- 1Department of Otolaryngology, University of Michigan, Ann Arbor, MI, United States
- 2Department of Speech, Language, and Hearing, University of Texas at Dallas, Richardson, TX, United States
- 3Department of Surgery, Southern Illinois University School of Medicine, Springfield, IL, United States
Calcitonin-gene-related peptide (CGRP) is a lateral olivocochlear (LOC) efferent neurotransmitter. Depression of sound-driven auditory brainstem response amplitude in CGRP-null mice suggests the potential for endogenous CGRP release to upregulate spontaneous and/or sound-driven auditory nerve (AN) activity. We chronically infused CGRP into the guinea pig cochlea and evaluated changes in AN activity as well as outer hair cell (OHC) function. The amplitude of both round window noise (a measure of ensemble spontaneous activity) and the synchronous whole-nerve response to sound (compound action potential, CAP) were enhanced. Lack of change in both onset adaptation and steady state amplitude of sound-evoked distortion product otoacoustic emission (DPOAE) responses indicated CGRP had no effect on OHCs, suggesting the origin of the observed changes was neural. Combined with results from the CGRP-null mice, these results appear to confirm that endogenous CGRP enhances auditory nerve activity when released by the LOC neurons. However, infusion of the CGRP receptor antagonist CGRP (8–37) did not reliably influence spontaneous or sound-driven AN activity, or OHC function, results that contrast with the decreased ABR amplitude measured in CGRP-null mice.
Introduction
A variety of evidence suggests a role for Calcitonin-gene-related-peptide (CGRP) in auditory function, with CGRP released by the lateral olivocochlear (LOC) efferent neurons mediating the neural response to sound. The guinea pig is a common model for studies of the LOC system. LOC efferents have been immunolabeled with antibodies to CGRP in the cochlea in guinea pigs (Takeda et al., 1987; Sliwinska-Kowalska et al., 1989; Ylikoski et al., 1989; Ohno et al., 1993; Cabanillas and Luebke, 2002). Other studies of the LOC system document CGRP-like immunoreactivity in additional species including mice (Maison et al., 2003a; Wu et al., 2018), rats (Kitajiri et al., 1985; Takeda et al., 1986; Lu et al., 1987; Tohyama et al., 1989; Ylikoski et al., 1989; Kuriyama et al., 1990; Merchan-Perez et al., 1990), hamsters (Simmons et al., 1996), cats (Lu et al., 1987), and humans (Kong et al., 2002).
Immunolabeling of the human cochlea revealed CGRP-like immunoreactivity in the inner spiral bundle and tunnel spiral bundle suggesting expression of CGRP in both the LOC and medial olivococlear (MOC) efferent neurons with no notable differences from base to apex (Schrott-Fischer et al., 2007). CGRP immunoreactive terminals are distributed under the inner hair cells (IHCs) in approximately equal numbers throughout the length of the cochlea in rats (Vetter et al., 1991) and mice (Maison et al., 2003a) although some mouse strains may have a gradient with less robust CGRP presence in the apex (Wu et al., 2018). There are some reports that CGRP-positive labeling of the LOC system is robust across the length of the guinea pig cochlea (Sliwinska-Kowalska et al., 1989; Ylikoski et al., 1989) although there are also reports that CGRP-positive cells are less abundant in the apex and upper third turn of the guinea pig cochlea (Safieddine and Eybalin, 1992). Sliwinska-Kowalska et al. (1989) investigated CGRP distribution in pigmented female guinea pigs weighing 150–300 g, Ylikoski et al. (1989) used 200–350 G guinea pigs but did not specify sex or strain, and Safieddine and Eybalin (1992) used pigmented guinea pigs weighing 200–250 g with sex not specified, thus it is not known if there are sex or strain (pigmented/albino) differences in CGRP distribution. In the only study assessing potential sex differences in the effects of CGRP within the LOC system, Allen and Luebke (2017) reported no functional differences between male and female CGRP-null mice. The lack of sex differences in that mouse study contrasts with sex differences in CGRP immunoreactivity observed in other tissues from rat and from human (Valdemarsson et al., 1990; Herbison and Spratt, 1995; Ji et al., 2019), with sex differences in humans potentially explaining sex differences in the efficacy of antimigraine medications that target CGRP receptors (Barbanti et al., 2021; de Vries Lentsch et al., 2021).
The mature CGRP receptor is a G-protein coupled receptor (GPCR) composed of three proteins, including calcitonin-like receptor (CLR), receptor activity-modifying protein (RAMP1), and CGRP-receptor component protein (RCP) (for additional detail, see Dickerson et al., 2016). Post-natal maturation of the CGRP receptor has been documented in the mouse (Dickerson et al., 2016). In mice, the CGRP receptor complex within the cochlea shows developmental maturation over the first 3-months (Dickerson et al., 2016). The guinea pig is precocial, whereas the mouse is altricial, and receptor development may follow different timelines in guinea pig and mouse. Post-natal receptor maturation of the CGRP receptor has not been studied in the guinea pig. Interestingly, observations of CGRP expression in the Type II SGNs have also been reported, although release of CGRP from type II afferent terminals in the cochlear nucleus has not been established and functional actions are not yet determined (Wu et al., 2018; Vyas et al., 2019).
The lateral superior olive (LSO) is the site of origin for the descending LOC efferent neurons. CGRP-like immunolabeling of the LSO is also well described in multiple species (Lu et al., 1987; Vetter et al., 1991; Simmons and Raji-Kubba, 1993; Safieddine et al., 1997; Simmons et al., 1997; Moore et al., 1999; Reuss et al., 1999). Co-localization of CGRP and other LOC transmitters has been described by several groups, and species differences have emerged with respect to colocalization as well as overall distribution. For example, in the human LSO, Moore et al. (1999) describe CGRP-positive terminals that are also positive for antibodies to choline acetyltransferase (ChAT); these cells were distinct from a second population which immunostained for ChAT but not CGRP. In the rat LSO and cochlea, Vetter et al. (1991) describe CGRP-positive terminals that are also positive for antibodies to ChAT; these cells were distinct from a second population which immunostained with antibodies to glutamic acid decarboxylase (GAD, the enzyme that decarboxylates glutamate to make GABA). In contrast, in the mouse, GABA and CGRP were extensively co-localized with ACh in LOC terminals (Maison et al., 2003a), whereas dopaminergic (DA) neurons were a separate subpopulation (Darrow et al., 2006). In studies evaluating CGRP co-localization with other LOC transmitters in guinea pigs, LSO cell bodies have been found to co-localize CGRP and encephalin (enk) (Tohyama et al., 1990), or CGRP, enk, and ACh (Safieddine and Eybalin, 1992). Finally, extensive colocalization has been observed with double and triple immunolabeling for ChAT and either CGRP, enk, GAD, or tyrosine hydroxylase (TH, the enzyme responsible for catalyzing the conversion of L-tyrosine to the DA precursor dihydroxyphenylalanine) (Safieddine et al., 1997).
That CGRP is present in the LSO and the LOC terminals in a variety of species suggests the potential for a functional role of CGRP in modulating the ascending auditory signal. This possibility has been explored, and supported, by data from a small number of studies. Depressed amplitude of auditory brainstem response (ABR) wave I in αCGRP-null mice (Maison et al., 2003b) has been shown, an effect that is consistent with excitatory modulation of sound-evoked auditory nerve (AN) activity by CGRP. One interpretation is that sound stimulation drives release of CGRP by LOC efferents during acoustic stimulation of the LOC reflex loop, and in the absence of CGRP release (in the αCGRP-null mice), sound-evoked responses are depressed. Alternatively, if CGRP is tonically released, the observed effects might also be explained by changes in AN spontaneous response properties. In mammals, AN fibers with higher spontaneous firing rates have lower (better) thresholds, are less sharply tuned, and adapt to sound more rapidly than other fibers with lower spontaneous firing rates (see Sachs and Abbas, 1974; Liberman, 1978; Rhode and Smith, 1985; Winter et al., 1990; Muller and Robertson, 1991; Yates, 1991). Consistent with the hypothesis that CGRP can increase spontaneous neural activity, application of CGRP produced an increase in spontaneous neural activity in the Xenopus lateral line organ (Adams et al., 1987; Sewell and Starr, 1991; Bailey and Swell, 2000a; Bailey and Swell, 2000b).
In the study by Maison et al. (2003b), which revealed that ABR amplitude was depressed in αCGRP-null mice, there were no corresponding changes in ABR threshold, distortion product otoacoustic emission (DPOAE) threshold and amplitude, or the strength of the MOC reflex. Allen and Luebke (2017) similarly report intact DPOAE responses in αCGRP-null mice. The lack of change in DPOAE outcomes [reflecting no change in outer hair cell (OHC) function] and the MOC metric are perhaps somewhat surprising. Earlier literature described CGRP-containing nerve endings on OHCs in rodents (Cabanillas and Luebke, 2002; Maison et al., 2003a) and humans (Kong et al., 2002; Schrott-Fischer et al., 2007), suggesting CGRP may function as an MOC transmitter as well as an LOC transmitter (see also Vyas et al., 2019). The limited DPOAE changes detected in the knock-out mice might provide an incomplete description of the role of CGRP in auditory physiology, however, if there is central nervous system compensation during development (as suggested by May et al., 2002, who evaluated auditory sensitivity in mice lacking alpha-9 receptors). In another recent investigation, mature CGRP-null mice (3 months of age) not only had reduced cochlear nerve activity, they were also observed to have increased thresholds both in quiet and in broadband noise background (with thresholds measured using pre-pulse inhibition of the acoustic startle reflex) (Allen and Luebke, 2017). Development of deficits from 1 to 3 months of age was consistent with the timing of CGRP receptor maturation in mice shown by Dickerson et al. (2016).
In contrast to earlier research using CGRP-null mice, the current study evaluated the effects of infusing CGRP into the cochlea on auditory function, including assessments for both LOC and MOC-like functional effects. Effects of CGRP on sound-evoked response were evaluated using both CGRP and CGRP fragment 8–37, which acts as an antagonist at CGRP receptors. To evaluate the hypothesis that CGRP mediates changes in spontaneous AN activity as well as sound-driven AN activity, we measured electrical activity at the round window membrane, which provides a measure of ensemble spontaneous activity of the AN fiber population (Kiang et al., 1976; Dolan et al., 1990; Groff and Liberman, 2003). Changes in DPOAE amplitude and onset adaptation were used to assess effects of the study drugs on OHC responses. The use of experimental designs that combine both agonists and antagonists has allowed the effects of exogenous drug delivery and tonic endogenous release to be distinguished for the LOC transmitter DA (Garrett et al., 2011); the current data extend understanding of putative LOC transmitter substances to the peptide CGRP.
Methods
Animal Model
Fourteen guinea pigs (Elm Hill Breeding Labs, Chelmsford, MA) were used in these experiments (5 female, 5 male, sex records not available for 4 animals). Two additional animals were excluded from the investigation based on permanent profound post-operative hearing loss. Animal weights were ∼250–300 g on arrival (approximately 4 weeks old) and ∼350–500 g (approximately 6 weeks old) at study entry. All animals were maintained with free access to food (Guinea Pig Chow, PMI Nutrition International Inc., Brentwood, MO) and water. The animal care program was AALAC accredited. Husbandry met or exceeded all applicable standards, including the Guide for the Use and Care of Laboratory Animals, prepared by the National Research Council (1996). The University Committee on Use and Care of Animals at the University of Michigan approved all animal care and testing protocols.
