- 1Institute of Reproductive Health, Tongji Medical College, Huazhong University of Science and Technology, Wuhan, China
- 2Guangdong and Shenzhen Key Laboratory of Male Reproductive Medicine and Genetics, Institute of Urology, Peking University Shenzhen Hospital, Shenzhen-Peking University – The Hong Kong University of Science and Technology Medical Center, Shenzhen, China
- 3Department of Obstetrics and Gynaecology, Xiangyang No. 1 People’s Hospital, Hubei University of Medicine, Xiangyang, China
- 4The Second Affiliated Hospital, College of Medicine, Zhejiang University, Hangzhou, China
- 5College of Pharmacy, Hubei University of Medicine, Shiyan, China
- 6Shenzhen Huazhong University of Science and Technology Research Institute, Shenzhen, China
Non-alcoholic fatty liver disease (NAFLD) affects obesity-associated metabolic syndrome, which exhibits hepatic steatosis, insulin insensitivity and glucose intolerance. Emerging evidence suggests that microRNAs (miRNAs) are essential for the metabolic homeostasis of liver tissues. Many hepatic miRNAs located in the miR-379/miR-544 cluster were significantly increased in leptin-receptor-deficient type 2 mice (db/db), a mouse model of diabetes. However, the function of the miR-379/miR-544 cluster in the process of hepatic steatosis remains unclear. Here, we report that the novel function of miR-379/miR-544 cluster in regulating obesity-mediated metabolic dysfunction. Genetical mutation of miR-379/miR-544 cluster in mice displayed resistance to high-fat diet (HFD)-induced obesity with moderate hepatic steatosis and hypertriglyceridemia. In vitro studies revealed that silencing of miR-379 in human hepatocellular carcinoma (HepG2) cells ameliorated palmitic acid-induced elevation of cellular triglycerides, and overexpression of miR-379 had the opposite effect. Moreover, Igf1r (Insulin-like growth factor 1 receptor) and Dlk1 (Delta-like homolog 1) were directly targeted by miR-379 and miR-329, respectively, and elevated in the livers of the miR-379/miR-544 cluster knockout mice fed on HFD. Further transcriptome analyses revealed that the hepatic gene expressions are dysregulated in miR-379/miR-544 knockout mice fed with HFD. Collectively, our findings identify the miR-379/miR-544 cluster as integral components of a regulatory circuit that functions under conditions of metabolic stress to control hepatic steatosis. Thus, this miRNA cluster provides potential targets for pharmacologic intervention in obesity and NAFLD.
Introduction
Non-alcoholic fatty liver disease (NAFLD) is one major cause of chronic liver disease in the world and characterized by excessive triglyceride accumulation in the liver clinically and pathologically (Adams et al., 2005; Samuel et al., 2010; Samuel and Shulman, 2012). The disease is closely related with many kinds of metabolic disorders, such as obesity, type 2 diabetes (T2D), and dyslipidemia (Younossi et al., 2011; Ajmal et al., 2014). NAFLD in rodents is generally induced by a high-fat diet (HFD), which causes excess accumulation of triglyceride in the liver and insulin resistance (Wang et al., 2018; Zhang et al., 2018; Zhou et al., 2018). In addition, the liver is a metabolic organ that regulates metabolism of glucose and lipid, and many hepatic genes have been found essential for the development of fatty liver and insulin resistance (Postic et al., 2004). Although the pathogenesis of NAFLD has been studied extensively, the mechanism underlying is still not fully uncovered yet.
MicroRNAs (miRNAs) are a class of endogenous small non-coding RNAs (ncRNA), which could silence gene expression at the post-transcriptional level through imperfect complementary binding with the 3′-untranslated regions (UTRs) of the target mRNAs (Ambros, 2004; Bartel, 2004). Importantly, miR-24, miR-30c, miR-33, miR-122, and miR-130 have been found as key regulators of lipid metabolism (Wen and Friedman, 2012; Ramirez et al., 2013; Soh et al., 2013; Ng et al., 2014; Xiao et al., 2015). Previous microarray screening showed that the miR-379, miR-411, miR-299, and miR-543 were upregulated in the liver of hyperglucocorticoidemia and obesity (db/db mouse model) as well as human liver in a GC/GR-dependent manner (de Guia et al., 2015). These upregulated miRNAs are all located in the miR-379/miR-544 genomic cluster with high conservation in mammalian species (Glazov et al., 2008), which resides on the human and mouse chromosomes 14 and 12, respectively. Another group reported that the level of serum miR-379 was significantly up-regulated in NAFLD patients compared to normal controls (Okamoto et al., 2020). Moreover, hepatocyte-specific silencing of miR-379 remarkably reduced the level of circulating very-low-density lipoprotein (VLDL)-associated triglyceride (TG) in healthy mice and normalized unusual lipid profiles in metabolically impaired animals (de Guia et al., 2015). Previous studies have suggested that deficiency of the miR-379/miR-544 cluster led to CLPG-like muscular hypertrophy (Gao et al., 2015), but no studies have linked this miRNA cluster to hepatic lipid accumulation and metabolic dysfunction.
The Delta-like homolog 1 (DLK1) is a single transmembrane protein homologous to the Notch pathway ligand Delta but lacks typical Notch interaction sequences (Schmidt et al., 2000; Takada et al., 2000), and has been reported to regulate nutrient metabolism and protect from steatosis (Charalambous et al., 2014). DLK1 is expressed in multiple embryonic tissues before birth, but only in preadipocytes after birth (Schmidt et al., 2000). Ectopic overexpression of DLK1 resulted in a lipodystrophic phenotype related to triglyceride accumulation and glucose intolerance (Lee et al., 2003; Villena et al., 2008). In addition, insulin-like growth factor 1 (IGF1) / insulin-like growth factor 1 receptor (IGF1R) signaling pathway has been demonstrated to be essential for the process of insulin resistance, which leading to metabolic diseases and type 2 diabetes (Fernandez et al., 2001; Le Roith et al., 2002). Mice with one of the Igf1r alleles deficient (Igf1r±) globally exhibited a 10% decrease in post-natal growth, and developed glucose intolerance and insulin resistance with age (Bokov et al., 2011). However, the underlying molecular links among DLK1, IGF1/IGF1R signaling pathway, and miRNA-379/miR-544 cluster in obesity-mediated metabolic dysfunction, like NAFLD, are mostly poorly understood.
Here, we identified miR-379/miR-544 cluster as the critical regulator to resist HFD-induced obesity and regulate moderate hepatic steatosis via targeting Igf1r and Dlk1 directly. Although knockout miR-379/miR-544 cluster in mice do not exhibit overt abnormalities except for muscular hypertrophy in this study, we found that miR-379/miR-544 knockout mice (KO) offer resistance to HFD-induced obesity and moderate hepatic steatosis. Furthermore, we demonstrated that miR-329 and miR-379, which belong to the miR-379/miR-544 cluster, could directly target Igf1r and Dlk1, respectively, and repress their expression. In vitro studies also showed that silencing of miR-379 in HepG2 cells ameliorated palmitic acid (PA)-induced elevation of cellular triglycerides. Further transcriptome analyses revealed that the hepatic gene expressions are dysregulated in miR-379/miR-544 KO mice fed with HFD by RNA-seq. Our data identify a novel function of the miR-379/miR-544 cluster in regulating liver steatosis and metabolic syndrome and provide a clue of this miRNA cluster as a potential therapeutic target for obesity and NAFLD.
Results
Upregulation of miR-379/miR-544 Cluster in the Steatotic Livers of db/db Mice and HFD-Fed Mice
A previous study has shown that many hepatic miRNAs were up- or down-regulated more than two-fold in db/db diabetic mice compared to wild-type (WT) mice by microarray (de Guia et al., 2015). To explore whether the miR-379/miR-544 cluster is involved in regulating hepatic metabolism, we performed miRNA expression profiling of livers from WT and db/db mice using their microarray data. The results showed that the expression levels of nine miRNAs (miR-379, miR-411, miR-299, miR-679, miR-543, miR-300, miR-134, miR-541, and miR-337-5p) located in the miR-379/miR-544 genomic cluster were significantly upregulated in db/db mice compared to WT mice (Figure 1A and Supplementary Table 1). Then we verified the expression of three miRNAs (miR-379, miR-411, and miR-543) selected from 9 upregulated miRNAs by RT-qPCR in the steatotic livers of db/db mice and mice fed a high-fat diet, respectively. Compared with the respective control mice, db/db mice and mice fed with HFD displayed significantly increased expression levels of hepatic miR-379, miR-411, and miR-543, consistent with microarray results (Figures 1B, C). In addition, RT-qPCR revealed that most of the miRNAs (such as miR-299, miR-300, miR-495, miR-544, etc.) in the miR-379/miR-544 cluster were significantly elevated in the livers of db/db mice and mice fed with HFD compared with controls (Supplementary Figures 1A,B). Interestingly, except for in livers, we also found that the expression levels of the primary transcript of the miR-379/miR-544 cluster in white adipose tissue (WBT) of HFD-feeding mice were significantly increased compared to that of control mice (Supplementary Figure 2A). These data suggest that miR-379/miR-544 cluster might be involved in regulating hepatic metabolism in mice.
