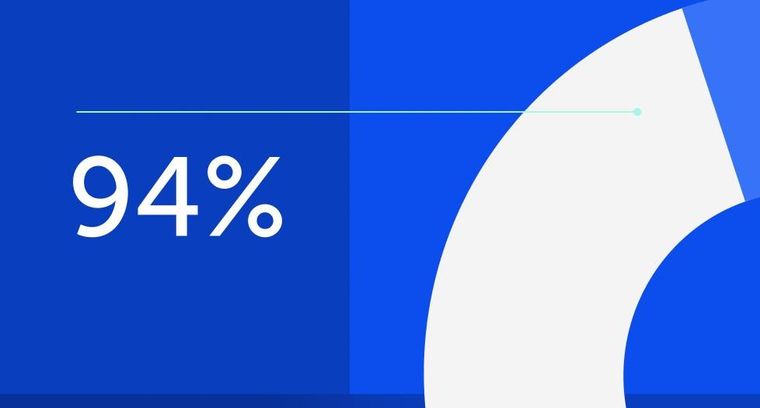
94% of researchers rate our articles as excellent or good
Learn more about the work of our research integrity team to safeguard the quality of each article we publish.
Find out more
REVIEW article
Front. Cell Dev. Biol., 08 September 2021
Sec. Cellular Biochemistry
Volume 9 - 2021 | https://doi.org/10.3389/fcell.2021.733751
This article is part of the Research TopicBiology and Pharmacological Effects of Extracellular Vesicles in CancerView all 16 articles
Cell death induction has become popular as a novel cancer treatment. Ferroptosis, a newly discovered form of cell death, features regulated, iron-dependent accumulation of lipid hydroperoxides. Since this word “ferroptosis” was coined, numerous studies have examined the complex relationship between ferroptosis and cancer. Here, starting from the intrinsic hallmarks of cancer and cell death, we discuss the theoretical basis of cell death induction as a cancer treatment. We review various aspects of the relationship between ferroptosis and cancer, including the genetic basis, epigenetic modification, cancer stem cells, and the tumor microenvironment, to provide information and support for further research on ferroptosis. We also note that exosomes can be applied in ferroptosis-based therapy. These extracellular vesicles can deliver different molecules to modulate cancer cells and cell death pathways. Using exosomes to control ferroptosis occurring in targeted cells is promising for cancer therapy.
Cancer has become one of the major threats to human health. A report estimated that in 2021 in the United States, there will be 1,898,160 new cancer cases and 608,570 cancer deaths (Siegel et al., 2021). Although the cancer mortality rate has decreased in recent years, access to healthcare has also decreased due to the COVID-19 pandemic, which has led to hampered cancer diagnosis and treatment (Siegel et al., 2021). As widely applied chemoradiotherapy is showing its drawbacks, such as frequent resistance and toxic side effects, cell death induction is becoming increasingly popular for developing novel cancer treatment.
Common forms of cell death, such as apoptosis, autophagy, and necroptosis, are all related to reactive oxygen species (ROS) and are regulated by ROS. For example, ROS can facilitate the extrinsic apoptosis pathway through negative regulation of the cellular FLICE-inhibitory protein (Wang et al., 2008) and can induce intrinsic apoptosis pathways by triggering quick release of Cyt-c (Madesh and Hajnoczky, 2001) and regulating the Bcl-2 protein family (Burlacu, 2003). Evidence shows that ROS generated from ETC and NOX can regulate several pathways that mediate autophagy induction (Li et al., 2011), and AMPK can be activated by AMPK kinase after H2O2 treatment, which also results in autophagy induction (Choi et al., 2001). In addition, ETC- and NOX-derived ROS are involved in necroptosis facilitation (Dixon and Stockwell, 2014). Evidence of cell death regulation, mediated by ROS, can also be found in ferroptosis and chemosensitization (Galadari et al., 2017).
Given the strong relationship between ROS and cell death, regulating ROS generation upward or controlling oxidative defense downward has become central for new cancer treatments, which have been enhanced by the finding that cancer cells process a higher level of ROS than do healthy cells (Galadari et al., 2017). A higher level of ROS, partly attributed to defective mitochondrial oxidative metabolism (Tafani et al., 2016), can lead to two opposite outcomes: the promotion of cancer and its suppression. Cancer suppression occurs because an elevated ROS level promotes various cell death processes, as mentioned above. Cancer promotion occurs because an elevated ROS level does the following:
(a) facilitates tumorigenesis through damaging or modifying cellular proteins, DNA, and lipids, leading to activation or inhibition of various tumorigenesis related signaling cascades (Tafani et al., 2016);
(b) promotes angiogenesis by mediating the proliferation, migration, and tube formation of endothelial cells (Potente et al., 2011) or by modulating various vascular endothelial growth factors;
(c) contributes to invasion and metastasis through active involvement in essential events including modulating signaling kinases and the cytoskeleton (Tochhawng et al., 2013); and
(d) participates in chemoresistance (Ledoux et al., 2003).
Cancer cells exhibiting a greater ROS level display increased activity of antioxidant enzymes, which help create a homeostasis for cell surviving. Therefore, it would be valuable to develop therapeutic strategies to break the redox homeostasis in cancer cells and activate cell death pathways to limit cancer progression. There are two possible approaches: the first is to decrease intracellular ROS. This can be done by, for example, hindering mitochondrial ETC and the activation of NOX, thus inhibiting ROS generation. This technique has been demonstrated in several cancer cell lines and has been proven to be beneficial. A study induced apoptosis in PANC-1 pancreatic cancer cells using diphenylene iodonium, which suppressed ROS generation through inhibiting NOX4 (Mochizuki et al., 2006). The opposite strategy consists of increasing the ROS to a toxic level and thus triggering cell death pathways. Researchers report that piperlongumine, a natural small molecule, can selectively induce ROS-dependent cell death in cancer (Chen et al., 2014). Moreover, glucose metabolism is thought to be related to ROS elimination, and a study has shown that glucose deprivation can induce cytotoxicity in MCF-7/ADR human multidrug-resistant breast cancer cells (Gupta et al., 1997; Lee et al., 1997).
Cancer therapy based on cell death induction has become an important research topic, and ferroptosis, a newly discovered form of cell death, has gained general attention. In this paper, we review the literature on ferroptosis and its relationship with cancer from different perspectives, including proto-oncogene and tumor suppressor gene, epigenetics, cancer stem cells (CSCs), and the tumor microenvironment (TME). Based on the new insights into cancer treatments using cell death induction, we believe ferroptosis to be a promising candidate for cancer treatment. As numerous molecules, ranging from RNAs to plant-derived natural compounds, have been demonstrated to have a therapeutic effect on cancer via the induction of ferroptosis-like cell death, drug delivery, which is a critical step in the application of ferroptosis as a cancer treatment, is still being discussed. Given various advantages, such as easy tissue penetration, low toxicity, and low immunogenicity, exosomes are believed to be a reliable drug delivery system able to selectively target specific cells (Figure 1). Here, we provide a simple overview of exosomes and their potential applications in ferroptosis-based therapy.
Figure 1. The schematic diagram of modulating ferroptosis in cancer inhibition based on an exosome delivery system. Cancer development and treatment efficacy are impacted by genetic factors, epigenetic modifications, cancer stem cells, and the tumor microenvironment (TME). Ferroptosis is a double-edged sword in cancer survival because it is regulated by different signaling pathways. Cancer treatment could benefit from using an exosome delivery system to control the onset of ferroptosis.
A newly discovered form of cell death, different from apoptosis, autophagy, and necroptosis, called ferroptosis has recently gained recognition for use in cancer treatment. Ferroptosis, a word coined in 2012 (Dixon et al., 2012), is a form of regulated cell death characterized by iron-dependent accumulation of lipid hydroperoxides to lethal levels. It was first used to describe a cell death process induced by a small molecule called erastin, which inhibits the intake of cystine, resulting in glutathione depletion and inactivation of the phospholipid potentially toxic lipid peroxidase 4 (GPX4) (Yang et al., 2014). GPX4 converts lipid hydroperoxide, which is potentially toxic, to a non-toxic form of lipid alcohol (Ursini et al., 1982). Therefore, inactivation or inhibition of the enzyme GPX4 triggers overwhelming lipid peroxidation that causes iron-dependent cell death. Regulation of ferroptosis can be achieved generally by interfering with iron metabolism and ROS metabolism, and the ferroptosis process can be suppressed by iron chelators, lipophilic antioxidants, lipid peroxidation, and the depletion of polyunsaturated fatty acids and correlates with the accumulation of lipid-peroxidation markers (Stockwell et al., 2017).
For the successful application of ferroptosis in cancer treatment, a more concrete understanding of ferroptosis and cancer is needed. The following section contains a review of recent research on ways in which ferroptosis interacts with cancer, especially as regards cancer-related genes, epigenetics, the TME, and so on, and provides a short review of research on ferroptosis regulation and its application to cancer treatment.
The RAS family of small GTPase, including HRAS, NRAS, and KRAS, is closely related to ferroptosis since the two most well-known ferroptosis inducers, erastin (eradicator of RAS and ST) and RSL3 (RAS-selective Lethal 3), are technically oncogenic RAS-selective lethal small molecules (Yagoda et al., 2007; Yang and Stockwell, 2008). The relationship between ferroptosis and RAS has been carefully investigated by numerous studies (Table 1). For example, researchers have found that HRASV12 expressing cancer cells are electively sensitive to ferroptosis, and KRAS silencing in KRAS mutant Calu-1 cells strongly reduces erastin sensibility (Yagoda et al., 2007). A possible explanation might be that constitutive RAS pathway activity promotes TFRC (a gene related to iron metabolism) expression while suppressing the expression of iron storage proteins. However, more evidence for the relation between RAS mutation and erastin sensibility cannot be found in some cancer cell lines (Yang et al., 2014). In contrast, RMS13 rhabdomyosarcoma cells that overexpress HRAS, KRAS, or NRAS are resistant to erastin and RAL3 (Schott et al., 2015), which means RAS does affect erastin sensitivity, while the oncogenic RAS pathway is not the sole determinant of ferroptosis sensitivity (Dixon and Stockwell, 2019). Other research has shown that, in an NRASQ61L expressing HL-60 cell line, high mobility group box 1 (HMGB1) is an essential regulator of erastin-induced ferroptosis (Ye et al., 2019). ADP Ribosylation Factor 6 (ARF6), a part of the RAS superfamily, facilitates high sensitivity to RSL3-induced lipid peroxidation (Ye et al., 2020). Reports have shown that oncogenic RAS induces rapid increase of ROS partly through upregulating NOX1 (Irani et al., 1997; Mitsushita et al., 2004). In mice with KRAS-driven pancreatic ductal adenocarcinoma (PDAC), high iron diets and GPX4 depletion, which results in 8-OHG release, lead to macrophage infiltration and activation (Dai E. et al., 2020).
Because of its role in cell cycle arrest, senescence, and apoptosis, as well as for its interesting role in metabolism, oxidative responses, and ferroptosis, p53 has long been an important focus of research. p53’s essential function of survival promotion is confirmed by the fact that cells are more sensitive to ferroptosis after p53 depletion through CRISPR/Cas9 (Tarangelo et al., 2018). By antagonizing p53 activity, such as the O-GlcNAcylated c-Jun (the first discovered oncogenic transcription factor), cell death can be prevented (Eferl et al., 2003). Recently, p53-related signal pathways have been shown to modulate ferroptosis in the following ways.
Research has shown that p53 can suppress the expression of SLC7A11, a key component of the cystine/glutamate antiporter (Xc– system), leading to the inhibition of cystine uptake and sensitization to ferroptosis. For example, the p53 mutants p53R237H and p53R175H promote sensitivity to ferroptosis-like cell death, most likely through the combination of p53 mutants with NRF2 and through the suppression of the NRF2-dependent transactivation of SLC7A11 together with other antioxidant genes that oppose ferroptosis (Sasaki et al., 2002; Habib et al., 2015; Liu D.S. et al., 2017). Moreover, overexpression of SLA7A11 in human tumor suppresses ROS-induced ferroptosis and inhibits p533KR-mediated tumor growth suppression in xenograft models. Though mutant p533KR effectively downregulates SLC7A11, it does not affect other p53 target genes involved in cell cycle regulation or apoptosis (Jiang et al., 2015). In contrast, mutant p534KR98 is unable to reduce SLC7A11 expression (Wang et al., 2016).
As a transcription target of p53, the activity of spermidine/spermine N1-acetyltransferase 1 (SAT1), the rate-limiting enzyme in polyamine catabolism, induces lipid peroxidation and sensitizes cells to undergo ferroptosis and the deletion of SAT1 suppresses p53 and p533KR-mediated ferroptosis. However, while p53 modulates SLC7A11 expression, the expression and activity of SLC7A11 and GPX4 are not associated with SAT1, and only ferrostatin-1 can inhibit ROS-induced ferroptosis in Tet-on cells (Ou et al., 2016). Research has also found that SAT1 induction is associated with the expression of arachidonate 15-lipoxygenase (ALOX15), which is essential in p53-mediated ferroptosis (Chu et al., 2019). Unfortunately, the p53–SAT1–ALOX15 axis has not been fully explained.
