- Biology Department, University of Massachusetts Boston, Boston, MA, United States
The eukaryotic structural maintenance of chromosomes (SMC) proteins are involved in key processes of chromosome structure and dynamics. SMC1β was identified as a component of the meiotic cohesin complex in vertebrates, which aids in keeping sister chromatids together prior to segregation in meiosis II and is involved in association of homologous chromosomes in meiosis I. The role of SMC1β in meiosis has primarily been studied in mice, where mutant male and female mice are infertile due to germ cell arrest at pachytene and metaphase II stages, respectively. Here, we investigate the function of zebrafish Smc1b to understand the role of this protein more broadly in vertebrates. We found that zebrafish smc1b is necessary for fertility and has important roles in meiosis, yet has no other apparent roles in development. Therefore, smc1b functions primarily in meiosis in both fish and mammals. In zebrafish, we showed that smc1b mutant spermatocytes initiated telomere clustering in leptotene, but failed to complete this process and progress into zygotene. Furthermore, mutant spermatocytes displayed a complete failure of synapsis between homologous chromosomes and homolog pairing only occurred at chromosome ends. Interestingly, meiotic DNA double strand breaks occurred in the absence of Smc1b despite failed pairing and synapsis. Overall, our findings point to an essential role of Smc1b in the leptotene to zygotene transition during zebrafish spermatogenesis. In addition, ovarian follicles failed to form in smc1b mutants, suggesting an essential role in female meiosis as well. Our results indicate that there are some key differences in Smc1b requirement in meiosis among vertebrates: while Smc1b is not required for homolog pairing and synapsis in mice, it is essential for these processes in zebrafish.
Introduction
Germ cells are unique since only they can undergo meiosis and contribute to the next generation by producing haploid gametes. Meiosis is a specialized cell division where DNA replicates once but chromosomes go through two rounds of segregation (Bolcun-Filas and Handel, 2018). The first meiotic division (meiosis-I) segregates homologous chromosome pairs whereas the second division (meiosis-II) is more similar to mitotic divisions, separating sister chromatids. To accomplish the unique segregation in meiosis-I, homologous chromosomes (each of which consists of two sister chromatids) must find each other, pair, and undergo synapsis. In many eukaryotic organisms, such dynamic chromosome movements that assist in homolog pairing are facilitated by telomeres (Alleva and Smolikove, 2017). Telomeres attach to the nuclear envelope during the leptotene stage via a meiotic-specific protein complex. This complex interacts with a protein chain that spans the nuclear envelope and interacts with the cytoskeleton to drive chromosome movements (Burke, 2018). The telomeres then cluster at one side of the nucleus, forming the “bouquet.” This process of chromosome movement and clustering is thought to be essential for homologous chromosomes to find each other and pair.
As homologous chromosomes pair, a proteinaceous structure assembles between them, called the synaptonemal complex (SC), which holds homologous chromosomes together and facilitates meiotic recombination (Bolcun-Filas and Handel, 2018). The vertebrate SC consists of three synaptonemal complex proteins (SYCP): SYPC2 and SYCP3 form the axial elements, which associates with the chromosome axes; and SYPC1 forms the transverse elements, which bridges the axial elements of homologous chromosomes. In addition, multiple SC central element proteins overlap with the transverse element as chromosomes synapse. The formation of the synaptonemal complex between homologous chromosomes is critical for segregation of homologous chromosomes to opposite daughter cells during the first meiotic division. In zebrafish, SC formation is initiated near the telomeres (Saito et al., 2014; Blokhina et al., 2019). The axial element of the SC assembles along chromosomes beginning near the chromosome ends, as has been visualized by Sycp2 and Sycp3 localization (Blokhina et al., 2019). Synapsis ensues, as seen by visualization of the transverse element protein, Sycp1, which follows Sycp3 localization (Blokhina et al., 2019; Imai et al., 2021). Thus, homolog pairing and synapsis begins at the chromosomes ends and zippers closed toward the chromosome center. As homolog pairing commences, meiotic double strand breaks, which are a prerequisite for homologous recombination, also initiate near the chromosome ends in zebrafish (Saito et al., 2011; Blokhina et al., 2019).
Formation of the SC in meiosis is dependent on the cohesin complex. The cohesin complex has a critical role in the faithful pairing and segregation of chromosomes during both mitosis and meiosis (Ishiguro, 2019). In both processes the cohesin complex promotes sister chromatid cohesion and proper chromosome segregation, however, in meiosis it has additional roles in homolog pairing, assembly of the synaptonemal complex, and chromosome architecture. The meiotic cohesin complex consist of four core subunits: SMC1β, SMC3, RAD21L or REC8, and STAG3 (Figure 1A; Rankin, 2015). Similarly, mitotic cohesin consists of SMC1α, SMC3, RAD21, and STAG1/2 (Nasmyth, 2001). The two SMC proteins form a ring surrounding the sister chromatids that is closed at one end by RAD21L/REC8 and STAG3 (or RAD21 and STAG1/2 in mitosis). This complex holds the sister chromatids together until anaphase, when separase cleaves RAD21L/REC8/RAD21, allowing sister chromosomes to move to opposite poles of the cell (Rankin, 2015). In meiosis, the cohesin complex is necessary for assembly of the SC axial element, and therefore promotes synaptonemal complex formation. The specific components of each cohesin complex contributes to their unique roles in meiosis and mitosis (Ishiguro, 2019).
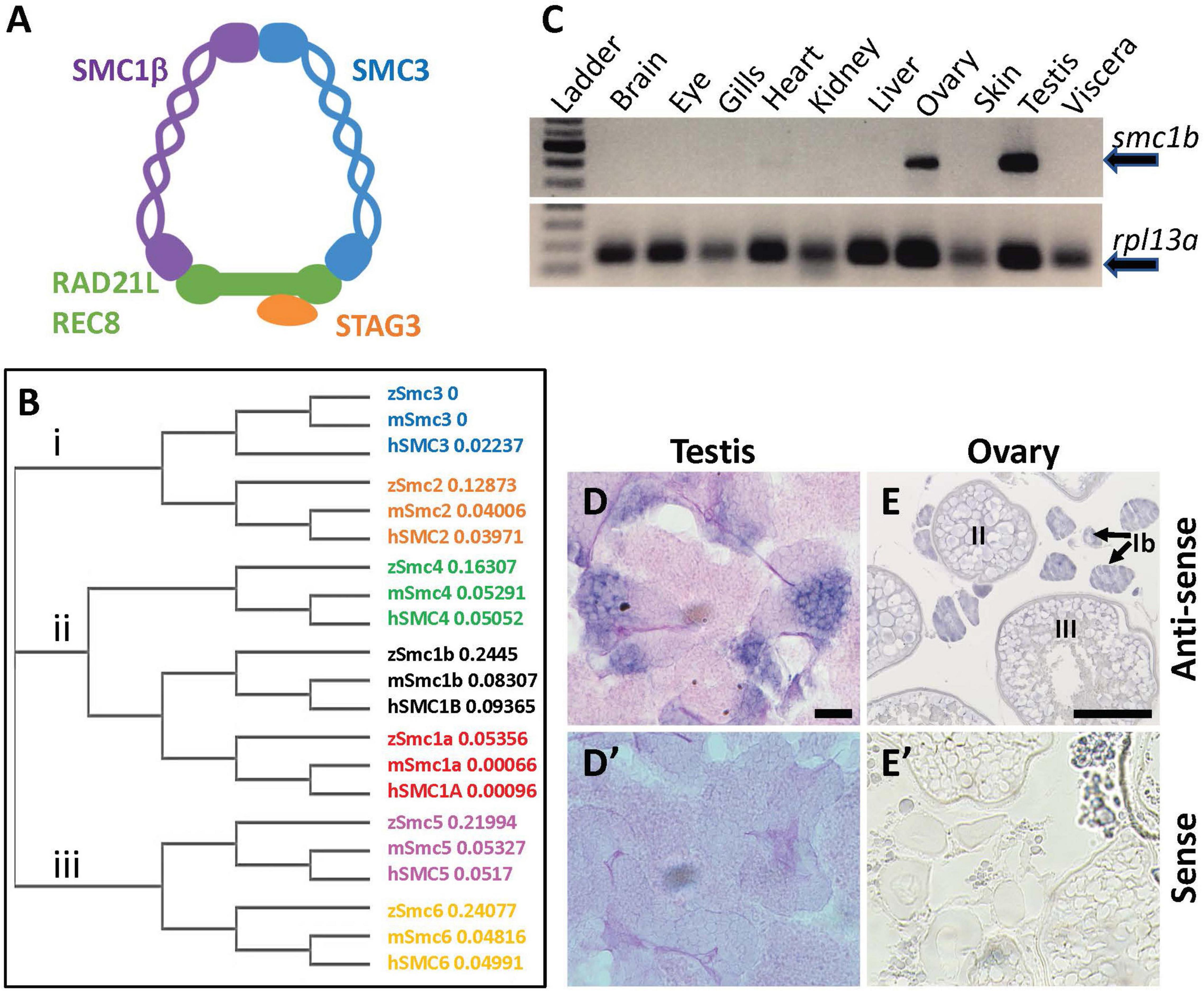
Figure 1. Zebrafish smc1b is conserved and expressed in germ cells. (A) Cartoon representing the meiotic cohesin complex. (B) Phylogenetic tree of zebrafish (z), mouse (m), and human (h) Smc protein family. Numbers to the right of protein names represent bootstrap confidence values. (C) RT-PCR of smc1b and control gene rpl13a using cDNA generated from brains, eyes, gills, hearts, kidneys, livers, ovaries, skin, testes, and viscera of wild-type adult zebrafish. (D,E) In situ hybridization (ISH) on testis (D) and ovary (E) sections of wild-type zebrafish. (D’,E’) ISH using sense probes were run as negative controls. Scale bars are 20 μm for testes and 100 μm for ovaries.
Vertebrate animals typically have two SMC1 proteins, the mitotic SMC1α and meiotic SMC1β, however, the distinct roles of these two proteins have not been studied outside of mammals. Mice lacking SMC1β fail to complete meiosis and present as infertile in both sexes, indicating that it is specifically necessary for meiosis (Revenkova et al., 2004; Takabayashi et al., 2009). In Smc1β mutant mice, meiosis is blocked in the pachytene stage in males whereas female meiosis progresses up to metaphase II. The other observed defects in the mutant mice were short prophase-I axial elements, incomplete synapsis, premature loss of sister chromatid cohesion, and reduced recombination foci (Revenkova et al., 2004). In addition, mammalian SMC1β has a role in telomere integrity and attachment of telomeres to the nuclear envelope during meiotic prophase-I. In mice, SMC1β is required for localization and enrichment of cohesin to telomeres in meiotic prophase-I and Smc1β deficient cells display various types of telomere abnormalities (Adelfalk et al., 2009). In addition, around 20% of telomeres in the Smc1β mutant meiocytes failed to attach to the nuclear envelope during meiosis. Thus, this protein is necessary for multiple processes required for proper chromosome segregation during meiosis.
Whether or not SMC1β functions in meiosis in non-mammalian vertebrates has not been investigated, although it was found to be expressed in medaka spermatocytes (Iwai et al., 2004). Here, we analyzed the function of zebrafish smc1β, which is named smc1b in zebrafish. We found that all smc1b mutant zebrafish develop as males with no other outward visible defects. Histological examination of the testes revealed that they failed to develop mature sperm and consequently were sterile. Smc1b mutant spermatocytes failed to progress through meiotic prophase-I, demonstrating that smc1b is specifically necessary for meiosis in zebrafish, similar to mice. Interestingly, zebrafish smc1b mutant males exhibited more severe meiotic defects than those reported in mice, displaying meiotic arrest at the leptotene stage, whereas mouse spermatocytes reached up to the pachytene stage. Furthermore, the cohesin complex did not appear to be enriched at telomeres in zebrafish spermatocytes, however, meiotic bouquet formation was still disrupted in mutants. This study broadens our understanding of how the meiotic cohesin complex functions in vertebrate animals.
Materials and Methods
Zebrafish Strains and Husbandry
All animal procedures were approved by the UMass Boston Animal Care and Use Committee. Zebrafish were maintained in a recirculating system on a 14 h light and 10 h dark light cycle. Strains and lines used in this study were: smc1bsa24632, smc1bsa24631, and Tuebingen (Tue). Embryos of two smc1b mutant lines (ID: sa24632 and sa24631) were generated by the Zebrafish Mutation Project and obtained from the Zebrafish International Resource Center (ZIRC). The smc1bsa24632 and smc1bsa24631 lines each carry a nonsense mutation which cause premature stop codons at codon 198 and 261, respectively, whereas the wild-type protein is 1,235 amino acids long. For simplicity, we refer to each mutant line as smc1bQ198X and smc1bQ261X.
