- Institute of Ophthalmology, Faculty of Brain Sciences, University College London, London, United Kingdom
The neurovascular unit (NVU) is a complex multi-cellular structure consisting of endothelial cells (ECs), neurons, glia, smooth muscle cells (SMCs), and pericytes. Each component is closely linked to each other, establishing a structural and functional unit, regulating central nervous system (CNS) blood flow and energy metabolism as well as forming the blood-brain barrier (BBB) and inner blood-retina barrier (BRB). As the name suggests, the “neuro” and “vascular” components of the NVU are well recognized and neurovascular coupling is the key function of the NVU. However, the NVU consists of multiple cell types and its functionality goes beyond the resulting neurovascular coupling, with cross-component links of signaling, metabolism, and homeostasis. Within the NVU, glia cells have gained increased attention and it is increasingly clear that they fulfill various multi-level functions in the NVU. Glial dysfunctions were shown to precede neuronal and vascular pathologies suggesting central roles for glia in NVU functionality and pathogenesis of disease. In this review, we take a “glio-centric” view on NVU development and function in the retina and brain, how these change in disease, and how advancing experimental techniques will help us address unanswered questions.
Introduction
The brain and retina, which constitute the central nervous system (CNS), are highly complex tissues, requiring high levels of energy for function and tight control for health. To achieve this, they contain a specialized vasculature that controls parenchymal homeostasis, transport of metabolites, and confers, in part, so-called immune privilege (Forrester et al., 2018; Taylor and Ng, 2018). Most importantly, the bi-directional movement of molecules across the blood-tissue barriers is strictly controlled to maintain CNS health and brain function. For many decades the focus of this regulatory capacity lay at the endothelial cell (EC), the predominant cell of the blood-brain barrier (BBB), but more recently the wider neurovascular unit (NVU) has been recognized as providing functionality. The NVU is a complex multi-hetero-cellular structure of EC, neurons, glia, smooth muscle cells (SMCs), pericytes, and extracellular matrix (ECM). Together, these components regulate blood flow and metabolism, thus allowing the controlled exchange of nutrients and metabolic waste products (Hawkins and Davis, 2005; Lok et al., 2007). To meet the high metabolic demand of the CNS, particularly in response to an intensification of physical or mental activity, increased neuronal activity leads to subsequent changes in cerebral blood flow (functional hyperemia), in a process called neurovascular coupling. While neurons can directly regulate this system (McConnell et al., 2017), glial cells are often in direct physical contact with the vasculature and neurons, thus are critically positioned to interface between these cellular components where they may contribute to the relay of information and act as a modulator of such crosstalk. Additionally, NVU components are crucial for brain protection and homeostasis as ECs form the BBB, and glia physically ensheathing the blood vessels are seen as a secondary barrier (Kutuzov et al., 2018). With this close physical interaction between ECs and glia, nutrients required for CNS function are delivered from the blood vessels to neurons mainly via glia cells (Hurley et al., 2015), while waste compounds are passed via glial cell to microglia or back into the bloodstream (Marina et al., 2018). Dysfunction of the NVU is characterized by dysregulation of neurovascular coupling, neuron death, gliosis, microglia activation, mural cell transmigration, and BBB breakdown (Zlokovic, 2005; Willis, 2011). Dysfunction of the BBB is associated with increased vascular leakage, transcellular transport, immune cell infiltration, and reduction of intercellular junctions (Figure 1). Accordingly, glial cells and other NVU cells work closely together to maintain CNS function and maintenance.
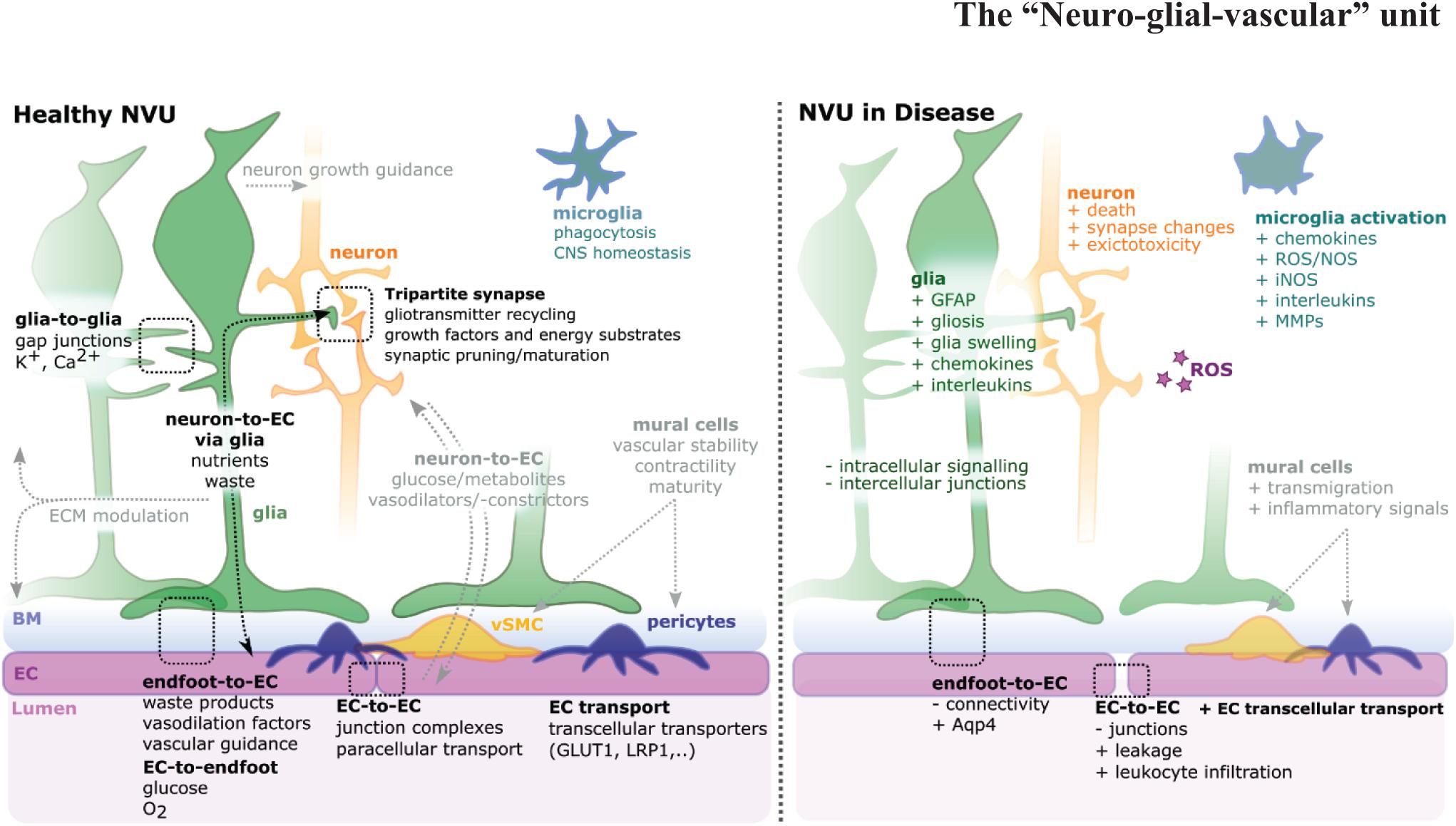
Figure 1. Schematic of the neurovascular unit (NVU) in health and disease. The NVU is a hetero-cellular complex formed by glia, neurons, vSMCs, pericytes, microglia, and blood vessels, that form the blood-brain barrier (BBB) and blood retina barrier (BRB). Glia cells (green) impact neurons (orange), endothelial cells (ECs) (magenta), and each other. For NVU functionality, various direct (i.e., glia-to-glia, tripartite synapse, endfoot-to-EC, EC-to-endfoot) and indirect (i.e., neuron-to-EC via glia, neuron-to-EC via mural cells, microglia) pathways need to be considered. Upon disease multi-level changes are observed, including altered cell shapes, function, and interactions [see also (Lécuyer et al., 2016; Jha et al., 2018)]. NVU component changes include gliosis, neuron death, EC-connectivity changes, mural cell transmigration, and microglia activation.
In this review, we discuss glia cell types and their role in the NVU, by examining glia specializations to support neurons, the vasculature, and neuro-vascular interactions in the NVU. Lastly, we will highlight how rapidly improving techniques and tools will help us answer pressing outstanding questions in the field. While this review is not meant to be an exhaustive list of the many types of glia within the CNS, we aim to highlight their importance for the development, function, and dysfunction of the NVU in the brain and retina.
Components of the Neurovascular Unit
The complex interaction between NVU cells requires each cellular component to operate in a complex and coordinated manner to ensure homeostatic control of the BBB and blood retina barrier (BRB). Each component exhibits specialized features that are critical to the overall maintenance of NVU function (Figure 1). Briefly, ECs form a single-layer lining of tubular blood vessels, which are specialized depending on the vascular bed in which they are situated (Chico and Kugler, 2021). At the BBB/BRB, ECs exhibit reduced pinocytosis/transcytosis (O’Brown et al., 2019; Villaseñor et al., 2019), increased expression of tight junction molecules, such as claudins, occludin, or zonula occludens 1 (ZO-1; Figure 2A; Fanning et al., 1999; Nitta et al., 2003), and exclude free transport of substances over 400kDa (Pardridge, 2001). Mural cells, which constitute pericytes and vascular smooth muscle cells (vSMCs), are positioned in the basement membrane shared with ECs, maintain vascular stability, provide structural support for blood vessels, govern vasodilation/constriction (Tong et al., 2021), as well as contribute to NVU function by BBB maintenance (Armulik et al., 2010; Bell et al., 2010; Henshall et al., 2015). Neurons in the NVU transduce signals and control local cerebral blood flow directly, such as via nitric oxide (NO), as well as indirectly via glia cells, such as via arachnoid acid or potassium (K+) (Attwell et al., 2010). Additionally, neuronal activity itself shapes vascular and BBB formation via levels of neurotransmitter release (Lacoste et al., 2014; Whiteus et al., 2014). Glial cells physically ensheath blood vessels with their endfeet, creating the semi-permeable glia limitans (Kutuzov et al., 2018). Importantly, glia physically connect vessels to neurons (Zonta et al., 2003), modulate neurotransmission, and impact neurogenesis (Argente-Arizón et al., 2017; Falk and Götz, 2017). Microglia, macrophages, and perivascular macrophages (PVM) play roles in phagocytosis and the CNS inflammatory response, ensuring CNS maintenance and health (Guillemin and Brew, 2004; Serrats et al., 2010). The vascular basement membrane, which encompasses blood vessels, acts as a passage for fluid transport (Morris et al., 2016), while the perivascular basal laminae and ECM molecules support the glio-vascular interface (Hoddevik et al., 2020). Together, these components form a spatially and functionally integrated NVU with bidirectional communication, namely neuro-vascular-coupling and vascular-neuro-coupling. However, the precise mechanisms of the diverse roles of glial cells in NVU form, maintenance and function remain unclear. Answering these fundamental questions will be of particular importance as the NVUs’ main role is considered neurovascular coupling, but indeed various other aspects such as integration of signaling, metabolism, and homeostasis occur across NVU components and thus must also be considered.
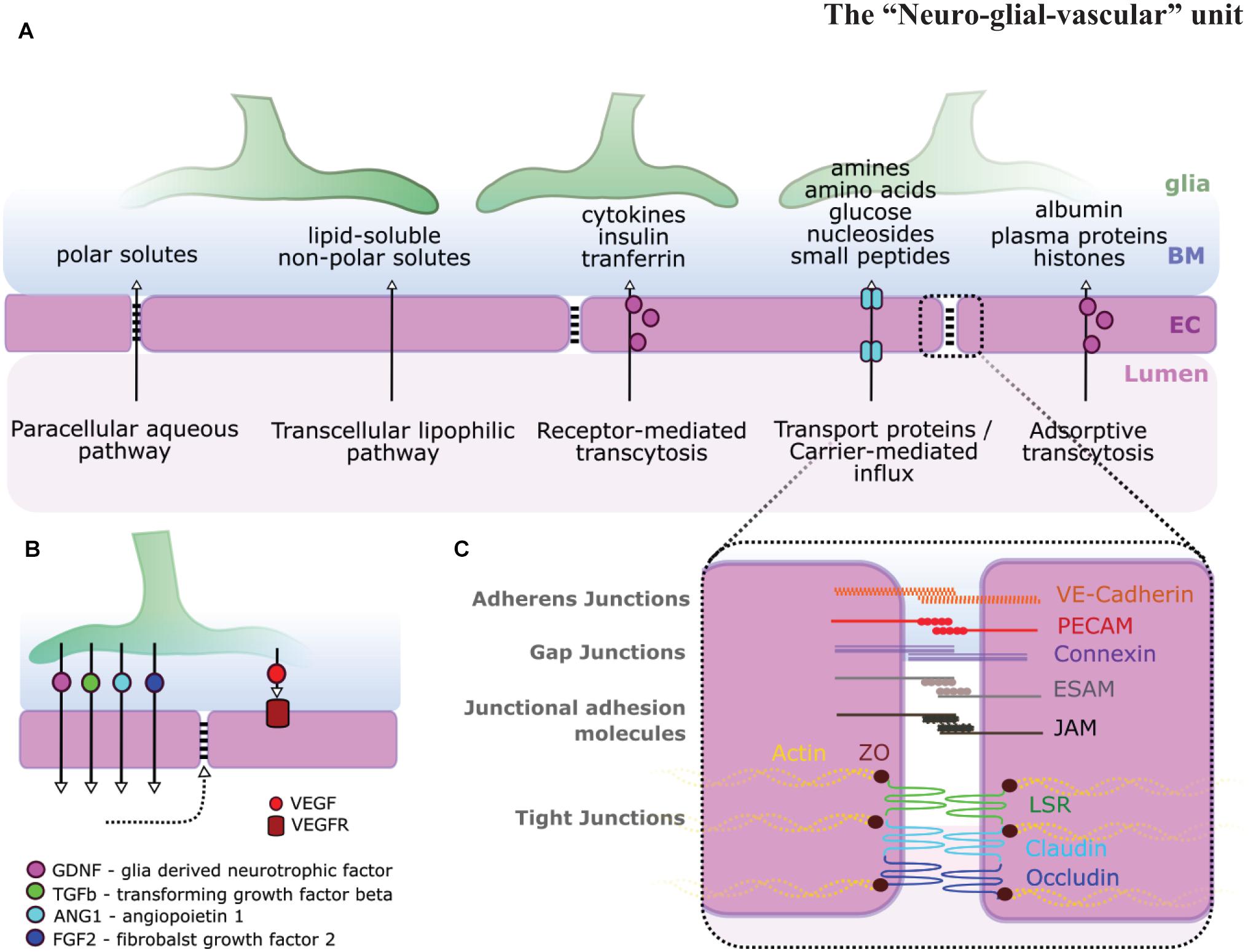
Figure 2. Glia-endothelial interactions. (A) Pathways across the BBB and BRB allow for the transport of various types of molecules. (B) Glial signaling impacts ECs [i.e., glial-derived neurotrophic factor (GDNF), transforming growth factor β (TGF-β), Ang1, fibroblast growth factor 2 (FGF2), and vascular endothelial growth factor (VEGF)] and in turn BBB stability (dotted arrow). (C) BBB stability is highly dependent on EC inter-cellular junction integrity including adherens junctions, gap junctions, junctional adhesion molecules, and tight junctions adapted from Abbott et al. (2006); Malik and Di Benedetto (2018).
