- 1Cancer Biology, Division of Cancer and Stem Cells, School of Medicine, Biodiscovery Institute, University of Nottingham, Nottingham, United Kingdom
- 2The Institute of Biomedical and Clinical Science, University of Exeter Medical School, St. Luke’s Campus, University of Exeter, Exeter, United Kingdom
- 3Biosciences, College of Life and Environmental Science, University of Exeter, Exeter, United Kingdom
- 4Academic Ophthalmology, Division of Clinical Neuroscience, School of Medicine, University of Nottingham, Nottingham, United Kingdom
Significantly reduced levels of the anti-inflammatory gaseous transmitter hydrogen sulfide (H2S) are observed in diabetic patients and correlate with microvascular dysfunction. H2S may protect the microvasculature by preventing loss of the endothelial glycocalyx. We tested the hypothesis that H2S could prevent or treat retinal microvascular endothelial dysfunction in diabetes. Bovine retinal endothelial cells (BRECs) were exposed to normal (NG, 5.5 mmol/L) or high glucose (HG, 25 mmol/L) ± the slow-release H2S donor NaGYY4137 in vitro. Glycocalyx coverage (stained with WGA-FITC) and calcein-labeled monocyte adherence were measured. In vivo, fundus fluorescein angiography (FFA) was performed in normal and streptozotocin-induced (STZ) diabetic rats. Animals received intraocular injection of NaGYY4137 (1 μM) or the mitochondrial-targeted H2S donor AP39 (100 nM) simultaneously with STZ (prevention) or on day 6 after STZ (treatment), and the ratio of interstitial to vascular fluorescence was used to estimate apparent permeability. NaGYY4137 prevented HG-induced loss of BREC glycocalyx, increased monocyte binding to BRECs (p ≤ 0.001), and increased overall glycocalyx coverage (p ≤ 0.001). In rats, the STZ-induced increase in apparent retinal vascular permeability (p ≤ 0.01) was significantly prevented by pre-treatment with NaGYY4137 and AP39 (p < 0.05) and stabilized by their post-STZ administration. NaGYY4137 also reduced the number of acellular capillaries (collagen IV + /IB4-) in the diabetic retina in both groups (p ≤ 0.05). We conclude that NaGYY4137 and AP39 protected the retinal glycocalyx and endothelial permeability barrier from diabetes-associated loss of integrity and reduced the progression of diabetic retinopathy (DR). Hydrogen sulfide donors that target the glycocalyx may therefore be a therapeutic candidate for DR.
Introduction
Loss of integrity of the vascular permeability barrier is associated with a range of pathological conditions. In particular, increased permeability is observed in diabetes, and in hyperglycemic conditions (Gillies et al., 1997; Brownlee, 2001; Allen and Bayraktutan, 2009; Saker et al., 2014) where it is associated with the pathogenesis of life-altering diabetic microvascular complications such as retinopathy (Sander et al., 2007). The vascular permeability barrier is formed of endothelial cells (ECs), their underlying basement membrane, and the endothelial glycocalyx. The latter is now recognized as a key regulator of permeability as demonstrated by a range of studies showing that selective loss of specific glycocalyx components is associated with altered permeability properties in various vascular beds (Jeansson and Haraldsson, 2003; Singh et al., 2007; Landsverk et al., 2012; Betteridge et al., 2017; Onions et al., 2019).
A role for endothelial glycocalyx damage in diabetes-associated vascular changes has been described. Glycocalyx reduction is associated with increased vascular permeability and increased leucocyte and platelet adhesion in acute hyperglycemia and diabetes (Nieuwdorp et al., 2006a,b; Broekhuizen et al., 2010; Kumase et al., 2010), which were reversed by glycocalyx restoration experimentally in animals (Henry and Duling, 1999; Constantinescu et al., 2003). Strategies to reverse or reduce glycocalyx damage in diabetes have the potential to rescue some of the potentially damaging vascular changes that underlie the development of vascular complications such as retinopathy.
We hypothesized that exogenous application of hydrogen sulfide (H2S) may protect the glycocalyx in diabetes, reversing or preventing the retinal vascular leakage observed in diabetic retinopathy (DR). Hydrogen sulfide has recently been identified as a seemingly ubiquitous “gaseous mediator” in mammals and humans where it is synthesized by multiple cell types by at least four distinct enzyme systems utilizing endogenous sulfur-containing amino acids. These systems include the cytosolic cystathionase (CSE), cystathionine-β-synthase (CBS), mitochondrial cysteine aminotransferase/3-mercaptopyruvate sulfurtransferase (CAT/3MST), and D-amino acid oxidase/3- mercaptopyruvate sulfurtransferase (DAO/3MST) (Whiteman et al., 2011; Szabo, 2012; Wang, 2012; Szabo et al., 2014). The first three, at least have been demonstrated in ECs (Wang, 2012; Tao et al., 2013; Mitidieri et al., 2020). Experimentally, manipulation of H2S level has been achieved by pharmacological inhibition or genetic removal of enzyme systems for H2S and the use of H2S delivery molecules, albeit generally limited to the use of simple inorganic sulfide salts [e.g., sodium hydrosulfide (NaSH) or disodium sulfide (Na2S)]. These studies have revealed that H2S and/or physiologically derived species exert a wide variety of effects on different organ systems including regulation of vascular tone (Yang et al., 2008; Kanagy et al., 2017; Szabo, 2017), inflammation (Whiteman and Winyard, 2011), aging and health span (Ng et al., 2018; Zivanovic et al., 2019), and more recently as a regulator of retinal physiology (Du et al., 2017).
There is increasing evidence for impaired vascular EC synthesis and/or bioavailability of H2S in diabetes (Szabo, 2012). Lower vascular and/or tissue levels of “H2S” have been observed in humans with diabetes (Whiteman et al., 2010a; Suzuki et al., 2017), and in several animal diabetic models, including streptozotocin (STZ)-treated rats (Yusuf et al., 2005; Si et al., 2013; Zhou et al., 2014) and mice (Suzuki et al., 2011) and in db/db (Peake et al., 2013; Sun et al., 2018), ob/ob (Zhao et al., 2017), Akita (Kundu et al., 2013; John et al., 2017), and NOD (Brancaleone et al., 2008) mice. Administration of often high doses of H2S via sulfide salts at least partially prevented the diabetic phenotype in some of these studies. In the STZ diabetes model specifically, retinal levels of H2S were lower in diabetic animals compared with controls (Si et al., 2013), and retinal capillary leakage, VEGF levels, and oxidative stress markers were partially normalized after NaSH treatment (Si et al., 2013). NaSH administration also partially restored mitochondrial function, e.g., increased ATP synthesis and complex II and III activity and prevented mitochondrial oxidant production and mitochondrial swelling, demonstrating a likely mitochondrial target for pharmacological H2S (Si et al., 2013). Collectively, these studies strongly suggest that diabetes and diabetic retinopathy (DR) result from “H2S deficiency,” which could at least be partially overcome by pharmacological manipulation of mitochondrial H2S levels.
It is important to note that these previous studies on H2S and diabetes have exclusively relied on the use of sulfide salts to generate H2S (e.g., NaSH and Na2S). While useful laboratory tools for H2S generation, they are not without severe limitations (Whiteman et al., 2011). These include generation of an instant, and unphysiological bolus of H2S by pH-dependent dissociation (and rapid decay), whereas endogenous organic enzymatic H2S synthesis produces low levels of H2S over prolonged periods of time (Li et al., 2008; Whiteman et al., 2010b, 2011; Kabil and Banerjee, 2014).
It is now recognized that mitochondrial oxidative stress may contribute to the pathogenesis of diabetic endothelial dysfunction (Brownlee, 2005) and that in diabetes, the primary target for H2S activity appears to be the mitochondria where it is used as an electron source for respiration to reduce oxidative stress (Módis et al., 2014; Szabo et al., 2014). This is supported by data indicating that mitochondrial-targeted slow-release H2S donors preserved cellular bioenergetics and increased levels of Nrf2 and peroxisome proliferator-activated receptor-gamma coactivator (PGC-1α), key regulators of mitochondrial antioxidant capacity, bioenergetics, and biogenesis, both in vitro and in vivo in response to UVA-induced photoaging (Lohakul et al., 2021).
However, H2S concentrations (derived from sulfide salts) that are required to reverse mitochondrial dysfunction in the vasculature (e.g., in diabetic rats) are unattainable (Suzuki et al., 2011; Montoya and Pluth, 2016). This is presumably because H2S from these tools is generated immediately, locally, and not targeted to mitochondria. With the development of the slow-release cytosolic donor sodium GYY4137 (Li et al., 2008) and the mitochondria-specific sulfide donor AP39 (Le Trionnaire et al., 2014) the limitations of NaSH/Na2S have been overcome. The effects on microvascular EC and in the kidney have been previously reported (Ahmad et al., 2016; Gerő et al., 2016), but to the best of our knowledge, no previous studies have reported on the effects of these molecules in DR.
In this current study, we investigated the effects of a single dose (administered by intravitreal injection) of two slow-release H2S donor molecules, the cytosolic sodium GYY4137 (Li et al., 2008) and the mitochondria-specific sulfide donor AP39 (Le Trionnaire et al., 2014), on retinal vascular permeability in vivo in the Norway Brown rat streptozotocin-induced DR model. In addition, the effects of these two H2S generators on in vitro EC glycocalyx thickness using bovine retinal endothelial cells (BRECs) were studied. Leucocyte adhesion to BRECs and the effects of the H2S modulation were also evaluated in normal and high-glucose conditions.
Materials and Methods
H2S Donor Synthesis
The mitochondria-targeted H2S donor AP39 was synthesized in our laboratories as previously described by our team (Le Trionnaire et al., 2014). Commercially available GYY4137 is a morpholine salt that contains unstated quantities of dichloromethane residual from its initial synthesis and which forms part of the lattice structure (at least 2 dichloromethane:1 GYY4137 molecule) (Alexander et al., 2015), i.e., it is commercially available as xCHCl2 or a dichloromethane complex. Dichloromethane is metabolized in vivo to carbon monoxide (Ratney et al., 1974; Takano and Miyazaki, 1988). Furthermore, the morpholinium counter ion (1 morpholine:1 GYY4137 molecule) is not biologically inert and has a half-life in rats of ∼90 min (Sohn et al., 1982). LD50 values for morpholine in rats and mice, e.g., 100–400 mg/kg for i.p. administration (Kielhorn and Rosner, 1996), are well within the commonly used doses of commercial GYY4137, e.g., 50–300 mg/kg (Lee et al., 2011; Li et al., 2013). In the eye, morpholine and dichloromethane have been reported to cause keratoconjunctivitis, focal/diffuse cataract formation, keratitis, iritis, conjunctival epithelial edema and detachment, and denudation of corneal epithelium (Ballantyne et al., 1976; Brandt and Okamoto, 1988; Toxicology–cosmetic ingredient review, 1989). To avoid these clinical indications and associated toxicity, a cleaner dichloromethane and morpholine-free sodium salt synthesized as previously described was used in this study (Alexander et al., 2015).
