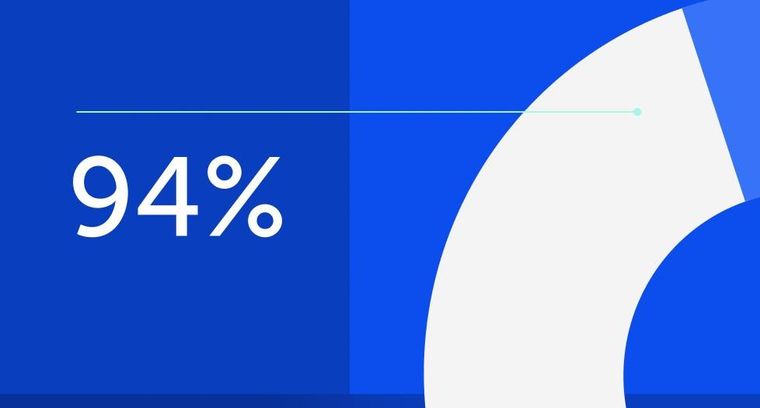
94% of researchers rate our articles as excellent or good
Learn more about the work of our research integrity team to safeguard the quality of each article we publish.
Find out more
REVIEW article
Front. Cell Dev. Biol., 17 November 2021
Sec. Molecular and Cellular Oncology
Volume 9 - 2021 | https://doi.org/10.3389/fcell.2021.740783
This article is part of the Research TopicThe Role of Steroid Hormones and Growth Factors in CancerView all 22 articles
Osteosarcoma (OS), a primary malignant bone tumor, stems from bone marrow-derived mesenchymal stem cells (BMSCs) and/or committed osteoblast precursors. Distant metastases, in particular pulmonary and skeletal metastases, are common in patients with OS. Moreover, extensive resection of the primary tumor and bone metastases usually leads to bone defects in these patients. Bone morphogenic protein-2 (BMP-2) has been widely applied in bone regeneration with the rationale that BMP-2 promotes osteoblastic differentiation of BMSCs. Thus, BMP-2 might be useful after OS resection to repair bone defects. However, the potential tumorigenicity of BMP-2 remains a concern that has impeded the administration of BMP-2 in patients with OS and in populations susceptible to OS with severe bone deficiency (e.g., in patients with genetic mutation diseases and aberrant activities of bone metabolism). In fact, some studies have drawn the opposite conclusion about the effect of BMP-2 on OS progression. Given the roles of BMSCs in the origination of OS and osteogenesis, we hypothesized that the responses of BMSCs to BMP-2 in the tumor milieu may be responsible for OS development. This review focuses on the relationship among BMSCs, BMP-2, and OS cells; a better understanding of this relationship may elucidate the accurate mechanisms of actions of BMP-2 in osteosarcomagenesis and thereby pave the way for clinically safer and broader administration of BMP-2 in the future. For example, a low dosage of and a slow-release delivery strategy for BMP-2 are potential topics for exploration to treat OS.
Although osteosarcoma (OS), a primary bone neoplasm, is rare, with an incidence of only one to three confirmed cases per 1 million people in the world each year, it comprises ∼20% of newly diagnosed bone tumors (Dorfman and Czerniak, 1995; Klein and Siegal, 2006; Mirabello et al., 2009b). Epidemiologically, OS presents in children, the youth, and the elderly with high frequency (Kansara et al., 2014); the morbidity of OS increases to 8–11 per million annually in 15–19-year-olds (Stiller et al., 2006; Mirabello et al., 2009a; Anfinsen et al., 2011). OS most often initiates in the metaphysis of long bones (Ritter and Bielack, 2010), implying a correlation with impaired bone growth. Currently, bone-marrow-derived mesenchymal stem cells (BMSCs) and/or committed osteoblast precursors with genomic mutations (e.g., TP53, RB1), chromosomal deletion, and chromosomal rearrangements are recognized as the cellular origins of OS (Chandar et al., 1992; Walkley et al., 2008; Mohseny et al., 2009; Rubio et al., 2013; Chen et al., 2014; Deng et al., 2019; Han et al., 2019). As an aggressive tumor, OS is insensitive to some chemotherapy agents (Pavlou et al., 2019; Belisario et al., 2020); MAP (methotrexate, doxorubicin, and cisplatin) is still the first-line drug for OS chemotherapy (Marina et al., 2016). To date, the OS has a 5-year survival rate of ∼50% (Smeland et al., 2019); the leading cause of death in OS is pulmonary metastasis (Bhattasali et al., 2015). Skeletal metastasis is also common in patients with OS and precipitates severe bone erosions. Extensive resection to remove OS is also responsible for voluminous bone defects, which may induce dysfunction and disfiguration. The rehabilitation of bone tissue is a huge challenge in clinical OS therapy. Although bone morphogenic protein-2 (BMP-2) has been widely used in bone repair and has shown promising results, its application in OS has not been reported because of its potential role in tumorigenesis.
BMP-2 was discovered by Urist (1965), and its cDNA was first cloned by Wozney et al. (1988). This growth factor is a member of bone morphogenic proteins (BMPs) belonging to the transforming growth factor-beta (TGF-β) superfamily that is important for diverse cellular processes (e.g., cell proliferation, differentiation, apoptosis, angiogenesis, migration, and extracellular matrix remodeling) (Bierie and Moses, 2006; Massagué, 2008). More than 20 BMPs have been identified in human tissues (Wozney and Rosen, 1998; Reddi, 2005). As the most well-studied one, BMP-2 has been widely used in bone formation because of its potent osteoinductivity and has been approved by the U.S. Food and Drug Administration for orthopedic and dental applications (Burkus et al., 2002; US Food and Drug Administration, 2002; Alonso et al., 2014). After continued clinical use, the adverse effects of BMP-2 (e.g., inflammatory, ectopic bone formation, infection, and potential tumorigenicity) have come into focus; the high dose and off-label application of BMP-2 have also aroused concern (Cahill et al., 2009; Tian et al., 2017; Pardali et al., 2018; Hashimoto et al., 2020; Hsu et al., 2020). Whether BMP-2 suppresses or stimulates tumor development remains a contentious issue (Weiss, 2015), and this controversy still challenges researchers (Kendal et al., 2020; Table 1). Using an orthotopic mouse model, Xiong et al. (2018) revealed that 2.5 μg of recombinant human BMP-2 (rhBMP-2) applied for 14 days not only induced bone formation but also suppressed OS growth and pulmonary metastasis in OS-bearing mice. Similar research also documented that rhBMP-2 constrained the tumorigenicity of cancer stem cells in human OS cell lines in vitro and in vivo (Wang et al., 2011; Gill et al., 2017). Conversely, opposite results from other studies have suggested that BMP-2 promotes OS migration and epithelial–mesenchymal transition (Sotobori et al., 2006; Tian et al., 2019). Because of this discrepancy in results, the use of BMP-2 in those at high risk of OS must be discreet and individualized based on the latest research.
Mesenchymal stem cells (MSCs) are identified as the origin of OS and are capable of differentiating into osteoblasts, a process that can be accelerated by BMP-2. However, BMP-2 is also involved in the progression of OS, suggesting that complicated crosstalk may exist among OS, BMP-2, and MSCs. As multipotent mesenchymal stromal cells, MSCs are universally found in almost all connective tissues (Horwitz et al., 2005; da Silva Meirelles et al., 2006). They possess the ability to differentiate into various mature somatic cells (e.g., osteoblasts, adipocytes, and chondrocytes) with appropriate stimulation (Pittenger et al., 1999) and the capacity to self-renew. BMSCs were first isolated by Owen and Friedenstein (1988) from bone marrow. These heterogeneous cells are involved in osteoblast differentiation through the spatiotemporal expression of osteogenesis-related genes (RUNX2, COL1A1, ALPL, SP7, BGLAP, etc.) (Ducy et al., 1997; Nakashima et al., 2002; Twine et al., 2014). A few signal pathways have proven to have pivotal roles in BMSC-induced osteogenesis; the canonical BMP-2 pathway (Figure 1) is a well-known example. A great body of research has focused on the effect of MSCs on or toward osteoblastic differentiation and OS progression; to date, though, the effect of BMP-2 on normal BMSCs and on mutated BMSC–induced osteosarcomagenesis is still elusive.
Figure 1. Canonical bone morphogenic protein-2 (BMP-2) signaling pathway. After BMP-2 binds to its transmembrane receptors [bone morphogenic protein receptor (BMPR)I and BMPRII], these phosphorylated receptors facilitate the phosphorylation of mothers against decapentaplegic and the Caenorhabditis elegans protein 1/5/8 (Smad1/5/8) in the cytoplasm. Then, the complex of pSmad1/5/8 and Smad 4 translocates to the nucleus, where phosphorylated Smad1/5/8 (pSmad1/5/8) and Smad 4 function as transcription factors, enhancing the transcription of osteoblastic genes, including COL1A1, RUNX2, ALPL, SP7, and BGLAP. As negative feedback, Smad7 inhibits the phosphorylation of Smad1/5/8, and Smad6 prevents the nucleus translocation of the complex of pSmad1/5/8 and Smad4.
MSCs play contradictory roles in copious cancer types (Devarasetty et al., 2017; Gyukity-Sebestyén et al., 2019; Xu et al., 2019; Zhang et al., 2020; Jia et al., 2021). In OS, MSCs are reportedly involved in not only chemoresistance, proliferation, and pulmonary metastases but also OS recession (Cortini et al., 2017). Thus, the effect of MSCs on OS might be converted according to the relevant OS niche. Herein, we summarize the literature and present the potential mechanism of the contradictory effects of MSCs on OS to provide direction for additional studies.
MSCs can suppress sarcoma progression. Gauthaman et al. (2012) found that umbilical cord-derived MSCs from Wharton’s jelly suppressed the proliferation and migration of MG-63 cells (a human OS cell line) in vitro; in a Kaposi sarcoma model, MSCs also inhibited tumor progression (Khakoo et al., 2006). BMP-2 also has inhibited OS progression, although the potential mechanism was not discussed (Xiong et al., 2018). Given the close link between MSCs and BMP-2 in osteoblastic differentiation and OS etiology, BMP-2 might suppress OS through BMSCs. We reviewed the literature to explore the ability of BMP-2 to inhibit OS through BMSCs and present three assumptions (Figure 2): (1) BMP-2 induces proliferation of BMSCs with the capacity to suppress OS; (2) BMP-2 induces differentiation of mutated BMSCs and/or OS cells to normal osteoblasts; (3) BMSC polarization shifts.
