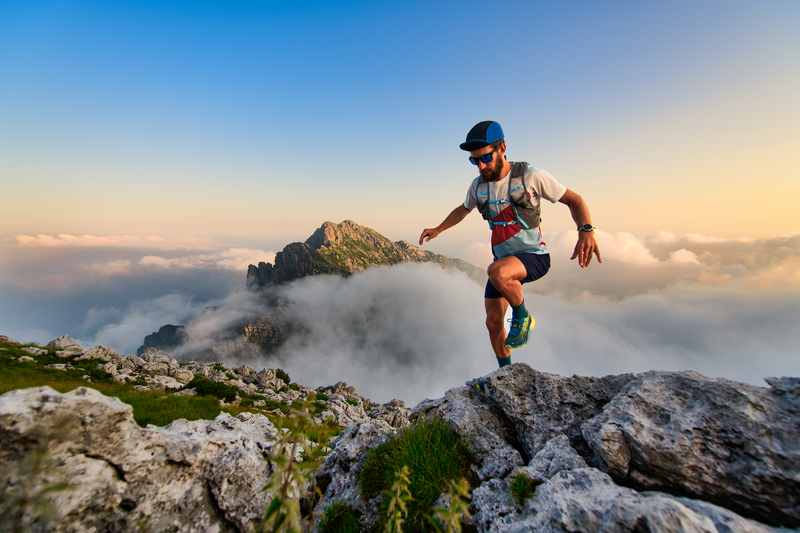
95% of researchers rate our articles as excellent or good
Learn more about the work of our research integrity team to safeguard the quality of each article we publish.
Find out more
REVIEW article
Front. Cell Dev. Biol. , 22 July 2021
Sec. Stem Cell Research
Volume 9 - 2021 | https://doi.org/10.3389/fcell.2021.711005
This article is part of the Research Topic Stem cells in Tissue Homeostasis and Disease View all 18 articles
Given the self-renewal, multi-differentiation, immunoregulatory, and tissue maintenance properties, mesenchymal stem cells (MSCs) are promising candidates for stem cell-based therapies. Breakthroughs have been made in uncovering MSCs as key contributors to homeostasis and the regenerative repair of tissues and organs derived from three germ layers. MSC differentiation into specialized cell types is sophisticatedly regulated, and accumulating evidence suggests long non-coding RNAs (lncRNAs) as the master regulators of various biological processes including the maintenance of homeostasis and multi-differentiation functions through epigenetic, transcriptional, and post-translational mechanisms. LncRNAs are ubiquitous and generally referred to as non-coding transcripts longer than 200 bp. Most lncRNAs are evolutionary conserved and species-specific; however, the weak conservation of their sequences across species does not affect their diverse biological functions. Although numerous lncRNAs have been annotated and studied, they are nevertheless only the tip of the iceberg; the rest remain to be discovered. In this review, we characterize MSC functions in homeostasis and highlight recent advances on the functions and mechanisms of lncRNAs in regulating MSC homeostasis and differentiation. We also discuss the current challenges and perspectives for understanding the roles of lncRNAs in MSC functions in homeostasis, which could help develop promising targets for MSC-based therapies.
Mesenchymal stem cells (MSCs) are heterogeneous, multipotent adult stem cells that originate in the mesoderm and that have been isolated from diverse tissues such as adipose tissue, bone marrow, and umbilical cord. Due to their self-renewal, multilineage differentiation potential, extensive immunomodulatory effects, and tissue maintenance properties, MSCs have emerged as attractive tools for cell-based therapies and have been involved as treatment options for hematological diseases, autoimmune diseases, and peripheral nerve injuries (Chen et al., 2019; Yousefi et al., 2019; Zoehler et al., 2020). Currently, there have been breakthroughs in uncovering MSCs as key contributors to homeostasis and the regenerative repair of tissues and organs derived from three germ layers (mesoderm, ectoderm, and endoderm) (Sui et al., 2020). Notably, the MSCs present in different embryonic development stages, including postembryonic and postnatal tissues, constitute a population of sub-totipotent stem cells or progenitors, which were recently defined as MSC systems, have been confirmed to have molecular heterogeneity at single-cell transcriptomic level. MSCs maintain tissue homeostasis in three main ways. First, the MSCs residing in the major tissues, including adipose, bone, cartilage, muscles, divide and differentiate into targeted cell types to support the expansion, regeneration, and homeostasis of these tissues (Hilgendorf et al., 2019). Second, MSCs residing in tissue perivascular niches interact closely with their surroundings, which harbor varied cell types and soluble factors that further influence MSC behavior (Crisan et al., 2008; Sui et al., 2020). Third, MSCs themselves also secrete abundant types of biofactors and extracellular vesicles (EVs) to potentially affect their surroundings, including supporting hematopoiesis and modulating immune responses (Wang et al., 2014; Kfoury and Scadden, 2015; Sui et al., 2020). These functional capabilities contribute to MSC modulation in tissue homeostasis. However, the regulation of MSC function in these processes is immensely complex and tightly controlled and warrants extensive studies.
Long non-coding RNAs (lncRNAs) are transcripts with an average length of >200 nucleotides, lack protein-coding potential, and were previously considered transcriptional noise (Djebali et al., 2012). Most lncRNAs are evolutionarily conserved and species-specific, albeit less conserved across species, and they have diverse biological functions (Jin et al., 2011). According to genome-wide association studies (GWAS), non-coding intervals cover over one-third of the phenotype-associated locations. Nevertheless, lncRNAs largely remain to be identified, and their association and their functions require intensive studies (Jin et al., 2011). With the development of high-throughput sequencing, microarrays, and bioinformatics, an increasing number of lncRNAs has been identified, and increasing evidence has confirmed their roles as master regulators of various biological processes, including the maintenance of MSC homeostasis and multi-differentiation functions through diverse mechanisms at the epigenetic, transcriptional, and translational level.
In this review, we provide an overview of the MSC characteristics and their contributions to tissue homeostasis, and highlight the role of lncRNAs in modulating MSC homeostasis and differentiation. We also discuss the challenges and perspectives underlying lncRNA usage in preclinical research and clinical application. We aim to elucidate the underlying mechanisms involved in this process, which could help provide promising targets for MSC-based therapies.
Mesenchymal stem cells were first identified from bone marrow by Friedenstein et al. (1976) in the 1950s; thereafter, scientists revealed that they are present in almost all connective tissues, and can also reside in fetal or adult somatic tissues, including the amniotic membrane (Parolini et al., 2008), umbilical cord (Romanov et al., 2003), adipose tissue (Zuk et al., 2002), skin (Orciani and Di Primio, 2013), peripheral blood (He et al., 2007), dental pulp (Huang et al., 2009), fetal liver (Zhang et al., 2005), and synovial membrane (De Bari et al., 2001). The source tissue from which MSCs are derived determines their differentiation potential (Xu et al., 2017). Bone marrow-derived MSCs (BMSCs) and adipose-derived MSCs (ADSCs) share similar morphological features and cell surface markers; however, many studies have indicated that significant biological differences exist, including differentiation potential. For example, BMSCs exhibit higher osteogenic but lower adipogenic differentiation capacity compared to ADSCs (Xu et al., 2017). ADSCs produce more neurosphere-derived neuron-like cells compared to BMSCs; therefore, ADSCs are a more suitable source for cell transplantation for treating spinal cord injury (Chung et al., 2013). Therefore, clarifying the intrinsic biological characteristic of MSCs derived from different sources and choosing the appropriate MSCs are important for their clinical application. To create a standard criterion for univocally defining the identity of MSCs used for scientific research and preclinical studies, the International Society for Cellular Therapy established the minimum criteria required for defining MSCs (Dominici et al., 2006; Wang et al., 2019): (1) MSCs must be fibroblast-like plastic-adherent cells when maintained in standard culture conditions; (2) ≥ 95% of the MSC population must express CD105, CD73, and CD90, and lack (≤2% positive) CD45, CD34, CD14 or CD11b, CD79a or CD19, and HLA-class II expression; (3) MSCs must have the capacity to differentiate into adipocytes, osteoblasts, and chondroblasts in vitro. Later studies have indicated that besides the capacity to differentiate into mesenchymal lineages, MSCs also have the potential to trans-differentiate into the unrelated germline ectodermal (neurocytes) and endodermal lineages (hepatocytes).
Recently, a new concept of MSC system was proposed by Wang et al. (2019), which was regarded as all MSCs derived from different stages of embryonic development, from postembryonic sub-totipotent stem cells to progenitors (Zhao, 2013). The MSC system well defined the important self-renewal and differentiation, immunomodulatory, and tissue homeostasis properties of MSCs, which provides a more comprehensive view of MSCs and better explains the heterogeneity of MSCs in differentiation potential and immunomodulatory functions. MSCs that reside in tissues such as bone marrow, adipose, cartilage, and muscle primarily form unique niches with a quiescent state. When exposed to stimulus such as injury, inflammation, and medicine, MSCs enter an active state to divide and differentiate into specialized cell types to support the expansion and homeostasis of these tissues (Méndez-Ferrer et al., 2010; Hilgendorf et al., 2019; Hu et al., 2020). Besides, MSCs interact closely with their surroundings by secreting variable biofactors and EVs to support hematopoiesis and modulate immune responses; the surrounding niches in which MSCs reside also influence their behavior (Crisan et al., 2008; Zhao et al., 2014). For example, during wound healing, skin residential ADSCs divide and migrate to injured sites and differentiate into skin cells such as dermal fibroblasts (DFs) to replace and regenerate damaged cells. On the other hand, ADSCs activate wound healing via the autocrine and paracrine pathways. Together with other skin cells such as DFs, ADSCs secrete factors to form the extracellular matrix and interact with each other to promote wound healing, maintain skin structure, and modulate skin homeostasis (Mazini et al., 2020). Another example is BMSCs, which express nestin, in the perivascular stroma, can self-renew and differentiate into osteochondral lineages that form a unique niche in the bone marrow to maintain hematopoietic stem cell (HSC) homeostasis, such as modulating HSC proliferation, differentiation, and recruitment (Méndez-Ferrer et al., 2010). In the endosteal niche, these BMSCs, together with osteoblasts, maintain HSCs in a quiescent state. When subjected to injury, MSCs expressing LepR and Gli1 divide and contribute to bone repair and regeneration (Zhou et al., 2014; Shi et al., 2017). In lethally irradiated mice, the injection of MSCs deficient in nestin expression notably reduced HSC homing to the bone marrow (Méndez-Ferrer et al., 2010). BMSC dysfunction, including aberrant proliferation and differentiation, is the crucial pathogenesis of bone degeneration and hematopoiesis suppression. Moreover, MSCs are indispensable in maintaining the homeostasis of other tissues, including intestinal (Stzepourginski et al., 2017) and skeletal muscle (Wosczyna et al., 2019).
