- Chemotaxis Signal Section, Laboratory of Immunogenetics, NIAID, NIH, Rockville, MD, United States
How phagocytes find invading microorganisms and eliminate pathogenic ones from human bodies is a fundamental question in the study of infectious diseases. About 2.5 billion years ago, eukaryotic unicellular organisms–protozoans–appeared and started to interact with various bacteria. Less than 1 billion years ago, multicellular animals–metazoans–appeared and acquired the ability to distinguish self from non-self and to remove harmful organisms from their bodies. Since then, animals have developed innate immunity in which specialized white-blood cells phagocytes- patrol the body to kill pathogenic bacteria. The social amoebae Dictyostelium discoideum are prototypical phagocytes that chase various bacteria via chemotaxis and consume them as food via phagocytosis. Studies of this genetically amendable organism have revealed evolutionarily conserved mechanisms underlying chemotaxis and phagocytosis and shed light on studies of phagocytes in mammals. In this review, we briefly summarize important studies that contribute to our current understanding of how phagocytes effectively find and kill pathogens via chemotaxis and phagocytosis.
Introduction
How eukaryotic cells interact with bacteria and viruses is a fundamental question in biology. Eukaryotic cells and their interactions with other life forms began when single-cell organisms such as protozoa appeared about 2.5 billion years ago. Since then, multicellular organisms with increasingly complex genomes have developed innate immune systems in which specialized phagocytic cells, such as neutrophils and macrophages in humans, patrol the body to detect, recognize and eliminate invading pathogens. Specialized eukaryotic cells that can recognize foreign objects by migrating toward them and engulfing them were discovered by Elie Metchnikoff in 1882 (Beck and Habicht, 1996). One day, he pierced the larva of a starfish with a rose thorn. When he examined the transparent larva the next day, he saw many cells covering the thorn and trying to engulf it. He imagined that these cells migrated from afar toward foreign invaders, a cellular process known as chemotaxis, and attempted to engulf the invaders, a cellular process known as phagocytosis. Both processes were also described in some specialized cells by others at the time. Engelmann T. W. (1881) and Pfeffer W. F. (1884) described chemotaxis of bacteria (Mc, 1946; Drews, 2005), and Leber T. (1888) discovered that leukocytes migrated in straight paths to sites of rabbit corneal irritation (Mc, 1946). Those observations gave rise to the hypothesis that diffusible substances guide the migration (chemotaxis) of those cells. Many reports of phagocytosis described the uptake of microorganisms, red cells, or various particles by cells in different animal systems. For example, Hayem (1870) and Klebs (1872) observed bacteria in white blood cells; Waldeyer (1876) found bacteria in peritoneal pus cells, Koch (1876) found anthrax bacilli in spleen and lymph node cells, and Osler (1875) reported that human alveolar cells engulfed coal dust particles (Ambrose, 2006). Metchnikoff proposed that recognizing foreign substances and engulfing them represents a fundamental mechanism by which organisms in the animal kingdom defend themselves against infections. Therefore, he created the discipline of cellular immunology, which is now called innate immunity.
It was generally believed that human phagocytes use at least two different types of receptors for defense against bacteria pathogens: one for detecting and chasing pathogens via chemotaxis and another for recognizing and engulfing them via phagocytosis. Detection and chasing are facilitated by chemoattractant G-protein-coupled-receptors (GPCRs) (Jin et al., 2008; Metzemaekers et al., 2020), whereas recognition and engulfing employ pattern-recognition receptors (PRRs), such as Toll-like receptors (TLRs), scavenger receptors, and C-type lectin receptors, as well as phagocytic receptors that include complement receptors and Fcγ receptors (Flannagan et al., 2012; Amarante-Mendes et al., 2018).
The social amoebae Dictyostelium discoideum inhabit soil and feed on diverse bacterial species, including gram-positive and gram-negative bacteria (Vogel et al., 1980; Gerisch, 1982; Devreotes and Zigmond, 1988; Soldati and Cardenal-Munoz, 2019). The amoebae locate bacteria by detecting metabolites, such as folic acid; move toward the bacteria via chemotaxis; then engulf the bacteria by recognizing their surface molecules and consume them through phagocytosis. Over the years, many molecular components involved in eukaryotic chemotaxis and phagocytosis have been discovered. Studies in D. discoideum have helped us to uncover molecular components and pathways and to develop new techniques. Importantly, molecular mechanisms are evolutionarily conserved allowing us to apply knowledge gained from the model organism to the study of chemotaxis and phagocytosis in human phagocytes. In this review, we will summarize landmark discoveries in phagocytic cells that shape our knowledge of innate immunity and highlight important studies in D. discoideum that provide novel insights to our current understanding of chemotaxis and phagocytosis.
Chemoattractants and Chemokines
The idea that eukaryotic cells can migrate in a gradient of diffusible substances and move toward their sources took root before 1900 (Mc, 1946). In 1882, Metchnikoff first noted that cells migrated from afar and covered a rose thorn inserted into the larva of a starfish (Beck and Habicht, 1996), and Leber (1888) discovered that leukocytes migrated in straight paths to sites of rabbit corneal irritation (Mc, 1946). Those observations gave rise to the hypothesis that diffusible substances generated by a foreign object or at the site of injury establish chemical gradients that guide cell migration.
It had been known for many years that some motile cells, including mammalian leukocytes and metazoan cells, move toward the sources of diffusible chemicals, but the chemical nature of the substances was not known and could be not be properly analyzed because experimental techniques did not yet exist. In 1962, Boyden reported a technique, now known as the Boyden chamber assay, to test for chemotactic activity of diffusible substances (Boyden, 1962). The first chemoattractant to be reported for eukaryotic cells was 3′5′-cyclic adenosine monophosphate (cAMP) for Dictyostelium discoideum (Table 1). In 1947, Bonner reported that the eukaryotic social amoebae, D. discoideum, migrate robustly toward the source of diffusible chemicals (Bonner, 1947). In 1969, it was discovered that cAMP is a chemoattractant for the social amoeba (Konijn et al., 1969). A couple of years later, the Bonner group found that the folic acid released from bacteria serves as another chemotactic substance used by D. discoideum cells to hunt their bacterial food (Pan et al., 1972). A chemotactic factor for leukocytes was found in the complement system of serum in 1968, later known as C5a (Snyderman et al., 1968). In 1975, N-formyl peptides, which are released from bacteria, were identified as chemoattractants for leukocytes (Schiffmann et al., 1975). By the early 1980s, the “classical” chemoattractants, including N-formyl peptides, C5a, and the lipid mediator leukotriene B4 (LTB4) (Borgeat et al., 1976; Fernandez et al., 1978), had been identified, and the cytokine-like-chemoattractants, chemokines, would be discovered in the coming years.
The chemokine field started with the cloning of the human gene encoding IL-8, also known as CXCL8 (Baggiolini, 2015). Several groups independently cloned the human gene encoding CXCL8 (Walz et al., 1987; Yoshimura et al., 1987). The amino acid sequences of CXCL8 and monocyte chemoattractant protein-1 (MCP-1, also known as CCL2) contained either CXC or CC, which led to the identification of the protein family of chemokines with at least two CXC or CC subgroups (Matsushima et al., 1989; Yoshimura et al., 1989). Interestingly, chemokine-like proteins, such as IP-10 (CXCL10) (Luster et al., 1985), LD78 (CCL3L1) (Obaru et al., 1986), and the mouse ortholog of human I-309 (CCL1) (Miller et al., 1989), had been identified several years before CXCL8. However, their chemoattractant activity remained unclear until the discovery of CXCL8.
By the early 1990s, the new field of chemokines took off. Today, we can easily identify genes encoding typical chemokines in genome sequences due to their size (about 70–90 amino acids) and conserved N-terminal, cysteine motifs (Murphy et al., 2000). Based on the number and spacing of cysteines in these motifs, chemokines have been classified into C, CC, CXC, and CX3C subfamilies. There are more than fifty chemokines that are produced by leukocytes and tissue cells. Some chemokines are involved in normal housekeeping functions, such as the maturation of leukocytes in the bone marrow, the trafficking and homing of lymphocytes, and the regeneration of circulating leukocytes, while others orchestrate inflammatory responses for the recruitment of immune cells to the inflamed regions. Interestingly, some chemokines have been shown to also play significant roles in cancer metastasis (Jin et al., 2008; Li et al., 2013).
Chemoattractant- and Chemokine-Receptors
The molecular identities of chemoattractant receptors for eukaryotic cells began to be revealed in the late 1980s when DNA cloning techniques became widely available for laboratories. The first breakthrough was the discovery of a cAMP chemoattractant receptor cAR1 in D. discoideum and the realization that it belongs to the family of G protein-coupled receptors (GPCRs) (Table 1), which contains seven transmembrane domains and (Klein et al., 1988). A couple of years later, the receptors for classical chemoattractants, such as fMLP and C5a, had been cloned and were found to be GPCRs (Boulay et al., 1990; Gerard and Gerard, 1991). Before the identification of the IL-8 (CXCL8) receptor, evidence suggested that the putative receptor would be a GPCR since activation of neutrophils by IL-8 (CXCL8) was shown to be inhibited by pertussis toxin, a drug blocking GPCR/Gi signaling. However, single transmembrane tyrosine kinase receptors, such as the receptor for platelet-derived growth factor (Williams, 1989), can mediate chemotaxis, therefore it was speculated that tyrosine kinase receptors could also detect other protein-ligands, such as chemokines, at the time.
In 1991, two back-to-back papers reported the discoveries of two GPCRs as IL-8 receptors (Holmes et al., 1991; Murphy and Tiffany, 1991). The two receptors are similar but not identical, sharing 77% amino acid identity. Each of them triggered transient Ca2+ responses upon IL-8 stimulation and had a high affinity for IL-8 (Kd = 2 nM). They were named IL-8RA and IL-8RB at the time and are now known as CXCR1 and CXCR2. Currently, about twenty chemokine GPCRs mediate signal events induced by more than 50 chemokines (Murphy et al., 2000). Interestingly, chemokines and their receptors, unlike other types of GPCRs, have overlapping specificities for each other, and many chemokine-receptor-mediated signals appear to be redundant. It became a paradigm that phagocytes use GPCRs to detect diffusible chemicals (both classical chemoattractants and chemokines) and to mediate signaling pathways that control the reorganization of the actin cytoskeleton for cell migration toward the sites of infection and pathogens via chemotaxis. Once reaching the pathogens, these phagocytes employ pattern-recognition receptors (PRRs) to recognize surface molecules of the pathogens and to mediate signaling pathways that regulate the reorganization of the actin cytoskeleton for the engulfment of particles via phagocytosis (Greenberg, 1995; Flannagan et al., 2012; Kaufmann and Dorhoi, 2016).
Phagocytosis and Opsonic- and Non-Opsonic Receptors
Phagocytosis was observed by Oster in 1875 and others, and later was studied and named by Metchnikoff in 1883 (Ambrose, 2006). Phagocytosis is a process of sensing and engulfing particles larger than 0.5 μm. The particle is internalized into an organelle, called a phagosome. The phagosome fuses with lysosomes to become a phagolysosome, which contains enzymes that can degrade the particle (Flannagan et al., 2012; Kaufmann and Dorhoi, 2016). Phagocytosis is a basic process for obtaining nutrition in unicellular organisms, such as free-living amoebae of D. discoideum (Dunn et al., 2017; King and Kay, 2019), and for the elimination of foreign microorganisms in multicellular organisms by a group of cells, such as neutrophils and macrophages in mammals.