Figure 2. Potential mechanism of bone morphogenic protein-2 (BMP-2) induced tumor suppression via bone marrow-derived mesenchymal stem cells (BMSCs). BMP-2 may induce mutated BMSC differentiation into normal osteoblasts. Conversely, BMP-2 may promote the proliferation of specific BMSCs with anticancer capacity and the shift of BMSC polarization from MSC2 (tumor promotion) to MSC1 (tumor inhibition). OS: osteosarcoma.
BMSCs are heterogeneous populations comprising various subpopulations with diverse properties (Horwitz et al., 2005). Except for Wharton’s jelly MSCs, BMSCs from rats and mice have demonstrated dose-dependent cytotoxicity to tumor cells (Otsu et al., 2009). Thus, specific BMSCs with anticancer capacity exist and may function according to the altered expression of some cytomembrane receptors (Ridge et al., 2017). It would make sense that BMP-2 could suppress OS through the proliferation of these specific BMSCs and that a BMP-2 and Wnt pathway autocrine loop (Figure 3) may be capable of explaining this process. The Wnt pathway is involved in diverse cellular events, including mitogenic stimulation, cell fate determination, differentiation, and proliferation (Huang and Niehrs, 2014; Yao et al., 2016; Steinhart and Angers, 2018). It is not surprising that the Wnt pathway, in particular the canonical Wnt pathway (i.e., the beta-catenin–dependent pathway), plays crucial roles in osteoblastic differentiation and osteogenesis (Liu et al., 2008; Wang et al., 2014b; Lerner and Ohlsson, 2015). Although the Wnt pathway is thought to inhibit MSC proliferation (Moon et al., 2018), an activated Wnt pathway facilitating BMSC proliferation has also been reported (Zhu et al., 2014). After the canonical Wnt pathway is activated, beta-catenin translocates from the cytoplasm into nuclei. In the nuclei, a complex consisting of beta-catenin and some transcription factors—for example, lymphoid enhancer-binding factor 1/T cell-specific transcription factor (LEF/TCF)—modulates the expression of target genes, including BMP2, RUNX2, and proliferation-related genes (Zhang R. et al., 2013). Conversely, BMP-2 can stimulate the accumulation of beta-catenin in nuclei (Yang et al., 2006; Hiyama et al., 2011), thereby activating the canonical Wnt pathway in turn. BMP-2–induced cell proliferation has been reported in murine preosteoblasts, rat BMSCs, and human pulmonary artery epithelial cells (de Jesus Perez et al., 2009; Rosen, 2009; An et al., 2017). The OS suppression properties of BMP-2 might result from the positive feedback of this loop via expansion of the specific BMSCs in the OS niche. In addition, aberrant activation of Wnt/beta-catenin signaling in OS cells has been detected (Chen et al., 2015). The identification of specific BMSCs in the OS niche is a precondition for OS suppression. Unfortunately, few studies about these specific BMSCs have been conducted in OS settings, so detailed information about their characteristics is still lacking.
Figure 3. Reciprocal activation between canonical bone morphogenic protein-2 (BMP-2) and canonical Wnt signaling pathway. After BMP-2 binds to its receptors on the cell surface, phosphorylated Smad1/5/8 and Smad4 translocate into nuclei, where this complex modulates the transcription of some target genes, including WNT3A (coding the Wnt3a protein) and some osteoblastic differentiation genes. However, with the autocrine signaling, Wnt3a binds to its receptors (i.e., LRP5/6 and Frizzled) on the membrane, so β-catenin accumulates in the nuclei and incorporates with T cell-specific transcription factor (TCF) to upregulate the transcription of target genes, including BMP2, RUNX-2, and proliferation-related genes. APC: adenomatous polyposis coli, CK1: casein kinase 1, Dvl: Disheveled, GSK3β: glycogen synthase kinase3β, LRP5/6: low-density lipoprotein receptor-related protein 5.
Cancer is a disease arising from failed cell differentiation (Honma and Akimoto, 2007). Thus, differentiation-inducing treatments have been proposed. With this strategy, tumor cells differentiate back into normal cells instead of being eliminated by chemotherapeutics and/or radiation. One well-known differentiation-inducing treatment is all-trans-retinoic acid in acute promyelocytic leukemia (Huang et al., 1988). Notably, OS is recognized as an osteoblast differentiation disruption disease (Tang et al., 2008). OS cells have characteristic properties that resemble undifferentiated osteoblasts (Carpio et al., 2001; Postiglione et al., 2003; Haydon et al., 2007), and activating RB1 transcription has reversed the disrupted osteoblastic differentiation (Thomas et al., 2001). In addition, BMP-2 has been tested for its efficacy as a differentiation-inducing treatment. Rampazzo et al. (2017) successfully induced astroglial differentiation of glioblastoma stem cells using a BMP-2 mimicking peptide. Moreover, BMP-2 has suppressed tumors and promoted bone formation simultaneously: Wang et al. (2012) indicated that renal cell cancer was inhibited and bone formation was induced with the application of BMP-2. Furthermore, BMP-2 has reduced tumor volume, attenuated OS-induced pulmonary metastases, and stimulated bone formation (Xiong et al., 2018). Applying 30 μg of BMP-2 to OS-bearing mice also increased the transcription of osteogenic genes and promoted osteogenesis (Wang et al., 2013). Taken together, these data suggest that BMP-2 may play a therapeutic role in OS by inducing osteogenic differentiation of mutated BMSCs and/or OS cells.
The polarization of macrophages in inflammatory conditions suggests that the effect of BMSCs on OS may also transform mutually between tumor promotion and tumor suppression (Ridge et al., 2017). This hypothesis has been verified by Waterman et al. (2012) in a study that activated different cytomembrane receptors. The researchers claimed that activation of toll-like receptor-4 (TLR-4) conferred an antitumor effect on human BMSCs, which were named MSC1; after TLR-3 activation, however, the human BMSCs were converted to MSC2, which promoted tumor growth and metastasis (Waterman et al., 2012). Although myriad studies have indicated that TLR-2 and TLR-4 can enhance the expression of BMP-2 in BMSCs and accelerate bone formation (Yang et al., 2009; Su et al., 2011; Oliveira et al., 2017; Zhou et al., 2019), the effect of BMP-2 on the expression of TLRs is still equivocal. The dosage of BMP-2 and the state of BMSCs in the tumor niche may draw contrasting conclusions. Thus, the hypothesis that BMP-2 suppresses OS by affecting TLRs must be explored in more detail.
More research has reported that BMPs, especially a supra-physiological dose of BMP-2, induces tumorigenesis, not tumor suppression (Figure 4; Jin et al., 2001; Kang et al., 2011; Wu J. B. et al., 2011; Nishimori et al., 2012; Tian et al., 2017; Zhang et al., 2018). The physiological concentration of BMP is ∼2 ng/g of bone. In most clinical trials, supra-physiological doses (mg concentrations) of BMP-2 have been applied, and these doses may disturb the normal BMP-2 signal pathway (Arrabal et al., 2013; Oryan et al., 2014). After BMP-2 binds to its receptors on the cell surface, the BMP-2 signaling pathway is activated. In the canonical BMP-2 pathway, transcription of osteogenic genes, including RUNX2, and SP7 (OSTERIX), is upregulated. Although these two genes are vital for bone formation, an increasing body of evidence implies that they are also engaged in tumorigenesis. Normally, RUNX2 expresses during the cell cycle in healthy osteoblasts to disturb cell growth and induce osteoblast maturation (Pratap et al., 2003). Overexpression of RUNX2 has been found in patients with OS and is correlated to poor prognosis (Pereira et al., 2009; Sadikovic et al., 2010; Gupta et al., 2019). van der Deen et al. (2012) used chromatin immunoprecipitations to detect RUNX2 target genes in U2OS cells; results indicated that some motility-related genes were downstream of RUNX2 and that cell motility decreased after RUNX2 depletion. Furthermore, an elevated RUNX2 protein level may also be responsible for pulmonary metastasis. After RUNX2 activates SPP1 (OPN), the RUNX2 target gene encodes a secreted matricellular protein, thereby remodeling the bone matrix, which leads to tumor metastasis (Villanueva et al., 2019). RUNX2 may also account for the chemotherapeutic resistance of OS. When RUNX2 was silenced by si/shRNA, OS cells were more sensitive to doxorubicin (Roos et al., 2015). Another osteogenic gene, SP7, has not been associated with osteosarcomagenesis, but it has been described as a stimulus in other tumors (Dai et al., 2015; Yao et al., 2019; Ricci et al., 2020). This finding suggests that sustained activation of the BMP-2 pathway causing increased SP7 transcription may also precipitate OS in bone tissues.
Figure 4. Potential mechanism of bone morphogenic protein-2 (BMP-2)–induced tumor progression via bone marrow-derived mesenchymal stem cells (BMSCs). (A) After the canonical BMP-2 pathway is activated, RUNX2 and SP7 transcription initiate. The overexpression of RUNX2 and SP7, as a result of continuous activation of the canonical BMP-2 pathway, may promote osteosarcoma (OS) progression. (B) BMSCs are recruited to OS by BMP-2. Then, BMSCs adapt to OS via OS-related cytokines and exosomes; in turn, the tumor-centered BMSCs will secrete growth factors and cytokines, such as vascular endothelial growth factor (VEGF) and CXC chemokine receptor 4 (CXCR4), to promote OS development.