So far, there have been breakthroughs in understanding the biological characteristics and potential therapeutic values of MSCs. In general, MSCs have multi-directional differentiation potential, can secrete bioactive molecules to migrate and home to injured or inflamed sites, and have powerful immunomodulating ability, thereby making them important contributors in tissue repair and homeostasis maintenance (Figure 1) (Vizoso et al., 2019; Wang et al., 2019; Bulati et al., 2020).
Figure 1. Implications of MSCs in homeostasis. MSCs can be isolated from a variety of tissues, including the amniotic membrane, umbilical cord, placenta, adipose tissue, skin, peripheral blood, dental pulp, and fetal liver. All MSCs derived from different stages of embryonic development, from postembryonic sub-totipotent stem cells to progenitors, are defined as MSC systems. During in vitro culture, MSCs must: (1) be fibroblast-like and plastic-adherent; (2) express CD105, CD73, and CD90, and lack CD45, CD34, CD14 or CD11b, CD79a or CD19, and HLA class II expression; (3) differentiate into adipocytes, osteoblasts, and chondroblasts. MSCs have trilineage differentiation potential, can secrete bioactive molecules and EVs (microvesicles and exosomes) to help tissue repair and maintain homeostasis. MSC dysfunction leads to disease-related MSC alterations that induce homeostasis disorder systemic disease.
Mesenchymal stem cells maintain tissue homeostasis based on their differentiation potential by serving as a source of renewable progenitor cells to repair injured tissues and replace cells in routine cellular turnover throughout adult life (Spees et al., 2016; Chen et al., 2017). MSCs are adult stem cells that present in many tissues and can differentiate into multiple mesenchymal lineage cell types such as adipocytes, osteoblasts, chondrocytes, and myoblasts under specific culture conditions (Boeuf and Richter, 2010; Scott et al., 2011; Westhrin et al., 2015; Chen et al., 2018). Besides, when exposed to certain extracellular cues, MSCs can also give rise to cross-lineage cell types like endodermal-hepatocyte and ectodermal-neurons, which is also known as trans-differentiate potential (Song and Tuan, 2004).
During bone tissue fracture, MSCs are recruited to the injury site and differentiate into osteoblasts to aid the repair and reconstitution of injured bone tissue (Freitas et al., 2019; Moura et al., 2020). MSCs can differentiate into cardiac cells under specific conditions in vitro; genetically manipulated MSCs with Akt1 and Wnt11 overexpression exhibit enhanced cardiac differentiation as verified by the elevated cardiac markers Nkx2.5, GATA4, α-MHC, and BNP, indicating that the transplantation of genetically engineered MSCs is a promising strategy for treating acute myocardial infarction (Chen et al., 2018). Moreover, MSCs also have the potential to trans-differentiate into endoderm and ectoderm cells to help repair specific tissues and organs. MSCs induced by chemically defined media containing specific cytokines and growth factors in vitro can trans-differentiate into hepatocyte-like cells with the functional properties of albumin synthesis and secretion, cytochrome P450 enzyme activity, glycogen storage, urea biosynthesis, and the expression of hepatocyte-specific genes (He et al., 2013; Fu et al., 2016; Maymó et al., 2018; Furuya et al., 2019), and can reconstitute liver function in vivo in experimental hepatic injury murine models (Xu et al., 2014; Fu et al., 2016). MSCs also have the capacity to produce pancreas-like cells under stepwise induction by cytokine cocktails (Yu et al., 2015; Mehrfarjam et al., 2016), via pancreatic extract or coculture with pancreatic adult stem cells (Lee et al., 2008; Hefei et al., 2015). MSC-derived insulin-producing cells express pancreatic β cell-related genes, respond to glucose challenge in vitro, and have the potential to improve glucose tolerance in diabetic 90% pancreatectomy rats in vivo (Yu et al., 2015). Further, MSCs can tans-differentiate into endothelial cells with the endothelial phenotype and express endothelial nitric oxide synthase, which contributed to improving endothelial function in a vascular injury rat model (Jiang et al., 2006; Yue et al., 2008).
Although the multi-differentiation capacity of MSCs ensures their tissue repair and regeneration function, the increasing application of MSCs clinically has reported that only a small amount of MSCs undergo subsequent differentiation into the targeted cell type after transplantation while still receiving functional improvement (Ferrand et al., 2011; Lai et al., 2015; Vizoso et al., 2019). Other mechanisms may confer MSCs efficacy in damaged tissues and the maintenance of tissue homeostasis.
Increasing evidence supports the idea that intravenously injected MSCs can home specifically to sites of ischemia, damage, or inflammation, while not requiring induction into a specific functional cell type in advance (Price et al., 2006; Ye and Zhang, 2017; Ben Menachem-Zidon et al., 2019). Yet, other studies have shown poor survival and transient retainment of transplanted MSCs within the host tissue (Yeo et al., 2013; Miao et al., 2017), indicating that MSCs may not exert their therapeutic effects directly; rather, it occurs through the secretion of bioactive factors to provide a conducive microenvironment to facilitate the repair and regeneration of injured tissues.
Mesenchymal stem cells with the potential for synthesizing and secreting a variety of bioactive factors (e.g., cytokines and chemokines), and to affect nearby cells were first described by Haynesworth et al. (1996). In 2009, Bruno et al. (2009) reported that a new form of MSC secretion, termed microvesicles (80 nm to 1 μm), was protective against acute tubular injury. The next year, Lai et al. (2010) demonstrated a specific class of extracellular vesicles (EVs) with a diameter of 40–100 nm, defined as exosomes. The multiple bioactive factors, together with the EVs (e.g., exosomes and microvesicles), are generally referred to as MSC secretome. Subsequent studies reported that the MSC secretome has important effects in promoting angiogenesis, modulating immunity, and hematopoietic support (Lai et al., 2015; Konala et al., 2016). The composition of the soluble factors of MSCs derived from different tissues may vary, but they often secrete cytokines (e.g., CCL2, CCL5, bFGF, IL-6, TGF-β, and VEGF), contributing to tissue development, cell differentiation, and tumor growth and metastasis (Wang et al., 2019). Some factors (e.g., IL-6, IL-10, PGE2, HGF, nitric oxide, and human HLA-G) account for the immunomodulatory functions of MSCs (Wang et al., 2019). MSCs can also secrete neurotrophic factors, such as brain- and glial-derived neurotrophic factors (e.g., nerve growth factor), making them attractive cellular sources for brain disorders (Lopatina et al., 2019). Moreover, MSC-derived EVs also exhibit tissue repair and immunomodulation functions. Our group demonstrated that MSC-derived exosomes can promote the angiogenesis of human brain microvascular endothelial cells and contribute to alleviating Parkinson disease (PD) in a mouse model (Xue et al., 2021). Further, MSC exosomes inhibited inflammatory responses and reactive astrogliosis in vitro and in vivo, and repaired learning and memory impairments induced by status epilepticus in a mouse model (Xian et al., 2019). In an allogeneic hematopoietic stem cell transplantation animal model, EVs derived from human umbilical cord-derived MSCs prevented acute graft-versus-host disease (GVHD) (Wang et al., 2016).
Accumulating evidence supports the role of lncRNAs as master regulators of various biological processes, including the maintenance of MSC homeostasis and multi-differentiation functions through diverse mechanisms. The recent development of genome technology opened the door to understanding their functional importance. Conventionally, lncRNAs are transcribed by RNA polymerase II, containing multi-exons, processed by alternative splicing, 3′ polyadenylated and 5′ capped, and present transcriptional activation activity like that of mRNAs (Djebali et al., 2012; Ma et al., 2013; Lagarde et al., 2017). Although lncRNAs are distributed widely across species, they are poorly conserved and exhibit low expression levels, making them species-specific features and easily regarded as transcriptional noise (Ma et al., 2013). Moreover, lncRNAs exhibit a spatiotemporal and cell-, tissue-, and development-specific expression pattern (Shi et al., 2020), and their subcellular location in the nucleus or the cytoplasm determines their functions and working mechanisms (Chen, 2016). Nuclear lncRNAs are usually involved in transcriptional regulation, including interaction with chromatin regulation and RNA processing. Cytoplasmic lncRNAs tend to affect translation, such as modulating mRNA stability and cellular signaling cascades (Schmitt and Chang, 2016).
Based on the genome location of protein-coding genes, lncRNAs can be classified into five groups: intergenic, intronic, sense, antisense, and bidirectional (Ma et al., 2013; Jarroux et al., 2017; Fernandes et al., 2019), which are described in Figure 2A. This classification is widely used by the GENCODE/Ensemble database in the annotation of transcript biotypes, as well as newly assembled lncRNA transcripts identified by laboratories. Initially, lncRNA transcripts can be classified as either intergenic or intragenic; the intragenic lncRNAs overlap with coding genes and are further classified into antisense, bidirectional, intronic, and overlapping sense lncRNAs. Additionally, lncRNAs commonly perform their gene expression regulatory functions by acting as signals, decoys, guides, and scaffolds through main mechanisms by interacting with DNA, protein, and RNA (Wang and Chang, 2011; Schmitt and Chang, 2016), as illustrated in Figures 2B,C. However, the mechanisms underlying lncRNA regulation of gene expression and biological processes are complex and not simply confined to one archetype as we have summarized, and await more extensive discoveries.
Figure 2. Classification and mechanism of lncRNAs. (A) Classification of lncRNAs according to the protein-coding genes as intergenic, intronic, sense, antisense, and bidirectional. (B) LncRNAs acting as guides, scaffolds, decoys, and signals to perform their functions with DNAs and proteins. (C) LncRNAs interact with RNAs to regulate RNA stability, and translation, and sponge miRNAs.
Accumulating studies have implicated lncRNAs as vital regulators of variable bioprocesses, including genomic imprinting, chromosome modification, transcriptional interference, cell cycle, proliferation, immunobiology, and differentiation (Bartolomei et al., 1991; Quinn and Chang, 2016; Yang et al., 2018). In terms of the important biological functions of lncRNAs, dysregulation such as overexpression, deficiency, or mutation is suspected in the occurrence and progression of many diseases, including autoimmune disease, cardiovascular disease, and cancer (Batista and Chang, 2013; Beermann et al., 2016; Atianand et al., 2017). Moreover, emerging evidence has confirmed the contribution of lncRNAs in MSC differentiation, homeostasis, and related diseases (Tye et al., 2015); clarifying the roles and innate mechanisms of MSC-related lncRNAs in homeostasis will help provide promising targets for MSC-based therapies.
