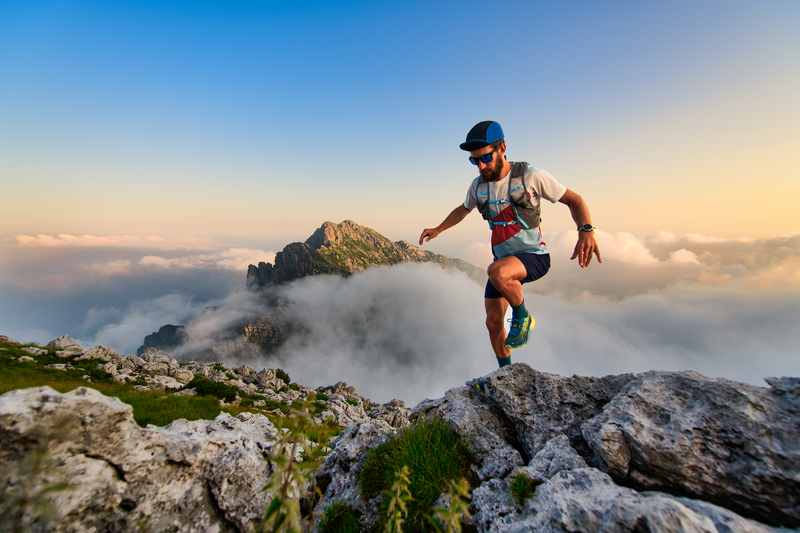
95% of researchers rate our articles as excellent or good
Learn more about the work of our research integrity team to safeguard the quality of each article we publish.
Find out more
MINI REVIEW article
Front. Cell Dev. Biol. , 29 October 2021
Sec. Cell Adhesion and Migration
Volume 9 - 2021 | https://doi.org/10.3389/fcell.2021.750775
This article is part of the Research Topic Space Mechanobiology and Medicine View all 9 articles
In recent years, there has been an increasing interest in space exploration, supported by the accelerated technological advancements in the field. This has led to a new potential environment that humans could be exposed to in the very near future, and therefore an increasing request to evaluate the impact this may have on our body, including health risks associated with this endeavor. A critical component in regulating the human pathophysiology is represented by the cardiovascular system, which may be heavily affected in these extreme environments of microgravity and radiation. This mini review aims to identify the impact of microgravity and radiation on the cardiovascular system. Being able to understand the effect that comes with deep space explorations, including that of microgravity and space radiation, may also allow us to get a deeper understanding of the heart and ultimately our own basic physiological processes. This information may unlock new factors to consider with space exploration whilst simultaneously increasing our knowledge of the cardiovascular system and potentially associated diseases.
Cardiovascular disease (CVD) is one of the leading causes of death in adults in many countries (Lozano et al., 2012). Due to the devastating rate of fatality, many researchers have been motivated to develop clinical models to simulate responses to treatment and drugs to CVD (Gentile, 2016; Polonchuk et al., 2017, 2021; Roche et al., 2021; Sharma and Gentile, 2021; Sharma et al., 2021). New approach and concept are needed to understand the complexity of cardiac function and disease onset. Interestingly, the effects of spaceflight on the human body appear to mimic an accelerated aging process that negatively impacts the heart and the cardiovascular system and closely resembles CVD (Hughson et al., 2016, 2018). Furthermore, the heart is also known to be sensitive to radiation; therefore, the response and any associated risk and diseases need to be considered when examining the impact of space exploration. As such, a deeper understanding of the cardiogenic effects of microgravity and radiation may have implications for human spaceflight as well as treat millions of heart disease patients on Earth. As microgravity and radiation happen simultaneously during space travel, it is of great interest to study the compounding impact on living matter exposed to microgravity and radiation environment at the same time. Due to the complexity of such research, there is still much unknown regarding the effect of spaceflight on the cardiovascular system. In this mini review, a new approach toward the understanding of cardiac function and disease can be found in space mechanobiology. Space mechanobiology can be defined as the study of how cells are influenced by their physical environment and in this case by the microgravity environment. To fully understand the function and underlying mechanisms of cardiac function and the mechanism and markers for CVD, it is important to first understand how cardiac cells transduce these mechanical stimuli. The extracellular matrix (ECM) plays a vital part in carrying and providing mechanical cues to these cardiac cells. These mechanical cues are then transformed into biological responses (Martino et al., 2018). As the heart is a mechanical organ that is highly responsive to different types of mechanical stimuli (Takahashi et al., 2013; Guo and Huebsch, 2020), this mini review will demonstrate the importance of mechanotransduction and how space microgravity and radiation can be leveraged to advance our understanding of cardiac function and disease.
Exposure to microgravity which differs from the normal state of gravity, puts the human body under critical physiological changes, some of the considerable importance to survival. Gravity is an important factor of fluid distribution and therefore plays an important factor in the cardiovascular system. Under normal gravity where the human body is in an upright position, there is higher arterial pressure (200 mmHg) in the feet and lower pressure (70 mmHg) in the head. Whereas the heart relative to arterial pressure is 100 mmHg. In a microgravity environment this fluid distribution is altered, losing the gradient and the fluid distribution becomes more uniform throughout the body (Hargens and Richardson, 2009; Demontis et al., 2017). This, in turn, reduces the demand for arterial blood pressure and decreases the amount of work the heart has to do leading to cardiovascular deconditioning and increased risk of CVD.
