- 1College of Animal Sciences, Zhejiang Provincial Key Laboratory of Preventive Veterinary Medicine, Institute of Preventive Veterinary Medicine, Zhejiang University, Hangzhou, China
- 2Department of Biomedical Sciences and One Health Center for Zoonoses and Tropical Veterinary Medicine, Ross University School of Veterinary Medicine, Basseterre, Saint Kitts and Nevis
Transthyretin (TTR)-like proteins play multi-function roles in nematode and are important component of excretory/secretory product in Haemonchus contortus. In this study, we functionally characterised a secretory transthyretin-like protein in the barber’s pole worm H. contortus. A full-length of transthyretin-like protein-coding gene (Hc-ttr-31) was identified in this parasitic nematode, representing a counterpart of Ce-ttr-31 in Caenorhabditis elegans. High transcriptional levels of Hc-ttr-31 were detected in the egg and early larval stages of H. contortus, with the lowest level measured in the adult stage, indicating a decreased transcriptional pattern of this gene during nematode development. Localisation analysis indicated a secretion of TTR-31 from the intestine to the gonad, suggesting additional roles of Hc-ttr-31 in nematode reproduction. Expression of Hc-ttr-31 and Ce-ttr-31 in C. elegans did not show marked influence on the nematode development and reproduction, whereas Hc-ttr-31 RNA interference-mediated gene knockdown of Ce-ttr-31 shortened the lifespan, decreased the brood size, slowed the pumping rate and inhibited the growth of treated worms. Particularly, gene knockdown of Hc-ttr-31 in C. elegans was linked to activated apoptosis signalling pathway, increased general reactive oxygen species (ROS) level, apoptotic germ cells and facultative vivipary phenotype, as well as suppressed germ cell removal signalling pathways. Taken together, Hc-ttr-31 appears to play roles in regulating post-embryonic larval development, and potentially in protecting gonad from oxidative stress and mediating engulfment of apoptotic germ cells. A better knowledge of these aspects should contribute to a better understanding of the developmental biology of H. contortus and a discovery of potential targets against this and related parasitic worms.
Introduction
The strongylid nematode Haemonchus contortus (also known as barber’s pole worm) is one of the most important parasitic nematodes of sheep, goats and other ruminants (Chilton et al., 2006). It feeds on blood in the abomasa of small ruminants and causes the parasitic disease haemonchosis characterised by anemia, hemorrhagic gastritis, oedema and associated complications (Zajac and Garza., 2020). This parasitic disease affects hundreds of millions of livestock animals, causing billions of dollars of losses to livestock husbandry globally (Roeber et al., 2013; Wang et al., 2017; Zajac and Garza., 2020). Current control strategies against H. contortus infection in ruminants rely heavily on anthelmintic chemotherapy, especially in the absence of efficient alternative methods in many countries (Blanchard et al., 2018). However, frequent and indiscriminate administration of anthelmintics has led to the development of drug resistance worldwide (Arsenopoulos et al., 2021), including drugs recently introduced into the market (Mederos et al., 2014; Niciura et al., 2019). Although the commercial vaccine Barbervax® against H. contortus has been registered for use in lambs in some countries (Teixeira et al., 2019; Kebeta et al., 2020), effective control of H. contortus and haemonchosis remains a major challenge globally. Revealing resistance mechanisms and discovering novel drug/vaccine targets are current priorities in the research field of H. contortus, which should be preferably based on a deep understanding of this important parasitic nematode at the molecular level (Wang et al., 2017; Ma et al., 2020).
Extensive studies on the excretory/secretory products (ESP) of parasitic nematodes (Hewitson et al., 2011; Vanhamme et al., 2020) have indicated a range of molecules that might play important roles at the host-parasite interface and are of potential value as vaccine targets. For instance, hundreds of ESPs of H. contortus have been identified by using advanced transcriptomic and proteomic tools, including proteolytic enzymes, glycoside hydrolases, C-type lectins, SCP/TAPS and transthyretin (TTR)-like proteins (Yatsuda et al., 2003; Gadahi et al., 2016; Wang et al., 2019). Particularly, TTR-like proteins have been consistently identified in the previous studies, which have been proposed to play a role in the developmental transition from the free-living stage to the parasitic stage of H. contortus as well as in the host-parasite interactions (see Cantacessi et al., 2010; Laing et al., 2013; Britton et al., 2016; Wang et al., 2019). In particularly, although it was proposed that TTR proteins play various roles in regulating apoptosis, modulating host immune responses and degenerative maladies in a range of organisms (Wang et al., 2010; Ankarcrona et al., 2016; Lin et al., 2016), few molecules have been studied at the molecular level in free-living or parasitic worms (Wang et al., 2010; Offenburger et al., 2018). Little is known about the biological function of TTR-like proteins in H. contortus and related parasitic nematodes of socioeconomic importance.
A recent proteomic study has confirmed 15 excreted/secreted TTR-like proteins in the parasitic stages of H. contortus (Wang et al., 2019), suggesting their roles in the key biological processes (e.g., development, reproduction and parasitism) of this important parasite. In this study, we elected to characterise one gene homologue (Hc-ttr-31) of C. elegans ttr-31 that exhibited strong phenotypic changes in previous genome-wide RNA interference (RNAi) studies. Real-time quantitative PCR (qRT-PCR) was used to analyse the developmental transcription of ttr-31 in H. contortus and C. elegans. Spatial expression of Hc-ttr-31 was assessed in the infective third-stage larvae (L3s), fourth-stage larvae (L4s) and adults of H. contortus by indirect immunofluorescence, and in C. elegans N2 strain by transgenesis. Gene knockdown was conducted to investigate the functional roles of Hc-ttr-31 in the development and reproduction as well as apoptosis of this important parasitic nematode.
Materials and Methods
Propagation of H. contortus and C. elegans
Adult worms of H. contortus (ZJ strain) were collected from a slaughterhouse in Jiaxing, Zhejiang, China. Female adult worms were dissected to collect eggs under a dissecting microscope (Motic, China). Eggs were cultured at 28°C for 7 days to obtain the L3s. Three helminth-free lambs (Hu sheep, 6 months old) were experimentally infected with ∼8000 L3s of H. contortus as described previously (Zhang et al., 2018). Faecal examination was performed to confirm the establishment of infection. Eggs of H. contortus were collected by flotation using saturated NaCl solution (Cox and Todd, 1962). The first-stage larvae (L1s), second-stage larvae (L2s) and L3s of H. contortus were obtained by incubating faecal samples at 28°C for 1, 3 and 7 days, respectively. L4s and adult worms were collected from the abomasum of euthanised lambs at 9 and 45 days after infection, washed in phosphate-buffered saline (PBS, pH7.4) and temporarily maintained in DMEM (Thermo Fisher Scientific, United States) with 10% FBS (Biological Industries, Israel) at 37°C (Shi et al., 2021).
The free-living nematode C. elegans N2 strain was maintained on nematode growth medium (NGM) agar plates at 20°C (Stiernagle, 2006). NGM agar plates were supplied with Escherichia coli OP50 or HT115 mutant bacteria as a food source.
Extraction of Deoxyribo Nucleic Acid and Ribo Nucleic Acid
Genomic DNA (gDNA) was extracted from snap-frozen adult worms of H. contortus using a small-scale DNA extraction kit (Takara Bio, Japan) according to the supplier’s instructions. Total RNA was extracted from eggs, L1s, L2s, L3s, L4s and adult worms of H. contortus using Trizol reagent (Invitrogen, United States). The first strand cDNA was synthesised from the total RNA using a first-strand cDNA synthesis kit (Toyobo, Japan). Extracted gDNA and synthesised cDNA were stored at −80°C until use.
Cloning and Characterization
The sequence of C. elegans ttr-31 (WormBase ID: WBGene00010225) was used to identify the gene homologue of H. contortus (designated as Hc-ttr-31) from the Sanger Helminths Database (https://www.sanger.ac.uk/resources/downloads/helminths/haemonchus-contortus.html). Based on the available transcript of Hc-ttr-31, primers (Supplementary Table S1) were designed using Primer Premier 5 (PREMIER Biosoft International, United States) to amplify the coding region of this gene. The amplification reaction mix contained 2.5 μL 10 × PCR buffer, 0.5 μL LA Taq (Takara Bio), 2 μL of 2.5 mM dNTPs, 2.0 μL of primer pair (10 μM), 1 μL gDNA of H. contortus and 17 μL of molecular grade water. The thermocycle program comprised of 94°C for 15 s, 62°C for 30 s and 72°C for 30 s. PCR amplification was also performed using cDNA as a template to obtain the transcript of Hc-ttr-31. PCR products were purified, ligated to PMD-19T (Takara Bio) and sequenced in both directions. Based on the obtained nucleotide acid sequence, exons and introns of Hc-ttr-31 were predicted by the “GT-AG” rule using DNASTAR (Version 7.1.0) (Breathnach and Chambon, 1981), with evidence from the obtained transcript sequences. Compara Gene Tree analysis and reciprocal blastp searching were performed based on resources available at WormBase ParaSite (https://parasite.wormbase.org/index.html). Functional domain architecture of the inferred proteins was predicted based on the InterPro database (Mitchell et al., 2018).
Quantitative Real-Time Polymerase Chain Reaction
Transcriptions of Hc-ttr-31 in all developmental stages (egg, L1, L2, L3, L4 and adult) of H. contortus were determined using Real-time SYBR Green Mix reagent (Toyobo) and a CFX96 Real-time PCR System (Bio-Rad, United States). qRT-PCR was also performed to assess the transcriptional level of ttr-31 in different developmental stages of C. elegans. The thermocycle program was 95°C for 10 min followed by 40 cycles of 95°C for 15 s, 60°C for 15 s and 72°C for 15 s. Actin (act-1) and β-tubulin were used as the internal controls for C. elegans and H. contortus, respectively. Transcriptional levels of key signalling components (i.e., ced-1, ced-4, ced-6, ced-7, ced-9, egl-1, ina-1, nrf-5, par-1, and ttr-52) involved in apoptosis and phagocytosis of apoptotic cells (Reddien and Horvitz, 2004; Wang et al., 2010; Palmisano and Meléndez, 2019) were also assessed. Primer sets used in this section can be found in Supplementary Table S1. Three replicates were included and performed independently. Transcriptional changes of genes were analysed using a 2-∆∆Ct method and presented as mean ± standard error of mean (SEM). Statistical analysis was conducted using a one-way ANOVA (p < 0.05).