The metabolism of glutamine, one of the essential components of ferroptosis, is catalyzed by cytosolic glutamine aminotransferases or by mitochondrial glutaminases (Gao et al., 2015; Altman et al., 2016). The expression of glutaminase 2 (GLS2), which has been identified as a transcriptional target of p53, mediates oxygen consumption, mitochondrial respiration, and ATP generation in cancer cells. Based on the evidence that GLS2 facilitates GSH production in several cancer cell lines, GLS2 is recognized as a negative regulator of ferroptosis (Hu et al., 2010).
Colorectal cancer (CRC) caused by a number of genetic disorders, including KRAS mutation, p53 mutation, and p53 depletion, which provides additional evidence for the survival-promoting function of p53. Interestingly, this p53 function might partly be achieved by modulating ferroptosis. Research has found that p53 can inhibit ferroptosis by modulating the localization and activity, but not expression, of dipeptidyl peptidase-4 (DPP4), leading to survival promoting functions. This process occurs through a post-translational interaction with protease DPP4, which strengthens membrane lipid peroxidation in a protease-independent way via interaction with an ROS-generating NOX (Xie et al., 2017).
Cyclin dependent kinase inhibitor 1A (CDKN1A/p21), also known as p21WAF1/Cip1, is a key mediator of p53-dependent cell cycle arrest after DNA damage (Abbas and Dutta, 2009). A recent study has shown that the expression of CDKN1A, mediated by p53, delays the onset of ferroptosis in response to subsequent cystine deprivation in cancer cells (Tarangelo et al., 2018). As two negative regulators of p53, MDM2 and MDMX facilitate ferroptosis with or without p53, most likely by altering the lipid profile of cells (Venkatesh et al., 2020), which is confirmed by evidence that with the treatment of MDM2 inhibitor nutlin-3, p53 expression increases and leads to the suppression of Xc– system inhibitor-induced ferroptosis in HT-1080 cells (Tarangelo et al., 2018). The function of CDKN1A in cell cycle arrest, which is unable to trigger ferroptosis, is primarily achieved by binding to and suppressing the kinase activity of the cyclin-dependent kinases (CDKs) (Abbas and Dutta, 2009).
Interestingly, a recent study reported that retention of p53 in the nucleus, mediated by the interaction of long non-coding RNA (lncRNA) P53RRA, and Ras GTPase-activating protein-binding protein 1 (G3BP1), leads to cell cycle arrest, apoptosis, and ferroptosis. This is because p53 is displaced from the G3BP1 complex (Mao et al., 2018).
Studies tend to view Myc proteins as transcriptional factors that exert tumorigenesis functions by activating and suppressing target genes (Lutz et al., 2002). Evidence has shown a relationship between Myc and ferroptosis. A recent study reported that egl nine homolog 1 (EGLN1) and Myc activate lymphoid-specific helicase (LSH) expression through HIF-1α, and that LSH suppresses ferroptosis through the interaction with WDR76, leading to the activation of lipid metabolism-associated genes (Jiang et al., 2017). Another study reported that the depletion of VHL, a major tumor suppressor of clear cell renal cell carcinoma (ccRCC), leads to the stabilization of the hypoxia inducible factors HIF-1α and HIF-2α. This paper also found that exogenous expression of pVHL can revert ccRCC cells to an oxidative metabolism and a state of insensitivity to ferroptosis induction. Myc-dependent tumor growth in mouse models can be inhibited by GSH synthesis suppression (Miess et al., 2018). A newly identified oncogene, DJ-1, displays ferroptosis resistance and can synergistically transform mouse NIH3T3 cells together with activated GTPase HRAS and MYC proto-oncogene (c-Myc) (Jiang et al., 2020).
Members of the Myb family are found in all eukaryotic lineages, the function of which is to regulate fundamental cellular processes, metabolism, and cellular differentiation (Prouse and Campbell, 2012). Evidence shows that c-Myb is involved in ferroptosis through a cysteine dioxygenase 1 (CDO1)–GPX4 axis. Silencing CDO1 leads to suppression of erastin-induced ferroptosis in vitro and in vivo, and inhibition of CDO1 restores cellular GSA levels, which prevents ROS generation. This paper demonstrates that c-Myb transcriptionally regulates CDO1 and inhibition of CDO1 expression upregulates GPX4 (Hao et al., 2017).
Cellular SRC (c-SRC), the product of the SRC gene, is involved in tumorigenesis, invasion, and the metastatic phenotype (Alper and Bowden, 2005). A recent study has found that the SRC gene is one of the targeting sites of GPX4, the differential expression of which regulates cell proliferation, cancer progression, apoptosis, and ferroptosis (Wei J. et al., 2020). Another report demonstrated that, mediated by α6β4 integrin, the activation of SRC and STAT3 could inhibit ACSL4 expression, leading to the protection of adherent epithelial and carcinoma cells form erastin-induced ferroptosis (Brown et al., 2017). This is partly because ferroptosis cannot be triggered while there is a lack membranes enriched by ACSL4-mediated long polyunsaturated fatty acids. It was also proved that matrix-detached epithelial and cancer cells cluster spontaneously through a pathway involved with Nectin-4 (also known as cell adhesion protein PVRL4), the process of which sustains GPX4 expression and buffers against lipid peroxidation by stimulating the PVRL4/α6β4/Src axis signal pathway (Brown et al., 2018).
The retinoblastoma (Rb) protein is the founding member of a protein family that exerts a strong regulatory function on the transcription of various genes in eukaryotes (Knudsen and Knudsen, 2008). Ferroptosis in hepatocellular carcinoma can be promoted, resulting in two or three times more cell death, by sorafenib treatment combining with Rb knockdown using RNA interference (Louandre et al., 2015).
Non-coding RNAs (ncRNAs) are RNAs in the transcriptome and will not be translated into proteins. They are identified as several subfamilies based on their molecular size and shape, including long non-coding RNAs (lncRNAs), microRNAs (miRNAs), small nuclear RNAs (snRNAs), and small interfering RNAs (siRNAs) (Hombach and Kretz, 2016). Non-coding RNAs are increasingly regarded as essential regulators of ferroptosis in cancer and a better understanding of them can provide novel ideas for cancer treatment.
MiRNAs exhibit functions by binding to the 3′-untranslated regions of their target mRNAs and thus prevent the expression process (Majidinia et al., 2020). Studies have demonstrated that miRNAs regulate ferroptosis through direct and indirect approaches. For example, miR-7-5p inhibits ferroptosis by downregulating mitoferrin and reducing iron levels in radio-resistant cells (Tomita et al., 2019). miR-6852, which is regulated by lncRNA linc00336, can inhibit lung cancer progression by promoting ferroptosis. Besides direct regulation, evidence shows that miRNAs affect the metabolism of GSH, a scavenger of ROS that protects lipid membrane (Hsu et al., 2019). For instance, miR-18a and miR-218 downregulate GSH levels in hepatocellular carcinoma and bladder cancer separately by targeting GCL (Anderton et al., 2017; Li P. et al., 2017), while miR-152 and miR-155 decrease GSH levels in hepatocellular carcinoma and lung cancer separately by targeting GST (Huang et al., 2010; Lv et al., 2016), the general pathway by which miRNAs modulate GSH level. GST can be targeted and modulated by various miRNAs, including miR-92b-3p, miR-124, miR129-5P, miR-130b, miR-133a/b, miR-144, miR-153-1/2, miR-186, miR-302c-5p, miR-513a-3p, miR-590-3p/5p, miR-36645p, miR-3714, and let-7a-5p (Zhang et al., 2020e). In the meantime, iron metabolism mainly includes the interaction between transferrin (TF) and TF receptor (TFR), which can also be regulated by miRNAs. For example, in CRC and hepatocellular cancer, TFR can be targeted by miRNAs including miR-22, miR-31, miR-141, miR-145, miR-152, miR-182, miR-200a, miR-320, miR-758, and miR19463–65, resulting in a disruption between TF and TFR and the following iron importing process (Zhang et al., 2020e). Moreover, iron can regulate miRNA levels. Levels of miR-107 and miR-125b can be suppressed by iron in hepatocellular carcinoma (Lobello et al., 2016; Zou et al., 2016), while levels of miR-146a and miR-150 can be increased by iron (Sriramoju et al., 2015; Lobello et al., 2016), which might be due to iron’s induction of excess ROS (Zhang et al., 2020e). Moreover, miRNAs regulate the NRF2 pathway through by targeting Kelch-like ECh-Associated Protein 1 (KEAP1) and NRF2 mRNAs (Zhang et al., 2020e).
LncRNAs generally serve as regulators of transcription factors in the nucleus or as sponges of miRNAs in the cytoplasm (Wu et al., 2020). The silence of lncRNA ZFAS1, which acts as a ceRNA and sponge for miR-150-5p, suppresses ferroptosis by downregulating SLC38A1 (Yang Y.N. et al., 2020). Besides the relationship between linc00336/miR-6852 and lncRNA P53rra/G3BP1 mentioned above, lncRNAs modulate ferroptosis indirectly by targeting ferroptosis-associated factors (Table 2). A study reported that the reduction of lncRNA ROR leads to reduced GST expression in breast cancer (Li Y.H. et al., 2017), and silencing lncRNA Neat1 contributes to an increase of GST (Wang et al., 2018). Other studies have shown that lncRNAs are associated with iron metabolism and that silencing lncRNA PVT1 suppresses TFR expression and obstructs iron intake via miR-150 (Xu et al., 2018). Evidence also shows that lncRNAs affect the expression of NRF2 by directly and indirectly modulating KEAP1 levels, while NRF2 is associated with lncRNA regulation (Zhang et al., 2020e). Besides the above factors, ROS levels can be regulated by lncRNAs. For instance, decreased expression of lncRNA H19 increases ROS via the MAPK/ERK signaling pathway (Ding et al., 2018), while the reduction of lncRNA growth arrest specific 5 in melanoma enhances intracellular ROS (Chen et al., 2019). Increased levels of lncRNA GABPB1-AS1 downregulate the peroxiredoxin-5 peroxidase gene and ultimately inhibits the antioxidant capacity of cells (Qi et al., 2019).
Other ncRNAs, such as circRNAs RNAs, rRNAs, piRNAs, snRNAs, and snoRNAs, also interact with ferroptosis in various cancer types (Table 3). For circRNAs, circIL4R facilitates tumorigenesis and prevents ferroptosis by regulating the miR-541-3p/GPX4 axis (Xu et al., 2020a). The reduction of circ-TTBK2 delays proliferation and invasion of glioma cells by regulating the miR-761/ITGB8 axis and triggering ferroptosis (Zhang et al., 2020b). Another study reports that circRNA clARs regulate ferroptosis through interacting with the RNA binding protein ALKBH5 (Liu et al., 2020). A recent study has shown that reduction of circ0008035 enhances the anticancer effects of erastin and RSL3 by increasing iron accumulation and lipid peroxidation (Li C. et al., 2020). Moreover, studies revealed that tRNA upregulates ferroptosis by suppressing GSH biosynthesis in a GPX4-independent pattern. However, in contrast, tRNAs can also downregulate ferroptosis by enhancing the antioxidant defense system (Zhang et al., 2020e). Moreover, rRNAs, piRNAs, snRNAs, and snoRNAs were recently found to be involved in ferroptosis-associated pathways (Zhang et al., 2020e).
Various studies have revealed the function of DNA or protein methylation in tumor progression, ROS metabolism, and iron metabolism; however, despite being one of the most common molecular modification in epigenetics, the direct relationship between methylation and ferroptosis has not been fully discussed.