Head-down tilt is one ground-based analog model for studying simulated microgravity and therefore cardiovascular deconditioning effects, where previous reports utilizing this model have shown a significant elevation of microvascular pressures in the head, and increased capillary fluid filtration (Parazynski et al., 1991; Christ et al., 2001). Short-duration spaceflight has shown increased capillary fluid filtration, as well; however, this phenomenon is unknown in the context of long-duration spaceflight (Leach et al., 1996). These microvascular adaptations are related with the fluid redistribution phenomenon highlighted previously, and in turn, suggest vascular adaptations. Several ex vivo studies have shown that the structure and function of blood vessels certainly adapts to spaceflight, real and simulated, however, there currently exist no in vivo rodent equivalent studies (Delp, 1999, 2007). There exists a much more thorough literature of in vivo studies, both of crew and rodent analog models, spaceflight and ground based. Observations from astronauts that have returned from a 6-month spaceflight have shown an increase in stiffness in the carotid artery, which is comparable to 20 years of aging (Kawasaki et al., 1987; Gepner et al., 2014; Hughson et al., 2016). This reduced cardiac function leads to a reduction in tissue mass of the heart, also known as cardiac atrophy, which ultimately causes debilitating changes in heart function. More recent in vivo vascular studies of crew during long-duration International Space Station (ISS) missions have shown spaceflight-induced structural and functional changes of the carotid and brachial arteries. From this study, no changes in carotid or brachial arteries were generally seen; however, it is important to note that crew flight studies have a small sample size and variability between crew responses that may influence certain measures. For indeed in this study, cardiovascular biomarkers of oxidative stress and inflammation (e.g., increased PGF2a, oxidized LDL, TNF-a, myeloperoxidase, etc.) were elevated during spaceflight (Lee et al., 2020). Indeed, there are suggestions that all astronauts more notably the Apollo astronauts that traveled to the Moon and back had comparatively greater CVD risks (Delp et al., 2016).
Although it remains unclear how future exploration missions, and as a result, extended altered gravity field durations and higher radiation exposures may exacerbate these effects. On-going and future studies and further in vivo rodent studies complemented with ex vivo and in vitro analyses to assess the functional, structural, and biochemical adaptations of cardiovascular adaptations to spaceflight can be utilized in the field of space mechanobiology for more in-depth study for the cardiovascular system.
Spaceflight exposure also leads to various cellular and organ adaptations, though the in vivo study of cardiovascular adaptations, whether through actual and/or ground simulated studies, is limited. Several studies have looked at the impact of microgravity on cell proliferation and differentiation. These studies have demonstrated that stem cells that were grown in a microgravity environment grow differently from those grown under normal gravity, showing changes in division, contraction, and migration (Zhang et al., 2015; Shinde et al., 2016).
Recent studies that involved the cells of the cardiovascular system looked at cardiac progenitor cells (CPCs) cultured on the ISS. Alterations to transcriptional control were measured and it was found that among both neonatal and adult CPCs, the significantly dysregulated microRNAs affect cytoskeletal remodeling and mechanotransduction pathways. Genes related to mechanotransduction were downregulated, while the expression of cytoskeletal genes and calcium signaling molecules was significantly elevated only in neonatal CPCs. Cytoskeletal organization and migration were both affected by spaceflight in neonatal and adult CPCs, however, only neonatal CPCs experienced increased expression of early developmental markers and a higher potential to proliferate (Baio et al., 2018; Camberos et al., 2019, 2021).
Wnorowski et al. (2019) have shown that monolayers of beating cardiomyocytes derived from human induced pluripotent stem cells (hiPSC-CMs) that were sent to the ISS for a period of 5.5 weeks, had no significant effect on the morphology. Looking at functional differences between the two samples, there were no significant difference in velocity during contraction and relaxation. However, some functional differences were observed, where the space-flown hiPSC-CMs had a decreased calcium recycling rate as well as an observed beating irregularity when the samples were assessed following their return to Earth. These results suggested that following the samples return to normal gravity, their calcium-handling-related parameters remained altered (Wnorowski et al., 2019).
Although Baio et al. (2018) and Wnorowski et al. (2019) had similar microgravity conditions with the samples flown to the ISS, there were discrepancies in results including the contradiction in the expression of DNA repair genes and this may be attributed by several experimental conditions, one of them being that the type of cells used by Baio et al. (2018) being progenitor cells and Wnorowski et al. (2019) used differentiated cardiomyocytes. There are other experimental conditions that can be considered between these and other experiments in the field, including the duration of exposure to microgravity and the way the cells were processed for the assays. Other studies including different cell types in the cardiovascular response to simulated microgravity is summarized in Table 1A and the response to spaceflight microgravity is summarized in Table 1B.
Space radiation consists of solar and cosmic galactic rays (CGR) mainly composed of respectively, low and high energy protons, alpha particles and a minority of heavy charged particles and nuclei. Solar Particle Events (SPE) are temporary phenomena that increase the flux of protons. Astronauts are exposed to these solar and cosmic components only during Extra Vehicular Activities (EVA) and most of the times they remain inside their spacecraft or the ISS, where SPE could still be considered. Another source of radiation is the interaction of solar and CGR with shielding, creating high Linear Energy Transfer (LET) but low energy metallic particles and protons. The inside of the vessel is also subjected to a higher constant low dose of gamma radiation, about twice the highest natural radiation background found on Earth (Restier-Verlet et al., 2021).
When discussing radiation, we measure the “activity” of radiation where we measure how much radiation is coming out of something and “exposure” to radiation that measure the effect of radiation that has been absorbed by the substance. Radiation exposure is measured in gray (Gy), and this allows for a common unit of measurement of different types of radiation by measuring their effect on materials. Since not all radiation has the same effect biologically, we use sieverts (Sv) to measure the absorbed dose in human tissue to the biological damage to radiation (Australian Radiation Protection and Nuclear Safety Agency [ARPANSA], n.d.). The radiation doses received for space explorers depend on the time and distance from earth. Estimated doses are 150 mSv/year for space flight in the ISS, 300–400 mSv for traveling to the Moon, and 300–400 mSv/year and 1600–2300 mSv for 2 years traveling to Mars (Shavers et al., 2004; Chancellor et al., 2018; Restier-Verlet et al., 2021) in higher doses of radiation (>100 mGy) it has been clinically shown to lead to cardiac dysfunction over time, where cases have been reported following radiotherapy. Short and long-term effects of radiation induced cardiovascular diseases were observed following radiotherapy treatment of cancers adjacent to heart including lungs, breasts, and esophagus. The hearts of patients received 1.6–3.9 Gy radiation during radiotherapy treatment of peptic ulcer disease increased risk of coronary heart disease (Carr et al., 2005). In breast cancer radiotherapy, women’s hearts received doses 2.7–6.3 Gy which significantly increased risk of developing ischemic heart disease (McGale et al., 2011). Hughson et al. (2016) studies have suggested that astronauts spending 6 months in spaceflight increased stiffness of carotid artery and insulin resistance. The increase stiffness of carotid artery and insulin resistance may cause by multiple and compounding factors including radiation and microgravity. In a rat model Soucy et al. (2011) have demonstrated the detrimental effects of ionizing radiation. The rats exposed 1 Gy high-energy iron-ion radiation at approximately 0.5 Gy/min exhibit higher aortic stiffness and a greater level of endothelial dysfunction 4 months post radiation. This mainly involves increased oxidative stress as well as inflammation, playing a role in radiation-induced cardiovascular damage, which has been highlighted by multiple studies (Soucy et al., 2011; Coleman et al., 2015; Seawright et al., 2017; Table 1C). The relationship between dosage and response for some biological effects are therefore not linear and not proportional to dose (Betlazar et al., 2016, 2020, 2021; Puukila et al., 2017; Tang and Loganovsky, 2018). Chronic low doses of radiation (<100 mGy) may not be detrimental and could even induce benefits to the central nervous system and possibly to the cardiovascular system. However, it is unknown what impact of primarily low-dose rate radiation will have on the cardiovascular system during space exploration (Boerma et al., 2016), particularly in the presence of compounding factors including microgravity. Therefore, the study by combing compounding factors radiation and microgravity is crucial to examine the impact of space exploration on the cardiovascular system.