Prokaryotic Expression and Preparation of Polyclonal Antibodies
Hc-ttr-31 was subcloned into pET30a vectors for prokaryotic expression of the recombinant protein rHc-TTR-31. In brief, the pET30a-Hc-trr-31 plasmids were transformed into E. coli BL 21 (DE3), which was induced by 0.5 mM isopropyl-β-d-1-thiogalactopyranoside (IPTG) at 37°C for 8 h. Bacteria were harvested by a centrifugation at 8,000 × g at 4°C for 5 min, suspended in 50 mM potassium phosphate (pH7.4), and lysed by sonication. The supernatant was separated by a centrifugation at 12,000 × g for 10 min at 4°C, filtered with a 0.22 μm filter (Merck Millipore, United States), then incubated with Ni-NTA resin column (Thermo Fisher Scientific) for 30 min. After washing with 20 mM imidazole, recombinant protein rHc-TTR-31 was eluted with 250 mM imidazole from the column. Purified protein was assessed by SDS-PAGE and a Bradford kit (Fdbio Science, China) using bovine serum albumin (BSA) as standard.
A New Zealand rabbit was immunised with the rHc-TTR-31 to generate polyclonal antibodies against the recombinant protein (Shi et al., 2021). In brief, the rabbit was subcutaneously injected with the rHc-TTR-31 and Freund’s Complete Adjuvant (Sigma-Aldrich, United States). Two boosting shots were conducted with the rHc-TTR-31 and Incomplete Fraud’s Adjuvant in a 2-week interval. Antiserum was collected 10 days after the final immunisation. Western blotting was performed using the anti-6 × His tag polyclonal antibodies (1:2,000; Proteintech, United States) and the generated anti-rHc-TTR-31 polyclonal antibodies to determine the specificity of antibodies.
Indirect Fluorescence Immunohistochemistry
Immunolocalisation of target protein was performed as described previously (Riou et al., 2005; Shi et al., 2021). Briefly, L3s of H. contortus were exsheathed by incubation with 0.15% sodium hypochlorite for 30 min (Ding et al., 2017); the exsheathed L3s (xL3s), L4s and adults were washed in PBS (pH7.4), fixed in 4% paraformaldehyde (Rothwell and Sangster, 1993) overnight, and then dehydrated. L4s and adults were further embedded in paraffin, sectioned at 5 μm in thickness, dried at 60°C overnight and deparaffinized with xylene and dehydrated as described previously (Qu et al., 2014). Sliced L4s and adults as well as xL3s of H. contortus were incubted in 1% BSA for 2 h, washed twice in PBS, and then incubated with 1:500 diluted anti-Hc-TTR-31 polyclonal antibodies at 4°C overnight. After washing, slides were incubated with goat anti-rabbit IgG (Invitrogen) at 1:1,000 dilution at 37°C for 1 h. Pre-immunisation serum from the same animal was used as a negative control. Fluorescence was detected with a Zeiss LSM710 laser confocal microscope (Zeiss Microscopy, Germany).
Transgenic Expression of Hc-ttr-31 in C. elegans
To confirm the spatial distribution of Hc-TTR-31, transgenesis of Hc-ttr-31 was conducted in C. elegans (Huang et al., 2021). Briefly, the promoter sequence of Ce-ttr-31 was amplified, ligated to the PMD-18T vector and subcloned into pPD95.67 plasmids (Ce-ttr-31p::gfp), with the Hc-ttr-31 coding sequence inserted into the multiple cloning site (Ce-ttr-31p::Hc-ttr-31::gfp). Transgenic expression of Hc-ttr-31 in C. elegans N2 strain was performed by micro-injection with the Ce-ttr-31p::Hc-ttr-31::gfp (Mello et al., 1991). A Ce-ttr-31p::Ce-ttr-31::gfp was used as a reference control. Considering the homology of Hc-ttr-31 and Ce-ttr-31, transgenesis of Hc-ttr-31 should result in an over-expression of TTR-31 in the treated C. elegans. To explore the effects of exogenous Hc-TTR-31 in C. elegans, transgenic worms were mounted on 2% agar pads containing 1% sodium azide (Solarbio, China) and imaged using a Zeiss LSM710 laser confocal microscope (Zeiss Microscopy).
RNA Interference
To explore the function of Hc-ttr-31 in H. contortus, Hc-ttr-31 RNAi-mediated gene knockdown of its orthologue Ce-ttr-31 was performed in C. elegans, using a method described previously (Kwon et al., 2010; Yan et al., 2014). In brief, Hc-ttr-31 was cloned into the plasmid L4440, which was then transformed into E. coli HT115 (DE3), with Ce-ttr-31 and empty L4440 vectors used as positive and negative controls, respectively. NGM plates containing 0.1 mM IPTG were seeded with the transformed HT115 and placed at room temperature for 5 days prior to RNAi assay. Worms of C. elegans N2 strain were synchronized and laid on standard NGM plates supplemented with OP50 (Kamath and Ahringer, 2003). The worms were washed off the plates after 30 h (i.e., L4 stage) and then centrifuged at 1,000 ×g for 5 min. From which, 20 worms were inoculated onto NGM plates with the transformed HT115, and incubated at 25°C for 3 days. Following transcriptional examinations of Hc-ttr-31 and Ce-ttr-31 by qRT-PCR, phenotypic changes of worms were assessed in aspects of brood size, pumping rate, body length and body width (see Wong et al., 1995; Mörck and Pilon, 2006; Papaevgeniou et al., 2019). Three replicates were performed for the RNAi and phenotypic assays. For the analysis of facultative vivipary phenotype, worms were checked using a dissecting microscope (Motic) and the ratio of bagging worms was calculated.
Apoptosis Assay
To check whether Hc-ttr-31 plays a role in the apoptosis, the number of apoptotic germ cells in the RNAi-treated worms was determined using a method described elsewhere (Kelly et al., 2000) with some modifications according to manufacturer’s instruction. Briefly, ∼ 100 synchronised adult worms were subjected to RNAi treatment for 36 h and then washed in M9 buffer for 5 times; Worms were suspended in 0.5 ml M9 buffer and stained with acridine orange (20 μg/ml; Solarbio) for 1.0 h; Stained worms were then transferred onto NGM plates seeded with OP50 for 1.5 h for recovery. Recovered worms were kept in 1.0% sodium azide solution at the centre of slide and examined under a Zeiss LSM710 laser confocal microscope (Zeiss Microscopy).
Measurement of Reactive Oxygen Species
Production of general ROS in RNAi-treated worms was determined using 2′, 7′-dichlorodihydrofluorescein diacetate (DCFH-DA) (Beyotime, China) based on the method reported elsewhere (Zhu et al., 2010; Guo et al., 2016). In brief, about 5,000 treated/untreated worms were sonicated in PBS, and the supernatant was collected after centrifugation at 15,000 × g for 45 min. Bradford protein assay kit (Fdbio Science) was performed to determine protein concentration. The lysates were incubated with 300 μM DCFH-DA in black-walled microtiter plate (Corning Incorporated, United States) at 37°C for 3 h. Rosup was used as a positive control in the production of reactive oxygen. Fluorescence intensity was measured using a Synergy H1 hybrid multimode microplate reader (BioTek, United States).
Statistical Analysis
At least three technical replicates were included in each assay and each experiment was repeated three times. Data are presented as mean ± standard error of mean (SEM). One-way ANOVA with Dunnett post-hoc test was performed using Excel 2016 (Microsoft, United States) and GraphPad Prism 5 (GraphPad Software, United States). p < 0.05 was considered statistically significant.
Results
cDNA-Confirmed Hc-ttr-31 is a 1-to-1 Orthologue of Ce-ttr-31
Hc-ttr-31 comprised three exons, encoding a transcript of 432 nt in length (GenBank accession number MW013314; Figure 1A), representing a predicted gene locus (hcontortus_chr2_Celeg_TT_arrow_pilon: 30031855–30032608) in the recently updated chromosome-level genome assembly of H. contortus (MHCO3ISE; WBPS15). The cDNA-confirmed gene model of Hc-ttr-31 was similar to that of Ce-ttr-31 in the free-living model organism C. elegans (Figure 1A), and was predicted a 1-to-1 orthologue of Ce-ttr-31. The inferred protein Hc-TTR-31 was 143 aa in length, contained a signal peptide (SignalP-noTM) and a transthyretin-related family domain, showing a 65% similarity to the amino acid sequence deduced from Ce-ttr-31 (Figure 1B).
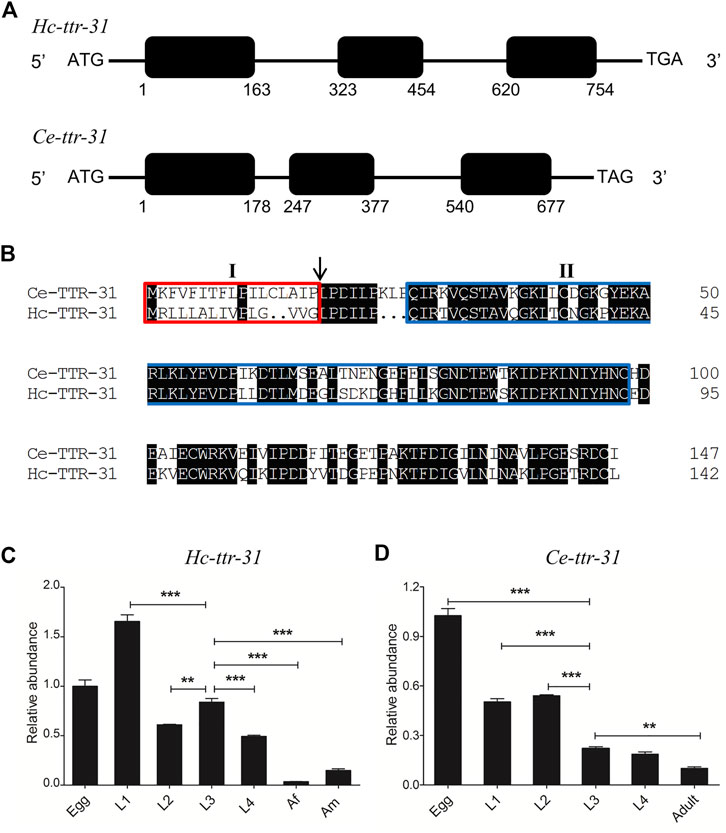
FIGURE 1. Sequence and transcription analyses of ttr-31 in Haemonchus contortus and Caenorhabditis elegans. (A) Gene structures of Hc-ttr-31 and Ce-ttr-31. Black boxes and the horizontal lines represent exons and introns, respectively. The numbers below the black boxes indicates the boundaries of exons. (B) Pair-wise sequence alignment of the predicted TTR-31 of C. elegans and H. contortus. Identical residues are shaded in black. The red box (I) indicates the predicted secretory signal, the arrow points the putative cleavage sites and the blue box (II) shows the transthyretin domain. Transcriptional patterns of Hc-ttr-31 (C) and Ce-ttr-31 (D) in different developmental stages of H. contortus and C. elegans. Egg, first (L1), second (L2), third (L3), fourth (L4) larval, female adult (Af) and male adult (Am) stages are indicated. *p < 0.05; **p < 0.01; ***p < 0.001.