Overview of Glial Cells Types and the Neurovascular Unit
Glia were originally described as scaffolds providing structural support and maintaining biophysical integrity (Virchow, 1856), making their role in supporting the structure and biophysical integrity of the CNS their most widely described function (Losada-Perez, 2018). However, glia are now being increasingly appreciated for their many other functional and regulatory roles, such as neurotransmission, BBB function, and controlling immunity (Table 1). To fulfill the variety of specialized functions required, glia cells are highly specialized according to each CNS region with respect to proteomic signatures, electrophysiology, Ca2+ signaling, morphology, and proximities to synapses (Tsai et al., 2012; Molofsky et al., 2014; Ben Haim and Rowitch, 2017; Chai et al., 2017). Moreover, even within morphological groups, such as astrocytes, there is heterogeneity of cell types and their pathological responses (John Lin et al., 2017; Hasel et al., 2021) that may also reflect regional differences in the structure and function of the NVU.
The most abundant and widespread glial cell type in the brain are astrocytes. Astrocytes are fivefold more numerous than neurons (Sofroniew and Vinters, 2010), with individual astrocytes contacting up to two million neuron synapses with elaborate morphologies (Oberheim et al., 2009). This high spatial correspondence between astrocytes and neurons is accompanied by astrocytes regulating neuron health by controlling neurotransmitters, such as glutamate or adenosine, as well as maintaining hydromineral brain homeostasis, such as Ca2+, Cl–, or water (Simard and Nedergaard, 2004; Keaney and Campbell, 2015; Price et al., 2018). In addition to contacting neurons, astrocytes also contact blood vessels, affecting local blood flow by regulating blood vessel diameters by vasoconstriction (e.g., by arachnoid acid) and vasodilation (e.g., by prostaglandins) (Kimelberg, 2010). Astrocytes also physically and functionally contribute to the BBB and its permeability for factors such as molecular traffic of glucose or proteins (Abbott et al., 2006). Thus, astrocytes are central to the function of a healthy functioning NVU, and overall CNS function.
In the retina, the principal glial cell type are Müller glia (MG), which contact blood vessels and neurons, fulfilling similar functions as astrocytes in the brain (Newman and Reichenbach, 1996; Subirada et al., 2018). The structure, morphology, and species-specific differences of retinal MGs are well described (Cajal, 1995) and MG exhibit at least five apico-basal domains that stretch from the apical stem around photoreceptors to the basal endfoot in the inner limiting membrane (Wang et al., 2017). However, MG are not just highly organized apico-basally, but also laterally to interact with other cells; this intercalation between cells is in a so-called tiled fashion, thereby contacting almost all cells within the retina (MacDonald et al., 2017; Wang et al., 2017). The retina is protected by two separate components of the BRB. The outer (oBRB) consists of retinal pigment epithelium and the inner (iBRB) is located at the level of the retinal capillaries. The latter is established by MGs and pericytes, with dysfunction being implicated in several retinal diseases such as diabetic retinopathy (DR; Cunha-Vaz et al., 2011; Frey and Antonetti, 2011; Díaz-Coránguez et al., 2017; Park et al., 2017). In the mammalian retina, there are also astrocytes that contact blood vessels and are pivotal as a structural growth template during angiogenesis, mainly via vascular endothelial growth factor (VEGF) and Hypoxia-inducible factor (HIF) pathways, following ganglion cell templates (O’Sullivan et al., 2017; Paisley and Kay, 2021). This suggests that MG and astrocytes may work together in the mammalian retina to influence the development of the NVU as well as to regulate its function. In humans, the retinal astrocytes are limited to the inner vascular plexus, while MG contact both plexi and are likely to induce the BRB functionality/maturity in the deep plexus, raising the likelihood for differential coupling between astrocytes and MG in the healthy and potentially diseased retina (Tout et al., 1993; Fruttiger, 2007; Ashraf et al., 2020). However, MG are the only glia found in the fovea, which is free of astrocytes, microglia, and vascular EC, suggesting that in some regions of the retina MG are sufficient to solely meet the functional needs required for high acuity vision (Reichenbach and Bringmann, 2020).
Besides the principal glial cells in the brain and retina (i.e., astrocytes and MG), other glial cells exist to fulfill crucial functions in the CNS. During development, progenitors, so-called radial glia, will divide to generate neurons and glia (Rakic, 2003), while also making contact with the vasculature where they contribute to the regulation of CNS angiogenesis. In certain CNS regions, radial glia persist into adulthood as stem cells, contributing to BBB maintenance via retinoic acid signaling (Sharif et al., 2018). Microglia are the primary immune cells in the CNS, surveying their environment and responding to insult, fulfilling roles in phagocytosis and inflammation where they express both pro-inflammatory (e.g., IL-1β) and anti-inflammatory (e.g., IL-10) molecules, with subsequent upregulation of factors such as Glial Fibrillary Acidic Protein (GFAP). Additionally, microglia-to-astrocyte crosstalk in response to glutamate plays a role in neuro-immune-interactions (Nimmerjahn et al., 2005; Macht, 2016), and microglia are required for normal neurogenesis, mostly by nerve growth factor (NGF) and tumor necrosis factor (TNF; Matejuk and Ransohoff, 2020). Another type of glia, called ependymal cells, line the brain ventricles, producing cerebrospinal fluid, and subpopulations acting as progenitors for astrocytes and oligodendrocytes (Bigio, 2010; Furube et al., 2020). Thus, there are several types of glia found within each CNS tissue, yet several pressing questions remain to understand the importance of glia in the NVU. These include what role do glial cells play in driving NVU formation during development, how are glial cells directed to contact and support multi-cellular units within the NVU, and what are the consequences of dysfunction of these glial components on NVU function.
Glial Support for Neurons in the Neurovascular Unit
The fact that glia are a structurally integral part of the NVU physically linking the vasculature and neurons, emphasizes their functionality in BBB/BRB formation and CNS development.
Glia cells provide structural support to neuronal tissues for anisotropic mechanical tension (Nagashima et al., 2017), and loss of MG in the retina results in tearing of the tissue due to the loss of their biophysical support (MacDonald et al., 2015). Another biophysical role of glia is that they can swell, which subsequently affects the NVU by spatial changes. The impact of glia volume changes on neurons is facilitated and relayed by the fact that glia ensheath pre- and post-synaptic terminals of neurons to form the “tripartite synapse” (Araque et al., 1999; Santello et al., 2012; Hillen et al., 2018). Due to this physical proximity, changes in glia cell size can modulate the extracellular space and subsequently neuron excitability (Florence et al., 2012; Vecino et al., 2016). This is achieved by the synergistic activity of Aquaporin 4 (Aqp4), a channel protein, which is needed for water transport and is enriched in astrocytic endfeet (Gleiser et al., 2016), as well as the transient receptor potential cation channel TRPV4 for Ca2+ influx (Jo et al., 2015). However, even though Aqp4 and TRPV4 are considered main factors, glia swelling is a complex process and, depending on the context, other factors were shown to play a role in glia volume changes. These include K+ ion transport via connexin 43, Kir 4.1, or Na+/K+-ATPase, and ion flux via Na+-K+-Cl– co-transporter (NKCC1), or glutamate movement via specialized transporters (Lafrenaye and Simard, 2019). These factors can also change in disease or upon injury, as exemplified by sulfonylurea receptor 1 – transient receptor potential melastatin 4 (SUR1-TRPM4) which is upregulated in CNS injury (Mehta et al., 2015), but the impact of such changes on NVU function remain poorly defined.
Besides this physical link, glia also functionally link NVU components, exemplified by their impact on EC junctions, transporters, and pathways (Figure 1; Hayashi et al., 1997; McAllister et al., 2001; Haseloff et al., 2005). This functional link is in part achieved by factors such as glial cell line-derived neurotrophic factor (GDNF), VEGF, fibroblast growth factor 2 (FGF2), or angiopoietin-1 (Figures 2B,C; Igarashi et al., 1999; Lee et al., 2003; Lécuyer et al., 2016; Blanco-Suarez et al., 2018; Jha et al., 2018). But glia cells are also key to supporting neurotransmission by removal of neurotransmitters to terminate synaptic transmission and reestablish neuronal excitability, thus avoiding toxic overstimulation, called excitotoxicity (Turner and Adamson, 2011). Glia also directly regulate neuronal activity within the synapse (Pannasch and Rouach, 2013; Sibille et al., 2014) and synchronize/modulate synaptic inputs (Fellin et al., 2004; Fellin, 2009) on the level of signaling via gliotransmitters, such as gamma-aminobutyric acid (GABA), glutamate, or cytokines (Kim et al., 2020). The major neurotransmitters are the excitatory glutamate and the inhibitory GABA, working together to regulate CNS function. Following the removal of neurotransmitters from the synaptic cleft, glia cells transfer these neurotransmitters back to neurons in a process called the glutamate-glutamine cycle that requires ammonia (NH3) and ammonium ion (NH4+) derived from NVU blood vessels (Bak et al., 2006; Limón et al., 2021), thus reestablishing functional neuron neurotransmitter pools. This maintenance of synaptic potentials comes at a very high metabolic cost with the energy for this provided by astrocytes and blood vessels (Vergara et al., 2019). While it was previously assumed that both glutamate and GABAergic neurons are under astrocytic control, a recent in vitro study challenges this, suggesting that GABAergic neurons establish functional synaptic transmission without glia (Turko et al., 2019). Another neuromodulator released from neurons or glia (Butt, 2011), impacting neuronal function, is adenosine derived from adenosine triphosphate (ATP) breakdown. Adenosine stimulates receptors that regulate the release of GABA, glutamate, acetylcholine, noradrenaline, 5-HT, and dopamine (Sperlágh and Vizi, 2011).
Thus, glia are specialized morphologically, biophysically, and molecularly to support and regulate the NVU. However, the multitude of glial functions within the NVU makes it challenging to discern which mechanisms are necessary and sufficient for NVU form and function. To understand the role of glia cells, further studies are needed where glia cells are disrupted (i.e., lacking, inhibited, or overactive). Such studies will allow us to disentangle NVU interactions, establish the exact role of glia cells within it and the pathophysiological consequences.
Glia Cells and the Neurovascular Unit Vasculature: Angiogenesis and Regulation of Blood Flow
As glia directly contact and ensheath blood vessels, they directly influence EC structure and function rather than passively co-exist. Indeed, in the last decade, it has become clear that glial cells play an active role in facilitating vascular angiogenesis via expression of factors, such as VEGF or transforming growth factor 1β (TGF-1β) in radial glia cells (Virgintino et al., 2003; Welser et al., 2010; Biswas et al., 2017; Siqueira et al., 2018). Moreover, radial glia were shown to stabilize murine nascent cortex vessels via inhibition of Wnt signaling and proliferation in EC in a contact- and age-dependent manner, a process which is potentially mediated by MMP-2 (Ma et al., 2013). Glia cells, specifically CNS-specific macrophages (microglia), were also shown to play pivotal roles in the fusion of blood vessels, called anastomosis. This is achieved by Notch1-expressing macrophages that link path-seeking dll4-expressing vascular tip cells (Outtz et al., 2011) as well as by acting as physical chaperones which express TIE2 and NRP1, regulating anastomosis downstream of VEGF-mediated endothelial tip cell induction (Fantin et al., 2010). After vessel- and NVU-formation, glia are also pivotal for BBB maturity, via factors such as retinoic acid supplied by radial glial cells, which increases BBB stability and the expression of BBB-specific genes such as p-glycoprotein (P-gp), occludin, and Glut-1 (Mizee et al., 2013) [see details for BBB transport systems and junctions (Zhao et al., 2015)]. Similarly, astrocytic Src suppressed C kinase substrate (SSeCKS) reduces VEGF and increases EC tight junctions (Lee et al., 2003), or astrocytic angiotensin-converting enzyme-1 (ACE-1), which produces angiotensin II that facilitates BBB maturation and junction protein stabilization (Lavoie and Sigmund, 2003; Wosik et al., 2007). ECs and glia interact bidirectionally in NVU development. Firstly, radial glia support EC maturation toward decreased proliferation, reduced tip cell marker DLL4, and reduced vascular permeability, thus supporting BBB maturation. Subsequently, ECs increase GFAP-positivity in radial glia in a VEGF-A dependent manner, leading to astrocyte differentiation and NVU formation (da Silva et al., 2019). In addition to these molecular impacts, the migration patterns of blood vessels, astrocytes, and neurons are closely associated with each other like scaffolds, with astrocytes providing VEGF for EC migration and, vice versa, ECs in turn provide oxygen for astrocyte differentiation (Bozoyan et al., 2012; Duan et al., 2017; O’Sullivan et al., 2017). Critically, in pathology, lactate-stimulated MG express G-protein–coupled receptor 81 (GPR81), which triggers neovascularization via pathways such as Wnt or Norrin (Madaan et al., 2019).