Microgravity and space radiation are unique and extreme environments which have been demonstrated to induce or model onsets of cardiovascular diseases and therefore has the potential to elucidate the underlying mechanisms of cardiovascular biology. By using microgravity and radiation as “tools” we could increase our understanding in the mechanotransduction, function and mechanisms of the cardiovascular system and subsequently the on-set of diseases.
The majority of studies have focused on utilizing stem cells and looking into their development (Table 1B). There are currently limited studies that have explored the effects of both microgravity and space radiation as a mechanism for CVD-causing processes. Both microgravity and space radiation, separately, have been shown to exhibit significant changes at both subcellular and tissue levels; however, exploring the effects of the two stressors in combination would be beneficial to our understanding of the space environment.
Endothelial cells (ECs) have been observed to form tube-like aggregates during both spaceflight and simulated gravity, confirming the influence of microgravity for 3D aggregation of ECs with and without the influence of radiation (Krüger et al., 2019). Tan et al. (2020) looked at human bronchial epithelial Beas-2B cells under simulated microgravity for 48 h followed by X-ray radiation. Although this is not specific to the cardiovascular system, the results were interesting in that the effects were shown to be additive. This study showed that the simulated microgravity on its own significantly reduced the survival of the cells and with the addition of radiation (1–6 Gy) it further inhibited cell survival -and cell proliferation (Tan et al., 2020). It is also well known that DNA double-strand breaks (DSBs) are a prominent consequence of radiation exposure, forming γH2AX foci as an early cellular response to DSBs (Sedelnikova et al., 2007; Mah et al., 2010). In this study, it was observed that the compound effect of simulated microgravity and radiation significantly promoted the formation of γH2AX foci compared to independent exposure of the two factors (Tan et al., 2020). Looking into studies done on the ISS, Wnorowski et al. (2019) observed that DNA damage and repair genes were significantly decreased in the flight samples when compared to the post-flight and ground samples. This is different to the observation from the study by Baio et al. (2018) where both the neonatal and adult CPCs showed increased expression of DNA repair genes, whilst in a study utilizing simulated microgravity on the ground, there is only increased expression of DNA repair genes in neonatal CPCs (Fuentes et al., 2015; Wnorowski et al., 2019). As results from ground simulated microgravity and spaceflight microgravity studies and the effect it has on the cardiovascular system has been conflicting there may be other factors to consider, one of them being the level of radiation that will be considerably higher aboard the ISS than on Earth (Moreno-Villanueva et al., 2017; Wnorowski et al., 2019). The difference in the level of radiation may impact the cell’s response aboard in-flight and on the ground. Molecularly, radiation and microgravity have been shown to have a negative impact on the integrity of DNA. To prevent DNA damage, cells have developed specific mechanisms that allow them to locate and repair DNA lesions. Usually, cells can withstand a moderate level of DNA damage with the help of these repair processes; however, the lack of gravity may unfavorably affect this process that tries and prevent the DNA damage, leading to the accumulation of DNA miss-matched repair (Moreno-Villanueva et al., 2017).
Previously mentioned study by Hughson et al. (2016) suggested that microgravity and radiation cause an increase in stiffness in carotid artery. The carotid artery stiffness was compared between pre- and postflight; it was seen that the stiffness was significantly increased postflight. Studies have shown that under normal gravity condition this increase in stiffness happens with the increase in age. However, this increase in stiffness could already be seen in the participants of the study as a result of the space condition (Hughson et al., 2016). Animal model further confirmed that space environment contributes to higher aortic stiffness, where rats exposed to high energy radiation that simulated the space environment also have significantly higher aortic stiffness over a 6-month period (Soucy et al., 2011). Although the mechanism for the change is different between spaceflight and aging, the magnitude of change is significant. Arterial stiffness has also been believed to be a precursor to atherosclerosis and a marker for increased cardiovascular disease risk such as myocardial infarction (O’Rourke, 1995; Safar et al., 2003; Zieman et al., 2005). The majority of upregulated proteins in the aortic wall are involved in the actin cytoskeleton organization and by identifying the exact molecular mechanism and mechanical regulators associated with arterial stiffness, it can aid in identifying therapeutic intervention (Miotto et al., 2021). Interestingly, the increased stiffness in the carotid artery that is normally seen in the aging cardiovascular system in the population can be simulated by spaceflight (Kawasaki et al., 1987; Lakatta and Levy, 2003; Gepner et al., 2014; Hughson et al., 2016). This process can be utilized in the field of space mechanobiology for more in-depth study of the mechanotransduction responses in the cardiovascular system in relation to cardiovascular diseases.
There are a number of proteins that are sensitive and responsive to changes in the mechanical environment and looking at these key proteins could elucidate the relationship between cell function and their responses to these mechanical cues. By looking at the expressions of these proteins, it can then be used as a measure of mechanotransduction (Chin et al., 2019).