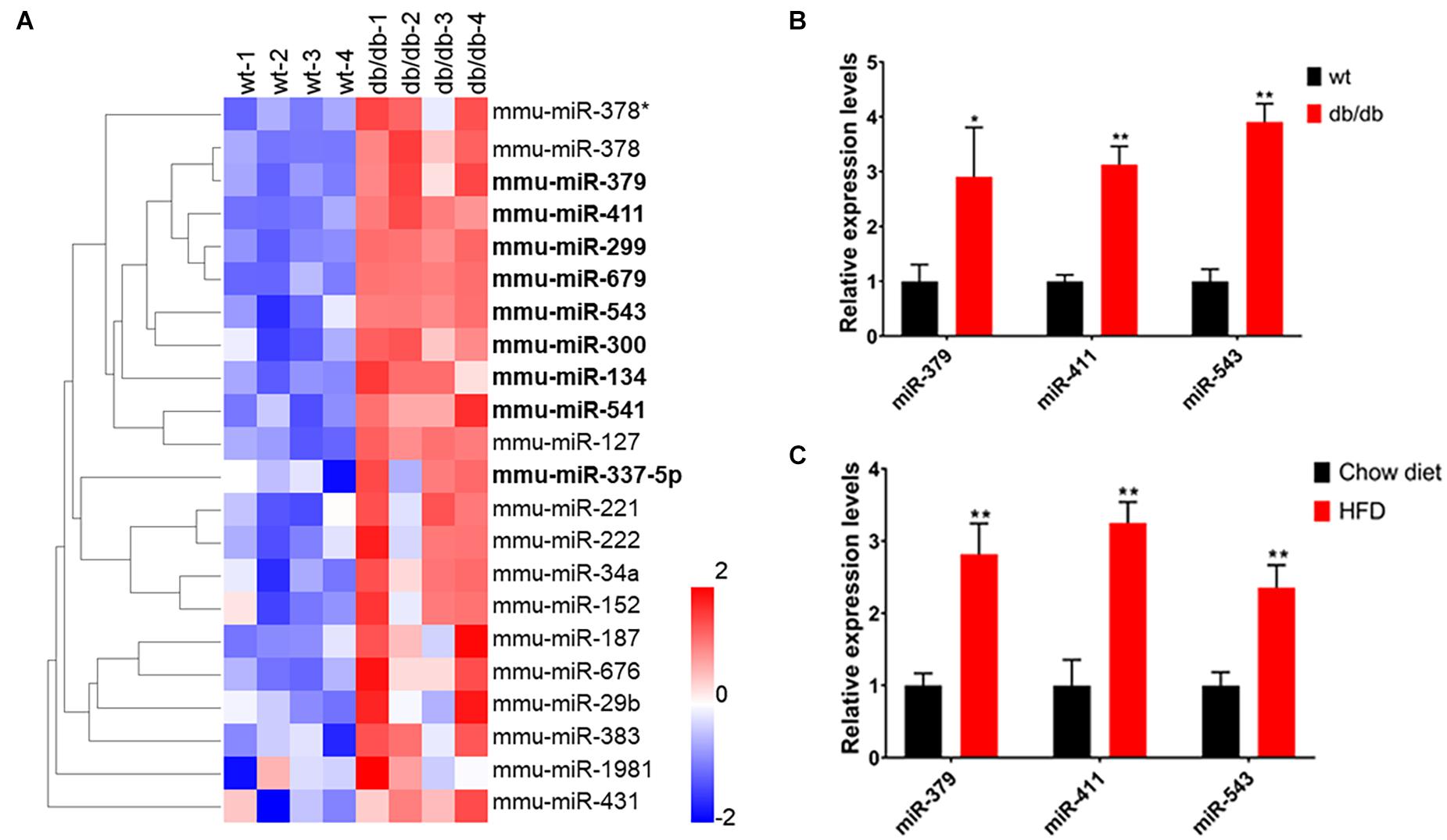
Figure 1. The miR-379/miR-544 cluster expression is upregulated in the steatotic liver of db/db mice and HFD-fed mice. (A) Heatmap showing relative miRNA expression between wild-type (wt) and db/db mice (n = 4). The higher and lower expression is showed with red and blue color, respectively. Nine significantly upregulated miRNAs (miR-379, miR-411, miR-299, miR-679, miR-543, miR-300, miR-134, miR-541, and miR-337-5p) located in the miR-379/miR-544 cluster are shown with bold font. Differentially regulated miRNAs are defined with fold changes ≥ 2-fold, P < 0.05. (B,C) Hepatic expression levels of miR-379, miR-411, and miR-543 were determined by real-time PCR in chow-fed 10-12-week-old db/db mice (B) and WT mice (n = 4) fed an HFD for 10 weeks starting at 4 weeks of age (C). *P < 0.05 and **P < 0.01.
Spatiotemporal Expression Pattern and Genetic Ablation of the miR-379/miR-544 Cluster in Mice
Since the miR-379/miR-544 locus is the largest known placental mammal-specific miRNA cluster containing 24 miRNA genes and located within the highly conserved imprinted Dlk1-Dio3 region (Figure 2A), we measured the expression pattern of the miR-379/miR-544 precursor transcript containing all 24 miRNAs in eight adult different mouse organs using semi-quantitative RT-PCR and quantitative RT-PCR (RT-qPCR). Interestingly, we found that the expression of the primary transcript of the miR-379/miR-544 cluster was mostly restricted to the adult brain, not in the adult liver (Figure 2B and Supplementary Figure 2B). We then measured the primary transcript levels of the miR-379/miR-544 cluster in the postnatal developing livers. A higher expression level of the miR-379/miR-544 cluster transcripts in perinatal livers was observed, whereas lower expression was detected in adult livers (Figure 2C and Supplementary Figure 2C). This expression pattern in the liver was similar to that reported for the miR-379/miR-544 cluster in testis (Cao et al., 2018). Given that, we assume that the miR-379/miR-544 cluster plays a role in mouse liver development. To investigate the physiological role of the miR-379/miR-544 cluster in vivo, we generated a knockout (KO) mouse line as our previous report described (Cao et al., 2018). Our breeding strategy was validated by showing the miR-379, miR-411, and miR-543 were not detected by RT-qPCR analysis in several tissues of WT and KO mice at postnatal day 20 (P20) (Figure 2D). The efficiency of miR-379/miR-544 cluster deletion in liver was further confirmed by semi-quantitative RT-PCR (Supplementary Figures 2D,E). The analyses showed that the primary transcript of the miR-379/miR-544 cluster had no expression in KO mice liver at P20, suggesting that we successfully deleted miR-379/miR-544 KO cluster in mouse liver.
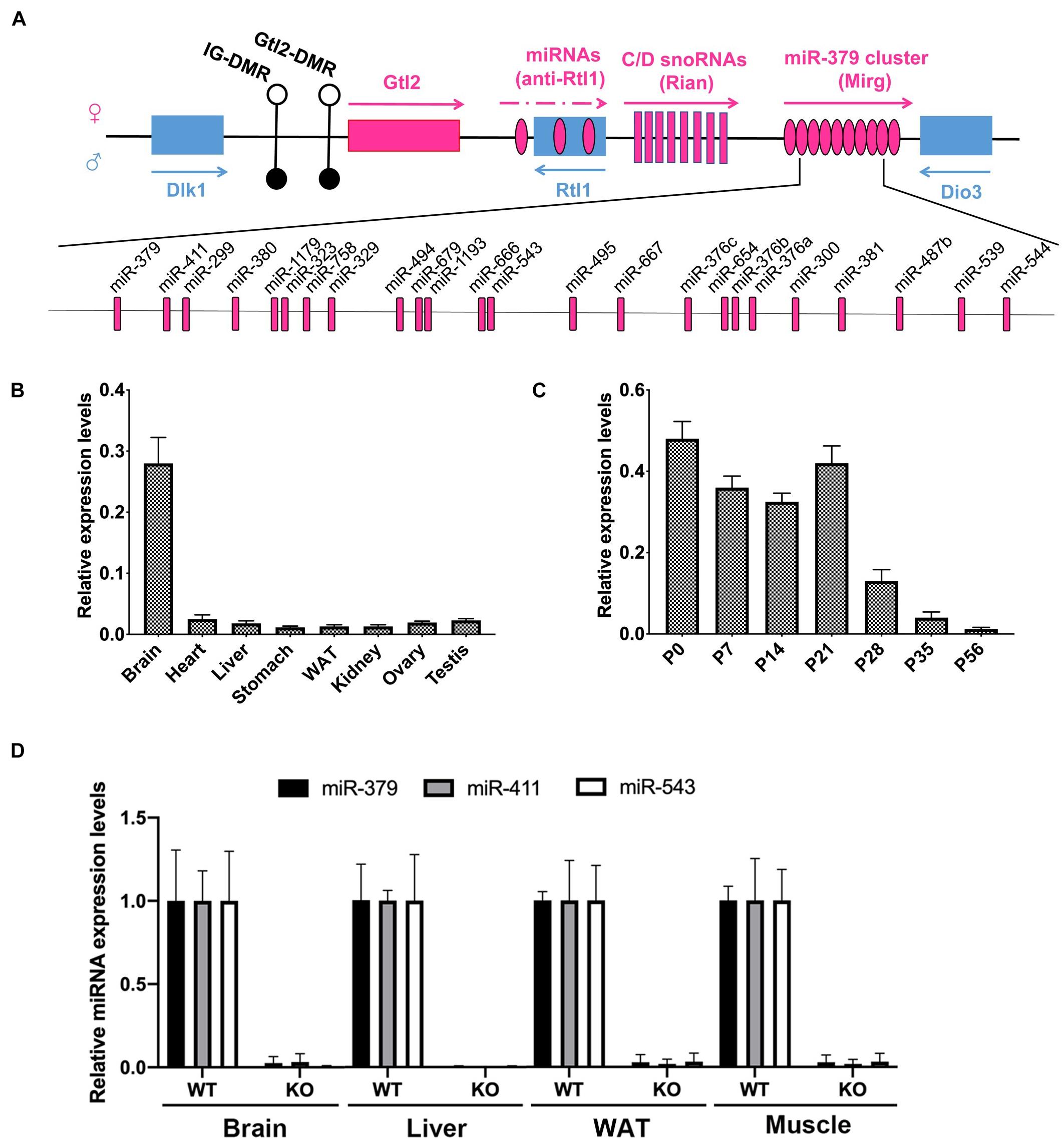
Figure 2. Expression of the miR-379/miR-544 cluster in multiple tissues and developing livers in mice. (A) Schematic representation of miR-379/miR-544 cluster at the imprinted Dlk1-Dio3 region on the mouse distal chromosome 12 (human 14q32). (B) Expression pattern of the pri-miR-379/miR-544 cluster transcript in eight different adult mouse tissues (n = 4), assayed via quantitative PCR. (C) Expression pattern of the pri-miR-379/miR-544 cluster transcript in developing mouse livers (n = 4). Livers at postnatal day 0 (P0, newborn), P7, P14, P21, P28, P35, and P56 were analyzed. (D) Expression of selected miRNAs scattered with miRNA cluster (miR-379, miR-411, and miR-543) in brain, liver, WAT (white adipose tissue), and muscle from WT and the miR-379/miR-544 cluster KO mice (n = 3) at P20 was determined by quantitative PCR.
Genetic Deletion of the miR-379/miR-544 Cluster Confers Resistance to HFD-Induced Obesity
Since the miR-379/miR-544 KO mice did not exhibit any overt abnormalities under basal conditions (Cao et al., 2018), we subjected WT and KO mice to metabolic stress by feeding an HFD for 10 weeks to further determine the role of miR-379/miR-544 in the steatotic liver (hereafter named WT-HFD and KO-HFD mice, respectively). Surprisingly, as shown in Figure 3A, WT mice displayed a significant increase in the body weight after HFD induced for 6 weeks, but KO mice gained much less body weight and were resistant to obesity induced by the HFD. Furthermore, unlike WT-HFD mice, the KO-HFD mice did not develop hepatic steatosis. And food intake remained unaltered in KO-HFD mice, compared with WT-HFD mice (Figure 3B). Interestingly, the weights of KO-HFD mouse livers exhibited a significant reduction compared to WT-HFD, but the ratio of liver weight to the body weight did not change significantly (Figure 3C). Moreover, compared with the WT-HFD mice, the KO-HFD mice had a smaller fat pad weight (Figure 3D). In addition, H&E and Oil Red O staining of hepatic histological sections provided further evidence of reduced liver fat deposition in KO-HFD mice compared with the WT-HFD mice (Figure 3E). H&E staining showed that the epididymal adipose cell size in KO-HFD mice was markedly smaller than that of in WT-HFD mice (Figures 3F,G). Consistent with these histological results, transmission electron microscope (TEM) revealed fewer lipid droplets accumulated in KO-HFD mouse liver than WT-HFD mouse liver (Supplementary Figure 3A). Notably, the levels of liver triglyceride (TG), serum TG, serum glucose, and serum cholesterol in KO-HFD mice were significantly lower than in WT-HFD mice (Figures 3H–K), suggesting global amelioration of the metabolic syndrome that accompanies obesity upon depletion of miR-379/miR-544 cluster. Together, these data indicate that genetic deletion of the miR-379/miR-544 cluster could confer resistance to HFD-induced obesity and moderate hepatic steatosis.