Detection of the target particles is the first step of phagocytosis. In 1903, Wright and Douglas discovered that the uptake of staphylococci by human leukocytes is enhanced by serum (Wright et al., 1989). They concluded that blood fluids have an opsonic power that modifies bacteria to be ingested by the leukocytes. The idea that phagocytosis begins with pathogenic bacteria being labeled with opsonins–host-derived proteins that bind specific receptors on phagocytes–was widely accepted. Nowadays it is well known that the major opsonins promoting efficient phagocytosis by leukocytes are complement components and immunoglobulin G (IgG) antibodies (Uribe-Querol and Rosales, 2020). These opsonins and their receptors are the best studied.
Complement is a system of more than 30 proteins in the plasma and on the cell surface, and it constitutes more than 15 of the globular fractions of plasma (Dunkelberger and Song, 2010). The complement system comprises vital components of innate immunity and influences T- and B-cell biology and adaptive responses. A major role of complement in innate immunity is providing complement opsonins, such as C4b, C3b, and C3bi, to decorate the surface of microorganisms, and phagocytes recognize these marked microorganisms via the complement receptors (CRs), including CR1, CR2, CR3, and CR4, and then eliminate them via phagocytosis (Walport, 2001a,b; Dunkelberger and Song, 2010). IgG antibodies are another family of opsonins that bind to the surface of pathogenic microorganisms, and on human cells, IgG receptors are Fcγ receptors, such as FcγRI, FcγRII, and FcγRIII (Uribe-Querol and Rosales, 2020). Fcγ receptors specifically bind to the Fc part of IgG molecules to form clusters on the surface of phagocytes, and these clusters then trigger phagocytosis and other cellular responses.
Janeway Jr. (1989) proposed that innate immune cells must express receptors that recognize specific molecules on foreign microorganisms. Since then, receptors directly recognizing pathogen-associated-molecular-patterns (PAMPs) have been discovered, and these so-called pattern recognition receptors (PRRs) are proteins capable of binding to molecules associated with pathogens (Walsh et al., 2013; Amarante-Mendes et al., 2018). PRRs are found associated with different subcellular compartments, such as on cellular and endosomal membranes, in the cytosol, and the bloodstream as the secreted forms. Four main classes of PRRs are recognized, including the Toll-like receptors (TLRs), the nucleotide-binding oligomerization domain (NOD)-leucine-rich-repeats (LRRs)-containing receptors (NLRs), the retinoic acid-inducible gene 1 (RIG-1)-like receptors (RLRs), and the C-type lectin receptors (CLRs). Some of them, such as TLRs, can bind to PAMPs but cannot induce phagocytosis, while others, such as some CLRs (Dectin-1, Dectin-3, and DC-SIGN) can induce phagocytosis; the latter type is called non-opsonic receptors.
When a particle is recognized by either opsonic- or non-opsonic receptors, many signaling pathways in phagocytic cells are activated, resulting in reorganization of the actin cytoskeleton and the formation of a phagocytic cup around the particle for engulfment. Opsonic- and non-opsonic receptors, unlike chemoattractant GPCRs that have seven transmembrane domains, are single transmembrane proteins with extracellular ligand-binding domains and intracellular signaling domains (Greenberg, 1995; Greenberg and Grinstein, 2002; Flannagan et al., 2012). Activation of these receptors mediates phosphorylation of tyrosine residues on the intracellular domain that induces signaling events that lead to the rearrangement of the actin cytoskeleton for the engulfment of particles. It appeared that chemotaxis and phagocytosis are two distinct cellular processes that are sequentially connected by phagocytes during the fight against pathogens. Studies of D. discoideum suggest that chemotaxis and phagocytosis may be originated from one cellular process in ancient phagocytes.
Chemotaxis of D. discoideum and Leukocytes
Before 1900, it was known that leukocytes migrated in straight paths to inflamed sites of rabbit corneal irritation (Mc, 1946). Leukocytes are amoeboid cells, and their movement closely resembles that of the amoebae of D. discoideum (Devreotes and Zigmond, 1988). In the 1970s, Gerisch used time-lapse microscopy to directly observe the behaviors of D. discoideum cells in response to cAMP stimuli (Gerisch et al., 1975). In response to a uniform cAMP increase, the cells became elongated and polarized, with clear leading fronts and trailing ends, and their random motility increased, a behavior termed chemokinesis. When placed in a cAMP gradient, the cells instantly migrated toward the source of cAMP by extending pseudopods (leading fronts) followed by retraction of the trailing ends (Gerisch et al., 1975; Gerisch, 1982; Swanson and Taylor, 1982). Zigmond observed movements of individual leukocytes in response to chemical gradients using time-lapse microscopy (Zigmond, 1974, 1978). Together, the studies of D. discoideum and leukocytes showed that these amoeboid cells can initiate locomotion by extending pseudopods in the direction of a higher concentration of chemoattractant (Devreotes and Zigmond, 1988).
Based on the observations, two different models have been proposed to explain how cells measure gradients (Parent and Devreotes, 1999; Rappel et al., 2002). In the first model, a cell detects changes in chemical concentration over time only and adjusts its movement in the chemical gradient based on the change in concentration it detects as it moves (Berg, 1975). Bacteria employ this temporal mechanism of chemotaxis (Berg, 1975). In the second model, a cell simultaneously compares differences in chemoattractant concentration around its perimeter, detecting spatial changes (Parent and Devreotes, 1999). Using this spatial mechanism, a cell does not need to move before judging the direction of a gradient. Zigmond proposed that amoeboid cells, unlike bacteria, utilize a spatial sensing mechanism to detect a chemical gradient and to guide their movement (Zigmond, 1974). Since then, the model system D. discoideum has been used to raise fundamental questions in eukaryotic chemotaxis, propose hypotheses of gradient sensing, discover essential components involved in chemotaxis, and develop techniques to study chemotaxis.
A GPCR-Mediated Signaling Network for Eukaryotic Chemotaxis
The receptor for chemoattractant cAMP in D. discoideum was discovered as a GPCR in 1988 (Klein et al., 1988). In the ensuing years, receptors for fMLP, C5a, and IL-8 (CXCL8) in human leukocytes were also found to be members of the GPCR family (Boulay et al., 1990; Gerard and Gerard, 1991; Holmes et al., 1991; Murphy and Tiffany, 1991). These findings established the paradigm that chemoattractants of eukaryotic cells are detected by GPCRs. Many components involved in chemotaxis were discovered in D. discoideum, and their homologs were then found to play similar roles in the chemotaxis of leukocytes.
Over the years, pathways involved in the cAMP receptor (cAR1)-mediated cell migration have been identified in D. discoideum (Figure 1). The binding of cAMP to cAR1 induces dissociation (activation) of heterotrimeric G proteins into Gα2 and Gβγ subunits (Firtel et al., 1989; Janetopoulos et al., 2001; Ueda et al., 2001), which, in turn, activate downstream signaling components to control the reorganization of the actin cytoskeleton for chemotaxis (Gerisch et al., 1993). Free Gβγ subunits activate small G protein Ras proteins (Sasaki et al., 2004; Charest et al., 2010; Kortholt et al., 2011; van Haastert et al., 2017), which activate PI3K to convert PIP2 to PIP3 (Sasaki et al., 2004). Activation of cAR1 also induces a transient membrane dissociation of PTEN and then a reassociation of PTEN to the trailing back of the cell (Funamoto et al., 2002; Iijima and Devreotes, 2002). PTEN dephosphorylates PIP3 and converts it to PIP2, and local PIP3 levels on the membrane provide an intracellular cue to recruit proteins containing pleckstrin homology (PH) domains from the cytosol to the membrane (Funamoto et al., 2002; Iijima and Devreotes, 2002; Matsuoka and Ueda, 2018). The recruited proteins include cytosolic regulator of adenylyl cyclase (CRAC) (Insall et al., 1994; Parent et al., 1998), Akt/PKB (Meili et al., 1999), myosin I (Chen et al., 2012), and Leep1 (Yang et al., 2021), each of which plays a role in regulating the reorganization of the actin cytoskeleton. It is still not clear how CRAC and Akt/PKB binding to PIP3 regulate the actin cytoskeleton. PIP3 binding proteins of myosin I, an actin-based motor protein, and Leep1 promote actin polymerization at the leading front of a migrating cell. Three myosin-I proteins, myosin ID, IE, and IF, bind to PIP3 and therefore are recruited to the leading edge of a cell to promote actin-polymerization for chemotaxis (Chen et al., 2012). Recently, it was found that Leep1 interacts with PIP3 and the Scar/WAVE complex to control the actin cytoskeleton at the leading edge of a migrating cell (Yang et al., 2021). In addition, several PIP3-independent pathways have been implicated in the chemotaxis of D. discoideum. One pathway is that the activation of G proteins mediates cGMP signaling that controls myosin phosphorylation and chemotaxis (Bosgraaf and Van Haastert, 2002; Kortholt et al., 2011). The second one is a GPCR-mediated mTORC2 and AKT signaling (Charest et al., 2010). Upon the activation of cAR1, the small G-protein Ras is activated and becomes Ras-GTP, and the small G-protein RacE is phosphorylated by GSK3. Ras-GTP, RacE-P, and mTORC2 form a signaling complex to promote AKT phosphorylation, which regulates cell migration (Senoo et al., 2019). In addition, activation of the small G-proteins RasC/G and Rap1 transduces signals to mTOR2 for chemotaxis (Khanna et al., 2016; Tariqul Islam et al., 2019). The fourth pathway is that the free Gβγ subunit associates with an ELMO/Dock complex to activate Rac leading to actin polymerization for cell migration (Yan et al., 2012). Finally, activation of cAR1 induces the phosphorylation of Erk2 that mediates signaling for both chemotaxis and phagocytosis (Hadwiger and Nguyen, 2011; Schwebs et al., 2018; Nichols et al., 2019; Tariqul Islam et al., 2019).
