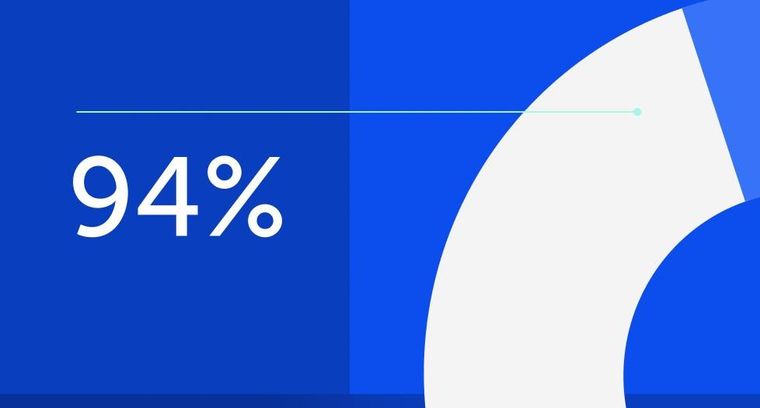
94% of researchers rate our articles as excellent or good
Learn more about the work of our research integrity team to safeguard the quality of each article we publish.
Find out more
BRIEF RESEARCH REPORT article
Front. Cell Dev. Biol., 28 October 2021
Sec. Cell Growth and Division
Volume 9 - 2021 | https://doi.org/10.3389/fcell.2021.713415
This article is part of the Research TopicNuclear Genome Stability: DNA Replication, Telomere Maintenance, and DNA RepairView all 21 articles
A correction has been applied to this article in:
Corrigendum: Possible Involvement of Hsp90 in the Regulation of Telomere Length and Telomerase Activity During the Leishmania Amazonensis Developmental Cycle and Population Proliferation
The Leishmania developmental cycle comprises three main life forms in two hosts, indicating that the parasite is continually challenged due to drastic environmental changes. The disruption of this cycle is critical for discovering new therapies to eradicate leishmaniasis, a neglected disease that affects millions worldwide. Telomeres, the physical ends of chromosomes, maintain genome stability and cell proliferation and are potential antiparasitic drug targets. Therefore, understanding how telomere length is regulated during parasite development is vital. Here, we show that telomeres form clusters spread in the nucleoplasm of the three parasite life forms. We also observed that amastigotes telomeres are shorter than metacyclic and procyclic promastigotes and that in parasites with continuous in vitro passages, telomere length increases over time. These observed differences in telomere length among parasite’s life stages were not due to lack/inhibition of telomerase since enzyme activity was detected in all parasite life stages, although the catalysis was temperature-dependent. These data led us to test if, similar to other eukaryotes, parasite telomere length maintenance could be regulated by Hsp83, the ortholog of Hsp90 in trypanosomatids, and Leishmania (LHsp90). Parasites were then treated with the Hsp90 inhibitor 17AAG. The results showed that 17AAG disturbed parasite growth, induced accumulation into G2/M phases, and telomere shortening in a time-dependent manner. It has also inhibited procyclic promastigote’s telomerase activity. Besides, LHsp90 interacts with the telomerase TERT component as shown by immunoprecipitation, strongly suggesting a new role for LHsp90 as a parasite telomerase component involved in controlling telomere length maintenance and parasite life span.
Leishmaniases are a group of infectious diseases caused by parasites of the genus Leishmania, affecting millions of people worldwide. The disease has a broad spectrum of clinical manifestations varying from asymptomatic, self-limited skin lesions to systemic and fatal infections (Kevric et al., 2015). The parasite’s sequential developmental cycle involves three main life forms: procyclic promastigotes (PP), metacyclic promastigotes (MP), and amastigotes (Am). In general, the non-infective (but highly proliferative) PP lives in the phlebotomine sandflies’ midgut (invertebrate host). They can transform into non-proliferative MP, infecting mammals (vertebrate hosts) and transforming into Am. Am live and multiply inside the vertebrate host cells and infect new cells and other sandflies in new rounds of infection (Sacks and Perkins, 1984; Alvar et al., 2012). Therefore, to survive and complete the developmental cycle, Leishmania spp. needs to adapt to drastic environmental changes during the transition from invertebrate to the vertebrate host. Alterations in the temperature and pH, which affect cell morphology, cell surface, and metabolism, including gene expression and the activity of some enzymes, are among the challenges parasites pass through to survive (Sacks and Kamhawi, 2001; Wheeler et al., 2010; Dillon et al., 2015; Inbar et al., 2017). Interestingly, Am and MP, the infective forms, are close-related regarding gene expression than PP, which cannot infect cells (Inbar et al., 2017).
Despite its global distribution, to date, there are few strategies to treat and control leishmaniasis. Most antileishmanial treatments are based on drugs developed several years ago, presenting side effects and leading to drug resistance. Also, few human vaccines in clinical trials demonstrate the urgency of finding new anti-parasite therapies that are less toxic and more active (Hotez et al., 2016; Moafi et al., 2019; World Health Organization [WHO], 2020). Thus, an increasing interest in studying telomeres as drug design targets has emerged (Olaussen et al., 2006; Chen et al., 2009; Sekaran et al., 2014; Dey and Chakrabarti, 2018). Telomeres are protein-DNA complexes that protect chromosome ends from degradation and fusion (Giardini et al., 2014). Chromosome ends in Leishmania, like most eukaryotes, are composed of conserved 5’-TTAGGG-3’ telomeric repeats maintained by telomerase (Fu and Barker, 1998; Cano et al., 1999; Conte and Cano, 2005). Telomerase is a ribonucleoprotein enzyme minimally composed of two catalytically subunits: the telomerase reverse transcriptase protein (TERT) and the telomerase RNA (TER), which contains the template that specifies the sequence of the telomeric repeats (Feng et al., 1995; Giardini et al., 2014). Both components were already identified and partially characterized in Leishmania sp., showing conserved and genus-specific features (Giardini et al., 2006; Vasconcelos et al., 2014). For example, the Leishmania telomerase RNA component (LeishTER), similarly to Trypanosoma brucei, was shown to be processed by trans-splicing. Still, its primary sequence, including the template sequence, is only conserved within the Leishmania genus (Vasconcelos et al., 2014). Also, although the purified parasite’s telomerase activity presents catalytic properties shared with telomerase described in model organisms (Cano et al., 1999), it also contains enzymatic characteristics specific to the genus (Giardini et al., 2011). All these identified features can elect parasite telomerase as a potential drug target.
Here we show that in L. amazonensis, telomeres’ foci are spread in the nucleoplasm of all life stages. Intriguingly, telomeres from Am and MP are shorter than PP, and telomere length increases in parasites with continuous in vitro passages. However, telomerase activity was detected in all parasite life stages. Furthermore, it showed to be life-stage dependent, strongly suggesting that parasite telomere length is regulated during development by a still unknown factor. Therefore, based on the literature, we speculate if Hsp90, considered one of the players involved in controlling telomerase activity in model organisms, could be implicated in parasite telomere maintenance (Holt et al., 1999; Forsythe et al., 2001; Keppler et al., 2006; Toogun et al., 2008; Viviescas et al., 2019). The ortholog of Hsp90 in Leishmania spp. and other trypanosomatids is Hsp83, which is important for parasite growth and the differentiation of PP into Am forms (Brandau et al., 1995; Wiesgigl and Clos, 2001; Zilka et al., 2001). Besides, it is known that heat shock stress and the inhibition of Leishmania Hsp90 (LHsp90) by geldanamycin and its analogs induce parasite death and, in some species, apoptosis-like death (Wiesgigl and Clos, 2001; Li et al., 2009; de Petersen et al., 2012; Santos et al., 2014). To test the hypothesis that LHsp90 could regulate telomere length maintenance in L. amazonensis, we treated parasites with 17AAG, a geldanamycin analog. 17AAG inhibits most Hsp90 by binding the ATP/ADP domain located at the N-terminal region of the protein (Grenert et al., 1997). In Leishmania spp., 17AAG shows a stronger preference to bind the N-terminal domain of LHsp90 (Palma et al., 2019). Our findings suggest that LHsp90 plays a conserved role in L. amazonensis telomeres maintenance since its inhibition impaired parasite growth, induced cell cycle arrest, caused telomere shortening, and inhibited in vitro telomerase activity. Besides, LHsp90 co-immunoprecipitated with the telomerase TERT component, strongly suggesting that it is part of the complex and may be involved in parasite telomere length maintenance.