Apparatus and Procedures
Surgical Procedure
The studies described here used a chronic unilateral left-ear drug-infusion paradigm. CGRP has a molecular weight of ∼3,800; it is a large enough molecule that it is unlikely to readily diffuse across the round window membrane (as larger molecules less readily diffuse across this membrane, see Goycoolea and Lundman, 1997; Mikulec et al., 2008). Therefore, it was delivered directly into the cochlea using a surgically implanted cannula. The surgical implant procedures were closely modeled after those we have described previously (Le Prell et al., 2004). Animals were anesthetized (40 mg/kg ketamine, 10 mg/kg xylazine), then a cannula filled with an artificial perilymph (AP) solution (145 mM NaCl, 2.7 mM KCl, 2.0 mM MgSO4, 1.2 mM CaCl2, 5.0 mM HEPES; pH = 7.40, osmolality = 280–285 mOsm) was inserted through the wall of the left cochlea via a small fenestra slightly lateral to the round window. The site of insertion was at a place with a best frequency of approximately 22.4 kHz (based on surgically-induced threshold deficits described in Le Prell et al., 2004). A silastic ball located 0.5 mm away from the end of the cannula prevented over-insertion of the cannula and prevented leaking of fluids from the cochlea. The other end of the cannula was connected to an osmotic mini-pump (model 2002, 0.5 μl/h x 14 days; Durect corporation, Cupertino, CA), also filled with the AP solution.
After inserting the cannula, a ball electrode (0.25 mm diameter, constructed of teflon-coated platinum-iridium wire) was carefully placed on the left round window membrane following procedures we have described previously (Le Prell et al., 2006). A ground wire was inserted into the middle ear via the defect in the bulla, and carboxylate cement (Durelon, ESPE, Germany) was used to seal the bulla defect and permanently fix both the cannula and the electrodes in place. The opposing ends of the electrodes were soldered to a two-pin connector (HSS-132-G2, Samtec Inc., IN) prior to the onset of the surgical procedure. Methyl methacrylate cement (Jet Repair Acrylic, Lang Dental Manufacturing, IL) was used to fix the cannula and the connector for the electrodes to the skull, and to seal the tissue edges surrounding the head-mounted connector. The post-auricular incision was then sutured and the incision cleaned.
AP was infused unilaterally for 12–14 days during which a variety of post-operative baseline data were collected. Guinea pigs then underwent a brief surgical procedure during which a small incision exposed the depleted osmotic pump, the depleted pump was extruded, the cannula clamped and cut, and a new osmotic-pump attached. The new pump was filled with either 200 μM CGRP (CGRP rat, Sigma Chemical C0292, CAS No. 96827-03-1; dissolved in AP) (n = 5; four female, sex not recorded for one animal) or 250 μM CGRP fragment 8–37 (synthetic human CGRP fragment 8–37, Sigma Chemical C2806, CAS No. 119911-68-1; dissolved in AP) (n = 7; four male, one female, sex not recorded for two animals).
Electrophysiology
Animals were anesthetized (20 mg/kg ketamine, 5 mg/kg xylazine), and placed on a warm heating pad to maintain body temperature. Acoustic stimuli were brief pure-tone stimuli (4, 8, 16, or 22.4 kHz) presented at levels ranging from 0 to 100-dB SPL in 5-dB increments (5-msec duration, 0.5-msec rise-fall; 10/sec). Acoustic stimuli were generated using Tucker-Davis Technology (TDT; Alachua, FL) System II/System III hardware and SigGen32 software. Signals were converted to analog (DA1), filtered (FT6-2, Fc = 40 kHz), attenuated (PA5), and presented using a 200-Ohm transducer (Beyer Dynamic, Farmingdale, NY) coupled to the animals’ ear canal via vinyl tubing. Cochlear potentials were filtered (300–3,000 Hz) and amplified (1,000x) using a Grass P55 amplifier. BioSig32 (TDT) was used to average 25 evoked responses for each frequency/level combination. Threshold to evoke a compound action potential (CAP) response of the auditory nerve was defined as the sound level that produced a 10-μV response; threshold estimates were determined using linear interpolation. CAP responses were measured using the implanted electrode; thus, testing was unilateral. CAP testing was repeated at 2–3 day intervals to monitor stability of post-surgical responses during AP infusion (the control condition), with additional testing at 2–3 day intervals during CGRP or CGRP (8–37) infusion (the experimental conditions) to monitor progressive changes as the experimental substances increased in concentration in the cochlea with continued infusion.
Round Window Noise
Baseline tests were conducted once during the second week of unilateral AP infusion (between days 9–11); repeat testing during drug infusion was conducted on the 11th day of either CGRP (n = 4; one animal not tested) or CGRP (8–37) (n = 7) infusion. RWN recordings were performed in anesthetized animals (20 mg/kg ketamine, 5 mg/kg xylazine) maintained on a warm heating pad. RWN was measured using the round window recording electrode. Using a digital oscilloscope, fast Fourier transformation of ensemble activity was conducted as in Dolan et al. (1990). The broad spectral peak (0.8–1.0 kHz) that is typical of round-window noise was measured. RWN was measured using the implanted electrode; thus, testing was unilateral.
DPOAE
Baseline DPOAE tests were conducted once during the second week of unilateral AP infusion (days 9–11); repeat testing during CGRP infusion was conducted on the 9th day of CGRP (n = 4; one animal not tested) or CGRP (8–37) (n = 5; two animals not tested). Animals were anesthetized (58.8 mg/kg ketamine, 2.4 mg/kg xylazine, and 1.2 mg/kg acepromazine), and placed on a warm heating pad to maintain body temperature, then time-dependent amplitude of the cubic DPOAE (2F2-F1) was assessed using procedures we have described in detail previously (Halsey et al., 2005; Le Prell et al., 2005). In brief, the DPOAE tests were conducted using TDT system II/III equipment in combination with an Etymotic Research microphone-earphone assembly (ER-10B + Low Noise Microphone) coupled to the subject’s ear by a short segment of flexible vinyl tubing, minimally smaller than the guinea pig ear canal and which sealed the ear canal to provide a closed field test condition. Testing was unilateral and limited to the experimental ear.
Onset adaptation of the DPOAE is a measure of the strength of the MOC reflex (see Liberman et al., 1996; Maison and Liberman, 2000; Kujawa and Liberman, 2001). Onset adaptation of the cubic distortion product was measured as described previously (Halsey et al., 2005; Le Prell et al., 2005); primary tone frequencies (F1, F2) were fixed at 8 kHz (F1) and 9.6 kHz (F2) and the cubic distortion product (2F1-F2) was 6.4 kHz. F1 and F2 levels (L1, L2) were initially set to approximately 92-dB SPL; L2 was then systematically decreased over a 12-dB range in 1-dB steps. This procedure was repeated for at least six levels of F1, with F1 decreasing in 1-dB steps, until the levels producing maximum DPOAE adaptation were determined for both positive and negative deflections. Additional tests were then conducted with L1 and L2 level increments changing in 0.4-dB increments over at least six levels of F1, with twelve F2 levels presented for each level of F1. A MatLab program was used to control stimulus generation (TDT hardware) and presentation (Beyer sound drivers), as well as data collection.
Primary tones were 1 s in duration, with a 1.5 s pause between presentations. Sound levels for F1, F2, and the DPOAE were determined during each level series using Fourier transform of the microphone input. For each level combination, responses to four stimulus presentations were collected and averaged. DPOAE amplitude was sampled at 50-msec intervals during the 1-s primary tone duration. If standard deviations exceeded 2-dB at any time point, the data were excluded and the level combination was repeated. Adaptation of the DPOAE response was defined as the difference between DPOAE amplitude at the onset of the primary tones and the steady-state amplitude of the DPOAE (defined as the average DPOAE amplitude during the final four time points).
Statistical Analysis
All data values in the text and figures are mean ± S.E.M.; all statistical comparisons were performed using SPSS 13.0. Statistical reliability of group differences was via ANOVA. Adjustment for violation of sphericity was accomplished using the Greenhouse-Geisser correction. Bonferroni corrections were applied for multiple comparisons.
CAP data collected during unilateral AP infusion were evaluated as a function of time post-implant (AP1: days 3–8; AP2: days 12–14) to assess possible post-operative changes in function. Functional data collected during unilateral CGRP infusion were evaluated as a function of the duration of drug delivery (CGRP1: days 2–5; CGRP2: days 6–8; CGRP3: days 13–14) to account for increasing intra-cochlear CGRP concentration during the 14-day infusion period.
Results
Changes in Evoked Potentials During AP Infusion
Threshold changes during AP were minimal, consistent with Brown et al. (1993) and Le Prell et al. (2004). Whereas Brown et al. (1993) monitored ABR threshold measures in saline-infused animals as a surgical control during the development of this surgical procedure, Le Prell et al. (2004) measured both ABR thresholds and behavioral thresholds during 14–56 days of AP infusion prior to drug manipulation (Le Prell et al., 2004). With respect to CAP amplitude, there was a statistically significant Frequency x Replication (AP1, AP2) effect (p = 0.042) of time post-implant on CAP amplitude. Pair-wise comparisons revealed that differences between AP1 and AP2 measurements were limited to a small but statistically reliable decrease in CAP amplitude at 4 (p = 0.037) and 8 (p = 0.045) kHz, with no time-related changes in CAP amplitude at 16 or 24 kHz (p’s > 0.05). Larger decreases in amplitude were evident in the surgical controls included by Sly et al. (2012). They reported small but largely reversible post-surgical reductions in DPOAE amplitude and slowly progressive decreases in ABR wave III amplitude over 4-week of infusion of Ringer’s solution which they attributed to surgical trauma to the cochlea. Effects of CGRP were measured relative to the AP2 baseline collected during days 12–14 of AP infusion.
Intra-Cochlear CGRP Improved Threshold Sensitivity
Threshold sensitivity during AP, CGRP, and CGRP (8–37) antagonist infusion are shown in Figures 1A,B; because each subject contributed both baseline (AP) and either CGRP or CGRP (8–37) data points, the within-subject change in threshold is also shown (Figure 1C). CAP threshold was lower (better) during CGRP infusion when compared to within-subject pre-drug (AP) baseline (p = 0.043); after Bonferroni correction, the only pair-wise comparison that approached statistical reliability was AP versus the latter CGRP time point (days 13–14, see Figure 1A) (F1,4 = 6.998, p = 0.057). To determine which frequencies had reliable differences between AP and CGRP days 13–14 thresholds, two-tailed paired t-tests were completed at each frequency. Differences were statistically significant at 4 kHz (t4 = 3.661, p = 0.0216) and 8 kHz (t4 = 7.682, p = 0.00159), and approached statistical significance at 16 kHz (t4 = 2.275p = 0.0853), but not at 24 kHz (t4 = 0.851, p = 0.443). CAP threshold measured during infusion of the CGRP receptor antagonist, CGRP (8–37), was not different from baseline (1B) (F1,6 = 0.187, p = 0.681) and no additional pair-wise comparisons were completed within test frequencies. When change in threshold (on day 13–14 of infusion) was compared as a function of the treatment agent [CGRP vs. CGRP (8–37)] using a two-way ANOVA with treatment and frequency as factors, there was a statistically significant difference between the treatment groups (F1,47 = 6.003, p = 0.019) with no effect of frequency (F3,47 = 0.532, p = 0.663) and no drug × frequency interaction (F3,47 = 0.0700, p = 0.976).