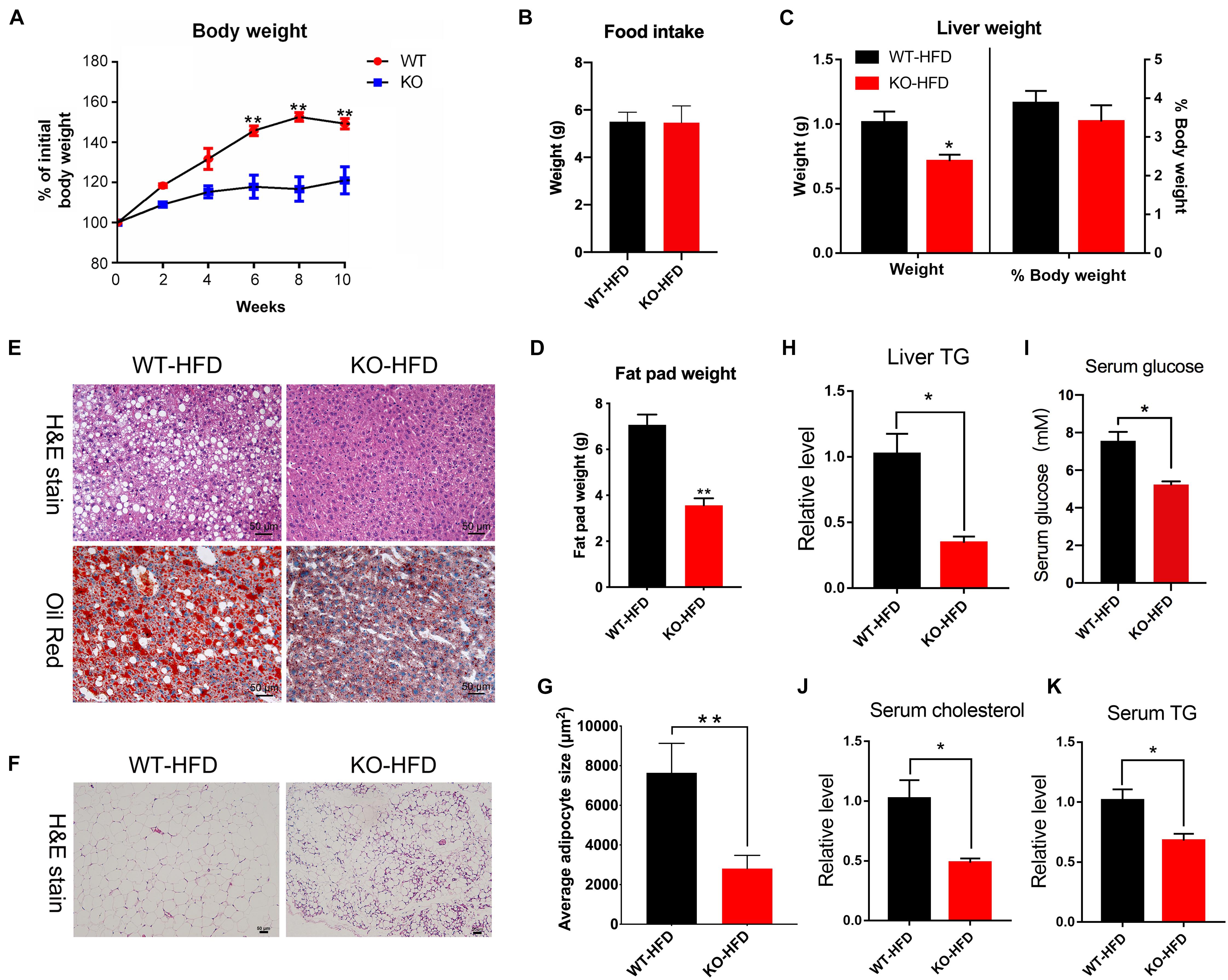
Figure 3. The miR-379/miR-544 cluster KO mice displayed resistance to HFD-induced obesity. (A) The liver growth curve shows that the miR-379/miR-544 cluster KO-HFD mice gain less weight than WT-HFD mice (n = 5). *P < 0.05 and **P < 0.01. (B) Measurement of food intake of miR-379/miR-544 cluster KO-HFD mice and WT-HFD mice (n = 3). (C) Liver weight and the ratio of liver weight to body weight in the miR-379/miR-544 cluster KO-HFD mice and WT-HFD mice (n = 3). *P < 0.05. (D) Representative images of H&E staining (top) and Oil-red O staining (bottom) of liver sections from WT-HFD (left) or the miR-379/miR-544 cluster KO-HFD (right) mice (n = 3). Scale bars = 50 μm. (E) Weight of total fat pads isolated from the miR-379/miR-544 cluster KO-HFD and WT-HFD mice (n = 3). **P < 0.01. (F) Representative images of H&E-staining of epididymal fat pads from WT-HFD and the miR-379/miR-544 cluster KO-HFD mice (n = 3). Scale bars = 50 μm. (G) Quantitation of adipocyte size between WT-HFD and KO-HFD mice as in panel (F) are shown (n = 3). **P < 0.01. (H–K) Measurement of liver TG (H), serum TG (I), random serum glucose (J), and serum cholesterol (K) levels in WT-HFD and KO-HFD mice (n = 3) are shown, respectively. *P < 0.05.
The miR-379/miR-544 Cluster Deletion Improves HFD-Induced Glucose Tolerance and Whole-Body Insulin Sensitivity
In humans and rodents, obesity is frequently associated with alterations in glucose homeostasis and insulin sensitivity. We thus carried out glucose- and insulin-tolerance tests (GTT and ITT) to determine whether glucose tolerance and clearance would be affected by genetic deletion of the miR-379/miR-544 cluster in mice under the HFD condition. The results showed that the KO-HFD mice exhibited improved glucose tolerance and clearance (Figures 4A,B). In addition, we observed KO-HFD mice exhibited reduced mRNA levels of lipogenesis-related genes (Pparγ, Fas, and Scd1) via RT-qPCR (Figure 4C). We also measured the protein level of PPARγ in WT-HFD and KO-HFD mouse livers, and the result was consistent with its mRNA level (Figures 4D,E).
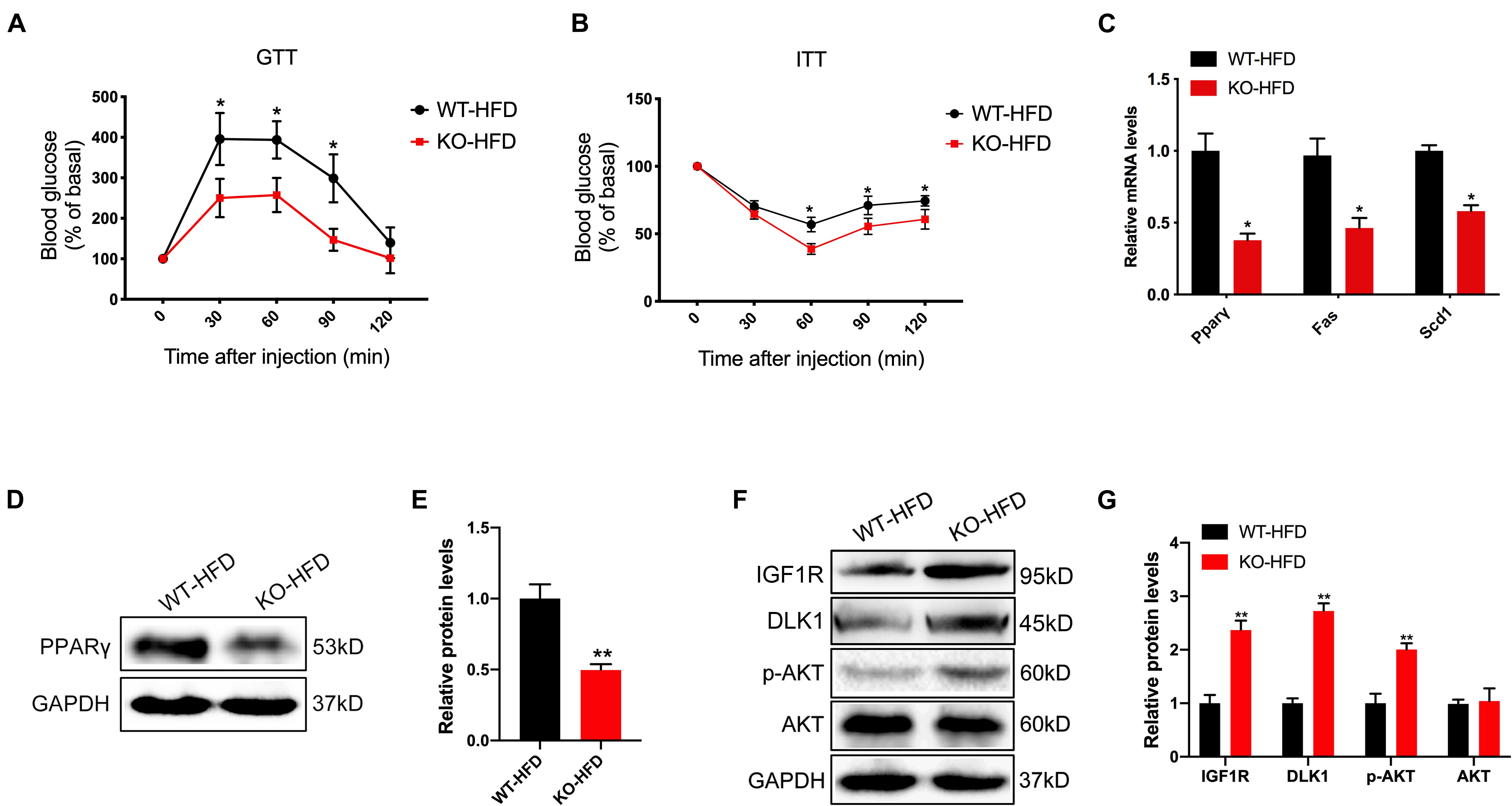
Figure 4. The miR-379/miR-544 cluster KO mice exhibit reduced obesity-associated insulin resistance after feeding with 10 weeks of HFD. (A,B) Blood glucose levels in WT-HFD and KO-HFD mice (n = 4) during a glucose-tolerance test (GTT) (A) and insulin-tolerance test (ITT) (B). *P < 0.05. (C) RT-qPCR analyses of lipogenic genes (Pparγ, Fas, and Scd1) expression in livers from WT-HFD and KO-HFD mice (n = 3). *P < 0.05. (D,E) The protein level of PPARγ in livers from WT-HFD and KO-HFD mice (n = 3) was determined (D) and quantified (E) by Western blot analysis. **P < 0.01. (F,G) Western blot analysis (F) and quantitation (G) of IGF1R, DLK1, phosphorylated AKT (p-AKT), and total AKT in the livers from WT-HFD and KO-HFD mice (n = 3). **P < 0.01.