The Yes-associated protein (YAP) and its paralog TAZ (transcription co-activator) have been demonstrated as major regulators of cardiac cell survival, proliferation, and differentiation (Xin et al., 2011; von Gise et al., 2012; Lin et al., 2014). It has been found that activation of YAP improves cardiac regeneration (Xin et al., 2013). Furthermore, when cardiomyocyte-specific activated YAP is overexpressed, there is an increase in the proliferation of cardiomyocytes which leads to a cardiac overgrowth in neonatal mice (Xin et al., 2011). In supporting this, it has been found that YAP protein can be detected in neonatal hearts; however, with age, the expression decreases, while the phosphorylation of YAP increases with age (von Gise et al., 2012). However, nuclear YAP expression that is usually missing in adult cardiomyocytes shows in infarcted cardiac tissue at the border of the infarcted region. The reason for this could be a result of the increased stiffness in the ECM that is exhibited in the infarcted area (Mosqueira et al., 2014). Interestingly, both spaceflight and simulated microgravity has been shown to upregulate YAP1 expression in adult CPCs and downregulation in neonatal CPCs (Baio et al., 2018; Camberos et al., 2019). This indicates that microgravity can induce functions and responses to damage on the heart.
Another key cardiac mechanotransducer associated with the mechanical stretching of cardiac tissues is Piezo1, which is a membrane mechanosensor. Activation of Piezo1 occurs due to the vascular shear stress in the human body, which is derived through the effects of heart contraction (Beech and Kalli, 2019). Recently it has been shown that Piezo1 in cardiomyocytes is triggered by cell stretching (Guo et al., 2020), which indicates that Piezo1 plays a vital part as an ion channel contributing to the feedback of the mechano-electric response of the cardiac cells utilizing its Ca2+ transient control during cardiac cell stretching. Another study confirmed that the Piezo1 channel directly converts the mechanical stretch of cardiomyocytes into calcium ion (Ca2+) and reactive oxygen species (ROS) signaling, and when Piezo1 is overexpressed or deleted, it resulted in heart dysfunction (Jiang et al., 2021).
It is evident that mechanotransduction in the form of mechanical cues, ECM and mechanical load in the form of gravity plays a pivotal role in cardiac homeostasis beyond just YAP and PIEZO. The current gap lies in the fundamental drivers of these functions and mechanisms remains unresolved.
The human heart is a vital organ that pumps blood throughout the body using the circulatory system to supply nutrients and remove waste from the tissues. As it is a vital part of the human body, it is important that the issue of cardiovascular health and diseases are understood and addressed. The complexity of the cellular anatomy of the human heart makes it challenging to develop a clinically relevant model. Therefore, future effort to address this could include the use of novel in vitro models, including organs on a chip to mature cells (Chen et al., 2013; Ren et al., 2013; Sharma et al., 2021). Even with an established in vitro and/or in vivo model, to initiate the onset of CVD conditions remains a challenge. As outlined in this mini review, cardiac mechanotransduction plays an important role in not only understanding the cardiovascular system, but also in the maturation and the functional response of the cardiac tissue. It has been demonstrated in studies how cardiac mechanosensing regulators including YAP and PIEZO1 regulate cardiac tissue function and dysfunction, including cardiac cell survival, proliferation, differentiation and responses to mechanical stimuli (Xin et al., 2011; von Gise et al., 2012; Lin et al., 2014; Beech and Kalli, 2019; Guo et al., 2020; Jiang et al., 2021). The main effects of microgravity and radiation on the cardiovascular system has been demonstrated to show changes can be seen from a physiological to a cellular level, including changes to cardiac and endothelial function, increased stiffness of carotid artery, oxidative stress and inflammation as well as altered fluid distribution and decreased calcium recycling rate. As further highlighted in this mini-review the complexity and cross-talk between different mechanosensors and regulators remain elusive and reinforce the opportunities for cardiac and space biologists in investigating this interface of cell biology and biomedical engineering.
A key element that was not discussed in this mini review is the technologies to conduct microgravity and/or simulated microgravity research but there are clear advancements in this area made available for researchers. Whilst there have been several studies in space on the cardiac responses and genetic and epigenetic profiling on the effects of microgravity, this sets the foundation and the stage to examine the underlying cellular mechanisms of the cardiovascular system. More comparison between experiments on Earth and ISS needs to be done to validate the simulations done on the ground and open more doors to research that simulate the space conditions which will allow us to look into space mechanobiology with greater control. Furthermore, as demonstrated in this mini review, the majority of research shown on cardiac cells have been done on microgravity and radiation independently; however, there are limited studies that combine these two external stressors on cells of cardiovascular origin. It is also important to note that the effects of microgravity and radiation has been varied depending on factors such as cell type, specific bioreactor/cultured environment used and the type of exposure.
The unwavering question of whether microgravity or radiation or the dual effects have on cardiac function remains open for discovery and ultimately both of these conditions are a new type of extreme environment in which researchers can uncover knowledge from cellular to organ level. As shown in this mini review, there is still a great amount of unknown to the underlying mechanisms of cardiac function and CVD onsets, and whilst there exist several novel and innovative approaches toward advancing this understanding, gap in knowledge remains. The research outlined in this mini review provides a glimpse into what can be achieved through microgravity research in advancing cardiac knowledge and with current CVD death toll still remaining high, it may be time to take an out of this world approach toward CVD.
CB wrote the manuscript. SN contributed to the section “The Effect of Microgravity on the Cardiovascular System.” MF, G-JL, RM, and NH contributed to the section “The Effect of Radiation on the Cardiovascular System.” CG, BM, KP, and JC initiated, conceptualized the review, and edited the manuscript. All authors have read and agreed to the published version of the manuscript.
The author acknowledges the support of the Australian Research Council Discovery Project (ARC DP) [DP190101973 to JC, the support of The University of Sydney (Cardiothoracic Surgery Research Grant)], UTS (Seed Funding), and Catholic Archdiocese of Sydney (Grant for Adult Stem Cell Research), Ian Potter Foundation Grant to CG.
The authors declare that the research was conducted in the absence of any commercial or financial relationships that could be construed as a potential conflict of interest.
All claims expressed in this article are solely those of the authors and do not necessarily represent those of their affiliated organizations, or those of the publisher, the editors and the reviewers. Any product that may be evaluated in this article, or claim that may be made by its manufacturer, is not guaranteed or endorsed by the publisher.