Besides these roles in angiogenesis, glia are also pivotal in regulating blood flow via regulating NVU synaptic activity as well as by releasing factors such as calcium, NO, arachidonic acid, and prostaglandins (Gordon et al., 2007; Attwell et al., 2010; Biesecker et al., 2016; Magaki et al., 2018). It was also shown that angiotensinogen-to-angiotensin II cleavage occurs in glia, with angiotensin I receptor (AT1-R) causing vasoconstriction (Kawamura et al., 2004), while AT2-R causing vasodilation (Fletcher et al., 2010). Moreover, glia contribute to vasodilation indirectly by interaction with other NVU components such as pericytes, which then, in turn, impacts the vasodilatory state (Nortley and Attwell, 2017). Here, one important molecule is calcium, with calcium signaling not only being coordinated between glia cells (Muñoz et al., 2015), but also ECs (Thakore et al., 2021). It remains to be understood to which extent glia-EC signaling is indirectly (via neurons or pericytes) or directly coupled. Lastly, neuron-derived NO regulates glia-mediated vasodilation via prostanoids and epoxyeicosatrienoic acids (Someya et al., 2019). Together, glial cells therefore not only play a role in angiogenesis, anastomosis, EC maturation, and blood flow regulation, with glia dysfunction potentially leading to BBB breakdown, pathological vascularization, dysregulation of vasoregulation and failure to deliver sufficient oxygenation.
Reciprocal Neuronal-To-Vascular Transport via Glia
As the CNS has high metabolic demands and lacks a carbohydrate storage system, the NVU is critical to serving the retina and brain metabolic needs as glucose has to be continuously supplied via the blood to meet the constant CNS energy demand. This is particularly crucial as neurons rely on oxidative metabolism, making them sensitive to changes in levels of oxygen and potentially ischemia (Turner and Adamson, 2011); on the other hand, astrocytes rely on glycolytic metabolism, and glucose can be stored in them in the form of glycogen, rendering them central players in NVU and CNS metabolism (Öz et al., 2007). Crucially, pyruvate carboxylase, an enzyme that is key to synthesizing the neurotransmitter glutamate from glucose via the anaplerotic pathway, is almost exclusively expressed in astrocytes, positioning them as essential producers of the neurotransmitters GABA and glutamate (Schousboe et al., 2019). Recently it was shown that retinal MG also conduct anaplerosis (Singh et al., 2020), suggesting that MG support retinal NVU metabolism similar to astrocytes in the brain.
While the majority of vascular-to-neuron glucose metabolism occurs directly via glia in the NVU, a minor proportion of the glucose flux happens directly between blood vessels and neurons (Maoz et al., 2018). At the end of glycolysis, lactate and pyruvate are produced, but instead of being merely “end-products” they are utilized further to generate energy. Indeed, lactate is taken up by neurons and is metabolized in preference to glucose when both are available (Bouzier-Sore et al., 2003; Bouzier-Sore et al., 2006). Once transported into the neuron, lactate is converted to pyruvate and used for ATP production. Thus, glia not only provide compounds for neurons but also complement them in their metabolic requirements. Besides glucose metabolism, astrocytes are also crucial for fatty acid oxidation (FAO) to generate ATP, and catabolic ketogenesis to generate ketone bodies for neuronal metabolism; with ketone bodies or lactate being used by neurons as an energy substrate to produce ATP (Bélanger and Magistretti, 2009; Souza et al., 2019). Importantly, neurons produce toxic fatty acids that are endocytosed by NVU astrocytes for cytoprotection and CNS health (Ioannou et al., 2019). Metabolically, astrocytes also play a critical role in L-serine de novo synthesis, which is converted to D-serine in neurons, acting as a co-agonist of N-methyl-D-aspartate (NMDA) receptors (Yamasaki et al., 2001). Together, these data demonstrate that glial cells play a key role in maintaining the homeostatic status of the metabolism of neurotransmitters, glucose, FAO, L-serine, as well as NO, essential for maintaining normal function of the NVU. Any disturbance of this fine-tuned balance, therefore, such as would occur in diseases as diverse as stroke and diabetes, will influence NVU function resulting in further failure to supply adequate essential substrates for normal neuronal function.
In addition to the above metabolic coupling, neuron-to-vascular coupling is achieved by NO, a gaseous neurotransmitter acting as a vasodilator that is needed for neurovascular coupling and regulating the vasodilatory vascular response, called functional hyperemia (Hoiland et al., 2020). In hyperemia, glia cells are essential in relaying either vasodilation or vasoconstriction depending on the available NO concentration (Metea and Newman, 2007). Further to physiological NO, glia-mediated NO and gliosis-related production of reactive oxygen (ROS) or nitrogen species (RNS), play a role in nitro-oxidative stress such as in neuroinflammation and disease such as epileptogenesis. Indeed, Sharma et al. suggest that epilepsy is preceded by a cascade of reactive gliosis, ROS/RNS, inflammatory cytokines, and neurodegeneration (Sharma et al., 2019). This neuro-vascular metabolic coupling of glucose, fatty acid, L-serine, and NO via glia is dependent on glia directly contacting ECs within the NVU. The required spatial connections between endfeet and ECs are partially achieved via connexins, which form intercellular gap junction channels and hemichannels that are expressed in ECs and astrocyte endfeet, as well as are associated with BBB maturation (Zhao et al., 2018). Also, Pannexins (Panx) that resemble connexin-based hemichannels, are thought to play a role in vasculo-neuro coupling although there is still a debate on their function in CNS development and health (Giaume et al., 2020).
Glial Cells and the Neurovascular Unit in Disease – Pathogenesis, and Dysfunction
Beyond their roles in physiological conditions, glia and microglia contribute to neuroinflammation in response to injury, stroke, or other neurological diseases (Cekanaviciute and Buckwalter, 2016). They act as primary initiators of the inflammation cascade by increased reactivity and the secretion of factors such as chemokines (Sofroniew and Vinters, 2010; Karve et al., 2016). One ubiquitous biomarker of so-called glial activation is increased expression of GFAP (Diaz-Arrastia et al., 2013). Despite the extensive list of definitions for different glia cell states, the field agrees that inactive and reactive glia cells display changes in molecular profiles, including alterations in the cytoskeleton, metabolism, chaperones, secreted proteins, signaling proteins, and transporters. These molecular changes are accompanied by morphological transformation in cellular phenotype, such as hypertrophy (Escartin et al., 2021), which may impact the ability of glial cells to provide multiple support functions for each NVU component(s) and hence its uncoupling.
Besides the physiological functions of glial cells in inflammation, their roles in pathological settings are of wider interest. For example, increasing evidence also shows glia as a link between vascular and neurological contributions in cognitive impairment, Alzheimer’s Disease (AD), and seizures (Burda and Sofroniew, 2014; Edison et al., 2018; Price et al., 2018; Diaz Verdugo et al., 2019). Neuropathologically, AD is characterized by intracellular neurofibrillary tangles and brain parenchymal amyloid β-peptide (Aβ) deposits. The latter form neuritic plaques and cerebral amyloid angiopathy, leading to angiopathy and NVU dysregulation (Soto-Rojas et al., 2021). Astrocytes have been shown to degrade amyloid-beta in an apolipoprotein E (APOE)-dependent manner, a process that could be impaired in AD (Koistinaho et al., 2004), leading to plaque formation. Neurodegenerative disorders are complex conditions with multiple underlying causes and the role of glia has not been fully elucidated (Gleichman and Carmichael, 2020). Nevertheless, several astrocyte/glia risk factor genes, such as APOE, particularly the E4 isoform (Pihlstrøm et al., 2018) or Clusterin (CLU) and FERM Domain Containing Kindlin 2 (FERMT2) (Verheijen and Sleegers, 2018), have been identified for AD. Also, it is generally acknowledged that AD involves inflammatory responses, initiated, or mediated via microglia and astrocytes that lead to BBB breakdown (Akiyama et al., 2000; Nagele et al., 2004). Throughout AD disease progression, different aspects were found to affect the NVU. In pre-senile AD, increased proliferation (Ki-67), gliosis (GFAP), and vascular changes, but not neurogenesis were shown (Boekhoorn et al., 2006). In late-onset AD, vascular dysregulation and BBB breakdown are considered as the earliest biomarker (Iturria-Medina et al., 2016; Sweeney et al., 2018), with vascular dysregulation preceding changes in amyloid beta deposition, metabolism, function, structure, and memory. However, future work is needed to link genetic and mechanistic causes of AD and the spatiotemporal impacts on individual NVU components and NVU function.
In the retina, understanding the NVU and glia contribution is of particular interest as NVU dysfunction can precede neural dysfunction in patient retinas, such as in patients with Type 1 Diabetes who exhibit DR (Lasta et al., 2013). In DR and other retinal diseases, inflammation and accompanying side effects are critical contributors to disease progression and vision loss. Crucially, it has been shown that MG provide VEGF, which contributes to the upregulation of inflammatory markers, such as ICAM1 and TNFα (Le, 2017). These pro-inflammatory compounds induce pathological vascular leakage and retinal neovascularization, making anti-VEGF therapies an important therapeutic strategy in DR. In DR, it was shown that the MGs that express VEGF display a distinct morphology (Pierce et al., 1995), suggesting that they enter a reactive phenotype with altered function before expressing VEGF, which in turn leads to changes in NVU and vascular function. Besides morphological changes and increased VEGF expression, MG also show altered expression of trophic factors in DR, such as NGF, brain-derived neurotrophic factor (BDNF), neurotrophin-3 (NT-3), neurotrophin-4 (NT-4), ciliary neurotrophic factor (CNTF), or glial cell line-derived neurotrophic factor (GDNF), as well as inflammatory factors such as interleukin 1β (IL-1β), IL-6, IL-8, and TNF-α. Together, these factors contribute to disease progression and neuron survival (Boss et al., 2017). Indeed, in diabetes, it is likely that such changes result in early NVU dysfunction and subsequent failure of neurovascular coupling and hence an insufficient supply of nutrients, which then cause further damage to the NVU. Another retinal disease with clear implications of MG dysfunction is macular telangiectasia 2 which causes loss of central vision due to vascular defects (MacTel 2) (Powner et al., 2010a,b). Critically, it was shown that loss of MG matches the area of macular pigment depletion in MacTel 2 patients (Powner et al., 2010b). Moreover, the loss of these MG impacts on the NVU as a secondary hallmark feature of MacTel 2 is the formation of dysfunctional telangiectatic vessels. One potentially crucial aspect when thinking about the regional restriction of MG loss and vascular defects is that macular MG rely more on serine biosynthesis than peripheral MG (Zhang T. et al., 2019), with serine synthesis disruption resulting in mitochondria dysfunction and oxidative stress and ultimately retinal pathology (Zhang et al., 2018). This retinal pathology is also associated with swelling-induced MG volume changes (Lafrenaye and Simard, 2019) and penetration of astrocytes into deeper layers (Coffey et al., 2007). Thus, rather than having a passive role in pathogenesis, the activation state of glia, together with changes in their morphology and molecular expression, is directly associated with disease progression of diseases such as DR and MacTel 2.
Finally, it is important to note that retinal demand for oxygen is even greater than that of the brain. This is especially so during dark adaptation when metabolic activity and oxygen demand is high. The configuration of the vascular supply to the retina means that during high demand the functional reserve of oxygen is minimal, even when neurovascular coupling maximizes functional hyperemia. This makes the retina extremely vulnerable to hypoxic damage and so even small alterations to the function of any component of the NVU, including MG, is likely to result in compromised retinal function.
Future Directions and Areas of Scientific Interest
Combining the Strengths of Models
As the NVU is a heterologous structure formed by different cell types, it is crucial to study the NVU, and the role of glial cells in it, in various models to understand how those cells integrate to form a functional NVU. Despite our increased understanding of NVU and BBB formation, our insight into the exact processes that glial cells regulate in NVU formation and function is still far from complete. This is emphasized by the fact that in vivo studies are still limited in experimental scope and flexibility.
As an in vivo model, rodents are an invaluable pre-clinical asset to study glia and their role in the NVU. However, caution has to be paid when wanting to draw direct conclusions from pre-clinical models. For example rodent glial cells are smaller and less complex than human glial cells, with human astrocytes being 2.6-fold larger, 10-fold more GFAP-positive primary processes, and relaying signals faster (Oberheim et al., 2009), suggesting that NVU metabolism and signaling dynamics are different between rodents and human. Similarly, certain glial cells are primate-specific, such as interlaminar astrocytes, and can therefore not be studied in rodents (Colombo et al., 1995; Colombo and Reisin, 2004). To complement NVU and glia studies in rodents, zebrafish have also been invaluable, particularly as NVU function and development can be studied in real-time in vivo and throughout the CNS (Gestri et al., 2012; Richardson et al., 2017; Angueyra and Kindt, 2018). Zebrafish characteristics, such as ex utero development, genetic tractability, and embryonic transparency render them a crucial asset (MacDonald et al., 2017; Richardson et al., 2017). Crucially, the site of the BBB is conserved in zebrafish and humans in capillary ECs (O’Brown et al., 2018). However, even though neurovascular coupling was shown to be conserved, it remains to be answered to which extent the NVU is truly conserved across species (Chhabria et al., 2018). Again, glia in zebrafish and mammals show differences, exemplified by the lack of typical stellate astrocytes in zebrafish (Grupp et al., 2010) as well as the fact that progenitor cells are widely maintained in the zebrafish adult CNS while being mostly transient in mammals (Than-Trong and Bally-Cuif, 2015). However, zebrafish are being increasingly used as an experimental model to contribute to the understanding of astrocyte development and function (Mu et al., 2019; Chen et al., 2020; Muñoz-Ballester et al., 2021). Importantly, zebrafish radial glia are capable of adult neurogenesis and harbor a very high regenerative capacity, making zebrafish a suitable model to study de- and re-generation (Thummel et al., 2008; Kroehne et al., 2011; Gemberling et al., 2013; Goldman, 2014; Powell et al., 2016). In vitro, reductionist models have also been invaluable in providing insights to study glia and their role in the NVU. However, glial cultures, originally established by dissociation and plating of brain homogenates (Booher and Sensenbrenner, 1972; McCarthy and de Vellis, 1980), lose their structural context, rendering the information obtained of limited relevance. Similarly, cultured MGs were previously shown to de-differentiate (Hauck et al., 2003; Otteson and Phillips, 2010) casting doubt on the translatability of the data to in vivo settings. Advancements in establishing 3D cell cultures, however, have allowed for more physiological insights into glia biology (Haycock, 2011; Watson et al., 2017). This is especially the case when considering recent NVU studies in organoids (Nzou et al., 2020) and organ-on-a-chip (Maoz et al., 2018) models. Still, in comparison to in vivo models, in vitro studies are often considered to provide limited insight into their tissue context and developmental processes only recapitulating single-timepoint and -context information (Duke et al., 2004; Helms et al., 2016).