Hc-ttr-31 Highly Transcribes in all Larval Stages but Not in Adult Stage
Different transcriptional levels of Hc-ttr-31 were detected among the developmental stages of H. contortus. Specifically, Hc-ttr-31 was highly transcribed in the egg and larval (L1, L2, L3 and L4) stages of H. contortus, with the highest level detected in the L1 stage and the lowest in the female adult stage (Figure 1C), showing a decreasing transcriptional pattern of Hc-ttr-31 during the development of this parasitic nematode. A similar developmental transcription of Ce-ttr-31 was also found in C. elegans (Figure 1D). Differently, the highest transcriptional level of Ce-ttr-31 was detected in the egg of the free-living nematode (Figure 1D).
Hc-TTR-31 is Localised in the Intestine and Gonad of H. contortus
Recombinant Hc-TTR-31 (rHc-TTR-31) was successfully expressed in E. coli BL21 (DE3) and was recognised by the anti-6×His tag polyclonal antibodies (Supplementary Figure S1). Using the recombinant protein, polyclonal antibodies against rHc-TTR-31 were generated and used to detect the native Hc-TTR-31 from the crude protein extract of H. contortus (Supplementary Figure S1A), facilitating immunolocalization of native Hc-TTR-31 in the L3s, L4s, and adults of this parasite (Figure 2 and Supplementary Figure S2). Specifically, predominant cephalic, cuticular/muscular and intestinal distributions of Hc-TTR-31 were observed in the xL3s of H. contortus, with punctate expression detected in the head and tail regions (Figure 2A). Muscular and intestinal distributions of Hc-TTR-31 were also found in L4s (Figure 2B) and adults (Figure 2C) of this parasitic nematode. Differently, the fluorescent intensities observed in the L4s and adults were lower than that in the xL3s of H. contortus (Figures 2B,C), which was in accordance with the decreasing transcriptional pattern of Hc-ttr-31 during the development of this parasite.
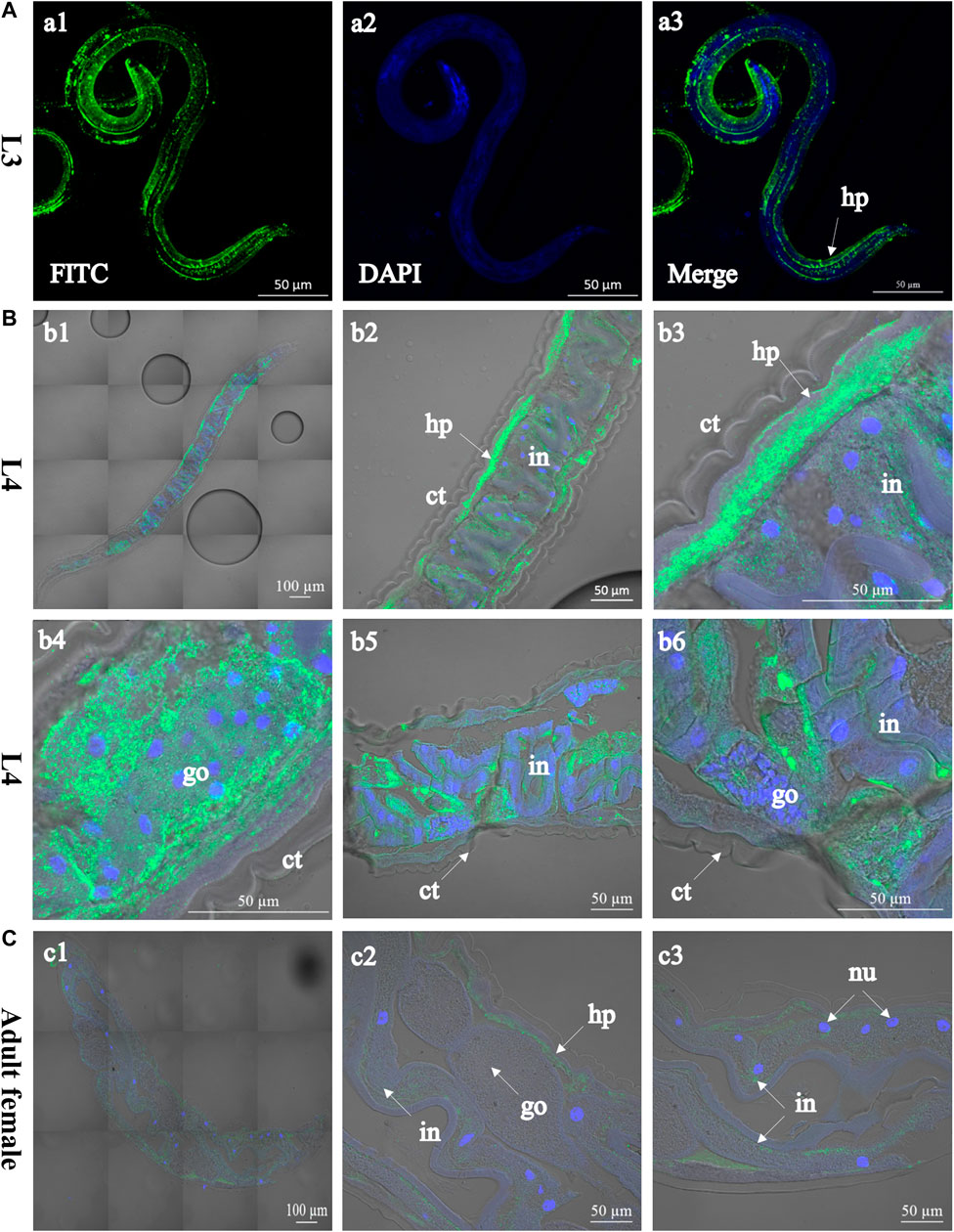
FIGURE 2. Immunolocalisation of Hc-TTR-31 in Haemonchus contortus. Green fluorescence shows the distribution of Hc-TTR-31 in the third- (A) and fourth stage larvae (B), and adult stage (female) (C) of H. contortus. Hc-TTR-31 is probed with rabbit anti-rHc-TTR-31 polyclonal antibodies followed by fluorescein (FITC) conjugated-goat anti-rabbit IgG as secondary antibody. Nuclei are stained with 4′,6-diamidino-2-phenylindole (DAPI). ct: cuticle, go: gonad, in: intestine, hp: hypodermis, nu: nucleus. Scale bar: 50 μm or 100 μm.
Expression of Ce-TTR-31 and Hc-TTR-31 Prolonged the Lifespan of C. elegans
Driven by the promoter of Ce-ttr-31 (Ce-ttr-31p), GFP was expressed in the intestine, muscle, and neurons in the pharynx and tail regions of C. elegans (Figure 3), suggesting functional activities of the promoter in these tissues. Therefore, by microinjection of the Ce-ttr-31p::Ce-ttr-31::gfp and Ce-ttr-31p::Hc-ttr-31::gfp plasmids, GFP-fused proteins were expressed in the transgenic worms. Specifically, the Ce-TTR-31-GFP fusion protein was observed in the pharyngeal neurons, muscle, and the U-shape gonad arms, but not detected in the intestine of adult worms (Figure 4A). A similar protein distribution was also found for the Hc-TTR-31-GFP fusion protein in the transgenic C. elegans, including pharyngeal neurons, gonad and musculature (Figures 4B,C).
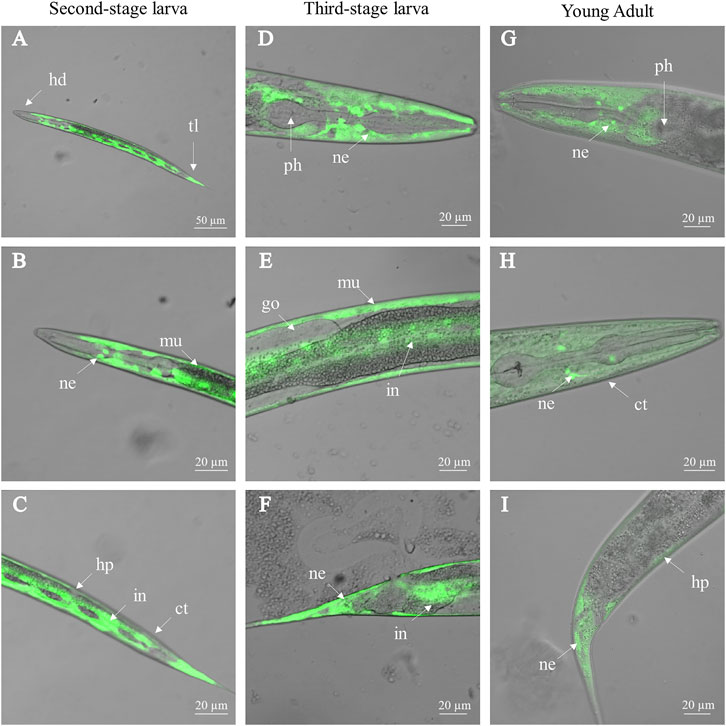
FIGURE 3. Activity of Ce-ttr-31 promoter in Caenorhabditis elegans. Ce-ttr-31p:gfp is expressed in the second-stage larva (A–C), third-stage larva (D–F) and adult (G–I) of C. elegans. Activities of Ce-ttr-31 promoter in neurons (ne), muscle layer (mu), intestine (in) and hypodermis (hp) are indicated. ct: cuticle, go: gonad, hd: head, ph: pharynx, tl: tail. Scale bar: 20 μm.