We would like to acknowledge Australian Government Research Training Program Scholarship and AINSE Limited for providing financial assistance (Award – Residential Student Scholarship 2020 to CB) to enable work on this research. MF received the support of Centre National d’etudes Spatiales (CNES). Figure 1 was provided by ©Chelsea Ly including illustrations from BioRender.com.
CVD, cardiovascular disease; ECM, extracellular matrix; ISS, International Space Station; CPCs, cardiac progenitor cells; hiPSC-CMs, human induced pluripotent stem cells; CGR, cosmic galactic rays; SPE, Solar Particle Events; EVA, Extra Vehicular Activities; LET, Linear Energy Transfer; ECs, endothelial cells; DSBs, DNA double strand breaks; YAP, yes-associated protein; TAZ, transcription co-activator; Ca2+, calcium ion; ROS, reactive oxygen species; RPM, random positioning machine; RWV, rotating wall vessel.
Australian Radiation Protection and Nuclear Safety Agency [ARPANSA] (n.d.). Units of Ionising Radiations Measurement. Available Online at: https://www.arpansa.gov.au/understanding-radiation/what-is-radiation/radiation/measurement (accessed 09 September 2021)
Baio, J., Martinez, A. F., Silva, I., Hoehn, C. V., Countryman, S., Bailey, L., et al. (2018). Cardiovascular progenitor cells cultured aboard the International Space Station exhibit altered developmental and functional properties. NPJ Microgravity 4:13. doi: 10.1038/s41526-018-0048-x
Beech, D. J., and Kalli, A. C. (2019). Force Sensing by Piezo Channels in Cardiovascular Health and Disease. Arterioscler. Thromb. Vasc. Biol. 39, 2228–2239. doi: 10.1161/ATVBAHA.119.313348
Betlazar, C., Middleton, R. J., Banati, R., and Liu, G.-J. (2020). The Translocator Protein (TSPO) in Mitochondrial Bioenergetics and Immune Processes. Cells 9:512. doi: 10.3390/cells9020512
Betlazar, C., Middleton, R. J., Banati, R. B., and Liu, G.-J. (2016). The impact of high and low dose ionisingionizing radiation on the central nervous system. Redox Biol. 9, 144–156. doi: 10.1016/j.redox.2016.08.002
Betlazar, C., Middleton, R. J., Howell, N. R., Storer, B., Davis, E., Davies, J., et al. (2021). Mitochondrial translocator protein (TSPO) expression in the brain after whole body gamma irradiation. Front. Cell Dev. Biol. (In press).
Boerma, M., Sridharan, V., Mao, X.-W., Nelson, G. A., Cheema, A. K., Koturbash, I., et al. (2016). Effects of ionizing radiation on the heart. Mutat. Res. 770, 319–327. doi: 10.1016/j.mrrev.2016.07.003
Camberos, V., Baio, J., Bailey, L., Hasaniya, N., Lopez, L. V., and Kearns-Jonker, M. (2019). Effects of Spaceflight and Simulated Microgravity on YAP1 Expression in Cardiovascular Progenitors: implications for Cell-Based Repair. Int. J. Mol. Sci. 20:2742. doi: 10.3390/ijms20112742
Camberos, V., Baio, J., Mandujano, A., Martinez, A. F., Bailey, L., Hasaniya, N., et al. (2021). The Impact of Spaceflight and Microgravity on the Human Islet-1+ Cardiovascular Progenitor Cell Transcriptome. Int. J. Mol. Sci. 22:3577. doi: 10.3390/ijms22073577
Carr, Z. A., Land, C. E., Kleinerman, R. A., Weinstock, R. W., Stovall, M., Griem, M. L., et al. (2005). Coronary heart disease after radiotherapy for peptic ulcer disease. Int. J. Radiat. Oncol. Biol. Phys. 61, 842–850. doi: 10.1016/j.ijrobp.2004.07.708
Chancellor, J. C., Blue, R. S., Cengel, K. A., Auñón-Chancellor, S. M., Rubins, K. H., Katzgraber, H. G., et al. (2018). Limitations in predicting the space radiation health risk for exploration astronauts. NPJ Microgravity 4:8. doi: 10.1038/s41526-018-0043-2
Chen, M. B., Srigunapalan, S., Wheeler, A. R., and Simmons, C. A. (2013). A 3D microfluidic platform incorporating methacrylated gelatin hydrogels to study physiological cardiovascular cell–cell interactions. Lab Chip 13, 2591–2598. doi: 10.1039/c3lc00051f
Chin, I. L., Hool, L., and Choi, Y. S. (2019). A Review of in vitro Platforms for Understanding Cardiomyocyte Mechanobiology. Front. Bioeng. Biotechnol. 7:133. doi: 10.3389/fbioe.2019.00133
Christ, F., Gamble, J., Baranov, V., Kotov, A., Chouker, A., Thiel, M., et al. (2001). Changes in microvascular fluid filtration capacity during 120 days of 6° head-down tilt. J. Appl. Physiol. 91, 2517–2522. doi: 10.1152/jappl.2001.91.6.2517
Coleman, M. A., Sasi, S. P., Onufrak, J., Natarajan, M., Manickam, K., Schwab, J., et al. (2015). Low-dose radiation affects cardiac physiology: gene networks and molecular signaling in cardiomyocytes. Am. J. Physiol. Heart Circ. Physiol. 309, H1947–H1963. doi: 10.1152/ajpheart.00050.2015
Delp, M. D. (1999). Myogenic and vasoconstrictor responsiveness of skeletal muscle arterioles is diminished by hindlimb unloading. J. Appl. Physiol. 86, 1178–1184. doi: 10.1152/jappl.1999.86.4.1178
Delp, M. D. (2007). Arterial adaptations in microgravity contribute to orthostatic tolerance. J. Appl. Physiol. 102, 836–836. doi: 10.1152/japplphysiol.01347.2006
Delp, M. D., Charvat, J. M., Limoli, C. L., Globus, R. K., and Ghosh, P. (2016). Apollo Lunar Astronauts Show Higher Cardiovascular Disease Mortality: possible Deep Space Radiation Effects on the Vascular Endothelium. Sci. Rep. 6:29901. doi: 10.1038/srep29901