Manipulating the Composition and Function of the Neurovascular Unit
Our morphological and functional understanding of the NVU, through examining the molecular composition and regional specifications of the different cell types in the CNS, has also advanced significantly. This has allowed us to begin to understand the molecular profiles of cellular components of the NVU as well as their specialization and conservation across species (Cahoy et al., 2008; Roesch et al., 2008; Zhang et al., 2014; Vanlandewijck et al., 2018; Ross et al., 2020). One aspect of particular interest is to examine glia-to-EC contacts, whether this is impacted by regional specialization, and whether this and NVU function, is influenced by glia/EC identities. Understanding such specializations will provide novel insights into the integration of CNS barriers provided by the ECs, basal lamina, and glia limitans, as their specialization might cause them to respond differently to stimulation, injury, or disease and how it affects the NVU. Regional specializations and barrier properties might also elucidate new routes for drug delivery. Thus, to understand how NVU components function as a unit, it is also crucial to establish the contribution of the individual components and how different NVU cell types combine spatially and functionally together. While the latter can be achieved by careful observation, the former usually requires system changes, ideally cell-specific, to be introduced (e.g., removal, over-expression, inhibition of proteins or cells, etc.). This is exemplified by a recent study that used tamoxifen-inducible astrocyte ablation in mice, showing that astrocytes are key to maintaining the integrity of the BBB as loss of astrocytes coincided with vascular leakage and decreased EC ZO-1 expression (Heithoff et al., 2021) and BRB (Puñal et al., 2019), thus confirming early work undertaken over three decades ago (Janzer and Raff, 1987). This BBB alteration could not be rescued by other cells. Moreover, these alterations were accompanied by non-proliferative astrogliosis which, over time, limited vascular leakage (Heithoff et al., 2021). In addition, functional imaging is allowing the unraveling of NVU functions such as neuronal activity using dynamic calcium imaging (Chhabria et al., 2018) and cellular relationships following metabolic compound exchanges (Kanow et al., 2017). Similarly, optokinetic response measurements are increasingly used to measure visual acuity (Dietrich et al., 2019; Sugita et al., 2020). Linked to performing functional imaging, understanding metabolic fluxes, storage, and turnover is crucial in beginning to understand how NVU components communicate and support each other’s function and how they may be disturbed in disease. This understanding of metabolic pathways is particularly challenging as metabolites are difficult to visualize, particularly over long periods of time. Multi-modal studies using live dyes, in vitro designs, as well as computational modeling, will help in the understanding metabolic pathways, fluxes via glia, as well as direct neuron-to-vascular transport.
In vivo Imaging and Objective Quantification to Understand Glial Cell Interactions
To understand the role of glia and the NVU, the correct tools are needed to visualize components with sufficient resolution to resolve subcellular structures. For this, zebrafish are particularly well suited as transgenic reporter lines exist to label as well as manipulate (i.e., upregulation, downregulation, and loss of function) each component of the NVU in vivo. As such, multi-transgenic reporter lines can be generated to visualize complex cell structures and interactions in the developing retina (Lieschke and Currie, 2007). Further, the function of the NVU can be visualized in real-time in vivo using several physiological readouts such as blood flow (e.g., red blood cell movement) or neuronal activity (calcium reporters). Elaborate integrations of NVU studies are needed to answer questions on the spatiotemporal integration of angiogenesis, barrierogenesis, gliogenesis, and NVU function. This is exemplified by recent studies (Rosa et al., 2015; Zhang R. et al., 2019) showing that neuronal activity during development impacts glial maturation at the synapse. However, it is unclear how these events may in turn influence glial support for neurons and ultimately NVU formation. Combining state-of-the-art visualization tools, examinations of NVU dynamics and functional studies in vivo will compliment high-resolution structural studies [e.g., electron microscopy (Willis, 2011)] and provide further insights into the roles of glia in the form and function of the NVU.
As glial morphology is tightly linked to their function, quantitative objective analysis of glia morphology can provide novel insights. Recent advances in NVU in vivo imaging, such as Two-photon microscopy (TPM), Intrinsic optical signal imaging (IOSI), Optical coherence tomography (OCT), or Laser speckle contrast imaging (LSCI) will greatly contribute to our understanding of NVU functionality and dynamics (Yoon and Jeong, 2019). This will enable imaging of functional readouts, such as blood flow, following neuronal stimuli including exposure to light flicker in the retina, to ascertain the impact of glial dysfunction on neurovascular coupling mediated events. This is complemented by ever more sophisticated tools in which to study the NVU in freely behaving animals (Cong et al., 2017; Senarathna et al., 2019) as well as the ability to investigate NVU function during development (Chhabria et al., 2018). The advancement of imaging modalities and spatio-temporal resolution offers great benefits for understanding these developmental and functional relationships in the NVU (Figure 3). However, one drawback to having this increase in resolution is the exponential increase in the amount of data that is generated per experiment. With the vast amount of experimental data, data analysis and computational expertise has become a limiting factor for many laboratories. Trained specialists and specialized data analysis training are needed to extract meaningful data in a high-throughput, standardized, and objective manner (Levet et al., 2021). Dedicated specialized computational approaches can address this analytical bottleneck to analyze data, and push scientific boundaries by computationally modeling what is experimentally impossible. This could be by in silico multi-transgenics (i.e., artificially overlaying transgene expression on a reference tissue) to establish a virtual retina atlas, similar to the zebrafish brain atlas, allowing for further neuronal activity mapping (Randlett et al., 2015) and co-localization analysis (Ronneberger et al., 2012) in silico. Similarly, deep learning approaches open new avenues from feature mining (Dollar et al., 2007), over retinal ganglion counting (Masin et al., 2021), to fundus and OCT analysis (Badar et al., 2020). Furthermore, this is accompanied by an increased assessment of feature and data connectivity and relationships, such as principal component analysis and Uniform Manifold Approximation and Projection (Allaoui et al., 2020). Additionally, carefully designed data analysis workflows will provide new insights into our data and the NVU, most likely in a way that is beyond current comprehension.
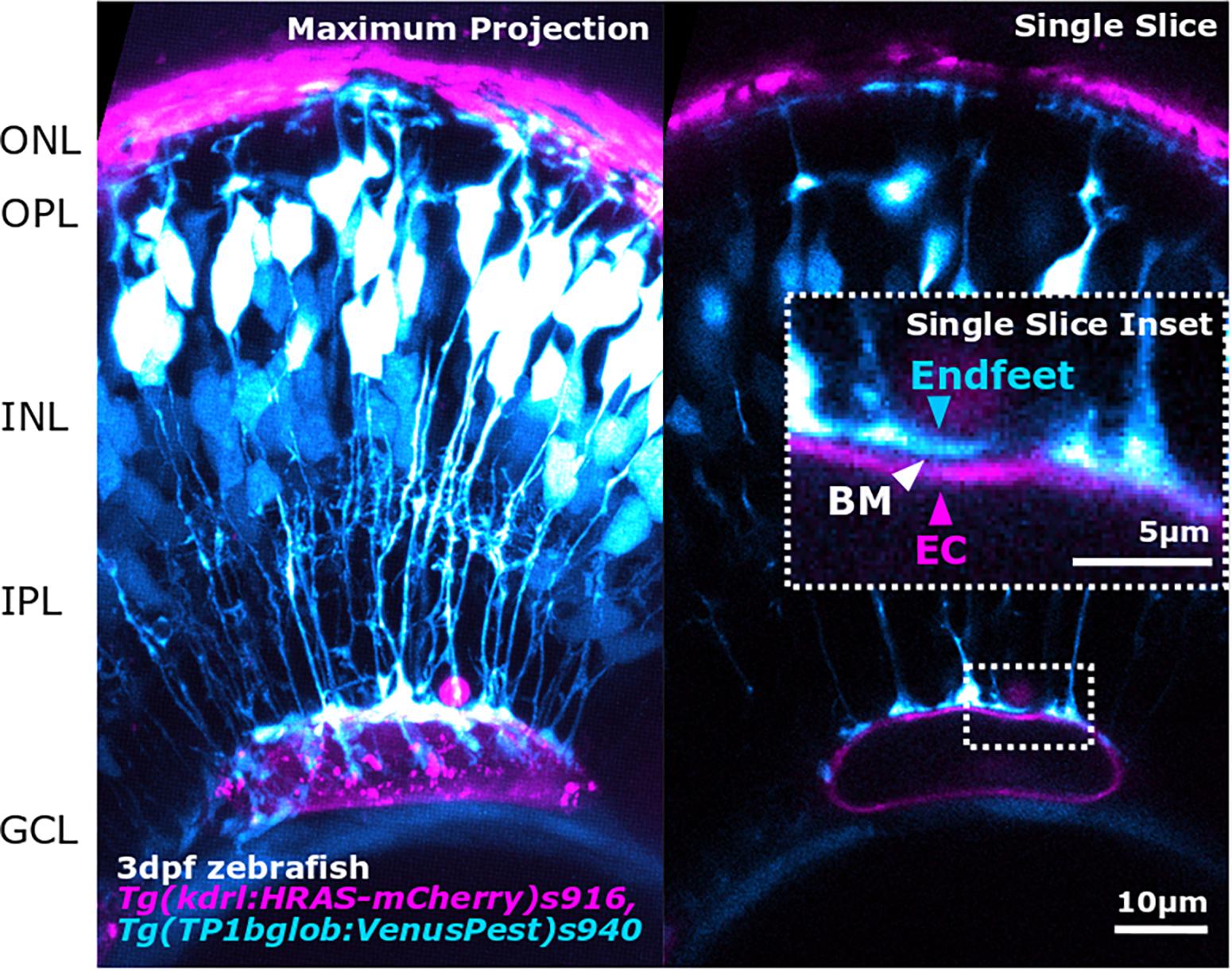
Figure 3. Advancements in image acquisition methods and resolution, enable the study of NVU component interactions as shown here by the interaction between MG endfeet (blue) and blood vessels (magenta), separated by the BM (white arrowhead; inset) in the developing zebrafish retina. The image was acquired with Zeiss LSM 900 AiryScan2 microscopy that allows in vivo acquisition with a resolution of 120 × 120 × 350 nm (x,y,z).
The coupling of quantitative analysis of glia shape and NVU function will also be essential in understanding the kinetics of NVU degeneration and dysfunction in disease (Luengo-Oroz et al., 2011). This is exemplified by studies that link cell feature information to genomic profiles (Yuan et al., 2012) or even link feature analysis to image cytometry, which measures cellular protein and DNA in images (Tárnok, 2006). Once robust and repeatable ways to characterize glia morphology are established, it will be possible to directly link cell morphology to function and to their molecular profiles, which could then in turn be linked to NVU functionality studies. One prominent example used transcriptomics to identify and classify retinal bipolar cells by matching their molecular expression with cell morphology (Shekhar et al., 2016). These comprehensive experimental paradigms could be used for each component in the NVU, throughout its development, to identify the molecular mechanisms regulating cell shape, function, and connectivity. These insights into precise cellular mechanisms that control the development and function of the NVU may also inform the pathogenesis of disease in mature tissues. As such, identification of the molecular and morphological changes (e.g., glial hypertrophy), that potentially precede pathology in disease, would facilitate the diagnosis of NVU dysfunction and provide the opportunity for early treatment and better clinical outcomes.
Conclusion
It is increasingly clear that glial cells are critical for NVU development, function, maintenance, and dysfunction in disease. However, uncovering the precise contribution of glial cells to the NVU has been challenging to date, particularly when trying to understand and integrate their dual contribution to neurons and the vasculature. With the advancement of imaging, computational and genetic tools, it will be possible to use multidimensional approaches (morphology, function, genetics, interactions, and dynamics) to clarify the exact role(s) of glial cells in the NVU. Examining the role of glia in the “neuro-glial-vascular unit” with such a holistic approach will enhance the understanding, diagnosis, and treatment of aging and disease in the nervous system.
Author Contributions
EK, JG, and RM contributed to the conception and design of the study. EK wrote the first draft of the manuscript. All authors contributed to manuscript revision, read, and approved the submitted version.
Funding
This project was funded by a Moorfields Eye Charity Springboard award (GR001208) and Biotechnology and Biological Sciences Research Council David Phillips Fellowship (BB/S010386/1) to RM.
Conflict of Interest
The authors declare that the research was conducted in the absence of any commercial or financial relationships that could be construed as a potential conflict of interest.
Publisher’s Note
All claims expressed in this article are solely those of the authors and do not necessarily represent those of their affiliated organizations, or those of the publisher, the editors and the reviewers. Any product that may be evaluated in this article, or claim that may be made by its manufacturer, is not guaranteed or endorsed by the publisher.
Acknowledgments
The authors are grateful to all funders who supported this work.
References
Abbott, N. J., Rönnbäck, L., and Hansson, E. (2006). Astrocyte–endothelial interactions at the blood–brain barrier. Nat. Rev. Neurosci. 7, 41–53. doi: 10.1038/nrn1824
Akiyama, H., Barger, S., Barnum, S., Bradt, B., Bauer, J., Cole, G. M., et al. (2000). Inflammation and Alzheimer’s disease. Neurobiol. Aging 21, 383–421. doi: 10.1016/S0197-4580(00)00124-X
Allaoui, M., Kherfi, M. L., and Cheriet, A. (2020). “Considerably improving clustering algorithms using UMAP dimensionality reduction technique: a comparative study,” in Image and Signal Processing Lecture Notes in Computer Science, eds A. El Moataz, D. Mammass, A. Mansouri, and F. Nouboud (Cham: Springer International Publishing), 317–325. doi: 10.1007/978-3-030-51935-3_34
Angueyra, J. M., and Kindt, K. S. (2018). Leveraging zebrafish to study retinal degenerations. Front. Cell Dev. Biol. 6:110. doi: 10.3389/fcell.2018.00110
Araque, A., Parpura, V., Sanzgiri, R. P., and Haydon, P. G. (1999). Tripartite synapses: glia, the unacknowledged partner. Trends Neurosci. 22, 208–215. doi: 10.1016/s0166-2236(98)01349-6
Argente-Arizón, P., Guerra-Cantera, S., Garcia-Segura, L. M., Argente, J., and Chowen, J. A. (2017). Glial cells and energy balance. J. Mol. Endocrinol. 58, R59–R71. doi: 10.1530/JME-16-0182
Armulik, A., Genové, G., Mäe, M., Nisancioglu, M. H., Wallgard, E., Niaudet, C., et al. (2010). Pericytes regulate the blood-brain barrier. Nature 468, 557–561. doi: 10.1038/nature09522
Ashraf, M., Sampani, K., Clermont, A., Abu-Qamar, O., Rhee, J., Silva, P. S., et al. (2020). Vascular density of deep, intermediate and superficial vascular plexuses are differentially affected by diabetic retinopathy severity. Invest. Ophthalmol. Vis. Sci. 61, 53–53. doi: 10.1167/iovs.61.10.53
Attwell, D., Buchan, A. M., Charpak, S., Lauritzen, M., MacVicar, B. A., and Newman, E. A. (2010). Glial and neuronal control of brain blood flow. Nature 468, 232–243. doi: 10.1038/nature09613
Badar, M., Haris, M., and Fatima, A. (2020). Application of deep learning for retinal image analysis: a review. Comput. Sci. Rev. 35:100203. doi: 10.1016/j.cosrev.2019.100203
Bak, L. K., Schousboe, A., and Waagepetersen, H. S. (2006). The glutamate/GABA-glutamine cycle: aspects of transport, neurotransmitter homeostasis and ammonia transfer. J. Neurochem. 98, 641–653. doi: 10.1111/j.1471-4159.2006.03913.x
Bélanger, M., and Magistretti, P. J. (2009). The role of astroglia in neuroprotection. Dialogues Clin. Neurosci. 11, 281–295.
