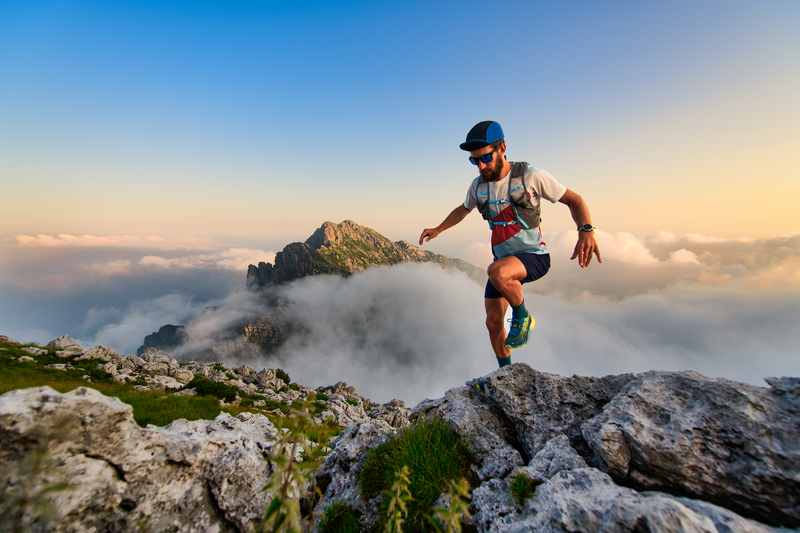
95% of researchers rate our articles as excellent or good
Learn more about the work of our research integrity team to safeguard the quality of each article we publish.
Find out more
REVIEW article
Front. Cell Dev. Biol. , 04 January 2022
Sec. Epigenomics and Epigenetics
Volume 9 - 2021 | https://doi.org/10.3389/fcell.2021.739511
Gestational diabetes mellitus (GDM) refers to different degrees of glucose tolerance abnormalities that occur during pregnancy or are discovered for the first time, which can have a serious impact on the mother and the offspring. The screening of GDM mainly relies on the oral glucose tolerance test (OGTT) at 24–28 weeks of gestation. The early diagnosis and intervention of GDM can greatly improve adverse pregnancy outcomes. However, molecular markers for early prediction and diagnosis of GDM are currently lacking. Therefore, looking for GDM-specific early diagnostic markers has important clinical significance for the prevention and treatment of GDM and the management of subsequent maternal health. Circular RNA (circRNA) is a new type of non-coding RNA. Recent studies have found that circRNAs were involved in the occurrence and development of malignant tumors, metabolic diseases, cardiovascular and cerebrovascular diseases, etc., and could be used as the molecular marker for early diagnosis. Our previous research showed that circRNAs are differentially expressed in serum of GDM pregnant women in the second and third trimester, placental tissues during cesarean delivery, and cord blood. However, the mechanism of circular RNA in GDM still remains unclear. This article focuses on related circRNAs involved in insulin resistance and β-cell dysfunction, speculating on the possible role of circRNAs in the pathophysiology of GDM under the current research context, and has the potential to serve as early molecular markers for the diagnosis of GDM.
Gestational diabetes mellitus (GDM) is defined as varying degrees of glucose intolerance identified for the first time during pregnancy (American Diabetes Association, 2019). In the past decade, with the increasing numbers of pregnant and parturient women who are obese or of advanced age, the incidence of GDM has increased worldwide (McIntyre et al., 2019). According to the latest data from the International Diabetes Federation in 2019 (International Diabetes Federation, 2019), the global prevalence of diabetes in pregnancy is 15.8%, affecting approximately 20.4 million women and newborns each year, with GDM accounting for 83.6% of these cases. In China, the incidence of GDM is 14.8% (Gao et al., 2019). GDM is associated with adverse pregnancy outcomes and long-term complications for the mother and fetus, and has certain effects on the long-term health management of postpartum mothers. Long-term exposure to a hyperglycemic uterine environment can lead to epigenetic changes for the fetus (Franzago et al., 2019), and increase the risk of metabolic and cardiovascular diseases in the offspring (Scholtens et al., 2019; Perak et al., 2021). Recent research demonstrates a 5–10 times higher risk of type 2 diabetes mellitus (T2DM) after childbirth in women with GDM than in women without GDM, with the highest risk at 3–6 years after childbirth (Song et al., 2018; Vounzoulaki et al., 2020).
The current diagnosis of GDM is based on the criteria defined by the International Association of Diabetes and Pregnancy Study Groups. The oral glucose tolerance test has some limitations, including repeated blood sampling, poor compliance of subjects and late diagnosis, because it is impossible to screen high-risk groups of GDM in the first trimester, However, those women with gestational diabetes have abnormal glucose metabolism before OGTT screening (Yefet et al., 2020). Early intervention for GDM, especially lifestyle modification, can effectively reduce fetal exposure to hyperglycemia in the uterus and greatly improve adverse pregnancy outcomes (Ehrlich et al., 2021). Therefore, looking for a specific early diagnostic marker to facilitate early, active, and appropriate intervention is of great significance for preventing and delaying the development and progression of long-term maternal diabetes in the early stage.
Non-coding RNAs, which do not encode proteins, include microRNAs (miRNAs), long non-coding RNAs (lncRNAs), circular RNAs (circRNAs), tRNA-derived small RNAs, and PIWI-interacting RNAs (Slack and Chinnaiyan, 2019). Non-coding RNAs such as miRNAs, lncRNAs, and circRNAs have been reported to serve as potential molecular markers for metabolic diseases, including GDM. Despite the large amount of research on miRNAs and lncRNAs in GDM, little is known about the role of circRNAs in this context. CircRNAs, which are formed by covalent joining of the 3′ and 5′ ends through exon or intron circularization, are more stable than linear RNA (Kristensen et al., 2019). In this review, we summarize recent research advances that have further elucidated the roles of circRNAs in the development and progression of GDM.
In a normal pregnancy, as the gestational age increases, the energy requirements of the fetus increase, and the mother’s basal metabolism increases (Catalano et al., 1999). Insulin resistance is an adaptive response, which promotes increased levels of glucose and free fatty acids in the maternal blood that can be transferred as sources of energy to the fetus (Plows et al., 2018). Although insulin response has increased, insulin secretion is still relatively insufficient. On the other hand, exogenous insulin sensitivity, defined as the ability of insulin to increase glucose uptake in skeletal muscle and adipose tissue, is reduced by about 50% in late pregnancy (Catalano et al., 1991). In addition, placenta, skeletal muscle, and adipose tissue produce factors related to insulin resistance, such as Human placental lactogen (hPL), human placental growth hormone (hPGH), leptin, TNF-α, etc., which may reduce the efficiency of glucose uptake or cause insulin signal transduction obstacle (Kirwan et al., 2002; Andersson-Hall et al., 2020). thus resulting in a progressive reduction in insulin sensitivity. Adenosine monophosphate (AMP)-activated kinase (AMPK), which is decreased in insulin resistant states (Hegarty et al., 2009). The expression of AMPK is decreased in the skeletal muscle and adipose tissue of pregnant women with GDM (Cantó et al., 2010). The elevated blood glucose levels stimulate a compensatory increase in the release of insulin, and there is an adaptive increase in pancreatic β-cell mass caused by increased cell proliferation and reduced apoptosis (Plows et al., 2018).
