- 1Biomedical Sciences, University of Missouri, Columbia, MO, United States
- 2MU Institute for Data Science and Informatics, University of Missouri, Columbia, MO, United States
- 3Thompson Center for Autism and Neurobehavioral Disorders, University of Missouri, Columbia, MO, United States
- 4Genetics Area Program, University of Missouri, Columbia, MO, United States
The conceptus is most vulnerable to developmental perturbation during its early stages when the events that create functional organ systems are being launched. As the placenta is in direct contact with maternal tissues, it readily encounters any xenobiotics in her bloodstream. Besides serving as a conduit for solutes and waste, the placenta possesses a tightly regulated endocrine system that is, of itself, vulnerable to pharmaceutical agents, endocrine disrupting chemicals (EDCs), and other environmental toxicants. To determine whether extrinsic factors affect placental function, transcriptomics and other omics approaches have become more widely used. In casting a wide net with such approaches, they have provided mechanistic insights into placental physiological and pathological responses and how placental responses may impact the fetus, especially the developing brain through the placenta-brain axis. This review will discuss how such omics technologies have been utilized to understand effects of EDCs, including the widely prevalent plasticizers bisphenol A (BPA), bisphenol S (BPS), and phthalates, other environmental toxicants, pharmaceutical agents, maternal smoking, and air pollution on placental gene expression, DNA methylation, and metabolomic profiles. It is also increasingly becoming clear that miRNA (miR) are important epigenetic regulators of placental function. Thus, the evidence to date that xenobiotics affect placental miR expression patterns will also be explored. Such omics approaches with mouse and human placenta will assuredly provide key biomarkers that may be used as barometers of exposure and can be targeted by early mitigation approaches to prevent later diseases, in particular neurobehavioral disorders, originating due to placental dysfunction.
Introduction
The placenta and uterine tissue directly interact, and thus, factors circulating in the maternal bloodstream easily transfer across the placenta, where they can affect this organ and secondarily the fetus. This close relationship between the placenta and maternal tissue is essential for nutrient, gas, and waste exchange. The placenta is also an endocrine organ that produces a range of hormones and cytokine factors that exert local paracrine effects in the placenta but can also act upon maternal and fetal tissues. Many biomedical studies examine effects on mouse or rat placenta as rodents have an invasive hemochorial type of placentation with syncytiotrophoblast (syncytioTB) cells that are involved in nutrient and gas exchange and are bathed in maternal blood, analogous to structural components of the human placenta (Rosenfeld, 2015a). However, the fetal placental cells, trophoblasts (TB), may also be immersed in compounds percolating through the maternal blood. For this reason, TB cells have some ability to detoxify select xenobiotic chemicals, which may help buffer the fetus against such chemical assaults (Myllynen et al., 2005; Obolenskaya et al., 2010; Corbel et al., 2015; Nahar et al., 2015). However, being an endocrine organ in of itself, the placenta is vulnerable to a myriad of exogenous chemicals. The ability to respond to such environmental challenges is also likely sexually dimorphic in nature (Mao et al., 2010; Rosenfeld, 2015a).
Individual gene or protein expression patterns were used to ascertain the effects of such chemical exposures on the placenta. Microarray technology was the first method employed to relate such chemicals exposures and transcriptomic changes in the placenta (e.g., Imanishi et al., 2003; Avissar-Whiting et al., 2010; Bruchova et al., 2010; Votavova et al., 2011; Tait et al., 2015; Grindler et al., 2018). However, such studies were confined to genes included on the arrays, and microarrays were only developed for a few select species whose genome was sequenced and annotated. RNA sequencing (RNAseq) is a high throughput approach that has greatly expanded our knowledge of how EDC, other environmental toxicants and pharmaceutical agents affect global gene expression patterns in the placenta (e.g., Green et al., 2020; Mao et al., 2020). Herein, we will consider the studies to date that have shown such extrinsic factors can affect transcriptomic profiles or protein expression in the placenta as determined by microarray analyses, RNAseq, or candidate gene/protein approaches. Further, we will consider other omics approaches, including metabolomics, proteomics, and methylomics, that have been used to characterize the effects of xenobiotics on the human and rodent placenta. The importance of miRNAs (miRs) is gaining currency as such small RNAs have the ability to block translation by binding to target mRNA (Van Wynsberghe et al., 2011; Moreno-Moya et al., 2014). The miR/mRNA complexes can then be degraded prior to the mRNA entering the cytoplasm to be translated into a protein. In this way, miR represent the final epigenetic regulators. Studies have thus examined how some of the above factors can regulate miR and other non-coding RNA profiles by using small RNAseq. Lastly, we will consider some of the recently developed transcriptomic technology that will continue to advance our understanding in placental toxicology and allow for pinpointing transcriptomic changes in individual TB cell populations.
Endocrine Disrupting Chemicals
Endocrine disrupting chemicals (EDC) are synthetic and natural compounds that can mimic or antagonize endogenous hormone responses. BPA is a widely prevalent synthetic chemical that can act through steroid and non-steroid receptor pathways (Schug et al., 2011). Current production estimates for BPA are around 20 billion pounds (Grand View Research, 2014). Approximately 93% of the U.S. population unknowingly has measurable amounts of BPA in their urine (Calafat et al., 2008). Exposure to BPA and, its analog, bisphenol S (BPS) is primarily dietary (Galloway et al., 2010; Sieli et al., 2011), but other routes of exposure are known (Xue et al., 2016; Hines et al., 2018). BPA readily can be transmitted from the maternal tissue to the fetal placenta (Vandenberg et al., 2007; vom Saal et al., 2007). BPA substitutes, such as BPS, are increasingly being used in a range of consumer products labeled BPA-free. Yet, BPS may lead to similar and potentially even more pronounced effects compared to BPA (Rosenfeld, 2017; Wu et al., 2018).
BPA has been shown to affect placental gene expression patterns (Imanishi et al., 2003; Kang et al., 2011; Susiarjo et al., 2013; Tan et al., 2013; Tait et al., 2015; Xu et al., 2015; Lee et al., 2016; Lan et al., 2017). Most of these reports though only used a candidate gene expression approach (Kang et al., 2011; Susiarjo et al., 2013; Tan et al., 2013; Tait et al., 2015; Xu et al., 2015; Lee et al., 2016; Lan et al., 2017), in particular for those known to be imprinted (Kang et al., 2011; Susiarjo et al., 2013). More recent studies employed microarrays to examine thousands of genes in a single experiment (Imanishi et al., 2003; Tait et al., 2015), but such studies may have been under-powered. One study that used microarrays to examine the effects of BPA on the placenta found that the high dosage of BPA tested resulted in significant degeneration and necrosis of giant cells, vacuolization in the junctional zone, and overall reduction of the spongioTB layer (Tait et al., 2015). Nuclear accumulation of β-catenin was evident in TB within the labyrinthine and spongioTB layers, suggestive of Wnt/β-catenin pathway activation (Tait et al., 2015). The microarray studies revealed that the low dosage of BPA tested promoted blood vessel development and arborization, whereas the high dose inhibited such angiogenic changes.
We used RNAseq analyses to examine the global transcriptomic profile in embryonic age (E) 12.5 mouse placenta following dietary exposure to BPA or BPS. BPA and BPS altered the expression of an identical set of 13 genes (Mao et al., 2020). Of which, 11 were downregulated and two (Actn2 and Efcab2) modestly upregulated. Four of the differentially expressed (DE) transcripts are typically enriched in the placenta (Sfrp4, Coch, Gm9513, and Calm4) as determined by the TissueEnrich program (Mao et al., 2020). Based on the DE gene-sets, WNT and chemokine signaling pathways, amino acid metabolism, and possibly neurotransmission are pathways predicted to be affected in the placental samples exposed to BPA/BPS.
In the same study, we examined for histopathological changes in the placenta following BPA and BPS exposure. Additionally, targeted and non-targeted metabolomics analyses were performed to determine the extent to which these EDCs affect other omics profiles and whether transcriptomics and metabolomic changes correlated with BPA/BPS-associated architectural modifications in the placenta (Mao et al., 2020). Both exposures reduced the area occupied by spongioTB relative to parietal trophoblast giant cells (pTGC) within the junctional zone. Both BPA and BPS markedly reduced placental serotonin (5-HT) concentrations and lowered 5-HT pTGC immunoreactivity. Concentrations of dopamine and 5-hydroxyindoleacetic acid), the main metabolite of 5-HT, however, were increased. Dopamine-immunoreactivity in pTGC was increased in BPA and BPS exposed placentas. By using mixOmics analyses (Rohart et al., 2017), we found a strong positive correlation between 5-HT positive pTGC cells and reductions in spongioTB to pTGC area, indicative that 5-HT is essential for maintaining cells within the junctional zone. In contrast, an inverse correlation existed between dopamine positive pTGC cells and reductions spongioTB to pTGC area. The collective findings suggest that BPS exposure causes almost identical placental effects as BPA. A major target of BPA/BPS is either spongioTB or pTGC within the junctional zone. BPA/BPS induced disruptions in placental 5-HT and dopamine may affect fetal brain development through the placenta- brain axis. It is clear that 5-HT as a morphogen may be one of the primary conductors regulating early neural crest formation, metamorphosis, neurogenesis, cell motility, synaptogenesis, and development of the nociceptive system (Lauder, 1988; Lauder et al., 1988; Whitaker-Azmitia, 1991; Herlenius and Lagercrantz, 2001). Strong evidence exists that the initial source of 5-HT to orchestrate such neural changes is the placenta (Huang et al., 1998; Bonnin and Levitt, 2011; Hadden et al., 2017). By using such omics approaches in the placenta, it may also thus shed light on how EDC compromise early neural development and thereby increase the risk for neurobehavioral disorders.
BPA might also affect DNA methylation patterns in the placenta. One study examined BPA concentrations, gene expression patterns of BPA-specific metabolizing enzymes, and global DNA methylations in the placenta of 2nd trimester human fetuses (Nahar et al., 2015). Average LINE1 and CCGG global methylation in the placenta were 58.3 and 59.2%, respectively, Total BPA concentrations positively correlated with global methylation for the placenta based on the LINE1 assay. BPA-specific metabolizing enzymes, such as GUSB, UGT2B15, STS, and SULT1A1 were identified in these placenta samples. The findings suggest that maternal exposure to BPA might promote hypermethylation of select genes in the placenta.
Phthalates are another class of EDCs found in commonly used household items, including children’s toys, plastic containers, and plastic wraps (Ferguson et al., 2011; Lioy et al., 2015; Schulz et al., 2015; Jo et al., 2018). They are associated with adverse pregnancy outcomes, including fetal loss and placental growth abnormalities (Zong et al., 2015; Gao et al., 2017; Mahaboob Basha and Radha, 2017), but the full range of mechanisms by which they induce such effects remains elusive. Examination of the DNA methylome (Illumina Infinium Human Methylation 850k BeadChip) and transcriptome (Agilent whole human genome array) in first-trimester human placenta revealed 39 genes that demonstrated altered methylation and gene expression patterns in women exposed to high amounts of phthalates with most showing reduced expression in this group (Grindler et al., 2018). The combined usage of methylomics and transcriptomics revealed epidermal growth factor receptor (EGFR) as a likely primary mediator of phthalates on placental function.