Protein Alignment
We used the online program MUSCLE1 to perform protein alignment and phylogenetic tree generation. The NCBI accession number of the proteins used in this study are as follows: zebrafish Smc1a (NP_001155103.1), Smc1b (XP_009296271.1), Smc2 (NP_955836.2), Smc3 (NP_999854.1), Smc4 (NP_775360.2), Smc5 (NP_001180470.1), and Smc6 (NP_001121806.1); mouse Smc1a (NP_062684.2), Smc1b (NP_536718.1), Smc2 (NP_001288341.1), Smc3 (NP_999854.1), Smc4 (AAH62939.1), Smc5 (AAH38345.1), and Smc6 (AAH90630.1); human [SMC1A (CAI42646.1), SMC1B (AAI26209.1), SMC2 (AAI44164.1), SMC3 (NP_005436.1), SMC4 (Q9NTJ3.2), SMC5 (NP_055925.2), and SMC6 (CAC39248.1).
RT-PCR
Total RNA was isolated from zebrafish organs using TRI-reagent, following the manufacturer’s procedure. Isolated RNA was treated with TURBO DNase to remove genomic DNA contamination. The first strand cDNA was synthesized using oligo-dT primers and AMV-Reverse Transcriptase. The ziwi transcript, which is expressed in the germ cells of both sexes, was used as a control for the gonad using previously published primers (Siegfried and Nüsslein-Volhard, 2008). The ubiquitously expressed gene ribosomal protein L13 alpha (rpl13a) was used as control for other tissues (Tang et al., 2007). The primers used for RT-PCR are listed in Table 1.
In situ Hybridization
Samples for in situ hybridization (ISH) were fixed in 4% paraformaldehyde (PFA) at 4°C overnight. To generate a probe for detecting smc1b, a 390 bp long partial cDNA was made using smc1b RT-PCR primers (Table 1) and sub-cloned into the pGEMT Easy vector (Promega). Both sense and anti-sense probes were synthesized using a DIG RNA Labeling kit. The ISH on paraffin-embedded sections were performed according to the protocol described by Webster et al. (2019). Alkaline phosphatase-conjugated anti-DIG antibody (Roche) and BM Purple substrate were used to visualize the probe.
Genotyping and PCR
To genotype the smc1b mutants, nested PCR reactions were performed, with the second PCR reaction using dCAPS (Derived Cleaved Amplified Polymorphic Sequences) primers, designed using the online tool dCAPS Finder 2.02 (Neff et al., 2002). In the first PCR, primers smc1b FP and smc1b RP1 were used to amplify a 751 bp amplicon, which contained both mutant alleles. The nested PCR reaction for the smc1bQ198X line was performed using primers smc1b_sa24632 FP and RP, followed by Hpy188I digestion. The digested PCR product was run on 7% acrylamide gel to distinguish mutant (127 bp) and wild-type (152 bp) bands. Primers smc1b_sa24631 FP and RP2 were used to amplify the smc1bQ261X mutation region, followed by DdeI digestion. To distinguish the mutant (182 bp) from wild-type (157 bp) band, the digested PCR product was run on a 7% acrylamide gel. Sanger sequencing was done on PCR products to confirm all of the mutations. We also performed sequencing on cDNA from homozygous mutant testes and detected the predicted mutations. The name and sequence of the genotyping primers are listed in Table 2.
Fertility Tests
Smc1bQ198X mutant males were paired with wild type Tue females. The following morning, embryos were collected and monitored under a dissecting microscope to see if embryonic cell cleavages took place. If eggs appeared unfertilized, they were kept up to 24 h to ensure development did not proceed. Eggs were scored as unfertilized if there was no apparent initiation of embryonic development. The embryos were raised in 1× E3 buffer in a 28°C incubator.
Histology
Fish were euthanized by tricaine overdose and torsos were isolated and fixed in Bouin’s solution overnight at room temperature. Following dehydration, the fixed tissues were embedded in paraffin for sectioning. A rotating microtome was used for obtaining 5 μm thick sections. We used Modified Harris’s hematoxylin and eosin for histological staining following standard protocols. Imaging was performed by a Zeiss Inverted Microscope and acquired using Zen Software.
Immunofluorescence and Telomere Staining on Sections
Testes were isolated and fixed in 4% PFA for overnight at 4°C. The fixed tissues were embedded in paraffin and cut into 5 μm thick sections. After de-paraffinization and rehydration, antigen retrieval was done by heating the sections in 10 mM sodium citrate, pH 6.0 solution for 30 min using a vegetable steamer. After 3 min × 5 min washes in PBST, the sections were circled with a barrier pen, covered with blocking buffer (1% bovine serum albumin in PBST) and incubated for 30 min in a humidified chamber at room temperature. Primary antibodies were diluted in the blocking buffer as follows: Sycp3 (NB300-232, Novus Biologicals) at 1:200, γ-H2AX (Neumann et al., 2011) at 1:200, and Caspase-3 (C8487, Sigma-Aldrich) at 1:1000. The sections were incubated overnight with the primary antibodies at 4°C. Incubation with anti-rabbit Alexa Fluor Plus 488 (Thermo Fisher Scientific) secondary antibody (1:500) was done for 1 h at room temperature. Telomeres were stained using a TelC-Cy3 probe (PNA Bio) following previously described protocol (Saito et al., 2014). To visualize nuclei, sections were counterstained with DAPI for 10 min and cover slipped using Fluoroshield (Sigma-Aldrich) mounting medium.
Chromosome Spread Preparation, Telomere Staining, and Immunofluorescence
Meiotic chromosome spreads from zebrafish testes were prepared according to the previously published protocol (Blokhina et al., 2019, 2020), except that cell membranes were disrupted by incubating isolated cells in 0.8% sodium citrate solution for 20 min instead of 0.1 M sucrose solution. Spreads were preserved in −20°C before performing further analysis. Telo-FISH on spreads was done following the same protocol applied to sections. For antibody labeling, primary antibody Sycp1 (Blokhina et al., 2019), Sycp3 (NB300-232, Novus Biologicals), Smc3 (PA529131, Thermo Fisher Scientific), and Rad51 (GTX100469, GeneTex) were diluted at 1:200 and incubated overnight at 4°C. Incubation in appropriate secondary antibody (1:500) was done for 1 h at room temperature. Spreads were kept at 4°C before imaging by a confocal microscopy (Zeiss LSM 880).
Image Analysis
Image acquisition was done with ZEN Black software attached to the confocal microscopy. Cell counting and further analysis was performed using open-sourced software Fiji/Image J. We performed Student’s t-test (p < 0.05) to see whether difference between wild-type and mutant caspase-3 positive cells were significant or not. The fluorescence intensity of γ-H2Ax and Rad51 in mutant and wild-type leptotene stage cells were quantified using Fiji. The signal for each cell was normalized against the nuclear stain DAPI. To see if the intensities were significantly different between wild-type and mutant cells, we have done a t-test at a significance level of p < 0.05.
Results
Zebrafish Smc1b Has Key Domains Conserved With Mammals
To investigate the evolutionary conservation of Smc proteins among the vertebrates, we generated a phylogenetic tree comparing zebrafish, mouse, and human Smc protein sequences. The tree has three major branches which consist of: (i) Smc2 and Smc3; (ii) Smc1a, Smc1b, and Smc4; (iii) Smc5 and Smc6 (all SMC1α/Smc1a and SMC1β/Smc1b are referred to as Smc1a and Smc1b, respectively, for simplicity) (Figure 1B). The tree demonstrates that each zebrafish Smc protein clusters together with the corresponding mammalian protein. We also found that most mouse and human SMC proteins are evolutionary closer to each other than to those of zebrafish. Surprisingly, mouse SMC3 grouped with zebrafish Smc3 rather than that of human (Figure 1B). We focused our attention to the Smc1b protein since previous studies demonstrated a role of this protein in meiosis and reproduction (Revenkova et al., 2004). To investigate Smc1b conservation among vertebrates, we performed a protein alignment, which showed that zebrafish Smc1b is 53.04 and 52.11% identical to human and mouse SMC1β, respectively (Supplementary Figure 1). Higher similarity was observed in several key domains, such as the N-terminal ATP binding cassette (ABC), the flexible hinge, and the C-terminal ABC domains, which are 62.75, 66.95, and 75.96% identical between zebrafish and human, respectively (Supplementary Figure 1B). However, coil-coiled domains, which are located between the ABCs and the hinge domain, are less conserved (ranging between 26.53 to 57.14%). Interestingly, we found four conserved motifs (Walker A and B, ABC transporter signature motif, and D-Loop) in zebrafish Smc1b, similar to mammals (Supplementary Figure 1) (Revenkova et al., 2001). Walker A motifs have been shown to bind with azido-ATP, an analog of ATP (Akhmedov et al., 1998). The signature motif is essential for head-to-head engagement of SMC dimers and the Walker B sequence is involved in ATP hydrolysis (Chao et al., 2017). These results suggest that the functions of zebrafish Smc1b could be similar to the mammalian protein.
Smc1b Is Required for Spermatogenesis and Oogenesis in Zebrafish
To ask which zebrafish tissues smc1b may function in, we assessed smc1b expression. We performed RT-PCR from brains, eyes, gills, hearts, kidneys, livers, ovaries, skin, testes, and viscera of wild-type adult zebrafish to test which organs of zebrafish express smc1b. Smc1b was detected in the testis and ovary but not in other organs tested (Figure 1C), suggesting that smc1b has important functions in zebrafish gonads. Next, we sought to answer which gonadal cells express smc1b by performing in situ hybridization (ISH) on testis and ovary sections. The ISH detected expression in the spermatogonia and some spermatocytes but not in the mature sperm of the testis (Figure 1D). In ovaries, smc1b was expressed in stage Ib, II, and III oocytes, which are all in meiosis-I (Figure 1E). The ISH results bolstered the notion that smc1b has important roles in zebrafish germ cells.
To test whether smc1b is required for meiosis in zebrafish, we analyzed two mutant lines. The smc1bsa24632 and smc1bsa24631 mutations each result in a premature stop codon after amino acid 197 and 260, respectively. Here, we refer to these mutant lines as smc1bQ198X and smc1bQ261X, respectively, reflecting the protein change caused by each mutation (Figure 2A). The homozygous mutants of both alleles developed normally without showing any outwardly visible defects. However, all smc1b mutant fish (N = 54) developed as phenotypic males (based on pigmentation and body shape). When mutant males were paired with wild-type females, they were able to induce spawning (N = 5), however, all eggs were unfertilized (Table 3). To identify the underlying problem, we performed histology on testes from adult mutants and wild-type siblings (Figure 2). The histology revealed complete lack of spermatozoa in both mutant lines, however, spermatogonia and spermatocytes were present suggesting that mutant germ cells had arrested as spermatocytes (Figures 2C,D). We also established a trans-heterozygous (smc1bQ198X/Q261X) line, which exhibited phenotypes indistinguishable to the homozygous mutants, i.e., all male and no sperm (Figure 2E). To test if smc1b mutant testes had abnormal cell death, we performed immunofluorescence (IF) on mutant and wild-type testes (N = 3) with cleaved caspase-3 antibody. There was no significant difference between wild-type and mutant testes in terms of apoptosis (Figures 2F–H). Therefore, smc1b mutant cells that arrest in meiosis die by a caspase-3 independent mechanism. These data point to a potential role of zebrafish smc1b in meiosis.