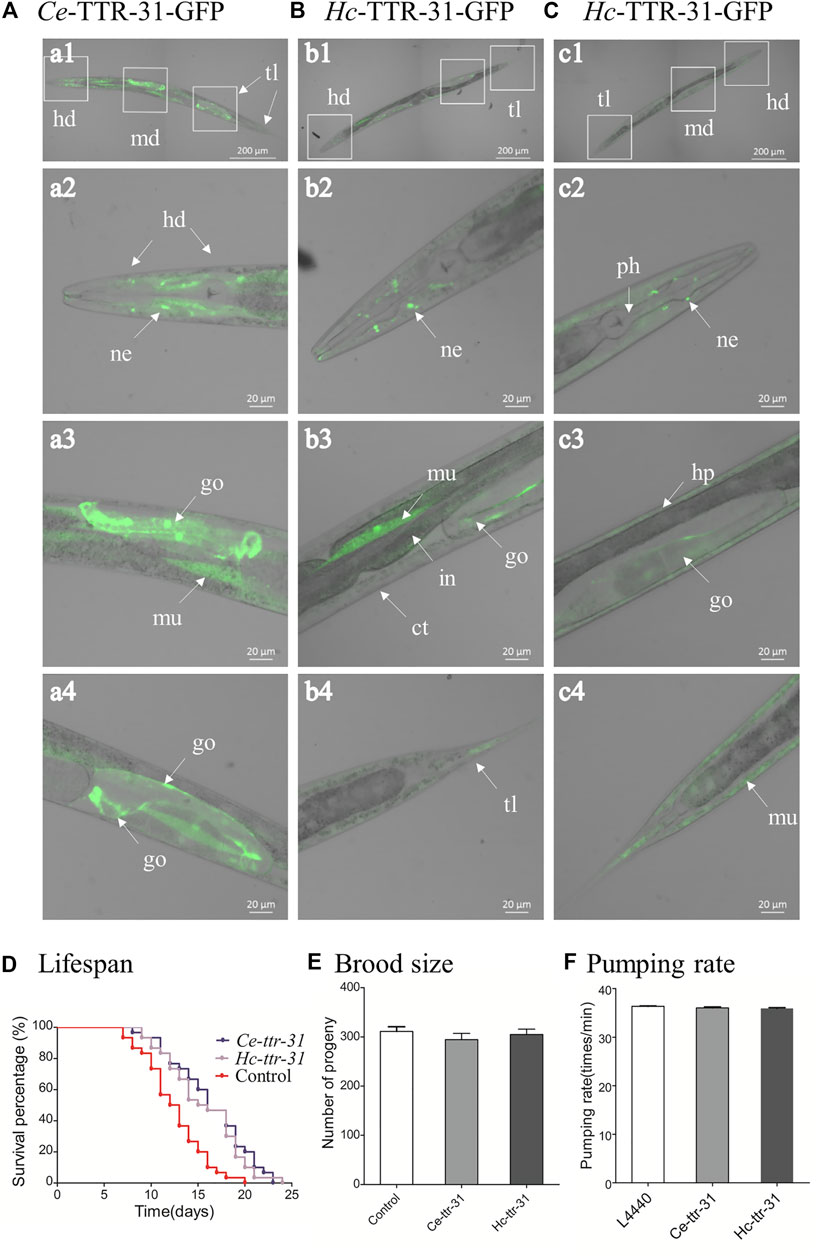
FIGURE 4. Transgenic expression of Hc-ttr-31 in Caenorhabditis elegans. Green fluorescence protein-fused Ce-TTR-31 (A) and Hc-TTR-31 (B,C) were expressed in adult worms of C. elegans by microinjection of pPD95.67-Ce-ttr-31p:gfp plasmids. Protein expression in neurons (ne), gonad (go), muscle layer (mu) and hypodermis (hp) are indicated. The influences of overexpressed TTR-31 on lifespan (D), brood size (E) and pumping rate (F) in transgenic worms are indicated. Data are presented as mean ± SEM (n = 30, 10, 10). ct: cuticle, hd: head, in: intestine, md: middle, ph: pharynx, tl: tail. Scale bar: 20 μm or 200 μm.
Microinjection of Ce-ttr-31p::Ce-ttr-31::gfp/Ce-ttr-31p::Hc-ttr-31::gfp in C. elegans N2 strain resulted in expression of Ce-TTR-31 and expression of both Ce-TTR-31 and Hc-TTR-31 in the transgenic worms. Compared with the non-transgenic C. elegans N2 strain, expression of Ce-TTR-31 prolonged the lifespan of transgenic worms (Figure 4D), but did not show any significant (p > 0.05) effect on brood size (Figure 4E) or pumping rate (Figure 4F) of the transgenic worms. Expression of both Ce-TTR-31 and Hc-TTR-31 in C. elegans N2 strain showed similar effects to the expression of Ce-TTR-31 (Figures 4D–F).
Downregulation of ttr-31 Leads to Facultative Vivipary Phenotype in C. elegans
To further explore the functional roles of Hc-ttr-31, gene knockdown was successfully conducted by feeding worms with HT115 bacteria that could express silencing RNAs targeting Ce-ttr-31 or Hc-ttr-31. Specifically, compared with negative control, RNAi of Ce-ttr-31 significantly reduced (>50%; p < 0.001) the transcriptional level of this gene in the treated worms (Figure 5A), shortened the lifespan of treated worms (Figure 5B), decreased the number of progeny (p < 0.001), and inhibited the pumping rate (p < 0.001) and growth (body length and width; p < 0.05) of the treated worms (Figures 5C–F). Hc-ttr-31 RNAi mediated gene knock down of Ce-ttr-31 also achieved effective gene silencing (>50%; p < 0.001) of Ce-ttr-31 (Figure 5A), and resulted in similar effects on treated worms, such as shortened lifespan, and reduced brood size, pumping rate, body length and width (Figures 5B–F), implying functional conservation of ttr-31 between C. elegans and H. contortus.
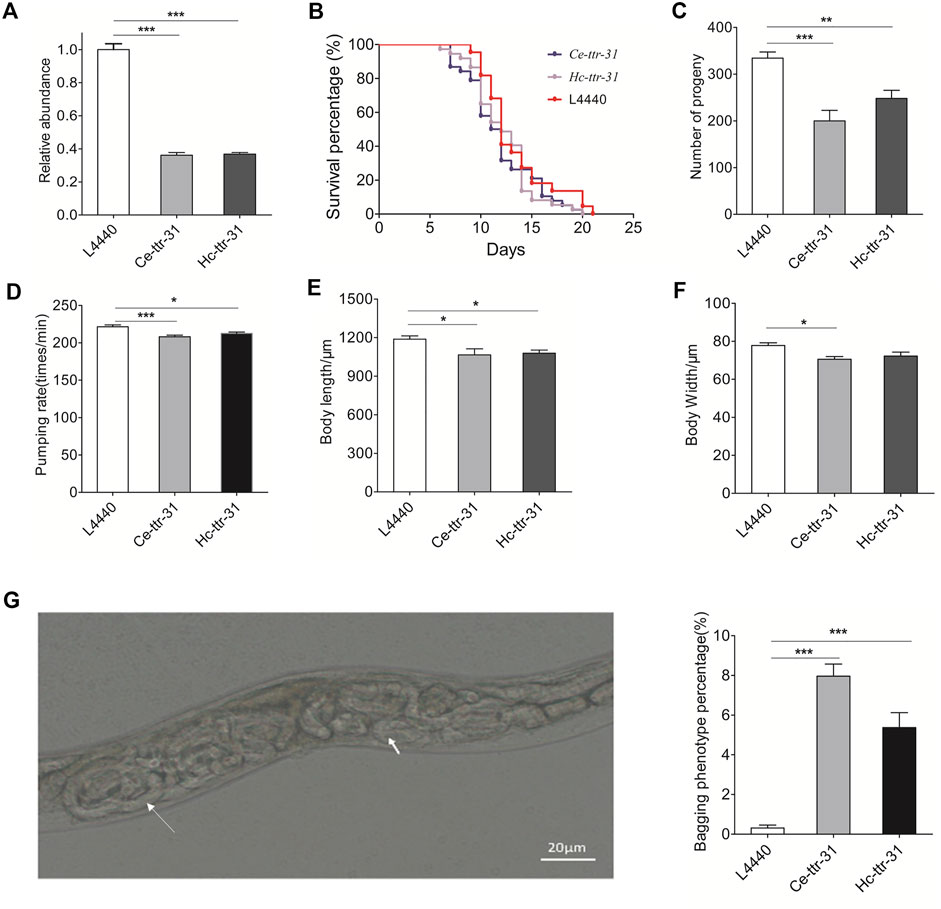
FIGURE 5. Hc-ttr-31 RNAi mediated gene knockdown of Ce-ttr-31 in Caenorhabditis elegans. (A) Transcriptional levels of Ce-ttr-31 in treated worms. Effects of gene knockdown on the lifespan (B), brood size (C), pumping rate (D), body length (E) and body width (F) are shown. Data are presented as mean ± SEM (n = 10). (G) Hatching in vivo phenotype and the percentage of bagging phenotype are shown. Data are presented as mean ± SEM (n = 6). *p < 0.05; **p < 0.01; ***p < 0.001.
Notably, compared with the untreated C. elegans, effective knockdown of Ce-ttr-31 and Hc-ttr-31-mediated knockdown of Ce-ttr-31 led to significantly increased (8 and 5 fold; p < 0.001) facultative vivipary phenotype (i.e., hatching in vivo or “bagging”; Chen and Caswell-Chen, 2004) in treated worms (Figure 5G), suggesting involvement of TTR-31 in the post-embryonic development and reproduction processes.