Another cohort study revealed that chorionic gonadotropin A (CGA) showed sex-dependent gene expression changes in the placenta that were linked to various phthalate concentrations detected in the urine of pregnant women (Adibi et al., 2017a). CY19A1, CYP11A1, CGA expression in the placenta correlated with maternal urinary concentrations of monobenzyl phthalate (MBzP), MnBP, mono-iso-butyl phthalate (MiBP), and conceptus sex (Adibi et al., 2017a).
In vitro culture approaches have aided our understanding of how EDCs affect placental cells. One study used TB stem cells from rhesus monkeys (Macaca mulatta) to screen global transcriptome changes induced by several EDCs, atrazine, tributyltin, bisphenol A, bis(2-ethylhexyl) phthalate, and perfluorooctanoic acid (PFOA) (Midic et al., 2018). Atrazine and tributylin, and to a lesser extent the other three EDCs, suppressed genes involved in cytokine signaling related to antiviral response, along with those involved in metabolism, DNA repair, and cell migration.
Another study isolated human TB progenitor cells at 7–14 weeks. of pregnancy from two female and three male concepti, as well as villous cytotTB cells (vCTBs) at 15–20 weeks. pregnancy from three female and four male concepti. Primary cell lines were cultured in the presence of one or more phthalates: mono-n-butyl (MnBP), monobenzyl (MBzP), mono-2-ethylhexyl (MEHP), and monoethyl (MEP) (Adibi et al., 2017b). Treatment of both TB lines with MnBP, MBzP and MEHP at concentrations that resemble those found in the urine of pregnant women altered CGB and PPARG expression in these primary placental cells, although the effects varied according to the sex from which the placental cells were derived (Adibi et al., 2017b).
Other Environmental Toxicants
Other environmental toxicants, including heavy metals, such as arsenic, and flame retardants can reach the placenta, whereupon they may induce transcriptomic changes. The earth’s crust contains arsenic, and it can also be found in the water, land, and air. However, it is highly toxic in the inorganic form (WHO, 2018). Common routes of exposure to inorganic arsenic are through drinking contaminated groundwater, using such water in food preparation and irrigation of food crops, manufacturing of it, consumption of contaminated food, and smoking tobacco (WHO, 2018). As with the EDCs, the placenta can accumulate high concentrations of arsenic that can lead to placental alterations, including in the glucocorticoid receptor pathway, oxidative stress, inflammation, linkages to pre-eclampsia, and epigenetic changes (Ahmed et al., 2011; Caldwell et al., 2015; Cardenas et al., 2015; Li et al., 2015; Green et al., 2016; Konkel, 2016; Appleton et al., 2017; Rahman et al., 2018; Punshon et al., 2019; Winterbottom et al., 2019b; Meakin et al., 2020; Stone et al., 2021).
To examine whether exposure to arsenic results in global transcriptomic changes in the placenta, 46 pregnant women were selected from the New Hampshire Birth Cohort Study (NHBCS), which is a US cohort known to have low-to-moderate arsenic levels in drinking water because of unregulated private wells (Winterbottom et al., 2019a). Potential sex-dependent gene expression changes in the placenta were correlated with prenatal exposure to arsenic, as determined by concentrations in the urine of pregnant mothers. While no genes were differentially expressed in female placenta based on arsenic exposure, several hundred genes were affected in the placenta of males. Two of the genes that showed the greatest downregulation in male placenta exposed to arsenic were FIBIN and RANBP3L (Ahmed et al., 2011; Caldwell et al., 2015; Cardenas et al., 2015; Li et al., 2015; Green et al., 2016; Konkel, 2016; Appleton et al., 2017; Rahman et al., 2018; Punshon et al., 2019; Winterbottom et al., 2019b; Meakin et al., 2020; Stone et al., 2021).
To understand how such gene expression patterns might originate in the placenta, a handful of studies have examined the expression of epigenetic regulator genes and DNA methylation profiles in the placenta of arsenic-exposed human cohorts. One study analyzed the expression of over a hundred epigenetic regulator genes, such as those that act as readers, writers and erasers of post-translational histone modifications, and chromatin remodelers (Winterbottom et al., 2019b). Several of these genes demonstrated differences based on the interaction between placental sex and arsenic exposure with the histone methyltransferase (PRDM6) negatively correlating with arsenic exposure. Placental glucocorticoid receptor (NR3C1) methylation positively associated with arsenic exposure (Appleton et al., 2017).
Global DNA methylation patterns based on CpG loci were examined in placental samples obtained from 343 individuals enrolled in the New Hampshire Birth Cohort Study and correlated based on arsenic levels in the urine and toenails samples of these pregnant mothers (Green et al., 2016). While no linkages were found based on arsenic in maternal urine, strong association were identified based on levels of this heavy metal in the toenail samples. Of the 163 differentially methylated loci, the primary one was for LYRM2 (Green et al., 2016). This study also found that allocation of placental cell sub-populations changed based on arsenic exposure.
Another study linked arsenic exposure and DNA methylation patterns, as determined by Infinium HumanMethylation450 BeadChip array, in the placenta, in the umbilical artery, and human umbilical vein endothelial cells (HUVEC) (Cardenas et al., 2015). Genes regulating melanogenesis and insulin signaling pathways were differentially methylated in the placenta and umbilical artery based on arsenic exposure (Cardenas et al., 2015).
The flame-retardant mixture, Firemaster 550 (FM 550) contains organophosphate flame retardants that was hypothesized to disrupt placental function. To examine how this environmental toxicant affected the placenta, pregnant Wistar rats were treated with varying concentrations of this chemical (Rock et al., 2020). This treatment altered the expression of genes involved in transport and synthesis of 5-HT in the placenta (Rock et al., 2020). Additionally, metabolites of 5-HT and the kynurenine metabolic pathway were increased.
The cellular and transcriptomic effects of another flame retardant, BDE-47- a polybrominated diphenyl ethers (PBDEs), was tested in human placental cytotTB cells (CTBs) (Robinson et al., 2019). This compound suppressed migration and invasion by CTBs. BDE-47 induced transcriptome changes that were dose dependent with genes involved in stress, inflammation, lipid/cholesterol metabolism, differentiation, migration, and vascular morphogenesis affected (Robinson et al., 2019). Hypermethylation of CpG islands for genes involved in cell adhesion and migration occurred in response to this treatment.
Pharmaceutical Agents
Pregnant women are often prescribed pharmaceutical agents to regulate such conditions as depression, epilepsy, and pain. While such drugs may be beneficial to the mother, they can have untoward consequences on the conceptus, including the placenta. Transcriptomics and other gene expression approaches have been useful tools in understanding how such xenobiotics alter the genetic machinery in this organ. In this section, we will consider three such pharmaceutical agents, serotonin-reuptake inhibitors (SSRI) used to treat depression, valproic acid (VPA) used to treat seizures, and oxycodone (OXY) that is a commonly prescribed analgesic agent.
Approximately 8–10% of pregnant women are prescribed selective serotonin-reuptake inhibitors (SSRI) to combat depression (Mitchell et al., 2011; Huybrechts et al., 2013). Such drugs act by binding to SLC6A4/SERT within the intracellular membrane, which in the central nervous system prevents the presynaptic cells from accruing 5-HT. Inhibition of SLC6A4/SERT results in increased concentrations of 5-HT in the synaptic space that can continue to bind and activates its cognate receptors. Such drugs though can also inhibit SLC6A4/SERT within placental TB, namely the pTGC that use this transporter to uptake maternal 5-HT. In vitro studies reveal SSRIs disrupt various structural and hormonal properties of placental cell lines (Hudon Thibeault et al., 2017; Clabault et al., 2018a,b). In rats, in utero exposure to venlafaxine reduced fetal placental weight (Laurent et al., 2016). Two SSRI, fluoxetine and sertraline, reduced cell proliferation of extravillous TB (JEG-3) cells (Clabault et al., 2018a). Norfluoxetine, a metabolite of fluoxetine, increased MMP-9 activity by these TB cells but suppressed MMP-9 activity in another cell line, HIPEC, derived from extravillous TB. TIMP-1 and ADAM-10 showed increased expression in JEG-3 cells treated with sertraline. Venlafaxine, another SSRI, increased ADAM-10 in HIPEC cells (Clabault et al., 2018a). Sertraline and venlafaxine induced fusion of cultured primary villous TB cells (Clabault et al., 2018b). Both compounds affect human chorionic gonadotropin beta (β-HCG) secretion by BeWo TB-derived cells. Norfluoxetine stimulated increased gene expression of chorionic gonadotropin beta (CGB) and gap junction protein alpha 1 (GJA1) which are considered biomarkers of syncytialization for these TB cells. Pregnant mothers consuming SSRIs have been reported to deliver lower birthweight infants and exhibit higher rates of placental-fetal vascular malperfusion than controls (Levy et al., 2020).
Valproic acid (VPA) is a short-chain-fatty acid commonly used as an antiepileptic drug and mood stabilizer (Chateauvieux et al., 2010). Such beneficial effects are ascribed to its inhibition of gamma amino butyric acid (GABA), transaminobutyrate, and ion channels (Chateauvieux et al., 2010). More recently, it has been shown that VPA can act as an histone deacetylase (HDAC) inhibitor that increases transcription by preventing deacetylation of histone proteins (Monti et al., 2009). Thus, some of the actions of VPA may be due to its epigenetic properties. Epilepsy is the most common neurological disorder in pregnant women, necessitating continued usage of antiepileptic drugs (AED) to prevent seizures. VPA is one of the primary AED prescribed to pregnant women, even though current data suggests that it may be associated with adverse fetal outcomes and behavioral deficits in children exposed in utero to this drug (Eadie, 2014; Elkjaer et al., 2018; Richards et al., 2019; Vajda et al., 2019; Daugaard et al., 2020). The placenta is also not immune to the effects of this AED (Khera, 1992; Meir et al., 2016; Tetro et al., 2019; Jinno et al., 2020; Shafique and Winn, 2021), and VPA-induced changes in the placenta may adversely affect fetal development, including the brain.
To examine the transcriptome changes in response to VPA, term placenta from women who delivered via cesarean were perfused with varying concentrations of VPA or vehicle. They were than analyzed with a customized gene array panel to examine the expression of carrier genes (Rubinchik-Stern et al., 2018). This drug treatment changed the mRNA expression patterns for transporters of folic acid, glucose, choline, thyroid hormone, and serotonin. Placental folate concentrations were also decreased with VPA treatment. VPA treatment to pregnant rats altered the expression of other transporter genes with Abcc4 and Slc22a4 reduced in late gestation, but Abcc5 was increased by VPA during mid-gestation (Jinno et al., 2020). Whether such changes on transporter gene expression patterns in the human and rat placenta is due to HDAC inhibition or other biological effects of VPA remains uncertain.