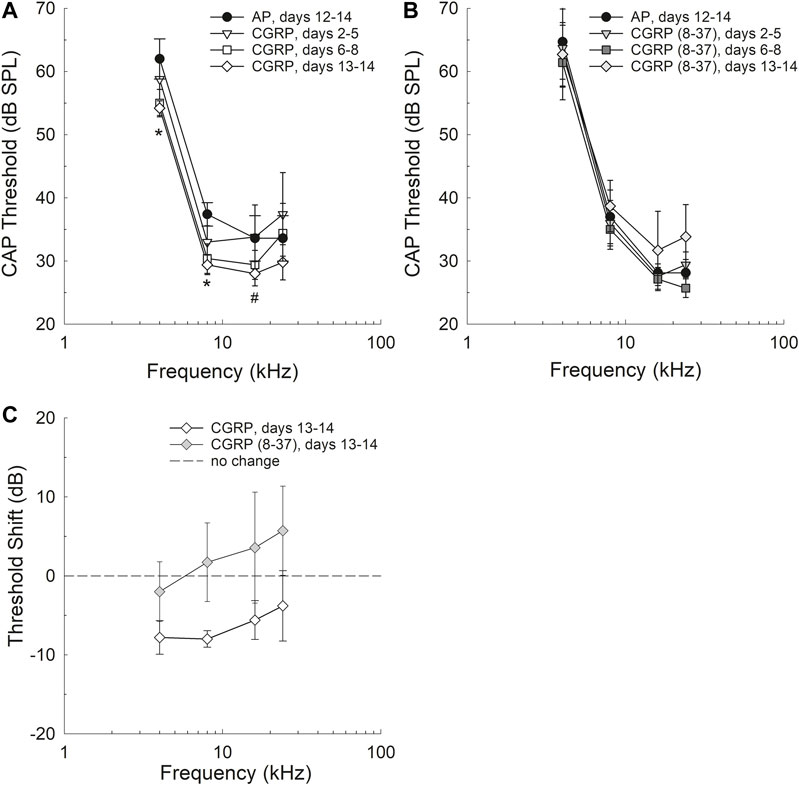
FIGURE 1. Threshold sensitivity was improved after 13–14 days of CGRP agonist infusion, but not at earlier infusion times including days 2–5 and 6–8 (A). Differences between AP and CGRP thresholds were statistically significant at 4 and 8 kHz (see asterisks) and approached statistical significance at 16 kHz (p = 0.0853). Threshold sensitivity after 13–14 days of CGRP antagonist [CGRP (8–37)] infusion was not reliably different from AP control (B) and no pair-wise comparisons were completed. The change in thresholds as a function of drug was significantly different when threshold shift as a function of drug assignment was compared (C).
Intra-Cochlear CGRP Enhanced CAP Amplitude
CGRP-induced increases in CAP amplitude were time-, frequency-, and intensity-dependent (see Figure 2).
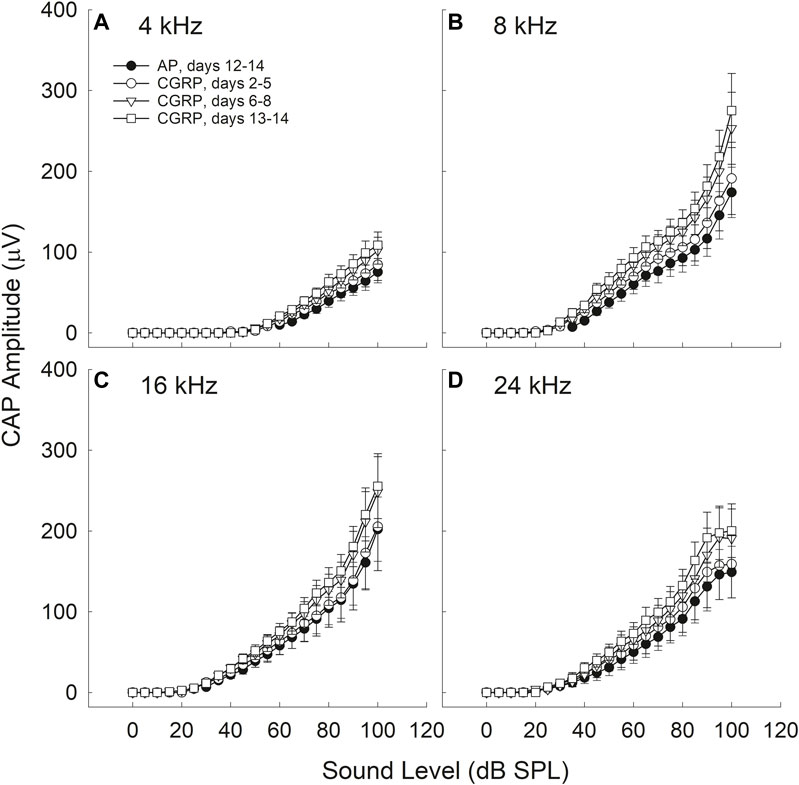
FIGURE 2. CAP amplitude increased during CGRP infusion; all comparisons are relative to control measurements on the 12th-14th day of artificial perilymph (AP) infusion, immediately prior to the pump change surgery during which the AP pump was replaced with a pump containing CGRP. Increases in CAP amplitude (30–35%) were statistically reliable at 4 (A), 8 (B), 16 (C), and 24 (D) kHz for the CGRP (days 13–14) comparisons with the AP data set (all p‘s < 0.005). CGRP-induced changes are shown as a function of duration of infusion; tests were conducted at least once from days 2–5, on days 6, 7, or 8, and on days 13 or 14.
Time-Dependence
There were no reliable CGRP-induced changes in CAP amplitude during the earliest infusion days (days 2–4). When CAP amplitude was assessed on days 6–8 of infusion, the increase in CAP amplitude (20–30%) approached statistical reliability (4 kHz, p = 0.055; 8 kHz, p = 0.071; 16 kHz; p = 0.136; 24 kHz, p = 0.053). The most robust increases in CAP amplitude (30–35%) were observed at the 13–14 days time point, and these amplitude increases were statistically reliable (4 kHz, p = 0.016; 8 kHz, p = 0.026; 16 kHz, p = 0.052; 24 kHz, p = 0.027). Time-dependent changes are likely a consequence of the slow infusion rate of the osmotic mini-pump, with significant drug accumulations building slowly over multiple days or weeks (for discussion see Le Prell et al., 2004).
Frequency-Dependence
For the data set collected on the 13th and 14th days of infusion, a time at which the effects of CGRP on CAP amplitude were highly reliable, there was a statistically significant Condition × Frequency interaction (p = 0.022). The difference between AP and CGRP was smaller at 4 kHz than at the other (higher) test frequencies. Smaller effects at the lowest frequencies, furthest from the site of infusion, are not surprising as drug diffusion depends on the slow cochlear fluid gradient (approximately 1.6 nl/min, see Ohyama et al., 1988; for discussion see Le Prell et al., 2004). LOC innervation has been sparser in the apex than in the base in some (Safieddine and Eybalin, 1992) but not all (Sliwinska-Kowalska et al., 1989; Ylikoski et al., 1989) reports thus frequency effects could be related not only to drug distribution but also to CGRP receptor distribution.
Intensity-dependence
The difference in CAP amplitude during AP and CGRP increased with increasing intensity (4 kHz: p = 0.028; 8 kHz: p = 0.077; 16 kHz: p = 0.069; 24 kHz: p = 0.019). In contrast to the statistically significant time, frequency, and intensity dependent effects of CGRP infusion, there were no statistically significant treatment effects for CGRP (8–37) (F1,6 = 0.004, p = 0.984) (Figure 3).
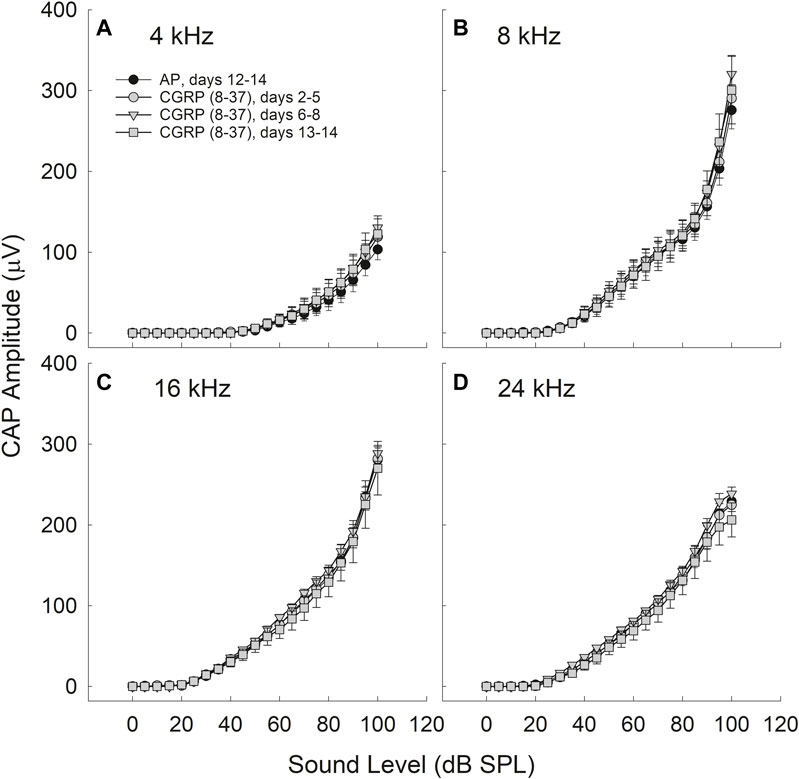
FIGURE 3. CAP amplitude did not change as a function of CGRP (8–37) infusion at 4 (A), 8 (B), 16 (C), and 24 (D) kHz. CAP tests were conducted at least once from days 2–5, on days 6, 7, or 8, and on days 13 or 14 for CGRP (8–37). All comparisons are relative to control measurements on the 12th–14th day of artificial perilymph (AP) infusion, immediately prior to the pump change surgery during which the AP pump was replaced with a pump containing CGRP (8–37).
Intra-Cochlear CGRP Increased Spontaneous Neural Activity
Electrical activity at the round window increased during CGRP infusion in the 800–1,200 Hz region (F1,3 = 13.025, p = 0.037); this “hump” region reflects spontaneous firing of multiple auditory nerve fibers (Figure 4A) (for discussion of ‘hump’ region, see Kiang et al., 1976; Dolan et al., 1990; Groff and Liberman, 2003). In contrast, comparisons between AP and the antagonist, CGRP (8–37), revealed no significant differences in electrical activity (F1,6 = 3.229, p = 0.122) (Figure 4B). Baseline measures in the group of guinea pigs that later received CGRP systematically differed from the baseline measures in the group that later received CGRP (8–37) (F1,9 = 4.830, p = 0.056 when all frequencies were used and F1,9 = 5.625, p = 0.042 when only frequencies less than 1,100 Hz were used).
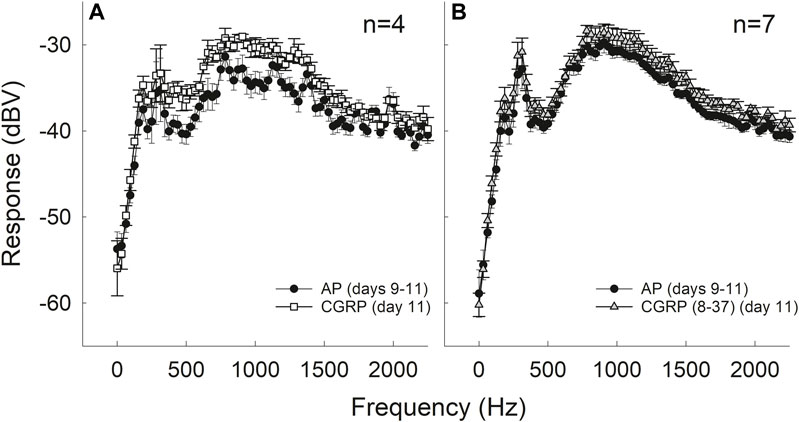
FIGURE 4. Intra-cochlear infusion of CGRP enhanced round window noise from approximately 500–1,200 Hz; the frequency range associated with spontaneous firing of the auditory nerve (A). There were no changes in round window noise during infusion of CGRP (8–37) (B).
Intra-Cochlear CGRP Does not Affect MOC Reflex Strength
The strength of the MOC reflex, measured using onset adaptation of the DPOAE, was essentially the same during AP, CGRP infusion, and CGRP (8–37). DPOAE adaptation is illustrated for one representative animal in Figure 5 (5A: AP; 5B: CGRP). Adaptation was clearly robust (>20 dB) in both conditions; mean adaptation data for all animals is illustrated in Figure 5 [5C: CGRP; 5D: CGRP (8–37)]. Although adaptation magnitude was seemingly reduced during infusion of the CGRP receptor antagonist, these differences were not statistically reliable (all p’s > 0.25). In contrast to the lack of effect shown here, manipulations known to disrupt the MOC system, including intra-venous strychnine and OCB transection, cause a profound depression of DPOAE adaptation (approximately 20-dB, see Le Prell et al., 2005).