All three L. amazonensis (strain MHOM/BR/1973/M2269) life forms were obtained from the same developmental cycle. PP was obtained from lesion-derived Am and cultivated at 28°C in M199 medium (Gibco) supplemented with 10% fetal calf serum (Cultilab). MP was obtained from PP stationary cultures using agglutination with peanut lectin (Sacks and Perkins, 1984). Am was obtained from mice footpad lesions as described before (Barbiéri et al., 1990). PP passage 1 (P1) was obtained from newly in vitro-transformed PP and was maintained in continuous passages. PP from passages 2, 4, 6, and 8 represent the continuous cultivation (every 4 days) of PP from passage 1 in exponential growth. MP from passages 1, 2, 4, 6, and 8 were obtained from PP passages 1, 2, 4, 6, and 8 in the stationary growth phase (tenth day).
The telomere fluorescence in situ hybridization was performed as described before (Siqueira Neto et al., 2007; da Silva et al., 2010), with minor modifications. Exponentially growing L. amazonensis PP from passage 6 (∼ 1 × 106 cells), ∼ 1 × 105 MP from passage 6, and ∼ 1 × 105 lesion-derived Am were harvested at 2,500 x g for 5 min at 4∘C, washed in PBS (137 mM NaCl, 2.7 mM KCl, 10 mM Na2HPO4, and 2 mM KH2PO4, pH 7.4) and fixed in 1% formaldehyde for 5 min at 4°C. After permeabilization with 0.1% Triton X-100 for 10 min at room temperature, the parasites were incubated with 0.1 M glycine for 5 min at room temperature. Parasites were attached to glass coverslips, and FISH reactions were done using an 18-mer PNA (Peptide Nucleic Acid) FITC-labeled telomeric oligoprobe (CCCTAA)3 (PANAGENE). DNA in the nucleus and kinetoplast were stained with Vectashield® mounting medium DAPI (Vector Labs). The images were analyzed using a Nikon 80i fluorescence microscope and were superimposed using NIS elements software (v. Ar 3.10).
To estimate the IC50 (Half-maximal inhibitory concentration) of 17AAG (Cayman Chemical) for L. amazonensis PP (passage 6), we used PrestoBlue (Invitrogen), supplied as a 10X solution, according to the manufacturer’s instructions. Briefly, exponentially growing PP from passage 6 (∼3 × 106 cells) in M199 medium supplemented with 10% FCS (80 μL), were incubated with 10 μL of increased concentrations of 17AAG (25, 50, 100, 150, 200, 250, 300, 350, 400, and 500 nM) diluted in 90% methanol and deposited in 96 well plates. After 48 h at 28°C, 10 μL of 10X PrestoBlue was added to each well followed by incubation for 10 min at 28°C. The viability of the cells was detected by measuring Absorbance 570 nm. The percentage of viable parasites was plotted against the log of the drug concentration, and the IC50 was analyzed using GraphPad Prism 8.0. Assays were done in triplicate, and as controls, we used wild-type cells (wt) and methanol-treated cells (meth-treated).
Genomic DNA (gDNA) from the three L. amazonensis life forms, PP and MP from continuous passages, and PP (passage 6) tested in all different conditions were obtained using the DNeasy Blood and Tissue kit (Qiagen). The integrity of each DNA sample was confirmed by fractionation in 0.8% agarose gels (Supplementary Figures 1A,B). DNA samples (1.0 μg each) were resuspended in 1X TE and digested with 10 U AfaI (Thermo Scientific) at 37°C overnight to liberate the chromosome end termini (Conte and Cano, 2005). Terminal restriction fragments (TRF) were fractionated onto a 0.8% agarose gel and transferred to Hybond N + nylon membranes. Southern blots were done using AfaI-digested DNA fragments hybridized with a DIG-labeled telomeric (TEL) probe (5′-TTAGGG3-3′). Membranes were stripped and re-hybridized with a PCR fragment of L. amazonensis GAPDH (Fw: 5′ GAAGGACTGGCGCGGTGGCCGCGCG 3′, and Rv: 5′ CCACGGCCTTGGCGGCGCCGGTCG 3′) labeled using the PCR DIG probe synthesis kit (Roche). The hybridization signals were developed with an anti-DIG-HRP conjugate antibody (Roche) and CPD-Star (Roche). The average TRF was determined by comparing the TRF location on the blot relative to the DNA molecular weight marker VII DIG-labeled (Roche).
Three biological replicates of each sample: L. amazonensis PP from passage 6 (∼ 2 × 106 cells) in exponential growth (wt), meth-treated, and treated for 48 and 96 h with 100 and 200 nM 17AAG were analyzed. Parasite samples were harvested at 2,500 x g for 5 min at 4∘C, washed in PBS, fixed in 90% methanol for 30 min at −20∘C, washed again with PBS, and suspended in PBS. Cells were incubated with 10 μg/ml RNase A (Invitrogen) for 30 min at 37°C. DNA content in each cell cycle phase was estimated by staining cells with 10 μg/ml propidium iodide for 40 min at 37°C (Sigma) followed by flow cytometry analysis using Accuri C6 plus flow cytometer (BD Biosciences). Flow Jo was used for data analysis and to construct the histograms (events x FL2 area). A total of 20,000 events were analyzed for each sample with a similar variance between the groups. Raw data from flow cytometry experiments are available from the corresponding author on request.
Flow-Fish was used to quantitatively estimate telomeres length from the three L. amazonensis life forms in different conditions: meth-treated PP (passage 6) and PP (passage 6) treated for 48 and 96 h with 100 and 200 nM 17AAG. Assays were done in triplicates using three biological replicates of each sample treated on different days, using a modification of the Baerlocher et al. method (Baerlocher et al., 2006; da Silva et al., 2017). As described before (da Silva et al., 2017), human leukocytes (1 × 106 cells) with a known telomere length were mixed with each parasite sample to allow proper discrimination of parasites and controls. FISH reactions were done using an 18 mer PNA FITC-labeled telomeric oligoprobe (CCCTAA)3 (PANAGENE). Results were analyzed using Accuri C6 plus flow cytometer (BayBioscences, Kobe, Japan). Quantum FITC beads (Bangs Laboratories) were used to calculate the fluorescence recorded for each sample, the MFI (Mean Fluorescence Intensity), which was converted into equivalent MESF (Molecules Equivalent of Soluble Fluorochrome) according to Baerlocher et al. (2006). The results were also represented as fluorescent histograms, and the morphometric parameters (FSC-area x FSC-height) allowed to discriminate and select cells within different groups. Raw data from flow fish experiments are available from the corresponding author on request.
Protein extracts were obtained from L. amazonensis lesion-derived Am and, PP and MP both from passage 6. Cells (∼ 1 × 109) were harvested by centrifugation, washed in ice-cold PBS supplemented with 2% glucose (PBS-G), and resuspended in buffer A (20 mM Tris-HCl pH 7.5, 1 mM EGTA pH 8.0, 1 mM EDTA pH 8.0, 1 mM spermidine, 0, 3 M spermine, 1 mM DTT, 15 mM NaCl) containing 1 X protease inhibitor cocktail (Sigma), followed by incubation for 30 min on ice. Protein extracts were prepared in buffer A containing 0.5% NP-40 v/v. Total protein concentration of each extract was determined by reading OD280nm. Finally, telomerase positive extracts were purified in DEAE-sepharose columns using a standard protocol (Cano et al., 1999; Giardini et al., 2011).
Western blot assays (Supplementary Figure 4) were done with approximately 300 μg of each telomerase-positive extracts fractionated in 10% SDS-PAGE and transferred to nitrocellulose membranes (BioRad). Extracts were probed with rabbit-produced polyclonal sera against recombinant L. amazonensis TERT (N-terminal region) (1:8,000 dilution) (produced by our research group), and recombinant L. braziliensis Hsp90 (1:5,000 dilution) (Seraphim et al., 2013). As secondary antibody, we used goat anti-rabbit HRP-conjugated (Bio-Rad) (1:30,000 dilution) and ECL reagent (GE).