BMP-2 may promote OS progression through modulation of the tumor microenvironment (TME), which plays an indispensable role in tumor progression (Hui and Chen, 2015; Yang et al., 2020). The bone microenvironment where OS grows is composed of hematopoietic stem cells, lymphoid progenitors, mature immune cells, bone cells, MSCs, mineralized extracellular matrix, and more (Tsukasaki and Takayanagi, 2019; Corre et al., 2020). The crosstalk in these items modulates the OS TME, which affects OS progression. Cancers are identified as “wounds that never heal” (Dvorak, 1986), so it is not surprising that MSCs are involved in tumor development, given the central role of MSCs in repairing wounds by altering the local inflammatory environment and secreting growth factors, immunoregulatory factors, and chemokines (Caplan and Correa, 2011; Wang et al., 2014a; Shi et al., 2017) after the tumor-specific tropism of MSCs (Kidd et al., 2009). However, MSCs are not always beneficial for healing; the fluctuation of their function depends on the milieu where they reside (Wang et al., 2014a). In the TME, MSCs can be converted into tumor-associated MSCs that have vast differences from normal MSCs (Le Nail et al., 2018) and that can promote tumor proliferation, migration, immunosuppression, and angiogenesis through extracellular vesicles (Quante et al., 2011; Baglio et al., 2017; Shi et al., 2017; Whiteside, 2018). In the OS niche, interleukin-6 (IL-6) and vascular endothelial growth factor (VEGF) secreted from BMSCs have been involved in OS progression (Tu et al., 2012; Zhang P. et al., 2013); BMSCs promoted pulmonary metastasis of OS by increasing the expression of CXC chemokine receptor 4 (CXCR4) and VEGF (Fontanella et al., 2016). Furthermore, extracellular vesicles, such as exosomes from BMSCs, are loaded with certain miRNAs involved in OS aggression and development (Xie et al., 2018). BMP-2, as a member of the TGF-β superfamily with the ability to recruit MSCs to inflammatory surroundings and the TME (Spaeth et al., 2008), may recruit BMSCs to OS, and BMP-2-induced chemotaxis has been reported in other conditions (Hiepen et al., 2014; Simões Sato et al., 2014; Pardali et al., 2018). BMP-2, particularly at high doses, induces inflammation (James et al., 2016), which may cause MSC homing as a result of inflammatory cytokines; in addition, MSCs have been recruited by BMP-2 through CXCR4, accelerating bone formation (Zwingenberger et al., 2014). Thus, BMP-2 might recruit BMSCs toward the OS phenotype. Together, these results suggest a tentative hypothesis. After BMSCs are recruited by BMP-2 to the OS niche, they will be educated directly or indirectly by OS cells. Afterward, the emergence of the educated BMSCs that can secrete some cytokines and growth factors will promote OS proliferation, migration, angiogenesis, and more.
The debate about BMP-2 is an obstacle to its clinical application, despite the potential value for those at high risk of OS and for patients with OS and bone defects. Illustrating the reasons for these controversies can deepen our understanding of the function of BMP-2 in OS and guide its clinical administration.
Diverse OS cell lines applied in the research contribute to the confusion about results. Histologically, several OS subtypes with distinct characteristics have been confirmed. At the cellular level, various in vitro OS cell lines have been used in research; great differences in these cell lines have been verified. Saos2 cells appear more identical to normal osteoblasts than other OS cell lines, as osteoblastic markers can be detected in these cells. Conversely, osteocalcin, an important marker in bone mature, was hardly expressed in MG-63 and U2OS cells. However, matrix metalloproteinase-9 (MMP-9), a well-known cytokine for tumor migration and metastasis (Deryugina and Quigley, 2006), was positive in most MG-63 cells (Pautke et al., 2004). In other research, researchers (Mohseny et al., 2011) compared differences in differentiation, tumorigenesis, and protein expressions among 19 OS cell lines. Only OSA, IOR/OS9, and IOR/OS18 could differentiate into osteoblasts, chondrocytes, and adipocytes; 13 of the 19 cell lines could differentiate toward osteoblasts. This finding may explain why some researchers claimed that OS cell lines could not be induced into osteoblasts by BMP-2, whereas other studies reported opposite results (Haydon et al., 2007). Moreover, in these 19 OS cell lines, HOS-14B cells had the greatest capacities of tumorigenesis and metastasis. These inherent disparities between various OS cell lines, to some extent, account for the conflicting conclusions about the role of BMP-2 in OS progression.
Variations in MSCs are also ubiquitous. MSCs are heterogeneous populations consisting of a few subtypes with diverse characteristics; the differences may come from individual differences and species differences (Peister et al., 2004). The proposed definition of MSCs suggests that they must (1) adhere to plastic, (2) express special surface markers, and (3) differentiate along the osteogenic, chondrogenic, and adipogenic lineages (Dominici et al., 2006; Lindsay and Barnett, 2017). Commonly, CD34, CD31, and CD45 are negative on both human and mouse MSCs (Dominici et al., 2006); some markers, such as STRO-1 and CD271, are only detected on human MSCs (Lv et al., 2014); these are specific and can be found on other cell types (Kuhn and Tuan, 2010). CD29, CD51, CD73, CD90, CD105, and CD146 are universal in human and mouse MSCs (Sacchetti et al., 2007; Zhang et al., 2019). BMSCs are the most used MSCs in research; they are heterogeneous as well, which complicates the research and weakens the conclusions. Although some specific isolation kits based on the cell surface markers have been applied to clarify results, it remains hard to purify the homogeneous BMSCs, as MSCs share cell-surface markers and localization with pericytes (Crisan et al., 2008). With the development of biotechnology, the function and characteristic identification of a single cell are practicable. Single-cell RNA sequencing has been used to detect immune cell heterogeneity (Papalexi and Satija, 2018); Zhou et al. (2020) assayed the intratumoral heterogeneity and immunosuppressive microenvironment in advanced OS and demonstrated the complex variations in OS.
Furthermore, the dose and the delivery strategy of BMP-2 affect the research conclusions (Wu G. et al., 2011). Most of the reported disadvantages of BMP-2 result from overdosage. The effective dose of BMP-2 in osteoblastic differentiation of MSCs, which is dose-dependent, is just 25–100 ng/mL in vitro (Rickard et al., 1994; Lecanda et al., 1997). However, the working concentration of BMP-2 for in vitro or in vivo research is not distinguished, and most doses are supra-physiological, which may confound the results and cause adverse effects. The delivery pattern of BMP-2 is also crucial. A continuous and slow release, rather than a burst stimulation, is more bionic and more closely resembles physiological conditions. Most recent research has administered rhBMP-2 protein directly into the culture medium or intravenously, which may cause stress conditions for cells and tissues. The advantages of a sustained, low-dose release of BMP-2, including less inflammation and ectopic ossification, have been verified (Wildemann et al., 2004; Ji et al., 2010; Seo et al., 2017; Berkmann et al., 2020; Xin et al., 2020). The mitigatory inflammatory surroundings can reduce the risk of tumorigenesis as well, which makes low-dose BMP-2 application more reasonable.
Currently, most in vitro studies are carried out on traditional two-dimensional (2D) culture models (i.e., flask- and petri-dish-based cultures). However, these 2D models hardly mimic tumor cell biology because of tumor heterogeneity and different responses to secreted cytokines, growth factors, and methylation states of the cells. Moreover, the 2D cell culture systems cannot sufficiently simulate a three-dimensional (3D) physiological microenvironment, so they fail to provide physiologically relevant information regarding cell–cell interactions, cell–extracellular matrix interactions, growth factor synthesis, or physical and chemical cues to oncogenesis (Hickman et al., 2014; Berkmann et al., 2020). Furthermore, the results obtained from gene expression analysis and drug resistance also differ substantially between 2D and 3D cell culture models (Zhao et al., 2014; Costa et al., 2016; Henriksson et al., 2017; Zhou et al., 2017; Fontoura et al., 2020; Mao et al., 2020; Sun et al., 2020; Xie et al., 2021). The disadvantages of the 2D culture reduce attempts to understand the authentic role of BMP-2 that may play in the formation and pathology of OS.
In most OS studies, rodents, such as mice or rats, have been used as experimental animal models in addition to the patient-derived xenograft or cell line-derived xenograft models. Normally, OS is rare in mice and rats, and these models may present limited information or misinformation. The OS incidence in dogs is ∼27-fold higher than in humans, which makes the canine model a more useful model to the human OS for research (Simpson et al., 2017). To date, preclinical research using dogs as animal models has suggested that a combination of canine BMSCs together with rhBMP-2 treatment suppressed OS by increasing p53 and some other pro-apoptotic proteins (Rici et al., 2012, 2018). However, using dogs as animal models to study the effects of BMP-2 on OS development is not well accepted in Western countries because of social and cultural reasons.
Large-scale and multicenter cohort studies for evaluating BMP-2 treatment effects on OS progression remain unavailable. Although some clinical retrospective studies have suggested that BMP-2 used in spine fusion surgery was not involved in tumorigenesis (Fahim et al., 2010; Cooper and Kou, 2013; Lad et al., 2013), these studies were performed with small sample sizes and had insufficient follow-up times. Large-scale and multicenter cohort studies are needed to draw a scientific conclusion and establish the effects of the BMP-2 on patients living with cancer.
To date, the exact role of BMP-2 in osteosarcomagenesis is still equivocal, although abundant studies have been carried out. This uncertainty is attributed to the intricacy of the OS genome, differences between OS subtypes, the complex TME, and the multifunctionality of BMP-2 activation of several signal transduction pathways. The response of MSCs, which have a pivotal effect on osteogenesis and osteosarcomagenesis, to BMP-2 remains a key to understanding this mystery. This review represents research focused on the BMP-2 effect on OS cell lines and OS animal models and the relevant potential mechanisms involved, and it provides some clues for additional research about OS biology and safe application of BMP-2 in clinical settings. For current clinical application, we recognize that a low-dose and slow-release strategy of BMP-2 applied in bone regeneration is acceptable, even in the tumor-caused bone defects, while in the OS treatment, we still maintain a prudent stand to the employment of BMP-2.
As a growth factor, BMP-2 plays a crucial role in various cell biology activities. BMP-2 use in populations with genetic mutation diseases may promote OS progression; mutations of some genes, particularly TP53 and RB1, and genomic alterations have been associated with osteosarcomagenesis. Likewise, using BMP-2 in patients with some bone metabolic diseases might increase the occurrence of OS, because aberrant activities of osteogenesis-related signaling pathways in these patients are very common; these pathologic activities may enhance the expression of RUNX2 and SP7, the latter of which is overexpressed in patients with OS and is correlated with poor prognosis.