Mesenchymal stem cell differentiation is intricately regulated by multiple factors, including transcriptional factors (Runx2, PPARγ, MyoD, and GATA6), growth factors (VEGF, HGF, and EGF), and epigenetic factors such as DNA methylation, histone modification, RNA modification, and non-coding RNAs (miRNAs and lncRNAs) (Almalki and Agrawal, 2016; Sui et al., 2020). Recent studies have shown that lncRNAs are relatively new differentiation regulators that exert their functions through variable mechanisms, and await extensive studies. Herein, we mainly focus on the MSC-associated lncRNAs in differentiation and homeostasis.
Long non-coding RNAs involved in MSC-derived lineage (adipocytes, osteoblasts and chondrocytes) differentiation have been extensively studied while remaining relatively less well studied in other directions such as endoderm and ectoderm lineage commitment and differentiation. Herein, we provide an overview of the essential lncRNAs involved in MSC lineage commitment (Figure 3 and Table 1) and elaborate on the representative lncRNAs below.
Figure 3. Representative MSC differentiation- and exosome-associated lncRNAs. Endoderm: ANCR (DANCR), (differentiation) antagonizing non-protein coding RNA; MALAT1, metastasis-associated lung adenocarcinoma transcript 1. Ectoderm: H19. Mesoderm-cardiac: Braveheart. Mesoderm-adipogenic: PGC1β-OT1, peroxisome proliferator-activated receptor γ coactivator-1β-OT1; lnc13728; H19; MEG3, maternally expressed 3. Mesoderm-osteogenic: H19; MEG3; MALAT1; DANCR; PGC1β-OT1. Mesoderm-chondrogenic: DANCR. Mesoderm-endothelial: MEG3; MIAT, myocardial infarction-associated transcript; HULC, highly upregulated in liver cancer. Exosomal lncRNAs: HIF3A-AS1, HIF3A antisense 1; MALAT1; UCA1, urothelial carcinoma-associated 1; NEAT1, nuclear paraspeckle assembly transcript 1; H19; KLF3-AS1, KLF3 antisense 1.
Mesenchymal stem cells tend to differentiate toward osteogenic, adipogenic, and chondrogenic lineages. Osteogenic and adipogenic MSC differentiation is a theoretically opposite process, during which the signaling pathways or transcription factors induced in adipogenesis occur at the cost of osteogenesis, and vice versa (Yuan et al., 2016). For example, peroxisome proliferator-activated receptor γ (PPARγ), a master regulator of MSC adipogenesis, and inhibits osteogenic differentiation. Bone morphogenetic protein (BMP) and Wnt, crucial inducers of MSC osteogenic differentiation, may hinder MSC adipogenic commitment by inactivating PPARγ. Many lncRNAs such as H19 and MEG3 act in the same manner. H19 is a paternally imprinted gene (Zhang and Tycko, 1992) that has been recently uncovered as an inhibitor during BMSC adipogenic differentiation through the epigenetic modulation of histone deacetylases (HDACs) (Huang et al., 2016). H19 also has the potential to promote MSC osteogenic differentiation by acting as a competing endogenous RNA (ceRNA) through sponging and inhibiting the expression of miR-22 and miR-141 (Liang et al., 2016). Similarly, H19 promotes tension-induced osteogenesis of BMSCs by sponging miR-138 and activates the downstream FAK pathway (Wu et al., 2018). Therefore, H19 is a key regulator in the multi-direction commitment of MSCs.
MEG3 is also an essential multi-functional regulator during MSC differentiation. During osteogenic differentiation, MSCs from patients with multiple myeloma (MM) had lower MEG3 expression compared to that from normal donors (Zhuang et al., 2015). MEG3 performs its function at multiple levels. At the transcriptional level, it may act as a decoy to dissociate SOX2 binding at the BMP4 promoter, repressing BMP4 expression, thereby transcriptionally activating BMP4 promotion of MSC osteogenic differentiation (Zhuang et al., 2015). MEG3 can also act as histone methylation mediators by binding to the enhancer of zeste homolog 2 (EZH2), which can inhibit the expression of Wnt pathway genes by inducing H3K27 trimethylation to inhibit the osteogenic differentiation of human dental follicle stem cells (hDFSCs) (Deng et al., 2018). At the post-transcriptional level, MEG3 may act as a ceRNA to regulate osteogenic gene expression, and its expression level is increased in postmenopausal osteoporosis (PMOP) patients as compared to that in healthy donors (Wang et al., 2017). During the osteogenic differentiation of BMSCs from PMOP, MEG3 may target miR-133a-3p to inhibit this process (Wang et al., 2017). In addition, MEG3 may control the balance between MSC adipogenic and osteogenic differentiation; its downregulation promotes adipogenic differentiation while inhibiting the osteogenic differentiation of human ADSCs via miR-140-5p (Li et al., 2017). Moreover, MEG3 is an inhibitor of the development of many bone disorders, such as bone tumors, osteoarthritis (OA), osteoporosis, RA, and ankylosing spondylitis (AS). These findings indicate that MEG3 may act as a novel target for diagnosing or treating such bone diseases (Sun et al., 2020).
DANCR was characterized as a differentiation-antagonizing lncRNA of progenitor cells (Kretz et al., 2012). It functions as a positive regulator of chondrogenesis of human synovium-derived MSCs (through the miR-1305–Smad4 axis) (Zhang et al., 2017) while acting as an inhibitor of periodontal ligament stem cell osteogenesis (Wang et al., 2020). Another study revealed that DANCR inhibited the osteogenic differentiation of human BMSCs through the p38–MAPK pathway (Zhang et al., 2018). The lncRNA MALAT1 is another well-known abundant and conserved imprinted gene that acts as a master regulator of osteogenic differentiation via the mechanism of sponging miRNAs such as miR-143 (Gao et al., 2018) and miR-34c (Yang et al., 2019). Another newly identified lncRNA, PGC1β-OT1, reciprocally modulates MSC adipogenic and osteogenic commitment by sponging miR-148a-3p and enhancing the effect of KDM6B (Yuan et al., 2019); the lncRNA ROA inhibits MSC adipogenic differentiation by destroying hnRNPA1 binding to the PTX3 promoter, thereby transcriptionally downregulating PTX3 and the ERK pathway (Pan et al., 2020). Moreover, our lab discovered that lncRNA13728 promoted ADSC adipogenic differentiation by upregulating ZBED3 and inhibiting the WNT–β-catenin pathway (Xu et al., 2021).
The disruption of the balance between MSC osteogenesis and adipogenesis leads to disorders such as osteoporosis (Hoshiba et al., 2012). Notably, lncRNAs such as MEG3 and PGC1β-OT1 reciprocally modulate MSC commitment to adipogenic and osteogenic cells; therefore, understanding the roles and underlying mechanisms of these lncRNAs may provide insights into improving the therapeutic method and effect of MSCs in diseases such as osteosarcoma, obesity, and OA.
In addition, MSCs can differentiate into mesoderm endotheliocytes and myocytes. The dysfunction of endothelial cell and myocyte generation leads to defects in angiogenesis and related cardiovascular disease. MEG3 inhibits BMSC endothelial differentiation by accelerating FOXM1 protein degradation via ubiquitination and decreasing VEGF expression (Sun et al., 2018). Moreover, the lncRNA MIAT, identified as a key contributor to development and disease, acts as a ceRNA of miR-200a and thereby targets VEGF to promote MSC endothelial differentiation (Wang et al., 2018). For MSC myogenesis, a recent study revealed that the lncRNA HULC promotes ADSC epithelial and smooth muscle-like cell differentiation by targeting BMP9, activating the Wnt–β-catenin pathway while inhibiting the Notch pathway (Li Y. et al., 2018). Another report showed the lncRNA Braveheart efficiently facilitates MSC cardiogenic differentiation by upregulating cardiac-specific transcription factors and epithelial-mesenchymal transition (EMT)-associated genes (Hou et al., 2017). Although MSCs have the potential to differentiate into all kinds of myocytes, functional lncRNAs in other types of myocyte commitment remain to be discovered.
Mesenchymal stem cells have tri-lineage differentiation potential; despite the mesodermal-lineage cells, MSCs can also trans-differentiate into ectodermal and endodermal lineages. Unlike the well-studied mesoderm lineage-associated lncRNAs described above, studies on the detailed functions of lncRNAs in MSC ectoderm and endoderm commitment are relatively rare (which are summarized in Table 1), and further exploration is warranted.
Generating definitive endoderm (DE) and its lineage hepatocytes is a prerequisite for cell replacement therapy for liver and pancreatic diseases as well as for drug testing and toxicology studies (Li et al., 2019). According to our findings, the lncRNA ANCR (DANCR) is an inhibitor during ADSC trans-differentiation toward DE, and the mechanism linked involves it acting as a scaffold to recruit PTBP1 to ID2 mRNA, enhancing the interaction between them and subsequently stabilizing the ID2 mRNA (Li et al., 2019). This finding reveals another function of ANCR in modulating MSC DE commitment besides regulating chondrogenesis and osteogenesis. MALAT1 also performs a function in MSC trans-differentiation into hepatocyte in addition to adipogenesis and osteogenesis. Tan et al. (2019) successfully induced BMSCs into hepatocytes using HGF in vitro and discovered that MALAT1 coordinated with β-catenin, sponging miR-217, and upregulating ZEB1 to enhance telomerase activity during MSC hepatic trans-differentiation.
Ectoderm lineage neural cells are the foundation of our nervous system; they are relatively difficult to generate in vitro. Generating abundant neural cells will help promote cell-based therapy for treating neurological disorders and nerve injuries. Many studies have demonstrated that MSCs have the potential to trans-differentiate into neural-like cells under specific stimulation, making them a novel therapy for treating nervous system diseases. A study that profiled lncRNAs during BMSC neural cell differentiation found that several lncRNAs were differentially expressed, suggesting their key roles in this process (Wu et al., 2015). A subsequent study confirmed that H19 has a negative effect on BMSC neural-like differentiation through the miR-675–IGFR axis (Farzi-Molan et al., 2018). In the future, the identification of new lncRNAs in MSC neurogenesis and studies of the extensive mechanisms involved, as well as in vivo experiments, are needed, which will contribute to improving MSC-based therapeutic effects in treating neurological disease.