Given that miR-379 could suppress the IGF1/IGF1R and PI3K/AKT signaling pathway in hepatocellular carcinoma (Chen et al., 2016; Huang et al., 2016), and DLK1 is the potential target gene of miR-329 that may be involved in IGF1 signaling and muscle growth through Akt phosphorylation (Glass, 2005; Nueda et al., 2008). We next measured the protein level of IGFIR, DLK1, and AKT phosphorylation in WT-HFD and the KO-HFD mouse livers. As expected, the expression levels of IGF1R, DLK1, and AKT phosphorylation (Ser 473) in KO-HFD mouse livers were significantly higher than those in WT-HFD mouse livers (Figures 4F,G). Together, these findings strongly indicate that the activation of IGF1/IGF1R and PI3K/AKT signaling pathway contributed to the altered glucose and lipid homeostasis in the miR-379/miR-544 cluster KO mice fed on HFD.
Suppression of miR-379 Expression Ameliorates Palmitic Acid-Induced Lipid Accumulation in HepG2 Cells
To further determine the underlying molecular mechanism of the miR-379/miR-544 cluster in regulating liver steatosis, we utilized the HepG2 cell line as a model to confirm the in vivo findings. First, we treated HepG2 cells with 300 μM palmitic acid (PA), a toxic lipid, for 24 h and found that the expression level of miR-379 increased three-folds (Figure 5A). Then, we added miR-379 inhibitor for 48 h and found that the expression of miR-379 was inhibited in HepG2 cells (Figure 5B), accompanied by reduced lipid accumulation and cellular triglyceride content in the presence of PA (Figures 5C,D). Moreover, the suppression of miR-379 reversed the PA-induced elevation of mRNA expression of Pparγ and Fas (Figure 5E), consistent with the in vivo results from miR-379/miR-544 KO-HFD mouse livers. To further ask whether miR-379 could induce triglyceride deposition in vitro, miR-379 was overexpressed in HepG2 cell line by transfection of a miR-379 mimic (Figure 5F). As revealed by Oil Red O staining, the miR-379 mimic dramatically facilitated lipid accumulation in HepG2 cells (Figure 5G). Similarly, the cellular triglyceride content increased in miR-379 overexpressed HepG2 cells (Figure 5H). This increase in cellular triglycerides was associated with increased expression of Pparγ and Fas (Figure 5I). Together, these in vitro data confirmed the reliability of the results obtained from the miR-379/miR-544 KO-HFD mouse model.
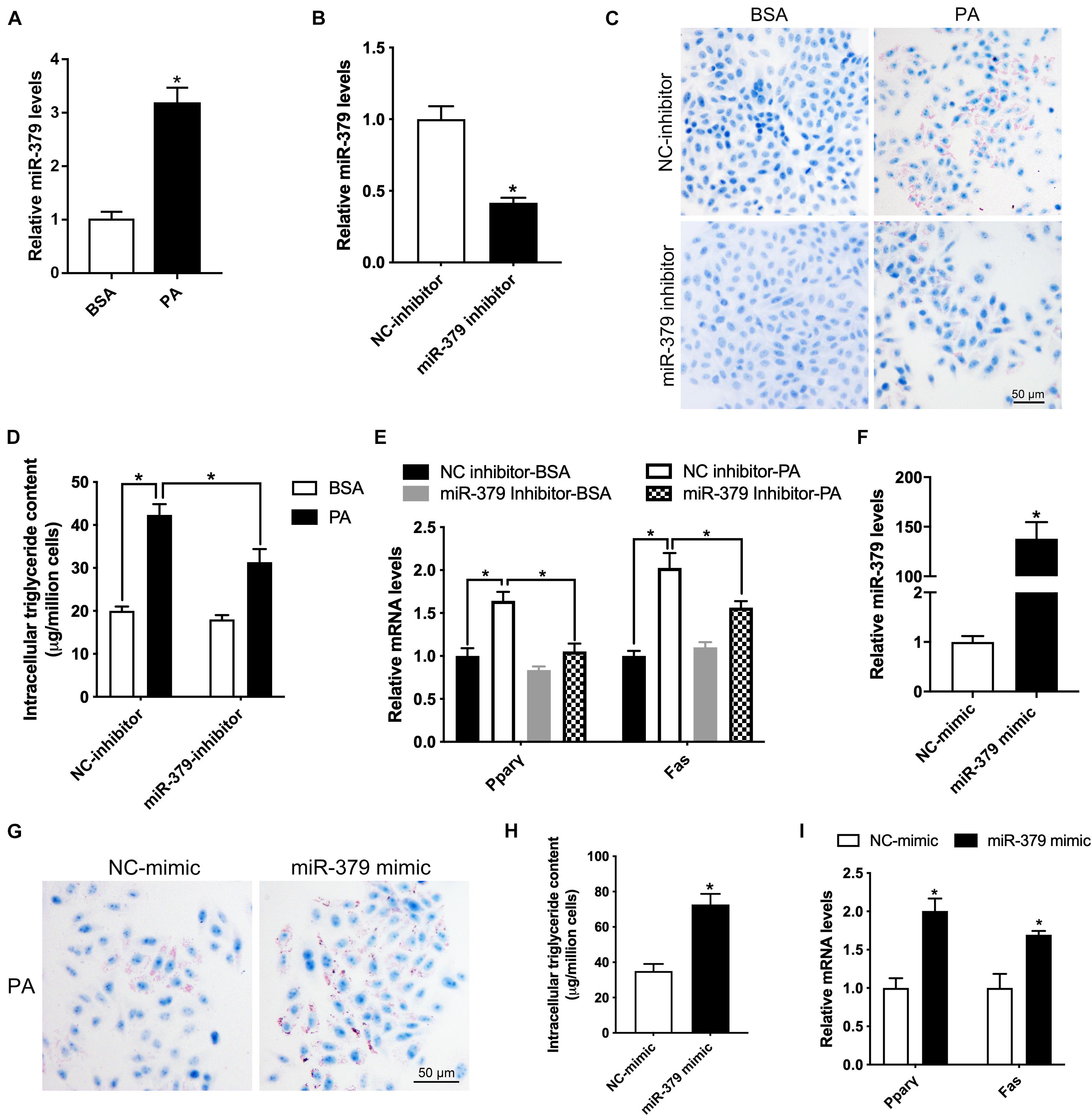
Figure 5. Suppression of miR-379 expression ameliorates palmitic acid-induced lipid accumulation in HepG2 cells. (A) Histogram showing the relative expression of miR-379 in HepG2 cells (n = 4) treated with BSA or 300 μM palmitic acid (PA) for 24 h. *P < 0.05. (B) Histogram showing the relative expression of miR-379 in HepG2 cells (n = 3) transfected with miR-379 inhibitor or negative control (NC) for 48 h. *P < 0.05. (C–E) Oil-red O staining (C), intracellular triglyceride content (D), and RT-qPCR analysis of Pparγ and Fas (E) in HepG2 cells (n = 3) transfected with a miR-379 inhibitor or NC for 48 h in the presence of PA or not. *P < 0.05. Scale bars = 50 μm. (F) HepG2 cells (n = 3) were transfected with miR-379 mimic (overexpression) or related negative control (NC) for 48 h, and RT-qPCR analyzed the expression level of miR-379. (G–I) Oil-red O staining (G), intracellular triglyceride content (H), and RT-qPCR analysis of Pparγ and Fas (I) in HepG2 cells (n = 3) transfected with a miR-379 mimic or NC for 48 h in the presence of palmitic acid. *P < 0.05.
Igf1r and Dlk1 Are Among the Metabolic Targets of the miR-379/miR-544 Cluster
Potential effects of miR-379/miR-544 cluster on glucose and lipid homeostasis promoted us to explore the downstream effectors of these miRNAs. By using the bioinformatics prediction (Lewis et al., 2005), we identified Igf1r, a key component of the critical node in the IGF1/IGF1R signaling pathway, contains a potential miRNA response element (MRE) for miR-379-5p in its 3′-untranslated region (3′-UTR). This MRE site is highly conserved in humans, mice, and rats (Figure 6A). To investigate whether Igf1r could be regulated by miR-379-5p, we generated luciferase reporter constructs encoding Igf1r wild-type and mutant 3′-UTR sequence, and co-transfected with miR-379-5p mimic or negative control into HepG2 cells. Through luciferase reporter assay, we found that luciferase activity with Igf1r 3′-UTR was significantly inhibited by miR-379-5p mimic, whereas its activity with the Igf1r mutated 3′-UTR did not alter significantly (Figure 6B). Moreover, an increase in IGF1R expression occurred in HepG2 cells incubated with a miR-379 inhibitor (Figures 6C,D), suggesting miR-379 could inhibit the protein level of endogenous IGF1R in HepG2 cells. Likewise, we found that another miRNA, miR-329-3p, located in the miR-379/miR-544 cluster, has a putative binding site in the 3′-UTR of Dlk1 (Figure 6E). In addition, like miR-379, the expression levels of hepatic miR-329 in db/db mice and mice fed with HFD were significantly increased compared with that of control mice (Supplementary Figures 3B,C). Luciferase reporter assay revealed that miR-329 could directly bind to Dlk1 3′-UTR, but not to mutant Dlk1 3′-UTR (Figure 6F) and repress the protein level of endogenous DLK1 in HepG2 cells (Figures 6G,H). Taken together, these data indicate that Igf1r and Dlk1 are direct target genes of the miR-379/miR-544 cluster, contributing to regulating the glucose and lipid homeostasis in miR-379/miR-544 KO-HFD mouse models.