Cell Culture and Treatments
Primary cultures of BRECs were isolated from bovine retinae dissected from eyes of freshly slaughtered cattle, by homogenization and a series of filtration steps as previously described (Chibber et al., 2000). Briefly, excess fat and muscle tissue was removed from the eye globes and the retina were dissected, placed in 20 ml of minimal essential medium (MEM) and homogenized using a hand-held sterile glass homogenizer to dissociate the neural retina. The resulting homogenate was filtered through 80-μm nylon mesh, the material remaining on the mesh was collected, and the trapped microvessels were resuspended in serum free MEM with collagenase-dispase (2 mg/ml) and digested for 90 min at 37°C on a rotator shaker. Following further filtration through a 45-μm polypropylene net filter, the trapped microvessel fragments were vigorously pipetted in MEM with 10% (v/v) pooled human serum, 10% (v/v) tryptose phosphate broth (TPB), L-glutamine 2 mM, penicillin (100 U/ml), and streptomycin (100 μg/ml) and plated in fibronectin-coated tissue culture flasks. After 24 h, the flasks were rinsed once with MEM to remove debris and unattached cells and refilled with fresh growth medium [MEM supplemented with 10% (v/v) pooled human serum, 10% (v/v) tryptose phosphate broth (TPB), L-glutamine 2 mmol/L, and penicillin (100 U/ml) and streptomycin (100 μg/ml)]. From passage 1 onward, the BRECs were cultured on gelatin-coated tissue culture flasks and human serum was replaced with 10% (v/v) horse serum. Pericyte contamination was removed by differential trypsinization. Cultures were >90% pure as assessed by morphology and staining for von Willebrand factor. Preliminary studies confirmed that treatment with 25 mmol/L D-mannitol as osmotic control had no effect on either the glycocalyx or leukocyte adhesion compared with the normal glucose (NG) treatment. U937 cells (human leukemic monocyte lymphoma cell line) were cultured in RPMI-1640 with 10% (v/v) fetal bovine serum, 5.6 mmol/L D-glucose, 25 mmol/L HEPES, and antibiotics. Based on our preliminary results, a final concentration of 500 μmol/L of NaGYY4137 was used in the definitive experiment. NaGYY4137 (500 μmol/L) generates approximately 1 μmol/L or less of H2S during the incubation period (Szabo, 2017).
Glycocalyx Assessment
A cell-based fluorescent assay, based on that described by Singh et al. (2007), was performed to quantify changes within the glycocalyx using fluorescein isothiocyanate-labeled wheat germ agglutinin (WGA-FITC). Bovine retinal endothelial cells were exposed to normal (NG, 5.5 mmol/L) or high glucose (HG, 25 mmol/L) ± GYY4137 (500 μmol/L) in MEM with 0.5% bovine serum albumin (BSA, w/v) for 24 h. Cells were washed 3× with phosphate buffered saline (PBS) and incubated with WGA-FITC [2 μg/ml in HEPES-buffered phenol red-free MEM containing 0.5% BSA (w/v)] for 30 min at 37°C. After washing with PBS (3×), fluorescence intensities were measured (Ex/Em, 485/520 nm) using a fluorescence microplate reader. Cells were lysed and the protein content of each well was measured using the bicinchoninic acid method. Results are presented as fluorescence unit/μg of protein.
Leukocyte Adhesion Assay
Bovine retinal endothelial cells (BRECs) were cultured to confluency in commercially available μ-slide VI0.4 perfusion slides (Ibidi, München, Germany) coated first with 2% (w/v) bovine gelatin (2 h) and then overnight with bovine fibronectin (50 μg/ml). Cells were treated with NG or HG as above. Calcein acetoxymethyl ester labeled (0.5 μmol/L for 30 min) U937 cells (1 × 106/ml) were then flowed over confluent BREC cultures at a laminar shear stress of 1 dyne/cm2 generated by a peristaltic pump. This shear stress was chosen to mimic venular wall shear stresses that favor leucocyte adhesion in vivo (Jones et al., 1995; Sundd et al., 2011). After 6 min, slides were washed with PBS for 1 min to remove non-adherent cells, fixed in 4% (w/v) paraformaldehyde, and examined using a Nikon Eclipse TS100 (Nikon UK Limited, Kingston upon Thames, Surrey, United Kingdom) fluorescence microscope to assess the number of adherent U937 cells (10 random fields of view/slide). All experiments were performed on at least three separate occasions. Statistical significance was tested using the Student t-test.
Animal Ethics
Experimental animals were treated in accordance with ARVO Statement for the Use of Animals in Ophthalmic and Vision Research and under a UK Home Office license at the University of Nottingham Biological Services Unit.
Streptozotocin-Induced Diabetes
A total of 12 male Norway Brown rats (250–300 g, Envigo, United States) were weighed and given a single intraperitoneal (i.p.) injection of STZ (50 mg/kg, Sigma–Aldrich, MO, United States). A total of six control rats were injected with 300 μl of saline i.p. On days 0 and 4 and prior to sacrifice (day 7), blood glucose levels were tested using a sample of blood taken from the tail vein and an Accuchek blood glucose monitor. Rats with blood glucose levels of 15 mmol/L and above were deemed diabetic. Streptozotocin-injected rats that did not become hyperglycemic on day 4 were re-injected with STZ the following morning and subsequently included if deemed diabetic following evaluation for diabetes as outlined above.
Intravitreal Injections
Rats were anaesthetized with a single 10 mg/ml i.p. injection of Domitor (medetomidine hydrochloride, Pfizer, United Kingdom) and Ketaset (ketamine hydrochloride, Zoetis, NJ, United States). Pupils were dilated with topical applications of 5% (w/v) phenylephrine hydrochloride (Bausch and Lomb) and 0.8% (w/v) tropicamide (Bausch and Lomb), and eyes were coated with Lubrithal (Dechra) to prevent dehydration. A 1.5-cm 34-gauge hypodermic needle (Hamilton, NV, United States) attached to a 5-μl syringe (World Precision Instruments, FL, United States) was inserted through pars plana at 3 mm from the limbus into the vitreous of the left eye at a 45° angle. Rats received 5 μl of sterile PBS, 1 μmol/L NaGYY4137 [sodium 4-methoxyphenyl(morpholino)-phosphinodithioate], or 100 nmol/L AP39 [(10-oxo-10-(4-(3-thioxo-3H-1,2-dithiol-5yl)phenoxy)decyl) triphenylphosphonium bromide] on day 0 (prevention arm) or day 6 (treatment arm).
Fundus Fluorescein Angiography
Angiography was performed as described (Allen et al., 2020). Sodium fluorescein (NaF) dye was prepared by dissolving in sterile PBS to give a final concentration of 100 mg/ml and sterile filtered (0.2 μm). Rats were anesthetized as previously described and fundus images of the retina were captured to check for any ocular abnormalities. Rats received a single 250 μl i.p. injection of sodium fluorescein (100 mg/ml), which was allowed to circulate for ∼60 s before imaging with a Micron IV Retinal Imaging Microscope (Phoenix Research Labs). The green filter was selected, and a 3-min video footage of the retina was recorded at 15 frames per second. This was carried out on days 0 and 7. Development of cataracts with resultant blurring of posterior segment view meant that some eyes (n = 2) were excluded from the consecutive fundus fluorescein angiography (FFA).
Retinal Permeability Model
Angiograms were imported into ImageJ software and fluorescence was measured in the interstitium and a major retinal vessel every 200 frames up to 2,400 frames. The ratio of interstitial to vascular fluorescence was adjusted for background level and plotted against time and the slope used to determine an estimate of permeability (Figure 1). Figure 1A shows images taken from rats given NaF and recording started within 1 min of injection. The large vessels of the retina are clearly seen, and the retina becomes brighter over time. To estimate a measure of permeability, the fluorescence intensity in an area with no large vessels was measured. The solute flux (number of fluorescence molecules entering the interstitium per unit time) was calculated from the change in fluorescence intensity in the window outside the major vessel, as a proportion of the fluorescence intensity in the blood vessel in the same frame, to account for any changes in focus, excitation intensity, or noise, plotted against the time in seconds. The permeability surface area product was calculated from the solute flux, the concentration gradient between the intravascular and extravascular compartments (difference in fluorescence intensity), and the area of the extravascular sample measurement according to Fick’s law. In healthy Norway Brown rats, the permeability was the same 7 days after intraocular injection with 2 μl saline (7.47 ± 1.74 × 10–4 s–1) as it was before injection (6.57 ± 2.13 × 10–4 s–1). Streptozotocin treatment resulted in a significant increase in blood glucose (29.73 ± 0.66 mmol/L) after 1 week (compared with 7.92 ± 0.37 mmol/L), which was accompanied by a significant increase in solute flux in the retina (Figure 4), which translated to an increase in estimated permeability from 8.19 ± 0.95 × 10–4 s–1 before STZ to 13.32 ± 1.65 × 10–4 s–1 after STZ induction (Allen et al., 2020).