Co-immunoprecipitation assays were performed using 20 μg of each telomerase positive protein extracts (input), 5 μg each polyclonal anti-LbHsp90 or anti-LaTERT sera, and DynaBeads protein A (Thermo Fisher). Protein extracts were incubated at 37∘C for 2 h with protein A-coupled with the specific serum in the presence of IP buffer (25 mM TRIS-HCl pH 8.0, 2 mM EDTA pH 8.0, 10% glycerol, 1 X protease inhibitor cocktail). Samples were collected and fractionated in 10% SDS-PAGE. The presence of TERT and Hsp90 in the Co-IP fractions was detected by western blot revealed with anti-LbHsp90 (1:5,000 dilution) and anti-LaTERT (1:8,000 dilution) sera.
Telomerase activity assay was performed using a modified one-tube telomere repeat amplification protocol (TRAP) assay and DEAE semi-purified extracts (Cano et al., 1999; Giardini et al., 2011) obtained from the three life forms (Am and, PP and MP both from passage 6). A 5′-DIG-labeled TS primer (5′-AATCCGTCGAGCAGAGTT-3′) was used as the template for the telomerase activity step. The Cx-extend primer [5′-GTG (CCCTTA)3CCCTAA-3′] was used as the reverse primer in the PCR reaction step. The amount of protein in the DEAE semi-purified extracts used in each reaction was around 1 μg and control reactions were done without extracts or TS primer. Telomerase activity was tested by pre-incubating the extracts with 200 ng DNase-free RNase A (Sigma) for 5 min at 37∘C. Amplified telomerase products were fractionated in 10% non-denaturing PAGE (19:1, acrylamide:bis-acrylamide) in 1X TBE (100 mM Tris, 100 mM Boric acid, 2 mM EDTA disodium salt), transferred to nylon membranes, and incubated with anti-DIG-HRP conjugate antibody (Roche). Assays were developed using CPDstar (Roche).
PP (passage 6) extracts (∼1 μg) were also pre-incubated with 100 nM 17AAG for 30 min at 28°C before performing the TRAP assay to test if the inhibition of LHsp90 could disturb telomerase activity.
Using fluorescence in situ hybridization (FISH), we found that parasite telomeres are distributed in the nucleus of the three main L. amazonensis life forms, organized in multiple foci, as well as individual dots, or forming indistinct clusters. Telomeres distribution showed similar patterns throughout the cell cycle phases in Am and PP (Figure 1A). However, in MP, they were observed only at G1/G0 phase because this is a non-replicative form (Sacks and Perkins, 1984; Alvar et al., 2012) and, therefore, stays in a quiescent-like state.
Figure 1. L. amazonensis telomeres distribution and size during development and continuous passages. (A) Distribution of L. amazonensis telomeres in three parasites developmental stage obtained using telomeric FISH. Telomeres were hybridized with a FITC-labeled telomeric oligoprobe (CCCTAA)3 (PANAGENE). DNA in the nucleus and kinetoplast were stained with Vectashield® mounting medium DAPI (Vector Labs). The images were analyzed using a Nikon 80i fluorescence microscope and were superimposed using NIS elements software (v. Ar 3.10). Bar 2 μm. Telomeres are shown to form indistinct clusters and individual foci independent of the cell cycle phase and parasite life stages. (B) gDNA (1.0 μg) of lesion-derived Am, PP (passages 1–8), and MP (passages 1–8) were digested with AfaI (10 U). DNA fragments were separated on 0.8% ethidium bromide (EtBr)-stained agarose gel. Southern blotting hybridization was done with a DIG-labeled telomeric probe (5′-TTAGGG3-3′). As the loading control, a DIG-labeled probe that recognizes the L. amazonensis GAPDH gene was used. Chemiluminescent detection was performed using an anti-DIG specific antibody covalently coupled to alkaline phosphate (Roche) and CPD-Star (Roche). In addition, 1 kb plus DNA ladder and VII DIG-labeled were used as molecular weight markers (MW). (C) Flow-FISH analyses were performed with the three main parasite life forms using a PNA FITC-labeled (CCCTAA)3 telomeric probe (PANAGENE). Histograms represent the average fluorescence intensity of non-hybridized parasites (at the left) and the telomeric probe (at the right). The data were analyzed using FlowJo software v.7.6.5. Vertical lines mark the difference between peaks, and horizontal lines with arrows, represent telomere length differences in the populations analyzed. The amount of fluorescence among samples was calculate using MESF (Supplementary Table 1).
Next, we used three different and complementary approaches to analyze telomere length during parasite development. The terminal restriction fragment (TRF) profiles of the three parasite life forms and from PP and MP maintained in continuous in vitro passages (1–8) were obtained using Southern blotting of gDNA hybridized with a telomeric probe using a standard protocol (Conte and Cano, 2005). The results shown in Figure 1B demonstrated that in lesion-derived Am, most of the telomere-containing fragments (Figure 1B, lane 1) range in size from ≤ 0.16 to 0.30 kb. Telomeres from PP with continuous passages (1–8) range in size from ≤ 0.16 to ≥ 0.38 kb (Figure 1B, lanes 2–6), whereas in MP from passages 1–8, telomeres range in size from ≤0.16 to 0.34 kb in length (Figure 1B, lanes 7–11; Supplementary Table 1). The results suggest that PP telomeres are slightly longer than Am and that parasite’s telomeres elongate after continuous in vitro passages. Telomere-associated sequences up to the first subtelomeric AfaI restriction site (Conte and Cano, 2005) appear as faint individual bands ≥ 1,0 kb (Figure 1B, lane 1–11). As the control, we hybridized the same DNA samples with a DIG-labeled fragment of L. amazonensis GAPDH (Figure 1B, bottom).
A qPCR assay was standardized to get a more precise result about the observed alterations in telomere length during parasite development (Aubert et al., 2012). It was possible to compare telomere amplification (T) with a single gene (S) amplification by qPCR to obtain the T/S ratio. Different L. amazonensis single gene candidates were tested: GAPDH, G6PDH, alpha-tubulin, and histone H2B (data not shown). The best parameters were obtained with GAPDH. The assay showed a confinable correlation of R2 = 0.99219, slope = −3.363 and amplification efficiency of 98% (Supplementary Figure 2). The qPCR results gave T/S ratios of, respectively, 1.64 and 0.54 for PP (passage 6) and Am (Supplementary Table 1). This result corroborates the TRF measurements shown in Supplementary Table 1, suggesting that PP telomeres are longer than Am.
Subsequently, we used a modified protocol of Flow-FISH assay (Baerlocher et al., 2006) to confirm the TRF measurements and qPCR results. Although all three methods are complementary, it is known that Flow-Fish is more precise and accurate than qPCR to measure telomere length in humans (Gutierrez-Rodrigues et al., 2014). Therefore, to ensure an accurate analysis of the data, human leukocytes were the internal control and processed together with parasite samples as described before (da Silva et al., 2017). Figure 1C shows FITC fluorescence histograms of the three L. amazonensis life forms hybridized with the PNA-FITC labeled telomeric probe. Each first peak in the graphs represents non-hybridized cells, whereas the second peaks represent the average fluorescence intensity emitted by the telomeric probe, which is proportional to telomere length. Therefore, the variation between peaks represents telomere length differences in the populations analyzed being, PP > MP > Am. The MESF values and the proportional change obtained for each sample are summarized in Supplementary Table 1.
In conclusion, it was possible to show that according to the average length of TRFs, the qPCR and Flow-FISH results (summarized in Supplementary Table 1), Am telomeres are shorter than PP and MP, strongly suggesting that telomere length is regulated during L. amazonensis development.