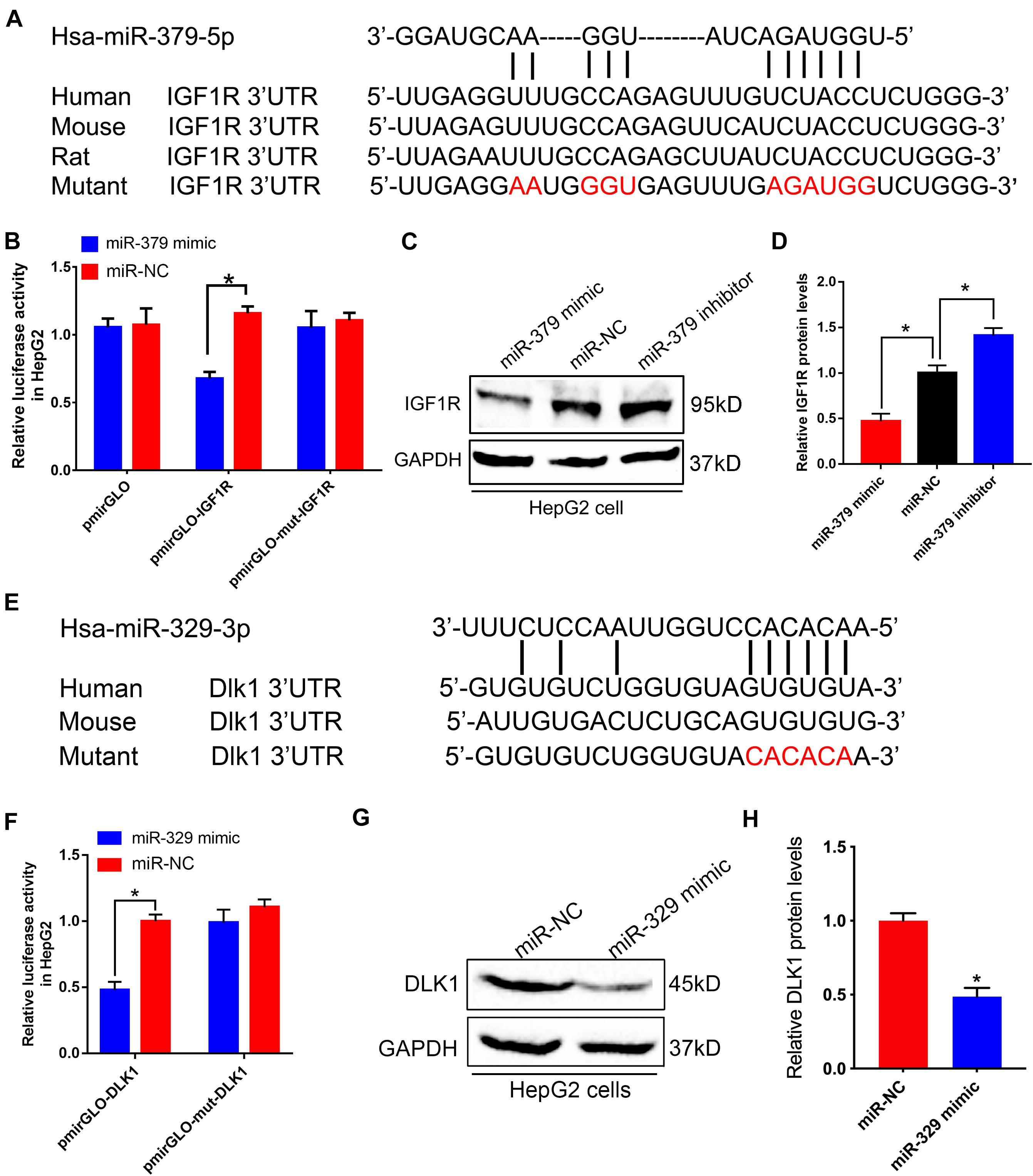
Figure 6. miR-379/miR-544 cluster directly targets Igf1r and Dlk1. (A) Sequence alignment of human miR-379-5p with 3′-UTRs of human, mouse, rat, and mutant Igf1r. (B) Luciferase reporter assay showing wild-type or mutant Igf1r 3′-UTR transfected with miR-379 mimic or negative control in HepG2 cells (n = 3). HepG2 cells transfected with the empty luciferase reporter vector served as control. *P < 0.05. (C,D) Western blot (C) and quantitative analysis (D) of the IGF1R protein compared with GAPDH when HepG2 cells (n = 3) were transfected with a miR-379 mimic or a miR-379 inhibitor. *P < 0.05. (E) Schematic representation of potential miR-329-3p binding sites and the designed mutant sites at Dlk1 3′-UTR is shown. (F) Luciferase reporter assay showing wild-type or mutant 3′-UTR transfected with miR-329 mimic or negative control in HepG2 cells (n = 3). *P < 0.05. (G,H) Western blot (G) and quantitative analysis (H) of the DLK1 protein when HepG2 cells (n = 3) were transfected with a miR-329 mimic. *P < 0.05.
Genetic Ablation of the miR-379/miR-544 Cluster in HFD Mice Causes the Dysregulation of Hepatic Gene Expression
To gain a better understanding of the molecular mechanisms underlying the specific function of miR-379/miR-544 cluster in hepatic steatosis, we next performed detailed gene expression analyses in livers of WT-HFD and KO-HFD mice by RNA-seq. The results revealed that a total of 761 genes are significantly deregulated in KO-HFD mouse livers compared to that of WT-HFD mouse livers (Figure 7A and Supplementary Table 2). Among them, 300 genes were upregulated, whereas 461 were genes downregulated in the KO-HFD mice compared to those in WT-HFD mice (Figure 7A and Supplementary Figure 4A). Further RT-qPCR analyses confirmed that 10 randomly selected mRNA expression levels were consistent with the RNA-seq data (Supplementary Figure 4B). We then adopted an alternative approach by analyzing the miR-379/miR-544 cluster targets predicted by TargetScan (Lewis et al., 2005) and microCosm (Griffiths-Jones et al., 2006) among upregulated genes in RNA-Seq to identify whether the effects on deregulated genes are caused by the primary targets of miR-379/miR-544 cluster. We found very few overlapping genes, which could indicate that either only a few upregulated genes are the real primary targets or that many targets are relevant only in the specific metabolic pathway (Figure 7B). Furthermore, we performed Sylamer analyses to identify the miRNAs responsible for the dysregulated mRNAs (van Dongen et al., 2008). In general, the enriched seed sequences (“words with the highest peak”) were not that of the miR-379/miR-544 cluster (Supplementary Figure 4C). These data implied that the transcriptomic changes observed in the miR-379/miR-544 KO-HFD mice were most likely representing secondary effects.
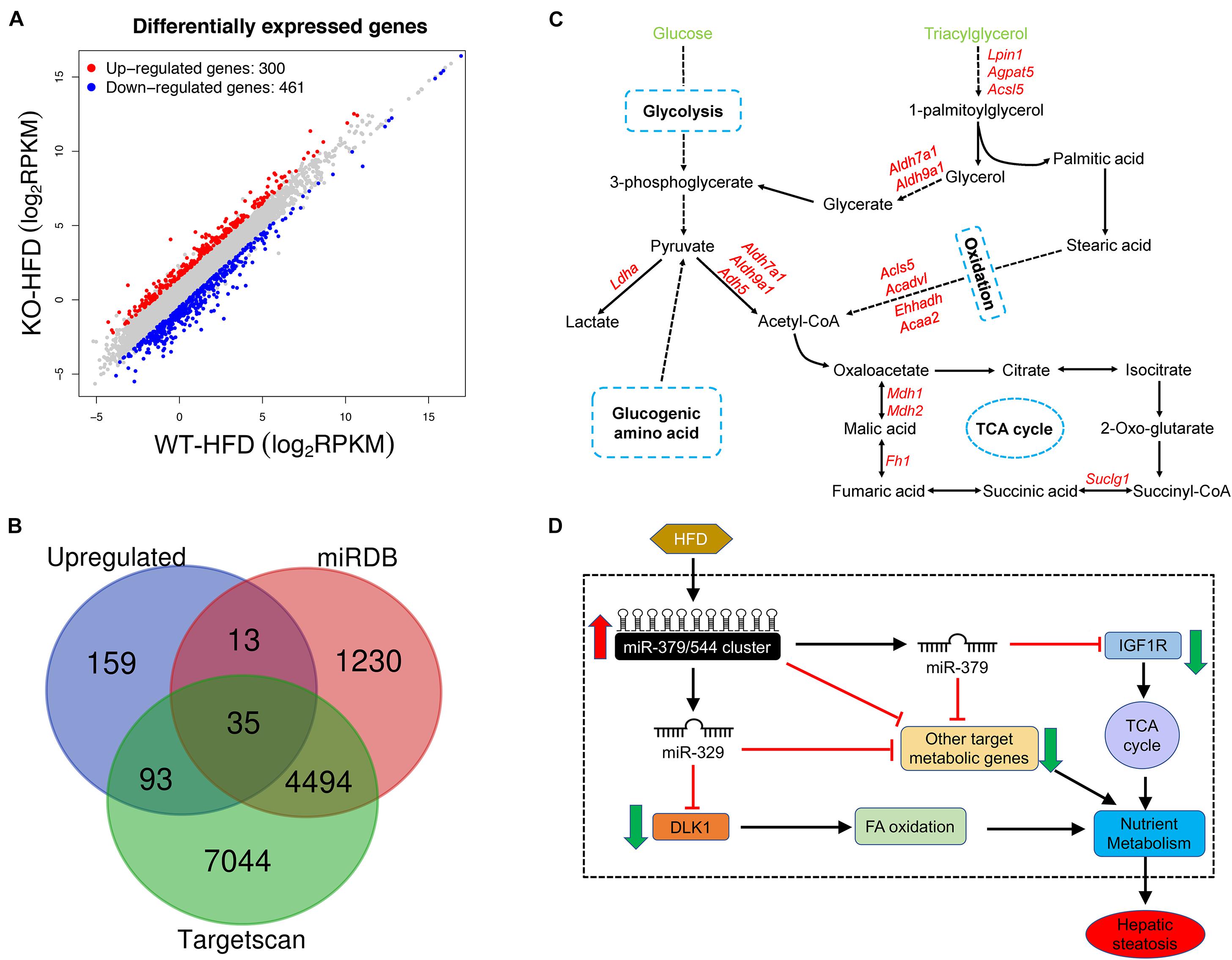
Figure 7. miR-379/miR-544 cluster KO-HFD mice display dysregulation of hepatic gene expression. (A) Volcano plot showing differential expression of 761 significantly altered genes that determined by RNA-seq (n = 3). (B) Venn diagram showing a comparison between significantly upregulated genes and genes predicted to be miR-379/miR-544 cluster targets by Targetscan and microCosm. (C) Schematic representation of the predicted metabolic genes targeted by miR-379/miR-544 cluster on glucose and triacylglycerol metabolism processes. (D) Schematic diagram of the working model of miR-379/miR-544 cluster in liver metabolic regulation. Igf1r, a transmembrane tyrosine kinase receptor, is a target of miR-379 that regulates the TCA cycle. Dlk1 is a target gene of miR-329 and involves in fatty acid oxidative metabolism. The miR-379/miR-544 cluster is proposed to be an integral component of a regulatory circuit that functions under conditions of metabolic stress to control the overall energy homeostasis.
