- Department of Orthopaedic Surgery, Shanghai Jiao Tong University Affiliated Sixth People’s Hospital, Shanghai, China
Macrophages are a group of heterogeneous cells widely present throughout the body. Under the influence of their specific environments, via both contact and noncontact signals, macrophages integrate into host tissues and contribute to their development and the functions of their constituent cells. Mitochondria are essential organelles that perform intercellular transfers to regulate cell homeostasis. Our review focuses on newly discovered roles of mitochondrial transfers between macrophages and surrounding cells and summarizes emerging functions of macrophages in transmitophagy, metabolic regulation, and immune defense. We also discuss the negative influence of mitochondrial transfers on macrophages, as well as current therapies targeting mitochondria in macrophages. Regulation of macrophages through mitochondrial transfers between macrophages and their surrounding cells is a promising therapy for various diseases, including cardiovascular diseases, inflammatory diseases, obesity, and cancer.
Introduction
Macrophages, which were once considered to be supplied only by adult monocytes, are now known to have both bone marrow myeloid and embryonic origins (Ginhoux and Guilliams, 2016). In addition to their immune surveillance function, macrophages show plasticity according to their environment in different tissues; thus, they have tissue-specific roles in maintaining homeostasis and tight interactions with surrounding cells (Gosselin et al., 2014; Lavin et al., 2014; Okabe and Medzhitov, 2016). Aberrant differentiation, polarization, and functions of macrophages give rise to diseases in various systems (Wynn et al., 2013; Byrne et al., 2016; Krenkel and Tacke, 2017; Peet et al., 2020). Deficient generation of anti-inflammatory macrophages and failed communication between macrophages and epithelial cells, endothelial cells, fibroblasts, and stem or tissue progenitor cells all contribute to a state of persistent injury; this can lead to pathological fibrosis (Wynn and Vannella, 2016) such as that occurring in chronic lung disease (Kim et al., 2008; O’Beirne et al., 2020). In atherosclerosis, macrophages participate in a non-resolving inflammatory response that expands the subendothelial layer and results in thrombosis (Moore and Tabas, 2011). Tumor-associated macrophages (TAMs) in the tumor microenvironment are often associated with poor prognosis and chemoresistance and have thus recently emerged as therapeutic targets (Cassetta and Pollard, 2018; Xiang et al., 2021).
Mitochondria are vital organelles that constantly undergo inner cellular movements and intracellular transfers to fulfill energy needs and promote cell survival (Liesa et al., 2009; Youle and van der Bliek, 2012; Zampieri et al., 2021). Macrophages rely strongly on mitochondria for their activation and functions (Weinberg et al., 2015; Tur et al., 2017). Recent studies have identified various tissue-resident macrophages as important participants in intercellular mitochondrial transfers, unveiling a new function of such macrophages (Phinney et al., 2015; Brestoff et al., 2020; Nicolas-Avila et al., 2020). Macrophage-related mitochondrial transfers have critical roles in tissue homeostasis, metabolic regulation, and immune defense under both physiological and pathological conditions (Tables 1, 2).
Dynamic Regulation of Mitochondria Within and Between Cells
Mitochondria are double-membrane organelles that are extensively involved in cell functions. They are widely known as the cell’s “power house” because they generate adenosine triphosphate (ATP) via oxidative phosphorylation (OXPHOS) and host essential lipid metabolism pathways (Schon et al., 2012). The mitochondrial respiratory chain on the inner membrane of mitochondria converts the power of nicotinamide adenine dinucleotide and dihydroflavine-adenine dinucleotide from the Krebs cycle to an electrochemical proton gradient across the inner membrane (Scarpulla, 2008); this electrochemical gradient fuels ATP synthase to catalyze cellular ATP (Friedman and Nunnari, 2014). Byproducts of mitochondrial redox reactions include reactive oxidative species (ROS), which can initiate diverse cellular responses ranging from cell protection to mitochondrial fission and autophagy (Zorov et al., 2014). The electrochemical proton gradient also powers Ca2+ uptake through uniporters on the inner membrane to regulate cytoplasmic Ca2+ levels (De Stefani et al., 2011). As semiautonomous organelles, mitochondria contain mitochondrial DNA (mtDNA) and are capable of self-replication. MtDNA is a 16.5-kb circular double-stranded DNA that is highly compacted within the mitochondrial matrix and encodes the core proteins of the mitochondrial respiratory chain (Anderson et al., 1981; Friedman and Nunnari, 2014).
Mitophagy: Mitochondrial Quantity and Quality Control
The integrity of mitochondria may be compromised owing to oxidative stress, starvation, ischemia–hypoxia, and aging (Spees et al., 2006; Gustafsson and Dorn, 2019; Liu et al., 2021), leading to energy exhaustion, ROS overproduction, and Ca2+-induced cell apoptosis (Bock and Tait, 2020). Mitophagy is an acute response to stress under changing developmental, bioenergetic, and environmental conditions that enable cells to meet the demands of metabolic reprogramming, mitochondrial quality control, and cell differentiation (Gustafsson and Dorn, 2019). Mitophagy is a process of cargo-specific autophagy that eliminates damaged mitochondria to regulate mitochondrial quality and quantity (Kim et al., 2007). During mitophagy, serine/threonine-protein kinase PINK1 is stabilized on the membranes of unwanted mitochondria for the subsequent recruitment of E3 ubiquitin-protein ligase parkin from the cytoplasm. Unwanted mitochondria then are marked by parkin-mediated ubiquitination in the outer mitochondrial membrane and recognized by autophagosomes (Pankiv et al., 2007; Matsuda et al., 2010; Okatsu et al., 2010). Other mitophagy pathways independent of ubiquitination are mediated by direct interaction between mitophagy receptors, including Bcl-2/adenovirus E1B 19-kDa protein-interacting protein 3-like (BINP3)/NIX, and several autophagosome proteins (Youle and Narendra, 2011; Zhang T. et al., 2016; Koentjoro et al., 2017).
Mitochondrial Transfer
As well as mitophagy, mitochondria constantly undergo changes in their position and morphology to deal with stress and to meet the cell’s demands. Changes in intracellular position are driven by the attachment and movement of mitochondria along dynamic cytoskeletal tracks (Liesa et al., 2009; Rafelski, 2013). Changes in track arrangements, interactions between mitochondria and organelles including the endoplasmic reticulum and plasma membrane, and mitochondrial fission and fusion are the main factors that influence mitochondrial intracellular movements (Chen et al., 2005; Lackner et al., 2013; Rafelski, 2013). Changes in morphology most commonly involve fission and fusion dynamics. Fusion reverses the effects of stress on the cell by allowing functional mitochondria to complement dysfunctional ones, whereas fission can lead to cleansing of daughter mitochondria by mitophagy (Youle and van der Bliek, 2012).
Mitochondria movements are not constrained within cells but also take place between cells. Mitochondrial transfers occur both under physiological conditions, e.g., in tissue homeostasis and stemness maintenance and in pathological conditions such as hypoxia, inflammation, and cancer (Liu et al., 2021). The transferred cargos may contain either healthy or damaged mitochondria. Healthy mitochondria are transferred from donor cells to protect recipient cells from oxidative stress and apoptosis and to enhance their mitochondrial respiration. In the case of stroke, astrocytes release healthy mitochondria that enter neurons to promote ATP production and viability (Islam et al., 2012; Liu et al., 2014; Hsu et al., 2016). Transfers of healthy mitochondria also occur between cancer cells to promote their survival during chemotherapy (Lou et al., 2012; Desir et al., 2016; Zampieri et al., 2021). On the other hand, stressed cells can transfer damaged mitochondria to recipient cells to ease their burden of impaired mitochondria. This occurs between injured retinal ganglion cells and adjacent astrocytes and between acute leukemia T cells and bone-marrow-derived stem cells (Davis et al., 2014; Wang et al., 2018).
Macrophage Derivation, Polarization, and Intercellular Communications
Macrophages are highly plastic cells and are present in almost all tissues, as exemplified by alveolar macrophages (AMs) in lung, Kupffer cells in liver, Langerhans cells in epidermal tissue, osteoclasts in bone, splenic macrophages in spleen red pulp, F4/80high peritoneal macrophages in peritoneum, and so on (Wynn et al., 2013). The classical definition of macrophages describes them as end cells of the mononuclear phagocytic lineage derived from circulating monocytes that originate in the bone marrow (Geissmann et al., 2010). However, more recent studies indicate heterogeneous origins of bone-marrow-derived macrophages (BMMs) in contrast to self-renewing embryo-derived ones, such as the yolk sac and fetal liver (Figure 1; Ginhoux et al., 2010; Hoeffel et al., 2012; Schulz et al., 2012). Many tissues contain both local self-renewing and peripheral monocyte-derived populations of macrophages (Schulz et al., 2012).
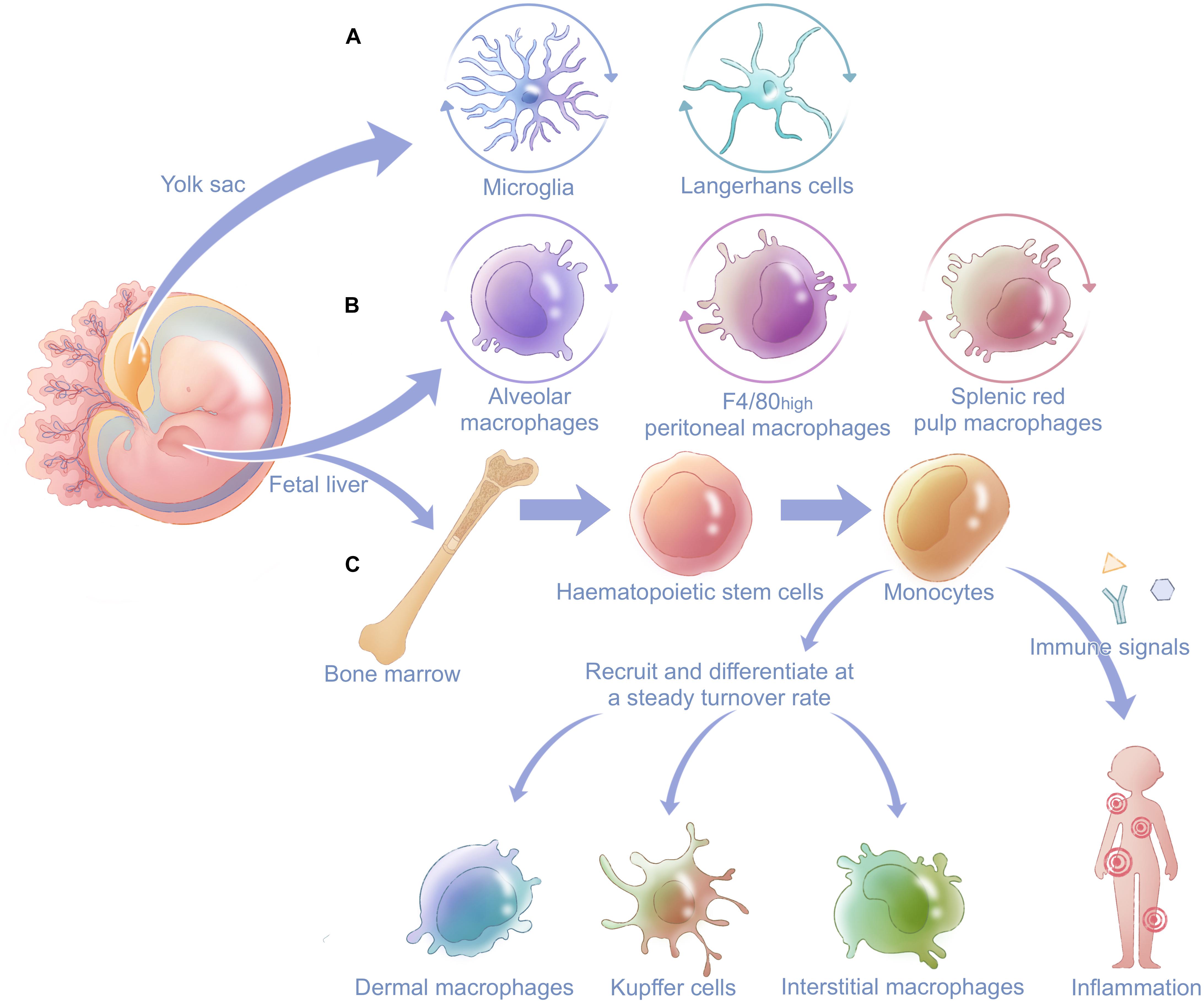
Figure 1. General derivation and distribution of macrophages in the body. Macrophages are derived from two main fetal organs: the fetal liver and yolk sac. (A) Microglia in the central neural system and Langerhans cells in epidermal tissue have prenatal origins from the yolk sac and renew themselves locally after being seeded in different systems. (B) Alveolar macrophages, F4/80high peritoneal macrophages, and splenic red pulp macrophages originate from the fetal liver and also have postnatal self-renewal capacity. Fetal liver gives rise to hematopoietic stem cells in bone marrow. (C) Hematopoietic stem cells can develop into monocytes and finally differentiate into other tissue-resident macrophages such as dermal macrophages, Kupffer cells, and interstitial macrophages after birth. When stimulated by immune signals, monocytes can be recruited and differentiate into macrophages at inflammatory sites and innate immune.