Potential metabolic abnormalities in gestational diabetes are insulin resistance and pancreatic β-cell dysfunction (Filardi et al., 2020). Insulin resistance in GDM is mediated by various factors, Compared with those in healthy pregnant women, glucose uptake is reduced by approximately half, and insulin sensitivity is decreased approximately 60% in patients with GDM (Catalano, 2014). Peripheral insulin resistance plays an important role in the pathophysiology of GDM. Some women have peripheral insulin resistance before pregnancy or early pregnancy, but it is asymptomatic and undetected. Moreover, in the second and third trimesters that GDM occurs may be due to increased insulin resistance (Catalano et al., 1999). Women with normal glucose tolerance in non-pregnant, insulin binds to the insulin receptor on the cell surface of surrounding tissues (such as skeletal muscle and fat) to activate the tyrosine kinase domain of the insulin receptor β subunit (IRβ), then activates the typical insulin-signaling cascade that induces the redistribution of glucose transporter type 4 (GLUT4) to the cell surface, allowing the cell’s glucose uptake (Tokarz et al., 2018). In pregnant women, the insulin-stimulated glucose transport rate dropped by about 40%, while in GDM women it reduced by 60% (Barbour et al., 2007). The total abundance of GLUT4 in the skeletal muscle did not change in normal pregnant women and GDM patients. Decreased glucose transport was associated with decreased Insulin receptor substrate-1(IRS-1) tyrosine phosphorylation (Barbour et al., 2007). However, in GDM subjects, the decrease of insulin receptor β subunit tyrosine phosphorylation level is related to the further decrease of glucose transport activity (Friedman et al., 1999). The increase in adipose tissue and impaired insulin signal transduction increase insulin resistance by approximately 56%, while progesterone can lower the expression of IRS-1 to inhibit glucose uptake by adipocytes (Nguyen-Ngo et al., 2019). In addition, endoplasmic reticulum stress or mitochondrial dysfunction in skeletal muscle, placental DNA methylation, and activation of adipose tissue inflammation are potential mechanisms of insulin resistance in GDM (Boyle et al., 2013; Lappas, 2014; Liong and Lappas, 2016; Hivert et al., 2020).
Pancreatic β-cells, which are the only insulin-secreting cells in the human body, are critical for blood glucose homeostasis. A reduction in the number and function of β-cells will lead to insufficient insulin release and increased blood glucose levels (Marchetti et al., 2017) Under normal pregnancy, the number of pancreatic islet β cells in women increases adaptively by 1.2∼2.4 fold (Assche et al., 1978; Butler et al., 2010). In the context, GDM is regarded as β-cell dysfunction and cannot compensate for insulin resistance during pregnancy (Xiang et al., 2013). The mechanism of β-cell dysfunction in GDM is not fully understood. Pre-pregnancy obesity, mother’s overnutrition, excessive weight gain during pregnancy, and genetic susceptibility may all cause β-cell dysfunction and hyperglycemia (Moyce and Dolinsky, 2018). The pregnancy hormones prolactin (PrL) and placental prolactin (PL) signal through the prolactin receptor (PRLR) and contribute to the beta cell adaptive response during pregnancy (Baeyens et al., 2016). In GDM, inactivation of prolactin receptors on the surface of islet β cells promotes apoptosis and inhibits proliferation of β cells (Banerjee et al., 2016). Moreover, down-regulation of prolactin action in non-beta cells during pregnancy negatively secondary affects beta cell gene expression and increases beta cell susceptibility to external injury (Shrivastava et al., 2021). Adiponectin plays an important role in the proliferation of beta cells during pregnancy by promoting prolactin expression in trophoblast cells (Qiao et al., 2021). Melatonin deficiency impairs pancreatic remodeling via apoptosis and proliferation of pancreatic islets and impairs beta cell glucose-induced insulin release during pregnancy and lactation (Gomes et al., 2021). In addition, under the mediation of obesity and systemic inflammation, a variety of cytokines (such as tumor necrosis factor α, interleukin-1β and interferon-γ are released, which induces β cells to undergo oxidative stress and endoplasmic reticulum Stress and mitochondrial damage interfere with cell dedifferentiation and cause cell apoptosis (Moyce and Dolinsky, 2018).
CircRNAs are created by a back-splicing process in which the downstream splice donor site is joined to the upstream splice acceptor site to form a covalently closed transcript. These molecules were first identified in a plant virus in 1976 (Sanger et al., 1976) and identified as an endogenous RNA splicing product in eukaryotes in 1979 (Hsu and Coca-Prados, 1979). CircRNAs were later found in the human hepatitis D virus in 1986 (Kos et al., 1986). In 2012, Salzman et al.(Salzman et al., 2012) analyzed the types and abundance of circRNAs in mammalian cells, and found that more than 10% of expressing genes could produce circRNAs. Based on the genomic source and sequence composition, circRNAs are classified into four types: exonic circRNAs derived from exons, intronic circRNAs derived from introns, exon-intron circRNAs containing both exons and introns, and intergenic circRNAs (Wang et al., 2016; Kristensen et al., 2019; Figure 1).
FIGURE 1. The type of circRNAs. CircRNAs are derived from precursor mRNA. (A) According to different biogenesis patterns from genomic regions, circRNAs can be divided into four categories: (1) Exonic circRNA (ecircRNA), ecircRNAs are predominantly generated from the back-splice exons (exon 1 or exon 2 and exon 3), where downstream 3 splice donors are covalently linked to upstream five splice acceptors in reverse order; (2) Circular intronic RNA (ciRNAs), intronic lariats that escape from debranching can lead to the formation of ciRNAs; (3) Exon-intron circRNAs(EIcircRNA), exons 3 and exon 4 are reversed spliced to form a loop containing an intron; (4) Intergenic circRNAs, the exons 3 from gene A and exons 2 from gene B were reversed spliced to form a loop. (B) A “cap” (m7G5'ppp5'Nm) was added to the 5 ‘end of pre-mRNA, and a “tail” Q18 (polyA) was added to the 3' end of precursor RNA to form linear RNA.
CircRNAs can escape degradation by exoribonucleases and the half-life of these molecules is 4.8 times longer than that of linear RNA (Jeck and Sharpless, 2014). CircRNAs are also more stable than linear RNAs (Stoll et al., 2020). CircRNAs are abundant in exosomes, and ubiquitous in extracellular fluids including saliva, blood, and urine (Hutchins et al., 2021; Wang et al., 2021a; Xiao et al., 2021). Their expression levels are 10-fold higher than those of linear mRNAs, and are highly conserved in the evolution of species (Jeck et al., 2013). CircRNA expression is time- and space-specific, showing distinct expression patterns in different tissues, cell types and developmental stages (Salzman et al., 2013).