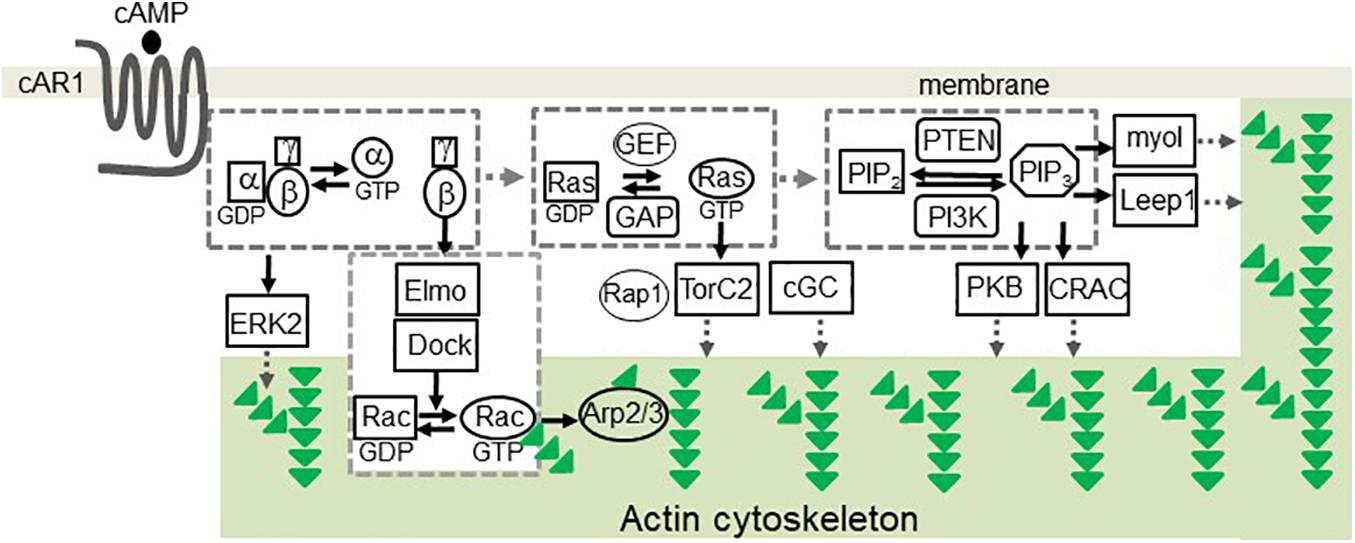
Figure 1. cAR1-mediated signaling pathways involved in chemotaxis in Dictyostelium discoideum. The binding of cAMP to cAR1 triggered signaling events leading to the reorganization of the actin cytoskeleton. The events include the dissociation of heterotrimeric G protein into Gα and Gβγ subunits, the activation of Ras, PIP3 production. PIP3 recruits signaling components, including PKB, CRAC, myoI, and Leep1, to transduce signals. Activation of heterotrimeric G-proteins activates Erk2 signaling to mediate chemotaxis and phagocytosis. Free Gβγs associate with the Elmo/Dock complex to activate Rac. Ras-GTP activates Rap1, TorC2 complex, and cGC. These signaling events regulate the actin cytoskeleton for cell migration. Green triangles represent G-actin molecules.
Chemoattractant GPCR-mediated signaling mechanisms for the regulation of chemotaxis have also been studied in leukocytes. Many molecular mechanisms involved in GPCR-mediated chemotaxis are evolutionarily conserved in D. discoideum and mammalian cells. Activation of GPCRs by chemoattractants triggers dissociation (activation) of heterotrimeric Gi proteins into Gαi and Gβγ subunits (Neptune and Bourne, 1997), which control several signaling pathways. Activation of Gi-proteins produces free Gβγ that activates PI3Ks to generate PIP3, regulates mTORC2, and produces active forms of small G-proteins (Servant et al., 2000; Suire et al., 2006; Liu et al., 2010), including Ras and Rac, to regulate the reorganization of the actin cytoskeleton. Leukocytes express several PIP3 phosphatases, such as PTEN and SHIP, that are involved in the regulation of chemotaxis (Nishio et al., 2007; Heit et al., 2008). In addition, a pathway consisting of CXCR4/Gi, ELMO1/Dock180, and Rac appears to play a role in CXCL12-mediated chemotaxis and metastasis of breast cancer cells (Li et al., 2013). Another GPCR-mediated pathway of PLC, PKC, and PKD is involved in the regulation of chemotaxis of neutrophils (Xu et al., 2015).
Chemotaxing cells, including D. discoideum and neutrophils, are morphologically polarized with leading pseudopods and trailing ends (Devreotes and Zigmond, 1988). Many components involved in chemotaxis are asymmetrically distributed in these polarized amoebae (Parent and Weiner, 2013). Eukaryotic chemotaxis is a complicated cell behavior that conceptually consists of three interconnected cellular processes: gradient sensing, cell polarization, and cell motility. Advances in fluorescence microscopy have allowed us to visualize subcellular localization of cAR1 and other signaling components in live D. discoideum cells since the 1990s. These studies shed new light on the question of how eukaryotic cells generate directional biochemical responses in response to a chemical gradient.
Gradient Sensing
Over the years, researchers have proposed different models, which involve either a temporal-sensing or a spatial-sensing mechanism (Zigmond, 1974; Parent and Devreotes, 1999; Rappel et al., 2002), to explain how eukaryotic cells detect and respond to chemical gradients. Many concepts have been developed and tested in the studies of D. discoideum chemotaxis. For example, one early hypothesis was that the accumulation of chemokine receptors at the front of a cell is essential for chemotaxis (Nieto et al., 1997). In 1997, cAR1 receptors were shown to be uniformly distributed on the membrane of chemotaxing D. discoideum cells (Xiao et al., 1997), and later, chemokine receptors were found to be the same on the surface of migrating neutrophils (Servant et al., 2000). In the ensuing years, studies showed that the D. discoideum cells can detect cAMP gradients to generate polarized responses even when cAR1 and its G-proteins are uninformedly distributed on the membrane (Parent et al., 1998; Jin et al., 2000). With the development of live-cell fluorescence microscopy, the spatiotemporal dynamics of key signaling events could be visualized (Gerisch and Muller-Taubenberger, 2003; Miyanaga et al., 2018). One breakthrough was the discovery that the chemoattractant sensing machinery works in cells without polarity and mobility (Parent et al., 1998). Specifically, D. discoideum cells become non-polar and immobile upon treatment with latrunculin, an inhibitor of actin polymerization. In latrunculin-treated cells, cAR1 and G proteins are uniformly distributed around the cell surface and PHcrac-GFP, a fluorescent probe for PIP3, is uniformly distributed in the cytosol (Parent et al., 1998; Jin et al., 2000; Matsuoka et al., 2006). When suddenly exposed to a cAMP gradient, a cell responds by initially inducing a transient PIP3 increase followed by a decrease around the membrane (a temporal adaptation) and producing a highly polarized distribution of PIP3 (a spatial amplification) in less than 2 min (Xu et al., 2005). During the application process, the membrane-bound PTENs gradually translocate from the front to the back (Iijima and Devreotes, 2002; Xu et al., 2007). The cAR1/G protein-controlled PIP3 responses display the key features of a spatial mechanism of gradient sensing. To truly understand gradient sensing mechanisms in chemotaxis, it is necessary to identify all regulatory components and to connect molecular mechanisms in a signaling network with a complexity that reflects that of chemotactic cells.
Over the last 20 years, many models have been proposed to explain GPCR-mediated gradient sensing and chemotaxis of eukaryotic cells (Parent and Devreotes, 1999; Postma and Van Haastert, 2001; Rappel et al., 2002; Van Haastert and Devreotes, 2004; Levine et al., 2006; Meier-Schellersheim et al., 2006; Iglesias and Devreotes, 2012; Takeda et al., 2012; Nakajima et al., 2014; Cheng and Othmer, 2016; Li et al., 2020). Most models were constructed by writing mathematical equations “by hand,” which is defining each reaction for molecule complex formation, association/de-association, or enzymatic transformation separately, and the tasks are time-consuming and very difficult due to a large number of reaction equations. It is almost impossible for experimental biologists to complete the tasks by themselves. The computer software packages have been developed and improved to allow researchers to construct signaling networks and to run simulations without dealing with mathematical equations. We hope that the software will be widely used by biologists to develop and analyze computational models that parallel live-cell experiments without writing reaction equations themselves, so more researchers can construct, examine, and validate their models. Future studies are needed to identify missing links and to understand how these components interact in a signaling network in time and space in a cell to achieve gradient sensing and chemotaxis.
Signal Relay and Self-Generated Gradients
How eukaryotic cells migrate over long distances toward the source of chemoattractants is an interesting question. Eukaryotic cells guide their movement by spatially detecting chemical gradients. Because diffusible gradients diminish quickly over a distance, eukaryotic cells have developed systems to generate signals and move collectively as a group of cells. This process has been studied in D. discoideum. D. discoideum amoebae ingest bacteria as food in the soil. When food is depleted, D. discoideum cells stop dividing and enter a developmental process during which individual cells aggregate and form multi-cellular structures (Devreotes, 1994). Within a few hours following starvation, a few cells spontaneously secrete cAMP. cAMP acts as a chemoattractant binding to cAR1 that leads to the dissociation of the heterotrimeric G-protein into Gα2 and Gβγ and the activation of the adenylyl cyclase A(ACA) that converts ATP into cAMP, most of which is secreted to relay chemotactic signals to neighboring cells. These cells respond to cAMP stimuli by moving toward the center (chemotaxis) and secreting additional cAMP (signal relay). The net result is that cAMP waves spread outward through a population of 106 cells as the cells moving toward the center. The cells migrate collectively toward secreted cAMPs in a head-to-tail fashion (streaming) and form an aggregation territory of about 0.5–1 cm in radius. It was found that ACA is not required for chemotaxis of individual cells, but ACA, specifically its asymmetric localization, is essential for cells to align head-to-tail in a stream and to migrate together as a group into aggregates (Kriebel et al., 2003).
A molecular system to relay chemotactic signals was discovered in neutrophils. Neutrophils are recruited to the site of an infection by primary chemoattractants, such as fMLP and C5a (Kolaczkowska and Kubes, 2013). After reaching the site, neutrophils respond to primary chemoattractants to produce and secrete secondary chemoattractants and recruit additional leukocytes to fight infection. A study showed that in response to an fMLP gradient, some neutrophils become polarized and migrate directionally, and they also release the secondary chemoattractant LTB4 (Afonso et al., 2012). All neutrophils, including those that have not yet responded to fMLP, respond to LTB4 and migrate toward the source of fMLP, indicating that LTB4 acts as a signal-relay molecule for neutrophils migrating collectively toward infection sites (Afonso et al., 2012; Lammermann et al., 2013). Interestingly, a recent study reported that D. discoideum cells can create chemoattractant gradients by degrading cAMP molecules and can achieve long-range chemotaxis by following these self-generated gradients (Tweedy et al., 2020). Mammalian cells may have systems to degrade attractants and make gradients for long-range migration toward the target sites.
Signaling Events in Phagocytosis
Once they reach the infection sites, leukocytes catch and eliminate pathogenic bacteria via phagocytosis. Phagocytosis consists of several phases. (1) Detection of the particles to be ingested. Phagocytic receptors recognize specific molecules on the surface of bacteria. (2) Activation of internalization. Ligands binding to the receptors induce the reorganization of the actin cytoskeleton, which mediates the formation of a phagocytic cup to engulf the bacteria. (3) Formation of the phagosome, a specialized vacuole. The membrane protrusions of a phagocytic cup cover the particle and fuse at the distal ends creating a new vacuole containing the engulfed particle that then separates from the plasma membrane. (4) Maturation of the phagosome into a phagolysosome. The new phagosomes fuse with early endosomes and late endosomes that have accumulated V-ATPase and lysosomal-associated proteins on the membrane and luminal proteases; and then fuse with lysosomes to become phagolysosomes, where the ingested bacteria are degraded (Amulic et al., 2012; Flannagan et al., 2012; Buckley et al., 2019). This review will focus mostly on phases 1 and 2, which share similarities with chemotaxis, and will not cover phases 3 and 4 and instead recommend two excellent reviews (Boulais et al., 2010; Dunn et al., 2017).
