- 1Institut de Cancérologie Strasbourg Europe (ICANS), Strasbourg, France
- 2Centre de Recherche en Biomédecine de Strasbourg (CRBS), Fédération de Médecine Translationnelle, UR 3072, Université de Strasbourg, Strasbourg, France
- 3Faculté des Sciences du Sport, Centre Européen d’Enseignement de Recherche et d’Innovation en Physiologie de l’Exercice (CEERIPE), Université de Strasbourg, Strasbourg, France
Breast cancer represents the most commonly diagnosed cancer while neoadjuvant and adjuvant chemotherapies are extensively used in order to reduce tumor development and improve disease-free survival. However, chemotherapy also leads to severe off-target side-effects resulting, together with the tumor itself, in major skeletal muscle deconditioning. This review first focuses on recent advances in both macroscopic changes and cellular mechanisms implicated in skeletal muscle deconditioning of breast cancer patients, particularly as a consequence of the chemotherapy treatment. To date, only six clinical studies used muscle biopsies in breast cancer patients and highlighted several important aspects of muscle deconditioning such as a decrease in muscle fibers cross-sectional area, a dysregulation of protein turnover balance and mitochondrial alterations. However, in comparison with the knowledge accumulated through decades of intensive research with many different animal and human models of muscle atrophy, more studies are necessary to obtain a comprehensive understanding of the cellular processes implicated in breast cancer-mediated muscle deconditioning. This understanding is indeed essential to ultimately lead to the implementation of efficient preventive strategies such as exercise, nutrition or pharmacological treatments. We therefore also discuss potential mechanisms implicated in muscle deconditioning by drawing a parallel with other cancer cachexia models of muscle wasting, both at the pre-clinical and clinical levels.
Introduction
Cancer represents the leading cause of death worldwide and a substantial barrier to increasing life expectancy. Among the different cancer sites, breast cancer is the most commonly diagnosed cancer, with 11.7% of total cases and 6.9% of cancer deaths (Sung et al., 2021). Effective therapy of breast cancer requires a multidisciplinary approach including surgery, radiotherapy, neoadjuvant and/or adjuvant therapies. Currently, neoadjuvant and adjuvant chemotherapies are extensively used in breast cancer patients to reduce tumor development and improve disease-free survival, but also leads to severe off-target side-effects (Maughan et al., 2010; Redden and Fuhrman, 2013; Fisusi and Akala, 2019; Schirrmacher, 2019). Among these treatment-related side effects, both pre-clinical and clinical studies highlighted that chemotherapeutic agents result in major skeletal muscle deconditioning and, together with exacerbated fatigue, are part of a vicious cycle which negatively impacts their quality of life (Berger et al., 2015; Caan et al., 2018; Aleixo et al., 2019; Cespedes Feliciano et al., 2019; Hiensch et al., 2019; Mallard et al., 2020). Although breast cancer represents the most deadly female cancer, 5-year survival rate is over 90% (National Cancer Institute, Surveillance, Epidemiology, and End Result program, 2019) emphasizing the critical need to fight long-lasting effects observed in survivors such as skeletal muscle deconditioning.
Skeletal muscle deconditioning is a direct consequence of global muscle homeostasis perturbation, leading to both structural and functional alterations that will translate into a decrease in muscle mass and/or force as well as an increase in fatigability (Chopard et al., 2009; Baldwin et al., 2013; Brioche et al., 2016; Cruz-Jentoft et al., 2019; Arc-Chagnaud et al., 2020). In the context of cancer patients, skeletal muscle atrophy represents a major characteristic of cachexia, which can be defined as an ongoing loss of skeletal muscle mass that cannot be fully reversed with nutrition and leading to functional alterations (Fearon et al., 2011). It is now well admitted that cancer cachexia is one of the most life-threatening aspects of cancer. Indeed, it has been shown that cachexia substantially increases sedentary behavior, functional impairment, loss of autonomy, quality of life degradation, surgical risks and overall adverse effects of chemotherapy (Fouladiun et al., 2007; Fearon et al., 2011; Roberts et al., 2013; Wallengren et al., 2013; Mason et al., 2016; Rutten et al., 2016; Schwarz et al., 2017; Baracos et al., 2018; Daly et al., 2018). Importantly, cachexia is also strongly correlated with a decrease in cancer patients survival and is actually the leading cause of death in cancer (Warren, 1932; Martin et al., 2015; Deluche et al., 2018; Huh et al., 2020). Thus, the management of skeletal muscle deconditioning during cancer and its treatment represents a major challenge for healthcare, particularly in breast cancer patients, considering both the high incidence of new cases (Sung et al., 2021) and the prevalence of cancer cachexia (∼25%) in breast cancer patients (Baracos et al., 2018). Even if, compared to other cancers, breast cancer does not display the highest prevalence of cachexia, it is important to note that cachexia diagnosis is based on global weight loss (Fearon et al., 2011), and not only muscle mass loss, which likely led to an underestimation of cachexia prevalence in clinical practice (Roeland et al., 2017).
To date, the cellular mechanisms of skeletal muscle deconditioning are of great importance and have been extensively reviewed in healthy people, elderly as well as in relation with many chronic diseases (Sandri, 2008; Chopard et al., 2009; Bodine, 2013; Bonaldo and Sandri, 2013; Schiaffino et al., 2013; Argilés et al., 2014; Bowen et al., 2015; Brioche et al., 2016; Petruzzelli and Wagner, 2016; Baracos et al., 2018; Larsson et al., 2019; Dolly et al., 2020; Silva et al., 2020; Vainshtein and Sandri, 2020; Sartori et al., 2021). However, in comparison with the knowledge accumulated through decades of intensive research with many different animal and human models, a comprehensive understanding of the cellular processes implicated in breast cancer-mediated muscle deconditioning is still needed in order to develop efficient strategies to counteract it.
This review focuses on recent advances in both macroscopic changes and cellular mechanisms implicated in skeletal muscle deconditioning of breast cancer patients, specifically as a consequence of chemotherapy treatment. This review also aims to highlight other potential mechanisms by drawing a parallel with cancer cachexia models of muscle wasting, both at the pre-clinical and clinical levels.
Chemotherapy-Induced Skeletal Muscle Macroscopic Alterations in Breast Cancer Patients
Two families of chemotherapeutic agents are commonly used in clinical practice for breast cancer patients: anthracyclines (i.e., doxorubicin or epirubicin) leading to DNA damage, and taxanes (i.e., docetaxel or paclitaxel) acting as cytoskeletal disruptors (Shah and Gradishar, 2018; Willson et al., 2019). Importantly, non-hormone-dependent (i.e., triple-negative or HER2-positive) breast cancer treatment also includes immunotherapy, a promising new field in breast cancer therapy (Emens, 2018; Keenan and Tolaney, 2020). If immunotherapy has been identified to induce severe cardiotoxicity (Behr et al., 2001; Rochette et al., 2015; Bregni et al., 2016; Varricchi et al., 2018), there is no study to date with a focus on skeletal muscle. On the other hand, chemotherapeutic agents are recognized to contribute to skeletal muscle deconditioning, resulting in an altered quality of life, increased treatment-related toxicity, and to an increased mortality risk (Rier et al., 2016; Shachar et al., 2017; Deluche et al., 2018; Trestini et al., 2018; Cespedes Feliciano et al., 2019; Huh et al., 2020). To date, several skeletal muscle structural and functional alterations were identified (loss of muscle mass and force, altered quality) with severe consequences on exercise tolerance.
Muscle Mass
Although it is widely accepted that chemotherapy induces skeletal muscle loss in breast cancer patients, very few studies clearly demonstrated it. Indeed, by excluding all non-longitudinal studies (i.e., with no pre vs. post-chemotherapy assessments) and lean body mass measurements (i.e., with no assessment of muscle mass in isolation), only two studies emerged (Rossi et al., 2020; Wiederin et al., 2020). Both studies demonstrated a decrease in pectoralis muscle area after chemotherapy. Wiederin et al. (2020) found a 10% reduction in muscle mass using magnetic resonance imaging in a cohort of breast cancer (N = 221), sarcoma (N = 115) and lymphoma (N = 216) female patients. In breast cancer only, Rossi et al. (2020) found a 15% reduction in muscle mass by using CT Scan. Surprisingly, we were unable to find any other longitudinal study on whole-body or locomotor muscle mass for breast-cancer patients undergoing chemotherapy. As a loss of skeletal muscle mass is strongly associated with poor functional outcomes (Fearon et al., 2011; Baracos et al., 2018; Cruz-Jentoft et al., 2019; Aleixo et al., 2020a) and chemotherapy efficacy (Caan et al., 2018; Lee et al., 2021) in breast cancer patients, further studies are needed to better characterize the loss of muscle mass in order to counteract it effectively thereafter.
Muscle Force
On the other hand, the impact of chemotherapy treatment on muscle force is more documented. Numerous studies, with various protocols of force evaluation (handgrip, isometric knee extension, mid-thigh pull, and shoulder strength, etc.), found inconsistent results on chemotherapy-treated breast cancer patients. Indeed, some longitudinal studies (Schmidt et al., 2015; Ramos da Silva et al., 2021) documented no change in isometric muscle force in both lower limbs (quadriceps femoris muscle) and upper limbs (latissimus dorsi, pectoralis, and handgrip muscles), while others found a significant reduction from −4 to −17% in handgrip or knee extensors muscle force (van Waart et al., 2015; Gadéa et al., 2018; Mijwel et al., 2018a; CeŠeiko et al., 2020; Toth et al., 2020). Discrepancies in study protocols (study duration, measurements timepoints, and treatments administered) and in the methods of force evaluation (isometric vs. isokinetic contractions, different muscle groups investigated) may explain these contrasting results. Other studies also highlighted a decrease in muscle force of breast cancer patients undergoing chemotherapy in comparison with healthy women (Klassen et al., 2017; Marques et al., 2020), supporting the fact that chemotherapeutic agents may affect skeletal muscle force production.
Muscle Quality
There is a growing body of evidence that the loss of muscle strength and power mostly exceeds the loss of muscle mass observed in many diseases or inactivity experiments, emphasizing that a deterioration in muscle quality could explain the loss in force and lead to functional impairments (di Prampero and Narici, 2003; Brioche et al., 2016; Pagano et al., 2018; CeŠeiko et al., 2020; Toth et al., 2020). Muscle quality can be assessed through different techniques, including magnetic resonance imaging, computed tomography or ultrasound echography (Karampinos et al., 2012; Addison et al., 2014; Aubrey et al., 2014; Khan et al., 2019; Stock and Thompson, 2021), that allows the detection and quantification of abnormalities in skeletal muscle composition. Among these abnormalities, intermuscular adipose tissue (IMAT) accumulation is particularly of interest. Indeed, these muscle fatty infiltrations (i.e., adipocytes located between muscle fibers and muscle groups), also referred as myosteatosis, are known to be associated with inactivity (Manini et al., 2007; Leskinen et al., 2009; Tuttle et al., 2011; Pagano et al., 2018), pathologies (Gorgey and Dudley, 2007; Wren et al., 2008; Karampinos et al., 2012; Gallagher et al., 2014; Uezumi et al., 2014b) and have been particularly investigated in sarcopenia (Goodpaster et al., 2000, 2001; Song et al., 2004; Marcus et al., 2010; Brioche et al., 2016). An accumulation of IMAT is closely linked to poor muscle quality and therefore muscle dysfunction (Jubrias et al., 1997; Visser et al., 2002, 2005; Delmonico et al., 2009; Marcus et al., 2010; Murphy et al., 2011; Tuttle et al., 2011; Beavers et al., 2013). In the specific context of cachexia, a reduction in muscle quality has been observed in breast cancer patients treated with chemotherapeutic agents. In a longitudinal study, metastatic breast cancer patients showed an altered muscle attenuation after taxane-based chemotherapy, indicating a decrease in muscle quality (Rier et al., 2018). In a cross-sectional study, breast cancer survivors who received anthracyclines were compared to control subjects and a clear increase in thigh IMAT content (∼30%) have been found and was interestingly correlated with an impaired cardiorespiratory fitness (Beaudry et al., 2020). Another cross sectional study highlighted an increased IMAT content in cancer patients (including breast-cancer patients) when compared to non-cancer individuals (Reding et al., 2019) and also showed a good correlation with the development of exercise intolerance.
Exercise Tolerance
As a consequence of the abovementioned skeletal muscle alterations, combined with a well-known cardiotoxicity (Bird and Swain, 2008; Kazemi-Bajestani et al., 2014; Nicolazzi et al., 2018; Varricchi et al., 2018; Jerusalem et al., 2019), chemotherapy is strongly impacting exercise tolerance. In clinical setting, the six-minute walk test (6MWT) represents a reference test reflecting exercise tolerance and is widely used in various pathologic populations (Enright, 2003; Agarwala and Salzman, 2020), including cancer patients (Galiano-Castillo et al., 2016; Wesolowski et al., 2020). A recent systematic-review reported, through the analysis of 21 original studies using the 6MWT, that 1,084 breast cancer patients (including both patients under treatment and survivors) showed a 24% reduction in performance compared to 878 healthy people (But-Hadzic et al., 2021). Aside the 6MWT, widely used as an indirect measurement of cardiorespiratory fitness, the assessment of the maximal oxygen consumption (V̇O2max) represents the gold standard measurement of exercise tolerance (Astrand and Saltin, 1961; Schumacher et al., 2019). Interestingly, consistent results between the 6MWT and V̇O2max were found in breast cancer patients. Indeed, another systematic review reported, from the analysis of 27 clinical trials, a significant 25% reduction in V̇O2max after chemotherapy treatment compared to healthy sedentary women (Peel et al., 2014). This cardiorespiratory deconditioning seems to strengthen the development of cancer-related fatigue and particularly physical fatigue (Neil et al., 2013), with consequences on exercise intolerance. Indeed, physical fatigue, assessed by the reduction in force during the repetition of maximal voluntary contractions, has been found to be exacerbated in breast cancer patients undergoing chemotherapy treatment compared to healthy individuals (Klassen et al., 2017), negatively impacting their exercise tolerance. Together with the decrease of skeletal muscle mass, a reduction in exercise capacity is also strongly associated with higher risk of adverse outcomes such as treatment-induced toxicity, mortality or functional impairment (Jones et al., 2012; Peel et al., 2014; Foulkes et al., 2019; Yu et al., 2020).
Cellular Mechanisms of Skeletal Muscle Deconditioning in Breast Cancer Patients: What Do We Know?
Skeletal muscle biopsy (e.g., using Bergström needle) is the only technique allowing full investigation of the cellular mechanisms of muscle deconditioning (Bergstrom, 1975; Tarnopolsky et al., 2011). To date, only six clinical studies, published in seven different publications, used muscle biopsies in early breast cancer patients (stage I–III) to decipher mechanisms of muscle deconditioning (Lønbro et al., 2017; Bohlen et al., 2018; Guigni et al., 2018; Mijwel et al., 2018b; Møller et al., 2019; Toth et al., 2020; Wilson et al., 2020). Altogether, these studies highlighted several important aspects of muscle deconditioning detailed below and outlined in Figure 1.
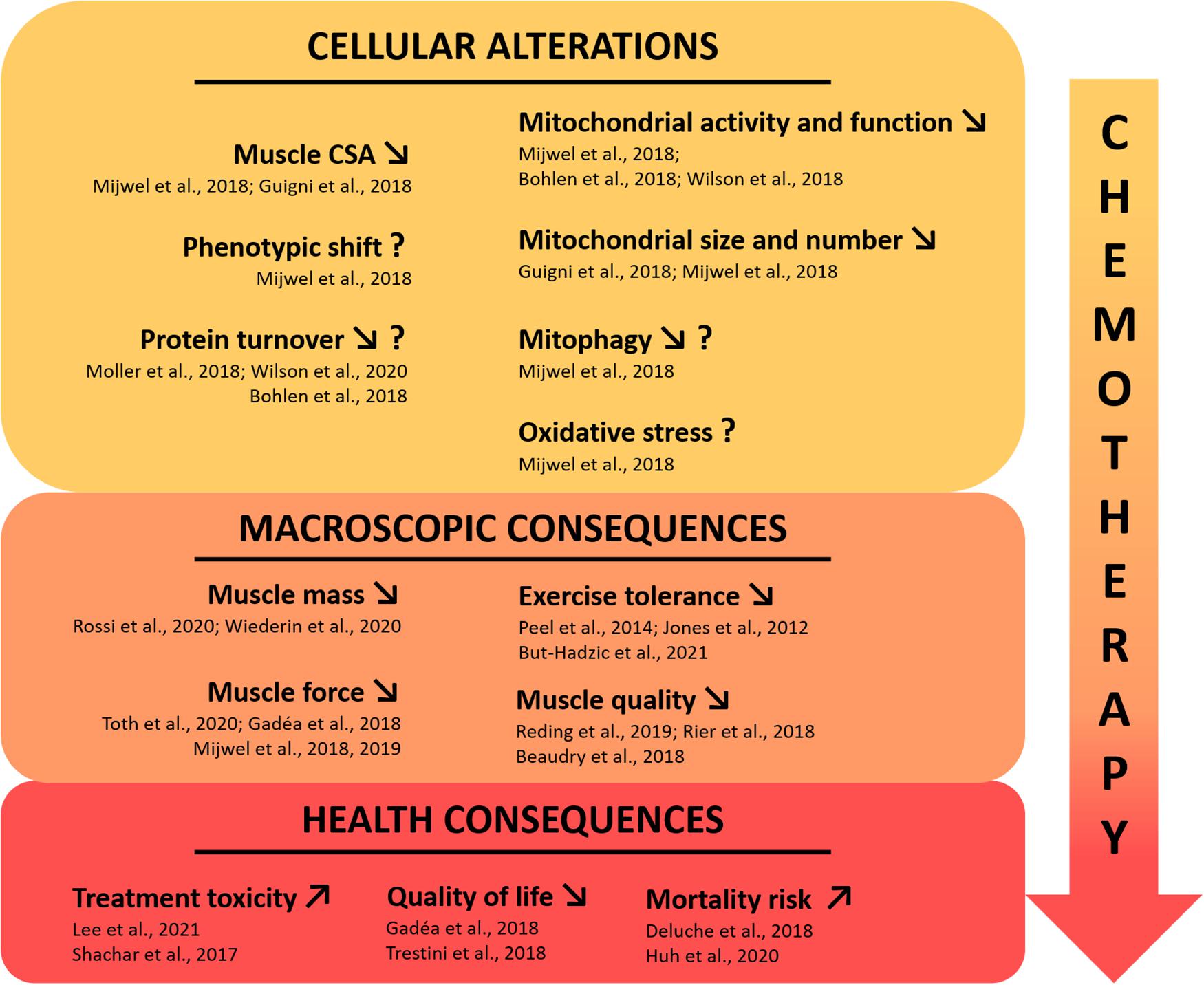
Figure 1. Current state of knowledge on skeletal muscle deconditioning in breast cancer patients. The use of chemotherapeutic agents in clinical breast cancer treatment is directly affecting skeletal muscle tissue, leading to major muscle deconditioning. To date, few clinical studies investigated the underlying cellular mechanisms that may be responsible for the macroscopic changes highlighted in breast cancer patients, affecting muscle function and having severe health consequences. The question mark indicates inconsistent results or a mechanism that needs to be consolidated with more studies. CSA, cross sectional area.
