- 1Department of Oncology, Xinqiao Hospital, Third Military Medical University, Chongqing, China
- 2Chongqing Key Laboratory of Immunotherapy, Chongqing, China
- 3Department of Thoracic Surgery, The Third Affiliated Hospital of Kunming Medical University, Kunming, China
Myeloid-derived suppressor cell (MDSC) is a heterogeneous population of immature myeloid cells, has a pivotal role in negatively regulating immune response, promoting tumor progression, creating pre-metastases niche, and weakening immunotherapy efficacy. The underlying mechanisms are complex and diverse, including immunosuppressive functions (such as inhibition of cytotoxic T cells and recruitment of regulatory T cells) and non-immunological functions (mediating stemness and promoting angiogenesis). Moreover, MDSC may predict therapeutic response as a poor prognosis biomarker among multiple tumors. Accumulating evidence indicates targeting MDSC can reverse immunosuppressive tumor microenvironment, and improve therapeutic response either single or combination with immunotherapy. This review summarizes the phenotype and definite mechanisms of MDSCs in tumor progression, and provide new insights of targeting strategies regarding to their clinical applications.
1 Introduction
Myeloid cells, which are derived from hematopoietic stem cells, have diverse functions such as protect human body against infection and help tissue repair. Mature myeloid cells, including macrophages, neutrophils, eosinophils, and basophils, are a key component of the innate immune system. However, the majority of these pro-inflammatory cells can be re-educated to become pro-tumor cells when tumorigenesis occurs. Significantly increased immature myeloid cells have been observed in the bone marrow and peripheral blood of patients with cancer (Yang et al., 2004). These immature myeloid cells are called myeloid-derived suppressor cells (MDSCs) because of their capacity to suppress the anti-tumor immune response (Talmadge and Gabrilovich, 2013).
The existence of enriched MDSCs have been shown to be related with poor prognosis for multiple types of cancer (Jiang et al., 2015; Tian et al., 2015). Various pro-tumor mechanisms have been reported: MDSCs suppress the proliferation and cytolysis of T cells (Chen et al., 2017) and promote the recruitment and expansion of Treg cells (Serafini et al., 2008). MDSCs are also involved in angiogenesis (Lee et al., 2005) and the maintenance of stemness (Wang et al., 2019a). However, in most studies the role of MDSCs have been studied independently and generate fragmented information. The limitations hinder our understanding of MDSCs in tumor microenvironment and optimize anti-tumor treatment.
In this review, we systematically summarize the characteristics and definite mechanisms of MDSCs in tumor progression and metastasis, highlight the role of MDSCs in clinical treatment, and further discuss the potential strategies to provide new insights regarding their clinical applications.
2 Definition of MDSCs
MDSCs comprise a heterogeneous population of immature myeloid cells that are divided into different subsets based on morphology and surface markers. In mice, MDSCs are mainly categorized into two groups: granulocytic MDSCs (G-MDSCs; or polymorphonuclear MDSCs [PMN-MDSCs]) and monocytic (M) MDSCs (M-MDSCs) (Zhao et al., 2016). Other subpopulations found in humans are immature MDSCs (i-MDSCs) (Mandruzzato et al., 2016) and fibrocytic MDSCs (f-MDSCs) (Zoso et al., 2014). G-MDSCs, which are characterized as CD11b+Ly6GhiLy6Clo cells, mainly produce reactive oxygen species (ROS) to suppress T-cell function (Tacke et al., 2012). The M-MDSCs referred to as CD11b+Ly6GloLy6Chi cells can inhibit the immune response by producing nitric oxide (NO), arginase 1 (Arg-1), and IL-10 (Maenhout et al., 2014). The f-MDSC subset, which is characterized by the expression of CD33+IL4Rα+, attenuates T-cell proliferation via indoleamine 2-3 dioxygenase (IDO) secretion and promotes regulatory T (Treg) cell expansion (Zoso et al., 2014). Nevertheless, the phenotype and function of i-MDSCs have not been determined (Figure 1).
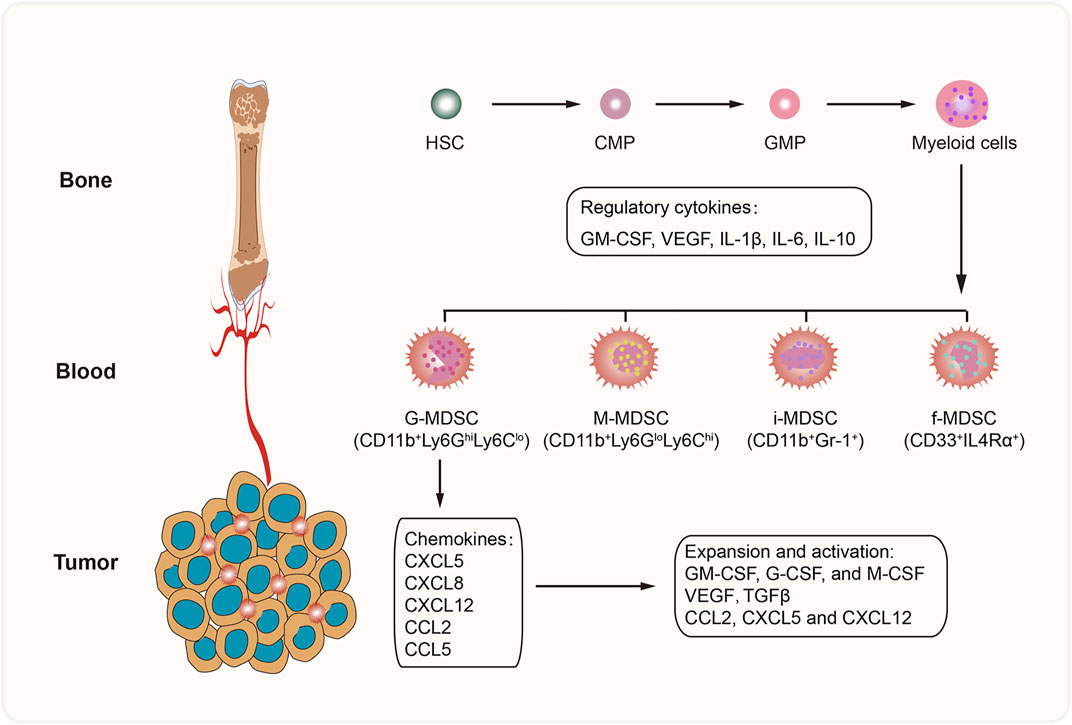
FIGURE 1. Development of MDSCs. MDSCs are derived from hematopoietic stem cells (HSC) in bone marrow, and differentiate into myeloid cells step by step. In the pathological conditions, a series of cytokines (GM-CSF, VEGF, IL-1β, IL-6, and IL-10) promote MDSC generation. Then suppressive MDSCs are recruited into TME by tumor-derived chemokines, such as CXCL5, CXCL8, CXCL12, CCL2, and CCL5. Further, the favorable TME accelerates the activation, survival and expansion of MDSCs. CMP, common myeloid progenitor; GMP, granulocyte and macrophage progenitor.
Although the generation of MDSCs might be explained by the interrupted development of normal myelopoiesis and abnormal expansion of immature myeloid cells (Netherby and Abrams, 2017), the underlying mechanisms are unclear. Several cytokines and growth factors secreted by tumor cells and immune cells have been shown to promote the accumulation and expansion of MDSCs by activating the JAK/STAT1 and JAK/STAT3 signaling pathways (Fleming et al., 2018), including granulocyte-macrophage colony-stimulating factor (GM-CSF), vascular endothelial growth factor (VEGF), transforming growth factor-β (TGF-β), interleukin-6 (IL-6), and interleukin-10 (IL-10). Moreover, these cells can recruit MDSCs into the tumor microenvironment (TME) by releasing certain chemokines, such as CXCL5, CXCL8, CXCL12, CCL2, and CCL5 (Ozga et al., 2021).
3 Contribution of MDSCs to Tumor Progression
3.1 Immunosuppressive Functions of MDSCs on Tumor Progression
MDSCs are potent suppressors of T-cell activation and function through various mechanisms, including the expression of ligands of negative immune checkpoint regulators, secretion of immunosuppressive cytokines, disruption of amino acid metabolism. Moreover, MDSCs also play a critical role in establishment and maintenance of immunosuppressive microenvironment by interacting with other immune cells, including nature killer cells (NK cells), dendritic cells (DCs), macrophages, and Treg cells (Figure 2).
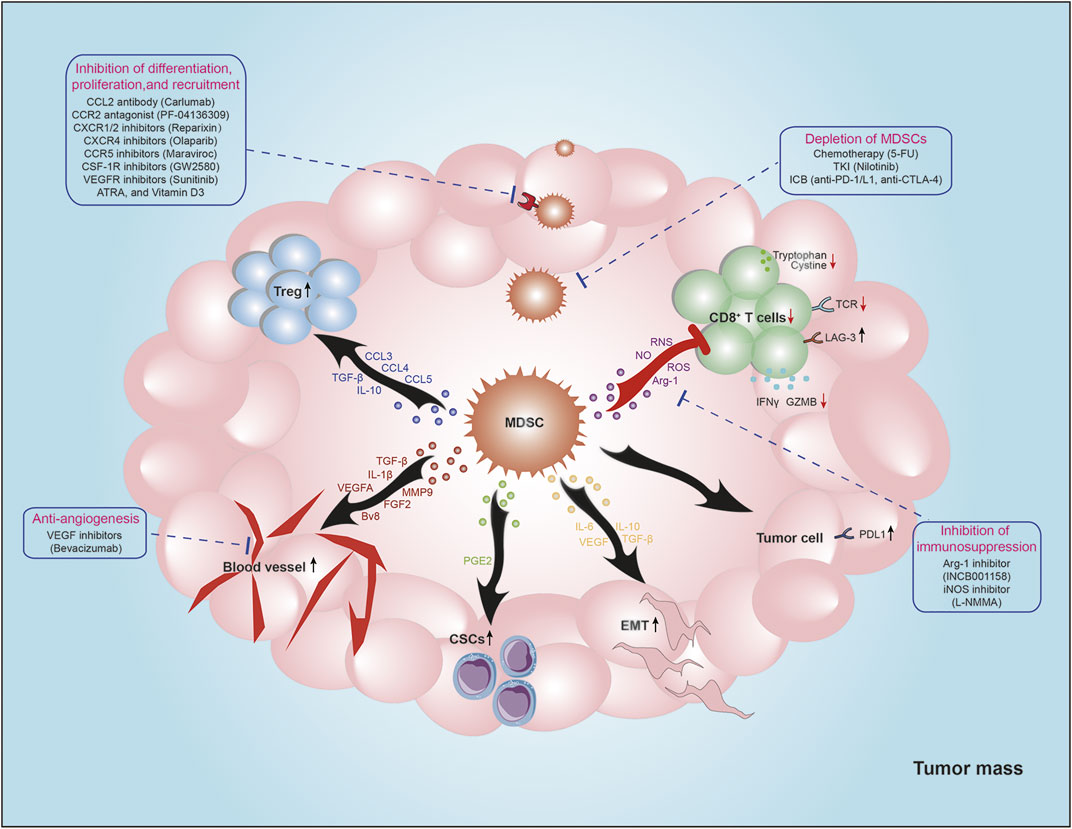
FIGURE 2. The roles of MDSCs in TME. MDSCs are potent suppressors of antitumor immunity. MDSCs decrease TCR expression and impair cytotoxic T cell function via producing Arg-1, NO, ROS, IL-10, and TGF-β. Moreover, MDSCs promote Tregs recruitment by secreting certain chemokines (CCL3, CCL4, and CCL5), and increase Tregs function via producing IL-10 and TGF-β. Additionally, MDSCs increase PD-L1 expression of tumor cells to mediate immune evasion. MDSCs also play non-immunological functions. MDSCs mediate EMT and stemness of tumor cells by producing VEGF, PEG2, IL-6, IL-10, and TGF-β. And MDSCs could induce angiogenesis via secreting VEGFA, FGF2, Bv8, TGF-β, IL-1β, and MMP9 to promote tumor metastasis. Strategies targeting MDSCs for cancer treatment are summarized around the main figure.