Some studies demonstrated the indirect regulation of ferroptosis via DNA and protein methylation. For example, lymphoid-specific helicase (LSH), a DNA methylation modifier, can activate lipid metabolism-associated genes to inhibit ferroptosis by interacting with WDR76 (Jiang et al., 2017), and together with another W40 protein DCAF8, they function as a crucial nexus in epigenetic regulation of ferroptosis, controlling LSH degradation by adapted oxidative damage sensing through DNA hydroxymethylation (Huang et al., 2020). The silencing of the DNA methylation of the elongation of very long-chain fatty acid protein 5 (ELOVL5) and fatty acid desaturase 1 (FADS1) leads to ferroptosis resistance, and these two enzymes are usually upregulated in mesenchymal-type gastric cancer cells (Lee J.-Y. et al., 2020). Besides, GPX4 methylation is also reported to be related to ferroptosis regulation. For example, homocysteine (Hcy), an amino acid involved in DNA methylation, facilitates GPX4 methylation that leads to upregulation of oxidative stress and ferroptosis in nucleus pulposus (Zhang et al., 2020c). Another study reported that the increased expression of GPX4 in cancer tissues might be partly attributed to a lower level of DNA methylation and histone acetylation (Zhang et al., 2020d). A report has shown that KDM3B, a histone H3 lysine 9 demethylase, can protect against erastin-induced ferroptosis and is thus considered a potential epigenetic regulator of ferroptosis (Wang et al., 2020). Meanwhile, the expression of iron metabolism-associated genes, including TRFC, FTH1, and FTL, can be modulated by the epigenetic silencing of the iron-responsive element binding protein 2 (IREB2) (Dixon et al., 2012), while other perturbations of mechanisms, including acetylation and methylation, have been observed to regulate iron metabolism in cancer cells by controlling transcript encoding proteins (Manz et al., 2016).
Some ferroptosis regulation pathways have been found recently in which tumor-associated factors are usually involved. For instance, in head and neck cancer cells, diminution of the hypermethylation of CDH1 results in increased E-cadherin expression and decreased ferroptosis susceptibility (Lee J. et al., 2020); this work also provides evidence that epithelial–mesenchymal transition (EMT) promotes ferroptosis via epigenetic regulation pathways. The lower promoter methylation of GPX1, a member of the GPX family that interact with oxidative stress, results in high expression levels of GPX1 in some cancer cell lines (Wei R. et al., 2020). Another study shows that JQ1 can inhibit BRD4 expression and ultimately induce ferroptosis through two pathways, either by inhibiting the histone methylase G9a or by activating the histone deacetylase SIRT1, which can recognize the acetylation site and recruit transcriptional factors (Sui et al., 2019).
A widely occurring post-translational modification, acetylation plays a role in ferroptosis mainly through direct and indirect interaction with ferroptosis regulators. The acetylation of genes and proteins involved in ferroptosis is reported to regulate iron-dependent cell death. For example, an acetylation defect is observed in mutant p533KR, which indirectly inhibits cysteine absorption and reduces GSH consumption, leading to lipid peroxidation and ferroptosis (Jiang et al., 2015). Acetylation absence in the mouse p53 K98 site and on other positions in the DNA-binding domain can result in the loss of tumor suppression functions in xenografts and ferroptosis (Wang et al., 2016). Another study reported that RSL3 promotes the protein expression and acetylation of ALOX12, the key protein in initiating membrane phospholipid oxidation (Wang Y. et al., 2021). Indirect regulation is also observable. It has been reported that suppression of EMT mediated by histone deacetylase SIRT1 gene silencing or pharmacological inhibition consequently decreases ferroptosis, which further suggests that EMT promotes ferroptosis through epigenetic regulation pathways (Lee J. et al., 2020). Moreover, acetylation of HMGB1, a damage-associated molecular pattern molecule (DAMP), is released by ferroptosis cells in an autophagy-dependent manner (Wen et al., 2019).
Ubiquitination is a post-translational modification involved in essential host processes that has been reported to regulate ferroptosis epigenetically. The most common regulation pathway involves interaction with SLC7A11, which is essential in the Xc– system. Evidence suggests that the deubiquitinase OTUB1, usually overexpressed in cancers, replicates the ferroptosis process and promotes tumor development by stabilizing the cystine transporter SLC7A11 (Gan, 2019). Once deubiquitinase is suppressed, caspase-dependent apoptosis and GPX4-degradation-dependent ferroptosis is activated, contributing to the accumulation of ubiquitination proteins that facilitates cell death (Yang L. et al., 2020). The tumor suppressor BAP1, an H2A deubiquitinating enzyme, can reduce SLC7A11 expression by inhibiting H2A ubiquitination (H2Aub) on the SLC7A11 promoter, thus controlling ferroptosis (Zhang Y.L. et al., 2019). Another study shows that p53 may also be involved in ubiquitination-dependent regulation of ferroptosis. For example, p53 decreases H2B ubiquitination occupancy in the SLC7A11 gene regulatory domain and represses its expression (Wang Y. et al., 2019). Ubiquitination also regulates ferroptosis by modulating ferritin degradation. In iron deficiency, nuclear receptor coactivator 4 (NCOA4) specifically binds iron-rich ferritin to autophagosomes through FTH1 and transports it to the lysosome for iron release, while NCOA4 can be degraded through ubiquitination, which affects that stability of ferritin. Therefore, suppressing NCOA4 can inhibit the degradation of ferritin and the occurrence of ferroptosis (Capelletti et al., 2020).
CSCs are a small section of tumor cells that possesses the ability to self-renew, initiate tumors, and cause resistance to conventional anticancer agents. Different from regular cancer cells, CSCs have a lower level of ROS, which might contribute to a slower growth rate, reduced oxidative metabolism, and elevated expression of the ROS scavenging system (Bystrom et al., 2014; Ding et al., 2015; Hyewon and Navdeep, 2018). Lipid intake pathways are upregulated in CSCs, providing energy essential for survival, which explains why interference with GPX4 pathways seems to render CSCs sensitive to ferroptosis (Recalcati et al., 2019; Visweswaran et al., 2020). Higher iron levels are another characteristic of CSCs, such that ferroptosis may be a good method for eliminating CSCs, which are less susceptible to classical anticancer apoptosis-inducing agents. Indications of higher iron levels consist of the expression levels of TFR1 and its ligand iron-loaded TF is upregulated in glioblastoma CSCs compared to non-CSCs (David et al., 2015). Furthermore, cellular iron, TFR1, and TF uptake are more robust in breast CSCs compared to non-CSCs (Mai et al., 2017). TFR1 and ferritin are essential for propagation and formation of tumors in vivo. On the other hand, forced reduction of intracellular iron reduces the proliferation and tumorigenicity of ovarian CSCs (Basuli et al., 2017). Evidence points to multiple roles of intracellular iron in CSC proliferation and stemness maintenance (Recalcati et al., 2019). For instance, in breast cancer cells, low iron levels are associated with a lower expression of EMT markers (Guo et al., 2015). Iron also mediates the downregulation of E-cadherin, a hallmark of EMT (Brookes et al., 2008).
Higher iron levels do not necessarily relate to ROS levels and ferroptosis, but it has been proven that CSCs are highly sensitive to ferroptosis due to increased expression levels of TFR1, and thus ferroptosis-based treatment and therapeutic interference of iron homeostasis can have a curing effect on cancer (Mai et al., 2017). Notably, recent studies indicate that triggering ferroptosis may specifically kill CSCs; for example, salinomycin can drive ferroptosis-based cell death in breast CSCs (Zhao et al., 2019), and ironmycin, a derivative of salinomycin, can specifically trigger iron accumulation in lysosomes, activating cell death pathways consistent with ferroptosis (Mai et al., 2017). Some small-molecule ferroptotic agents also have the potential to selectively kill breast CSCs (Taylor et al., 2019). The blocking of the lysosomal iron translocation of CSCs by inhibiting the divalent metal transporter 1 (DMT1) leads to iron accumulation and cell death with features of ferroptosis (Turcu et al., 2020). In colorectal CSCs, knockdown or inhibition of SLC7A11 significantly and specifically kills cancer cells and thus attenuates chemoresistance in CRC (Xu et al., 2020c). Besides, two nitroimidazoles (Koike et al., 2020), itraconazole (Xu et al., 2021), and dichloroacetate (Sun et al., 2021) are also proven to have therapeutic potential through promoting ferroptosis in CSCs.
The TME functions as a cradle for tumorigenesis and cancer progression. Understanding the TME and ferroptosis interaction may provide novel and effective anticancer strategies.
A recent study reports that ferroptosis can promote tumor growth by driving macrophage polarization in the TME (Dai E.Y. et al., 2020). Hypoxia is one of the known characteristics of the TME, which is controlled by the hypoxia-inducible factor (HIF) (Labiano et al., 2015). Researchers have found that hypoxia is an essential positive trigger for ferroptosis, and HIF-2α enhances lipid peroxidation while the depletion of HIF-1α decreases sensitivity to ferroptosis (Zou et al., 2019). Moreover, iron metabolism-associated genes, including FTH, TFR1, and SLC11A2, are regulated by hypoxia-responsive elements (HREs) in the promotor region (Li et al., 2019).
Ferroptosis is thought to be linked to antitumor immunity. This was first proved by the study that immunotherapy-activated CD8+ T lymphocytes can induce ferroptosis in cancer cells by downregulating SLC7A11 and SLC3A2, encoding subunits of system Xc–. Technically, this study has shown that tumor cell coculture with IFN-γ-rich supernatant obtained from activated T cells induces lipid peroxidation and ferroptosis (Wang W.M. et al., 2019). Overexpression of ferroptosis suppressor protein 1 (FSP1) or cytosolic GPX4 stimulates the genesis of ferroptosis-resistant CD8+ T cells without compromising their function, while the depletion of ferroptosis sensitivity-promoting enzyme acyl-CoA synthetase long-chain family member 4 (ACSL4) protected CD8+ T cells from ferroptosis but impaired antitumor CD8+ T cell response (Drijvers et al., 2021).
Other studies have demonstrated that cancer cells that have undergone ferroptosis can release high mobility group Box 1 (HMGB1) in an autophagy-dependent manner (Yu et al., 2015; Wen et al., 2019). When HMGB1 is released into the TME because of cancer cell death, it can stimulate the innate immune system by interacting with several pattern recognition receptors (Sims et al., 2010; Yamazaki et al., 2014). Evidence shows that during ferroptosis, tumor cells supply arachidonic acid for eicosanoid synthesis, which can strengthen antitumor immunity (Angeli et al., 2019). Moreover, ferroptosis induction in tumor cells is thought to be related to the release of prostaglandin E2 (PGE2), which facilities the evasion from immune surveillance (Yang et al., 2014).
The synergism between ferroptosis and immunomodulation in cancer has been widely investigated in recent decades. On the one hand, TME immunomodulation can trigger macrophage polarization from alternately activated macrophages M2 to classically activated macrophages M1, offering intertumoral H2O2 for the Fenton reaction (Zanganeh et al., 2016), which effectively generates ROS and triggers lipid peroxidation (Yang and Stockwell, 2016; Sun et al., 2017). On the other hand, ferroptosis in tumor cells can release tumor antigens and generate an immunogenic TME, thus enhancing the immunomodulation response (Zhang F. et al., 2019). Nanoparticles (NPs), which can passively infiltrate tumor tissues because of the enhanced permeability and retention, act as a drug-loading platform with high loading efficiency, and release specific cargos in tumor issues, are gaining recognition in immunotherapy.
Some metal elements are especially popular for their inherent physicochemical properties, and metal-containing nanomaterials are designed for ferroptosis-driven therapy. They can function in different manners, including facilitating Fenton-like reactions, providing hydrogen peroxide, damaging the reducing system, and disturbing cellular communication (Fei et al., 2020). For example, biomimetic magnetosome, composed of an Fe3O4 magnetic nanocluster with a TGF-β inhibitor loaded inside and a PD-1 antibody anchored on the membrane surface, was developed to promote ferroptosis/immunomodulation synergism in cancer (Zhang F. et al., 2019). MnOx nanospikes, as TME-responsive nano-adjuvants and immunogenic cell death drugs, were also designed for cancer nanovaccine-based immunotherapy (Ding et al., 2020). In another study, in which ultrasmall CaO2 and Fe3O4 were co-loaded on to dendritic mesoporous silica NPs, researchers showed that these particles can achieve tumor specialized localization and induction of Fenton reaction, thus triggering ferroptosis (Li and Rong, 2020). The Fe3O4-PLGA-Ce6 nanosystem, which dissociates in acidic TME, and the Fe2+-based metal–organic framework, which delivers Fe2+ to cancer cells, can also promote the Fenton reaction and facilitate ferroptosis (Xu et al., 2020b; Chen Q. et al., 2021).
Although nanotechnology is increasingly used in cancer treatment, the application of NP-based therapy faces various issues, such as intrinsic immunogenicity and residual cytotoxicity (Shen et al., 2018). In a new approach that has high biocompatibility, low immunogenicity, preferred tumor homing, and high efficiency in cargo delivery, the 30- to 120-nm endocytic lipid bilayer membrane-derived vesicles is attracting attention as a novel drug carrier for ferroptosis induction (Qin and Xu, 2014; Kibria et al., 2018). Attempts have been made to use exosomes as carriers for ferroptosis-inducing drugs to trigger cell death among cancer cells. For example, engineered M1 macrophages, with CCR2 overexpression, are employed as Fe3O4 NP carriers (Li et al., 2021). Moreover, a well-known ferroptosis inducer, erastin, can be loaded into exosomes labeled with folate and delivered to cancer cells that express the folate receptor to generate ROS and glutathione depletion (Yu et al., 2019).