Opioid drugs, especially oxycodone (OxyContin, OXY), are widely prescribed analgesic agents to control pain in pregnant women. This abuse is one of the leading non-infectious disease public health concerns and economic challenges facing the United States (Reinhart et al., 2018). Opioid use disorder (OUD), is a particular health concern in women of child-bearing age (SAMHSA, 2015) with OUD during pregnancy estimated to affect 5.6 per 1000 live birth infants (Patrick et al., 2012). Neonates exposed during gestation to opioids are at risk for neonatal abstinence syndrome (NAS) (Jones et al., 2019). Maternal OUD has been associated with poor fetal growth, increased risk for premature births, low birthweight offspring, and congenital defects (Yazdy et al., 2015; CDC, 2019). Adult-onset diseases due to developmental origin of health and disease (DOHaD) effects of these drugs are also possible (Grandjean et al., 2015; Rosenfeld, 2015b). The placenta may be bathed and affected by any opioids circulating in the maternal blood, whereupon it can affect this organ and be transmitted to the fetus.
An endogenous opioid system is present in the placenta that mediates several placental responses, including production of maternal recognition of pregnancy factors, such as HCG and placental lactogens (Cemerikic et al., 1991; Ahmed et al., 1992; Cemerikic et al., 1992; Petit et al., 1993; Cemerikic et al., 1994). Exogenous opioids that transit from the maternal blood to the placenta can thus impact this system. Effects of OXY and other opioids have been examined in cultured TB cells and shown to affect production of steroid hormones, HCG, and other placental factors (Cemerikic et al., 1988; Zharikova et al., 2007; Neradugomma et al., 2017; Serra et al., 2017).
We tested whether maternal OXY exposure affects the morphology and transcriptome profile as determined by RNAseq in E 12.5 mice placenta (Green et al., 2020). Maternal OXY treatment reduced pTGC area and maternal blood vessel area within the labyrinth region. OXY exposure altered placental gene expression profiles in a sexually dimorphic manner with female placenta exhibiting up-regulation of several placental enriched genes, including Ceacam11, Ceacam14, Ceacam12, Ceacam13, Prl7b1, Prl2b1, Ctsq, and Tpbpa. Placenta of OXY exposed males had alterations of many ribosomal proteins. Weighted correlation network analysis revealed that in OXY females vs. CTL females, select modules correlated with placental histological changes induced by OXY. Such associations were lacking in the male OXY vs. CTL male comparison. Pathways that are likely affected in OXY females based on gene-sets in these modules include extracellular matrix reorganization, VEGF signaling, and regulation of actin bioskeleton, collagen biosynthesis, peptide hormone signaling, interferon signaling, interferon gamma signaling, and triglyceride metabolism and catabolism.
Smoking
Inorganic arsenic in cigarettes can affect placental architecture and function as discussed below. Other chemicals within cigarettes may also act though upon the placenta. The placenta expresses nicotinic acetylcholine receptor (nAChR) subunits that regulate TB cell invasion but whose expression and signaling pathway can be usurped by nicotine contained within tobacco smoke (Lips et al., 2005; Machaalani et al., 2014, 2018; Aishah et al., 2017; Chen et al., 2020). For instance, nicotine acting through nAChR induced endoplasmic reticulum stress in rat pTGC (Wong et al., 2016). Maternal smoking has been linked with changes in gross placental weight and microanatomical structure, especially for the extravillous TB cells (Heidari et al., 2018a,b; Larsen et al., 2018). Metabolism of lipids, namely long-chain polyunsaturated fatty acids and transporters for glucose uptake transporters (SLC2A1 and SLC2A3), amino acids (SLC7A8), and lipid gene expression patterns in the placenta correlate with maternal smoking (Walker et al., 2019; Weinheimer et al., 2020). Circulating levels of placental-associated proteins, pregnancy-associated plasma protein A (PAPP-A) and free (fβHCG) are reduced in the serum of pregnant mothers who smoke (Jauniaux et al., 2013).
Transcriptomic and DNA methylation studies have been undertaken to determine whether maternal smoking affects these parameters in the placenta. An Illumina Expression Beadchip v3 that contained 24,526 transcripts was used to survey placental samples and cord blood from women who smoked while pregnant vs. non-smokers (Votavova et al., 2011). Pathways that were likely affected in placental and cord blood samples included xenobiotic metabolism, oxidative stress, inflammation, immunity, hematopoiesis, and vascularization. An earlier array-based study by this same research group found that maternal smoking induced several genes involved in xenobiotic metabolism (CYP1A1, CYP1B1, CYB5A, and COX412) collagen-associated genes (COL6A3, COL1A1, and COL1A2), coagulation genes (F5 and F13A1), and thrombosis-related genes (CD36, ADAMTS9, and GAS6) (Bruchova et al., 2010). Another study that considered effects of maternal smoking on gene expression and the proteome in the placenta found that smoking down-regulated SERPINB2, FGA, and HBB but upregulated SERPINA1, EFHD1, and KRT8 (Huuskonen et al., 2016). Transcript expression for CYP1A1 and CYP4B1 were elevated, whereas HSD17B2, NFKB, and TGFB1 were suppressed by maternal smoking (Huuskonen et al., 2016).
A handful of studies characterized DNA methylation changes in the placenta based on maternal smoking (Suter et al., 2011; Chhabra et al., 2014; Tsaprouni et al., 2014; Maccani and Maccani, 2015; Fa et al., 2016; Morales et al., 2016; Cardenas et al., 2019; Rousseaux et al., 2020). Exposure to maternal smoking during the first trimester increased methylation of the AHRR gene but did not alter its gene expression pattern (Fa et al., 2018). In contrast, maternal smoking during this period did not alter DNA methylation of CYP1A1 but expression of this gene was upregulated (Fa et al., 2018). DNA methylation analyses with the Illumina HumanMethylation450 BeadChip for participants in the Infancia y Medio Ambiente (INMA) birth cohort revealed that maternal smoking decreased methylation levels of cg27402634 in the placenta, and this change was also associated with decreased birthweight (Morales et al., 2016). Another group that used this same BeadChip reported CpG sites mapping to GTF2H2C and GTF2H2D in the placental methylome strongly associated with maternal smoking (Chhabra et al., 2014). Usage of the Illumina HumanMethylation BeadChip technology in another cohort population showed that methylation patterns within the RUNX3 gene were linked to maternal smoking during pregnancy with one of the loci correlating with decreased gestational age (Maccani et al., 2013).
One study considered whether maternal cessation of smoking prior to pregnancy would prevent some of the harmful DNA methylation marks relative to women who continued to smoke throughout their pregnancy (Rousseaux et al., 2020). The placenta from both groups of women showed similar epigenetic changes, including demethylation of LINE-1 sequences, enrichment in epigenetic marks for enhancer regions (H3K4me1 and H3K27ac), and regions in proximity or overlapping imprinted genes (NNAT, SGCE, PEG10, H19.MIR675). The persistence of DNA methylation changes in those women who quit smoking before becoming pregnant is worrying as it is suggests that events even during the periconception period can lead to a permanent stamp on the DNA methylome of the placenta. Further work is clearly needed to determine the extent to when such changes become irreversible and whether these same methylation alterations are conferred to the placenta of subsequent generations, i.e., potential transgenerational effects. In this aspect, another study reported that while cigarette smoking by pregnant mothers reduced DNA methylation for several genes, including CPOX near GPR15, PRSS23, AVPR1B, PSEN2, LINC00299, RPS6KA2, and KIAA0087, cessation of smoking 3 months prior to pregnancy partially reversed such methylation alterations in the placenta (Tsaprouni et al., 2014).
Air Pollution
Particular matter that is around 2.5 μm in diameter (PM2.5) in air pollution easily crosses the maternal-placental interface. As such, the placenta is vulnerable to such environmental toxicants. We will consider the evidence to date that air pollution disrupts the placental transcriptome and methylome profiles. Culturing of JEG-3 human placental cells in the presence of such PM affected genes involved in immune response, apoptosis regulation, calcium signaling pathway, steroid hormone biosynthesis, and cytokine-cytokine receptor interaction (Kim et al., 2018). Protein levels for mitogen activated protein kinases (MAPK) and COX2 were reduced in the PM2.5 exposed JEG-3 cells. A Rhode Island Child Health Study (RICHS) revealed two developmentally sensitive windows to PM2.5, with 12 weeks prior to and 13 weeks into gestation also being associated with reduced infant birthweight (Deyssenroth et al., 2021). This same study analyzed effects of PM2.5 on placental gene expression patterns and relation to birthweight. Two placental modules enriched for genes involved in amino acid transport and cellular respiration correlated with maternal PM2.5 exposure and infant birthweight (Deyssenroth et al., 2021). Additional findings from this cohort revealed that maternal exposure to PM2.5 or black carbon (based on proximity to major roadways) changed the placental expression if several imprinted genes with CHD7 showing interactions between PM2.5 exposure/black carbon and infant sex being linked to placental expression of ZDBF2 (Kingsley et al., 2017). The ENVironmental Influences ON early AGEing birth cohort was used to examine placental DNA methylation patterns (via a bisulfite -PCR pyrosequencing approach) in response to exposure to PM2.5 or black carbon (Neven et al., 2018). Promoter methylation for APEX1, ERCC4, and p53 were positively linked with maternal PM2.5 exposure, whereas DAPK1 showed a negative association to this extrinsic factor (Neven et al., 2018). Maternal exposure to black carbon was associated with increased promoter methylation for APEX1 and ERCC4.
MicroRNAs and Long Non-Coding RNAs
MicroRNAs (miR) and long non-coding (lnc)RNAs were once considered junk, but this “rubbish” RNA is now known to exhibit critical regulatory roles, including acting as the goalie as to which mRNAs are allowed to exist out of the nucleus and be translated to a protein vs. those that are instead targeted for degradation (Clancy et al., 2007; Van Wynsberghe et al., 2011; Moreno-Moya et al., 2014). Thus, increasing number of researchers are studying the expression of small and long non-coding RNAs in the placenta following maternal exposure to various xenobiotics. The expression pattern of such RNAs in the placenta might also provide key insights into infant diseases. For instance, several miRs, such as mR-379-3p, miR-335-3p, miR-4532, miR-519e-3p, miR-3065-5p, and miR-105-5p, were found to be down-regulated in the placenta of infants born small for gestational age (Östling et al., 2019).
BPA exposure has been linked to changes in miR expression patterns in whole placenta and TB cell lines (Avissar-Whiting et al., 2010; De Felice et al., 2015; Gao et al., 2018; Kaur et al., 2021). BPA treatment of human immortalized cytoTB cell lines and subsequent microarray analysis showed that several miRs had altered expression following this treatment, in particular miR-146a expression was strongly upregulated by BPA (Avissar-Whiting et al., 2010). Overexpression of miR-146a in these cell lines reduced cellular proliferation and rendered the cells more vulnerable to a DNA mutagenic agent (Avissar-Whiting et al., 2010). Genome-wide miR expression profiling revealed that maternal exposure to BPA significantly correlated with overexpression of miR-146a in whole placenta (De Felice et al., 2015). In experiments with California mice exposed to BPA, genistein, or the combination of these two EDCs, we found that developmental exposure to one or both compounds upregulated miR-146 in the hypothalamus of males and females (Kaur et al., 2021). In the testes, BPA induced miR-146a-5p that in turn impaired steroidogenesis through negative regulation of Metastatic tumor antigen 3 (MTA3) signaling (Gao et al., 2018). Taken together, miR-146 might be a biomarker for xenoestrogen exposure in mammals.