3.1.1 Suppression of T Cells by Expression of Ligands of Negative Immune Checkpoint Regulators
MDSCs are the main suppressive cells in the anti-tumor immune response; their main role is regulating the quantity and functions of T cells. Tumor-infiltrating MDSCs express high levels of Fas ligand (Fas-L) to induce apoptosis of CD8+ T cells by binding to the Fas receptor (Rashid et al., 2021). A previous study found that PMN-MDSCs rather than M-MDSCs could specially induce CD8+ T cells apoptosis through Fas-FasL axis and result in local immune suppression (Zhu J et al., 2017). And activated CD8+ T cells might also lead to MDSC apoptosis via the Fas-FasL axis (Sinha et al., 2011). Therefore, inducing apoptosis between MDSCs and T cells might result in reciprocal actions. Additionally, MDSCs in TME highly express several ligands of negative immune checkpoint regulators, programmed cell death protein ligand 1 (PD-L1) (Lu et al., 2016) and Galectin-9 (Dardalhon et al., 2010), which bind to programmed cell death protein 1 (PD-1) or T-cell immunoglobulin and mucin domain-containing protein 3 (TIM3) to induce effector T-cell anergy.
3.1.2 Suppression of T Cells by Secretion of Immunosuppressive Cytokines
In response to complex tumor microenvironment, MDSCs develop formidable plasticity, including cytokines, chemokines, and growth factors which are responsible for the suppressive capacity (Lechner et al., 2010). These products are potential therapeutic targets for inhibition of MDSC.
IL-10
Increased level of IL-10 has been reported in many cancers. In 2011, Hart et al have identified MDSCs as the predominant producers of IL-10 in a mouse model of ovarian cancer (Hart et al., 2011). They also found that IL-10 produced by MDSCs increased lymphocyte activation gene 3 (LAG-3) expression and decreased interferon (IFN)-γ secretion by T cells (Hart et al., 2011). MDSCs can activate the immunosuppressive capacity through IL-10 in pathological conditions and contribute to disease progression (Yaseen et al., 2020). The conclusion was further supported by a previous finding that IL-10 level was significantly reduced by MDSCs depletion in ovarian cancer-bearing mice (Hart et al., 2009). It has been revealed that IL-10 level was positively correlated with MDSCs expansion and tumor progression in patients with anaplastic thyroid cancer, ovarian cancer, gastric cancer and no-small cell lung cancer (Suzuki et al., 2013; Li et al., 2015; Pogoda et al., 2016; Wu et al., 2017). Further studies reported that increased production of IL-10 by MDSCs could inhibit the production of IL-2, IL-12 and IFN-γ by CD4+ or CD8+ T cells, which lead to their impaired proliferation and anti-tumor immunity (Vuk-Pavlovic et al., 2010; Li et al., 2015). Interestingly, IL-10/IL-10 receptor signaling is also critical for phenotypic and functional maintenance of MDSCs (Bah et al., 2018). It has been reported that blocking IL-10/IL-10R could decrease the infiltration of MDSCs in ascites of ovarian cancer-bearing mice (Lamichhane et al., 2017). Moreover, IL-10 has been shown to strength the immunosuppressive functions of MDSCs by upregulating the expression of ARG-1 and programmed cell death protein 1 (PD-1) (Xiu et al., 2015; Lamichhane et al., 2017). These results indicated that there was positive feedback between MDSCs and IL-10. In addition, IL-10 is also involved in the differentiation and expansion of Treg cells (Heo et al., 2010). Researchers found that IL-10 treatment could induce Foxp3 expression of CD4+ T cell population and increase the number of Treg cells. Further studies found that histone deacetylase 11 (HDAC11) and alarmin high mobility group box 1 (HMGB1) are key negative regulators of IL-10 transcription in MDSCs (Villagra et al., 2009; Parker et al., 2014), indicating a promising therapeutic strategy for enhancing anti-tumor immunity.
TGF-β
Increased TGF-β production in MDSCs has been identified in various tumor types. Chikamatsu et al reported that CD14+ HLA-DR− MDSCs produced high level of TGF-β to inhibit T cell proliferation and IFN-γ production in squamous cell carcinoma of the head and neck. And blocking TGF-β with antibody could partially the immunosuppressive functions of MDSCs (Chikamatsu et al., 2012; Novitskiy et al., 2012). Moreover, in a mouse model with specific deletion of Tgfbr2 in myeloid cells, the suppressive function of CD11b+Gr1+ MDSCs was found to decreased significantly (Novitskiy et al., 2012). The TGF-β family of proteins is a potent immune regulator that can inhibit proliferation, differentiation, activation, and cytotoxicity of effector T cells. It has been reported that TGF-β blocked naïve T cells differentiated into Th1 cells, which is the most important subset to mediate anti-tumor response (Sad and Mosmann 1994). Further studies revealed that the impaired differentiation might due to the silence of the expression of two Th1 master transcription factors, TBET and STAT4 (Gorelik et al., 2002; Lin et al., 2005). Chen and colleagues found that TGF-β inhibited T cell activation by dampening the initial Ca2+ influx triggered T Cell Receptor (TCR) stimulation (Chen et al., 2003). In addition to inhibiting differentiation and activation, TGF-β blocks T cell proliferation and effector functions. TGF-β could decrease IL-2 expression of T cells to inhibit proliferation by Smad3 signaling (McKarns et al., 2004). Thomas and Massague revealed that the TGF-β/Smad pathway could directly bind to their promoter regions to downregulate the expression of granzyme B and IFN-γ (Thomas and Massague, 2005), which are responsible for cytotoxic T-lymphocyte-mediated tumor cytotoxicity. Moreover, depletion of Tgfbr2 promoted the expression of receptor KLRG1 and production of granzyme-B and IFN-γ (Zhang and Bevan, 2012). Furthermore, TGF-β has a significant role in the generation and expansion of Treg cells with powerful immunosuppressive potential (Wan and Flavell 2008). Further evidence demonstrated that TGF-β could induce CD25 and Foxp3 expression to convert native CD4+ T cells to Treg cells (Fu et al., 2004).
Chemokines
In addition to releasing immunosuppressive cytokines, MDSCs can produce high levels of CCL3, CCL4, and CCL5, which attract Treg cells into the TME via preferential expression of CCR5 on the Treg cell surface (Schlecker et al., 2012).
3.1.3 Suppression of T Cells by Disruption of Amino Acid Metabolism
As previously mentioned, MDSCs produce Arg-1, inducible nitric oxide synthase (iNOS), and ROS to impair T-cell-mediated immune responses. The underlying mechanisms have been determined by the exhaustion of certain amino acids and the generation of free radicals.
Arg1
Arg-1 hyperproduction of MDSCs in human cancer was first reported by Zea et al., in 2005 (Zea et al., 2005); they found that increased Arg activity was limited to a specific subset of CD11b+CD14−CD15+ cells in the peripheral blood of patients with metastatic renal cell carcinoma instead of macrophages or dendritic cells described in mouse models. Arg-1 utilizes L-arginine to produce urea, causing L-arginine deficiency in the TME. L-arginine deficiency leads to cell cycle arrest during the G0-G1 phase of T cells by upregulating cyclin D3 and cyclin-dependent kinase 4 (cdk4), resulting in decreased phosphorylation of Rb protein and low expression and binding of E2F1 (Rodriguez et al., 2007; Rodriguez et al., 2009). The absence of L-arginine also appears to downregulate T-cell receptor (TCR) expression by decreasing CD3ζ chain biosynthesis to induce T-cell dysfunction (Rodriguez et al., 2002).
iNOS and ROS
Similarly, L-arginine is also a substrate for iNOS to produce NO (Lee et al., 2003). In addition to depleting L-arginine, NO can suppress T cells by inhibiting JAK3/STAT5 activation (Bingisser et al., 1998), decreasing MHC class II molecule expression (Harari and Liao 2004), and inducing T-cell apoptosis (Vig et al., 2004). Additionally, NO can cooperate with ROS to form the reactive nitrogen species (RNS) peroxynitrite (Gabrilovich et al., 2012), which nitrates tyrosine residue in proteins involved in T-cell function. Lu et al. reported that lymphocyte-specific protein tyrosine kinase (LCK) was nitrated at Tyr394 by MDSCs as an initiating tyrosine kinase in the TCR signaling cascade (Feng et al., 2018). LCK nitration reduced IL-2 production to inhibit the proliferation and activation of T cells. Moreover, RNS nitrates the tyrosine of TCR to modify its conformational flexibility and affect its interaction with MHC class I molecules, thus causing the decreased response of CD8+ T cells to antigen-specific stimulation (Nagaraj et al., 2007). Additionally, RNS induces the nitration of CCL2 chemokines to inhibit antigen-specific cytotoxic T-cell trafficking into the tumor (Molon et al., 2011). Upregulated ROS have been identified in the MDSCs of many tumors (Ohl and Tenbrock 2018). Other studies have found that MDSCs with decreased ROS production failed to inhibit IFN-γ secretion and proliferation of antigen-specific CD8+ T cells (Corzo et al., 2009). In addition to direct toxicity, ROS might be involved in TCR CD3ζ expression (Otsuji et al., 1996), which limits the activation and expression of IFN-γ in T cells.
IDO1
The abnormal metabolisms of tryptophan and cystine induced by MDSCs also mediate T-cell suppression. MDSCs highly express indoleamine 2,3-dioxygenase 1 (IDO1), which is an enzyme that hydrolyzes tryptophan along the kynurenine pathway (Yu et al., 2013). A shortage of tryptophan causes T-cell proliferation arrest during the G1 phase of the cell cycle by stimulating general control nonderepressible 2 (GCN2) activation (Munn et al., 2005). Furthermore, GCN2 activation could reduce the expression of the TCR CD3ζ chain to inhibit antigen presentation (Fallarino et al., 2006). Metz et al. reported that the IDO-mediated catabolism of tryptophan also inhibited the mammalian target of rapamycin (mTOR) and protein kinase C (PKC), along with autophagy of effector T cells (Metz et al., 2012). Furthermore, IDO1 promotes the expansion of Treg cells and enhances their immunosuppressive functions to mediate immune tolerance (Curti et al., 2007). A recent study found that IDO1 knockout in myeloid cells increased ROS levels to aggravate graft-versus-host disease (Ju et al., 2021); however, whether IDO1 could suppress ROS production in tumors remains unclear. MDSCs also limit T-cell activation by consuming cystine in the TME (Srivastava et al., 2010).
Cystine depriving
It is well-known that cysteine is an essential amino acid for T-cell activation. Because of the lack of cystathionase and an intact Xc− transporter, T cells cannot import cystine or convert intracellular methionine to cysteine(Ishii et al., 2004). Antigen presentation cells (APCs), including dendritic cells and macrophages, can import extracellular cystine and export cysteine to the TME, which is utilized by T cells. However, MDSCs import cystine but do not export cysteine because they do not express alanine-serine-cysteine transporters. Therefore, MDSCs competitively import cystine with APCs and reduce the level of cysteine in the TME to impair the uptake of T cells.
3.1.4 Crosstalk Between MDSCs and Other Immune Cells
There are many other mechanisms that mediate T-cell suppression by MDSCs. Zheng et al. reported that the V-domain Ig suppressor of T-cell activation (VISTA) is highly expressed on MDSCs in the peripheral blood of patients with acute myeloid leukemia (Wang et al., 2018). VISTA knockdown in MDSCs impairs MDSC-mediated inhibition of CD8 T-cell activity. Recently, it was found that MDSCs could release a large number of exosomes to induce exhaustion and apoptosis of CD8+ T cells by increasing ROS production (Rashid et al., 2021).