Hc-TTR-31 is Likely to Play a Role in the Apoptotic Germ Cell Removal
By staining with acridine orange, apoptotic cells were observed (brighter than the normal ones due to pyknosis) in the gonad arms of the RNAi-treated C. elegans (Figure 6). Specifically, compared with negative control (Figure 6A), there was an increase (4.28 and 3.77 folds) of apoptotic germ cells in the gonad arms of Ce-ttr-31 and Hc-ttr-31 RNAi-treated worms (Figures 6B,C). In addition to the increased number of apoptotic germ cells, lower transcriptional level of Ce-ttr-31 and Hc-ttr-31 was linked to a significant increase of general ROS (p < 0.01) and transcriptional level of ced-4 (cell death abnormal 4, encodes a key component in the apoptosis activation pathway) (p < 0.001) in RNAi-treated worms (Figure 7A), suggesting activated apoptosis and involvement of ttr-31 in apoptosis.
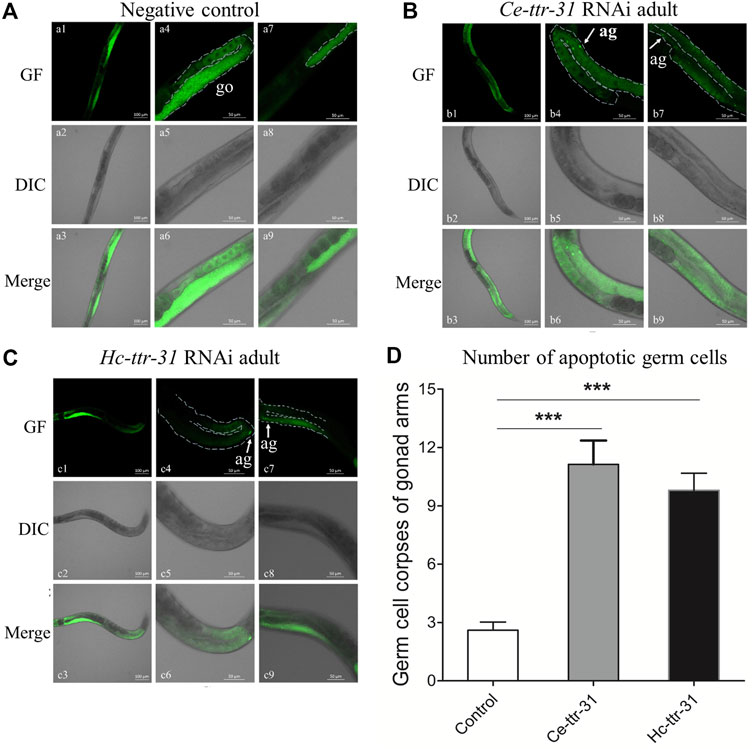
FIGURE 6. Effect of ttr-31 RNA interference on the germ cells in Caenorhabditis elegans. Apoptotic germ cells in the negative control (A), Ce-ttr-31 (B) and Hc-ttr-31 (C) RNAi-treated worms are indicated. Apoptotic germ cells (ag) are pointed by white arrows. (A–C) a1-a3 shows the whole gonad of C. elegans stained by acridine orange including two gonad arms (a4-a6 and a7-a9). b4-b6 and b7-b9 are two gonad arms of a worm in b1-b3. c4-c6 and c7-c9 are two gonad arms of a worm in c1-c3. (D) The number of apoptotic germ cells in the treated and untreated worms is shown. Data are presented as mean ± SEM (n = 15). GF: green fluorescence; DIC: differential interference contrast; Merge: GF merges with DIC. Scale bars: 50 μm ***p < 0.001.
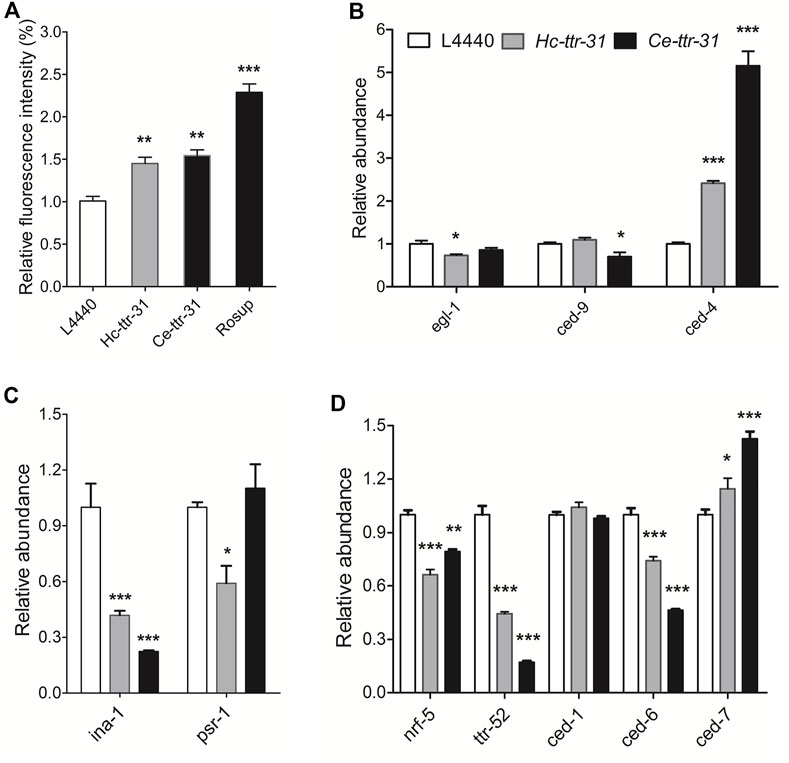
FIGURE 7. Effect of ttr-31 knockdown on the apoptotic signalling pathway in Caenorhabditis elegans. (A) Relative levels of reactive oxygen species (ROS) in RNA interference (RNAi)-treated worms, negative and positive (Rosup) controls are shown. (B–D) Transcriptional levels of genes involved in apoptotic signalling pathways in RNAi-treated C. elegans worms. ced-4: cell death abnormal 4, egl-1: egg-laying defective-1, ina-1: integrin-α, nrf-5: nose resistant to fluoxetine 5, psr-1: phosphatidyl serine receptor-1. *p < 0.05; **p < 0.01; ***p < 0.001.
As the homologous gene ttr-52 (encodes an extracellular phosphatidylserine-binding protein on the apoptotic cell) was reported to be required for cell corpse engulfment (Wang et al., 2010), the function of ttr-31 in apoptosis or clearance of cell corpse was determined by exploring the transcriptional alterations of signalling components involved in phagocytosis of apoptotic cells (Figures 7B–D). Specifically, compared with untreated worms, significant lower transcriptions were detected for ina-1 (encodes an engulfment receptor integrin-1 that functions upstream of CED-2/CED-5/CED-10/CED-12 apoptotic cell removal signalling pathway) (p < 0.001) (Figure 7C), and nrf-5 (encodes a secreted lipid-binding protein) (p < 0.01), ttr-52 (p < 0.001) and ced-6 (encodes CED-6, a key factor in CED-1/CED-6/CED-7 cell corpse engulfment pathway) (p < 0.001) in both Ce-ttr-31 and Hc-ttr-31 RNAi-treated C. elegans (Figure 7D). Differently, a significant higher transcription of ced-7 (encodes an ABC transporter that transfers phosphatidylserine from apoptotic cells to engulfing cells) (p < 0.05) was found in the treated worms (Figure 7C). Transcriptional changes of egl-1 (egg-laying defective-1), ced-9 (an orthologue of bcl-2), psr-1 (encodes a phosphatidylserine-recognizing receptor) and ced-1 (encodes a single-pass transmembrane protein that acts in engulfing cells to promote removal of apoptotic cells) were not consistent between Ce-ttr-31 and Hc-ttr-31 RNAi-treated worms (Figures 7B–D). In summary, ttr-31 is likely to regulate and recognise the apoptotic cells.
Discussion
The biological role of TTR-like proteins is not clear in parasitic nematodes, although such molecules have been commonly identified in the excretory/secretory products of parasitic worms. In this study, we reported the functional roles of Hc-ttr-31 in a strongylid nematode H. contortus. We found that this gene was transcribed in all developmental stages of H. contortus, with a decreasing transcriptional pattern during the development from the egg to the adult stage. Immunolocalisation and transgenic expression analyses of Hc-TTR-31 indicated pharyngeal, muscular and gonad protein distributions of this protein. Gene knockdown of Hc-ttr-31 in C. elegans was linked to an increase of ROS level and apoptotic germ cells, which resulted in a “worm bagging” phenotype, suggesting essential roles in development and reproduction of nematodes.
Hc-ttr-31 is an orthologue to the Ce-ttr-31 of the free-living nematode C. elegans. Although several TTR-like proteins or protein-coding genes have been reported in H. contortus (see Laing et al., 2013; Britton et al., 2016; Wang et al., 2019) and other parasitic nematodes (Parkinson et al., 2004; Saverwyns et al., 2008; Vieira et al., 2020), most of these molecules have not yet been identified and functionally characterised. In the current study, gene model validation, reciprocal homology searching, and domain architecture analysis confirmed the orthologous relationship between Hc-ttr-31 and Ce-ttr-31. This statement was also supported by the similar transcriptional patterns and protein localisation of the two genes. In particular, RNAi targeting the transgenic Hc-ttr-31 in C. elegans also resulted in efficient knockdown of Ce-ttr-31 and the same phenotypes in transgenic worms, strongly suggesting sequence and functional conservation between the two genes. However, considering the nature of differences between the free-living nematode C. elegans and the parasitic nematode H. contortus, detailed biological exploration of Hc-ttr-31 is still required.