Subsequently, protein extracts of all three parasite life forms were tested for the presence of telomerase activity. Parasite extracts were obtained using an improved version of a previously standardized TRAP Assay protocol (Cano et al., 1999; Giardini et al., 2011). In this case, DEAE semi-purified protein extracts were tested for the presence of telomerase activity using the temperatures that parasite life forms live in their hosts (Wheeler et al., 2010). The results showed that PP telomerase was active at 28∘C but not at 37∘C (Figure 2A). In contrast, MP’s and Am’s telomerase was detected at 37∘C but not at 28∘C (Figures 2B,C). As controls, extracts were pre-treated with RNase A, which abolishes enzyme activity, and reactions were done in the absence of extract (NE, no extract) (Figures 2A–C). The bands in the lanes where the PP extracts were incubated at 37∘C (Figure 2A) and Am and MP extracts at 28∘C (Figures 2B,C) are not due to telomerase activity. The corresponding bands are probably artifacts or spurious PCR products (i.e., primer-dimers) (Kim and Wu, 1997; Krupp et al., 1997; Cano et al., 1999; Herbert et al., 2006; Giardini et al., 2011). TSR8 primer was used as an amplification marker since it produces a ladder of products with 6-base increments starting at 50 bp, the shortest band in the telomerase-positive extracts (Figures 2A–C). The results obtained show that telomerase activity can be detected in all L. amazonensis life forms. Also, for the first time, we present strong evidence that telomerase activity in L. amazonensis is probably life-stage dependent since in PP enzyme activity is detected at 28∘C, and in MP and Am, enzyme activity is detected at 37∘C.
Figure 2. A temperature-dependent telomerase activity maintains telomeres in L. amazonensis spp. (A–C). Telomerase activity was detected in semi-purified extracts using a modified one-tube Telomere Repeat Amplification Protocol (TRAP) assay (Cano et al., 1999; Giardini et al., 2011). Telomerase products were fractionated in a 10% non-denaturing PAGE in 1X TBE. Products were visualized using chemiluminescence. The amplification of a DIG-labeled TSR8 oligonucleotide was used as the 6-base increment ladder marker. (A) Telomerase activity detected in PP extracts. Lane 1, DIG-labeled TSR8. Lane 2, the reaction was done at 28∘C with the purified protein extract. Lane 3, the reaction was done at 28∘C with RNase A pre-treated extract. Lane 4, the reaction was done at 37∘C with the purified protein extract. Lane 5, the reaction was done at 37∘C with RNase A pre-treated extract. Lane 6, the reaction was done in the absence of extract (NE, no extract). (B) Telomerase activity detected in MP extracts. Lane 1, the reaction was done in the absence of extract (NE, no extract). Lane 2, DIG-labeled TSR8. Lane 3, the reaction was done at 37∘C with the purified protein extract. Lane 4, the reaction was done at 37∘C with RNase A pre-treated extract. Lane 5, the reaction was done at 28∘C with the purified protein extract. Lane 6, the reaction was done at 28∘C with RNase A pre-treated extract. (C) Telomerase activity detected in Am extracts. Lane 1, the reaction was done in the absence of extract (NE, no extract). Lane 2, DIG-labeled TSR8. Lane 3, the reaction was done at 37∘C with the purified protein extract. Lane 4, the reaction was done at 37∘C with RNase A pre-treated extract. Lane 5, the reaction was done at 28∘C with the purified protein extract. Lane 6, the reaction was done at 28∘C with RNase A pre-treated extract.
To analyze if, similar to Hsp90 from model organisms (Holt et al., 1999; Toogun et al., 2008), LHsp90 could also be a telomerase partner, parasites were treated with increased concentrations of 17AAG, an analog of geldanamycin. 17AAG inhibits Hsp90 in model eukaryotes (Neckers and Neckers, 2002; Guo et al., 2005) and shows lethal effects on L. amazonensis life forms depending on the concentration used (Wiesgigl and Clos, 2001; Li et al., 2009; de Petersen et al., 2012; Santos et al., 2014).
The IC50 of 17AAG for L. amazonensis PP (passage 6) was estimated as ∼100 nM (Supplementary Figure 3). After that, parasites in exponential growth cultures were treated with 100 and 200 nM 17AAG for 48 and 96 h, followed by cell counting (Figure 3A), analyses of cell viability (Figure 3B) and the cell cycle (Figure 3C and Supplementary Table 2). The results showed a decrease in the number of cells after treating parasites with 100 and 200 nM 17AAG for 48 < 96 h, meaning that LHsp90 inhibition was time-dependent. As shown after DNA content analysis by flow cytometer, part of the cells treated for 48 and 96 h were arrested in G2/M (Figure 3C and Supplementary Table 2). The quantitative differences in DNA content in each cell cycle phase (G1, S, and G2/M) between treated, meth-treated, and wt parasites were obtained from the histograms (events × FL2-area) are shown in Figure 3D and summarized in Supplementary Table 2. It is worth informing that PP grown in the presence of 90% methanol (meth-treated) and wild-type cells (wt) showed identical DNA content profiles explaining why the experiments in Figures 3C–G were done with meth-treated parasites as the control.
Figure 3. The inhibitor of HSP90, 17AAG, induces cell death, cell cycle arrest, telomere shortening, and telomerase inhibition in L. amazonensis PP. (A) Growth curves of PP non-treated (Wt and meth-treated parasites) and treated with 17AAG), maintained in exponential growth for 96 h and counted in the Neubauer chamber every 24 h. Wt and meth-treated parasites were used as the control. Treated parasites were cultivated in the presence of 100 and 200 nM 17AAG. (B) Cells’ viability test. L. amazonensis PP (∼3 × 106 cells) in exponential growth were treated for 48 h at 28°C with increased concentrations of 17AAG. As a control, cells were grown in the absence (0) and the presence of methanol (0 Meth). The assay was done using PrestoBlue (Invitrogen) according to the manufacturer’s instructions. The percentage (%) of viable cells was estimated and plotted using Graphpad Prism 8. The error bars indicate the S.D. of the mean of triplicate samples. (C) The histograms represent cells non-treated and treated with 17AAG in each cell cycle phase based on a flow cytometer’s DNA content analysis of propidium iodide stained cells. The data were analyzed using FlowJo software v.7.6.5. (D) The graphs represent the percentage of PP (non-treated and treated with 17AAG) distributed in each cell cycle phase. As in (C) treated cells were incubated with 100 and 200 nM 17AAG for 48 and 96 h. Control represents cells grown in the presence of 90% methanol (meth-treated). (E) AfaI digested gDNA (1.0 μg) of meth-treated parasites and parasites treated for 48 and 96 h with 100 and 200 nM 17AAG. DNA fragments were separated on 0.8% EtBr (ethidium bromide)-stained agarose gels followed by Southern blotting hybridization with a DIG-labeled TEL probe (5′-TTAGGG3-3′). As the loading control, a DIG-labeled probe that recognizes the L. amazonensis GAPDH gene was used. Chemiluminescent detection was performed using an anti-DIG specific antibody (Roche) and CPD-Star (Roche). 1 kb plus DNA ladder (Invitrogen) and VII DIG-labeled Molecular weight (Roche) were used as molecular weight markers (MW). (F) Flow-FISH analyses were performed in triplicates using three different biological replicas. Parasite samples treated for 48 and 96 h with 100 and 200 nM 17AAG and meth-treated controls were hybridized using a PNA FITC-labeled (CCCTAA)3 telomeric probe (PANAGENE). Histograms represent the average fluorescence intensity emitted by non-hybridized parasites (control, blue) and by the telomeric probe (100 nM, yellow and 200 nM, orange). The data were analyzed using FlowJo software v.7.6.5. The amount of fluorescence among samples was calculate using MESF (Supplementary Table 3). (G) Telomerase activity was detected in DEAE-purified PP extracts using a modification of the one-tube TRAP assay (Cano et al., 1999; Giardini et al., 2011). Telomerase products were fractionated in a 10% non-denaturing PAGE in 1X TBE. Products were visualized using chemiluminescence. A DIG-labeled TSR8 oligonucleotide was used as the 6-base increment ladder marker. Lane 1, the reaction was done in the absence of extract (NE, no extract). Lane 2, the reaction was done at 28∘C with the telomerase positive protein extract. Lane 3, the reaction was done with the telomerase-positive protein extract pre-treated with RNase A. Lane 4, reaction done with the telomerase-positive extract pre-treated with 100 nM 17AAG.