In recent years, there have been significant advances in our understanding of the function of circRNAs. Accumulating evidence shows that circRNAs are the main factors that control gene expression by regulating transcription and translation. Rich in miRNA binding sites, circRNAs can act as a miRNA sponges, or competing endogenous RNAs (Hansen et al., 2013). By binding to RNA binding proteins, circRNAs can alter splicing patterns or mRNA stability, and inhibit the activity of proteins (Conn et al., 2015). CircRNAs can also serve as templates for the translation of proteins, and become a source of pseudogenes that regulate gene expression (Liu and Chen, 2021). However, the molecular mechanisms of the various biological functions of circRNAs remain to be fully elucidated.
With the recent advances in gene sequencing and bioinformatics technologies, circRNAs have been found to play an important role in various diseases such as cancer, metabolic diseases (e.g., T2DM and hyperlipidemia) as well as disorders of the cardiovascular and immune systems (Lei et al., 2020), (Garikipati et al., 2019). In malignant tumors including gastric cancer, liver cancer, breast cancer, lung cancer, bladder cancer, and cervical cancer, circRNAs are abnormally expressed, and regulate tumor proliferation and invasion, thus representing potential molecular markers for diagnosis and outcome prediction and also as therapeutic targets (Li et al., 2020) (Table 1). Furthermore, numerous studies have indicated that circRNAs are key molecules in metabolic homeostasis, regulate multiple genes related to glucose and lipid metabolism, and mediate the development and progression of metabolic disease. Thus, circRNAs are also implicated as promising targets for resolving metabolic disturbance as well as novel molecular diagnostic markers (Zeng et al., 2021).
CircRNA is involved in insulin resistance and pancreatic β-cell dysfunction (Zhang et al., 2021; Lin et al., 2021), provides certain insights for exploring the pathogenesis of GDM, and provides a possible future direction for the diagnosis of GDM and the prediction of complications.
The expression patterns of circRNAs in patients with GDM show distinct differences compared with healthy individuals (Yan et al., 2018). The predicted target genes of circRNAs are involved in glucose and lipid metabolism in GDM. AMPK signaling pathway is involved in the pathophysiology of GDM (Han et al., 2020), AMPK is critical for energy homeostasis, but its activity is decreased in the context of increasing hyperglycemic stress as the gestational age increases (Kumagai et al., 2018). In the skeletal muscle induced by the bacterial endotoxin lipopolysaccharide (LPS) and the pro-inflammatory cytokine IL-1β, activating AMPK could ameliorate inflammation and insulin resistance in GDM (Kumagai et al., 2018). CircACC1, a circRNA derived from the human acetyl-CoA carboxylase 1 gene, is involved in the formation of AMPK complex. Under metabolic stress, circACC1 activates the AMPK signaling pathway through JNK signaling, and promotes fatty acid β-oxidation and glycolysis in cells (Li et al., 2019). Unfortunately, the role of CircACC1 in GDM requires to be verified by further experiments. PAPPA is a metalloproteinase secreted from the human placenta that regulates the bioavailability of insulin-like growth factor (IGF) through the hydrolysis of IGF binding proteins (IGFBPs)-2, 3, and 5 (Boldt and Conover, 2007). In vitro, recombinant PAPPA has been shown to stimulate human adipose tissue to expand in an IGFBP-5 and IGF-1-dependent manner (Gyrup and Oxvig, 2007). Rojas–Rodriguez et al. (Rojas-Rodriguez et al., 2020) reported that insulin resistance is closely associated with endothelial dysfunction (Aziz et al., 2018), and hsa_circ_0010283 is involved in vascular smooth muscle cell dysfunction by regulating the miR-133a-3p/pregnancy-associated plasma protein A (PAPPA) pathway (Feng et al., 2020). PAPPA-deficient mice develop insulin resistance in pregnancy. Moreover, a study of 6,361 pregnant women demonstrated that the concentration of PAPPA in the circulation is negatively correlated with blood glucose levels and the incidence of GDM (Rojas-Rodriguez et al., 2020). As a miR-149-5p sponge, hsa_circ_0124644 regulates the expression of PAPPA indirectly, resulting in endothelial cell injury (Wang et al., 2020b). The circRNA SCAR is downregulated in liver fibroblasts, and is negatively correlated with insulin resistance (Zhao et al., 2020). CircHIPK3(one of the most abundant circRNAs present in β-cells (Stoll et al., 2018)) regulates hyperglycemia and insulin resistance by increasing the mRNA levels of two key enzymes in glucose metabolism. However, it is uncertain whether these circRNAs have the same regulatory mechanism in peripheral insulin resistance. The circRNA SAMD4A regulates fat formation via the miR-138-5p/EZH2 axis in obesity (Liu et al., 2020), which is a high-risk factor for GDM. Nevertheless, the role of these circRNAs in insulin resistance in GDM merits further investigation.
CircRNAs are abundant in human pancreatic islets, and highly conserved between species. As novel regulators for β-cell activity, circRNAs are involved in β-cell dysfunction in hyperglycemia. CircRNA-CDR1as was the first circRNA studied in pancreatic islet cells, and is predominantly located in the cytoplasm (Hansen et al., 2011). The researchers found that CircRNA-CDR1 acts as a miR-7 sponge, regulating insulin secretion, pancreatic β cell proliferation and insulin signal transduction (Xu et al., 2015). Since then, researchers have continued to explore the role of circRNA in beta cell dysfunction. Using microarrays, Stoll L et al.(Stoll et al., 2018) found circHIPK3 regulates the apoptosis, proliferation, and insulin secretion of β-cells through sponging miR-124-3p and miR-338-3p and regulating the expression of key genes such as SLC2A2, AKT1, and MTPN (Brozzi and Regazzi, 2021). As shown above, diminished levels of circHIPK3 and CircRNA-CDR1as cause β cells to be unable to release enough insulin to meet the organism’s needs. CircRNA is involved in β cell dysfunction mediated by lipotoxic and pro-inflammatory cytokines (Zhang et al., 2021; Wang et al., 2021b). Wu et al.(Wu et al., 2020) revealed that circTulp4 regulated β-cell proliferation to lipotoxicity via the miR-7222-3p/soat1/cyclinD1 signaling pathway. In addition, Stoll et al. (Stoll et al., 2020) discovered an intron circular RNA in mice, rats, and human pancreatic islets—ci-Ins2/ci-INS, which was specifically expressed in pancreatic β cells. It regulated insulin-releasing genes by interacting with RBP TDP-43. Meanwhile, ci-INS was found significantly lower in the islets of type 2 diabetes and to be inversely correlated to HbA1c levels. Sun et al. (Sun et al., 2021) found hsa_circ_0,054,633 mediates apoptosis and insulin secretion via miR-409-3p/caspase-8 axis in human pancreatic β cells. Under the premise that the pathogenesis of GDM is not clear, the reduction in β-cell mass adaptability in patients with GDM may be related to circRNA-mediated regulation of β-cell function and insulin secretion disorder.