Decrease in Skeletal Muscle Fibers Cross-Sectional Area and Phenotypic Shift
Muscle fibers CSA is one of the most substantial measurement of muscle deconditioning at the cellular level. Recently, Mijwel et al. (2018b) and Guigni et al. (2018) showed a clear decrease in both type I and type II vastus lateralis muscle fibers CSA after anthracycline-cyclophosphamide and taxane-based chemotherapy treatment. Interestingly, Mijwel et al. (2018b) demonstrated it through a longitudinal study while Guigni et al. (2018) have done it with a cross-sectional study design, by comparing breast cancer patients to healthy individuals. To highlight the substantial magnitude of the decrease in overall muscle fibers CSA during chemotherapy in breast cancer patients, it should be noted that this decrease was comparable to the considerable effect of 60 years of healthy aging (Lexell et al., 1988). If comparing muscle CSA of breast cancer patients under chemotherapy for 4 months with 60 years of aging is insightful to emphasize the profound impact of chemotherapy on the skeletal muscle apparatus, it is not fully accurate as other mechanisms are involved and interact with CSA differently in cancer vs. aging. Two other longitudinal studies found no reduction in muscle fibers vastus lateralis CSA during chemotherapy including taxanes, cyclophosphamide, doxorubicin, and carboplatin (Lønbro et al., 2017; Toth et al., 2020). However, in these studies, the second muscle biopsy was performed after ∼5 weeks and might explain the lack of atrophy as the effects of chemotherapeutic agents on skeletal muscle are strongly suggested to be cumulative. It is important to note that in vitro and in vivo studies also demonstrated the negative impact of both chemotherapeutic agents (McLoon et al., 1998; Gouspillou et al., 2015; Min et al., 2015; Barreto et al., 2016; Guigni et al., 2018) and breast cancer-bearing mice models (Hesse et al., 2019; Wang et al., 2021) on skeletal muscle structure, strengthening the results obtained in clinical studies.
Concerning fiber type distribution, only Mijwel et al. (2018b) reported significant changes, with a reduced proportion of type I muscle fibers after chemotherapy treatment. This potential type I to type II phenotypic shift is classically found in muscle disuse models (Baldwin et al., 2013) while the opposite is observed with aging (Larsson et al., 2019). This suggests that muscle deconditioning in breast cancer patients might also be driven by a decrease in overall physical activity during their treatment (De Groef et al., 2018; Gadéa et al., 2018; Yildiz Kabak et al., 2020), a well-known trigger of protein turnover dysregulation.
Protein Turnover
If a large number of excellent reviews have already documented the critical role of protein turnover homeostasis in the mechanisms related to skeletal muscle atrophy (Sandri, 2008; Chopard et al., 2009; Bodine, 2013; Bonaldo and Sandri, 2013; Schiaffino et al., 2013; Brioche et al., 2016; Larsson et al., 2019; Vainshtein and Sandri, 2020; Sartori et al., 2021) including in cancer cachexia (Argilés et al., 2014; Bowen et al., 2015; Petruzzelli and Wagner, 2016; Baracos et al., 2018; Dolly et al., 2020; Silva et al., 2020), little is known in the unique context of breast cancer. Indeed, only four studies investigated the mechanisms related to protein turnover homeostasis in breast cancer patients (Bohlen et al., 2018; Mijwel et al., 2018b; Møller et al., 2019; Wilson et al., 2020). Two publications from the same research team showed, through RNAseq analysis on pectoralis muscle, an increased expression of genes related to ubiquitin-mediated proteolysis and a decreased expression of genes related to ribosomes (Bohlen et al., 2018; Wilson et al., 2020). These results potentially indicate an altered protein turnover balance, with a reduced protein synthesis and an increased protein breakdown. Mijwel et al. (2018b) did not find any changes in MuRF1 protein expression (a key E3 ligase implicated in the ubiquitin-proteasome system) after chemotherapy in breast cancer patients, nor concerning the autophagy pathway, with no changes in the protein expression of different key markers implicated in this pathway (i.e., p-Ulk1, LC3B-II/I ratio, beclin-1, all reflecting autophagosome formation). These results could be explained by the “late” time-point of biopsy collection in this study as cellular processes triggering muscle atrophy, particularly those related to protein breakdown, tend to go back to “normal” expression profiles when the muscle atrophy is well established (Ferreira et al., 2008; Hanson et al., 2013; Atherton et al., 2016; Kawanishi et al., 2018). Finally, the study conducted by Møller et al. (2019) also investigated proteins involved in signaling pathways implicated in protein turnover from vastus lateralis muscle. Very surprisingly, they found a decreased protein expression of the E3 Ligases MAFbx and MuRF1 as well as an increase in p62 and phosphorylated-Ulk1 expression (Ser757), suggesting a decreased activity of the ubiquitin proteasome and autophagy systems, respectively. However, it is important to highlight that 9 out of 10 patients included in this study performed the baseline biopsy after at least one cycle of chemotherapy with epirubicin and doxorubicin (Lønbro et al., 2017). Given the aggressiveness of chemotherapy treatments, this is a serious methodological bias that likely altered “baseline” measures, and therefore, conclusions. Another limitation lays in the heterogeneous population of cancer patients investigated (i.e., seven patients with breast-cancer, one patient with head and neck cancer, one patient with rectal cancer, and one patient with sarcoma). To sum up, there are strong discrepancies between studies that investigated pathways of protein synthesis and breakdown in breast cancer patients undergoing chemotherapy. Further studies are needed as the understanding of these processes is critical to counteract the skeletal muscle atrophy outlined above.
Mitochondrial Alterations
Mitochondrial alterations represent, to date, one of the most investigated aspect of muscle deconditioning in breast cancer, especially in response to chemotherapeutic agents. In clinical studies, the RNAseq analysis used by both Bohlen et al. (2018) and Wilson et al. (2020) showed a clear dysregulation of genes implicated in mitochondrial function and oxidative phosphorylation. Interestingly, the authors showed a decrease in multiple genes implicated in the electron transport chain, antioxidant capacity, and altered PPAR signaling (including PGC-1α), emphasizing that mitochondria and overall energy homeostasis may be perturbed in breast cancer patients treated with chemotherapeutic agents. Guigni et al. (2018) confirmed a clear decrease in mitochondrial content and size for breast cancer patients compared to healthy matched controls, in both the intermyofibrillar and subsarcolemmal compartments. The authors concluded that these alterations, due to the mitotoxic effects of antineoplastic drugs, may constitute a possible explanation to the high prevalence of exercise intolerance and fatigue in all cancer’s types, including those not typically prone to cachexia such as breast cancer patients. Finally, the longitudinal study of Mijwel et al. (2018b) highlighted a decrease in citrate synthase activity with chemotherapy. The decrease in citrate synthase activity, a marker for mitochondrial quantity (Larsen et al., 2012), is in line with the results of Guigni et al. (2018) and confirms the likely lower mitochondria quantity in breast cancer patients. This study also reports a decreased protein expression of PINK1, an essential protein implicated in the final stages of mitophagy, therefore suggesting a lower mitophagy process in breast cancer patients. In addition, no variation in protein levels of Parkin has been detected in this study, nor those of the autophagy pathway, clearly indicating that mitophagy is not upregulated and that future studies should investigate this mitochondrial quality control pathway. Finally, an increased protein expression of SOD2, an essential antioxidant enzyme and redox signaling trigger through H2O2 production (Zou et al., 2017), was also found. Alone, this result does not permit to raise any conclusion whether it reflects an increase in antioxidant defenses or, at the opposite, a compensation for an increase in oxidative stress (i.e., superoxide anion) linked to the chemotherapeutic treatment. Clearly, future studies with protein expression analysis of oxidative stress and antioxidant pathways as well as enzymes activities are still necessary to understand the potential implication of redox balance in skeletal muscle deconditioning of breast cancer patients.
Potential Other Cellular Mechanisms of Muscle Deconditioning in Breast Cancer Patients: What Can We Learn From Other Cancers?
Based on the knowledge accumulated through decades of intensive research, this part of the review aims to identify potential cellular mechanisms responsible for skeletal muscle deconditioning in breast cancer patients by drawing a parallel with pre-clinical studies and other cancers models of muscle wasting. As summarized in Figure 2, we have limited our review to the main and well admitted mechanisms of muscle wasting in cancer; our list is therefore not exhaustive. Among the large variety of studies discussed hereafter, we found few studies related to skeletal muscle plasticity conducted on mouse models of breast cancer while several pre-clinical studies explored the effect of doxorubicin administration, one of the most commonly used chemotherapeutic agents to treat breast cancer patients. This lack of specific investigations indicates a major imbalance in comparison with other cancers and also emphasizes the need to remain cautious with the mechanisms identified thereafter as they mainly stem from the analysis of different cancers and treatments. However, it will provide future directions for researchers willing to investigate specifically the mechanisms of muscle deconditioning in breast cancer.
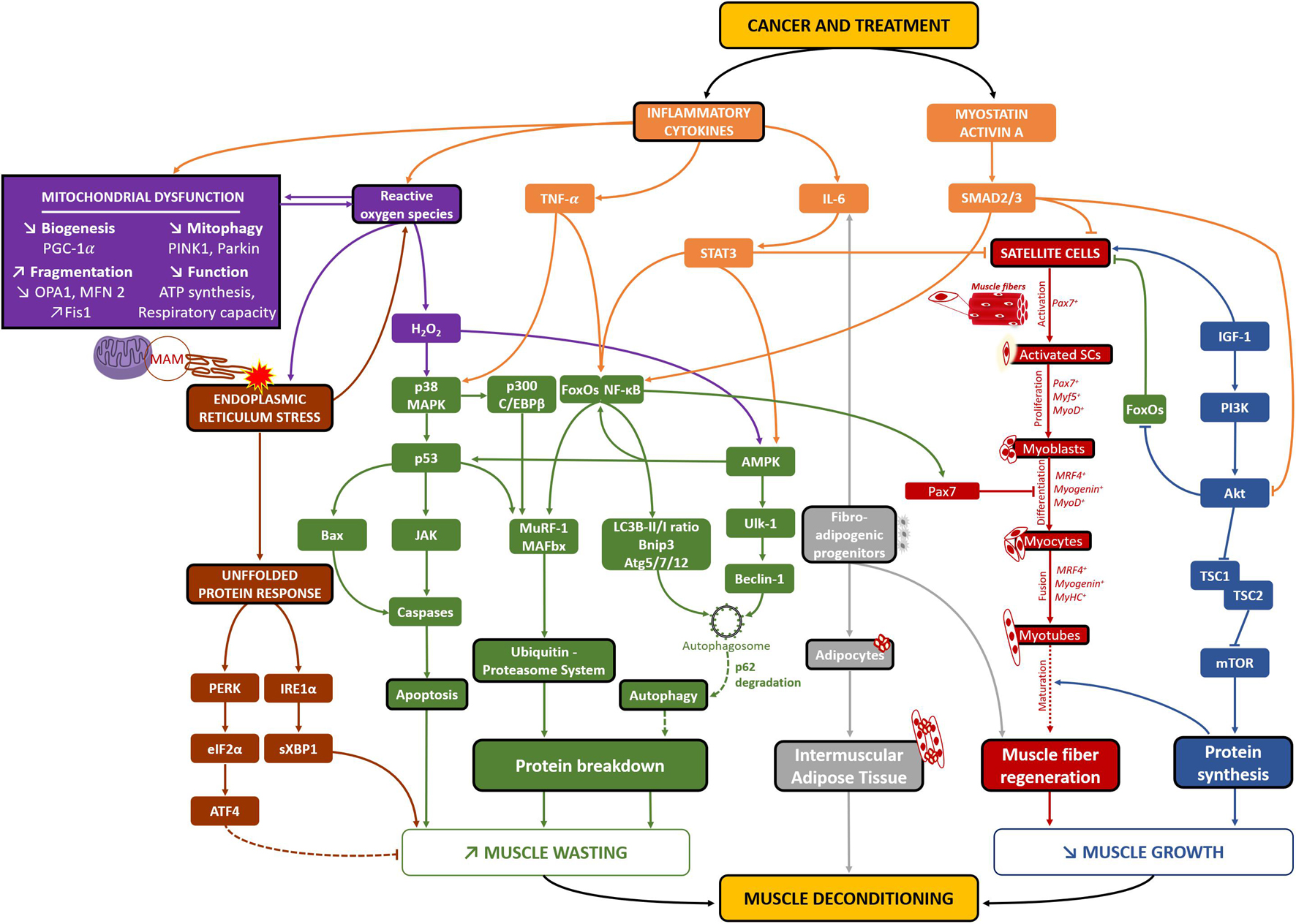
Figure 2. Potential cellular mechanisms of skeletal muscle deconditioning in breast cancer patients. Through the analysis of clinical and pre-clinical studies related to all cancer’s types, several different pathways may be implicated in skeletal muscle deconditioning in breast cancer patients. Both the cancer per se and its treatment lead to increased levels of inflammatory cytokines and Myostatin/Activin A pathways (in orange), consequently decreasing protein synthesis (in blue) and increasing pathways implicated in protein breakdown (in green). The activation of the autophagy-lysosomal system needs to be elucidated as an increase in autophagosomes formation has been consistently found as well as increased levels of p62, suggesting that lysosome activity might be disrupted in cancer cachexia, leading to no change in autophagy flux (green dotted line). The combination of high levels of inflammatory cytokines, ROS and mitochondrial altered dynamics, biogenesis and function (in purple) would also lead to increased protein breakdown and apoptosis. On the other hand, it would also lead to high levels of endoplasmic reticulum stress, resulting in an increase in the UPR system (in brown) and consequently protein breakdown. The involvement of the PERK/eiF2α/ATF4 pathway, known to be implicated in non-cancer models of muscle atrophy, needs to be clarified, as it might surprisingly be necessary to counteract muscle atrophy in cancer-related models of muscle atrophy (brown dotted line). Moreover, different studies also highlighted a reduction in the number of satellite cells (SCs), their capacity to differentiate and skeletal muscle capacity to regenerate, emphasizing that cancer-related muscle atrophy may also result from muscle altered repair/regrowth (in red). Finally, increased presence of fibro-adipogenic progenitors might also be implicated in muscle deconditioning as they are implicated in both IL-6-mediated muscle atrophy (in non-cancer models of muscle atrophy) and Intermuscular adipose tissue development (in gray). Altogether, these cellular mechanisms might play an important role in breast cancer-related skeletal muscle deconditioning and clearly need to be further investigated through clinical studies using muscle biopsies. MAM, mitochondria-associated ER membranes.
Protein Turnover
The sensitive balance between protein synthesis and protein breakdown is the major mechanism regulating muscle mass (Chopard et al., 2009; Schiaffino et al., 2013; Bowen et al., 2015; Argilés et al., 2019; Silva et al., 2020; Vainshtein and Sandri, 2020).
Skeletal muscle protein synthesis is mainly promoted by the PI3K-Akt-mTOR pathway and cachexia patients with pancreatic carcinoma or lung cancer demonstrated an altered PI3K-Akt-mTOR signaling (Schmitt et al., 2007; Murton et al., 2017), a result also found in various pre-clinical models (White et al., 2011; Padrão et al., 2013; Gallot et al., 2014; Puppa et al., 2014; Bohnert et al., 2016; Chen M. C. et al., 2016; de Lima Junior et al., 2016; Sun et al., 2016; Chacon-Cabrera et al., 2017; Quan-Jun et al., 2017; Nissinen et al., 2018; Salazar-Degracia et al., 2018). Importantly, both clinical (Bennegård et al., 1984; Emery et al., 1984; Dworzak et al., 1998) and pre-clinical studies (Beck et al., 1991; Smith and Tisdale, 1993; Samuels et al., 2001; Smith et al., 2004; Constantinou et al., 2011; Nissinen et al., 2016, 2018; Toledo et al., 2016; Antoun and Raynard, 2018; Cruz et al., 2019; Costamagna et al., 2020) highlighted a reduction in muscle protein synthesis, emphasizing that a reduction in protein synthesis may explain, at least in part, the muscle deconditioning occurring in cancer patients. Among all these studies, only two worked on rodents treated with doxorubicin and showed a reduced PI3K-Akt-mTOR signaling (de Lima Junior et al., 2016; Nissinen et al., 2016). Moreover, through RNAseq analysis, Wilson et al. (2019) also found an altered skeletal muscle mTOR signaling in breast cancer-bearing mice. These important studies clearly demonstrated that both breast cancer per se and the chemotherapeutic agents used in clinical setting to treat it may alter the main protein synthesis pathway in skeletal muscle, possibly leading to altered protein turnover.
On the other hand, protein breakdown includes two major pathways, the ubiquitin-proteasome and autophagy-lysosomal systems (UPS and autophagy, respectively), that are responsible for the degradation of most proteins and organelles in skeletal muscle cells. First, numerous pre-clinical studies observed an increase in skeletal muscle protein breakdown (Beck et al., 1991; Smith and Tisdale, 1993; Temparis et al., 1994; Baracos et al., 1995; Samuels et al., 2001; Smith et al., 2004; Silva et al., 2015; Toledo et al., 2016), demonstrating that a decrease in protein synthesis is not the only mechanism that could explain the loss muscle mass in cancer cachexia. UPS and autophagy pathways have been largely investigated both in clinical and pre-clinical studies. The UPS is almost unanimously found to be increased in cancer patients, particularly the “atrogenes” MAFbx and MuRF1 and the overall ubiquitination profile (Williams et al., 1999; Bossola et al., 2003; DeJong et al., 2005; Khal et al., 2005; Constantinou et al., 2011; Puig-Vilanova et al., 2015; Zhang et al., 2020). Importantly, the increase in UPS activity as well as mRNA/proteins implicated in this pathway is also consistently found in a large number of pre-clinical studies (Baracos et al., 1995; Gomes et al., 2001; Lecker et al., 2004; Acharyya et al., 2005; Khal et al., 2005; Moore-Carrasco et al., 2007; Zhou et al., 2010; Julienne et al., 2012; Padrão et al., 2013; Chacon-Cabrera et al., 2014, 2017; Gallot et al., 2014; Johnston et al., 2015; Silva et al., 2015; Bohnert et al., 2016; Chen M. C. et al., 2016; Hatakeyama et al., 2016; Sun et al., 2016; Toledo et al., 2016; Guo et al., 2017; Damrauer et al., 2018; Pin et al., 2018; Salazar-Degracia et al., 2018; Chen L. et al., 2019; Lee et al., 2019; Liu et al., 2019; Ranjbar et al., 2019; Bae et al., 2020; Huot et al., 2020), strengthening the fact that the UPS plays a major role in the protein breakdown aggravation. Concerning the autophagy system, clinical studies also demonstrated an increase in several important markers such as beclin1, Atg5, or LC3B-II/I ratio (Op den Kamp et al., 2012; Johns et al., 2014; Aversa et al., 2016; Pigna et al., 2016; de Castro et al., 2019; Zhang et al., 2020). Together with pre-clinical studies showing the same results (Penna et al., 2013, 2019a; Chacon-Cabrera et al., 2014; Bohnert et al., 2016; Salazar-Degracia et al., 2016, 2018; Sirago et al., 2017; Ballarò et al., 2019; Ranjbar et al., 2019), autophagy might also play a significant role in the increased protein breakdown of cancer patients. However, it appears of great importance to highlight that the majority of these studies also found an increase in p62 mRNA/protein expression, suggesting that if autophagosome formation is certainly increased, lysosome activity might be disrupted in cancer patients, leading to no modifications in autophagy flux (Penna et al., 2014; Klionsky et al., 2021). Furthermore, different studies showed that skeletal muscle protein breakdown is mostly ATP-dependent (i.e., UPS) in pre-clinical models of cancer cachexia (Temparis et al., 1994; Baracos et al., 1995; White et al., 2011), emphasizing again that autophagy might not be implicated or has a minor role in cancer-induced skeletal muscle wasting. As it is well known that the loss of autophagy leads to muscle wasting exacerbation in response to atrophic stimulus (Vainshtein and Sandri, 2020), further studies are needed to elucidate the variations of autophagic flux, whether it is an increase or a decrease, in cancer cachexia.