However, BMP-2 is highly likely to be used in OS treatments because of the BMP-2-induced proliferation of specific BMSCs with anticancer capacity. This strategy is based on the isolation and identification of these specific BMSCs. However, to our knowledge, no research on the isolation and identification of characteristics of these specific BMSCs has been carried out. Moreover, BMP-2 may inhibit OS through the osteoblastic differentiation of OS cells and/or mutated BMSCs. In addition, in line with the current consensus, although an overdose of BMP-2 could lead to over-proliferation of cells, which may increase the risk of neoplasm formation and tumorigenesis, using a low dose and a slow-release delivery pattern of BMP-2 appears safe for oncogenesis-related research.
For additional investigations, researchers should pay attention to the differences between various OS cell lines and the diverse OS subtypes. These differences are responsible for the contradictory roles of BMP-2 in OS development. Caution is needed to interpret data about the function of BMP-2 in OS progression when only one subtype of OS cell line is investigated. Because of the various limitations and factors involved, the relationship between BMP-2—in particular, the supra-physiological concentration of BMP-2—and OS has not been determined thoroughly; more research in this field is necessary.
CX contributed to the conception of this work and drafted the manuscript. MW performed literature search. BZ-D made important revisions. WS revised this manuscript and polished language. LW revised this manuscript and supplemented it with important information. YL edited and revised this manuscript and ultimately approved the publication. All authors contributed to the article and approved the submitted version.
This work was supported by the Dutch NWO grant (No. 729001041) and Dutch ZonMW grant on LSH 2TREAT (No. 436001004).
The authors declare that the research was conducted in the absence of any commercial or financial relationships that could be construed as a potential conflict of interest.
All claims expressed in this article are solely those of the authors and do not necessarily represent those of their affiliated organizations, or those of the publisher, the editors and the reviewers. Any product that may be evaluated in this article, or claim that may be made by its manufacturer, is not guaranteed or endorsed by the publisher.
We thank Prof. Changsheng Liu for the constructive suggestions in the completion of this review. We are also grateful for the contribution from the Shandong Taishan Scholar Program to YL.
Alonso, N., Risso, G. H., Denadai, R., and Raposo-Amaral, C. E. (2014). Effect of maxillary alveolar reconstruction on nasal symmetry of cleft lip and palate patients: a study comparing iliac crest bone graft and recombinant human bone morphogenetic protein-2. J. Plast. Reconstr. Aesthet. Surg. 67, 1201–1208. doi: 10.1016/j.bjps.2014.05.014
An, G., Zhang, W. B., Ma, D. K., Lu, B., Wei, G. J., Guang, Y., et al. (2017). Influence of VEGF/BMP-2 on the proliferation and osteogenetic differentiation of rat bone mesenchymal stem cells on PLGA/gelatin composite scaffold. Eur. Rev. Med. Pharmacol. Sci. 21, 2316–2328.
Anfinsen, K. P., Devesa, S. S., Bray, F., Troisi, R., Jonasdottir, T. J., Bruland, O. S., et al. (2011). Age-period-cohort analysis of primary bone cancer incidence rates in the United States (1976-2005). Cancer Epidemiol. Biomarkers Prev. 20, 1770–1777. doi: 10.1158/1055-9965.Epi-11-0136
Arrabal, P. M., Visser, R., Santos-Ruiz, L., Becerra, J., and Cifuentes, M. (2013). Osteogenic molecules for clinical applications: improving the BMP-collagen system. Biol. Res. 46, 421–429. doi: 10.4067/s0716-97602013000400013
Baglio, S. R., Lagerweij, T., Pérez-Lanzón, M., Ho, X. D., Léveillé, N., Melo, S. A., et al. (2017). Blocking tumor-educated MSC paracrine activity halts osteosarcoma progression. Clin. Cancer Res. 23, 3721–3733. doi: 10.1158/1078-0432.Ccr-16-2726
Belisario, D. C., Akman, M., Godel, M., Campani, V., Patrizio, M. P., Scotti, L., et al. (2020). ABCA1/ABCB1 ratio determines chemo- and immune-sensitivity in human osteosarcoma. Cells 9:647. doi: 10.3390/cells9030647
Berkmann, J. C., Herrera Martin, A. X., Pontremoli, C., Zheng, K., Bucher, C. H., Ellinghaus, A., et al. (2020). In vivo validation of spray-dried mesoporous bioactive glass microspheres acting as prolonged local release systems for BMP-2 to support bone regeneration. Pharmaceutics 12:823. doi: 10.3390/pharmaceutics12090823
Bhattasali, O., Vo, A. T., Roth, M., Geller, D., Randall, R. L., Gorlick, R., et al. (2015). Variability in the reported management of pulmonary metastases in osteosarcoma. Cancer Med. 4, 523–531. doi: 10.1002/cam4.407
Bierie, B., and Moses, H. L. (2006). Tumour microenvironment: TGFbeta: the molecular Jekyll and Hyde of cancer. Nat. Rev. Cancer 6, 506–520. doi: 10.1038/nrc1926
Burkus, J. K., Gornet, M. F., Dickman, C. A., and Zdeblick, T. A. (2002). Anterior lumbar interbody fusion using rhBMP-2 with tapered interbody cages. J. Spinal Disord. Tech. 15, 337–349. doi: 10.1097/00024720-200210000-00001
Cahill, K. S., Chi, J. H., Day, A., and Claus, E. B. (2009). Prevalence, complications, and hospital charges associated with use of bone-morphogenetic proteins in spinal fusion procedures. JAMA 302, 58–66. doi: 10.1001/jama.2009.956
Caplan, A. I., and Correa, D. (2011). The MSC: an injury drugstore. Cell Stem Cell 9, 11–15. doi: 10.1016/j.stem.2011.06.008
Carpio, L., Gladu, J., Goltzman, D., and Rabbani, S. A. (2001). Induction of osteoblast differentiation indexes by PTHrP in MG-63 cells involves multiple signaling pathways. Am. J. Physiol. Endocrinol. Metab. 281, E489–E499. doi: 10.1152/ajpendo.2001.281.3.E489
Chandar, N., Billig, B., McMaster, J., and Novak, J. (1992). Inactivation of p53 gene in human and murine osteosarcoma cells. Br. J. Cancer 65, 208–214. doi: 10.1038/bjc.1992.43
Chen, C., Zhao, M., Tian, A., Zhang, X., Yao, Z., and Ma, X. (2015). Aberrant activation of Wnt/β-catenin signaling drives proliferation of bone sarcoma cells. Oncotarget 6, 17570–17583. doi: 10.18632/oncotarget.4100
Chen, X., Bahrami, A., Pappo, A., Easton, J., Dalton, J., Hedlund, E., et al. (2014). Recurrent somatic structural variations contribute to tumorigenesis in pediatric osteosarcoma. Cell Rep. 7, 104–112. doi: 10.1016/j.celrep.2014.03.003
Cooper, G. S., and Kou, T. D. (2013). Risk of cancer after lumbar fusion surgery with recombinant human bone morphogenic protein-2 (rh-BMP-2). Spine (Phila Pa 1976) 38, 1862–1868. doi: 10.1097/BRS.0b013e3182a3d3b4
Corre, I., Verrecchia, F., Crenn, V., Redini, F., and Trichet, V. (2020). The osteosarcoma microenvironment: a complex but targetable ecosystem. Cells 9:976. doi: 10.3390/cells9040976
Cortini, M., Avnet, S., and Baldini, N. (2017). Mesenchymal stroma: role in osteosarcoma progression. Cancer Lett. 405, 90–99. doi: 10.1016/j.canlet.2017.07.024
Costa, E. C., Moreira, A. F., de Melo-Diogo, D., Gaspar, V. M., Carvalho, M. P., and Correia, I. J. (2016). 3D tumor spheroids: an overview on the tools and techniques used for their analysis. Biotechnol. Adv. 34, 1427–1441. doi: 10.1016/j.biotechadv.2016.11.002
Crisan, M., Yap, S., Casteilla, L., Chen, C. W., Corselli, M., Park, T. S., et al. (2008). A perivascular origin for mesenchymal stem cells in multiple human organs. Cell Stem Cell 3, 301–313. doi: 10.1016/j.stem.2008.07.003
da Silva Meirelles, L., Chagastelles, P. C., and Nardi, N. B. (2006). Mesenchymal stem cells reside in virtually all post-natal organs and tissues. J. Cell Sci. 119(Pt 11), 2204–2213. doi: 10.1242/jcs.02932
Dai, Q. S., Zhou, H. Y., Wu, Z. H., Long, J. T., Shao, N., Cheang, T. Y., et al. (2015). Osterix transcriptional factor is involved in the metastasis of human breast cancers. Oncol. Lett. 10, 1870–1874. doi: 10.3892/ol.2015.3448
de Jesus Perez, V. A., Alastalo, T. P., Wu, J. C., Axelrod, J. D., Cooke, J. P., Amieva, M., et al. (2009). Bone morphogenetic protein 2 induces pulmonary angiogenesis via Wnt-beta-catenin and Wnt-RhoA-Rac1 pathways. J. Cell Biol. 184, 83–99. doi: 10.1083/jcb.200806049
Deng, Q., Li, P., Che, M., Liu, J., Biswas, S., Ma, G., et al. (2019). Activation of hedgehog signaling in mesenchymal stem cells induces cartilage and bone tumor formation via Wnt/beta-Catenin. eLife 8:e50208. doi: 10.7554/eLife.50208
Deryugina, E. I., and Quigley, J. P. (2006). Matrix metalloproteinases and tumor metastasis. Cancer Metastasis Rev. 25, 9–34. doi: 10.1007/s10555-006-7886-9
Devarasetty, M., Wang, E., Soker, S., and Skardal, A. (2017). Mesenchymal stem cells support growth and organization of host-liver colorectal-tumor organoids and possibly resistance to chemotherapy. Biofabrication 9:021002. doi: 10.1088/1758-5090/aa7484