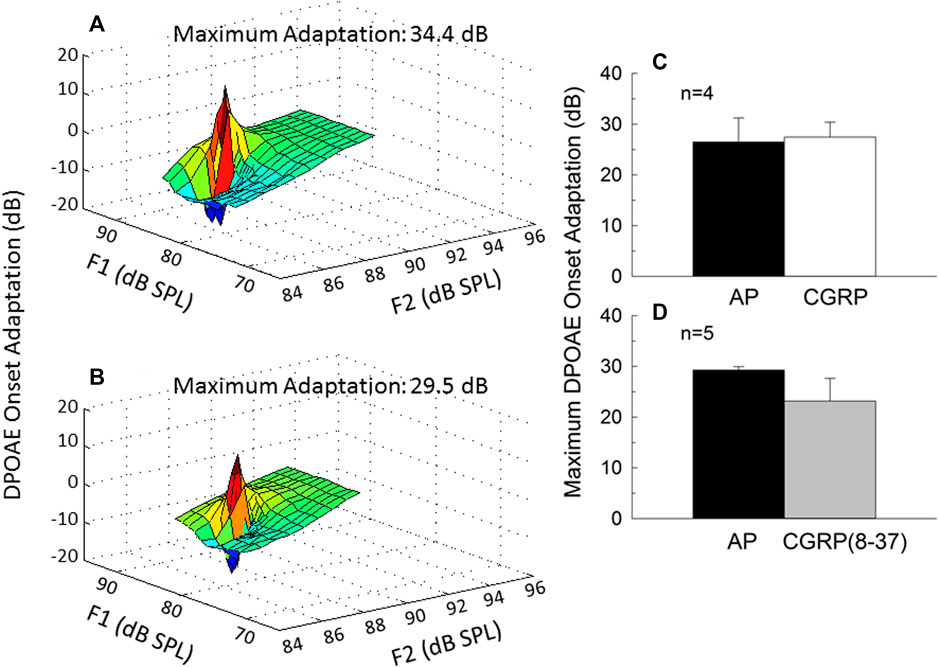
FIGURE 5. Onset adaptation of the outer hair cell distortion product otoacoustic emission (DPOAE) shows a rapid level-dependent adaptation shortly after signal onset when the medial olivocochlear pathway is intact. DPOAE adaptation is depicted on day 9 of artificial perilymph (A) and CGRP (B) infusion for one representative animal. The maximum adaptation was extracted from the fine-structure plots shown in (A,B). Onset adaptation was not reliably affected by either CGRP (C) or CGRP (8–37) (D) (all p’s > 0.25).
Intra-Cochlear CGRP Does not Affect Outer Hair Cell Function
DPOAE amplitude, a measure of the functional integrity of the OHC population, is illustrated for one representative animal in Figure 6 (6A: AP; 6B: CGRP). Mean steady-state amplitude data for all animals is illustrated in Figure 6 [6C: CGRP; 6D: CGRP (8–37)]. The lack of effect of CGRP and CGRP (8–37) on DPOAE amplitude indicates that OHC function was not influenced by intra-cochlear infusion of CGRP ligands, at least within the range of levels tested here. Lack of effect of CGRP and CGRP (8–37) contrasts with profound depression of DPOAE steady-state amplitude following intra-cochlear neomycin (Le Prell et al., 2004) or euthanasia with an overdose of sodium pentobarbital (Le Prell et al., 2005).
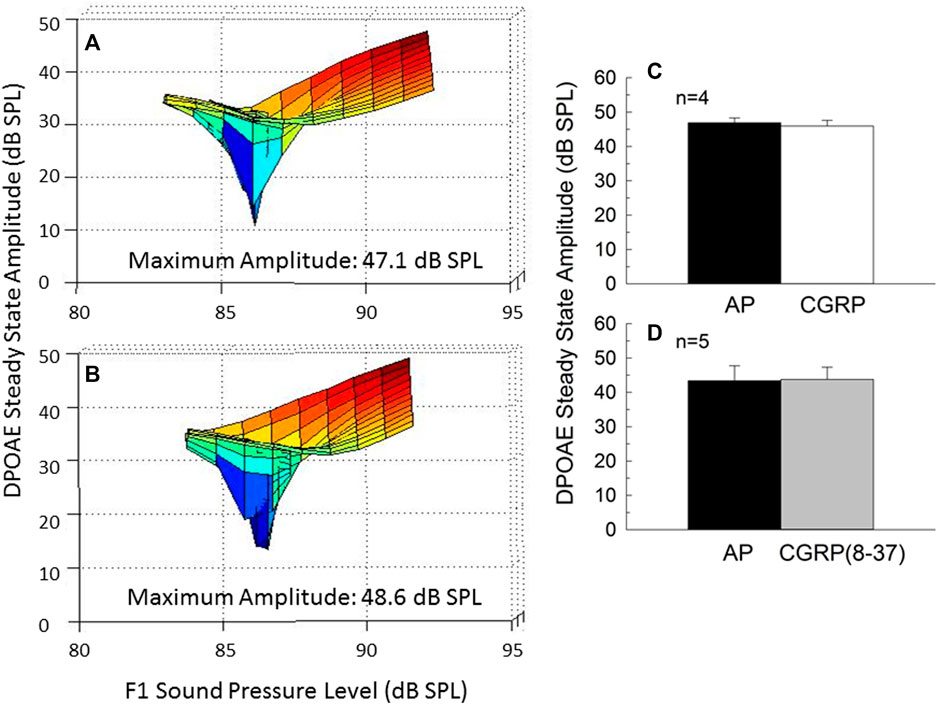
FIGURE 6. Steady-state amplitude of the outer hair cell distortion product otoacoustic emission (DPOAE) varies with F1 and F2 sound level, and provides as a measure of the integrity of the outer hair cell population. DPOAE amplitude is depicted on day 9 of artificial perilymph (A) and CGRP (B) infusion for one representative animal. The steady state amplitude was extracted from the fine-structure plots shown in (A,B). The level-dependent steady-state DPOAE amplitude was unchanged by either CGRP (C) or CGRP (8–37) (D) infusion (all p’s > 0.05).
Discussion
The primary outcome reported here is an increase in CAP amplitude accompanied by small improvements in CAP threshold during CGRP infusion into the cochlea. These results are consistent with the decreases in ABR Wave I amplitude reported by Maison et al. (2003b), who observed a decrease in sound-driven auditory nerve activity in the absence of endogenous αCGRP. Although no changes in thresholds were detected in the 16-week old mutant mice described by Maison et al. (2003b), changes in thresholds were detected in the 3-month old mutant mice described by Allen and Luebke (2017), with CGRP-null mice having poorer hearing in both quiet and in noise. The current results in guinea pigs are consistent with Allen and Luebke (2017) in that thresholds were better when exogenous CGRP was infused; however, threshold deficits did not emerge when CGRP (8–37) was infused. In addition to sound-evoked neural response, the current study evaluated spontaneous neural activity using round window noise. The increased round window noise described here during CGRP infusion suggests that CGRP induced an increase in spontaneous AN firing. The observed effects are consistent not only with data from CGRP-null mice, but also with the increase in spontaneous activity observed after bath application of CGRP to Xenopus lateral line organ (Adams et al., 1987; Sewell and Starr, 1991; Bailey and Swell, 2000a; Bailey and Swell, 2000b). Having demonstrated excitatory effects of CGRP in the current study, additional studies are warranted to determine a dose-response curve. A preliminary test with a single subject infused with a lower dose (125 μM) showed no effects of CGRP on CAP threshold or amplitude at any of the test times over the 14-day infusion, but the lowest effective dose is not known, and the effects of increasing either the concentration of CGRP or the duration of CGRP infusion are not known.
In their description of the effects of CGRP-null mutations, Maison et al. (2003b) reported they did not observe any changes in DPOAE threshold or amplitude, or the strength of the MOC reflex. Allen and Luebke (2017) similarly reported DPOAE responses to be intact in CGRP-null mice. The lack of effect of CGRP-null mutations on measures of OHC function is perhaps surprising given robust CGRP-positive immunolabeling of the MOC efferents that innervate the OHCs (Cabanillas and Luebke, 2002; Kong et al., 2002; Maison et al., 2003a). However, the data collected here using both CGRP and the CGRP (8–37) fragment, a receptor antagonist, were consistent with the reports by Maison et al. (2003b) and Allen and Luebke (2017). Just as CGRP-null mice did not have any changes with respect to DPOAE metrics, no changes were observed here in guinea pigs that underwent chemical manipulation of the cochlea fluids with CGRP ligands. Taken together, the data show CGRP effects on the AN but not the OHCs, although we cannot exclude the possibility for as-yet unknown effects of CGRP on the OHCs. Future studies should continue to include detailed input-output functions for DPOAE responses across a wide range of sound levels to assure that OHC function is well characterized.
The earlier data from Maison et al. (2003b) and Allen and Luebke (2017) reflect the loss of CGRP-mediated modulation of AN activity that is present in the wild-type controls. In mutant CGRP-null mice there is a loss of excitation; here, with CGRP infusion, there is evidence of increased spontaneous and sound driven AN activity. While we interpret these data to reflect direct effects of CGRP on the AN, the possibility that CGRP-mediated changes in blood flow could also contribute to the observed results must be considered. The spiral modiolar artery, a primary supplier of blood to the cochlea, contains CGRP-positive terminals (Ylikoski et al., 1989; Carlisle et al., 1990; Lyon and Payman, 2000; Qiu et al., 2001; Herzog et al., 2002) as well as CLR mRNA (Herzog et al., 2002). Micro-infusion of CGRP directly into the arterial network of the cochlea increases cochlear blood flow (Quirk et al., 1994), as does intra-venous CGRP infusion (Hillerdal and Andersson, 1991). However, when drugs that influence cochlear blood flow have been delivered directly into the cochlear fluids via round window application in the rat, instead of being delivered directly into the bloodstream, the otherwise vasoactive drugs failed to reduce cochlear blood flow (Laurikainen et al., 1993). Because the CGRP ligands were administered into the cochlear fluids in this study, the data from Laurikainen et al. (1993) suggest the current infusions to be unlikely to influence cochlear blood flow. However, even if the CGRP ligands did move into the blood supply, intra-arterial CGRP has no effect on CAP (Quirk et al., 1994), and other blood-flow promoting drugs have also failed to affect CAP amplitude after intra-venous drug delivery (Lamm and Arnold, 2000).
Changes in CAP have accompanied cochlear blood flow alterations only if the drug treatment resulted in arterial blockade, ischemia, and excitotoxic AN swelling, in which case CAP decreases (e.g., Asai et al., 1996; Kong et al., 1996; Scheibe et al., 1997; Tabuchi et al., 1998). Thus, even if intra-cochlear CGRP did influence cochlear blood flow, the observed increases in CAP amplitude are not readily explained by changes in cochlear blood flow. It is possible that inflammation affecting the cochlear vascular system could occur after cochlear surgery, and inflammation has the potential to influence cochlear blood flow (Sharaf et al., 2016). However, normal post-operative hearing thresholds suggest inflammation not to be a major concern as inflammation is increasingly understood to be associated with hearing loss (Frye et al., 2019; Zhang et al., 2021). Here, thresholds were not only normal, they showed statistically significant decreases (improvements) during CGRP infusion, which would not be expected in the presence of surgical trauma and significant inflammation. Taken together, the overall pattern of results is more consistent with direct effects on the AN than with indirect effects on the AN mediated via changes in cochlear blood flow.
Previous studies have showed that cutting the olivocochlear bundle (OCB), which eliminates both MOC and LOC innervation, depressed spontaneous (Liberman, 1990; Walsh et al., 1998; Zheng et al., 1999) and sound-driven (Zheng et al., 1999) AN activity. When the LSO is lesioned in the guinea pig brainstem using melittin (Le Prell et al., 2003b) or the LOC system is lesioned in the guinea pig cochlea using MPTP (Le Prell et al., 2005), sound-driven AN activity is decreased. When the guinea pig LOC system is lesioned using MPTP, spontaneous AN firing is also decreased (Le Prell et al., 2014a). We have long speculated that the decreases in spontaneous and sound driven AN firing after OCB transection, LSO lesion, or LOC lesion could be the result of compromised CGRP release when the LOC system is disrupted (Le Prell et al., 2003b; Le Prell et al., 2005; Le Prell, 2007; Le Prell et al., 2014a). The observation that CGRP infusion directly increased ensemble spontaneous and sound-driven AN activity in this study adds new support for the hypothesis that endogenous CGRP release by the LOC efferent neurons has an excitatory effect on the AN.