Analyses of miR expression patterns in placenta derived from a National Children’s Study (NCS) sought to link such profiles to maternal exposure to a variety of environmental toxicants, dichlorodiphenyldichloroethylene (DDE), bisphenol A (BPA), polybrominated diphenyl ethers (PBDEs), polychlorinated biphenyls (PCBs), arsenic (As), mercury (Hg), lead (Pb), and cadmium (Cd) (Li et al., 2015). PBDE 209 positively correlated with miR-188-5p but inversely associated with let-7c. PCBs and Cd positively associated with miR-1537 expression. Hg and Pb exposure were linked with down-regulation of several let-7 family members. However, maternal exposure to DDE or BPA levels were not associated with any changes in miR expression. The conflicting results between the one cohort study above that found a linkage between BPA and expression of miR-146a in the placenta and the NCS findings might relate to variation in BPA exposure for the cohort population of pregnant women examined, number of women enrolled, and sequencing technique, including depth of coverage.
The aforementioned Rhode Island Child Healthy Study explored the linkage between placental cadmium concentrations and lncRNA expression in the placenta (Hussey et al., 2020). MIR22HG and ERVH48-showed increasing expression corresponding to cadmium exposure and was linked with elevated odds of small for gesational age birth. In contrast, A114763.2 and LINC02595 demonstrated reduced expression relative to cadmium concentrations but increased odds for large for gestational age birth with increasing expression. In a Bangladesh cohort population with known exposure to arsenic, several placental miRs, miR-1290, miR-195, and miR27a, were negatively associated with birthweight, and miR-1290 expression varied based on arsenic exposure (Rahman et al., 2018).
One study explored whether maternal exposure to PM2.5 air pollution during different pregnancy trimesters was linked with varying changes in placental miR (Tsamou et al., 2018). Accordingly, miR-21 and miR-222 expression in the placenta was inversely associated with PM2.5 exposure during the 2nd trimester of pregnancy. However, exposure during the first trimester appeared to increase placental expression of miR-20a and miR-21. Based on the miR expression patterns, target mRNAs can be predicted, and the expression of tumor suppressor phosphatase and tensin homolog (PTEN) seems to be affected by the miRs that changed in response to maternal exposure to PM. Correspondingly, placental PTEN expression was positively associated with 3rd trimester PM2.5 exposure (Tsamou et al., 2018).
Conclusion
In acting as the gatekeeper, the placenta is vulnerable to xenobiotics circulating in the maternal bloodstream, especially in rodents and humans who exhibit an invasive, hemochorial type of placentation. In this review, we have considered the impact of EDCs, other environmental toxicants, pharmaceutical agents, maternal smoking, and air pollution on the placental transcriptome and other omics, including miR profiles, along with associated changes such compounds induced on placental morphology. What emerges from many of these studies is that such xenobiotics act upon endogenous receptors and transporters, e.g., steroid receptors, SERT inhibitors, opioid receptors, and nicotinic acetylcholine receptor, naturally expressed by the placenta that when bound by their endogenous ligands are crucial in regulating placental responses. Binding of xenobiotics to such receptors or transporters evades normally homeostatic regulation and prevents the endogenous ligands from binding and activating their cognate receptors.
The placenta is an ephemeral organ but how it responds to such xenobiotics can lead to longstanding effects on fetal health. Such is particularly true in the case of the fetal brain that depends upon placental hormones, especially 5-HT, for initial forebrain development (Bonnin and Levitt, 2011; Rosenfeld, 2020, 2021). The current studies show that exposure to BPA, VPA, and flame retardants suppress placental 5-HT, dopamine, and likely other neurotransmitters (Rubinchik-Stern et al., 2018; Mao et al., 2020; Rock et al., 2020). Such changes assumingly disrupt paracrine actions that these factors would otherwise stimulate in the placenta, but such placental disturbances can also lead to ramifications on the fetal brain whose initial development depends upon placental transfer of such substances, in particular placental derived 5-HT (Bonnin and Levitt, 2011; Rosenfeld, 2020, 2021). 5-HT acting as a morphogen may be one of the primary conductors regulating early neural crest formation, metamorphosis, neurogenesis, cell motility, synaptogenesis, and development of the nociceptive system (Lauder, 1988; Lauder et al., 1988; Whitaker-Azmitia, 1991; Herlenius and Lagercrantz, 2001). Definitive evidence that the placenta is the initial source of 5-HT for the developing fetal brain comes from studies that blocked placental tryptophan hydroxylase 1 (TPH1) enzymatic activity by in utero injecting the TPH inhibitor p-chlorophenyalanine (PCPA) into the labyrinth region of E14.5 placentas (Bonnin and Levitt, 2011). Predictably, direct and short exposure to this pharmacological inhibitor suppressed 5-HT levels in the placenta, as well as notably in the fetal forebrain. Thus, factors that affect placental synthesis and ability to amass 5-HT can also dramatically shape early brain development that can result in longstanding neurobehavioral changes. This inter-connection between the two organ systems, has been branded, the placenta-brain-axis (Rosenfeld, 2021). Placental transmission of 5-HT is likely one of many ways the placenta influences fetal brain development, as shown in Figure 1. Many neurobehavioral disorders likely trace their genesis to pathophysiological changes in the placenta (Marsit et al., 2012; Lesseur et al., 2014; Rosenfeld, 2015a).
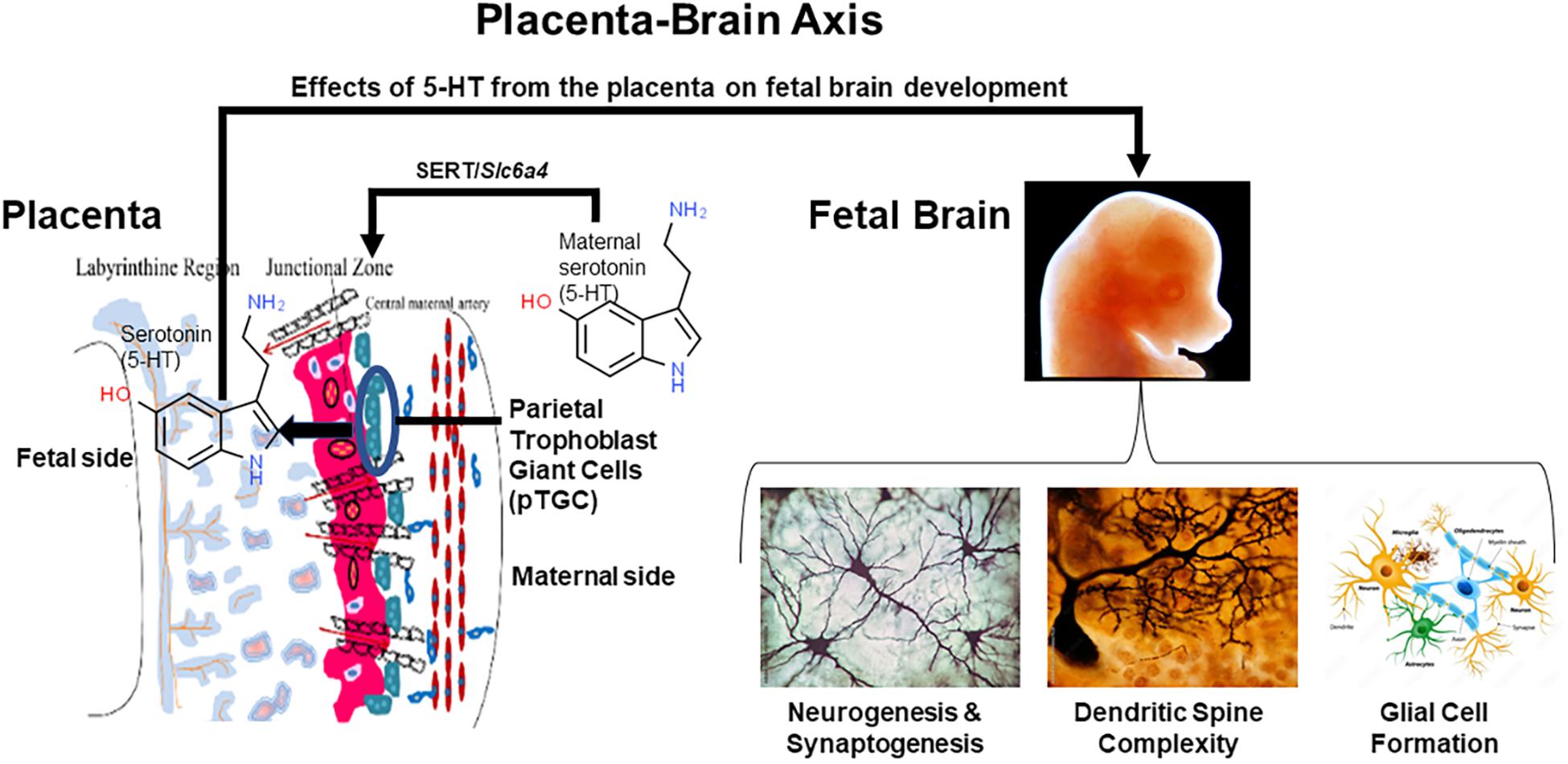
Figure 1. The placenta-brain axis and effects of placental 5-HT on fetal brain development. The parietal trophoblast giant cells (pTGC) in the fetal placenta may amass maternal 5-HT via SERT or may synthesize this morphogen. Placental-derived 5-HT may then act upon the developing fetal brain to promote neurogenesis, synaptogenesis, increase dendritic spine complexity, and glial cell formation. In essence, early embryology and brain development may depend upon 5-HT originating from the placenta.
One thing that also stands out in tracing the journey of discovery based on these omics approaches is that the experiments detailed herein employed techniques considered innovative for their time. However, ideas and technologies continue to evolve, and we must adapt our approaches accordingly. The studies described in this review were done with either whole placenta or isolated TB cell lines. Yet, the placenta in rodents and humans is a complex mixture of cells, and it is important to pinpoint how xenobiotics affect individual placental cell populations and how such changes may affect neighboring cells. The recently developed Visium spatial transcriptomics (ST) technology from 10X Genomics allows quantification of mRNA populations in the spatial context of intact tissue (Berglund et al., 2018; Maniatis et al., 2019). We have recently used this approach with mouse uteri (Mesa et al., 2021), but no studies to date have used novel method with placental samples. Single cell and single nuclei RNA-seq have been performed to understand human and mouse TB differentiation (Liu et al., 2018; Suryawanshi et al., 2018; Pique-Regi et al., 2019; West et al., 2019; Marsh and Blelloch, 2020). The architectural landscape of the placenta though is destroyed with both of these approaches. Thus, ST technology where the histoarchitecture is retained may complement these other techniques that allow for examination of gene expression patterns at the cellular or tissue level. Such approaches are predicted to become available to be able to characterize how varying extrinsic and intrinsic factors affect the DNA methylome, proteome, and global miR/other small RNA expression patterns down to the cellular level. MixOmics analyses (Rohart et al., 2017) and other integrative correlation analyses to be developed will permit integration of omics data and establish linkages between such molecular alterations to phenotypic changes in the placenta.