Dominici, M., Le Blanc, K., Mueller, I., Slaper-Cortenbach, I., Marini, F., Krause, D., et al. (2006). Minimal criteria for defining multipotent mesenchymal stromal cells. The international society for cellular therapy position statement. Cytotherapy 8, 315–317. doi: 10.1080/14653240600855905
Ducy, P., Zhang, R., Geoffroy, V., Ridall, A. L., and Karsenty, G. (1997). Osf2/Cbfa1: a transcriptional activator of osteoblast differentiation. Cell 89, 747–754. doi: 10.1016/s0092-8674(00)80257-3
Dvorak, H. F. (1986). Tumors: wounds that do not heal—similarities between tumor stroma generation and wound healing. N. Engl. J. Med. 315, 1650–1659. doi: 10.1056/nejm198612253152606
Fahim, D. K., Whitehead, W. E., Curry, D. J., Dauser, R. C., Luerssen, T. G., and Jea, A. (2010). Routine use of recombinant human bone morphogenetic protein-2 in posterior fusions of the pediatric spine: safety profile and efficacy in the early postoperative period. Neurosurgery 67, 1195–1204;discussion1204. doi: 10.1227/NEU.0b013e3181f258ba
Fontanella, R., Pelagalli, A., Nardelli, A., D’Alterio, C., Ieranò, C., Cerchia, L., et al. (2016). A novel antagonist of CXCR4 prevents bone marrow-derived mesenchymal stem cell-mediated osteosarcoma and hepatocellular carcinoma cell migration and invasion. Cancer Lett. 370, 100–107. doi: 10.1016/j.canlet.2015.10.018
Fontoura, J. C., Viezzer, C., Dos Santos, F. G., Ligabue, R. A., Weinlich, R., Puga, R. D., et al. (2020). Comparison of 2D and 3D cell culture models for cell growth, gene expression and drug resistance. Mater. Sci. Eng. C Mater. Biol. Appl. 107:110264. doi: 10.1016/j.msec.2019.110264
Gauthaman, K., Yee, F. C., Cheyyatraivendran, S., Biswas, A., Choolani, M., and Bongso, A. (2012). Human umbilical cord Wharton’s jelly stem cell (hWJSC) extracts inhibit cancer cell growth in vitro. J. Cell Biochem. 113, 2027–2039. doi: 10.1002/jcb.24073
Gill, J., Connolly, P., Roth, M., Chung, S. H., Zhang, W., Piperdi, S., et al. (2017). The effect of bone morphogenetic protein-2 on osteosarcoma metastasis. PLoS One 12:e0173322. doi: 10.1371/journal.pone.0173322
Gupta, S., Ito, T., Alex, D., Vanderbilt, C. M., Chang, J. C., Islamdoust, N., et al. (2019). RUNX2 (6p21.1) amplification in osteosarcoma. Hum. Pathol. 94, 23–28. doi: 10.1016/j.humpath.2019.09.010
Gyukity-Sebestyén, E., Harmati, M., Dobra, G., Németh, I. B., Mihály, J., and Zvara, Á, et al. (2019). Melanoma-derived exosomes induce PD-1 overexpression and tumor progression via mesenchymal stem cell oncogenic reprogramming. Front. Immunol. 10:2459. doi: 10.3389/fimmu.2019.02459
Han, Y., Feng, H., Sun, J., Liang, X., Wang, Z., Xing, W., et al. (2019). Lkb1 deletion in periosteal mesenchymal progenitors induces osteogenic tumors through mTORC1 activation. J. Clin. Invest. 129, 1895–1909. doi: 10.1172/JCI124590
Hashimoto, K., Kaito, T., Furuya, M., Seno, S., Okuzaki, D., Kikuta, J., et al. (2020). In vivo dynamic analysis of BMP-2-induced ectopic bone formation. Sci. Rep. 10:4751. doi: 10.1038/s41598-020-61825-2
Haydon, R. C., Luu, H. H., and He, T. C. (2007). Osteosarcoma and osteoblastic differentiation: a new perspective on oncogenesis. Clin. Orthop. Relat. Res. 454, 237–246. doi: 10.1097/BLO.0b013e31802b683c
Henriksson, I., Gatenholm, P., and Hägg, D. A. (2017). Increased lipid accumulation and adipogenic gene expression of adipocytes in 3D bioprinted nanocellulose scaffolds. Biofabrication 9:015022. doi: 10.1088/1758-5090/aa5c1c
Hickman, J. A., Graeser, R., de Hoogt, R., Vidic, S., Brito, C., Gutekunst, M., et al. (2014). Three-dimensional models of cancer for pharmacology and cancer cell biology: capturing tumor complexity in vitro/ex vivo. Biotechnol. J. 9, 1115–1128. doi: 10.1002/biot.201300492
Hiepen, C., Benn, A., Denkis, A., Lukonin, I., Weise, C., Boergermann, J. H., et al. (2014). BMP2-induced chemotaxis requires PI3K p55γ/p110α-dependent phosphatidylinositol (3,4,5)-triphosphate production and LL5β recruitment at the cytocortex. BMC Biol. 12:43. doi: 10.1186/1741-7007-12-43
Hiyama, A., Sakai, D., Tanaka, M., Arai, F., Nakajima, D., Abe, K., et al. (2011). The relationship between the Wnt/beta-catenin and TGF-beta/BMP signals in the intervertebral disc cell. J. Cell Physiol. 226, 1139–1148. doi: 10.1002/jcp.22438
Honma, Y., and Akimoto, M. (2007). Therapeutic strategy using phenotypic modulation of cancer cells by differentiation-inducing agents. Cancer Sci. 98, 1643–1651. doi: 10.1111/j.1349-7006.2007.00575.x
Horwitz, E. M., Le Blanc, K., Dominici, M., Mueller, I., Slaper-Cortenbach, I., Marini, F. C., et al. (2005). Clarification of the nomenclature for MSC: the international society for cellular therapy position statement. Cytotherapy 7, 393–395. doi: 10.1080/14653240500319234
Hsu, Y. H., Lin, R. M., Chiu, Y. S., Liu, W. L., and Huang, K. Y. (2020). Effects of IL-1β, IL-20, and BMP-2 on intervertebral disc inflammation under hypoxia. J. Clin. Med. 9:140. doi: 10.3390/jcm9010140
Huang, M. E., Ye, Y. C., Chen, S. R., Chai, J. R., Lu, J. X., Zhoa, L., et al. (1988). Use of all-trans retinoic acid in the treatment of acute promyelocytic leukemia. Blood 72, 567–572.
Huang, Y. L., and Niehrs, C. (2014). Polarized Wnt signaling regulates ectodermal cell fate in Xenopus. Dev. Cell 29, 250–257. doi: 10.1016/j.devcel.2014.03.015
Hui, L., and Chen, Y. (2015). Tumor microenvironment: sanctuary of the devil. Cancer Lett. 368, 7–13. doi: 10.1016/j.canlet.2015.07.039
James, A. W., LaChaud, G., Shen, J., Asatrian, G., Nguyen, V., Zhang, X., et al. (2016). A review of the clinical side effects of bone morphogenetic protein-2. Tissue Eng. Part B Rev. 22, 284–297. doi: 10.1089/ten.TEB.2015.0357
Ji, Y., Xu, G. P., Zhang, Z. P., Xia, J. J., Yan, J. L., and Pan, S. H. (2010). BMP-2/PLGA delayed-release microspheres composite graft, selection of bone particulate diameters, and prevention of aseptic inflammation for bone tissue engineering. Ann. Biomed. Eng. 38, 632–639. doi: 10.1007/s10439-009-9888-6
Jia, Y., Ding, X., Zhou, L., Zhang, L., and Yang, X. (2021). Mesenchymal stem cells-derived exosomal microRNA-139-5p restrains tumorigenesis in bladder cancer by targeting PRC1. Oncogene 40, 246–261. doi: 10.1038/s41388-020-01486-7
Jin, Y., Tipoe, G. L., Liong, E. C., Lau, T. Y., Fung, P. C., and Leung, K. M. (2001). Overexpression of BMP-2/4, -5 and BMPR-IA associated with malignancy of oral epithelium. Oral Oncol. 37, 225–233. doi: 10.1016/s1368-8375(00)00087-7
Kang, M. H., Oh, S. C., Lee, H. J., Kang, H. N., Kim, J. L., Kim, J. S., et al. (2011). Metastatic function of BMP-2 in gastric cancer cells: the role of PI3K/AKT, MAPK, the NF-κB pathway, and MMP-9 expression. Exp. Cell Res. 317, 1746–1762. doi: 10.1016/j.yexcr.2011.04.006
Kansara, M., Teng, M. W., Smyth, M. J., and Thomas, D. M. (2014). Translational biology of osteosarcoma. Nat. Rev. Cancer 14, 722–735. doi: 10.1038/nrc3838
Kendal, J. K., Singla, A., Affan, A., Hildebrand, K., Al-Ani, A., Ungrin, M., et al. (2020). Is use of BMP-2 associated with tumor growth and osteoblastic differentiation in murine models of osteosarcoma? Clin. Orthop. Relat. Res. 478, 2921–2933. doi: 10.1097/corr.0000000000001422
Khakoo, A. Y., Pati, S., Anderson, S. A., Reid, W., Elshal, M. F., Rovira, I. I., et al. (2006). Human mesenchymal stem cells exert potent antitumorigenic effects in a model of Kaposi’s sarcoma. J. Exp. Med. 203, 1235–1247. doi: 10.1084/jem.20051921
Kidd, S., Spaeth, E., Dembinski, J. L., Dietrich, M., Watson, K., Klopp, A., et al. (2009). Direct evidence of mesenchymal stem cell tropism for tumor and wounding microenvironments using in vivo bioluminescent imaging. Stem Cells 27, 2614–2623. doi: 10.1002/stem.187
Klein, M. J., and Siegal, G. P. (2006). Osteosarcoma: anatomic and histologic variants. Am. J. Clin. Pathol. 125, 555–581. doi: 10.1309/uc6k-qhld-9lv2-kenn
Kuhn, N. Z., and Tuan, R. S. (2010). Regulation of stemness and stem cell niche of mesenchymal stem cells: implications in tumorigenesis and metastasis. J. Cell Physiol. 222, 268–277. doi: 10.1002/jcp.21940
Lad, S. P., Bagley, J. H., Karikari, I. O., Babu, R., Ugiliweneza, B., Kong, M., et al. (2013). Cancer after spinal fusion: the role of bone morphogenetic protein. Neurosurgery 73, 440–449. doi: 10.1227/NEU.0000000000000018
Le Nail, L. R., Brennan, M., Rosset, P., Deschaseaux, F., Piloquet, P., Pichon, O., et al. (2018). Comparison of tumor- and bone marrow-derived mesenchymal stromal/stem cells from patients with high-grade osteosarcoma. Int. J. Mol. Sci. 19, 707. doi: 10.3390/ijms19030707
Lecanda, F., Avioli, L. V., and Cheng, S. L. (1997). Regulation of bone matrix protein expression and induction of differentiation of human osteoblasts and human bone marrow stromal cells by bone morphogenetic protein-2. J. Cell Biochem. 67, 386–396.