It appears that Hc-TTR-31 was produced in the intestine and then secreted into the gonad of a worm. Given the extensive identification of TTR-like proteins in excretory/secretory products, it is interesting that transgenic expression of GFP (driven by the promoter of Ce-ttr-31) was observed in the intestine of C. elegans, whereas the fusion protein Hc-TTR-31-GFP was detected in the gonads of worms. It is likely that Hc-TTR-31 was synthesised in the intestinal cells, secreted into the body cavity, and then diffused to the gonad, which can be supported by the identification of signal peptide and the localisation of Hc-TTR-31 in H. contortus. Particularly, TTR-52 (a homologous to TTR-31) has been confirmed as a secretory protein that was found expressed in the intestine of C. elegans (Wang et al., 2010; Raiders et al., 2021). Additionally, TTRs are proteins commonly found in the serum and cerebrospinal fluid in mammals (Goodman, 1986; Herbert et al., 1986; Buxbaum and Reixach, 2009), suggesting the secretory nature of these proteins. However, it is still not clear whether there is a polarity of Hc-TTR-31 secretion (exclusive secretion into body cavity or intestine lumen), since it has been identified in the excretory/secretory products of H. contortus (Wang et al., 2019) and potentially other parasitic nematodes.
The secretory Hc-TTR-31 might play a role in nematode development, particularly post-embryonic larval development. This statement can be strongly supported by the transcriptional analysis, protein localisation and RNAi analysis in the current study. First, Hc-ttr-31 was highly transcribed in the early developmental stages (e.g., egg, L1, L2 and L3) and then significantly downregulated when worms enter parasitic stages (e.g., L4 and adult) of H. contortus. More transcriptional evidence can be found in a previous study in which significant differences in transthyretin-like gene families were found between the free-living and the CO2-activated L3s of H. contortus (Cantacessi et al., 2010). Second, Hc-TTR-31 was consistently found in the pharyngeal neurons of H. contortus L3s and C. elegans larvae (i.e., L2s and L3s), whereas expression of both Hc-ttr-31 and Ce-ttr-31 in adult C. elegans extended the lifespan but did not influence the pumping rate and brood size of the transgenic worms. TTRs were also found in cerebrospinal fluid of mammals and mutations of these proteins may cause Alzheimer’s disease in human beings (Schwarzman et al., 1994; Sousa et al., 2007; Gião et al., 2020). So, it is very likely that ttr-31 plays a role in signalling pathways, such as insulin/insulin-like growth factor 1 (insulin/IGF-1) and steroid hormone signalling (Murphy and Hu, 2013; Antebi, 2015), to regulate worm growth and lifespan. However, this statement still needs further exploration and verification at the molecular level. Third, Hc-ttr-31 RNAi-mediated gene knockdown Ce-ttr-31 resulted in slower pumping rate and growth as well as a significant increase of embryos in the utero and egg hatching in vivo in C. elegans (facultative vivipary; see Chen and Caswell-Chen, 2004). Post-embryonic development variant, larval arrest and slow growth have also been reported in the previous RNAi experiments of C. elegans (Kamath et al., 2003; Rual et al., 2004; Dalton and Curran, 2018). Therefore, it is clear that Hc-ttr-31 also plays potential roles in regulating the post-embryonic development, larval growth and lifespan of H. contortus. Nevertheless, the mechanism of ttr-31 underlying the developmental regulation of parasitic worms is still unclear and warrants further investigation.
In addition, Hc-TTR-31 might play dual roles in protecting germ cells from oxidative stress and mediating clearance of apoptotic cells. It has been reported that a transthyretin-like protein (TTR-52) was required to mediate recognition and clearance of apoptotic cells in C. elegans (Wang et al., 2010; Palmisano and Meléndez, 2019). In the current study, gene knockdown of ttr-31 led to an increase of ROS production and increased number of apoptotic germ cells, as well as facultative vivipary (also known as worm bagging, a survival-enhancing response to stress; Chen and Caswell-Chen, 2004). Therefore, it is likely a relationship between low TTR-31 expression and oxidative stress which then causes DNA damage and apoptosis in the gonadal tissue of RNAi-treated worms (see Stergiou and Hengartner, 2004; Balaban et al., 2005; Hubbard and Schedl, 2019). Indeed, the functional role of another transthyretin-like protein (TTR-33) in protecting dopaminergic neurons from oxidative stress-induced degeneration has been reported in C. elegans (Offenburger et al., 2018). However, it is still plausible that dying cells might be recognised and cleared by phagocytes (phagocytosis) via TTR-31 (Lockshin and Zakeri, 2001; Reddien and Horvitz, 2004), as no apoptotic germ cell was found in the wild-type C. elegans. To further distinguish or confirm the involvement of TTR-31 in apoptosis and in apoptotic cell clearance, we assessed the transcriptional status of key signalling components involved in the apoptosis signalling pathways (see Savill and Fadok, 2000; Hochreiter-Hufford and Ravichandran, 2013; Palmisano and Meléndez, 2019). First, ced-4 (a key component in the apoptosis activation pathway) was up-regulated in ttr-31 RNAi treated worms, suggesting an initiation of apoptosis (see Stergiou and Hengartner, 2004; Hochreiter-Hufford and Ravichandran, 2013). Second, ina-1 (an engulfment receptor functions upstream of CED-2/CED-5/CED-10/CED-12 apoptotic cell removal signalling pathway), nrf-5 and ttr-52 (molecules mediating engulfment signal into CED-1/CED-6/CED-7 pathway) were down-regulated in response to gene knockdown of ttr-31 RNAi, suggesting a suppressed phagocytosis of apoptotic cells (Wang et al., 2003; Hsu and Wu, 2010; Zhang et al., 2012; Haley et al., 2018). Third, ced-7 (an ABC transporter that transfers phosphatidylserine from apoptotic cells to engulfing cells; Wang et al., 2003) was found upregulated in ttr-31 knock-down worms, which might be associated with the increased apoptotic germ cells. Therefore, Hc-TTR-31 is likely to play roles not only in protecting germ cells from oxidative stress-induced apoptosis and but also in mediating apoptotic germ cell clearance. However, this functional interpretation is based on results derived from heterologous expression of Hc-ttr-31 and heterologous RNAi of Ce-ttr-31 in C. elegans. A rescuing experiment of Ce-ttr-31 loss-of-function by Hc-ttr-31 in C. elegans, or direct gene knockout assay in H. contortus should be preferably conducted. Further investigations such as binding assays (TTR-31 and receptors), molecular changes at the protein levels, as well as time-lapse assays to distinguish between the role of TTR-31 in triggering germ cell apoptosis or in germ cell corpse clearance should provide novel insights into the functional roles of this TTR-like protein in parasitic nematodes.
In conclusion, we functionally characterised a secretory protein Hc-TTR-31 in the important parasitic nematode H. contortus. This protein appeared to play roles in regulating post-embryonic larval development, and likely in protecting germ cells from oxidative stress and mediating clearance of apoptotic germ cells. Detailed involvement of Hc-TTR-31 in the development and reproduction of H. contortus and related parasitic nematodes of socioeconomic importance warrants further investigation. A better understanding of these aspects at the molecular level is likely to indicate potential targets for the control of parasitic diseases.
Data Availability Statement
The datasets presented in this study can be found in online repositories. The names of the repository/repositories and accession number(s) can be found below: https://www.ncbi.nlm.nih.gov/genbank/, MW013314.
Ethics Statement
The animal study was reviewed and approved by the Zhejiang University Experimental Animal Ethics Committee.
Author Contributions
HS, XH, YY and AD designed the research. HS and XH conducted the experiment. HS and GM wrote the manuscript. XH, FW and XC analysed the data. YY, FW and XC provided reagents. CY, GM and AD revised the manuscript. All the authors contributed to the article and approved the submitted version.
Funding
This study was supported by the National Key Research and Development Program of China (grant number 2017YFD0501200), the National Natural Science Foundation of China (NSFC) (grant numbers 320023043 and 1602041), the National Basic Research Program of China (973 program) (grant number 2015CB150300), Science and Technology Program of Jiaxing (grant number 2016AY23025) and State Key Laboratory of Pathogen Biology of Livestock Diseases Open Foundation (grant number SKLVEB2016KFKT018). All funders have no role in the study design and collection, analysis, and interpretation of the results.
Conflict of Interest
The authors declare that the research was conducted in the absence of any commercial or financial relationships that could be construed as a potential conflict of interest.
Publisher’s Note
All claims expressed in this article are solely those of the authors and do not necessarily represent those of their affiliated organizations, or those of the publisher, the editors and the reviewers. Any product that may be evaluated in this article, or claim that may be made by its manufacturer, is not guaranteed or endorsed by the publisher.
Acknowledgments
We would like to thank Prof. Caiyong Chen (MOE Key Laboratory of Biosystems Homeostasis and Protection, College of Life Sciences, Zhejiang University) for providing the nematode expression vector pPD95.67.
Supplementary Material
The Supplementary Material for this article can be found online at: https://www.frontiersin.org/articles/10.3389/fcell.2021.753667/full#supplementary-material
References
Albert Hubbard, E. J., and Schedl, T. (2019). Biology of the Caenorhabditis elegans Germline Stem Cell System. Genetics 213 (4), 1145–1188. doi:10.1534/genetics.119.300238
Ankarcrona, M., Winblad, B., Monteiro, C., Fearns, C., Powers, E. T., Johansson, J., et al. (2016). Current and Future Treatment of Amyloid Diseases. J. Intern. Med. 280, 177–202. doi:10.1111/joim.12506
Antebi, A. (2015). Nuclear Receptor Signal Transduction in C. elegans. WormBook, 1–49. doi:10.1895/wormbook.1.64.2
Arsenopoulos, K. V., Fthenakis, G. C., Katsarou, E. I., and Papadopoulos, E. (2021). Haemonchosis: A Challenging Parasitic Infection of Sheep and Goats. Animals 11 (2), 363. doi:10.3390/ani11020363
Balaban, R. S., Nemoto, S., and Finkel, T. (2005). Mitochondria, Oxidants, and Aging. Cell 120, 483–495. doi:10.1016/j.cell.2005.02.001
Blanchard, A., Guégnard, F., Charvet, C. L., Crisford, A., Courtot, E., Sauvé, C., et al. (2018). Deciphering the Molecular Determinants of Cholinergic Anthelmintic Sensitivity in Nematodes: When Novel Functional Validation Approaches Highlight Major Differences between the Model Caenorhabditis elegans and Parasitic Species. Plos Pathog. 14, e1006996. doi:10.1371/journal.ppat.1006996
Breathnach, R., and Chambon, P. (1981). Organization and Expression of Eucaryotic Split Genes Coding for Proteins. Annu. Rev. Biochem. 50, 349–383. doi:10.1146/annurev.bi.50.070181.002025
Britton, C., Roberts, B., and Marks, N. D. (2016). Functional Genomics Tools for Haemonchus contortus and Lessons from Other Helminths. Adv. Parasitol. 93, 599–623. doi:10.1016/bs.apar.2016.02.017
Buxbaum, J. N., and Reixach, N. (2009). Transthyretin: the Servant of many Masters. Cell. Mol. Life Sci. 66, 3095–3101. doi:10.1007/s00018-009-0109-0
Cantacessi, C., Campbell, B. E., Young, N. D., Jex, A. R., Hall, R. S., Presidente, P. J., et al. (2010). Differences in Transcription between Free-Living and CO2-activated Third-Stage Larvae of Haemonchus contortus. BMC Genomics 11, 266. doi:10.1186/1471-2164-11-266
Chen, J., and Caswell-Chen, E. P. (2004). Facultative Vivipary Is a Life-History Trait in Caenorhabditis elegans. J. Nematol. 36, 107–113.