We also checked if the inhibition of LHsp90 caused alteration in parasite telomere length. Southern blots using gDNA obtained from meth-treated, and PP treated for 48 and 96 h with 100 and 200 nM 17AAG were hybridized with a telomeric probe (TEL), and as the control, the same DNA samples were hybridized with an L. amazonensis DIG-labeled GAPDH probe. The results showed that LHsp90 inhibition induces minor alteration in PP telomere length (Figure 3E). However, Flow-FISH (Figure 3F) analysis showed that LHsp90 inhibition induced telomere shortening in L. amazonensis PP in a time-dependent manner. Furthermore, MESF analysis (Supplementary Table 3) showed a more accentuated decrease in telomere length after 96 h of treatment independent of drug concentration. Besides, 100 nM 17AAG abolished PP’s telomerase activity in vitro (Figure 3G), strongly suggesting that LHsp90 regulates parasite telomere length.
Therefore, to check if LHsp90 is part of the L. amazonensis telomerase complex, we carried out co-immunoprecipitation assays (Figure 4) using telomerase-positive extracts obtained from the three main parasite life forms. The results showed that LHsp90 and TERT co-immunoprecipitated in the three extracts, evidencing that both proteins are part of the same protein complex. The presence of co-migrating bands in the extracts (input) of all three parasite forms revealed with anti-LdHsp90 serum is noted. Post-translational modifications are commonly observed in Hsp90 from most organisms, including Leishmania spp. (Mollapour and Neckers, 2012; Hombach-Barrigah et al., 2019). Whether post-translational modifications are determinant for LHsp90/TERT interactions is still an open question. Curiously, TERT is apparently expressed in lower levels in all parasite forms, being detected only in highly concentrated extracts (∼300 μg/gel lane) (Supplementary Figure 4). On the other hand, LHsp90 can be detected in ten times less concentrated protein extracts (input lanes in Figure 4). This finding corroborates other studies that evidenced LHsp90 as a highly abundant protein in Leishmania spp. promastigotes, representing 2.8% of its total protein content (Brandau et al., 1995). It is worth mentioning that the intense non-specific bands presented below the hsp90 band in the western blot of the IP using anti-Lbhsp90 IgG, and below TERT in the western blot of the IP using anti-LaTERT, are probably IgGs that were detected by the HRP-conjugated anti-rabbit IgG secondary antibody since the primary antibodies used (α-LbHSP90 and α-LaTERT) are polyclonal.
Figure 4. LHSP90 and the telomerase TERT component co-immunoprecipitated in the three L. amazonensis life forms. Co-immunoprecipitation (IP) assays were done using DynaBeads protein A, 20 μg of each telomerase positive extracts (input) obtained from Am, PP and MP, and specific polyclonal sera against LbHSP90 (left) or LaTERT (right). The total amount of IP eluates and 20 μg of each protein extracts (used as input) were fractionated in 10% SDS-PAGE and submitted to western blot analysis revealed with anti-LaTERT and anti-LbHSP90 sera. Goat anti-rabbit HRP-conjugate was used as the secondary antibody (Bio-Rad). The results were detected using enhanced chemiluminescence (ECL), according to the manufacturer’s instructions.
Altogether, the results presented here strongly suggest that LHsp90 can be part of the telomerase ribonucleoprotein complex and acts as a potential regulator of L. amazonensis telomere length.
The present study used the three main parasite life forms (PP, MP, and Am) to investigate telomere length maintenance in L. amazonensis. We figured out that L. amazonensis telomeres are distributed in nuclear clusters in the three life forms regardless of the cell cycle phase (Figure 1A). Curiously, as revealed by qPCR, telomeres from Am are shorter than PP, and using Flow-FISH, we confirmed that Am telomeres are shorter than MP and PP (Supplementary Figures 1C, 2 and Supplementary Table 1). It was also observed by TRF analysis that telomeres from PP and MP maintained in continuous in vitro passages increased over time (Figure 1B). These differences in telomere length are not due to lack/inhibition of telomerase since enzyme activity was detected in the three life forms. However, the catalysis seems to be life-stage dependent (Figures 2A–C). It is worth reminding that MP is pre-adapted to face 37°C during PP-to-Am differentiation and that Am is fully adapted at 37°C, but not PP, nectomonads, and other promastigote forms (Inbar et al., 2017). Also, Genest and Borst (2007) had previously shown that telomeres from Leishmania PP elongate over time, and Bussotti et al. (2018) recently demonstrated that Leishmania spp. amplifies the subtelomeric/telomeric regions during adaptation in the host, using probably an unknown replication/recombination mechanism. Thus, unknown factors should be involved in parasite telomere length regulation. Here we speculate that the telomere length differences among parasite life forms and parasites in continuous in vitro passages may also be due to variations in telomerase holoenzyme biogenesis and composition during parasite development and growth. However, further assays are necessary to determine if the enzyme’s catalysis is dependent on the parasite life stage or the composition of the RNP complex, or both.
In model eukaryotes, besides the telomeric proteins, telomerase activity can be regulated by protein partners involved in the assembly/disassembly of the telomerase ribonucleoprotein complex (Smogorzewska and de Lange, 2004; Collins, 2006; Podlevsky and Chen, 2012; Viviescas et al., 2019; Nguyen and Wong, 2020). Some of these partners are conserved, and among them, there are molecular chaperones such as Hsp90 (Toogun et al., 2008) and chaperone-like proteins (Viviescas et al., 2019). It was previously demonstrated that human Hsp90 (hHsp90) remains associated with a functional telomerase complex. Hsp90 is responsible for the nuclear transport of TERT by importin alpha and enhances telomerase activity by helping the phosphorylation of TERT by the AKT protein kinase. Hsp90/AKT, in its turn, regulates TERT subcellular distribution (Haendeler et al., 2003; Keppler et al., 2006; Xi et al., 2013), avoiding the degradation of TERT by the ubiquitin-proteasome system (Viviescas et al., 2019). Besides, the reconstitution of an active human telomerase ribonucleoprotein complex is dependent on Hsp90 and its co-chaperone p23 (Toogun et al., 2008). The dissociation of p23 from the complex, in contrast, retains the TERT component in the cytoplasm (Akalin et al., 2001; Keppler et al., 2006). Early studies in budding yeast demonstrated that the overexpression of Hsp82 (the ortholog of Hsp90 in yeast) induced telomere shortening without affecting telomerase activity (Grandin and Charbonneau, 2001). Later on, Hsp82 was shown to help the association of telomerase with telomeres facilitating telomere elongation through its interaction with CDC13, an essential component of the telomere capping complex (Dezwaan et al., 2009). Thus, in budding yeast, Hsp82, besides telomerase, may affect other factors involved in telomere length maintenance (DeZwaan and Freeman, 2010). Notably, the pharmacological inhibition of Hsp90 by geldanamycin or one of its analogs (e.g., 17AAG) triggers telomere shortening and abolishes telomerase activity in both humans and yeast (Holt et al., 1999; Toogun et al., 2008), confirming the influence of the chaperone in important telomere functions (Holt et al., 1999; Forsythe et al., 2001).
Based on this knowledge, we hypothesize that the ortholog of Hsp90 in L. amazonensis (LHsp90) could be a telomerase partner responsible for regulating telomerase activity. Our findings argue in favor of a conserved role for LHsp90 at parasite telomeres since its inhibition induces parasite growth arrest, telomere shortening, and abolishes PP telomerase activity in vitro (Figures 3A–F). We also showed that LHsp90 and the telomerase TERT component co-immunoprecipitated in telomerase positive extracts obtained from the three main parasite life forms (Figure 4), evidencing that LHsp90 can be part of the telomerase complex. Moreover, the western blot analysis showed that LHsp90, like other Hsp90, probably suffers post-translational modifications (co-migrating bands in input lanes in Figure 4 and Supplementary Figure 4), which can be important to regulate its activity, and also its interaction with other client proteins, such as the TERT. As highly documented, post-translational modifications regulate Hsp90 functions in the different biological processes (Backe et al., 2020). However, further studies are needed to evidence if LHsp90 is part of the parasite telomerase ribonucleoprotein complex and affects other parasite telomeric proteins.