In conclusion, the studies to date provide strong evidence that xenobiotics affect placenta structure and molecular processes that assumingly affect placental morphology. The coming years will assuredly be devoted to tracing xenobiotic affects to individual TB cell populations and how such effects change over the course of pregnancy. How xenobiotics modify the placenta-brain axis is also predicted to become an important avenue of research. Identification of such placental changes may provide a mechanistic understanding of the fetal origin of neurobehavioral disorders in general and open up new avenues for early diagnosis and potential treatments for patients with autism spectrum disorders (ASD) caused by placental dysfunction during pregnancy.
Author Contributions
CR researched, wrote, and edited the article.
Funding
CR is supported by 1R01ES025547.
Conflict of Interest
The author declares that the research was conducted in the absence of any commercial or financial relationships that could be construed as a potential conflict of interest.
Publisher’s Note
All claims expressed in this article are solely those of the authors and do not necessarily represent those of their affiliated organizations, or those of the publisher, the editors and the reviewers. Any product that may be evaluated in this article, or claim that may be made by its manufacturer, is not guaranteed or endorsed by the publisher.
References
Adibi, J. J., Buckley, J. P., Lee, M. K., Williams, P. L., Just, A. C., Zhao, Y., et al. (2017a). Maternal urinary phthalates and sex-specific placental mRNA levels in an urban birth cohort. Environ. Health 16:35.
Adibi, J. J., Zhao, Y., Zhan, L. V., Kapidzic, M., Larocque, N., Koistinen, H., et al. (2017b). An investigation of the single and combined phthalate metabolite effects on human chorionic gonadotropin expression in placental cells. Environ. Health Perspect. 125:107010. doi: 10.1289/ehp1539
Ahmed, M. S., Cemerikic, B., and Agbas, A. (1992). Properties and functions of human placental opioid system. Life Sci. 50, 83–97. doi: 10.1016/0024-3205(92)90290-6
Ahmed, S., Mahabbat-E Khoda, S., Rekha, R. S., Gardner, R. M., Ameer, S. S., Moore, S., et al. (2011). Arsenic-associated oxidative stress, inflammation, and immune disruption in human placenta and cord blood. Environ. Health Perspect. 119, 258–264. doi: 10.1289/ehp.1102086
Aishah, A., Hinton, T., and Machaalani, R. (2017). Cellular protein and mRNA expression of β1 nicotinic acetylcholine receptor (nAChR) subunit in brain, skeletal muscle and placenta. Int. J. Dev. Neurosci. 58, 9–16. doi: 10.1016/j.ijdevneu.2017.01.011
Appleton, A. A., Jackson, B. P., Karagas, M., and Marsit, C. J. (2017). Prenatal exposure to neurotoxic metals is associated with increased placental glucocorticoid receptor DNA methylation. Epigenetics 12, 607–615. doi: 10.1080/15592294.2017.1320637
Avissar-Whiting, M., Veiga, K. R., Uhl, K. M., Maccani, M. A., Gagne, L. A., Moen, E. L., et al. (2010). Bisphenol A exposure leads to specific microRNA alterations in placental cells. Reprod. Toxicol. 29, 401–406. doi: 10.1016/j.reprotox.2010.04.004
Berglund, E., Maaskola, J., Schultz, N., Friedrich, S., Marklund, M., Bergenstrahle, J., et al. (2018). Spatial maps of prostate cancer transcriptomes reveal an unexplored landscape of heterogeneity. Nat. Commun. 9:2419.
Bonnin, A., and Levitt, P. (2011). Fetal, maternal, and placental sources of serotonin and new implications for developmental programming of the brain. Neuroscience 197, 1–7. doi: 10.1016/j.neuroscience.2011.10.005
Bruchova, H., Vasikova, A., Merkerova, M., Milcova, A., Topinka, J., Balascak, I., et al. (2010). Effect of maternal tobacco smoke exposure on the placental transcriptome. Placenta 31, 186–191. doi: 10.1016/j.placenta.2009.12.016
Calafat, A. M., Ye, X., Wong, L. Y., Reidy, J. A., and Needham, L. L. (2008). Exposure of the U.S. population to bisphenol A and 4-tertiary-octylphenol: 2003-2004. Environ. Health Perspect. 116, 39–44. doi: 10.1289/ehp.10753
Caldwell, K. E., Labrecque, M. T., Solomon, B. R., Ali, A., and Allan, A. M. (2015). Prenatal arsenic exposure alters the programming of the glucocorticoid signaling system during embryonic development. Neurotoxicol. Teratol. 47, 66–79. doi: 10.1016/j.ntt.2014.11.006
Cardenas, A., Houseman, E. A., Baccarelli, A. A., Quamruzzaman, Q., Rahman, M., Mostofa, G., et al. (2015). In utero arsenic exposure and epigenome-wide associations in placenta, umbilical artery, and human umbilical vein endothelial cells. Epigenetics 10, 1054–1063. doi: 10.1080/15592294.2015.1105424
Cardenas, A., Lutz, S. M., Everson, T. M., Perron, P., Bouchard, L., and Hivert, M. F. (2019). Mediation by Placental DNA Methylation of the association of prenatal maternal smoking and birth weight. Am. J. Epidemiol. 188, 1878–1886. doi: 10.1093/aje/kwz184
CDC (2019). Basics About Opioid Use During Pregnancy. Available online at: https://Www.Cdc.Gov/Pregnancy/Opioids/Basics.Html (accessed September 13, 2021).
Cemerikic, B., Cheng, J., Agbas, A., and Ahmed, M. S. (1991). Opioids regulate the release of human chorionic gonadotropin hormone from trophoblast tissue. Life Sci. 49, 813–824. doi: 10.1016/0024-3205(91)90246-8
Cemerikic, B., Genbacev, O., Sulovic, V., and Beaconsfield, R. (1988). Effect of morphine on hCG release by first trimester human trophoblast in vitro. Life Sci. 42, 1773–1779. doi: 10.1016/0024-3205(88)90044-6
Cemerikic, B., Schabbing, R., and Ahmed, M. S. (1992). Selectivity and potency of opioid peptides in regulating human chorionic gonadotropin release from term trophoblast tissue. Peptides 13, 897–903. doi: 10.1016/0196-9781(92)90047-7
Cemerikic, B., Zamah, R., and Ahmed, M. S. (1994). Opioids regulation of human chorionic gonadotropin release from trophoblast tissue is mediated by gonadotropin releasing hormone. J. Pharmacol. Exp. Ther. 268, 971–977.
Chateauvieux, S., Morceau, F., Dicato, M., and Diederich, M. (2010). Molecular and therapeutic potential and toxicity of valproic acid. J. Biomed. Biotechnol. 2010:479364.
Chen, J., Qiu, M., Huang, Z., Chen, J., Zhou, C., Han, F., et al. (2020). Nicotine suppresses the invasiveness of human trophoblasts by downregulation of CXCL12 expression through the alpha-7 subunit of the nicotinic acetylcholine receptor. Reprod. Sci. 27, 916–924. doi: 10.1007/s43032-019-00095-4
Chhabra, D., Sharma, S., Kho, A. T., Gaedigk, R., Vyhlidal, C. A., Leeder, J. S., et al. (2014). Fetal lung and placental methylation is associated with in utero nicotine exposure. Epigenetics 9, 1473–1484. doi: 10.4161/15592294.2014.971593
Clabault, H., Cohen, M., Vaillancourt, C., and Sanderson, J. T. (2018a). Effects of selective serotonin-reuptake inhibitors (SSRIs) in JEG-3 and HIPEC cell models of the extravillous trophoblast. Placenta 72-73, 62–73.
Clabault, H., Flipo, D., Guibourdenche, J., Fournier, T., Sanderson, J. T., and Vaillancourt, C. (2018b). Effects of selective serotonin-reuptake inhibitors (SSRIs) on human villous trophoblasts syncytialization. Toxicol. Appl. Pharmacol. 349, 8–20. doi: 10.1016/j.taap.2018.04.018
Clancy, J. L., Nousch, M., Humphreys, D. T., Westman, B. J., Beilharz, T. H., and Preiss, T. (2007). Methods to analyze microRNA-mediated control of mRNA translation. Methods Enzymol. 431, 83–111. doi: 10.1016/s0076-6879(07)31006-9
Corbel, T., Perdu, E., Gayrard, V., Puel, S., Lacroix, M. Z., Viguié, C., et al. (2015). Conjugation and deconjugation reactions within the fetoplacental compartment in a sheep model: a key factor determining bisphenol A fetal exposure. Drug Metab. Dispos. 43, 467–476. doi: 10.1124/dmd.114.061291
Daugaard, C. A., Pedersen, L., Sun, Y., Dreier, J. W., and Christensen, J. (2020). Association of prenatal exposure to valproate and other antiepileptic drugs with intellectual disability and delayed childhood milestones. JAMA Netw. Open 3:e2025570. doi: 10.1001/jamanetworkopen.2020.25570
De Felice, B., Manfellotto, F., Palumbo, A., Troisi, J., Zullo, F., Di Carlo, C., et al. (2015). Genome-wide microRNA expression profiling in placentas from pregnant women exposed to BPA. BMC Med. Genomics 8:56. doi: 10.1186/s12920-015-0131-z
Deyssenroth, M. A., Rosa, M. J., Eliot, M. N., Kelsey, K. T., Kloog, I., Schwartz, J. D., et al. (2021). Placental gene networks at the interface between maternal PM(2.5) exposure early in gestation and reduced infant birthweight. Environ. Res. 199:111342. doi: 10.1016/j.envres.2021.111342
Eadie, M. J. (2014). Treating epilepsy in pregnant women. Expert Opin. Pharmacother. 15, 841–850. doi: 10.1517/14656566.2014.896902
Elkjaer, L. S., Bech, B. H., Sun, Y., Laursen, T. M., and Christensen, J. (2018). Association between prenatal valproate exposure and performance on standardized language and mathematics tests in school-aged children. JAMA Neurol. 75, 663–671. doi: 10.1001/jamaneurol.2017.5035
Fa, S., Larsen, T. V., Bilde, K., Daugaard, T. F., Ernst, E. H., Lykke-Hartmann, K., et al. (2018). Changes in first trimester fetal CYP1A1 and AHRR DNA methylation and mRNA expression in response to exposure to maternal cigarette smoking. Environ. Toxicol. Pharmacol. 57, 19–27. doi: 10.1016/j.etap.2017.11.007
Fa, S., Larsen, T. V., Bilde, K., Daugaard, T. F., Ernst, E. H., Olesen, R. H., et al. (2016). Assessment of global DNA methylation in the first trimester fetal tissues exposed to maternal cigarette smoking. Clin. Epigenetics 8:128.
Ferguson, K. K., Loch-Caruso, R., and Meeker, J. D. (2011). Urinary phthalate metabolites in relation to biomarkers of inflammation and oxidative stress: NHANES 1999-2006. Environ. Res. 111, 718–726. doi: 10.1016/j.envres.2011.02.002
Galloway, T., Cipelli, R., Guralnick, J., Ferrucci, L., Bandinelli, S., Corsi, A. M., et al. (2010). Daily bisphenol A excretion and associations with sex hormone concentrations: results from the InCHIANTI adult population study. Environ. Health Perspect. 118, 1603–1608.