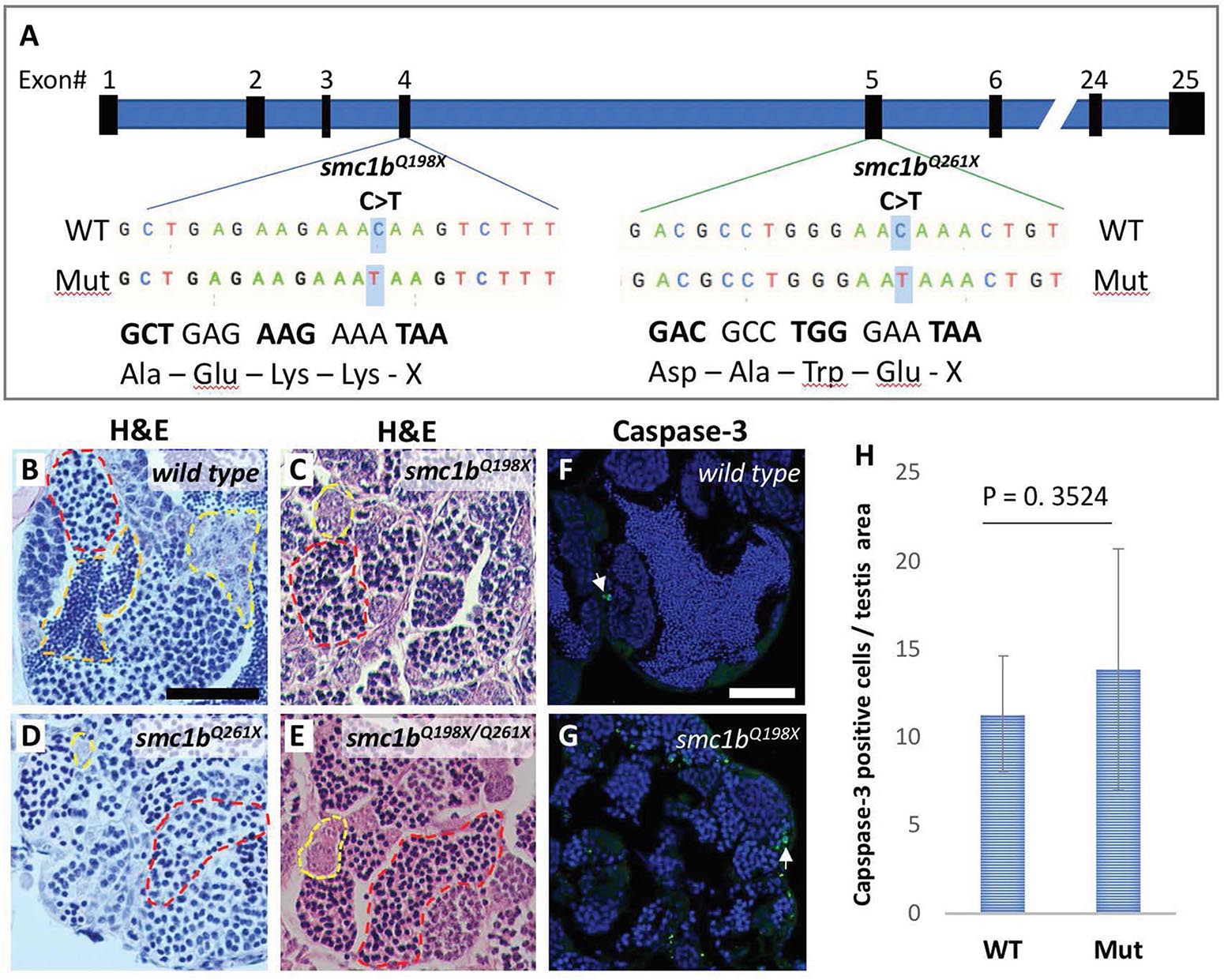
Figure 2. Smc1b mutant zebrafish display defects in spermatogenesis. (A) Smc1b mutations used in this study: smc1bQ198X located in exon 4 and smc1bQ261X in exon 5. Both mutations cause premature termination codons. (B–E) Hematoxylin-eosin (H&E) staining on adult testes of wild type (B), smc1bQ261X(–/–) (C), smc1bQ198X(–/–) (D), and smc1bQ198X/Q261X (E). Dashed lines denote examples of: spermatogonia (yellow), spermatocytes (red), and spermatozoa (orange). (F,G) Caspase-3 (green) and DAPI (blue) staining on adult testes from wild type (F) and smc1b mutant (G). Caspase-3 positive cells are indicated by arrows. (H) Quantification of caspase-3 positive cells per testis area in the mutant (N = 3 testes) and wild type (N = 3 testes). Student’s t-test shows no significant (p = 0.3524) difference between wild-type and mutant caspase-3 positive cells numbers. Scale bars are 50 μm.
Since all smc1b mutants were male as adults, we asked if mutants underwent sex reversal earlier in development. During zebrafish development, the undifferentiated gonads first pass through a “juvenile ovary” stage, in which the gonads contain immature oocytes, before undergoing sex-differentiation to form either an ovary or testis (Takahashi, 1977). The initiation of oogenesis and continued development of oocytes is required for female sex differentiation—when oogenesis fails the gonad adopts a testis fate and the fish develops as a male (Rodríguez-Marí et al., 2010; Dranow et al., 2013). To test if smc1b mutants generated immature oocytes and initiated female differentiation, we performed histology on mutants and wild-type siblings at 4, 5, and 6 wpf. Analysis of wild types showed that sex-differentiation of the gonads was histologically apparent at 5 weeks post fertilization (wpf) in our lines (Figure 3). At 4 wpf, all mutant and wild-type fish had undifferentiated gonads with no oocytes, indicating that they had not yet reached the juvenile ovary stage (Figures 3A,B). However, histology at 5 and 6 wpf revealed that 100% of smc1b mutant gonads were developing as testes, whereas the gonads of the wild-type siblings were either testes or ovaries (Figures 3C–H). These results indicate that smc1b mutants did not undergo sex reversal rather directly developed as male. Since we never detected ovarian follicles in the smc1b mutants, we conclude that this gene is also necessary for oogenesis in zebrafish.
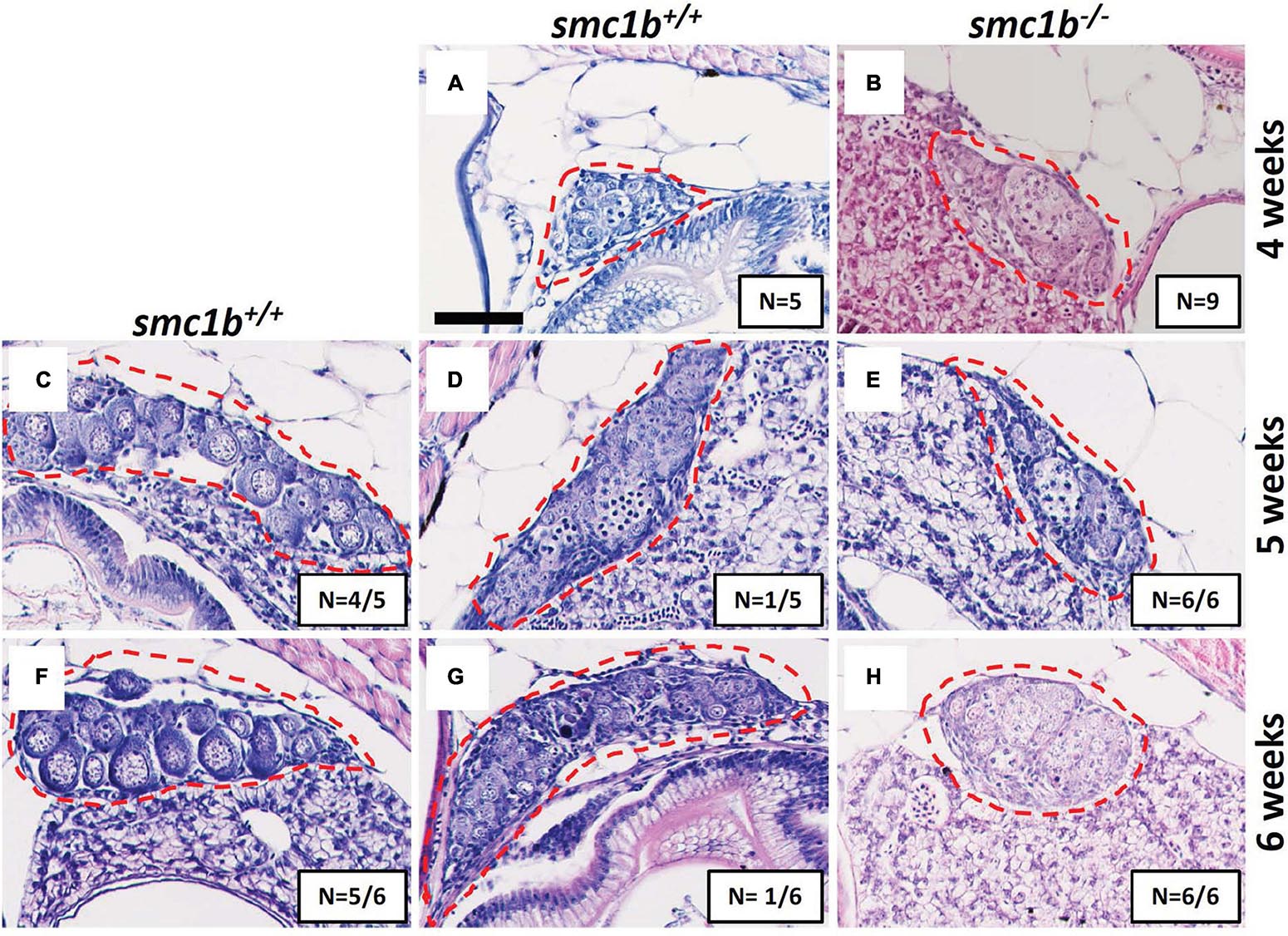
Figure 3. Absence of oogenesis in smc1bQ198X mutants. Hematoxylin-eosin stain of wild-type and smc1b mutant gonads during sex-differentiation. (A,B) Undifferentiated gonads in wild-type (A) and mutant (B) fish at 4 weeks old. (C–H) Although oogenesis (C,F) and spermatogenesis (D,G) took place in wild-type gonads at 5- and 6-weeks old, only spermatogenesis progressed in the mutants (E,H). Gonads are outlined by red dashed line, scale is 50 μm. N = total number of individuals with the gonad histology pictured per total number of fish analyzed for each age group.
Smc1b Is Necessary for Interstitial Pairing and Synapsis of Homologous Chromosomes
Since smc1b mutant testes had spermatocytes but no mature sperm (Figures 2C–E), we wanted to know when the spermatocytes arrested in meiosis. We stained mutant and wild-type testis sections with Sycp3 antibody and telomere fluorescent in situ hybridization (Telo-FISH) to determine the stage of meiosis in spermatocytes. In wild-type zebrafish, the telomeres attach to the nuclear envelope then cluster at one side of the nucleus to form the bouquet (Saito et al., 2014; Blokhina et al., 2019). Sycp3 begins to associate with the chromosomes in late leptotene to early zygotene stages, starting near the telomeres, then extends along the chromosomes as homologous chromosomes pair (Figures 4A–D; Saito et al., 2014; Blokhina et al., 2019). We found that Sycp3-positive cells were present in the smc1b mutants, indicating that meiosis initiated in mutant testes (Figures 4E,G). However, in mutant spermatocytes, Sycp3 localization was comparable to wild type leptotene stage cells and spermatocytes resembling subsequent stages were not present (Figures 4A,C). Telomere dynamics were also affected in smc1b mutants. In wild type, telomeres begin clustering in late leptotene and form a tight cluster in early zygotene, forming the meiotic bouquet. We found some mutant spermatocytes with clustered telomeres, however, these appeared less tightly clustered than wild-type bouquet-stage spermatocytes (Figures 4B,F). Moreover, Sycp3 did not tightly cluster near telomeres in these “bouquet-like” mutant cells, unlike wild type, indicating incomplete bouquet formation (Figures 4A,E). These data suggest that smc1b is necessary for progression from leptonema to zygonema.