Phagocytosis begins when a particle interacts with phagocytic receptors. The activation of these receptors initiates intracellular signaling pathways that lead to the reorganization of the actin cytoskeleton, the extension of pseudopods, and the engulfment of the particles. In leukocytes, phagocytic receptor-mediated signaling events include activation of tyrosine kinases and PI3K that regulate the actin cytoskeleton (Greenberg, 1995; Underhill and Ozinsky, 2002; Uribe-Querol and Rosales, 2020). For Fcγ receptors, the initial intracellular signaling event is phosphorylation of tyrosine residuals of the receptors and association of the receptors containing immunoreceptor tyrosine-based activating motifs (ITAMs) with members of the Src family. The phosphorylated receptors and ITAMs serve as docking sites for tyrosine kinases, such as Syk, to activate downstream components leading to the reorganization of actin cytoskeleton for the engulfment of the particles. Activation of the Fcγ receptor also promotes PI3K activities that contribute to the engulfment. As mentioned earlier, PI3Ks may regulate actin cytoskeleton for pseudopod extensions for both cell migration and particle ingestion. For a long time, it was generally thought that phagocytes use GPCRs to detect soluble chemoattractants derived from infection sites and to migrate toward bacteria, and then switch to phagocytic receptors to bind the bacteria for phagocytosis. The social amoebae D. discoideum, which detect folic acid released from bacteria and pursue them via chemotaxis and consume them through phagocytosis, have a simpler solution.
Dictyostelium discoideum cells do not encode orthologs of any known phagocytic receptors (non-opsonic- or opsonic-receptors) or tyrosine kinases (Goldberg et al., 2006), yet they are highly evolved as professional phagocytes (Dunn et al., 2017; King and Kay, 2019). In the 1990s, one study showed that a heterotrimeric G protein β subunit is required for both chemotaxis and phagocytosis (Peracino et al., 1998). Two other studies reported that either diffusible cAMPs or one yeast particle induced the accumulation of coronin, an actin-associated protein, in a leading pseudopod for either cell migration or the formation of a phagocytic cup to engulf the yeast (Gerisch et al., 1995; Maniak et al., 1995). These studies suggested heterotrimeric G-proteins are involved in signal transduction for the reorganization of actin cytoskeleton for both chemotaxis and phagocytosis. More than two decades later, it was discovered that D. discoideum cells utilize a GPCR (fAR1) linking to heterotrimeric G proteins to detect both diffusible folic acids released from bacteria and lipopolysaccharide (LPS) on the surface of the bacteria (Pan et al., 2016, 2018). Thus, this simple organism uses one GPCR/G protein machinery to regulate a signaling network for both “chemoattractant-mediated cell migration” to chase bacteria and “microbe-associated molecular pattern (MAMP)-mediated engulfment” to ingest bacteria. Interestingly, recent studies have suggested that neutrophils can utilize the chemoattractant GPCR/Gi signaling to mediate (Dunn et al., 2017) not only cell migration but also particle ingestion to fight against invading bacteria, indicating that mammalian phagocytes retain this mechanism to chase and interact with bacteria through the evolution (Wood Jr., Smith and Watson, 1946; Osei-Owusu et al., 2019; Wen et al., 2019).
A Class C GPCR Is a New Members of Pattern Recognition Receptors
Dictyostelium discoideum amoebae utilize fAR1 and cognate G-proteins to detect folate for chasing bacteria and the immobile LPS on the bacterial surface for engulfing them (Figure 2). fAR1 contains a Venus-Flytrap (VFT) extracellular domain and belongs to the class C GPCR family (Pan et al., 2018). More than 800 human GPCRs have been identified so far and are classified into five main families: class A, which includes chemokine receptors, class B1, class B2, class C, and class F (Fredriksson et al., 2003; Foord et al., 2005). Based on phylogenetic sequence analysis and structure similarity, cAMP receptors (cAR1-cAR4) in D. discoideum constitute their own class (Saxe III, Johnson et al., 1991; Ginsburg et al., 1995; Moran et al., 2016), while fAR1 is a member of the class C family.
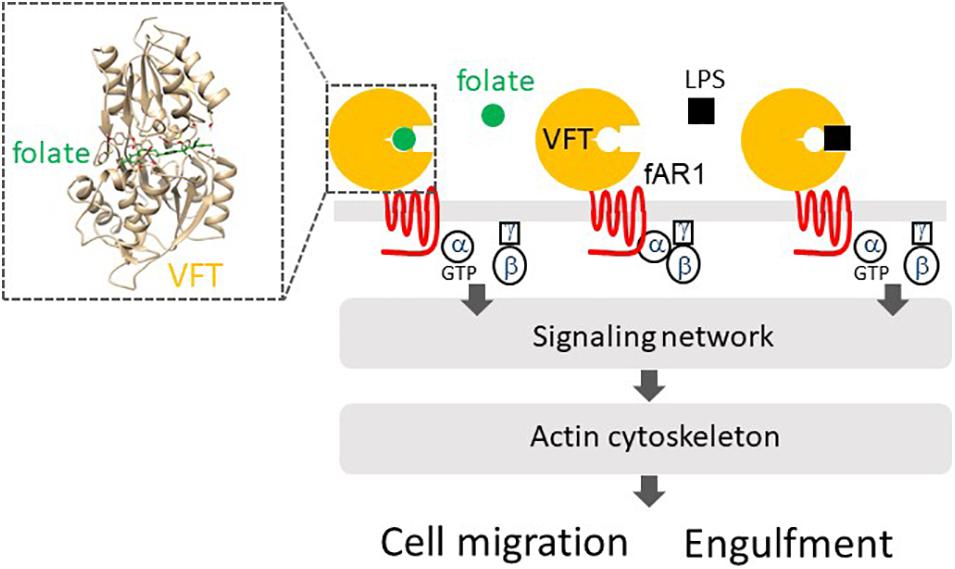
Figure 2. fAR1 binds to folate or LPS to activate heterotrimeric G-proteins that mediate a signaling network, which regulates the actin cytoskeleton for cell migration or particle engulfment. fAR1 contains a Venus-flytrap domain (VFT) for ligand binding. On the right box, Structural modeling and computational docking predict the extracellular domain of fAR1 folding into a VFT structure as the binding site for folate (green).
Class C GPCRs have a common structure consisting of an N-terminal extracellular Venus-flytrap (VFT) domain for ligand recognition and binding (Cao et al., 2009). VFT architecture is a typical structure of bacterial periplasmic binding proteins (PBPs) that sense small molecules and transport them into the cytoplasm (Borrok et al., 2009). A PBP consists of two globular domains connected by a small hinge region and exists in an open form in the absence of a ligand. The binding of a ligand induces a dramatic conformational change from an open to a close form, in which the ligand is clamped between the two lobes just like the carnivorous plant Venus-flytrap closing itself when a bug moving into the trap (Borrok et al., 2009). The class C GPCRs in humans are mainly composed of metabotropic glutamate receptors (mGluRs), gamma aminobutyric acid type B receptors (GABABRs), Ca2+-sensing receptors (CaSRs), taste receptors (T1Rs), pheromone receptors, and olfactory receptors (Cao et al., 2009). D. discoideum genome encodes 14 class C GPCRs, including fAR1 (Prabhu and Eichinger, 2006; Fey et al., 2013). The VFT domains of class C GPCRs in D. discoideum are closers to bacterial PBPs than those of human class C GPCRs (Cao et al., 2009). Interestingly, a bacterial PBP binds multiple ligands, while most human class C GPCRs expressed in the central nerve system bind only one natural ligand (Cao et al., 2009). However, the VFT in fAR1 retains the ability to recognize different molecules: folate released by bacteria and the sugar part of LPS (Figure 2), which is a major microbe-associated-molecular-pattern (MAMP) on the gram-negative bacteria (Pan et al., 2018). It appears is that the fusion of a PBP and an ancestral rhodopsin-like receptor (a class A GPCR) formed the common ancestor of the class C GPCRs, and fAR1 represents a prototype of class C GPCRs and is a new member of PRRs. Future studies will reveal the receptors of D. discoideum that recognize gram-positive bacteria and commune them as food.
Human Leukocytes Acquired Features for Fighting Foreign Pathogens
Chemotaxis and phagocytosis can be observed in amoeboid cells from many eukaryotic organisms, including D. discoideum, Caenorhabditis elegans, fruit fly, sea urchin, zebrafish, frog, chicken, mouse, and human. Some molecular components involved in these two processes are found in the early life forms, such as protozoans that go back about 2.5 billion years. Other components are unique to higher vertebrates but bear similarities to those of invertebrates (Figure 3). Collectively, these links represent compelling evidence that human leukocytes evolved from ancient life forms and acquired new features over a billion years.
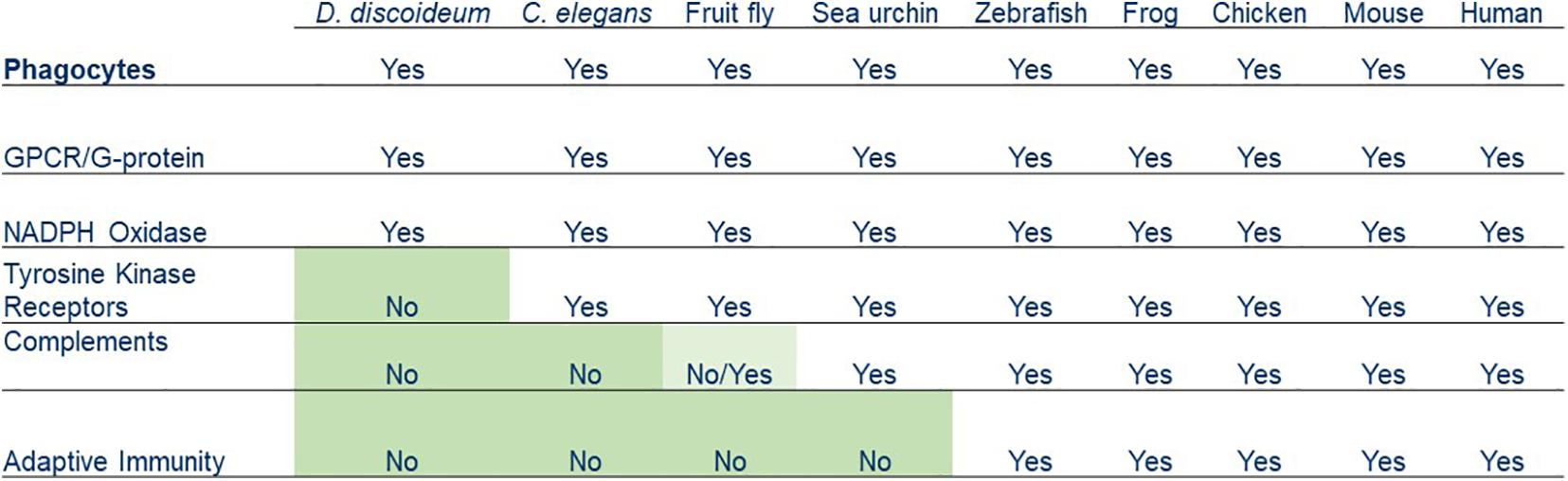
Figure 3. Summary of various phagocytosis components that have been acquired by phagocytes from simple organisms to humans.