Demontis, G. C., Germani, M. M., Caiani, E. G., Barravecchia, I., Passino, C., and Angeloni, D. (2017). Human Pathophysiological Adaptations to the Space Environment. Front. Physiol. 8:547. doi: 10.3389/fphys.2017.00547
Feger, B. J., Thompson, J. W., Dubois, L. G., Kommaddi, R. P., Foster, M. W., Mishra, R., et al. (2016). Microgravity induces proteomics changes involved in endoplasmic reticulum stress and mitochondrial protection. Sci. Rep. 6:34091. doi: 10.1038/srep34091
Freed, L. E., and Vunjak-Novakovic, G. (1997). Microgravity tissue engineering. In Vitro Cell. Dev. Biol. Animal. 33, 381–385. doi: 10.1007/s11626-997-0009-2
Fuentes, T. I., Appleby, N., Raya, M., Bailey, L., Hasaniya, N., Stodieck, L., et al. (2015). Simulated Microgravity Exerts an Age-Dependent Effect on the Differentiation of Cardiovascular Progenitors Isolated from the Human Heart. PLoS One 10:e0132378. doi: 10.1371/journal.pone.0132378
Gentile, C. (2016). Filling the Gaps between the In Vivo and In Vitro Microenvironment: engineering of Spheroids for Stem Cell Technology. Curr. Stem Cell Res. Ther. 11, 652–665. doi: 10.2174/1574888X10666151001114848
Gepner, A. D., Korcarz, C. E., Colangelo, L. A., Hom, E. K., Tattersall, M. C., Astor, B. C., et al. (2014). Longitudinal Effects of a Decade of Aging on Carotid Artery Stiffness: the Multiethnic Study of Atherosclerosis. Stroke 45, 48–53. doi: 10.1161/STROKEAHA.113.002649
Grabham, P., Sharma, P., Bigelow, A., and Geard, C. (2013). Two distinct types of the inhibition of vasculogenesis by different species of charged particles. Vasc. Cell 5:16. doi: 10.1186/2045-824X-5-16
Grimm, D., Infanger, M., Westphal, K., Ulbrich, C., Pietsch, J., Kossmehl, P., et al. (2009). A Delayed Type of Three-Dimensional Growth of Human Endothelial Cells Under Simulated Weightlessness. Tissue Eng. Part A 15, 2267–2275. doi: 10.1089/ten.tea.2008.0576
Guo, J., and Huebsch, N. (2020). Modeling the Response of Heart Muscle to Mechanical Stimulation In Vitro. Curr. Tissue Microenviron. Rep. 1, 61–72. doi: 10.1007/s43152-020-00007-8
Guo, Y., Merten, A.-L., Schöler, U., Yu, Z.-Y., Cvetkovska, J., Fatkin, D., et al. (2020). In vitro cell stretching technology (IsoStretcher) as an approach to unravel Piezo1-mediated cardiac mechanotransduction. Prog. Biophys. Mol. Biol. 159, 22–33. doi: 10.1016/j.pbiomolbio.2020.07.003
Hargens, A. R., and Richardson, S. (2009). Cardiovascular adaptations, fluid shifts, and countermeasures related to space flight. Respir. Physiol. Neurobiol. 169, S30–S33. doi: 10.1016/j.resp.2009.07.005
Hughson, R. L., Helm, A., and Durante, M. (2018). Heart in space: effect of the extraterrestrial environment on the cardiovascular system. Nat. Rev. Cardiol. 15, 167–180. doi: 10.1038/nrcardio.2017.157
Hughson, R. L., Robertson, A. D., Arbeille, P., Shoemaker, J. K., Rush, J. W. E., Fraser, K. S., et al. (2016). Increased postflight carotid artery stiffness and inflight insulin resistance resulting from 6-mo spaceflight in male and female astronauts. Am. J. Physiol. Heart Circ. Physiol. 310, H628–H638. doi: 10.1152/ajpheart.00802.2015
Infanger, M., Kossmehl, P., Shakibaei, M., Baatout, S., Witzing, A., Grosse, J., et al. (2006). Induction of three-dimensional assembly and increase in apoptosis of human endothelial cells by simulated microgravity: impact of vascular endothelial growth factor. Apoptosis 11, 749–764. doi: 10.1007/s10495-006-5697-7
Jha, R., Wu, Q., Singh, M., Preininger, M. K., Han, P., Ding, G., et al. (2016). Simulated Microgravity and 3D Culture Enhance Induction, Viability, Proliferation and Differentiation of Cardiac Progenitors from Human Pluripotent Stem Cells. Sci. Rep. 6:30956. doi: 10.1038/srep30956
Jiang, F., Yin, K., Wu, K., Zhang, M., Wang, S., Cheng, H., et al. (2021). The mechanosensitive Piezo1 channel mediates heart mechano-chemo transduction. Nat. Commun. 12:869. doi: 10.1038/s41467-021-21178-4
Kang, C.-Y., Zou, L., Yuan, M., Wang, Y., Li, T.-Z., Zhang, Y., et al. (2011). Impact of simulated microgravity on microvascular endothelial cell apoptosis. Eur. J. Appl. Physiol. 111, 2131–2138. doi: 10.1007/s00421-011-1844-0
Kawasaki, T., Sasayama, S., Yagi, S.-I., Asakawa, T., and Hirai, T. (1987). Non-invasive assessment of the age related changes in stiffness of major branches of the human arteries. Cardiovasc. Res. 21, 678–687. doi: 10.1093/cvr/21.9.678
Koturbash, I., Miousse, I. R., Sridharan, V., Nzabarushimana, E., Skinner, C. M., Melnyk, S. B., et al. (2016). Radiation-induced changes in DNA methylation of repetitive elements in the mouse heart. Mutat. Res. 787, 43–53. doi: 10.1016/j.mrfmmm.2016.02.009
Krüger, M., Pietsch, J., Bauer, J., Kopp, S., Carvalho, D. T. O., Baatout, S., et al. (2019). Growth of Endothelial Cells in Space and in Simulated Microgravity – a Comparison on the Secretory Level. Cell. Physiol. Biochem. 52, 1039–1060. doi: 10.33594/000000071
Lakatta, E. G., and Levy, D. (2003). Arterial and Cardiac Aging: major Shareholders in Cardiovascular Disease Enterprises: part I: aging Arteries: a “Set Up” for Vascular Disease. Circulation 107, 139–146. doi: 10.1161/01.CIR.0000048892.83521.58