The FoxO family of transcription factors (FoxO1 and FoxO3 particularly) and NF-κB are known to be essential transcription factors implicated in the regulation of numerous genes of both UPS and autophagy pathways in various models of skeletal muscle atrophy (Vainshtein and Sandri, 2020). These transcription factors have been found to be upregulated in different cancer cachexia models (Cai et al., 2004; Lecker et al., 2004; White et al., 2011; Op den Kamp et al., 2013; Chacon-Cabrera et al., 2014, 2017; Gallot et al., 2014; Puppa et al., 2014; Chen M. C. et al., 2016; Sun et al., 2016; Sirago et al., 2017; Salazar-Degracia et al., 2018; Lee et al., 2019; Bae et al., 2020) including in cancer patients (Rhoads et al., 2010; Skorokhod et al., 2012; Puig-Vilanova et al., 2015; Johns et al., 2017).
Aside from studies presented in the section of this review dedicated to breast cancer clinical studies (Lønbro et al., 2017; Bohlen et al., 2018; Guigni et al., 2018; Mijwel et al., 2018b; Møller et al., 2019; Wilson et al., 2020), no preclinical study has been done in order to explore protein breakdown in breast cancer models. However, some pre-clinical studies explored the effect of doxorubicin on skeletal muscle and an increase in both UPS and autophagy pathways was suggested as MAFbx, beclin1, Atg12, Atg7, and LC3B-II/I ratio increased with doxorubicin treatment in mice and rats (Smuder et al., 2011; Kavazis et al., 2014; Hulmi et al., 2018; Montalvo et al., 2020). Importantly, only Montalvo et al. (2020) explored p62 protein levels and found no change in its expression, again emphasizing the need to obtain a more precise understanding of the autophagic pathway. Interestingly, Yu et al. (2014) also treated mice with doxorubicin and found no change in numerous autophagy markers. Altogether, pre-clinical studies demonstrated altered protein synthesis and breakdown mainly in response to doxorubicin administration in rodents, again emphasizing that these mechanisms may induce skeletal muscle wasting in breast cancer patients.
Pro-inflammatory and TGF-β Family Cytokines
As a critical upstream of protein turnover alteration, inflammation plays a key role in the development of muscle wasting in cancer patients. Indeed, either released by the tumor or immune cells, pro-inflammatory cytokines like TNF-α, TWEAK, IL-6, IL-1β, IL-8, and INFγ have been found to be upregulated at a systemic level in animals (Costelli et al., 1993; Baltgalvis et al., 2008; Zhou et al., 2010; Toledo et al., 2016; Guo et al., 2017; Chen T. et al., 2018; Bae et al., 2020; Bernardo et al., 2020; Huot et al., 2020) and in cancer patients (Scott et al., 1996; DeJong et al., 2005; Moses et al., 2009; Skipworth et al., 2011; Op den Kamp et al., 2013; Puig-Vilanova et al., 2015; Johns et al., 2017; Riccardi et al., 2020). Importantly, from a study that included 661 breast cancer patients, systemic inflammatory cytokines were associated with a poor survival, reduced disease-specific survival and disease-free survival (Cho et al., 2018). These inflammatory cytokines have been also found to be upregulated within skeletal muscle in pre-clinical studies (Skipworth et al., 2011; Johnston et al., 2015; Chen M. C. et al., 2016; Hatakeyama et al., 2016; Chen L. et al., 2019; Lee et al., 2019; Bae et al., 2020), but none of these has been investigated in breast cancer models.
In addition to pro-inflammatory cytokines, two particular members of the TGF-β family have been particularly explored in cancer cachexia: myostatin (MSTN) and Activin A. MSTN clearly represents one of the most potent negative regulator of muscle growth and is known to act through its receptor ActRIIB and the subsequent activation of the SMAD2/SMAD3 cascade (Rodriguez et al., 2014). MSTN and/or its downstream targets have been found to be upregulated in many experiments on cancer cachexia (Costelli et al., 2008; Bonetto et al., 2009; Zhou et al., 2010; Murphy et al., 2011; Aversa et al., 2012; Padrão et al., 2013; Chacon-Cabrera et al., 2014; Silva et al., 2015; Chen M. C. et al., 2016; Sun et al., 2016; Chen M. C. et al., 2018; Salazar-Degracia et al., 2018; Lee et al., 2019; Huot et al., 2020), as well as in studies exploring the effect of doxorubicin administration (Kavazis et al., 2014; Liu et al., 2019). Acting through the same receptor than MSTN (ActRIIB), Activin A is also found to be increased in cancer cachexia (Leto et al., 2006; Loumaye et al., 2015; Matsuyama et al., 2015; Chen J. L. et al., 2016; Chen M. C. et al., 2016; Barreto et al., 2017; Zhong et al., 2019; Bernardo et al., 2020) and an independent prognosis factor of survival in cancer patients (Loumaye et al., 2017). Several authors conducted experiments with inhibition of the MSTN/Activin A pathway and found a reduction, or even a complete reversal, in the decrease of muscle mass and function in pre-clinical models (Liu et al., 2008; Benny Klimek et al., 2010; Murphy et al., 2011; Busquets et al., 2012a, b; Gallot et al., 2014; Hatakeyama et al., 2016; Levolger et al., 2019; Ojima et al., 2020; Pettersen et al., 2020), leading to the consideration of this pharmacological strategy for human cancer patients.
Mitochondrial Alterations, Oxidative Stress, and Unfolded Protein Response
Mitochondrial alterations represent a major aspect of muscle deconditioning that have been already associated with skeletal muscle atrophy in breast cancer patients (Bohlen et al., 2018; Guigni et al., 2018; Mijwel et al., 2018b; Wilson et al., 2020), and in other cancers such as gastrointestinal and lung cancer patients (Op den Kamp et al., 2015; de Castro et al., 2019). Triggered by both structural and functional mitochondrial impairments, mitochondrial alterations have been particularly studied in pre-clinical studies. First, altered morphology and/or mitochondria loss have been found in different models of cancer in animals and/or with chemotherapeutic agents (Shum et al., 2012; White et al., 2012; Fontes-Oliveira et al., 2013; Barreto et al., 2016; Brown et al., 2017; Sorensen et al., 2017) as well as in gastric cancer patients (Zhang et al., 2020). Taken together, these results showing mitochondrial alterations on other cancer types strengthen the abovementioned results specifically observed in breast cancer (Guigni et al., 2018; Mijwel et al., 2018b) and might be a specific maladaptation between cancers. Concerning mitochondrial function, the overall oxidative pathway is clearly affected by both cancer and chemotherapeutic agents (Ushmorov et al., 1999; Constantinou et al., 2011; Julienne et al., 2012; Fermoselle et al., 2013; Gilliam et al., 2013, 2016; Padrão et al., 2013; Tzika et al., 2013; McLean et al., 2014; Gouspillou et al., 2015; Op den Kamp et al., 2015; Puig-Vilanova et al., 2015; de Lima Junior et al., 2016; Brown et al., 2017; Crouch et al., 2017; Pin et al., 2018; Ryan et al., 2018; Neyroud et al., 2019; Penna et al., 2019b; Hulmi et al., 2020; Kunzke et al., 2020). Among these studies, only two showed the potent negative impact of doxorubicin on complexes respiratory capacity (Gilliam et al., 2013; Gouspillou et al., 2015) while Crouch et al. (2017) highlighted a decrease in ATP production with cyclophosphamide administration, an immunosuppressor commonly associated with doxorubicin in breast cancer treatment. Interestingly, various authors also found altered mitochondrial dynamics, with a decreased fusion and increased fission, leading to mitochondria fragmentation in cancer cachexia (White et al., 2011, 2012; Barreto et al., 2016; Brown et al., 2017; Marzetti et al., 2017; Pin et al., 2018; de Castro et al., 2019; Huot et al., 2020). Surprisingly, although it was found that breast cancer patients lost mitochondria during their chemotherapeutic treatment (Guigni et al., 2018), mitochondria dynamics has not been investigated to date in specific preclinical models of breast cancer patients. Even if it is well known that mitochondria fission is prerequisite for the activation of the mitophagy process, it seems that mitophagy is also dysfunctional in cancer as several authors showed a decrease in key markers such as PINK1 or Parkin (Aversa et al., 2016; Marzetti et al., 2017). This statement has been also confirmed in the study of Gouspillou et al. (2015) with mice treated with doxorubicin (reduced Parkin protein levels) as well as in the study of Mijwel et al. (2018b) with breast cancer patients (reduced PINK1 protein levels).
As a consequence of mitochondrial dysfunction and potential reduced mitophagy, fragmented and damaged mitochondria accumulate in skeletal muscle and, in addition to being less bioenergetically efficient, produce excessive amounts of oxidative stress, mediated through increases in reactive oxygen species (ROS). Indeed, many different studies found an increase in ROS (Gilliam et al., 2013, 2016; Gouspillou et al., 2015; Min et al., 2015; Chacon-Cabrera et al., 2017; Pin et al., 2018; Ballarò et al., 2019; Montalvo et al., 2020), more specifically elevated levels of hydrogen peroxide (H2O2). Unanimously, several studies reported that doxorubicin administration in rodents led to an increase in H2O2 production (Gilliam et al., 2013, 2016; Min et al., 2015; Montalvo et al., 2020), while there is still no clinical study available to confirm this increase in breast cancer patients. One of the consequences of the increase in oxidative stress is the alteration of protein turnover pathways, with a decrease in protein synthesis, supported by an altered PI3k-Akt-mTOR pathway, and an increase in protein breakdown systems (i.e., UPS and autophagy). Aside the protein turnover deregulation, mitochondria-mediated oxidative stress is also a potent initiator of apoptosis [see reviews from Powers et al. (2016),Aggarwal et al. (2019), Sies and Jones (2020), and Hyatt and Powers (2021)]. Many studies showed an increase in key markers of apoptosis in various pre-clinical models (Belizário et al., 2001; Ishiko et al., 2001; Yoshida et al., 2001; Tsang et al., 2003; Figueras et al., 2004; Schwarzkopf et al., 2006; Baltgalvis et al., 2008; Murphy et al., 2011; Smuder et al., 2011; Chacon-Cabrera et al., 2014; Salazar-Degracia et al., 2016, 2018) and in cancer patients (Busquets et al., 2007; de Castro et al., 2019). Three other studies also explored the effect of doxorubicin treatment in rodents and in vitro (C2C12) and found increased levels of caspase 3 (both its activity and cleaved form of caspase 3 protein expression) and of Bax (Gilliam et al., 2012; Yu et al., 2014; Min et al., 2015). Finally, the study of Ahmadabadi et al. (2020) also observed a decrease in Bcl-2/Bax ratio in breast cancer-bearing mice, showing once again that apoptosis might be upregulated in breast cancer patients. Intuitively, the loss of muscle cells or myonuclei would appear like one of the causes of muscle atrophy, and studies have already shown associations between loss of muscle mass/CSA and the number of apoptotic cells (Allen et al., 1997; Borisov and Carlson, 2000; Smith et al., 2000; Dupont-Versteegden, 2005; Andrianjafiniony et al., 2010; Guo et al., 2012; Chacon-Cabrera et al., 2014; Cheema et al., 2015; Salazar-Degracia et al., 2016).
Increased levels in unfolded or misfolded proteins and oxidative stress (due to the potential deficit in autophagy/mitophagy and mitochondrial dysfunction) will lead to endoplasmic reticulum stress and trigger the unfolded protein response (UPR) that might represent another major maladaptation taking place during cancer cachexia. Acting through three pathways (PERK-eIf2α-ATF4, IRE1α-sXBP1, and ATF6-ATF6N) the UPR contributes to skeletal muscle atrophy by decreasing protein synthesis, increasing protein breakdown and, ultimately, inducing apoptosis (Urbina-Varela et al., 2020; Vainshtein and Sandri, 2020; Gallot and Bohnert, 2021). The UPR has been shown to be upregulated in several pre-clinical studies of cancer cachexia (Bohnert et al., 2016, 2019; Gallot et al., 2019; Straughn et al., 2021) and in response to doxorubicin treatment (Montalvo et al., 2020) leading to the conclusion that the increased activity of the UPR system would trigger the muscle atrophy program and contribute to muscle wasting. However, as clearly described in the review of Gallot and Bohnert (2021), specific increase in the PERK-eIf2α-ATF4 pathway might also be necessary during skeletal muscle atrophy to counteract it, as both pharmacological (Bohnert et al., 2016) or genetical tools (Gallot et al., 2019) aiming to inhibit this pathway aggravated cancer-related muscle atrophy. On the contrary, muscle-specific deletion of XBP1 in LLC-bearing mice exhibited a reduced muscle atrophy, demonstrating that the IRE1α-sXBP1 axis of the UPR system seems to be implicated in cancer-mediated muscle atrophy.
Satellite Cells
The capacity of skeletal muscle to regenerate is another key parameter of its functionality. After injury, successful skeletal muscle regeneration appears to be driven by complex and precisely orchestrated processes involving multiple cell types. Of these cell types, satellite cells (SCs), localized between the sarcolemma and the basal lamina of myofibers (Mauro, 1961), represents the most studied and essential stem cells in order to support the regeneration process. In the context of cancer cachexia, several studies already showed that skeletal muscle tissue exhibited signs of ongoing degeneration/regeneration cycles, including ultrastructural damage, central nuclei localization, increased macrophages abundance as well as SCs proliferation in patients (Zampieri et al., 2010; He et al., 2013) and in pre-clinical models (Mehl et al., 2005; Chacon-Cabrera et al., 2014, 2017; Salazar-Degracia et al., 2016, 2018; Judge et al., 2018), including in breast cancer-bearing mice (Ahmadabadi et al., 2020). These signs of damage and regeneration might indicate an increased fragility of the skeletal muscle and an environment prone to lead to more degeneration/regeneration cycles. Having in mind that several authors also highlighted a clear decrease in regeneration capacity (He et al., 2013; Coletti et al., 2016; Inaba et al., 2018; Costamagna et al., 2020), cancer-related muscle atrophy may also result from muscle decreased repair/regrowth after injury and not only from different pathways causing protein turnover dysregulation. More specifically, the excellent study of He et al. (2013) demonstrated that SCs were able to proliferate and commit to the myogenic lineage, but unable to differentiate properly due to an NF-κB dependent increase in Pax7 expression. This increase in Pax7 expression was also found in breast cancer-bearing mice (Hesse et al., 2019) as well as in other cancers pre-clinical studies (Penna et al., 2010; Coletti et al., 2016; Costamagna et al., 2020), ultimately leading to muscle regeneration dysfunction. Importantly, D’Lugos et al. (2019) found that chronic doxorubicin administration drastically reduced SCs content in rats, suggesting that if cancer per se would inhibit myogenic differentiation process, the combination of both the disease and chemotherapeutic drugs administration might lead to global SCs dysfunction and loss in breast cancer patients. However, as highlighted in our section dedicated to clinical studies in breast cancer patients, only Mijwel et al. (2018b) investigated Pax7+-labeled SCs and found no change in their number. Therefore, more studies are necessary to clarify SCs fate and implication in breast cancer patients and/or pre-clinical models.
Intermuscular Adipose Tissue and Fibro-Adipogenic Progenitors
The abnormal development of fibrotic and/or IMAT deposits within skeletal muscle is a strong marker of regenerative failure. As documented above, breast cancer patients exhibit an increase in IMAT (Rier et al., 2018; Reding et al., 2019; Beaudry et al., 2020), a result also found in other types of cancers [for a systematic review see Aleixo et al. (2020b)]. However, we did not find any study exploring the cellular mechanisms related to IMAT development in preclinical models of breast cancer or with the administration of commonly used chemotherapeutic agents. In muscle disuse or pathological conditions, such as Duchenne muscular dystrophy, FAPs proliferate and differentiate into adipose and/or fibrous tissue (Uezumi et al., 2011, 2014a; Ieronimakis et al., 2016) and are currently accepted to represent the major population that appears to play a role in IMAT development (Brioche et al., 2016; Biferali et al., 2019; Theret et al., 2021). In the context of cancer cachexia, one study found an increased presence of FAPs in the muscle environment of pancreatic cancer patients (Judge et al., 2018) that might explain the development of myosteatosis observed in overall cancer patients. Considering the increase in IMAT development found in breast cancer patients (Rier et al., 2018; Reding et al., 2019; Beaudry et al., 2020), it thus appears essential to explore FAPs fate in this specific context.
Other than their important role in muscle regeneration and abnormal development of IMAT, FAPs have been recently shown to promote skeletal muscle atrophy. Indeed, the study of Madaro et al. (2018) demonstrated that FAPs progressively accumulate and exhibit increased IL-6/STAT3 signaling, promoting muscle atrophy in different mouse models. Interestingly, inactivation of this pathway effectively countered the muscle atrophy and fibrosis observed in these models, emphasizing a potential role of FAPs secretome and paracrine effects on skeletal muscle fibers. Considering the ambivalent role of FAPs in the development of IMAT and muscle atrophy, further studies should focus on these stem cells in order to elucidate their potential role in both pre-clinical and clinical models of cancer-related skeletal muscle wasting.