The major components involved in GPCR-mediated chemotaxis are evolutionarily conserved from D. discoideum to human cells. Earlier we have briefly mentioned the molecular components involved in a GPCR-mediated signaling network of eukaryotic chemotaxis. We know that the molecular components of the actin cytoskeleton and their regulators are evolutionarily conserved from amoebae of simple organisms to human leukocytes. We will not review these components and regulators of the actin cytoskeleton and instead recommend several outstanding reviews (Pollard and Borisy, 2003; Insall and Machesky, 2009; Devreotes and Horwitz, 2015).
Dictyostelium discoideum phagocytosis is not very different from humans (Dunn et al., 2017; King and Kay, 2019). After binding to a particle, amoebae of D. discoideum perform the internalization, formation of the phagosome, and maturation of the phagosome into phagolysosome. The D. discoideum genome encodes evolutionarily conserved components required for these processes, including particle internalization, phagosome formation, and maturation. For example, the D. discoideum genome encodes catalytic NOX (NADPH oxidase) subunits, which are homologs of NOX2 in humans. NOX2 generates superoxide by transferring an electron from cytosolic NADPH to O2 in the lumen of the phagosome. Reactions convert superoxide into reactive oxygen species (ROS), which contribute to the killing of internalized bacteria. D. discoideum and mammalian phagocytes use conserved strategies to kill bacteria. They both have V-ATPase for phagosome acidification. In addition, their phagosome acquires a series of proteases, hydrolases, and lysozymes to break down bacterial components. Interestingly, D. discoideum has a primitive innate immune system. In the multicellular slug stage, specialized phagocytic cells, sentinel (S) cells, circulate within the slug to trap, engulf, and kill bacteria. Recent studies revealed that S cells use Toll/interleukin-1 receptor (TIR) domain protein, TirA, to recognize bacteria, produce extracellular traps (ETs) and reactive oxygen species to kill bacteria in the slug of D. discoideum (Chen et al., 2007; Zhang et al., 2016).
Several features found in leukocytes for pathogen recognition are missing from the primitive phagocytes of D. discoideum. The D. discoideum genome does not encode complements, IgGs, or phagocytic receptors, such as tyrosine kinases/tyrosine kinase receptors (Goldberg et al., 2006; Cosson and Soldati, 2008). D. discoideum amoebae utilize a GPCR/G-protein machinery and signaling pathways for recognizing both the chemoattractant folic acid and a phagocytic cue LPS for chasing and ingesting Klebsiella aerogenes (Pan et al., 2016, 2018). Recent reports suggested that D. discoideum encodes lectins and the scavenger receptor, LmpB (Dinh et al., 2018; Sattler et al., 2018), but it is not clear how (or whether) they form a functional signaling pathway to mediate phagocytosis. Caenorhabditis elegans does not encode complements or adaptive immune system but expresses tyrosine kinases that have the potential to associate with scavenger receptors for phagocytosis (Stuart and Ezekowitz, 2008). Animals ranging from sea urchins to humans have a version of the complement system, and vertebrates from fish to humans have both a complement system and adaptive immunity. Since phagocytes from all organisms have chemoattractant GPCR/G-protein machinery, we propose that the GPCR-mediated signaling network is an ancient system for regulating the actin cytoskeleton for both chemotaxis and the engulfment of particles during phagocytosis. The molecular machinery required for phagosome formation and maturation also existed in ancient phagocytes. Although having the ability to distinguish of self from non-self, the simpler machinery of an ancient phagocyte might not work sufficiently when multicellular organisms encounter more complicated situations. New features were acquired by phagocytes of multicellular organisms to fight against various foreign microorganisms during the evolution. A receptor system that links to tyrosine kinases was added in C. elegans, a complement system was added in sea urchins and retained in animals with a fluid-filled body cavity or a circulatory system, and adaptive immunity was then acquired by vertebrates as the latest feature. Human phagocytes have evolved by retaining early features and acquiring new ones that have gradually increased the complexity of their machinery and enhanced their ability to fight foreign pathogens in different situations.
Pathogens Hijack Receptors on Immune Cells
Throughout their long co-evolution with their eukaryotic hosts, infectious pathogens, such as bacteria and viruses, have developed strategies for evading and (or) invading the immune system (Alcami, 2003). For example, Yersinia pestis, the bacterial agent of plague, selectively kills immune cells, enabling its multiplication and systemic spread (Osei-Owusu et al., 2019). The plague receptor on human immune cells is FPR1, a chemoattractant GPCR of N-formyl peptides released from the bacteria. Once it has attracted immune cells, Y. pestis uses its cap protein in the type III secretion system (T3SS) to bind FPR1 and then delivers effector proteins to the host immune cells. The injected effector proteins inhibit host cell signaling and activate apoptosis of these host cells.
Many viruses have also exploited chemokine receptor systems for their entry and replication in the host cells (Alcami, 2003; Lisco et al., 2007). For example, the binding of the HIV envelope proteins to CXCR4 or CCR5 triggers membrane fusion and signal transduction and promotes the entry of HIV into T cells or macrophages (Berger et al., 1999). A previous study showed that HIV-mediated CXCR4/Gi signaling activates cofilin, which regulates actin dynamics and is known to be involved in cell migration, to facilitate remodeling of the actin cytoskeleton for viral entry (Yoder et al., 2008). Therefore, HIV hijacks chemokine receptor systems to actively promote its entry and its nuclear localization to infect and multiply in host cells. Large DNA viruses, such as herpesvirus, encode a set of proteins that mimic chemokines and chemokine receptors, or secreted proteins that bind chemokines. These viral proteins have various functions to modulate immune systems for viral spread and replication (Alcami, 2003).
Chemokines and their receptors are indispensable for time- and site-dependent trafficking of human phagocytes to fight pathogens in the human body. On the other hand, pathogens have exploited chemokines and their receptors by hijacking and reprogramming chemokine signaling systems for their entry and replication in human cells. One challenge in fighting infectious diseases is to develop therapies that efficiently stop replication and the spread of pathogens without compromising the effectiveness of the human immune system or hyper-activating the system.
Concluding Remarks
In this review, we have highlighted some important findings in the research on chemotaxis and phagocytosis of D. discoideum and human phagocytes. We believe that the intricacies of human innate immune response can only be fully understood by analyzing simpler organisms. The discoveries made over the last 100 years on the social amoebae and human phagocytes have shed a light on our understanding of not only the basic evolution of the innate immune system but also problems of human disease. Future research on D. discoideum and new findings from this simple organism will further enrich our knowledge of innate immunity and human health.
Author Contributions
All authors listed have made a substantial, direct and intellectual contribution to the work, and approved it for publication.
Funding
This work was supported by an intramural fund from NIAID, NIH.
Conflict of Interest
The authors declare that the research was conducted in the absence of any commercial or financial relationships that could be construed as a potential conflict of interest.
Publisher’s Note
All claims expressed in this article are solely those of the authors and do not necessarily represent those of their affiliated organizations, or those of the publisher, the editors and the reviewers. Any product that may be evaluated in this article, or claim that may be made by its manufacturer, is not guaranteed or endorsed by the publisher.
References
Afonso, P. V., Janka-Junttila, M., Lee, Y. J., McCann, C. P., Oliver, C. M., Aamer, K. A., et al. (2012). LTB4 is a signal-relay molecule during neutrophil chemotaxis. Dev. Cell 22, 1079–1091. doi: 10.1016/j.devcel.2012.02.003
Alcami, A. (2003). Viral mimicry of cytokines, chemokines and their receptors. Nat. Rev. Immunol. 3, 36–50. doi: 10.1038/nri980
Amarante-Mendes, G. P., Adjemian, S., Branco, L. M., Zanetti, L. C., Weinlich, R., and Bortoluci, K. R. (2018). Pattern Recognition Receptors and the Host Cell Death Molecular Machinery. Front. Immunol. 9:2379. doi: 10.3389/fimmu.2018.02379
Ambrose, C. T. (2006). The Osler slide, a demonstration of phagocytosis from 1876 Reports of phagocytosis before Metchnikoff’s 1880 paper. Cell Immunol. 240, 1–4. doi: 10.1016/j.cellimm.2006.05.008
Amulic, B., Cazalet, C., Hayes, G. L., Metzler, K. D., and Zychlinsky, A. (2012). Neutrophil function: from mechanisms to disease. Annu. Rev. Immunol. 30, 459–489. doi: 10.1146/annurev-immunol-020711-074942
Baggiolini, M. (2015). CXCL8 - The First Chemokine. Front. Immunol. 6:285. doi: 10.3389/fimmu.2015.00285
Berger, E. A., Murphy, P. M., and Farber, J. M. (1999). Chemokine receptors as HIV-1 coreceptors: roles in viral entry, tropism, and disease. Annu. Rev. Immunol. 17, 657–700. doi: 10.1146/annurev.immunol.17.1.657
Bonner, J. T. (1947). Evidence for the formation of cell aggregates by chemotaxis in the development of the slime mold Dictyostelium discoideum. J. Exp. Zool. 106, 1–26. doi: 10.1002/jez.1401060102
Borgeat, P., Hamberg, M., and Samuelsson, B. (1976). Transformation of arachidonic acid and homo-gamma-linolenic acid by rabbit polymorphonuclear leukocytes. Monohydroxy acids from novel lipoxygenases. J. Biol. Chem. 251, 7816–7820. doi: 10.1016/s0021-9258(19)57008-9
Borrok, M. J., Zhu, Y., Forest, K. T., and Kiessling, L. L. (2009). Structure-based design of a periplasmic binding protein antagonist that prevents domain closure. ACS Chem. Biol. 4, 447–456. doi: 10.1021/cb900021q
Bosgraaf, L., and Van Haastert, P. J. (2002). A model for cGMP signal transduction in Dictyostelium in perspective of 25 years of cGMP research. J. Muscle Res. Cell Motil. 23, 781–791.