Macrophage Polarization and Function
Macrophages have two main activation states: M1 and M2 polarization (Figure 2; Locati et al., 2020). M1-polarized macrophages are activated by interferon-γ (IFN-γ), lipopolysaccharide (LPS), granulocyte-macrophage colony-stimulating factor, or tumor necrosis factor (TNF; Biswas and Mantovani, 2010). The toll-like receptor (TLR) for LPS and receptors for cytokines such as IFN-γ are thus activated and induce subsequent expression of transcription factors nuclear factor kappa-B (NF-κB), interferon regulatory factor 3 (IRF-3), and signal transducer and activator of transcription 1 (STAT1; Sengupta et al., 1996; Sica and Bronte, 2007). These transcription factors are transported into the nucleus, where they upregulate genes related to M1-polarized macrophages (Taniguchi et al., 2001; Gao et al., 2018). M1-polarized macrophages exhibit enhanced phagocytosis mediated by increased secretion of pro-inflammatory cytokines and chemotactic factors; thus, they facilitate the removal of non-self components (Sica and Mantovani, 2012) and play important parts in Th1-mediated immune responses (Biswas and Mantovani, 2010). M2-polarized macrophages are stimulated by interleukin-4 (IL-4) or interleukin-10 (IL-10) signaling, which induces signal transducer and activator of transcription 6 (STAT6), interferon regulatory factor 4 (IRF-4), and peroxisome proliferator-activated receptor γ (PPARγ; Odegaard et al., 2007; Czimmerer et al., 2018). M2-polarized macrophages can be further divided into M2a, M2b, and M2c subgroups. The M2a and M2b phenotypes are activated by IL-4 and promote an immune response mediated by Th2 (Stein et al., 1992). By contrast, M2c inhibits the immune response and favors tissue remodeling after activation by IL-10 or glucocorticoids (Curtale et al., 2013, 2017).
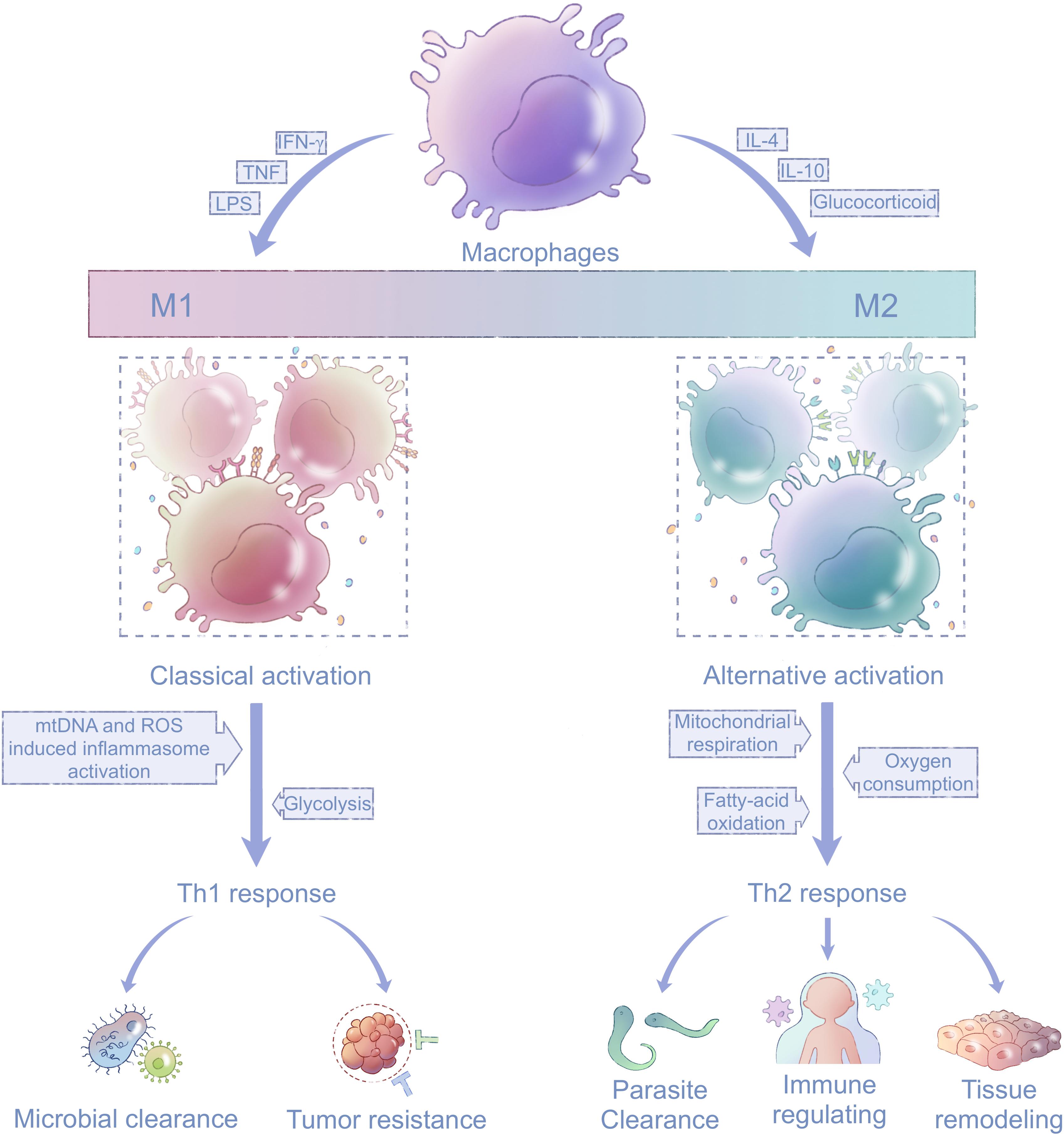
Figure 2. Mitochondria in macrophage polarization. Macrophages are activated and polarized in response to different stimuli, including TNF, IFN-γ, LPS, IL-4, IL-10, and glucocorticoids. The states of macrophages fall between two extremes, M1 and M2 phenotypes, which participate in the Th1 and Th2 immune responses, respectively. Energy metabolism in M1-polarized macrophages shifts to glycolysis compared with their precursors, and M1-polarized macrophages are activated by mtDNA and mitochondria-produced ROS (left). Energy metabolism in M2-polarized macrophages mainly depends on mitochondrial respiration fueled by oxygen and fatty acids (right). TNF, tumor necrosis factor; IFN-γ, interferon-γ; LPS, lipopolysaccharide; IL-4, interleukin-4; IL-10, interleukin-10; ROS, reactive oxidative species; and mtDNA, mitochondrial DNA.
Mitochondria in Macrophage Polarization and Signal Transduction
Macrophages undergo mitochondria-related metabolic reprogramming during activation (Haschemi et al., 2012; Huang et al., 2014; Jin et al., 2014). M1-polarized macrophages depend mainly on glycolysis as the first line of defense, whereas M2-polarized macrophages largely rely on oxygen consumption by mitochondrial respiration for their long-term functions (Haschemi et al., 2012; Galvan-Pena and O’Neill, 2014). Increased glucose utilization in IL-4-stimulated macrophages requires activation of the mechanistic/mammalian target of rapamycin complex 2 pathway, which operates in parallel with the IL-4Rα-STAT6 pathway to facilitate M2 activation via induction of IRF-4 (Huang et al., 2016). PPARγ-coactivator-1b (PGC-1b) induces mitochondrial biogenesis and is also indispensable for M2 polarization (Vats et al., 2006), and cell-autonomous lysosomal-based lipolysis and fatty-acid oxidation fuel the mitochondrial metabolism to maintain the M2 phenotype (Huang et al., 2014).
In addition to metabolism alteration, mitochondrial damage-associated molecular patterns (DAMPs) such as mtDNA and byproducts of mitochondrial respiration such as ROS have important roles in the initiation and transduction of signals in the immune response, especially in M1 activation (Nakahira et al., 2011; Zhou et al., 2011). The synthesis of mtDNA, which is induced after the engagement of TLRs, is crucial for NACHT and leucine-rich repeat protein 3 (NLRP3) signaling in M1-polarized macrophages; dysregulated NLRP3 inflammasome activity results in uncontrolled inflammation (Zhong et al., 2018). ROS are essential bactericidal components generated primarily via the phagosomal NADPH oxidase machinery by phagocytes including macrophages (Lambeth, 2004; West et al., 2011). ROS promote production of pro-inflammatory cytokines in response to LPS via decreasing the dephosphorylation of mitogen-activated protein kinases (MAPKs) including c-Jun N-terminal kinase, extracellular signal-regulated kinase, and p38 MAPK phosphorylation (Bulua et al., 2011). ROS also contribute to NLRP3 inflammasome activation (Sorbara and Girardin, 2011). Moreover, mitochondrial ROS are critical to the differentiation of M2-polarized macrophages (Angajala et al., 1605). In a study of TAMs, which are similar to M2-polarized macrophages in terms of their pro-angiogenic and immune-suppressive functions, inhibition of superoxide production was shown to specifically block the differentiation of M2 macrophages (Zhang et al., 2013). However, the inhibitory effect of ROS elimination on macrophage differentiation was overcome when macrophages were polarized to the M1 phenotype (Zhang et al., 2013).
Cell–Cell Communications Between Macrophages and Surrounding Cells
Cell–cell communications occur frequently between macrophages and adjacent tissue cells. Macrophages integrate into host tissues; this entails their specialization in response to the local environment (Varol et al., 2015). Local cells imprint the specific functions of macrophages (Varol et al., 2015), as exemplified by AMs, osteoclasts, and microglia. Alveolar epithelial cells are a major source of colony-stimulating factor 2 (CSF-2), which is necessary for the differentiation of AMs (Guilliams et al., 2013). The differentiation and function of osteoclasts are regulated by the balance of receptor activator of NF-κB ligand (RANKL) and osteoprotegerin produced by osteoblasts (Takayanagi et al., 2002; Boyle et al., 2003; Ikebuchi et al., 2018). Molecules of neuronal origin control microglial motility and functions via chemotaxis, neurotransmitters, and purinergic and adenosine signaling pathways (Crain et al., 2009; Mead et al., 2012; Limatola and Ransohoff, 2014).
Cell–cell communications between macrophages and tissue cells also facilitate tissue-specific functions of macrophages and contribute to the development and specific functions of resident tissues (Figure 3). The most direct type of cell–cell communication is based on the prototypical macrophage function, phagocytosis (Nagata, 2018). For example, macrophages in spleen red pulp phagocytose red blood cells (RBCs) to facilitate iron circulation (Rodrigues et al., 2017). Slight modifications of the cell membranes of RBCs, such as those associated with RBC senescence or damage, are sensed by macrophages, which phagocytose such RBCs and return iron to erythroid progenitors (Korolnek and Hamza, 2015). Microglia (macrophages in the central neural system) contribute to neural synapse maturation and brain development by synaptic pruning (Paolicelli et al., 2011).