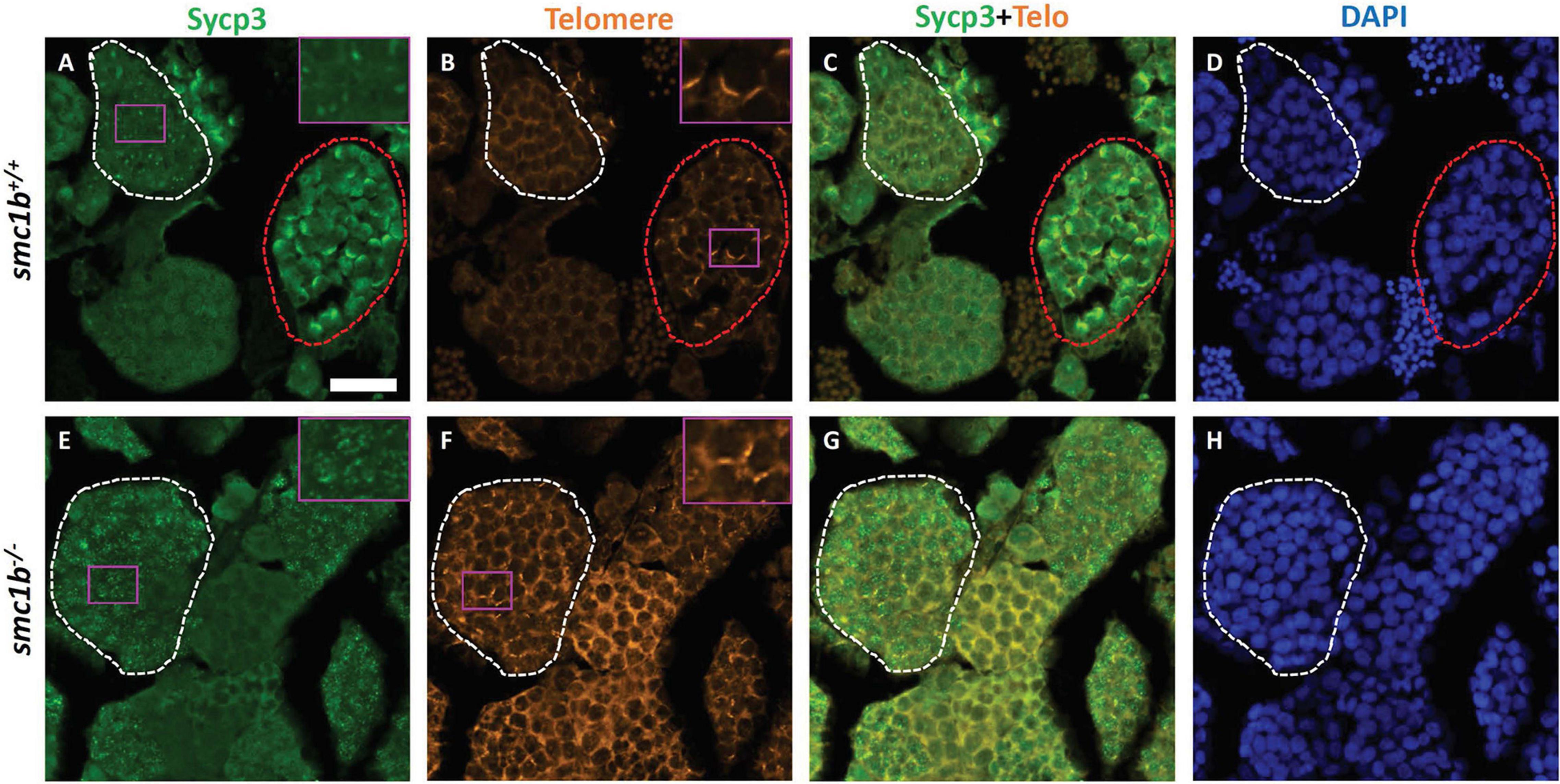
Figure 4. Spermatocytes in smc1bQ198X mutants failed to progress beyond the leptotene stage. Labeling of Sycp3 and telomeres in adult wild-type and mutant testes sections: Sycp3 (green), telomere in situ hybridization (orange), DAPI nuclear stain (blue). (A–H) Leptotene stage spermatocytes are present in both wild-type and mutant spermatocytes, examples circled in white. Bouquet stage cells (circled red) are present in wild type but not in the mutant, however, partial telomere clustering is apparent in some mutant cells [e.g., panel (F), inset]. Insets are zoomed images of the areas boxed in purple. Scale bar is 20 μm.
To assess the stage at which smc1b mutant spermatocytes arrest in more depth, we performed Telo-FISH combined with IF for Sycp1 and Sycp3 antibodies on chromosome spreads (Figure 5). In wild type, leptotene stage cells exhibited Sycp3 localized near the chromosome ends and had either no or small puncta of Sycp1 protein, as previously reported (Figure 5A; Saito et al., 2014; Blokhina et al., 2019). Zygotene stage cells had elongated Sycp3 and Sycp1 labeling as the SC assembled and chromosomes synapsed (Figures 5B,C). Pachytene stage cells had completely synapsed chromosomes with end-to-end labeling of SC proteins (Figure 5D). Similar to what we observed on sectioned testes, in smc1b mutant spermatocytes, Sycp3 only associated at the ends of chromosomes, resembling the leptotene stage in wild types (Figures 5E,F). To ask if pairing was initiated in smc1b mutants, we counted the number of Sycp3 foci in wild-type bouquet and mutant “bouquet-like” spermatocytes (e.g., Figures 5B,F). Zebrafish have 25 chromosomes, therefore we expect 50 Sycp3 foci if all homolog ends were paired (two ends per pair) and 100 foci if they were all unpaired. We found an average of 48 Sycp3 spots in wild-type and 40 in mutant spermatocytes suggesting that pairing at chromosome ends was initiated in smc1b mutants but failed to progress interstitially along homologous chromosomes (Figure 5H). Mutant spermatocytes had slightly less Sycp3 foci than wild type, suggesting either that some homologs fail to initiate pairing or that more than two chromosome ends were clustered together. Higher resolution microscopy will be needed to distinguish these possibilities. Regardless, interstitial Sycp3 was absent demonstrating that only chromosome ends could pair in smc1b mutants. Furthermore, mutant spermatocytes had no elongated stretches of Sycp1 protein associated with chromosomes, indicating that chromosomes failed to synapse in smc1b mutants (Figures 5E,F). Interestingly, we found about 5% of mutant spermatocytes exhibited some telomere clustering, which we refer to as “bouquet like” (Figure 5F). However, no mutant spermatocytes had tightly clustered telomeres characteristic of a fully formed bouquet, and elongated Sycp1 labeling indicative of SC assembly was absent. We therefore classified these “bouquet-like” spermatocytes as leptotene stage cells (Figures 5F,G). Overall, smc1b mutant spermatocytes failed to initiate synapsis between homologous chromosomes and progress to the zygotene stage, whereas 41% of wild-type primary spermatocytes were in the zygotene stage (Figure 5G). These results indicate that Smc1b is not necessarily required for initiation of bouquet formation, but is needed to form tightly clustered telomeres as well as stable pairing and synapsis of homologous chromosomes in zebrafish spermatocytes.
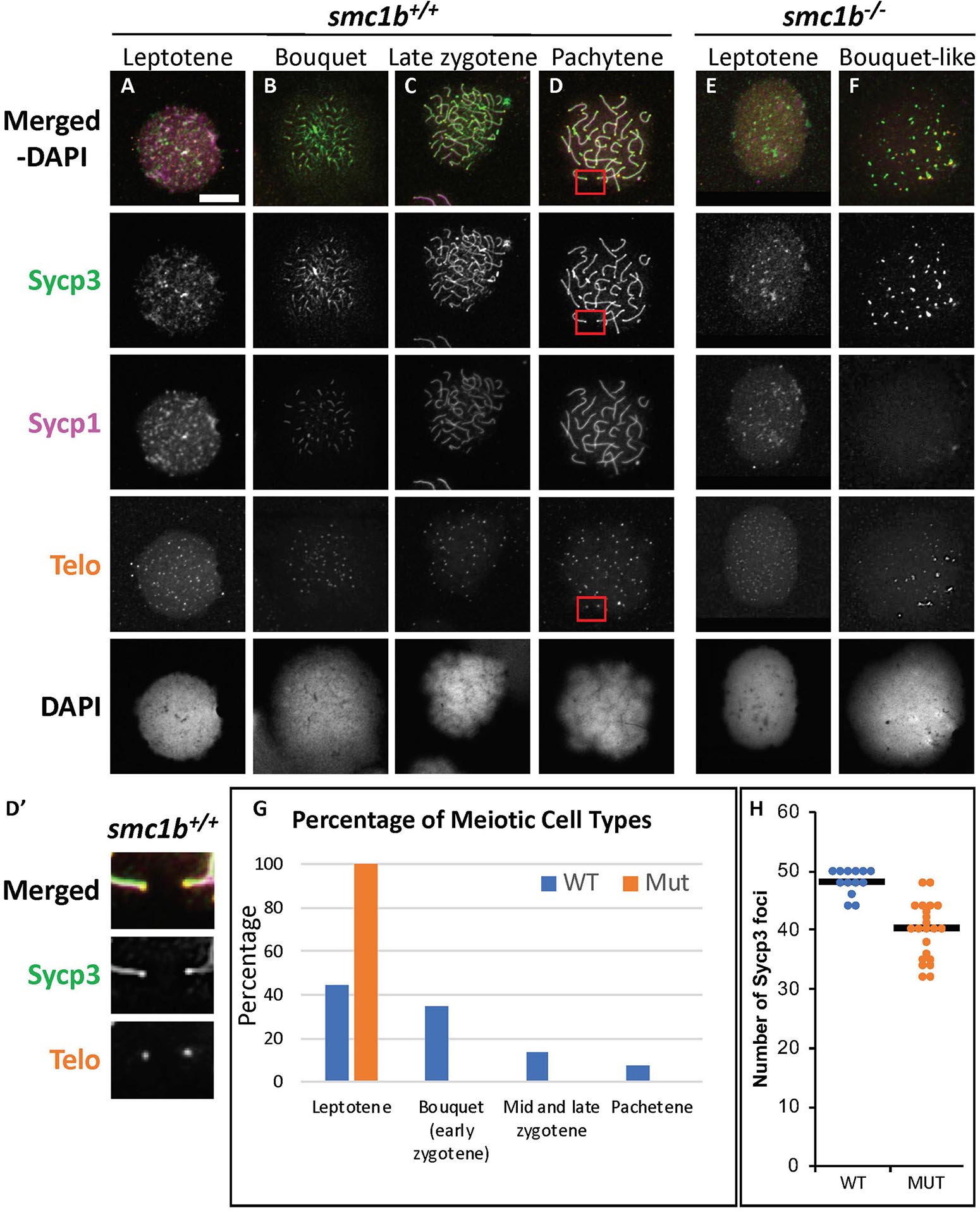
Figure 5. Failed synapsis and anomalous pairing in smc1bQ198X mutant spermatocytes. (A–F) Labeling of Sycp3 and Sycp1 proteins and telomeres (telo) on meiotic chromosome spreads from adult testes. (A–D) In wild-type spermatocytes, leptotene stage cells had Sycp3 protein localized near chromosome ends and exhibited either no Sycp1 signal or Sycp1 puncta. The synaptonemal complex proteins continued to assemble between homologous pairs through pachytene stages as homolog pairing and synapsis occurred. (D′) Sycp3 exhibited enrichment at or near telomeres. Images show enlarged views of the red boxed areas in D. (E,F) Smc1b mutant spermatocytes leptotene stage cells expressed Sycp3 in with a pattern similar to that of wild-type leptotene stage cells and Sycp1 was either absent or present in puncta. However, Sycp3 only labeled chromosomes near the ends and Sycp1 did not extend along chromosomes. A small percentage of mutant cells exhibited some telomere clustering one side of the nucleus (F), which we called bouquet-like and classified as leptotene stage as there was either no Sycp1 or only Sycp1 puncta visible. (G) Quantification of spermatocytes showed that all mutant cells are in leptotene stage compared to about 40% in wild type. Later stages were not observed in the mutants (N = 52 wild-type and 130 mutant cells). (H) Quantification of Sycp3 foci in wild-type bouquet (N = 13) and mutant “bouquet-like” (N = 23) nuclei shows abnormal pairing in smc1b mutant spermatocytes. Mutants have significantly fewer Sycp3 puncta than wild types (T-test, p = 0.000007). Horizontal bars in (H) show the averages. Scale bar is 10 μm.
To ask if loss of Smc1b affects association of the cohesin complex with meiotic chromosomes, we assayed localization of the cohesin complex protein Smc3 (Figure 6). In wild–type spermatocytes, Smc3 localization resembled to that of Sycp3 (Figures 5A–D, 6A–D). Smc3 associated with the synaptonemal complex in the bouquet stage starting near the chromosome ends (Figure 6B). By pachytene stage, Smc3 was localized along the entire synaptonemal complex (Figure 6D). Unlike what has been reported in mouse spermatocytes, Smc3 did not appear to be enriched near the telomeres in zebrafish, whereas Sycp3 was enriched (Figures 5D′, 6D′; Adelfalk et al., 2009). In the smc1b mutant leptotene stage cells, Smc3 protein was detected in nuclei but was not yet associated with chromosomes, similar to that of wild type (Figures 6A,E). However, while Smc3 associated with chromosomes at the bouquet stage of wild–type spermatocytes, Smc3 did not associate with chromosome axes in bouquet–like mutant spermatocytes (Figure 6F). These observations demonstrate that Smc1b is essential for cohesin complex formation during zebrafish meiosis.