To further examine whether these differentially expressed genes (DEGs) were related to glucose and lipid homeostasis, we tried to perform Gene Ontology (GO) and Kyoto Encyclopedia of Genes and Genomes (KEGG) analyses using our RNA-seq data. Many upregulated DEGs were enriched for genes involved in metabolic processes through the GO biological process analysis, including oxidation-reduction process, lipid metabolic process, etc. (Figure 3C and Supplementary Table 3). Moreover, the GO biological process analysis of downregulated genes was related to cell differentiation and MAPK cascade (Supplementary Figure 4E and Supplementary Table 4). Through the KEGG pathway analysis, these DEGs were correlated with many metabolic pathways such as non-alcoholic fatty liver disease, glycolysis/gluconeogenesis, and citrate cycle (Supplementary Figures 4F,G and Supplementary Tables 5, 6). In addition, we further analyzed miRNAs target genes that were upregulated in the RNA-Seq data and found many targets that participated in the metabolic processes such as glycolysis, oxidation, and tricarboxylic acid cycle (Figure 7C).
Taken together, our unbiased transcriptome-wide studies uncover a miRNA-controlled gene expression program whose sustained perturbation is very likely to underlie the inhibitors in the setting of obesity observed in the miR-379/miR-544 cluster KO mice fed on HFD (Figure 7D).
Discussion
In the current study, we identified a potential function of the miR-379/miR-544 cluster in regulating nutrient metabolism. First, we found that the expression of many miRNAs located in the miR-379/miR-544 cluster are significantly upregulated in the liver of mice fed on HFD as well as db/db mice. Second, our in vivo results suggested that the miR-379/miR-544 cluster knockout mice are protected from HFD-induced obesity and alleviated hepatic steatosis. Third, our in vitro data showed that suppression of miR-379 expression ameliorates palmitic acid-induced lipid accumulation in HepG2 cells, and overexpression of miR-379 promotes lipid accumulation in HepG2 cells. Finally, among the members of this cluster, we identify miR-379 and miR-329 could inhibit the expression of the IGF1R and DLK1 directly via binding with the 3′-UTR of their mRNAs. In addition, the differentially expressed genes between WT and the miR-379/miR-544 cluster KO mice fed on HFD are involved in metabolic processes, including oxidation-reduction process, lipid metabolic process, and cell differentiation. Interestingly, although the miR-379/miR-544 mutants did not exhibit other overt phenotypes except CLPG-like muscular hypertrophy under normal laboratory conditions (Gao et al., 2015; Cao et al., 2018), the miR-379/miR-544 KO-HFD mice displayed resistance to obesity and moderate hepatic steatosis in this study. These consequences broadened the vision of the role of miR-379/miR-544 under HFD conditions, which supports the notion that the function of miRNAs could be enhanced under conditions of stress (Leung and Sharp, 2010).
Accumulated evidence has showed that the abnormal expression of miRNAs in the liver was associated with the pathogenesis of metabolic disease, including fatty liver disease, type 2 diabetes, and hepatocellular carcinoma (Cheung et al., 2008; Li et al., 2009). Among them, mammalian conserved miR-379/miR-410 genomic cluster induced in hepatic tissue has been identified as a critical component of GC/GR-driven metabolic dysfunction. Notably, miR-379 was up-regulated in obesity mouse models in a GC/GR-dependent manner, consistent with our results. Specific silencing of miR-379 in hepatocytes substantially decreased the levels of triglyceride in healthy mice (de Guia et al., 2015). Using a mouse knockout model, one group identified a deletion of the miR-379/miR-410 cluster caused abnormalities in energy homeostasis maintenance, and finally partially penetrant neonatal lethality (Labialle et al., 2014). Based on the previous findings, the current study had proposed for the first time that genetic deletion of miR-379/miR-544 cluster attenuated high fat diet-induced hepatic steatosis and metabolic dysfunction.
Insulin-like growth factor 1 receptor is a tyrosine kinase receptor involved in liver injury and hepatic cell growth (Caro et al., 1988; Scharf et al., 1998) and could regulate (hepatocyte) metabolism indirectly through affecting the development and function of vital metabolic tissues. Moreover, in the liver, IGF1/IGF1R can regulate hormonal inputs via changing extra-hepatocyte function and/or change nutrient flux through altering tissue-specific metabolism of carbohydrate and lipid. These actions are not mutually exclusive, but are used to integrate the functions of all tissues to meet the metabolic needs of the organism (Boucher et al., 2016; Softic et al., 2016; Kineman et al., 2018). Our study showed that upregulation of the IGF1/IGF1R signaling pathway protected the miR-379/miR-544 cluster KO mice against high-fat diet-induced obesity. Luciferase reporter activity assays confirmed that Igf1r was a direct target of miR-379 in hepatocytes by using Igf1r 3-′UTR reporter constructs carrying binding site mutation, in the presence or absence of miR-379. Interestingly, a previous study showed that exogenous uptaken of DLK1 attenuated hepatic steatosis, hyperglycemia and glucose intolerance in the diabetic mice (Charalambous et al., 2014). It was also found that the metabolic effects of DLK1, including augmented fatty acid oxidation and decreased gluconeogenesis in the liver, work via AMPK activation (Lee et al., 2016). In our study, we identified Dlk1 as a direct target of miR-329, another member of miR-379/miR-544 cluster, in hepatocytes in this study. Importantly, similar to the phenotypes of miR-379/miR-544 KO-HFD mice, both the genetic and the positive energy balance dietary model suggested that the reduction of hepatic lipid synthesis and increase in skeletal muscle lipid oxidation enabled the DLK1-overexpressing mice to be protected from steatosis (Charalambous et al., 2014). Moreover, the production of pituitary growth hormone was elevated in DLK1-overexpressing mice due to a local defect in IGF1 feedback (Charalambous et al., 2014). Interestingly, in our study, we found that when the expression DLK1 was increased, the activation of AKT signaling also increased consistently in the miR-379/miR-544 cluster KO-HFD mouse livers. Therefore, these results clearly suggested that the miR-379/miR-544 cluster, which lay within the Dlk1-Dio3 imprinting region, might regulate the expression of Dlk1 negatively.
Importantly, increased expression level of miR-379 was not only found in obesity mouse models of NAFLD and HepG2 cells treated with PA, but also detected in human patients, implying that the level of hepatic miR-379 correlated with both serum cortisol and TG levels in NAFLD patients and exhibits high potential as a biomarker for NAFLD (de Guia et al., 2015; Okamoto et al., 2020). Moreover, our results showed that the binding sites of miR-379 within the Igf1r 3′-UTRs and miR-329 within the Dlk1 3′-UTRs were conserved between humans and mice, which further indicated the potential of miR-379/miR-544 cluster as a therapeutic target for human NAFLD. Furthermore, the miR-379/miR-544 cluster deficiency probably leads to variability in some metabolic genes’ expression and, beyond a certain threshold, to misexpression of some genes by epigenetic regulation, affecting both glucose and lipid metabolism.
In summary, our study illustrated the important role of the miR-379/miR-544 cluster in the regulation of glucose and lipid homeostasis. Disruption of the miR-379/miR-544 cluster dosage might have important consequences for obesity and metabolic disease. Our results revealed a previously unknown function of the miR-379/miR-544 cluster in resistance to high-fat induced obesity and moderate hepatic steatosis, suggesting that the miR-379/miR-544 cluster might be a potential target for the treatment of lipid-related disorders.
Materials and Methods
Mice
The miR-379/miR-544 cluster knockout (KO) mice generated as described previously (Cao et al., 2018) and maintained under a 12-h dark/light cycle in a specific pathogen-free animal facility. Mice were fed a chow diet with 10% kcal fat (Research Diet D12450B) or a high-fat diet with 60% kcal fat-containing diet (Research Diet D12492) for 10 weeks.
Glucose and Insulin Tolerance Tests
Glucose-tolerance tests were carried out on mice that had been fasted overnight for 16 h. After determining fasted blood glucose levels, glucose (2 g/kg of body weight) was intraperitoneally injected into each animal. Blood glucose levels were detected after 30, 60, 90, and 120 min. Insulin-tolerance tests were performed on mice following a 5-hour fast. After determining fasted blood glucose levels, animals were injected intraperitoneally with 0.75 U/kg body weight of insulin. Blood glucose levels were detected after 30, 60, 90, and 120 min.
Measurement of Metabolic Profile
The levels of triglyceride and cholesterol in livers, serum, and cultured cells were determined according to the manufacturer’s instructions (Njjcbio, China). Briefly, 50-100 mg of liver tissue was homogenized in 450-900 ml absolute ethyl alcohol, and centrifuged supernatants were harvested. The extracted contents from the liver, serum, and cells were enzymatically measured with respective kits and were expressed relative to liver weight or serum volume.
Histological Analysis
Liver tissues, epididymal fat pads, and cultured cells were harvested, fixed in methanol-free 4% paraformaldehyde for paraffin embedding. Tissue specimens were sectioned at 5 μm and then were processed for hematoxylin and eosin (H&E) staining. For oil-red O staining, the subset of fixed livers was sequential 10 and 20% sucrose equilibration for 12 h each, and then cryo-embedded in optimal cutting temperature medium following by 20 μm cryosection cutting with oil red O for evaluating the lipid accumulation. Each image of cultured cells, liver, and fat pad sections was obtained using a light microscope.
RNA Isolation and Quantitative PCR Analysis
Total RNA was extracted from mouse tissues or cultured cells using Trizol reagent (Invitrogen), according to the manufacturer’s protocol. The purified RNA samples were then quantified with a fluorimeter and reverse transcribed using the PrimeScript RT Reagent Kit with gDNA Eraser (Takara). Then cDNAs were quantified using ABI Prism Step-One System (Applied Biosystems) with SYBR Green Master Mix reagents (Takara). For miRNA detection, total RNA was reverse-transcribed using a miRNA-specific stem-loop primer and subsequently measured by real-time PCR using the miRNA-specific primer. Relative gene expression levels were calculated using the ΔΔCT method (with U6, miR-16, or Gapdh used as the reference gene) and normalized as indicated. The information of primers for quantitative RT-PCR (qRT-PCR) are all listed in Supplementary Table 7.
Western Blotting Analysis
Protein was extracted from frozen liver tissues and cultured HepG2 cell lines by using RIPA buffer. 30 μg of protein was loaded onto SDS-polyacrylamide gel electrophoresis (PAGE) gel and transferred to PVDF membranes (Bio-Rad). Then the membranes were blocked in 5% non-fat milk (blocking solution) for 1h and incubated with anti-PPARγ polyclonal antibody (1:1000, A0270, ABclonal), anti-AKT antibody (1:1000, no.9272, Cell Signaling Technology), anti–phospho-Akt (Ser 473) antibody (1:1000, no.9271, Cell Signaling Technology), anti-IGF1R polyclonal antibody (1:1000, A0243, ABclonal), anti-DLK1 polyclonal antibody (1:1000, A6578, ABclonal), and anti-GAPDH polyclonal antibody (1:6000, 10494-1-AP, proteinteach) overnight at 4°C. Membranes were washed with TBST three times and incubated with HRP-conjugated goat anti-rabbit IgG (1:5000, 1706515, Bio-Rad) for 1 h, and the immunoreactive bands were visualized with ECL plus Western Detection System (Bio-Rad). Band optical densities were quantified using Image J software (NIH).