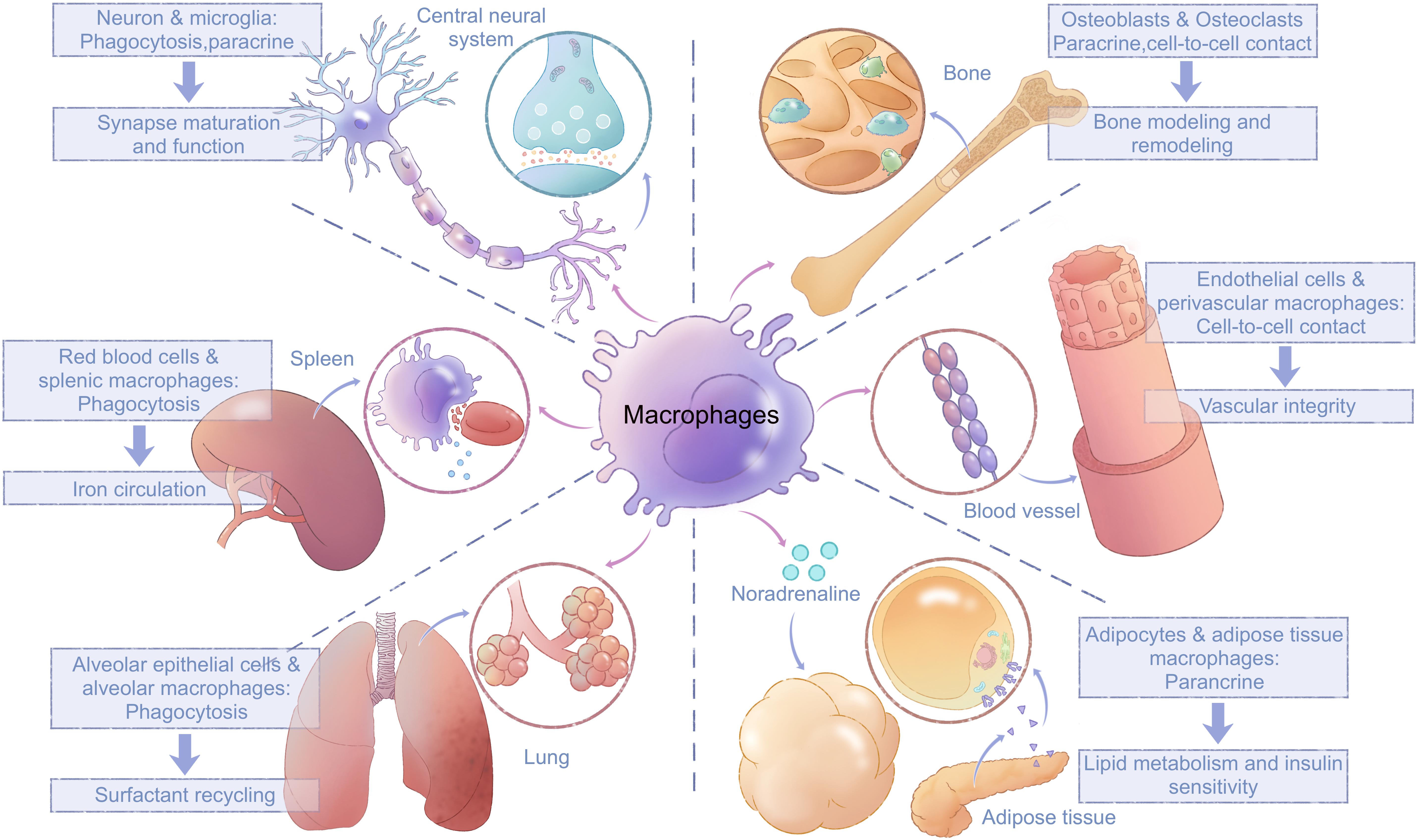
Figure 3. Interactions between macrophages and tissue cells. Macrophages interact closely with surrounding tissue cells through paracrine mechanisms, cell-to-cell contacts, and direct phagocytosis; these interactions are vital for tissue development and their normal function. Microglia trim synapses and release signal molecules to promote neural synapse maturation and function. Splenic macrophages phagocytose red blood cells and facilitate iron circulation. Alveolar macrophages phagocytose surfactants produced by alveolar epithelial cells. Bone-resident osteoclasts interact with osteoblasts through both cell-to-cell contacts and paracrine communication. Perivascular macrophages maintain vascular integrity by attenuating phosphorylation of VE-cadherin in endothelial cells via cell-to-cell contact. Macrophages in white adipose tissue regulate lipid metabolism and insulin sensitivity in adipocytes via paracrine effects of noradrenalin. VE, vascular endothelial.
In addition to phagocytosis, other forms of cell–cell communication exist between macrophages and their surrounding cells. Microglia can also release signaling molecules including brain-derived neurotrophic factor and microvesicles (MVs) containing cytosolic proteins, lipids, and microRNAs to regulate synaptic activity (Parkhurst et al., 2013; Maas et al., 2017). Macrophages in adipose tissues regulate lipid metabolism and insulin sensitivity in adipocytes via paracrine effects of noradrenalin (Pirzgalska et al., 2017; Flaherty et al., 2019). Perivascular macrophages in capillaries attenuate vascular endothelial-cadherin phosphorylation in endothelial cells to limit blood vessel permeability and maintain vascular integrity (He et al., 2016; Lapenna et al., 2018). BMMs develop into osteoclasts, where they coordinate with osteoblasts for bone modeling and remodeling through both cell contacts and ligand–receptor interactions (Boyle et al., 2003). The best-studied such mutual interactions are RANKL signaling in osteoclasts and its reverse signaling in osteoblasts, which maintains the balance between osteoclast maturation and function (Takayanagi et al., 2002; Ikebuchi et al., 2018). After osteoclast maturation, the proton pump in osteoclasts acidifies the resorption organelle and releases lytic enzymes to realize bone resorption (Boyle et al., 2003). In general, macrophages and tissue cells interact both directly and indirectly to maintain physiological functions of tissues.
Macrophage-Related Mitochondrial Transfer
The involvement of macrophages in intracellular mitochondrial transfers is emerging as a critical phenomenon in various tissues. Macrophages often function as recipients that digest depolarized or fragmented mitochondria, thereby favoring the survival and maintaining the functions of surrounding cells. Transfers of healthy mitochondria also contribute to the polarization and homeostasis of both recipient and donor macrophages. In general, macrophage-related mitochondrial transfers have mutual effects on both macrophages and their surrounding cells (Figure 4A).
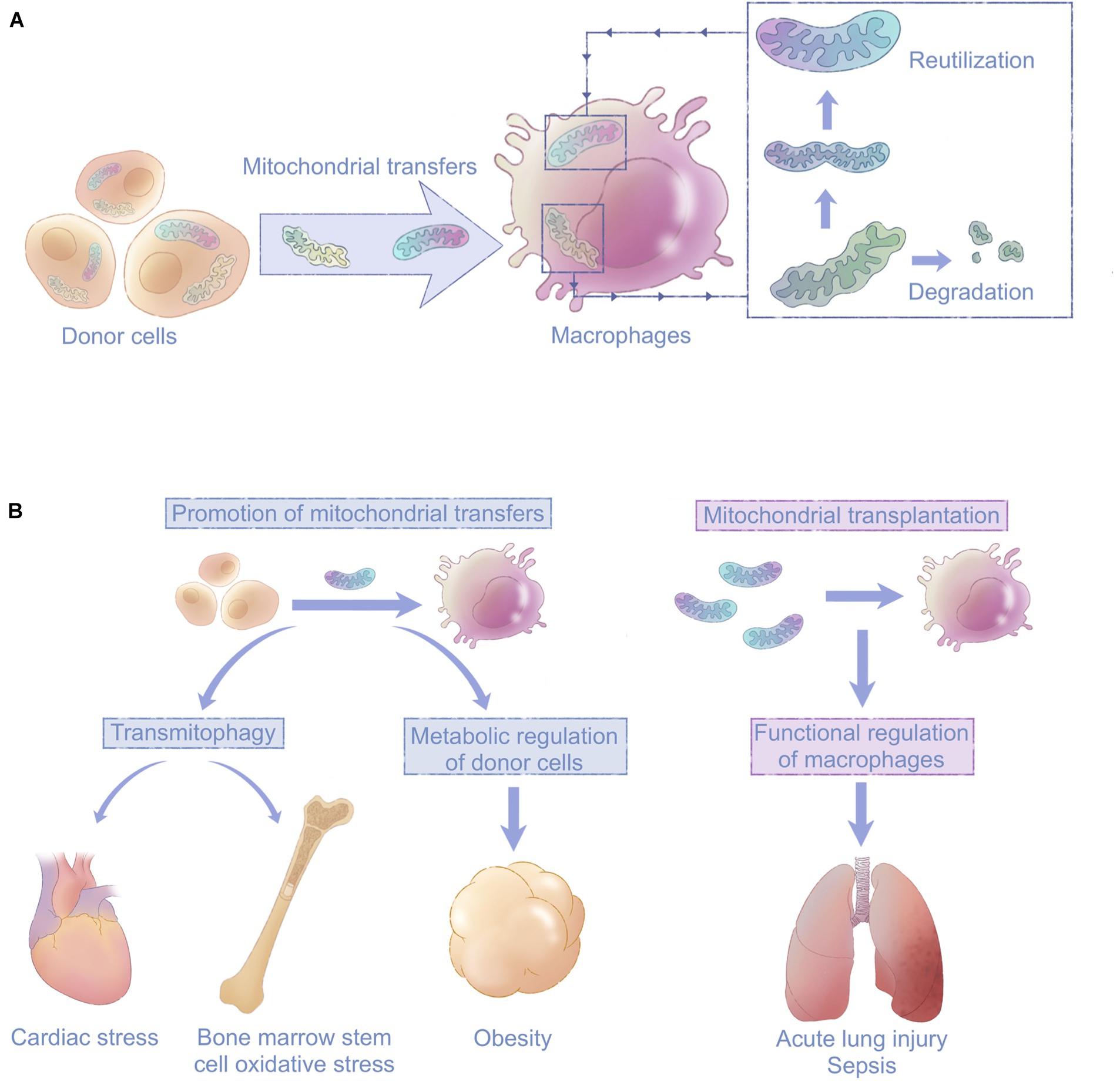
Figure 4. Macrophages are emerging as key players in mitochondrial transfers with clinical implications. (A) Macrophages mediate intercellular mitochondrial transfers of either healthy or damaged mitochondria from surrounding tissue cells. Transferred healthy mitochondria improve the function of recipient macrophages. Damaged mitochondria are degraded via mitophagy to ease donor cells’ stress or reutilized in macrophages. (B) Mitochondrial transfers involving macrophages can be targeted for therapeutic purposes through promotion of mitochondrial transfers or mitochondrial transplantation; this is a promising approach for diseases including cardiac stress, obesity, acute lung injury, and sepsis.
Macrophages Mediate Transmitophagy
Damaged mitochondria are generally degraded by mitophagy, a cell-autonomous activity (Davis and Marsh-Armstrong, 2014). In the case of ganglion cells and astrocytes, transcellular mitophagy (hereafter called transmitophagy) has been proposed as a means of extracellular degradation that lightens the burden of stressed cells (Davis et al., 2014). Recently, different groups of macrophages have been reported as potential handlers of damaged mitochondria during transmitophagy. Accumulating evidence also indicates that the mitophagy level inside macrophages influences macrophage polarization and function (Table 3; Kim et al., 2016; Larson-Casey et al., 2016; Zhao et al., 2017; Bhatia et al., 2019; Patoli et al., 2020; Zhang et al., 2020). Thus, transmitophagy may exert influences on both donor cells and recipient macrophages to regulate tissue homeostasis.
Cardiomyocytes Transfer Mitochondria to Macrophages
Cardiomyocytes (CMs) are highly specialized smooth muscle cells with extremely long lifespan and low turnover rate, which rely on a large pool of mitochondria to meet their intensive energy demands (Bergmann et al., 2009). Accumulation of damaged mitochondria leads to cardiac hypertrophy and heart failure (Bertero and Maack, 2018). Thus, elimination of dysfunctional mitochondria is vital for CM survival and function. Although most studies of CMs have focused on their intracellular clearance of fragmented mitochondria (Chen and Dorn, 2013; Gong et al., 2015; Tong et al., 2019), Nicolas-Avila et al. (2020) recently proposed intercellular mitochondrial transfer between CMs and surrounding cardiac-resident macrophages (cMACs) as an extracellular route by which CMs dispose of dysfunctional mitochondria.