Boulais, J., Trost, M., Landry, C. R., Dieckmann, R., Levy, E. D., Soldati, T., et al. (2010). Molecular characterization of the evolution of phagosomes. Mol. Syst. Biol. 6:423. doi: 10.1038/msb.2010.80
Boulay, F., Tardif, M., Brouchon, L., and Vignais, P. (1990). Synthesis and use of a novel N-formyl peptide derivative to isolate a human N-formyl peptide receptor cDNA. Biochem. Biophys. Res. Commun. 168, 1103–1109. doi: 10.1016/0006-291x(90)91143-g
Boyden, S. (1962). The chemotactic effect of mixtures of antibody and antigen on polymorphonuclear leucocytes. J. Exp. Med. 115, 453–466. doi: 10.1084/jem.115.3.453
Buckley, C. M., Heath, V. L., Gueho, A., Bosmani, C., Knobloch, P., Sikakana, P., et al. (2019). PIKfyve/Fab1 is required for efficient V-ATPase and hydrolase delivery to phagosomes, phagosomal killing, and restriction of Legionella infection. PLoS Pathog. 15:e1007551. doi: 10.1371/journal.ppat.1007551
Cao, J., Huang, S., Qian, J., Huang, J., Jin, L., Su, Z., et al. (2009). Evolution of the class C GPCR Venus flytrap modules involved positive selected functional divergence. BMC Evol. Biol. 9:67. doi: 10.1186/1471-2148-9-67
Charest, P. G., Shen, Z., Lakoduk, A., Sasaki, A. T., Briggs, S. P., and Firtel, R. A. (2010). A Ras signaling complex controls the RasC-TORC2 pathway and directed cell migration. Dev. Cell 18, 737–749. doi: 10.1016/j.devcel.2010.03.017
Chen, C. L., Wang, Y., Sesaki, H., and Iijima, M. (2012). Myosin I links PIP3 signaling to remodeling of the actin cytoskeleton in chemotaxis. Sci. Signal 5:ra10. doi: 10.1126/scisignal.2002446
Chen, G., Zhuchenko, O., and Kuspa, A. (2007). Immune-like phagocyte activity in the social amoeba. Science 317, 678–681. doi: 10.1126/science.1143991
Cheng, Y., and Othmer, H. (2016). A Model for Direction Sensing in Dictyostelium discoideum: Ras Activity and Symmetry Breaking Driven by a Gbetagamma-Mediated, Galpha2-Ric8 – Dependent Signal Transduction Network. PLoS Comput. Biol. 12:e1004900. doi: 10.1371/journal.pcbi.100490
Cosson, P., and Soldati, T. (2008). Eat, kill or die: when amoeba meets bacteria. Curr. Opin. Microbiol. 11, 271–276. doi: 10.1016/j.mib.2008.05.005
Devreotes, P. N. (1994). G protein-linked signaling pathways control the developmental program of Dictyostelium. Neuron 12, 235–241. doi: 10.1016/0896-6273(94)90267-4
Devreotes, P. N., and Zigmond, S. H. (1988). Chemotaxis in eukaryotic cells: a focus on leukocytes and Dictyostelium. Annu. Rev. Cell Biol. 4, 649–686. doi: 10.1146/annurev.cb.04.110188.003245
Devreotes, P., and Horwitz, A. R. (2015). Signaling networks that regulate cell migration. Cold Spring Harb. Perspect. Biol. 7:a005959. doi: 10.1101/cshperspect.a005959
Dinh, C., Farinholt, T., Hirose, S., Zhuchenko, O., and Kuspa, A. (2018). Lectins modulate the microbiota of social amoebae. Science 361, 402–406. doi: 10.1126/science.aat2058
Drews, G. (2005). Contributions of Theodor Wilhelm Engelmann on phototaxis, chemotaxis, and photosynthesis. Photosynth. Res. 83, 25–34. doi: 10.1007/s11120-004-6313-8
Dunkelberger, J. R., and Song, W. C. (2010). Complement and its role in innate and adaptive immune responses. Cell Res. 20, 34–50. doi: 10.1038/cr.2009.139
Dunn, J. D., Bosmani, C., Barisch, C., Raykov, L., Lefrancois, L. H., Cardenal-Munoz, E., et al. (2017). Eat Prey, Live: Dictyostelium discoideum As a Model for Cell-Autonomous Defenses. Front. Immunol. 8:1906. doi: 10.3389/fimmu.2017.01906
Fernandez, H. N., Henson, P. M., Otani, A., and Hugli, T. E. (1978). Chemotactic response to human C3a and C5a anaphylatoxins. I. Evaluation of C3a and C5a leukotaxis in vitro and under stimulated in vivo conditions. J. Immunol. 120, 109–115.
Fey, P., Dodson, R. J., Basu, S., and Chisholm, R. L. (2013). One stop shop for everything Dictyostelium: dictyBase and the Dicty Stock Center in 2012. Methods Mol. Biol. 983, 59–92. doi: 10.1007/978-1-62703-302-2_4
Firtel, R. A., van Haastert, P. J., Kimmel, A. R., and Devreotes, P. N. (1989). G protein linked signal transduction pathways in development: dictyostelium as an experimental system. Cell 58, 235–239. doi: 10.1016/0092-8674(89)90837-4
Flannagan, R. S., Jaumouille, V., and Grinstein, S. (2012). The cell biology of phagocytosis. Annu. Rev. Pathol. 7, 61–98.
Foord, S. M., Bonner, T. I., Neubig, R. R., Rosser, E. M., Pin, J. P., Davenport, A. P., et al. (2005). International Union of Pharmacology. XLVI. G protein-coupled receptor list. Pharmacol. Rev. 57, 279–288. doi: 10.1124/pr.57.2.5
Fredriksson, R., Lagerstrom, M. C., Lundin, L. G., and Schioth, H. B. (2003). The G-protein-coupled receptors in the human genome form five main families. Phylogenetic analysis, paralogon groups, and fingerprints. Mol. Pharmacol. 63, 1256–1272. doi: 10.1124/mol.63.6.1256
Funamoto, S., Meili, R., Lee, S., Parry, L., and Firtel, R. A. (2002). Spatial and temporal regulation of 3-phosphoinositides by PI 3-kinase and PTEN mediates chemotaxis. Cell 109, 611–623. doi: 10.1016/s0092-8674(02)00755-9
Gerard, N. P., and Gerard, C. (1991). The chemotactic receptor for human C5a anaphylatoxin. Nature 349, 614–617. doi: 10.1038/349614a0
Gerisch, G., Albrecht, R., De Hostos, E., Wallraff, E., Heizer, C., Kreitmeier, M., et al. (1993). Actin-associated proteins in motility and chemotaxis of Dictyostelium cells. Symp. Soc. Exp. Biol. 47, 297–315.
Gerisch, G., Albrecht, R., Heizer, C., Hodgkinson, S., and Maniak, M. (1995). Chemoattractant-controlled accumulation of coronin at the leading edge of Dictyostelium cells monitored using a green fluorescent protein-coronin fusion protein. Curr. Biol. 5, 1280–1285. doi: 10.1016/s0960-9822(95)00254-5
Gerisch, G., and Muller-Taubenberger, A. (2003). GFP-fusion proteins as fluorescent reporters to study organelle and cytoskeleton dynamics in chemotaxis and phagocytosis. Methods Enzymol. 361, 320–337. doi: 10.1016/s0076-6879(03)61017-7
Gerisch, G., Hulser, D., Malchow, D., and Wick, U. (1975). Cell communication by periodic cyclic-AMP pulses. Philos. Trans. R. Soc. Lond. B Biol. Sci. 272, 181–192. doi: 10.1098/rstb.1975.0080
Ginsburg, G. T., Gollop, R., Yu, Y., Louis, J. M., Saxe, C. L., and Kimmel, A. R. (1995). The regulation of Dictyostelium development by transmembrane signalling. J. Eukaryot. Microbiol. 42, 200–205. doi: 10.1111/j.1550-7408.1995.tb01565.x
Goldberg, J. M., Manning, G., Liu, A., Fey, P., Pilcher, K. E., Xu, Y., et al. (2006). The dictyostelium kinome–analysis of the protein kinases from a simple model organism. PLoS Genet. 2:e38. doi: 10.1371/journal.pgen.0020038
Greenberg, S. (1995). Signal transduction of phagocytosis. Trends Cell Biol. 5, 93–99. doi: 10.1016/s0962-8924(00)88957-6
Greenberg, S., and Grinstein, S. (2002). Phagocytosis and innate immunity. Curr. Opin. Immunol. 14, 136–145. doi: 10.1016/s0952-7915(01)00309-0
Hadwiger, J. A., and Nguyen, H. N. (2011). MAPKs in development: insights from Dictyostelium signaling pathways. Biomol. Concepts 2, 39–46. doi: 10.1515/bmc.2011.004
Heit, B., Robbins, S. M., Downey, C. M., Guan, Z., Colarusso, P., Miller, B. J., et al. (2008). PTEN functions to ‘prioritize’ chemotactic cues and prevent ‘distraction’ in migrating neutrophils. Nat. Immunol. 9, 743–752. doi: 10.1038/ni.1623
Holmes, W. E., Lee, J., Kuang, W. J., Rice, G. C., and Wood, W. I. (1991). Structure and functional expression of a human interleukin-8 receptor. Science 253, 1278–1280. doi: 10.1126/science.1840701
Iglesias, P. A., and Devreotes, P. N. (2012). Biased excitable networks: how cells direct motion in response to gradients. Curr. Opin. Cell Biol. 24, 245–253. doi: 10.1016/j.ceb.2011.11.009
Iijima, M., and Devreotes, P. (2002). Tumor suppressor PTEN mediates sensing of chemoattractant gradients. Cell 109, 599–610.
Insall, R. H., and Machesky, L. M. (2009). Actin dynamics at the leading edge: from simple machinery to complex networks. Dev. Cell 17, 310–322. doi: 10.1016/j.devcel.2009.08.012
Insall, R., Kuspa, A., Lilly, P. J., Shaulsky, G., Levin, L. R., Loomis, W. F., et al. (1994). CRAC, a cytosolic protein containing a pleckstrin homology domain, is required for receptor and G protein-mediated activation of adenylyl cyclase in Dictyostelium. J. Cell Biol. 126, 1537–1545. doi: 10.1083/jcb.126.6.1537
Janetopoulos, C., Jin, T., and Devreotes, P. (2001). Receptor-mediated activation of heterotrimeric G-proteins in living cells. Science 291, 2408–2411. doi: 10.1126/science.1055835
Janeway, C. A. Jr. (1989). Approaching the asymptote? Evolution and revolution in immunology. Cold Spring Harb. Symp. Quant. Biol. 54(Pt 1), 1–13. doi: 10.1101/sqb.1989.054.01.003
Jin, T., Xu, X., and Hereld, D. (2008). Chemotaxis, chemokine receptors and human disease. Cytokine 44, 1–8. doi: 10.1016/j.cyto.2008.06.017
Jin, T., Zhang, N., Long, Y., Parent, C. A., and Devreotes, P. N. (2000). Localization of the G protein betagamma complex in living cells during chemotaxis. Science 287, 1034–1036. doi: 10.1126/science.287.5455.1034
Kaufmann, S. H. E., and Dorhoi, A. (2016). Molecular Determinants in Phagocyte-Bacteria Interactions. Immunity 44, 476–491. doi: 10.1016/j.immuni.2016.02.014
Khanna, A., Lotfi, P., Chavan, A. J., Montano, N. M., Bolourani, P., Weeks, G., et al. (2016). The small GTPases Ras and Rap1 bind to and control TORC2 activity. Sci. Rep. 6:25823.