Transmission Electron Microscopy (TEM)
Liver tissues were fixed in 2.5% glutaraldehyde in 0.1 M sodium cacodylate buffer (pH 7.4) for 1 h. After being washed three times with 0.1 M phosphate buffer, the tissues were fixed with 1% osmium tetroxide in 0.1 M phosphate buffer for another 1 h. The samples were dehydrated with increasing ethanol concentration, embedded in epoxy resin, and cut to a thickness of 70 nm. Electron photomicrographs were taken of the ultrastructure of the liver tissues with a TEM (Hitachi, Japan), with the imaging parameters of the lens mode set as zoom-1 HC1, an acceleration voltage of 80.0 kV, a spot size of micro 8 and ×24,500 magnification.
Cell Culture, Cell Transfections, and Palmitic Acid (PA) Treatment
The HepG2 cell line was cultured in low-glucose Dulbecco’s modified Eagle’s medium (L-DMEM) (HyClone) supplemented with 10% horse serum (HyClone), 100 units/ml penicillin (HyClone), and 0.1 mg/ml streptomycin (HyClone) at 37°C with humidified air and 5% CO2. miRNA mimics and inhibitors or the negative control (NC) were purchased from GenePharma (Shanghai). Transfection of miRNAs was performed with HiPerFect transfection reagent (Qiagen). 0.25 M palmitic acid (Sigma) were dissolved in 100% ethyl alcohol. Before using, 0.25 M palmitic acid stock and 5% BSA were incubated in a 60°C water bath for 5-10 min. Then, 320 μl palmitic acid stock was dropwise added into 20 ml of 5% BSA to make 4 mM palmitic acid. Before experiments, 4 mM palmitic acid was incubated in a 60°C water bath for 5-10 min, and a mixture of palmitic acid was added at a total concentration of 300 μM.
Luciferase Reporter Assay
The annealed oligonucleotides with the WT Igf1r-3′UTR binding site or the oligonucleotides with mutated miR-379-5p-binding sites were cloned into pmirGLO control luciferase reporter vector (Promega, Madison, WI, United States). And the annealed oligonucleotides with the WT Dlk1-3′UTR binding site or the oligonucleotides with mutated miR-329-3p-binding sites were cloned into pmirGLO control luciferase reporter vector. After being seeded into 96-well plates, HepG2 cells were co-transfected with pmirGLO-Igf1r or pmirGLO-mut-Igf1r plasmids and miR-379 mimic or negative control using lipofectamine 2000 (Invitrogen). Meanwhile, pmirGLO-Dlk1 or pmirGLO-mut-Dlk1 plasmids and miR-329 mimic or negative control were also co-transfected into HepG2 cells. Luciferase activity was detected after 2 days of post-transfection using a dual-luciferase reporter kit (Catalog no. E1910; Promega) in which Firefly luciferase activity was normalized against Renilla luciferase activity according to the manufacturer’s instructions.
RNA-Seq Analysis
Total RNA was extracted from liver tissues of WT-HFD and KO-HFD mice using TRIzol (Invitrogen). The quality of RNA was determined using an Agilent 2100 Bioanalyzer (Agilent RNA 6000 Nano Chip). High-quality total RNA [RNA integrity number (RIN).7] was used as the starting material. All samples were in biological triplicates. RNA-Seq was performed using an Illumina HiSeq ×10 sequencing system according to the manufacturer’s instructions. The DESeq software used negative binomial distribution and a shrinkage estimator for the distribution’s variance to detect differential expression of mRNAs from high-throughput sequencing assay.
Statistical Analysis
All data are expressed as means ± SEMs. Significant differences were assessed either by a two-tailed Student t-test or one-way ANOVA followed by the Student-Newman-Keuls (SNK) test. P < 0.05 was considered statistically significant.
Data Availability Statement
All RNA sequencing data were deposited in the NCBI SRA (Sequence Read Achieve) database with the accession number of PRJNA699696.
Ethics Statement
The animal study was reviewed and approved by the Institutional Animal Care and Use Committee (IACUC) of the Tongji Medical College, Huazhong University of Science and Technology.
Author Contributions
SY and CC conceived and designed the research. CC, PD, WL, YGuo, JZ, and YGui performed all bench experiments and data analyses. CC and PD wrote the manuscript. SY revised the manuscript and supervised the project. All authors read and approved the manuscript.
Funding
This work was supported by grants from the Science Technology and Innovation Commission of Shenzhen Municipality (JCYJ20170244 to SY) and the Natural Science Foundation of Hubei Provincial Department of Education (Q20202105 to PD).
Conflict of Interest
The authors declare that the research was conducted in the absence of any commercial or financial relationships that could be construed as a potential conflict of interest.
Publisher’s Note
All claims expressed in this article are solely those of the authors and do not necessarily represent those of their affiliated organizations, or those of the publisher, the editors and the reviewers. Any product that may be evaluated in this article, or claim that may be made by its manufacturer, is not guaranteed or endorsed by the publisher.
Acknowledgments
The authors would like to thank the Yuan lab members for the discussion in the very initial phase of the project.
Supplementary Material
The Supplementary Material for this article can be found online at: https://www.frontiersin.org/articles/10.3389/fcell.2021.720900/full#supplementary-material
Supplementary Figure 1 | The expression levels of miRNAs located in the miR-379/miR-544 cluster were determined by RT-qPCR in the steatotic liver of db/db mice (A) and HFD-fed mice (B). ∗P < 0.05 and ∗∗P < 0.01. n = 4.
Supplementary Figure 2 | Expression of pri–miR-379/miR-544 cluster transcript in multiple tissues and developing mouse livers. (A) The expression level of the pri–miR-379/miR-544 cluster transcript in multiple tissues from chow-feeding and HFD-feeding mice (n = 4). ∗P < 0.05. (B) Expression pattern of the pri–miR-379/miR-544 cluster transcript in eight different adult mouse tissues (n = 4), assayed via semi-quantitative PCR. (C) Expression pattern of the pri–miR-379/miR-544 cluster transcript in mouse livers (n = 4) at various postnatal days, assayed via semi-quantitative PCR. (D,E) Semi-quantitative RT-PCR (C) and quantitative RT-PCR (D) analyses of the pri–miR-379/miR-544 cluster in livers from WT and KO mice (n = 3) at postnatal day 20 (P20). Gapdh was detected as a control in panels (A–C).
Supplementary Figure 3 | (A) Representative transmission electron microscopy images of WT-HFD and KO-HFD mouse livers are shown. The red arrows indicate lipid droplets. (B,C) The relative expression levels of hepatic miR-329 in db/db mice and mice fed with HFD are shown, respectively.
Supplementary Figure 4 | RNA-seq revealed differential gene expression in KO-HFD mouse livers compared with that of in WT-HFD. (A) Heatmap and hierarchical clustering of 761 significantly altered genes in livers from WT-HFD and KO-HFD mice (n = 3). The blue-to-red colors of the heatmap are linearly mapped to the Z-scores, which range from −2 to 2. (B) The expression levels of 10 selected genes from RNA-seq in WT-HFD and KO-HFD mice livers by RT-qPCR analyses are shown. (C) A sylamer plot of motif enrichment in the 3′-UTRs of the dysregulated mRNAs assayed by RNA-seq. (D) Gene Ontology (GO) biological process analyses of significantly upregulated differential expressed genes (DEGs). (E) GO biological process analyses of significantly downregulated DEGs. (F) Kyoto Encyclopedia of Genes and Genomes (KEGG) pathway analyses of significantly upregulated DEGs. (G) KEGG pathway analyses of significantly downregulated DEGs.
References
Adams, L. A., Lymp, J. F., St Sauver, J., Sanderson, S. O., Lindor, K. D., Feldstein, A., et al. (2005). The natural history of nonalcoholic fatty liver disease: a population-based cohort study. Gastroenterology 129, 113–121. doi: 10.1053/j.gastro.2005.04.014
Ajmal, M. R., Yaccha, M., Malik, M. A., Rabbani, M. U., Ahmad, I., Isalm, N., et al. (2014). Prevalence of nonalcoholic fatty liver disease (NAFLD) in patients of cardiovascular diseases and its association with hs-CRP and TNF-alpha. Indian Heart J. 66, 574–579. doi: 10.1016/j.ihj.2014.08.006
Bokov, A. F., Neha, G., Yuji, I., Sachin, T., Nicolas, M., Defronzo, R. A., et al. (2011). Does Reduced IGF-1R Signaling in Igf1r+/− Mice Alter Aging? PLoS One 6:e26891. doi: 10.1371/journal.pone.0026891
Boucher, J., Softic, S., El Ouaamari, A., Krumpoch, M. T., Kleinridders, A., Kulkarni, R. N., et al. (2016). Differential roles of insulin and IGF-1 receptors in adipose tissue development and function. Diabetes 65, 2201–2213. doi: 10.2337/db16-0212
Cao, C., Wen, Y., Dong, J., Wang, X., Qin, W., Huang, X., et al. (2018). Maternally expressed miR-379/miR-544 cluster is dispensable for testicular development and spermatogenesis in mice. Mol. Reprod. Dev. 85, 175–177. doi: 10.1002/mrd.22957
Caro, J. F., Poulos, J., Ittoop, O., Pories, W. J., Flickinger, E. G., and Sinha, M. K. (1988). Insulin-like growth factor I binding in hepatocytes from human liver, human hepatoma, and normal, regenerating, and fetal rat liver. J. Clin. Invest. 81, 976–981. doi: 10.1172/jci113451
Charalambous, M., Da Rocha, S. T., Radford, E. J., Medina-Gomez, G., Curran, S., Pinnock, S. B., et al. (2014). DLK1/PREF1 regulates nutrient metabolism and protects from steatosis. Proc. Natl. Acad. Sci. U.S.A. 111, 16088–16093. doi: 10.1073/pnas.1406119111
Chen, J. S., Li, H. S., Huang, J. Q., Dong, S. H., Huang, Z. J., Yi, W., et al. (2016). MicroRNA-379-5p inhibits tumor invasion and metastasis by targeting FAK/AKT signaling in hepatocellular carcinoma. Cancer Lett. 375, 73–83. doi: 10.1016/j.canlet.2016.02.043
Cheung, O., Puri, P., Eicken, C., Contos, M. J., Mirshahi, F., Maher, J. W., et al. (2008). Nonalcoholic steatohepatitis is associated with altered hepatic MicroRNA expression. Hepatology 48, 1810–1820. doi: 10.1002/hep.22569
de Guia, R. M., Rose, A. J., Sommerfeld, A., Seibert, O., Strzoda, D., Zota, A., et al. (2015). microRNA-379 couples glucocorticoid hormones to dysfunctional lipid homeostasis. EMBO J. 34, 344–360. doi: 10.15252/embj.201490464
Fernandez, A. M., Kim, J. K., Yakar, S., Dupont, J., Hernandez-Sanchez, C., Castle, A. L., et al. (2001). Functional inactivation of the IGF-I and insulin receptors in skeletal muscle causes type 2 diabetes. Genes Dev. 15, 1926–1934. doi: 10.1101/gad.908001
Gao, Y. Q., Chen, X., Wang, P., Lu, L., Zhao, W., Chen, C., et al. (2015). Regulation of DLK1 by the maternally expressed miR-379/miR-544 cluster may underlie callipyge polar overdominance inheritance. Proc. Natl. Acad. Sci. U.S.A. 112, 13627–13632. doi: 10.1073/pnas.1511448112
Glass, D. J. (2005). Skeletal muscle hypertrophy and atrophy signaling pathways. Int. J. Biochem. Cell Biol. 37, 1974–1984. doi: 10.1016/j.biocel.2005.04.018
Glazov, E. A., Mcwilliam, S., Barris, W. C., and Dalrymple, B. P. (2008). Origin, evolution, and biological role of miRNA cluster in DLK-DIO3 genomic region in placental mammals. Mol. Biol. Evol. 25, 939–948. doi: 10.1093/molbev/msn045
Griffiths-Jones, S., Grocock, R. J., Van Dongen, S., Bateman, A., and Enright, A. J. (2006). miRBase: microRNA sequences, targets and gene nomenclature. Nucleic Acids Res. 34, D140–D144.