CircRNA plays an important role in early diagnosis of GDM, assessment of placental function, and prediction of complications. In order to explore the value of circRNA in the diagnosis of GDM, our team found that circRNAs such as hsa_circ_0054633, hsa_circ_103410, hsa_circ_063981, and hsa_circ_102682 were differentially expressed in the placenta, cord blood and maternal plasma during the second and third trimester in women with GDM. At the same time, hsa_circ_0054633 was highly correlated with glycosylated hemoglobin (Wu et al., 2019) and hsa_circ_102682 was closely related to lipid metabolism in GDM(Wu et al., 2021). Nonetheless, if circular RNA is used as a marker for early diagnosis of GDM, whether it changes before OGTT diagnosis in the second trimester. And it is also unclear whether expression in placenta is related to expression in early pregnancy, more experiments are required to explore this. Then, Wang et al. (Wang et al., 2020a) discovered hsa_circ_0005243 was significantly downregulated in the placenta and plasma in the third trimester of patients with GDM, and its downregulation induced trophoblast dysfunction and inflammation in a hyperglycemic environment via the β-catenin and NF-κB pathways. Similarly, the dynamic changes of the circular RNA during pregnancy and its value as a molecular marker for early diagnosis or predicting placental functionstill also need to be verified. Increasingly, more and more circRNAs are identified to be expressed differently in the serum of GDM women during the third trimester of pregnancy and the placental tissues during delivery. Meanwhile, the mechanism of action of the corresponding circRNA is gradually being explored. Wang et al. (Wang et al., 2019) identified 8,321 circRNAs in GDM and normal placenta tissues, and revealed significantly reduced expression of hsa_circ_5824, hsa_circ_3636, and hsa_circ_0395 in the GDM group. Moreover, the reduced expression of hsa_circRNA_0395 in the placenta of patients with GDM overlapped with the expression of the gene encoding PAPPA2, which can be used to predict the development of fetal macrosomia in GDM. Yang et al.(Yang et al., 2020b) found that hsa_circRNA_102893 was downregulated in the plasma of women with GDM in the 15–24 weeks and bound to miR-33a-5p, miR-2115-3p, miR-197-3p, miR-5187-5p, and miR-198 to regulate the expression of 24 target genes. In addition, it was predicted that hsa_circRNA_102893 contained six flanking region binding proteins, including EIF4A3 protein (involved in promoter translation) and AGO protein (involved in the processing and maturation of small RNAs). Tang et al.(Tang et al., 2020)found various differentially expressed circRNAs in the placental tissue of patients with GDM by transcriptome sequencing. GO and KEGG pathway analyses indicated that the main function of the circRNAs was to activate phospholipase C and regulate insulin secretion. Furthermore, the circRNAs had one or more target sites for specific binding of miRNAs that facilitated regulation of the development and progression of GDM via the sponge mechanism. Although the abnormal expression of these circRNAs were found, at which stage of pregnancy circRNA changes, whether even occurs before pregnancy, and how they will change after delivery, these string of problems are still unknown at present, and more experiments are needed to clarify in vivo and in vitro.
Although circRNAs are a focus of research in many fields, their exact roles and regulatory mechanisms in GDM are preliminary remain to be fully elucidated. In-depth and systematic investigations are required to address the specific location of GDM-related circRNAs in cells and the signaling pathways by which they interact with proteins and miRNAs. Based on their characteristic stability and abundance as well as their biological functions, circRNAs show promise as molecular markers for early diagnosis of GDM and prediction of its complications, which will facilitate early intervention for GDM to reduce adverse perinatal outcomes. CircRNAs are also implicated as molecular markers for predicting the long-term risk of GDM and identifying patients at high risk to deliver intervention and guidance to provide a better long-term quality of life.
Y-PZ, S-ZY, Y-XL, J-LC contributed to wrote the review. Y-SZ contributed to final approval of the manuscript. All authors read and approved the final manuscript.
This work was supported by Science and Technology Project of Ningbo City (No. 2019C50091), Medical and health science and Technology project of Zhejiang Province (2021KY1038) and Medical and health science and Technology project of Zhejiang Province (2022KY297).
The authors declare that the research was conducted in the absence of any commercial or financial relationships that could be construed as a potential conflict of interest.
All claims expressed in this article are solely those of the authors and do not necessarily represent those of their affiliated organizations, or those of the publisher, the editors, and the reviewers. Any product that may be evaluated in this article, or claim that may be made by its manufacturer, is not guaranteed or endorsed by the publisher.
American Diabetes Association (2019). Classification and Diagnosis of Diabetes: Standards of Medical Care in Diabetes-2019. Diabetes Care 42 (Suppl. 1), S13–S28. doi:10.2337/dc19-S002
Andersson-Hall, U., Svedin, P., Svensson, H., Lönn, M., Mallard, C., and Holmäng, A. (2020). Longitudinal Changes in Adipokines and Free Leptin index during and after Pregnancy in Women with Obesity. Int. J. Obes. 44 (3), 675–683. doi:10.1038/s41366-019-0452-7
Assche, F. A., Aerts, L., and Prins, F. D. (1978). A Morphological Study of the Endocrine Pancreas in Human Pregnancy. BJOG:An Int. J. O&G 85 (11), 818–820. doi:10.1111/j.1471-0528.1978.tb15835.x
Aziz, A., Haywood, N. J., Cordell, P. A., Smith, J., Yuldasheva, N. Y., Sengupta, A., et al. (2018). Insulinlike Growth Factor-Binding Protein-1 Improves Vascular Endothelial Repair in Male Mice in the Setting of Insulin Resistance. Endocrinology 159 (2), 696–709. doi:10.1210/en.2017-00572
Baeyens, L., Hindi, S., Sorenson, R. L., and German, M. S. (2016). β-Cell Adaptation in Pregnancy. Diabetes Obes. Metab. 18 (Suppl. 1), 63–70. doi:10.1111/dom.12716
Banerjee, R. R., Cyphert, H. A., Walker, E. M., Chakravarthy, H., Peiris, H., Gu, X., et al. (2016). Gestational Diabetes Mellitus from Inactivation of Prolactin Receptor and MafB in Islet β-Cells. Diabetes 65 (8), 2331–2341. doi:10.2337/db15-1527