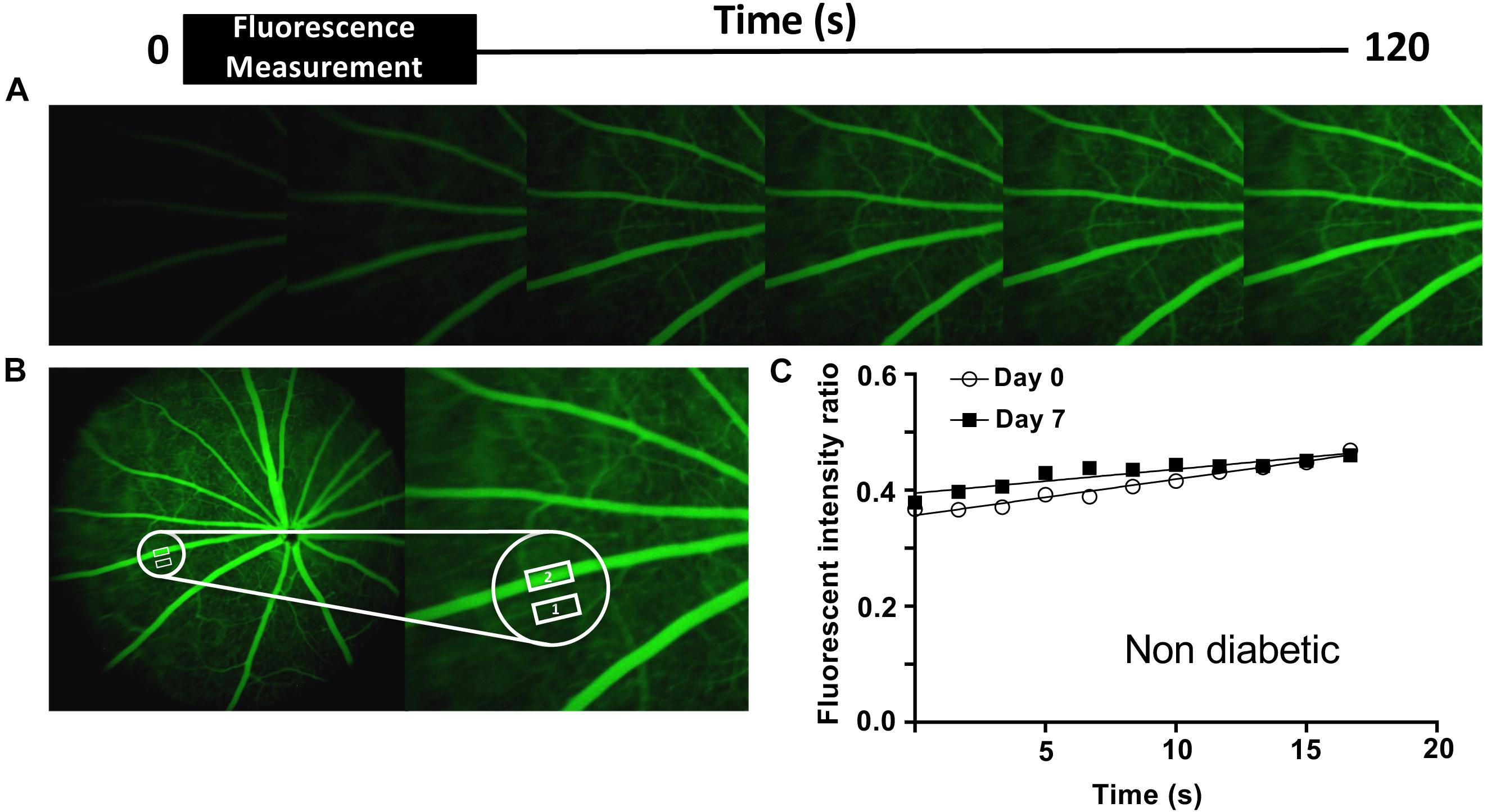
Figure 1. Retinal permeability analysis model. (A) Fundus fluorescein angiography was performed in non- and diabetic Norway Brown rats treated ± H2S donors, on days 0 and 7. The angiograms shown are representative of a non-diabetic animal. (B) The mean intensity of NaF in the retinal tissue (Adamis and Berman, 2008) and a main retinal vessel (Ahmad et al., 2016) were measured every 200 frames for 3 min. (C) Apparent permeability (Permeability.Surface Area product, PA) was calculated from the rate of change of extravascular fluorescence (= ΔIf/Δt) per unit concentration difference (ΔC) between blood and tissue. P = ΔIf/Δt/(ΔC x A). ΔIf/Δt ∼ slope. ΔC = difference between intra- and extravascular intensity.
Immunofluorescence
After animal termination and ocular dissection (day 14), retinae were flat-mounted and blocked in 5% (v/v) goat serum, 3% (v/v) Triton X-100, 1% (w/v) BSA, and stained with isolectin-B4 (IB4) (Sigma Aldrich, biotin conjugated) 5 μg/ml and rabbit anti-collagen IV (Abcam) 5 μg/ml overnight at 4°C. Streptavidin conjugated Alexafluor 488 2 μg/ml and donkey anti-rabbit Alexafluor 555 4 μg/ml were used to detect IB4 and collagen IV staining, respectively. Coverslips were mounted with Fluoroshield with DAPI. Images were obtained using a Leica TCS SPE confocal microscope, and all settings were maintained between images.
Statistics
All statistics and graphs were produced in GraphPad Prism 6 and statistical tests are shown in figure legends. Significant differences are indicated on graphs as asterisks, where: ∗p ≤ 0.05; ∗∗p ≤ 0.01; ∗∗∗p ≤ 0.001.
Results
Glycocalyx Integrity
Two hydrogen sulfide generating molecules were investigated, NaGYY4137 and AP39. The former is a slow-releasing hydrogen sulfide donor (Alexander et al., 2015) that does not target any specific part of the cell, whereas AP39 is a mitochondrial targeted donor (Le Trionnaire et al., 2014).
HG significantly reduced BREC glycocalyx in vitro (assessed by FITC-WGA staining) to 89.67% ± 6.6% compared with NG control conditions (100%), p ≤ 0.001, n = 12–30 (Figure 2). Simultaneous treatment with the slow-release H2S donor NaGYY4137 completely reversed this loss and actually restored the glycocalyx to higher levels than observed in the NG control (107.49 ± 8.13% vs. 100%, p ≤ 0.001, n = 12–30). Interestingly, cells incubated in NG conditions in the presence of NaGYY4137 also had significantly higher glycocalyx staining than control NG-treated cells (106.7 ± 6.35 vs. 100%, p ≤ 0.001, n = 12–30).
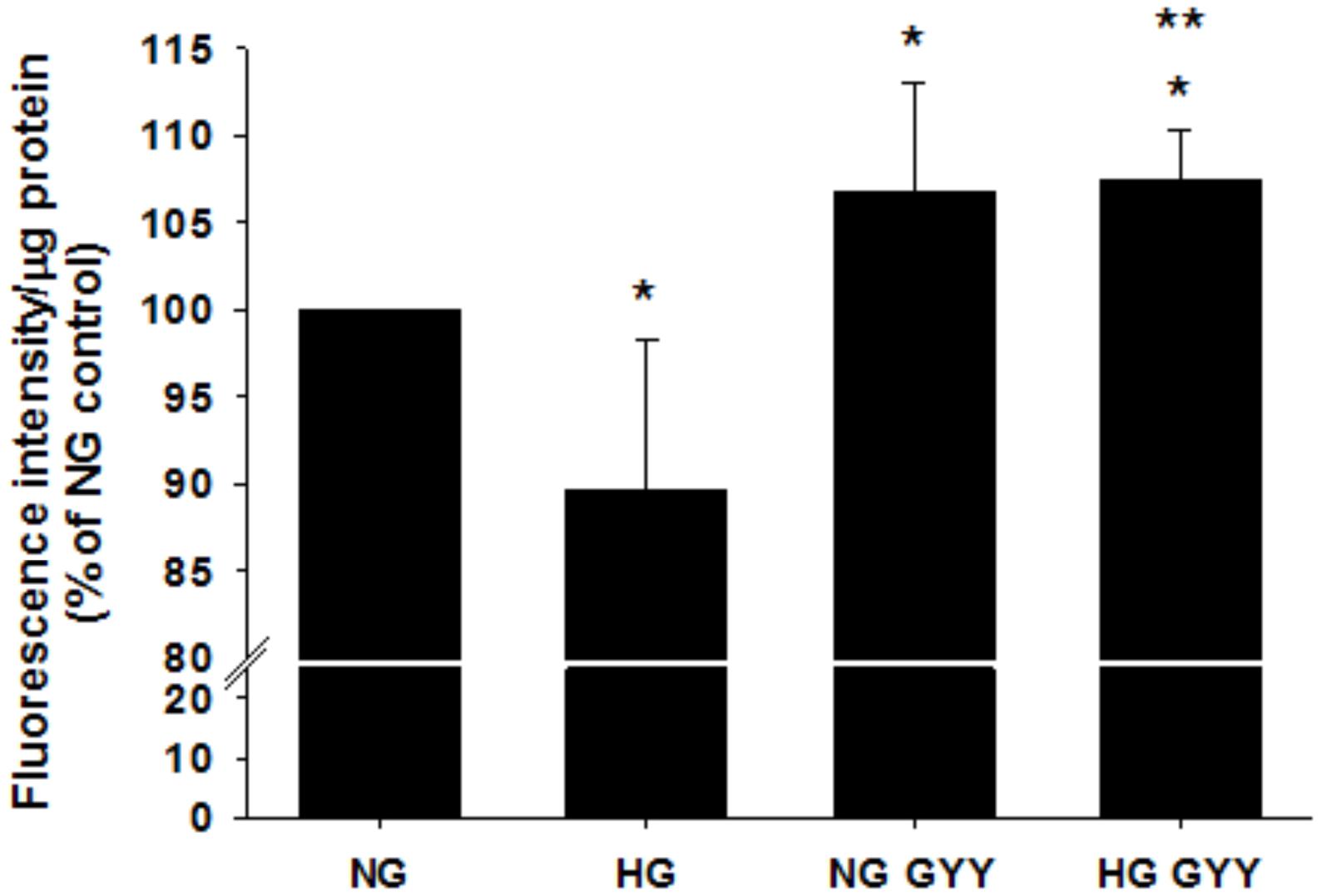
Figure 2. Administration of the slow-release H2S donor NaGYY4137 completely reversed the loss of retinal EC glycocalyx integrity induced by hyperglycemia. Retinal ECs were incubated with normal (5.5 mmol/L, NG) or high glucose (25 mmol/L, HG) ± GYY4137 (500 μmol/L) for 24 h before assessment of glycocalyx integrity with fluorescently labeled lectin (WGA-FITC). Data are mean ± SD, presented as fluorescence intensity/μg protein. *p ≤ 0.01 vs. control, **p ≤ 0.01 vs. HG, n = 12–30. One-way ANOVA.
Since the integrity of the glycocalyx may alter endothelial cell adhesion molecule exposure and thus influence leukocyte/endothelial binding, adhesion of U937 leukocytes to a BREC monolayer was examined under the same experimental conditions. HG significantly increased leukocyte adhesion to 348 ± 110% vs. NG control (100%), p ≤ 0.01, n = 4 (Figure 3). This increase was fully attenuated to control levels by incubation with NaGYY4137 (HG + NaGYY4137; 124 ± 47% vs. HG; 348 ± 110%, p ≤ 0.01, n = 4). NaGYY4137 had no significant effect on leukocyte binding in NG conditions.