In this study, altered mitochondrial morphology and reduced cristae density were observed in cMAC-deficient mice, suggesting impaired cardiac mitochondria homeostasis. Moreover, cMAC-deficient mice showed impaired systolic function, which could be restored by supplementation with cMACs; this finding emphasized the crucial role of cMACs in ensuring the mitochondria health of CMs (Nicolas-Avila et al., 2020). Further investigation revealed that cMACs took up mitochondria with compromised membrane integrity from CMs via exophers under physiological conditions (Nicolas-Avila et al., 2020). Under pathological conditions, as in an isoproterenol-induced mouse model of cardiac ischemia, dysfunctional mitochondrial transfer from CMs to cMACs was significantly increased. These results imply that the outsourcing of dysfunctional mitochondria from CMs to cMACs might have a protective function against cardiac stress (Nicolas-Avila et al., 2020). Nevertheless, as mitophagy and mitochondria influence the activation state of cMACs, how cMACs endure damaged mitochondria from CMs under stress remains unknown, as does how stress itself influences ability of cMACs to deal with dysfunctional mitochondria. Moreover, considering the heterogeneity of macrophages, single-cell sequencing may help to identify distinct subgroups responsible for transmitophagy.
Bone Marrow Stem Cells Transfer Mitochondria to Macrophages
Bone marrow mesenchymal stem cells (BMSCs) naturally reside in a hypoxic stem cell niche and regulate self-renewal and mobilization of hematopoietic stem cells via crosstalk with adjacent macrophages (Mendez-Ferrer et al., 2010; Chow et al., 2013; Morrison and Scadden, 2014). In an ex vivo co-culture system, macrophages were shown to receive depolarized mitochondria from BMSC exosomes, thus enhancing the ability of BMSCs to deal with oxidative stress by improving mitochondrial bioenergetics. Depolarized mitochondria are first loaded into LC3-positive vesicles and then migrate toward the cell periphery, where they are incorporated into outward budding blebs and subsequently taken up by macrophages (Phinney et al., 2015). This phenomenon may also occur in vivo between local macrophages and BMSCs administered to patients. BMSC-to-macrophage mitochondrial transfers represent a possible mechanism by which macrophages in stem cell niches protect BMSCs under stress, yet direct in vivo evidence for this mechanism is still lacking (Phinney et al., 2015).
Metabolic Regulation of Donor Cells
As mitochondria are centers of energy metabolism and coordinate aerobic respiration fueled by either glucose or lipids (Schon et al., 2012), mitochondrial transfers involving macrophages are not only used for transmitophagy but also meet metabolic ends, as exemplified by adipocytes in white adipose tissue (WAT) and adipose tissue macrophages (ATMs). In a recent study focusing on mitochondrial transfers between adipocytes in WAT and ATMs, nearly half of the ATMs in mice internalized mitochondria from neighboring adipocytes under physiological conditions (Brestoff et al., 2020). The significance of these mitochondrial transfers was confirmed in a mouse model of obesity fed a high-fat diet, where adipocyte-to-macrophage mitochondrial transfers were drastically decreased (Brestoff et al., 2020).
Adipose tissue macrophages regulate the glucose utilization and energy expenditure of adipocytes (Pirzgalska et al., 2017; Flaherty et al., 2019), and obesity induces a phenotype switch in ATMs (Lumeng et al., 2007). In turn, changes in ATM phenotypes contribute to WAT inflammation and obesity-induced insulin resistance (Han et al., 2013; Kratz et al., 2014). Indeed, decreased adipocyte-to-macrophage mitochondrial transfers are related to an obesity-induced inflammatory state in WAT. First, the pro-inflammatory environment induced by IFN-γ, LPS activation, and M1 polarization contributes to a macrophage-intrinsic impairment in mitochondrial uptake (Brestoff et al., 2020). Second, the uptake ability of adipose-resident macrophages depends on the heparan sulfate biosynthesis pathway, which has been reported to show anti-inflammatory effects (Brestoff et al., 2020). Inhibition of heparan sulfate biosynthesis leads to aberrant mitochondrial uptake, accompanied by decreased energy expenditure and fat accumulation in adipose tissue (Brestoff et al., 2020).
Intriguingly, gene enrichment analysis of mitochondria-recipient macrophages has defined a transcriptionally distinct subgroup of macrophages that are capable of mitochondrial internalization, with traits resembling those of anti-inflammatory macrophages (Brestoff et al., 2020). More investigations are needed to trace the derivation and characteristics of the transcriptionally distinct macrophage subgroups, as well as their response to the metabolic state in obesity, in order to understand the complexity of ATMs. Moreover, as evidence suggests that ATMs are highly plastic according to their surrounding environment, and obesity is strongly associated with the number, derivation, and functional changes of ATMs (Lumeng et al., 2008; Xu et al., 2013), regulation of ATM subgroups toward mitochondrial uptake subgroups is a promising therapeutic approach for obesity and related metabolic syndromes.
Functional Regulation of Macrophages by Mitochondrial Transfers
In addition to the favorable effects on surrounding cells of mitochondrial transfers from macrophages, macrophages themselves are influenced by transferred mitochondria. BMSCs modulate the function of AMs by transferring mitochondria via both contact-dependent and paracrine routes; this modulation can be exploited as a mechanism for BMSC therapy for acute lung injury (Jackson et al., 2016; Morrison et al., 2017). In Escherichia coli-treated co-culture system, MSCs were shown to transfer mitochondria to human macrophages via tunneling nanotubes (TNTs); this enhanced their phagocytic capacity and facilitated the antimicrobial effects of the BMSCs (Jackson et al., 2016). In a later study using a transwell system for macrophage and BMSC co-culture, BMSCs significantly increased the proportion of M2-polarized and phagocytic macrophages via transferring BMSC-derived extracellular vesicles (EVs) containing healthy mitochondria (Morrison et al., 2017).
In murine models, adoptive transfer of murine AMs treated with MSC-derived EVs protects mice from LPS-induced lung injury by alleviating the inflammatory cell recruitment, suggesting that anti-inflammatory M2 polarization occurs when AMs receive healthy mitochondria (Morrison et al., 2017). Importantly, BMSC-conditioned medium taken from rhodamine-6G-pretreated MSCs with dysfunctional mitochondria could not cause such changes, indicating the presence of functional mitochondria rather than mitochondrial components that induce such activation changes (Morrison et al., 2017).
Instead of mitochondria-derived immune signals, an increased energy supply from functional mitochondria is responsible for enhanced phagocytosis in AMs; the ATPase inhibitor oligomycin completely reversed the effect of MSC-conditioned medium on BMM phagocytosis (Morrison et al., 2017). However, AMs are a heterogeneous group of cells, especially in acute lung injury, which is characterized by acute immune response and subsequent tissue repair (Duan et al., 2012; Short et al., 2014). Whether self-replicative tissue-resident AMs or monocyte-derived AMs recruited during the acute immune response enable mitochondrial recipients to undergo M2 polarization and change of function remains unclear. In addition, mitochondrial transfers enhance the function of recipient macrophages by improving their mitochondrial bioenergetics (Phinney et al., 2015). Unhealthy mitochondria extruded by BMSCs still exhibit residual membrane potential, which provides evidence for their mitochondrial membrane integrity and fusion ability (Phinney et al., 2015). Depolarized but not totally fragmented mitochondria undergo mitochondrial fusion in macrophages for reutilization (Phinney et al., 2015). In addition to mitochondria, these vesicles contain miR451, miR1202, miR630, and miR638, which represses TLR expression, thereby tolerizing macrophages to mitochondrial-transfer-induced inflammation caused by excessive mtDNA (Phinney et al., 2015).
How Macrophages Mediate Mitochondrial Transfers
Macrophages mediate mitochondrial transfers through various mechanisms in different systems. Phagocytosis, the most typical macrophage function, contributes substantially to the mediation of mitochondrial transfers by macrophages (Phinney et al., 2015; Nicolas-Avila et al., 2020). In an ex vivo oxidative stress model of BMSCs, BMMs nibbled the surfaces of human BMSCs, enabling uptake of mitochondria-containing phagosomes budding from the plasma membrane (Phinney et al., 2015). Pre-incubation with dextran sulfate, an inhibitor of phagocytosis, significantly reduced uptake of MVs from BMSCs by BMMs (Phinney et al., 2015). Isolated cMACs feature large phagolysosome-like vacuoles and have been shown to actively phagocytose materials from CMs; these materials were later proven to be mitochondria-contained exophers (Nicolas-Avila et al., 2020). The engulfed exophers become Lamp1+ phagolysosomes in cMACs (Nicolas-Avila et al., 2020). Western blotting analyses revealed inflammasome activation in the hearts of cMAC-depleted mice, possibly owing to the presence of free mitochondria and mtDNA caused by abrogated mitochondria transfers in the absence of cMACs (Oka et al., 2012; Nicolas-Avila et al., 2020). Inflammasome activation in turn caused autophagic arrest and impaired exopher production in CMs (Nicolas-Avila et al., 2020). Therefore, cMACs prevent inflammasome activation and protect autophagy flux in CMs to support exopher formation for mitochondrial transfers (Nicolas-Avila et al., 2020).
Whether macrophages obtain mitochondria through cell–cell contact, such as through TNTs, in addition to phagocytosis was also investigated in the case of BMSC antimicrobial therapy (Jackson et al., 2016). Depletion of AMs abrogates the antimicrobial effects of BMSCs (Jackson et al., 2016), and TNTs containing mitochondria are extended from BMSCs to AMs (Jackson et al., 2016). It seems that BMSCs play an active part in this case. However, mitochondrial transfers were reduced but still evident after blockage of TNT formation in BMSCs by cytochalasin B (Jackson et al., 2016); in a later study, this was attributed to AMs also acquiring BMSC mitochondria through EVs in a manner independent of TNT formation by BMSCs (Islam et al., 2012). Therefore, although TNTs formed by BMSCs are partially responsible for the acquisition of mitochondria by AMs, the AMs also acquire mitochondria from BMSCs via EVs (Islam et al., 2012; Jackson et al., 2016).
Furthermore, several critical molecules that may contribute to macrophage-mediated mitochondrial transfer have been identified. For example, AMs selectively uptake mitochondria containing EVs from BMSCs by recognizing CD44 on the surfaces of EVs (Islam et al., 2012). Anti-CD44 antibody partially abrogated the effects of BMSC-conditioned medium on macrophages, whereas antibodies administered to AMs in the absence of MSC-conditioned medium had no influence (Islam et al., 2012). In addition, EXT1, an important gene in the heparan sulfate biosynthesis pathway, has been reported to be indispensable for ATMs to obtain mitochondria from adipocytes (Brestoff et al., 2020). Conditional deletion of EXT1 in myeloid cells reduces heparan sulfate levels in ATMs, impairs mitochondria transfer, and promotes fat mass accumulation (Brestoff et al., 2020). However, the direct association between the heparan sulfate biosynthesis pathway and the function of macrophages remains unclear (Brestoff et al., 2020). More studies are needed to decipher the mechanisms underlying macrophage-mediated mitochondrial transfers.
Targeting Mitochondria in Macrophages for Therapeutic Purposes
Great progress has been made in recent years in modulating the tissue environment via macrophages, particularly in the field of antitumor immunotherapy (Tacke, 2017; Xia et al., 2020). Most of those therapies target molecular pathways related to the recruitment and phenotypes of macrophages (Pathria et al., 2019), for instance, the CSF-1 receptor (Cassier et al., 2015; Cannarile et al., 2017) and agonistic CD40 therapy (Wiehagen et al., 2017). Targeting energy metabolism and mitochondria-related signal transduction in macrophages shows good prospects for developing efficient interventions.
Targeting glycolysis and mitochondrial ROS have been reported as effective therapeutic strategies for controlling inflammation mediated by M1-polarized macrophages. Dimethyl fumarate, a derivative of Krebs cycle intermediate fumarate, downregulates aerobic glycolysis in activated peritoneal macrophages to inhibit inflammation; thus, it has a critical role in the treatment of multiple sclerosis (Linker and Haghikia, 2016). Similarly, itaconate, an endogenous metabolite, is required for activation of the anti-inflammatory transcription factor Nrf2 in LPS-activated mouse and human macrophages (Mills et al., 2018). In addition, 4-octyl itaconate, a cell-permeable itaconate derivative, protects against LPS-induced cytokine production and inflammation in vivo (Mills et al., 2018). On the other hand, diphenyliodonium, a global and mitochondrial ROS scavenger, was shown to impair LPS-induced NLRP3 expression, thereby inhibiting IL-1β and IL-18 production in macrophages (Sazanov, 2007). Other promising candidates include metformin and rotenone, which regulate glycolysis and ROS via targeting pyruvate kinase and could also inhibit inflammation induced by M1-polarized macrophages (Palsson-McDermott et al., 2015; Mills and O’Neill, 2016; Peruzzotti-Jametti and Pluchino, 2018). Activation of M2-polarized macrophages can be regulated by OXPHOS. Acute inhibition of the polyamine-eIF5A-hypusine axis by Eif5a small interfering RNA (siRNA), Dhps-siRNA, and deoxyhypusine synthase inhibitor GC7 blunts OXPHOS-dependent M2 activation while leaving aerobic glycolysis-dependent M1 activation intact (Puleston et al., 2019). Genetic and GC7-driven inhibition of eIF5AH silenced mitochondria has been reported to prevent anoxic death of kidney cells and to improve outcomes of kidney transplants (Melis et al., 2017).