NK cells
NK cells play important roles in anti-tumor immune response though directly killing tumor cells and indirectly activating Th1 immunity. Li et al reported that coculture with MDSCs induced anergy of NK cells, including decreased cytotoxicity, reduced production of IFN-γ, and downregulated expression of NKG2D (Li et al., 2009). They also found that the anergy of NK cells induced by MDSCs was dependent on membrane-bound TGF-β1, implying in a cell-cell contact manner. Additionally, another study showed that MDSCs could downregulate expression of CD247 on the surface of NK cells to inhibit their development and cytotoxicity (Vaknin et al., 2008).
DCs
As one of professional antigen-presenting cells (APCs), DCs are vital in adaptive immunity to suppress tumor progression. Emerging evidences have shown that MDSCs-DCs crosstalk contributed to DCs dysfunction. In tumor-bearing mouse model, the number of mature DCs decreased proportionately to the increasing number of MDSCs, which might be due to their competitively differentiation from common progenitor cells (Sinha et al., 2007). In addition, MDSCs might inhibit DCs maturation to induce immune tolerance and evasion. It has been reported that MDSCs-producing VEGF and IL-10 could downregulate expression of major histocompatibility complex (MHC) II and co-stimulators on DCs by activating STAT3 signaling (Heim et al., 2014). In another study, researchers found that MDSCs could suppress the process of antigen capture and the migration of immature DC to secondary lymphoid organs, which were essential for DCs maturation (Greifenberg et al., 2009). Moreover, MDSCs have been reported to alter cytokine production of DCs, including decreased secretion of IL-12 and increased secretion of IL-23 (Hu et al., 2011). These alterations might transform DCs from anti-tumor cells into pro-tumor cells by driving the proliferation and inflammatory function of Th17 cells (Langrish et al., 2005).
Macrophages
Macrophage are also one of the professional APCs to facilitate Th1 cells-mediated anti-tumor immunity. MDSCs have been demonstrated to alter the cytokine production, phenotype and antigen-presenting capacity of macrophages. It has been reported that IL-10 produced by MDSCs could decrease the secretion of IL-6, IL-12, and tumor necrosis factor-α (TNF-α) of macrophages, which remarkably suppress their anti-tumor activity (Beury et al., 2014). Moreover, Rosenberg et al found that MDSCs might switch macrophages phenotype from anti-tumor M1 subtype into pro-tumor M2 subtype, giving rise to so-called “tumor associated macrophages” (TAMs) (Ostrand-Rosenberg et al., 2012). Additionally, coculture with MDSCs could downregulate expression of MHC II molecular to interfere with antigen-presenting process of macrophages (Shin et al., 2006), resulting in immune tolerance or immune evasion.
Treg cells
Treg cells are also potent suppressor of T cells proliferation and cytotoxicity. As mentioned above, IL-10 and TGF-β could induce generation and expansion of Treg cells through increasing expression of CD25 and Foxp3. In addition, a series of chemokines produced by MDSCs, such as CCL3, CCL4, and CCL5, could promote recruitment of Treg cells into TME.
3.2 Non-immunological Functions of MDSCs in Tumor Progression
In general, tumor cells produce certain growth factors and chemokines to recruit MDSCs into the TME. In addition to suppressing T-cell-mediated immunity, MDSCs promote tumor progression and metastasis by mediating stemness of tumor cells, angiogenesis, and degradation of the extracellular matrix (ECM).
3.2.1 Effects on EMT and Stemness
Numerous studies have indicated that MDSCs mediate the epithelial-to-mesenchymal transition (EMT) of tumor cells in many cancers (Zhu H et al., 2017; Pang et al., 2020). EMT is a critical biological process in the formation of cancer stem cells (CSCs), which harbor stem cell properties such as self-renewal, multi-lineage differentiation, and tumorigenicity (Dave et al., 2012). Many immunosuppressive factors produced by MDSCs, such as TGF-β (Katsuno et al., 2013), VEGF (Oka et al., 2007), IL-10 (Yang et al., 2019), and IL-6 (Kim et al., 2013), have been shown to induce EMT and stemness in various tumor cells. Liu et al. reported that CXCR2+ MDSCs induce EMT in breast cancer cells by secreting IL-6 (Zhu H et al., 2017). In a melanoma model, MDSCs promoted EMT via TGF-β, epidermal growth factor, and hepatocyte growth factor signaling pathways (Schlegel et al., 2015). In 2013, Zou et al. first reported the interaction between MDSCs and CSCs (Cui et al., 2013). They found that MDSCs triggered miRNA101 expression in ovarian cancer cells to repress the co-repressor gene C-terminal binding protein-2 (CtBP2), which directly targets stem cell core genes, resulting in increased cancer cell stemness and increased metastatic and tumorigenic potential. Another study indicated that MDSCs increased ALDHhigh CSCs in ovarian cancer by producing PEG2. Moreover, PEG2 produced by MDSCs upregulated PD-L1 expression in ovarian cancer cells (Komura et al., 2020). Linehan et al. demonstrated that MDSCs significantly increased the frequency of ALDH1Bright CSCs in a mouse model of pancreatic cancer through activation of the STAT3 pathway (Panni et al., 2014). In addition to inducing EMT and stemness, MDSCs upregulate caspase-1 to directly promote the proliferation of multiple squamous carcinoma cells in vitro and in vivo (Zeng et al., 2018).
3.2.2 Effects on Angiogenesis
MDSCs have been proven to facilitate angiogenesis in tumor progression through many different mechanisms. MDSCs produce high levels of VEGF (Shojaei et al., 2009), which is the most important cytokine involved in angiogenesis. VEGF binds to its receptor (VEGFR) on adjacent epithelial cells to promote neovascularization by activating the JAK2/STAT3 pathway. Interestingly, MDSCs in TME also express high levels of VEGFR2 (Min et al., 2017), which may be activated by VEGF secreted by tumor cells or themselves to produce more VEGF. Therefore, the VEGF/VEGFR pathway establishes a positive feedback loop in MDSCs to maintain their angiogenic activity. Moreover, MDSCs are able to produce an abundance of proteolytic enzymes, such as matrix metalloproteinases (MMPs) (Zhang et al., 2020), which are involved in tumor metastasis by degrading the ECM. MMPs are also key regulators of angiogenesis. Yang et al. reported that MMP9 was highly secreted by Gr-1+CD11b+ MDSCs, and that deletion of MMP9 decreased vascular density, vascular maturation, and tumor growth (Yang et al., 2004). Additionally, other factors contribute to MDSC-induced angiogenesis, including Bombina variegata peptide 8 (Bv8), TGF-β, fibroblast growth factor 2, and IL-1β. Ferrara et al. reported that Gr-1+CD11b+ myeloid cells upregulated the expression of Bv8 to promote tumor angiogenesis (Shojaei and Ferrara, 2008). As mentioned, MDSCs can produce TGF-β. TGF-β induces tumor angiogenesis by activating fibroblasts to produce ECM adhesion and stimulating tube formation by endothelial cells (van Meeteren et al., 2011). Further studies have indicated that TGF-β may mediate acquired resistance to anti-VEGF therapy (Aguilera et al., 2014). Yu et al. reported that STAT3-activated MDSCs upregulated the expression of basic fibroblast growth factor 2 and IL-1β (Kujawski et al., 2008), which are critical for tumor-derived MDSC–mediated angiogenesis.
Some RNA contents of MDSC-derived exosomes also contribute to tumor angiogenesis. Zhang et al. showed that MDSC miR-126a rescues doxorubicin-induced MDSC death in a S100A8/A9-dependent manner and promotes tumor angiogenesis (Deng et al., 2017). In another study, miR-9 contained in MDSC-derived exosomes was found to promote tumor angiogenesis by reprogramming endothelial cells (Baroni et al., 2016). However, other exosomes involved in MDSC-mediated angiogenesis require further study.
3.2.3 Effects on Pre-metastatic Niche Formation
Clinical data have proven that MDSCs positively correlated with distant metastasis in various tumors, including NSCLC, breast cancer, melanoma, prostate cancer and so on (Huang et al., 2013; Yu et al., 2013; Weide et al., 2014; Hossain et al., 2015). Besides the promotion of EMT and angiogenesis, MDSCs have been demonstrated to the formation of pre-metastatic niche. Wang et al. reported that CXCL1 produced by TAMs in tumor microenvironment could recruit CXCR2+ MDSCs to liver and establish a pre-metastatic niche that expedited liver metastasis (Wang et al., 2017). In another mouse model of breast cancer, CCL2 secreted by tumor cells could recruit MDSCs to the lung and result in formation of pre-metastatic niche of lung metastases (Sceneay et al., 2013). These functions might be explained by the degradation of the extracellular matrix (ECM) to make the local microenvironment more permissive for the seeding of circulating tumor cells (Wang et al., 2019b). Moreover, MDSCs-produced cytokines and chemokines, such as TGF-β, VEGFA, S100A8/A9, and MMP-9, might play important role in pre-metastatic niche formation through facilitating angiogenesis and tumor cell invasion (Shojaei et al., 2009).
4 MDSCs Act as a Negative Biomarker of Immunotherapy
The emergence of immunotherapy has achieved a great breakthrough in cancer therapy. In particular, T cell-based immunotherapy such as immune checkpoint blockers (ICBs), and the infiltration and function of cytotoxic T lymphocytes (CTLs) directly determines the efficacy of immunotherapy. Accumulating evidence has shown that MDSCs impair the patient response and survival, to clarify the role of MDSCs in immunotherapy has profound significance.
Several clinical studies have identified MDSCs as predictive biomarkers of poor prognosis for immunotherapy in many cancers. It has been reported that the responders to ipilimumab have lower frequency of MDSCs in peripheral blood than the non-responders in patients with melanoma (Meyer et al., 2014). The result of IMmotion150 identified that high myeloid inflammation gene signature expression (MyeloidHigh) was associated with reduced PFS in atezolizumab-treated patients with metastatic renal cell carcinoma (mRCC) (McDermott et al., 2018). Moreover, MDSCs have been shown to predict the efficacy of anti-PD-1 immunotherapy. Youn et al. demonstrated that the ratio of Treg cells to Lox-1+ MDSCs was positively correlated with anti-PD-1 efficacy in non-small cell lung cancer (NSCLC) (Kim et al., 2019). Another study found that the ratio of NK cells to Lox-1+ PMN-MDSCs was also an ideal biomarker for PD-1 antibody treatment of NSCLC (Youn et al., 2020). Recently, low frequency of MDSCs in the peripheral blood has been identified to be associated with longer progression free survival and overall survival in NSCLC patients after PD-1 treatment (Koh et al., 2020). Therefore, MDSCs might be a potential tool for cancer treatment or for improving the efficacy of immunotherapy.
5 Strategies Targeting MDSCs for Optimizing Cancer Treatment
To date, several clinical trials have been established to specifically target MDSCs to inhibit proliferation, decrease the expression of immunosuppressive mediators, block recruitment, and promote differentiation.
5.1 Blockade of MDSCs’ Recruitment
MDSCs express high levels of certain chemokine receptors, such as CXCR1, CXCR2, CXCR4, CCR2, and CCR5. Therefore, MDSCs can be recruited into the TME by corresponding chemokines from tumor cells and immune cells, including CXCL5, CXCL8, CXCL12, CCL2, and CCL5. Many preclinical and clinical trials have attempted to block MDSC recruitment with antagonists of these chemokine receptors. In 2016, a phase Ib study investigated the safety and efficacy of PF-04136309 (a CCR2 antagonist) and FOLFIRINOX for locally advanced and borderline resectable pancreatic cancer (Nywening et al., 2016). The results showed that the ORR and DCR for the combined group were 49 and 97%, respectively, but they were only 0 and 80%, respectively, for the chemotherapy alone group. Although the authors speculated that the regression of tumors was attributable to decreased TAMs by CCR2 blockade, the contribution of reduced MDSCs cannot be ignored. Another CCR2 antagonist, 747, has been proven to exhibit anti-cancer properties alone and potentiate the anti-tumor efficacy of sorafenib in a hepatocellular carcinoma model (Yao et al., 2017); however, further clinical studies are needed. A phase Ib study was established by Goldstein et al. to investigate the efficacy of the CXCR1/2 inhibitor, reparixin, in combination with weekly paclitaxel for metastatic HER2 negative breast cancer (Schott et al., 2017). They reported an ORR of 27.8% (5/18 patients). CXCR4 antagonists and antibodies are designed to treat acute myeloid leukemia, multiple myeloma (MM), and solid tumors. Thornton et al. published the results of a phase I trial of LY2510924, a CXCR4 peptide antagonist, for patients with solid tumors (Galsky et al., 2014). For the 45 patients in this trial, the DCR was 20% and the ORR was not observed. Phase II and phase III trials are required to enroll more patients. Recently, the efficacy of a CXCR4 antibody, ulocuplumab (BMS-936564), in combination with lenalidomide or bortezomib plus dexamethasone for MM was studied. The results showed that the ORR of combined therapy was 55.2% and the DCR was 72.4% (Ghobrial et al., 2020).