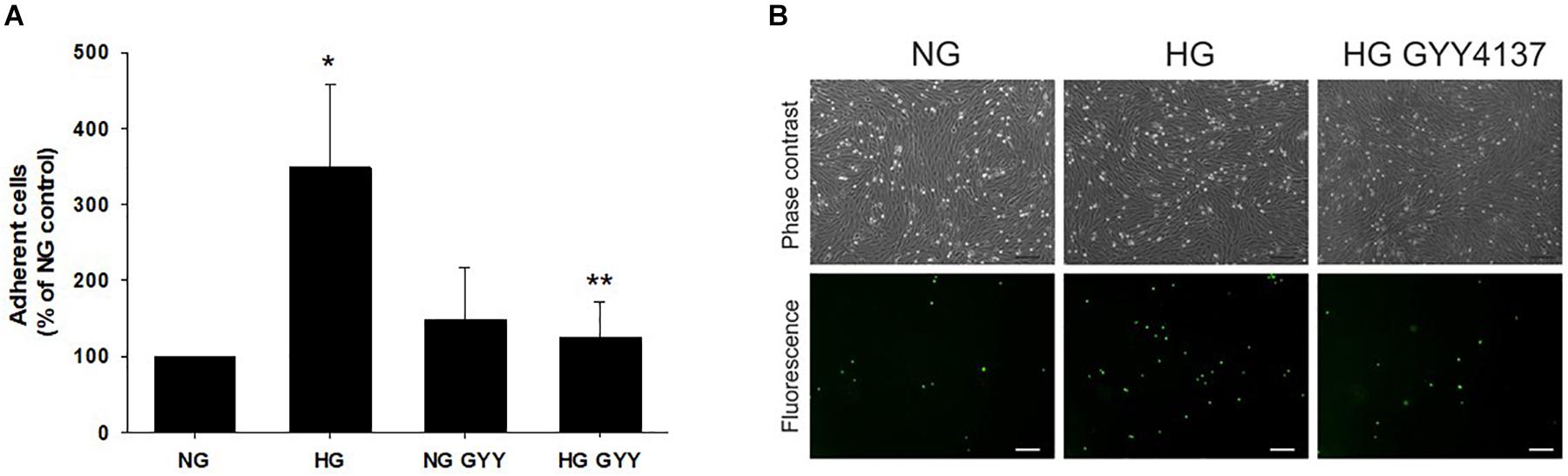
Figure 3. Administration of the slow-release H2S donor NaGYY4137 completely attenuated the increased leukocyte adhesion to retinal ECs induced by hyperglycemia. Retinal ECs were incubated with normal (5.5 mmol/L, NG) or high glucose (25 mmol/L, HG) ± NaGYY4137 (500 μmol/L) for 24 h before examination of leukocyte adhesion under flow (1 dyne/cm2). (A) Number of adherent U937 cells. Data are mean ± SD. *p ≤ 0.001 vs. control, **p ≤ 0.001 vs. HG, n = 4. One-way ANOVA. (B) Representative bright field, phase contrast (upper panel) and corresponding fluorescence images (lower panel) of adherent U937 cells stained with calcein acetoxymethyl ester adhering to a BREC monolayer. Scale bar, 75 μm; original magnification, ×100.
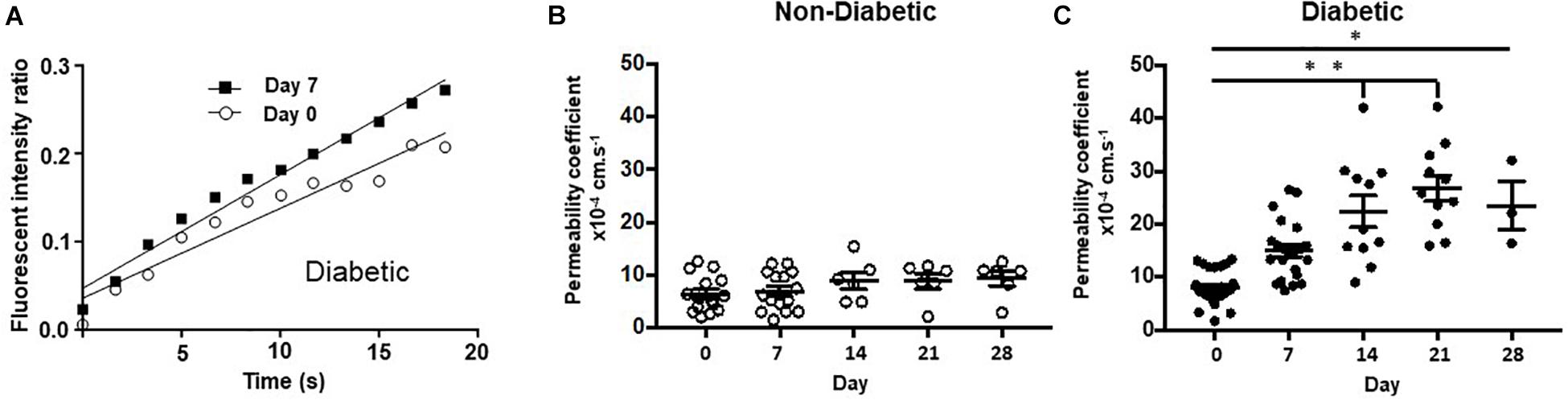
Figure 4. Chemically induced diabetes significantly increased retinal vascular permeability in Norway Brown rats compared to non-diabetic controls. (A) Fundus fluorescein angiography measurements from a single rat carried out on day 0 (before STZ) and day 7 (1 week of STZ treatment). (B) Permeability was measured from control (blood glucose 7.99 ± 0.21 mmol/L), and (C) diabetic (blood glucose 29.75 ± 1.63 mmol/L) Norway Brown rats on days 0, 7, 14, 21, and 28. Apparent permeability was calculated as described in the methodology text and depicted in Figure 1. Data including mean ± SEM. *p ≤ 0.001 vs. day 0, **p ≤ 0.0001 vs. diabetic day 0. One-way ANOVA.
Retinal Permeability
To determine the effect of hydrogen sulfide donors on retinal permeability, fluorescein fundus angiography was carried out before and after induction of diabetes with STZ. NaF was injected intraperitoneally into Norway Brown rats and the fluorescence intensity inside a large vessel and in the tissue outside a large vessel was determined over time by video-microscopy.
Retinal vascular permeability was significantly increased in the diabetic Norway Brown rats (23.51 ± 4.59 × 10–4 s–1, n = 3) compared with normal control rats (9.38 ± 1.40 × 10–4 s–1, n = 6, Figure 4) on day 28 (p ≤ 0.001) on day 28 and all other time points. In the H2S donor treatment study no changes in retinal permeability were measured on day 7 compared to baseline in non-diabetic animals treated with vehicle control (saline) (Figure 5A). However, a significant (p ≤ 0.05) increase in retinal permeability was measured in non-insulin treated diabetic animals on day 7 (12.16 ± 0.98 × 10−4 s−1) compared to baseline (9.23 ± 1.54 × 10−4 s−1) (Figures 5B,C). The slow-release hydrogen sulfide donor NaGYY4137 significantly reduced retinal vascular permeability in control, non-diabetic rats after 7 days (3.24 ± 0.66 × 10–4 s–1, p ≤ 0.05, n = 6) vs. day 0 (10.12 ± 1.85 × 10–4 s–1) when administered as a single preventative intraocular dose pre-diabetes (Figure 5D). Similarly, NaGYY4137 given in diabetic rats significantly reduced retinal permeability on day 7 (6.62 ± 1.16 × 10–4 s–1, p ≤ 0.05, n = 6) vs. day 0 (13.08 ± 2.61 × 10–4 s–1) when given as a preventative treatment (Figure 5D). When NaGYY4137 was administered therapeutically, retinal permeability was reduced in control, non-diabetic animals on day 7 (5.31 ± 1.24 × 10–4 s–1, n = 6) vs. day 0 (8.90 ± 1.19 × 10–4 s–1, n = 6). More importantly, in animals with pre-existing diabetes, retinal permeability stabilized on day 7 (10.27 ± 1.21 × 10–4 s–1, n = 6) compared to day 0 (8.65 ± 1.23 × 10–4 s–1, n = 6) (Figure 5E). Administering NaGYY4137 treatment prior to the onset of diabetes gave a 68% benefit in reducing retinal permeability compared with administration to animals with pre-existing diabetes (Figure 5F).
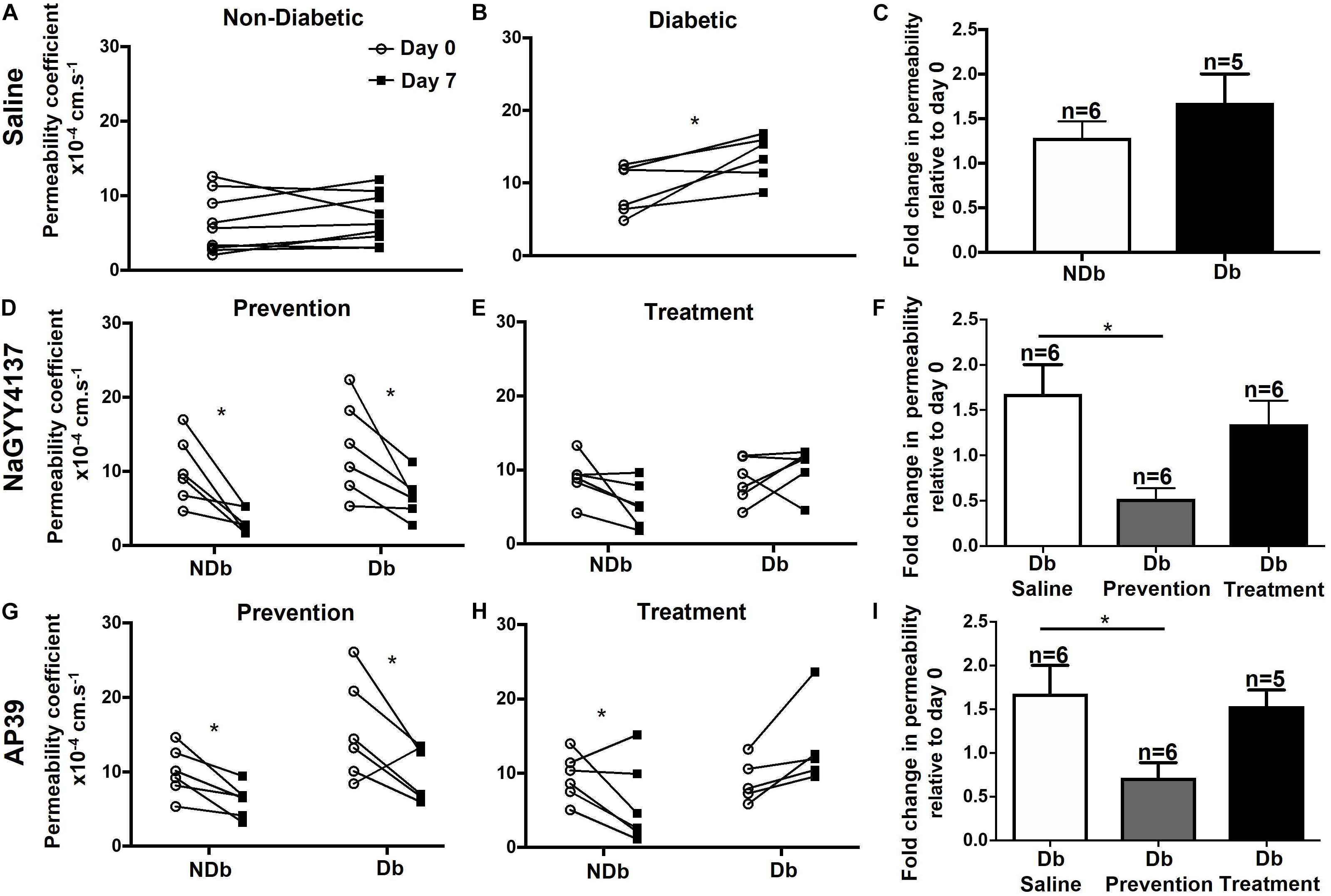
Figure 5. Ocular administration of the slow-release H2S donors reduced retinal permeability in chemically induced diabetic rats. Type I diabetes was successfully induced in rats following a single dose of STZ and animals were maintained for 1 week without insulin supplementation. Retinal vascular leak was measured on day 0 and day 7 in non- and diabetic animals following treatment. No observed increases in retinal permeability were measured on day 7 in non-diabetic animals treated with saline (A,C). In diabetic animals treated with saline a significant (*p ≤ 0.05) increase in retinal permeability was measured on day 7 vs. day 0 (B,C). NaGYY4137 and AP39 significantly (*p < 0.05, paired t-test) reduced retinal permeability in non- and diabetic rats (D,G,F) on day 7 when given as a single intraocular preventative dose, on day 0. In addition, NaGYY4137 and AP39 reduced retinal permeability in non-diabetic rats (E,F,H,I) when administered therapeutically (day 6). In diabetic rats, NaGYY4137 and AP39 stabilized retinal permeability on day 7 in rats with pre-existing diabetes (E,F,H,I).