In addition to conventional M1/M2 polarization, high levels of fatty acid oxidation in TAMs promote mitochondrial OXPHOS, ROS production, and JAK1 phosphorylation, leading to STAT6 activation and transcription of genes that regulate TAM generation and function (Su et al., 2020). Given the importance of fatty acid oxidation in TAMs, interfering in lipid metabolism could be a promising therapeutic approach for cancer (Su et al., 2020). In atherosclerosis, Dicer plays a protective part in coordinately regulating the inflammatory response in lesional macrophages through enhancing fatty-acid-fueled mitochondrial respiration. Promoting Dicer/miR-10a-dependent metabolic reprogramming in macrophages has potential therapeutic applications for the prevention of atherosclerosis (Wei et al., 2018).
Studies of mitochondrial transfer provide new strategies for modification of macrophages. In the cases of the myocardium and adipose, where spontaneous cell-to-macrophage mitochondrial transfers occur under physiological conditions (Brestoff et al., 2020; Nicolas-Avila et al., 2020), impaired ability of macrophages to take up mitochondria from surrounding cells could contribute to pathogenesis. Enhancing macrophage-related mitochondrial transfer is thus a promising therapeutic strategy. In acute lung injury and sepsis (Islam et al., 2012; Jackson et al., 2016), mitochondrial transplantation into macrophages could promote macrophage phagocytosis and train the immune system (Figure 4B; Yamada et al., 2020). The development of new treatments calls for more studies on the basic mechanisms underlying mitochondrial transfers involving macrophages. Key molecules responsible for mitochondrial transfer signaling in macrophages and mitochondrial transfer routes should be identified.
Conclusion and Perspectives
Mitochondrial transfers from donor cells promote the survival of recipient cells by enabling the recovery of mitochondrial function, as exemplified by neurons and osteocytes with low self-renewal rates (Hayakawa et al., 2016; Gao et al., 2019), chemoresistant cancer cells (Pasquier et al., 2013; Tan et al., 2015; Moschoi et al., 2016), and therapeutic use of stem cells (Zhang Y. L. et al., 2016; Yao et al., 2018). Recent studies have reported that macrophage-related mitochondrial transfers have important roles in processing unhealthy mitochondria as well as utilizing healthy mitochondria. Notably, mitochondria transferred from tissue cells to macrophages could also function as important messengers. In acute inflammation, activated monocytes give out mitochondria-related DAMPs including mitochondrial membrane components and mitochondrial 16S ribosomal RNA to activate an inflammatory response in endothelial cells (Pober and Sessa, 2007; Ait-Oufella et al., 2010; Puhm et al., 2019). Macrophages, which are important immune cells, can also be activated by mitochondria-related DAMPs (Nakahira et al., 2011; Zhou et al., 2011); therefore, mitochondrial transfers could function as immune signals. Besides, since macrophages in acute inflammatory responses are derived from recruited monocytes (Geissmann et al., 2010), macrophages might play a similar role as monocytes to give out mitochondrial components as immune signals.
Unhealthy mitochondria received by macrophages usually undergo either reutilization or degradation (Phinney et al., 2015; Brestoff et al., 2020; Nicolas-Avila et al., 2020). Reutilization of unhealthy mitochondria in macrophages resident in the stem cell niche is achieved by mitochondrial fusion to enhance OXPHOS in these macrophages (Phinney et al., 2015). Alternatively, degradation of unhealthy mitochondria can be achieved by transmitophagy in macrophages (Phinney et al., 2015; Brestoff et al., 2020; Nicolas-Avila et al., 2020). In addition, unhealthy mitochondria received by macrophages may be extruded by migrasomes, which are newly identified vesicular structures that discharge cellular contents during migration (Ma et al., 2015). In an ex vivo study, BMMs exposed to mild mitochondria stress induced by carbonyl cyanide 3-chlorophenylhydrazone were observed to leave behind migrasomes containing damaged mitochondria (Jiao et al., 2021), indicating that BMMs may be donor cells for mitochondrial transfers (Jiao et al., 2021). Further studies are required to determine whether migrasomes containing mitochondria are received by surrounding cells as a route of mitochondrial transfer.
Macrophages are widely distributed, enabling them to maintain tissue homeostasis, and related to various diseases (Wynn et al., 2013). However, studies to date have only described limited situations in which macrophage-related transfers exert their effects. This is the beginning of a conversation, not the final word. Targeting mitochondrial transfers in macrophages is a strategy that shows great potential in a range of fields including cancer and infectious diseases (Na et al., 2018). Therefore, future studies should focus on the development of techniques to regulate macrophage-related mitochondrial transfers for therapeutic purposes (Figure 4B).
Author Contributions
YP and JG provided the essential ideas for this work and performed the literature search and drafted the article. CZ and JG critically revised the manuscript. All authors contributed to the article and approved the submitted version.
Funding
This work was supported by the National Natural Science Foundation of China (No. 82002339).
Conflict of Interest
The authors declare that the research was conducted in the absence of any commercial or financial relationships that could be construed as a potential conflict of interest.
Publisher’s Note
All claims expressed in this article are solely those of the authors and do not necessarily represent those of their affiliated organizations, or those of the publisher, the editors and the reviewers. Any product that may be evaluated in this article, or claim that may be made by its manufacturer, is not guaranteed or endorsed by the publisher.
Abbreviations
AM, alveolar macrophage; ATM, adipose tissue macrophage; BMM, bone-marrow-derived macrophages; BNIP-3, Bcl-2/adenovirus E1B 19 kDa interacting with protein-3; BMSC, bone marrow mesenchymal stem cell; CCCP, carbonyl cyanide 3-chlorophenylhydrazone; CM, cardiomyocyte; cMAC, cardiac-resident macrophage; DAMP, damage-associated molecular pattern; E. coli, Escherichia coli; FADH2, dihydroflavine-adenine dinucleotide; GM-CSF, granulocyte-macrophage colony-stimulating factor; IFN- γ, interferon- γ; IL-4, interleukin-4; IL-10, interleukin-10; LPS, lipopolysaccharide; mtDNA, mitochondrial DNA; MV, microvesicle; NADH, nicotinamide adenine dinucleotide; OXPHOS, oxidative phosphorylation; RM, renal macrophages; ROS, reactive oxidative species; SM, splenic macrophages; TAM, tumor associated macrophages; TLR, toll-like receptor; TNF, tumor necrosis factor; TNT, tunneling nanotube; WAT, white adipose tissue.
References
Ait-Oufella, H., Maury, E., Lehoux, S., Guidet, B., and Offenstadt, G. (2010). The endothelium: physiological functions and role in microcirculatory failure during severe sepsis. Intensive Care Med. 36, 1286–1298. doi: 10.1007/s00134-010-1893-6
Anderson, S., Bankier, A. T., Barrell, B. G., de Bruijn, M. H., Coulson, A. R., Drouin, J., et al. (1981). Sequence and organization of the human mitochondrial genome. Nature 290, 457–465.
Angajala, A., Lim, S. B., Phillips, J. B., Kim, J. H., Yates, C., You, Z. B., et al. (1605). Diverse roles of mitochondria in immune responses: novel insights into immuno-metabolism. Front. Immunol. 2018:9. doi: 10.3389/fimmu.2018.01605
Bergmann, O., Bhardwaj, R. D., Bernard, S., Zdunek, S., Barnabe-Heider, F., Walsh, S., et al. (2009). Evidence for cardiomyocyte renewal in humans. Science 324, 98–102. doi: 10.1126/science.1164680
Bertero, E., and Maack, C. (2018). Metabolic remodelling in heart failure. Nat. Rev. Cardiol. 15, 457–470. doi: 10.1038/s41569-018-0044-6
Bhatia, D., Chung, K. P., Nakahira, K., Patino, E., Rice, M. C., Torres, L. K., et al. (2019). Mitophagy-dependent macrophage reprogramming protects against kidney fibrosis. JCI Insight 4:e132826.
Biswas, S. K., and Mantovani, A. (2010). Macrophage plasticity and interaction with lymphocyte subsets: cancer as a paradigm. Nat. Immunol. 11, 889–896. doi: 10.1038/ni.1937
Bock, F. J., and Tait, S. W. G. (2020). Mitochondria as multifaceted regulators of cell death. Nat. Rev. Mol. Cell. Bio. 21, 85–100. doi: 10.1038/s41580-019-0173-8
Boyle, W. J., Simonet, W. S., and Lacey, D. L. (2003). Osteoclast differentiation and activation. Nature 423, 337–342. doi: 10.1038/nature01658
Brestoff, J. R., Wilen, C. B., Moley, J. R., Li, Y., Zou, W., Malvin, N. P., et al. (2020). Intercellular mitochondria transfer to macrophages regulates white adipose tissue homeostasis and is impaired in obesity. Cell Metab. 33, 270–282.e8.
Bulua, A. C., Simon, A., Maddipati, R., Pelletier, M., Park, H., Kim, K. Y., et al. (2011). Mitochondrial reactive oxygen species promote production of proinflammatory cytokines and are elevated in TNFR1-associated periodic syndrome (TRAPS). J. Exp. Med. 208, 519–533. doi: 10.1084/jem.20102049
Byrne, A. J., Maher, T. M., and Lloyd, C. M. (2016). Pulmonary macrophages: a new therapeutic pathway in fibrosing lung disease? Trends Mol. Med. 22, 303–316. doi: 10.1016/j.molmed.2016.02.004
Cannarile, M. A., Weisser, M., Jacob, W., Jegg, A. M., Ries, C. H., and Ruttinger, D. (2017). Colony-stimulating factor 1 receptor (CSF1R) inhibitors in cancer therapy. J. Immunother. Cancer 5:53.
Cassetta, L., and Pollard, J. W. (2018). Targeting macrophages: therapeutic approaches in cancer. Nat. Rev. Drug Discov. 17, 887–904.
Cassier, P. A., Italiano, A., Gomez-Roca, C. A., Le Tourneau, C., Toulmonde, M., Cannarile, M. A., et al. (2015). CSF1R inhibition with emactuzumab in locally advanced diffuse-type tenosynovial giant cell tumours of the soft tissue: a dose-escalation and dose-expansion phase 1 study. Lancet Oncol. 16, 949–956. doi: 10.1016/s1470-2045(15)00132-1
Chen, H., Chomyn, A., and Chan, D. C. (2005). Disruption of fusion results in mitochondrial heterogeneity and dysfunction. J. Biol. Chem. 280, 26185–26192. doi: 10.1074/jbc.m503062200
Chen, Y., and Dorn, G. W. (2013). PINK1-phosphorylated mitofusin 2 is a parkin receptor for culling damaged mitochondria. Science 340, 471–475. doi: 10.1126/science.1231031
Chow, A., Huggins, M., Ahmed, J., Hashimoto, D., Lucas, D., Kunisaki, Y., et al. (2013). CD169(+) macrophages provide a niche promoting erythropoiesis under homeostasis and stress. Nat. Med. 19, 429–436. doi: 10.1038/nm.3057
Crain, J. M., Nikodemova, M., and Watters, J. J. (2009). Expression of P2 nucleotide receptors varies with age and sex in murine brain microglia. J. Neuroinflammation 6:24.