Gao, G. Z., Zhao, Y., Li, H. X., and Li, W. (2018). Bisphenol A-elicited miR-146a-5p impairs murine testicular steroidogenesis through negative regulation of Mta3 signaling. Biochem. Biophys. Res. Commun. 501, 478–485. doi: 10.1016/j.bbrc.2018.05.017
Gao, H., Zhang, Y. W., Huang, K., Yan, S. Q., Mao, L. J., Ge, X., et al. (2017). Urinary concentrations of phthalate metabolites in early pregnancy associated with clinical pregnancy loss in Chinese women. Sci. Rep. 7:6800.
Grand View Research (2014). Global Bisphenol A (BPA) Market by Appliation (Appliances, Automotive, Consumer, Construction, Electrical & Electronics) Expected to Reach USD 20.03 Billion by 2020. Available online at: http://www.digitaljournal.com/pr/2009287 (accessed September 13, 2021).
Grandjean, P., Barouki, R., Bellinger, D. C., Casteleyn, L., Chadwick, L. H., Cordier, S., et al. (2015). Life-long implications of developmental exposure to environmental stressors: new perspectives. Endocrinology 156, 3408–3415.
Green, B. B., Karagas, M. R., Punshon, T., Jackson, B. P., Robbins, D. J., Houseman, E. A., et al. (2016). Epigenome-wide assessment of DNA methylation in the placenta and arsenic exposure in the New Hampshire birth cohort study (USA). Environ. Health Perspect. 124, 1253–1260. doi: 10.1289/ehp.1510437
Green, M. T., Martin, R. E., Kinkade, J. A., Schmidt, R. R., Bivens, N. J., Tuteja, G., et al. (2020). Maternal oxycodone treatment causes pathophysiological changes in the mouse placenta. Placenta 100, 96–110. doi: 10.1016/j.placenta.2020.08.006
Grindler, N. M., Vanderlinden, L., Karthikraj, R., Kannan, K., Teal, S., Polotsky, A. J., et al. (2018). Exposure to phthalate, an endocrine disrupting chemical, alters the first trimester placental methylome and transcriptome in women. Sci. Rep. 8:6086.
Hadden, C., Fahmi, T., Cooper, A., Savenka, A. V., Lupashin, V. V., Roberts, D. J., et al. (2017). Serotonin transporter protects the placental cells against apoptosis in caspase 3-independent pathway. J. Cell. Physiol. 232, 3520–3529. doi: 10.1002/jcp.25812
Heidari, Z., Mahmoudzadeh-Sagheb, H., and Sheibak, N. (2018a). Placenta structural changes in heavy smoking mothers: a stereological aspect. Curr. Med. Res. Opin. 34, 1893–1897. doi: 10.1080/03007995.2018.1444590
Heidari, Z., Mahmoudzadeh-Sagheb, H., and Sheibak, N. (2018b). Quantitative changes of extravillous trophoblast cells in heavy smoker mothers compared with healthy controls. Reprod. Fertil. Dev. 30, 409–414. doi: 10.1071/rd17041
Herlenius, E., and Lagercrantz, H. (2001). Neurotransmitters and neuromodulators during early human development. Early Hum. Dev. 65, 21–37. doi: 10.1016/s0378-3782(01)00189-x
Hines, C. J., Christianson, A. L., Jackson, M. V., Ye, X., Pretty, J. R., Arnold, J. E., et al. (2018). An evaluation of the relationship among urine, air, and hand measures of exposure to bisphenol A (BPA) in US manufacturing workers. Ann. Work Expo. Health 62, 840–851. doi: 10.1093/annweh/wxy042
Huang, W. Q., Zhang, C. L., Di, X. Y., and Zhang, R. Q. (1998). Studies on the localization of 5-hydroxytryptamine and its receptors in human placenta. Placenta 19, 655–661. doi: 10.1016/s0143-4004(98)90027-3
Hudon Thibeault, A. A., Laurent, L., Vo Duy, S., Sauve, S., Caron, P., Guillemette, C., et al. (2017). Fluoxetine and its active metabolite norfluoxetine disrupt estrogen synthesis in a co-culture model of the feto-placental unit. Mol. Cell. Endocrinol. 442, 32–39. doi: 10.1016/j.mce.2016.11.021
Hussey, M. R., Burt, A., Deyssenroth, M. A., Jackson, B. P., Hao, K., Peng, S., et al. (2020). Placental lncRNA expression associated with placental cadmium concentrations and birth weight. Environ. Epigenet. 6:dvaa003.
Huuskonen, P., Amezaga, M. R., Bellingham, M., Jones, L. H., Storvik, M., Häkkinen, M., et al. (2016). The human placental proteome is affected by maternal smoking. Reprod. Toxicol. 63, 22–31. doi: 10.1016/j.reprotox.2016.05.009
Huybrechts, K. F., Palmsten, K., Mogun, H., Kowal, M., Avorn, J., Setoguchi-Iwata, S., et al. (2013). National trends in antidepressant medication treatment among publicly insured pregnant women. Gen. Hosp. Psychiatry 35, 265–271. doi: 10.1016/j.genhosppsych.2012.12.010
Imanishi, S., Manabe, N., Nishizawa, H., Morita, M., Sugimoto, M., Iwahori, M., et al. (2003). Effects of oral exposure of bisphenol A on mRNA expression of nuclear receptors in murine placentae assessed by DNA microarray. J. Reprod. Dev. 49, 329–336. doi: 10.1262/jrd.49.329
Jauniaux, E., Suri, S., and Muttukrishna, S. (2013). Evaluation of the impact of maternal smoking on ultrasound and endocrinological markers of first trimester placentation. Early Hum. Dev. 89, 777–780. doi: 10.1016/j.earlhumdev.2013.06.005
Jinno, N., Furugen, A., Kurosawa, Y., Kanno, Y., Narumi, K., Kobayashi, M., et al. (2020). Effects of single and repetitive valproic acid administration on the gene expression of placental transporters in pregnant rats: an analysis by gestational period. Reprod. Toxicol. 96, 47–56. doi: 10.1016/j.reprotox.2020.04.077
Jo, S. H., Lee, M. H., Kim, K. H., and Kumar, P. (2018). Characterization and flux assessment of airborne phthalates released from polyvinyl chloride consumer goods. Environ. Res. 165, 81–90. doi: 10.1016/j.envres.2018.04.007
Jones, H. E., Kaltenbach, K., Benjamin, T., Wachman, E. M., and O’grady, K. E. (2019). Prenatal opioid exposure, neonatal abstinence syndrome/neonatal opioid withdrawal syndrome, and later child development research: shortcomings and solutions. J. Addict. Med. 13, 90–92. doi: 10.1097/adm.0000000000000463
Kang, E. R., Iqbal, K., Tran, D. A., Rivas, G. E., Singh, P., Pfeifer, G. P., et al. (2011). Effects of endocrine disruptors on imprinted gene expression in the mouse embryo. Epigenetics 6, 937–950. doi: 10.4161/epi.6.7.16067
Kaur, S., Kinkade, J. A., Green, M. T., Martin, R. E., Willemse, T. E., Bivens, N. J., et al. (2021). Disruption of global hypothalamic microRNA (miR) profiles and associated behavioral changes in California mice (Peromyscus californicus) developmentally exposed to endocrine disrupting chemicals. Horm. Behav. 128:104890. doi: 10.1016/j.yhbeh.2020.104890
Khera, K. S. (1992). Valproic acid-induced placental and teratogenic effects in rats. Teratology 45, 603–610. doi: 10.1002/tera.1420450605
Kim, W., Cho, Y., Song, M. K., Lim, J. H., Kim, J. Y., Gye, M. C., et al. (2018). Effect of particulate matter 2.5 on gene expression profile and cell signaling in JEG-3 human placenta cells. Environ. Toxicol. 33, 1123–1134. doi: 10.1002/tox.22591
Kingsley, S. L., Deyssenroth, M. A., Kelsey, K. T., Awad, Y. A., Kloog, I., Schwartz, J. D., et al. (2017). Maternal residential air pollution and placental imprinted gene expression. Environ. Int. 108, 204–211. doi: 10.1016/j.envint.2017.08.022
Konkel, L. (2016). Arsenic and the placental epigenome: unlocking the secrets of prenatal exposure. Environ. Health Perspect. 124:A148.
Lan, X., Fu, L. J., Zhang, J., Liu, X. Q., Zhang, H. J., Zhang, X., et al. (2017). Bisphenol A exposure promotes HTR-8/SVneo cell migration and impairs mouse placentation involving upregulation of integrin-beta1 and MMP-9 and stimulation of MAPK and PI3K signaling pathways. Oncotarget 8, 51507–51521. doi: 10.18632/oncotarget.17882
Larsen, S., Haavaldsen, C., Bjelland, E. K., Dypvik, J., Jukic, A. M., and Eskild, A. (2018). Placental weight and birthweight: the relations with number of daily cigarettes and smoking cessation in pregnancy. A population study. Int. J. Epidemiol. 47, 1141–1150. doi: 10.1093/ije/dyy110
Lauder, J. M. (1988). Neurotransmitters as morphogens. Prog. Brain Res. 73, 365–387. doi: 10.1016/s0079-6123(08)60516-6
Lauder, J. M., Tamir, H., and Sadler, T. W. (1988). Serotonin and morphogenesis. I. Sites of serotonin uptake and -binding protein immunoreactivity in the midgestation mouse embryo. Development 102, 709–720. doi: 10.1242/dev.102.4.709
Laurent, L., Huang, C., Ernest, S. R., Berard, A., Vaillancourt, C., and Hales, B. F. (2016). In utero exposure to venlafaxine, a serotonin-norepinephrine reuptake inhibitor, increases cardiac anomalies and alters placental and heart serotonin signaling in the rat. Birth Defects Res. A Clin. Mol. Teratol. 106, 1044–1055. doi: 10.1002/bdra.23537
Lee, J. H., Ahn, C., Kang, H. Y., Hong, E. J., Hyun, S. H., Choi, K. C., et al. (2016). Effects of octylphenol and bisphenol A on the metal cation transporter channels of mouse placentas. Int. J. Environ. Res. Public Health 13:965. doi: 10.3390/ijerph13100965
Lesseur, C., Armstrong, D. A., Murphy, M. A., Appleton, A. A., Koestler, D. C., Paquette, A. G., et al. (2014). Sex-specific associations between placental leptin promoter DNA methylation and infant neurobehavior. Psychoneuroendocrinology 40, 1–9. doi: 10.1016/j.psyneuen.2013.10.012
Levy, M., Kovo, M., Miremberg, H., Anchel, N., Herman, H. G., Bar, J., et al. (2020). Maternal use of selective serotonin reuptake inhibitors (SSRI) during pregnancy-neonatal outcomes in correlation with placental histopathology. J. Perinatol. 40, 1017–1024. doi: 10.1038/s41372-020-0598-0
Li, Q., Kappil, M. A., Li, A., Dassanayake, P. S., Darrah, T. H., Friedman, A. E., et al. (2015). Exploring the associations between microRNA expression profiles and environmental pollutants in human placenta from the National Children’s Study (NCS). Epigenetics 10, 793–802. doi: 10.1080/15592294.2015.1066960
Lioy, P. J., Hauser, R., Gennings, C., Koch, H. M., Mirkes, P. E., Schwetz, B. A., et al. (2015). Assessment of phthalates/phthalate alternatives in children’s toys and childcare articles: review of the report including conclusions and recommendation of the Chronic Hazard Advisory Panel of the Consumer Product Safety Commission. J. Expo. Sci. Environ. Epidemiol. 25, 343–353. doi: 10.1038/jes.2015.33
Lips, K. S., Brüggmann, D., Pfeil, U., Vollerthun, R., Grando, S. A., and Kummer, W. (2005). Nicotinic acetylcholine receptors in rat and human placenta. Placenta 26, 735–746. doi: 10.1016/j.placenta.2004.10.009
Liu, Y., Fan, X., Wang, R., Lu, X., Dang, Y. L., Wang, H., et al. (2018). Single-cell RNA-seq reveals the diversity of trophoblast subtypes and patterns of differentiation in the human placenta. Cell Res. 28, 819–832. doi: 10.1038/s41422-018-0066-y
Maccani, J. Z., and Maccani, M. A. (2015). Altered placental DNA methylation patterns associated with maternal smoking: current perspectives. Adv. Genomics Genet. 2015, 205–214. doi: 10.2147/agg.s61518
Maccani, J. Z., Koestler, D. C., Houseman, E. A., Marsit, C. J., and Kelsey, K. T. (2013). Placental DNA methylation alterations associated with maternal tobacco smoking at the RUNX3 gene are also associated with gestational age. Epigenomics 5, 619–630. doi: 10.2217/epi.13.63
Machaalani, R., Ghazavi, E., Hinton, T., Makris, A., and Hennessy, A. (2018). Immunohistochemical expression of the nicotinic acetylcholine receptor (nAChR) subunits in the human placenta, and effects of cigarette smoking and preeclampsia. Placenta 71, 16–23. doi: 10.1016/j.placenta.2018.09.008
Machaalani, R., Ghazavi, E., Hinton, T., Waters, K. A., and Hennessy, A. (2014). Cigarette smoking during pregnancy regulates the expression of specific nicotinic acetylcholine receptor (nAChR) subunits in the human placenta. Toxicol. Appl. Pharmacol. 276, 204–212. doi: 10.1016/j.taap.2014.02.015
Mahaboob Basha, P., and Radha, M. J. (2017). Gestational di-n-butyl phthalate exposure induced developmental and teratogenic anomalies in rats: a multigenerational assessment. Environ. Sci. Pollut. Res. Int. 24, 4537–4551. doi: 10.1007/s11356-016-8196-6
Maniatis, S., Aijo, T., Vickovic, S., Braine, C., Kang, K., Mollbrink, A., et al. (2019). Spatiotemporal dynamics of molecular pathology in amyotrophic lateral sclerosis. Science 364, 89–93.