Similarly, the mitochondrial-targeted hydrogen sulfide donor AP39 significantly reduced retinal permeability in control, non-diabetic (6.14 ± 0.90 × 10–4 s–1, p ≤ 0.05, n = 6) and diabetic rats (9.84 ± 1.48 × 10–4 s–1, p ≤ 0.05, n = 6) compared with day 0 (10.01 ± 1.34 × 10–4 s–1 and 15.52 ± 2.75 × 10–4 s–1, respectively) when administered as a single preventative dose (Figure 5G). In addition, AP39 reduced retinal permeability after a week (5.93 ± 2.25 × 10–4 s–1, n = 6) compared with baseline (9.49 ± 1.28 × 10–4 s–1, p ≤ 0.05, n = 6) in control animals when administered therapeutically. However, in diabetic animals, AP39, when administered therapeutically, stabilized retinal permeability (13.63 ± 2.56 × 10–4 s–1, n = 5) on day 7 versus baseline (8.99 ± 1.31 × 10–4 s–1, n = 5) (Figure 5H). Similar to NaGYY4137, administering AP39 treatment prior to the onset of diabetes gave an 18% benefit in reducing retinal permeability when compared with animals with pre-existing diabetes (Figure 5I). Overall, both H2S donors were more effective in reducing retinal permeability when given to animals prior to the onset of diabetes. In addition, NaGYY4137 showed greater efficacy than a 10-fold lower dose of the mitochondrial targeted AP39 compound.
Acellular Capillary Formation
One of the contributing factors to the pathogenesis of DR is the presence of acellular capillaries within the retina. These vessels maintain a basement membrane but have lost the supporting pericytes and EC cells and thus have no blood flow, promoting retinal ischemia. In order to assess the effects of NaGYY4137 on acellular capillary formation, retinae were collected at the end of the retinal permeability study and stained for isolectin B4, an EC marker, and collagen IV, a major constituent of the basement membrane. NaGYY4137 was found to reduce the number of acellular capillaries (collagen IV + /IB4-) in the diabetic retinae in both the prevention and treatment group to 80.2 ± 13.9 mm–2 and 66.1 ± 7.1 mm–2, respectively, compared with 113.6 ± 11.7 mm–2 in the diabetic retinae (p ≤ 0.05, n = 3, Figure 6). Despite the increased effectiveness of NaGYY4137 in reducing retinal permeability when administered prior to diabetes onset, the time of administration appeared to have no difference in acellular capillary formation.
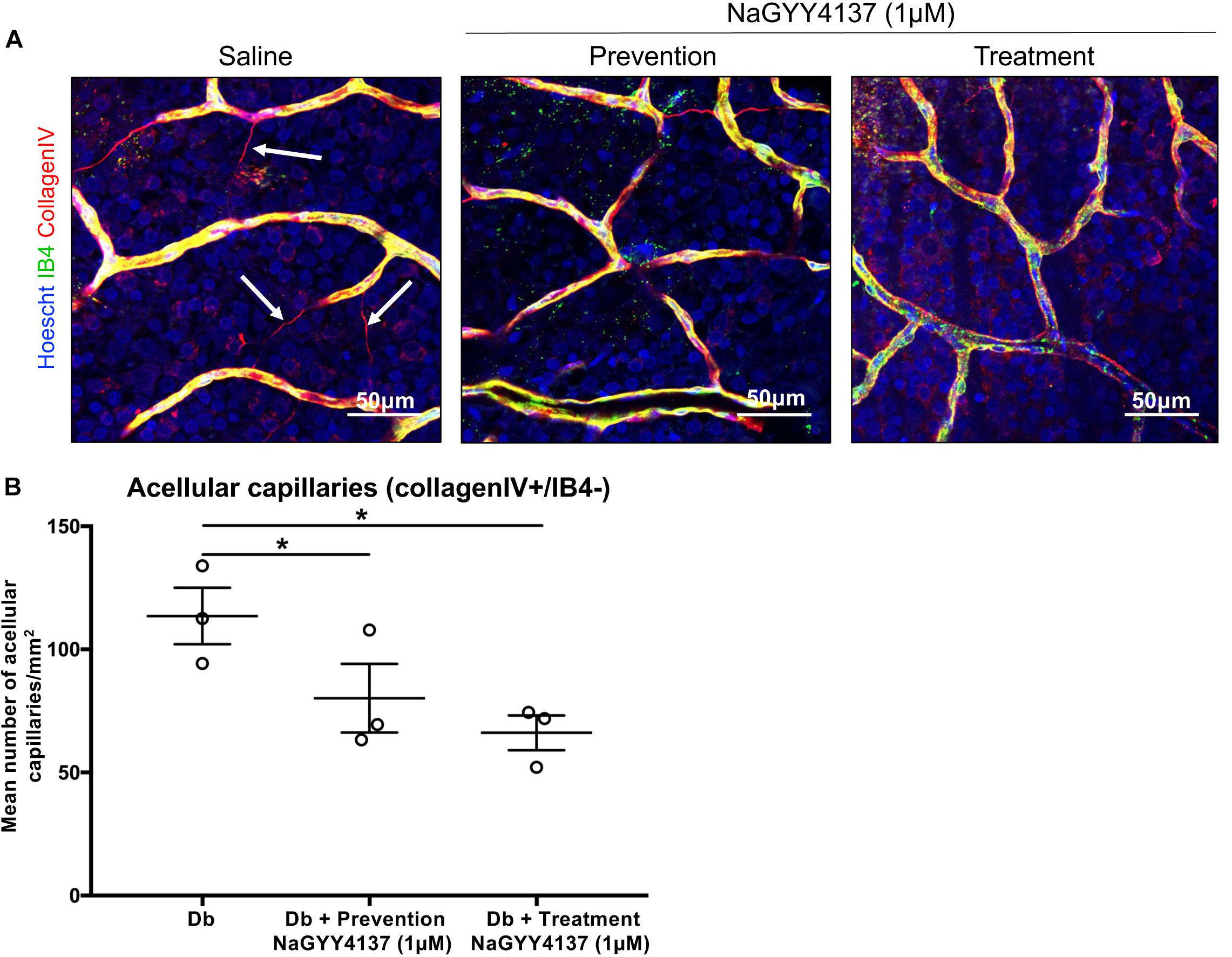
Figure 6. NaGYY4137 protected against diabetes-induced increase in acellular capillaries. Norway Brown rats were given a single dose of streptozotocin (50 mg/kg) to induce Type I diabetes. Rats were treated with an intraocular injection of either saline or 1 μmol/L H2S donor NaGYY4137 at day 0 (prevention) or day 6 (treatment). (A) Rats were enucleated at day 7 and whole retinae were mounted and stained with Hoescht, IB4, and collagen IV. The tissue was imaged using 20 × lens. The number of capillaries positively stained for collagen IV but lacking IB4 (white arrows) were counted in three areas of the retina. (B) Acellular capillaries are expressed per mm2. Error bars represent standard errors of the mean. Statistical analysis was performed using a one-way analysis of variance corrected for false discovery rate. *p ≤ 0.05.
Discussion
The data presented here indicate, for the first time, that (a) high glucose-driven glycocalyx loss, (b) high glucose-enhanced leukocyte adhesion to the endothelium, and (c) diabetes-associated increases in retinal permeability can all be reversed by administration of a slow-release H2S donor.
Hyperglycemia-related low-grade persistent inflammation is thought to contribute to the pathology of early DR (Adamis and Berman, 2008). Patients with DR have elevated ocular levels of inflammatory mediators and the diabetic retina displays characteristic features of inflammation including increased vascular permeability and leukocyte adhesion (Adamis and Berman, 2008). Since a healthy glycocalyx is critical to maintenance of both the anti-inflammatory and permeability characteristics of a healthy endothelium, our data highlight the potential of H2S donors as new therapeutic tools for the treatment of DR. Further investigations into optimal dosing and comparing other H2S delivery molecules are needed to further explore this potential.
The available literature describing other research in this area is scarce. However, glycocalyx loss and enhanced leukocyte adhesion have been reported in a rat model of diabetes (Kumase et al., 2010). Additionally, a clear link between in vivo retinal glycocalyx integrity and vascular permeability has been reported by Leskova et al. (2019), who demonstrated, in mice, that enzymatic degradation of the glycocalyx is associated with enhanced retinal permeability. These data support the hypothesis that maintaining glycocalyx integrity could be a viable therapeutic strategy for DR. EC glycocalyx thickness depends on the rate of shedding and circulatory levels of glycosaminoglycans (GAG)-degrading enzyme levels (Ikegami-Kawai et al., 2003; Maxhimer et al., 2005). The previously reported association of glycocalyx reduction with increased vascular permeability and leucocyte and platelet adhesion and subsequent reversal by glycocalyx restoration in experimental animals through increased GAG synthesis (Henry and Duling, 1999; Constantinescu et al., 2003) corroborate our findings. Furthermore, Ciszewicz et al. (2009), have shown that sulodexide attenuates hyperglycemia-associated EC permeability and inflammation. Similarly, Broekhuizen et al. (2010), demonstrated that sulodexide increased vascular EC glycocalyx and reversed the increased vascular permeability in type 2 diabetes. Additionally, Niu et al. (2019), using the STZ-induced diabetic rat model used here, showed that endomucin overexpression restored the diabetes-associated loss of retinal endothelial glycocalyx and that this restoration was associated with decreased leukocyte–endothelial adhesion and a reduction in vessel leakage in rats with DR.
The effects of H2S on the glycocalyx are still unknown. Although early studies showed potential antioxidant effects of H2S, more recent work has shown that the reaction rate between H2S and oxidant species, e.g., peroxide and peroxynitrite, may be too slow for oxidant scavenging activity alone to be primarily responsible for glycocalyx- and vaso-protection, especially given the low in vivo concentrations of H2S, which we have modeled using NaGYY4317 (Carballal et al., 2011). This strongly suggests that H2S is acting via other mechanisms to maintain the glycocalyx during hyperglycemic damage, e.g., enhancing synthesis of glycocalyx components or preventing hyperglycemia-induced glycocalyx degradation. For instance, the glycocalyx component syndecan-1 is cleaved by matrix metalloproteinase-9 (MMP-9) and both activity and expression of the protease are known to be reduced by H2S administration (Du et al., 2017). It has also been reported that MMP-7 can cleave chondroitin sulfate (Lipowsky, 2011) and syndecans 1 and 4 (Manon-Jensen et al., 2013) and our unpublished data indicate that NaGYY4137 inhibits MMP-7 in in vitro assays at concentrations similar to those used in the studies described here. Interestingly, our additional preliminary data indicating that NaGYY4137 reduces shedding of hyaluronic acid from cultured ECs suggest another potential action of H2S since the significant reduction in EC glycocalyx observed in acute hyperglycemia (Nieuwdorp et al., 2006b) and in types 1 and type 2 diabetes (Nieuwdorp et al., 2006a; Broekhuizen et al., 2010) is thought to be related to increased hyaluronidase catabolism (Nieuwdorp et al., 2006a).