Bell, R. D., Winkler, E. A., Sagare, A. P., Singh, I., LaRue, B., Deane, R., et al. (2010). Pericytes control key neurovascular functions and neuronal phenotype in the adult brain and during brain aging. Neuron 68, 409–427. doi: 10.1016/j.neuron.2010.09.043
Ben Haim, L., and Rowitch, D. H. (2017). Functional diversity of astrocytes in neural circuit regulation. Nat. Rev. Neurosci. 18, 31–41. doi: 10.1038/nrn.2016.159
Biesecker, K. R., Srienc, A. I., Shimoda, A. M., Agarwal, A., Bergles, D. E., Kofuji, P., et al. (2016). Glial cell calcium signaling mediates capillary regulation of blood flow in the retina. J. Neurosci. 36, 9435–9445. doi: 10.1523/JNEUROSCI.1782-16.2016
Bigio, M. R. D. (2010). Ependymal cells: biology and pathology. Acta Neuropathol. 119, 55–73. doi: 10.1007/s00401-009-0624-y
Biswas, S., Bachay, G., Chu, J., Hunter, D. D., and Brunken, W. J. (2017). Laminin-dependent interaction between astrocytes and microglia: a role in retinal angiogenesis. Am. J. Pathol. 187, 2112–2127. doi: 10.1016/j.ajpath.2017.05.016
Blanco-Suarez, E., Liu, T.-F., Kopelevich, A., and Allen, N. J. (2018). Astrocyte-secreted chordin-like 1 drives synapse maturation and limits plasticity by increasing synaptic GluA2 AMPA receptors. Neuron 100, 1116–1132.e13. doi: 10.1016/j.neuron.2018.09.043
Boekhoorn, K., Joels, M., and Lucassen, P. J. (2006). Increased proliferation reflects glial and vascular-associated changes, but not neurogenesis in the presenile Alzheimer hippocampus. Neurobiol. Dis. 24, 1–14. doi: 10.1016/j.nbd.2006.04.017
Booher, J., and Sensenbrenner, M. (1972). Growth and cultivation of dissociated neurons and glial cells from embryonic chick, rat and human brain in flask cultures. Neurobiology 2, 97–105.
Boss, J. D., Singh, P. K., Pandya, H. K., Tosi, J., Kim, C., Tewari, A., et al. (2017). Assessment of neurotrophins and inflammatory mediators in vitreous of patients with diabetic retinopathy. Invest. Ophthalmol. Vis. Sci. 58, 5594–5603. doi: 10.1167/iovs.17-21973
Bouzier-Sore, A.-K., Voisin, P., Bouchaud, V., Bezancon, E., Franconi, J.-M., and Pellerin, L. (2006). Competition between glucose and lactate as oxidative energy substrates in both neurons and astrocytes: a comparative NMR study. Eur. J. Neurosci. 24, 1687–1694. doi: 10.1111/j.1460-9568.2006.05056.x
Bouzier-Sore, A.-K., Voisin, P., Canioni, P., Magistretti, P. J., and Pellerin, L. (2003). Lactate is a preferential oxidative energy substrate over glucose for neurons in culture. J. Cereb. Blood Flow Metab. 23, 1298–1306. doi: 10.1097/01.WCB.0000091761.61714.25
Bozoyan, L., Khlghatyan, J., and Saghatelyan, A. (2012). Astrocytes control the development of the migration-promoting vasculature scaffold in the postnatal brain via VEGF signaling. J. Neurosci. 32, 1687–1704. doi: 10.1523/JNEUROSCI.5531-11.2012
Burda, J. E., and Sofroniew, M. V. (2014). Reactive gliosis and the multicellular response to CNS damage and disease. Neuron 81, 229–248. doi: 10.1016/j.neuron.2013.12.034
Butt, A. M. (2011). ATP: a ubiquitous gliotransmitter integrating neuron–glial networks. Semin. Cell Dev. Biol. 22, 205–213. doi: 10.1016/j.semcdb.2011.02.023
Cahoy, J. D., Emery, B., Kaushal, A., Foo, L. C., Zamanian, J. L., Christopherson, K. S., et al. (2008). A transcriptome database for astrocytes, neurons, and oligodendrocytes: a new resource for understanding brain development and function. J. Neurosci. 28, 264–278. doi: 10.1523/JNEUROSCI.4178-07.2008
Cajal, R. (1995). Cajal’s Histology of the Nervous System of Man and Vertebrates. Oxford: Oxford University Press.
Cekanaviciute, E., and Buckwalter, M. S. (2016). Astrocytes: integrative regulators of neuroinflammation in stroke and other neurological diseases. Neurotherapeutics 13, 685–701. doi: 10.1007/s13311-016-0477-8
Chai, H., Diaz-Castro, B., Shigetomi, E., Monte, E., Octeau, J. C., Yu, X., et al. (2017). Neural circuit-specialized astrocytes: transcriptomic, proteomic, morphological, and functional evidence. Neuron 95, 531–549.e9. doi: 10.1016/j.neuron.2017.06.029
Chen, J., Poskanzer, K. E., Freeman, M. R., and Monk, K. R. (2020). Live-imaging of astrocyte morphogenesis and function in zebrafish neural circuits. Nat. Neurosci. 23, 1297–1306. doi: 10.1038/s41593-020-0703-x
Chhabria, K., Plant, K., Bandmann, O., Wilkinson, R. N., Martin, C., Kugler, E., et al. (2018). The effect of hyperglycemia on neurovascular coupling and cerebrovascular patterning in zebrafish. J. Cereb. Blood Flow Metab. 40, 298–313. doi: 10.1177/0271678X18810615
Chico, T. J. A., and Kugler, E. C. (2021). Cerebrovascular development: mechanisms and experimental approaches. Cell. Mol. Life Sci. 78, 4377–4398. doi: 10.1007/s00018-021-03790-1
Coffey, P. J., Gias, C., McDermott, C. J., Lundh, P., Pickering, M. C., Sethi, C., et al. (2007). Complement factor H deficiency in aged mice causes retinal abnormalities and visual dysfunction. Proc. Natl. Acad. Sci. U.S.A. 104, 16651–16656. doi: 10.1073/pnas.0705079104
Colombo, J. A., and Reisin, H. D. (2004). Interlaminar astroglia of the cerebral cortex: a marker of the primate brain. Brain Res. 1006, 126–131. doi: 10.1016/j.brainres.2004.02.003
Colombo, J. A., Yáñez, A., Puissant, V., and Lipina, S. (1995). Long, interlaminar astroglial cell processes in the cortex of adult monkeys. J. Neurosci. Res. 40, 551–556. doi: 10.1002/jnr.490400414
Cong, L., Wang, Z., Chai, Y., Hang, W., Shang, C., Yang, W., et al. (2017). Rapid whole brain imaging of neural activity in freely behaving larval zebrafish (Danio rerio). eLife 6:e28158. doi: 10.7554/eLife.28158
Cunha-Vaz, J., Bernardes, R., and Lobo, C. (2011). Blood-retinal barrier. Eur. J. Ophthalmol. 21, 3–9. doi: 10.5301/EJO.2010.6049
da Silva, S. M., Campos, G. D., Gomes, F. C. A., and Stipursky, J. (2019). Radial glia-endothelial cells’ bidirectional interactions control vascular maturation and astrocyte differentiation: impact for blood-brain barrier formation. Curr. Neurovasc. Res. 16, 291–300. doi: 10.2174/1567202616666191014120156
Diaz Verdugo, C., Myren-Svelstad, S., Aydin, E., Van Hoeymissen, E., Deneubourg, C., Vanderhaeghe, S., et al. (2019). Glia-neuron interactions underlie state transitions to generalized seizures. Nat. Commun. 10:3830. doi: 10.1038/s41467-019-11739-z
Diaz-Arrastia, R., Wang, K. K. W., Papa, L., Sorani, M. D., Yue, J. K., Puccio, A. M., et al. (2013). Acute biomarkers of traumatic brain injury: relationship between plasma levels of ubiquitin C-terminal hydrolase-L1 and glial fibrillary acidic protein. J. Neurotrauma 31, 19–25. doi: 10.1089/neu.2013.3040
Díaz-Coránguez, M., Ramos, C., and Antonetti, D. A. (2017). The inner blood-retinal barrier: cellular basis and development. Vis. Res. 139, 123–137. doi: 10.1016/j.visres.2017.05.009
Dietrich, M., Hecker, C., Hilla, A., Cruz-Herranz, A., Hartung, H.-P., Fischer, D., et al. (2019). Using optical coherence tomography and optokinetic response as structural and functional visual system readouts in mice and rats. J. Vis. Exp. 143:e58571. doi: 10.3791/58571
Dollar, P., Tu, Z., Tao, H., and Belongie, S. (2007). “Feature mining for image classification,” in Proceedings of the 2007 IEEE Conference on Computer Vision and Pattern Recognition, Minneapolis, MN, 1–8. doi: 10.1109/CVPR.2007.383046
Duan, L.-J., Pan, S. J., Sato, T. N., and Fong, G.-H. (2017). Retinal angiogenesis regulates astrocytic differentiation in neonatal mouse retinas by oxygen dependent mechanisms. Sci. Rep. 7:17608. doi: 10.1038/s41598-017-17962-2
Duke, D. C., Moran, L. B., Turkheimer, F. E., Banati, R., and Graeber, M. B. (2004). Microglia in culture: what genes do they express? DNE 26, 30–37. doi: 10.1159/000080709
Edison, P., Donat, C. K., and Sastre, M. (2018). In vivo imaging of glial activation in Alzheimer’s disease. Front. Neurol. 9:625. doi: 10.3389/fneur.2018.00625
Elbaz, B., and Popko, B. (2019). Molecular control of oligodendrocyte development. Trends Neurosci. 42, 263–277. doi: 10.1016/j.tins.2019.01.002
Escartin, C., Galea, E., Lakatos, A., O’Callaghan, J. P., Petzold, G. C., Serrano-Pozo, A., et al. (2021). Reactive astrocyte nomenclature, definitions, and future directions. Nat. Neurosci. 24, 312–325. doi: 10.1038/s41593-020-00783-4
Falk, S., and Götz, M. (2017). Glial control of neurogenesis. Curr. Opin. Neurobiol. 47, 188–195. doi: 10.1016/j.conb.2017.10.025
Fanning, A. S., Mitic, L. L., and Anderson, J. M. (1999). Transmembrane proteins in the tight junction barrier. J. Am. Soc. Nephrol. 10, 1337–1345.