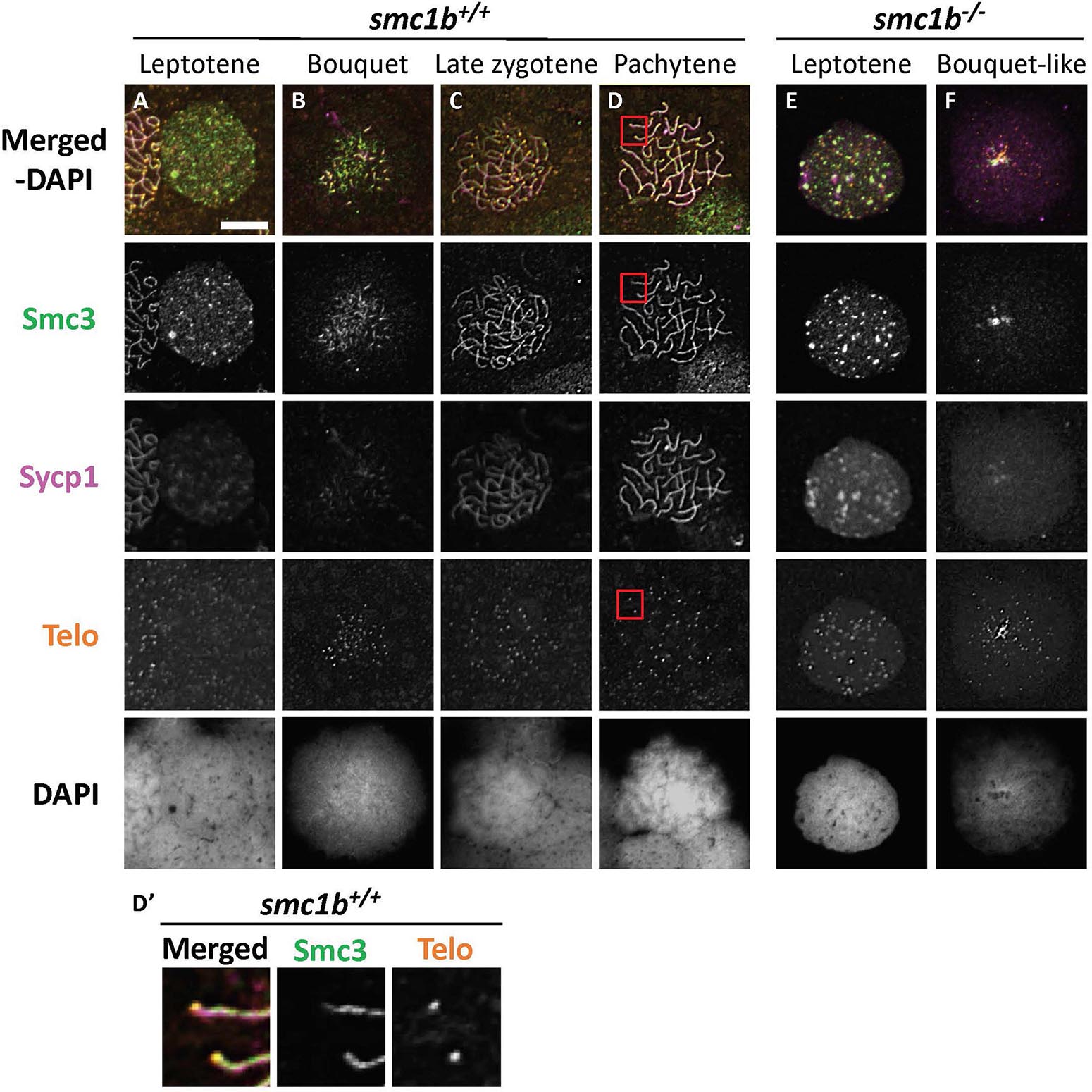
Figure 6. The cohesin complex does not associate with meiotic chromosomes in zebrafish spermatocytes. (A–E) Immunolabeling of Smc3, and Sycp1 in wild-type (A–D) and smc1b mutant (E,F) chromosome spreads. (A–D) In wild-type cells, Smc3 showed a localization pattern similar to Sycp1. (D′) Smc3 was not enriched at telomeres. Images show enlarged views of the red-boxed areas in panel (D). (E,F) In mutant cells, Smc3 was expressed in leptotene stage but failed to load onto chromosomes. Scale is 10 μm.
Meiotic Double Strand Breaks Form in the Absence of Smc1b
Formation of double strand breaks (DSB) is a pre-requisite of DNA recombination during meiosis. In meiotic cells, several proteins including histone variant γ-H2AX and Rad51 bind to DSBs and mediate exchange of DNA between homologous chromosomes (Hamer et al., 2003; Howard-Till et al., 2011). Therefore, expression and localization of these proteins are common markers of DSBs. To test whether zebrafish smc1b is required for meiotic DSB formation, we first performed IF on smc1b mutant testis sections with γ-H2AX antibody. In wild-type spermatocytes, γ-H2AX protein was initially localized near telomeres, as has been previously reported, which is most apparent in bouquet-stage cells in sectioned tissue (Figures 7A–D; Saito et al., 2011; Blokhina et al., 2019). In tissue sections, early bouquet-stage cells, which are in late leptonema, appeared to have γ-H2AX localized more broadly within nuclei (Figures 7A,D). In early zygotene stage, the telomeres became more concentrated on one side of the cell to form the late-bouquet. In these late-bouquet stage spermatocytes, γ-H2AX localization was also condensed on one side of the nucleus (Figures 7A,D). In smc1b mutant spermatocytes, γ-H2AX was detected but was not visibly concentrated to one side of the nuclei of spermatocytes (Figures 7E–H). Co-labeling with Telo-FISH, Sycp1, and γ-H2AX on nuclear spreads clearly demonstrated that γ-H2AX localization initiated near chromosome ends in late-leptotene and clustered near telomeres in the zygotene bouquet stage in wild-type spermatocytes and continued to exhibit chromosome labeling through the pachytene stage (Figures 8A–D). Similar to our observations of testes sections, in smc1b mutant spermatocytes, γ-H2AX was present but generally not concentrated to one side of the nucleus, resembling leptotene stage cells (Figures 8E,F). To test if γ-H2AX was abnormally expressed in mutant spermatocytes compared to similar stages of wild-type cells (leptonema), we measured fluorescence intensity on chromosome spreads. Because we could not co-label with Sycp3, we categorized mutant cells as leptotene stage if they had some γ-H2AX staining as well as either no Sycp1 or small Sycp1 puncta. The quantification showed no significant difference in γ-H2AX intensity in the mutant cells compared to wild-type leptotene stage cells, suggesting that γ-H2AX labeling was indicative of meiotic DSBs (Figure 8G). These data indicate that DSBs could form in smc1b mutants despite lack of cohesin and synaptonemal complexes.
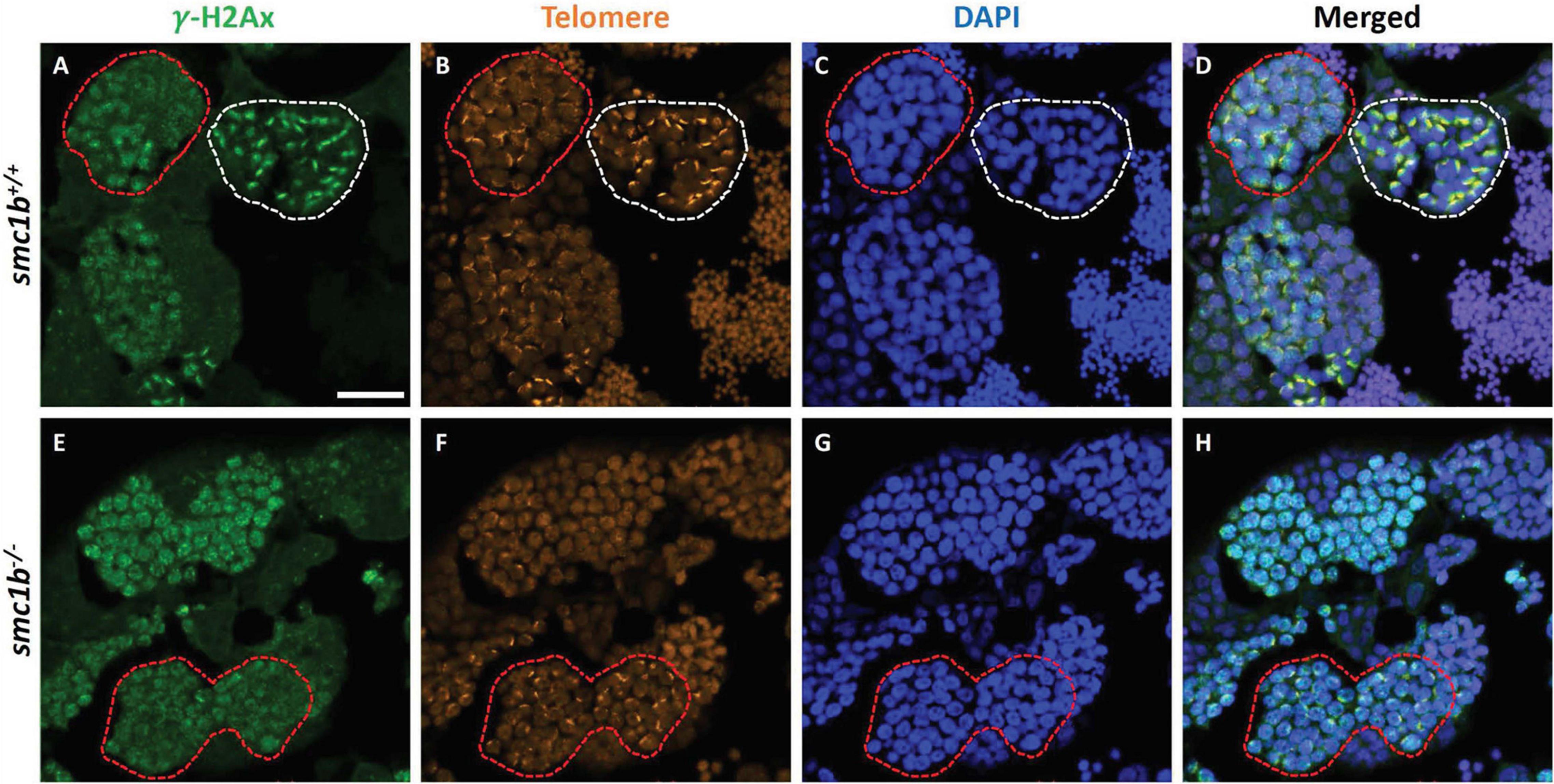
Figure 7. DNA double strand break marker γ–H2Ax was expressed in smc1bQ198X mutant testes. Immunolabeling of γ-H2Ax (green) and Telo-FISH (orange) on wild-type and mutant testis sections. (A–D) In wild-type early bouquet stage spermatocytes, γ-H2Ax appeared to localize more broadly in nuclei on testis sections (red dashed lines). Bouquet stage spermatocytes with tightly clustered telomeres (white dashed lines) exhibited γ-H2Ax localization near telomeres. (E–H) Early bouquet-stage spermatocytes in smc1b mutants display broad γ-H2Ax expression similar to wild type, however, tight clustering of telomeres and γ-H2Ax to one side of the nucleus was not observed. Scale bar is 20 μm.