Barbour, L. A., McCurdy, C. E., Hernandez, T. L., Kirwan, J. P., Catalano, P. M., and Friedman, J. E. (2007). Cellular Mechanisms for Insulin Resistance in normal Pregnancy and Gestational Diabetes. Diabetes Care 30 (Suppl. 2), S112–S119. doi:10.2337/dc07-s202
Boldt, H. B., and Conover, C. A. (2007). Pregnancy-associated Plasma Protein-A (PAPP-A): a Local Regulator of IGF Bioavailability through Cleavage of IGFBPs. Growth Horm. IGF Res. 17 (1), 10–18. doi:10.1016/j.ghir.2006.11.003
Boyle, K. E., Newsom, S. A., Janssen, R. C., Lappas, M., and Friedman, J. E. (2013). Skeletal Muscle MnSOD, Mitochondrial Complex II, and SIRT3 Enzyme Activities Are Decreased in Maternal Obesity during Human Pregnancy and Gestational Diabetes Mellitus. J. Clin. Endocrinol. Metab. 98 (10), E1601–E1609. doi:10.1210/jc.2013-1943
Brozzi, F., and Regazzi, R. (2021). Circular RNAs as Novel Regulators of β-Cell Functions under Physiological and Pathological Conditions. Int. J. Mol. Sci 22 (4), 1503. doi:10.3390/ijms22041503
Butler, A. E., Cao-Minh, L., Galasso, R., Rizza, R. A., Corradin, A., Cobelli, C., et al. (2010). Adaptive Changes in Pancreatic Beta Cell Fractional Area and Beta Cell Turnover in Human Pregnancy. Diabetologia 53 (10), 2167–2176. doi:10.1007/s00125-010-1809-6
Cantó, C., Jiang, L. Q., Deshmukh, A. S., Mataki, C., Coste, A., Lagouge, M., et al. (2010). Interdependence of AMPK and SIRT1 for Metabolic Adaptation to Fasting and Exercise in Skeletal Muscle. Cel Metab. 11 (3), 213–219. doi:10.1016/j.cmet.2010.02.006
Catalano, P. M., Tyzbir, E. D., Roman, N. M., Amini, S. B., and Sims, E. A. H. (1991). Longitudinal Changes in Insulin Release and Insulin Resistance in Nonobese Pregnant Women. Am. J. Obstet. Gynecol. 165 (6), 1667–1672. doi:10.1016/0002-9378(91)90012-G
Catalano, P. M., Huston, L., Amini, S. B., and Kalhan, S. C. (1999). Longitudinal Changes in Glucose Metabolism during Pregnancy in Obese Women with normal Glucose Tolerance and Gestational Diabetes Mellitus. Am. J. Obstet. Gynecol. 180 (4), 903–916. doi:10.1016/S0002-9378(99)70662-9
Catalano, P. M. (2014). Trying to Understand Gestational Diabetes. Diabet. Med. 31 (3), 273–281. doi:10.1111/dme.12381
Conn, S. J., Pillman, K. A., Toubia, J., Conn, V. M., Salmanidis, M., Phillips, C. A., et al. (2015). The RNA Binding Protein Quaking Regulates Formation of circRNAs. Cell 160 (6), 1125–1134. doi:10.1016/j.cell.2015.02.014
Ehrlich, S. F., Ferrara, A., Hedderson, M. M., Feng, J., and Neugebauer, R. (2021). Exercise During the First Trimester of Pregnancy and the Risks of Abnormal Screening and Gestational Diabetes Mellitus. Dia Care 44 (2), 425–432. doi:10.2337/dc20-1475
Feng, Z., Zhu, Y., Zhang, J., Yang, W., Chen, Z., and Li, B. (2020). Hsa-circ_0010283 Regulates Oxidized Low-Density Lipoprotein-Induced Proliferation and Migration of Vascular Smooth Muscle Cells by Targeting the miR-133a-3p/Pregnancy-Associated Plasma Protein A Axis. Circ. J. 84 (12), 2259–2269. doi:10.1253/circj.CJ-20-0345
Filardi, T., Catanzaro, G., Mardente, S., Zicari, A., Santangelo, C., Lenzi, A., et al. (2020). Non-Coding RNA: Role in Gestational Diabetes Pathophysiology and Complications. Int. J. Mol. Sci 21 (11), 4020. doi:10.3390/ijms21114020
Franzago, M., Fraticelli, F., Stuppia, L., and Vitacolonna, E. (2019). Nutrigenetics, Epigenetics and Gestational Diabetes: Consequences in Mother and Child. Epigenetics 14 (3), 215–235. doi:10.1080/15592294.2019.1582277
Friedman, J. E., Ishizuka, T., Shao, J., Huston, L., Highman, T., and Catalano, P. (1999). Impaired Glucose Transport and Insulin Receptor Tyrosine Phosphorylation in Skeletal Muscle from Obese Women with Gestational Diabetes. Diabetes 48 (9), 1807–1814. doi:10.2337/diabetes.48.9.1807
Gao, C., Sun, X., Lu, L., Liu, F., and Yuan, J. (2019). Prevalence of Gestational Diabetes Mellitus in mainland China: A Systematic Review and Meta-Analysis. J. Diabetes Investig. 10 (1), 154–162. doi:10.1111/jdi.12854
Garikipati, V. N. S., Verma, S. K., Cheng, Z., Liang, D., Truongcao, M. M., Cimini, M., et al. (2019). Circular RNA CircFndc3b Modulates Cardiac Repair after Myocardial Infarction via FUS/VEGF-A axis. Nat. Commun. 10 (1), 4317. doi:10.1038/s41467-019-11777-7
Gomes, P. R. L., Vilas‐Boas, E. A., Leite, E. d. A., Munhoz, A. C., Lucena, C. F., Amaral, F. G. D., et al. (2021). Melatonin Regulates Maternal Pancreatic Remodeling and B‐Cell Function during Pregnancy and Lactation. J. Pineal Res. 71 (1), e12717. doi:10.1111/jpi.12717
Gyrup, C., and Oxvig, C. (2007). Quantitative Analysis of Insulin-Like Growth Factor-Modulated Proteolysis of Insulin-Like Growth Factor Binding Protein-4 and -5 by Pregnancy-Associated Plasma Protein-A. Biochemistry 46 (7), 1972–1980. doi:10.1021/bi062229i
Han, D., Jiang, L., Gu, X., Huang, S., Pang, J., Wu, Y., et al. (2020). SIRT3 Deficiency Is Resistant to Autophagy‐Dependent Ferroptosis by Inhibiting the AMPK/mTOR Pathway and Promoting GPX4 Levels. J. Cel Physiol 235 (11), 8839–8851. doi:10.1002/jcp.29727
Hansen, T. B., Wiklund, E. D., Bramsen, J. B., Villadsen, S. B., Statham, A. L., Clark, S. J., et al. (2011). miRNA-Dependent Gene Silencing Involving Ago2-Mediated Cleavage of a Circular Antisense RNA. EMBO J. 30 (21), 4414–4422. doi:10.1038/emboj.2011.359
Hansen, T. B., Jensen, T. I., Clausen, B. H., Bramsen, J. B., Finsen, B., Damgaard, C. K., et al. (2013). Natural RNA Circles Function as Efficient microRNA Sponges. Nature 495 (7441), 384–388. doi:10.1038/nature11993
Hegarty, B. D., Turner, N., Cooney, G. J., and Kraegen, E. W. (2009). Insulin Resistance and Fuel Homeostasis: the Role of AMP-Activated Protein Kinase. Acta Physiol. 196 (1), 129–145. doi:10.1111/j.1748-1716.2009.01968.x
Hivert, M.-F., Cardenas, A., Allard, C., Doyon, M., Powe, C. E., Catalano, P. M., et al. (2020). Interplay of Placental DNA Methylation and Maternal Insulin Sensitivity in Pregnancy. Diabetes 69 (3), 484–492. doi:10.2337/db19-0798
Hsu, M.-T., and Coca-Prados, M. (1979). Electron Microscopic Evidence for the Circular Form of RNA in the Cytoplasm of Eukaryotic Cells. Nature 280 (5720), 339–340. doi:10.1038/280339a0
Hutchins, E., Reiman, R., Winarta, J., Beecroft, T., Richholt, R., De Both, M., et al. (2021). Extracellular Circular RNA Profiles in Plasma and Urine of Healthy, Male College Athletes. Sci. Data 8 (1), 276. doi:10.1038/s41597-021-01056-w
International Diabetes Federation (2019). IDF Diabetes Atlas. International Diabetes Federation. Available at: https://www.idf.org (Accessed May 10, 2021).