These lncRNAs, i.e., DANCR, MALAT1 MEG3, and H19, represent a subset of lncRNAs that exert various functions through multiple mechanisms in specific cell types under specific stimulations, which subsequently attach MSC unique capabilities to meet the qualifications in vivo and for clinical usage in vitro.
Increasing evidence suggests that the efficacy of MSC therapies is largely attributed to their paracrine secretion function, especially the exosomes (Dong et al., 2019). MSC-derived exosomes can shuttle a variety of bioactive molecules such as proteins, lipids, miRNA, lncRNAs, circular RNAs (circRNAs), and DNA to influence various bioprocesses, including development, immunity, and tissue homeostasis (Dong et al., 2019; Pegtel and Gould, 2019). Due to the advantages of low tumorigenic potential and low immunogenicity, exosomes are becoming novel, promising cell-free tools for tissue repair and diseases (Pegtel and Gould, 2019). Recently, functional lncRNAs derived from MSC exosomes have drawn increased attention, and some of these lncRNAs have been discovered. For example, the MSC exosomal lncRNA HIF3A-AS1 exhibits increased capacity in chondrocyte proliferation and cartilage repair in OA, which may be achieved through the miR-206–GIT1 axis (Liu et al., 2018a,b). Another study found that the exosomal lncRNA KLF3-AS1 alleviates cardiomyocyte pyroptosis and myocardial infarction through the miR-138-5p–Sirt1 axis (Mao et al., 2019). MALAT1 also resides in MSC exosomes; functional studies have shown that exosomal MALAT1 ameliorates osteoporotic by modulating the miR-34c–SATB2 axis (Yang et al., 2019) and can sponge miR-92a-3p and target ATG4a to fulfill its cardioprotective roles in doxorubicin-induced cardiac senescence and damage (Xia et al., 2020). Other exosomal lncRNAs such as UCA1 (Chen H. et al., 2020) and NEAT1 (Chen H. et al., 2020) also have a cardioprotective function by acting as ceRNAs.
The transfer of exosomes or microvesicles containing RNAs or other molecules between MSCs and the target cell type is one of the mechanisms by which MSCs perform their tissue repair functions (Spees et al., 2016). For example, H19 derived from MSC exosomes was transferred from MSCs to fibroblasts, thereby inhibiting fibroblast apoptosis and inflammation and activating the wound healing process in diabetic foot ulcers (Li et al., 2020). H19 could also be transferred to trophoblast cells via MSC-derived exosomes, enhancing trophoblast cell invasion and migration while inhibiting their apoptosis in preeclampsia (Chen Y. et al., 2020). Conversely, MSCs could also be the target cells during exosomal lncRNA transfer. MSCs derived from patients with MM had abundant exosomal lncRNA RUNX2-AS1; further studies revealed that it could be transferred from MM cells to MSCs and thereby prevent MSC osteogenesis by downregulating RUNX2 (Li B. et al., 2018), which provides a novel pathological mechanism of the bone lesion in patients with MM and could be a potential therapeutic target in the future.
These findings suggest that MSC-derived exosomes overexpressing lncRNAs such as H19 might be a novel direction for developing cell-free therapeutic strategies. Moreover, these exosomal lncRNAs are promising novel targets or biomarkers for treating and diagnosing diseases such as cardiomyopathy. In addition, understanding the tumor–stroma stem cell interactions, molecular transfer, and communication is also critical for developing novel and more effective strategies against cancer and other diseases.
Mesenchymal stem cells are key contributors in maintaining tissue homeostasis (Figure 1). The regulatory mechanisms underlying MSC functions are complicated, and are intricately regulated by multiple factors, i.e., transcriptional factors, growth factors, and epigenetic factors such as DNA methylation, histone modification, RNA modification, and non-coding RNAs (lncRNAs, miRNAs, and circRNAs). Recently, lncRNAs have emerged as prominent modulators of MSC fate commitment and functional homeostasis (Table 1) through variable mechanisms (Figure 2). Understanding the roles of lncRNAs in MSC functions in homeostasis will aid the development of promising targets for MSC-based therapies. However, issues and challenges remain to be investigated, including the conditions of MSCs used in basic research and clinical application, as well as the complex characteristics and mechanisms underlying lncRNA function.
As MSCs play an important role in tissue repair, regeneration, and homeostasis, their dysfunction may cause various systemic diseases. Clinical observation of allogenic MSC treatment of patients with autoimmune diseases, including systemic lupus erythematosus (SLE), diabetes mellitus (DM), rheumatoid arthritis (RA), and multiple sclerosis (MS) (Vizoso et al., 2019) indicates that the transplantation of external MSCs in good condition restores internal homeostasis. Further, MSC dysfunction indicates the onset of many diseases, including metabolic syndrome, DM, and RA, and aging syndromes such as Werner syndrome and Hutchinson–Gilford progeria syndrome (Liu et al., 2011; Zhang et al., 2015). Conversely, the continued inflammatory environment in these diseases may hinder MSC homing to the damage sites and probably result in MSC pool reduction and exhaustion (Shi et al., 2010), which contributes to the deterioration in MSC function and limits their use in autologous therapy.
To date, significant progress has been made in utilizing MSCs in basic preclinical research and clinical studies. However, some challenges should be overcome before the final clinical application (Wang et al., 2019). First, there is an urgent need for standard and consensus production (e.g., sources, medium, and culture conditions) to ensure the safety, reproducibility, and efficiency of MSCs administered to patients, which is also required in basic research. Second, MSCs derived from different tissues may have varying characteristics and functions; therefore, it is important to uncover the genetic background of different MSC sources and understand the specific innate characteristics of MSCs, which would aid the selection of the best seeds for fulfilling the specific clinical usage. Third, there is an urgent need to discover new genes or regulators such as the lncRNAs, as well as outstanding technologies to be developed to genetically modify MSCs and enhance their functions to boost their clinical application. Besides, the signals and mechanisms that modulate MSCs in tissue expansion, repair, and regeneration remain to be clarified, including the program that determines the balance between self-renewal and differentiation, the growth factors or signals that destroy the balance and trigger MSC expansion or differentiation, and how MSCs communicate with their surrounding niches to support a functional environment.
Mesenchymal stem cells maintain tissue homeostasis based on their differentiation potential to produce renewable progenitor cells to repair tissues and to replace cells in routine cellular turnover. MSCs tend to differentiate into mesenchymal lineage cells, while their trans-differentiation into endodermal and ectodermal lineage cells is limited. There are persistent challenges to fully understanding the underlying mechanisms in MSC differentiation, including identifying new signal and master transcription factors, and crosstalk between the signaling pathways involved in mediating and promoting MSC lineage differentiation and trans-differentiation rate. Manipulating MSCs with the overexpression of transcriptional factors increases their potential to differentiate into an intended cell type (Chen et al., 2018). However, a long journey remains before these genetically manipulated MSCs enter clinical application for treating diseases, unless safer methods are developed for manipulating MSCs with forced gene expression and to avoid activating the innate tendency of MSCs to differentiate into other unintended cell types.
Numerous lncRNAs participate in MSC lineage commitment, and lncRNAs derived from MSC exosomes exhibit enhanced tissue-protective and repair function. However, some challenges remain. On one hand, lncRNAs have multiple and varied functions and mechanisms of action, and lncRNAs largely remain unknown. e.g., H19 contributes to adipogenesis and osteogenesis, and resides in MSC exosomes to accelerate wound healing through different mechanisms. Moreover, lncRNAs may have an opposite effect on the same biological process, such as MEG3, which promotes and inhibits MSC osteogenic differentiation. First, the source of MSCs may confer the bidirectional effect on the lncRNA. lncRNAs usually display tissue- and spatiotemporal-specific expression patterns, and their aberrant expression is highly associated with disease and cancer occurrence. Therefore, lncRNAs may be differentially expressed at different stages of development, which confers their variable roles. Second, the MSC culture conditions in vitro may influence their stemness and functions, and the passage of MSCs used also matters. Therefore, as discussed above, there is an urgent need to establish a gold-standard approach for MSC basic research and clinical application. Taken together, extensive functional studies on one particular lncRNA can be performed in the future, and accompanying advanced molecular biotechnologies are being developed to better clarify and identify lncRNA targets and pathways and to screen for unknown lncRNA-interacting proteins. In addition, lncRNAs comprise a large proportion of the genome, and myriad functional lncRNAs remain to be discovered and studied. Moreover, most mechanisms of the existing studies on lncRNAs are focused on the downstream targets and pathways; the upstream stimulators and regulators that modulate lncRNA expression should be discovered. On the other hand, lncRNAs are poorly conserved among different species (Mirza et al., 2014), rendering it difficult or complicated to generate conditional knockout animal models to study the full function of lncRNAs, and complicating the development of lncRNAs as drug targets (Matsui and Corey, 2017). Despite these challenges, MSC-associated lncRNAs are promising targets and biomarkers for treating and diagnosing diseases. Nevertheless, opportunities coexist with challenges. There are emerging studies on lncRNA-based or -targeted drugs are emerging (Matsui and Corey, 2017), making them attractive therapeutic interventions in the future.
Mesenchymal stem cell exosome-derived lncRNAs such as H19 shuttle between MSCs and fibroblasts to perform their function in facilitating wound healing in diabetic foot ulcers (Li et al., 2020), which indicates that MSC-derived exosomes with lncRNA overexpression might be a novel direction for developing cell-free therapeutic strategies and will improve MSC efficacy. With continued research in the future, genetically modified MSCs with improved tissue repair and regeneration functions will be achieved soon.
Over the last decade, non-coding RNAs (e.g., miRNAs and lncRNAs) have emerged as significant new therapeutic targets; many efforts have been dedicated to developing new oligonucleotide-based therapies aimed at promoting or antagonizing them. To date, over 100 antisense oligonucleotide (ASO)-based therapies have been developed and tested in clinical trials. The US Food and Drug Administration (FDA) has approved fomivirsen for treating cytomegalovirus retinitis and mipomersen for treating familial hypercholesterolemia (Adams et al., 2017). Unlike miRNAs, which are small and advantageous for delivering their mimics or inhibitors through synthetically modified oligoribonucleotides, lncRNAs are relatively large and usually are of a structured nature that makes it difficult to design effective mimics or inhibitors (Scacalossi et al., 2019). Although no clinical advances have been made with lncRNAs, they remain striking targets for clinical therapeutic intervention in the future. In addition, lncRNAs are relatively large and therefore more stable, rendering them suitable diagnostic and prognostic biomarkers for cancer. In recent years, it has been confirmed that circulating lncRNAs are valuable for detecting cancer types, as they are quite easily detected by common methods such as qRT-PCR, RNA sequencing (RNA-seq), and microarray in whole blood, plasma, serum, urine, saliva, and gastric juice samples; some circulating lncRNAs have been proven as sensitive biomarkers. More lncRNAs are being identified as diagnostic and prognostic biomarkers for varied diseases, especially for those caused by aberrant MSC dysfunction.