Conclusion
Breast cancer patients undergoing chemotherapy definitively experience skeletal muscle deconditioning, mainly characterized by both a decrease in muscle mass and function. Despite the fact that mechanisms of muscle deconditioning are well known in many other muscle wasting models, including in other pre-clinical or clinical models of cancers, they still remain relatively unknown in breast cancer patients. In fact, some studies using muscle biopsies highlighted protein turnover and mitochondrial alterations in breast cancer patients, but other studies are clearly needed to obtain a more precise understanding of the cellular processes implicated in breast cancer-mediated muscle deconditioning. This lack of knowledge inevitably leads to difficulties for the implementation of efficient preventive strategies such as exercise, nutrition or pharmacological treatments.
Author Contributions
JM, EH, TH, and AP contributed to writing or editing the manuscript and approved the final version of the manuscript. All authors contributed to the article and approved the submitted version.
Funding
This work was supported by the Institut de Cancérologie Strasbourg Europe. This work has been published under the framework of the IdEx Unistra supported by the investments for the future program of the French Government.
Conflict of Interest
The authors declare that the research was conducted in the absence of any commercial or financial relationships that could be construed as a potential conflict of interest.
Publisher’s Note
All claims expressed in this article are solely those of the authors and do not necessarily represent those of their affiliated organizations, or those of the publisher, the editors and the reviewers. Any product that may be evaluated in this article, or claim that may be made by its manufacturer, is not guaranteed or endorsed by the publisher.
References
Acharyya, S., Butchbach, M. E. R., Sahenk, Z., Wang, H., Saji, M., Carathers, M., et al. (2005). Dystrophin glycoprotein complex dysfunction: a regulatory link between muscular dystrophy and cancer cachexia. Cancer Cell 8, 421–432. doi: 10.1016/j.ccr.2005.10.004
Addison, O., Marcus, R. L., Lastayo, P. C., and Ryan, A. S. (2014). Intermuscular fat: a review of the consequences and causes. Int. J. Endocrinol. 2014:309570. doi: 10.1155/2014/309570
Agarwala, P., and Salzman, S. H. (2020). Six-Minute walk test: clinical role, technique, coding, and reimbursement. Chest 157, 603–611. doi: 10.1016/j.chest.2019.10.014
Aggarwal, V., Tuli, H. S., Varol, A., Thakral, F., Yerer, M. B., Sak, K., et al. (2019). Role of reactive oxygen species in cancer progression: molecular mechanisms and recent advancements. Biomolecules 9:735. doi: 10.3390/biom9110735
Ahmadabadi, F., Saghebjoo, M., Huang, C.-J., Saffari, I., and Zardast, M. (2020). The effects of high-intensity interval training and saffron aqueous extract supplementation on alterations of body weight and apoptotic indices in skeletal muscle of 4T1 breast cancer-bearing mice with cachexia. Appl. Physiol. Nutr. Metab. Physiol. Appl. Nutr. Metab. 45, 555–563. doi: 10.1139/apnm-2019-0352
Aleixo, G. F. P., Deal, A. M., Nyrop, K. A., Muss, H. B., Damone, E. M., Williams, G. R., et al. (2020a). Association of body composition with function in women with early breast cancer. Breast Cancer Res. Treat. 181, 411–421. doi: 10.1007/s10549-020-05624-3
Aleixo, G. F. P., Shachar, S. S., Nyrop, K. A., Muss, H. B., Malpica, L., and Williams, G. R. (2020b). Myosteatosis and prognosis in cancer: systematic review and meta-analysis. Crit. Rev. Oncol. Hematol. 145:102839. doi: 10.1016/j.critrevonc.2019.102839
Aleixo, G. F. P., Williams, G. R., Nyrop, K. A., Muss, H. B., and Shachar, S. S. (2019). Muscle composition and outcomes in patients with breast cancer: meta-analysis and systematic review. Breast Cancer Res. Treat. 177, 569–579. doi: 10.1007/s10549-019-05352-3
Allen, D. L., Linderman, J. K., Roy, R. R., Grindeland, R. E., Mukku, V., and Edgerton, V. R. (1997). Growth hormone/IGF-I and/or resistive exercise maintains myonuclear number in hindlimb unweighted muscles. J. Appl. Physiol. Bethesda Md 1985 83, 1857–1861. doi: 10.1152/jappl.1997.83.6.1857
Andrianjafiniony, T., Dupré-Aucouturier, S., Letexier, D., Couchoux, H., and Desplanches, D. (2010). Oxidative stress, apoptosis, and proteolysis in skeletal muscle repair after unloading. Am. J. Physiol. Cell Physiol. 299, C307–C315. doi: 10.1152/ajpcell.00069.2010
Antoun, S., and Raynard, B. (2018). Muscle protein anabolism in advanced cancer patients: response to protein and amino acids support, and to physical activity. Ann. Oncol. Off. J. Eur. Soc. Med. Oncol. 29, ii10–ii17. doi: 10.1093/annonc/mdx809
Arc-Chagnaud, C., Py, G., Fovet, T., Roumanille, R., Demangel, R., Pagano, A. F., et al. (2020). Evaluation of an antioxidant and anti-inflammatory cocktail against human hypoactivity-induced skeletal muscle deconditioning. Front. Physiol. 11:71. doi: 10.3389/fphys.2020.00071
Argilés, J. M., Busquets, S., Stemmler, B., and López-Soriano, F. J. (2014). Cancer cachexia: understanding the molecular basis. Nat. Rev. Cancer 14, 754–762. doi: 10.1038/nrc3829
Argilés, J. M., López-Soriano, F. J., and Busquets, S. (2019). Mediators of cachexia in cancer patients. Nutrition 66, 11–15. doi: 10.1016/j.nut.2019.03.012
Astrand, P. O., and Saltin, B. (1961). Maximal oxygen uptake and heart rate in various types of muscular activity. J. Appl. Physiol. 16, 977–981. doi: 10.1152/jappl.1961.16.6.977
Atherton, P. J., Greenhaff, P. L., Phillips, S. M., Bodine, S. C., Adams, C. M., and Lang, C. H. (2016). Control of skeletal muscle atrophy in response to disuse: clinical/preclinical contentions and fallacies of evidence. Am. J. Physiol. Endocrinol. Metab. 311, E594–E604. doi: 10.1152/ajpendo.00257.2016
Aubrey, J., Esfandiari, N., Baracos, V. E., Buteau, F. A., Frenette, J., Putman, C. T., et al. (2014). Measurement of skeletal muscle radiation attenuation and basis of its biological variation. Acta Physiol. Oxf. Engl. 210, 489–497. doi: 10.1111/apha.12224
Aversa, Z., Bonetto, A., Penna, F., Costelli, P., Di Rienzo, G., Lacitignola, A., et al. (2012). Changes in myostatin signaling in non-weight-losing cancer patients. Ann. Surg. Oncol. 19, 1350–1356. doi: 10.1245/s10434-011-1720-5
Aversa, Z., Pin, F., Lucia, S., Penna, F., Verzaro, R., Fazi, M., et al. (2016). Autophagy is induced in the skeletal muscle of cachectic cancer patients. Sci. Rep. 6:30340. doi: 10.1038/srep30340
Bae, T., Jang, J., Lee, H., Song, J., Chae, S., Park, M., et al. (2020). Paeonia lactiflora root extract suppresses cancer cachexia by down-regulating muscular NF-κB signalling and muscle-specific E3 ubiquitin ligases in cancer-bearing mice. J. Ethnopharmacol. 246:112222. doi: 10.1016/j.jep.2019.112222
Baldwin, K. M., Haddad, F., Pandorf, C. E., Roy, R. R., and Edgerton, V. R. (2013). Alterations in muscle mass and contractile phenotype in response to unloading models: role of transcriptional/pretranslational mechanisms. Front. Physiol. 4:284. doi: 10.3389/fphys.2013.00284
Ballarò, R., Beltrà, M., De Lucia, S., Pin, F., Ranjbar, K., Hulmi, J. J., et al. (2019). Moderate exercise in mice improves cancer plus chemotherapy-induced muscle wasting and mitochondrial alterations. FASEB J. 33, 5482–5494. doi: 10.1096/fj.201801862R
Baltgalvis, K. A., Berger, F. G., Pena, M. M. O., Davis, J. M., Muga, S. J., and Carson, J. A. (2008). Interleukin-6 and cachexia in ApcMin/+ mice. Am. J. Physiol. Regul. Integr. Comp. Physiol. 294, R393–R401. doi: 10.1152/ajpregu.00716.2007
Baracos, V. E., DeVivo, C., Hoyle, D. H., and Goldberg, A. L. (1995). Activation of the ATP-ubiquitin-proteasome pathway in skeletal muscle of cachectic rats bearing a hepatoma. Am. J. Physiol. 268, E996–E1006. doi: 10.1152/ajpendo.1995.268.5.E996
Baracos, V. E., Martin, L., Korc, M., Guttridge, D. C., and Fearon, K. C. H. (2018). Cancer-associated cachexia. Nat. Rev. Dis. Primers 4:17105. doi: 10.1038/nrdp.2017.105
Barreto, R., Kitase, Y., Matsumoto, T., Pin, F., Colston, K. C., Couch, K. E., et al. (2017). ACVR2B/Fc counteracts chemotherapy-induced loss of muscle and bone mass. Sci. Rep. 7:14470. doi: 10.1038/s41598-017-15040-1
Barreto, R., Waning, D. L., Gao, H., Liu, Y., Zimmers, T. A., and Bonetto, A. (2016). Chemotherapy-related cachexia is associated with mitochondrial depletion and the activation of ERK1/2 and p38 MAPKs. Oncotarget 7, 43442–43460. doi: 10.18632/oncotarget.9779
Beaudry, R. I., Kirkham, A. A., Thompson, R. B., Grenier, J. G., Mackey, J. R., and Haykowsky, M. J. (2020). Exercise intolerance in anthracycline-treated breast cancer survivors: the role of skeletal muscle bioenergetics, oxygenation, and composition. The Oncologist 25, e852–e860. doi: 10.1634/theoncologist.2019-0777
Beavers, K. M., Beavers, D. P., Houston, D. K., Harris, T. B., Hue, T. F., Koster, A., et al. (2013). Associations between body composition and gait-speed decline: results from the Health, Aging, and Body Composition study. Am. J. Clin. Nutr. 97, 552–560. doi: 10.3945/ajcn.112.047860
Beck, S. A., Smith, K. L., and Tisdale, M. J. (1991). Anticachectic and antitumor effect of eicosapentaenoic acid and its effect on protein turnover. Cancer Res. 51, 6089–6093.
Behr, T. M., Béhé, M., and Wörmann, B. (2001). Trastuzumab and breast cancer. N. Engl. J. Med. 345, 995–996. doi: 10.1056/NEJM200109273451312
Belizário, J. E., Lorite, M. J., and Tisdale, M. J. (2001). Cleavage of caspases-1, -3, -6, -8 and -9 substrates by proteases in skeletal muscles from mice undergoing cancer cachexia. Br. J. Cancer 84, 1135–1140. doi: 10.1054/bjoc.2001.1700
Bennegård, K., Lindmark, L., Edén, E., Svaninger, G., and Lundholm, K. (1984). Flux of amino acids across the leg in weight-losing cancer patients. Cancer Res. 44, 386–393.
Benny Klimek, M. E., Aydogdu, T., Link, M. J., Pons, M., Koniaris, L. G., and Zimmers, T. A. (2010). Acute inhibition of myostatin-family proteins preserves skeletal muscle in mouse models of cancer cachexia. Biochem. Biophys. Res. Commun. 391, 1548–1554. doi: 10.1016/j.bbrc.2009.12.123
Berger, A. M., Mitchell, S. A., Jacobsen, P. B., and Pirl, W. F. (2015). Screening, evaluation, and management of cancer-related fatigue: ready for implementation to practice? CA Cancer J. Clin. 65, 190–211. doi: 10.3322/caac.21268
Bergstrom, J. (1975). Percutaneous needle biopsy of skeletal muscle in physiological and clinical research. Scand. J. Clin. Lab. Invest. 35, 609–616.
Bernardo, B., Joaquim, S., Garren, J., Boucher, M., Houle, C., LaCarubba, B., et al. (2020). Characterization of cachexia in the human fibrosarcoma HT-1080 mouse tumour model. J. Cachexia Sarcopenia Muscle 11, 1813–1829. doi: 10.1002/jcsm.12618
Biferali, B., Proietti, D., Mozzetta, C., and Madaro, L. (2019). Fibro-Adipogenic progenitors cross-talk in skeletal muscle: the social network. Front. Physiol. 10:1074. doi: 10.3389/fphys.2019.01074
Bird, B. R. J. H., and Swain, S. M. (2008). Cardiac toxicity in breast cancer survivors: review of potential cardiac problems. Clin. Cancer Res. 14, 14–24. doi: 10.1158/1078-0432.CCR-07-1033
Bodine, S. C. (2013). Disuse-induced muscle wasting. Int. J. Biochem. Cell Biol. 45, 2200–2208. doi: 10.1016/j.biocel.2013.06.011
Bohlen, J., McLaughlin, S. L., Hazard-Jenkins, H., Infante, A. M., Montgomery, C., Davis, M., et al. (2018). Dysregulation of metabolic-associated pathways in muscle of breast cancer patients: preclinical evaluation of interleukin-15 targeting fatigue. J. Cachexia Sarcopenia Muscle 9, 701–714. doi: 10.1002/jcsm.12294
Bohnert, K. R., Gallot, Y. S., Sato, S., Xiong, G., Hindi, S. M., and Kumar, A. (2016). Inhibition of ER stress and unfolding protein response pathways causes skeletal muscle wasting during cancer cachexia. FASEB J. Off. Publ. Fed. Am. Soc. Exp. Biol. 30, 3053–3068. doi: 10.1096/fj.201600250RR
Bohnert, K. R., Goli, P., Roy, A., Sharma, A. K., Xiong, G., Gallot, Y. S., et al. (2019). The toll-like receptor/MyD88/XBP1 signaling axis mediates skeletal muscle wasting during cancer cachexia. Mol. Cell. Biol. 39:e00184-19. doi: 10.1128/MCB.00184-19
Bonaldo, P., and Sandri, M. (2013). Cellular and molecular mechanisms of muscle atrophy. Dis. Model. Mech. 6, 25–39. doi: 10.1242/dmm.010389
Bonetto, A., Penna, F., Minero, V. G., Reffo, P., Bonelli, G., Baccino, F. M., et al. (2009). Deacetylase inhibitors modulate the myostatin/follistatin axis without improving cachexia in tumor-bearing mice. Curr. Cancer Drug Targets 9, 608–616. doi: 10.2174/156800909789057015
Borisov, A. B., and Carlson, B. M. (2000). Cell death in denervated skeletal muscle is distinct from classical apoptosis. Anat. Rec. 258, 305–318.