Generated from the plasma membrane (Simons and Raposo, 2009), exosomes were first used for carrying clotting suppressors (Wolf, 1967). Since then, these extracellular vesicles have been shown to be secreted by various kinds of cells, including dendritic cells, macrophages, T cells, B cells, mesenchymal stem cells, endothelial cells, epithelial cells, and various cancer cells (Song H. et al., 2021).
Exosomes are generated from late endosomes through several different pathways. Endosomal-sorting complexes required for transport (ESCRTs), which recognize ubiquitylated proteins, are the most characterized one among genesis pathways, while others may involve sphingomyelinases (Trajkovic et al., 2008), sphingosine-1-phosphate, and tetraspanin-enriched domains (Brinton et al., 2015). Four ESCRTs, numbered from 0 to 3, consist of many proteins able to recognize ubiquitinated cargoes. Technically, ESCRT-0 subunits recruit proteins for internalization, such as ubiquitinated proteins and clathrin. ESCRT-1 and ESCRT-2 control the initiation of the budding process and facilitate the enzymatic de-ubiquitination of cargo proteins before the formation of intraluminal vesicles (ILVs). ILVs then gather to form larger membranous vesicles in the intracellular compartment. ESCRT-3 drives membrane invagination and separation (Ha et al., 2016; McGough and Vincent, 2016). According to the genesis process, the composition pattern of exosomes faithfully reflects their parent cells. For proteins displayed on the surface, adhesion molecules, which belong to the tetraspanin and integrin families, are the most abundant. These proteins, which are generally membrane crossing, include CD9, CD63, CD81, and CD82 and regulate processes like fusion, migration, and adhesion. They usually attach to each other or associate with nearby proteins, such as integrins, to form a tetraspanin membrane domain (Farooqi et al., 2018). The major histocompatibility complex II (MHC-II) may be present on the surface of exosomes and is involved in promoting certain T-cell responses (Elena and Myung Soo, 2015). Moreover, tumor-derived exosomes are able to promote cancer cell migration and metastasis, containing various kinds of integrin, such as exosomal integrins αvβ6 for prostate cancer (Carmine et al., 2015), α6β4 and α6β1 for lung cancer, and αvβ5 for liver cancer metastasis (Hoshino et al., 2015). Other protein molecules, such as annexins, flotillin, and GTPases, are associated with lipid fractions on exosomes and serve transportation and fusion functions (Colombo et al., 2014). Besides proteins, lipids are another main component of exosomes, which depend on the type of parent cell plasma membrane. Phosphatidylethanolamines, phosphatidylcholines, phosphatidylinositols, phosphatidylserines, sphingomyelins, lysobisphosphatidic acid (bis-monoacylglycerol phosphate), phosphatidic acid, cholesterol, lysophosphatidylcholines, ceramide, and phosphoglycerides have been found in these membranes (Caroline et al., 2007). The intraluminal composition of the exosomal membrane also depends on the parent cells and particularly on their cytoplasmatic content. Exosomes shuttle through the body, allowing the horizontal transfer of their cargo while fusing with target cells and releasing their content via an endocytosis process, and thus participate in various regulation pathways (Escrevente et al., 2011). A wide range of molecules have been found in different cell-derived exosomes, such as heat shock proteins, cytoskeletal proteins, lipids, and enzymes, along with nucleic acid molecules, such as miRNAs, mRNAs, ncRNAs, mitochondrial DNA, and single-strand DNA (Farooqi et al., 2018).
Among the numerous biological roles played by exosomes, their function in cancer is becoming increasingly apparent. A number of studies have revealed that exosomes can regulate the function of target cells by secreting their contents into the TME, using crosstalk, and/or influencing major tumor-related pathways, including EMT, CSCs, angiogenesis, and metastasis involving several cell types (Ha et al., 2016; Wu et al., 2017). Moreover, drug resistance is partly attributed to exosomes, for cancer cells can encapsulate therapeutic drugs in exosomes and transport them out of tumor cells (Arrighetti et al., 2019). Evidence shows that exosomes also overlap with ferroptosis modulation. For example, mesenchymal stromal cells (MSCs) derived from human umbilical cord blood (HUCB-MSCs) tend to significantly inhibit the expression of DMT1 by miR-23a-3p to inhibit ferroptosis (Song Y. et al., 2021). The miR-522 inside exosomes, generated from cancer-associated fibroblasts (CAFs), can block lipid-ROS accumulation by targeting ALOX15 and thus inhibit ferroptosis (Zhang H. et al., 2020a). In a recent study, researchers found that ferroptosis promotes tumor growth by driving macrophage polarization in the TME. One kind of common KRAS mutant, KRASG12D, is secreted into the TME from tumor cells after succumbing to autophagy-dependent ferroptosis. This extracellular protein is then packaged into tumor-derived exosomes and is absorbed by macrophages, leading to the switch from the M1 phenotype to the M2 phenotype and accelerating cancer progression (Dai E.Y. et al., 2020). Prominin 2 is a pentaspanin protein involved in lipid dynamics regulation. It promotes the formation of ferritin-containing multivesicular bodies (MVBs) and exosomes that transport iron out of the cell and thus inhibits ferroptosis (Brown et al., 2019). Exosomes themselves are also proved to have some curing functions; for instance, rat plasma-derived exosomes can enhance cell proliferation and radio-resistance-related genes and yet downregulate ferroptosis in irradiated fibroblasts (Gan et al., 2021).
Despite the therapeutic potential of nucleic acid and protein drugs, their clinical application has been limited partly by a lack of appropriate delivery systems. Proteins and small RNAs can be loaded onto exosomes and delivered to target cells, interfering with various pathways. For example, a research team engineered human embryonic kidney (HEK) cells to produce exosomes able to target breast cancer cells overexpressing epidermal growth factor receptor (EGFR). In order to achieve elective targeting, researchers have engineered donor HEK cells to express the transmembrane domain of platelet-derived growth factor receptors fused to the GE11 peptide. Let-7a miRNA was introduced into GE11-positive exosomes using the lipofection method and HEK cells. Results show that miRNA exosomes have a curing effect on breast cancer (Ohno et al., 2013). As with ferroptosis, we reviewed a number of proteins and RNAs regulating ferroptosis-based cell death in the previous section. These molecules can be easily introduced to donor cells, and tumor targeting exosomes carrying these molecules can be used for cancer treatment. However, related research is lacking. Although the use of exosomes as a delivery system has its drawback (for example, quickly eliminating by the reticuloendothelial system, lack of efficient encapsulation methods, and potential immune responses), exosomes targeted at tumors may allow systemic administration of miRNA as cancer treatment and are thus worthy of attention.
Nanotechnology has been developed for drug delivery, but intrinsic immunogenicity and residual cytotoxicity have hindered its application. During recent decades, researchers turned to delivery systems based on natural and synthetic polymers and lipids because such liposomes possess valuable qualities, such as the incorporation of hydrophilic and hydrophobic drugs, and membrane penetration (Farooqi et al., 2018). However, disadvantages, such as lower circulation stability, rapid clearance by phagocytosis, and increased toxicity, challenge the application of liposomes (Ha et al., 2016). In this respect, exosomes display better tolerance and lower toxicity due to their ubiquitous presence and similarity in structure and composition to biological membranes (Bang and Thum, 2012). Exosomes can penetrate through tissues, deliver contents directly into cellular compartments, and evade the immune system. They are also able to target specific organs and tissues (Hood et al., 2011). The application of engineered cell strains with special plasmid vectors that encode fusion proteins helps to develop targeted exosome-based delivery systems by achieving amenable membrane modifications and desirable attributes especially when targeting a specific cell type (Farooqi et al., 2018).
Modified exosomes can be selectively used to deliver drugs to specific cells and present advantages, such as high effectiveness and reduced toxicity. Exosomes generated by genetically engineered cell stains present designed proteins on the surface, which can selectively drive exosomes and their contents to targeted cells. For example, engineered immature dendritic cells (imDCs) in mice express a well-characterized exosomal membrane protein (Lamp2b) fused to αv integrin-specific iRGD peptide (CRGDKGPDC). Doxorubicin (Dox), produced by engineering imDCs, can be loaded to exosomes through electroporation. In vivo and in vitro experiments have shown that the Dox-exosomes process possesses high efficacy in Dox delivery and targeting to breast cancer, effective cancer suppression, and low toxicity (Tian et al., 2014). One study showed that exosomes derived from brain cells that expressed brain specific surface proteins can cross the blood–brain barrier and deliver drugs to the other side (Yang et al., 2015). For ferroptosis, therapeutic drugs, such as erastin and newly recognized natural ferroptosis-inducing compounds, can be loaded onto tumor targeting exosomes. This may provide new avenues for cancer treatment. Attempts have been made and the results are positive. Nevertheless, challenging issues remain to be solved, such as poor encapsulation efficiency and the interference form exosomal endogenous nucleic acids and proteins.
Apoptosis, necroptosis, pyroptosis, and ferroptosis are the most widely studied types of programmed cell death. These types of programmed cell death are all involved in cancer progression and therapy. In our lab, we focus on regulating the crosstalk among different types of programmed cell death to broaden the application of anti-tumor drugs (Liu S. et al., 2017; Huang et al., 2018, 2021a,b; Xiang et al., 2019; Li T. et al., 2020). Inducing a certain type of programmed cell death specifically can have profound significance for cancer treatment. Ferroptosis is an iron-dependent form of programmed cell death triggered by unrestricted lipid peroxidation and subsequent plasma membrane rupture. It is well known that cancer development and treatment can be affected by genetic factors, epigenetic modifiers, CSCs, and the TME (Xiang et al., 2019). As mentioned above, ferroptosis could be induced to exert anti-tumor functions via signaling pathway modulation, non-coding RNA expression, DNA methylation, histone modification, CSCs, microenvironment remodeling, and so on. However, it is not clear how to utilize and manipulate ferroptosis in cancer treatment, specifically. On the one hand, studies need to deeply exploit the molecular and cellular mechanisms underlying ferroptosis; on the other hand, combining ferroptosis with biological materials is a promising alternative strategy. As the smallest extracellular vesicles and endogenous source of nanocarriers, exosomes show great potential for cargo delivery, including RNA, protein, drugs, and ions. Most importantly, exosomes have been shown to transport iron out of the cell to regulate ferroptosis (Brown et al., 2019). In addition, gene engineered exosomes exhibit promising characteristics in cancer treatment (Cheng et al., 2021). Therefore, adjusting the cargo of exosomes and/or engineering their spreading pathways could target cancer cells (especially CSCs) or the TME in order to induce ferroptosis, thus achieving a positive therapeutic outcome.
SW and YH wrote the manuscript. TL and WL reviewed the manuscript. All authors approved the manuscript.
This study was funded by the National Natural Science Foundation of China (No. 81502582) and the Fundamental Research Funds for the Central Universities (N182004002).
The authors declare that the research was conducted in the absence of any commercial or financial relationships that could be construed as a potential conflict of interest.
All claims expressed in this article are solely those of the authors and do not necessarily represent those of their affiliated organizations, or those of the publisher, the editors and the reviewers. Any product that may be evaluated in this article, or claim that may be made by its manufacturer, is not guaranteed or endorsed by the publisher.