Lerner, U. H., and Ohlsson, C. (2015). The WNT system: background and its role in bone. J. Intern. Med. 277, 630–649. doi: 10.1111/joim.12368
Lindsay, S. L., and Barnett, S. C. (2017). Are nestin-positive mesenchymal stromal cells a better source of cells for CNS repair? Neurochem. Int. 106, 101–107. doi: 10.1016/j.neuint.2016.08.001
Liu, F., Kohlmeier, S., and Wang, C. Y. (2008). Wnt signaling and skeletal development. Cell Signal. 20, 999–1009. doi: 10.1016/j.cellsig.2007.11.011
Lv, F. J., Tuan, R. S., Cheung, K. M., and Leung, V. Y. (2014). Concise review: the surface markers and identity of human mesenchymal stem cells. Stem Cells 32, 1408–1419. doi: 10.1002/stem.1681
Mao, S., He, J., Zhao, Y., Liu, T., Xie, F., Yang, H., et al. (2020). Bioprinting of patient-derived in vitro intrahepatic cholangiocarcinoma tumor model: establishment, evaluation and anti-cancer drug testing. Biofabrication 12:045014. doi: 10.1088/1758-5090/aba0c3
Marina, N. M., Smeland, S., Bielack, S. S., Bernstein, M., Jovic, G., Krailo, M. D., et al. (2016). Comparison of MAPIE versus MAP in patients with a poor response to preoperative chemotherapy for newly diagnosed high-grade osteosarcoma (EURAMOS-1): an open-label, international, randomised controlled trial. Lancet Oncol. 17, 1396–1408. doi: 10.1016/s1470-2045(16)30214-5
Mirabello, L., Troisi, R. J., and Savage, S. A. (2009a). International osteosarcoma incidence patterns in children and adolescents, middle ages and elderly persons. Int. J. Cancer 125, 229–234. doi: 10.1002/ijc.24320
Mirabello, L., Troisi, R. J., and Savage, S. A. (2009b). Osteosarcoma incidence and survival rates from 1973 to 2004: data from the surveillance, epidemiology, and end results program. Cancer 115, 1531–1543. doi: 10.1002/cncr.24121
Mohseny, A. B., Machado, I., Cai, Y., Schaefer, K. L., Serra, M., Hogendoorn, P. C., et al. (2011). Functional characterization of osteosarcoma cell lines provides representative models to study the human disease. Lab. Invest. 91, 1195–1205. doi: 10.1038/labinvest.2011.72
Mohseny, A. B., Szuhai, K., Romeo, S., Buddingh, E. P., Briaire-de Bruijn, I., de Jong, D., et al. (2009). Osteosarcoma originates from mesenchymal stem cells in consequence of aneuploidization and genomic loss of Cdkn2. J. Pathol. 219, 294–305. doi: 10.1002/path.2603
Moon, J. S., Ko, H. M., Park, J. I., Kim, J. H., Kim, S. H., and Kim, M. S. (2018). Inhibition of human mesenchymal stem cell proliferation via Wnt signaling activation. J. Cell Biochem. 119, 1670–1678. doi: 10.1002/jcb.26326
Nakashima, K., Zhou, X., Kunkel, G., Zhang, Z., Deng, J. M., Behringer, R. R., et al. (2002). The novel zinc finger-containing transcription factor osterix is required for osteoblast differentiation and bone formation. Cell 108, 17–29. doi: 10.1016/s0092-8674(01)00622-5
Nishimori, H., Ehata, S., Suzuki, H. I., Katsuno, Y., and Miyazono, K. (2012). Prostate cancer cells and bone stromal cells mutually interact with each other through bone morphogenetic protein-mediated signals. J. Biol. Chem. 287, 20037–20046. doi: 10.1074/jbc.M112.353094
Oliveira, M. I., Pinto, M. L., Gonçalves, R. M., Martins, M. C. L., Santos, S. G., and Barbosa, M. A. (2017). Adsorbed fibrinogen stimulates TLR-4 on monocytes and induces BMP-2 expression. Acta Biomater. 49, 296–305. doi: 10.1016/j.actbio.2016.11.034
Oryan, A., Alidadi, S., Moshiri, A., and Bigham-Sadegh, A. (2014). Bone morphogenetic proteins: a powerful osteoinductive compound with non-negligible side effects and limitations. Biofactors 40, 459–481. doi: 10.1002/biof.1177
Otsu, K., Das, S., Houser, S. D., Quadri, S. K., Bhattacharya, S., and Bhattacharya, J. (2009). Concentration-dependent inhibition of angiogenesis by mesenchymal stem cells. Blood 113, 4197–4205. doi: 10.1182/blood-2008-09-176198
Owen, M., and Friedenstein, A. J. (1988). Stromal stem cells: marrow-derived osteogenic precursors. Ciba Found Symp. 136, 42–60. doi: 10.1002/9780470513637.ch4
Papalexi, E., and Satija, R. (2018). Single-cell RNA sequencing to explore immune cell heterogeneity. Nat. Rev. Immunol. 18, 35–45. doi: 10.1038/nri.2017.76
Pardali, E., Makowski, L. M., Leffers, M., Borgscheiper, A., and Waltenberger, J. (2018). BMP-2 induces human mononuclear cell chemotaxis and adhesion and modulates monocyte-to-macrophage differentiation. J. Cell Mol. Med. 22, 5429–5438. doi: 10.1111/jcmm.13814
Pautke, C., Schieker, M., Tischer, T., Kolk, A., Neth, P., Mutschler, W., et al. (2004). Characterization of osteosarcoma cell lines MG-63, Saos-2 and U-2 OS in comparison to human osteoblasts. Anticancer Res. 24, 3743–3748.
Pavlou, M., Shah, M., Gikas, P., Briggs, T., Roberts, S. J., and Cheema, U. (2019). Osteomimetic matrix components alter cell migration and drug response in a 3D tumour-engineered osteosarcoma model. Acta Biomater. 96, 247–257. doi: 10.1016/j.actbio.2019.07.011
Peister, A., Mellad, J. A., Larson, B. L., Hall, B. M., Gibson, L. F., and Prockop, D. J. (2004). Adult stem cells from bone marrow (MSCs) isolated from different strains of inbred mice vary in surface epitopes, rates of proliferation, and differentiation potential. Blood 103, 1662–1668. doi: 10.1182/blood-2003-09-3070
Pereira, B. P., Zhou, Y., Gupta, A., Leong, D. T., Aung, K. Z., Ling, L., et al. (2009). Runx2, p53, and pRB status as diagnostic parameters for deregulation of osteoblast growth and differentiation in a new pre-chemotherapeutic osteosarcoma cell line (OS1). J. Cell Physiol. 221, 778–788. doi: 10.1002/jcp.21921
Pittenger, M. F., Mackay, A. M., Beck, S. C., Jaiswal, R. K., Douglas, R., Mosca, J. D., et al. (1999). Multilineage potential of adult human mesenchymal stem cells. Science 284, 143–147. doi: 10.1126/science.284.5411.143
Postiglione, L., Domenico, G. D., Montagnani, S., Spigna, G. D., Salzano, S., Castaldo, C., et al. (2003). Granulocyte-macrophage colony-stimulating factor (GM-CSF) induces the osteoblastic differentiation of the human osteosarcoma cell line SaOS-2. Calcif. Tissue Int. 72, 85–97. doi: 10.1007/s00223-001-2088-5
Pratap, J., Galindo, M., Zaidi, S. K., Vradii, D., Bhat, B. M., Robinson, J. A., et al. (2003). Cell growth regulatory role of Runx2 during proliferative expansion of preosteoblasts. Cancer Res. 63, 5357–5362.