Fantin, A., Vieira, J. M., Gestri, G., Denti, L., Schwarz, Q., Prykhozhij, S., et al. (2010). Tissue macrophages act as cellular chaperones for vascular anastomosis downstream of VEGF-mediated endothelial tip cell induction. Blood 116, 829–840. doi: 10.1182/blood-2009-12-257832
Fellin, T. (2009). Communication between neurons and astrocytes: relevance to the modulation of synaptic and network activity. J. Neurochem. 108, 533–544. doi: 10.1111/j.1471-4159.2008.05830.x
Fellin, T., Pascual, O., Gobbo, S., Pozzan, T., Haydon, P. G., and Carmignoto, G. (2004). Neuronal synchrony mediated by astrocytic glutamate through activation of extrasynaptic NMDA receptors. Neuron 43, 729–743. doi: 10.1016/j.neuron.2004.08.011
Fletcher, E. L., Phipps, J. A., Ward, M. M., Vessey, K. A., and Wilkinson-Berka, J. L. (2010). The renin–angiotensin system in retinal health and disease: its influence on neurons, glia and the vasculature. Prog. Retin. Eye Res. 29, 284–311. doi: 10.1016/j.preteyeres.2010.03.003
Florence, C. M., Baillie, L. D., and Mulligan, S. J. (2012). Dynamic volume changes in astrocytes are an intrinsic phenomenon mediated by bicarbonate ion flux. PLoS One 7:e51124. doi: 10.1371/journal.pone.0051124
Forrester, J. V., McMenamin, P. G., and Dando, S. J. (2018). CNS infection and immune privilege. Nat. Rev. Neurosci. 19, 655–671. doi: 10.1038/s41583-018-0070-8
Frey, T., and Antonetti, D. A. (2011). Alterations to the blood–retinal barrier in diabetes: cytokines and reactive oxygen species. Antioxid. Redox Signal. 15, 1271–1284. doi: 10.1089/ars.2011.3906
Fruttiger, M. (2007). Development of the retinal vasculature. Angiogenesis 10, 77–88. doi: 10.1007/s10456-007-9065-1
Furube, E., Ishii, H., Nambu, Y., Kurganov, E., Nagaoka, S., Morita, M., et al. (2020). Neural stem cell phenotype of tanycyte-like ependymal cells in the circumventricular organs and central canal of adult mouse brain. Sci. Rep. 10:2826. doi: 10.1038/s41598-020-59629-5
Gemberling, M., Bailey, T. J., Hyde, D. R., and Poss, K. D. (2013). The zebrafish as a model for complex tissue regeneration. Trends Genet. 29, 611–620. doi: 10.1016/j.tig.2013.07.003
Gestri, G., Link, B. A., and Neuhauss, S. C. F. (2012). The visual system of zebrafish and its use to model human ocular Diseases. Dev. Neurobiol. 72, 302–327. doi: 10.1002/dneu.20919
Giaume, C., Naus, C. C., Sáez, J. C., and Leybaert, L. (2020). Glial connexins and pannexins in the healthy and diseased brain. Physiol. Rev. 101, 93–145. doi: 10.1152/physrev.00043.2018
Gleichman, A. J., and Carmichael, S. T. (2020). Glia in neurodegeneration: drivers of disease or along for the ride? Neurobiol. Dis. 142:104957. doi: 10.1016/j.nbd.2020.104957
Gleiser, C., Wagner, A., Fallier-Becker, P., Wolburg, H., Hirt, B., and Mack, A. F. (2016). Aquaporin-4 in astroglial cells in the CNS and supporting cells of sensory organs—a comparative perspective. Int. J. Mol. Sci. 17:1411. doi: 10.3390/ijms17091411
Goldman, D. (2014). Müller glial cell reprogramming and retina regeneration. Nat. Rev. Neurosci. 15, 431–442. doi: 10.1038/nrn3723
Gordon, G. R. J., Mulligan, S. J., and MacVicar, B. A. (2007). Astrocyte control of the cerebrovasculature. Glia 55, 1214–1221. doi: 10.1002/glia.20543
Grupp, L., Wolburg, H., and Mack, A. F. (2010). Astroglial structures in the zebrafish brain. J. Comp. Neurol. 518, 4277–4287. doi: 10.1002/cne.22481
Guillemin, G. J., and Brew, B. J. (2004). Microglia, macrophages, perivascular macrophages, and pericytes: a review of function and identification. J. Leukoc. Biol. 75, 388–397. doi: 10.1189/jlb.0303114
Hasel, P., Rose, I. V. L., Sadick, J. S., Kim, R. D., and Liddelow, S. A. (2021). Neuroinflammatory astrocyte subtypes in the mouse brain. Nat. Neurosci. 1–13. doi: 10.1038/s41593-021-00905-6
Haseloff, R. F., Blasig, I. E., Bauer, H.-C., and Bauer, H. (2005). In search of the astrocytic factor(s) modulating blood–brain barrier functions in brain capillary endothelial cells in vitro. Cell. Mol. Neurobiol. 25, 25–39. doi: 10.1007/s10571-004-1375-x
Hauck, S. M., Suppmann, S., and Ueffing, M. (2003). Proteomic profiling of primary retinal Müller glia cells reveals a shift in expression patterns upon adaptation to in vitro conditions. Glia 44, 251–263. doi: 10.1002/glia.10292
Hawkins, B. T., and Davis, T. P. (2005). The blood-brain barrier/neurovascular unit in health and disease. Pharmacol. Rev. 57, 173–185. doi: 10.1124/pr.57.2.4
Hayashi, Y., Nomura, M., Yamagishi, S.-I., Harada, S.-I., Yamashita, J., and Yamamoto, H. (1997). Induction of various blood-brain barrier properties in non-neural endothelial cells by close apposition to co-cultured astrocytes. Glia 19, 13–26. doi: 10.1002/(SICI)1098-1136(199701)19:1<13::AID-GLIA2<3.0.CO;2-B
Haycock, J. W. (2011). 3D cell culture: a review of current approaches and techniques. Methods Mol. Biol. 695, 1–15. doi: 10.1007/978-1-60761-984-0_1
Heithoff, B. P., George, K. K., Phares, A. N., Zuidhoek, I. A., Munoz-Ballester, C., and Robel, S. (2021). Astrocytes are necessary for blood–brain barrier maintenance in the adult mouse brain. Glia 69, 436–472. doi: 10.1002/glia.23908
Helms, H. C., Abbott, N. J., Burek, M., Cecchelli, R., Couraud, P.-O., Deli, M. A., et al. (2016). In vitro models of the blood–brain barrier: an overview of commonly used brain endothelial cell culture models and guidelines for their use. J. Cereb. Blood Flow Metab. 36, 862–890. doi: 10.1177/0271678X16630991
Henshall, T. L., Keller, A., He, L., Johansson, B. R., Wallgard, E., Raschperger, E., et al. (2015). Notch3 is necessary for blood vessel integrity in the central nervous system. Arterioscler. Thromb. Vasc. Biol. 35, 409–420. doi: 10.1161/ATVBAHA.114.304849
Hillen, A. E. J., Burbach, J. P. H., and Hol, E. M. (2018). Cell adhesion and matricellular support by astrocytes of the tripartite synapse. Prog. Neurobiol. 165–167, 165–167; 66–86. doi: 10.1016/j.pneurobio.2018.02.002
Hoddevik, E. H., Rao, S. B., Zahl, S., Boldt, H. B., Ottersen, O. P., and Amiry-Moghaddam, M. (2020). Organisation of extracellular matrix proteins laminin and agrin in pericapillary basal laminae in mouse brain. Brain Struct. Funct. 225, 805–816. doi: 10.1007/s00429-020-02036-3
Hoiland, R. L., Caldwell, H. G., Howe, C. A., Nowak-Flück, D., Stacey, B. S., Bailey, D. M., et al. (2020). Nitric oxide is fundamental to neurovascular coupling in humans. J. Physiol. 598, 4927–4939. doi: 10.1113/JP280162
Hurley, J. B., Lindsay, K. J., and Du, J. (2015). Glucose, lactate, and shuttling of metabolites in vertebrate retinas. J. Neurosci. Res. 93, 1079–1092. doi: 10.1002/jnr.23583
Igarashi, Y., Utsumi, H., Chiba, H., Yamada-Sasamori, Y., Tobioka, H., Kamimura, Y., et al. (1999). Glial cell line-derived neurotrophic factor induces barrier function of endothelial cells forming the blood–brain barrier. Biochem. Biophys. Res. Commun. 261, 108–112. doi: 10.1006/bbrc.1999.0992
Ioannou, M. S., Jackson, J., Sheu, S.-H., Chang, C.-L., Weigel, A. V., Liu, H., et al. (2019). Neuron-astrocyte metabolic coupling protects against activity-induced fatty acid toxicity. Cell 177, 1522–1535.e14. doi: 10.1016/j.cell.2019.04.001
Iturria-Medina, Y., Sotero, R. C., Toussaint, P. J., Mateos-Pérez, J. M., and Evans, A. C. (2016). Early role of vascular dysregulation on late-onset Alzheimer’s disease based on multifactorial data-driven analysis. Nat. Commun. 7:11934. doi: 10.1038/ncomms11934
Janzer, R. C., and Raff, M. C. (1987). Astrocytes induce blood-brain barrier properties in endothelial cells. Nature 325, 253–257. doi: 10.1038/325253a0
Jha, M. K., Kim, J.-H., Song, G. J., Lee, W.-H., Lee, I.-K., Lee, H.-W., et al. (2018). Functional dissection of astrocyte-secreted proteins: implications in brain health and diseases. Prog. Neurobiol. 162, 37–69. doi: 10.1016/j.pneurobio.2017.12.003
Jo, A. O., Ryskamp, D. A., Phuong, T. T. T., Verkman, A. S., Yarishkin, O., MacAulay, N., et al. (2015). TRPV4 and AQP4 channels synergistically regulate cell volume and calcium homeostasis in retinal Müller glia. J. Neurosci. 35, 13525–13537. doi: 10.1523/JNEUROSCI.1987-15.2015
John Lin, C.-C., Yu, K., Hatcher, A., Huang, T.-W., Lee, H. K., Carlson, J., et al. (2017). Identification of diverse astrocyte populations and their malignant analogs. Nat. Neurosci. 20, 396–405. doi: 10.1038/nn.4493
Kanow, M. A., Giarmarco, M. M., Jankowski, C. S., Tsantilas, K., Engel, A. L., Du, J., et al. (2017). Biochemical adaptations of the retina and retinal pigment epithelium support a metabolic ecosystem in the vertebrate eye. eLife 6:e28899. doi: 10.7554/eLife.28899
Karve, I. P., Taylor, J. M., and Crack, P. J. (2016). The contribution of astrocytes and microglia to traumatic brain injury. Br. J. Pharmacol. 173, 692–702. doi: 10.1111/bph.13125
Kawamura, H., Kobayashi, M., Li, Q., Yamanishi, S., Katsumura, K., Minami, M., et al. (2004). Effects of angiotensin II on the pericyte-containing microvasculature of the rat retina. J. Physiol. 561, 671–683. doi: 10.1113/jphysiol.2004.073098
Keaney, J., and Campbell, M. (2015). The dynamic blood-brain barrier. FEBS J. 282, 4067–4079. doi: 10.1111/febs.13412
Kim, Y. S., Choi, J., and Yoon, B.-E. (2020). Neuron-glia interactions in neurodevelopmental disorders. Cells 9:2176. doi: 10.3390/cells9102176
Kimelberg, H. K. (2010). Functions of mature mammalian astrocytes: a current view. Neuroscientist 16, 79–106. doi: 10.1177/1073858409342593
Koistinaho, M., Lin, S., Wu, X., Esterman, M., Koger, D., Hanson, J., et al. (2004). Apolipoprotein E promotes astrocyte colocalization and degradation of deposited amyloid-β peptides. Nat. Med. 10, 719–726. doi: 10.1038/nm1058
Kroehne, V., Freudenreich, D., Hans, S., Kaslin, J., and Brand, M. (2011). Regeneration of the adult zebrafish brain from neurogenic radial glia-type progenitors. Development 138, 4831–4841. doi: 10.1242/dev.072587
Kutuzov, N., Flyvbjerg, H., and Lauritzen, M. (2018). Contributions of the glycocalyx, endothelium, and extravascular compartment to the blood–brain barrier. Proc. Natl. Acad. Sci. U.S.A. 115, E9429–E9438. doi: 10.1073/pnas.1802155115
Lacoste, B., Comin, C. H., Ben-Zvi, A., Kaeser, P. S., Xu, X., Costa, L., et al. (2014). Sensory-related neural activity regulates the structure of vascular networks in the cerebral cortex. Neuron 83, 1117–1130. doi: 10.1016/j.neuron.2014.07.034
Lafrenaye, A. D., and Simard, J. M. (2019). Bursting at the seams: molecular mechanisms mediating astrocyte swelling. Int. J. Mol. Sci. 20:330. doi: 10.3390/ijms20020330
Lasta, M., Pemp, B., Schmidl, D., Boltz, A., Kaya, S., Palkovits, S., et al. (2013). Neurovascular dysfunction precedes neural dysfunction in the retina of patients with type 1 diabetes. Invest. Ophthalmol. Vis. Sci. 54, 842–847. doi: 10.1167/iovs.12-10873
Lavoie, J. L., and Sigmund, C. D. (2003). Minireview: overview of the renin-angiotensin system—an endocrine and paracrine system. Endocrinology 144, 2179–2183. doi: 10.1210/en.2003-0150
Le, Y.-Z. (2017). VEGF production and signaling in Müller glia are critical to modulating vascular function and neuronal integrity in diabetic retinopathy and hypoxic retinal vascular diseases. Vis. Res. 139, 108–114. doi: 10.1016/j.visres.2017.05.005