Mao, J., Jain, A., Denslow, N. D., Nouri, M. Z., Chen, S., Wang, T., et al. (2020). Bisphenol A and bisphenol S disruptions of the mouse placenta and potential effects on the placenta-brain axis. Proc. Natl. Acad. Sci. U.S.A. 117, 4642–4652. doi: 10.1073/pnas.1919563117
Mao, J., Zhang, X., Sieli, P. T., Falduto, M. T., Torres, K. E., and Rosenfeld, C. S. (2010). Contrasting effects of different maternal diets on sexually dimorphic gene expression in the murine placenta. Proc. Natl. Acad. Sci. U.S.A. 107, 5557–5562. doi: 10.1073/pnas.1000440107
Marsh, B., and Blelloch, R. (2020). Single nuclei RNA-seq of mouse placental labyrinth development. eLife 9:e60266.
Marsit, C. J., Lambertini, L., Maccani, M. A., Koestler, D. C., Houseman, E. A., Padbury, J. F., et al. (2012). Placenta-imprinted gene expression association of infant neurobehavior. J. Pediatr. 160, 854–860.e2.
Meakin, C. J., Szilagyi, J. T., Avula, V., and Fry, R. C. (2020). Inorganic arsenic and its methylated metabolites as endocrine disruptors in the placenta: mechanisms underpinning glucocorticoid receptor (GR) pathway perturbations. Toxicol. Appl. Pharmacol. 409:115305. doi: 10.1016/j.taap.2020.115305
Meir, M., Bishara, A., Mann, A., Udi, S., Portnoy, E., Shmuel, M., et al. (2016). Effects of valproic acid on the placental barrier in the pregnant mouse: optical imaging and transporter expression studies. Epilepsia 57, e108–e112.
Mesa, A. M., Mao, J., Medrano, T. I., Bivens, N. J., Jurkevich, A., Tuteja, G., et al. (2021). Spatial transcriptomics analysis of uterine gene expression in enhancer of zeste homolog 2 (Ezh2) conditional knockout mice. Biol. Reprod. ioab147. doi: 10.1093/biolre/ioab147
Midic, U., Goheen, B., Vincent, K. A., Vandevoort, C. A., and Latham, K. E. (2018). Changes in gene expression following long-term in vitro exposure of Macaca mulatta trophoblast stem cells to biologically relevant levels of endocrine disruptors. Reprod. Toxicol. 77, 154–165. doi: 10.1016/j.reprotox.2018.02.012
Mitchell, A. A., Gilboa, S. M., Werler, M. M., Kelley, K. E., Louik, C., and Hernandez-Diaz, S. (2011). Medication use during pregnancy, with particular focus on prescription drugs: 1976-2008. Am. J. Obstet. Gynecol. 205, 51.e1–51.e8.
Monti, B., Polazzi, E., and Contestabile, A. (2009). Biochemical, molecular and epigenetic mechanisms of valproic acid neuroprotection. Curr. Mol. Pharmacol. 2, 95–109. doi: 10.2174/1874467210902010095
Morales, E., Vilahur, N., Salas, L. A., Motta, V., Fernandez, M. F., Murcia, M., et al. (2016). Genome-wide DNA methylation study in human placenta identifies novel loci associated with maternal smoking during pregnancy. Int. J. Epidemiol. 45, 1644–1655. doi: 10.1093/ije/dyw196
Moreno-Moya, J. M., Vilella, F., and Simon, C. (2014). MicroRNA: key gene expression regulators. Fertil. Steril. 101, 1516–1523. doi: 10.1016/j.fertnstert.2013.10.042
Myllynen, P., Pasanen, M., and Pelkonen, O. (2005). Human placenta: a human organ for developmental toxicology research and biomonitoring. Placenta 26, 361–371. doi: 10.1016/j.placenta.2004.09.006
Nahar, M. S., Liao, C., Kannan, K., Harris, C., and Dolinoy, D. C. (2015). In utero bisphenol A concentration, metabolism, and global DNA methylation across matched placenta, kidney, and liver in the human fetus. Chemosphere 124, 54–60. doi: 10.1016/j.chemosphere.2014.10.071
Neradugomma, N. K., Liao, M. Z., and Mao, Q. (2017). Buprenorphine, norbuprenorphine, R-methadone, and S-methadone upregulate BCRP/ABCG2 expression by activating aryl hydrocarbon receptor in human placental trophoblasts. Mol. Pharmacol. 91, 237–249. doi: 10.1124/mol.116.107367
Neven, K. Y., Saenen, N. D., Tarantini, L., Janssen, B. G., Lefebvre, W., Vanpoucke, C., et al. (2018). Placental promoter methylation of DNA repair genes and prenatal exposure to particulate air pollution: an ENVIRONAGE cohort study. Lancet Planet Health 2, e174–e183.
Obolenskaya, M. Y., Teplyuk, N. M., Divi, R. L., Poirier, M. C., Filimonova, N. B., Zadrozna, M., et al. (2010). Human placental glutathione S-transferase activity and polycyclic aromatic hydrocarbon DNA adducts as biomarkers for environmental oxidative stress in placentas from pregnant women living in radioactivity- and chemically-polluted regions. Toxicol. Lett. 196, 80–86. doi: 10.1016/j.toxlet.2010.03.1115
Östling, H., Kruse, R., Helenius, G., and Lodefalk, M. (2019). Placental expression of microRNAs in infants born small for gestational age. Placenta 81, 46–53. doi: 10.1016/j.placenta.2019.05.001
Patrick, S. W., Schumacher, R. E., Benneyworth, B. D., Krans, E. E., Mcallister, J. M., and Davis, M. M. (2012). Neonatal abstinence syndrome and associated health care expenditures: United States, 2000-2009. J. Am. Med. Assoc. 307, 1934–1940.
Petit, A., Gallo-Payet, N., Bellabarba, D., Lehoux, J. G., and Belisle, S. (1993). The modulation of placental lactogen release by opioids: a role for extracellular calcium. Mol. Cell. Endocrinol. 90, 165–170. doi: 10.1016/0303-7207(93)90148-d
Pique-Regi, R., Romero, R., Tarca, A. L., Sendler, E. D., Xu, Y., Garcia-Flores, V., et al. (2019). Single cell transcriptional signatures of the human placenta in term and preterm parturition. eLife 8:e52004.
Punshon, T., Li, Z., Jackson, B. P., Parks, W. T., Romano, M., Conway, D., et al. (2019). Placental metal concentrations in relation to placental growth, efficiency and birth weight. Environ. Int. 126, 533–542. doi: 10.1016/j.envint.2019.01.063
Rahman, M. L., Liang, L., Valeri, L., Su, L., Zhu, Z., Gao, S., et al. (2018). Regulation of birthweight by placenta-derived miRNAs: evidence from an arsenic-exposed birth cohort in Bangladesh. Epigenetics 13, 573–590. doi: 10.1080/15592294.2018.1481704
Reinhart, M., Scarpati, L. M., Kirson, N. Y., Patton, C., Shak, N., and Erensen, J. G. (2018). The economic burden of abuse of prescription opioids: a systematic literature review from 2012 to 2017. Appl. Health Econ. Health Policy 16, 609–632. doi: 10.1007/s40258-018-0402-x
Richards, N., Reith, D., Stitely, M., and Smith, A. (2019). Developmental outcomes at age four following maternal antiepileptic drug use. Epilepsy Behav. 93, 73–79. doi: 10.1016/j.yebeh.2019.01.018
Robinson, J. F., Kapidzic, M., Hamilton, E. G., Chen, H., Puckett, K. W., Zhou, Y., et al. (2019). Genomic profiling of BDE-47 effects on human placental cytotrophoblasts. Toxicol. Sci. 167, 211–226. doi: 10.1093/toxsci/kfy230
Rock, K. D., St Armour, G., Horman, B., Phillips, A., Ruis, M., Stewart, A. K., et al. (2020). Effects of prenatal exposure to a mixture of organophosphate flame retardants on placental gene expression and serotonergic innervation in the fetal rat brain. Toxicol. Sci. 176, 203–223. doi: 10.1093/toxsci/kfaa046
Rohart, F., Gautier, B., Singh, A., and Le Cao, K.-A. (2017). mixOmics: an R package for ‘omics feature selection and multiple data integration. PLoS Comp. Biol. 13:e1005752. doi: 10.1371/journal.pcbi.1005752
Rosenfeld, C. S. (2015a). Sex-specific placental responses in fetal development. Endocrinology 156, 3422–3434. doi: 10.1210/en.2015-1227
Rosenfeld, C. S. (2015b). The Epigenome and Developmental Origns of Health and Disease. Cambridge, MA: Academic Press.