Abbas, T., and Dutta, A. (2009). p21 in cancer: intricate networks and multiple activities. Nat. Rev. Cancer 9, 400–414. doi: 10.1038/nrc2657
Alper, O., and Bowden, E. T. (2005). Novel insights into c-Src. Curr. Pharm. Des. 11, 1119–1130. doi: 10.2174/1381612053507576
Altman, B. J., Stine, Z. E., and Dang, C. V. (2016). From krebs to clinic: glutamine metabolism to cancer therapy. Nat. Rev. Cancer 16, 619–634. doi: 10.1038/nrc.2016.71
Anderton, B., Camarda, R., Balakrishnan, S., Balakrishnan, A., Kohnz, R., Lim, L., et al. (2017). MYC-driven inhibition of the glutamate-cysteine ligase promotes glutathione depletion in liver cancer. EMBO Rep. 18:e201643068. doi: 10.15252/embr.201643068
Angeli, J. P. F., Krysko, D. V., and Conrad, M. (2019). Ferroptosis at the crossroads of cancer-acquired drug resistance and immune evasion. Nat. Rev. Cancer 19, 405–414. doi: 10.1038/s41568-019-0149-1
Arrighetti, N., Corbo, C., Evangelopoulos, M., Pasto, A., Zuco, V., and Tasciotti, E. (2019). Exosome-like nanovectors for drug delivery in cancer. Curr. Med. Chem. 26, 6132–6148. doi: 10.2174/0929867325666180831150259
Bang, C., and Thum, T. (2012). Exosomes: new players in cell-cell communication. Int. J. Biochem. Cell Biol. 44, 2060–2064. doi: 10.1016/j.biocel.2012.08.007
Basuli, D., Tesfay, L., Deng, Z., Paul, B., Yamamoto, Y., Ning, G., et al. (2017). Iron addiction: a novel therapeutic target in ovarian cancer. Oncogene 36, 4089–4099. doi: 10.1038/onc.2017.11
Brinton, L. T., Sloane, H. S., Kester, M., and Kelly, K. A. (2015). Formation and role of exosomes in cancer. Cell. Mol. Life Sci. 72, 659–671. doi: 10.1007/s00018-014-1764-3
Brookes, M. J., Boult, J., Roberts, K., Cooper, B. T., Hotchin, N. A., Matthews, G., et al. (2008). A role for iron in Wnt signalling. Oncogene 27, 966–975. doi: 10.1038/sj.onc.1210711
Brown, C. W., Amante, J. J., and Mercurio, A. M. (2018). Cell clustering mediated by the adhesion protein PVRL4 is necessary for alpha6beta4 integrin-promoted ferroptosis resistance in matrix-detached cells. J. Biol. Chem. 293, 12741–12748. doi: 10.1074/jbc.RA118.003017
Brown, C. W., Amante, J. J., Chhoy, P., Elaimy, A. L., Liu, H., Zhu, L. J., et al. (2019). Prominin2 drives ferroptosis resistance by stimulating iron export. Dev. Cell 51, 575–586.e4. doi: 10.1016/j.devcel.2019.10.007
Brown, C. W., Amante, J. J., Goel, H. L., and Mercurio, A. M. (2017). The alpha6beta4 integrin promotes resistance to ferroptosis. J. Cell Biol. 216, 4287–4297. doi: 10.1083/jcb.201701136
Burlacu, A. (2003). Regulation of apoptosis by Bcl-2 family proteins. J. Cell. Mol. Med. 7, 249–257. doi: 10.1111/j.1582-4934.2003.tb00225.x
Bystrom, L. M., Guzman, M. L., and Rivella, S. (2014). Iron and reactive oxygen species: friends or foes of cancer cells? Antioxid. Redox Signal. 20, 1917–1924. doi: 10.1089/ars.2012.5014
Capelletti, M. M., Manceau, H., Puy, H., and Peoc’h, K. (2020). Ferroptosis in liver diseases: an overview. Int. J. Mol. Sci. 21:23. doi: 10.3390/ijms21144908
Carmine, F., Amrita, S., Brad, J. Z., Renato, V. I., and Lucia, R. L. (2015). The αvβ6 Integrin Is Transferred Intercellularly via Exosomes∗. J. Biol. Chem. 290, 4545–4551. doi: 10.1074/jbc.C114.617662
Caroline, S., Karine, L., Bertrand, P., and Michel, R. (2007). Exosome lipidomics unravels lipid sorting at the level of multivesicular bodies. Biochimie 89, 205–212. doi: 10.1016/j.biochi.2006.10.014
Chen, L., Yang, H. X., Yi, Z. H., Jiang, L., Li, Y. Q., Han, Q. Q., et al. (2019). LncRNA GAS5 regulates redox balance and dysregulates the cell cycle and apoptosis in malignant melanoma cells. J. Cancer Res. Clin. Oncol. 145, 637–652. doi: 10.1007/s00432-018-2820-4
Chen, Q., Ma, X., Xie, L., Chen, W., Xu, Z., Song, E., et al. (2021). Iron-based nanoparticles for MR imaging-guided ferroptosis in combination with photodynamic therapy to enhance cancer treatment. Nanoscale 13, 4855–4870. doi: 10.1039/d0nr08757b
Chen, W. B., Balakrishnan, K., Kuang, Y. Y., Han, Y. Y., Fu, M., Gandhi, V., et al. (2014). Reactive Oxygen Species (ROS) inducible DNA cross-linking agents and their effect on cancer cells and normal lymphocytes. J. Med. Chem. 57, 4498–4510. doi: 10.1021/jm401349g
Chen, W., Fu, J., Chen, Y., Li, Y., Ning, L., Huang, D., et al. (2021). Circular RNA circKIF4A facilitates the malignant progression and suppresses ferroptosis by sponging miR-1231 and upregulating GPX4 in papillary thyroid cancer. Aging 13, 16500–16512. doi: 10.18632/aging.203172
Cheng, L., Zhang, X., Tang, J., Lv, Q., and Liu, J. (2021). Gene-engineered exosomes-thermosensitive liposomes hybrid nanovesicles by the blockade of CD47 signal for combined photothermal therapy and cancer immunotherapy. Biomaterials 275:120964. doi: 10.1016/j.biomaterials.2021.120964
Choi, S.-L., Kim, S.-J., Lee, K.-T., Kim, J., Mu, J., Birnbaum, M. J., et al. (2001). The regulation of AMP-activated protein kinase by H2O2. Biochem. Biophys. Res. Commun. 287, 92–97. doi: 10.1006/bbrc.2001.5544
Chu, B., Kon, N., Chen, D., Li, T., Liu, T., Jiang, L., et al. (2019). ALOX12 is required for p53-mediated tumour suppression through a distinct ferroptosis pathway. Nat. Cell Biol. 21, 579–591. doi: 10.1038/s41556-019-0305-6
Colombo, M., Raposo, G., and Théry, C. (2014). Biogenesis, secretion, and intercellular interactions of exosomes and other extracellular vesicles. Annu. Rev. Cell Dev. Biol. 30, 255–289. doi: 10.1146/annurev-cellbio-101512-122326
Dai, E., Han, L., Liu, J., Xie, Y., Zeh, H. J., Kang, R., et al. (2020). Ferroptotic damage promotes pancreatic tumorigenesis through a TMEM173/STING-dependent DNA sensor pathway. Nat. Commun. 11:6339. doi: 10.1038/s41467-020-20154-8
Dai, E. Y., Han, L., Liu, J., Xie, Y. C., Kroemer, G., Klionsky, D. J., et al. (2020). Autophagy-dependent ferroptosis drives tumor-associated macrophage polarization via release and uptake of oncogenic KRAS protein. Autophagy 16, 2069–2083. doi: 10.1080/15548627.2020.1714209
David, L. S., Tyler, E. M., Qiulian, W., William, A. F., Nupur, K. D., James, S. H., et al. (2015). Preferential iron trafficking characterizes glioblastoma stem-like cells. Cancer Cell 28, 441–455. doi: 10.1016/j.ccell.2015.09.002
Ding, B., Zheng, P., Jiang, F., Zhao, Y., Wang, M., Chang, M., et al. (2020). MnOx nanospikes as nanoadjuvants and immunogenic cell death drugs with enhanced antitumor immunity and antimetastatic effect. Angew. Chem. Int. Ed. Engl. 59, 16381–16384. doi: 10.1002/anie.202005111
Ding, K., Liao, Y. N., Gong, D. H., Zhao, X., and Ji, W. (2018). Effect of long non-coding RNA H19 on oxidative stress and chemotherapy resistance of CD133+cancer stem cells via the MAPK/ERK signaling pathway in hepatocellular carcinoma. Biochem. Biophys. Res. Commun. 502, 194–201. doi: 10.1016/j.bbrc.2018.05.143
Ding, S., Li, C., Cheng, N., Cui, X., Xu, X., and Zhou, G. (2015). Redox regulation in cancer stem cells. Oxid. Med. Cell. Longev. 2015:750798. doi: 10.1155/2015/750798
Dixon, S. J., and Stockwell, B. R. (2014). The role of iron and reactive oxygen species in cell death. Nat. Chem. Biol. 10, 9–17. doi: 10.1038/nchembio.1416
Dixon, S. J., and Stockwell, B. R. (2019). The hallmarks of ferroptosis. Annu. Rev. Cancer Biol. 3, 35–54. doi: 10.1146/annurev-cancerbio-030518-055844
Dixon, S., Lemberg, K., Lamprecht, M., Skouta, R., Zaitsev, E., Gleason, C., et al. (2012). Ferroptosis: an iron-dependent form of non-apoptotic cell death. Cell 149, 1060–1072. doi: 10.1016/j.cell.2012.03.042
Drijvers, J. M., Gillis, J. E., Muijlwijk, T., Nguyen, T. H., Gaudiano, E. F., Harris, I. S., et al. (2021). Pharmacologic screening identifies metabolic vulnerabilities of CD8(+) T cells. Cancer Immunol. Res. 9, 184–199. doi: 10.1158/2326-6066.CIR-20-0384
Eferl, R., Ricci, R., Kenner, L., Zenz, R., David, J.-P., Rath, M., et al. (2003). Liver tumor development: c-jun antagonizes the proapoptotic activity of p53. Cell 112, 181–192. doi: 10.1016/S0092-8674(03)00042-4
Elena, V. B., and Myung Soo, K. (2015). Using exosomes, naturally-equipped nanocarriers, for drug delivery. J. Control. Release 219, 396–405. doi: 10.1016/j.jconrel.2015.07.030
Escrevente, C., Keller, S., Altevogt, P., and Costa, J. (2011). Interaction and uptake of exosomes by ovarian cancer cells. BMC Cancer 11:108. doi: 10.1186/1471-2407-11-108
Farooqi, A. A., Desai, N. N., Qureshi, M. Z., Librelotto, D. R. N., Gasparri, M. L., Bishayee, A., et al. (2018). Exosome biogenesis, bioactivities and functions as new delivery systems of natural compounds. Biotechnol. Adv. 36, 328–334. doi: 10.1016/j.biotechadv.2017.12.010
Fei, W., Zhang, Y., Ye, Y., Li, C., Yao, Y., Zhang, M., et al. (2020). Bioactive metal-containing nanomaterials for ferroptotic cancer therapy. J. Mater. Chem. B 8, 10461–10473. doi: 10.1039/d0tb02138e
Gai, C., Liu, C., Wu, X., Yu, M., Zheng, J., Zhang, W., et al. (2020). MT1DP loaded by folate-modified liposomes sensitizes erastin-induced ferroptosis via regulating miR-365a-3p/NRF2 axis in non-small cell lung cancer cells. Cell Death Dis. 11:751. doi: 10.1038/s41419-020-02939-3
Galadari, S., Rahman, A., Pallichankandy, S., and Thayyullathil, F. (2017). Reactive oxygen species and cancer paradox: to promote or to suppress? Free Radic. Biol. Med. 104, 144–164. doi: 10.1016/j.freeradbiomed.2017.01.004
Gan, B. Y. (2019). DUBbing ferroptosis in cancer cells. Cancer Res. 79, 1749–1750. doi: 10.1158/0008-5472.Can-19-0487
Gan, F., Wang, R., Lyu, P., Li, Y., Fu, R., Du, Y., et al. (2021). Plasma-derived exosomes boost the healing of irradiated wound by regulating cell proliferation and ferroptosis. J. Biomed. Nanotechnol. 17, 100–114. doi: 10.1166/jbn.2021.3008
Gao, M., Monian, P., Quadri, N., Ramasamy, R., and Jiang, X. (2015). Glutaminolysis and transferrin regulate ferroptosis. Mol. Cell 59, 298–308. doi: 10.1016/j.molcel.2015.06.011
Guo, W., Zhang, S., Chen, Y., Zhang, D., Yuan, L., Cong, H., et al. (2015). An important role of the hepcidin–ferroportin signaling in affecting tumor growth and metastasis. Acta Biochim. Biophys. Sin. 47, 703–715. doi: 10.1093/abbs/gmv063
Gupta, A. K., Lee, Y. J., Galoforo, S. S., Berns, C. M., Martinez, A. A., Corry, P. M., et al. (1997). Differential effect of glucose derivation on MAPK activation in drug sensitive human breast carcinoma MCF-7 and multidrug resistant MCF-7/ADR cells. Mol. Cell. Biochem. 170, 23–30. doi: 10.1023/a:1006890316102
Ha, D., Yang, N., and Nadithe, V. (2016). Exosomes as therapeutic drug carriers and delivery vehicles across biological membranes: current perspectives and future challenges. Acta Pharm. Sin. B 6, 287–296. doi: 10.1016/j.apsb.2016.02.001
Habib, E., Linher-Melville, K., Lin, H.-X., and Singh, G. (2015). Expression of xCT and activity of system xc– are regulated by NRF2 in human breast cancer cells in response to oxidative stress. Redox Biol. 5, 33–42. doi: 10.1016/j.redox.2015.03.003
Hao, S., Yu, J., He, W., Huang, Q., Zhao, Y., Liang, B., et al. (2017). Cysteine dioxygenase 1 mediates erastin-induced ferroptosis in human gastric cancer cells. Neoplasia 19, 1022–1032. doi: 10.1016/j.neo.2017.10.005
Hombach, S., and Kretz, M. (2016). Non-coding RNAs: classification, biology and functioning. Adv. Exp. Med. Biol. 937, 3–17.