Lécuyer, M.-A., Kebir, H., and Prat, A. (2016). Glial influences on BBB functions and molecular players in immune cell trafficking. Biochim. Biophys. Acta Mol. Basis Dis. 1862, 472–482. doi: 10.1016/j.bbadis.2015.10.004
Lee, S.-W., Kim, W. J., Choi, Y. K., Song, H. S., Son, M. J., Gelman, I. H., et al. (2003). SSeCKS regulates angiogenesis and tight junction formation in blood-brain barrier. Nat. Med. 9, 900–906. doi: 10.1038/nm889
Levet, F., Carpenter, A. E., Eliceiri, K. W., Kreshuk, A., Bankhead, P., and Haase, R. (2021). Developing open-source software for bioimage analysis: opportunities and challenges. F1000Res 10:302. doi: 10.12688/f1000research.52531.1
Lieschke, G. J., and Currie, P. D. (2007). Animal models of human disease: zebrafish swim into view. Nat. Rev. Genet. 8, 353–367. doi: 10.1038/nrg2091
Limón, I. D., Angulo-Cruz, I., Sánchez-Abdon, L., and Patricio-Martínez, A. (2021). Disturbance of the glutamate-glutamine cycle, secondary to hepatic damage, compromises memory function. Front. Neurosci. 15:578922. doi: 10.3389/fnins.2021.578922
Lok, J., Gupta, P., Guo, S., Kim, W. J., Whalen, M. J., van Leyen, K., et al. (2007). Cell–cell signaling in the neurovascular unit. Neurochem. Res. 32, 2032–2045. doi: 10.1007/s11064-007-9342-9
Losada-Perez, M. (2018). Glia: from ‘just glue’ to essential players in complex nervous systems: a comparative view from flies to mammals. J. Neurogenet. 32, 78–91. doi: 10.1080/01677063.2018.1464568
Luengo-Oroz, M., Ledesma-Carbayo, M., Peyriéras, N., and Santos, A. (2011). Image analysis for understanding embryo development: a bridge from microscopy to biological insights. Curr. Opin. Genet. Dev. 21, 630–637. doi: 10.1016/j.gde.2011.08.001
Ma, S., Kwon, H. J., Johng, H., Zang, K., and Huang, Z. (2013). Radial glial neural progenitors regulate nascent brain vascular network stabilization via inhibition of Wnt signaling. PLoS Biol. 11:e1001469. doi: 10.1371/journal.pbio.1001469
MacDonald, R. B., Charlton-Perkins, M., and Harris, W. A. (2017). Mechanisms of Müller glial cell morphogenesis. Curr. Opin. Neurobiol. 47, 31–37. doi: 10.1016/j.conb.2017.08.005
MacDonald, R. B., Randlett, O., Oswald, J., Yoshimatsu, T., Franze, K., and Harris, W. A. (2015). Müller glia provide essential tensile strength to the developing retina. J. Cell Biol. 210, 1075–1083. doi: 10.1083/jcb.201503115
Macht, V. A. (2016). Neuro-immune interactions across development: a look at glutamate in the prefrontal cortex. Neurosci. Biobehav. Rev. 71, 267–280. doi: 10.1016/j.neubiorev.2016.08.039
Madaan, A., Chaudhari, P., Nadeau-Vallée, M., Hamel, D., Zhu, T., Mitchell, G., et al. (2019). Müller cell–localized G-protein–coupled receptor 81 (Hydroxycarboxylic Acid Receptor 1) regulates inner retinal vasculature via Norrin/Wnt pathways. Am. J. Pathol. 189, 1878–1896. doi: 10.1016/j.ajpath.2019.05.016
Magaki, S. D., Williams, C. K., and Vinters, H. V. (2018). Glial function (and dysfunction) in the normal & ischemic brain. Neuropharmacology 134, 218–225. doi: 10.1016/j.neuropharm.2017.11.009
Malik, V. A., and Di Benedetto, B. (2018). The blood-brain barrier and the EphR/Ephrin system: perspectives on a link between neurovascular and neuropsychiatric disorders. Front. Mol. Neurosci. 11:127. doi: 10.3389/fnmol.2018.00127
Maoz, B. M., Herland, A., FitzGerald, E. A., Grevesse, T., Vidoudez, C., Pacheco, A. R., et al. (2018). A linked organ-on-chip model of the human neurovascular unit reveals the metabolic coupling of endothelial and neuronal cells. Nat. Biotechnol. 36, 865–874. doi: 10.1038/nbt.4226
Marina, N., Turovsky, E., Christie, I. N., Hosford, P. S., Hadjihambi, A., Korsak, A., et al. (2018). Brain metabolic sensing and metabolic signaling at the level of an astrocyte. Glia 66, 1185–1199. doi: 10.1002/glia.23283
Masin, L., Claes, M., Bergmans, S., Cools, L., Andries, L., Davis, B. M., et al. (2021). A novel retinal ganglion cell quantification tool based on deep learning. Sci. Rep. 11:702. doi: 10.1038/s41598-020-80308-y
Matejuk, A., and Ransohoff, R. M. (2020). Crosstalk between astrocytes and microglia: an overview. Front. Immunol. 11:1416. doi: 10.3389/fimmu.2020.01416
McAllister, M. S., Krizanac-Bengez, L., Macchia, F., Naftalin, R. J., Pedley, K. C., Mayberg, M. R., et al. (2001). Mechanisms of glucose transport at the blood–brain barrier: an in vitro study. Brain Res. 904, 20–30. doi: 10.1016/S0006-8993(01)02418-0
McCarthy, K. D., and de Vellis, J. (1980). Preparation of separate astroglial and oligodendroglial cell cultures from rat cerebral tissue. J. Cell Biol. 85, 890–902. doi: 10.1083/jcb.85.3.890
McConnell, H. L., Kersch, C. N., Woltjer, R. L., and Neuwelt, E. A. (2017). The translational significance of the neurovascular unit ∗. J. Biol. Chem. 292, 762–770. doi: 10.1074/jbc.R116.760215
Mehta, R. I., Tosun, C., Ivanova, S., Tsymbalyuk, N., Famakin, B. M., Kwon, M. S., et al. (2015). Sur1-Trpm4 cation channel expression in human cerebral infarcts. J. Neuropathol. Exp. Neurol. 74, 835–849. doi: 10.1097/NEN.0000000000000223
Metea, M. R., and Newman, E. A. (2007). Signalling within the neurovascular unit in the mammalian retina. Exp. Physiol. 92, 635–640. doi: 10.1113/expphysiol.2006.036376
Mizee, M. R., Wooldrik, D., Lakeman, K. A. M., van het Hof, B., Drexhage, J. A. R., Geerts, D., et al. (2013). Retinoic acid induces blood–brain barrier development. J. Neurosci. 33, 1660–1671. doi: 10.1523/JNEUROSCI.1338-12.2013
Molofsky, A. V., Kelley, K. W., Tsai, H.-H., Redmond, S. A., Chang, S. M., Madireddy, L., et al. (2014). Astrocyte-encoded positional cues maintain sensorimotor circuit integrity. Nature 509, 189–194. doi: 10.1038/nature13161
Morris, A. W. J., Sharp, M. M., Albargothy, N. J., Fernandes, R., Hawkes, C. A., Verma, A., et al. (2016). Vascular basement membranes as pathways for the passage of fluid into and out of the brain. Acta Neuropathol. 131, 725–736. doi: 10.1007/s00401-016-1555-z
Mu, Y., Bennett, D. V., Rubinov, M., Narayan, S., Yang, C.-T., Tanimoto, M., et al. (2019). Glia accumulate evidence that actions are futile and suppress unsuccessful behavior. Cell 178, 27–43.e19. doi: 10.1016/j.cell.2019.05.050
Muñoz, M. F., Puebla, M., and Figueroa, X. F. (2015). Control of the neurovascular coupling by nitric oxide-dependent regulation of astrocytic Ca(2+) signaling. Front. Cell. Neurosci. 9:59. doi: 10.3389/fncel.2015.00059
Muñoz-Ballester, C., Umans, R. A., and Robel, S. (2021). Leveraging zebrafish to study bona fide astrocytes. Trends Neurosci. 44, 77–79. doi: 10.1016/j.tins.2020.10.013
Nagashima, M., Hadidjojo, J., Barthel, L. K., Lubensky, D. K., and Raymond, P. A. (2017). Anisotropic Müller glial scaffolding supports a multiplex lattice mosaic of photoreceptors in zebrafish retina. Neural Dev. 12:20. doi: 10.1186/s13064-017-0096-z
Nagele, R. G., Wegiel, J., Venkataraman, V., Imaki, H., Wang, K.-C., and Wegiel, J. (2004). Contribution of glial cells to the development of amyloid plaques in Alzheimer’s disease. Neurobiol. Aging 25, 663–674. doi: 10.1016/j.neurobiolaging.2004.01.007
Newman, E., and Reichenbach, A. (1996). The Müller cell: a functional element of the retina. Trends Neurosci. 19, 307–312. doi: 10.1016/0166-2236(96)10040-0
Nimmerjahn, A., Kirchhoff, F., and Helmchen, F. (2005). Resting microglial cells are highly dynamic surveillants of brain parenchyma in vivo. Science 308, 1314–1318. doi: 10.1126/science.1110647
Nitta, T., Hata, M., Gotoh, S., Seo, Y., Sasaki, H., Hashimoto, N., et al. (2003). Size-selective loosening of the blood-brain barrier in claudin-5–deficient mice. J. Cell Biol. 161, 653–660. doi: 10.1083/jcb.200302070
Nortley, R., and Attwell, D. (2017). Control of brain energy supply by astrocytes. Curr. Opin. Neurobiol. 47, 80–85. doi: 10.1016/j.conb.2017.09.012
Nzou, G., Wicks, R. T., VanOstrand, N. R., Mekky, G. A., Seale, S. A., El-Taibany, A., et al. (2020). Multicellular 3D neurovascular unit model for assessing hypoxia and neuroinflammation induced blood-brain barrier dysfunction. Sci. Rep. 10:9766. doi: 10.1038/s41598-020-66487-8
O’Brown, N. M., Megason, S. G., and Gu, C. (2019). Suppression of transcytosis regulates zebrafish blood-brain barrier function. eLife 8:e47326. doi: 10.7554/eLife.47326
O’Brown, N. M., Pfau, S. J., and Gu, C. (2018). Bridging barriers: a comparative look at the blood–brain barrier across organisms. Genes Dev. 32, 466–478. doi: 10.1101/gad.309823.117
O’Sullivan, M. L., Puñal, V. M., Kerstein, P. C., Brzezinski, J. A., Glaser, T., Wright, K. M., et al. (2017). Astrocytes follow ganglion cell axons to establish an angiogenic template during retinal development. Glia 65, 1697–1716. doi: 10.1002/glia.23189
Oberheim, N. A., Takano, T., Han, X., He, W., Lin, J. H. C., Wang, F., et al. (2009). Uniquely hominid features of adult human astrocytes. J. Neurosci. 29, 3276–3287. doi: 10.1523/JNEUROSCI.4707-08.2009
Otteson, D. C., and Phillips, M. J. (2010). A conditional immortalized mouse muller glial cell line expressing glial and retinal stem cell genes. Invest. Ophthalmol. Vis. Sci. 51, 5991–6000. doi: 10.1167/iovs.10-5395
Outtz, H. H., Tattersall, I. W., Kofler, N. M., Steinbach, N., and Kitajewski, J. (2011). Notch1 controls macrophage recruitment and Notch signaling is activated at sites of endothelial cell anastomosis during retinal angiogenesis in mice. Blood 118, 3436–3439. doi: 10.1182/blood-2010-12-327015
Öz, G., Seaquist, E. R., Kumar, A., Criego, A. B., Benedict, L. E., Rao, J. P., et al. (2007). Human brain glycogen content and metabolism: implications on its role in brain energy metabolism. Am. J. Physiol. Endocrinol. Metab. 292, E946–E951. doi: 10.1152/ajpendo.00424.2006
Paisley, C. E., and Kay, J. N. (2021). Seeing stars: development and function of retinal astrocytes. Dev. Biol. 478, 144–154. doi: 10.1016/j.ydbio.2021.07.007
Pannasch, U., and Rouach, N. (2013). Emerging role for astroglial networks in information processing: from synapse to behavior. Trends Neurosci. 36, 405–417. doi: 10.1016/j.tins.2013.04.004
Pardridge, W. (2001). Brain Drug Targeting: The Future of Brain Drug Development. Cambridge: Cambridge University Press.
Park, D. Y., Lee, J., Kim, J., Kim, K., Hong, S., Han, S., et al. (2017). Plastic roles of pericytes in the blood–retinal barrier. Nat. Commun. 8:15296. doi: 10.1038/ncomms15296
Pierce, E. A., Avery, R. L., Foley, E. D., Aiello, L. P., and Smith, L. E. (1995). Vascular endothelial growth factor/vascular permeability factor expression in a mouse model of retinal neovascularization. Proc. Natl. Acad. Sci. U.S.A. 92, 905–909. doi: 10.1073/pnas.92.3.905
Pihlstrøm, L., Wiethoff, S., and Houlden, H. (2018). “Chapter 22 – Genetics of neurodegenerative diseases: an overview,” in Handbook of Clinical Neurology Neuropathology, eds G. G. Kovacs and I. Alafuzoff (Amsterdam: Elsevier), 309–323. doi: 10.1016/B978-0-12-802395-2.00022-5
Powell, C., Cornblath, E., Elsaeidi, F., Wan, J., and Goldman, D. (2016). Zebrafish Müller glia-derived progenitors are multipotent, exhibit proliferative biases and regenerate excess neurons. Sci. Rep. 6:24851. doi: 10.1038/srep24851
Powner, M. B., Gillies, M. C., Tretiach, M., Scott, A., Guymer, R. H., Hageman, G. S., et al. (2010a). Perifoveal Müller cell depletion in a case of macular telangiectasia type 2. Ophthalmology 117, 2407–2416.