Chilton, N. B., Huby-Chilton, F., Gasser, R. B., and Beveridge, I. (2006). The Evolutionary Origins of Nematodes within the Order Strongylida Are Related to Predilection Sites within Hosts. Mol. Phylogenet. Evol. 40, 118–128. doi:10.1016/j.ympev.2006.01.003
Cox, D. D., and Todd, A. C. (1962). Survey of Gastrointestinal Parasitism in Wisconsin Dairy Cattle. J. Am. Vet. Med. Assoc. 141, 706–709.
Dalton, H. M., and Curran, S. P. (2018). Hypodermal Responses to Protein Synthesis Inhibition Induce Systemic Developmental Arrest and AMPK-dependent Survival in Caenorhabditis elegans. Plos Genet. 14 (7), e1007520. doi:10.1371/journal.pgen.1007520
Ding, H., Shi, H., Shi, Y., Guo, X., Zheng, X., Chen, X., et al. (2017). Characterization and Function Analysis of a Novel Gene, Hc-Maoc-1, in the Parasitic Nematode Haemonochus Contortus. Parasites Vectors 10 (1), 67. doi:10.1186/s13071-017-1991-1
Gadahi, J. A., Wang, S., Bo, G., Ehsan, M., Yan, R., Song, X., et al. (2016). Proteomic Analysis of the Excretory and Secretory Proteins of Haemonchus contortus (HcESP) Binding to Goat PBMCs In Vivo Revealed Stage-specific Binding Profiles. PLoS One 11, e0159796. doi:10.1371/journal.pone.0159796
Gião, T., Saavedra, J., Cotrina, E., Quintana, J., Llop, J., Arsequell, G., et al. (2020). Undiscovered Roles for Transthyretin: From a Transporter Protein to a New Therapeutic Target for Alzheimer's Disease. Ijms 21 (6), 2075. doi:10.3390/ijms21062075
Goodman, D. S. (1986). “Statement Regarding Nomenclature for the Protein Known as Prealbumin, Which Is Also (Recently) Called Transthyretin”, in Amyloidosis, Springer, 287-288. doi:10.1007/978-1-4613-2199-6_36
Guo, X., Li, Q., Shi, J., Shi, L., Li, B., Xu, A., et al. (2016). Perfluorooctane Sulfonate Exposure Causes Gonadal Developmental Toxicity in Caenorhabditis elegans through ROS-Induced DNA Damage. Chemosphere 155, 115–126. doi:10.1016/j.chemosphere.2016.04.046
Haley, R., Wang, Y., and Zhou, Z. (2018). The Small GTPase RAB-35 Defines a Third Pathway that Is Required for the Recognition and Degradation of Apoptotic Cells. Plos Genet. 14 (8), e1007558. doi:10.1371/journal.pgen.1007558
Herbert, J., Wilcox, J. N., Pham, K.-T. C., Fremeau, R. T., Zeviani, M., Dwork, A., et al. (1986). Transthyretin: A Choroid Plexus-specific Transport Protein in Human Brain: The 1986 S. Weir Mitchell Award. Neurology 36, 900. doi:10.1212/wnl.36.7.900
Hewitson, J. P., Harcus, Y., Murray, J., van Agtmaal, M., Filbey, K. J., Grainger, J. R., et al. (2011). Proteomic Analysis of Secretory Products from the Model Gastrointestinal Nematode Heligmosomoides Polygyrus Reveals Dominance of Venom Allergen-like (VAL) Proteins. J. Proteomics 74, 1573–1594. doi:10.1016/j.jprot.2011.06.002
Hochreiter-Hufford, A., and Ravichandran, K. S. (2013). Clearing the Dead: Apoptotic Cell Sensing, Recognition, Engulfment, and Digestion. Cold Spring Harbor Perspect. Biol. 5, a008748. doi:10.1101/cshperspect.a008748
Hsu, T.-Y., and Wu, Y.-C. (2010). Engulfment of Apoptotic Cells in C. elegans Is Mediated by Integrin α/SRC Signaling. Curr. Biol. 20, 477–486. doi:10.1016/j.cub.2010.01.062
Huang, Y., Wu, J., Chen, X., Tong, D., Zhou, J., Wu, F., et al. (2021). A Zinc Metalloprotease Nas-33 Is Required for Molting and Survival in Parasitic Nematode Haemonchus contortus. Front. Cell Dev. Biol. 9, 695003. doi:10.3389/fcell.2021.695003
Kamath, R., and Ahringer, J. (2003). Genome-wide RNAi Screening in Caenorhabditis elegans. Methods 30, 313–321. doi:10.1016/S1046-2023(03)00050-1
Kamath, R. S., Fraser, A. G., Dong, Y., Poulin, G., Durbin, R., Gotta, M., et al. (2003). Systematic Functional Analysis of the Caenorhabditis elegans Genome Using RNAi. Nature 421, 231–237. doi:10.1038/nature01278
Kebeta, M. M., Hine, B. C., Walkden-Brown, S. W., Kahn, L. P., and Doyle, E. K. (2020). Evaluation of Barbervax Vaccination for Lambing Merino Ewes. Vet. Parasitol. 283, 109187. doi:10.1016/j.vetpar.2020.109187
Kelly, K. O., Dernburg, A. F., Stanfield, G. M., and Villeneuve, A. M. (2000). Caenorhabditis elegans Msh-5 Is Required for Both normal and Radiation-Induced Meiotic Crossing over but Not for Completion of Meiosis. Genetics 156, 617–630. doi:10.1017/S001667230000461410.1093/genetics/156.2.617
Kwon, E.-S., Narasimhan, S. D., Yen, K., and Tissenbaum, H. A. (2010). A New DAF-16 Isoform Regulates Longevity. Nature 466, 498–502. doi:10.1038/nature09184
Laing, R., Kikuchi, T., Martinelli, A., Tsai, I. J., Beech, R. N., Redman, E., et al. (2013). The Genome and Transcriptome of Haemonchus contortus, a Key Model Parasite for Drug and Vaccine Discovery. Genome Biol. 14, R88–R16. doi:10.1186/gb-2013-14-8-r88
Lin, B., Zhuo, K., Chen, S., Hu, L., Sun, L., Wang, X., et al. (2016). A Novel Nematode Effector Suppresses Plant Immunity by Activating Host Reactive Oxygen Species‐scavenging System. New Phytol. 209, 1159–1173. doi:10.1111/nph.13701
Lockshin, R. A., and Zakeri, Z. (2001). Programmed Cell Death and Apoptosis: Origins of the Theory. Nat. Rev. Mol. Cell Biol. 2, 545–550. doi:10.1038/35080097
Ma, G., Gasser, R. B., Wang, T., Korhonen, P. K., and Young, N. D. (2020). Toward Integrative ‘omics of the Barber's Pole Worm and Related Parasitic Nematodes. Infect. Genet. Evol. 85, 104500. doi:10.1016/j.meegid.2020.104500
Mederos, A. E., Ramos, Z., and Banchero, G. E. (2014). First Report of Monepantel Haemonchus contortus Resistance on Sheep Farms in Uruguay. Parasites Vectors 7, 598. doi:10.1186/s13071-014-0598-z
Mello, C. C., Kramer, J. M., Stinchcomb, D., and Ambros, V. (1991). Efficient Gene Transfer in C.Elegans: Extrachromosomal Maintenance and Integration of Transforming Sequences. EMBO J. 10, 3959–3970.