It is interesting to note that NaGYY4137 treatment in NG enhanced glycocalyx staining above the levels observed in NG alone (Figure 2), suggesting that H2S supplementation alone increased glycocalyx density. As mentioned previously, the glycocalyx is a dynamic structure and thus this observation could potentially be explained by the ability of H2S to modify glycocalyx-degrading enzymes to reduce their activity. Interestingly, H2S is the only molecule known to induce protein S-persulfidation (S-sulfhydration), a recently identified and unique post-translational protein modification. It is possible that the activity of glycocalyx-degrading enzymes such as heparinase might be altered by persulfidation of the cysteine residues of its active site. Additionally, cysteine residues in proteins have the potential to be redox modified by H2S in a non-S-persulfidation manner and several potential glycocalyx-degrading enzymes, including the proteinases MMP-1 (Lohakul et al., 2021), MMP-2, MMP-7, and MMP-9, have a cysteine in their active site. Thus, as has been shown with other zinc proteases including angiotensin-converting enzyme (Laggner et al., 2007) and TNF-α-converting enzyme (Li et al., 2013), H2S has the potential to modulate the glycocalyx-degrading enzyme activity of the proteinases, although further work is needed to confirm this hypothesis.
The effectiveness of our mitochondrial targeted H2S donor in decreasing retinal leakage in vivo raises the possibility of mitochondrial involvement in the observed effects. Modis et al. reported that endogenous mitochondrial H2S production was governed by MST (Módis et al., 2013). Additionally, Si et al. (2013) reported that DR was associated with significant decreases in retinal H2S levels and expression of the H2S synthesizing enzymes CSE, CBS, and 3MST (i.e., DR resulted in retinal H2S deficiency) and also with increased mitochondrial permeability and respiration (Si et al., 2013), strongly suggesting extensive mitochondrial dysfunction and H2S deficiency in the retina in DR. Vascular mitochondrial impairment induced by diabetes has previously been shown to be inhibited/reversed by sulfide administration albeit at high doses/concentrations (e.g., 300 μmol/L) (Módis et al., 2013; Coletta et al., 2015), suggesting that pharmacological sulfide could overcome impaired retinal bioenergetics in DR. Although NaSH administration has previously been shown to reduce retinal vascular abnormalities associated with DR and “correct” mitochondrial dysfunction (Si et al., 2013), this earlier study is intriguing for several reasons. Firstly, the NaSH was given as a daily intraperitoneal injection (over 14 weeks) at a dose equivalent to 280 mmol/kg. This was an exceptionally high and surprisingly non-lethal dose (although toxicity was not examined) given that 14 μmol/kg i.p. induces systemic inflammation and vascular collapse (Li et al., 2005) and the LD50 for NaSH is 52.6 μmol/kg for the same route of administration (Strickland et al., 2003). Possible toxicological constraints aside, the half-life of bolus sulfide in blood is less than a minute as it is rapidly metabolized/removed (Wang, 2002, 2012), so the precise mechanisms by which a bolus of H2S (from NaSH), administered intraperitoneally survives intact to penetrate the blood–brain barrier and selectively increase sulfide levels in the retina via this route remain elusive. Given that plasma H2S levels have been measured at nanomolar to low micromolar concentrations, our approach to prevent/reverse DR-induced retinal vascular leakage using slow-release H2S donors (Wei et al., 2014; John et al., 2017; Qiu et al., 2018) or H2S delivery molecules that selectively target mitochondria (Le Trionnaire et al., 2014; Szczesny et al., 2014; Ikeda et al., 2015; Ahmad et al., 2016; Karwi et al., 2017) would overcome these concerns.
Conclusion
The data presented here together with previous in vivo data (Whiteman et al., 2010a) suggest that, in health, H2S is vasculoprotective and that microvascular dysfunction in diabetes, including DR, is associated with reduced circulating and retinal H2S levels. Thus, diabetes may be a condition of H2S deficiency and H2S supplementation using slow-release and/or mitochondrial-targeted H2S delivery molecules may represent a novel, cost-effective alternative therapy for DR.
Data Availability Statement
The original contributions presented in the study are included in the article/supplementary material. The raw data supporting the conclusions of this article will be made available by the authors, without undue reservation.
Ethics Statement
The animal study was reviewed and approved by University of Nottingham Biological Services Unit.
Author Contributions
JW, DB, and MWh conceived and planned the experiments. CA, JW, DB, and MWh took the lead in writing the manuscript. CA, KW, and NM carried out the experiments. RT and MWo synthesized the slow-release hydrogen sulfide donors. AB and WA contributed to the interpretation of the results. All authors provided critical feedback and helped shape the research, analysis, and manuscript.
Funding
We wish to thank the Medical Research Council, UK (MR/M022706/1 to MWh and MWo; MR/L01985X/1 to JW, DB, and MWh), British Heart Foundation (PG/18/31/33759 to AB and DB), Royal Society (RGS\R1\191221 to AB) and the Brian Ridge Scholarship (RT), and the National Eye Research Centre and Masonic Charitable Foundation for funding this study.
Conflict of Interest
MWh, RT, and MWo, and the University of Exeter have intellectual property (patent filings) related to hydrogen sulfide delivery molecules and their therapeutic use. MWh was a consultant to MitoRx Therapeutics (Oxford).
The remaining authors declare that the research was conducted in the absence of any commercial or financial relationships that could be construed as a potential conflict of interest.
Publisher’s Note
All claims expressed in this article are solely those of the authors and do not necessarily represent those of their affiliated organizations, or those of the publisher, the editors and the reviewers. Any product that may be evaluated in this article, or claim that may be made by its manufacturer, is not guaranteed or endorsed by the publisher.
Acknowledgments
We wish to thank Bridget Fox for her assistance with the preliminary studies.
References
Adamis, A. P., and Berman, A. J. (2008). Immunological mechanisms in the pathogenesis of diabetic retinopathy. Semin. Immunopathol. 30, 65–84. doi: 10.1007/s00281-008-0111-x
Ahmad, A., Olah, G., Szczesny, B., Wood, M. E., Whiteman, M., and Szabo, C. (2016). AP39, a mitochondrially targeted hydrogen sulfide donor, exerts protective effects in renal epithelial cells subjected to oxidative stress in vitro and in acute renal injury in vivo. Shock 45, 88–97. doi: 10.1097/shk.0000000000000478
Alexander, B. E., Coles, S. J., Fox, B. C., Khan, T. F., Maliszewski, J., Perry, A., et al. (2015). Investigating the generation of hydrogen sulfide from the phosphonamidodithioate slow-release donor GYY4137. Med. Chem. Commun. 6, 1649–1655. doi: 10.1039/c5md00170f
Allen, C. L., and Bayraktutan, U. (2009). Antioxidants attenuate hyperglycaemia-mediated brain endothelial cell dysfunction and blood-brain barrier hyperpermeability. Diabetes Obes. Metab. 11, 480–490. doi: 10.1111/j.1463-1326.2008.00987.x
Allen, C. L., Malhi, N. K., Whatmore, J. L., Bates, D. O., and Arkill, K. P. (2020). Non-invasive measurement of retinal permeability in a diabetic rat model. Microcirculation. 27:e12623.
Ballantyne, B., Gazzard, M. F., and Swanston, D. W. (1976). The opthalmic toxicology of dichloromethane. Toxicology 6, 173–187. doi: 10.1016/0300-483x(76)90019-6
Betteridge, K. B., Arkill, K. P., Neal, C. R., Harper, S. J., Foster, R. R., Satchell, S. C., et al. (2017). Sialic acids regulate microvessel permeability, revealed by novel in vivo studies of endothelial glycocalyx structure and function. J. Physiol. 595, 5015–5035. doi: 10.1113/JP274167
Brancaleone, V., Roviezzo, F., Vellecco, V., De Gruttola, L., Bucci, M., and Cirino, G. (2008). Biosynthesis of H2S is impaired in non-obese diabetic (n.d.) mice. Br. J. Pharmacol. 155, 673–680. doi: 10.1038/bjp.2008.296
Brandt, K. R., and Okamoto, M. Y. (1988). Final report on the safety assessment of methylene chloride. J. Am. Coll. Toxicol. 7, 741–835. doi: 10.3109/10915818809078710
Broekhuizen, L. N., Lemkes, B. A., Mooij, H. L., Meuwese, M. C., Verberne, H., Holleman, F., et al. (2010). Effect of sulodexide on endothelial glycocalyx and vascular permeability in patients with type 2 diabetes mellitus. Diabetologia 53, 2646–2655. doi: 10.1007/s00125-010-1910-x
Brownlee, M. (2001). Biochemistry and molecular cell biology of diabetic complications. Nature 414, 813–820. doi: 10.1038/414813a
Brownlee, M. (2005). The pathobiology of diabetic complications: a unifying mechanism. Diabetes 54, 1615–1625. doi: 10.2337/diabetes.54.6.1615
Carballal, S., Trujillo, M., Cuevasanta, E., Bartesaghi, S., Möller, M. N., Folkes, L. K., et al. (2011). Reactivity of hydrogen sulfide with peroxynitrite and other oxidants of biological interest. Free Radic. Biol. Med. 50, 196–205. doi: 10.1016/j.freeradbiomed.2010.10.705
Chibber, R., Ben-Mahmud, B. M., Coppini, D., Christ, E., and Kohner, E. M. (2000). Activity of the glycosylating enzyme, core 2 GlcNAc (beta1,6) transferase, is higher in polymorphonuclear leukocytes from diabetic patients compared with age-matched control subjects: relevance to capillary occlusion in diabetic retinopathy. Diabetes 49, 1724–1730. doi: 10.2337/diabetes.49.10.1724
Ciszewicz, M., Polubinska, A., Antoniewicz, A., Suminska-Jasinska, K., and Breborowicz, A. (2009). Sulodexide suppresses inflammation in human endothelial cells and prevents glucose cytotoxicity. Transl. Res. 153, 118–123. doi: 10.1016/j.trsl.2008.12.007
Coletta, C., Módis, K., Szczesny, B., Brunyánszki, A., Oláh, G., Rios, E. C., et al. (2015). Regulation of vascular tone, angiogenesis and cellular bioenergetics by the 3-mercaptopyruvate sulfurtransferase/H2S pathway: functional impairment by hyperglycemia and restoration by DL-α-lipoic acid. Mol. Med. 21, 1–14. doi: 10.2119/molmed.2015.00035
Constantinescu, A. A., Vink, H., and Spaan, J. A. (2003). Endothelial cell glycocalyx modulates immobilization of leukocytes at the endothelial surface. Arterioscler. Thromb. Vasc. Biol. 23, 1541–1547. doi: 10.1161/01.atv.0000085630.24353.3d
Du, J., Jin, H., and Yang, L. (2017). Role of hydrogen sulfide in retinal diseases. Front. Pharmacol. 8:588. doi: 10.3389/fphar.2017.00588
Gerő, D., Torregrossa, R., Perry, A., Waters, A., Le-Trionnaire, S., Whatmore, J. L., et al. (2016). The novel mitochondria-targeted hydrogen sulfide (H2S) donors AP123 and AP39 protect against hyperglycemic injury in microvascular endothelial cells in vitro. Pharmacol. Res. 113, 186–198. doi: 10.1016/j.phrs.2016.08.019
Gillies, M. C., Su, T., Stayt, J., Simpson, J. M., Naidoo, D., and Salonikas, C. (1997). Effect of high glucose on permeability of retinal capillary endothelium in vitro. Invest. Ophthalmol. Vis. Sci. 38, 635–642.