Jeck, W. R., and Sharpless, N. E. (2014). Detecting and Characterizing Circular RNAs. Nat. Biotechnol. 32 (5), 453–461. doi:10.1038/nbt.2890
Jeck, W. R., Sorrentino, J. A., Wang, K., Slevin, M. K., Burd, C. E., Liu, J., et al. (2013). Circular RNAs Are Abundant, Conserved, and Associated with ALU Repeats. RNA 19 (2), 141–157. doi:10.1261/rna.035667.112
Kirwan, J. P., Hauguel-De Mouzon, S., Lepercq, J., Challier, J.-C., Huston-Presley, L., Friedman, J. E., et al. (2002). TNF- Is a Predictor of Insulin Resistance in Human Pregnancy. Diabetes 51 (7), 2207–2213. doi:10.2337/diabetes.51.7.2207
Kos, A., Dijkema, R., Arnberg, A. C., van der Meide, P. H., and Schellekens, H. (1986). The Hepatitis delta (δ) Virus Possesses a Circular RNA. Nature 323 (6088), 558–560. doi:10.1038/323558a0
Kristensen, L. S., Andersen, M. S., Stagsted, L. V. W., Ebbesen, K. K., Hansen, T. B., and Kjems, J. (2019). The Biogenesis, Biology and Characterization of Circular RNAs. Nat. Rev. Genet. 20 (11), 675–691. doi:10.1038/s41576-019-0158-7
Kumagai, A., Itakura, A., Koya, D., and Kanasaki, K. (2018). AMP-Activated Protein (AMPK) in Pathophysiology of Pregnancy Complications. Ijms 19 (10), 3076. doi:10.3390/ijms19103076
Lappas, M. (2014). Activation of Inflammasomes in Adipose Tissue of Women with Gestational Diabetes. Mol. Cell Endocrinol. 382 (1), 74–83. doi:10.1016/j.mce.2013.09.011
Lei, M., Zheng, G., Ning, Q., Zheng, J., and Dong, D. (2020). Translation and Functional Roles of Circular RNAs in Human Cancer. Mol. Cancer 19 (1), 30. doi:10.1186/s12943-020-1135-7
Li, X., Zhao, Z., Jian, D., Li, W., Tang, H., and Li, M. (2017). Hsa-circRNA11783-2 in Peripheral Blood Is Correlated with Coronary Artery Disease and Type 2 Diabetes Mellitus. Diabetes Vasc. Dis. Res. 14 (6), 510–515. doi:10.1177/1479164117722714
Li, Q., Wang, Y., Wu, S., Zhou, Z., Ding, X., Shi, R., et al. (2019). CircACC1 Regulates Assembly and Activation of AMPK Complex under Metabolic Stress. Cel Metab. 30 (1), 157–173. doi:10.1016/j.cmet.2019.05.009
Li, J., Sun, D., Pu, W., Wang, J., and Peng, Y. (2020). Circular RNAs in Cancer: Biogenesis, Function, and Clinical Significance. Trends Cancer 6 (4), 319–336. doi:10.1016/j.trecan.2020.01.012
Lin, X., Du, Y., Lu, W., Gui, W., Sun, S., Zhu, Y., et al. (2021). CircRNF111 Protects Against Insulin Resistance and Lipid Deposition via Regulating miR-143-3p/IGF2R Axis in Metabolic Syndrome. Front. Cel Dev. Biol. 9, 663148. doi:10.3389/fcell.2021.663148
Liong, S., and Lappas, M. (2016). Endoplasmic Reticulum Stress Regulates Inflammation and Insulin Resistance in Skeletal Muscle from Pregnant Women. Mol. Cell Endocrinol. 425, 11–25. doi:10.1016/j.mce.2016.02.016
Liu, C.-X., and Chen, L.-L. (2021). Expanded Regulation of Circular RNA Translation. Mol. Cel 81 (20), 4111–4113. doi:10.1016/j.molcel.2021.09.023
Liu, Y., Liu, H., Li, Y., Mao, R., Yang, H., Zhang, Y., et al. (2020). Circular RNA SAMD4A Controls Adipogenesis in Obesity through the miR-138-5p/EZH2 Axis. Theranostics 10 (10), 4705–4719. doi:10.7150/thno.42417
Lu, Y.-K., Chu, X., Wang, S., Sun, Y., Zhang, J., Dong, J., et al. (2021). Identification of Circulating Hsa_circ_0063425 and Hsa_circ_0056891 as Novel Biomarkers for Detection of Type 2 Diabetes. J. Clin. Endocrinol. Metab. 106 (7), e2688–e2699. doi:10.1210/clinem/dgab101
Marchetti, P., Bugliani, M., De Tata, V., Suleiman, M., and Marselli, L. (2017). Pancreatic Beta Cell Identity in Humans and the Role of Type 2 Diabetes. Front. Cel Dev. Biol. 5, 55. doi:10.3389/fcell.2017.00055
McIntyre, H. D., Catalano, P., Zhang, C., Desoye, G., Mathiesen, E. R., and Damm, P. (2019). Gestational Diabetes Mellitus. Nat. Rev. Dis. Primers 5 (1), 4711. doi:10.1038/s41572-019-0098-8
Moyce, B., and Dolinsky, V. (2018). Maternal β-Cell Adaptations in Pregnancy and Placental Signalling: Implications for Gestational Diabetes. Int. J. Mol. Sci. 19 (11), 3467. doi:10.3390/ijms19113467
Nguyen-Ngo, C., Jayabalan, N., Salomon, C., and Lappas, M. (2019). Molecular Pathways Disrupted by Gestational Diabetes Mellitus. J. Mol. Endocrinol. 63 (3), R51–R72. doi:10.1530/JME-18-0274
Perak, A. M., Lancki, N., Kuang, A., Labarthe, D. R., Allen, N. B., Shah, S. H., et al. (2021). Associations of Maternal Cardiovascular Health in Pregnancy with Offspring Cardiovascular Health in Early Adolescence. JAMA 325 (7), 658–668. doi:10.1001/jama.2021.0247