King, J. S., and Kay, R. R. (2019). The origins and evolution of macropinocytosis. Philos. Trans. R. Soc. Lond. B Biol. Sci. 374:20180158. doi: 10.1098/rstb.2018.0158
Klein, P. S., Sun, T. J., Saxe, C. L. III, Kimmel, A. R., Johnson, R. L., and Devreotes, P. N. (1988). A chemoattractant receptor controls development in Dictyostelium discoideum. Science 241, 1467–1472. doi: 10.1126/science.3047871
Kolaczkowska, E., and Kubes, P. (2013). Neutrophil recruitment and function in health and inflammation. Nat. Rev. Immunol. 13, 159–175. doi: 10.1038/nri3399
Konijn, T. M., van de Meene, J. G., Chang, Y. Y., Barkley, D. S., and Bonner, J. T. (1969). Identification of adenosine-3′,5′-monophosphate as the bacterial attractant for myxamoebae of Dictyostelium discoideum. J. Bacteriol. 99, 510–512. doi: 10.1128/jb.99.2.510-512.1969
Kortholt, A., Kataria, R., Keizer-Gunnink, I., Van Egmond, W. N., Khanna, A., and Van Haastert, P. J. (2011). Dictyostelium chemotaxis: essential Ras activation and accessory signalling pathways for amplification. EMBO Rep. 12, 1273–1279. doi: 10.1038/embor.2011.210
Kriebel, P. W., Barr, V. A., and Parent, C. A. (2003). Adenylyl cyclase localization regulates streaming during chemotaxis. Cell 112, 549–560. doi: 10.1016/s0092-8674(03)00081-3
Lammermann, T., Afonso, P. V., Angermann, B. R., Wang, J. M., Kastenmuller, W., Parent, C. A., et al. (2013). Neutrophil swarms require LTB4 and integrins at sites of cell death in vivo. Nature 498, 371–375. doi: 10.1038/nature12175
Levine, H., Kessler, D. A., and Rappel, W. J. (2006). Directional sensing in eukaryotic chemotaxis: a balanced inactivation model. Proc. Natl. Acad. Sci. U S A. 103, 9761–9766. doi: 10.1073/pnas.0601302103
Li, H., Yang, L., Fu, H., Yan, J., Wang, Y., Guo, H., et al. (2013). Association between Galphai2 and ELMO1/Dock180 connects chemokine signalling with Rac activation and metastasis. Nat. Commun. 4, 1706.
Li, X., Miao, Y., Pal, D. S., and Devreotes, P. N. (2020). Excitable networks controlling cell migration during development and disease. Semin. Cell Dev. Biol. 100, 133–142. doi: 10.1016/j.semcdb.2019.11.001
Lisco, A., Grivel, J. C., Biancotto, A., Vanpouille, C., Origgi, F., Malnati, M. S., et al. (2007). Viral interactions in human lymphoid tissue: Human herpesvirus 7 suppresses the replication of CCR5-tropic human immunodeficiency virus type 1 via CD4 modulation. J. Virol. 81, 708–717. doi: 10.1128/jvi.01367-06
Liu, L., Das, S., Losert, W., and Parent, C. A. (2010). mTORC2 regulates neutrophil chemotaxis in a cAMP- and RhoA-dependent fashion. Dev. Cell 19, 845–857. doi: 10.1016/j.devcel.2010.11.004
Luster, A. D., Unkeless, J. C., and Ravetch, J. V. (1985). Gamma-interferon transcriptionally regulates an early-response gene containing homology to platelet proteins. Nature 315, 672–676. doi: 10.1038/315672a0
Maniak, M., Rauchenberger, R., Albrecht, R., Murphy, J., and Gerisch, G. (1995). Coronin involved in phagocytosis: dynamics of particle-induced relocalization visualized by a green fluorescent protein Tag. Cell 83, 915–924. doi: 10.1016/0092-8674(95)90207-4
Matsuoka, S., and Ueda, M. (2018). Mutual inhibition between PTEN and PIP3 generates bistability for polarity in motile cells. Nat. Commun. 9:4481.
Matsuoka, S., Iijima, M., Watanabe, T. M., Kuwayama, H., Yanagida, T., Devreotes, P. N., et al. (2006). Single-molecule analysis of chemoattractant-stimulated membrane recruitment of a PH-domain-containing protein. J. Cell Sci. 119(Pt 6), 1071–1079. doi: 10.1242/jcs.02824
Matsushima, K., Larsen, C. G., DuBois, G. C., and Oppenheim, J. J. (1989). Purification and characterization of a novel monocyte chemotactic and activating factor produced by a human myelomonocytic cell line. J. Exp. Med. 169, 1485–1490. doi: 10.1084/jem.169.4.1485
Mc, C. M. (1946). Chemotaxis in leukocytes. Physiol. Rev. 26, 319–336. doi: 10.1152/physrev.1946.26.3.319
Meier-Schellersheim, M., Xu, X., Angermann, B., Kunkel, E. J., Jin, T., and Germain, R. N. (2006). Key role of local regulation in chemosensing revealed by a new molecular interaction-based modeling method. PLoS Comput. Biol. 2:e82. doi: 10.1371/journal.pcbi.0020082
Meili, R., Ellsworth, C., Lee, S., Reddy, T. B., Ma, H., and Firtel, R. A. (1999). Chemoattractant-mediated transient activation and membrane localization of Akt/PKB is required for efficient chemotaxis to cAMP in Dictyostelium. EMBO J. 18, 2092–2105. doi: 10.1093/emboj/18.8.2092
Metzemaekers, M., Gouwy, M., and Proost, P. (2020). Neutrophil chemoattractant receptors in health and disease: double-edged swords. Cell Mol. Immunol. 17, 433–450. doi: 10.1038/s41423-020-0412-0
Miller, M. D., Hata, S., De Waal Malefyt, R., and Krangel, M. S. (1989). A novel polypeptide secreted by activated human T lymphocytes. J. Immunol. 143, 2907–2916.
Miyanaga, Y., Kamimura, Y., Kuwayama, H., Devreotes, P. N., and Ueda, M. (2018). Chemoattractant receptors activate, recruit and capture G proteins for wide range chemotaxis. Biochem. Biophys. Res. Commun. 507, 304–310. doi: 10.1016/j.bbrc.2018.11.029
Moran, B. M., Flatt, P. R., and McKillop, A. M. (2016). G protein-coupled receptors: signalling and regulation by lipid agonists for improved glucose homoeostasis. Acta Diabetol. 53, 177–188. doi: 10.1007/s00592-015-0826-9
Murphy, P. M., and Tiffany, H. L. (1991). Cloning of complementary DNA encoding a functional human interleukin-8 receptor. Science 253, 1280–1283. doi: 10.1126/science.1891716
Murphy, P. M., Baggiolini, M., Charo, I. F., Hebert, C. A., Horuk, R., Matsushima, K., et al. (2000). International union of pharmacology. XXII. Nomenclature for chemokine receptors. Pharmacol. Rev. 52, 145–176.
Nakajima, A., Ishihara, S., Imoto, D., and Sawai, S. (2014). Rectified directional sensing in long-range cell migration. Nat. Commun. 5:5367.
Neptune, E. R., and Bourne, H. R. (1997). Receptors induce chemotaxis by releasing the betagamma subunit of Gi, not by activating Gq or Gs. Proc. Natl. Acad. Sci. U S A. 94, 14489–14494. doi: 10.1073/pnas.94.26.14489
Nichols, J. M. E., Paschke, P., Peak-Chew, S., Williams, T. D., Tweedy, L., Skehel, M., et al. (2019). The Atypical MAP Kinase ErkB Transmits Distinct Chemotactic Signals through a Core Signaling Module. Dev. Cell 48, 491–505e499.
Nieto, M., Frade, J. M., Sancho, D., Mellado, M., Martinez, A. C., and Sanchez-Madrid, F. (1997). Polarization of chemokine receptors to the leading edge during lymphocyte chemotaxis. J. Exp. Med. 186, 153–158. doi: 10.1084/jem.186.1.153
Nishio, M., Watanabe, K., Sasaki, J., Taya, C., Takasuga, S., Iizuka, R., et al. (2007). Control of cell polarity and motility by the PtdIns(3,4,5)P3 phosphatase SHIP1. Nat. Cell Biol. 9, 36–44. doi: 10.1038/ncb1515
Obaru, K., Fukuda, M., Maeda, S., and Shimada, K. (1986). A cDNA clone used to study mRNA inducible in human tonsillar lymphocytes by a tumor promoter. J. Biochem. 99, 885–894. doi: 10.1093/oxfordjournals.jbchem.a135549
Osei-Owusu, P., Charlton, T. M., Kim, H. K., Missiakas, D., and Schneewind, O. (2019). FPR1 is the plague receptor on host immune cells. Nature 574, 57–62. doi: 10.1038/s41586-019-1570-z
Pan, M., Neilson, M. P., Grunfeld, A. M., Cruz, P., Wen, X., Insall, R. H., et al. (2018). A G-protein-coupled chemoattractant receptor recognizes lipopolysaccharide for bacterial phagocytosis. PLoS Biol. 16:e2005754. doi: 10.1371/journal.pbio.2005754
Pan, M., Xu, X., Chen, Y., and Jin, T. (2016). Identification of a Chemoattractant G-Protein-Coupled Receptor for Folic Acid that Controls Both Chemotaxis and Phagocytosis. Dev. Cell 36, 428–439. doi: 10.1016/j.devcel.2016.01.012
Pan, P., Hall, E. M., and Bonner, J. T. (1972). Folic acid as second chemotactic substance in the cellular slime moulds. Nat. New Biol. 237, 181–182. doi: 10.1038/newbio237181a0
Parent, C. A., and Weiner, O. D. (2013). The symphony of cell movement: how cells orchestrate diverse signals and forces to control migration. Curr. Opin. Cell Biol. 25, 523–525. doi: 10.1016/j.ceb.2013.07.011
Parent, C. A., Blacklock, B. J., Froehlich, W. M., Murphy, D. B., and Devreotes, P. N. (1998). G protein signaling events are activated at the leading edge of chemotactic cells. Cell 95, 81–91. doi: 10.1016/s0092-8674(00)81784-5
Peracino, B., Borleis, J., Jin, T., Westphal, M., Schwartz, J. M., Wu, L., et al. (1998). G protein beta subunit-null mutants are impaired in phagocytosis and chemotaxis due to inappropriate regulation of the actin cytoskeleton. J. Cell Biol. 141, 1529–1537. doi: 10.1083/jcb.141.7.1529
Pollard, T. D., and Borisy, G. G. (2003). Cellular motility driven by assembly and disassembly of actin filaments. Cell 112, 453–465. doi: 10.1016/s0092-8674(03)00120-x
Postma, M., and Van Haastert, P. J. (2001). A diffusion-translocation model for gradient sensing by chemotactic cells. Biophys. J. 81, 1314–1323. doi: 10.1016/s0006-3495(01)75788-8
Prabhu, Y., and Eichinger, L. (2006). The Dictyostelium repertoire of seven transmembrane domain receptors. Eur. J. Cell Biol. 85, 937–946. doi: 10.1016/j.ejcb.2006.04.003
Rappel, W. J., Thomas, P. J., Levine, H., and Loomis, W. F. (2002). Establishing direction during chemotaxis in eukaryotic cells. Biophys. J. 83, 1361–1367. doi: 10.1016/s0006-3495(02)73906-4
Sasaki, A. T., Chun, C., Takeda, K., and Firtel, R. A. (2004). Localized Ras signaling at the leading edge regulates PI3K, cell polarity, and directional cell movement. J. Cell Biol. 167, 505–518. doi: 10.1083/jcb.200406177
Sattler, N., Bosmani, C., Barisch, C., Gueho, A., Gopaldass, N., Dias, M., et al. (2018). Functions of the Dictyostelium LIMP-2 and CD36 homologues in bacteria uptake, phagolysosome biogenesis and host cell defence. J. Cell Sci. 131:17.