5.2 Inhibition of MDSCs’ Activation
Inducing immature MDSCs into mature myeloid cells may be a potential strategy for treating cancer. All-trans retinoic acid (ATRA) has been recognized as an ideal inducer of differentiation and has been widely used for many hematopoietic tumors (Mirza et al., 2006; Iclozan et al., 2013). Moreover, McCarter et al. found that ATRA could decrease the frequency and suppressive functions of MDSCs and increase the frequency of circulating mature myeloid cells in the peripheral blood of patients with melanoma (Tobin et al., 2018). However, the efficacy of ATRA alone or in combination with ipilimumab for melanoma patients requires further study. Furthermore, 1,25 dihydroxy vitamin D (1,25(OH)2D), the active form of vitamin D, is involved in cell differentiation. The use of 1,25(OH)2D has been proven to block tumor progression and prolong disease-free survival by increasing CD4+ and CD8+ T-cell infiltration in patients with head and neck squamous cell carcinoma (Makitie et al., 2020). Other studies reported that MDSCs in the TME expressed high levels of vitamin D receptor, and that 1,25(OH)2D treatment could significantly inhibit their suppressive functions (Fleet et al., 2020). However, the effects of 1,25(OH)2D on MDSC differentiation require further study.
5.3 Depletion of MDSCs’ Expansion
The CSF-1/CSF-1R pathway has a key role in MDSC proliferation, and CSF-1R inhibitor treatment has resulted in exciting results. Tyner et al. identified GW-2580, a CSF-1R inhibitor, as a potential anti-tumor agent for acute myeloid leukemia by decreasing CD33+ MDSC infiltration (Edwards et al., 2019). Imatinib, a TKI approved for the treatment of chronic myeloid leukemia and gastrointestinal stromal tumors, was also found to target GSF1R. Imatinib has been shown to significantly reduce the frequency of circulating G-MDSCs in patients with chronic myeloid leukemia (Giallongo et al., 2018). In 2019, Rugo et al. published the results of a phase Ib study of the combination of pexidartinib (another CSF-1R inhibitor) and paclitaxel for patients with advanced solid tumors (Wesolowski et al., 2019). They found that of 38 evaluable patients, 1 (3%) had a complete response, 5 (13%) had a partial response, and 13 (34%) had stable disease. The overall response rate (ORR) and disease control rate (DCR) were 16 and 55%, respectively. They also found that combined therapy reduced CD14dim/CD16+ monocyte levels by 57–100% in peripheral blood; however, the effect on MDSCs was not studied further.
5.4 Target on MDSCs’ Metabolic Products
As mentioned, Arg-1 and iNOS are critical factors in MDSC-mediated immune suppression. A phase I study of the arginase inhibitor INCB001158 (1,158) alone and in combination with pembrolizumab for patients with solid tumors revealed that the ORR and DCR for the 1,158 alone group and the combined group were 3 and 28% and 6 and 37%, respectively (Naing et al., 2019). Recently, a phase I/II study of INCB001158 plus chemotherapy for patients with advanced biliary tract cancers reported that the ORR was 24% (8/33 patients; 95% confidence interval [CI], 11.1–42.3%), the DCR was 67%, and the median progression-free survival was 8.5 months (95% CI, 5.7–10.1 months) (Javle et al., 2021). Similarly, a phase I/II clinical trial performed to study the efficacy of the iNOS inhibitor NG-monomethyl-L-arginine (L-NMMA) combined with docetaxel treatment reported that the ORR was 22.2% for patients with triple-negative breast cancer (Chung et al., 2019). These results suggest that inhibitors of Arg-1 and iNOS combined with immunotherapy or chemotherapy might be better choices for some solid cancers (Table 1).
In fact, reductions in MDSC frequency and function have been demonstrated during regular anti-tumor therapies, including chemotherapy, targeted therapy, immunotherapy, and combined therapy. However, other studies and our previous studies have shown that radiotherapy could induce an increase in MDSCs in the TME and spleen (van Meir et al., 2017; Cheng et al., 2021). Regarding chemotherapeutic agents, 5-fluorouracil (5-FU), gemcitabine, and pemetrexed could decrease MDSC accumulation in various cancers. However, Baniyash et al. reported that CPT-11 (irinotecan) increased the abundance and immunosuppressive features of MDSCs in colorectal cancer by blocking MDSC apoptosis and myeloid cell differentiation (Kanterman et al., 2014). Anti-angiogenic drugs, such as bevacizumab and some small molecular tyrosine kinase inhibitors (TKIs), can also reduce MDSC accumulation. Kotsakis et al. found that bevacizumab-based chemotherapy significantly reduced the percentage of MDSCs compared with non-bevacizumab regimens in the peripheral blood of patients with NSCLC (Koinis et al., 2016). In a previous study, nilotinib, dasatinib, and sorafenib, but not sunitinib, inhibited the differentiation of MDSCs and promoted their immunosuppressive capacity (Heine et al., 2016). However, in a model of mRCC, both sunitinib alone and in combination with PD-1 antibody significantly reduced a large proportion of G-MDSCs and M-MDSCs (Rayman et al., 2015). And another study also found that sunitinib treatment reduces intratumoral MDSC content, especially significantly shrinkage of G-MDSC in RCC patients (Guislain et al., 2015). Aerts et al. found that axitinib with αCTLA-4 increased the number of MDSCs but decreased their suppressive capacity in a melanoma brain metastasis model (Du Four et al., 2016). Olaparib, targeting poly (ADP-ribose) polymerase (PARP), also suppressed MDSC recruitment by dampening the CXCL12/CXCR4 pathway (Sun et al., 2021). Although epidermal growth factor receptor TKIs, including gefitinib and osimertinib, were found to increase the level of MDSCs in NSCLC (Jia et al., 2019), whether their suppressive functions are modified requires further study. Additionally, immune checkpoint inhibitors (anti-PD-1, anti-PD-L1, and anti-CTLA-4) have been reported to reduce the frequency of MDSCs and decrease the production of suppressive factors and chemokines. Grivas et al. found that PD-L1 antibody (atezolizumab/avelumab) and PD-1 antibody (pembrolizumab) were correlated with a decreased percentage of PD-L1+ MDSCs in patients with metastatic urothelial carcinoma (Tzeng et al., 2018). Kiessling et al. reported that ipilimumab, a CTLA-4 antibody, reduced the infiltration of MDSCs and iNOS expression in melanoma patients (de Coana et al., 2017).
6 Conclusion and Perspectives
Emerging evidence has implied the suppressive role of MDSCs in pathological conditions including tumor progression. Although we have a better knowledge of the origin and development of MDSC, the genomic and metabolic differentiation mechanisms are still unclear. Recent advances in genomic of high-resolution and metabolic technologies may allow for further study development and differentiation. In consideration of the pro-tumor contribution of MDSCs from immunological and non-immunological view in tumor progression and metastasis just as we have discussed in this review, to clarify their role in anti-tumor treatment especially immunotherapy is particularly important. In clinic, MDSCs are negative prognostic biomarkers for multiple tumors, and may mediate acquired resistance to immunotherapy. Although many clinical trials have attempted to target MDSCs for improving clinical benefit, their efficacy is far from our expectations. The major obstacle is to understand the complex mechanisms and target MDSCs selectively. Therefore, more clinical studies and basic research involving targeting MDSCs alone or in combination with immunotherapy are needed.
Author Contributions
J-NC and Y-XY drafted the manuscript. QJ and BZ revised manuscript.
Conflict of Interest
The authors declare that the research was conducted in the absence of any commercial or financial relationships that could be construed as a potential conflict of interest.
Publisher’s Note
All claims expressed in this article are solely those of the authors and do not necessarily represent those of their affiliated organizations, or those of the publisher, the editors and the reviewers. Any product that may be evaluated in this article, or claim that may be made by its manufacturer, is not guaranteed or endorsed by the publisher.
Acknowledgments
The authors would like to thank Dr. Tao Yang for critical review of the manuscript. We also thank all lab members for helpful discussions.
References
Aguilera, K. Y., Rivera, L. B., Hur, H., Carbon, J. G., Toombs, J. E., Goldstein, C. D., et al. (2014). Collagen Signaling Enhances Tumor Progression after Anti-VEGF Therapy in a Murine Model of Pancreatic Ductal Adenocarcinoma. Cancer Res. 74 (4), 1032–1044. doi:10.1158/0008-5472.can-13-2800
Bah, I., Kumbhare, A., Nguyen, L., McCall, C. E., and El Gazzar, M. (2018). IL-10 Induces an Immune Repressor Pathway in Sepsis by Promoting S100A9 Nuclear Localization and MDSC Development. Cell Immunol. 332, 32–38. doi:10.1016/j.cellimm.2018.07.003
Baroni, S., Romero-Cordoba, S., Plantamura, I., Dugo, M., D’Ippolito, E., Cataldo, A., et al. (2016). Exosome-Mediated Delivery of miR-9 Induces Cancer-Associated Fibroblast-Like Properties in Human Breast Fibroblasts. Cell Death Dis 7 (7), e2312. doi:10.1038/cddis.2016.224
Beury, D. W., Parker, K. H., Nyandjo, M., Sinha, P., Carter, K. A., and Ostrand-Rosenberg, S. (2014). Cross-Talk Among Myeloid-Derived Suppressor Cells, Macrophages, and Tumor Cells Impacts the Inflammatory Milieu of Solid Tumors. J. Leukoc. Biol. 96 (6), 1109–1118. doi:10.1189/jlb.3a0414-210r
Bingisser, R. M., Tilbrook, P. A., Holt, P. G., and Kees, U. R. (1998). Macrophage-Derived Nitric Oxide Regulates T Cell Activation via Reversible Disruption of the Jak3/STAT5 Signaling Pathway. J. Immunol. 160 (12), 5729–5734.