Curtale, G., Mirolo, M., Renzi, T. A., Rossato, M., Bazzoni, F., and Locati, M. (2013). Negative regulation of toll-like receptor 4 signaling by IL-10-dependent microRNA-146b. Proc. Natl. Acad. Sci. U.S.A. 110, 11499–11504. doi: 10.1073/pnas.1219852110
Curtale, G., Renzi, T. A., Drufuca, L., Rubino, M., and Locati, M. (2017). Glucocorticoids downregulate TLR4 signaling activity via its direct targeting by miR-511-5p. Eur. J. Immunol. 47, 2080–2089. doi: 10.1002/eji.201747044
Czimmerer, Z., Daniel, B., Horvath, A., Ruckerl, D., Nagy, G., Kiss, M., et al. (2018). The transcription factor STAT6 mediates direct repression of inflammatory enhancers and limits activation of alternatively polarized macrophages. Immunity 48:75. doi: 10.1016/j.immuni.2017.12.010
Davis, C. H., Kim, K. Y., Bushong, E. A., Mills, E. A., Boassa, D., Shih, T., et al. (2014). Transcellular degradation of axonal mitochondria. Proc. Natl. Acad. Sci. U.S.A. 111, 9633–9638. doi: 10.1073/pnas.1404651111
Davis, C. H., and Marsh-Armstrong, N. (2014). Discovery and implications of transcellular mitophagy. Autophagy 10, 2383–2384. doi: 10.4161/15548627.2014.981920
De Stefani, D., Raffaello, A., Teardo, E., Szabo, I., and Rizzuto, R. (2011). A forty-kilodalton protein of the inner membrane is the mitochondrial calcium uniporter. Nature 476, 336–340. doi: 10.1038/nature10230
Desir, S., Dickson, E. L., Vogel, R. I., Thayanithy, V., Wong, P., Teoh, D., et al. (2016). Tunneling nanotube formation is stimulated by hypoxia in ovarian cancer cells. Oncotarget 7, 43150–43161. doi: 10.18632/oncotarget.9504
Duan, M., Li, W. C., Vlahos, R., Maxwell, M. J., Anderson, G. P., and Hibbs, M. L. (2012). Distinct macrophage subpopulations characterize acute infection and chronic inflammatory lung disease. J. Immunol. 189, 946–955. doi: 10.4049/jimmunol.1200660
Flaherty, S. E. III, Grijalva, A., Xu, X., Ables, E., Nomani, A., and Ferrante, A. W. Jr. (2019). A lipase-independent pathway of lipid release and immune modulation by adipocytes. Science 363, 989–993. doi: 10.1126/science.aaw2586
Friedman, J. R., and Nunnari, J. (2014). Mitochondrial form and function. Nature 505, 335–343. doi: 10.1038/nature12985
Galvan-Pena, S., and O’Neill, L. A. (2014). Metabolic reprograming in macrophage polarization. Front. Immunol. 5:420. doi: 10.3389/fimmu.2014.00420
Gao, J. J., Qin, A., Liu, D. L., Ruan, R., Wang, Q. Y., Yuan, J., et al. (2019). Endoplasmic reticulum mediates mitochondrial transfer within the osteocyte dendritic network. Sci. Adv. 5:eaaw7215. doi: 10.1126/sciadv.aaw7215
Gao, Y., Zhao, H., Wang, P., Wang, J., and Zou, L. (2018). The roles of SOCS3 and STAT3 in bacterial infection and inflammatory diseases. Scand. J. Immunol. 88:e12727. doi: 10.1111/sji.12727
Geissmann, F., Manz, M. G., Jung, S., Sieweke, M. H., Merad, M., and Ley, K. (2010). Development of monocytes, macrophages, and dendritic cells. Science 327, 656–661. doi: 10.1126/science.1178331
Ginhoux, F., Greter, M., Leboeuf, M., Nandi, S., See, P., Gokhan, S., et al. (2010). Fate mapping analysis reveals that adult microglia derive from primitive macrophages. Science 330, 841–845. doi: 10.1126/science.1194637
Ginhoux, F., and Guilliams, M. (2016). Tissue-resident macrophage ontogeny and homeostasis. Immunity 44, 439–449. doi: 10.1016/j.immuni.2016.02.024
Gong, G., Song, M., Csordas, G., Kelly, D. P., Matkovich, S. J., and Dorn, G. W. II (2015). Parkin-mediated mitophagy directs perinatal cardiac metabolic maturation in mice. Science 350:aad2459. doi: 10.1126/science.aad2459
Gosselin, D., Link, V. M., Romanoski, C. E., Fonseca, G. J., Eichenfield, D. Z., Spann, N. J., et al. (2014). Environment drives selection and function of enhancers controlling tissue-specific macrophage identities. Cell 159, 1327–1340. doi: 10.1016/j.cell.2014.11.023
Guilliams, M., De Kleer, I., Henri, S., Post, S., Vanhoutte, L., De Prijck, S., et al. (2013). Alveolar macrophages develop from fetal monocytes that differentiate into long-lived cells in the first week of life via GM-CSF. J. Exp. Med. 210, 1977–1992. doi: 10.1084/jem.20131199
Gustafsson, A. B., and Dorn, G. W. (2019). Evolving and expanding the roles of mitophagy as a homeostatic and pathogenic process. Physiol. Rev. 99, 853–892. doi: 10.1152/physrev.00005.2018
Han, M. S., Jung, D. Y., Morel, C., Lakhani, S. A., Kim, J. K., Flavell, R. A., et al. (2013). JNK expression by macrophages promotes obesity-induced insulin resistance and inflammation. Science 339, 218–222. doi: 10.1126/science.1227568
Haschemi, A., Kosma, P., Gille, L., Evans, C. R., Burant, C. F., Starkl, P., et al. (2012). The sedoheptulose kinase CARKL directs macrophage polarization through control of glucose metabolism. Cell Metab. 15, 813–826. doi: 10.1016/j.cmet.2012.04.023
Hayakawa, K., Esposito, E., Wang, X., Terasaki, Y., Liu, Y., Xing, C., et al. (2016). Transfer of mitochondria from astrocytes to neurons after stroke. Nature 535, 551–555. doi: 10.1038/nature18928
He, H., Mack, J. J., Guc, E., Warren, C. M., Squadrito, M. L., Kilarski, W. W., et al. (2016). Perivascular macrophages limit permeability. Arterioscler. Thromb. Vasc. Biol. 36, 2203–2212. doi: 10.1161/atvbaha.116.307592
Hoeffel, G., Wang, Y. L., Greter, M., See, P., Teo, P., Malleret, B., et al. (2012). Adult Langerhans cells derive predominantly from embryonic fetal liver monocytes with a minor contribution of yolk sac-derived macrophages. J. Exp. Med. 209, 1167–1181. doi: 10.1084/jem.20120340
Hsu, Y. C., Wu, Y. T., Yu, T. H., and Wei, Y. H. (2016). Mitochondria in mesenchymal stem cell biology and cell therapy: from cellular differentiation to mitochondrial transfer. Semin. Cell. Dev. Biol. 52, 119–131. doi: 10.1016/j.semcdb.2016.02.011
Huang, S. C. C., Everts, B., Ivanova, Y., O’Sullivan, D., Nascimento, M., Smith, A. M., et al. (2014). Cell-intrinsic lysosomal lipolysis is essential for alternative activation of macrophages. Nat. Immunol. 15, 846–855. doi: 10.1038/ni.2956
Huang, S. C. C., Smith, A. M., Everts, B., Colonna, M., Pearce, E. L., Schilling, J. D., et al. (2016). Metabolic reprogramming mediated by the mTORC2-IRF4 signaling axis is essential for macrophage alternative activation. Immunity 45, 817–830. doi: 10.1016/j.immuni.2016.09.016
Ikebuchi, Y., Aoki, S., Honma, M., Hayashi, M., Sugamori, Y., Khan, M., et al. (2018). Coupling of bone resorption and formation by RANKL reverse signalling. Nature 561:195. doi: 10.1038/s41586-018-0482-7
Islam, M. N., Das, S. R., Emin, M. T., Wei, M., Sun, L., Westphalen, K., et al. (2012). Mitochondrial transfer from bone-marrow-derived stromal cells to pulmonary alveoli protects against acute lung injury. Nat. Med. 18, 759–765. doi: 10.1038/nm.2736
Jackson, M. V., and Krasnodembskaya, A. D. (2017). Analysis of mitochondrial transfer in direct co-cultures of human Monocyte-Derived Macrophages (MDM) and Mesenchymal Stem Cells (MSC). Bio. Protoc. 7:e2255.
Jackson, M. V., Morrison, T. J., Doherty, D. F., Mcauley, D. F., Matthay, M. A., Kissenpfennig, A., et al. (2016). Mitochondrial transfer via tunneling nanotubes is an important mechanism by which mesenchymal stem cells enhance macrophage phagocytosis in the in vitro and in vivo models of ARDS. Stem Cells 34, 2210–2223. doi: 10.1002/stem.2372
Jiao, H., Jiang, D., Hu, X., Du, W., Ji, L., Yang, Y., et al. (2021). Mitocytosis, a migrasome-mediated mitochondrial quality-control process. Cell 184, 2896–910 e13.
Jin, Z. X., Wei, W., Yang, M., Du, Y., and Wan, Y. H. (2014). Mitochondrial complex I activity suppresses inflammation and enhances bone resorption by shifting macrophage-osteoclast polarization. Cell Metab. 20, 483–498. doi: 10.1016/j.cmet.2014.07.011
Kim, E. Y., Battaile, J. T., Patel, A. C., You, Y., Agapov, E., Grayson, M. H., et al. (2008). Persistent activation of an innate immune response translates respiratory viral infection into chronic lung disease. Nat. Med. 14, 633–640. doi: 10.1038/nm1770
Kim, I., Rodriguez-Enriquez, S., and Lemasters, J. J. (2007). Selective degradation of mitochondria by mitophagy. Arch. Biochem. Biophys. 462, 245–253. doi: 10.1016/j.abb.2007.03.034
Kim, M. J., Bae, S. H., Ryu, J. C., Kwon, Y., Oh, J. H., Kwon, J., et al. (2016). SESN2/sestrin2 suppresses sepsis by inducing mitophagy and inhibiting NLRP3 activation in macrophages. Autophagy 12, 1272–1291. doi: 10.1080/15548627.2016.1183081
Koentjoro, B., Park, J. S., and Sue, C. M. (2017). Nix restores mitophagy and mitochondrial function to protect against PINK1/Parkin-related Parkinson’s disease. Sci. Rep. 7:44373.
Korolnek, T., and Hamza, I. (2015). Macrophages and iron trafficking at the birth and death of red cells. Blood 125, 2893–2897. doi: 10.1182/blood-2014-12-567776
Kratz, M., Coats, B. R., Hisert, K. B., Hagman, D., Mutskov, V., Peris, E., et al. (2014). Metabolic dysfunction drives a mechanistically distinct proinflammatory phenotype in adipose tissue macrophages. Cell Metab. 20, 614–625. doi: 10.1016/j.cmet.2014.08.010
Krenkel, O., and Tacke, F. (2017). Liver macrophages in tissue homeostasis and disease. Nat. Rev. Immunol. 17, 306–321.
Lackner, L. L., Ping, H., Graef, M., Murley, A., and Nunnari, J. (2013). Endoplasmic reticulum-associated mitochondria-cortex tether functions in the distribution and inheritance of mitochondria. Proc. Natl. Acad. Sci. U.S.A. 110, E458–E467.
Lambeth, J. D. (2004). NOX enzymes and the biology of reactive oxygen. Nat. Rev. Immunol. 4, 181–189. doi: 10.1038/nri1312
Lapenna, A., De Palma, M., and Lewis, C. E. (2018). Perivascular macrophages in health and disease. Nat. Rev. Immunol. 18, 689–702. doi: 10.1038/s41577-018-0056-9
Larson-Casey, J. L., Deshane, J. S., Ryan, A. J., Thannickal, V. J., and Carter, A. B. (2016). Macrophage Akt1 kinase-mediated mitophagy modulates apoptosis resistance and pulmonary fibrosis. Immunity 44, 582–596.