The chemical transmitters that might mediate the net-excitatory effect in the intact (undamaged) system are a question of significant interest (for review and discussion see Le Prell et al., 2003a; Le Prell et al., 2003b; Le Prell et al., 2005; Reijntjes and Pyott, 2016). In brief, most data suggest that DA is largely inhibitory with respect to AN activity (d’Aldin et al., 1995a; d’Aldin et al., 1995b; Oestreicher et al., 1997; Ruel et al., 2001), although new data using selective agonists and antagonists acting at D2 receptors suggest tonic release of endogenous DA has an excitatory action at the D2 receptor (Garrett et al., 2011). Similar, but more limited, documentation of inhibitory effects on AN activity have been reported for enk (Burki et al., 1993) and GABA (Felix and Ehrenberger, 1992; Arnold et al., 1998). Comprehensive discussion of these diverse pharmacological studies is available in Reijntjes and Pyott (2016). Recent data suggest differential release of ACh and DA in different sound conditions and a “fine-tuning” of the activity of the auditory nerve via the release of these two LOC neurotransmitters (Wu et al., 2020).
Overall understanding of the LOC system will require improved understanding of other LOC transmitters that are co-localized with CGRP in the LOC terminals, including, for example, ACh and dyn. The effects of ACh on the OHCs have been carefully characterized, although the potential effects of ACh on the AN remain less well understood (for detailed reviews, see Puel, 1995; Le Prell et al., 2001; Reijntjes and Pyott, 2016; Fuchs and Lauer, 2019). Some studies have used cochlear perfusion, and others have used microiontophoresis to restrict ACh delivery to the vicinity of the AN dendrites; chemical antagonists and genetic manipulations have also been employed. Release of ACh from the MOC efferents onto the OHCs inhibits OHC electromotile action. This body of work suggests that the α9/α10 nicotinic receptors may provide a novel pharmacotherapeutic target (Elgoyhen et al., 2009). Early data collected with ACh delivered via intracochlear perfusion indicated that 250 μM ACh had no effect on the cochlear microphonic (CM) or CAP; however, 250 μM ACh delivered in combination with 10 μM eserine (which blocks the metabolism of ACh) augmented CM amplitude and depressed CAP amplitude, suggesting neural effects may be secondary to changes in OHC response to sound (Bobbin and Konishi, 1971). In contrast to the decreases in CAP with cochlear perfusion, when 0.5 M ACh was applied in the vicinity of the AN dendrites using microiontophoresis, increased subsynaptic spiking and enhanced glutamate-induced AN activity were observed, suggesting direct excitatory effects of ACh on the AN (Felix and Ehrenberger, 1992). Other recent data also suggest direct excitatory effects of ACh on the AN; specifically, selective deletion of several muscarinic receptor (M1 and/or M4) subtypes decreased neural response amplitude without effecting DPOAEs (Maison et al., 2010). Taken together, this data suggests the potential that ACh may also serve as an excitatory transmitter when released from the LOC efferent neurons.
The effects of dynorphin (dyn) have been less clear, with data from Sahley and colleagues, who assessed supra-physiological doses, suggesting excitatory (Sahley et al., 1991; Sahley and Nodar, 1994), or biphasic (Sahley et al., 2008) effects. In contrast, data collected using biologically plausible drug concentrations instead suggested that dyn inhibits sound-evoked AN activity (Le Prell et al., 2014b). More recently, Sahley et al. (2019) suggested that release of dyn by the LOC neurons may in part drive well-observed cascades including glutamate-induced swelling, inflammation, excitotoxicity, and hearing loss as dyn has been linked to such responses (immune, inflammatory, and excitotoxic) in other neural systems. Taken together, the transmitter data collected using DA, GABA, enk, and dyn have largely failed to explain the net excitatory effects of LOC innervation on the AN that are suggested by the net-inhibitory effects of LOC lesions, as well as observation that electrical current applied at some locations within the LSO enhances CAP amplitude (Groff and Liberman, 2003). The data from Maison et al. (2003b) and Allen and Luebke (2017) using CGRP-null mice suggest CGRP as a candidate transmitter substance, and the current data using chemical manipulations confirm CGRP has the potential to upregulate AN activity, with ACh also remaining a potentially excitatory neurotransmitter substance within the LOC system.
The extent to which endogenous CGRP is released from the LOC efferents in either a tonic state or stimulus-driven (sound) condition is not currently known; thus, important questions remain. In an effort to identify endogenous release, we assessed the effects of a CGRP receptor antagonist, CGRP (8–37). The finding that CGRP (8–37) did not influence AN or OHC outcomes may suggest there is no appreciable tonic or sound-driven release of CGRP by the LOC or MOC efferents, at least under these test conditions. Caution is required with respect to this interpretation however, as the extent to which CGRP (8–37) is an effective antagonist in the guinea pig inner ear remains an open question. It would be worthwhile to include combinations of agents in future studies to directly assess whether the antagonist selected for use competitively blocks the effects attributed to agonist infusion, and we strongly encourage this approach in future investigations to reduce questions about the potential effectiveness of the antagonist selected for investigation. Another approach that might be used to more precisely localize the observed drug effects to the LOC system would be repeating the drug studies in guinea pigs with efferent lesions, although LOC lesions are often incomplete which would make data from such studies difficult to interpret. LSO lesions induced in the brainstem using melittin do not completely eliminate the LOC innervation in the cochlea (Le Prell et al., 2003b), and LOC lesions induced in the cochlea using MPTP are also incomplete (Le Prell et al., 2005).
We selected CGRP (8–37) as competitive blockade of CGRP1 receptors has been shown in guinea pig basilar artery using CGRP (8–37) (Jansen-Olesen et al., 2001). In addition, CGRP (8–37) has been an effective antagonist when delivered in equimolar concentrations with CGRP (Buffelli et al., 2001). From a practical perspective, CGRP (8–37) was the only antagonist readily available through common commercial sources. However, CGRP (8–37) is widely regarded to not be very potent as an antagonist (Doods et al., 2000), and there have been significant efforts to develop more potent antagonists. The novel CGRP receptor antagonist BIBN4096BS (olcegepant) has been an effective antagonist when applied to brainstem slices from the mouse (Zheng et al., 2021), resulted in decreased bone volume when administered systematically in the mouse (Köhli et al., 2021), and decreased CGRP-induced light aversion when olcegepant was co-administered in the mouse (Russo et al., 2009). In contrast, neither olcegepant nor CGRP (8–37) affected uptake of CGRP in rat dura mater preparations (Gupta et al., 2010). Olcegepant has not been well studied in guinea pigs and thus it is not clear if olcegepant would be more effective than CGRP (8–37) when small drug volumes are infused into guinea pig cochlea. However, olcegepant binding in rat, rabbit, dog, and guinea pig models has been reported to be is as much as 100 times less than that observed in primate models (rhesus macaque, marmoset) (Doods et al., 2000; Hershey et al., 2005).
Given better binding in non-human primates and in humans, multiple drugs that antagonize CGRP release or the CGRP receptor are either in clinical trials or approved for use for antimigraine purposes (Mohanty and Lippmann, 2020). Despite the availability of drugs that are potent in non-human primates and humans, there continues to be a need for antagonists that are effective in rodent models for use in basic (pre-clinical) investigation. In addition to binding issues, stability of the antagonists must be considered for chronic infusion paradigms such as this one. The stability of CGRP (8–37) in vivo has been questioned (Miranda et al., 2013). However, CGRP (8–37) was biologically effective when delivered via osmotic mini-pump for 7-days in vivo in rats (Reinshagen et al., 1998; Buffelli et al., 2001; Yin et al., 2004). Our infusions extended over a longer period, to 14 days. However, even if CGRP (8–37) were inactivated at later test times, we did not observe any functional changes within the first 6–8 days (see Figure 3), times at which the data from rats suggest the CGRP (8–37) fragment to be stable via osmotic mini-pump delivery.
In summary, this is the first direct demonstration that CGRP infusion enhances spontaneous and sound-driven AN activity. Based on these results, we conclude that CGRP has the potential to play an excitatory role in the AN response, confirming and extending data from knock-out mice (see Maison et al., 2003b). These key data set the stage for psychophysical data to be collected during periods of chronic cochlear drug infusion using osmotic mini-pumps (as in Le Prell et al., 2004) to confirm the possible role of CGRP in modulation of hearing in noise, following the decreased hearing-in-noise documented in CGRP-null mice by Allen and Luebke (2017). Studies in awake animals would have the added benefit of precluding the possibility that ketamine anesthesia may attenuate responses to CGRP. The effects of CGRP are commonly measured in ketamine-anesthetized animals (Radziszewski et al., 2003; Sakamoto et al., 2014; Sakamoto et al., 2017; Søndergaard et al., 2019). However, intra-venous ketamine attenuated CGRP-induced vasodilation of the dural artery in rats (Chan et al., 2010). Possible effects of ketamine on the excitatory effects of CGRP in the cochlea are speculative; however, if CGRP has less excitatory effect when measured under ketamine anesthesia, then the effect of CGRP on AN activity could be even more robust than was shown in this report. Taken together, the data provide direct confirmation of excitatory effects of CGRP on the AN, and support a potentially important role for CGRP in auditory function.
Data Availability Statement
The raw data supporting the conclusion of this article will be made available by the authors, without undue reservation.
Ethics Statement
The animal study was reviewed and approved by the University Committee on Use and Care of Animals at the University of Michigan.
Author Contributions
CL, DD, and SB designed the experiments; CL and DD collected data; LH performed statistical analyses; CL, DD, SB, and LH interpreted results; CL and LH wrote the manuscript; DD and SB reviewed and revised the manuscript.
Funding
Financial support was provided by the American Hearing Research Foundation (CL), the National Organization for Hearing Research (CL), and NIH-NIDCD grants R03-DC007342 (CL) and P30-DC05188 (DD).
Conflict of Interest
The authors declare that the research was conducted in the absence of any commercial or financial relationships that could be construed as a potential conflict of interest.
Publisher’s Note
All claims expressed in this article are solely those of the authors and do not necessarily represent those of their affiliated organizations, or those of the publisher, the editors and the reviewers. Any product that may be evaluated in this article, or claim that may be made by its manufacturer, is not guaranteed or endorsed by the publisher.
Acknowledgments
The authors thank Chris Ellinger, Kärin Halsey, Beth Hand, Lisa Kabara, Diane Prieskorn, and Sarah Weisbuch, for their invaluable technical assistance.
