- 1Key Laboratory of Carbohydrate Chemistry and Biotechnology, Ministry of Education, School of Biotechnology, Jiangnan University, Wuxi, China
- 2Department of Pathobiochemistry, Faculty of Pharmacy, Meijo University, Nagoya, Japan
Glycosaminoglycans (GAGs) including chondroitin sulfate, dermatan sulfate, heparan sulfate, and keratan sulfate, except for hyaluronan that is a free polysaccharide, are covalently attached to core proteins to form proteoglycans. More than 50 gene products are involved in the biosynthesis of GAGs. We recently developed a comprehensive glycosylation mapping tool, GlycoMaple, for visualization and estimation of glycan structures based on gene expression profiles. Using this tool, the expression levels of GAG biosynthetic genes were analyzed in various human tissues as well as tumor tissues. In brain and pancreatic tumors, the pathways for biosynthesis of chondroitin and dermatan sulfate were predicted to be upregulated. In breast cancerous tissues, the pathways for biosynthesis of chondroitin and dermatan sulfate were predicted to be up- and down-regulated, respectively, which are consistent with biochemical findings published in the literature. In addition, the expression levels of the chondroitin sulfate-proteoglycan versican and the dermatan sulfate-proteoglycan decorin were up- and down-regulated, respectively. These findings may provide new insight into GAG profiles in various human diseases including cancerous tumors as well as neurodegenerative disease using GlycoMaple analysis.
Introduction
Glycosaminoglycans (GAGs) are linear polysaccharides consisting of repeating disaccharide units. Among these are heparin, heparan sulfate (HS), chondroitin sulfate (CS), dermatan sulfate (DS), and keratan sulfate (KS), which are covalently bound to core proteins, forming proteoglycans (PGs) (Kjellen and Lindahl, 1991; Iozzo, 1998; Lindahl et al., 2015). Hyaluronan (HA) is a free polysaccharide (Lindahl and Rodén, 1972; Hascall, 2019). PGs are distributed in the extracellular matrix and on cell surfaces, and are crucially involved in a wide range of biological processes such as cell adhesion, the regulation of cellular signaling, and assembly of the extracellular matrix (Esko and Selleck, 2002; Häcker et al., 2005; Bülow and Hobert, 2006; Bishop et al., 2007; Sarrazin et al., 2011; Thelin et al., 2013; Xu and Esko, 2014; Mizumoto et al., 2015; Neill et al., 2015).
CS, DS, and HS are covalently linked to specific serine residues usually flanked by a glycine residue on core proteins via a common linker tetrasaccharide region, GlcAβ1–3Gaβ1–3Galβ1–4Xylβ1–O–, where GlcA, Gal, and Xyl stand for glucuronic acid, galactose, and xylose, respectively (Lindahl and Rodén, 1972; Sugahara and Kitagawa, 2000). The biosynthesis of the linker tetrasaccharide is initiated by the transfer of β-Xyl from uridine diphosphate (UDP)-Xyl to the specific serine residue(s) on the core proteins of PGs by β-xylosyltransferase (XYLT) encoded by XYLT1 or XYLT2 (Götting et al., 2000; Pönighaus et al., 2007) at the endoplasmic reticulum, endoplasmic reticulum-Golgi intermediate compartment, or cis-Golgi apparatus (Prydz, 2015). Then, a second Gal is transferred from UDP-Gal to Xylβ-O-serine by β4-galactosyltransferase-I encoded by B4GALT7 (Almeida et al., 1999; Okajima et al., 1999). The C2-position of Xyl is phosphorylated by xylosylkinase encoded by FAM20B (Koike et al., 2009). A third Gal is added to the second Gal residue on the Galβ1–4Xylβ-O-serine from UDP-Gal by β3-galactosyltransferase-II encoded by B3GALT6 (Bai et al., 2001). β3-Glucuronosyltransferase-I encoded by B3GAT3 transfers GlcA to Galβ1–3Galβ1–4Xylβ-O-serine from UDP-GlcA (Kitagawa et al., 1998). Then, a phosphate group in GlcAβ1–3Galβ1–3Galβ1–4Xyl(2-O-phosphate) is removed by 2-O-phosphoxylose phosphatase encoded by PXYLP1 (Koike et al., 2014). The types of GAGs including CS, DS, or HS extended from the linker tetrasaccharide are determined by the structure of the core protein, sulfation and phosphorylation status on the tetrasaccharide, and the biochemical environment of GAGosome, which is a complex of enzymes and regulatory factors in the Golgi apparatus (Sugahara and Kitagawa, 2000; Presto et al., 2008; Prydz, 2015; Izumikawa, 2019).