Quante, M., Tu, S. P., Tomita, H., Gonda, T., Wang, S. S., Takashi, S., et al. (2011). Bone marrow-derived myofibroblasts contribute to the mesenchymal stem cell niche and promote tumor growth. Cancer Cell 19, 257–272. doi: 10.1016/j.ccr.2011.01.020
Rampazzo, E., Dettin, M., Maule, F., Scabello, A., Calvanese, L., D’Auria, G., et al. (2017). A synthetic BMP-2 mimicking peptide induces glioblastoma stem cell differentiation. Biochim. Biophys. Acta Gen. Subj. 1861, 2282–2292. doi: 10.1016/j.bbagen.2017.07.001
Reddi, A. H. (2005). BMPs: from bone morphogenetic proteins to body morphogenetic proteins. Cytokine Growth Factor Rev. 16, 249–250. doi: 10.1016/j.cytogfr.2005.04.003
Ricci, B., Tycksen, E., Celik, H., Belle, J. I., Fontana, F., Civitelli, R., et al. (2020). Osterix-Cre marks distinct subsets of CD45- and CD45+ stromal populations in extra-skeletal tumors with pro-tumorigenic characteristics. eLife 9:e54659. doi: 10.7554/eLife.54659
Rici, R. E., Alcântara, D., Fratini, P., Wenceslau, C. V., Ambrósio, C. E., Miglino, M. A., et al. (2012). Mesenchymal stem cells with rhBMP-2 inhibits the growth of canine osteosarcoma cells. BMC Vet. Res. 8:17. doi: 10.1186/1746-6148-8-17
Rici, R. E. G., Will, S., Luna, A. C. L., Melo, L. F., Santos, A. C., Rodrigues, R. F., et al. (2018). Combination therapy of canine osteosarcoma with canine bone marrow stem cells, bone morphogenetic protein and carboplatin in an in vivo model. Vet. Comp. Oncol. 16, 478–488. doi: 10.1111/vco.12404
Rickard, D. J., Sullivan, T. A., Shenker, B. J., Leboy, P. S., and Kazhdan, I. (1994). Induction of rapid osteoblast differentiation in rat bone marrow stromal cell cultures by dexamethasone and BMP-2. Dev. Biol. 161, 218–228. doi: 10.1006/dbio.1994.1022
Ridge, S. M., Sullivan, F. J., and Glynn, S. A. (2017). Mesenchymal stem cells: key players in cancer progression. Mol. Cancer 16:31. doi: 10.1186/s12943-017-0597-8
Ritter, J., and Bielack, S. S. (2010). Osteosarcoma. Ann. Oncol. 21(Suppl. 7), vii320–vii325. doi: 10.1093/annonc/mdq276
Roos, A., Satterfield, L., Zhao, S., Fuja, D., Shuck, R., Hicks, M. J., et al. (2015). Loss of Runx2 sensitises osteosarcoma to chemotherapy-induced apoptosis. Br. J. Cancer 113, 1289–1297. doi: 10.1038/bjc.2015.305
Rosen, V. (2009). BMP2 signaling in bone development and repair. Cytokine Growth Factor Rev. 20, 475–480. doi: 10.1016/j.cytogfr.2009.10.018
Rubio, R., Gutierrez-Aranda, I., Sáez-Castillo, A. I., Labarga, A., Rosu-Myles, M., Gonzalez-Garcia, S., et al. (2013). The differentiation stage of p53-Rb-deficient bone marrow mesenchymal stem cells imposes the phenotype of in vivo sarcoma development. Oncogene 32, 4970–4980. doi: 10.1038/onc.2012.507
Sacchetti, B., Funari, A., Michienzi, S., Di Cesare, S., Piersanti, S., Saggio, I., et al. (2007). Self-renewing osteoprogenitors in bone marrow sinusoids can organize a hematopoietic microenvironment. Cell 131, 324–336. doi: 10.1016/j.cell.2007.08.025
Sadikovic, B., Thorner, P., Chilton-Macneill, S., Martin, J. W., Cervigne, N. K., Squire, J., et al. (2010). Expression analysis of genes associated with human osteosarcoma tumors shows correlation of RUNX2 overexpression with poor response to chemotherapy. BMC Cancer 10:202. doi: 10.1186/1471-2407-10-202
Seo, B. B., Koh, J. T., and Song, S. C. (2017). Tuning physical properties and BMP-2 release rates of injectable hydrogel systems for an optimal bone regeneration effect. Biomaterials 122, 91–104. doi: 10.1016/j.biomaterials.2017.01.016
Shi, Y., Du, L., Lin, L., and Wang, Y. (2017). Tumour-associated mesenchymal stem/stromal cells: emerging therapeutic targets. Nat. Rev. Drug Discov. 16, 35–52. doi: 10.1038/nrd.2016.193
Simões Sato, A. Y., Bub, G. L., and Campos, A. H. (2014). BMP-2 and -4 produced by vascular smooth muscle cells from atherosclerotic lesions induce monocyte chemotaxis through direct BMPRII activation. Atherosclerosis 235, 45–55. doi: 10.1016/j.atherosclerosis.2014.03.030
Simpson, S., Dunning, M. D., de Brot, S., Grau-Roma, L., Mongan, N. P., and Rutland, C. S. (2017). Comparative review of human and canine osteosarcoma: morphology, epidemiology, prognosis, treatment and genetics. Acta Vet. Scand. 59:71. doi: 10.1186/s13028-017-0341-9
Smeland, S., Bielack, S. S., Whelan, J., Bernstein, M., Hogendoorn, P., Krailo, M. D., et al. (2019). Survival and prognosis with osteosarcoma: outcomes in more than 2000 patients in the EURAMOS-1 (European and American Osteosarcoma Study) cohort. Eur. J. Cancer 109, 36–50. doi: 10.1016/j.ejca.2018.11.027
Sotobori, T., Ueda, T., Myoui, A., Yoshioka, K., Nakasaki, M., Yoshikawa, H., et al. (2006). Bone morphogenetic protein-2 promotes the haptotactic migration of murine osteoblastic and osteosarcoma cells by enhancing incorporation of integrin beta1 into lipid rafts. Exp. Cell Res. 312, 3927–3938. doi: 10.1016/j.yexcr.2006.08.024
Spaeth, E., Klopp, A., Dembinski, J., Andreeff, M., and Marini, F. (2008). Inflammation and tumor microenvironments: defining the migratory itinerary of mesenchymal stem cells. Gene Ther. 15, 730–738. doi: 10.1038/gt.2008.39
Steinhart, Z., and Angers, S. (2018). Wnt signaling in development and tissue homeostasis. Development 145:dev146589. doi: 10.1242/dev.146589
Stiller, C. A., Bielack, S. S., Jundt, G., and Steliarova-Foucher, E. (2006). Bone tumours in European children and adolescents, 1978-1997. Report from the Automated Childhood Cancer Information System project. Eur. J. Cancer 42, 2124–2135. doi: 10.1016/j.ejca.2006.05.015
Su, X., Ao, L., Shi, Y., Johnson, T. R., Fullerton, D. A., and Meng, X. (2011). Oxidized low density lipoprotein induces bone morphogenetic protein-2 in coronary artery endothelial cells via Toll-like receptors 2 and 4. J. Biol. Chem. 286, 12213–12220. doi: 10.1074/jbc.M110.214619
Sun, L., Yang, H., Wang, Y., Zhang, X., Jin, B., Xie, F., et al. (2020). Application of a 3D bioprinted hepatocellular carcinoma cell model in antitumor drug research. Front. Oncol. 10:878. doi: 10.3389/fonc.2020.00878
Tang, N., Song, W. X., Luo, J., Haydon, R. C., and He, T. C. (2008). Osteosarcoma development and stem cell differentiation. Clin. Orthop. Relat. Res. 466, 2114–2130. doi: 10.1007/s11999-008-0335-z
Thomas, D. M., Carty, S. A., Piscopo, D. M., Lee, J. S., Wang, W. F., Forrester, W. C., et al. (2001). The retinoblastoma protein acts as a transcriptional coactivator required for osteogenic differentiation. Mol. Cell 8, 303–316. doi: 10.1016/s1097-2765(01)00327-6
Tian, H., Zhao, J., Brochmann, E. J., Wang, J. C., and Murray, S. S. (2017). Bone morphogenetic protein-2 and tumor growth: diverse effects and possibilities for therapy. Cytokine Growth Factor Rev. 34, 73–91. doi: 10.1016/j.cytogfr.2017.01.002
Tian, H., Zhou, T., Chen, H., Li, C., Jiang, Z., Lao, L., et al. (2019). Bone morphogenetic protein-2 promotes osteosarcoma growth by promoting epithelial-mesenchymal transition (EMT) through the Wnt/β-catenin signaling pathway. J. Orthop. Res. 37, 1638–1648. doi: 10.1002/jor.24244
Tsukasaki, M., and Takayanagi, H. (2019). Osteoimmunology: evolving concepts in bone-immune interactions in health and disease. Nat. Rev. Immunol. 19, 626–642. doi: 10.1038/s41577-019-0178-8
Tu, B., Du, L., Fan, Q. M., Tang, Z., and Tang, T. T. (2012). STAT3 activation by IL-6 from mesenchymal stem cells promotes the proliferation and metastasis of osteosarcoma. Cancer Lett. 325, 80–88. doi: 10.1016/j.canlet.2012.06.006
Twine, N. A., Chen, L., Pang, C. N., Wilkins, M. R., and Kassem, M. (2014). Identification of differentiation-stage specific markers that define the ex vivo osteoblastic phenotype. Bone 67, 23–32. doi: 10.1016/j.bone.2014.06.027
Urist, M. R. (1965). Bone: formation by autoinduction. Science 150, 893–899. doi: 10.1126/science.150.3698.893
US Food and Drug Administration (2002). Premarket Approval (PMA): Infuse Bone Graft/lt-Cage Lumbar Tapered Fusion Device. Available online at: https://www.accessdata.fda.gov/scripts/cdrh/cfdocs/cfpma/pma.cfm?id=P000058 (accessed November 26, 2008).
van der Deen, M., Akech, J., Lapointe, D., Gupta, S., Young, D. W., Montecino, M. A., et al. (2012). Genomic promoter occupancy of runt-related transcription factor RUNX2 in Osteosarcoma cells identifies genes involved in cell adhesion and motility. J. Biol. Chem. 287, 4503–4517. doi: 10.1074/jbc.M111.287771
Villanueva, F., Araya, H., Briceno, P., Varela, N., Stevenson, A., Jerez, S., et al. (2019). The cancer-related transcription factor RUNX2 modulates expression and secretion of the matricellular protein osteopontin in osteosarcoma cells to promote adhesion to endothelial pulmonary cells and lung metastasis. J. Cell Physiol. 234, 13659–13679. doi: 10.1002/jcp.28046
Walkley, C. R., Qudsi, R., Sankaran, V. G., Perry, J. A., Gostissa, M., Roth, S. I., et al. (2008). Conditional mouse osteosarcoma, dependent on p53 loss and potentiated by loss of Rb, mimics the human disease. Genes Dev. 22, 1662–1676. doi: 10.1101/gad.1656808
Wang, L., Park, P., La Marca, F., Than, K., Rahman, S., and Lin, C. Y. (2013). Bone formation induced by BMP-2 in human osteosarcoma cells. Int. J. Oncol. 43, 1095–1102. doi: 10.3892/ijo.2013.2030
Wang, L., Park, P., Zhang, H., La Marca, F., Claeson, A., Than, K., et al. (2012). BMP-2 inhibits tumor growth of human renal cell carcinoma and induces bone formation. Int. J. Cancer 131, 1941–1950. doi: 10.1002/ijc.27444
Wang, L., Park, P., Zhang, H., La Marca, F., Claeson, A., Valdivia, J., et al. (2011). BMP-2 inhibits the tumorigenicity of cancer stem cells in human osteosarcoma OS99-1 cell line. Cancer Biol. Ther. 11, 457–463. doi: 10.4161/cbt.11.5.14372
Wang, Y., Chen, X., Cao, W., and Shi, Y. (2014a). Plasticity of mesenchymal stem cells in immunomodulation: pathological and therapeutic implications. Nat. Immunol. 15, 1009–1016. doi: 10.1038/ni.3002
Wang, Y., Li, Y. P., Paulson, C., Shao, J. Z., Zhang, X., Wu, M., et al. (2014b). Wnt and the Wnt signaling pathway in bone development and disease. Front. Biosci. 19:379–407.