Lavin, Y., Winter, D., Blecher-Gonen, R., David, E., Keren-Shaul, H., Merad, M., et al. (2014). Tissue-resident macrophage enhancer landscapes are shaped by the local microenvironment. Cell 159, 1312–1326. doi: 10.1016/j.cell.2014.11.018
Liesa, M., Palacin, M., and Zorzano, A. (2009). Mitochondrial dynamics in mammalian health and disease. Physiol. Rev. 89, 799–845. doi: 10.1152/physrev.00030.2008
Limatola, C., and Ransohoff, R. M. (2014). Modulating neurotoxicity through CX3CL1/CX3CR1 signaling. Front. Cell Neurosci. 8:229. doi: 10.3389/fncel.2014.00229
Linker, R. A., and Haghikia, A. (2016). Dimethyl fumarate in multiple sclerosis: latest developments, evidence and place in therapy. Ther. Adv. Chronic Dis. 7, 198–207. doi: 10.1177/2040622316653307
Liu, C. S., Chang, J. C., Kuo, S. J., Liu, K. H., Lin, T. T., Cheng, W. L., et al. (2014). Delivering healthy mitochondria for the therapy of mitochondrial diseases and beyond. Int. J. Biochem. Cell. Biol. 53, 141–146. doi: 10.1016/j.biocel.2014.05.009
Liu, D. L., Gao, Y. S., Liu, J., Huang, Y. G., Yin, J. H., Feng, Y. Y., et al. (2021). Intercellular mitochondrial transfer as a means of tissue revitalization. Signal Transduct. Target.Ther. 6:65.
Locati, M., Curtale, G., and Mantovani, A. (2020). Diversity, mechanisms, and significance of macrophage plasticity. Annu. Rev. Pathol. 15, 123–147. doi: 10.1146/annurev-pathmechdis-012418-012718
Lou, E., Fujisawa, S., Morozov, A., Barlas, A., Romin, Y., Dogan, Y., et al. (2012). Tunneling nanotubes provide a unique conduit for intercellular transfer of cellular contents in human malignant pleural mesothelioma. PLoS One 7:e33093. doi: 10.1371/journal.pone.0033093
Lumeng, C. N., Bodzin, J. L., and Saltiel, A. R. (2007). Obesity induces a phenotypic switch in adipose tissue macrophage polarization. J. Clin. Invest. 117, 175–184. doi: 10.1172/JCI29881
Lumeng, C. N., DelProposto, J. B., Westcott, D. J., and Saltiel, A. R. (2008). Phenotypic switching of adipose tissue macrophages with obesity is generated by spatiotemporal differences in macrophage subtypes. Diabetes 57, 3239–3246. doi: 10.2337/db08-0872
Ma, L., Li, Y., Peng, J., Wu, D., Zhao, X., Cui, Y., et al. (2015). Discovery of the migrasome, an organelle mediating release of cytoplasmic contents during cell migration. Cell. Res. 25, 24–38. doi: 10.1038/cr.2014.135
Maas, S. L., Broekman, M. L., and de Vrij, J. (2017). Tunable resistive pulse sensing for the characterization of extracellular vesicles. Methods Mol. Biol. 1545, 21–33. doi: 10.1007/978-1-4939-6728-5_2
Matsuda, N., Sato, S., Shiba, K., Okatsu, K., Saisho, K., Gautier, C. A., et al. (2010). PINK1 stabilized by mitochondrial depolarization recruits Parkin to damaged mitochondria and activates latent Parkin for mitophagy. J. Cell. Biol. 189, 211–221. doi: 10.1083/jcb.200910140
Mead, E. L., Mosley, A., Eaton, S., Dobson, L., Heales, S. J., and Pocock, J. M. (2012). Microglial neurotransmitter receptors trigger superoxide production in microglia; consequences for microglial-neuronal interactions. J. Neurochem. 121, 287–301. doi: 10.1111/j.1471-4159.2012.07659.x
Melis, N., Rubera, I., Cougnon, M., Giraud, S., Mograbi, B., Belaid, A., et al. (2017). Targeting eIF5A hypusination prevents anoxic cell death through mitochondrial silencing and improves kidney transplant outcome. J. Am. Soc. Nephrol. 28, 811–822. doi: 10.1681/ASN.2016010012
Mendez-Ferrer, S., Michurina, T. V., Ferraro, F., Mazloom, A. R., Macarthur, B. D., Lira, S. A., et al. (2010). Mesenchymal and haematopoietic stem cells form a unique bone marrow niche. Nature 466, 829–834. doi: 10.1038/nature09262
Mills, E. L., and O’Neill, L. A. (2016). Reprogramming mitochondrial metabolism in macrophages as an anti-inflammatory signal. Eur. J. Immunol. 46, 13–21. doi: 10.1002/eji.201445427
Mills, E. L., Ryan, D. G., Prag, H. A., Dikovskaya, D., Menon, D., Zaslona, Z., et al. (2018). Itaconate is an anti-inflammatory metabolite that activates Nrf2 via alkylation of KEAP1. Nature 556:113. doi: 10.1038/nature25986
Moore, K. J., and Tabas, I. (2011). Macrophages in the pathogenesis of atherosclerosis. Cell 145, 341–355. doi: 10.1016/j.cell.2011.04.005
Morrison, S. J., and Scadden, D. T. (2014). The bone marrow niche for haematopoietic stem cells. Nature 505, 327–334. doi: 10.1038/nature12984
Morrison, T. J., Jackson, M. V., Cunningham, E. K., Kissenpfennig, A., McAuley, D. F., O’Kane, C. M., et al. (2017). Mesenchymal stromal cells modulate macrophages in clinically relevant lung injury models by extracellular vesicle mitochondrial transfer. Am. J. Respir. Crit. Care Med. 196, 1275–1286. doi: 10.1164/rccm.201701-0170OC
Moschoi, R., Imbert, V., Nebout, M., Chiche, J., Mary, D., Prebet, T., et al. (2016). Protective mitochondrial transfer from bone marrow stromal cells to acute myeloid leukemic cells during chemotherapy. Blood 128, 253–264. doi: 10.1182/blood-2015-07-655860
Na, Y. R., Je, S., and Seok, S. H. (2018). Metabolic features of macrophages in inflammatory diseases and cancer. Cancer Lett. 413, 46–58. doi: 10.1016/j.canlet.2017.10.044
Nagata, S. (2018). Apoptosis and clearance of apoptotic cells. Annu. Rev. Immunol. 36, 489–517. doi: 10.1146/annurev-immunol-042617-053010
Nakahira, K., Haspel, J. A., Rathinam, V. A. K., Lee, S. J., Dolinay, T., Lam, H. C., et al. (2011). Autophagy proteins regulate innate immune responses by inhibiting the release of mitochondrial DNA mediated by the NALP3 inflammasome. Nat. Immunol. 12, 222–230. doi: 10.1038/ni.1980
Nicolas-Avila, J. A., Lechuga-Vieco, A. V., Esteban-Martinez, L., Sanchez-Diaz, M., Diaz-Garcia, E., Santiago, D. J., et al. (2020). A network of macrophages supports mitochondrial homeostasis in the heart. Cell 183:94. doi: 10.1016/j.cell.2020.08.031
O’Beirne, S. L., Kikkers, S. A., Oromendia, C., Salit, J., Rostmai, M. R., Ballman, K. V., et al. (2020). Alveolar macrophage immunometabolism and lung function impairment in smoking and chronic obstructive pulmonary disease. Am. J. Respir. Crit. Care Med. 201, 735–739. doi: 10.1164/rccm.201908-1683LE
Odegaard, J. I., Ricardo-Gonzalez, R. R., Goforth, M. H., Morel, C. R., Subramanian, V., Mukundan, L., et al. (2007). Macrophage-specific PPARgamma controls alternative activation and improves insulin resistance. Nature 447, 1116–1120. doi: 10.1038/nature05894
Oka, T., Hikoso, S., Yamaguchi, O., Taneike, M., Takeda, T., Tamai, T., et al. (2012). Mitochondrial DNA that escapes from autophagy causes inflammation and heart failure. Nature 485, 251–255. doi: 10.1038/nature10992
Okabe, Y., and Medzhitov, R. (2016). Tissue biology perspective on macrophages. Nat. Immunol. 17, 9–17. doi: 10.1038/ni.3320
Okatsu, K., Saisho, K., Shimanuki, M., Nakada, K., Shitara, H., Sou, Y. S., et al. (2010). p62/SQSTM1 cooperates with Parkin for perinuclear clustering of depolarized mitochondria. Genes Cells 15, 887–900. doi: 10.1111/j.1365-2443.2010.01426.x
Palsson-McDermott, E. M., Curtis, A. M., Goel, G., Lauterbach, M. A. R., Sheedy, F. J., Gleeson, L. E., et al. (2015). Pyruvate kinase M2 regulates Hif-1 alpha activity and IL-1 beta induction and is a critical determinant of the warburg effect in LPS-activated macrophages. Cell Metab. 21:347. doi: 10.1016/j.cmet.2014.12.005
Pankiv, S., Clausen, T. H., Lamark, T., Brech, A., Bruun, J. A., Outzen, H., et al. (2007). p62/SQSTM1 binds directly to Atg8/LC3 to facilitate degradation of ubiquitinated protein aggregates by autophagy. J. Biol. Chem. 282, 24131–24145. doi: 10.1074/jbc.M702824200
Paolicelli, R. C., Bolasco, G., Pagani, F., Maggi, L., Scianni, M., Panzanelli, P., et al. (2011). Synaptic pruning by microglia is necessary for normal brain development. Science 333, 1456–1458. doi: 10.1126/science.1202529
Parkhurst, C. N., Yang, G., Ninan, I., Savas, J. N., Yates, J. R., Lafaille, J. J., et al. (2013). Microglia promote learning-dependent synapse formation through brain-derived neurotrophic factor. Cell 155, 1596–1609. doi: 10.1016/j.cell.2013.11.030
Pasquier, J., Guerrouahen, B. S., Al Thawadi, H., Abu Kaoud, N., Maleki, M., Le Foll, F., et al. (2013). Preferential transfer of mitochondria from endothelial to cancer cells through tunneling nanotubes modulates chemoresistance. J. Transl. Med. 11:94. doi: 10.1158/1078-0432.OVCA13-B69
Pathria, P., Louis, T. L., and Varner, J. A. (2019). Targeting tumor-associated macrophages in cancer. Trends Immunol. 40, 310–327. doi: 10.1016/j.it.2019.02.003
Patoli, D., Mignotte, F., Deckert, V., Dusuel, A., Dumont, A., Rieu, A., et al. (2020). Inhibition of mitophagy drives macrophage activation and antibacterial defense during sepsis. J. Clin. Invest. 130, 5858–5874. doi: 10.1172/JCI130996
Peet, C., Ivetic, A., Bromage, D. I., and Shah, A. M. (2020). Cardiac monocytes and macrophages after myocardial infarction. Cardiovasc. Res. 116, 1101–1112. doi: 10.1093/cvr/cvz336
Peruzzotti-Jametti, L., and Pluchino, S. (2018). Targeting mitochondrial metabolism in neuroinflammation: towards a therapy for progressive multiple sclerosis. Trends Mol. Med. 24, 838–855. doi: 10.1016/j.molmed.2018.07.007
Phinney, D. G., Di Giuseppe, M., Njah, J., Sala, E., Shiva, S., St Croix, C. M., et al. (2015). Mesenchymal stem cells use extracellular vesicles to outsource mitophagy and shuttle microRNAs. Nat. Commun. 6:8472. doi: 10.1038/ncomms9472
Pirzgalska, R. M., Seixas, E., Seidman, J. S., Link, V. M., Sanchez, N. M., Mahu, I., et al. (2017). Sympathetic neuron-associated macrophages contribute to obesity by importing and metabolizing norepinephrine. Nat. Med. 23, 1309–1318. doi: 10.1038/nm.4422
Pober, J. S., and Sessa, W. C. (2007). Evolving functions of endothelial cells in inflammation. Nat. Rev. Immunol. 7, 803–815. doi: 10.1038/nri2171
Puhm, F., Afonyushkin, T., Resch, U., Obermayer, G., Rohde, M., Penz, T., et al. (2019). Mitochondria are a subset of extracellular vesicles released by activated monocytes and induce Type I IFN and TNF responses in endothelial cells. Circ. Res. 125, 43–52.