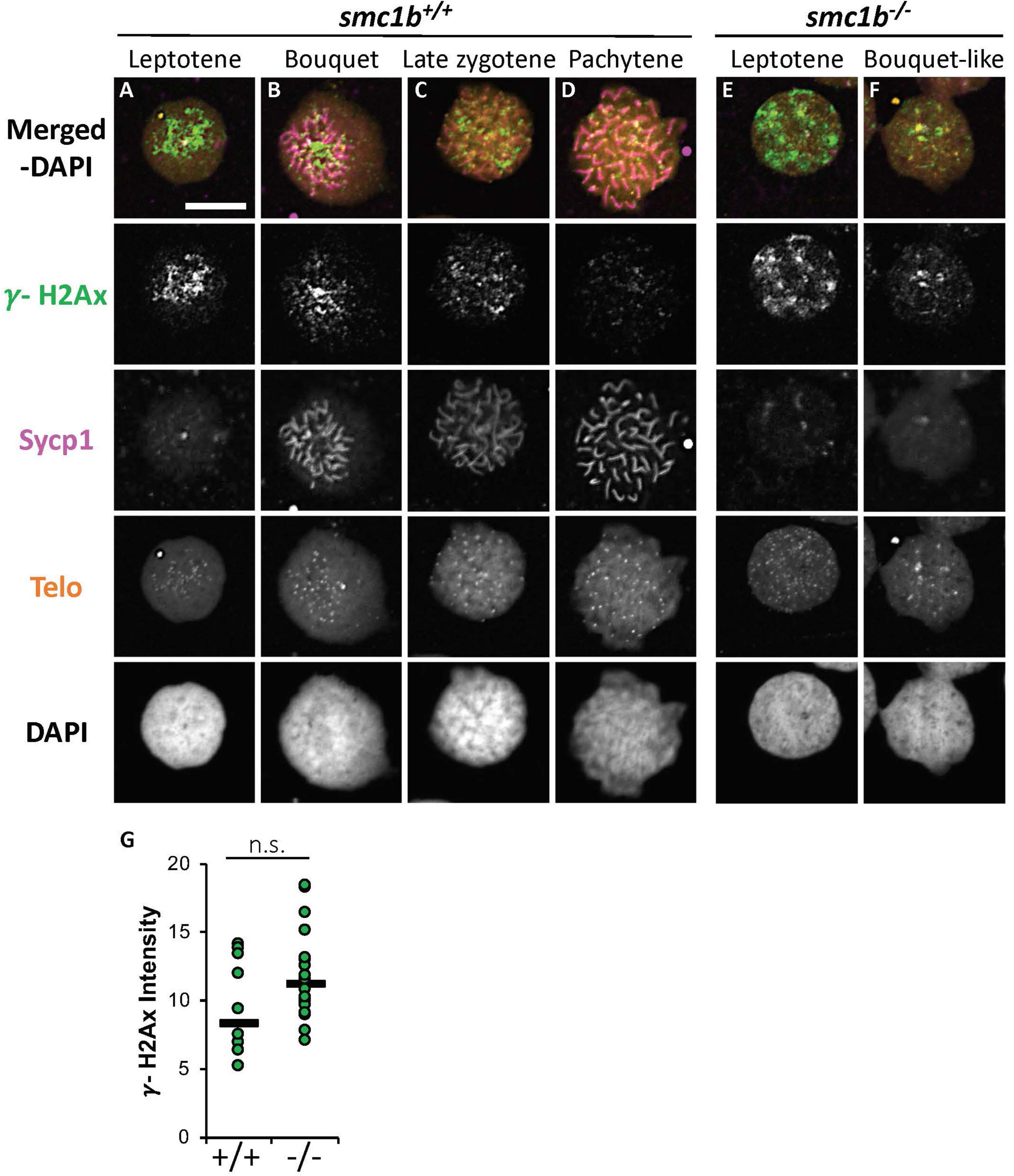
Figure 8. DNA double strand break marker γ-H2Ax was expressed in smc1bQ198X mutant testes. (A–F) Labeling of γ-H2Ax (green), Sycp1 (magenta), and Telo-FISH (orange) on wild-type (A–D) and mutant (E,F) chromosome spreads from testes. γ-H2Ax protein is expressed in both wild-type and mutant nuclei. (G) Quantification of γ-H2Ax fluorescence intensity in wild-type (N = 10) and mutant (N = 18) leptotene stage cells shows no statistically significant difference (P = 0.0945). The horizontal bars show the medians. Scale bar is 10 μm.
Finally, we stained chromosome spreads with Rad51 antibody to further assay the initiation of DSB repair of meiotic chromosomes in smc1b mutant spermatocytes. Following DSB formation, 5′–3′ end resection results in formation of single-stranded DNA that is bound by Dmc1 and Rad51. These proteins function to initiate DSB repair and strand invasion during meiotic recombination (Crickard and Greene, 2018). In wild-type spermatocytes, Rad51 protein was first localized near the chromosome ends in the bouquet-stage and then resolved into one or two spots per chromosome as early recombination nodules formed, as previously reported (Figures 9A–D; Blokhina et al., 2019). In smc1b mutant spermatocytes, Rad51 was present in nuclei indicating that early DSB repair machinery necessary for meiotic recombination was active (Figures 9E,F). Quantification of Rad51 signal in mutant and wild-type leptotene stage cells demonstrated no significant differences (Figure 9G). Therefore, the meiotic cohesin complex was not required for initiation of meiotic DSBs in zebrafish spermatocytes.
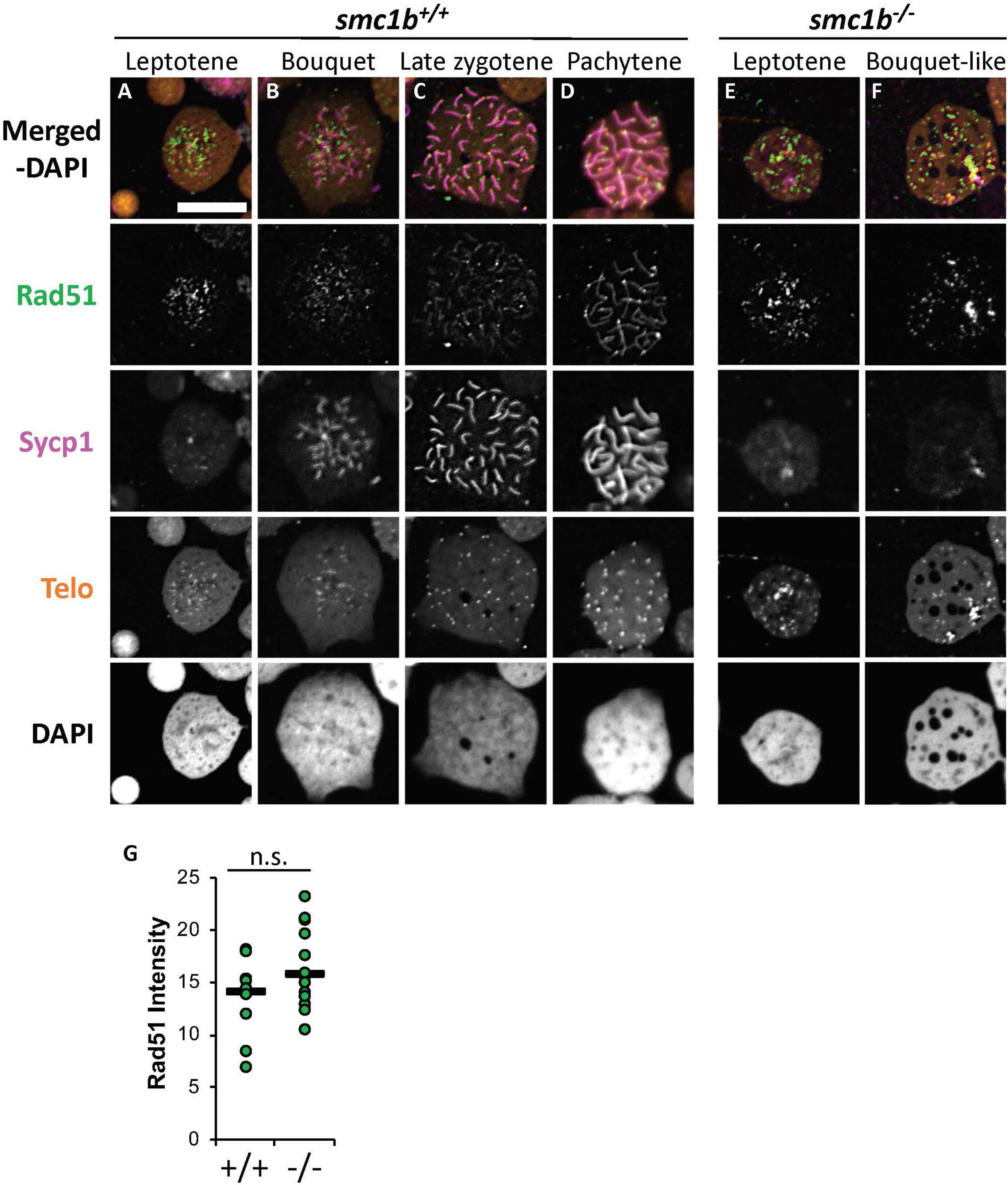
Figure 9. DNA double strand break and recombination marker Rad51 was expressed in smc1bQ198X mutant spermatocytes. Immunolabeling of Rad51 (green) and Sycp1 (magenta) in wild-type and mutant spreads, combined with telomere (telo) in situ hybridization (orange). (A,B) Rad51 protein was detected in wild-type beginning in leptotene stage and was initially located near the chromosome ends in leptotene and bouquet stages. (C,D) In more advanced wild-type spermatocytes, Rad51 localization become more distinct and resolved to one or two spots per chromosome. (E,F) Mutant spermatocytes had detectable levels of Rad51. (G) Quantification of Rad51 signal in wild-type (N = 10) and mutant (N = 15) leptotene stage cells demonstrates there is no significant difference (P = 0.0614). The horizontal bars show the medians. Scale bar is 10 μm.
Discussion
Vertebrate animals harbor two smc1 genes, Smc1α and Smc1β (named smc1a and smc1b in zebrafish). In mammals, SMC1α functions primarily in mitotic divisions, whereas SMC1β predominantly functions in meiosis, although SMC1α has partially overlapping roles with SMC1β in mammalian meiosis (Biswas et al., 2018). The subfunctionalization of these two proteins as mitotic versus meiotic regulators has not been investigated outside of mammals. In this study, we demonstrate that the meiotic cohesin complex protein Smc1b is necessary for meiosis and fertility in zebrafish. Smc1b mutant zebrafish developed as sterile males without any other apparent defects. Histology showed that smc1b mutant spermatocytes entered meiosis but failed to complete the process as there was no sperm. We found that smc1b mutant spermatocytes arrested at the leptotene stage with aberrant homolog pairing and complete failure of synapsis in the mutant cells. Nonetheless, meiotic DSBs still happened in smc1b mutant spermatocytes. Overall, our results indicate that Smc1b is essential for meiotic homologous chromosome pairing and synapsis in zebrafish, demonstrating that Smc1b functions as a meiotic cohesin protein in non-mammalian vertebrates, as has been found in mammals.
Differences in Smc1b Requirement Among Vertebrates
We have shown that smc1b mutant zebrafish are infertile because of a failure to complete meiosis and an inability to produce mature sperm. Our finding is in agreement with previous studies in mammals, which showed that Smc1β mutant male mice were sterile due to lack of spermatids and spermatozoa (Revenkova et al., 2004; Takabayashi et al., 2009). However, zebrafish smc1b mutants displayed more severe phenotypes compared to what has been reported in mouse mutants. For example, zebrafish smc1b–/– spermatocytes arrested at leptotene stage whereas mouse mutant spermatocytes were arrested in pachytene stage and oocytes in metaphase II stage. We found that zebrafish smc1b–/– spermatocytes failed to complete meiotic bouquet formation (early zygonema), even though some cells initiated telomere clustering. Furthermore, failure of synapsis and abnormal pairing between homologous chromosomes occurred in zebrafish smc1b–/– spermatocytes. By contrast, mice lacking SMC1β were able to form a synaptonemal complex (SC) between homologous chromosomes, although it was shorter than in the wild type and showed defects in late recombination markers but not early markers (Revenkova et al., 2004). Zebrafish smc1b–/– spermatocytes arrested in the leptotene stage, which is prior to when recombination takes place. However, we found that meiotic DSBs, which are a pre-requisite of recombination, formed in mutant spermatocytes and are therefore independent of smc1b.
One possible explanation for the discrepancies between the onset of the phenotype in zebrafish and mouse mutants is potential differences in Smc1a protein expression during meiosis. In mice, cohesin complex proteins, including SMC1α and SMC3, are localized to the homologous chromosome pairs in Smc1β mutant spermatocytes, albeit at reduced levels compared to wild type (Revenkova et al., 2004). Furthermore, expression of SMC1α in meiosis-I can partly substitute for SMC1β (Biswas et al., 2018). Zebrafish smc1b mutants may have earlier and more severe defects compared to mouse mutants due to a possible lack of Smc1a expression in spermatocytes. We attempted to label Smc1a protein in zebrafish to ask if Smc1a expression was absent in zebrafish spermatocytes, however, the commercial antibodies we tested (abcam137707) did not work in zebrafish. Smc3 labeling demonstrated that zebrafish Smc3 did not localize to chromosomes in smc1b–/– spermatocytes, indicating that Smc1b is essential for formation of the meiotic cohesin complex in zebrafish. Interestingly, mutations that disrupt the mouse meiotic cohesin complex have similar defects to the zebrafish smc1b mutants. For example, mice that were double mutant for Rec8 and Rad21L or single mutant for Stag3 failed to form axial elements or SCs and arrested in leptonema (Llano et al., 2012; Winters et al., 2014). These data suggest that, in contrast to mice, Smc1b is the only functional Smc1 cohesin subunit in meiotic germ cells in zebrafish.