References
Adams, J. C., Mroz, E. A., and Sewell, W. F. (1987). A Possible Neurotransmitter Role for CGRP in a Hair-Cell Sensory Organ. Brain Res. 419, 347–351. doi:10.1016/0006-8993(87)90606-8
Allen, P. D., and Luebke, A. E. (2017). Reflex Modification Audiometry Reveals Dual Roles for Olivocochlear Neurotransmission. Front. Cel. Neurosci. 11, 361. doi:10.3389/fncel.2017.00361
Arnold, T., Oestreicher, E., Ehrenberger, K., and Felix, D. (1998). GABAA Receptor Modulates the Activity of Inner Hair Cell Afferents in Guinea Pig Cochlea. Hear. Res. 125, 147–153. doi:10.1016/s0378-5955(98)00144-0
Asai, Y., Umemura, K., and Nakashima, M. (1996). Reversibility of Compound Action Potential during the Acute Phase after Transitory Local Ischemia. Ann. Otol. Rhinol. Laryngol. 105, 472–475. doi:10.1177/000348949610500610
Bailey, G. P., and Sewell, W. F. (2000b). Calcitonin Gene-Related Peptide Suppresses Hair Cell Responses to Mechanical Stimulation in the Xenopus Lateral Line Organ. J. Neurosci. 20, 5163–5169. doi:10.1523/JNEUROSCI.20-13-05163.2000
Bailey, G. P., and Sewell, W. F. (2000a). Pharmacological Characterization of the CGRP Receptor in the Lateral Line Organ of Xenopus laevis. J. Assoc. Res. Otolaryngol. 1, 82–88. doi:10.1007/s101620010007
Barbanti, P., Aurilia, C., Cevoli, S., Egeo, G., Fofi, L., Messina, R., et al. (2021). Long‐term (48 Weeks) Effectiveness, Safety, and Tolerability of Erenumab in the Prevention of High‐frequency Episodic and Chronic Migraine in a Real World: Results of the EARLY 2 Study. Headache 61 (9), 1351–1363. doi:10.1111/head.14194
Bobbin, R. P., and Konishi, T. (1971). Acetylcholine Mimics Crossed Olivocochlear Bundle Stimulation. Nat. New Biol. 231, 222–223. doi:10.1038/newbio231222a0
Brown, J. N., Miller, J. M., Altschuler, R. A., and Nuttall, A. L. (1993). Osmotic Pump Implant for Chronic Infusion of Drugs into the Inner Ear. Hear. Res. 70, 167–172. doi:10.1016/0378-5955(93)90155-t
Buffelli, M., Pasino, E., and Cangiano, A. (2001). In Vivo Acetylcholine Receptor Expression Induced by Calcitonin Gene-Related Peptide in Rat Soleus Muscle. Neuroscience 104, 561–567. doi:10.1016/s0306-4522(01)00090-2
Bürki, C., Felix, D., and Ehrenberger, K. (1993). Enkephalin Suppresses Afferent Cochlear Neurotransmission. ORL 55, 3–6. doi:10.1159/000276344
Cabanillas, L. A., and Luebke, A. E. (2002). CGRP- and Cholinergic-Containing Fibers Project to Guinea Pig Outer Hair Cells. Hear. Res. 172, 14–17. doi:10.1016/s0378-5955(02)00305-2
Carlisle, L., Aberdeen, J., Forge, A., and Burnstock, G. (1990). Neural Basis for Regulation of Cochlear Blood Flow: Peptidergic and Adrenergic Innervation of the Spiral Modiolar Artery of the Guinea Pig. Hear. Res. 43, 107–113. doi:10.1016/0378-5955(90)90219-f
Chan, K., Gupta, S., de Vries, R., Danser, A., Villalón, C., Muñoz-Islas, E., et al. (2010). Effects of Ionotropic Glutamate Receptor Antagonists on Rat Dural Artery Diameter in an Intravital Microscopy Model. Br. J. Pharmacol. 160, 1316–1325. doi:10.1111/j.1476-5381.2010.00733.x
d'Aldin, C., Eybalin, M., Puel, J.-L., Charachon, G., Ladrech, S., Renard, N., et al. (1995a). Synaptic Connections and Putative Functions of the Dopaminergic Innervation of the Guinea Pig Cochlea. Eur. Arch. Otorhinolaryngol. 252, 270–274. doi:10.1007/BF00185388
d'Aldin, C., Puel, J.-L., Leducq, R., Crambes, O., Eybalin, M., and Pujol, R. (1995b). Effects of a Dopaminergic Agonist in the Guinea Pig Cochlea. Hear. Res. 90, 202–211. doi:10.1016/0378-5955(95)00167-5
Darrow, K. N., Simons, E. J., Dodds, L., and Liberman, M. C. (2006). Dopaminergic Innervation of the Mouse Inner Ear: Evidence for a Separate Cytochemical Group of Cochlear Efferent Fibers. J. Comp. Neurol. 498, 403–414. doi:10.1002/cne.21050
de Vries Lentsch, S., Rubio-Beltrán, E., and MaassenVanDenBrink, A. (2021). Changing Levels of Sex Hormones and Calcitonin Gene-Related Peptide (CGRP) during a Woman's Life: Implications for the Efficacy and Safety of Novel Antimigraine Medications. Maturitas 145, 73–77. doi:10.1016/j.maturitas.2020.12.012
Dickerson, I. M., Bussey-Gaborski, R., Holt, J. C., Jordan, P. M., and Luebke, A. E. (2016). Maturation of Suprathreshold Auditory Nerve Activity Involves Cochlear CGRP-Receptor Complex Formation. Physiol. Rep. 4, e12869. doi:10.14814/phy2.12869
Dolan, D. F., Nuttall, A. L., and Avinash, G. (1990). Asynchronous Neural Activity Recorded from the Round Window. J. Acoust. Soc. Am. 87, 2621–2627. doi:10.1121/1.399054
Doods, H., Hallermayer, G., Wu, D., Entzeroth, M., Rudolf, K., Engel, W., et al. (2000). Pharmacological Profile of BIBN4096BS, the First Selective Small Molecule CGRP Antagonist. Br. J. Pharmacol. 129, 420–423. doi:10.1038/sj.bjp.0703110
Elgoyhen, A. B., Katz, E., and Fuchs, P. A. (2009). The Nicotinic Receptor of Cochlear Hair Cells: A Possible Pharmacotherapeutic Target? Biochem. Pharmacol. 78, 712–719. doi:10.1016/j.bcp.2009.05.023
Felix, D., and Ehrenberger, K. (1992). The Efferent Modulation of Mammalian Inner Hair Cell Afferents. Hear. Res. 64, 1–5. doi:10.1016/0378-5955(92)90163-h
Frye, M. D., Ryan, A. F., and Kurabi, A. (2019). Inflammation Associated with Noise-Induced Hearing Loss. J. Acoust. Soc. Am. 146, 4020–4032. doi:10.1121/1.5132545
Fuchs, P. A., and Lauer, A. M. (2019). Efferent Inhibition of the Cochlea. Cold Spring Harb. Perspect. Med. 9, a033530. doi:10.1101/cshperspect.a033530
Garrett, A. R., Robertson, D., Sellick, P. M., and Mulders, W. H. A. M. (2011). The Actions of Dopamine Receptors in the Guinea Pig Cochlea. Audiol. Neurotol. 16, 145–157. doi:10.1159/000316674
Goycoolea, M. V., and Lundman, L. (1997). Round Window Membrane. Structure Function and Permeability: A Review. Microsc. Res. Tech. 36, 201–211. doi:10.1002/(sici)1097-0029(19970201)36:3<201:aid-jemt8>3.0.co;2-r
Groff, J. A., and Liberman, M. C. (2003). Modulation of Cochlear Afferent Response by the Lateral Olivocochlear System: Activation via Electrical Stimulation of the Inferior Colliculus. J. Neurophysiol. 90, 3178–3200. doi:10.1152/jn.00537.2003
Gupta, S., Amrutkar, D. V., Mataji, A., Salmasi, H., Hay-Schmidt, A., Sheykhzade, M., et al. (2010). Evidence for CGRP Re-uptake in Rat Dura Mater Encephali. Br. J. Pharmacol. 161, 1885–1898. doi:10.1111/j.1476-5381.2010.01012.x
Halsey, K., Skjönsberg, A., Ulfendahl, M., and Dolan, D. F. (2005). Efferent-mediated Adaptation of the DPOAE as a Predictor of Aminoglycoside Toxicity. Hear. Res. 201, 99–108. doi:10.1016/j.heares.2004.09.010
Herbison, A. E., and Spratt, D. P. (1995). Sexually Dimorphic Expression of Calcitonin Gene-Related Peptide (CGRP) mRNA in Rat Medial Preoptic Nucleus. Mol. Brain Res. 34, 143–148. doi:10.1016/0169-328x(95)00144-h
Hershey, J. C., Corcoran, H. A., Baskin, E. P., Salvatore, C. A., Mosser, S., Williams, T. M., et al. (2005). Investigation of the Species Selectivity of a Nonpeptide CGRP Receptor Antagonist Using a Novel Pharmacodynamic Assay. Regul. Peptides 127, 71–77. doi:10.1016/j.regpep.2004.10.010
Herzog, M., Scherer, E. Q., Albrecht, B., Rorabaugh, B., Scofield, M. A., and Wangemann, P. (2002). CGRP Receptors in the Gerbil Spiral Modiolar Artery Mediate a Sustained Vasodilation via a Transient cAMP-Mediated Ca2+-Decrease. J. Membr. Biol. 189, 225–236. doi:10.1007/s00232-002-1017-5
Hillerdal, M., and Andersson, S. E. (1991). The Effects of Calcitonin Gene-Related Peptide (CGRP) on Cochlear and Mucosal Blood Flow in the Albino Rabbit. Hear. Res. 52, 321–328. doi:10.1016/0378-5955(91)90022-2
Jansen-Olesen, I., Kaarill, L., and Edvinsson, L. (2001). Characterization of CGRP1 Receptors in the Guinea Pig Basilar Artery. Eur. J. Pharmacol. 414, 249–258. doi:10.1016/s0014-2999(01)00760-9
Ji, Y., Rizk, A., Voulalas, P., Aljohani, H., Akerman, S., Dussor, G., et al. (2019). Sex Differences in the Expression of Calcitonin Gene-Related Peptide Receptor Components in the Spinal Trigeminal Nucleus. Neurobiol. Pain 6, 100031. doi:10.1016/j.ynpai.2019.100031
Kiang, N. Y. S., Moxon, E. C., and Kahn, A. R. (1976). “The Relationship of Gross Potentials Recorded from the Cochlea to Single Unit Activity in the Auditory Nerve,” in Electrocochleography. Editors R. J. Ruben, C. Elberling, and G. Solomon (Baltimore: University Park), 95–115.
Kitajiri, M., Yamashita, T., Tohyama, Y., Kumazawa, T., Takeda, N., Kawasaki, Y., et al. (1985). Localization of Calcitonin Gene-Related Peptide in the Organ of Corti of the Rat: An Immunohistochemical Study. Brain Res. 358, 394–397. doi:10.1016/0006-8993(85)90992-8
Köhli, P., Appelt, J., Otto, E., Jahn, D., Baranowsky, A., Bahn, A., et al. (2021). Effects of CGRP Receptor Antagonism on Glucose and Bone Metabolism in Mice with Diet-Induced Obesity. Bone 143, 115646. doi:10.1016/j.bone.2020.115646
Kong, W.-J., Ren, T., and Nuttall, A. L. (1996). Electrophysiological and Morphological Evaluation of the Acute Ototoxicity of Sodium Nitroprusside. Hear. Res. 99, 22–30. doi:10.1016/s0378-5955(96)00076-7
Kong, W.-J., Scholtz, A. W., Kammen-Jolly, K., Glückert, R., Hussl, B., von Cauvenberg, P. B., et al. (2002). Ultrastructural Evaluation of Calcitonin Gene-Related Peptide Immunoreactivity in the Human Cochlea and Vestibular Endorgans. Eur. J. Neurosci. 15, 487–497. doi:10.1046/j.0953-816x.2001.01880.x
Kujawa, S. G., and Liberman, M. C. (2001). Effects of Olivocochlear Feedback on Distortion Product Otoacoustic Emissions in Guinea Pig. J. Assoc. Res. Otolaryngol. 2, 268–278. doi:10.1007/s101620010047
Kuriyama, H., Shiosaka, S., Sekitani, M., Tohyama, Y., Kitajiri, M., Yamashita, T., et al. (1990). Electron Microscopic Observation of Calcitonin Gene-Related Peptide-like Immunoreactivity in the Organ of Corti of the Rat. Brain Res. 517, 76–80. doi:10.1016/0006-8993(90)91010-e
Lamm, K., and Arnold, W. (2000). The Effect of Blood Flow Promoting Drugs on Cochlear Blood Flow, Perilymphatic pO2 and Auditory Function in the Normal and Noise-Damaged Hypoxic and Ischemic Guinea Pig Inner Ear. Hear. Res. 141, 199–219. doi:10.1016/s0378-5955(00)00005-8
Laurikainen, E. A., Miller, J. M., Quirk, W. S., Kallinen, J., Ren, T., Nuttall, A. L., et al. (1993). Betahistine-induced Vascular Effects in the Rat Cochlea. Am. J. Otol. 14, 24–30.