Chen, C.-H., Seguin-Devaux, C., Burke, N. A., Oriss, T. B., Watkins, S. C., Clipstone, N., et al. (2003). Transforming Growth Factor β Blocks Tec Kinase Phosphorylation, Ca2+ Influx, and NFATc Translocation Causing Inhibition of T Cell Differentiation. J. Exp. Med. 197 (12), 1689–1699. doi:10.1084/jem.20021170
Chen, J., Ye, Y., Liu, P., Yu, W., Wei, F., Li, H., et al. (2017). Suppression of T Cells by Myeloid-Derived Suppressor Cells in Cancer. Hum. Immunol. 78 (2), 113–119. doi:10.1016/j.humimm.2016.12.001
Cheng, J. N., Luo, W., Sun, C., Jin, Z., Zeng, X., Alexander, P. B., et al. (2021). Radiation-Induced Eosinophils Improve Cytotoxic T Lymphocyte Recruitment and Response to Immunotherapy. Sci. Adv. 7 (5), eabc7609. doi:10.1126/sciadv.abc7609
Chikamatsu, K., Sakakura, K., Toyoda, M., Takahashi, K., Yamamoto, T., and Masuyama, K. (2012). Immunosuppressive Activity of CD14+ HLA-DR− Cells in Squamous Cell Carcinoma of the Head and Neck. Cancer Sci. 103 (6), 976–983. doi:10.1111/j.1349-7006.2012.02248.x
Chung, A., Ensor, J., Darcourt, J., Belcheva, A., Patel, T., Chang, J., et al. (2019). Abstract OT3-08-01: A Phase Ib/II Clinical Trial Investigating the Efficacy of Nitric Oxide Deprivation and Docetaxel in Triple Negative Breast Cancer. Cancer Res. 79 (4 Suppl. ment), OT3-08-01-OT03-08-01. doi:10.1158/1538-7445.sabcs18-ot3-08-01
Corzo, C. A., Cotter, M. J., Cheng, P., Cheng, F., Kusmartsev, S., Sotomayor, E., et al. (2009). Mechanism Regulating Reactive Oxygen Species in Tumor-Induced Myeloid-Derived Suppressor Cells. J. Immunol. 182 (9), 5693–5701. doi:10.4049/jimmunol.0900092
Cui, T. X., Kryczek, I., Zhao, L., Zhao, E., Kuick, R., Roh, M. H., et al. (2013). Myeloid-Derived Suppressor Cells Enhance Stemness of Cancer Cells by Inducing microRNA101 and Suppressing the Corepressor CtBP2. Immunity 39 (3), 611–621. doi:10.1016/j.immuni.2013.08.025
Curti, A., Pandolfi, S., Valzasina, B., Aluigi, M., Isidori, A., Ferri, E., et al. (2007). Modulation of Tryptophan Catabolism by Human Leukemic Cells Results in the Conversion of CD25− into CD25+ T Regulatory Cells. Blood 109 (7), 2871–2877. doi:10.1182/blood-2006-07-036863
Dardalhon, V., Anderson, A. C., Karman, J., Apetoh, L., Chandwaskar, R., Lee, D. H., et al. (2010). Tim-3/Galectin-9 Pathway: Regulation of Th1 Immunity through Promotion of CD11b+Ly-6G+ Myeloid Cells. J. Immunol. 185 (3), 1383–1392. doi:10.4049/jimmunol.0903275
Dave, B., Mittal, V., Tan, N. M., and Chang, J. C. (2012). Epithelial-Mesenchymal Transition, Cancer Stem Cells and Treatment Resistance. Breast Cancer Res. 14 (1), 202. doi:10.1186/bcr2938
de Coaña, Y. P., Wolodarski, M., Poschke, I., Yoshimoto, Y., Yang, Y., Nyström, M., et al. (2017). Ipilimumab Treatment Decreases Monocytic MDSCs and Increases CD8 Effector Memory T Cells in Long-Term Survivors with Advanced Melanoma. Oncotarget 8 (13), 21539–21553. doi:10.18632/oncotarget.15368
Deng, Z., Rong, Y., Teng, Y., Zhuang, X., Samykutty, A., Mu, J., et al. (2017). Exosomes miR-126a Released from MDSC Induced by DOX Treatment Promotes Lung Metastasis. Oncogene 36 (5), 639–651. doi:10.1038/onc.2016.229
Du Four, S., Maenhout, S. K., Niclou, S. P., Thielemans, K., Neyns, B., and Aerts, J. L. (2016). Combined VEGFR and CTLA-4 Blockade Increases the Antigen-Presenting Function of Intratumoral DCs and Reduces the Suppressive Capacity of Intratumoral MDSCs. Am. J. Cancer Res. 6 (11), 2514–2531.
Edwards, D. K., Watanabe-Smith, K., Rofelty, A., Damnernsawad, A., Laderas, T., Lamble, A., et al. (2019). CSF1R Inhibitors Exhibit Antitumor Activity in Acute Myeloid Leukemia by Blocking Paracrine Signals from Support Cells. Blood 133 (6), 588–599. doi:10.1182/blood-2018-03-838946
Fallarino, F., Grohmann, U., You, S., McGrath, B. C., Cavener, D. R., Vacca, C., et al. (2006). The Combined Effects of Tryptophan Starvation and Tryptophan Catabolites Down-Regulate T Cell Receptor ζ-Chain and Induce a Regulatory Phenotype in Naive T Cells. J. Immunol. 176 (11), 6752–6761. doi:10.4049/jimmunol.176.11.6752
Feng, S., Cheng, X., Zhang, L., Lu, X., Chaudhary, S., Teng, R., et al. (2018). Myeloid-Derived Suppressor Cells Inhibit T Cell Activation through Nitrating LCK in Mouse Cancers. Proc. Natl. Acad. Sci. USA 115 (40), 10094–10099. doi:10.1073/pnas.1800695115
Fleet, J. C., Burcham, G. N., Calvert, R. D., Elzey, B. D., and Ratliff, T. L. (2020). 1α, 25 Dihydroxyvitamin D (1,25(OH)2D) Inhibits the T Cell Suppressive Function of Myeloid Derived Suppressor Cells (MDSC). J. Steroid Biochem. Mol. Biol. 198, 105557. doi:10.1016/j.jsbmb.2019.105557
Fleming, V., Hu, X., Weber, R., Nagibin, V., Groth, C., Altevogt, P., et al. (2018). Targeting Myeloid-Derived Suppressor Cells to Bypass Tumor-Induced Immunosuppression. Front. Immunol. 9, 398. doi:10.3389/fimmu.2018.00398
Fu, S., Zhang, N., Yopp, A. C., Chen, D., Mao, M., Chen, D., et al. (2004). TGF-Beta Induces Foxp3 + T-Regulatory Cells from CD4 + CD25 - Precursors. Am. J. Transpl. 4 (10), 1614–1627. doi:10.1111/j.1600-6143.2004.00566.x
Gabrilovich, D. I., Ostrand-Rosenberg, S., and Bronte, V. (2012). Coordinated Regulation of Myeloid Cells by Tumours. Nat. Rev. Immunol. 12 (4), 253–268. doi:10.1038/nri3175
Galsky, M. D., Vogelzang, N. J., Conkling, P., Raddad, E., Polzer, J., Roberson, S., et al. (2014). A Phase I Trial of LY2510924, a CXCR4 Peptide Antagonist, in Patients with Advanced Cancer. Clin. Cancer Res. 20 (13), 3581–3588. doi:10.1158/1078-0432.ccr-13-2686
Ghobrial, I. M., Liu, C.-J., Redd, R. A., Perez, R. P., Baz, R., Zavidij, O., et al. (2020). A Phase Ib/II Trial of the First-In-Class Anti-CXCR4 Antibody Ulocuplumab in Combination with Lenalidomide or Bortezomib Plus Dexamethasone in Relapsed Multiple Myeloma. Clin. Cancer Res. 26 (2), 344–353. doi:10.1158/1078-0432.ccr-19-0647
Giallongo, C., Parrinello, N. L., La Cava, P., Camiolo, G., Romano, A., Scalia, M., et al. (2018). Monocytic Myeloid-Derived Suppressor Cells as Prognostic Factor in Chronic Myeloid Leukaemia Patients Treated with Dasatinib. J. Cel Mol Med 22 (2), 1070–1080. doi:10.1111/jcmm.13326
Gorelik, L., Constant, S., and Flavell, R. A. (2002). Mechanism of Transforming Growth Factor β-Induced Inhibition of T Helper Type 1 Differentiation. J. Exp. Med. 195 (11), 1499–1505. doi:10.1084/jem.20012076
Greifenberg, V., Ribechini, E., Rößner, S., and Lutz, M. B. (2009). Myeloid-Derived Suppressor Cell Activation by Combined LPS and IFN-γ Treatment Impairs DC Development. Eur. J. Immunol. 39 (10), 2865–2876. doi:10.1002/eji.200939486
Guislain, A., Gadiot, J., Kaiser, A., Jordanova, E. S., Broeks, A., Sanders, J., et al. (2015). Sunitinib Pretreatment Improves Tumor-Infiltrating Lymphocyte Expansion by Reduction in Intratumoral Content of Myeloid-Derived Suppressor Cells in Human Renal Cell Carcinoma. Cancer Immunol. Immunother. 64 (10), 1241–1250. doi:10.1007/s00262-015-1735-z
Harari, O., and Liao, J. (2004). Inhibition of MHC II Gene Transcription by Nitric Oxide and Antioxidants. Curr. Pharm. Des. 10 (8), 893–898. doi:10.2174/1381612043452893
Hart, K. M., Bak, S. P., Alonso, A., and Berwin, B. (2009). Phenotypic and Functional Delineation of Murine CX3CR1+ Monocyte-Derived Cells in Ovarian Cancer. Neoplasia 11 (6), 564–573. doi:10.1593/neo.09228
Hart, K. M., Byrne, K. T., Molloy, M. J., Usherwood, E. M., and Berwin, B. (2011). IL-10 Immunomodulation of Myeloid Cells Regulates a Murine Model of Ovarian Cancer. Front. Immun. 2, 29. doi:10.3389/fimmu.2011.00029
Heim, C. E., Vidlak, D., Scherr, T. D., Kozel, J. A., Holzapfel, M., Muirhead, D. E., et al. (2014). Myeloid-derived Suppressor Cells Contribute to Staphylococcus A Orthopedic Biofilm Infection. J. Immunol. 192 (8), 3778–3792. doi:10.4049/jimmunol.1303408
Heine, A., Schilling, J., Grünwald, B., Krüger, A., Gevensleben, H., Held, S. A. E., et al. (2016). The Induction of Human Myeloid Derived Suppressor Cells through Hepatic Stellate Cells Is Dose-Dependently Inhibited by the Tyrosine Kinase Inhibitors Nilotinib, Dasatinib and Sorafenib, but Not Sunitinib. Cancer Immunol. Immunother. 65 (3), 273–282. doi:10.1007/s00262-015-1790-5
Heo, Y.-J., Joo, Y.-B., Oh, H.-J., Park, M.-K., Heo, Y.-M., Cho, M.-L., et al. (2010). IL-10 Suppresses Th17 Cells and Promotes Regulatory T Cells in the CD4+ T Cell Population of Rheumatoid Arthritis Patients. Immunol. Lett. 127 (2), 150–156. doi:10.1016/j.imlet.2009.10.006
Hossain, D. M. S., Pal, S. K., Moreira, D., Duttagupta, P., Zhang, Q., Won, H., et al. (2015). TLR9-Targeted STAT3 Silencing Abrogates Immunosuppressive Activity of Myeloid-Derived Suppressor Cells from Prostate Cancer Patients. Clin. Cancer Res. 21 (16), 3771–3782. doi:10.1158/1078-0432.ccr-14-3145
Hu, C.-E., Gan, J., Zhang, R.-D., Cheng, Y.-R., and Huang, G.-J. (2011). Up-Regulated Myeloid-Derived Suppressor Cell Contributes to Hepatocellular Carcinoma Development by Impairing Dendritic Cell Function. Scand. J. Gastroenterol. 46 (2), 156–164. doi:10.3109/00365521.2010.516450
Huang, A., Zhang, B., Wang, B., Zhang, F., Fan, K.-X., and Guo, Y.-J. (2013). Increased CD14+HLA-DR-/Low Myeloid-Derived Suppressor Cells Correlate with Extrathoracic Metastasis and Poor Response to Chemotherapy in Non-Small Cell Lung Cancer Patients. Cancer Immunol. Immunother. 62 (9), 1439–1451. doi:10.1007/s00262-013-1450-6