Hood, J. L., Roman, S. S., and Wickline, S. A. (2011). Exosomes released by melanoma cells prepare sentinel lymph nodes for tumor metastasis. Cancer Res. 71, 3792–3801. doi: 10.1158/0008-5472.Can-10-4455
Hoshino, A., Costa-Silva, B., Shen, T.-L., Rodrigues, G., Hashimoto, A., Tesic Mark, M., et al. (2015). Tumour exosome integrins determine organotropic metastasis. Nature 527, 329–335. doi: 10.1038/nature15756
Hsu, J. L., Chou, J. W., Chen, T. F., Hsu, J. T., Fang-Yi, S., Lan, J. L., et al. (2019). Glutathione peroxidase 8 negatively regulates caspase-4/11 to protect against colitis. EMBO Mol. Med. 12:e9386. doi: 10.15252/emmm.201809386
Hu, W., Zhang, C., Wu, R., Sun, Y., Levine, A., and Feng, Z. (2010). Glutaminase 2, a novel p53 target gene regulating energy metabolism and antioxidant function. Proc. Natl. Acad. Sci. U.S.A. 107:7455. doi: 10.1073/pnas.1001006107
Huang, D., Li, Q., Sun, X., Sun, X., Tang, Y., Qu, Y., et al. (2020). CRL4DCAF8 dependent opposing stability control over the chromatin remodeler LSH orchestrates epigenetic dynamics in ferroptosis. Cell Death Differ. 28, 1593–1609. doi: 10.1038/s41418-020-00689-5
Huang, J., Wang, Y., Guo, Y., and Sun, S. (2010). Down-regulated microRNA-152 induces aberrant DNA methylation in hepatitis B virus-related hepatocellular carcinoma by targeting DNA methyltransferase 1. Hepatology 52, 60–70. doi: 10.1002/hep.23660
Huang, Y., Du, J., Mi, Y., Li, T., Gong, Y., Ouyang, H., et al. (2018). Long non-coding RNAs contribute to the inhibition of proliferation and EMT by pterostilbene in human breast cancer. Front. Oncol. 8:629. doi: 10.3389/fonc.2018.00629
Huang, Y., Yuan, K., Tang, M., Yue, J., Bao, L., Wu, S., et al. (2021a). Melatonin inhibiting the survival of human gastric cancer cells under ER stress involving autophagy and Ras-Raf-MAPK signalling. J. Cell Mol. Med. 25, 1480–1492. doi: 10.1111/jcmm.16237
Huang, Y., Zhou, Z., Zhang, J., Hao, Z., He, Y., Wu, Z., et al. (2021b). lncRNA MALAT1 participates in metformin inhibiting the proliferation of breast cancer cell. J. Cell Mol. Med. 25, 7135–7145. doi: 10.1111/jcmm.16742
Hyewon, K., and Navdeep, S. C. (2018). Regulation of redox balance in cancer and T cells. J. Biol. Chem. 293, 7499–7507. doi: 10.1074/jbc.TM117.000257
Irani, K., Xia, Y., Zweier, J. L., Sollott, S. J., Der, C. J., Fearon, E. R., et al. (1997). Mitogenic signaling mediated by oxidants in Ras-transformed fibroblasts. Science 275, 1649–1652. doi: 10.1126/science.275.5306.1649
Jiang, L., Chen, X. B., Wu, Q., Zhu, H. Y., Du, C. Y., Ying, M. D., et al. (2020). The C terminus of DJ-1 determines its homodimerization, MGO detoxification activity and suppression of ferroptosis. Acta Pharmacol. Sin. 42, 1150–1159. doi: 10.1038/s41401-020-00531-1
Jiang, L., Kon, N., Li, T., Wang, S. J., Su, T., Hibshoosh, H., et al. (2015). Ferroptosis as a p53-mediated activity during tumour suppression. Nature 520, 57–62. doi: 10.1038/nature14344
Jiang, Y., Mao, C., Yang, R., Yan, B., Shi, Y., Liu, X., et al. (2017). EGLN1/c-Myc induced lymphoid-specific helicase inhibits ferroptosis through lipid metabolic gene expression changes. Theranostics 7, 3293–3305. doi: 10.7150/thno.19988
Kibria, G., Ramos, E. K., Wan, Y., Gius, D. R., and Liu, H. P. (2018). Exosomes as a drug delivery system in cancer therapy: potential and challenges. Mol. Pharm. 15, 3625–3633. doi: 10.1021/acs.molpharmaceut.8b00277
Knudsen, E. S., and Knudsen, K. E. (2008). Tailoring to RB: tumour suppressor status and therapeutic response. Nat. Rev. Cancer 8, 714–724. doi: 10.1038/nrc2401
Koike, N., Kota, R., Naito, Y., Hayakawa, N., Matsuura, T., Hishiki, T., et al. (2020). 2-Nitroimidazoles induce mitochondrial stress and ferroptosis in glioma stem cells residing in a hypoxic niche. Commun. Biol. 3:450. doi: 10.1038/s42003-020-01165-z
Labiano, S., Palazon, A., and Melero, I. (2015). Immune response regulation in the tumor microenvironment by hypoxia. Semin. Oncol. 42, 378–386. doi: 10.1053/j.seminoncol.2015.02.009
Ledoux, S., Yang, R., Friedlander, G., and Laouari, D. (2003). Glucose depletion enhances P-glycoprotein expression in hepatoma cells: role of endoplasmic reticulum stress response. Cancer Res. 63, 7284–7290.
Lee, J., You, J. H., Kim, M. S., and Roh, J. L. (2020). Epigenetic reprogramming of epithelial-mesenchymal transition promotes ferroptosis of head and neck cancer. Redox Biol. 37:101697. doi: 10.1016/j.redox.2020.101697
Lee, J.-Y., Nam, M., Son, H. Y., Hyun, K., Jang, S. Y., Kim, J. W., et al. (2020). Polyunsaturated fatty acid biosynthesis pathway determines ferroptosis sensitivity in gastric cancer. Proc. Natl. Acad. Sci. U.S.A. 117, 32433–32442. doi: 10.1073/pnas.2006828117
Lee, Y. J., Galoforo, S. S., Berns, C. M., Tong, W. P., Kim, H. R. C., and Corry, P. M. (1997). Glucose deprivation-induced cytotoxicity in drug resistant human breast carcinoma MCF-7/ADR cells: role of c-myc and bcl-2 in apoptotic cell death. J. Cell Sci. 110, 681–686.
Li, C., Tian, Y., Liang, Y., and Li, Q. C. (2020). Circ_0008035 contributes to cell proliferation and inhibits apoptosis and ferroptosis in gastric cancer via miR-599/EIF4A1 axis. Cancer Cell Int. 20:15. doi: 10.1186/s12935-020-01168-0
Li, P., Gao, M., Hu, Z., Xu, T., Chen, J., Ma, Y., et al. (2021). Synergistic ferroptosis and macrophage re-polarization using engineering exosome-mimic M1 nanovesicles for cancer metastasis suppression. Chem. Eng. J. 409:128217. doi: 10.1016/j.cej.2020.128217
Li, P., Yang, X., Cheng, Y., Zhang, X., Yang, C., Deng, X., et al. (2017). MicroRNA-218 increases the sensitivity of bladder cancer to cisplatin by targeting Glut1. Cell Physiol. Biochem. 41, 921–932. doi: 10.1159/000460505
Li, T., Yu, Y., Shi, H., Cao, Y., Liu, X., Hao, Z., et al. (2020). Magnesium in combinatorial with valproic acid suppressed the proliferation and migration of human bladder cancer cells. Front. Oncol. 10:58911. doi: 10.3389/fonc.2020.589112
Li, Y. H., Jiang, B. H., Zhu, H. B., Qu, X. F., Zhao, L. Q., Tan, Y. R., et al. (2017). Inhibition of long non-coding RNA ROR reverses resistance to Tamoxifen by inducing autophagy in breast cancer. Tumor Biol. 39:11. doi: 10.1177/1010428317705790
Li, Z., and Rong, L. (2020). Cascade reaction-mediated efficient ferroptosis synergizes with immunomodulation for high-performance cancer therapy. Biomater. Sci. 8, 6272–6285. doi: 10.1039/d0bm01168a
Li, Z., Jiang, L., Chew, S. H., Hirayama, T., Sekido, Y., and Toyokuni, S. (2019). Carbonic anhydrase 9 confers resistance to ferroptosis/apoptosis in malignant mesothelioma under hypoxia. Redox Biol. 26:11. doi: 10.1016/j.redox.2019.101297
Li, Z.-Y., Yang, Y., Ming, M., and Liu, B. (2011). Mitochondrial ROS generation for regulation of autophagic pathways in cancer. Biochem. Biophys. Res. Commun. 414, 5–8. doi: 10.1016/j.bbrc.2011.09.046
Liu, D. S., Duong, C. P., Haupt, S., Montgomery, K. G., House, C. M., Azar, W. J., et al. (2017). Inhibiting the system xC-/glutathione axis selectively targets cancers with mutant-p53 accumulation. Nat. Commun. 8:14844. doi: 10.1038/ncomms14844
Liu, S., Liang, B., Jia, H., Jiao, Y., Pang, Z., and Huang, Y. (2017). Evaluation of cell death pathways initiated by antitumor drugs melatonin and valproic acid in bladder cancer cells. FEBS Open Biol. 7, 798–810. doi: 10.1002/2211-5463.12223
Liu, Z., Wang, Q., Wang, X., Xu, Z., Wei, X., and Li, J. (2020). Circular RNA cIARS regulates ferroptosis in HCC cells through interacting with RNA binding protein ALKBH5. Cell Death Discov. 6:72. doi: 10.1038/s41420-020-00306-x
Lobello, N., Biamonte, F., Pisanu, M. E., Faniello, M. C., Jakopin, Z., Chiarella, E., et al. (2016). Ferritin heavy chain is a negative regulator of ovarian cancer stem cell expansion and epithelial to mesenchymal transition. Oncotarget 7, 62019–62033. doi: 10.18632/oncotarget.11495
Louandre, C., Marcq, I., Bouhlal, H., Lachaier, E., Godin, C., Saidak, Z., et al. (2015). The retinoblastoma (Rb) protein regulates ferroptosis induced by sorafenib in human hepatocellular carcinoma cells. Cancer Lett. 356(2 Pt. B), 971–977. doi: 10.1016/j.canlet.2014.11.014
Lutz, W., Leon, J., and Eilers, M. (2002). Contributions of myc to tumorigenesis. Biochim. Biophys. Acta 1602, 61–71. doi: 10.1016/S0304-419X(02)00036-7
Lv, L. X., An, X. M., Li, H. Y., and Ma, L. X. (2016). Effect of miR-155 knockdown on the reversal of doxorubicin resistance in human lung cancer A549/dox cells. Oncol. Lett. 11, 1161–1166. doi: 10.3892/ol.2015.3995
Lyu, N., Zeng, Y., Kong, Y., Chen, Q., Deng, H., Ou, S., et al. (2021). Ferroptosis is involved in the progression of hepatocellular carcinoma through the circ0097009/miR-1261/SLC7A11 axis. Ann. Transl. Med. 9:675. doi: 10.21037/atm-21-997
Madesh, M., and Hajnoczky, G. (2001). VDAC-dependent permeabilization of the outer mitochondrial membrane by superoxide induces rapid. J. Cell Biol. 155:1003. doi: 10.1083/jcb.200105057
Mai, T. T., Hamaï, A., Hienzsch, A., Cañeque, T., Müller, S., Wicinski, J., et al. (2017). Salinomycin kills cancer stem cells by sequestering iron in lysosomes. Nat. Chem. 9, 1025–1033. doi: 10.1038/nchem.2778
Majidinia, M., Karimian, A., Alemi, F., Yousefi, B., and Safa, A. (2020). Targeting miRNAs by polyphenols: novel therapeutic strategy for aging. Biochem. Pharmacol. 173:113688. doi: 10.1016/j.bcp.2019.113688
Manz, D. H., Blanchette, N. L., Paul, B. T., Torti, F. M., and Torti, S. V. (2016). Iron and cancer: recent insights. Ann. N. Y. Acad. Sci. 1368, 149–161. doi: 10.1111/nyas.13008
Mao, C., Wang, X., Liu, Y., Wang, M., Yan, B., Jiang, Y., et al. (2018). A G3BP1-interacting lncRNA promotes ferroptosis and apoptosis in cancer via nuclear sequestration of p53. Cancer Res. 78, 3484–3496. doi: 10.1158/0008-5472.CAN-17-3454
McGough, I. J., and Vincent, J.-P. (2016). Exosomes in developmental signalling. Development 143, 2482–2493. doi: 10.1242/dev.126516