Le Prell, C. G., Dolan, D. F., Schacht, J., Miller, J. M., Lomax, M. I., and Altschuler, R. A. (2003a). Pathways for Protection from Noise Induced Hearing Loss. Noise Health 5, 1–17.
Le Prell, C. G., Bledsoe, S. C., Bobbin, R. P., and Puel, J. L. (2001). “Neurotransmission in the Inner Ear: Functional and Molecular Analyses,” in Physiology of the Ear. Editors A. F. Jahn, and J. Santos-Sacchi. Second ed (New York: Singular Publishing), 575–611.
Le Prell, C. G., Dolan, D. F., Hughes, L. F., Altschuler, R. A., Shore, S. E., and Bledsoe, S. C. (2014a). Disruption of Lateral Olivocochlear Neurons with a Dopaminergic Neurotoxin Depresses Spontaneous Auditory Nerve Activity. Neurosci. Lett. 582, 54–58. doi:10.1016/j.neulet.2014.08.040
Le Prell, C. G., Halsey, K., Hughes, L. F., Dolan, D. F., and Bledsoe, S. C. (2005). Disruption of Lateral Olivocochlear Neurons via a Dopaminergic Neurotoxin Depresses Sound-Evoked Auditory Nerve Activity. J. Assoc. Res. Otolaryngol. 6, 48–62. doi:10.1007/s10162-004-5009-2
Le Prell, C. G., Hughes, L. F., and Bledsoe, S. C. (2014b). Dynorphin Release by the Lateral Olivocochlear Efferents May Inhibit Auditory Nerve Activity: A Cochlear Drug Delivery Study. Neurosci. Lett. 571, 17–22. doi:10.1016/j.neulet.2014.04.024
Le Prell, C. G., Kawamoto, K., Raphael, Y., and Dolan, D. F. (2006). Electromotile Hearing: Acoustic Tones Mask Psychophysical Response to High-Frequency Electrical Stimulation of Intact Guinea Pig Cochlea. J. Acoust. Soc. Am. 120, 3889–3900. doi:10.1121/1.2359238
Le Prell, C. G. (2007). Role for the Lateral Olivocochlear Neurons in Auditory Function. Focus on "Selective Removal of Lateral Olivocochlear Efferents Increases Vulnerability to Acute Acoustic Injury". J. Neurophysiol. 97, 963–965. doi:10.1152/jn.01223.2006
Le Prell, C. G., Shore, S. E., Hughes, L. F., and Bledsoe, S. C. (2003b). Disruption of Lateral Efferent Pathways: Functional Changes in Auditory Evoked Responses. J. Assoc. Res. Otolaryngol. 4, 276–290. doi:10.1007/s10162-002-3018-6
Le Prell, C. G., Yagi, M., Kawamoto, K., Beyer, L. A., Atkin, G., Raphael, Y., et al. (2004). Chronic Excitotoxicity in the Guinea Pig Cochlea Induces Temporary Functional Deficits without Disrupting Otoacoustic Emissions. J. Acoust. Soc. Am. 116, 1044–1056. doi:10.1121/1.1772395
Liberman, M. C. (1978). Auditory‐nerve Response from Cats Raised in a Low‐noise Chamber. J. Acoust. Soc. Am. 63, 442–455. doi:10.1121/1.381736
Liberman, M. C. (1990). Effects of Chronic Cochlear De-efferentation on Auditory-Nerve Response. Hear. Res. 49, 209–223. doi:10.1016/0378-5955(90)90105-x
Liberman, M. C., Puria, S., and Guinan, J. J. (1996). The Ipsilaterally Evoked Olivocochlear Reflex Causes Rapid Adaptation of the 2 F1−f2 Distortion Product Otoacoustic Emission. J. Acoust. Soc. Am. 99, 3572–3584. doi:10.1121/1.414956
Lu, S. M., Schweitzer, L., Cant, N. B., and Dawbarn, D. (1987). Immunoreactivity to Calcitonin Gene-Related Peptide in the Superior Olivary Complex and Cochlea of Cat and Rat. Hear. Res. 31, 137–146. doi:10.1016/0378-5955(87)90119-5
Lyon, M. J., and Payman, R. N. (2000). Comparison of the Vascular Innervation of the Rat Cochlea and Vestibular System. Hear. Res. 141, 189–198. doi:10.1016/s0378-5955(00)00004-6
Maison, S. F., Emeson, R. B., Adams, J. C., Luebke, A. E., and Liberman, M. C. (2003b). Loss of αCGRP Reduces Sound-Evoked Activity in the Cochlear Nerve. J. Neurophysiol. 90, 2941–2949. doi:10.1152/jn.00596.2003
Maison, S. F., and Liberman, M. C. (2000). Predicting Vulnerability to Acoustic Injury with a Noninvasive Assay of Olivocochlear Reflex Strength. J. Neurosci. 20, 4701–4707. doi:10.1523/JNEUROSCI.20-12-04701.2000
Maison, S. F., Liu, X. P., Vetter, D. E., Eatock, R. A., Nathanson, N. M., Wess, J., et al. (2010). Muscarinic Signaling in the Cochlea: Presynaptic and Postsynaptic Effects on Efferent Feedback and Afferent Excitability. J. Neurosci. 30, 6751–6762. doi:10.1523/JNEUROSCI.5080-09.2010
Maison, S. p. F., Adams, J. C., and Liberman, M. C. (2003a). Olivocochlear Innervation in the Mouse: Immunocytochemical Maps, Crossed versus Uncrossed Contributions, and Transmitter Colocalization. J. Comp. Neurol. 455, 406–416. doi:10.1002/cne.10490
May, B. J., Prosen, C. A., Weiss, D., and Vetter, D. (2002). Behavioral Investigation of Some Possible Effects of the Central Olivocochlear Pathways in Transgenic Mice. Hear. Res. 171, 142–157. doi:10.1016/s0378-5955(02)00495-1
Merchan-Perez, A., Gil-Loyzaga, P., and Eybalin, M. (1990). Immunocytochemical Detection of Calcitonin Gene-Related Peptide in the Postnatal Developing Rat Cochlea. Int. J. Develop. Neurosci. 8, 603–612. doi:10.1016/0736-5748(90)90052-4
Mikulec, A. A., Hartsock, J. J., and Salt, A. N. (2008). Permeability of the Round Window Membrane is Influenced by the Composition of Applied Drug Solutions and by Common Surgical Procedures. Otol. Neurotol. 29, 1020–1026. doi:10.1097/MAO.0b013e31818658ea
Miranda, L. P., Shi, L., Holder, J. R., Wright, M., Gegg, C. V., Johnson, E., et al. (2013). Peptide Antagonists of the Calcitonin Gene-Related Peptide (CGRP) Receptor with Improved Pharmacokinetics and Pharmacodynamics. Biopolymers 100, 422–430. doi:10.1002/bip.22270
Mohanty, D., and Lippmann, S. (2020). CGRP Inhibitors for Migraine. Innov. Clin. Neurosci. 17, 39–40.
Moore, J. K., Simmons, D. D., and Guan, Y.-L. (1999). The Human Olivocochlear System: Organization and Development. Audiol. Neurotol. 4, 311–325. doi:10.1159/000013855
Müller, M., and Robertson, D. (1991). Relationship between Tone Burst Discharge Pattern and Spontaneous Firing Rate of Auditory Nerve Fibres in the Guinea Pig. Hear. Res. 57, 63–70. doi:10.1016/0378-5955(91)90075-k
National Research Council (1996). Guide for the Care and Use of Laboratory Animals. Washington D.C.: National Academy Press.
Oestreicher, E., Arnold, W., Ehrenberger, K., and Felix, D. (1997). Dopamine Regulates the Glutamatergic Inner Hair Cell Activity in Guinea Pigs. Hear. Res. 107, 46–52. doi:10.1016/s0378-5955(97)00023-3
Ohno, K., Takeda, N., Tanaka-Tsuji, M., and Matsunaga, T. (1993). Calcitonin Gene-Related Peptide in the Efferent System of the Inner Ear: A Review. Acta Otolaryngol. 113, 16–20. doi:10.3109/00016489309126206
Ohyama, K., Salt, A. N., and Thalmann, R. (1988). Volume Flow Rate of Perilymph in the Guinea-pig Cochlea. Hear. Res. 35, 119–129. doi:10.1016/0378-5955(88)90111-6
Puel, J.-L. (1995). Chemical Synaptic Transmission in the Cochlea. Prog. Neurobiol. 47, 449–476. doi:10.1016/0301-0082(95)00028-3
Qiu, J.-H., Steyger, P. S., Trune, D. R., and Nuttall, A. L. (2001). Co-existence of Tyrosine Hydroxylase and Calcitonin Gene-Related Peptide in Cochlear Spiral Modiolar Artery of Guinea Pigs. Hear. Res. 155, 152–160. doi:10.1016/s0378-5955(01)00231-3
Quirk, W. S., Seidman, M. D., Laurikainen, E. A., Nuttall, A. L., and Miller, J. M. (1994). Influence of Calcitonin-Gene Related Peptide on Cochlear Blood Flow and Electrophysiology. Am. J. Otol. 15, 56–60.
Radziszewski, P., Soller, W., and Mattiasson, A. (2003). Calcitonin Gene-Related Peptide and Substance P Induce Pronounced Motor Effects in the Female Rat Urethra In Vivo. Scand. J. Urol. Nephrol. 37, 275–280. doi:10.1080/00365590310004761
Reijntjes, D. O. J., and Pyott, S. J. (2016). The Afferent Signaling Complex: Regulation of Type I Spiral Ganglion Neuron Responses in the Auditory Periphery. Hear. Res. 336, 1–16. doi:10.1016/j.heares.2016.03.011
Reinshagen, M., Flämig, G., Ernst, S., Geerling, I., Wong, H., Walsh, J. H., et al. (1998). Calcitonin Gene-Related Peptide Mediates the Protective Effect of Sensory Nerves in a Model of Colonic Injury. J. Pharmacol. Exp. Ther. 286, 657–661.
Reuss, S., Disque-Kaiser, U., De Liz, S., Ruffer, M., and Riemann, R. (1999). Immunfluorescence Study of Neuropeptides in Identified Neurons of the Rat Auditory Superior Olivary Complex. Cel Tissue Res. 297, 13–21. doi:10.1007/s004410051329
Rhode, W. S., and Smith, P. H. (1985). Characteristics of Tone-Pip Response Patterns in Relationship to Spontaneous Rate in Cat Auditory Nerve Fibers. Hear. Res. 18, 159–168. doi:10.1016/0378-5955(85)90008-5
Ruel, J., Nouvian, R., D'Aldin, C. G., Pujol, R., Eybalin, M., and Puel, J.-L. (2001). Dopamine Inhibition of Auditory Nerve Activity in the Adult Mammalian Cochlea. Eur. J. Neurosci. 14, 977–986. doi:10.1046/j.0953-816x.2001.01721.x
Russo, A. F., Kuburas, A., Kaiser, E. A., Raddant, A. C., and Recober, A. (2009). A Potential Preclinical Migraine Model: CGRP-Sensitized Mice. Mol. Cel. Pharmacol. 1, 264–270.