Iclozan, C., Antonia, S., Chiappori, A., Chen, D.-T., and Gabrilovich, D. (2013). Therapeutic Regulation of Myeloid-Derived Suppressor Cells and Immune Response to Cancer Vaccine in Patients with Extensive Stage Small Cell Lung Cancer. Cancer Immunol. Immunother. 62 (5), 909–918. doi:10.1007/s00262-013-1396-8
Ishii, I., Akahoshi, N., Yu, X. N., Kobayashi, Y., Namekata, K., Komaki, G., et al. (2004). Murine Cystathionine Gamma-Lyase: Complete cDNA and Genomic Sequences, Promoter Activity, Tissue Distribution and Developmental Expression. Biochem. J. 381 (Pt 1), 113–123. doi:10.1042/BJ20040243
Javle, M. M., Bridgewater, J. A., Gbolahan, O. B., Jungels, C., Cho, M. T., Papadopoulos, K. P., et al. (2021). A Phase I/II Study of Safety and Efficacy of the Arginase Inhibitor INCB001158 Plus Chemotherapy in Patients (Pts) with Advanced Biliary Tract Cancers. J. Clin. Oncol. 39 (3_Suppl. l), 311. doi:10.1200/jco.2021.39.3_suppl.311
Jia, Y., Li, X., Jiang, T., Zhao, S., Zhao, C., Zhang, L., et al. (2019). EGFR‐targeted Therapy Alters the Tumor Microenvironment in EGFR‐driven Lung Tumors: Implications for Combination Therapies. Int. J. Cancer 145 (5), 1432–1444. doi:10.1002/ijc.32191
Jiang, H., Gebhardt, C., Umansky, L., Beckhove, P., Schulze, T. J., Utikal, J., et al. (2015). Elevated Chronic Inflammatory Factors and Myeloid-Derived Suppressor Cells Indicate Poor Prognosis in Advanced Melanoma Patients. Int. J. Cancer 136 (10), 2352–2360. doi:10.1002/ijc.29297
Ju, J. M., Nam, G., Lee, Y. K., Jung, M., Chang, H., Kim, W., et al. (2021). Ido1 Scavenges Reactive Oxygen Species in Myeloid-Derived Suppressor Cells to Prevent Graft-Versus-Host Disease. Proc. Natl. Acad. Sci. U S A. 118 (10), e2011170118. doi:10.1073/pnas.2011170118
Kanterman, J., Sade-Feldman, M., Biton, M., Ish-Shalom, E., Lasry, A., Goldshtein, A., et al. (2014). Adverse Immunoregulatory Effects of 5FU and CPT11 Chemotherapy on Myeloid-Derived Suppressor Cells and Colorectal Cancer Outcomes. Cancer Res. 74 (21), 6022–6035. doi:10.1158/0008-5472.can-14-0657
Katsuno, Y., Lamouille, S., and Derynck, R. (2013). TGF-β Signaling and Epithelial-Mesenchymal Transition in Cancer Progression. Curr. Opin. Oncol. 25 (1), 76–84. doi:10.1097/cco.0b013e32835b6371
Kim, S.-Y., Kang, J. W., Song, X., Kim, B. K., Yoo, Y. D., Kwon, Y. T., et al. (2013). Role of the IL-6-JAK1-STAT3-Oct-4 Pathway in the Conversion of Non-stem Cancer Cells into Cancer Stem-like Cells. Cell Signal. 25 (4), 961–969. doi:10.1016/j.cellsig.2013.01.007
Kim, H. R., Park, S.-M., Seo, S.-U., Jung, I., Yoon, H. I., Gabrilovich, D. I., et al. (2019). The Ratio of Peripheral Regulatory T Cells to Lox-1+Polymorphonuclear Myeloid-Derived Suppressor Cells Predicts the Early Response to Anti-PD-1 Therapy in Patients with Non-small Cell Lung Cancer. Am. J. Respir. Crit. Care Med. 199 (2), 243–246. doi:10.1164/rccm.201808-1502le
Koh, J., Kim, Y., Lee, K. Y., Hur, J. Y., Kim, M. S., Kim, B., et al. (2020). MDSC Subtypes and CD39 Expression on CD8 + T Cells Predict the Efficacy of anti‐PD‐1 Immunotherapy in Patients with Advanced NSCLC. Eur. J. Immunol. 50 (11), 1810–1819. doi:10.1002/eji.202048534
Koinis, F., Vetsika, E. K., Aggouraki, D., Skalidaki, E., Koutoulaki, A., Gkioulmpasani, M., et al. (2016). Effect of First-Line Treatment on Myeloid-Derived Suppressor Cells' Subpopulations in the Peripheral Blood of Patients with Non-Small Cell Lung Cancer. J. Thorac. Oncol. 11 (8), 1263–1272. doi:10.1016/j.jtho.2016.04.026
Komura, N., Mabuchi, S., Shimura, K., Yokoi, E., Kozasa, K., Kuroda, H., et al. (2020). The Role of Myeloid-Derived Suppressor Cells in Increasing Cancer Stem-Like Cells and Promoting PD-L1 Expression in Epithelial Ovarian Cancer. Cancer Immunol. Immunother. 69 (12), 2477–2499. doi:10.1007/s00262-020-02628-2
Kujawski, M., Kortylewski, M., Lee, H., Herrmann, A., Kay, H., and Yu, H. (2008). Stat3 Mediates Myeloid Cell-Dependent Tumor Angiogenesis in Mice. J. Clin. Invest. 118 (10), 3367–3377. doi:10.1172/jci35213
Lamichhane, P., Karyampudi, L., Shreeder, B., Krempski, J., Bahr, D., Daum, J., et al. (2017). IL10 Release upon PD-1 Blockade Sustains Immunosuppression in Ovarian Cancer. Cancer Res. 77 (23), 6667–6678. doi:10.1158/0008-5472.can-17-0740
Langrish, C. L., Chen, Y., Blumenschein, W. M., Mattson, J., Basham, B., Sedgwick, J. D., et al. (2005). IL-23 Drives a Pathogenic T Cell Population that Induces Autoimmune Inflammation. J. Exp. Med. 201 (2), 233–240. doi:10.1084/jem.20041257
Lechner, M. G., Liebertz, D. J., and Epstein, A. L. (2010). Characterization of Cytokine-Induced Myeloid-Derived Suppressor Cells from normal Human Peripheral Blood Mononuclear Cells. J. Immunol. 185 (4), 2273–2284. doi:10.4049/jimmunol.1000901
Lee, J., Ryu, H., Ferrante, R. J., Morris, S. M., and Ratan, R. R. (2003). Translational Control of Inducible Nitric Oxide Synthase Expression by Arginine Can Explain the Arginine Paradox. Proc. Natl. Acad. Sci. 100 (8), 4843–4848. doi:10.1073/pnas.0735876100
Lee, F. T., Mountain, A. J., Kelly, M. P., Hall, C., Rigopoulos, A., Johns, T. G., et al. (2005). Enhanced Efficacy of Radioimmunotherapy with 90Y-CHX-A''-DTPA-hu3S193 by Inhibition of Epidermal Growth Factor Receptor (EGFR) Signaling with EGFR Tyrosine Kinase Inhibitor AG1478. Clin. Cancer Res. 11 (19 Pt 2), 7080s–7086s. doi:10.1158/1078-0432.CCR-1004-0019
Li, H., Han, Y., Guo, Q., Zhang, M., and Cao, X. (2009). Cancer-Expanded Myeloid-Derived Suppressor Cells Induce Anergy of NK Cells through Membrane-Bound TGF-Β1. J. Immunol. 182 (1), 240–249. doi:10.4049/jimmunol.182.1.240
Li, G., Wu, K., Tao, K., Lu, X., Ma, J., Mao, Z., et al. (2015). Vasoactive Intestinal Peptide Induces CD14+HLA-DR−/low Myeloid-Derived Suppressor Cells in Gastric Cancer. Mol. Med. Rep. 12 (1), 760–768. doi:10.3892/mmr.2015.3374
Lin, J. T., Martin, S. L., Xia, L., and Gorham, J. D. (2005). TGF-β1 Uses Distinct Mechanisms to Inhibit IFN-γ Expression in CD4+ T Cells at Priming and at Recall: Differential Involvement of Stat4 and T-Bet. J. Immunol. 174 (10), 5950–5958. doi:10.4049/jimmunol.174.10.5950
Lu, C., Redd, P. S., Lee, J. R., Savage, N., and Liu, K. (2016). The Expression Profiles and Regulation of PD-L1 in Tumor-Induced Myeloid-Derived Suppressor Cells. Oncoimmunology 5 (12), e1247135. doi:10.1080/2162402x.2016.1247135
Maenhout, S. K., Thielemans, K., and Aerts, J. L. (2014). Location, Location, Location: Functional and Phenotypic Heterogeneity between Tumor-Infiltrating and Non-Infiltrating Myeloid-Derived Suppressor Cells. Oncoimmunology 3 (10), e956579. doi:10.4161/21624011.2014.956579
Mäkitie, A., Tuokkola, I., Laurell, G., Mäkitie, O., Olsen, K., Takes, R. P., et al. (2020). Vitamin D in Head and Neck Cancer: A Systematic Review. Curr. Oncol. Rep. 23 (1), 5. doi:10.1007/s11912-020-00996-7
Mandruzzato, S., Brandau, S., Britten, C. M., Bronte, V., Damuzzo, V., Gouttefangeas, C., et al. (2016). Toward Harmonized Phenotyping of Human Myeloid-Derived Suppressor Cells by Flow Cytometry: Results from an Interim Study. Cancer Immunol. Immunother. 65 (2), 161–169. doi:10.1007/s00262-015-1782-5
McDermott, D. F., Huseni, M. A., Atkins, M. B., Motzer, R. J., Rini, B. I., Escudier, B., et al. (2018). Clinical Activity and Molecular Correlates of Response to Atezolizumab Alone or in Combination with Bevacizumab versus Sunitinib in Renal Cell Carcinoma. Nat. Med. 24 (6), 749–757. doi:10.1038/s41591-018-0053-3
McKarns, S. C., Schwartz, R. H., and Kaminski, N. E. (2004). Smad3 Is Essential for TGF-Β1 to Suppress IL-2 Production and TCR-Induced Proliferation, but Not IL-2-Induced Proliferation. J. Immunol. 172 (7), 4275–4284. doi:10.4049/jimmunol.172.7.4275
Metz, R., Rust, S., Duhadaway, J. B., Mautino, M. R., Munn, D. H., Vahanian, N. N., et al. (2012). Ido Inhibits a Tryptophan Sufficiency Signal that Stimulates mTOR: A Novel Ido Effector Pathway Targeted by D-1-Methyl-Tryptophan. Oncoimmunology 1 (9), 1460–1468. doi:10.4161/onci.21716
Meyer, C., Cagnon, L., Costa-Nunes, C. M., Baumgaertner, P., Montandon, N., Leyvraz, L., et al. (2014). Frequencies of Circulating MDSC Correlate with Clinical Outcome of Melanoma Patients Treated with Ipilimumab. Cancer Immunol. Immunother. 63 (3), 247–257. doi:10.1007/s00262-013-1508-5
Min, Y., Li, J., Qu, P., and Lin, P. C. (2017). C/EBP-δ Positively Regulates MDSC Expansion and Endothelial VEGFR2 Expression in Tumor Development. Oncotarget 8 (31), 50582–50593. doi:10.18632/oncotarget.16410
Mirza, N., Fishman, M., Fricke, I., Dunn, M., Neuger, A. M., Frost, T. J., et al. (2006). All-Trans-Retinoic Acid Improves Differentiation of Myeloid Cells and Immune Response in Cancer Patients. Cancer Res. 66 (18), 9299–9307. doi:10.1158/0008-5472.can-06-1690
Molon, B., Ugel, S., Del Pozzo, F., Soldani, C., Zilio, S., Avella, D., et al. (2011). Chemokine Nitration Prevents Intratumoral Infiltration of Antigen-Specific T Cells. J. Exp. Med. 208 (10), 1949–1962. doi:10.1084/jem.20101956