Miess, H., Dankworth, B., Gouw, A. M., Rosenfeldt, M., Schmitz, W., Jiang, M., et al. (2018). The glutathione redox system is essential to prevent ferroptosis caused by impaired lipid metabolism in clear cell renal cell carcinoma. Oncogene 37, 5435–5450. doi: 10.1038/s41388-018-0315-z
Mitsushita, J., Lambeth, J. D., and Kamata, T. (2004). The superoxide-generating oxidase Nox1 is functionally required for Ras oncogene transformation. Cancer Res. 64, 3580–3585. doi: 10.1158/0008-5472.Can-03-3909
Mochizuki, T., Furuta, S., Mitsushita, J., Shang, W., Ito, M., Yokoo, Y., et al. (2006). Inhibition of NADPH oxidase 4 activates apoptosis via the AKT/apoptosis signal-regulating kinase 1 pathway in pancreatic cancer PANC-1 cells. Oncogene 25, 3699–3707. doi: 10.1038/sj.onc.1209406
Ohno, S., Takanashi, M., Sudo, K., Ueda, S., Ishikawa, A., Matsuyama, N., et al. (2013). Systemically injected exosomes targeted to EGFR deliver antitumor MicroRNA to breast cancer cells. Mol. Ther. 21, 185–191. doi: 10.1038/mt.2012.180
Ou, Y., Wang, S. J., Li, D., Chu, B., and Gu, W. (2016). Activation of SAT1 engages polyamine metabolism with p53-mediated ferroptotic responses. Proc. Natl. Acad. Sci. U.S.A. 113, E6806–E6812. doi: 10.1073/pnas.1607152113
Potente, M., Gerhardt, H., and Carmeliet, P. (2011). Basic and therapeutic aspects of angiogenesis. Cell 146, 873–887. doi: 10.1016/j.cell.2011.08.039
Prouse, M. B., and Campbell, M. M. (2012). The interaction between MYB proteins and their target DNA binding sites. Biochim. Biophys. Acta 1819, 67–77. doi: 10.1016/j.bbagrm.2011.10.010
Qi, W. C., Li, Z. H., Xia, L. J., Dai, J. S., Zhang, Q., Wu, C. F., et al. (2019). LncRNA GABPB1-AS1 and GABPB1 regulate oxidative stress during erastin-induced ferroptosis in HepG2 hepatocellular carcinoma cells. Sci. Rep. 9:12. doi: 10.1038/s41598-019-52837-8
Recalcati, S., Gammella, E., and Cairo, G. (2019). Dysregulation of iron metabolism in cancer stem cells. Free Radic. Biol. Med. 133, 216–220. doi: 10.1016/j.freeradbiomed.2018.07.015
Sasaki, H., Sato, H., Kuriyama-Matsumura, K., Sato, K., Maebara, K., Wang, H., et al. (2002). Electrophile response element-mediated induction of the cystine/glutamate exchange transporter gene expression∗. J. Biol. Chem. 277, 44765–44771. doi: 10.1074/jbc.M208704200
Schott, C., Graab, U., Cuvelier, N., Hahn, H., and Fulda, S. (2015). Oncogenic RAS mutants confer resistance of RMS13 rhabdomyosarcoma cells to oxidative stress-induced ferroptotic cell death. Front. Oncol. 5:131. doi: 10.3389/fonc.2015.00131
Shen, Z. Y., Song, J. B., Yung, B. C., Zhou, Z. J., Wu, A. G., and Chen, X. Y. (2018). Emerging strategies of cancer therapy based on ferroptosis. Adv. Mater. 30:15. doi: 10.1002/adma.201704007
Siegel, R. L., Miller, K. D., Fuchs, H. E., and Jemal, A. (2021). Cancer statistics, 2021. CA Cancer J. Clin. 71, 7–33. doi: 10.3322/caac.21654
Simons, M., and Raposo, G. (2009). Exosomes - vesicular carriers for intercellular communication. Curr. Opin. Cell Biol. 21, 575–581. doi: 10.1016/j.ceb.2009.03.007
Sims, G. P., Rowe, D. C., Rietdijk, S. T., Herbst, R., and Coyle, A. J. (2010). HMGB1 and RAGE in inflammation and cancer. Annu. Rev. Immunol. 28, 367–388.
Song, H., Liu, B., Dong, B., Xu, J., Zhou, H., Na, S., et al. (2021). Exosome-based delivery of natural products in cancer therapy. Front. Cell Dev. Biol. 9:650426. doi: 10.3389/fcell.2021.650426
Song, Y., Wang, B., Zhu, X., Hu, J., Sun, J., Xuan, J., et al. (2021). Human umbilical cord blood-derived MSCs exosome attenuate myocardial injury by inhibiting ferroptosis in acute myocardial infarction mice. Cell Biol. Toxicol. 37, 51–64. doi: 10.1007/s10565-020-09530-8
Sriramoju, B., Kanwar, R. K., and Kanwar, J. R. (2015). Lactoferrin induced neuronal differentiation: a boon for brain tumours. Int. J. Dev. Neurosci. 41, 28–36. doi: 10.1016/j.ijdevneu.2014.12.005
Stockwell, B. R., Friedmann Angeli, J. P., Bayir, H., Bush, A. I., Conrad, M., Dixon, S. J., et al. (2017). Ferroptosis: a regulated cell death nexus linking metabolism, redox biology, and disease. Cell 171, 273–285. doi: 10.1016/j.cell.2017.09.021
Sui, S. Y., Zhang, J., Xu, S. P., Wang, Q., Wang, P. Y., and Pang, D. (2019). Ferritinophagy is required for the induction of ferroptosis by the bromodomain protein BRD4 inhibitor (+)-JQ1 in cancer cells. Cell Death Dis. 10:17. doi: 10.1038/s41419-019-1564-7
Sun, J., Cheng, X., Pan, S., Wang, L., Dou, W., Liu, J., et al. (2021). Dichloroacetate attenuates the stemness of colorectal cancer cells via trigerring ferroptosis through sequestering iron in lysosomes. Environ. Toxicol. 36, 520–529. doi: 10.1002/tox.23057
Sun, W. J., Hu, Q. Y., Ji, W. Y., Wright, G., and Gu, Z. (2017). Leveraging physiology for precision drug delivery. Physiol. Rev. 97, 189–225. doi: 10.1152/physrev.00015.2016
Tafani, M., Sansone, L., Limana, F., Arcangeli, T., De Santis, E., Polese, M., et al. (2016). The interplay of reactive oxygen species, hypoxia, inflammation, and sirtuins in cancer initiation and progression. Oxid. Med. Cell. Longev. 2016:3907147. doi: 10.1155/2016/3907147
Tarangelo, A., Magtanong, L., Bieging-Rolett, K. T., Li, Y., Ye, J., Attardi, L. D., et al. (2018). p53 Suppresses metabolic stress-induced ferroptosis in cancer cells. Cell Rep. 22, 569–575. doi: 10.1016/j.celrep.2017.12.077
Taylor, W. R., Fedorka, S. R., Gad, I., Shah, R., Alqahtani, H. D., Koranne, R., et al. (2019). Small-molecule ferroptotic agents with potential to selectively target cancer stem cells. Sci. Rep. 9:14. doi: 10.1038/s41598-019-42251-5
Tian, Y. H., Li, S. P., Song, J., Ji, T. J., Zhu, M. T., Anderson, G. J., et al. (2014). A doxorubicin delivery platform using engineered natural membrane vesicle exosomes for targeted tumor therapy. Biomaterials 35, 2383–2390. doi: 10.1016/j.biomaterials.2013.11.083
Tochhawng, L., Deng, S., Pervaiz, S., and Yap, C. T. (2013). Redox regulation of cancer cell migration and invasion. Mitochondrion 13, 246–253. doi: 10.1016/j.mito.2012.08.002
Tomita, K., Fukumoto, M., Itoh, K., Kuwahara, Y., Igarashi, K., Nagasawa, T., et al. (2019). MiR-7-5p is a key factor that controls radioresistance via intracellular Fe2+ content in clinically relevant radioresistant cells. Biochem. Biophys. Res. Commun. 518, 712–718. doi: 10.1016/j.bbrc.2019.08.117
Trajkovic, K., Hsu, C., Chiantia, S., Rajendran, L., Wenzel, D., Wieland, F., et al. (2008). Ceramide triggers budding of exosome vesicles into multivesicular endosomes. Science 319, 1244–1247. doi: 10.1126/science.1153124
Turcu, A. L., Versini, A., Khene, N., Gaillet, C., Caneque, T., Muller, S., et al. (2020). DMT1 inhibitors kill cancer stem cells by blocking lysosomal iron translocation. Chemistry 26, 7369–7373. doi: 10.1002/chem.202000159
Ursini, F., Maiorino, M., Valente, M., Ferri, L., and Gregolin, C. (1982). Purification from pig liver of a protein which protects liposomes and biomembranes from peroxidative degradation and exhibits glutathione peroxidase activity on phosphatidylcholine hydroperoxides. Biochim. Biophys. Acta 710, 197–211. doi: 10.1016/0005-2760(82)90150-3
Venkatesh, D., O’Brien, N. A., Zandkarimi, F., Tong, D. R., Stokes, M. E., Dunn, D. E., et al. (2020). MDM2 and MDMX promote ferroptosis by PPARalpha-mediated lipid remodeling. Genes Dev. 34, 526–543. doi: 10.1101/gad.334219.119
Visweswaran, M., Arfuso, F., Warrier, S., and Dharmarajan, A. (2020). Aberrant lipid metabolism as an emerging therapeutic strategy to target cancer stem cells. Stem Cells 38, 6–14. doi: 10.1002/stem.3101
Wang, L., Azad, N., Kongkaneramit, L., Chen, F., Lu, Y., Jiang, B.-H., et al. (2008). The fas death signaling pathway connecting reactive oxygen species generation and FLICE inhibitory protein down-regulation. J. Immunol. 180, 3072–3080. doi: 10.4049/jimmunol.180.5.3072
Wang, M., Mao, C., Ouyang, L., Liu, Y., Lai, W., Liu, N., et al. (2019). Long noncoding RNA LINC00336 inhibits ferroptosis in lung cancer by functioning as a competing endogenous RNA. Cell Death Differ. 26, 2329–2343. doi: 10.1038/s41418-019-0304-y
Wang, S., Zhang, Q., Wang, Q. L., Shen, Q. C., Chen, X., Li, Z. Y., et al. (2018). NEAT1 paraspeckle promotes human hepatocellular carcinoma progression by strengthening IL-6/STAT3 signaling. Oncoimmunology 7:13. doi: 10.1080/2162402x.2018.1503913
Wang, S.-J., Li, D., Ou, Y., Jiang, L., Chen, Y., Zhao, Y., et al. (2016). Acetylation is crucial for p53-mediated ferroptosis and tumor suppression. Cell Rep. 17, 366–373. doi: 10.1016/j.celrep.2016.09.022
Wang, W. M., Green, M., Choi, J. E., Gijon, M., Kennedy, P. D., Johnson, J. K., et al. (2019). CD8(+) T cells regulate tumour ferroptosis during cancer immunotherapy. Nature 569, 270–274. doi: 10.1038/s41586-019-1170-y
Wang, Y., Yang, L., Zhang, X., Cui, W., Liu, Y., Sun, Q. R., et al. (2019). Epigenetic regulation of ferroptosis by H2B monoubiquitination and p53. EMBO Rep. 20:e47563. doi: 10.15252/embr.201847563
Wang, Y., Yu, R., Wu, L., and Yang, G. (2021). Hydrogen sulfide guards myoblasts from ferroptosis by inhibiting ALOX12 acetylation. Cell Signal. 78:109870. doi: 10.1016/j.cellsig.2020.109870
Wang, Y., Zhao, Y., Wang, H., Zhang, C., Wang, M., Yang, Y., et al. (2020). Histone demethylase KDM3B protects against ferroptosis by upregulating SLC7A11. FEBS Open Biol. 10, 637–643. doi: 10.1002/2211-5463.12823
Wang, Z., Chen, X., Liu, N., Shi, Y., Liu, Y., Ouyang, L., et al. (2021). A nuclear long non-coding RNA LINC00618 accelerates ferroptosis in a manner dependent upon apoptosis. Mol. Ther. 29, 263–274. doi: 10.1016/j.ymthe.2020.09.024
Wei, J., Xie, Q., Liu, X., Wan, C., Wu, W., Fang, K., et al. (2020). Identification the prognostic value of glutathione peroxidases expression levels in acute myeloid leukemia. Ann. Transl. Med. 8:678. doi: 10.21037/atm-20-3296
Wei, R., Qiu, H., Xu, J., Mo, J., Liu, Y., Gui, Y., et al. (2020). Expression and prognostic potential of GPX1 in human cancers based on data mining. Ann. Transl. Med. 8:124. doi: 10.21037/atm.2020.02.36
Wen, Q., Liu, J., Kang, R., Zhou, B., and Tang, D. (2019). The release and activity of HMGB1 in ferroptosis. Biochem. Biophys. Res. Commun. 510, 278–283. doi: 10.1016/j.bbrc.2019.01.090