Huang, D. J., Huang, J. Z., Yang, J., Li, Y. H., Luo, Y. C., He, H. Y., et al. (2016). Bioinformatic identification of IGF1 as a hub gene in hepatocellular carcinoma (HCC) and in-vitro analysis of the chemosensitizing effect of miR-379 via suppressing the IGF1/IGF1R signaling pathway. Eur. Rev. Med. Pharm. Sci. 20, 5098–5106.
Kineman, R. D., Del Rio-Moreno, M., and Sarmento-Cabral, A. (2018). 40 YEARS of IGF1: Understanding the tissue-specific roles of IGF1/IGF1R in regulating metabolism using the Cre/loxP system. J. Mol. Endocrinol. 61, T187–T198.
Labialle, S., Marty, V., Bortolin-Cavaillé, M. L., Hoareau-Osman, M., Pradère, J. P., Valet, P., et al. (2014). The miR-379/miR-410 cluster at the imprinted Dlk1-Dio3 domain controls neonatal metabolic adaptation. EMBO J. 33, 2216–2230. doi: 10.15252/embj.201387038
Le Roith, D., Kim, H., Fernandez, A. M., and Accili, D. (2002). Inactivation of muscle insulin and IGF-I receptors and insulin responsiveness. Curr. Opin. Clin. Nutr. Metab. Care 5, 371–375. doi: 10.1097/00075197-200207000-00004
Lee, K., Villena, J. A., Moon, Y. S., Kim, K. H., Lee, S., Kang, C., et al. (2003). Inhibition of adipogenesis and development of glucose intolerance by soluble preadipocyte factor-1 (Pref-1). J. Clin. Invest. 111, 453–461. doi: 10.1172/jci15924
Lee, Y. H., Yun, M. R., Kim, H. M., Jeon, B. H., Park, B. C., Lee, B. W., et al. (2016). Exogenous administration of DLK1 ameliorates hepatic steatosis and regulates gluconeogenesis via activation of AMPK. Int. J. Obes. (Lond) 40, 356–365. doi: 10.1038/ijo.2015.173
Leung, A. K., and Sharp, P. A. (2010). MicroRNA functions in stress responses. Mol. Cell 40, 205–215. doi: 10.1016/j.molcel.2010.09.027
Lewis, B. P., Burge, C. B., and Bartel, D. P. (2005). Conserved seed pairing, often flanked by adenosines, indicates that thousands of human genes are microRNA targets. Cell 120, 15–20. doi: 10.1016/j.cell.2004.12.035
Li, S., Chen, X., Zhang, H., Liang, X., Xiang, Y., Yu, C., et al. (2009). Differential expression of microRNAs in mouse liver under aberrant energy metabolic status. J. Lipid. Res. 50, 1756–1765. doi: 10.1194/jlr.m800509-jlr200
Ng, R., Wu, H., Xiao, H., Chen, X., Willenbring, H., Steer, C. J., et al. (2014). Inhibition of microRNA-24 expression in liver prevents hepatic lipid accumulation and hyperlipidemia. Hepatology 60, 554–564. doi: 10.1002/hep.27153
Nueda, M. L., Garcia-Ramirez, J. J., Laborda, J., and Baladron, V. (2008). dlk1 specifically interacts with insulin-like growth factor binding protein 1 to modulate adipogenesis of 3T3-L1 cells. J. Mol. Biol. 379, 428–442. doi: 10.1016/j.jmb.2008.03.070
Okamoto, K., Koda, M., Okamoto, T., Onoyama, T., Miyoshi, K., Kishina, M., et al. (2020). Serum miR-379 expression is related to the development and progression of hypercholesterolemia in non-alcoholic fatty liver disease. PLoS One 15:e0219412. doi: 10.1371/journal.pone.0219412
Postic, C., Dentin, R., and Girard, J. (2004). Role of the liver in the control of carbohydrate and lipid homeostasis. Diabetes Metab. 30, 398–408. doi: 10.1016/s1262-3636(07)70133-7
Ramirez, C. M., Goedeke, L., Rotllan, N., Yoon, J. H., Cirera-Salinas, D., Mattison, J. A., et al. (2013). MicroRNA 33 regulates glucose metabolism. Mol. Cell Biol. 33, 2891–2902. doi: 10.1128/mcb.00016-13
Samuel, V. T., and Shulman, G. I. (2012). Mechanisms for insulin resistance: common threads and missing links. Cell 148, 852–871. doi: 10.1016/j.cell.2012.02.017
Samuel, V. T., Petersen, K. F., and Shulman, G. I. (2010). Lipid-induced insulin resistance: unravelling the mechanism. Lancet 375, 2267–2277. doi: 10.1016/s0140-6736(10)60408-4
Scharf, J. G., Schmidt-Sandte, W., Pahernik, S. A., Ramadori, G., Braulke, T., and Hartmann, H. (1998). Characterization of the insulin-like growth factor axis in a human hepatoma cell line (PLC). Carcinogenesis 19, 2121–2128. doi: 10.1093/carcin/19.12.2121
Schmidt, J. V., Matteson, P. G., Jones, B. K., Guan, X. J., and Tilghman, S. M. (2000). The Dlk1 and Gtl2 genes are linked and reciprocally imprinted. Genes Dev. 14, 1997–2002.
Softic, S., Boucher, J., Solheim, M. H., Fujisaka, S., Haering, M. F., Homan, E. P., et al. (2016). Lipodystrophy due to adipose tissue-specific insulin receptor knockout results in progressive NAFLD. Diabetes 65, 2187–2200. doi: 10.2337/db16-0213
Soh, J., Iqbal, J., Queiroz, J., Fernandez-Hernando, C., and Hussain, M. M. (2013). MicroRNA-30c reduces hyperlipidemia and atherosclerosis in mice by decreasing lipid synthesis and lipoprotein secretion. Nat. Med. 19, 892–900. doi: 10.1038/nm.3200
Takada, S., Tevendale, M., Baker, J., Georgiades, P., Campbell, E., Freeman, T., et al. (2000). Delta-like and gtl2 are reciprocally expressed, differentially methylated linked imprinted genes on mouse chromosome 12. Curr. Biol. 10, 1135–1138. doi: 10.1016/s0960-9822(00)00704-1
van Dongen, S., Abreu-Goodger, C., and Enright, A. J. (2008). Detecting microRNA binding and siRNA off-target effects from expression data. Nat. Methods 5, 1023–1025. doi: 10.1038/nmeth.1267
Villena, J. A., Choi, C. S., Wang, Y., Kim, S., Hwang, Y. J., Kim, Y. B., et al. (2008). Resistance to high-fat diet-induced obesity but exacerbated insulin resistance in mice overexpressing preadipocyte factor-1 (Pref-1): a new model of partial lipodystrophy. Diabetes 57, 3258–3266. doi: 10.2337/db07-1739
Wang, L., Chen, J., Ning, C., Lei, D., and Ren, J. (2018). Endoplasmic reticulum stress related molecular mechanisms in nonalcoholic fatty liver disease (NAFLD). Curr. Drug Targets 19, 1087–1094. doi: 10.2174/1389450118666180516122517
Wen, J., and Friedman, J. R. (2012). miR-122 regulates hepatic lipid metabolism and tumor suppression. J. Clin. Invest. 122, 2773–2776. doi: 10.1172/jci63966
Xiao, F., Yu, J., Liu, B., Guo, Y., Li, K., Deng, J., et al. (2015). A novel function of MicroRNA 130a-3p in hepatic insulin sensitivity and liver steatosis. Sci. Found. China 63, 2631–2642. doi: 10.2337/db13-1689
Younossi, Z. M., Stepanova, M., Afendy, M., Fang, Y., Younossi, Y., Mir, H., et al. (2011). Changes in the prevalence of the most common causes of chronic liver diseases in the united states from 1988 to 2008 – sciencedirect. Clin. Gastroenterol. Hepatol. 9, 524–530. doi: 10.1016/j.cgh.2011.03.020
Zhang, Y., Sowers, J. R., and Ren, J. (2018). Targeting autophagy in obesity: from pathophysiology to management. Nat. Rev. Endocrinol. 14, 356–376. doi: 10.1038/s41574-018-0009-1
Keywords: miR-379/miR-544, obesity, liver, mouse, knockout, high-fat diet
Citation: Cao C, Duan P, Li W, Guo Y, Zhang J, Gui Y and Yuan S (2021) Lack of miR-379/miR-544 Cluster Resists High-Fat Diet-Induced Obesity and Prevents Hepatic Triglyceride Accumulation in Mice. Front. Cell Dev. Biol. 9:720900. doi: 10.3389/fcell.2021.720900
Received: 05 June 2021; Accepted: 10 August 2021;
Published: 30 August 2021.
Edited by:
Joaquim S. L. Vong, The Chinese University of Hong Kong, ChinaReviewed by:
Vivien Chavanelle, Independent Researcher, Paris, FranceWeiping Jia, Shanghai Diabetes Research Institute, Shanghai Sixth People’s Hospital, China
Jun Ren, University of Washington, United States
Copyright © 2021 Cao, Duan, Li, Guo, Zhang, Gui and Yuan. This is an open-access article distributed under the terms of the Creative Commons Attribution License (CC BY). The use, distribution or reproduction in other forums is permitted, provided the original author(s) and the copyright owner(s) are credited and that the original publication in this journal is cited, in accordance with accepted academic practice. No use, distribution or reproduction is permitted which does not comply with these terms.
*Correspondence: Shuiqiao Yuan, c2h1aXFpYW95dWFuQGh1c3QuZWR1LmNu