Plows, J., Stanley, J., Baker, P., Reynolds, C., and Vickers, M. (2018). The Pathophysiology of Gestational Diabetes Mellitus. Int. J. Mol. Sci. 19 (11), 3342. doi:10.3390/ijms19113342
Qiao, L., Saget, S., Lu, C., Hay, W. W., Karsenty, G., and Shao, J. (2021). Adiponectin Promotes Maternal β-Cell Expansion through Placental Lactogen Expression. Diabetes 70 (1), 132–142. doi:10.2337/db20-0471
Rezaeinejad, F., Mirzaei, A., Khalvati, B., Sabz, G., and Alipoor, B. (2021). Circulating Expression Levels of CircHIPK3 and CDR1as Circular-RNAs in Type 2 Diabetes Patients. Mol. Biol. Rep. Online ahead of print. doi:10.1007/s11033-021-06850-8
Rojas-Rodriguez, R., Ziegler, R., DeSouza, T., Majid, S., Madore, A. S., Amir, N., et al. (2020). PAPPA-mediated Adipose Tissue Remodeling Mitigates Insulin Resistance and Protects against Gestational Diabetes in Mice and Humans. Sci. Transl. Med. 12 (571), eaay4145. doi:10.1126/scitranslmed.aay4145
Salzman, J., Gawad, C., Wang, P. L., Lacayo, N., and Brown, P. O. (2012). Circular RNAs Are the Predominant Transcript Isoform from Hundreds of Human Genes in Diverse Cell Types. PloS one 7 (2), e30733. Available at: https://pubmed.ncbi.nlm.nih.gov/22319583/(accessed Mar. 11, 2021). doi:10.1371/journal.pone.0030733
Salzman, J., Chen, R. E., Olsen, M. N., Wang, P. L., and Brown, P. O. (2013). Cell-Type Specific Features of Circular RNA Expression. Plos Genet. 9 (9), e1003777. doi:10.1371/journal.pgen.1003777
Sanger, H. L., Klotz, G., Riesner, D., Gross, H. J., and Kleinschmidt, A. K. (1976). Viroids Are Single-Stranded Covalently Closed Circular RNA Molecules Existing as Highly Base-Paired Rod-Like Structures. Proc. Natl. Acad. Sci. 73 (11), 3852–3856. doi:10.1073/pnas.73.11.3852
Scholtens, W. L. D. M., Kuang, A., Linder, B., Lawrence, J. M., Lebenthal, Y., McCance, D., et al. (2019). Hyperglycemia and Adverse Pregnancy Outcome Follow-Up Study (HAPO FUS): Maternal Gestational Diabetes Mellitus and Childhood Glucose Metabolism. Dia Care 42 (3), 372–380. doi:10.2337/dc18-1646
Shrivastava, V., Lee, M., Lee, D., Pretorius, M., Radford, B., Makkar, G., et al. (2021). Beta Cell Adaptation to Pregnancy Requires Prolactin Action on Both Beta and Non-beta Cells. Sci. Rep. 11 (1), 10372. doi:10.1038/s41598-021-89745-9
Slack, F. J., and Chinnaiyan, A. M. (2019). The Role of Non-Coding RNAs in Oncology. Cell 179 (5), 1033–1055. doi:10.1016/j.cell.2019.10.017
Song, C., Lyu, Y., Li, C., Liu, P., Li, J., Ma, R. C., et al. (2018). Long-Term Risk of Diabetes in Women at Varying Durations after Gestational Diabetes: a Systematic Review and Meta-Analysis with More Than 2 Million Women. Obes. Rev. 19 (3), 421–429. doi:10.1111/obr.12645
Stoll, L., Sobel, J., Rodriguez-Trejo, A., Guay, C., Lee, K., Venø, M. T., et al. (2018). Circular RNAs as Novel Regulators of β-Cell Functions in Normal and Disease Conditions. Mol. Metab. 9, 69–83. doi:10.1016/j.molmet.2018.01.010
Stoll, L., Rodríguez-Trejo, A., Guay, C., Brozzi, F., Bayazit, M. B., Gattesco, S., et al. (2020). A Circular RNA Generated from an Intron of the Insulin Gene Controls Insulin Secretion. Nat. Commun. 11. doi:10.1038/s41467-020-19381-w
Sun, R., Xue, W., and Zhao, J. (2021). Hsa_circ_0054633 Mediates Apoptosis and Insulin Secretion in Human Pancreatic β Cells through miR-409-3p/caspase-8 axis. Diabetes Res. Clin. Pract. 176, 108837. doi:10.1016/j.diabres.2021.108837
Tang, L., Li, P., and Li, L. (2020). Whole Transcriptome Expression Profiles in Placenta Samples from Women with Gestational Diabetes Mellitus. J. Diabetes Invest. 11 (5), 1307–1317. Available at: https://pubmed.ncbi.nlm.nih.gov/32174045/(accessed Jul. 30, 2020). doi:10.1111/jdi.13250
Tokarz, V. L., MacDonald, P. E., and Klip, A. (2018). The Cell Biology of Systemic Insulin Function. J. Cel Biol 217 (7), 2273–2289. doi:10.1083/jcb.201802095
Vounzoulaki, E., Khunti, K., Abner, S. C., Tan, B. K., Davies, M. J., and Gillies, C. L. (2020). Progression to Type 2 Diabetes in Women with a Known History of Gestational Diabetes: Systematic Review and Meta-Analysis. BMJ 369, m1361. doi:10.1136/bmj.m1361
Wang, F., Nazarali, A. J., and Ji, S. (2016). Circular RNAs as Potential Biomarkers for Cancer Diagnosis and Therapy. Am. J. Cancer Res. 6 (6), 1167–1176.