Powner, M. B., Gillies, M. C., Zhu, M., Vevis, K., Hunyor, A. P., and Fruttiger, M. (2010b). Loss of Müller’s cells and photoreceptors in macular telangiectasia type 2. Ophthalmology 120, 2344–2352. doi: 10.1016/j.ophtha.2013.04.013
Price, B. R., Norris, C. M., Sompol, P., and Wilcock, D. M. (2018). An emerging role of astrocytes in vascular contributions to cognitive impairment and dementia. J. Neurochem. 144, 644–650. doi: 10.1111/jnc.14273
Puñal, V. M., Paisley, C. E., Brecha, F. S., Lee, M. A., Perelli, R. M., Wang, J., et al. (2019). Large-scale death of retinal astrocytes during normal development is non-apoptotic and implemented by microglia. PLoS Biol. 17:e3000492. doi: 10.1371/journal.pbio.3000492
Rakic, P. (2003). Elusive radial glial cells: historical and evolutionary perspective. Glia 43, 19–32. doi: 10.1002/glia.10244
Randlett, O., Wee, C. L., Naumann, E. A., Nnaemeka, O., Schoppik, D., Fitzgerald, J. E., et al. (2015). Whole-brain activity mapping onto a zebrafish brain atlas. Nat. Methods 12, 1039–1046. doi: 10.1038/nmeth.3581
Reichenbach, A., and Bringmann, A. (2020). Glia of the human retina. Glia 68, 768–796. doi: 10.1002/glia.23727
Richardson, R., Tracey-White, D., Webster, A., and Moosajee, M. (2017). The zebrafish eye—a paradigm for investigating human ocular genetics. Eye 31, 68–86. doi: 10.1038/eye.2016.198
Roesch, K., Jadhav, A. P., Trimarchi, J. M., Stadler, M. B., Roska, B., Sun, B. B., et al. (2008). The transcriptome of retinal Müller glial cells. J. Comp. Neurol. 509, 225–238. doi: 10.1002/cne.21730
Ronneberger, O., Liu, K., Rath, M., Ruess, D., Mueller, T., Skibbe, H., et al. (2012). ViBE-Z: a framework for 3D virtual colocalization analysis in zebrafish larval brains. Nat. Methods 9, 735–742. doi: 10.1038/nmeth.2076
Rosa, J. M., Bos, R., Sack, G. S., Fortuny, C., Agarwal, A., Bergles, D. E., et al. (2015). Neuron-glia signaling in developing retina mediated by neurotransmitter spillover. elife 4:e09590. doi: 10.7554/eLife.09590
Ross, J. M., Kim, C., Allen, D., Crouch, E. E., Narsinh, K., Cooke, D. L., et al. (2020). The expanding cell diversity of the brain vasculature. Front. Physiol. 11:600767. doi: 10.3389/fphys.2020.600767
Santello, M., Calì, C., and Bezzi, P. (2012). “Gliotransmission and the tripartite synapse,” in Synaptic Plasticity: Dynamics, Development and Disease Advances in Experimental Medicine and Biology, eds M. R. Kreutz and C. Sala (Vienna: Springer), 307–331. doi: 10.1007/978-3-7091-0932-8_14
Schousboe, A., Waagepetersen, H. S., and Sonnewald, U. (2019). Astrocytic pyruvate carboxylation: status after 35 years. J. Neurosci. Res. 97, 890–896. doi: 10.1002/jnr.24402
Senarathna, J., Yu, H., Deng, C., Zou, A. L., Issa, J. B., Hadjiabadi, D. H., et al. (2019). A miniature multi-contrast microscope for functional imaging in freely behaving animals. Nat. Commun. 10:99. doi: 10.1038/s41467-018-07926-z
Serrats, J., Schiltz, J. C., García-Bueno, B., van Rooijen, N., Reyes, T. M., and Sawchenko, P. E. (2010). Dual roles for perivascular macrophages in immune-to-brain signaling. Neuron 65, 94–106. doi: 10.1016/j.neuron.2009.11.032
Sharif, Y., Jumah, F., Coplan, L., Krosser, A., Sharif, K., and Tubbs, R. S. (2018). Blood brain barrier: a review of its anatomy and physiology in health and disease. Clin. Anat. 31, 812–823. doi: 10.1002/ca.23083
Sharma, S., Puttachary, S., and Thippeswamy, T. (2019). Glial source of nitric oxide in epileptogenesis: a target for disease modification in epilepsy. J. Neurosci. Res. 97, 1363–1377. doi: 10.1002/jnr.24205
Shekhar, K., Lapan, S. W., Whitney, I. E., Tran, N. M., Macosko, E. Z., Kowalczyk, M., et al. (2016). Comprehensive classification of retinal bipolar neurons by single-cell transcriptomics. Cell 166, 1308–1323.e30. doi: 10.1016/j.cell.2016.07.054
Sibille, J., Pannasch, U., and Rouach, N. (2014). Astroglial potassium clearance contributes to short-term plasticity of synaptically evoked currents at the tripartite synapse. J. Physiol. 592, 87–102. doi: 10.1113/jphysiol.2013.261735
Simard, M., and Nedergaard, M. (2004). The neurobiology of glia in the context of water and ion homeostasis. Neuroscience 129, 877–896. doi: 10.1016/j.neuroscience.2004.09.053
Singh, C., Tran, V., McCollum, L., Bolok, Y., Allan, K., Yuan, A., et al. (2020). Hyperoxia induces glutamine-fuelled anaplerosis in retinal Müller cells. Nat. Commun. 11:1277. doi: 10.1038/s41467-020-15066-6
Siqueira, M., Francis, D., Gisbert, D., Gomes, F. C. A., and Stipursky, J. (2018). Radial glia cells control angiogenesis in the developing cerebral cortex through TGF-β1 signaling. Mol. Neurobiol. 55, 3660–3675. doi: 10.1007/s12035-017-0557-8
Sofroniew, M. V., and Vinters, H. V. (2010). Astrocytes: biology and pathology. Acta Neuropathol. 119, 7–35. doi: 10.1007/s00401-009-0619-8
Someya, E., Akagawa, M., Mori, A., Morita, A., Yui, N., Asano, D., et al. (2019). Role of neuron–glia signaling in regulation of retinal vascular tone in rats. Int. J. Mol. Sci. 20:1952. doi: 10.3390/ijms20081952
Soto-Rojas, L. O., Pacheco-Herrero, M., Martínez-Gómez, P. A., Campa-Córdoba, B. B., Apátiga-Pérez, R., Villegas-Rojas, M. M., et al. (2021). The neurovascular unit dysfunction in Alzheimer’s disease. Int. J. Mol. Sci. 22:2022. doi: 10.3390/ijms22042022
Souza, D. G., Almeida, R. F., Souza, D. O., and Zimmer, E. R. (2019). The astrocyte biochemistry. Semin. Cell Dev. Biol. 95, 142–150. doi: 10.1016/j.semcdb.2019.04.002
Sperlágh, B., and Vizi, E. S. (2011). The role of extracellular adenosine in chemical neurotransmission in the hippocampus and basal ganglia: pharmacological and clinical aspects. Curr. Top. Med. Chem. 11, 1034–1046. doi: 10.2174/156802611795347564
Subirada, P. V., Paz, M. C., Ridano, M. E., Lorenc, V. E., Vaglienti, M. V., Barcelona, P. F., et al. (2018). A journey into the retina: Müller glia commanding survival and death. Eur. J. Neurosci. 47, 1429–1443. doi: 10.1111/ejn.13965
Sugita, Y., Miura, K., and Furukawa, T. (2020). Retinal ON and OFF pathways contribute to initial optokinetic responses with different temporal characteristics. Eur. J. Neurosci. 52, 3160–3165. doi: 10.1111/ejn.14697
Sweeney, M. D., Sagare, A. P., and Zlokovic, B. V. (2018). Blood–brain barrier breakdown in Alzheimer disease and other neurodegenerative disorders. Nat. Rev. Neurol. 14, 133–150. doi: 10.1038/nrneurol.2017.188
Tárnok, A. (2006). Slide-based cytometry for cytomics—a minireview. Cytometry A 69A, 555–562. doi: 10.1002/cyto.a.20317
Taylor, A. W., and Ng, T. F. (2018). Negative regulators that mediate ocular immune privilege. J. Leukoc. Biol. 103, 1179–1187. doi: 10.1002/JLB.3MIR0817-337R
Thakore, P., Alvarado, M. G., Ali, S., Mughal, A., Pires, P. W., Yamasaki, E., et al. (2021). Brain endothelial cell TRPA1 channels initiate neurovascular coupling. eLife 10:e63040. doi: 10.7554/eLife.63040
Than-Trong, E., and Bally-Cuif, L. (2015). Radial glia and neural progenitors in the adult zebrafish central nervous system. Glia 63, 1406–1428. doi: 10.1002/glia.22856
Thummel, R., Kassen, S. C., Enright, J. M., Nelson, C. M., Montgomery, J. E., and Hyde, D. R. (2008). Characterization of Müller glia and neuronal progenitors during adult zebrafish retinal regeneration. Exp. Eye Res. 87, 433–444. doi: 10.1016/j.exer.2008.07.009
Tong, L., Hill, R. A., Damisah, E. C., Murray, K. N., Yuan, P., Bordey, A., et al. (2021). Imaging and optogenetic modulation of vascular mural cells in the live brain. Nat. Protoc. 16, 472–496. doi: 10.1038/s41596-020-00425-w
Tout, S., Chan-Ling, T., Holländer, H., and Stone, J. (1993). The role of müller cells in the formation of the blood-retinal barrier. Neuroscience 55, 291–301. doi: 10.1016/0306-4522(93)90473-S
Tsai, H.-H., Li, H., Fuentealba, L. C., Molofsky, A. V., Taveira-Marques, R., Zhuang, H., et al. (2012). Regional astrocyte allocation regulates CNS synaptogenesis and repair. Science 337, 358–362. doi: 10.1126/science.1222381
Turko, P., Groberman, K., Browa, F., Cobb, S., and Vida, I. (2019). Differential dependence of GABAergic and glutamatergic neurons on glia for the establishment of synaptic transmission. Cereb. Cortex 29, 1230–1243. doi: 10.1093/cercor/bhy029
Turner, D. A., and Adamson, D. C. (2011). Neuronal-astrocyte metabolic interactions: understanding the transition into abnormal astrocytoma metabolism. J. Neuropathol. Exp. Neurol. 70, 167–176. doi: 10.1097/NEN.0b013e31820e1152
Vanlandewijck, M., He, L., Mäe, M. A., Andrae, J., Ando, K., Del Gaudio, F., et al. (2018). A molecular atlas of cell types and zonation in the brain vasculature. Nature 554, 475–480. doi: 10.1038/nature25739
Vecino, E., Rodriguez, F. D., Ruzafa, N., Pereiro, X., and Sharma, S. C. (2016). Glia–neuron interactions in the mammalian retina. Prog. Retin. Eye Res. 51, 1–40. doi: 10.1016/j.preteyeres.2015.06.003
Vergara, R. C., Jaramillo-Riveri, S., Luarte, A., Moënne-Loccoz, C., Fuentes, R., Couve, A., et al. (2019). The energy homeostasis principle: neuronal energy regulation drives local network dynamics generating behavior. Front. Comput. Neurosci. 13:49. doi: 10.3389/fncom.2019.00049
Verheijen, J., and Sleegers, K. (2018). Understanding Alzheimer disease at the interface between genetics and transcriptomics. Trends Genet. 34, 434–447. doi: 10.1016/j.tig.2018.02.007
Villaseñor, R., Lampe, J., Schwaninger, M., and Collin, L. (2019). Intracellular transport and regulation of transcytosis across the blood–brain barrier. Cell. Mol. Life Sci. 76, 1081–1092. doi: 10.1007/s00018-018-2982-x
Virchow, R. (1856). Gesammelte Abhandlungen zur Wissenschaftlischen Medizin. Frankfurt: Staatsdruckerei.
Virgintino, D., Errede, M., Robertson, D., Girolamo, F., Masciandaro, A., and Bertossi, M. (2003). VEGF expression is developmentally regulated during human brain angiogenesis. Histochem. Cell Biol. 119, 227–232. doi: 10.1007/s00418-003-0510-y
Wang, J., O’Sullivan, M. L., Mukherjee, D., Puñal, V. M., Farsiu, S., and Kay, J. N. (2017). Anatomy and spatial organization of Müller glia in mouse retina. J. Comp. Neurol. 525, 1759–1777. doi: 10.1002/cne.24153
Watson, P. M. D., Kavanagh, E., Allenby, G., and Vassey, M. (2017). Bioengineered 3D glial cell culture systems and applications for neurodegeneration and neuroinflammation. SLAS Discov. 22, 583–601. doi: 10.1177/2472555217691450
Welser, J. V., Li, L., and Milner, R. (2010). Microglial activation state exerts a biphasic influence on brain endothelial cell proliferation by regulating the balance of TNF and TGF-β1. J. Neuroinflammation 7:89. doi: 10.1186/1742-2094-7-89
Whiteus, C., Freitas, C., and Grutzendler, J. (2014). Perturbed neural activity disrupts cerebral angiogenesis during a postnatal critical period. Nature 505, 407–411. doi: 10.1038/nature12821
Willis, C. L. (2011). Glia-induced reversible disruption of blood–brain barrier integrity and neuropathological response of the neurovascular unit. Toxicol. Pathol. 39, 172–185. doi: 10.1177/0192623310385830
Wosik, K., Cayrol, R., Dodelet-Devillers, A., Berthelet, F., Bernard, M., Moumdjian, R., et al. (2007). Angiotensin II controls occludin function and is required for blood–brain barrier maintenance: relevance to multiple sclerosis. J. Neurosci. 27, 9032–9042. doi: 10.1523/JNEUROSCI.2088-07.2007
Yamasaki, M., Yamada, K., Furuya, S., Mitoma, J., Hirabayashi, Y., and Watanabe, M. (2001). 3-phosphoglycerate dehydrogenase, a key enzyme forl-serine biosynthesis, is preferentially expressed in the radial glia/astrocyte lineage and olfactory ensheathing glia in the mouse brain. J. Neurosci. 21, 7691–7704. doi: 10.1523/JNEUROSCI.21-19-07691.2001
Yoon, J.-H., and Jeong, Y. (2019). In vivo imaging for neurovascular disease research. Arch. Pharm. Res. 42, 263–273. doi: 10.1007/s12272-019-01128-x
Yuan, Y., Failmezger, H., Rueda, O. M., Ali, H. R., Gräf, S., Chin, S.-F., et al. (2012). Quantitative image analysis of cellular heterogeneity in breast tumors complements genomic profiling. Sci. Transl. Med. 4:157ra143. doi: 10.1126/scitranslmed.3004330
Zhang, R., Du, W., Prober, D. A., and Du, J. (2019). Müller glial cells participate in retinal waves via glutamate transporters and AMPA receptors. Cell Rep. 27, 2871–2880.e2. doi: 10.1016/j.celrep.2019.05.011
Zhang, T., Gillies, M. C., Madigan, M. C., Shen, W., Du, J., Grünert, U., et al. (2018). Disruption of de novo serine synthesis in Müller cells induced mitochondrial dysfunction and aggravated oxidative damage. Mol. Neurobiol. 55, 7025–7037. doi: 10.1007/s12035-017-0840-8
Zhang, T., Zhu, L., Madigan, M. C., Liu, W., Shen, W., Cherepanoff, S., et al. (2019). Human macular Müller cells rely more on serine biosynthesis to combat oxidative stress than those from the periphery. eLife 8:e43598. doi: 10.7554/eLife.43598
Zhang, Y., Chen, K., Sloan, S. A., Bennett, M. L., Scholze, A. R., O’Keeffe, S., et al. (2014). An RNA-sequencing transcriptome and splicing database of glia, neurons, and vascular cells of the cerebral cortex. J. Neurosci. 34, 11929–11947. doi: 10.1523/JNEUROSCI.1860-14.2014
Zhao, Y., Xin, Y., He, Z., and Hu, W. (2018). Function of connexins in the interaction between glial and vascular cells in the central nervous system and related neurological diseases. Neural Plast. 2018:6323901. doi: 10.1155/2018/6323901
Zhao, Z., Nelson, A. R., Betsholtz, C., and Zlokovic, B. V. (2015). Establishment and dysfunction of the blood-brain barrier. Cell 163, 1064–1078. doi: 10.1016/j.cell.2015.10.067
Zlokovic, B. V. (2005). Neurovascular mechanisms of Alzheimer’s neurodegeneration. Trends Neurosci. 28, 202–208. doi: 10.1016/j.tins.2005.02.001
Keywords: astrocytes, brain, Müller glia, neurovascular unit, retina
Citation: Kugler EC, Greenwood J and MacDonald RB (2021) The “Neuro-Glial-Vascular” Unit: The Role of Glia in Neurovascular Unit Formation and Dysfunction. Front. Cell Dev. Biol. 9:732820. doi: 10.3389/fcell.2021.732820
Received: 29 June 2021; Accepted: 01 September 2021;
Published: 27 September 2021.
Edited by:
Chunyi Wen, Hong Kong Polytechnic University, Hong Kong, SAR ChinaReviewed by:
Ruifang Sui, Peking Union Medical College Hospital (CAMS), ChinaLasse Dahl Ejby Jensen, Linköping University, Sweden
Copyright © 2021 Kugler, Greenwood and MacDonald. This is an open-access article distributed under the terms of the Creative Commons Attribution License (CC BY). The use, distribution or reproduction in other forums is permitted, provided the original author(s) and the copyright owner(s) are credited and that the original publication in this journal is cited, in accordance with accepted academic practice. No use, distribution or reproduction is permitted which does not comply with these terms.
*Correspondence: Ryan B. MacDonald, cnlhbi5tYWNkb25hbGRAdWNsLmFjLnVr