Like most protozoa parasites, Leishmania uses LHsp90 during stage transitions in their developmental cycles (Zilka et al., 2001). Since Leishmania lives and multiplies in different environments and temperatures, these stressful living conditions are probably detrimental to the interactions between protein partners and the assembly of specific protein complexes. Thus, we speculate that the composition of the telomerase ribonucleoprotein complex alters during parasite development, and LHsp90 could be an important player in the control of telomere length maintenance and parasite life span. Therefore, a detailed understanding of telomerase biogenesis and LHsp90 intersections with the telomeric machinery will greatly contribute to our knowledge of Leishmania spp. telomeres and how they might be subject to manipulation for therapeutic purposes. Telomeres and the proteins involved with telomere length maintenance in humans have been used as important targets for developing therapies against cancer and other telomere-related diseases (Martínez and Blasco, 2017; Fernandes S. G. et al., 2020). The Leishmania spp. telomeric machine, although share some conserved features with most eukaryotes, presents genus-specific characteristics, such as unique telomeric proteins (Morea et al., 2017; Fernandes et al., 2019, Fernandes C. A. H. et al., 2020), a singular telomerase RNA component (Vasconcelos et al., 2014), and amino acid substitutions in the TERT functional domains (Giardini et al., 2006), increasing the chance of finding a good and parasite-specific therapeutic target among these molecules.
The original contributions presented in the study are included in the article/Supplementary Material, further inquiries can be directed to the corresponding author/s.
BO, MES, and SP: investigation, methodology, validation, and writing—original draft preparation. MV: data curation, validation, and writing—original draft preparation. EM, CS, MS, and FG-R: investigation, methodology, and validation. MdS: investigation, methodology, validation, writing—reviewing, and editing. JB: resources, visualization, writing—reviewing, and editing. RC: resources, conceptualization, supervision, writing—reviewing, and editing. MINC: conceptualization, funding acquisition, project administration, supervision, writing—reviewing, and editing. All authors contributed to the article and approved the submitted version.
This work was supported by the São Paulo Research Foundation (FAPESP, Fundação de Amparo a Pesquisa do Estado de São Paulo) under grant 2018/04375-2 to MINC. BO and MES are doctoral fellows from FAPESP (grants 2019/25985-6 and 2020/00316-1), and SP is a master fellow from CAPES (Coordenação de Aperfeiçoamento de Pessoal de Nível Superior-Brazil). MdS is a young researcher from FAPESP (grants 2019/10753-2 and 2020/10277-3). MINC is a PQ-CNPq fellow (The National Council for Scientific and Technological Development) (grant 302433/2019-8). The funders had no role in study design, data collection, analysis, decision to publish, or manuscript preparation.
The authors declare that the research was conducted in the absence of any commercial or financial relationships that could be construed as a potential conflict of interest.
The reviewer JA declared a shared affiliation with one of the authors, JB, to the handling editor at time of review.
All claims expressed in this article are solely those of the authors and do not necessarily represent those of their affiliated organizations, or those of the publisher, the editors and the reviewers. Any product that may be evaluated in this article, or claim that may be made by its manufacturer, is not guaranteed or endorsed by the publisher.
We acknowledge Mrs. Barbara A. A. Santana for helping with the MESF calculations.
The Supplementary Material for this article can be found online at: https://www.frontiersin.org/articles/10.3389/fcell.2021.713415/full#supplementary-material
Akalin, A., Elmore, L. W., Forsythe, H. L., Amaker, B. A., Mccollum, E. D., Nelson, P. S., et al. (2001). A novel mechanism for chaperone-mediated telomerase regulation during prostate Cancer progression. Cancer Res. 61, 4791–4796.
Alvar, J., Vélez, I. D., Bern, C., Herrero, M., Desjeux, P., and Cano, J. (2012). Leishmaniasis worldwide and global estimates of its incidence. PLoS One 7:e35671. doi: 10.1371/journal.pone.0035671
Aubert, G., Hills, M., and Lansdorp, P. M. (2012). Telomere length measurement-caveats and a critical assessment of the available technologies and tools. Mutat. Res. 730, 59–67. doi: 10.1016/j.mrfmmm.2011.04.003
Backe, S. J., Sager, R. A., Woodford, M. R., Makedon, A. M., and Mollapour, M. (2020). Post-translational modifications of Hsp90 and translating the chaperone code. J. Biol. Chem. 295, 11099–11117. doi: 10.1074/jbc.REV120.011833
Baerlocher, G. M., Vulto, I., de Jong, G., and Lansdorp, P. M. (2006). Flow cytometry and FISH to measure the average length of telomeres (flow FISH). Nat. Protoc. 1, 2365–2376. doi: 10.1038/nprot.2006.263
Barbiéri, C. L., Doine, A. I., and Freymuller, E. (1990). Lysosomal depletion in macrophages from spleen and foot lesions of Leishmania-infected hamster. Exp. Parasitol. 71, 218–228. doi: 10.1016/0014-4894(90)90024-7
Brandau, S., Dresel, A., and Clos, J. (1995). High constitutive levels of heat-shock proteins in human-pathogenic parasites of the genus Leishmania. Biochem. J. 310(Pt 1), 225–232. doi: 10.1042/bj3100225
Bussotti, G., Gouzelou, E., Côrtes Boité, M., Kherachi, I., Harrat, Z., Eddaikra, N., et al. (2018). Leishmania genome dynamics during environmental adaptation reveal strain-specific differences in gene copy number variation, karyotype instability, and telomeric amplification. mBio 9:e01399-18. doi: 10.1128/mbio.01399-18
Cano, M. I. N., Dungan, J. M., Agabian, N., and Blackburn, E. H. (1999). Telomerase in kinetoplastid parasitic protozoa. Proc. Natl. Acad. Sci. U. S. A. 96, 3616–3621. doi: 10.1073/pnas.96.7.3616
Chen, H., Li, Y., and Tollefsbol, T. O. (2009). Strategies targeting telomerase inhibition. Mol. Biotechnol. 41, 194–199. doi: 10.1007/s12033-008-9117-9
Collins, K. (2006). The biogenesis and regulation of telomerase holoenzymes. Nat. Rev. Mol. Cell Biol. 7, 484–494. doi: 10.1038/nrm1961