Wang, H., She, G., Zhou, W., Liu, K., Miao, J., and Yu, B. (2019). Expression Profile of Circular RNAs in Placentas of Women with Gestational Diabetes Mellitus. Endocr. J. 66 (5), 431–441. doi:10.1507/endocrj.EJ18-0291
Wang, H., Zhou, W., She, G., Yu, B., and Sun, L. (2020). Downregulation of Hsa_circ_0005243 Induces Trophoblast Cell Dysfunction and Inflammation via the β-catenin and NF-Κb Pathways. Reprod. Biol. Endocrinol. 18 (1), 51. doi:10.1186/s12958-020-00612-0
Wang, G., Li, Y., Liu, Z., Ma, X., Li, M., Lu, Q., et al. (2020). Circular RNA Circ_0124644 Exacerbates the Ox-LDL-Induced Endothelial Injury in Human Vascular Endothelial Cells through Regulating PAPP-A by Acting as a Sponge of miR-149-5p. Mol. Cel Biochem 471 (1–2), 51–61. doi:10.1007/s11010-020-03764-0
Wang, M., Zhang, L., Ren, W., Li, S., Zhi, K., Zheng, J., et al. (2021). Diagnostic Value of CircRNAs as Potential Biomarkers in Oral Squamous Cell Carcinoma: A Meta-Analysis. Front. Oncol. 11, 693284. doi:10.3389/fonc.2021.693284
Wang, Z., Deng, C., and Zheng, Y. (2021). Involvement of circRNAs in Proinflammatory Cytokines-Mediated β-Cell Dysfunction. Mediators Inflamm. 2021, 5566453. doi:10.1155/2021/5566453
Wu, H., Wu, S., Zhu, Y., Ye, M., Shen, J., Liu, Y., et al. (2019). Hsa_circRNA_0054633 Is Highly Expressed in Gestational Diabetes Mellitus and Closely Related to Glycosylation index. Clin. Epigenet 11 (1), 22. doi:10.1186/s13148-019-0610-8
Wu, L., Xiong, L., Li, J., Peng, Z., Zhang, L., Shi, P., et al. (2020). Circ-Tulp4 Promotes β-Cell Adaptation to Lipotoxicity by Regulating Soat1 Expression. J. Mol. Endocrinol. 65 (4), 149–161. doi:10.1530/JME-20-0079
Wu, H., Zheng, X., Liu, Y., Shen, J., Ye, M., and Zhang, Y. (2021). Hsa_circRNA_102682 Is Closely Related to Lipid Metabolism in Gestational Diabetes Mellitus. Gynecol. Endocrinol. Online ahead of print, 1–5. doi:10.1080/09513590.2021.1991911
Xiang, A. H., Takayanagi, M., Black, M. H., Trigo, E., Lawrence, J. M., Watanabe, R. M., et al. (2013). Longitudinal Changes in Insulin Sensitivity and Beta Cell Function between Women with and without a History of Gestational Diabetes Mellitus. Diabetologia 56 (12), 2753–2760. doi:10.1007/s00125-013-3048-0
Xiao, Q., Yin, R., Wang, Y., Yang, S., Ma, A., Pan, X., et al. (2021). Comprehensive Analysis of Peripheral Exosomal circRNAs in Large Artery Atherosclerotic Stroke. Front. Cel Dev. Biol. 9, 685741. doi:10.3389/fcell.2021.685741
Xu, H., Guo, S., Li, W., and Yu, P. (2015). The Circular RNA Cdr1as, via miR-7 and its Targets, Regulates Insulin Transcription and Secretion in Islet Cells. Sci. Rep. 5, 12453. doi:10.1038/srep12453
Yan, L., Feng, J., Cheng, F., Cui, X., Gao, L., Chen, Y., et al. (2018). Circular RNA Expression Profiles in Placental Villi from Women with Gestational Diabetes Mellitus. Biochem. Biophysical Res. Commun. 498 (4), 743–750. doi:10.1016/j.bbrc.2018.03.051
Yang, L., Han, X., Zhang, C., Sun, C., Huang, S., Xiao, W., et al. (2020). Hsa_circ_0060450 Negatively Regulates Type I Interferon-Induced Inflammation by Serving as miR-199a-5p Sponge in Type 1 Diabetes Mellitus. Front. Immunol. 11, 576903. doi:10.3389/fimmu.2020.576903
Yang, H., Ye, W., Chen, R., Zeng, F., Long, Y., Zhang, X., et al. (2020). Circulating Expression of Hsa_circRNA_102893 Contributes to Early Gestational Diabetes Mellitus Detection. Sci. Rep. 10 (1), 19046. doi:10.1038/s41598-020-76013-5
Yefet, E., Jeda, E., Tzur, A., and Nachum, Z. (2020). Markers for Undiagnosed Type 2 Diabetes Mellitus during Pregnancy-A Population‐based Retrospective Cohort Study. J. Diabetes 12 (3), 205–214. doi:10.1111/1753-0407.12985
Yingying, Z., Yongji, Y., Qiuting, C., Rifang, L., and Zhuanping, Z. (2021). hsa_circ_0071106 Can Be Used as a Diagnostic Marker for Type 2 Diabetes. Int. J. Med. Sci. 18 (11), 2312–2320. doi:10.7150/ijms.52575
Zeng, Y., Zheng, Z., Liu, F., and Yi, G. (2021). Circular RNAs in Metabolism and Metabolic Disorders. Obes. Rev. 22, e13220. doi:10.1111/obr.13220
Zhang, C., Han, X., Yang, L., Fu, J., Sun, C., Huang, S., et al. (2020). Circular RNA circPPM1F Modulates M1 Macrophage Activation and Pancreatic Islet Inflammation in Type 1 Diabetes Mellitus. Theranostics 10 (24), 10908–10924. doi:10.7150/thno.48264
Zhang, Y., Zhao, Q., Su, S., Dan, L., Li, X., Wang, Y., et al. (2021). Comparative Analysis of circRNA Expression Profile and circRNA-miRNA-mRNA Regulatory Network between Palmitic and Stearic Acid-Induced Lipotoxicity to Pancreatic β Cells. Bioengineered 12 (1), 9031–9045. doi:10.1080/21655979.2021.1992333
Zhao, Z., Li, X., Jian, D., Hao, P., Rao, L., and Li, M. (2017). Hsa_circ_0054633 in Peripheral Blood Can Be Used as a Diagnostic Biomarker of Pre-diabetes and Type 2 Diabetes Mellitus. Acta Diabetol. 54 (3), 237–245. doi:10.1007/s00592-016-0943-0
Keywords: gestational diabetes mellitus, circRNA, early diagnosis, GDM, pathophysiology
Citation: Zhang Y-p, Ye S-z, Li Y-x, Chen J-l and Zhang Y-s (2022) Research Advances in the Roles of Circular RNAs in Pathophysiology and Early Diagnosis of Gestational Diabetes Mellitus. Front. Cell Dev. Biol. 9:739511. doi: 10.3389/fcell.2021.739511
Received: 11 July 2021; Accepted: 02 December 2021;
Published: 04 January 2022.
Edited by:
Michael E. Symonds, University of Nottingham, United KingdomReviewed by:
Alison J. Eastman, Vanderbilt University Medical Center, United StatesCopyright © 2022 Zhang, Ye, Li, Chen and Zhang. This is an open-access article distributed under the terms of the Creative Commons Attribution License (CC BY). The use, distribution or reproduction in other forums is permitted, provided the original author(s) and the copyright owner(s) are credited and that the original publication in this journal is cited, in accordance with accepted academic practice. No use, distribution or reproduction is permitted which does not comply with these terms.
*Correspondence: Yi-sheng Zhang, bmJkb2N0b3J6aGFuZ0AxNjMuY29t
Disclaimer: All claims expressed in this article are solely those of the authors and do not necessarily represent those of their affiliated organizations, or those of the publisher, the editors and the reviewers. Any product that may be evaluated in this article or claim that may be made by its manufacturer is not guaranteed or endorsed by the publisher.
Research integrity at Frontiers
Learn more about the work of our research integrity team to safeguard the quality of each article we publish.