FZ and RZ conceived the project. YY, SL, CH, ZC, TL, LZ, and LW collected the data. YY wrote and revised the manuscript. FZ, HC, and RZ provided guidelines and edited the manuscript. All authors read and approved the final manuscript.
This study was supported by the National Key R&D Program of China (2016YFA0101003 and 2016YFC0903901), National Natural Science Fund (81771764), CAMS Innovation Fund for Medical Sciences (2017-I2M-3-007), and project funded by China Postdoctoral Science Foundation (2021M690461).
The authors declare that the research was conducted in the absence of any commercial or financial relationships that could be construed as a potential conflict of interest.
Adams, B. D., Parsons, C., Walker, L., Zhang, W. C., and Slack, F. J. (2017). Targeting noncoding RNAs in disease. J. Clin. Invest. 127, 761–771. doi: 10.1172/jci84424
Almalki, S. G., and Agrawal, D. K. (2016). Key transcription factors in the differentiation of mesenchymal stem cells. Differentiation 92, 41–51. doi: 10.1016/j.diff.2016.02.005
Atianand, M. K., Caffrey, D. R., and Fitzgerald, K. A. (2017). Immunobiology of Long Noncoding RNAs. Annu. Rev. Immunol. 35, 177–198. doi: 10.1146/annurev-immunol-041015-055459
Bartolomei, M. S., Zemel, S., and Tilghman, S. M. (1991). Parental imprinting of the mouse H19 gene. Nature 351, 153–155. doi: 10.1038/351153a0
Batista, P. J., and Chang, H. Y. (2013). Long noncoding RNAs: cellular address codes in development and disease. Cell 152, 1298–1307. doi: 10.1016/j.cell.2013.02.012
Beermann, J., Piccoli, M. T., Viereck, J., and Thum, T. (2016). Non-coding RNAs in development and disease: background, mechanisms, and therapeutic approaches. Physiol. Rev. 96, 1297–1325. doi: 10.1152/physrev.00041.2015
Ben Menachem-Zidon, O., Gropp, M., Ben Shushan, E., Reubinoff, B., and Shveiky, D. (2019). Systemically transplanted mesenchymal stem cells induce vascular-like structure formation in a rat model of vaginal injury. PLoS One 14:e0218081. doi: 10.1371/journal.pone.0218081
Boeuf, S., and Richter, W. (2010). Chondrogenesis of mesenchymal stem cells: role of tissue source and inducing factors. Stem Cell Res. Ther. 1:31. doi: 10.1186/scrt31
Bruno, S., Grange, C., Deregibus, M. C., Calogero, R. A., Saviozzi, S., Collino, F., et al. (2009). Mesenchymal stem cell-derived microvesicles protect against acute tubular injury. J. Am. Soc. Nephrol. 20, 1053–1067. doi: 10.1681/asn.2008070798
Bulati, M., Miceli, V., Gallo, A., Amico, G., Carcione, C., Pampalone, M., et al. (2020). The Immunomodulatory Properties of the Human Amnion-Derived Mesenchymal Stromal/Stem Cells Are Induced by INF-γ Produced by Activated Lymphomonocytes and Are Mediated by Cell-To-Cell Contact and Soluble Factors. Front. Immunol. 11:54. doi: 10.3389/fimmu.2020.00054
Chen, B., Chen, X., Liu, C., Li, J., Liu, F., and Huang, Y. (2018). Co-expression of Akt1 and Wnt11 promotes the proliferation and cardiac differentiation of mesenchymal stem cells and attenuates hypoxia/reoxygenation-induced cardiomyocyte apoptosis. Biomed. Pharmacother. 108, 508–514. doi: 10.1016/j.biopha.2018.09.047
Chen, H., Xia, W., and Hou, M. (2020). LncRNA-NEAT1 from the competing endogenous RNA network promotes cardioprotective efficacy of mesenchymal stem cell-derived exosomes induced by macrophage migration inhibitory factor via the miR-142-3p/FOXO1 signaling pathway. Stem Cell Res. Ther. 11:31. doi: 10.1186/s13287-020-1556-7
Chen, Y., Ding, H., Wei, M., Zha, W., Guan, S., Liu, N., et al. (2020). MSC-Secreted Exosomal H19 Promotes Trophoblast Cell Invasion and Migration by Downregulating let-7b and Upregulating FOXO1. Mol. Ther. Nucleic Acids 19, 1237–1249. doi: 10.1016/j.omtn.2019.11.031
Chen, H. S., Hsu, C. Y., Chang, Y. C., Chuang, H. Y., Long, C. Y., Hsieh, T. H., et al. (2017). Benzyl butyl phthalate decreases myogenic differentiation of endometrial mesenchymal stem/stromal cells through miR-137-mediated regulation of PITX2. Sci. Rep. 7:186. doi: 10.1038/s41598-017-00286-6
Chen, L. L. (2016). Linking Long Noncoding RNA Localization and Function. Trends Biochem. Sci. 41, 761–772. doi: 10.1016/j.tibs.2016.07.003
Chen, Y., Yu, Q., Hu, Y., and Shi, Y. (2019). Current Research and Use of Mesenchymal Stem Cells in the Therapy of Autoimmune Diseases. Curr. Stem Cell Res. Ther. 14, 579–582. doi: 10.2174/1574888x14666190429141421
Chung, C. S., Fujita, N., Kawahara, N., Yui, S., Nam, E., and Nishimura, R. (2013). A comparison of neurosphere differentiation potential of canine bone marrow-derived mesenchymal stem cells and adipose-derived mesenchymal stem cells. J. Vet. Med. Sci. 75, 879–886. doi: 10.1292/jvms.12-0470
Crisan, M., Yap, S., Casteilla, L., Chen, C. W., Corselli, M., Park, T. S., et al. (2008). A perivascular origin for mesenchymal stem cells in multiple human organs. Cell Stem Cell 3, 301–313. doi: 10.1016/j.stem.2008.07.003
De Bari, C., Dell’Accio, F., Tylzanowski, P., and Luyten, F. P. (2001). Multipotent mesenchymal stem cells from adult human synovial membrane. Arthritis Rheum. 44, 1928–1942. doi: 10.1002/1529-0131(200108)44:8<1928::Aid-art331<3.0.Co;2-p
Deng, L., Hong, H., Zhang, X., Chen, D., Chen, Z., Ling, J., et al. (2018). Down-regulated lncRNA MEG3 promotes osteogenic differentiation of human dental follicle stem cells by epigenetically regulating Wnt pathway. Biochem. Biophys. Res. Commun. 503, 2061–2067. doi: 10.1016/j.bbrc.2018.07.160
Djebali, S., Davis, C. A., Merkel, A., Dobin, A., Lassmann, T., Mortazavi, A., et al. (2012). Landscape of transcription in human cells. Nature 489, 101–108. doi: 10.1038/nature11233
Dominici, M., Le Blanc, K., Mueller, I., Slaper-Cortenbach, I., Marini, F., Krause, D., et al. (2006). Minimal criteria for defining multipotent mesenchymal stromal cells. The International Society for Cellular Therapy position statement. Cytotherapy 8, 315–317. doi: 10.1080/14653240600855905
Dong, R., Liu, Y., Yang, Y., Wang, H., Xu, Y., and Zhang, Z. (2019). MSC-Derived Exosomes-Based therapy for peripheral nerve injury: a novel therapeutic strategy. Biomed. Res. Int. 2019:6458237. doi: 10.1155/2019/6458237
Farzi-Molan, A., Babashah, S., Bakhshinejad, B., Atashi, A., and Fakhr Taha, M. (2018). Down-regulation of the non-coding RNA H19 and its derived miR-675 is concomitant with up-regulation of insulin-like growth factor receptor type 1 during neural-like differentiation of human bone marrow mesenchymal stem cells. Cell Biol. Int. 42, 940–948. doi: 10.1002/cbin.10960
Fernandes, J. C. R., Acuña, S. M., Aoki, J. I., Floeter-Winter, L. M., and Muxel, S. M. (2019). Long Non-Coding RNAs in the Regulation of Gene Expression: physiology and Disease. Noncoding RNA 5:17. doi: 10.3390/ncrna5010017
Ferrand, J., Noël, D., Lehours, P., Prochazkova-Carlotti, M., Chambonnier, L., Ménard, A., et al. (2011). Human bone marrow-derived stem cells acquire epithelial characteristics through fusion with gastrointestinal epithelial cells. PLoS One 6:e19569. doi: 10.1371/journal.pone.0019569
Freitas, J., Santos, S. G., Gonçalves, R. M., Teixeira, J. H., Barbosa, M. A., and Almeida, M. I. (2019). Genetically Engineered-MSC Therapies for Non-unions, Delayed Unions and Critical-size Bone Defects. Int. J. Mol. Sci. 20:3430. doi: 10.3390/ijms20143430
Friedenstein, A. J., Gorskaja, J. F., and Kulagina, N. N. (1976). Fibroblast precursors in normal and irradiated mouse hematopoietic organs. Exp. Hematol. 4, 267–274.