Mitchell, A. L., Attwood, T. K., Babbitt, P. C., Blum, M., Bork, P., Bridge, A., et al. (2018). InterPro in 2019: Improving Coverage, Classification and Access to Protein Sequence Annotations. Nucleic Acids Res. 47, D351–D360. doi:10.1093/nar/gky1100
Mörck, C., and Pilon, M. (2006). C. elegans Feeding Defective Mutants Have Shorter Body Lengths and Increased Autophagy. BMC Dev. Biol. 6, 39. doi:10.1186/1471-213X-6-39
Murphy, C. T., and Hu, P. J. (2013). Insulin/insulin-like Growth Factor Signaling in C. elegans. WormBook, 1–43. doi:10.1895/wormbook.1.164.1
Niciura, S. C. M., Tizioto, P. C., Moraes, C. V., Cruvinel, G. G., de Albuquerque, A. C. A., Santana, R. C. M., et al. (2019). Extreme-QTL Mapping of Monepantel Resistance in Haemonchus contortus. Parasites Vectors 12 (1), 403. doi:10.1186/s13071-019-3663-9
Offenburger, S.-L., Ho, X. Y., Tachie-Menson, T., Coakley, S., Hilliard, M. A., and Gartner, A. (2018). 6-OHDA-induced Dopaminergic Neurodegeneration in Caenorhabditis elegans Is Promoted by the Engulfment Pathway and Inhibited by the Transthyretin-Related Protein TTR-33. Plos Genet. 14, e1007125. doi:10.1371/journal.pgen.1007125
Palmisano, N. J., and Meléndez, A. (2019). Autophagy in C. elegans Development. Developmental Biol. 447 (1), 103–125. doi:10.1016/j.ydbio.2018.04.009
Papaevgeniou, N., Hoehn, A., Tur, J. A., Klotz, L.-O., Grune, T., and Chondrogianni, N. (2019). Sugar-derived AGEs Accelerate Pharyngeal Pumping Rate and Increase the Lifespan of Caenorhabditis elegans. Free Radic. Res. 53, 1056–1067. doi:10.1080/10715762.2019.1661403
Parkinson, J., Mitreva, M., Whitton, C., Thomson, M., Daub, J., Martin, J., et al. (2004). A Transcriptomic Analysis of the Phylum Nematoda. Nat. Genet. 36, 1259–1267. doi:10.1038/ng1472
Qu, G., Fetterer, R., Leng, L., Du, X., Zarlenga, D., Shen, Z., et al. (2014). Ostertagia Ostertagi Macrophage Migration Inhibitory Factor Is Present in All Developmental Stages and May Cross-Regulate Host Functions through Interaction with the Host Receptor. Int. J. Parasitol. 44, 355–367. doi:10.1016/j.ijpara.2014.01.009
Raiders, S., Black, E. C., Bae, A., MacFarlane, S., Klein, M., Shaham, S., et al. (2021). Glia Actively Sculpt Sensory Neurons by Controlled Phagocytosis to Tune Animal Behavior. eLife 10, e63532. doi:10.7554/eLife.63532
Reddien, P. W., and Horvitz, H. R. (2004). The Engulfment Process of Programmed Cell Death in Caenorhabditis elegans. Annu. Rev. Cell Dev. Biol. 20, 193–221. doi:10.1146/annurev.cellbio.20.022003.114619
Riou, M., Koch, C., Delaleu, B., Berthon, P., and Kerboeuf, D. (2005). Immunolocalisation of an ABC Transporter, P-Glycoprotein, in the Eggshells and Cuticles of Free-Living and Parasitic Stages of Haemonchus contortus. Parasitol. Res. 96, 142–148. doi:10.1007/s00436-005-1345-3
Roeber, F., Jex, A. R., and Gasser, R. B. (2013). Impact of Gastrointestinal Parasitic Nematodes of Sheep, and the Role of Advanced Molecular Tools for Exploring Epidemiology and Drug Resistance - an Australian Perspective. Parasites Vectors 6, 153. doi:10.1186/1756-3305-6-153
Rothwell, J. T., and Sangster, N. C. (1993). An In Vitro Assay Utilising Parasitic Larval Haemonchus contortus to Detect Resistance to Closantel and Other Anthelmintics. Int. J. Parasitol. 23, 573–578. doi:10.1016/0020-7519(93)90162-r
Rual, J.-F., Ceron, J., Koreth, J., Hao, T., Nicot, A. S., Hirozane-Kishikawa, T., et al. (2004). Toward Improving Caenorhabditis elegans Phenome Mapping with an ORFeome-Based RNAi Library. Genome Res. 14, 2162–2168. doi:10.1101/gr.2505604
Saverwyns, H., Visser, A., Van Durme, J., Power, D., Morgado, I., Kennedy, M. W., et al. (2008). Analysis of the Transthyretin-like (TTL) Gene Family in Ostertagia Ostertagi - Comparison with Other Strongylid Nematodes and Caenorhabditis elegans. Int. J. Parasitol. 38, 1545–1556. doi:10.1016/j.ijpara.2008.04.004
Savill, J., and Fadok, V. (2000). Corpse Clearance Defines the Meaning of Cell Death. Nature 407, 784–788. doi:10.1038/35037722
Schwarzman, A. L., Gregori, L., Vitek, M. P., Lyubski, S., Strittmatter, W. J., Enghilde, J. J., et al. (1994). Transthyretin Sequesters Amyloid Beta Protein and Prevents Amyloid Formation. Proc. Natl. Acad. Sci. 91, 8368–8372. doi:10.1073/pnas.91.18.8368
Shi, H., Huang, X., Chen, X., Yang, Y., Wang, Z., Yang, Y., et al. (2021). Acyl-CoA Oxidase ACOX-1 Interacts with a Peroxin PEX-5 to Play Roles in Larval Development of Haemonchus contortus. Plos Pathog. 17, e1009767. doi:10.1371/journal.ppat.1009767
Sousa, J. C., Cardoso, I., Marques, F., Saraiva, M. J., and Palha, J. A. (2007). Transthyretin and Alzheimer's Disease: where in the Brain? Neurobiol. Aging 28, 713–718. doi:10.1016/j.neurobiolaging.2006.03.015
Stergiou, L., and Hengartner, M. O. (2004). Death and More: DNA Damage Response Pathways in the Nematode C. elegans. Cell Death Differ 11, 21–28. doi:10.1038/sj.cdd.4401340
Teixeira, M., Matos, A. F. I. M., Albuquerque, F. H. M. A., Bassetto, C. C., Smith, W. D., and Monteiro, J. P. (2019). Strategic Vaccination of Hair Sheep against Haemonchus contortus. Parasitol. Res. 118, 2383–2388. doi:10.1007/s00436-019-06367-x
Vanhamme, L., Souopgui, J., Ghogomu, S., and Ngale Njume, F. (2020). The Functional Parasitic Worm Secretome: Mapping the Place of Onchocerca Volvulus Excretory Secretory Products. Pathogens 9 (11), 975. doi:10.3390/pathogens9110975
Vieira, P., Shao, J., Vijayapalani, P., Maier, T. R., Pellegrin, C., Eves-van den Akker, S., et al. (2020). A New Esophageal Gland Transcriptome Reveals Signatures of Large Scale De Novo Effector Birth in the Root Lesion Nematode Pratylenchus Penetrans. BMC genomics 21 (1), 738. doi:10.1186/s12864-020-07146-0
Wang, C., Li, F., Zhang, Z., Yang, X., Ahmad, A. A., Li, X., et al. (2017). Recent Research Progress in China on Haemonchus contortus. Front. Microbiol. 8, 1509. doi:10.3389/fmicb.2017.01509
Wang, T., Ma, G., Ang, C.-S., Korhonen, P. K., Koehler, A. V., Young, N. D., et al. (2019). High Throughput LC-MS/MS-based Proteomic Analysis of Excretory-Secretory Products from Short-Term In Vitro Culture of Haemonchus contortus. J. Proteomics 204, 103375. doi:10.1016/j.jprot.2019.05.003
Wang, X., Li, W., Zhao, D., Liu, B., Shi, Y., Chen, B., et al. (2010). Caenorhabditis elegans Transthyretin-like Protein TTR-52 Mediates Recognition of Apoptotic Cells by the CED-1 Phagocyte Receptor. Nat. Cell Biol. 12, 655–664. doi:10.1038/ncb2068
Wang, X., Wu, Y.-C., Fadok, V. A., Lee, M.-C., Gengyo-Ando, K., Cheng, L.-C., et al. (2003). Cell Corpse Engulfment Mediated by C. elegans Phosphatidylserine Receptor through CED-5 and CED-12. Science 302, 1563–1566. doi:10.1126/science.1087641
Wong, A., Boutis, P., and Hekimi, S. (1995). Mutations in the Clk-1 Gene of Caenorhabditis elegans Affect Developmental and Behavioral Timing. Genetics 139, 1247–1259. doi:10.1093/genetics/139.3.1247
Yan, B., Guo, X., Zhou, Q., Yang, Y., Chen, X., Sun, W., et al. (2014). Hc-fau, a Novel Gene Regulating Diapause in the Nematode Parasite Haemonchus contortus. Int. J. Parasitol. 44, 775–786. doi:10.1016/j.ijpara.2014.05.011
Yatsuda, A. P., Krijgsveld, J., Cornelissen, A. W. C. A., Heck, A. J. R., and de Vries, E. (2003). Comprehensive Analysis of the Secreted Proteins of the Parasite Haemonchus contortus Reveals Extensive Sequence Variation and Differential Immune Recognition. J. Biol. Chem. 278, 16941–16951. doi:10.1074/jbc.M212453200
Zajac, A. M., and Garza, J. (2020). Biology, Epidemiology, and Control of Gastrointestinal Nematodes of Small Ruminants. Vet. Clin. North America: Food Anim. Pract. 36 (1), 73–87. doi:10.1016/j.cvfa.2019.12.005
Zhang, L., Mou, L., Chen, X., Yang, Y., Hu, M., Li, X., et al. (2018). Identification and Preliminary Characterization of Hc-Clec-160, a Novel C-type Lectin Domain-Containing Gene of the Strongylid Nematode Haemonchus contortus. Parasites Vectors 11, 1–8. doi:10.1186/s13071-018-3005-3
Zhang, Y., Wang, H., Kage-Nakadai, E., Mitani, S., and Wang, X. (2012). C. elegans Secreted Lipid-Binding Protein NRF-5 Mediates PS Appearance on Phagocytes for Cell Corpse Engulfment. Curr. Biol. 22, 1276–1284. doi:10.1016/j.cub.2012.06.004
Keywords: Haemonchus contortus, transthyretin-like protein, post-embryonic larval development, apoptosis, phagocytosis
Citation: Shi H, Huang X, Chen X, Yang Y, Wu F, Yao C, Ma G and Du A (2021) Haemonchus contortus Transthyretin-Like Protein TTR-31 Plays Roles in Post-Embryonic Larval Development and Potentially Apoptosis of Germ Cells. Front. Cell Dev. Biol. 9:753667. doi: 10.3389/fcell.2021.753667
Received: 05 August 2021; Accepted: 22 October 2021;
Published: 03 November 2021.
Edited by:
Qingfeng Zhang, Tongji University, ChinaReviewed by:
Shuai Wang, Xinxiang Medical University, ChinaChunqun Wang, Huazhong Agricultural University, China
Copyright © 2021 Shi, Huang, Chen, Yang, Wu, Yao, Ma and Du. This is an open-access article distributed under the terms of the Creative Commons Attribution License (CC BY). The use, distribution or reproduction in other forums is permitted, provided the original author(s) and the copyright owner(s) are credited and that the original publication in this journal is cited, in accordance with accepted academic practice. No use, distribution or reproduction is permitted which does not comply with these terms.
*Correspondence: Guangxu Ma, Z3htYTFAemp1LmVkdS5jbg==; Aifang Du, YWZkdUB6anUuZWR1LmNu
†These authors have contributed equally to this work and share first authorship