Conte, F. F., and Cano, M. I. N. (2005). Genomic organization of telomeric and subtelomeric sequences of Leishmania (Leishmania) amazonensis. Internat. J. Parasitol. 35, 1435–1443. doi: 10.1016/j.ijpara.2005.05.011
da Silva, M. S., Perez, A. M., Da Silveira, R. C. V., Moraes, C. E., Siqueira Neto, J. L., Freitas Júnior, L. H., et al. (2010). The Leishmania amazonensis TRF (TTAGGG repeat binding factor) homologue co-localizes and binds telomeres in vivo. BMC Microbiol. 10:136. doi: 10.1186/1471-2180-10-136
da Silva, M. S., Segatto, M., Pavani, R. S., Gutierrez-Rodrigues, F., Bispo, V. S., De Medeiros, M. H. G., et al. (2017). Consequences of acute oxidative stress in Leishmania amazonensis: from telomere shortening to the selection of the fittest parasites. BBA Mol. Cell Res. 186, 138–150. doi: 10.1016/j.bbamcr.2016.11.001
de Petersen, A. L. O. A., Guedes, C. E. S., Versoza, C. L., Lima, J. G. B., de Freitas, L. A. R., Borges, V. M., et al. (2012). 17-AAG kills intracellular Leishmania amazonensis while reducing inflammatory responses in infected macrophages. PLoS One 7:e49496. doi: 10.1371/journal.pone.0049496
Dey, A., and Chakrabarti, K. (2018). Current perspectives of telomerase structure and function in eukaryotes with emerging views on telomerase in human parasites. Internat. J. Mol Sci. 19:333. doi: 10.3390/ijms19020333
DeZwaan, D. C., and Freeman, B. C. (2010). HSP90 manages the ends. Trends Biochem. Sci. 35, 384–391. doi: 10.1016/j.tibs.2010.02.005
Dezwaan, D. C., Toogun, O. A., Echtenkamp, F. J., and Freeman, B. C. (2009). The Hsp82 molecular chaperone promotes a switch between unextendable and extendable telomere states. Nat. Struct. Mol. Biol. 16, 711–716. doi: 10.1038/nsmb.1616
Dillon, L. A. L., Okrah, K., Hughitt, V. K., Suresh, R., Li, Y., Fernandes, M. C., et al. (2015). Transcriptomic profiling of gene expression and RNA processing during Leishmania major differentiation. Nucleic Acids Res. 43, 6799–6813. doi: 10.1093/nar/gkv656
Feng, J., Funk, W. D., Wang, S. S., Weinrich, S. L., Avilion, A. A., et al. (1995). The RNA component of human telomerase. Science 269, 1236–1241. doi: 10.1126/science.7544491
Fernandes, C. A. H., Morea, E. G. O., Dos Santos, G. A., Vieira, M. R., Da Silva, V. L., Viviescas, M. A., et al. (2020). A multi-approach analysis highlights the relevance of RPA-1 as a Telomere end-binding protein (TEBP) in Leishmania amazonensis. BBA Gen. Subj. 1864:129607. doi: 10.1016/j.bbagen.2020.129607
Fernandes, C. A. H., Perez, A. M., Barros, A. C., Dreyer, T. R., Da Silva, M. S., Morea, E. G. O., et al. (2019). Dual cellular localization of the Leishmania amazonensis Rbp38 (LaRbp38) explains its affinity for telomeric and mitochondrial DNA. Biochimie 162, 15–25. doi: 10.1016/j.biochi.2019.03.017
Fernandes, S. G., Dsouza, R., Pandya, G., Kirtonia, A., Tergaonkar, V., Lee, S. Y., et al. (2020). Role of telomeres and telomeric proteins in human malignancies and their therapeutic potential. Cancers (Basel) 12:1901. doi: 10.3390/cancers12071901
Forsythe, H. L., Jarvis, J. L., Turner, J. W., Elmore, L. W., and Holt, S. E. (2001). Stable association of hsp90 and p23, but Not hsp70, with active human telomerase. J. Biol. Chem. 276, 15571–15574. doi: 10.1074/jbc.c100055200
Fu, G., and Barker, D. C. (1998). Characterization of Leishmania telomeres reveals unusual telomeric repeats and conserved telomere-associated sequence. Nucleic Acids Res. 26, 2161–2167. doi: 10.1093/nar/26.9.2161
Genest, P. A., and Borst, P. (2007). Analysis of telomere length variation in Leishmania over time. Mol. Biochem. Parasitol. 151, 213–215. doi: 10.1016/j.molbiopara.2006.10.015
Giardini, M. A., Fernández, M. F., Lira, C. B. B., and Cano, M. I. N. (2011). Leishmania amazonensis: partial purification and study of the biochemical properties of the telomerase reverse transcriptase activity from promastigote-stage. Exp. Parasitol. 127, 243–248. doi: 10.1016/j.exppara.2010.08.001
Giardini, M. A., Lira, C. B. B., Conte, F. F., Camillo, L. R., Siqueira Neto, J. L., Ramos, C. H. I., et al. (2006). The putative telomerase reverse transcriptase component of Leishmania amazonensis: gene cloning and characterization. Parasitol. Res. 98, 447–454. doi: 10.1007/s00436-005-0036-34
Giardini, M. A., Segatto, M., da Silva, M. S., Nunes, V. S., and Cano, M. I. N. (2014). Telomere and telomerase biology. in: telomeres in health and disease, prog. Mol. Biol. Transl. Sci. 125, 1–40. doi: 10.1016/b978-0-12-397898-1.00001-3
Grandin, N., and Charbonneau, M. (2001). HSP90 levels affect telomere length in yeast. Mol. Genet. Genom. 265, 126–134. doi: 10.1007/s004380000398
Grenert, J. P., Sullivan, W. P., Fadden, P., Haystead, T. A., Clark, J., et al. (1997). The amino-terminal domain of heat shock protein 90 (HSP90) that binds geldanamycin is an ATP/ADP switch domain that regulates Hsp90 conformation. J. Biol. Chem. 272, 23843–23850. doi: 10.1074/jbc.272.38.23843
Guo, W., Reigan, P., Siegel, D., Zirrolli, J., Gustafson, D., and Ross, D. (2005). Formation of 17-allylamino-demethoxygeldanamycin (17-AAG) hydroquinone by NAD(P)H:quinone oxidoreductase 1: role of 17-AAG hydroquinone in heat shock protein 90 inhibition. Cancer Res. 65, 10006–10015. doi: 10.1158/0008-5472.CAN-05-2029
Gutierrez-Rodrigues, F., Santana-Lemos, B. A., Scheucher, P. S., Alves-Paiva, R. M., and Calado, R. T. (2014). Direct comparison of Flow-FISH and qPCR as diagnostic tests for telomere length measurement in humans. PLoS One 9:e113747. doi: 10.1371/journal.pone.0113747
Haendeler, J., Ho_mann, J., Rahman, S., Zeiher, A. M., and Dimmeler, S. (2003). Regulation of telomerase activity and anti-apoptotic function by protein-protein interaction and phosphorylation. FEBS Lett. 536, 180–186. doi: 10.1016/s0014-5793(03)00058-9
Herbert, B. S., Hochreiter, A., Wright, W., et al. (2006). Nonradioactive detection of telomerase activity using the telomeric repeat amplification protocol. Nat Protoc. 1, 1583–1590. doi: 10.1038/nprot.2006.239
Holt, S. E., Aisner, D. L., Baur, J., Tesmer, V. M., Dy, M., Ouellette, M., et al. (1999). Functional requirement of p23 and Hsp90 in telomerase complexes. Genes Dev. 13, 13–817.