Puleston, D. J., Buck, M. D., Klein Geltink, R. I., Kyle, R. L., Caputa, G., O’Sullivan, D., et al. (2019). Polyamines and eIF5A hypusination modulate mitochondrial respiration and macrophage activation. Cell Metab. 30, 352–63 e8. doi: 10.1016/j.cmet.2019.05.003
Rafelski, S. M. (2013). Mitochondrial network morphology: building an integrative, geometrical view. BMC Biol. 11:71. doi: 10.1186/1741-7007-11-71
Rodrigues, D., Freitas, M., Marisa Costa, V., Arturo Lopez-Quintela, M., Rivas, J., Freitas, P., et al. (2017). Quantitative histochemistry for macrophage biodistribution on mice liver and spleen after the administration of a pharmacological-relevant dose of polyacrylic acid-coated iron oxide nanoparticles. Nanotoxicology 11, 256–266. doi: 10.1080/17435390.2017.1291865
Sazanov, L. A. (2007). Respiratory complex I: mechanistic and structural insights provided by the crystal structure of the hydrophilic domain. Biochemistry 46, 2275–2288. doi: 10.1021/bi602508x
Scarpulla, R. C. (2008). Transcriptional paradigms in mammalian mitochondrial biogenesis and function. Physiol. Rev. 88, 611–638. doi: 10.1152/physrev.00025.2007
Schon, E. A., DiMauro, S., and Hirano, M. (2012). Human mitochondrial DNA: roles of inherited and somatic mutations. Nat. Rev. Genet. 13, 878–890. doi: 10.1038/nrg3275
Schulz, C., Gomez Perdiguero, E., Chorro, L., Szabo-Rogers, H., Cagnard, N., Kierdorf, K., et al. (2012). A lineage of myeloid cells independent of Myb and hematopoietic stem cells. Science 336, 86–90. doi: 10.1126/science.1219179
Sengupta, T. K., Schmitt, E. M., and Ivashkiv, L. B. (1996). Inhibition of cytokines and JAK-STAT activation by distinct signaling pathways. Proc. Natl. Acad. Sci. U.S.A. 93, 9499–9504. doi: 10.1073/pnas.93.18.9499
Short, K. R., Kroeze, E., Fouchier, R. A. M., and Kuiken, T. (2014). Pathogenesis of influenza-induced acute respiratory distress syndrome. Lancet Infect. Dis. 14, 57–69. doi: 10.1016/S1473-3099(13)70286-X
Sica, A., and Bronte, V. (2007). Altered macrophage differentiation and immune dysfunction in tumor development. J. Clin. Invest. 117, 1155–1166. doi: 10.1172/JCI31422
Sica, A., and Mantovani, A. (2012). Macrophage plasticity and polarization: in vivo veritas. J. Clin. Invest. 122, 787–795. doi: 10.1172/JCI59643
Sorbara, M. T., and Girardin, S. E. (2011). Mitochondrial ROS fuel the inflammasome. Cell. Res. 21, 558–560. doi: 10.1038/cr.2011.20
Spees, J. L., Olson, S. D., Whitney, M. J., and Prockop, D. J. (2006). Mitochondrial transfer between cells can rescue aerobic respiration. Proc. Natl. Acad. Sci. U.S.A. 103, 1283–1288. doi: 10.1073/pnas.0510511103
Stein, M., Keshav, S., Harris, N., and Gordon, S. (1992). Interleukin 4 potently enhances murine macrophage mannose receptor activity: a marker of alternative immunologic macrophage activation. J. Exp. Med. 176, 287–292. doi: 10.1084/jem.176.1.287
Su, P., Wang, Q., Bi, E., Ma, X., Liu, L., Yang, M., et al. (2020). Enhanced lipid accumulation and metabolism are required for the differentiation and activation of tumor-associated macrophages. Cancer Res. 80, 1438–1450. doi: 10.1158/0008-5472.CAN-19-2994
Tacke, F. (2017). Targeting hepatic macrophages to treat liver diseases. J. Hepatol. 66, 1300–1312. doi: 10.1016/j.jhep.2017.02.026
Takayanagi, H., Kim, S., Matsuo, K., Suzuki, H., Suzuki, T., Sato, K., et al. (2002). RANKL maintains bone homeostasis through c-Fos-dependent induction of interferon-beta. Nature 416, 744–749. doi: 10.1038/416744a
Tan, A. S., Baty, J. W., Dong, L. F., Bezawork-Geleta, A., Endaya, B., Goodwin, J., et al. (2015). Mitochondrial genome acquisition restores respiratory function and tumorigenic potential of cancer cells without mitochondrial DNA. Cell Metab. 21, 81–94. doi: 10.1016/j.cmet.2014.12.003
Taniguchi, T., Ogasawara, K., Takaoka, A., and Tanaka, N. (2001). IRF family of transcription factors as regulators of host defense. Annu. Rev. Immunol. 19, 623–655. doi: 10.1146/annurev.immunol.19.1.623
Tong, M. M., Saito, T., Zhai, P. Y., Oka, S., Mizushima, W., Nakamura, M., et al. (2019). Mitophagy is essential for maintaining cardiac function during high fat diet-induced diabetic cardiomyopathy. Circ. Res. 124, 1360–1371. doi: 10.1161/CIRCRESAHA.118.314607
Tur, J., Vico, T., Lloberas, J., Zorzano, A., and Celada, A. (2017). Macrophages and mitochondria: a critical interplay between metabolism, signaling, and the functional activity. Adv. Immunol. 133, 1–36. doi: 10.1016/bs.ai.2016.12.001
Varol, C., Mildner, A., and Jung, S. (2015). Macrophages: development and tissue specialization. Annu. Rev. Immunol. 33, 643–675. doi: 10.1146/annurev-immunol-032414-112220
Vats, D., Mukundan, L., Odegaard, J. I., Zhang, L., Smith, K. L., Morel, C. R., et al. (2006). Oxidative metabolism and PGC-1 beta attenuate macrophage-mediated inflammation. Cell Metab. 4:255. doi: 10.1016/j.cmet.2006.08.006
Wang, J., Liu, X., Qiu, Y., Shi, Y., Cai, J., Wang, B., et al. (2018). Cell adhesion-mediated mitochondria transfer contributes to mesenchymal stem cell-induced chemoresistance on T cell acute lymphoblastic leukemia cells. J. Hematol. Oncol. 11:11. doi: 10.1186/s13045-018-0554-z
Wei, Y., Corbalan-Campos, J., Gurung, R., Natarelli, L., Zhu, M., Exner, N., et al. (2018). Dicer in macrophages prevents atherosclerosis by promoting mitochondrial oxidative metabolism. Circulation 138, 2007–2020. doi: 10.1161/CIRCULATIONAHA.117.031589
Weinberg, S. E., Sena, L. A., and Chandel, N. S. (2015). Mitochondria in the regulation of innate and adaptive immunity. Immunity 42, 406–417. doi: 10.1016/j.immuni.2015.02.002
West, A. P., Brodsky, I. E., Rahner, C., Woo, D. K., Erdjument-Bromage, H., Tempst, P., et al. (2011). TLR signalling augments macrophage bactericidal activity through mitochondrial ROS. Nature 472, 476–480. doi: 10.1038/nature09973
Wiehagen, K. R., Girgis, N. M., Yamada, D. H., Smith, A. A., Chan, S. R., Grewal, I. S., et al. (2017). Combination of CD40 agonism and CSF-1R blockade reconditions tumor-associated macrophages and drives potent antitumor immunity. Cancer Immunol. Res. 5, 1109–1121. doi: 10.1158/2326-6066.CIR-17-0258
Wynn, T. A., Chawla, A., and Pollard, J. W. (2013). Macrophage biology in development, homeostasis and disease. Nature 496, 445–455. doi: 10.1038/nature12034
Wynn, T. A., and Vannella, K. M. (2016). Macrophages in tissue repair, regeneration, and fibrosis. Immunity 44, 450–462. doi: 10.1016/j.immuni.2016.02.015
Xia, Y., Rao, L., Yao, H., Wang, Z., Ning, P., and Chen, X. (2020). Engineering macrophages for cancer immunotherapy and drug delivery. Adv. Mater. 32:e2002054. doi: 10.1002/adma.202002054
Xiang, X. N., Wang, J. G., Lu, D., and Xu, X. (2021). Targeting tumor-associated macrophages to synergize tumor immunotherapy. Signal Transduct. Target Ther. 6:75.
Xu, X., Grijalva, A., Skowronski, A., van Eijk, M., Serlie, M. J., and Ferrante, A. W. Jr. (2013). Obesity activates a program of lysosomal-dependent lipid metabolism in adipose tissue macrophages independently of classic activation. Cell Metab. 18, 816–830.
Yamada, Y., Ito, M., Arai, M., Hibino, M., Tsujioka, T., and Harashima, H. (2020). Challenges in promoting mitochondrial transplantation therapy. Int. J. Mol. Sci. 21:6365.
Yao, Y., Fan, X. L., Jiang, D., Zhang, Y. L., Li, X., Xu, Z. B., et al. (2018). Connexin 43-mediated mitochondrial transfer of iPSC-MSCs alleviates asthma inflammation. Stem Cell Rep. 11, 1120–1135.
Youle, R. J., and Narendra, D. P. (2011). Mechanisms of mitophagy. Nat. Rev. Mol. Cell. Biol. 12, 9–14.
Youle, R. J., and van der Bliek, A. M. (2012). Mitochondrial fission, fusion, and stress. Science 337, 1062–1065.
Zampieri, L. X., Silva-Almeida, C., Rondeau, J. D., and Sonveaux, P. (2021). Mitochondrial transfer in cancer: a comprehensive review. Int. J. Mol. Sci. 22:3245. doi: 10.3390/ijms22063245
Zhang, T., Xue, L., Li, L., Tang, C., Wan, Z., Wang, R., et al. (2016). BNIP3 protein suppresses PINK1 kinase proteolytic cleavage to promote mitophagy. J. Biol. Chem. 291, 21616–21629. doi: 10.1074/jbc.M116.733410
Zhang, X. Y., Sergin, I., Evans, T. D., Jeong, S. J., Rodriguez-Velez, A., Kapoor, D., et al. (2020). High-protein diets increase cardiovascular risk by activating macrophage mTOR to suppress mitophagy. Nat. Metab. 2:110. doi: 10.1038/s42255-019-0162-4
Zhang, Y., Choksi, S., Chen, K., Pobezinskaya, Y., Linnoila, I., and Liu, Z. G. (2013). ROS play a critical role in the differentiation of alternatively activated macrophages and the occurrence of tumor-associated macrophages. Cell Res. 23, 898–914.
Zhang, Y. L., Yu, Z. D., Jiang, D., Liang, X. T., Liao, S. Y., Zhang, Z., et al. (2016). iPSC-MSCs with high intrinsic MIRO1 and sensitivity to TNF-alpha yield efficacious mitochondrial transfer to rescue anthracycline-induced cardiomyopathy. Stem Cell Rep. 7, 749–763.
Zhao, Y., Guo, Y. F., Jiang, Y. T., Zhu, X. D., Liu, Y. Q., and Zhang, X. L. (2017). Mitophagy regulates macrophage phenotype in diabetic nephropathy rats. Biochem. Biophys. Res. Commun. 494, 42–50. doi: 10.1016/j.bbrc.2017.10.088
Zhong, Z., Liang, S., Sanchez-Lopez, E., He, F., Shalapour, S., Lin, X. J., et al. (2018). New mitochondrial DNA synthesis enables NLRP3 inflammasome activation. Nature 560, 198–203. doi: 10.1038/s41586-018-0372-z
Zhou, R. B., Yazdi, A. S., Menu, P., and Tschopp, J. (2011). A role for mitochondria in NLRP3 inflammasome activation. Nature 475:221.
Keywords: macrophage, mitochondrial transfer, mitophagy, adipocyte, cardiomyocyte
Citation: Pang Y, Zhang C and Gao J (2021) Macrophages as Emerging Key Players in Mitochondrial Transfers. Front. Cell Dev. Biol. 9:747377. doi: 10.3389/fcell.2021.747377
Received: 26 July 2021; Accepted: 21 September 2021;
Published: 13 October 2021.
Edited by:
Amanda Maree Clark, University of Pittsburgh, United StatesReviewed by:
Silvia Jiménez-Morales, Instituto Nacional de Medicina Genómica (INMEGEN), MexicoChao Zhao, Fudan University, China
Copyright © 2021 Pang, Zhang and Gao. This is an open-access article distributed under the terms of the Creative Commons Attribution License (CC BY). The use, distribution or reproduction in other forums is permitted, provided the original author(s) and the copyright owner(s) are credited and that the original publication in this journal is cited, in accordance with accepted academic practice. No use, distribution or reproduction is permitted which does not comply with these terms.
*Correspondence: Changqing Zhang, emhhbmdjcUBzanR1LmVkdS5jbg==; Junjie Gao, Y29saW5nampAMTYzLmNvbQ==