Waterman, R. S., Henkle, S. L., and Betancourt, A. M. (2012). Mesenchymal stem cell 1 (MSC1)-based therapy attenuates tumor growth whereas MSC2-treatment promotes tumor growth and metastasis. PLoS One 7:e45590. doi: 10.1371/journal.pone.0045590
Weiss, K. R. (2015). To B(MP-2) or not to B(MP-2): cytokines and tumor surgery—response. Clin. Cancer Res. 21:4023. doi: 10.1158/1078-0432.Ccr-15-0875
Whiteside, T. L. (2018). Exosome and mesenchymal stem cell cross-talk in the tumor microenvironment. Semin. Immunol. 35, 69–79. doi: 10.1016/j.smim.2017.12.003
Wildemann, B., Kandziora, F., Krummrey, G., Palasdies, N., Haas, N. P., Raschke, M., et al. (2004). Local and controlled release of growth factors (combination of IGF-I and TGF-beta I, and BMP-2 alone) from a polylactide coating of titanium implants does not lead to ectopic bone formation in sheep muscle. J. Control Release 95, 249–256. doi: 10.1016/j.jconrel.2003.11.014
Wozney, J. M., and Rosen, V. (1998). Bone morphogenetic protein and bone morphogenetic protein gene family in bone formation and repair. Clin. Orthop. Relat. Res. 346, 26–37.
Wozney, J. M., Rosen, V., Celeste, A. J., Mitsock, L. M., Whitters, M. J., Kriz, R. W., et al. (1988). Novel regulators of bone formation: molecular clones and activities. Science 242, 1528–1534. doi: 10.1126/science.3201241
Wu, G., Hunziker, E. B., Zheng, Y., Wismeijer, D., and Liu, Y. (2011). Functionalization of deproteinized bovine bone with a coating-incorporated depot of BMP-2 renders the material efficiently osteoinductive and suppresses foreign-body reactivity. Bone 49, 1323–1330. doi: 10.1016/j.bone.2011.09.046
Wu, J. B., Fu, H. Q., Huang, L. Z., Liu, A. W., and Zhang, J. X. (2011). Effects of siRNA-targeting BMP-2 on the abilities of migration and invasion of human liver cancer SMMC7721 cells and its mechanism. Cancer Gene Ther. 18, 20–25. doi: 10.1038/cgt.2010.55
Xie, B., Li, Y., Zhao, R., Xu, Y., Wu, Y., Wang, J., et al. (2018). Identification of key genes and miRNAs in osteosarcoma patients with chemoresistance by bioinformatics analysis. Biomed. Res. Int. 2018:4761064. doi: 10.1155/2018/4761064
Xie, F., Sun, L., Pang, Y., Xu, G., Jin, B., Xu, H., et al. (2021). Three-dimensional bio-printing of primary human hepatocellular carcinoma for personalized medicine. Biomaterials 265:120416. doi: 10.1016/j.biomaterials.2020.120416
Xin, T., Mao, J., Liu, L., Tang, J., Wu, L., Yu, X., et al. (2020). Programmed sustained release of recombinant human bone morphogenetic protein-2 and inorganic ion composite hydrogel as artificial periosteum. ACS Appl. Mater. Interf. 12, 6840–6851. doi: 10.1021/acsami.9b18496
Xiong, Q., Wang, X., Wang, L., Huang, Y., Tian, X., Fan, Y., et al. (2018). BMP-2 inhibits lung metastasis of osteosarcoma: an early investigation using an orthotopic model. Onco Targets Ther. 11, 7543–7553. doi: 10.2147/ott.S176724
Xu, H., Zhao, G., Zhang, Y., Jiang, H., Wang, W., Zhao, D., et al. (2019). Mesenchymal stem cell-derived exosomal microRNA-133b suppresses glioma progression via Wnt/β-catenin signaling pathway by targeting EZH2. Stem Cell Res. Ther. 10:381. doi: 10.1186/s13287-019-1446-z
Yang, C., Tian, Y., Zhao, F., Chen, Z., Su, P., Li, Y., et al. (2020). Bone microenvironment and osteosarcoma metastasis. Int. J. Mol. Sci. 21:6985. doi: 10.3390/ijms21196985
Yang, L., Yamasaki, K., Shirakata, Y., Dai, X., Tokumaru, S., Yahata, Y., et al. (2006). Bone morphogenetic protein-2 modulates Wnt and frizzled expression and enhances the canonical pathway of Wnt signaling in normal keratinocytes. J. Dermatol. Sci. 42, 111–119. doi: 10.1016/j.jdermsci.2005.12.011
Yang, X., Fullerton, D. A., Su, X., Ao, L., Cleveland, J. C. Jr., and Meng, X. (2009). Pro-osteogenic phenotype of human aortic valve interstitial cells is associated with higher levels of Toll-like receptors 2 and 4 and enhanced expression of bone morphogenetic protein 2. J. Am. Coll. Cardiol. 53, 491–500. doi: 10.1016/j.jacc.2008.09.052
Yao, B., Wang, J., Qu, S., Liu, Y., Jin, Y., Lu, J., et al. (2019). Upregulated osterix promotes invasion and bone metastasis and predicts for a poor prognosis in breast cancer. Cell Death Dis. 10:28. doi: 10.1038/s41419-018-1269-3
Yao, K., Qiu, S., Tian, L., Snider, W. D., Flannery, J. G., Schaffer, D. V., et al. (2016). Wnt regulates proliferation and neurogenic potential of Müller glial cells via a Lin28/let-7 miRNA-dependent pathway in adult mammalian retinas. Cell Rep. 17, 165–178. doi: 10.1016/j.celrep.2016.08.078
Zhang, G., Huang, P., Chen, A., He, W., Li, Z., Liu, G., et al. (2018). How BMP-2 induces EMT and breast cancer stemness through Rb and CD44? Cell Death Dis. 9:20. doi: 10.1038/s41419-017-0037-0
Zhang, H., Wang, J., Ren, T., Huang, Y., Liang, X., Yu, Y., et al. (2020). Bone marrow mesenchymal stem cell-derived exosomal miR-206 inhibits osteosarcoma progression by targeting TRA2B. Cancer Lett. 490, 54–65. doi: 10.1016/j.canlet.2020.07.008
Zhang, K., Zhou, Y., Xiao, C., Zhao, W., Wu, H., Tang, J., et al. (2019). Application of hydroxyapatite nanoparticles in tumor-associated bone segmental defect. Sci. Adv. 5:eaax6946. doi: 10.1126/sciadv.aax6946
Zhang, P., Dong, L., Yan, K., Long, H., Yang, T. T., Dong, M. Q., et al. (2013). CXCR4-mediated osteosarcoma growth and pulmonary metastasis is promoted by mesenchymal stem cells through VEGF. Oncol. Rep. 30, 1753–1761. doi: 10.3892/or.2013.2619
Zhang, R., Oyajobi, B. O., Harris, S. E., Chen, D., Tsao, C., Deng, H. W., et al. (2013). Wnt/beta-catenin signaling activates bone morphogenetic protein 2 expression in osteoblasts. Bone 52, 145–156. doi: 10.1016/j.bone.2012.09.029
Zhao, Y., Yao, R., Ouyang, L., Ding, H., Zhang, T., Zhang, K., et al. (2014). Three-dimensional printing of Hela cells for cervical tumor model in vitro. Biofabrication 6:035001. doi: 10.1088/1758-5082/6/3/035001
Zhou, Q., Gu, X., Dong, J., Zhu, C., Cai, Z., He, D., et al. (2019). The use of TLR2 modified BMSCs for enhanced bone regeneration in the inflammatory micro-environment. Artif. Cells Nanomed. Biotechnol. 47, 3329–3337. doi: 10.1080/21691401.2019.1626867
Zhou, Y., Chen, H., Li, H., and Wu, Y. (2017). 3D culture increases pluripotent gene expression in mesenchymal stem cells through relaxation of cytoskeleton tension. J. Cell Mol. Med. 21, 1073–1084. doi: 10.1111/jcmm.12946
Zhou, Y., Yang, D., Yang, Q., Lv, X., Huang, W., Zhou, Z., et al. (2020). Single-cell RNA landscape of intratumoral heterogeneity and immunosuppressive microenvironment in advanced osteosarcoma. Nat. Commun. 11:6322. doi: 10.1038/s41467-020-20059-6
Zhu, Z., Yin, J., Guan, J., Hu, B., Niu, X., Jin, D., et al. (2014). Lithium stimulates human bone marrow derived mesenchymal stem cell proliferation through GSK-3β-dependent β-catenin/Wnt pathway activation. FEBS J. 281, 5371–5389. doi: 10.1111/febs.13081
Keywords: osteosarcoma, bone morphogenetic protein-2, mesenchymal stem cells, osteogenic differentiation, osteogenesis, tumor heterogeneity, bone-marrow-derived mesenchymal stem cell
Citation: Xu C, Wang M, Zandieh-Doulabi B, Sun W, Wei L and Liu Y (2021) To B (Bone Morphogenic Protein-2) or Not to B (Bone Morphogenic Protein-2): Mesenchymal Stem Cells May Explain the Protein’s Role in Osteosarcomagenesis. Front. Cell Dev. Biol. 9:740783. doi: 10.3389/fcell.2021.740783
Received: 13 July 2021; Accepted: 11 October 2021;
Published: 17 November 2021.
Edited by:
Gustavo Cernera, University of Naples Federico II, ItalyReviewed by:
Tianyi Liu, University of California, San Francisco, United StatesCopyright © 2021 Xu, Wang, Zandieh-Doulabi, Sun, Wei and Liu. This is an open-access article distributed under the terms of the Creative Commons Attribution License (CC BY). The use, distribution or reproduction in other forums is permitted, provided the original author(s) and the copyright owner(s) are credited and that the original publication in this journal is cited, in accordance with accepted academic practice. No use, distribution or reproduction is permitted which does not comply with these terms.
*Correspondence: Yuelian Liu, eS5saXVAYWN0YS5ubA==
Disclaimer: All claims expressed in this article are solely those of the authors and do not necessarily represent those of their affiliated organizations, or those of the publisher, the editors and the reviewers. Any product that may be evaluated in this article or claim that may be made by its manufacturer is not guaranteed or endorsed by the publisher.
Research integrity at Frontiers
Learn more about the work of our research integrity team to safeguard the quality of each article we publish.