Henry, C. B., and Duling, B. R. (1999). Permeation of the luminal capillary glycocalyx is determined by hyaluronan. Am. J. Physiol. 277, H508–H514.
Ikeda, K., Marutani, E., Hirai, S., Wood, M. E., Whiteman, M., and Ichinose, F. (2015). Mitochondria-targeted hydrogen sulfide donor AP39 improves neurological outcomes after cardiac arrest in mice. Nitric Oxide 49, 90–96. doi: 10.1016/j.niox.2015.05.001
Ikegami-Kawai, M., Suzuki, A., Karita, I., and Takahashi, T. (2003). Increased hyaluronidase activity in the kidney of streptozotocin-induced diabetic rats. J. Biochem. 134, 875–880. doi: 10.1093/jb/mvg214
Jeansson, M., and Haraldsson, B. (2003). Glomerular size and charge selectivity in the mouse after exposure to glucosaminoglycan-degrading enzymes. J. Am. Soc. Nephrol. 14, 1756–1765. doi: 10.1097/01.asn.0000072742.02714.6e
John, A., Kundu, S., Pushpakumar, S., Fordham, M., Weber, G., Mukhopadhyay, M., et al. (2017). GYY4137, a hydrogen sulfide donor modulates miR194-dependent collagen realignment in diabetic kidney. Sci. Rep. 7:10924.
Jones, D. A., Smith, C. W., and McIntire, L. V. (1995). Effects of Fluid Shear Stress on Leukocyte Adhesion to Endothelial Cells. Physiology and Pathophysiology of Leukocyte Adhesion. New York, NY: Oxford University Press, 148–168.
Kabil, O., and Banerjee, R. (2014). Enzymology of H2S biogenesis, decay and signaling. Antioxid. Redox Signal. 20, 770–782. doi: 10.1089/ars.2013.5339
Kanagy, N. L., Szabo, C., and Papapetropoulos, A. (2017). Vascular biology of hydrogen sulfide. Am. J. Physiol. Cell Physiol. 312, C537–C549.
Karwi, Q. G., Bornbaum, J., Boengler, K., Torregrossa, R., Whiteman, M., Wood, M. E., et al. (2017). AP39, a mitochondria-targeting hydrogen sulfide (H2S) donor, protects against myocardial reperfusion injury independently of salvage kinase signalling. Br. J. Pharmacol. 174, 287–301. doi: 10.1111/bph.13688
Kielhorn, J., and Rosner, G. (1996). Environmental Health Criteria: Morpholine. International Programme on Chemical Safety. Geneva: World Health Organization.
Kumase, F., Morizane, Y., Mohri, S., Takasu, I., Ohtsuka, A., and Ohtsuki, H. (2010). Glycocalyx degradation in retinal and choroidal capillary endothelium in rats with diabetes and hypertension. Acta Med. Okayama 64, 277–283.
Kundu, S., Pushpakumar, S. B., Tyagi, A., Coley, D., and Sen, U. (2013). Hydrogen sulfide deficiency and diabetic renal remodeling: role of matrix metalloproteinase-9. Am. J. Physiol. Endocrinol. Metab. 304, E1365–E1378.
Laggner, H., Hermann, M., Esterbauer, H., Muellner, M. K., Exner, M., Gmeiner, B. M., et al. (2007). The novel gaseous vasorelaxant hydrogen sulfide inhibits angiotensin-converting enzyme activity of endothelial cells. J. Hypertens. 25, 2100–2104. doi: 10.1097/hjh.0b013e32829b8fd0
Landsverk, S. A., Tsai, A. G., Cabrales, P., and Intaglietta, M. (2012). Impact of enzymatic degradation of the endothelial glycocalyx on vascular permeability in an awake hamster model. Crit. Care Res. Pract. 2012:842545.
Le Trionnaire, S., Perry, A., Szczesny, B., Szabo, C., Winyard, P. G., Whatmore, J. L., et al. (2014). The synthesis and functional evaluation of a mitochondria-targeted hydrogen sulfide donor, (10-oxo-10-(4-(3-thioxo-3H-1,2-dithiol-5-yl)phenoxy)decyl)triphenylphosphonium bromide (AP39). Med. Chem. Comm. 5, 728–736. doi: 10.1039/c3md00323j
Lee, Z. W., Zhou, J., Chen, C. S., Zhao, Y., Tan, C. H., Li, L., et al. (2011). The slow-releasing hydrogen sulfide donor, GYY4137, exhibits novel anti-cancer effects in vitro and in vivo. PloS One. 6:e21077. doi: 10.1371/journal.pone.0021077
Leskova, W., Pickett, H., Eshaq, R. S., Shrestha, B., Pattillo, C. B., and Harris, N. R. (2019). Effect of diabetes and hyaluronidase on the retinal endothelial glycocalyx in mice. Exp. Eye Res. 179, 125–131. doi: 10.1016/j.exer.2018.11.012
Li, L., Bhatia, M., Zhu, Y. Z., Zhu, Y. C., Ramnath, R. D., Wang, Z. J., et al. (2005). Hydrogen sulfide is a novel mediator of lipopolysaccharide-induced inflammation in the mouse. FASEB J. 19, 1196–1198. doi: 10.1096/fj.04-3583fje
Li, L., Fox, B., Keeble, J., Salto-Tellez, M., Winyard, P. G., Wood, M. E., et al. (2013). The complex effects of the slow-releasing hydrogen sulfide donor GYY4137 in a model of acute joint inflammation and in human cartilage cells. J. Cell Mol. Med. 17, 365–376. doi: 10.1111/jcmm.12016
Li, L., Whiteman, M., Guan, Y. Y., Neo, K. L., Cheng, Y., Lee, S. W., et al. (2008). Characterization of a novel, water-soluble hydrogen sulfide-releasing molecule (GYY4137): new insights into the biology of hydrogen sulfide. Circulation 117, 2351–2360. doi: 10.1161/circulationaha.107.753467
Lipowsky, H. H. (2011). Protease activity and the role of the endothelial glycocalyx in inflammation. Drug Discov. Today Dis. Models 8, 57–62. doi: 10.1016/j.ddmod.2011.05.004
Lohakul, J., Jeayeng, S., Chaiprasongsuk, A., Torregrossa, R., Wood, M., Saelim, M., et al. (2021). Mitochondria-targeted hydrogen sulfide delivery molecules protect against UVA-induced photoimaging in dermal fibroblasts, and in mouse skin in vivo. Antioxid. Redox Signal. [Epub ahead of print].
Manon-Jensen, T., Multhaupt, H. A., and Couchman, J. R. (2013). Mapping of matrix metalloproteinase cleavage sites on syndecan-1 and syndecan-4 ectodomains. FEBS J. 280, 2320–2331. doi: 10.1111/febs.12174
Maxhimer, J. B., Somenek, M., Rao, G., Pesce, C. E., Baldwin, D. Jr., Gattuso, P., et al. (2005). Heparanase-1 gene expression and regulation by high glucose in renal epithelial cells: a potential role in the pathogenesis of proteinuria in diabetic patients. Diabetes 54, 2172–2178. doi: 10.2337/diabetes.54.7.2172
Mitidieri, E., Gurgone, D., Caiazzo, E., Tramontano, T., Cicala, C., Sorrentino, R., et al. (2020). L-cysteine/cystathionine-β-synthase-induced relaxation in mouse aorta involves a L-serine/sphingosine-1-phosphate/NO pathway. Br. J. Pharmacol. 177, 734–744. doi: 10.1111/bph.14654
Módis, K., Asimakopoulou, A., Coletta, C., Papapetropoulos, A., and Szabo, C. (2013). Oxidative stress suppresses the cellular bioenergetic effect of the 3-mercaptopyruvate sulfurtransferase/hydrogen sulfide pathway. Biochem. Biophys. Res. Commun. 433, 401–407. doi: 10.1016/j.bbrc.2013.02.131
Módis, K., Bos, E. M., Calzia, E., van Goor, H., Coletta, C., Papapetropoulos, A., et al. (2014). Regulation of mitochondrial bioenergetic function by hydrogen sulfide. Part II. Pathophysiological and therapeutic aspects. Br. J. Pharmacol. 171, 2123–2146. doi: 10.1111/bph.12368
Montoya, L. A., and Pluth, M. D. (2016). Organelle-targeted H2S probes enable visualization of the subcellular distribution of H2S donors. Anal. Chem. 88, 5769–5774. doi: 10.1021/acs.analchem.6b00087
Ng, L. T., Gruber, J., and Moore, P. K. (2018). Is there a role of H2S in mediating health span benefits of caloric restriction? Biochem. Pharmacol. 149, 91–100. doi: 10.1016/j.bcp.2018.01.030
Nieuwdorp, M., Mooij, H. L., Kroon, J., Atasever, B., Spaan, J. A., Ince, C., et al. (2006a). Endothelial glycocalyx damage coincides with microalbuminuria in type 1 diabetes. Diabetes 55, 1127–1132. doi: 10.2337/diabetes.55.04.06.db05-1619
Nieuwdorp, M., van Haeften, T. W., Gouverneur, M. C., Mooij, H. L., van Lieshout, M. H., Levi, M., et al. (2006b). Loss of endothelial glycocalyx during acute hyperglycemia coincides with endothelial dysfunction and coagulation activation in vivo. Diabetes 55, 480–486. doi: 10.2337/diabetes.55.02.06.db05-1103
Niu, T., Zhao, M., Jiang, Y., Xing, X., Shi, X., Cheng, L., et al. (2019). Endomucin restores depleted endothelial glycocalyx in the retinas of streptozotocin-induced diabetic rats. FASEB J. 33, 13346–13357. doi: 10.1096/fj.201901161r
Onions, K. L., Gamez, M., Buckner, N. R., Baker, S. L., Betteridge, K. B., Desideri, S., et al. (2019). VEGFC reduces glomerular albumin permeability and protects against alterations in VEGF receptor expression in diabetic nephropathy. Diabetes 68, 172–187. doi: 10.2337/db18-0045
Peake, B. F., Nicholson, C. K., Lambert, J. P., Hood, R. L., Amin, H., Amin, S., et al. (2013). Hydrogen sulfide preconditions the db/db diabetic mouse heart against ischemia-reperfusion injury by activating Nrf2 signaling in an Erk-dependent manner. Am. J. Physiol. Heart Circ. Physiol. 304, H1215–H1224.