Bossola, M., Muscaritoli, M., Costelli, P., Grieco, G., Bonelli, G., Pacelli, F., et al. (2003). Increased muscle proteasome activity correlates with disease severity in gastric cancer patients. Ann. Surg. 237, 384–389. doi: 10.1097/01.SLA.0000055225.96357.71
Bowen, T. S., Schuler, G., and Adams, V. (2015). Skeletal muscle wasting in cachexia and sarcopenia: molecular pathophysiology and impact of exercise training. J. Cachexia Sarcopenia Muscle 6, 197–207. doi: 10.1002/jcsm.12043
Bregni, G., Galli, G., Gevorgyan, A., de Braud, F., and Di Cosimo, S. (2016). Trastuzumab cardiac toxicity: a problem we put our heart into. Tumori 102, 1–5. doi: 10.5301/tj.5000393
Brioche, T., Pagano, A. F., Py, G., and Chopard, A. (2016). Muscle wasting and aging: experimental models, fatty infiltrations, and prevention. Mol. Aspects Med. 50, 56–87. doi: 10.1016/j.mam.2016.04.006
Brown, J. L., Rosa-Caldwell, M. E., Lee, D. E., Blackwell, T. A., Brown, L. A., Perry, R. A., et al. (2017). Mitochondrial degeneration precedes the development of muscle atrophy in progression of cancer cachexia in tumour-bearing mice. J. Cachexia Sarcopenia Muscle 8, 926–938. doi: 10.1002/jcsm.12232
Busquets, S., Deans, C., Figueras, M., Moore-Carrasco, R., López-Soriano, F. J., Fearon, K. C. H., et al. (2007). Apoptosis is present in skeletal muscle of cachectic gastro-intestinal cancer patients. Clin. Nutr. Edinb. Scotl. 26, 614–618. doi: 10.1016/j.clnu.2007.06.005
Busquets, S., Toledo, M., Marmonti, E., Orpí, M., Capdevila, E., Betancourt, A., et al. (2012a). Formoterol treatment downregulates the myostatin system in skeletal muscle of cachectic tumour-bearing rats. Oncol. Lett. 3, 185–189. doi: 10.3892/ol.2011.442
Busquets, S., Toledo, M., Orpí, M., Massa, D., Porta, M., Capdevila, E., et al. (2012b). Myostatin blockage using actRIIB antagonism in mice bearing the Lewis lung carcinoma results in the improvement of muscle wasting and physical performance. J. Cachexia Sarcopenia Muscle 3, 37–43. doi: 10.1007/s13539-011-0049-z
But-Hadzic, J., Dervisevic, M., Karpljuk, D., Videmsek, M., Dervisevic, E., Paravlic, A., et al. (2021). Six-Minute walk distance in breast cancer survivors-a systematic review with meta-analysis. Int. J. Environ. Res. Public. Health 18:2591. doi: 10.3390/ijerph18052591
Caan, B. J., Feliciano, E. M. C., Prado, C. M., Alexeeff, S., Kroenke, C. H., Bradshaw, P., et al. (2018). Association of muscle and adiposity measured by computed tomography with survival in patients with nonmetastatic breast cancer. JAMA Oncol. 4, 798–804. doi: 10.1001/jamaoncol.2018.0137
Cai, D., Frantz, J. D., Tawa, N. E., Melendez, P. A., Oh, B.-C., Lidov, H. G. W., et al. (2004). IKKbeta/NF-kappaB activation causes severe muscle wasting in mice. Cell 119, 285–298. doi: 10.1016/j.cell.2004.09.027
CeŠeiko, R., Thomsen, S. N., Tomsone, S., EglĪtis, J., Vçtra, A., Srebnijs, A., et al. (2020). Heavy resistance training in breast cancer patients undergoing adjuvant therapy. Med. Sci. Sports Exerc. 52, 1239–1247. doi: 10.1249/MSS.0000000000002260
Cespedes Feliciano, E. M., Chen, W. Y., Lee, V., Albers, K. B., Prado, C. M., Alexeeff, S., et al. (2019). Body composition, adherence to anthracycline and taxane-based chemotherapy, and survival after nonmetastatic breast cancer. JAMA Oncol. 6, 264-270. doi: 10.1001/jamaoncol.2019.4668
Chacon-Cabrera, A., Fermoselle, C., Urtreger, A. J., Mateu-Jimenez, M., Diament, M. J., de Kier Joffé, E. D. B., et al. (2014). Pharmacological strategies in lung cancer-induced cachexia: effects on muscle proteolysis, autophagy, structure, and weakness. J. Cell. Physiol. 229, 1660–1672. doi: 10.1002/jcp.24611
Chacon-Cabrera, A., Mateu-Jimenez, M., Langohr, K., Fermoselle, C., García-Arumí, E., Andreu, A. L., et al. (2017). Role of PARP activity in lung cancer-induced cachexia: effects on muscle oxidative stress, proteolysis, anabolic markers, and phenotype. J. Cell. Physiol. 232, 3744–3761. doi: 10.1002/jcp.25851
Cheema, N., Herbst, A., McKenzie, D., and Aiken, J. M. (2015). Apoptosis and necrosis mediate skeletal muscle fiber loss in age-induced mitochondrial enzymatic abnormalities. Aging Cell 14, 1085–1093. doi: 10.1111/acel.12399
Chen, J. L., Walton, K. L., Qian, H., Colgan, T. D., Hagg, A., Watt, M. J., et al. (2016). Differential effects of IL6 and activin a in the development of cancer-associated cachexia. Cancer Res. 76, 5372–5382. doi: 10.1158/0008-5472.CAN-15-3152
Chen, L., Chen, L., Wan, L., Huo, Y., Huang, J., Li, J., et al. (2019). Matrine improves skeletal muscle atrophy by inhibiting E3 ubiquitin ligases and activating the Akt/mTOR/FoxO3α signaling pathway in C2C12 myotubes and mice. Oncol. Rep. 42, 479–494. doi: 10.3892/or.2019.7205
Chen, M. C., Hsu, W.-L., and Chou, T.-C. (2018). Anti-cachectic effect of Antrodia cinnamomea extract in lung tumor-bearing mice under chemotherapy. Oncotarget 9, 19584–19596. doi: 10.18632/oncotarget.24680
Chen, M. C., Hsu, W.-L., Hwang, P.-A., Chen, Y.-L., and Chou, T.-C. (2016). Combined administration of fucoidan ameliorates tumor and chemotherapy-induced skeletal muscle atrophy in bladder cancer-bearing mice. Oncotarget 7, 51608–51618. doi: 10.18632/oncotarget.9958
Chen, T., Li, B., Xu, Y., Meng, S., Wang, Y., and Jiang, Y. (2018). Luteolin reduces cancer-induced skeletal and cardiac muscle atrophy in a Lewis lung cancer mouse model. Oncol. Rep. 40, 1129–1137. doi: 10.3892/or.2018.6453
Cho, U., Park, H. S., Im, S. Y., Yoo, C. Y., Jung, J. H., Suh, Y. J., et al. (2018). Prognostic value of systemic inflammatory markers and development of a nomogram in breast cancer. PLoS One 13:e0200936. doi: 10.1371/journal.pone.0200936
Chopard, A., Hillock, S., and Jasmin, B. J. (2009). Molecular events and signalling pathways involved in skeletal muscle disuse-induced atrophy and the impact of countermeasures. J. Cell. Mol. Med. 13, 3032–3050. doi: 10.1111/j.1582-4934.2009.00864.x
Coletti, D., Aulino, P., Pigna, E., Barteri, F., Moresi, V., Annibali, D., et al. (2016). Spontaneous physical activity downregulates Pax7 in cancer cachexia. Stem Cells Int. 2016:6729268. doi: 10.1155/2016/6729268
Constantinou, C., Fontes de Oliveira, C. C., Mintzopoulos, D., Busquets, S., He, J., Kesarwani, M., et al. (2011). Nuclear magnetic resonance in conjunction with functional genomics suggests mitochondrial dysfunction in a murine model of cancer cachexia. Int. J. Mol. Med. 27, 15–24. doi: 10.3892/ijmm.2010.557
Costamagna, D., Duelen, R., Penna, F., Neumann, D., Costelli, P., and Sampaolesi, M. (2020). Interleukin-4 administration improves muscle function, adult myogenesis, and lifespan of colon carcinoma-bearing mice. J. Cachexia Sarcopenia Muscle 11, 783–801. doi: 10.1002/jcsm.12539
Costelli, P., Carbó, N., Tessitore, L., Bagby, G. J., Lopez-Soriano, F. J., Argilés, J. M., et al. (1993). Tumor necrosis factor-alpha mediates changes in tissue protein turnover in a rat cancer cachexia model. J. Clin. Invest. 92, 2783–2789. doi: 10.1172/JCI116897
Costelli, P., Muscaritoli, M., Bonetto, A., Penna, F., Reffo, P., Bossola, M., et al. (2008). Muscle myostatin signalling is enhanced in experimental cancer cachexia. Eur. J. Clin. Invest. 38, 531–538. doi: 10.1111/j.1365-2362.2008.01970.x
Crouch, M.-L., Knowels, G., Stuppard, R., Ericson, N. G., Bielas, J. H., Marcinek, D. J., et al. (2017). Cyclophosphamide leads to persistent deficits in physical performance and in vivo mitochondria function in a mouse model of chemotherapy late effects. PLoS One 12:e0181086. doi: 10.1371/journal.pone.0181086
Cruz, B., Oliveira, A., Ventrucci, G., and Gomes-Marcondes, M. C. C. (2019). A leucine-rich diet modulates the mTOR cell signalling pathway in the gastrocnemius muscle under different Walker-256 tumour growth conditions. BMC Cancer 19:349. doi: 10.1186/s12885-019-5448-0
Cruz-Jentoft, A. J., Bahat, G., Bauer, J., Boirie, Y., Bruyère, O., Cederholm, T., et al. (2019). Sarcopenia: revised European consensus on definition and diagnosis. Age Ageing 48, 16–31. doi: 10.1093/ageing/afy169
D’Lugos, A. C., Fry, C. S., Ormsby, J. C., Sweeney, K. R., Brightwell, C. R., Hale, T. M., et al. (2019). Chronic doxorubicin administration impacts satellite cell and capillary abundance in a muscle-specific manner. Physiol. Rep. 7:e14052. doi: 10.14814/phy2.14052
Daly, L. E., Bhuachalla, N., Ní Bhuachalla, ÉB., Power, D. G., Cushen, S. J., James, K., et al. (2018). Loss of skeletal muscle during systemic chemotherapy is prognostic of poor survival in patients with foregut cancer. J. Cachexia Sarcopenia Muscle 9, 315–325. doi: 10.1002/jcsm.12267
Damrauer, J. S., Stadler, M. E., Acharyya, S., Baldwin, A. S., Couch, M. E., and Guttridge, D. C. (2018). Chemotherapy-induced muscle wasting: association with NF-κB and cancer cachexia. Eur. J. Transl. Myol. 28:7590. doi: 10.4081/ejtm.2018.7590
de Castro, G. S., Simoes, E., Lima, J. D. C. C., Ortiz-Silva, M., Festuccia, W. T., Tokeshi, F., et al. (2019). Human cachexia induces changes in mitochondria, autophagy and apoptosis in the skeletal muscle. Cancers 11:1264. doi: 10.3390/cancers11091264
De Groef, A., Geraerts, I., Demeyer, H., Van der Gucht, E., Dams, L., de Kinkelder, C., et al. (2018). Physical activity levels after treatment for breast cancer: two-year follow-up. Breast Edinb. Scotl. 40, 23–28. doi: 10.1016/j.breast.2018.04.009
de Lima Junior, E. A., Yamashita, A. S., Pimentel, G. D., De Sousa, L. G. O., Santos, R. V. T., Gonçalves, C. L., et al. (2016). Doxorubicin caused severe hyperglycaemia and insulin resistance, mediated by inhibition in AMPk signalling in skeletal muscle. J. Cachexia Sarcopenia Muscle 7, 615–625. doi: 10.1002/jcsm.12104
DeJong, C. H. C., Busquets, S., Moses, A. G. W., Schrauwen, P., Ross, J. A., Argiles, J. M., et al. (2005). Systemic inflammation correlates with increased expression of skeletal muscle ubiquitin but not uncoupling proteins in cancer cachexia. Oncol. Rep. 14, 257–263.
Delmonico, M. J., Harris, T. B., Visser, M., Park, S. W., Conroy, M. B., Velasquez-Mieyer, P., et al. (2009). Longitudinal study of muscle strength, quality, and adipose tissue infiltration. Am. J. Clin. Nutr. 90, 1579–1585. doi: 10.3945/ajcn.2009.28047
Deluche, E., Leobon, S., Desport, J. C., Venat-Bouvet, L., Usseglio, J., and Tubiana-Mathieu, N. (2018). Impact of body composition on outcome in patients with early breast cancer. Support. Care Cancer 26, 861–868. doi: 10.1007/s00520-017-3902-6
di Prampero, P. E., and Narici, M. V. (2003). Muscles in microgravity: from fibres to human motion. J. Biomech. 36, 403–412. doi: 10.1016/s0021-9290(02)00418-9
Dolly, A., Dumas, J.-F., and Servais, S. (2020). Cancer cachexia and skeletal muscle atrophy in clinical studies: what do we really know? J. Cachexia Sarcopenia Muscle 11, 1413–1428. doi: 10.1002/jcsm.12633
Dupont-Versteegden, E. E. (2005). Apoptosis in muscle atrophy: relevance to sarcopenia. Exp. Gerontol. 40, 473–481. doi: 10.1016/j.exger.2005.04.003
Dworzak, F., Ferrari, P., Gavazzi, C., Maiorana, C., and Bozzetti, F. (1998). Effects of cachexia due to cancer on whole body and skeletal muscle protein turnover. Cancer 82, 42–48.
Emens, L. A. (2018). Breast cancer immunotherapy: facts and hopes. Clin. Cancer Res. Off. J. Am. Assoc. Cancer Res. 24, 511–520. doi: 10.1158/1078-0432.CCR-16-3001
Emery, P. W., Edwards, R. H., Rennie, M. J., Souhami, R. L., and Halliday, D. (1984). Protein synthesis in muscle measured in vivo in cachectic patients with cancer. Br. Med. J. Clin. Res. Ed 289, 584–586. doi: 10.1136/bmj.289.6445.584
Fearon, K., Strasser, F., Anker, S. D., Bosaeus, I., Bruera, E., Fainsinger, R. L., et al. (2011). Definition and classification of cancer cachexia: an international consensus. Lancet Oncol. 12, 489–495. doi: 10.1016/S1470-2045(10)70218-7
Fermoselle, C., García-Arumí, E., Puig-Vilanova, E., Andreu, A. L., Urtreger, A. J., de Kier Joffé, E. D. B., et al. (2013). Mitochondrial dysfunction and therapeutic approaches in respiratory and limb muscles of cancer cachectic mice. Exp. Physiol. 98, 1349–1365. doi: 10.1113/expphysiol.2013.072496
Ferreira, R., Neuparth, M. J., Vitorino, R., Appell, H. J., Amado, F., and Duarte, J. A. (2008). Evidences of apoptosis during the early phases of soleus muscle atrophy in hindlimb suspended mice. Physiol. Res. 57, 601–611. doi: 10.33549/physiolres.931272
Figueras, M., Busquets, S., Carbó, N., Barreiro, E., Almendro, V., Argilés, J. M., et al. (2004). Interleukin-15 is able to suppress the increased DNA fragmentation associated with muscle wasting in tumour-bearing rats. FEBS Lett. 569, 201–206. doi: 10.1016/j.febslet.2004.05.066
Fisusi, F. A., and Akala, E. O. (2019). Drug combinations in breast cancer therapy. Pharm. Nanotechnol. 7, 3–23. doi: 10.2174/2211738507666190122111224
Fontes-Oliveira, C. C., Busquets, S., Toledo, M., Penna, F., Paz Aylwin, M., Sirisi, S., et al. (2013). Mitochondrial and sarcoplasmic reticulum abnormalities in cancer cachexia: altered energetic efficiency? Biochim. Biophys. Acta 1830, 2770–2778. doi: 10.1016/j.bbagen.2012.11.009
Fouladiun, M., Körner, U., Gunnebo, L., Sixt-Ammilon, P., Bosaeus, I., and Lundholm, K. (2007). Daily physical-rest activities in relation to nutritional state, metabolism, and quality of life in cancer patients with progressive cachexia. Clin. Cancer Res. Off. J. Am. Assoc. Cancer Res. 13, 6379–6385. doi: 10.1158/1078-0432.CCR-07-1147
Foulkes, S. J., Howden, E. J., Bigaran, A., Janssens, K., Antill, Y., Loi, S., et al. (2019). Persistent impairment in cardiopulmonary fitness after breast cancer chemotherapy. Med. Sci. Sports Exerc. 51, 1573–1581. doi: 10.1249/MSS.0000000000001970
Gadéa, E., Thivat, E., Dubray-Longeras, P., Arbre, M., Van-praagh, I., Mouret-Reynier, M.-A., et al. (2018). Prospective study on body composition, energy balance and biological factors changes in post-menopausal women with breast cancer receiving adjuvant chemotherapy including taxanes. Nutr. Cancer 70, 997–1006. doi: 10.1080/01635581.2018.1502330
Galiano-Castillo, N., Arroyo-Morales, M., Ariza-Garcia, A., Sánchez-Salado, C., Fernández-Lao, C., Cantarero-Villanueva, I., et al. (2016). The six-minute walk test as a measure of health in breast cancer patients. J. Aging Phys. Act. 24, 508–515. doi: 10.1123/japa.2015-0056
Gallagher, D., Heshka, S., Kelley, D. E., Thornton, J., Boxt, L., Pi-Sunyer, F. X., et al. (2014). Changes in adipose tissue depots and metabolic markers following a 1-year diet and exercise intervention in overweight and obese patients with type 2 diabetes. Diabetes Care 37, 3325–3332. doi: 10.2337/dc14-1585
Gallot, Y. S., and Bohnert, K. R. (2021). Confounding roles of ER stress and the unfolded protein response in skeletal muscle atrophy. Int. J. Mol. Sci. 22:2567. doi: 10.3390/ijms22052567
Gallot, Y. S., Bohnert, K. R., Straughn, A. R., Xiong, G., Hindi, S. M., and Kumar, A. (2019). PERK regulates skeletal muscle mass and contractile function in adult mice. FASEB J. Off. Publ. Fed. Am. Soc. Exp. Biol. 33, 1946–1962. doi: 10.1096/fj.201800683RR
Gallot, Y. S., Durieux, A.-C., Castells, J., Desgeorges, M. M., Vernus, B., Plantureux, L., et al. (2014). Myostatin gene inactivation prevents skeletal muscle wasting in cancer. Cancer Res. 74, 7344–7356. doi: 10.1158/0008-5472.CAN-14-0057
Gilliam, L. A. A., Fisher-Wellman, K. H., Lin, C.-T., Maples, J. M., Cathey, B. L., and Neufer, P. D. (2013). The anticancer agent doxorubicin disrupts mitochondrial energy metabolism and redox balance in skeletal muscle. Free Radic. Biol. Med. 65, 988–996. doi: 10.1016/j.freeradbiomed.2013.08.191
Gilliam, L. A. A., Lark, D. S., Reese, L. R., Torres, M. J., Ryan, T. E., Lin, C.-T., et al. (2016). Targeted overexpression of mitochondrial catalase protects against cancer chemotherapy-induced skeletal muscle dysfunction. Am. J. Physiol. Endocrinol. Metab. 311, E293–E301. doi: 10.1152/ajpendo.00540.2015
Gilliam, L. A. A., Moylan, J. S., Patterson, E. W., Smith, J. D., Wilson, A. S., Rabbani, Z., et al. (2012). Doxorubicin acts via mitochondrial ROS to stimulate catabolism in C2C12 myotubes. Am. J. Physiol. Cell Physiol. 302, C195–C202. doi: 10.1152/ajpcell.00217.2011
Gomes, M. D., Lecker, S. H., Jagoe, R. T., Navon, A., and Goldberg, A. L. (2001). Atrogin-1, a muscle-specific F-box protein highly expressed during muscle atrophy. Proc. Natl. Acad. Sci. U.S.A. 98, 14440–14445. doi: 10.1073/pnas.251541198
Goodpaster, B. H., Carlson, C. L., Visser, M., Kelley, D. E., Scherzinger, A., Harris, T. B., et al. (2001). Attenuation of skeletal muscle and strength in the elderly: the Health ABC Study. J. Appl. Physiol. Bethesda Md 1985 90, 2157–2165. doi: 10.1152/jappl.2001.90.6.2157
Goodpaster, B. H., Thaete, F. L., and Kelley, D. E. (2000). Composition of skeletal muscle evaluated with computed tomography. Ann. N. Y. Acad. Sci. 904, 18–24. doi: 10.1111/j.1749-6632.2000.tb06416.x
Gorgey, A. S., and Dudley, G. A. (2007). Skeletal muscle atrophy and increased intramuscular fat after incomplete spinal cord injury. Spinal Cord 45, 304–309. doi: 10.1038/sj.sc.3101968
Gouspillou, G., Scheede-Bergdahl, C., Spendiff, S., Vuda, M., Meehan, B., Mlynarski, H., et al. (2015). Anthracycline-containing chemotherapy causes long-term impairment of mitochondrial respiration and increased reactive oxygen species release in skeletal muscle. Sci. Rep. 5:8717. doi: 10.1038/srep08717