Fu, Y., Deng, J., Jiang, Q., Wang, Y., Zhang, Y., Yao, Y., et al. (2016). Rapid generation of functional hepatocyte-like cells from human adipose-derived stem cells. Stem Cell Res. Ther. 7:105. doi: 10.1186/s13287-016-0364-6
Furuya, K., Zheng, Y. W., Sako, D., Iwasaki, K., Zheng, D. X., Ge, J. Y., et al. (2019). Enhanced hepatic differentiation in the subpopulation of human amniotic stem cells under 3D multicellular microenvironment. World J. Stem Cells 11, 705–721. doi: 10.4252/wjsc.v11.i9.705
Gao, Y., Xiao, F., Wang, C., Wang, C., Cui, P., Zhang, X., et al. (2018). Long noncoding RNA MALAT1 promotes osterix expression to regulate osteogenic differentiation by targeting miRNA-143 in human bone marrow-derived mesenchymal stem cells. J. Cell Biochem. 119, 6986–6996. doi: 10.1002/jcb.26907
Haynesworth, S. E., Baber, M. A., and Caplan, A. I. (1996). Cytokine expression by human marrow-derived mesenchymal progenitor cells in vitro: effects of dexamethasone and IL-1 alpha. J. Cell Physiol. 166, 585–592. doi: 10.1002/(sici)1097-4652(199603)166:3<585::aid-jcp13<3.0.co;2-6
He, H., Liu, X., Peng, L., Gao, Z., Ye, Y., Su, Y., et al. (2013). Promotion of hepatic differentiation of bone marrow mesenchymal stem cells on decellularized cell-deposited extracellular matrix. Biomed. Res. Int. 2013:406871. doi: 10.1155/2013/406871
He, Q., Wan, C., and Li, G. (2007). Concise review: multipotent mesenchymal stromal cells in blood. Stem Cells 25, 69–77. doi: 10.1634/stemcells.2006-0335
Hefei, W., Yu, R., Haiqing, W., Xiao, W., Jingyuan, W., and Dongjun, L. (2015). Morphological characteristics and identification of islet-like cells derived from rat adipose-derived stem cells cocultured with pancreas adult stem cells. Cell Biol. Int. 39, 253–263. doi: 10.1002/cbin.10387
Hilgendorf, K. I., Johnson, C. T., Mezger, A., Rice, S. L., Norris, A. M., Demeter, J., et al. (2019). Omega-3 Fatty Acids Activate Ciliary FFAR4 to Control Adipogenesis. Cell 179, 1289–1305.e21. doi: 10.1016/j.cell.2019.11.005
Hoshiba, T., Kawazoe, N., and Chen, G. (2012). The balance of osteogenic and adipogenic differentiation in human mesenchymal stem cells by matrices that mimic stepwise tissue development. Biomaterials 33, 2025–2031. doi: 10.1016/j.biomaterials.2011.11.061
Hou, J., Long, H., Zhou, C., Zheng, S., Wu, H., Guo, T., et al. (2017). Long noncoding RNA Braveheart promotes cardiogenic differentiation of mesenchymal stem cells in vitro. Stem Cell Res. Ther. 8:4. doi: 10.1186/s13287-016-0454-5
Hu, B., Lv, X., Chen, H., Xue, P., Gao, B., Wang, X., et al. (2020). Sensory nerves regulate mesenchymal stromal cell lineage commitment by tuning sympathetic tones. J. Clin. Invest. 130, 3483–3498. doi: 10.1172/jci131554
Huang, G. T., Gronthos, S., and Shi, S. (2009). Mesenchymal stem cells derived from dental tissues vs. those from other sources: their biology and role in regenerative medicine. J. Dent. Res. 88, 792–806. doi: 10.1177/0022034509340867
Huang, Y., Zheng, Y., Jin, C., Li, X., Jia, L., and Li, W. (2016). Long Non-coding RNA H19 Inhibits Adipocyte Differentiation of Bone Marrow Mesenchymal Stem Cells through Epigenetic Modulation of Histone Deacetylases. Sci. Rep. 6:28897. doi: 10.1038/srep28897
Jarroux, J., Morillon, A., and Pinskaya, M. (2017). History, Discovery, and Classification of lncRNAs. Adv. Exp. Med. Biol. 1008, 1–46. doi: 10.1007/978-981-10-5203-3_1
Jiang, W., Ma, A., Wang, T., Han, K., Liu, Y., Zhang, Y., et al. (2006). Intravenous transplantation of mesenchymal stem cells improves cardiac performance after acute myocardial ischemia in female rats. Transpl. Int. 19, 570–580. doi: 10.1111/j.1432-2277.2006.00307.x
Jin, G., Sun, J., Isaacs, S. D., Wiley, K. E., Kim, S. T., Chu, L. W., et al. (2011). Human polymorphisms at long non-coding RNAs (lncRNAs) and association with prostate cancer risk. Carcinogenesis 32, 1655–1659. doi: 10.1093/carcin/bgr187
Kfoury, Y., and Scadden, D. T. (2015). Mesenchymal cell contributions to the stem cell niche. Cell Stem Cell 16, 239–253. doi: 10.1016/j.stem.2015.02.019
Konala, V. B., Mamidi, M. K., Bhonde, R., Das, A. K., Pochampally, R., and Pal, R. (2016). The current landscape of the mesenchymal stromal cell secretome: a new paradigm for cell-free regeneration. Cytotherapy 18, 13–24. doi: 10.1016/j.jcyt.2015.10.008
Kretz, M., Webster, D. E., Flockhart, R. J., Lee, C. S., Zehnder, A., Lopez-Pajares, V., et al. (2012). Suppression of progenitor differentiation requires the long noncoding RNA ANCR. Genes Dev. 26, 338–343. doi: 10.1101/gad.182121.111
Lagarde, J., Uszczynska-Ratajczak, B., Carbonell, S., Pérez-Lluch, S., Abad, A., Davis, C., et al. (2017). High-throughput annotation of full-length long noncoding RNAs with capture long-read sequencing. Nat. Genet. 49, 1731–1740. doi: 10.1038/ng.3988
Lai, R. C., Arslan, F., Tan, S. S., Tan, B., Choo, A., Lee, M. M., et al. (2010). Derivation and characterization of human fetal MSCs: an alternative cell source for large-scale production of cardioprotective microparticles. J. Mol. Cell Cardiol. 48, 1215–1224. doi: 10.1016/j.yjmcc.2009.12.021
Lai, R. C., Yeo, R. W., and Lim, S. K. (2015). Mesenchymal stem cell exosomes. Semin Cell Dev. Biol. 40, 82–88. doi: 10.1016/j.semcdb.2015.03.001
Lee, J., Han, D. J., and Kim, S. C. (2008). In vitro differentiation of human adipose tissue-derived stem cells into cells with pancreatic phenotype by regenerating pancreas extract. Biochem. Biophys. Res. Commun. 375, 547–551. doi: 10.1016/j.bbrc.2008.08.064
Li, B., Luan, S., Chen, J., Zhou, Y., Wang, T., Li, Z., et al. (2020). The MSC-Derived Exosomal lncRNA H19 Promotes Wound Healing in Diabetic Foot Ulcers by Upregulating PTEN via MicroRNA-152-3p. Mol. Ther. Nucleic Acids 19, 814–826. doi: 10.1016/j.omtn.2019.11.034
Li, B., Xu, H., Han, H., Song, S., Zhang, X., Ouyang, L., et al. (2018). Exosome-mediated transfer of lncRUNX2-AS1 from multiple myeloma cells to MSCs contributes to osteogenesis. Oncogene 37, 5508–5519. doi: 10.1038/s41388-018-0359-0
Li, Y., Shan, Z., Yang, B., Yang, D., Men, C., Cui, Y., et al. (2018). LncRNA HULC promotes epithelial and smooth-muscle-like differentiation of adipose-derived stem cells by upregulation of BMP9. Pharmazie 73, 49–55. doi: 10.1691/ph.2018.7634
Li, J., Yang, Y., Fan, J., Xu, H., Fan, L., Li, H., et al. (2019). Long noncoding RNA ANCR inhibits the differentiation of mesenchymal stem cells toward definitive endoderm by facilitating the association of PTBP1 with ID2. Cell Death Dis. 10:492. doi: 10.1038/s41419-019-1738-3
Li, Z., Jin, C., Chen, S., Zheng, Y., Huang, Y., Jia, L., et al. (2017). Long non-coding RNA MEG3 inhibits adipogenesis and promotes osteogenesis of human adipose-derived mesenchymal stem cells via miR-140-5p. Mol. Cell Biochem. 433, 51–60. doi: 10.1007/s11010-017-3015-z
Liang, W. C., Fu, W. M., Wang, Y. B., Sun, Y. X., Xu, L. L., Wong, C. W., et al. (2016). H19 activates Wnt signaling and promotes osteoblast differentiation by functioning as a competing endogenous RNA. Sci. Rep. 6:20121. doi: 10.1038/srep20121
Liu, G. H., Barkho, B. Z., Ruiz, S., Diep, D., Qu, J., Yang, S. L., et al. (2011). Recapitulation of premature ageing with iPSCs from Hutchinson-Gilford progeria syndrome. Nature 472, 221–225. doi: 10.1038/nature09879
Liu, Y., Lin, L., Zou, R., Wen, C., Wang, Z., and Lin, F. (2018a). MSC-derived exosomes promote proliferation and inhibit apoptosis of chondrocytes via lncRNA-KLF3-AS1/miR-206/GIT1 axis in osteoarthritis. Cell Cycle 17, 2411–2422. doi: 10.1080/15384101.2018.1526603
Liu, Y., Zou, R., Wang, Z., Wen, C., Zhang, F., and Lin, F. (2018b). Exosomal KLF3-AS1 from hMSCs promoted cartilage repair and chondrocyte proliferation in osteoarthritis. Biochem. J. 475, 3629–3638. doi: 10.1042/bcj20180675
Lopatina, T., Kalinina, N., Karagyaur, M., Stambolsky, D., Rubina, K., Revischin, A., et al. (2019). Correction: adipose-Derived Stem Cells Stimulate Regeneration of Peripheral Nerves: BDNF Secreted by These Cells Promotes Nerve Healing and Axon Growth De Novo. PLoS One 14:e0219946. doi: 10.1371/journal.pone.0219946
Ma, L., Bajic, V. B., and Zhang, Z. (2013). On the classification of long non-coding RNAs. RNA Biol. 10, 925–933. doi: 10.4161/rna.24604
Mao, Q., Liang, X. L., Zhang, C. L., Pang, Y. H., and Lu, Y. X. (2019). LncRNA KLF3-AS1 in human mesenchymal stem cell-derived exosomes ameliorates pyroptosis of cardiomyocytes and myocardial infarction through miR-138-5p/Sirt1 axis. Stem Cell Res. Ther. 10:393. doi: 10.1186/s13287-019-1522-4
Matsui, M., and Corey, D. R. (2017). Non-coding RNAs as drug targets. Nat. Rev. Drug Discov. 16, 167–179. doi: 10.1038/nrd.2016.117