Sachs, M. B., and Abbas, P. J. (1974). Rate versus Level Functions for Auditory‐nerve Fibers in Cats: Tone‐burst Stimuli. J. Acoust. Soc. Am. 56, 1835–1847. doi:10.1121/1.1903521
Safieddine, S., and Eybalin, M. (1992). Triple Immunofluorescence Evidence for the Coexistence of Acetylcholine, Enkephalins and Calcitonin Gene-Related Peptide within Efferent (Olivocochlear) Neurons of Rats and Guinea-pigs. Eur. J. Neurosci. 4, 981–992. doi:10.1111/j.1460-9568.1992.tb00124.x
Safieddine, S., Prior, A. M. S., and Eybalin, M. (1997). Choline Acetyltransferase, Glutamate Decarboxylase, Tyrosine Hydroxylase, Calcitonin Gene-Related Peptide and Opioid Peptides Coexist in Lateral Efferent Neurons of Rat and Guinea-pig. Eur. J. Neurosci. 9, 356–367. doi:10.1111/j.1460-9568.1997.tb01405.x
Sahley, T. L., Anderson, D. J., and Chernicky, C. L. (2008). Bi-phasic Intensity-dependent Opioid-Mediated Neural Amplitude Changes in the Chinchilla Cochlea: Partial Blockade by an N-Methyl-D-Aspartate (NMDA)-receptor Antagonist. Eur. J. Pharmacol. 580, 100–115. doi:10.1016/j.ejphar.2007.10.038
Sahley, T. L., Anderson, D. J., Hammonds, M. D., Chandu, K., and Musiek, F. E. (2019). Evidence for a Dynorphin-Mediated Inner Ear Immune/inflammatory Response and Glutamate-Induced Neural Excitotoxicity: An Updated Analysis. J. Neurophysiol. 122, 1421–1460. doi:10.1152/jn.00595.2018
Sahley, T. L., Kalish, R. B., Musiek, F. E., and Hoffman, D. W. (1991). Effects of Opioid Be Drugs on Auditory Evoked Potentials Suggest a Role of Lateral Olivocochlear Dynorphins in Auditory Function. Hear. Res. 55, 133–142. doi:10.1016/0378-5955(91)90099-u
Sahley, T. L., and Nodar, R. H. (1994). Improvement in Auditory Function Following Pentazocine Suggests a Role for Dynorphins in Auditory Sensitivity. Ear Hear. 15, 422–431. doi:10.1097/00003446-199412000-00003
Sakamoto, K., Kuroki, T., Okuno, Y., Sekiya, H., Watanabe, A., Sagawa, T., et al. (2014). Activation of the TRPV1 Channel Attenuates N-Methyl-D-Aspartic Acid-Induced Neuronal Injury in the Rat Retina. Eur. J. Pharmacol. 733, 13–22. doi:10.1016/j.ejphar.2014.03.035
Sakamoto, K., Kuroki, T., Sagawa, T., Ito, H., Mori, A., Nakahara, T., et al. (2017). Opioid Receptor Activation is Involved in Neuroprotection Induced by TRPV1 Channel Activation against Excitotoxicity in the Rat Retina. Eur. J. Pharmacol. 812, 57–63. doi:10.1016/j.ejphar.2017.07.002
Scheibe, F., Haupt, H., and Baumgärtl, H. (1997). Effects of Experimental Cochlear Thrombosis on Oxygenation and Auditory Function of the Inner Ear. Eur. Arch. Otorhinolaryngol. 254, 91–94. doi:10.1007/BF01526187
Schrott-Fischer, A., Kammen-Jolly, K., Scholtz, A., Rask-Andersen, H., Glueckert, R., and Eybalin, M. (2007). Efferent Neurotransmitters in the Human Cochlea and Vestibule. Acta Otolaryngol. 127, 13–19. doi:10.1080/00016480600652123
Sewell, W. F., and Starr, P. A. (1991). Effects of Calcitonin Gene-Related Peptide and Efferent Nerve Stimulation on Afferent Transmission in the Lateral Line Organ. J. Neurophysiol. 65, 1158–1169. doi:10.1152/jn.1991.65.5.1158
Sharaf, K., Ihler, F., Bertlich, M., Reichel, C. A., Berghaus, A., and Canis, M. (2016). Tumor Necrosis Factor-Induced Decrease of Cochlear Blood Flow can be Reversed by Etanercept or JTE-013. Otol. Neurotol. 37, e203–e208. doi:10.1097/mao.0000000000001095
Simmons, D. D., Moulding, H. D., and Zee, D. (1996). Olivocochlear Innervation of Inner and Outer Hair Cells during Postnatal Maturation: An Immunocytochemical Study. Develop. Brain Res. 95, 213–226. doi:10.1016/0165-3806(96)00084-3
Simmons, D. D., Raji-Kubba, J., Popper, P., and Micevych, P. E. (1997). Developmentally Regulated Expression of Calcitonin Gene-Related Peptide in the Superior Olive. J. Comp. Neurol. 377, 207–216. doi:10.1002/(sici)1096-9861(19970113)377:2<207:aid-cne4>3.0.co;2-7
Simmons, D. D., and Raji-Kubba, J. (1993). Postnatal Calcitonin Gene-Related Peptide in the Superior Olivary Complex. J. Chem. Neuroanat. 6, 407–418. doi:10.1016/0891-0618(93)90015-v
Sliwinska-Kowalska, M., Parakkal, M., Schneider, M. E., and Fex, J. (1989). CGRP-like Immunoreactivity in the Guinea Pig Organ of Corti: A Light and Electron Microscopy Study. Hear. Res. 42, 83–95. doi:10.1016/0378-5955(89)90119-6
Sly, D. J., Hampson, A. J., Minter, R. L., Heffer, L. F., Li, J., Millard, R. E., et al. (2012). Brain-derived Neurotrophic Factor Modulates Auditory Function in the Hearing Cochlea. J. Assoc. Res. Otolaryngol. 13, 1–16. doi:10.1007/s10162-011-0297-9
Søndergaard, A. M., Overgaard, C. B., Mazur, A., Postnov, D. D., Matchkov, V. V., and Aalkjaer, C. (2019). Rat Mesenteric Small Artery Neurogenic Dilatation is Predominantly Mediated by β 1 ‐adrenoceptors In Vivo. J. Physiol. 597, 1819–1831. doi:10.1113/jp277368
Tabuchi, K., Ito, Z., Wada, T., Hara, A., and Kusakari, J. (1998). The Effect of Mannitol upon Cochlear Dysfunction Induced by Transient Local Anoxia. Hear. Res. 126, 28–36. doi:10.1016/s0378-5955(98)00142-7
Takeda, N., Doi, K., Mori, N., Yamazaki, H., Tohyama, M., and Matsunaga, T. (1987). Localization and Fine Structure of Calcitonin Gene-Related Peptide (CGRP)-like Immunoreactive Nerve Fibres in the Organ of Corti of Guinea Pigs by Immunohistochemistry. Acta Otolaryngol. 103, 567–571.
Takeda, N., Kitajiri, M., Girgis, S., Hillyard, C. J., MacIntyre, I., Emson, P. C., et al. (1986). The Presence of a Calcitonin Gene-Related Peptide in the Olivocochlear Bundle in Rat. Exp. Brain Res. 61, 575–578. doi:10.1007/BF00237583
Tohyama, Y., Kiyama, H., Kitajiri, M., Yamashita, T., Kumazawa, T., and Tohyama, M. (1989). Ontogeny of Calcitonin Gene-Related Peptide in the Organ of Cortl of the Rat. Develop. Brain Res. 45, 309–312. doi:10.1016/0165-3806(89)90050-3
Tohyama, Y., Senba, E., Yamashita, T., Kitajiri, M., Kuriyama, H., Kumazawa, T., et al. (1990). Coexistence of Calcitonin Gene-Related Peptide and Enkephalin in Single Neurons of the Lateral Superior Olivary Nucleus of the Guinea Pig that Project to the Cochlea as Lateral Olivocochlear System. Brain Res. 515, 312–314. doi:10.1016/0006-8993(90)90613-g
Valdemarsson, S., Edvinsson, L., Hedner, P., and Ekman, R. (1990). Hormonal Influence on Calcitonin Gene-Related Peptide in Man: Effects of Sex Difference and Contraceptive Pills. Scand. J. Clin. Lab. Invest. 50, 385–388. doi:10.3109/00365519009091595
Vetter, D. E., Adams, J. C., and Mugnaini, E. (1991). Chemically Distinct Rat Olivocochlear Neurons. Synapse 7, 21–43. doi:10.1002/syn.890070104
Vyas, P., Wu, J. S., Jimenez, A., Glowatzki, E., and Fuchs, P. A. (2019). Characterization of Transgenic Mouse Lines for Labeling Type I and Type II Afferent Neurons in the Cochlea. Sci. Rep. 9, 5549. doi:10.1038/s41598-019-41770-5
Walsh, E. J., McGee, J., McFadden, S. L., and Liberman, M. C. (1998). Long-term Effects of Sectioning the Olivocochlear Bundle in Neonatal Cats. J. Neurosci. 18, 3859–3869. doi:10.1523/JNEUROSCI.18-10-03859.1998
Winter, I. M., Robertson, D., and Yates, G. K. (1990). Diversity of Characteristic Frequency Rate-Intensity Functions in Guinea Pig Auditory Nerve Fibres. Hear. Res. 45, 191–202. doi:10.1016/0378-5955(90)90120-e
Wu, J. S., Vyas, P., Glowatzki, E., and Fuchs, P. A. (2018). Opposing Expression Gradients of Calcitonin-Related Polypeptide Alpha (Calca/Cgrp α) and Tyrosine Hydroxylase (Th) in Type II Afferent Neurons of the Mouse Cochlea. J. Comp. Neurol. 526, 425–438. doi:10.1002/cne.24341
Wu, J. S., Yi, E., Manca, M., Javaid, H., Lauer, A. M., and Glowatzki, E. (2020). Sound Exposure Dynamically Induces Dopamine Synthesis in Cholinergic LOC Efferents for Feedback to Auditory Nerve Fibers. Elife 9, e52419. doi:10.7554/eLife.52419
Yang Zheng, X., Henderson, D., McFadden, S. L., Ding, D. L., and Salvi, R. J. (1999). Auditory Nerve Fiber Responses Following Chronic Cochlear De-efferentation. J. Comp. Neurol. 406, 72–86. doi:10.1002/(sici)1096-9861(19990329)406:1<72:aid-cne5>3.3.co;2-1
Yates, G. K. (1991). Auditory-nerve Spontaneous Rates Vary Predictably with Threshold. Hear. Res. 57, 57–62. doi:10.1016/0378-5955(91)90074-j
Yin, H., Chao, L., and Chao, J. (2004). Adrenomedullin Protects against Myocardial Apoptosis after Ischemia/reperfusion through Activation of Akt-GSK Signaling. Hypertension 43, 109–116. doi:10.1161/01.hyp.0000103696.60047.55
Ylikoski, J., Pirvola, U., Häppölä, O., Panula, P., and Virtanen, I. (1989). Immunohistochemical Demonstration of Neuroactive Substances in the Inner Ear of Rat and Guinea Pig. Acta Otolaryngol. 107, 417–423. doi:10.3109/00016488909127533
Zhang, Y., Li, Y., Fu, X., Wang, P., Wang, Q., Meng, W., et al. (2021). The Detrimental and Beneficial Functions of Macrophages after Cochlear Injury. Front. Cell Dev. Biol. 9, 631904. doi:10.3389/fcell.2021.631904
Keywords: cochlea, CGRP, auditory nerve, auditory brainstem response, lateral olivocochlear efferent
Citation: Le Prell CG, Hughes LF, Dolan DF and Bledsoe SC (2021) Effects of Calcitonin-Gene-Related-Peptide on Auditory Nerve Activity. Front. Cell Dev. Biol. 9:752963. doi: 10.3389/fcell.2021.752963
Received: 04 August 2021; Accepted: 20 October 2021;
Published: 12 November 2021.
Edited by:
Isabel Varela-Nieto, Consejo Superior de Investigaciones Científicas (CSIC), SpainReviewed by:
Anne E. Luebke, University of Rochester, United StatesRudolf Glueckert, Innsbruck Medical University, Austria
Copyright © 2021 Le Prell, Hughes, Dolan and Bledsoe. This is an open-access article distributed under the terms of the Creative Commons Attribution License (CC BY). The use, distribution or reproduction in other forums is permitted, provided the original author(s) and the copyright owner(s) are credited and that the original publication in this journal is cited, in accordance with accepted academic practice. No use, distribution or reproduction is permitted which does not comply with these terms.
*Correspondence: Colleen G. Le Prell, Y29sbGVlbi5sZXByZWxsQHV0ZGFsbGFzLmVkdQ==