Leach, C. S., Alfrey, C. P., Suki, W. N., Leonard, J. I., Rambaut, P. C., Inners, L. D., et al. (1996). Regulation of body fluid compartments during short-term spaceflight. J. Appl. Physiol. 81, 105–116. doi: 10.1152/jappl.1996.81.1.105
Lee, S. M. C., Ribeiro, L. C., Martin, D. S., Zwart, S. R., Feiveson, A. H., Laurie, S. S., et al. (2020). Arterial structure and function during and after long-duration spaceflight. J. Appl. Physiol. 129, 108–123. doi: 10.1152/japplphysiol.00550.2019
Lin, Z., von Gise, A., Zhou, P., Gu, F., Ma, Q., Jiang, J., et al. (2014). Cardiac-Specific YAP Activation Improves Cardiac Function and Survival in an Experimental Murine MI Model. Circ. Res. 115, 354–363. doi: 10.1161/CIRCRESAHA.115.303632
Liu, C., Zhong, G., Zhou, Y., Yang, Y., Tan, Y., Li, Y., et al. (2020). Alteration of calcium signalling in cardiomyocyte induced by simulated microgravity and hypergravity. Cell Prolif. 53:e12783. doi: 10.1111/cpr.12783
Lozano, R., Naghavi, M., Foreman, K., Lim, S., Shibuya, K., Aboyans, V., et al. (2012). Global and regional mortality from 235 causes of death for 20 age groups in 1990 and 2010: a systematic analysis for the Global Burden of Disease Study 2010. Lancet 380, 2095–2128. doi: 10.1016/S0140-6736(12)61728-0
Mah, L.-J., El-Osta, A., and Karagiannis, T. C. (2010). γH2AX: a sensitive molecular marker of DNA damage and repair. Leukemia 24, 679–686. doi: 10.1038/leu.2010.6
Martino, F., Perestrelo, A. R., Vinarský, V., Pagliari, S., and Forte, G. (2018). Cellular Mechanotransduction: from Tension to Function. Front. Physiol. 9:824. doi: 10.3389/fphys.2018.00824
McGale, P., Darby, S. C., Hall, P., Adolfsson, J., Bengtsson, N.-O., Bennet, A. M., et al. (2011). Incidence of heart disease in 35,000 women treated with radiotherapy for breast cancer in Denmark and Sweden. Radiother. Oncol. 100, 167–175. doi: 10.1016/j.radonc.2011.06.016
Miotto, D. S., Dionizio, A., Jacomini, A. M., Zago, A. S., Buzalaf, M. A. R., and Amaral, S. L. (2021). Identification of Aortic Proteins Involved in Arterial Stiffness in Spontaneously Hypertensive Rats Treated With Perindopril:A Proteomic Approach. Front. Physiol. 12:624515. doi: 10.3389/fphys.2021.624515
Moreno-Villanueva, M., Wong, M., Lu, T., Zhang, Y., and Wu, H. (2017). Interplay of space radiation and microgravity in DNA damage and DNA damage response. NPJ Microgravity 3:14. doi: 10.1038/s41526-017-0019-7
Mosqueira, D., Pagliari, S., Uto, K., Ebara, M., Romanazzo, S., Escobedo-Lucea, C., et al. (2014). Hippo pathway effectors control cardiac progenitor cell fate by acting as dynamic sensors of substrate mechanics and nanostructure. ACS Nano 8, 2033–2047. doi: 10.1021/nn4058984
O’Rourke, M. (1995). Mechanical Principles in Arterial Disease. Hypertension 26, 2–9. doi: 10.1161/01.HYP.26.1.2
Parazynski, S. E., Hargens, A. R., Tucker, B., Aratow, M., Styf, J., and Crenshaw, A. (1991). Transcapillary fluid shifts in tissues of the head and neck during and after simulated microgravity. J. Appl. Physiol. 71, 2469–2475. doi: 10.1152/jappl.1991.71.6.2469
Polonchuk, L., Chabria, M., Badi, L., Hoflack, J.-C., Figtree, G., Davies, M. J., et al. (2017). Cardiac spheroids as promising in vitro models to study the human heart microenvironment. Sci. Rep. 7:7005. doi: 10.1038/s41598-017-06385-8
Polonchuk, L., Surija, L., Lee, M. H., Sharma, P., Liu Chung Ming, C., Richter, F., et al. (2021). Towards engineering heart tissues from bioprinted cardiac spheroids. Biofabrication 13:045009. doi: 10.1088/1758-5090/ac14ca
Puukila, S., Lemon, J. A., Lees, S. J., Tai, T. C., Boreham, D. R., and Khaper, N. (2017). Impact of Ionizing Radiation on the Cardiovascular System: a Review. Radiat. Res. 188, 539–546. doi: 10.1667/RR14864.1
Ren, L., Liu, W., Wang, Y., Wang, J.-C., Tu, Q., Xu, J., et al. (2013). Investigation of hypoxia-induced myocardial injury dynamics in a tissue interface mimicking microfluidic device. Anal. Chem. 85, 235–244. doi: 10.1021/ac3025812
Restier-Verlet, J., El-Nachef, L., Ferlazzo, M. L., Al-Choboq, J., Granzotto, A., Bouchet, A., et al. (2021). Radiation on Earth or in Space: what Does It Change? Int. J. Mol. Sci. 22:3739. doi: 10.3390/ijms22073739
Roche, C. D., Sharma, P., Ashton, A. W., Jackson, C., Xue, M., and Gentile, C. (2021). Printability, Durability, Contractility and Vascular Network Formation in 3D Bioprinted Cardiac Endothelial Cells Using Alginate–Gelatin Hydrogels. Front. Bioeng. Biotechnol. 9:636257. doi: 10.3389/fbioe.2021.636257
Safar, M. E., Levy, B. I., and Struijker-Boudier, H. (2003). Current Perspectives on Arterial Stiffness and Pulse Pressure in Hypertension and Cardiovascular Diseases. Circulation 107, 2864–2869. doi: 10.1161/01.CIR.0000069826.36125.B4
Seawright, J. W., Samman, Y., Sridharan, V., Mao, X. W., Cao, M., Singh, P., et al. (2017). Effects of low-dose rate γ-irradiation combined with simulated microgravity on markers of oxidative stress, DNA methylation potential, and remodeling in the mouse heart. PLoS One 12:e0180594. doi: 10.1371/journal.pone.0180594