Heparan sulfate consists of repeating disaccharide units of N-acetylglucosamine (GlcNAc) (Rodén et al., 1992), and GlcA that are polymerized onto the linker tetrasaccharide region of specific core proteins (Lidholt et al., 1989; Lindahl, 1989; Esko and Selleck, 2002; Kim et al., 2003). The initial GlcNAc residue is transferred from UDP-GlcNAc to the tetrasaccharide by α4-N-acetylglucosaminyltransferase (GlcNAcT)-I encoded by exostosin-like 2 (EXTL2) or EXTL3 (Kitagawa et al., 1999; Kim et al., 2001). Thereafter, polymerization of the HS-repeating disaccharide region, [–3GalNAcα1–4GlcAβ1–]n, occurs by enzymatic activities designated as HS-β4-glucuronosyltransferase-II (HS-GlcAT-II) and GlcNAcT-II, which are catalyzed by a HS polymerase enzyme hetero-complex composed of exostosin 1 (EXT1) and EXT2 (Lind et al., 1998; McCormick et al., 1998; Kim et al., 2003). EXTL1 and EXTL3 also show GlcNAcT-II activity (Kim et al., 2001). The GlcNAc residue on the repeating unit is partially converted to N-sulfated glucosamine by a dual enzyme, N-deacetylase/N-sulfotransferase encoded by NDST1, NDST2, NDST3, or NDST4 (Hashimoto et al., 1992; Eriksson et al., 1994; Aikawa and Esko, 1999; Aikawa et al., 2001). HS C5-epimerase encoded by GLCE converts GlcA residues, which are located on the non-reducing side of N-sulfated glucosamine in the repeating disaccharide region of HS, iduronic acid (IdoA) (Li et al., 1997). The disaccharide region can be further O-sulfated at the C2 position of IdoA by HS 2-O-sulfotransferases, and O-sulfated at C3 and C6 positions of GlcNAc or N-sulfated glucosamine by HS 3-O-sulfotransferases and HS 6-O-sulfotransferases, respectively. 3′-phosphoadenosine 5′-phosphosulfate (PAPS) is utilized as the substrate for the sulfation reaction (Kusche-Gullberg and Kjellen, 2003). PAPS is synthesized from ATP and an inorganic sulfate in the cytosol by PAPS synthase encoded by PAPSS1 or PAPSS2 (Venkatachalam, 2003). PAPS is transported into the Golgi lumen from the cytosol by two PAPS transporters encoded by SLC35B2 and SLC35B3 (Kamiyama et al., 2003, 2006).
The repeating disaccharide region of CS and DS, [–4GlcAβ1–3N-acetylgalactosamine (GalNAc)β1–]n and [–4IdoAβ1–3GalNAcβ1–]n, respectively, is also attached to a linker tetrasaccharide, GlcA-Gal-Gal-Xyl, on a serine residue of specific core proteins (Lindahl and Rodén, 1972; Fransson et al., 1993; Lamari and Karamanos, 2006). The initial GalNAc residue is transferred from UDP-GalNAc to the tetrasaccharide by β4-N-acetylgalactosaminyltransferase (GalNAcT)-I encoded by CSGALNACT1 or CSGALNACT2 (Uyama et al., 2002, 2003). The CS-repeating disaccharide region is formed by the alternative addition of GlcA and GalNAc residues from UDP-GlcA and UDP-GalNAc to the non-reducing end of the linker region tetrasaccharide, GlcA-Gal-Gal-Xyl, by CS-GlcAT-II and GalNAcT-II activities, respectively, of a chondroitin synthase (CHSY) family member including CHSY1, CHSY3, chondroitin polymerizing factor (CHPF), and CHPF2 (Kitagawa et al., 2001, 2003; Izumikawa et al., 2007, 2008). The combination of any two heterocomplexes of these five proteins exerts polymerization activity to build the repeating disaccharide region of CS (Kitagawa et al., 2001, 2003; Izumikawa et al., 2007, 2008). After or during biosynthesis of the disaccharide region, GlcA residues in the chondroitin precursor chain are epimerized to IdoA by DS-epimerase encoded by DSE or DSEL (Maccarana et al., 2006; Pacheco et al., 2009). The CS and DS repeating disaccharide regions, [–4GlcAβ1–3GalNAcβ1–]n and [–4IdoAβ1–3GalNAcβ1–]n, respectively, can be modified by sulfation at C4 and C6 positions of GalNAc and C2 position of GlcA and IdoA by chondroitin 4-O-sulfotransferases, chondroitin 6-O-sulfotransferases, and uronyl 2-O-sulfotransferase, respectively (Kusche-Gullberg and Kjellen, 2003).
Keratan sulfate consists of sulfated poly-N-acetyllactosamine, [–4GlcNAcβ1–3Galβ1–]n, which is bound to serine, threonine, or asparagine on specific core proteins through the linkage region such as O-linked or N-linked oligosaccharides, respectively (Stuhlsatz et al., 1989; Caterson and Melrose, 2018). The biosynthesis of the repeating disaccharide region of KS is initiated by β3-N-acetylglucosaminyltransferase encoded by B3GNT7 (Seko and Yamashita, 2004; Kitayama et al., 2007). Thereafter, GlcNAc 6-O-sulfotransferase encoded by CHST5 or CHST6 transfers a sulfate group from PAPS to the GlcNAc residue (Akama et al., 2001, 2002; Narentuya et al., 2019), following the addition of a Gal residue to the GlcNAc6-O-sulfate residue by β4-galactosyltransferase encoded by B4GALT4 (Seko et al., 2003; Kitayama et al., 2007). After the construction of polysaccharide, [–4GlcNAc(6S)β1–3Galβ1–]n, KS Gal 6-O-sulfotransferase is encoded by CHST1 (Fukuta et al., 1997; Akama et al., 2002). KS-PGs are distributed widely in the cornea, cartilage, and brain, and play important roles in collagen fibrillogenesis, tissue hydration, neurotransmission, and nerve regeneration (Caterson and Melrose, 2018).