We also found that there are some differences in smc1b gene expression between zebrafish and mice in non-gonadal tissues. Previous studies reported that mouse SMC1β protein was expressed in the brain, heart, and spleen in addition to gonads (Mannini et al., 2015), but our result showed it primarily expressed in the gonads (Figure 1). In medaka, Smc1b protein was also detected specifically in the ovary and testis, however, the only non-gonadal tissues assayed were the liver and OL32 cells (derived from fin tissue) (Iwai et al., 2004). Further exploration of differences in Smc1b expression across different vertebrate species would be informative in understanding the subfunctionalization of Smc1a and Smc1b between mitotically and meiotically dividing cell types in vertebrates.
We found that Smc3 colocalized with the synaptonemal complex in zebrafish, showing a similar localization pattern to that of mouse spermatocytes (Revenkova et al., 2001). However, in contrast to mice, we did not see Smc3 enrichment near telomeres in wild-type zebrafish spermatocytes (Figure 6). In mice, SMC1β, SMC3, STAG3, and SYCP2 have all been reported to be enriched near the telomeres in meiotic prophase-I (Liebe et al., 2004; Adelfalk et al., 2009). Interestingly, we detected enrichment of Sycp3 near the telomeres, but did not detect enrichment of Smc3 in zebrafish (Figures 5, 6). Cohesin complex enrichment at or near telomeres is thought to maintain telomere integrity and telomere attachment to the nuclear envelope during meiosis—Smc1β mutant spermatocytes exhibited loss of SMC3 telomere enrichment and had defects in telomere attachment and integrity in mice (Adelfalk et al., 2009). The presence of enriched Sycp3 instead of Smc3 in zebrafish indicates that there could be different mechanisms of telomere attachment and maintenance during meiosis in these two species.
Crosstalk Between Cohesin, the Synaptonemal Complex, and DSBs During Zebrafish Meiosis
The reason for failed homolog synapsis and interstitial pairing in zebrafish Smc1b mutants could be impaired interactions between cohesin complex proteins and SC proteins. The vertebrate SC consists of axial element proteins Sycp2 and Sycp3, transverse filament protein Sycp1, and multiple central element proteins (Gao and Colaiácovo, 2018). In mice, cohesin subunits SMC1 and SMC3 physically interact with SC axial element proteins SYCP2 and SYCP3 (Yang and Wang, 2009). In zebrafish spermatocytes, Smc1b is required for Sycp3 and Sycp1 to associate along chromosomes suggesting that interactions between these proteins is necessary for SC formation. This notion is further supported by recent analysis of zebrafish SC mutants, which also show defects in homolog pairing and synapsis (Takemoto et al., 2020; Imai et al., 2021). For instance, spermatocytes mutant for sycp2, encoding an SC axial element protein, failed to form an SC, which disrupted homolog pairing (Takemoto et al., 2020). Interestingly, these mutants did not have the axial element protein Sycp3 associated with chromosomes whereas the transverse filament protein Sycp1 did associate with chromosomes. In the sycp2 mutants, the meiotic cohesin complex protein Rad21l1 co-localized with Sycp1 on chromosomes (Takemoto et al., 2020). We found that Smc1b was necessary for the cohesin complex (Smc3) and Sycp3 to extend along the chromosomes in meiosis and for Sycp1 to associate with chromosomes. Together, these data suggest that the cohesin complex may be involved in recruiting Sycp1 to chromosomes, even in the absence of axial element proteins. Mutations disrupting the SC transverse element protein Sycp1 displayed somewhat less severe defects than those observed for sycp2 and smc1b mutants. Homologous chromosomes initially paired in Sycp1 mutant spermatocytes, however, synapsis did not occur which caused loss of pairing and germ cell arrest at late zygotene/early pachytene stage (Imai et al., 2021). Analysis of these mutants demonstrates that the zebrafish cohesin complex is intimately associated with SC formation and function during meiosis, as has been observed in other organisms.
Smc1b was not required for DSBs suggesting that a functional meiotic cohesin complex is not a prerequisite for meiotic DSBs in zebrafish spermatocytes. DSB formation during meiosis is induced by topoisomerase-type enzyme Spo11 (Keeney et al., 1997). Zebrafish lacking Spo11 formed the meiotic bouquet despite failed DSB formation, however, homolog pairing and synapsis were disrupted in the mutant spermatocytes (Blokhina et al., 2019). Formation of meiotic DSBs was also disrupted in sycp2 mutants. These mutants also displayed failure of the axial element protein, Sycp3, to associate with chromosomes (Takemoto et al., 2020). By contrast, we found that smc1b mutant spermatocytes lacked formation of the chromosome axes but could still form DSBs. Based on these observations, we propose that failure to form chromosome axes does not preclude meiotic DSBs, however, axial element proteins may facilitate Spo11-mediated DSB formation. Despite DSB formation, smc1b mutants spermatocytes arrested in late-leptotene stage with failed pairing and synapsis. Therefore, it is unlikely that DSBs that formed in zebrafish smc1b–/– spermatocytes would resolve into recombination events. These findings are consistent with mouse mutants lacking a meiotic cohesin complex (e.g., Stag3–/– or Rad21l–/–Rec8–/–), where DSBs occurred despite a failure to form chromosome axial elements and a synaptonemal complex (Llano et al., 2012; Winters et al., 2014).
Failure of Female Development in Zebrafish Meiotic Mutants
We found that all smc1b mutant zebrafish developed as male, demonstrating that smc1b is required for female development. In zebrafish, formation of ovarian follicles is a prerequisite for establishment and maintenance of the ovary (Kossack and Draper, 2019). Folliculogenesis involves the separation of oocytes, which are initially clustered together within nests, into individual oocytes each of which is surrounded by somatic cells. Generally, oocytes initiate meiosis while in the nests and arrest in meiosis-I after follicles form (Elkouby and Mullins, 2017). In zebrafish, folliculogenesis begins when oocytes are in the pachytene stage and meiosis arrests in the diplotene stage as oocytes continue to grow and follicles develop (Elkouby et al., 2016). Meiosis-I resumes at the maturation stage, which occurs just prior to ovulation in zebrafish (stage IV), then arrests again in meiosis-II until fertilization takes place (Selman et al., 1993). Multiple meiotic mutants have all or mostly male phenotypes in zebrafish, including mutants disrupting SC encoding genes sypc1 and sycp2, as well as a cohesin complex encoding gene rad21l1 (Takemoto et al., 2020; Blokhina et al., 2021; Imai et al., 2021). In mutants affecting SC components, oogenesis was initiated, as juvenile fish had ovarian follicles at 40 dpf (sycp1 –/–) and 28 dpf (sycp2 –/–) (Takemoto et al., 2020; Imai et al., 2021). Therefore, in the sycp1 and sycp2 mutants, oogenesis did not proceed to stages that could support ovary development into adulthood. In rad21l1 mutants, which is the zebrafish ortholog of mouse Rad21l, nearly all fish developed as fertile adult males, suggesting a function in oogenesis but not spermatogenesis (Blokhina et al., 2021). During development, most rad21l1 mutant fish initially had immature oocytes demonstrating that, similar to sycp1 and sycp2 mutants, oogenesis could proceed past the pachytene stage but did not mature to stages capable of supporting oogenesis in most fish. Male rad21l1 mutants had minor defects in synapsis, however, this did not have a major impact on spermatogenesis or fertility (Blokhina et al., 2021). These data suggest that there could be sex-specific functions of different meiotic cohesin complexes in zebrafish with Rad21l1 containing complexes being essential for oogenesis whereas meiotic cohesin complexes consisting of Rec8a and/or Rec8b may be important for spermatogenesis or for both spermatogenesis and oogenesis. In both spo11 and mlh1 mutant zebrafish, fish developed into adult males and females (Feitsma et al., 2007; Blokhina et al., 2019). The mlh1 mutant zebrafish could complete meiosis but showed recombination defects and produced aneuploid progeny (Feitsma et al., 2007; Leal et al., 2008). Thus, sex reversal is not universal in zebrafish meiotic mutants, but it is a common phenotype in mutants that fail to complete meiosis. Previous studies found that smc1b was expressed in bipotential gonads of juvenile zebrafish, therefore it is likely to play an important role in meiosis in the juvenile ovary (Tzung et al., 2015). We found that ovarian follicles failed to form in smc1b–/– gonads. Because smc1b mutants did not form ovarian follicles in juvenile fish, it is likely that oocytes arrested prior to the pachytene stage.
Data Availability Statement
The original contributions presented in the study are included in the article/Supplementary Material, further inquiries can be directed to the corresponding author/s.
Ethics Statement
The animal study was reviewed and approved by UMass Boston Institutional Animal Care and Use Committee.
Author Contributions
KI contributed to the data shown in all figures. MM contributed to the data shown in Figures 2, 3. KI and KS designed the experiments, analyzed the data, and wrote the manuscript. All authors contributed to the article and approved the submitted version.
Funding
This work was supported by the National Institute of Health—NICHD award 1R15HD095735-01. KI was also supported by a CSM Dean’s Doctoral Research Fellowship. MM was supported by a McCone and Alumni Research Fellowship from the Department of Biology and a CSM Research Fellowship from the College of Science and Mathematics at UMass Boston.
Conflict of Interest
The authors declare that the research was conducted in the absence of any commercial or financial relationships that could be construed as a potential conflict of interest.
Publisher’s Note
All claims expressed in this article are solely those of the authors and do not necessarily represent those of their affiliated organizations, or those of the publisher, the editors and the reviewers. Any product that may be evaluated in this article, or claim that may be made by its manufacturer, is not guaranteed or endorsed by the publisher.
Acknowledgments
We would like to thank Sean Burgess (U. C. Davis) and James Amatruda (University of Southern California) for providing Sycp1 and γ-H2AX antibodies, respectively. We would also like to thank the Zebrafish Mutation Project for generating the smc1b mutants and to the Zebrafish International Resource Center (ZIRC) for supplying smc1b mutant zebrafish. We would also like to thank Jessica MacNeil and Kavita Venkataramani for helpful discussions about the manuscript.
Supplementary Material
The Supplementary Material for this article can be found online at: https://www.frontiersin.org/articles/10.3389/fcell.2021.714245/full#supplementary-material
Supplementary Figure 1 | Conservation of key motifs in vertebrate Smc1b proteins. (A) Graphical representation of zebrafish Smc1b protein. Numbers inside boxes represent amino acid position in the corresponding domain. (B) Percentage of identical amino acid residues between zebrafish (z) and either human (h) or mouse (m) Smc1b proteins. (C–E) Protein sequence alignments of the N-terminal ATP binding domain (C), flexible hinge domain (D), and C-terminal ATP binding domain (E). The conserved motifs are highlighted in green. The consensus motif sequences are Walker A: GxxGxGK (S/T), ABC transporter signature motif: LSGG(E/Q) (K/R), Walker B: hhhhDE, where x is any of 20 amino acid and h is any hydrophobic residue.