Hombach-Barrigah, A., Bartsch, K., Smirlis, D., et al. (2019). Leishmania donovani 90 kD heat shock protein – impact of phosphosites on parasite fitness. infectivity and casein kinase affinity. Sci. Rep. 9:5074. doi: 10.1038/s41598-019-41640-0
Hotez, P. J., Pecoul, B., Rijal, S., Boehme, C., Aksoy, S., Malecela, M., et al. (2016). Eliminating the neglected tropical diseases: translational science and new technologies. PLoS Negl. Trop. Dis. 10:e0003895. doi: 10.1371/journal.pntd.0003895
Inbar, E., Hughitt, V. K., Dillon, L. A. L., Ghosh, K., El-Sayed, N. M., and Sacks, D. L. (2017). The transcriptome of Leishmania major developmental stages in their natural sand fly vector. mBio 8:e00029-17. doi: 10.1128/mbio.00029-17
Keppler, B. R., Grady, A. T., and Jarstfer, M. B. (2006). The biochemical role of the heat shock protein 90 chaperone complex in establishing human telomerase activity. J. Biol. Chem. 281, 19840–19848. doi: 10.1074/jbc.m511067200
Kevric, I., Cappel, M. A., and Keeling, J. H. (2015). New world and old world Leishmania infections. Dermatol. Clin. 33, 579–593. doi: 10.1016/j.det.2015.03.018
Kim, N. W., and Wu, F. (1997). Advances in quantification and characterization of telomerase activity by the telomeric repeat amplification protocol (TRAP). Nucleic Acids Res. 25, 2595–2597. doi: 10.1093/nar/25.13.2595
Krupp, G., Kühne, K., Tamm, S., et al. (1997). Molecular basis of artifacts in the detection of telomerase activity and a modified primer for a more robust ‘TRAP’ assay. Nucleic Acids Res. 25, 919–921. doi: 10.1093/nar/25.4.919
Li, Q., Zhou, Y., Yao, C., Ma, X., Wang, L., et al. (2009). Apoptosis caused by Hsp90 inhibitor geldanamycin in Leishmania donovani during promastigote-to-amastigote transformation stage. Parasitol. Res. 105, 1539–1548. doi: 10.1007/s00436-009-1582-y
Martínez, P., and Blasco, M. A. (2017). Telomere-driven diseases and telomere-targeting therapies. J. Cell Biol. 216, 875–887. doi: 10.1083/jcb.201610111
Moafi, M., Rezvan, H., Sherkat, R., and Taleban, R. (2019). Leishmania vaccines entered in clinical trials: a review of literature. Int. J. Prev. Med. 10:95. doi: 10.4103/ijpvm.IJPVM_116_18
Mollapour, M., and Neckers, L. (2012). Post-translational modifications of Hsp90 and their contributions to chaperone regulation. Biochim. Biophys. Acta 1823, 648–655. doi: 10.1016/j.bbamcr.2011.07.018
Morea, E. G. O., Viviescas, M. A., Fernandes, C. A. H., Matioli, F. F., Lira, C. B. B., Fernandez, M. F., et al. (2017). A calmodulin-like protein (LCalA) is a new Leishmania amazonensis telomere end-binding protein. BBA Gen. Subj. 1861, 2583–2597. doi: 10.1016/j.bbagen.2017.08.011
Neckers, L., and Neckers, K. (2002). Heat-shock protein 90 inhibitors as novel cancer chemotherapeutic agents. Expert Opin. Emerg. Drugs 7, 277–288. doi: 10.1517/14728214.7.2.277
Nguyen, K. T. T. T., and Wong, J. M. Y. (2020). Telomerase biogenesis and activities from the perspective of its direct interacting partners. Cancers 12:1679. doi: 10.3390/cancers12061679
Olaussen, K. A., Dunant, A., Fouret, P., et al. (2006). DNA repair by ERCC1 in non-small-cell lung cancer and cisplatin-based adjuvant chemotherapy. N. Engl. J. Med. 355, 983–991. doi: 10.1056/NEJMoa060570
Palma, L. C., Ferreira, L. F. G. R., Petersen, A. L. D. O., et al. (2019). A docking-based structural analysis of geldanamycin-derived inhibitor binding to human or Leishmania Hsp90. Sci. Rep. 9:14756. doi: 10.1038/s41598-019-51239-0
Podlevsky, J. D., and Chen, J. J. (2012). It all comes together at the ends: telomerase structure, function, and biogenesis. Mut. Res. 730, 3–11. doi: 10.1016/j.mrfmmm.2011.11.002
Sacks, D., and Kamhawi, S. (2001). Molecular aspects of parasite-vector and vector-host interactions in leishmaniasis. Ann. Rev. Microbiol. 55, 453–483. doi: 10.1146/annurev.micro.55.1.453
Sacks, D., and Perkins, P. (1984). Identification of an infective stage of Leishmania promastigotes. Science 223, 1417–1419. doi: 10.1126/science.6701528
Santos, D. M., Petersen, A. L. O. A., Celes, F. S., Borges, V. M., Veras, P. S. T., et al. (2014). Chemotherapeutic potential of 17-AAG against cutaneous leishmaniasis caused by Leishmania (Viannia) braziliensis. PLoS Negl. Trop. Dis. 8:e3275. doi: 10.1371/journal.pntd.0003275
Sekaran, V., Soares, J., and Jarstfer, M. B. (2014). Telomere maintenance as a target for drug discovery. J. Med. Chem. 57, 521–538. doi: 10.1021/jm400528t
Seraphim, T. V., Alves, M. M., Silva, I. M., Gomes, F. E. R., Silva, K. P., Murta, S. M. F., et al. (2013). Low resolution structural studies indicate that the activator of Hsp90 ATPase 1 (Aha1) of Leishmania braziliensis has an elongated shape which allows its interaction with both N- and M-Domains of Hsp90. PLoS One 8:e66822. doi: 10.1371/journal.pone.0066822
Siqueira Neto, J. L., Lira, C. B. B., Giardini, M. A., Khater, L., Perez, A. M., Peroni, L. A., et al. (2007). Leishmania replication protein A-1 binds in vivo single-stranded telomeric DNA. Biochem. Biophys. Res. Comm. 358, 417–423. doi: 10.1016/j.bbrc.2007.04.144
Smogorzewska, A., and de Lange, T. (2004). Regulation of telomerase by telomeric proteins. Ann. Rev. Biochem. 73, 177–208. doi: 10.1146/annurev.biochem.73.071403.1600
Toogun, O. A., DeZwaan, D. C., and Freeman, B. C. (2008). The Hsp90 molecular chaperone modulates multiple telomerase activities. Mol. Cell. Biol. 28, 457–467. doi: 10.1128/mcb.01417-07
Vasconcelos, E. J. R., Nunes, V. S., da Silva, M. S., Segatto, M., Myler, P. J., and Cano, M. I. N. (2014). The putative Leishmania telomerase RNA (LeishTER) undergoes trans-splicing and contains a conserved template sequence. PLoS One 9:e112061. doi: 10.1371/journal.pone.0112061
Viviescas, M. A., Segato, M., and Cano, M. I. N. (2019). Chaperones and their role in telomerase ribonucleoprotein biogenesis and telomere maintenance. Curr. Proteom. 16, 31–43. doi: 10.2174/1570164615666180713103133
Wheeler, R. J., Gluenz, E., and Gull, K. (2010). The cell cycle of Leishmania: morphogenetic events and their implications for parasite biology. Mol. Microbiol. 79, 647–662. doi: 10.1111/j.1365-2958.2010.07479.x
Wiesgigl, M., and Clos, J. (2001). Heat shock protein 90 homeostasis controls stage differentiation in Leishmania donovani. Mol. Biol. Cell 12, 3307–3316. doi: 10.1091/mbc.12.11.3307
World Health Organization [WHO] (2020). Leishmaniasis, WHO, World Health Organization. Geneva: World Health Organization.
Xi, P., Zhou, L., Wang, M., Liu, J.-P., and Cong, Y.-S. (2013). Serine/Threonine-Protein Phosphatase 2A physically interacts with human telomerase reverse transcriptase HTERT and regulates its subcellular distribution. J. Cell. Biochem. 114, 409–417. doi: 10.1002/jcb.24378
Zilka, A., Garlapati, S., Dahan, E., Yaolsky, V., and Shapira, M. (2001). Developmental regulation of heat shock protein 83 in Leishmania 3′ processing and mRNA stability control transcript abundance, and translation is directed by a determinant in the 3′-untranslated region. J. Biol. Chem. 276, 47922–47929.
Keywords: Leishmania life forms, continuous in vitro passages, telomeres maintenance, telomerase ribonucleoprotein complex, LHsp90
Citation: de Oliveira BCD, Shiburah ME, Paiva SC, Vieira MR, Morea EGO, da Silva MS, Alves CS, Segatto M, Gutierrez-Rodrigues F, Borges JC, Calado RT and Cano MIN (2021) Possible Involvement of Hsp90 in the Regulation of Telomere Length and Telomerase Activity During the Leishmania amazonensis Developmental Cycle and Population Proliferation. Front. Cell Dev. Biol. 9:713415. doi: 10.3389/fcell.2021.713415
Received: 22 May 2021; Accepted: 29 September 2021;
Published: 28 October 2021.
Edited by:
Andrew Burgess, Anzac Research Institute, AustraliaReviewed by:
Juliana Ide Aoki, University of São Paulo, BrazilCopyright © 2021 de Oliveira, Shiburah, Paiva, Vieira, Morea, da Silva, Alves, Segatto, Gutierrez-Rodrigues, Borges, Calado and Cano. This is an open-access article distributed under the terms of the Creative Commons Attribution License (CC BY). The use, distribution or reproduction in other forums is permitted, provided the original author(s) and the copyright owner(s) are credited and that the original publication in this journal is cited, in accordance with accepted academic practice. No use, distribution or reproduction is permitted which does not comply with these terms.
*Correspondence: Maria Isabel N. Cano, bWFyaWEuaW4uY2Fub0B1bmVzcC5icg==
†These authors have contributed equally to this work and share first authorship
Disclaimer: All claims expressed in this article are solely those of the authors and do not necessarily represent those of their affiliated organizations, or those of the publisher, the editors and the reviewers. Any product that may be evaluated in this article or claim that may be made by its manufacturer is not guaranteed or endorsed by the publisher.
Research integrity at Frontiers
Learn more about the work of our research integrity team to safeguard the quality of each article we publish.