Sedelnikova, O. A., Nakamura, A., Kovalchuk, O., Koturbash, I., Mitchell, S. A., Marino, S. A., et al. (2007). DNA Double-Strand Breaks Form in Bystander Cells after Microbeam Irradiation of Three-dimensional Human Tissue Models. Cancer Res. 67, 4295–4302. doi: 10.1158/0008-5472.CAN-06-4442
Sharma, P., and Gentile, C. (2021). Cardiac Spheroids as in vitro Bioengineered Heart Tissues to Study Human Heart Pathophysiology. J. Vis. Exp. 167. doi: 10.3791/61962
Sharma, P., Wang, X., Ming, C. L. C., Vettori, L., Figtree, G., Boyle, A., et al. (2021). Considerations for the Bioengineering of Advanced Cardiac In Vitro Models of Myocardial Infarction. Small 17:2003765. doi: 10.1002/smll.202003765
Shavers, M. R., Zapp, N., Barber, R. E., Wilson, J. W., Qualls, G., Toupes, L., et al. (2004). Implementation of ALARA radiation protection on the ISS through polyethylene shielding augmentation of the Service Module Crew Quarters. Adv. Space Res. 34, 1333–1337. doi: 10.1016/j.asr.2003.10.051
Shinde, V., Brungs, S., Henry, M., Wegener, L., Nemade, H., Rotshteyn, T., et al. (2016). Simulated Microgravity Modulates Differentiation Processes of Embryonic Stem Cells. Cell. Physiol. Biochem. 38, 1483–1499. doi: 10.1159/000443090
Soucy, K. G., Lim, H. K., Kim, J. H., Oh, Y., Attarzadeh, D. O., Sevinc, B., et al. (2011). HZE 56 Fe-Ion Irradiation Induces Endothelial Dysfunction in Rat Aorta: role of Xanthine Oxidase. Radiat. Res. 176, 474–485. doi: 10.1667/RR2598.1
Takahashi, K., Kakimoto, Y., Toda, K., and Naruse, K. (2013). Mechanobiology in cardiac physiology and diseases. J. Cell. Mol. Med. 17, 225–232. doi: 10.1111/jcmm.12027
Tan, S., Pei, W., Huang, H., Zhou, G., and Hu, W. (2020). Additive effects of simulated microgravity and ionizing radiation in cell death, induction of ROS and expression of RAC2 in human bronchial epithelial cells. NPJ Microgravity 6, 1–6. doi: 10.1038/s41526-020-00123-7
Tang, F. R., and Loganovsky, K. (2018). Low dose or low dose rate ionizing radiation-induced health effect in the human. J. Environ. Radioact. 192, 32–47. doi: 10.1016/j.jenvrad.2018.05.018
von Gise, A., Lin, Z., Schlegelmilch, K., Honor, L. B., Pan, G. M., Buck, J. N., et al. (2012). YAP1, the nuclear target of Hippo signaling, stimulates heart growth through cardiomyocyte proliferation but not hypertrophy. Proc. Natl. Acad. Sci. U. S. A. 109, 2394–2399. doi: 10.1073/pnas.1116136109
Wnorowski, A., Sharma, A., Chen, H., Wu, H., Shao, N.-Y., Sayed, N., et al. (2019). Effects of Spaceflight on Human Induced Pluripotent Stem Cell-Derived Cardiomyocyte Structure and Function. Stem Cell Rep. 13, 960–969. doi: 10.1016/j.stemcr.2019.10.006
Xin, M., Kim, Y., Sutherland, L. B., Murakami, M., Qi, X., McAnally, J., et al. (2013). Hippo pathway effector Yap promotes cardiac regeneration. Proc. Natl. Acad. Sci. U. S. A. 110, 13839–13844. doi: 10.1073/pnas.1313192110
Xin, M., Kim, Y., Sutherland, L. B., Qi, X., McAnally, J., Schwartz, R. J., et al. (2011). Regulation of Insulin-Like Growth Factor Signaling by Yap Governs Cardiomyocyte Proliferation and Embryonic Heart Size. Sci. Signal. 4:ra70. doi: 10.1126/scisignal.2002278
Zhang, C., Li, L., Chen, J., and Wang, J. (2015). Behavior of stem cells under outer-space microgravity and ground-based microgravity simulation: microgravity and stem cells. Cell Biol. Int. 39, 647–656. doi: 10.1002/cbin.10452
Keywords: mechanobiology, microgravity, cardiovascular, mechanotransduction, cardiac disease, radiation
Citation: Basirun C, Ferlazzo ML, Howell NR, Liu G-J, Middleton RJ, Martinac B, Narayanan SA, Poole K, Gentile C and Chou J (2021) Microgravity × Radiation: A Space Mechanobiology Approach Toward Cardiovascular Function and Disease. Front. Cell Dev. Biol. 9:750775. doi: 10.3389/fcell.2021.750775
Received: 31 July 2021; Accepted: 11 October 2021;
Published: 29 October 2021.
Edited by:
Claudia Tanja Mierke, Leipzig University, GermanyReviewed by:
Maria A. Mariggiò, University of Studies G. d’Annunzio Chieti and Pescara, ItalyCopyright © 2021 Basirun, Ferlazzo, Howell, Liu, Middleton, Martinac, Narayanan, Poole, Gentile and Chou. This is an open-access article distributed under the terms of the Creative Commons Attribution License (CC BY). The use, distribution or reproduction in other forums is permitted, provided the original author(s) and the copyright owner(s) are credited and that the original publication in this journal is cited, in accordance with accepted academic practice. No use, distribution or reproduction is permitted which does not comply with these terms.
*Correspondence: Joshua Chou, Sm9zaHVhLmNob3VAdXRzLmVkdS5hdQ==
Disclaimer: All claims expressed in this article are solely those of the authors and do not necessarily represent those of their affiliated organizations, or those of the publisher, the editors and the reviewers. Any product that may be evaluated in this article or claim that may be made by its manufacturer is not guaranteed or endorsed by the publisher.
Research integrity at Frontiers
Learn more about the work of our research integrity team to safeguard the quality of each article we publish.