Saxe, C. L. III, Johnson, R., Devreotes, P. N., and Kimmel, A. R. (1991). Multiple genes for cell surface cAMP receptors in Dictyostelium discoideum. Dev. Genet. 12, 6–13. doi: 10.1002/dvg.1020120104
Schiffmann, E., Corcoran, B. A., and Wahl, S. M. (1975). N-formylmethionyl peptides as chemoattractants for leucocytes. Proc. Natl. Acad. Sci. U S A. 72, 1059–1062. doi: 10.1073/pnas.72.3.1059
Schwebs, D. J., Pan, M., Adhikari, N., Kuburich, N. A., Jin, T., and Hadwiger, J. A. (2018). Dictyostelium Erk2 is an atypical MAPK required for chemotaxis. Cell Signal 46, 154–165. doi: 10.1016/j.cellsig.2018.03.006
Senoo, H., Kamimura, Y., Kimura, R., Nakajima, A., Sawai, S., Sesaki, H., et al. (2019). Phosphorylated Rho-GDP directly activates mTORC2 kinase towards AKT through dimerization with Ras-GTP to regulate cell migration. Nat. Cell Biol. 21, 867–878. doi: 10.1038/s41556-019-0348-8
Servant, G., Weiner, O. D., Herzmark, P., Balla, T., Sedat, J. W., and Bourne, H. R. (2000). Polarization of chemoattractant receptor signaling during neutrophil chemotaxis. Science 287, 1037–1040. doi: 10.1126/science.287.5455.1037
Snyderman, R., Gewurz, H., and Mergenhagen, S. E. (1968). Interactions of the complement system with endotoxic lipopolysaccharide. Generation of a factor chemotactic for polymorphonuclear leukocytes. J. Exp. Med. 128, 259–275. doi: 10.1084/jem.128.2.259
Soldati, T., and Cardenal-Munoz, E. (2019). A brief historical and evolutionary perspective on the origin of cellular microbiology research. Cell Microbiol. 21:e13083.
Stuart, L. M., and Ezekowitz, R. A. (2008). Phagocytosis and comparative innate immunity: learning on the fly. Nat. Rev. Immunol. 8, 131–141. doi: 10.1038/nri2240
Suire, S., Condliffe, A. M., Ferguson, G. J., Ellson, C. D., Guillou, H., Davidson, K., et al. (2006). Gbetagammas and the Ras binding domain of p110gamma are both important regulators of PI(3)Kgamma signalling in neutrophils. Nat. Cell Biol. 8, 1303–1309. doi: 10.1038/ncb1494
Swanson, J. A., and Taylor, D. L. (1982). Local and spatially coordinated movements in Dictyostelium discoideum amoebae during chemotaxis. Cell 28, 225–232. doi: 10.1016/0092-8674(82)90340-3
Takeda, K., Shao, D., Adler, M., Charest, P. G., Loomis, W. F., Levine, H., et al. (2012). Incoherent feedforward control governs adaptation of activated ras in a eukaryotic chemotaxis pathway. Sci. Signal 5:ra2. doi: 10.1126/scisignal.2002413
Tariqul Islam, A. F. M., Scavello, M., Lotfi, P., Daniel, D., Haldeman, P., and Charest, P. G. (2019). Caffeine inhibits PI3K and mTORC2 in Dictyostelium and differentially affects multiple other cAMP chemoattractant signaling effectors. Mol. Cell Biochem. 457, 157–168. doi: 10.1007/s11010-019-03520-z
Tweedy, L., Thomason, P. A., Paschke, P. I., Martin, K., Machesky, L. M., Zagnoni, M., et al. (2020). Seeing around corners: Cells solve mazes and respond at a distance using attractant breakdown. Science 369:6507.
Ueda, M., Sako, Y., Tanaka, T., Devreotes, P., and Yanagida, T. (2001). Single-molecule analysis of chemotactic signaling in Dictyostelium cells. Science 294, 864–867. doi: 10.1126/science.1063951
Underhill, D. M., and Ozinsky, A. (2002). Phagocytosis of microbes: complexity in action. Annu. Rev. Immunol. 20, 825–852. doi: 10.1146/annurev.immunol.20.103001.114744
Uribe-Querol, E., and Rosales, C. (2020). Phagocytosis: Our Current Understanding of a Universal Biological Process. Front. Immunol. 11:1066. doi: 10.3389/fimmu.2020.01066
Van Haastert, P. J., and Devreotes, P. N. (2004). Chemotaxis: signalling the way forward. Nat. Rev. Mol. Cell Biol. 5, 626–634. doi: 10.1038/nrm1435
van Haastert, P. J., Keizer-Gunnink, I., and Kortholt, A. (2017). Coupled excitable Ras and F-actin activation mediates spontaneous pseudopod formation and directed cell movement. Mol. Biol. Cell 28, 922–934. doi: 10.1091/mbc.e16-10-0733
Vogel, G., Thilo, L., Schwarz, H., and Steinhart, R. (1980). Mechanism of phagocytosis in Dictyostelium discoideum: phagocytosis is mediated by different recognition sites as disclosed by mutants with altered phagocytotic properties. J. Cell Biol. 86, 456–465. doi: 10.1083/jcb.86.2.456
Walsh, D., McCarthy, J., O’Driscoll, C., and Melgar, S. (2013). Pattern recognition receptors–molecular orchestrators of inflammation in inflammatory bowel disease. Cytokine Growth Factor Rev. 24, 91–104. doi: 10.1016/j.cytogfr.2012.09.003
Walz, A., Peveri, P., Aschauer, H., and Baggiolini, M. (1987). Purification and amino acid sequencing of NAF, a novel neutrophil-activating factor produced by monocytes. Biochem. Biophys. Res. Commun. 149, 755–761. doi: 10.1016/0006-291x(87)90432-3
Wen, X., Xu, X., Sun, W., Chen, K., Pan, M., Wang, J. M., et al. (2019). G-protein-coupled formyl peptide receptors play a dual role in neutrophil chemotaxis and bacterial phagocytosis. Mol. Biol. Cell 30, 346–356. doi: 10.1091/mbc.e18-06-0358
Williams, L. T. (1989). Signal transduction by the platelet-derived growth factor receptor. Science 243, 1564–1570. doi: 10.1126/science.2538922
Wood, W. B. Jr., Smith, M. R., and Watson, B. (1946). Surface phagocytosis; its relation to the mechanism of recovery in pneumococcal pneumonia. Science 104:28.
Wright, A. E., Douglas, S. R., and Sanderson, J. B. (1989). An experimental investigation of the role of the blood fluids in connection with phagocytosis. 1903. Rev. Infect. Dis. 11, 827–834. doi: 10.1093/clinids/11.5.827
Xiao, Z., Zhang, N., Murphy, D. B., and Devreotes, P. N. (1997). Dynamic distribution of chemoattractant receptors in living cells during chemotaxis and persistent stimulation. J. Cell Biol. 139, 365–374. doi: 10.1083/jcb.139.2.365
Xu, X., Gera, N., Li, H., Yun, M., Zhang, L., Wang, Y., et al. (2015). GPCR-mediated PLCbetagamma/PKCbeta/PKD signaling pathway regulates the cofilin phosphatase slingshot 2 in neutrophil chemotaxis. Mol. Biol. Cell 26, 874–886. doi: 10.1091/mbc.e14-05-0982
Xu, X., Meier-Schellersheim, M., Jiao, X., Nelson, L. E., and Jin, T. (2005). Quantitative imaging of single live cells reveals spatiotemporal dynamics of multistep signaling events of chemoattractant gradient sensing in Dictyostelium. Mol. Biol. Cell 16, 676–688. doi: 10.1091/mbc.e04-07-0544
Xu, X., Meier-Schellersheim, M., Yan, J., and Jin, T. (2007). Locally controlled inhibitory mechanisms are involved in eukaryotic GPCR-mediated chemosensing. J. Cell Biol. 178, 141–153. doi: 10.1083/jcb.200611096
Yan, J., Mihaylov, V., Xu, X., Brzostowski, J. A., Li, H., Liu, L., et al. (2012). A Gbetagamma effector, ElmoE, transduces GPCR signaling to the actin network during chemotaxis. Dev. Cell 22, 92–103. doi: 10.1016/j.devcel.2011.11.007
Yang, Y., Li, D., Chao, X., Singh, S. P., Thomason, P., Yan, Y., et al. (2021). Leep1 interacts with PIP3 and the Scar/WAVE complex to regulate cell migration and macropinocytosis. J. Cell Biol. 220:7.
Yoder, A., Yu, D., Dong, L., Iyer, S. R., Xu, X., Kelly, J., et al. (2008). HIV envelope-CXCR4 signaling activates cofilin to overcome cortical actin restriction in resting CD4 T cells. Cell 134, 782–792. doi: 10.1016/j.cell.2008.06.036
Yoshimura, T., Matsushima, K., Tanaka, S., Robinson, E. A., Appella, E., Oppenheim, J. J., et al. (1987). Purification of a human monocyte-derived neutrophil chemotactic factor that has peptide sequence similarity to other host defense cytokines. Proc. Natl. Acad. Sci. U S A. 84, 9233–9237. doi: 10.1073/pnas.84.24.9233
Yoshimura, T., Robinson, E. A., Tanaka, S., Appella, E., Kuratsu, J., and Leonard, E. J. (1989). Purification and amino acid analysis of two human glioma-derived monocyte chemoattractants. J. Exp. Med. 169, 1449–1459. doi: 10.1084/jem.169.4.1449
Zhang, X., Zhuchenko, O., Kuspa, A., and Soldati, T. (2016). Social amoebae trap and kill bacteria by casting DNA nets. Nat. Commun. 7:10938.
Zigmond, S. H. (1974). Mechanisms of sensing chemical gradients by polymorphonuclear leukocytes. Nature 249, 450–452. doi: 10.1038/249450a0
Keywords: chemotaxis, phagocytosis, innate immunity, D. discoideum, phagocyte
Citation: Xu X, Pan M and Jin T (2021) How Phagocytes Acquired the Capability of Hunting and Removing Pathogens From a Human Body: Lessons Learned From Chemotaxis and Phagocytosis of Dictyostelium discoideum (Review). Front. Cell Dev. Biol. 9:724940. doi: 10.3389/fcell.2021.724940
Received: 14 June 2021; Accepted: 15 July 2021;
Published: 20 August 2021.
Edited by:
Robin S. B. Williams, University of London, United KingdomReviewed by:
Thierry Soldati, Université de Genève, SwitzerlandPascale G. Charest, University of Arizona, United States
Copyright © 2021 Xu, Pan and Jin. This is an open-access article distributed under the terms of the Creative Commons Attribution License (CC BY). The use, distribution or reproduction in other forums is permitted, provided the original author(s) and the copyright owner(s) are credited and that the original publication in this journal is cited, in accordance with accepted academic practice. No use, distribution or reproduction is permitted which does not comply with these terms.
*Correspondence: Tian Jin, VGppbkBuaWFpZC5uaWguZ292