Wolf, P. (1967). The nature and significance of platelet products in human plasma. Br. J. Haematol. 13, 269–288. doi: 10.1111/j.1365-2141.1967.tb08741.x
Wu, C. Y., Du, S. L., Zhang, J., Liang, A. L., and Liu, Y. J. (2017). Exosomes and breast cancer: a comprehensive review of novel therapeutic strategies from diagnosis to treatment. Cancer Gene Ther. 24, 6–12. doi: 10.1038/cgt.2016.69
Wu, H., and Liu, A. (2021). Long non-coding RNA NEAT1 regulates ferroptosis sensitivity in non-small-cell lung cancer. J. Int. Med. Res. 49:300060521996183. doi: 10.1177/0300060521996183
Wu, P., Li, C., Ye, D. M., Yu, K., Li, Y., Tang, H., et al. (2021). Circular RNA circEPSTI1 accelerates cervical cancer progression via miR-375/409-3P/515-5p-SLC7A11 axis. Aging 13, 4663–4673. doi: 10.18632/aging.202518
Wu, Z. Y., Trenner, M., Boon, R. A., Spin, J. M., and Maegdefessel, L. (2020). Long noncoding RNAs in key cellular processes involved in aortic aneurysms. Atherosclerosis 292, 112–118. doi: 10.1016/j.atherosclerosis.2019.11.013
Xian, Z. Y., Hu, B., Wang, T., Cai, J. L., Zeng, J. Y., Zou, Q., et al. (2020). CircABCB10 silencing inhibits the cell ferroptosis and apoptosis by regulating the miR-326/CCL5 axis in rectal cancer. Neoplasma 67, 1063–1073. doi: 10.4149/neo_2020_191024N1084
Xiang, Y., Guo, Z., Zhu, P., Chen, J., and Huang, Y. (2019). Traditional Chinese medicine as a cancer treatment: modern perspectives of ancient but advanced science. Cancer Med. 8, 1958–1975. doi: 10.1002/cam4.2108
Xie, Y., Zhu, S., Song, X., Sun, X., Fan, Y., Liu, J., et al. (2017). The tumor suppressor p53 limits ferroptosis by blocking DPP4 activity. Cell Rep. 20, 1692–1704. doi: 10.1016/j.celrep.2017.07.055
Xu, Q. H., Zhou, L. J., Yang, G. H., Meng, F. D., Wan, Y., Wang, L., et al. (2020a). CircIL4R facilitates the tumorigenesis and inhibits ferroptosis in hepatocellular carcinoma by regulating the miR-541-3p/GPX4 axis. Cell Biol. Int. 44, 2344–2356. doi: 10.1002/cbin.11444
Xu, X., Chen, Y., Zhang, Y., Yao, Y., and Ji, P. (2020b). Highly stable and biocompatible hyaluronic acid-rehabilitated nanoscale MOF-Fe(2+) induced ferroptosis in breast cancer cells. J. Mater. Chem. B 8, 9129–9138. doi: 10.1039/d0tb01616k
Xu, X., Zhang, X., Wei, C., Zheng, D., Lu, X., Yang, Y., et al. (2020c). Targeting SLC7A11 specifically suppresses the progression of colorectal cancer stem cells via inducing ferroptosis. Eur. J. Pharm. Sci. 152:105450. doi: 10.1016/j.ejps.2020.105450
Xu, Y. X. X., Luo, X. X., He, W. G., Chen, G. C., Li, Y. S., Li, W. X., et al. (2018). Long non-coding RNA PVT1/miR-150/HIG2 axis regulates the proliferation, invasion and the balance of iron metabolism of hepatocellular carcinoma. Cell. Physiol. Biochem. 49, 1403–1419. doi: 10.1159/000493445
Xu, Y., Wang, Q., Li, X., Chen, Y., and Xu, G. (2021). Itraconazole attenuates the stemness of nasopharyngeal carcinoma cells via triggering ferroptosis. Environ. Toxicol, 36, 257–266. doi: 10.1002/tox.23031
Yagoda, N., von Rechenberg, M., Zaganjor, E., Bauer, A. J., Yang, W. S., Fridman, D. J., et al. (2007). RAS-RAF-MEK-dependent oxidative cell death involving voltage-dependent anion channels. Nature 447, 864–868. doi: 10.1038/nature05859
Yamazaki, T., Hannani, D., Poirier-Colame, V., Ladoire, S., Locher, C., Sistigu, A., et al. (2014). Defective immunogenic cell death of HMGB1-deficient tumors: compensatory therapy with TLR4 agonists. Cell Death Differ. 21, 69–78. doi: 10.1038/cdd.2013.72
Yang, L., Chen, X., Yang, Q. Q., Chen, J. H., Huang, Q. T., Yao, L. Y., et al. (2020). Broad spectrum deubiquitinase inhibition induces both apoptosis and ferroptosis in cancer cells. Front. Oncol. 10:15. doi: 10.3389/fonc.2020.00949
Yang, T. Z., Martin, P., Fogarty, B., Brown, A., Schurman, K., Phipps, R., et al. (2015). Exosome delivered anticancer drugs across the blood-brain barrier for brain cancer therapy in danio rerio. Pharm. Res. 32, 2003–2014. doi: 10.1007/s11095-014-1593-y
Yang, W. S., and Stockwell, B. R. (2008). Synthetic lethal screening identifies compounds activating iron-dependent, nonapoptotic cell death in oncogenic-RAS-harboring cancer cells. Chem. Biol. 15, 234–245. doi: 10.1016/j.chembiol.2008.02.010
Yang, W. S., and Stockwell, B. R. (2016). Ferrootosis: death by lipid peroxidation. Trends Cell Biol. 26, 165–176. doi: 10.1016/j.tcb.2015.10.014
Yang, W. S., SriRamaratnam, R., Welsch, M. E., Shimada, K., Skouta, R., Viswanathan, V. S., et al. (2014). Regulation of ferroptotic cancer cell death by GPX4. Cell 156, 317–331. doi: 10.1016/j.cell.2013.12.010
Yang, Y. N., Tai, W. L., Lu, N. H., Li, T., Liu, Y. J., Wu, W. J., et al. (2020). lncRNA ZFAS1 promotes lung fibroblast-to-myofibroblast transition and ferroptosis via functioning as a ceRNA through miR-150-5p/SLC38A1 axis. Aging 12, 9085–9102. doi: 10.18632/aging.103176
Ye, F., Chai, W., Xie, M., Yang, M., Yu, Y., Cao, L., et al. (2019). HMGB1 regulates erastin-induced ferroptosis via RAS-JNK/p38 signaling in HL-60/NRAS(Q61L) cells. Am. J. Cancer Res. 9, 730–739.
Ye, Z., Hu, Q., Zhuo, Q., Zhu, Y., Fan, G., Liu, M., et al. (2020). Abrogation of ARF6 promotes RSL3-induced ferroptosis and mitigates gemcitabine resistance in pancreatic cancer cells. Am. J. Cancer Res. 10, 1182–1193.
Yu, M., Gai, C., Li, Z., Ding, D., Zheng, J., Zhang, W., et al. (2019). Targeted exosome-encapsulated erastin induced ferroptosis in triple negative breast cancer cells. Cancer Sci. 110, 3173–3182. doi: 10.1111/cas.14181
Yu, Y., Xie, Y., Cao, L., Yang, L., Yang, M., Lotze, M. T., et al. (2015). The ferroptosis inducer erastin enhances sensitivity of acute myeloid leukemia cells to chemotherapeutic agents. Mol. Cell Oncol. 2:e1054549. doi: 10.1080/23723556.2015.1054549
Zanganeh, S., Hutter, G., Spitler, R., Lenkov, O., Mahmoudi, M., Shaw, A., et al. (2016). Iron oxide nanoparticles inhibit tumour growth by inducing pro-inflammatory macrophage polarization in tumour tissues. Nat. Nanotechnol. 11, 986–994. doi: 10.1038/nnano.2016.168
Zhang, F., Li, F., Lu, G. H., Nie, W., Zhang, L., Lv, Y., et al. (2019). Engineering magnetosomes for ferroptosis/immunomodulation synergism in cancer. ACS Nano 13, 5662–5673. doi: 10.1021/acsnano.9b00892
Zhang, H., Deng, T., Liu, R., Ning, T., Yang, H., Liu, D., et al. (2020a). CAF secreted miR-522 suppresses ferroptosis and promotes acquired chemo-resistance in gastric cancer. Mol. Cancer 19:43. doi: 10.1186/s12943-020-01168-8
Zhang, H. Y., Zhang, B. W., Zhang, Z. B., and Deng, Q. J. (2020b). Circular RNA TTBK2 regulates cell proliferation, invasion and ferroptosis via miR-761/ITGB8 axis in glioma. Eur. Rev. Med. Pharmacol. Sci. 24, 2585–2600. doi: 10.26355/eurrev_202003_20528
Zhang, H., Ge, Z., Wang, Z., Gao, Y., Wang, Y., and Qu, X. (2021). Circular RNA RHOT1 promotes progression and inhibits ferroptosis via mir-106a-5p/STAT3 axis in breast cancer. Aging 13, 8115–8126. doi: 10.18632/aging.202608
Zhang, X., Huang, Z., Xie, Z., Chen, Y., Zheng, Z., Wei, X., et al. (2020c). Homocysteine induces oxidative stress and ferroptosis of nucleus pulposus via enhancing methylation of GPX4. Free Radic. Biol. Med. 160, 552–565. doi: 10.1016/j.freeradbiomed.2020.08.029
Zhang, X., Sui, S., Wang, L., Li, H., Zhang, L., Xu, S., et al. (2020d). Inhibition of tumor propellant glutathione peroxidase 4 induces ferroptosis in cancer cells and enhances anticancer effect of cisplatin. J. Cell Physiol. 235, 3425–3437. doi: 10.1002/jcp.29232
Zhang, X., Wang, L., Li, H., Zhang, L., Zheng, X., and Cheng, W. (2020e). Crosstalk between noncoding RNAs and ferroptosis: new dawn for overcoming cancer progression. Cell Death Dis. 11:580. doi: 10.1038/s41419-020-02772-8
Zhang, Y. L., Koppula, P., and Gan, B. Y. (2019). Regulation of H2A ubiquitination and SLC7A11 expression by BAP1 and PRC1. Cell Cycle 18, 773–783. doi: 10.1080/15384101.2019.1597506
Zhang, Y., Guo, S., Wang, S., Li, X., Hou, D., Li, H., et al. (2021). LncRNA OIP5-AS1 inhibits ferroptosis in prostate cancer with long-term cadmium exposure through miR-128-3p/SLC7A11 signaling. Ecotoxicol. Environ. Saf. 220:112376. doi: 10.1016/j.ecoenv.2021.112376
Zhao, Y. M., Zhao, W., Lim, Y. C., and Liu, T. Q. (2019). Salinomycin-loaded gold nanoparticles for treating cancer stem cells by ferroptosis-induced cell death. Mol. Pharm. 16, 2532–2539. doi: 10.1021/acs.molpharmaceut.9b00132
Zou, C., Zou, C., Cheng, W., Li, Q., Han, Z., Wang, X., et al. (2016). Heme oxygenase-1 retards hepatocellular carcinoma progression through the microRNA pathway. Oncol. Rep. 36, 2715–2722. doi: 10.3892/or.2016.5056
Keywords: ferroptosis, apoptosis, cancer, cell death, exosomes
Citation: Wu S, Li T, Liu W and Huang Y (2021) Ferroptosis and Cancer: Complex Relationship and Potential Application of Exosomes. Front. Cell Dev. Biol. 9:733751. doi: 10.3389/fcell.2021.733751
Received: 30 June 2021; Accepted: 13 August 2021;
Published: 08 September 2021.
Edited by:
Jian-ye Zhang, Guangzhou Medical University, ChinaCopyright © 2021 Wu, Li, Liu and Huang. This is an open-access article distributed under the terms of the Creative Commons Attribution License (CC BY). The use, distribution or reproduction in other forums is permitted, provided the original author(s) and the copyright owner(s) are credited and that the original publication in this journal is cited, in accordance with accepted academic practice. No use, distribution or reproduction is permitted which does not comply with these terms.
*Correspondence: Yongye Huang, aHVhbmd5b25neWU4OEAxNjMuY29t
Disclaimer: All claims expressed in this article are solely those of the authors and do not necessarily represent those of their affiliated organizations, or those of the publisher, the editors and the reviewers. Any product that may be evaluated in this article or claim that may be made by its manufacturer is not guaranteed or endorsed by the publisher.
Research integrity at Frontiers
Learn more about the work of our research integrity team to safeguard the quality of each article we publish.