Qiu, Y., Wu, Y., Meng, M., Luo, M., Zhao, H., Sun, H., et al. (2018). GYY4137 protects against myocardial ischemia/reperfusion injury via activation of the PHLPP-1/Akt/Nrf2 signaling pathway in diabetic mice. J. Surg. Res. 225, 29–39. doi: 10.1016/j.jss.2017.12.030
Ratney, R. S., Wegman, D. H., and Elkins, H. B. (1974). In vivo conversion of methylene chloride to carbon monoxide. Arch. Environ. Health 28, 223–226. doi: 10.1080/00039896.1974.10666472
Saker, S., Stewart, E. A., Browning, A. C., Allen, C. L., and Amoaku, W. M. (2014). The effect of hyperglycaemia on permeability and the expression of junctional complex molecules in human retinal and choroidal endothelial cells. Exp. Eye Res. 121, 161–167. doi: 10.1016/j.exer.2014.02.016
Sander, B., Thornit, D. N., Colmorn, L., Strøm, C., Girach, A., Hubbard, L. D., et al. (2007). Progression of diabetic macular edema: correlation with blood retinal barrier permeability, retinal thickness, and retinal vessel diameter. Invest. Ophthalmol. Vis. Sci. 48, 3983–3987. doi: 10.1167/iovs.06-1102
Si, Y. F., Wang, J., Guan, J., Zhou, L., Sheng, Y., and Zhao, J. (2013). Treatment with hydrogen sulfide alleviates streptozotocin-induced diabetic retinopathy in rats. Br. J. Pharmacol. 169, 619–631. doi: 10.1111/bph.12163
Singh, A., Satchell, S. C., Neal, C. R., McKenzie, E. A., Tooke, J. E., and Mathieson, P. W. (2007). Glomerular endothelial glycocalyx constitutes a barrier to protein permeability. J. Am. Soc. Nephrol. 18, 2885–2893. doi: 10.1681/asn.2007010119
Sohn, O. S., Fiala, E. S., Conaway, C. C., and Weisburger, J. H. (1982). Metabolism and disposition of morphine in the rat, hamster, and guinea pig. Toxicol. Appl. Pharmacol. 64, 486–491. doi: 10.1016/0041-008x(82)90246-0
Strickland, J., Cummings, A., Spinnato, J. A., and Liccione, J. J. (2003). Toxicological Review of Hydrogen Sulfide (CAS No. 7783-06-4). In Support of Summary Information on the Integrated Risk Information System (IRIS). Washington, DC: U.S. Environmental Protection Agency.
Sun, Y., Tian, Z., Liu, N., Zhang, L., Gao, Z., Sun, X., et al. (2018). Exogenous H2S switches cardiac energy substrate metabolism by regulating SIRT3 expression in db/db mice. J. Mol. Med. (Berl). 96, 281–299. doi: 10.1007/s00109-017-1616-3
Sundd, P., Pospieszalska, M. K., Cheung, L. S., Konstantopoulos, K., and Ley, K. (2011). Biomechanics of leukocyte rolling. Biorheology 48, 1–35. doi: 10.3233/bir-2011-0579
Suzuki, K., Olah, G., Modis, K., Coletta, C., Kulp, G., Gerö, D., et al. (2011). Hydrogen sulfide replacement therapy protects the vascular endothelium in hyperglycemia by preserving mitochondrial function. Proc. Natl. Acad. Sci. U.S.A. 108, 13829–13834. doi: 10.1073/pnas.1105121108
Suzuki, K., Sagara, M., Aoki, C., Tanaka, S., and Aso, Y. (2017). Clinical implication of plasma hydrogen sulfide levels in Japanese patients with Type 2 diabetes. Intern. Med. 56, 17–21. doi: 10.2169/internalmedicine.56.7403
Szabo, C. (2012). Roles of hydrogen sulfide in the pathogenesis of diabetes mellitus and its complications. Antioxid. Redox Signal. 17, 68–80. doi: 10.1089/ars.2011.4451
Szabo, C. (2017). Hydrogen sulfide, an enhancer of vascular nitric oxide signaling: mechanisms and implications. Am. J. Physiol. Cell Physiol. 312, C3–C15.
Szabo, C., Ransy, C., Módis, K., Andriamihaja, M., Murghes, B., Coletta, C., et al. (2014). Regulation of mitochondrial bioenergetic function by hydrogen sulfide. Part I. Biochemical and physiological mechanisms. Br. J. Pharmacol. 171, 2099–2122. doi: 10.1111/bph.12369
Szczesny, B., Módis, K., Yanagi, K., Coletta, C., Le Trionnaire, S., Perry, A., et al. (2014). AP39, a novel mitochondria-targeted hydrogen sulfide donor, stimulates cellular bioenergetics, exerts cytoprotective effects and protects against the loss of mitochondrial DNA integrity in oxidatively stressed endothelial cells in vitro. Nitric Oxide 41, 120–130.
Takano, T., and Miyazaki, Y. (1988). Metabolism of dichloromethane and the subsequent binding of its product, carbon monoxide, to cytochrome P-450 in perfused rat liver. Toxicol. Lett. 40, 93–96. doi: 10.1016/0378-4274(88)90187-7
Tao, B. B., Liu, S. Y., Zhang, C. C., Fu, W., Cai, W. J., Wang, Y., et al. (2013). VEGFR2 functions as an H2S-targeting receptor protein kinase with its novel Cys1045-Cys1024 disulfide bond serving as a specific molecular switch for hydrogen sulfide actions in vascular endothelial cells. Antioxid. Redox Signal. 19, 448–464. doi: 10.1089/ars.2012.4565
Toxicology–cosmetic ingredient review (1989). 6 Final report on the safety assessment of morpholine. J. Am. Coll. Toxicol. 8:707. doi: 10.3109/10915818909010528
Wang, R. (2002). Two’s company, three’s a crowd: can H2S be the third endogenous gaseous transmitter? FASEB J. 16, 1792–1798. doi: 10.1096/fj.02-0211hyp
Wang, R. (2012). Physiological implications of hydrogen sulfide: a whiff exploration that blossomed. Physiol. Rev. 92, 791–896. doi: 10.1152/physrev.00017.2011
Wei, W. B., Hu, X., Zhuang, X. D., Liao, L. Z., and Li, W. D. (2014). GYY4137, a novel hydrogen sulfide-releasing molecule, likely protects against high glucose-induced cytotoxicity by activation of the AMPK/mTOR signal pathway in H9c2 cells. Mol. Cell Biochem. 389, 249–256. doi: 10.1007/s11010-013-1946-6
Whiteman, M., Gooding, K. M., Whatmore, J. L., Ball, C. I., Mawson, D., Skinner, K., et al. (2010a). Adiposity is a major determinant of plasma levels of the novel vasodilator hydrogen sulphide. Diabetologia 53, 1722–1726. doi: 10.1007/s00125-010-1761-5
Whiteman, M., Le Trionnaire, S., Chopra, M., Fox, B., and Whatmore, J. (2011). Emerging role of hydrogen sulfide in health and disease: critical appraisal of biomarkers and pharmacological tools. Clin. Sci. (Lond). 121, 459–488. doi: 10.1042/cs20110267
Whiteman, M., Li, L., Rose, P., Tan, C. H., Parkinson, D. B., and Moore, P. K. (2010b). The effect of hydrogen sulfide donors on lipopolysaccharide-induced formation of inflammatory mediators in macrophages. Antioxid. Redox Signal. 12, 1147–1154. doi: 10.1089/ars.2009.2899
Whiteman, M., and Winyard, P. G. (2011). Hydrogen sulfide and inflammation: the good, the bad, the ugly and the promising. Expert. Rev. Clin. Pharmacol. 4, 13–32. doi: 10.1586/ecp.10.134
Yang, G., Wu, L., Jiang, B., Yang, W., Qi, J., Cao, K., et al. (2008). H2S as a physiologic vasorelaxant: hypertension in mice with deletion of cystathionine gamma-lyase. Science 322, 587–590. doi: 10.1126/science.1162667
Yusuf, M., Kwong Huat, B. T., Hsu, A., Whiteman, M., Bhatia, M., and Moore, P. K. (2005). Streptozotocin-induced diabetes in the rat is associated with enhanced tissue hydrogen sulfide biosynthesis. Biochem. Biophys. Res. Commun. 333, 1146–1152. doi: 10.1016/j.bbrc.2005.06.021
Zhao, H., Lu, S., Chai, J., Zhang, Y., Ma, X., Chen, J., et al. (2017). Hydrogen sulfide improves diabetic wound healing in ob/ob mice via attenuating inflammation. J. Diabetes Complications 31, 1363–1369. doi: 10.1016/j.jdiacomp.2017.06.011
Zhou, X., Feng, Y., Zhan, Z., and Chen, J. (2014). Hydrogen sulfide alleviates diabetic nephropathy in a streptozotocin-induced diabetic rat model. J. Biol. Chem. 289, 28827–28834. doi: 10.1074/jbc.m114.596593
Keywords: glycocalyx, retinal permeability, diabetes, hydrogen sulfide, inflammation, mitochondria, slow-release hydrogen sulfide donors
Citation: Allen CL, Wolanska K, Malhi NK, Benest AV, Wood ME, Amoaku W, Torregrossa R, Whiteman M, Bates DO and Whatmore JL (2021) Hydrogen Sulfide Is a Novel Protector of the Retinal Glycocalyx and Endothelial Permeability Barrier. Front. Cell Dev. Biol. 9:724905. doi: 10.3389/fcell.2021.724905
Received: 14 June 2021; Accepted: 29 July 2021;
Published: 07 September 2021.
Edited by:
Ye Zeng, Sichuan University, ChinaReviewed by:
Rocío Salceda, National Autonomous University of Mexico, MexicoHongyan Kang, Beihang University, China
Copyright © 2021 Allen, Wolanska, Malhi, Benest, Wood, Amoaku, Torregrossa, Whiteman, Bates and Whatmore. This is an open-access article distributed under the terms of the Creative Commons Attribution License (CC BY). The use, distribution or reproduction in other forums is permitted, provided the original author(s) and the copyright owner(s) are credited and that the original publication in this journal is cited, in accordance with accepted academic practice. No use, distribution or reproduction is permitted which does not comply with these terms.
*Correspondence: David O. Bates, RGF2aWQuQmF0ZXNAbm90dGluZ2hhbS5hYy51aw==; Jacqueline L. Whatmore, Si5MLldoYXRtb3JlQGV4ZXRlci5hYy51aw==