Guigni, B. A., Callahan, D. M., Tourville, T. W., Miller, M. S., Fiske, B., Voigt, T., et al. (2018). Skeletal muscle atrophy and dysfunction in breast cancer patients: role for chemotherapy-derived oxidant stress. Am. J. Physiol. Cell Physiol. 315, C744–C756. doi: 10.1152/ajpcell.00002.2018
Guo, B.-S., Cheung, K.-K., Yeung, S. S., Zhang, B.-T., and Yeung, E. W. (2012). Electrical stimulation influences satellite cell proliferation and apoptosis in unloading-induced muscle atrophy in mice. PLoS One 7:e30348. doi: 10.1371/journal.pone.0030348
Guo, D., Wang, C., Wang, Q., Qiao, Z., and Tang, H. (2017). Pantoprazole blocks the JAK2/STAT3 pathway to alleviate skeletal muscle wasting in cancer cachexia by inhibiting inflammatory response. Oncotarget 8, 39640–39648. doi: 10.18632/oncotarget.17387
Hanson, A. M., Harrison, B. C., Young, M. H., Stodieck, L. S., and Ferguson, V. L. (2013). Longitudinal characterization of functional, morphologic, and biochemical adaptations in mouse skeletal muscle with hindlimb suspension. Muscle Nerve 48, 393–402. doi: 10.1002/mus.23753
Hatakeyama, S., Summermatter, S., Jourdain, M., Melly, S., Minetti, G. C., and Lach-Trifilieff, E. (2016). ActRII blockade protects mice from cancer cachexia and prolongs survival in the presence of anti-cancer treatments. Skelet. Muscle 6:26. doi: 10.1186/s13395-016-0098-2
He, W. A., Berardi, E., Cardillo, V. M., Acharyya, S., Aulino, P., Thomas-Ahner, J., et al. (2013). NF-κB-mediated Pax7 dysregulation in the muscle microenvironment promotes cancer cachexia. J. Clin. Invest. 123, 4821–4835. doi: 10.1172/JCI68523
Hesse, E., Schröder, S., Brandt, D., Pamperin, J., Saito, H., and Taipaleenmäki, H. (2019). Sclerostin inhibition alleviates breast cancer-induced bone metastases and muscle weakness. JCI Insight 5:125543. doi: 10.1172/jci.insight.125543
Hiensch, A. E., Bolam, K. A., Mijwel, S., Jeneson, J. A. L., Huitema, A. D. R., Kranenburg, O., et al. (2019). Doxorubicin-induced skeletal muscle atrophy: elucidating the underlying molecular pathways. Acta Physiol. Oxf. Engl. 229:e13400. doi: 10.1111/apha.13400
Huh, J., Park, B., Lee, H., An, Y.-S., Jung, Y., Kim, J. Y., et al. (2020). Prognostic value of skeletal muscle depletion measured on computed tomography for overall survival in patients with non-metastatic breast cancer. J. Breast Cancer 23, 80–92. doi: 10.4048/jbc.2020.23.e8
Hulmi, J. J., Nissinen, T. A., Räsänen, M., Degerman, J., Lautaoja, J. H., Hemanthakumar, K. A., et al. (2018). Prevention of chemotherapy-induced cachexia by ACVR2B ligand blocking has different effects on heart and skeletal muscle. J. Cachexia Sarcopenia Muscle 9, 417–432. doi: 10.1002/jcsm.12265
Hulmi, J. J., Penna, F., Pöllänen, N., Nissinen, T. A., Hentilä, J., Euro, L., et al. (2020). Muscle NAD+ depletion and Serpina3n as molecular determinants of murine cancer cachexia-the effects of blocking myostatin and activins. Mol. Metab. 41:101046. doi: 10.1016/j.molmet.2020.101046
Huot, J. R., Pin, F., Narasimhan, A., Novinger, L. J., Keith, A. S., Zimmers, T. A., et al. (2020). ACVR2B antagonism as a countermeasure to multi-organ perturbations in metastatic colorectal cancer cachexia. J. Cachexia Sarcopenia Muscle 11, 1779–1798. doi: 10.1002/jcsm.12642
Hyatt, H. W., and Powers, S. K. (2021). Mitochondrial dysfunction is a common denominator linking skeletal muscle wasting due to disease, aging, and prolonged inactivity. Antioxid. Basel Switz. 10:588. doi: 10.3390/antiox10040588
Ieronimakis, N., Hays, A., Prasad, A., Janebodin, K., Duffield, J. S., and Reyes, M. (2016). PDGFRα signalling promotes fibrogenic responses in collagen-producing cells in Duchenne muscular dystrophy. J. Pathol. 240, 410–424. doi: 10.1002/path.4801
Inaba, S., Hinohara, A., Tachibana, M., Tsujikawa, K., and Fukada, S.-I. (2018). Muscle regeneration is disrupted by cancer cachexia without loss of muscle stem cell potential. PLoS One 13:e0205467. doi: 10.1371/journal.pone.0205467
Ishiko, O., Sumi, T., Yoshida, H., Hyun, Y., and Ogita, S. (2001). Expression of apoptosis regulatory proteins in the skeletal muscle of tumor-bearing rabbits compared with diet-restricted rabbits. Int. J. Mol. Med. 8, 279–283. doi: 10.3892/ijmm.8.3.279
Jerusalem, G., Lancellotti, P., and Kim, S.-B. (2019). HER2+ breast cancer treatment and cardiotoxicity: monitoring and management. Breast Cancer Res. Treat. 177, 237–250. doi: 10.1007/s10549-019-05303-y
Johns, N., Hatakeyama, S., Stephens, N. A., Degen, M., Degen, S., Frieauff, W., et al. (2014). Clinical classification of cancer cachexia: phenotypic correlates in human skeletal muscle. PLoS One 9:e83618. doi: 10.1371/journal.pone.0083618
Johns, N., Stretch, C., Tan, B. H. L., Solheim, T. S., Sørhaug, S., Stephens, N. A., et al. (2017). New genetic signatures associated with cancer cachexia as defined by low skeletal muscle index and weight loss. J. Cachexia Sarcopenia Muscle 8, 122–130. doi: 10.1002/jcsm.12138
Johnston, A. J., Murphy, K. T., Jenkinson, L., Laine, D., Emmrich, K., Faou, P., et al. (2015). Targeting of Fn14 prevents cancer-induced cachexia and prolongs survival. Cell 162, 1365–1378. doi: 10.1016/j.cell.2015.08.031
Jones, L. W., Hornsby, W. E., Goetzinger, A., Forbes, L. M., Sherrard, E. L., Quist, M., et al. (2012). Prognostic significance of functional capacity and exercise behavior in patients with metastatic non-small cell lung cancer. Lung Cancer 76, 248–252. doi: 10.1016/j.lungcan.2011.10.009
Jubrias, S. A., Odderson, I. R., Esselman, P. C., and Conley, K. E. (1997). Decline in isokinetic force with age: muscle cross-sectional area and specific force. Pflugers Arch. 434, 246–253. doi: 10.1007/s004240050392
Judge, S. M., Nosacka, R. L., Delitto, D., Gerber, M. H., Cameron, M. E., Trevino, J. G., et al. (2018). Skeletal muscle fibrosis in pancreatic cancer patients with respect to survival. JNCI Cancer Spectr. 2:ky043. doi: 10.1093/jncics/pky043
Julienne, C. M., Dumas, J.-F., Goupille, C., Pinault, M., Berri, C., Collin, A., et al. (2012). Cancer cachexia is associated with a decrease in skeletal muscle mitochondrial oxidative capacities without alteration of ATP production efficiency. J. Cachexia Sarcopenia Muscle 3, 265–275. doi: 10.1007/s13539-012-0071-9
Karampinos, D. C., Baum, T., Nardo, L., Alizai, H., Yu, H., Carballido-Gamio, J., et al. (2012). Characterization of the regional distribution of skeletal muscle adipose tissue in type 2 diabetes using chemical shift-based water/fat separation. J. Magn. Reson. Imaging JMRI 35, 899–907. doi: 10.1002/jmri.23512
Kavazis, A. N., Smuder, A. J., and Powers, S. K. (2014). Effects of short-term endurance exercise training on acute doxorubicin-induced FoxO transcription in cardiac and skeletal muscle. J. Appl. Physiol. Bethesda Md 1985 117, 223–230. doi: 10.1152/japplphysiol.00210.2014
Kawanishi, N., Funakoshi, T., and Machida, S. (2018). Time-course study of macrophage infiltration and inflammation in cast immobilization-induced atrophied muscle of mice. Muscle Nerve 57, 1006–1013. doi: 10.1002/mus.26061
Kazemi-Bajestani, S. M. R., Becher, H., Fassbender, K., Chu, Q., and Baracos, V. E. (2014). Concurrent evolution of cancer cachexia and heart failure: bilateral effects exist. J. Cachexia Sarcopenia Muscle 5, 95–104. doi: 10.1007/s13539-014-0137-y
Keenan, T. E., and Tolaney, S. M. (2020). Role of immunotherapy in triple-negative breast cancer. J. Natl. Compr. Cancer Netw. JNCCN 18, 479–489. doi: 10.6004/jnccn.2020.7554
Khal, J., Hine, A. V., Fearon, K. C. H., Dejong, C. H. C., and Tisdale, M. J. (2005). Increased expression of proteasome subunits in skeletal muscle of cancer patients with weight loss. Int. J. Biochem. Cell Biol. 37, 2196–2206. doi: 10.1016/j.biocel.2004.10.017
Khan, A. I., Reiter, D. A., Sekhar, A., Sharma, P., Safdar, N. M., Patil, D. H., et al. (2019). MRI quantitation of abdominal skeletal muscle correlates with CT-based analysis: implications for sarcopenia measurement. Appl. Physiol. Nutr. Metab. Physiol. Appl. Nutr. Metab. 44, 814–819. doi: 10.1139/apnm-2018-0473
Klassen, O., Schmidt, M. E., Ulrich, C. M., Schneeweiss, A., Potthoff, K., Steindorf, K., et al. (2017). Muscle strength in breast cancer patients receiving different treatment regimes. J. Cachexia Sarcopenia Muscle 8, 305–316. doi: 10.1002/jcsm.12165
Klionsky, D. J., Abdel-Aziz, A. K., Abdelfatah, S., Abdellatif, M., Abdoli, A., Abel, S., et al. (2021). Guidelines for the use and interpretation of assays for monitoring autophagy (4th edition). Autophagy 17, 1–382. doi: 10.1080/15548627.2020.1797280
Kunzke, T., Buck, A., Prade, V. M., Feuchtinger, A., Prokopchuk, O., Martignoni, M. E., et al. (2020). Derangements of amino acids in cachectic skeletal muscle are caused by mitochondrial dysfunction. J. Cachexia Sarcopenia Muscle 11, 226–240. doi: 10.1002/jcsm.12498
Larsen, S., Nielsen, J., Hansen, C. N., Nielsen, L. B., Wibrand, F., Stride, N., et al. (2012). Biomarkers of mitochondrial content in skeletal muscle of healthy young human subjects. J. Physiol. 590, 3349–3360. doi: 10.1113/jphysiol.2012.230185
Larsson, L., Degens, H., Li, M., Salviati, L., Lee, Y. I., Thompson, W., et al. (2019). Sarcopenia: aging-related loss of muscle mass and function. Physiol. Rev. 99, 427–511. doi: 10.1152/physrev.00061.2017
Lecker, S. H., Jagoe, R. T., Gilbert, A., Gomes, M., Baracos, V., Bailey, J., et al. (2004). Multiple types of skeletal muscle atrophy involve a common program of changes in gene expression. FASEB J. Off. Publ. Fed. Am. Soc. Exp. Biol. 18, 39–51. doi: 10.1096/fj.03-0610com
Lee, B. M., Cho, Y., Kim, J. W., Ahn, S. G., Kim, J. H., Jeung, H. C., et al. (2021). Association between skeletal muscle loss and the response to neoadjuvant chemotherapy for breast cancer. Cancers 13:1806. doi: 10.3390/cancers13081806
Lee, H., Heo, J.-W., Kim, A.-R., Kweon, M., Nam, S., Lim, J.-S., et al. (2019). Z-ajoene from crushed garlic alleviates cancer-induced skeletal muscle atrophy. Nutrients 11:2724. doi: 10.3390/nu11112724
Leskinen, T., Sipilä, S., Alen, M., Cheng, S., Pietiläinen, K. H., Usenius, J.-P., et al. (2009). Leisure-time physical activity and high-risk fat: a longitudinal population-based twin study. Int. J. Obes. 2005 33, 1211–1218. doi: 10.1038/ijo.2009.170
Leto, G., Incorvaia, L., Badalamenti, G., Tumminello, F. M., Gebbia, N., Flandina, C., et al. (2006). Activin A circulating levels in patients with bone metastasis from breast or prostate cancer. Clin. Exp. Metastasis 23, 117–122. doi: 10.1007/s10585-006-9010-5
Levolger, S., Wiemer, E. A. C., van Vugt, J. L. A., Huisman, S. A., van Vledder, M. G., van Damme-van Engel, S., et al. (2019). Inhibition of activin-like kinase 4/5 attenuates cancer cachexia associated muscle wasting. Sci. Rep. 9:9826. doi: 10.1038/s41598-019-46178-9
Lexell, J., Taylor, C. C., and Sjöström, M. (1988). What is the cause of the ageing atrophy? Total number, size and proportion of different fiber types studied in whole vastus lateralis muscle from 15- to 83-year-old men. J. Neurol. Sci. 84, 275–294. doi: 10.1016/0022-510x(88)90132-3
Liu, C.-M., Yang, Z., Liu, C.-W., Wang, R., Tien, P., Dale, R., et al. (2008). Myostatin antisense RNA-mediated muscle growth in normal and cancer cachexia mice. Gene Ther. 15, 155–160. doi: 10.1038/sj.gt.3303016
Liu, D., Qiao, X., Ge, Z., Shang, Y., Li, Y., Wang, W., et al. (2019). IMB0901 inhibits muscle atrophy induced by cancer cachexia through MSTN signaling pathway. Skelet. Muscle 9:8. doi: 10.1186/s13395-019-0193-2
Lønbro, S., Farup, J., Bentsen, S., Voss, T., Rittig, N., Wang, J., et al. (2017). Lean body mass, muscle fibre size and muscle function in cancer patients during chemotherapy and 10 weeks exercise. JCSM Clin. Rep. 2, 1–15. doi: 10.17987/jcsm-cr.v2i1.26
Loumaye, A., de Barsy, M., Nachit, M., Lause, P., Frateur, L., van Maanen, A., et al. (2015). Role of Activin A and myostatin in human cancer cachexia. J. Clin. Endocrinol. Metab. 100, 2030–2038. doi: 10.1210/jc.2014-4318
Loumaye, A., de Barsy, M., Nachit, M., Lause, P., van Maanen, A., Trefois, P., et al. (2017). Circulating Activin A predicts survival in cancer patients. J. Cachexia Sarcopenia Muscle 8, 768–777. doi: 10.1002/jcsm.12209
Madaro, L., Passafaro, M., Sala, D., Etxaniz, U., Lugarini, F., Proietti, D., et al. (2018). Denervation-activated STAT3-IL-6 signalling in fibro-adipogenic progenitors promotes myofibres atrophy and fibrosis. Nat. Cell Biol. 20, 917–927. doi: 10.1038/s41556-018-0151-y
Mallard, J., Hucteau, E., Schott, R., Petit, T., Demarchi, M., Belletier, C., et al. (2020). Evolution of physical status from diagnosis to the end of first-line treatment in breast, lung, and colorectal cancer patients: the PROTECT-01 cohort study protocol. Front. Oncol. 10:1304. doi: 10.3389/fonc.2020.01304
Manini, T. M., Clark, B. C., Nalls, M. A., Goodpaster, B. H., Ploutz-Snyder, L. L., and Harris, T. B. (2007). Reduced physical activity increases intermuscular adipose tissue in healthy young adults. Am. J. Clin. Nutr. 85, 377–384. doi: 10.1093/ajcn/85.2.377
Marcus, R. L., Addison, O., Kidde, J. P., Dibble, L. E., and Lastayo, P. C. (2010). Skeletal muscle fat infiltration: impact of age, inactivity, and exercise. J. Nutr. Health Aging 14, 362–366. doi: 10.1007/s12603-010-0081-2
Marques, V. A., Ferreira-Junior, J. B., Lemos, T. V., Moraes, R. F., Junior, J. R., de, S., et al. (2020). Effects of chemotherapy treatment on muscle strength, quality of life, fatigue, and anxiety in women with breast cancer. Int. J. Environ. Res. Public. Health 17:7289. doi: 10.3390/ijerph17197289
Martin, L., Senesse, P., Gioulbasanis, I., Antoun, S., Bozzetti, F., Deans, C., et al. (2015). Diagnostic criteria for the classification of cancer-associated weight loss. J. Clin. Oncol. Off. J. Am. Soc. Clin. Oncol. 33, 90–99. doi: 10.1200/JCO.2014.56.1894
Marzetti, E., Lorenzi, M., Landi, F., Picca, A., Rosa, F., Tanganelli, F., et al. (2017). Altered mitochondrial quality control signaling in muscle of old gastric cancer patients with cachexia. Exp. Gerontol. 87, 92–99. doi: 10.1016/j.exger.2016.10.003
Mason, M. C., Garcia, J. M., Sansgiry, S., Walder, A., Berger, D. H., and Anaya, D. A. (2016). Preoperative cancer cachexia and short-term outcomes following surgery. J. Surg. Res. 205, 398–406. doi: 10.1016/j.jss.2016.06.076
Matsuyama, T., Ishikawa, T., Okayama, T., Oka, K., Adachi, S., Mizushima, K., et al. (2015). Tumor inoculation site affects the development of cancer cachexia and muscle wasting. Int. J. Cancer 137, 2558–2565. doi: 10.1002/ijc.29620
Maughan, K. L., Lutterbie, M. A., and Ham, P. S. (2010). Treatment of breast cancer. Am. Fam. Physician 81, 1339–1346.
Mauro, A. (1961). Satellite cell of skeletal muscle fibers. J. Biophys. Biochem. Cytol. 9, 493–495. doi: 10.1083/jcb.9.2.493
McLean, J. B., Moylan, J. S., and Andrade, F. H. (2014). Mitochondria dysfunction in lung cancer-induced muscle wasting in C2C12 myotubes. Front. Physiol. 5:503. doi: 10.3389/fphys.2014.00503
McLoon, L. K., Falkenberg, J. H., Dykstra, D., and Iaizzo, P. A. (1998). Doxorubicin chemomyectomy as a treatment for cervical dystonia: histological assessment after direct injection into the sternocleidomastoid muscle. Muscle Nerve 21, 1457–1464.
Mehl, K. A., Davis, J. M., Berger, F. G., and Carson, J. A. (2005). Myofiber degeneration/regeneration is induced in the cachectic ApcMin/+ mouse. J. Appl. Physiol. Bethesda Md 1985 99, 2379–2387. doi: 10.1152/japplphysiol.00778.2005
Mijwel, S., Backman, M., Bolam, K. A., Olofsson, E., Norrbom, J., Bergh, J., et al. (2018a). Highly favorable physiological responses to concurrent resistance and high-intensity interval training during chemotherapy: the OptiTrain breast cancer trial. Breast Cancer Res. Treat. 169, 93–103. doi: 10.1007/s10549-018-4663-8
Mijwel, S., Cardinale, D. A., Norrbom, J., Chapman, M., Ivarsson, N., Wengström, Y., et al. (2018b). Exercise training during chemotherapy preserves skeletal muscle fiber area, capillarization, and mitochondrial content in patients with breast cancer. FASEB J. 32, 5495–5505. doi: 10.1096/fj.201700968R
Min, K., Kwon, O.-S., Smuder, A. J., Wiggs, M. P., Sollanek, K. J., Christou, D. D., et al. (2015). Increased mitochondrial emission of reactive oxygen species and calpain activation are required for doxorubicin-induced cardiac and skeletal muscle myopathy. J. Physiol. 593, 2017–2036. doi: 10.1113/jphysiol.2014.286518
Møller, A. B., Lønbro, S., Farup, J., Voss, T. S., Rittig, N., Wang, J., et al. (2019). Molecular and cellular adaptations to exercise training in skeletal muscle from cancer patients treated with chemotherapy. J. Cancer Res. Clin. Oncol. 145, 1449–1460. doi: 10.1007/s00432-019-02911-5
Montalvo, R. N., Doerr, V., Min, K., Szeto, H. H., and Smuder, A. J. (2020). Doxorubicin-induced oxidative stress differentially regulates proteolytic signaling in cardiac and skeletal muscle. Am. J. Physiol. Regul. Integr. Comp. Physiol. 318, R227–R233. doi: 10.1152/ajpregu.00299.2019
Moore-Carrasco, R., Busquets, S., Almendro, V., Palanki, M., López-Soriano, F. J., and Argilés, J. M. (2007). The AP-1/NF-kappaB double inhibitor SP100030 can revert muscle wasting during experimental cancer cachexia. Int. J. Oncol. 30, 1239–1245.