Maymó, J. L., Riedel, R., Pérez-Pérez, A., Magatti, M., Maskin, B., Dueñas, J. L., et al. (2018). Proliferation and survival of human amniotic epithelial cells during their hepatic differentiation. PLoS One 13:e0191489. doi: 10.1371/journal.pone.0191489
Mazini, L., Rochette, L., Admou, B., Amal, S., and Malka, G. (2020). Hopes and Limits of Adipose-Derived Stem Cells (ADSCs) and Mesenchymal Stem Cells (MSCs) in Wound Healing. Int. J. Mol. Sci. 21:1306. doi: 10.3390/ijms21041306
Mehrfarjam, Z., Esmaeili, F., Shabani, L., and Ebrahimie, E. (2016). Induction of pancreatic β cell gene expression in mesenchymal stem cells. Cell Biol. Int. 40, 486–500. doi: 10.1002/cbin.10567
Méndez-Ferrer, S., Michurina, T. V., Ferraro, F., Mazloom, A. R., Macarthur, B. D., Lira, S. A., et al. (2010). Mesenchymal and haematopoietic stem cells form a unique bone marrow niche. Nature 466, 829–834. doi: 10.1038/nature09262
Miao, C., Lei, M., Hu, W., Han, S., and Wang, Q. (2017). A brief review: the therapeutic potential of bone marrow mesenchymal stem cells in myocardial infarction. Stem Cell Res. Ther. 8:242. doi: 10.1186/s13287-017-0697-9
Mirza, A. H., Kaur, S., Brorsson, C. A., and Pociot, F. (2014). Effects of GWAS-associated genetic variants on lncRNAs within IBD and T1D candidate loci. PLoS One 9:e105723. doi: 10.1371/journal.pone.0105723
Moura, S. R., Bras, J. P., Freitas, J., Osório, H., Barbosa, M. A., Santos, S. G., et al. (2020). miR-99a in bone homeostasis: regulating osteogenic lineage commitment and osteoclast differentiation. Bone 134:115303. doi: 10.1016/j.bone.2020.115303
Orciani, M., and Di Primio, R. (2013). Skin-derived mesenchymal stem cells: isolation, culture, and characterization. Methods Mol. Biol. 989, 275–283. doi: 10.1007/978-1-62703-330-5_21
Pan, Y., Xie, Z., Cen, S., Li, M., Liu, W., Tang, S., et al. (2020). Long noncoding RNA repressor of adipogenesis negatively regulates the adipogenic differentiation of mesenchymal stem cells through the hnRNP A1-PTX3-ERK axis. Clin. Transl. Med. 10:e227. doi: 10.1002/ctm2.227
Parolini, O., Alviano, F., Bagnara, G. P., Bilic, G., Bühring, H. J., Evangelista, M., et al. (2008). Concise review: isolation and characterization of cells from human term placenta: outcome of the first international Workshop on Placenta Derived Stem Cells. Stem Cells 26, 300–311. doi: 10.1634/stemcells.2007-0594
Pegtel, D. M., and Gould, S. J. (2019). Exosomes. Annu. Rev. Biochem. 88, 487–514. doi: 10.1146/annurev-biochem-013118-111902
Price, M. J., Chou, C. C., Frantzen, M., Miyamoto, T., Kar, S., Lee, S., et al. (2006). Intravenous mesenchymal stem cell therapy early after reperfused acute myocardial infarction improves left ventricular function and alters electrophysiologic properties. Int. J. Cardiol. 111, 231–239. doi: 10.1016/j.ijcard.2005.07.036
Quinn, J. J., and Chang, H. Y. (2016). Unique features of long non-coding RNA biogenesis and function. Nat. Rev. Genet. 17, 47–62. doi: 10.1038/nrg.2015.10
Romanov, Y. A., Svintsitskaya, V. A., and Smirnov, V. N. (2003). Searching for alternative sources of postnatal human mesenchymal stem cells: candidate MSC-like cells from umbilical cord. Stem Cells 21, 105–110. doi: 10.1634/stemcells.21-1-105
Scacalossi, K. R., van Solingen, C., and Moore, K. J. (2019). Long non-coding RNAs regulating macrophage functions in homeostasis and disease. Vascul. Pharmacol. 114, 122–130. doi: 10.1016/j.vph.2018.02.011
Schmitt, A. M., and Chang, H. Y. (2016). Long Noncoding RNAs in Cancer Pathways. Cancer Cell 29, 452–463. doi: 10.1016/j.ccell.2016.03.010
Scott, M. A., Nguyen, V. T., Levi, B., and James, A. W. (2011). Current methods of adipogenic differentiation of mesenchymal stem cells. Stem Cells Dev. 20, 1793–1804. doi: 10.1089/scd.2011.0040
Shi, T., Hu, W., Hou, H., Zhao, Z., Shang, M., and Zhang, L. (2020). Identification and Comparative Analysis of Long Non-Coding RNA in the Skeletal Muscle of Two Dezhou Donkey Strains. Genes 11:508. doi: 10.3390/genes11050508
Shi, Y., He, G., Lee, W. C., McKenzie, J. A., Silva, M. J., and Long, F. (2017). Gli1 identifies osteogenic progenitors for bone formation and fracture repair. Nat. Commun. 8:2043. doi: 10.1038/s41467-017-02171-2
Shi, Y., Hu, G., Su, J., Li, W., Chen, Q., Shou, P., et al. (2010). Mesenchymal stem cells: a new strategy for immunosuppression and tissue repair. Cell Res. 20, 510–518. doi: 10.1038/cr.2010.44
Song, L., and Tuan, R. S. (2004). Transdifferentiation potential of human mesenchymal stem cells derived from bone marrow. FASEB J. 18, 980–982. doi: 10.1096/fj.03-1100fje
Spees, J. L., Lee, R. H., and Gregory, C. A. (2016). Mechanisms of mesenchymal stem/stromal cell function. Stem Cell Res. Ther. 7:125. doi: 10.1186/s13287-016-0363-7
Stzepourginski, I., Nigro, G., Jacob, J. M., Dulauroy, S., Sansonetti, P. J., Eberl, G., et al. (2017). CD34+ mesenchymal cells are a major component of the intestinal stem cells niche at homeostasis and after injury. Proc. Natl. Acad. Sci. U. S. A. 114, E506–E513. doi: 10.1073/pnas.1620059114
Sui, B. D., Zheng, C. X., Li, M., Jin, Y., and Hu, C. H. (2020). Epigenetic Regulation of Mesenchymal Stem Cell Homeostasis. Trends Cell Biol. 30, 97–116. doi: 10.1016/j.tcb.2019.11.006
Sun, H., Peng, G., Wu, H., Liu, M., Mao, G., Ning, X., et al. (2020). Long non-coding RNA MEG3 is involved in osteogenic differentiation and bone diseases (Review). Biomed. Rep. 13, 15–21. doi: 10.3892/br.2020.1305
Sun, X., Luo, L. H., Feng, L., and Li, D. S. (2018). Down-regulation of lncRNA MEG3 promotes endothelial differentiation of bone marrow derived mesenchymal stem cells in repairing erectile dysfunction. Life Sci. 208, 246–252. doi: 10.1016/j.lfs.2018.07.024
Tan, Y. F., Tang, L., OuYang, W. X., Jiang, T., Zhang, H., and Li, S. J. (2019). β-catenin-coordinated lncRNA MALAT1 up-regulation of ZEB-1 could enhance the telomerase activity in HGF-mediated differentiation of bone marrow mesenchymal stem cells into hepatocytes. Pathol. Res. Pract. 215, 546–554. doi: 10.1016/j.prp.2019.01.002
Tye, C. E., Gordon, J. A., Martin-Buley, L. A., Stein, J. L., Lian, J. B., and Stein, G. S. (2015). Could lncRNAs be the missing links in control of mesenchymal stem cell differentiation? J. Cell Physiol. 230, 526–534. doi: 10.1002/jcp.24834
Vizoso, F. J., Eiro, N., Costa, L., Esparza, P., Landin, M., Diaz-Rodriguez, P., et al. (2019). Mesenchymal stem cells in Homeostasis and systemic diseases: hypothesis, evidences, and therapeutic opportunities. Int. J. Mol. Sci. 20:3738. doi: 10.3390/ijms20153738
Wang, H., Ding, X. G., Yang, J. J., Li, S. W., Zheng, H., Gu, C. H., et al. (2018). LncRNA MIAT facilitated BM-MSCs differentiation into endothelial cells and restored erectile dysfunction via targeting miR-200a in a rat model of erectile dysfunction. Eur. J. Cell Biol. 97, 180–189. doi: 10.1016/j.ejcb.2018.02.001
Wang, K. C., and Chang, H. Y. (2011). Molecular mechanisms of long noncoding RNAs. Mol. Cell 43, 904–914. doi: 10.1016/j.molcel.2011.08.018
Wang, L., Gu, Z., Zhao, X., Yang, N., Wang, F., Deng, A., et al. (2016). Extracellular Vesicles Released from Human Umbilical Cord-Derived Mesenchymal Stromal Cells Prevent Life-Threatening Acute Graft-Versus-Host Disease in a Mouse Model of Allogeneic Hematopoietic Stem Cell Transplantation. Stem Cells Dev. 25, 1874–1883. doi: 10.1089/scd.2016.0107
Wang, Q., Li, Y., Zhang, Y., Ma, L., Lin, L., Meng, J., et al. (2017). LncRNA MEG3 inhibited osteogenic differentiation of bone marrow mesenchymal stem cells from postmenopausal osteoporosis by targeting miR-133a-3p. Biomed. Pharmacother. 89, 1178–1186. doi: 10.1016/j.biopha.2017.02.090
Wang, S., Zhu, R., Li, H., Li, J., Han, Q., and Zhao, R. C. (2019). Mesenchymal stem cells and immune disorders: from basic science to clinical transition. Front. Med. 13, 138–151. doi: 10.1007/s11684-018-0627-y
Wang, Y., Chen, X., Cao, W., and Shi, Y. (2014). Plasticity of mesenchymal stem cells in immunomodulation: pathological and therapeutic implications. Nat. Immunol. 15, 1009–1016. doi: 10.1038/ni.3002
Wang, Z., Huang, Y., and Tan, L. (2020). Downregulation of lncRNA DANCR promotes osteogenic differentiation of periodontal ligament stem cells. BMC Dev. Biol. 20:2. doi: 10.1186/s12861-019-0206-8
Westhrin, M., Xie, M., Olderøy, M., Sikorski, P., Strand, B. L., and Standal, T. (2015). Osteogenic differentiation of human mesenchymal stem cells in mineralized alginate matrices. PLoS One 10:e0120374. doi: 10.1371/journal.pone.0120374