Munn, D. H., Sharma, M. D., Baban, B., Harding, H. P., Zhang, Y., Ron, D., et al. (2005). GCN2 Kinase in T Cells Mediates Proliferative Arrest and Anergy Induction in Response to Indoleamine 2,3-Dioxygenase. Immunity 22 (5), 633–642. doi:10.1016/j.immuni.2005.03.013
Nagaraj, S., Gupta, K., Pisarev, V., Kinarsky, L., Sherman, S., Kang, L., et al. (2007). Altered Recognition of Antigen Is a Mechanism of CD8+ T Cell Tolerance in Cancer. Nat. Med. 13 (7), 828–835. doi:10.1038/nm1609
Naing, A., Bauer, T., Papadopoulos, K. P., Rahma, O., Tsai, F., Garralda, E., et al. (2019). Phase I Study of the Arginase Inhibitor INCB001158 (1158) Alone and in Combination with Pembrolizumab (PEM) in Patients (Pts) with Advanced/metastatic (Adv/met) Solid Tumours. Ann. Oncol. 30, v160. doi:10.1093/annonc/mdz244.002
Netherby, C. S., and Abrams, S. I. (2017). Mechanisms Overseeing Myeloid-Derived Suppressor Cell Production in Neoplastic Disease. Cancer Immunol. Immunother. 66 (8), 989–996. doi:10.1007/s00262-017-1963-5
Novitskiy, S. V., Pickup, M. W., Chytil, A., Polosukhina, D., Owens, P., and Moses, H. L. (2012). Deletion of TGF- Signaling in Myeloid Cells Enhances Their Anti-tumorigenic Properties. J. Leukoc. Biol. 92 (3), 641–651. doi:10.1189/jlb.1211639
Nywening, T. M., Wang-Gillam, A., Sanford, D. E., Belt, B. A., Panni, R. Z., Cusworth, B. M., et al. (2016). Targeting Tumour-Associated Macrophages with CCR2 Inhibition in Combination with FOLFIRINOX in Patients with Borderline Resectable and Locally Advanced Pancreatic Cancer: A single-Centre, Open-Label, Dose-Finding, Non-randomised, Phase 1b Trial. Lancet Oncol. 17 (5), 651–662. doi:10.1016/s1470-2045(16)00078-4
Ohl, K., and Tenbrock, K. (2018). Reactive Oxygen Species as Regulators of MDSC-Mediated Immune Suppression. Front. Immunol. 9, 2499. doi:10.3389/fimmu.2018.02499
Oka, N., Soeda, A., Inagaki, A., Onodera, M., Maruyama, H., Hara, A., et al. (2007). VEGF Promotes Tumorigenesis and Angiogenesis of Human Glioblastoma Stem Cells. Biochem. Biophysical Res. Commun. 360 (3), 553–559. doi:10.1016/j.bbrc.2007.06.094
Ostrand-Rosenberg, S., Sinha, P., Beury, D. W., and Clements, V. K. (2012). Cross-Talk between Myeloid-Derived Suppressor Cells (MDSC), Macrophages, and Dendritic Cells Enhances Tumor-Induced Immune Suppression. Semin. Cancer Biol. 22 (4), 275–281. doi:10.1016/j.semcancer.2012.01.011
Otsuji, M., Kimura, Y., Aoe, T., Okamoto, Y., and Saito, T. (1996). Oxidative Stress by Tumor-Derived Macrophages Suppresses the Expression of CD3 Chain of T-Cell Receptor Complex and Antigen-specific T-Cell Responses. Proc. Natl. Acad. Sci. 93 (23), 13119–13124. doi:10.1073/pnas.93.23.13119
Ozga, A. J., Chow, M. T., and Luster, A. D. (2021). Chemokines and the Immune Response to Cancer. Immunity 54 (5), 859–874. doi:10.1016/j.immuni.2021.01.012
Pang, X., Fan, H.-Y., Tang, Y.-L., Wang, S.-S., Cao, M.-X., Wang, H.-F., et al. (2020). Myeloid Derived Suppressor Cells Contribute to the Malignant Progression of Oral Squamous Cell Carcinoma. PLoS One 15 (2), e0229089. doi:10.1371/journal.pone.0229089
Panni, R. Z., Sanford, D. E., Belt, B. A., Mitchem, J. B., Worley, L. A., Goetz, B. D., et al. (2014). Tumor-Induced STAT3 Activation in Monocytic Myeloid-Derived Suppressor Cells Enhances Stemness and Mesenchymal Properties in Human Pancreatic Cancer. Cancer Immunol. Immunother. 63 (5), 513–528. doi:10.1007/s00262-014-1527-x
Parker, K. H., Sinha, P., Horn, L. A., Clements, V. K., Yang, H., Li, J., et al. (2014). HMGB1 Enhances Immune Suppression by Facilitating the Differentiation and Suppressive Activity of Myeloid-Derived Suppressor Cells. Cancer Res. 74 (20), 5723–5733. doi:10.1158/0008-5472.can-13-2347
Pogoda, K., Pyszniak, M., Rybojad, P., and Tabarkiewicz, J. (2016). Monocytic Myeloid-Derived Suppressor Cells as a Potent Suppressor of Tumor Immunity in Non-Small Cell Lung Cancer. Oncol. Lett. 12 (6), 4785–4794. doi:10.3892/ol.2016.5273
Rashid, M., Borin, T., Ara, R., Piranlioglu, R., Achyut, B., Korkaya, H., et al. (2021). Critical Immunosuppressive Effect of MDSC-Derived Exosomes in the Tumor Microenvironment. Oncol. Rep. 45 (3), 1171–1181. doi:10.3892/or.2021.7936
Rayman, P., Diaz-Montero, C. M., Yang, Y., Rini, B., Elson, P., and Finke, J. (2015). Modulation of Immune Cell Infiltrate with Sunitinib to Improve Anti-PD1 Therapy in Preclinical Tumor Model. J. ImmunoTherapy Cancer 3 (S2), P310. doi:10.1186/2051-1426-3-s2-p310
Rodriguez, P. C., Zea, A. H., Culotta, K. S., Zabaleta, J., Ochoa, J. B., and Ochoa, A. C. (2002). Regulation of T Cell Receptor CD3ζ Chain Expression Byl-Arginine. J. Biol. Chem. 277 (24), 21123–21129. doi:10.1074/jbc.m110675200
Rodriguez, P. C., Quiceno, D. G., and Ochoa, A. C. (2007). L-arginine Availability Regulates T-Lymphocyte Cell-Cycle Progression. Blood 109 (4), 1568–1573. doi:10.1182/blood-2006-06-031856
Rodriguez, P. C., Ernstoff, M. S., Hernandez, C., Atkins, M., Zabaleta, J., Sierra, R., et al. (2009). Arginase I-Producing Myeloid-Derived Suppressor Cells in Renal Cell Carcinoma Are a Subpopulation of Activated Granulocytes. Cancer Res. 69 (4), 1553–1560. doi:10.1158/0008-5472.can-08-1921
Sad, S., and Mosmann, T. R. (1994). Single IL-2-Secreting Precursor CD4 T Cell Can Develop into Either Th1 or Th2 Cytokine Secretion Phenotype. J. Immunol. 153 (8), 3514–3522.
Sceneay, J., Parker, B. S., Smyth, M. J., and Möller, A. (2013). Hypoxia-Driven Immunosuppression Contributes to the Pre-Metastatic Niche. Oncoimmunology 2 (1), e22355. doi:10.4161/onci.22355
Schlecker, E., Stojanovic, A., Eisen, C., Quack, C., Falk, C. S., Umansky, V., et al. (2012). Tumor-Infiltrating Monocytic Myeloid-Derived Suppressor Cells Mediate CCR5-Dependent Recruitment of Regulatory T Cells Favoring Tumor Growth. J. Immunol. 189 (12), 5602–5611. doi:10.4049/jimmunol.1201018
Schlegel, N. C., von Planta, A., Widmer, D. S., Dummer, R., and Christofori, G. (2015). PI3K Signalling Is Required for a TGFβ-Induced Epithelial-Mesenchymal-Like Transition (EMT-Like) in Human Melanoma Cells. Exp. Dermatol. 24 (1), 22–28. doi:10.1111/exd.12580
Schott, A. F., Goldstein, L. J., Cristofanilli, M., Ruffini, P. A., McCanna, S., Reuben, J. M., et al. (2017). Phase Ib Pilot Study to Evaluate Reparixin in Combination with Weekly Paclitaxel in Patients with HER-2-Negative Metastatic Breast Cancer. Clin. Cancer Res. 23 (18), 5358–5365. doi:10.1158/1078-0432.ccr-16-2748
Serafini, P., Mgebroff, S., Noonan, K., and Borrello, I. (2008). Myeloid-Derived Suppressor Cells Promote Cross-Tolerance in B-Cell Lymphoma by Expanding Regulatory T Cells. Cancer Res. 68 (13), 5439–5449. doi:10.1158/0008-5472.can-07-6621
Shin, J.-S., Ebersold, M., Pypaert, M., Delamarre, L., Hartley, A., and Mellman, I. (2006). Surface Expression of MHC Class II in Dendritic Cells Is Controlled by Regulated Ubiquitination. Nature 444 (7115), 115–118. doi:10.1038/nature05261
Shojaei, F., and Ferrara, N. (2008). Refractoriness to Antivascular Endothelial Growth Factor Treatment: Role of Myeloid Cells: Figure 1. Cancer Res. 68 (14), 5501–5504. doi:10.1158/0008-5472.can-08-0925
Shojaei, F., Wu, X., Qu, X., Kowanetz, M., Yu, L., Tan, M., et al. (2009). G-CSF-Initiated Myeloid Cell Mobilization and Angiogenesis Mediate Tumor Refractoriness to Anti-VEGF Therapy in Mouse Models. Proc. Natl. Acad. Sci. 106 (16), 6742–6747. doi:10.1073/pnas.0902280106
Sinha, P., Clements, V. K., Fulton, A. M., and Ostrand-Rosenberg, S. (2007). Prostaglandin E2 Promotes Tumor Progression by Inducing Myeloid-Derived Suppressor Cells. Cancer Res. 67 (9), 4507–4513. doi:10.1158/0008-5472.can-06-4174
Sinha, P., Chornoguz, O., Clements, V. K., Artemenko, K. A., Zubarev, R. A., and Ostrand-Rosenberg, S. (2011). Myeloid-Derived Suppressor Cells Express the Death Receptor Fas and Apoptose in Response to T Cell-Expressed FasL. Blood 117 (20), 5381–5390. doi:10.1182/blood-2010-11-321752
Srivastava, M. K., Sinha, P., Clements, V. K., Rodriguez, P., and Ostrand-Rosenberg, S. (2010). Myeloid-Derived Suppressor Cells Inhibit T-Cell Activation by Depleting Cystine and Cysteine. Cancer Res. 70 (1), 68–77. doi:10.1158/0008-5472.can-09-2587
Sun, R., Luo, H., Su, J., Di, S., Zhou, M., Shi, B., et al. (2021). Olaparib Suppresses MDSC Recruitment via SDF1α/CXCR4 Axis to Improve the Anti-tumor Efficacy of CAR-T Cells on Breast Cancer in Mice. Mol. Ther. 29 (1), 60–74. doi:10.1016/j.ymthe.2020.09.034
Suzuki, S., Shibata, M., Gonda, K., Kanke, Y., Ashizawa, M., Ujiie, D., et al. (2013). Immunosuppression Involving Increased Myeloid-Derived Suppressor Cell Levels, Systemic Inflammation and Hypoalbuminemia Are Present in Patients with Anaplastic Thyroid Cancer. Mol. Clin. Oncol. 1 (6), 959–964. doi:10.3892/mco.2013.170
Tacke, R. S., Lee, H.-C., Goh, C., Courtney, J., Polyak, S. J., Rosen, H. R., et al. (2012). Myeloid Suppressor Cells Induced by Hepatitis C Virus Suppress T-Cell Responses through the Production of Reactive Oxygen Species. Hepatology 55 (2), 343–353. doi:10.1002/hep.24700
Talmadge, J. E., and Gabrilovich, D. I. (2013). History of Myeloid-Derived Suppressor Cells. Nat. Rev. Cancer 13 (10), 739–752. doi:10.1038/nrc3581