Moses, A. G. W., Maingay, J., Sangster, K., Fearon, K. C. H., and Ross, J. A. (2009). Pro-inflammatory cytokine release by peripheral blood mononuclear cells from patients with advanced pancreatic cancer: relationship to acute phase response and survival. Oncol. Rep. 21, 1091–1095. doi: 10.3892/or_00000328
Murphy, J. C., McDaniel, J. L., Mora, K., Villareal, D. T., Fontana, L., and Weiss, E. P. (2011). Preferential reductions in intermuscular and visceral adipose tissue with exercise-induced weight loss compared with calorie restriction. J. Appl. Physiol. 112, 79–85. doi: 10.1152/japplphysiol.00355.2011
Murton, A. J., Maddocks, M., Stephens, F. B., Marimuthu, K., England, R., and Wilcock, A. (2017). Consequences of late-stage non-small-cell lung cancer cachexia on muscle metabolic processes. Clin. Lung Cancer 18, e1–e11. doi: 10.1016/j.cllc.2016.06.003
National Cancer Institute, Surveillance, Epidemiology, and End Result program (2019). Cancer Stat Facts: Female Breast Cancer. Bethesda, MD: National Cancer Institute.
Neil, S. E., Klika, R. J., Garland, S. J., McKenzie, D. C., and Campbell, K. L. (2013). Cardiorespiratory and neuromuscular deconditioning in fatigued and non-fatigued breast cancer survivors. Support. Care Cancer Off. J. Multinatl. Assoc. Support. Care Cancer 21, 873–881. doi: 10.1007/s00520-012-1600-y
Neyroud, D., Nosacka, R. L., Judge, A. R., and Hepple, R. T. (2019). Colon 26 adenocarcinoma (C26)-induced cancer cachexia impairs skeletal muscle mitochondrial function and content. J. Muscle Res. Cell Motil. 40, 59–65. doi: 10.1007/s10974-019-09510-4
Nicolazzi, M. A., Carnicelli, A., Fuorlo, M., Scaldaferri, A., Masetti, R., Landolfi, R., et al. (2018). Anthracycline and trastuzumab-induced cardiotoxicity in breast cancer. Eur. Rev. Med. Pharmacol. Sci. 22, 2175–2185. doi: 10.26355/eurrev_201804_14752
Nissinen, T. A., Degerman, J., Räsänen, M., Poikonen, A. R., Koskinen, S., Mervaala, E., et al. (2016). Systemic blockade of ACVR2B ligands prevents chemotherapy-induced muscle wasting by restoring muscle protein synthesis without affecting oxidative capacity or atrogenes. Sci. Rep. 6:32695. doi: 10.1038/srep32695
Nissinen, T. A., Hentilä, J., Penna, F., Lampinen, A., Lautaoja, J. H., Fachada, V., et al. (2018). Treating cachexia using soluble ACVR2B improves survival, alters mTOR localization, and attenuates liver and spleen responses. J. Cachexia Sarcopenia Muscle 9, 514–529. doi: 10.1002/jcsm.12310
Ojima, C., Noguchi, Y., Miyamoto, T., Saito, Y., Orihashi, H., Yoshimatsu, Y., et al. (2020). Peptide-2 from mouse myostatin precursor protein alleviates muscle wasting in cancer-associated cachexia. Cancer Sci. 111, 2954–2964. doi: 10.1111/cas.14520
Op den Kamp, C. M., Gosker, H. R., Lagarde, S., Tan, D. Y., Snepvangers, F. J., Dingemans, A.-M. C., et al. (2015). Preserved muscle oxidative metabolic phenotype in newly diagnosed non-small cell lung cancer cachexia. J. Cachexia Sarcopenia Muscle 6, 164–173. doi: 10.1002/jcsm.12007
Op den Kamp, C. M., Langen, R. C., Minnaard, R., Kelders, M. C., Snepvangers, F. J., Hesselink, M. K., et al. (2012). Pre-cachexia in patients with stages I–III non-small cell lung cancer: systemic inflammation and functional impairment without activation of skeletal muscle ubiquitin proteasome system. Lung Cancer 76, 112–117. doi: 10.1016/j.lungcan.2011.09.012
Op den Kamp, C. M., Langen, R. C., Snepvangers, F. J., de Theije, C. C., Schellekens, J. M., Laugs, F., et al. (2013). Nuclear transcription factor κ B activation and protein turnover adaptations in skeletal muscle of patients with progressive stages of lung cancer cachexia. Am. J. Clin. Nutr. 98, 738–748. doi: 10.3945/ajcn.113.058388
Padrão, A. I., Oliveira, P., Vitorino, R., Colaço, B., Pires, M. J., Márquez, M., et al. (2013). Bladder cancer-induced skeletal muscle wasting: disclosing the role of mitochondria plasticity. Int. J. Biochem. Cell Biol. 45, 1399–1409. doi: 10.1016/j.biocel.2013.04.014
Pagano, A. F., Brioche, T., Arc-Chagnaud, C., Demangel, R., Chopard, A., and Py, G. (2018). Short-term disuse promotes fatty acid infiltration into skeletal muscle. J. Cachexia Sarcopenia Muscle 9, 335–347. doi: 10.1002/jcsm.12259
Peel, A. B., Thomas, S. M., Dittus, K., Jones, L. W., and Lakoski, S. G. (2014). Cardiorespiratory fitness in breast cancer patients: a call for normative values. J. Am. Heart Assoc. 3:e000432. doi: 10.1161/JAHA.113.000432
Penna, F., Baccino, F. M., and Costelli, P. (2014). Coming back: autophagy in cachexia. Curr. Opin. Clin. Nutr. Metab. Care 17, 241–246. doi: 10.1097/MCO.0000000000000048
Penna, F., Ballarò, R., Beltrà, M., De Lucia, S., García Castillo, L., and Costelli, P. (2019a). The skeletal muscle as an active player against cancer cachexia. Front. Physiol. 10:41. doi: 10.3389/fphys.2019.00041
Penna, F., Ballarò, R., Martinez-Cristobal, P., Sala, D., Sebastian, D., Busquets, S., et al. (2019b). Autophagy exacerbates muscle wasting in cancer cachexia and impairs mitochondrial function. J. Mol. Biol. 431, 2674–2686. doi: 10.1016/j.jmb.2019.05.032
Penna, F., Costamagna, D., Fanzani, A., Bonelli, G., Baccino, F. M., and Costelli, P. (2010). Muscle wasting and impaired myogenesis in tumor bearing mice are prevented by ERK inhibition. PLoS One 5:e13604. doi: 10.1371/journal.pone.0013604
Penna, F., Costamagna, D., Pin, F., Camperi, A., Fanzani, A., Chiarpotto, E. M., et al. (2013). Autophagic degradation contributes to muscle wasting in cancer cachexia. Am. J. Pathol. 182, 1367–1378. doi: 10.1016/j.ajpath.2012.12.023
Petruzzelli, M., and Wagner, E. F. (2016). Mechanisms of metabolic dysfunction in cancer-associated cachexia. Genes Dev. 30, 489–501. doi: 10.1101/gad.276733.115
Pettersen, K., Andersen, S., van der Veen, A., Nonstad, U., Hatakeyama, S., Lambert, C., et al. (2020). Autocrine activin A signalling in ovarian cancer cells regulates secretion of interleukin 6, autophagy, and cachexia. J. Cachexia Sarcopenia Muscle 11, 195–207. doi: 10.1002/jcsm.12489
Pigna, E., Berardi, E., Aulino, P., Rizzuto, E., Zampieri, S., Carraro, U., et al. (2016). Aerobic exercise and pharmacological treatments counteract cachexia by modulating autophagy in colon cancer. Sci. Rep. 6:26991. doi: 10.1038/srep26991
Pin, F., Barreto, R., Kitase, Y., Mitra, S., Erne, C. E., Novinger, L. J., et al. (2018). Growth of ovarian cancer xenografts causes loss of muscle and bone mass: a new model for the study of cancer cachexia. J. Cachexia Sarcopenia Muscle 9, 685–700. doi: 10.1002/jcsm.12311
Powers, S. K., Morton, A. B., Ahn, B., and Smuder, A. J. (2016). Redox control of skeletal muscle atrophy. Free Radic. Biol. Med. 98, 208–217. doi: 10.1016/j.freeradbiomed.2016.02.021
Puig-Vilanova, E., Rodriguez, D. A., Lloreta, J., Ausin, P., Pascual-Guardia, S., Broquetas, J., et al. (2015). Oxidative stress, redox signaling pathways, and autophagy in cachectic muscles of male patients with advanced COPD and lung cancer. Free Radic. Biol. Med. 79, 91–108. doi: 10.1016/j.freeradbiomed.2014.11.006
Puppa, M. J., Gao, S., Narsale, A. A., and Carson, J. A. (2014). Skeletal muscle glycoprotein 130’s role in Lewis lung carcinoma-induced cachexia. FASEB J. Off. Publ. Fed. Am. Soc. Exp. Biol. 28, 998–1009. doi: 10.1096/fj.13-240580
Quan-Jun, Y., Yan, H., Yong-Long, H., Li-Li, W., Jie, L., Jin-Lu, H., et al. (2017). Selumetinib attenuates skeletal muscle wasting in murine cachexia model through ERK inhibition and AKT activation. Mol. Cancer Ther. 16, 334–343. doi: 10.1158/1535-7163.MCT-16-0324
Ramos da Silva, B., Mialich, M. S., Cruz, L. P., Rufato, S., Gozzo, T., and Jordao, A. A. (2021). Performance of functionality measures and phase angle in women exposed to chemotherapy for early breast cancer. Clin. Nutr. ESPEN 42, 105–116. doi: 10.1016/j.clnesp.2021.02.007
Ranjbar, K., Ballarò, R., Bover, Q., Pin, F., Beltrà, M., Penna, F., et al. (2019). Combined exercise training positively affects muscle wasting in tumor-bearing mice. Med. Sci. Sports Exerc. 51, 1387–1395. doi: 10.1249/MSS.0000000000001916
Redden, M. H., and Fuhrman, G. M. (2013). Neoadjuvant chemotherapy in the treatment of breast cancer. Surg. Clin. North Am. 93, 493–499. doi: 10.1016/j.suc.2013.01.006
Reding, K. W., Brubaker, P., D’Agostino, R., Kitzman, D. W., Nicklas, B., Langford, D., et al. (2019). Increased skeletal intermuscular fat is associated with reduced exercise capacity in cancer survivors: a cross-sectional study. Cardio Oncol. 5:3. doi: 10.1186/s40959-019-0038-5
Rhoads, M. G., Kandarian, S. C., Pacelli, F., Doglietto, G. B., and Bossola, M. (2010). Expression of NF-kappaB and IkappaB proteins in skeletal muscle of gastric cancer patients. Eur. J. Cancer Oxf. Engl. 1990 46, 191–197. doi: 10.1016/j.ejca.2009.10.008
Riccardi, D. M., dos, R., das Neves, R. X., de Matos-Neto, E. M., Camargo, R. G., Lima, J. D. C. C., et al. (2020). Plasma lipid profile and systemic inflammation in patients with cancer cachexia. Front. Nutr. 7:4. doi: 10.3389/fnut.2020.00004
Rier, H. N., Jager, A., Sleijfer, S., Maier, A. B., and Levin, M.-D. (2016). The prevalence and prognostic value of low muscle mass in cancer patients: a review of the literature. The Oncologist 21, 1396–1409. doi: 10.1634/theoncologist.2016-0066
Rier, H. N., Jager, A., Sleijfer, S., van Rosmalen, J., Kock, M. C. J. M., and Levin, M.-D. (2018). Changes in body composition and muscle attenuation during taxane-based chemotherapy in patients with metastatic breast cancer. Breast Cancer Res. Treat. 168, 95–105. doi: 10.1007/s10549-017-4574-0
Roberts, B. M., Frye, G. S., Ahn, B., Ferreira, L. F., and Judge, A. R. (2013). Cancer cachexia decreases specific force and accelerates fatigue in limb muscle. Biochem. Biophys. Res. Commun. 435, 488–492. doi: 10.1016/j.bbrc.2013.05.018
Rochette, L., Guenancia, C., Gudjoncik, A., Hachet, O., Zeller, M., Cottin, Y., et al. (2015). Anthracyclines/trastuzumab: new aspects of cardiotoxicity and molecular mechanisms. Trends Pharmacol. Sci. 36, 326–348. doi: 10.1016/j.tips.2015.03.005
Rodriguez, J., Vernus, B., Chelh, I., Cassar-Malek, I., Gabillard, J. C., Hadj Sassi, A., et al. (2014). Myostatin and the skeletal muscle atrophy and hypertrophy signaling pathways. Cell. Mol. Life Sci. CMLS 71, 4361–4371. doi: 10.1007/s00018-014-1689-x
Roeland, E. J., Ma, J. D., Nelson, S. H., Seibert, T., Heavey, S., Revta, C., et al. (2017). Weight loss versus muscle loss: re-evaluating inclusion criteria for future cancer cachexia interventional trials. Support. Care Cancer 25, 365–369. doi: 10.1007/s00520-016-3402-0
Rossi, F., Torri, L., Lambertini, M., De Giorgis, S., Calabrese, M., and Tagliafico, A. S. (2020). Muscle mass loss after neoadjuvant chemotherapy in breast cancer: estimation on breast magnetic resonance imaging using pectoralis muscle area. Eur. Radiol. 30, 4234–4241. doi: 10.1007/s00330-020-06799-5
Rutten, I. J. G., van Dijk, D. P. J., Kruitwagen, R. F. P. M., Beets-Tan, R. G. H., Olde Damink, S. W. M., and van Gorp, T. (2016). Loss of skeletal muscle during neoadjuvant chemotherapy is related to decreased survival in ovarian cancer patients. J. Cachexia Sarcopenia Muscle 7, 458–466. doi: 10.1002/jcsm.12107
Ryan, Z. C., Craig, T. A., Wang, X., Delmotte, P., Salisbury, J. L., Lanza, I. R., et al. (2018). 1α,25-dihydroxyvitamin D3 mitigates cancer cell mediated mitochondrial dysfunction in human skeletal muscle cells. Biochem. Biophys. Res. Commun. 496, 746–752. doi: 10.1016/j.bbrc.2018.01.092
Salazar-Degracia, A., Blanco, D., Vilà-Ubach, M., de Biurrun, G., de Solórzano, C. O., Montuenga, L. M., et al. (2016). Phenotypic and metabolic features of mouse diaphragm and gastrocnemius muscles in chronic lung carcinogenesis: influence of underlying emphysema. J. Transl. Med. 14:244. doi: 10.1186/s12967-016-1003-9
Salazar-Degracia, A., Busquets, S., Argilés, J. M., Bargalló-Gispert, N., López-Soriano, F. J., and Barreiro, E. (2018). Effects of the beta2 agonist formoterol on atrophy signaling, autophagy, and muscle phenotype in respiratory and limb muscles of rats with cancer-induced cachexia. Biochimie 149, 79–91. doi: 10.1016/j.biochi.2018.04.009
Samuels, S. E., Knowles, A. L., Tilignac, T., Debiton, E., Madelmont, J. C., and Attaix, D. (2001). Higher skeletal muscle protein synthesis and lower breakdown after chemotherapy in cachectic mice. Am. J. Physiol. Regul. Integr. Comp. Physiol. 281, R133–R139. doi: 10.1152/ajpregu.2001.281.1.R133
Sandri, M. (2008). Signaling in muscle atrophy and hypertrophy. Physiol. Bethesda Md 23, 160–170. doi: 10.1152/physiol.00041.2007
Sartori, R., Romanello, V., and Sandri, M. (2021). Mechanisms of muscle atrophy and hypertrophy: implications in health and disease. Nat. Commun. 12:330. doi: 10.1038/s41467-020-20123-1
Schiaffino, S., Dyar, K. A., Ciciliot, S., Blaauw, B., and Sandri, M. (2013). Mechanisms regulating skeletal muscle growth and atrophy. FEBS J. 280, 4294–4314. doi: 10.1111/febs.12253
Schirrmacher, V. (2019). From chemotherapy to biological therapy: a review of novel concepts to reduce the side effects of systemic cancer treatment (Review). Int. J. Oncol. 54, 407–419. doi: 10.3892/ijo.2018.4661
Schmidt, T., Weisser, B., Dürkop, J., Jonat, W., Van Mackelenbergh, M., Röcken, C., et al. (2015). Comparing endurance and resistance training with standard care during chemotherapy for patients with primary breast cancer. Anticancer Res. 35, 5623–5629.