Wosczyna, M. N., Konishi, C. T., Perez Carbajal, E. E., Wang, T. T., Walsh, R. A., Gan, Q., et al. (2019). Mesenchymal Stromal Cells Are Required for Regeneration and Homeostatic Maintenance of Skeletal Muscle. Cell Rep. 27, 2029–2035.e5. doi: 10.1016/j.celrep.2019.04.074
Wu, A. M., Ni, W. F., Huang, Z. Y., Li, Q. L., Wu, J. B., Xu, H. Z., et al. (2015). Analysis of differentially expressed lncRNAs in differentiation of bone marrow stem cells into neural cells. J. Neurol. Sci. 351, 160–167. doi: 10.1016/j.jns.2015.03.011
Wu, J., Zhao, J., Sun, L., Pan, Y., Wang, H., and Zhang, W. B. (2018). Long non-coding RNA H19 mediates mechanical tension-induced osteogenesis of bone marrow mesenchymal stem cells via FAK by sponging miR-138. Bone 108, 62–70. doi: 10.1016/j.bone.2017.12.013
Xia, W., Chen, H., Xie, C., and Hou, M. (2020). Long-noncoding RNA MALAT1 sponges microRNA-92a-3p to inhibit doxorubicin-induced cardiac senescence by targeting ATG4a. Aging 12, 8241–8260. doi: 10.18632/aging.103136
Xian, P., Hei, Y., Wang, R., Wang, T., Yang, J., Li, J., et al. (2019). Mesenchymal stem cell-derived exosomes as a nanotherapeutic agent for amelioration of inflammation-induced astrocyte alterations in mice. Theranostics 9, 5956–5975. doi: 10.7150/thno.33872
Xu, D., Nishimura, T., Zheng, M., Wu, M., Su, H., Sato, N., et al. (2014). Enabling autologous human liver regeneration with differentiated adipocyte stem cells. Cell Transplant. 23, 1573–1584. doi: 10.3727/096368913x673432
Xu, H., Yang, Y., Fan, L., Deng, L., Fan, J., Li, D., et al. (2021). Lnc13728 facilitates human mesenchymal stem cell adipogenic differentiation via positive regulation of ZBED3 and downregulation of the WNT/β-catenin pathway. Stem Cell Res. Ther. 12:176. doi: 10.1186/s13287-021-02250-8
Xu, L., Liu, Y., Sun, Y., Wang, B., Xiong, Y., Lin, W., et al. (2017). Tissue source determines the differentiation potentials of mesenchymal stem cells: a comparative study of human mesenchymal stem cells from bone marrow and adipose tissue. Stem Cell Res. Ther. 8:275. doi: 10.1186/s13287-017-0716-x
Xue, C., Li, X. L., Ba, L., Zhang, M., Yang, Y., Gao, Y., et al. (2021). MSC-derived exosomes can enhance the engiogenesis of euman erain MECs and show eherapeutic eotential in a mouse model of Parkinson’s disease. Aging Dis. (in press). doi: 10.14336/ad.2020.1221
Yang, Q., Jia, L., Li, X., Guo, R., Huang, Y., Zheng, Y., et al. (2018). Long Noncoding RNAs: new Players in the Osteogenic Differentiation of Bone Marrow- and Adipose-Derived Mesenchymal Stem Cells. Stem Cell Rev. Rep. 14, 297–308. doi: 10.1007/s12015-018-9801-5
Yang, X., Yang, J., Lei, P., and Wen, T. (2019). LncRNA MALAT1 shuttled by bone marrow-derived mesenchymal stem cells-secreted exosomes alleviates osteoporosis through mediating microRNA-34c/SATB2 axis. Aging 11, 8777–8791. doi: 10.18632/aging.102264
Ye, X., and Zhang, C. (2017). Effects of Hyperlipidemia and Cardiovascular Diseases on Proliferation, Differentiation and Homing of Mesenchymal Stem Cells. Curr. Stem Cell Res. Ther. 12, 377–387. doi: 10.2174/1574888x12666170316105805
Yeo, R. W., Lai, R. C., Zhang, B., Tan, S. S., Yin, Y., Teh, B. J., et al. (2013). Mesenchymal stem cell: an efficient mass producer of exosomes for drug delivery. Adv. Drug Deliv. Rev. 65, 336–341. doi: 10.1016/j.addr.2012.07.001
Yousefi, F., Lavi Arab, F., Nikkhah, K., Amiri, H., and Mahmoudi, M. (2019). Novel approaches using mesenchymal stem cells for curing peripheral nerve injuries. Life Sci. 221, 99–108. doi: 10.1016/j.lfs.2019.01.052
Yu, Y. B., Bian, J. M., and Gu, D. H. (2015). Transplantation of insulin-producing cells to treat diabetic rats after 90% pancreatectomy. World J. Gastroenterol. 21, 6582–6590. doi: 10.3748/wjg.v21.i21.6582
Yuan, H., Xu, X., Feng, X., Zhu, E., Zhou, J., Wang, G., et al. (2019). A novel long noncoding RNA PGC1β-OT1 regulates adipocyte and osteoblast differentiation through antagonizing miR-148a-3p. Cell Death Differ. 26, 2029–2045. doi: 10.1038/s41418-019-0296-7
Yuan, Z., Li, Q., Luo, S., Liu, Z., Luo, D., Zhang, B., et al. (2016). PPARγ and Wnt Signaling in Adipogenic and Osteogenic Differentiation of Mesenchymal Stem Cells. Curr. Stem Cell Res. Ther. 11, 216–225. doi: 10.2174/1574888x10666150519093429
Yue, W. M., Liu, W., Bi, Y. W., He, X. P., Sun, W. Y., Pang, X. Y., et al. (2008). Mesenchymal stem cells differentiate into an endothelial phenotype, reduce neointimal formation, and enhance endothelial function in a rat vein grafting model. Stem Cells Dev. 17, 785–793. doi: 10.1089/scd.2007.0243
Zhang, H., Miao, Z., He, Z., Yang, Y., Wang, Y., and Feng, M. (2005). The existence of epithelial-to-mesenchymal cells with the ability to support hematopoiesis in human fetal liver. Cell Biol. Int. 29, 213–219. doi: 10.1016/j.cellbi.2004.12.007
Zhang, J., Tao, Z., and Wang, Y. (2018). Long non-coding RNA DANCR regulates the proliferation and osteogenic differentiation of human bone-derived marrow mesenchymal stem cells via the p38 MAPK pathway. Int. J. Mol. Med. 41, 213–219. doi: 10.3892/ijmm.2017.3215
Zhang, L., Sun, X., Chen, S., Yang, C., Shi, B., Zhou, L., et al. (2017). Long noncoding RNA DANCR regulates miR-1305-Smad 4 axis to promote chondrogenic differentiation of human synovium-derived mesenchymal stem cells. Biosci. Rep. 37:BSR20170347. doi: 10.1042/bsr20170347
Zhang, W., Li, J., Suzuki, K., Qu, J., Wang, P., Zhou, J., et al. (2015). Aging stem cells. A Werner syndrome stem cell model unveils heterochromatin alterations as a driver of human aging. Science 348, 1160–1163. doi: 10.1126/science.aaa1356
Zhang, Y., and Tycko, B. (1992). Monoallelic expression of the human H19 gene. Nat. Genet. 1, 40–44. doi: 10.1038/ng0492-40
Zhao, C. H. (2013). [Concept of mesenchymal stem cells: bring more insights into functional research of MSC]. Zhongguo Shi Yan Xue Ye Xue Za Zhi 21, 263–267. doi: 10.7534/j.issn.1009-2137.2013.02.001
Zhao, H., Feng, J., Seidel, K., Shi, S., Klein, O., Sharpe, P., et al. (2014). Secretion of shh by a neurovascular bundle niche supports mesenchymal stem cell homeostasis in the adult mouse incisor. Cell Stem Cell 14, 160–173. doi: 10.1016/j.stem.2013.12.013
Zhou, B. O., Yue, R., Murphy, M. M., Peyer, J. G., and Morrison, S. J. (2014). Leptin-receptor-expressing mesenchymal stromal cells represent the main source of bone formed by adult bone marrow. Cell Stem Cell 15, 154–168. doi: 10.1016/j.stem.2014.06.008
Zhuang, W., Ge, X., Yang, S., Huang, M., Zhuang, W., Chen, P., et al. (2015). Upregulation of lncRNA MEG3 Promotes Osteogenic Differentiation of Mesenchymal Stem Cells From Multiple Myeloma Patients By Targeting BMP4 Transcription. Stem Cells 33, 1985–1997. doi: 10.1002/stem.1989
Zoehler, B., Fracaro, L., Senegaglia, A. C., and Bicalho, M. D. G. (2020). Infusion of Mesenchymal Stem Cells to Treat Graft Versus Host Disease: the Role of HLA-G and the Impact of its Polymorphisms. Stem Cell Rev. Rep. 16, 459–471. doi: 10.1007/s12015-020-09960-1
Keywords: mesenchymal stem cells, long non-coding RNAs, differentiation, homeostasis, exosomes
Citation: Yang Y, Liu S, He C, Chen Z, Lyu T, Zeng L, Wang L, Zhang F, Chen H and Zhao RC (2021) Long Non-coding RNA Regulation of Mesenchymal Stem Cell Homeostasis and Differentiation: Advances, Challenges, and Perspectives. Front. Cell Dev. Biol. 9:711005. doi: 10.3389/fcell.2021.711005
Received: 17 May 2021; Accepted: 21 June 2021;
Published: 22 July 2021.
Edited by:
Wencheng Zhang, Tongji University, ChinaReviewed by:
Zhipeng Fan, Capital Medical University, ChinaCopyright © 2021 Yang, Liu, He, Chen, Lyu, Zeng, Wang, Zhang, Chen and Zhao. This is an open-access article distributed under the terms of the Creative Commons Attribution License (CC BY). The use, distribution or reproduction in other forums is permitted, provided the original author(s) and the copyright owner(s) are credited and that the original publication in this journal is cited, in accordance with accepted academic practice. No use, distribution or reproduction is permitted which does not comply with these terms.
*Correspondence: Fengchun Zhang, emhhbmdmY2NyYUBhbGl5dW4uY29t; Hua Chen, Y2hlbmh1YUBwdW1jaC5jbg==; Robert Chunhua Zhao, emhhb2NodW5odWFAaWJtcy5wdW1jLmVkdS5jbg==
Disclaimer: All claims expressed in this article are solely those of the authors and do not necessarily represent those of their affiliated organizations, or those of the publisher, the editors and the reviewers. Any product that may be evaluated in this article or claim that may be made by its manufacturer is not guaranteed or endorsed by the publisher.
Research integrity at Frontiers
Learn more about the work of our research integrity team to safeguard the quality of each article we publish.