Thomas, D. A., and Massagué, J. (2005). TGF-β Directly Targets Cytotoxic T Cell Functions during Tumor Evasion of Immune Surveillance. Cancer Cell 8 (5), 369–380. doi:10.1016/j.ccr.2005.10.012
Tian, T., Gu, X., Zhang, B., Liu, Y., Yuan, C., Shao, L., et al. (2015). Increased Circulating CD14(+)HLA-DR-/low Myeloid-Derived Suppressor Cells Are Associated with Poor Prognosis in Patients with Small-Cell Lung Cancer. Cancer Biomark 15 (4), 425–432. doi:10.3233/cbm-150473
Tobin, R. P., Jordan, K. R., Robinson, W. A., Davis, D., Borges, V. F., Gonzalez, R., et al. (2018). Targeting Myeloid-Derived Suppressor Cells Using All-Trans Retinoic Acid in Melanoma Patients Treated with Ipilimumab. Int. Immunopharmacology 63, 282–291. doi:10.1016/j.intimp.2018.08.007
Tzeng, A., Diaz-Montero, C. M., Rayman, P. A., Kim, J. S., Pavicic, P. G., Finke, J. H., et al. (2018). Immunological Correlates of Response to Immune Checkpoint Inhibitors in Metastatic Urothelial Carcinoma. Targ Oncol. 13 (5), 599–609. doi:10.1007/s11523-018-0595-9
Vaknin, I., Blinder, L., Wang, L., Gazit, R., Shapira, E., Genina, O., et al. (2008). A Common Pathway Mediated through Toll-like Receptors Leads to T- and Natural Killer-Cell Immunosuppression. Blood 111 (3), 1437–1447. doi:10.1182/blood-2007-07-100404
van Meeteren, L. A., Goumans, M.-J., and ten Dijke, P. (2011). TGF-β Receptor Signaling Pathways in Angiogenesis; Emerging Targets for Anti-Angiogenesis Therapy. Curr. Pharm. Biotechnol 12 (12), 2108–2120. doi:10.2174/138920111798808338
van Meir, H., Nout, R. A., Welters, M. J. P., Loof, N. M., de Kam, M. L., van Ham, J. J., et al. (2017). Impact of (Chemo)radiotherapy on Immune Cell Composition and Function in Cervical Cancer Patients. Oncoimmunology 6 (2), e1267095. doi:10.1080/2162402x.2016.1267095
Vig, M., Srivastava, S., Kandpal, U., Sade, H., Lewis, V., Sarin, A., et al. (2004). Inducible Nitric Oxide Synthase in T Cells Regulates T Cell Death and Immune Memory. J. Clin. Invest. 113 (12), 1734–1742. doi:10.1172/jci20225
Villagra, A., Cheng, F., Wang, H.-W., Suarez, I., Glozak, M., Maurin, M., et al. (2009). The Histone Deacetylase HDAC11 Regulates the Expression of Interleukin 10 and Immune Tolerance. Nat. Immunol. 10 (1), 92–100. doi:10.1038/ni.1673
Vuk-Pavlovic, S., Bulur, P. A., Lin, Y., Qin, R., Szumlanski, C. L., Zhao, X., et al. (2010). Immunosuppressive CD14+HLA-DRlow/- Monocytes in Prostate Cancer. Prostate 70 (4), 443–455. doi:10.1002/pros.21078
Wan, Y. Y., and Flavell, R. A. (2008). TGF-β and Regulatory T Cell in Immunity and Autoimmunity. J. Clin. Immunol. 28 (6), 647–659. doi:10.1007/s10875-008-9251-y
Wang, D., Sun, H., Wei, J., Cen, B., and DuBois, R. N. (2017). CXCL1 Is Critical for Premetastatic Niche Formation and Metastasis in Colorectal Cancer. Cancer Res. 77 (13), 3655–3665. doi:10.1158/0008-5472.can-16-3199
Wang, Y., Yin, K., Tian, J., Xia, X., Ma, J., Tang, X., et al. (2019a). Granulocytic Myeloid‐Derived Suppressor Cells Promote the Stemness of Colorectal Cancer Cells through Exosomal S100A9. Adv. Sci. 6 (18), 1901278. doi:10.1002/advs.201901278
Wang, Y., Ding, Y., Guo, N., and Wang, S. (2019b). MDSCs: Key Criminals of Tumor Pre-metastatic Niche Formation. Front. Immunol. 10, 172. doi:10.3389/fimmu.2019.00172
Wang, L., Jia, B., Claxton, D. F., Ehmann, W. C., Rybka, W. B., Mineishi, S., et al. (2018). VISTA Is Highly Expressed on MDSCs and Mediates an Inhibition of T Cell Response in Patients with AML. Oncoimmunology 7 (9), e1469594. doi:10.1080/2162402x.2018.1469594
Weide, B., Martens, A., Zelba, H., Stutz, C., Derhovanessian, E., Di Giacomo, A. M., et al. (2014). Myeloid-Derived Suppressor Cells Predict Survival of Patients with Advanced Melanoma: Comparison with Regulatory T Cells and NY-ESO-1- or Melan-A-specific T Cells. Clin. Cancer Res. 20 (6), 1601–1609. doi:10.1158/1078-0432.ccr-13-2508
Wesolowski, R., Sharma, N., Reebel, L., Rodal, M. B., Peck, A., West, B. L., et al. (2019). Phase Ib Study of the Combination of Pexidartinib (PLX3397), a CSF-1R Inhibitor, and Paclitaxel in Patients with Advanced Solid Tumors. Ther. Adv. Med. Oncol. 11, 1758835919854238. doi:10.1177/1758835919854238
Wu, L., Deng, Z., Peng, Y., Han, L., Liu, J., Wang, L., et al. (2017). Ascites-Derived IL-6 and IL-10 Synergistically Expand CD14+HLA-DR-/low Myeloid-Derived Suppressor Cells in Ovarian Cancer Patients. Oncotarget 8 (44), 76843–76856. doi:10.18632/oncotarget.20164
Xiu, B., Lin, Y., Grote, D. M., Ziesmer, S. C., Gustafson, M. P., Maas, M. L., et al. (2015). IL-10 Induces the Development of Immunosuppressive CD14+HLA-DRlow/− Monocytes in B-Cell Non-hodgkin Lymphoma. Blood Cancer J. 5, e328. doi:10.1038/bcj.2015.56
Yang, L., DeBusk, L. M., Fukuda, K., Fingleton, B., Green-Jarvis, B., Shyr, Y., et al. (2004). Expansion of Myeloid Immune Suppressor Gr+CD11b+ Cells in Tumor-Bearing Host Directly Promotes Tumor Angiogenesis. Cancer Cell 6 (4), 409–421. doi:10.1016/j.ccr.2004.08.031
Yang, L., Dong, Y., Li, Y., Wang, D., Liu, S., Wang, D., et al. (2019). IL‐10 Derived from M2 Macrophage Promotes Cancer Stemness via JAK1/STAT1/NF‐κB/Notch1 Pathway in Non‐small Cell Lung Cancer. Int. J. Cancer 145 (4), 1099–1110. doi:10.1002/ijc.32151
Yao, W., Ba, Q., Li, X., Li, H., Zhang, S., Yuan, Y., et al. (2017). A Natural CCR2 Antagonist Relieves Tumor-Associated Macrophage-Mediated Immunosuppression to Produce a Therapeutic Effect for Liver Cancer. EBioMedicine 22, 58–67. doi:10.1016/j.ebiom.2017.07.014
Yaseen, M. M., Abuharfeil, N. M., Darmani, H., and Daoud, A. (2020). Mechanisms of Immune Suppression by Myeloid-Derived Suppressor Cells: the Role of Interleukin-10 as a Key Immunoregulatory Cytokine. Open Biol. 10 (9), 200111. doi:10.1098/rsob.200111
Youn, J.-I., Park, S.-M., Park, S., Kim, G., Lee, H.-J., Son, J., et al. (2020). Peripheral Natural Killer Cells and Myeloid-Derived Suppressor Cells Correlate with Anti-PD-1 Responses in Non-Small Cell Lung Cancer. Sci. Rep. 10 (1), 9050. doi:10.1038/s41598-020-65666-x
Yu, J., Du, W., Yan, F., Wang, Y., Li, H., Cao, S., et al. (2013). Myeloid-Derived Suppressor Cells Suppress Antitumor Immune Responses through Ido Expression and Correlate with Lymph Node Metastasis in Patients with Breast Cancer. J. Immunol. 190 (7), 3783–3797. doi:10.4049/jimmunol.1201449
Zea, A. H., Rodriguez, P. C., Atkins, M. B., Hernandez, C., Signoretti, S., Zabaleta, J., et al. (2005). Arginase-Producing Myeloid Suppressor Cells in Renal Cell Carcinoma Patients: A Mechanism of Tumor Evasion. Cancer Res. 65 (8), 3044–3048. doi:10.1158/0008-5472.can-04-4505
Zeng, Q., Fu, J., Korrer, M., Gorbounov, M., Murray, P. J., Pardoll, D., et al. (2018). Caspase-1 from Human Myeloid-Derived Suppressor Cells Can Promote T Cell-Independent Tumor Proliferation. Cancer Immunol. Res. 6 (5), 566–577. doi:10.1158/2326-6066.cir-17-0543
Zhang, N., and Bevan, M. J. (2012). TGF-β Signaling to T Cells Inhibits Autoimmunity during Lymphopenia-Driven Proliferation. Nat. Immunol. 13 (7), 667–673. doi:10.1038/ni.2319
Zhang, J., Han, X., Shi, H., Gao, Y., Qiao, X., Li, H., et al. (2020). Lung Resided Monocytic Myeloid-Derived Suppressor Cells Contribute to Premetastatic Niche Formation by Enhancing MMP-9 Expression. Mol. Cell Probes 50, 101498. doi:10.1016/j.mcp.2019.101498
Zhao, Y., Wu, T., Shao, S., Shi, B., and Zhao, Y. (2016). Phenotype, Development, and Biological Function of Myeloid-Derived Suppressor Cells. Oncoimmunology 5 (2), e1004983. doi:10.1080/2162402x.2015.1004983
Zhu, H., Gu, Y., Xue, Y., Yuan, M., Cao, X., and Liu, Q. (2017). CXCR2+ MDSCs Promote Breast Cancer Progression by Inducing EMT and Activated T Cell Exhaustion. Oncotarget 8 (70), 114554–114567. doi:10.18632/oncotarget.23020
Zhu, J., Powis de Tenbossche, C. G., Cané, S., Colau, D., van Baren, N., Lurquin, C., et al. (2017). Resistance to Cancer Immunotherapy Mediated by Apoptosis of Tumor-Infiltrating Lymphocytes. Nat. Commun. 8 (1), 1404. doi:10.1038/s41467-017-00784-1
Keywords: MDSC, Treg, EMT, angiogenesis, immunotherapy
Citation: Cheng J-N, Yuan Y-X, Zhu B and Jia Q (2021) Myeloid-Derived Suppressor Cells: A Multifaceted Accomplice in Tumor Progression. Front. Cell Dev. Biol. 9:740827. doi: 10.3389/fcell.2021.740827
Received: 13 July 2021; Accepted: 03 December 2021;
Published: 23 December 2021.
Edited by:
Sanjay Mishra, The Ohio State University, United StatesReviewed by:
Rachna Shah, Memorial Sloan Kettering Cancer Center, United StatesPratibha Bhalla, University of Texas Southwestern Medical Center, United States
Copyright © 2021 Cheng, Yuan, Zhu and Jia. This is an open-access article distributed under the terms of the Creative Commons Attribution License (CC BY). The use, distribution or reproduction in other forums is permitted, provided the original author(s) and the copyright owner(s) are credited and that the original publication in this journal is cited, in accordance with accepted academic practice. No use, distribution or reproduction is permitted which does not comply with these terms.
*Correspondence: Bo Zhu, Ym8uemh1QHRtbXUuZWR1LmNu; Qingzhu Jia, cWluZ3podS5qaWFAdG1tdS5lZHUuY24=
†These authors have contributed equally to this work and share first authorship