Schmitt, T. L., Martignoni, M. E., Bachmann, J., Fechtner, K., Friess, H., Kinscherf, R., et al. (2007). Activity of the Akt-dependent anabolic and catabolic pathways in muscle and liver samples in cancer-related cachexia. J. Mol. Med. Berl. Ger. 85, 647–654. doi: 10.1007/s00109-007-0177-2
Schumacher, A. N., Shackelford, D. Y. K., Brown, J. M., and Hayward, R. (2019). Validation of the 6-min walk test for predicting peak V⋅O2 in cancer survivors. Med. Sci. Sports Exerc. 51, 271–277. doi: 10.1249/MSS.0000000000001790
Schwarz, S., Prokopchuk, O., Esefeld, K., Gröschel, S., Bachmann, J., Lorenzen, S., et al. (2017). The clinical picture of cachexia: a mosaic of different parameters (experience of 503 patients). BMC Cancer 17:130. doi: 10.1186/s12885-017-3116-9
Schwarzkopf, M., Coletti, D., Sassoon, D., and Marazzi, G. (2006). Muscle cachexia is regulated by a p53–PW1/Peg3-dependent pathway. Genes Dev. 20, 3440–3452. doi: 10.1101/gad.412606
Scott, H. R., McMillan, D. C., Crilly, A., McArdle, C. S., and Milroy, R. (1996). The relationship between weight loss and interleukin 6 in non-small-cell lung cancer. Br. J. Cancer 73, 1560–1562. doi: 10.1038/bjc.1996.294
Shachar, S. S., Deal, A. M., Weinberg, M., Williams, G. R., Nyrop, K. A., Popuri, K., et al. (2017). Body composition as a predictor of toxicity in patients receiving anthracycline and taxane–based chemotherapy for early-stage breast cancer. Clin. Cancer Res. 23, 3537–3543. doi: 10.1158/1078-0432.CCR-16-2266
Shah, A. N., and Gradishar, W. J. (2018). Adjuvant anthracyclines in breast cancer: what is their role? The Oncologist 23, 1153–1161. doi: 10.1634/theoncologist.2017-0672
Shum, A. M. Y., Mahendradatta, T., Taylor, R. J., Painter, A. B., Moore, M. M., Tsoli, M., et al. (2012). Disruption of MEF2C signaling and loss of sarcomeric and mitochondrial integrity in cancer-induced skeletal muscle wasting. Aging 4, 133–143. doi: 10.18632/aging.100436
Sies, H., and Jones, D. P. (2020). Reactive oxygen species (ROS) as pleiotropic physiological signalling agents. Nat. Rev. Mol. Cell Biol. 21, 363–383. doi: 10.1038/s41580-020-0230-3
Silva, K. A. S., Dong, J., Dong, Y., Dong, Y., Schor, N., Tweardy, D. J., et al. (2015). Inhibition of Stat3 activation suppresses caspase-3 and the ubiquitin-proteasome system, leading to preservation of muscle mass in cancer cachexia. J. Biol. Chem. 290, 11177–11187. doi: 10.1074/jbc.M115.641514
Silva, S. P., Santos, J. M., Silva, M. P., Costa, R. M., and Medeiros, R. (2020). Cancer cachexia and its pathophysiology: links with sarcopenia, anorexia and asthenia. J. Cachexia Sarcopenia Muscle 11, 619–635. doi: 10.1002/jcsm.12528
Sirago, G., Conte, E., Fracasso, F., Cormio, A., Fehrentz, J.-A., Martinez, J., et al. (2017). Growth hormone secretagogues hexarelin and JMV2894 protect skeletal muscle from mitochondrial damages in a rat model of cisplatin-induced cachexia. Sci. Rep. 7:13017. doi: 10.1038/s41598-017-13504-y
Skipworth, R. J. E., Moses, A. G. W., Sangster, K., Sturgeon, C. M., Voss, A. C., Fallon, M. T., et al. (2011). Interaction of gonadal status with systemic inflammation and opioid use in determining nutritional status and prognosis in advanced pancreatic cancer. Support. Care Cancer Off. J. Multinatl. Assoc. Support. Care Cancer 19, 391–401. doi: 10.1007/s00520-010-0832-y
Skorokhod, A., Bachmann, J., Giese, N. A., Martignoni, M. E., and Krakowski-Roosen, H. (2012). Real-imaging cDNA-AFLP transcript profiling of pancreatic cancer patients: Egr-1 as a potential key regulator of muscle cachexia. BMC Cancer 12:265. doi: 10.1186/1471-2407-12-265
Smith, H. J., Greenberg, N. A., and Tisdale, M. J. (2004). Effect of eicosapentaenoic acid, protein and amino acids on protein synthesis and degradation in skeletal muscle of cachectic mice. Br. J. Cancer 91, 408–412. doi: 10.1038/sj.bjc.6601981
Smith, H. K., Maxwell, L., Martyn, J. A., and Bass, J. J. (2000). Nuclear DNA fragmentation and morphological alterations in adult rabbit skeletal muscle after short-term immobilization. Cell Tissue Res. 302, 235–241. doi: 10.1007/s004410000280
Smith, K. L., and Tisdale, M. J. (1993). Increased protein degradation and decreased protein synthesis in skeletal muscle during cancer cachexia. Br. J. Cancer 67, 680–685. doi: 10.1038/bjc.1993.126
Smuder, A. J., Kavazis, A. N., Min, K., and Powers, S. K. (2011). Exercise protects against doxorubicin-induced markers of autophagy signaling in skeletal muscle. J. Appl. Physiol. Bethesda Md 1985 111, 1190–1198. doi: 10.1152/japplphysiol.00429.2011
Song, M.-Y., Ruts, E., Kim, J., Janumala, I., Heymsfield, S., and Gallagher, D. (2004). Sarcopenia and increased adipose tissue infiltration of muscle in elderly African American women. Am. J. Clin. Nutr. 79, 874–880. doi: 10.1093/ajcn/79.5.874
Sorensen, J. C., Petersen, A. C., Timpani, C. A., Campelj, D. G., Cook, J., Trewin, A. J., et al. (2017). BGP-15 protects against oxaliplatin-induced skeletal myopathy and mitochondrial reactive oxygen species production in mice. Front. Pharmacol. 8:137. doi: 10.3389/fphar.2017.00137
Stock, M. S., and Thompson, B. J. (2021). Echo intensity as an indicator of skeletal muscle quality: applications, methodology, and future directions. Eur. J. Appl. Physiol. 121, 369–380. doi: 10.1007/s00421-020-04556-6
Straughn, A. R., Kelm, N. Q., and Kakar, S. S. (2021). Withaferin a and ovarian cancer antagonistically regulate skeletal muscle mass. Front. Cell Dev. Biol. 9:636498. doi: 10.3389/fcell.2021.636498
Sun, R., Zhang, S., Hu, W., Lu, X., Lou, N., Yang, Z., et al. (2016). Valproic acid attenuates skeletal muscle wasting by inhibiting C/EBPβ-regulated atrogin1 expression in cancer cachexia. Am. J. Physiol. Cell Physiol. 311, C101–C115. doi: 10.1152/ajpcell.00344.2015
Sung, H., Ferlay, J., Siegel, R. L., Laversanne, M., Soerjomataram, I., Jemal, A., et al. (2021). Global cancer statistics 2020: GLOBOCAN estimates of incidence and mortality worldwide for 36 cancers in 185 countries. CA. Cancer J. Clin. 71, 209–249. doi: 10.3322/caac.21660
Tarnopolsky, M. A., Pearce, E., Smith, K., and Lach, B. (2011). Suction-modified Bergström muscle biopsy technique: experience with 13,500 procedures. Muscle Nerve 43, 717–725. doi: 10.1002/mus.21945
Temparis, S., Asensi, M., Taillandier, D., Aurousseau, E., Larbaud, D., Obled, A., et al. (1994). Increased ATP-ubiquitin-dependent proteolysis in skeletal muscles of tumor-bearing rats. Cancer Res. 54, 5568–5573.
Theret, M., Rossi, F. M. V., and Contreras, O. (2021). Evolving roles of muscle-resident fibro-adipogenic progenitors in health, regeneration, neuromuscular disorders, and aging. Front. Physiol. 12:673404. doi: 10.3389/fphys.2021.673404
Toledo, M., Busquets, S., Penna, F., Zhou, X., Marmonti, E., Betancourt, A., et al. (2016). Complete reversal of muscle wasting in experimental cancer cachexia: additive effects of activin type II receptor inhibition and β-2 agonist. Int. J. Cancer 138, 2021–2029. doi: 10.1002/ijc.29930
Toth, M. J., Voigt, T. B., Tourville, T. W., Prior, S. M., Guigni, B. A., Schlosberg, A. V., et al. (2020). Effect of neuromuscular electrical stimulation on skeletal muscle size and function in patients with breast cancer receiving chemotherapy. J. Appl. Physiol. 128, 1654–1665. doi: 10.1152/japplphysiol.00203.2020
Trestini, I., Carbognin, L., Monteverdi, S., Zanelli, S., De Toma, A., Bonaiuto, C., et al. (2018). Clinical implication of changes in body composition and weight in patients with early-stage and metastatic breast cancer. Crit. Rev. Oncol. Hematol. 129, 54–66. doi: 10.1016/j.critrevonc.2018.06.011
Tsang, W. P., Chau, S. P. Y., Kong, S. K., Fung, K. P., and Kwok, T. T. (2003). Reactive oxygen species mediate doxorubicin induced p53-independent apoptosis. Life Sci. 73, 2047–2058. doi: 10.1016/s0024-3205(03)00566-6
Tuttle, L. J., Sinacore, D. R., Cade, W. T., and Mueller, M. J. (2011). Lower physical activity is associated with higher intermuscular adipose tissue in people with type 2 diabetes and peripheral neuropathy. Phys. Ther. 91, 923–930. doi: 10.2522/ptj.20100329
Tzika, A. A., Fontes-Oliveira, C. C., Shestov, A. A., Constantinou, C., Psychogios, N., Righi, V., et al. (2013). Skeletal muscle mitochondrial uncoupling in a murine cancer cachexia model. Int. J. Oncol. 43, 886–894. doi: 10.3892/ijo.2013.1998
Uezumi, A., Fukada, S., Yamamoto, N., Ikemoto-Uezumi, M., Nakatani, M., Morita, M., et al. (2014a). Identification and characterization of PDGFRα+ mesenchymal progenitors in human skeletal muscle. Cell Death Dis. 5:e1186. doi: 10.1038/cddis.2014.161
Uezumi, A., Ikemoto-Uezumi, M., and Tsuchida, K. (2014b). Roles of nonmyogenic mesenchymal progenitors in pathogenesis and regeneration of skeletal muscle. Front. Physiol. 5:68. doi: 10.3389/fphys.2014.00068
Uezumi, A., Ito, T., Morikawa, D., Shimizu, N., Yoneda, T., Segawa, M., et al. (2011). Fibrosis and adipogenesis originate from a common mesenchymal progenitor in skeletal muscle. J. Cell Sci. 124, 3654–3664. doi: 10.1242/jcs.086629
Urbina-Varela, R., Castillo, N., Videla, L. A., and Del Campo, A. (2020). Impact of mitophagy and mitochondrial unfolded protein response as new adaptive mechanisms underlying old pathologies: sarcopenia and non-alcoholic fatty liver disease. Int. J. Mol. Sci. 21:7704. doi: 10.3390/ijms21207704
Ushmorov, A., Hack, V., and Dröge, W. (1999). Differential reconstitution of mitochondrial respiratory chain activity and plasma redox state by cysteine and ornithine in a model of cancer cachexia. Cancer Res. 59, 3527–3534.
Vainshtein, A., and Sandri, M. (2020). Signaling pathways that control muscle mass. Int. J. Mol. Sci. 21:4759. doi: 10.3390/ijms21134759
van Waart, H., Stuiver, M. M., van Harten, W. H., Geleijn, E., Kieffer, J. M., Buffart, L. M., et al. (2015). Effect of low-intensity physical activity and moderate- to high-intensity physical exercise during adjuvant chemotherapy on physical fitness, fatigue, and chemotherapy completion rates: results of the PACES randomized clinical trial. J. Clin. Oncol. Off. J. Am. Soc. Clin. Oncol. 33, 1918–1927. doi: 10.1200/JCO.2014.59.1081
Varricchi, G., Ameri, P., Cadeddu, C., Ghigo, A., Madonna, R., Marone, G., et al. (2018). Antineoplastic drug-induced cardiotoxicity: a redox perspective. Front. Physiol. 9:167. doi: 10.3389/fphys.2018.00167
Visser, M., Goodpaster, B. H., Kritchevsky, S. B., Newman, A. B., Nevitt, M., Rubin, S. M., et al. (2005). Muscle mass, muscle strength, and muscle fat infiltration as predictors of incident mobility limitations in well-functioning older persons. J. Gerontol. A. Biol. Sci. Med. Sci. 60, 324–333. doi: 10.1093/gerona/60.3.324
Visser, M., Kritchevsky, S. B., Goodpaster, B. H., Newman, A. B., Nevitt, M., Stamm, E., et al. (2002). Leg muscle mass and composition in relation to lower extremity performance in men and women aged 70 to 79: the health, aging and body composition study. J. Am. Geriatr. Soc. 50, 897–904. doi: 10.1046/j.1532-5415.2002.50217.x
Wallengren, O., Lundholm, K., and Bosaeus, I. (2013). Diagnostic criteria of cancer cachexia: relation to quality of life, exercise capacity and survival in unselected palliative care patients. Support. Care Cancer Off. J. Multinatl. Assoc. Support. Care Cancer 21, 1569–1577. doi: 10.1007/s00520-012-1697-z
Wang, R., Kumar, B., Bhat-Nakshatri, P., Prasad, M. S., Jacobsen, M. H., Ovalle, G., et al. (2021). Aging-associated skeletal muscle defects in HER2/Neu transgenic mammary tumor model. JCSM Rapid Commun. 4, 24–39. doi: 10.1002/rco2.23
Warren, S. (1932). The immediate causes of death in cancer. Am. J. Med. Sci. 184, 610–615. doi: 10.1097/00000441-193211000-00002
Wesolowski, S., Orlowski, T. M., and Kram, M. (2020). The 6-min walk test in the functional evaluation of patients with lung cancer qualified for lobectomy. Interact. Cardiovasc. Thorac. Surg. 30, 559–564. doi: 10.1093/icvts/ivz313
White, J. P., Baynes, J. W., Welle, S. L., Kostek, M. C., Matesic, L. E., Sato, S., et al. (2011). The regulation of skeletal muscle protein turnover during the progression of cancer cachexia in the Apc(Min/+) mouse. PLoS One 6:e24650. doi: 10.1371/journal.pone.0024650
White, J. P., Puppa, M. J., Sato, S., Gao, S., Price, R. L., Baynes, J. W., et al. (2012). IL-6 regulation on skeletal muscle mitochondrial remodeling during cancer cachexia in the ApcMin/+ mouse. Skelet. Muscle 2:14. doi: 10.1186/2044-5040-2-14
Wiederin, J., Gu, C., Jewett, P., and Blaes, A. H. (2020). Pectoralis muscle wasting during chemotherapy. J. Clin. Oncol. 38:e24069. doi: 10.1200/JCO.2020.38.15_suppl.e24069
Williams, A., Sun, X., Fischer, J. E., and Hasselgren, P. O. (1999). The expression of genes in the ubiquitin-proteasome proteolytic pathway is increased in skeletal muscle from patients with cancer. Surgery 126, 744–749; discussion 749-750.
Willson, M. L., Burke, L., Ferguson, T., Ghersi, D., Nowak, A. K., and Wilcken, N. (2019). Taxanes for adjuvant treatment of early breast cancer. Cochrane Database Syst. Rev. 9:CD004421. doi: 10.1002/14651858.CD004421.pub3
Wilson, H. E., Rhodes, K. K., Rodriguez, D., Chahal, I., Stanton, D. A., Bohlen, J., et al. (2019). Human breast cancer Xenograft model implicates peroxisome proliferator-activated receptor signaling as driver of cancer-induced muscle fatigue. Clin. Cancer Res. Off. J. Am. Assoc. Cancer Res. 25, 2336–2347. doi: 10.1158/1078-0432.CCR-18-1565
Wilson, H. E., Stanton, D. A., and Pistilli, E. E. (2020). Breast cancer-associated skeletal muscle mitochondrial dysfunction and lipid accumulation is reversed by PPARG. BioRxiv [preprint]. doi: 10.1101/2020.04.05.026617
Wren, T. A. L., Bluml, S., Tseng-Ong, L., and Gilsanz, V. (2008). Three-point technique of fat quantification of muscle tissue as a marker of disease progression in Duchenne muscular dystrophy: preliminary study. AJR Am. J. Roentgenol. 190, W8–W12. doi: 10.2214/AJR.07.2732
Yildiz Kabak, V., Gursen, C., Aytar, A., Akbayrak, T., and Duger, T. (2020). Physical activity level, exercise behavior, barriers, and preferences of patients with breast cancer-related lymphedema. Support. Care Cancer Off. J. Multinatl. Assoc. Support. Care Cancer 29, 3593–3602. doi: 10.1007/s00520-020-05858-3
Yoshida, H., Ishiko, O., Sumi, T., Honda, K. I., Hirai, K., and Ogita, S. (2001). Expression of apoptosis regulatory proteins in the skeletal muscle of tumor-bearing rabbits. Jpn. J. Cancer Res. Gann 92, 631–637. doi: 10.1111/j.1349-7006.2001.tb01141.x
Yu, A. F., Flynn, J. R., Moskowitz, C. S., Scott, J. M., Oeffinger, K. C., Dang, C. T., et al. (2020). Long-term cardiopulmonary consequences of treatment-induced cardiotoxicity in survivors of ERBB2-positive breast cancer. JAMA Cardiol. 5, 309–317. doi: 10.1001/jamacardio.2019.5586
Yu, A. P., Pei, X. M., Sin, T. K., Yip, S. P., Yung, B. Y., Chan, L. W., et al. (2014). Acylated and unacylated ghrelin inhibit doxorubicin-induced apoptosis in skeletal muscle. Acta Physiol. Oxf. Engl. 211, 201–213. doi: 10.1111/apha.12263
Zampieri, S., Doria, A., Adami, N., Biral, D., Vecchiato, M., Savastano, S., et al. (2010). Subclinical myopathy in patients affected with newly diagnosed colorectal cancer at clinical onset of disease: evidence from skeletal muscle biopsies. Neurol. Res. 32, 20–25. doi: 10.1179/016164110X12556180205997
Zhang, Y., Wang, J., Wang, X., Gao, T., Tian, H., Zhou, D., et al. (2020). The autophagic-lysosomal and ubiquitin proteasome systems are simultaneously activated in the skeletal muscle of gastric cancer patients with cachexia. Am. J. Clin. Nutr. 111, 570–579. doi: 10.1093/ajcn/nqz347
Zhong, X., Pons, M., Poirier, C., Jiang, Y., Liu, J., Sandusky, G. E., et al. (2019). The systemic activin response to pancreatic cancer: implications for effective cancer cachexia therapy. J. Cachexia Sarcopenia Muscle 10, 1083–1101. doi: 10.1002/jcsm.12461
Zhou, X., Wang, J. L., Lu, J., Song, Y., Kwak, K. S., Jiao, Q., et al. (2010). Reversal of cancer cachexia and muscle wasting by ActRIIB antagonism leads to prolonged survival. Cell 142, 531–543. doi: 10.1016/j.cell.2010.07.011
Keywords: cancer cachexia, muscle atrophy, protein turnover, intermuscular adipose tissue, inflammatory cytokines, mitochondria, oxidative stress, satellite cells
Citation: Mallard J, Hucteau E, Hureau TJ and Pagano AF (2021) Skeletal Muscle Deconditioning in Breast Cancer Patients Undergoing Chemotherapy: Current Knowledge and Insights From Other Cancers. Front. Cell Dev. Biol. 9:719643. doi: 10.3389/fcell.2021.719643
Received: 02 June 2021; Accepted: 10 August 2021;
Published: 14 September 2021.
Edited by:
Yann Simon Gallot, University of Évry Val d’Essonne, FranceReviewed by:
Paola Costelli, University of Turin, ItalyBert Blaauw, University of Padua, Italy
Amélie Rébillard, Laboratoire des Sciences du Mouvement, du Sport et de la Santé (M2S), France
Copyright © 2021 Mallard, Hucteau, Hureau and Pagano. This is an open-access article distributed under the terms of the Creative Commons Attribution License (CC BY). The use, distribution or reproduction in other forums is permitted, provided the original author(s) and the copyright owner(s) are credited and that the original publication in this journal is cited, in accordance with accepted academic practice. No use, distribution or reproduction is permitted which does not comply with these terms.
*Correspondence: Allan F. Pagano, YWxsYW4ucGFnYW5vQHVuaXN0cmEuZnI=; cGFnYW5vLmFsbGFuQGdtYWlsLmNvbQ==