Rosenfeld, C. S. (2017). Neuroendocrine disruption in animal models due to exposure to bisphenol A analogues. Front. Neuroendocrinol. 47, 123–133. doi: 10.1016/j.yfrne.2017.08.001
Rosenfeld, C. S. (2020). Placental serotonin signaling, pregnancy outcomes, and regulation of fetal brain development. Biol. Reprod. 102, 532–538. doi: 10.1093/biolre/ioz204
Rosenfeld, C. S. (2021). The placenta-brain-axis. J. Neurosci. Res. 99, 271–283. doi: 10.1002/jnr.24603
Rousseaux, S., Seyve, E., Chuffart, F., Bourova-Flin, E., Benmerad, M., Charles, M. A., et al. (2020). Immediate and durable effects of maternal tobacco consumption alter placental DNA methylation in enhancer and imprinted gene-containing regions. BMC Med. 18:306. doi: 10.1186/s12916-020-01736-1
Rubinchik-Stern, M., Shmuel, M., Bar, J., Kovo, M., and Eyal, S. (2018). Adverse placental effects of valproic acid: studies in perfused human placentas. Epilepsia 59, 993–1003. doi: 10.1111/epi.14078
Schug, T. T., Janesick, A., Blumberg, B., and Heindel, J. J. (2011). Endocrine disrupting chemicals and disease susceptibility. J. Steroid Biochem. Mol. Biol. 127, 204–215.
Schulz, S., Wagner, S., Gerbig, S., Wächter, H., Sielaff, D., Bohn, D., et al. (2015). DESI MS based screening method for phthalates in consumer goods. Analyst 140, 3484–3491. doi: 10.1039/c5an00338e
Serra, A. E., Lemon, L. S., Mokhtari, N. B., Parks, W. T., Catov, J. M., Venkataramanan, R., et al. (2017). Delayed villous maturation in term placentas exposed to opioid maintenance therapy: a retrospective cohort study. Am. J. Obstet. Gynecol. 216, 418.e1–418.e5.
Shafique, S., and Winn, L. M. (2021). Gestational valproic acid exposure induces epigenetic modifications in murine decidua. Placenta 107, 31–40. doi: 10.1016/j.placenta.2021.03.004
Sieli, P. T., Jasarevic, E., Warzak, D. A., Mao, J., Ellersieck, M. R., Liao, C., et al. (2011). Comparison of serum bisphenol A concentrations in mice exposed to bisphenol A through the diet versus oral bolus exposure. Environ. Health Perspect. 119, 1260–1265. doi: 10.1289/ehp.1003385
Stone, J., Sutrave, P., Gascoigne, E., Givens, M. B., Fry, R. C., and Manuck, T. A. (2021). Exposure to toxic metals and per- and polyfluoroalkyl substances and the risk of preeclampsia and preterm birth in the United States: a review. Am. J. Obstet. Gynecol. MFM 3:100308. doi: 10.1016/j.ajogmf.2021.100308
Suryawanshi, H., Morozov, P., Straus, A., Sahasrabudhe, N., Max, K. E. A., Garzia, A., et al. (2018). A single-cell survey of the human first-trimester placenta and decidua. Sci. Adv. 4:eaau4788. doi: 10.1126/sciadv.aau4788
Susiarjo, M., Sasson, I., Mesaros, C., and Bartolomei, M. S. (2013). Bisphenol a exposure disrupts genomic imprinting in the mouse. PLoS Genet. 9:e1003401. doi: 10.1371/journal.pgen.1003401
Suter, M., Ma, J., Harris, A., Patterson, L., Brown, K. A., Shope, C., et al. (2011). Maternal tobacco use modestly alters correlated epigenome-wide placental DNA methylation and gene expression. Epigenetics 6, 1284–1294. doi: 10.4161/epi.6.11.17819
Tait, S., Tassinari, R., Maranghi, F., and Mantovani, A. (2015). Toxicogenomic analysis of placenta samples from mice exposed to different doses of BPA. Genom. Data 4, 109–111. doi: 10.1016/j.gdata.2015.04.004
Tan, W., Huang, H., Wang, Y., Wong, T. Y., Wang, C. C., and Leung, L. K. (2013). Bisphenol A differentially activates protein kinase C isoforms in murine placental tissue. Toxicol. Appl. Pharmacol. 269, 163–168. doi: 10.1016/j.taap.2013.03.016
Tetro, N., Imbar, T., Wohl, D., Eisenberg, I., Yagel, S., Shmuel, M., et al. (2019). The effects of valproic acid on early pregnancy human placentas: pilot ex vivo analysis in cultured placental villi. Epilepsia 60, e47–e51.
Tsamou, M., Vrijens, K., Madhloum, N., Lefebvre, W., Vanpoucke, C., and Nawrot, T. S. (2018). Air pollution-induced placental epigenetic alterations in early life: a candidate miRNA approach. Epigenetics 13, 135–146. doi: 10.1080/15592294.2016.1155012
Tsaprouni, L. G., Yang, T. P., Bell, J., Dick, K. J., Kanoni, S., Nisbet, J., et al. (2014). Cigarette smoking reduces DNA methylation levels at multiple genomic loci but the effect is partially reversible upon cessation. Epigenetics 9, 1382–1396.
Vajda, F. J. E., O’brien, T. J., Graham, J. E., Hitchcock, A. A., Lander, C. M., and Eadie, M. J. (2019). Valproate-associated foetal malformations-rates of occurrence, risks in attempted avoidance. Acta Neurol. Scand. 139, 42–48. doi: 10.1111/ane.13005
Van Wynsberghe, P. M., Chan, S. P., Slack, F. J., and Pasquinelli, A. E. (2011). Analysis of microRNA expression and function. Methods Cell Biol. 106, 219–252.
Vandenberg, L. N., Hauser, R., Marcus, M., Olea, N., and Welshons, W. V. (2007). Human exposure to bisphenol A (BPA). Reprod. Toxicol. 24, 139–177.
vom Saal, F. S., Akingbemi, B. T., Belcher, S. M., Birnbaum, L. S., Crain, D. A., Eriksen, M., et al. (2007). Chapel Hill bisphenol A expert panel consensus statement: integration of mechanisms, effects in animals and potential to impact human health at current levels of exposure. Reprod. Toxicol. 24, 131–138. doi: 10.1016/j.reprotox.2007.07.005
Votavova, H., Dostalova Merkerova, M., Fejglova, K., Vasikova, A., Krejcik, Z., Pastorkova, A., et al. (2011). Transcriptome alterations in maternal and fetal cells induced by tobacco smoke. Placenta 32, 763–770. doi: 10.1016/j.placenta.2011.06.022
Walker, N., Filis, P., O’shaughnessy, P. J., Bellingham, M., and Fowler, P. A. (2019). Nutrient transporter expression in both the placenta and fetal liver are affected by maternal smoking. Placenta 78, 10–17. doi: 10.1016/j.placenta.2019.02.010
Weinheimer, C., Wang, H., Comstock, J. M., Singh, P., Wang, Z., Locklear, B. A., et al. (2020). Maternal tobacco smoke exposure causes sex-divergent changes in placental lipid metabolism in the rat. Reprod. Sci. 27, 631–643.
West, R. C., Ming, H., Logsdon, D. M., Sun, J., Rajput, S. K., Kile, R. A., et al. (2019). Dynamics of trophoblast differentiation in peri-implantation-stage human embryos. Proc. Natl. Acad. Sci. U.S.A. 116, 22635–22644. doi: 10.1073/pnas.1911362116
Whitaker-Azmitia, P. M. (1991). Role of serotonin and other neurotransmitter receptors in brain development: basis for developmental pharmacology. Pharmacol. Rev. 43, 553–561.
WHO (2018). Available online at: https://Www.Who.Int/News-Room/Fact-Sheets/Detail/Arsenic (accessed February 15, 2018).
Winterbottom, E. F., Ban, Y., Sun, X., Capobianco, A. J., Marsit, C. J., Chen, X., et al. (2019a). Transcriptome-wide analysis of changes in the fetal placenta associated with prenatal arsenic exposure in the New Hampshire Birth Cohort Study. Environ. Health 18:100.
Winterbottom, E. F., Moroishi, Y., Halchenko, Y., Armstrong, D. A., Beach, P. J., Nguyen, Q. P., et al. (2019b). Prenatal arsenic exposure alters the placental expression of multiple epigenetic regulators in a sex-dependent manner. Environ. Health 18:18.
Wong, M. K., Holloway, A. C., and Hardy, D. B. (2016). Nicotine directly induces endoplasmic reticulum stress response in rat placental trophoblast giant cells. Toxicol. Sci. 151, 23–34. doi: 10.1093/toxsci/kfw019
Wu, L. H., Zhang, X. M., Wang, F., Gao, C. J., Chen, D., Palumbo, J. R., et al. (2018). Occurrence of bisphenol S in the environment and implications for human exposure: a short review. Sci. Total Environ. 615, 87–98. doi: 10.1016/j.scitotenv.2017.09.194
Xu, X., Chiung, Y. M., Lu, F., Qiu, S., Ji, M., and Huo, X. (2015). Associations of cadmium, bisphenol A and polychlorinated biphenyl co-exposure in utero with placental gene expression and neonatal outcomes. Reprod. Toxicol. 52, 62–70. doi: 10.1016/j.reprotox.2015.02.004
Xue, J., Wan, Y., and Kannan, K. (2016). Occurrence of bisphenols, bisphenol A diglycidyl ethers (BADGEs), and novolac glycidyl ethers (NOGEs) in indoor air from Albany, New York, USA, and its implications for inhalation exposure. Chemosphere 151, 1–8. doi: 10.1016/j.chemosphere.2016.02.038
Yazdy, M. M., Desai, R. J., and Brogly, S. B. (2015). Prescription opioids in pregnancy and birth outcomes: a review of the literature. J. Pediatr. Genet. 4, 56–70.
Zharikova, O. L., Deshmukh, S. V., Kumar, M., Vargas, R., Nanovskaya, T. N., Hankins, G. D., et al. (2007). The effect of opiates on the activity of human placental aromatase/CYP19. Biochem. Pharmacol. 73, 279–286. doi: 10.1016/j.bcp.2006.08.019
Keywords: trophoblast, serotonin, bisphenol A, endocrine disruptors, environmental chemicals, placenta-brain axis, pharmaceutical agents, smoking
Citation: Rosenfeld CS (2021) Transcriptomics and Other Omics Approaches to Investigate Effects of Xenobiotics on the Placenta. Front. Cell Dev. Biol. 9:723656. doi: 10.3389/fcell.2021.723656
Received: 11 June 2021; Accepted: 31 August 2021;
Published: 24 September 2021.
Edited by:
Michael J. Soares, University of Kansas Medical Center Research Institute, United StatesReviewed by:
Alina Maloyan, Oregon Health and Science University, United StatesKhursheed Iqbal, University of Kansas Medical Center, United States
Copyright © 2021 Rosenfeld. This is an open-access article distributed under the terms of the Creative Commons Attribution License (CC BY). The use, distribution or reproduction in other forums is permitted, provided the original author(s) and the copyright owner(s) are credited and that the original publication in this journal is cited, in accordance with accepted academic practice. No use, distribution or reproduction is permitted which does not comply with these terms.
*Correspondence: Cheryl S. Rosenfeld, cm9zZW5mZWxkY0BtaXNzb3VyaS5lZHU=