Footnotes
References
Adelfalk, C., Janschek, J., Revenkova, E., Blei, C., Liebe, B., Göb, E., et al. (2009). Cohesin SMC1β protects telomeres in meiocytes. J. Cell Biol. 187, 185–199. doi: 10.1083/jcb.200808016
Akhmedov, A. T., Frei, C., Tsai-Pflugfelder, M., Kemper, B., Gasser, S. M., and Jessberger, R. (1998). Structural maintenance of chromosomes protein C-terminal domains bind preferentially to DNA with secondary structure. J. Biol. Chem. 273, 24088–24094. doi: 10.1074/jbc.273.37.24088
Alleva, B., and Smolikove, S. (2017). Moving and stopping: regulation of chromosome movement to promote meiotic chromosome pairing and synapsis. Nucleus 8, 613–624. doi: 10.1080/19491034.2017.1358329
Biswas, U., Stevense, M., and Jessberger, R. (2018). SMC1α Substitutes for Many Meiotic Functions of SMC1β but Cannot Protect Telomeres from Damage. Curr. Biol. 28, 249–261.e4. doi: 10.1016/j.cub.2017.12.020
Blokhina, Y. P., Frees, M. A., Nguyen, A., Sharifi, M., Chu, D. B., Bispo, K., et al. (2021). Rad21l1 cohesin subunit is dispensable for spermatogenesis but not oogenesis in zebrafish. PLoS Genet. 17:e1009127. doi: 10.1371/journal.pgen.1009127
Blokhina, Y. P., Nguyen, A. D., Draper, B. W., and Burgess, S. M. (2019). The telomere bouquet is a hub where meiotic double-strand breaks, synapsis, and stable homolog juxtaposition are coordinated in the zebrafish, Danio rerio. PLoS Genet. 15:e1007730. doi: 10.1371/journal.pgen.1007730
Blokhina, Y. P., Olaya, I., and Burgess, S. M. (2020). Preparation of meiotic chromosome spreads from zebrafish spermatocytes. J. Vis. Exp. 157:10.3791/60671. doi: 10.3791/60671
Bolcun-Filas, E., and Handel, M. A. (2018). Meiosis: the chromosomal foundation of reproduction. Biol. Reprod. 99, 112–126. doi: 10.1093/biolre/ioy021
Burke, B. (2018). LINC complexes as regulators of meiosis. Curr. Opin. Cell Biol. 52, 22–29. doi: 10.1016/j.ceb.2018.01.005
Chao, L. F. I., Singh, M., Thompson, J., Yates, J. R., and Hagstrom, K. A. (2017). An SMC-like protein binds and regulates Caenorhabditis elegans condensins. PLoS Genet. 13:e1006614. doi: 10.1371/journal.pgen.1006614
Crickard, J. B., and Greene, E. C. (2018). Biochemical attributes of mitotic and meiotic presynaptic complexes. DNA Repair (Amst). 71, 148–157. doi: 10.1016/j.dnarep.2018.08.018
Dranow, D. B., Tucker, R. P., and Draper, B. W. (2013). Germ cells are required to maintain a stable sexual phenotype in adult zebrafish. Dev. Biol. 376, 43–50. doi: 10.1016/j.ydbio.2013.01.016
Elkouby, Y. M., Jamieson-Lucy, A., and Mullins, M. C. (2016). Oocyte Polarization Is Coupled to the Chromosomal Bouquet, a Conserved Polarized Nuclear Configuration in Meiosis. PLoS Biol. 14:e1002335. doi: 10.1371/journal.pbio.1002335
Elkouby, Y. M., and Mullins, M. C. (2017). Coordination of cellular differentiation, polarity, mitosis and meiosis – New findings from early vertebrate oogenesis. Dev. Biol. 430, 275–287. doi: 10.1016/j.ydbio.2017.06.029
Feitsma, H., Leal, M. C., Moens, P. B., Cuppen, E., and Schulz, R. W. (2007). Mlh1 Deficiency in Zebrafish Results in Male Sterility and Aneuploid as Well as Triploid Progeny in Females. Genetics 175, 1561–1569. doi: 10.1534/genetics.106.068171
Gao, J., and Colaiácovo, M. P. (2018). Zipping and Unzipping: protein modifications regulating synaptonemal complex dynamics. Trends Genet. 34, 232–245. doi: 10.1016/j.tig.2017.12.001
Hamer, G., Roepers-Gajadien, H. L., Van Duyn-Goedhart, A., Gademan, I. S., Kal, H. B., Van Buul, P. P. W., et al. (2003). DNA double-strand breaks and γ-H2AX signaling in the testis. Biol. Reprod. 68, 628–634. doi: 10.1095/biolreprod.102.008672
Howard-Till, R. A., Lukaszewicz, A., and Loidl, J. (2011). The recombinases Rad51 and Dmc1 play distinct roles in DNA break repair and recombination partner choice in the meiosis of Tetrahymena. PLoS Genet. 7:e1001359. doi: 10.1371/journal.pgen.1001359
Imai, Y., Saito, K., Takemoto, K., Velilla, F., Kawasaki, T., Ishiguro, K. I., et al. (2021). Sycp1 Is Not Required for Subtelomeric DNA Double-Strand Breaks but Is Required for Homologous Alignment in Zebrafish Spermatocytes. Front. Cell Dev. Biol. 9:664377. doi: 10.3389/fcell.2021.664377
Ishiguro, K. I. (2019). The cohesin complex in mammalian meiosis. Genes Cells 24, 6–30. doi: 10.1111/gtc.12652
Iwai, T., Lee, J., Yoshii, A., Yokota, T., Mita, K., and Yamashita, M. (2004). Changes in the expression and localization of cohesin subunits during meiosis in a non-mammalian vertebrate, the medaka fish. Gene Expr. Patterns 4, 495–504. doi: 10.1016/j.modgep.2004.03.004
Keeney, S., Giroux, C. N., and Kleckner, N. (1997). Meiosis-specific DNA double-strand breaks are catalyzed by Spo11, a member of a widely conserved protein family. Cell 88, 375–384. doi: 10.1016/S0092-8674(00)81876-0
Kossack, M. E., and Draper, B. W. (2019). Genetic regulation of sex determination and maintenance in zebrafish (Danio rerio). Curr. Top. Dev. Biol. 134, 119–149. doi: 10.1016/bs.ctdb.2019.02.004
Leal, M. C., Feitsma, H., Cuppen, E., França, L. R., and Schulz, R. W. (2008). Completion of meiosis in male zebrafish (Danio rerio) despite lack of DNA mismatch repair gene mlh1. Cell Tissue Res. 332, 133–139. doi: 10.1007/s00441-007-0550-z
Liebe, B., Alsheimer, M., Höög, C., Benavente, R., and Scherthan, H. (2004). Telomere Attachment, Meiotic Chromosome Condensation, Pairing, and Bouquet Stage Duration Are Modified in Spermatocytes Lacking Axial Elements. Mol. Biol. Cell 15, 827–837. doi: 10.1091/mbc.E03-07-0524
Llano, E., Herrán, Y., García-Tuñón, I., Gutiérrez-Caballero, C., de Álava, E., Barbero, J. L., et al. (2012). Meiotic cohesin complexes are essential for the formation of the axial element in mice. J. Cell Biol. 197, 877–885. doi: 10.1083/jcb.201201100
Mannini, L., Cucco, F., Quarantotti, V., Amato, C., Tinti, M., Tana, L., et al. (2015). SMC1B is present in mammalian somatic cells and interacts with mitotic cohesin proteins. Sci. Rep. 5, 1–11. doi: 10.1038/srep18472
Nasmyth, K. (2001). Disseminating the genome: joining, resolving, and separating sister chromatids during mitosis and meiosis. Annu. Rev. Genet. 35, 673–745. doi: 10.1146/annurev.genet.35.102401.091334
Neff, M. M., Turk, E., and Kalishman, M. (2002). Web-based primer design for single nucleotide polymorphism analysis. Trends Genet. 18, 613–615. doi: 10.1016/S0168-9525(02)02820-2
Neumann, J. C., Chandler, G. L., Damoulis, V. A., Fustinoa, N. J., Lillard, K., Looijenga, L., et al. (2011). Mutation in the type IB bone morphogenetic protein receptor alk6b impairs germ-cell differentiation and causes germ-cell tumors in zebrafish. Proc. Natl. Acad. Sci. U. S. A. 108, 13153–13158. doi: 10.1073/pnas.1102311108
Rankin, S. (2015). Complex elaboration: making sense of meiotic cohesin dynamics. FEBS J. 282, 2413–2430. doi: 10.1111/febs.13301
Revenkova, E., Eijpe, M., Heyting, C., Gross, B., and Jessberger, R. (2001). Novel Meiosis-Specific Isoform of Mammalian SMC1. Mol. Cell. Biol. 21, 6984–6998. doi: 10.1128/mcb.21.20.6984-6998.2001
Revenkova, E., Eijpe, M., Heyting, C., Hodges, C. A., Hunt, P. A., Liebe, B., et al. (2004). Cohesin SMC1β is required for meiotic chromosome dynamics, sister chromatid cohesion and DNA recombination. Nat. Cell Biol. 6, 555–562. doi: 10.1038/ncb1135
Rodríguez-Marí, A., Cañestro, C., Bremiller, R. A., Nguyen-Johnson, A., Asakawa, K., Kawakami, K., et al. (2010). Sex reversal in zebrafish fancl mutants is caused by Tp53-mediated germ cell apoptosis. PLoS Genet. 6:e1001034. doi: 10.1371/journal.pgen.1001034
Saito, K., Sakai, C., Kawasaki, T., and Sakai, N. (2014). Telomere distribution pattern and synapsis initiation during spermatogenesis in zebrafish. Dev. Dyn. 243, 1448–1456. doi: 10.1002/dvdy.24166
Saito, K., Siegfried, K. R., Nüsslein-Volhard, C., and Sakai, N. (2011). Isolation and cytogenetic characterization of zebrafish meiotic prophase I mutants. Dev. Dyn. 240, 1779–1792. doi: 10.1002/dvdy.22661
Selman, K., Wallace, R. A., Sarka, A., and Qi, X. (1993). Stages of oocyte development in the zebrafish, Brachydanio rerio. J. Morphol. 218, 203–224. doi: 10.1002/jmor.1052180209
Siegfried, K. R., and Nüsslein-Volhard, C. (2008). Germ line control of female sex determination in zebrafish. Dev. Biol. 324, 277–287. doi: 10.1016/j.ydbio.2008.09.025
Takabayashi, S., Yamauchi, Y., Tsume, M., Noguchi, M., and Katoh, H. (2009). A spontaneous Smc1b mutation causes cohesin protein dysfunction and sterility in mice. Exp. Biol. Med. 234, 994–1001. doi: 10.3181/0808-RM-244
Takahashi, H. (1977). Juvenile Hermaphroditism in the Zebrafish, Brachydanio rerio. Bull. Fac. Fish. Hokkaido Univ. 28, 57–65.
Takemoto, K., Imai, Y., Saito, K., Kawasaki, T., Carlton, P. M., Ishiguro, K. I., et al. (2020). Sycp2 is essential for synaptonemal complex assembly, early meiotic recombination and homologous pairing in zebrafish spermatocytes. PLoS Genet. 16:e1008640. doi: 10.1371/journal.pgen.1008640
Tang, R., Dodd, A., Lai, D., McNabb, W. C., and Love, D. R. (2007). Validation of zebrafish (Danio rerio) reference genes for quantitative real-time RT-PCR normalization. Acta Biochim. Biophys. Sin. (Shanghai) 39, 384–390. doi: 10.1111/j.1745-7270.2007.00283.x
Tzung, K.-W., Goto, R., Saju, J. M., Sreenivasan, R., Saito, T., Arai, K., et al. (2015). Early Depletion of Primordial Germ Cells in Zebrafish Promotes Testis Formation. Stem Cell Rep. 4, 61–73. doi: 10.1016/j.stemcr.2014.10.011
Webster, K. A., Henke, K., Ingalls, D. M., Nahrin, A., Harris, M. P., and Siegfried, K. R. (2019). Cyclin-dependent kinase 21 is a novel regulator of proliferation and meiosis in the male germline of zebrafish. Reproduction 157, 383–398. doi: 10.1530/REP-18-0386
Winters, T., McNicoll, F., and Jessberger, R. (2014). Meiotic cohesin STAG3 is required for chromosome axis formation and sister chromatid cohesion. EMBO J. 33, 1256–1270. doi: 10.1002/embj.201387330
Keywords: cohesin, smc1b, meiosis, zebrafish, testis, spermatogenesis, germ cells
Citation: Islam KN, Modi MM and Siegfried KR (2021) The Zebrafish Meiotic Cohesin Complex Protein Smc1b Is Required for Key Events in Meiotic Prophase I. Front. Cell Dev. Biol. 9:714245. doi: 10.3389/fcell.2021.714245
Received: 24 May 2021; Accepted: 15 July 2021;
Published: 09 August 2021.
Edited by:
Sean Burgess, University of California, Davis, United StatesCopyright © 2021 Islam, Modi and Siegfried. This is an open-access article distributed under the terms of the Creative Commons Attribution License (CC BY). The use, distribution or reproduction in other forums is permitted, provided the original author(s) and the copyright owner(s) are credited and that the original publication in this journal is cited, in accordance with accepted academic practice. No use, distribution or reproduction is permitted which does not comply with these terms.
*Correspondence: Kellee Renee Siegfried, a2VsbGVlLnNpZWdmcmllZEB1bWIuZWR1