Hyaluronan is a high molecular weight, natural polymer composed of a repeating disaccharide, [–4GlcAβ1–3GlcNAcβ1–]n (Rodén, 1980; Hascall and Esko, 2015; Abbruzzese et al., 2017; Hascall, 2019). HA is synthesized from cytosolic UDP-GlcNAc and UDP-GlcA by hyaluronan synthase 1 (HAS1), HAS2, or HAS3 at the plasma membrane (Itano and Kimata, 2002), whereas other GAGs are synthesized in the secretory pathway. HAS1 and HAS2 contribute to the synthesis of longer hyaluronan (∼2 × 106 Da and >2 × 106 Da, respectively), whereas HAS3 synthesizes a markedly shorter hyaluronan (∼1 × 106 Da) (Itano et al., 1999). HA is a major component of the extracellular matrix, and is not covalently attached to a core protein (Lindahl and Rodén, 1972; Hascall, 2019). During the tissue injury and repair process, HA is actively produced and plays important roles (Liang et al., 2016).
The abundance of GAGs and their pattern of sulfation are dynamically altered, affecting GAG–protein interactions (Kjellen and Lindahl, 2018) during a number of physiological and pathological processes, such as differentiation, tumorigenesis, inflammation, and bacterial and viral infections (Sasisekharan et al., 2002; Bishop et al., 2007; Iozzo and Sanderson, 2011; Jiang et al., 2011; Kam and Alexander, 2014; Baghy et al., 2016; Morla, 2019). Therefore, the analyses of changes in content as well as sulfation pattern of GAGs are required to elucidate the pathogenesis. However, these analyses are complicated and require special techniques. We previously established GlycoMaple, a comprehensive glycosylation mapping tool based on genetic expression profiles (Huang et al., 2021). With this tool, the expression profiles of glycan-related genes are mapped into glycan metabolic pathways to visualize and estimate glycan biosynthesis or degradation using transcriptional data. Transcripts per million transcripts (TPM) values of genes in RNA-Seq analyses are commonly utilized to evaluate and compare gene expression levels among samples from distinct cells or tissues. By uploading RNA-Seq data, glycan metabolic pathways in the tissue of interest can be automatically drawn to understand the glycosylation process. In this study, the expression levels of genes involved in GAG biosynthetic pathways in various tissues and tumor tissues have been addressed using gene expression profile data available in public databases, thereby allowing the estimation and comparison of amounts as well as sulfation pattern of GAGs between normal and disease states.
Methods
Expression data of GAG-related genes (Supplementary Table 1) in 37 human tissues were downloaded from the Human Protein Atlas database1 (Supplementary Table 2). TPM values of GAG-related genes (Supplementary Table 1) and PG genes (Supplementary Table 3) from healthy tissue in GTEx and primary tumor tissues in TCGA were obtained from UCSC Xena2 (Goldman et al., 2020). Raw data (log2[TPM + 0.001]) in Xena were converted to TPM values (Supplementary Tables 4, 6). TPM values of samples were input in GlycoMaple, and then pathways were visualized (Huang et al., 2021). When several genes overlapped in a reaction, the maximum TPM value among the values of overlapping genes was used to represent this reaction. When several gene products comprised a reaction complex, the minimum TPM value of the subunit genes was used to represent the reaction. To compare GAG biosynthetic pathways between tumor and normal tissues, fold changes in expression of genes, whose median TPM + 1 values increased by >1.5 and decreased by <0.667, are shown as pink and green arrows, respectively, in the pathways. The heatmaps and boxplots for gene expression profiles were drawn by R (ver. 3.6.2) (R Foundation for Statistical Computing, Vienna, Austria). The data used for boxplots were converted to log2 (TPM + 1). The Wilcoxon matched-pairs signed rank test was used to compare gene expression levels between primary tumor and normal tissues.
Results and Discussion
GAG Expression Profiles of Human Tissues
We previously listed 950 human glycan-related genes and established a tool named GlycoMaple, which can visualize 19 human glycan metabolic pathways and estimate the glycan structures synthesized in cells or tissues (Huang et al., 2021). In this study, we focused on GAG biosynthetic pathways. Among 950 glycan-related genes, those involved in: (1) biosynthesis of GAG backbones, (2) sulfation modification of HS, (3) sulfation modification of CS and DS, (4) biosynthesis of KS, and (5) biosynthesis and catabolism of HA in GlycoMaple pathways, were selected as GAG-related genes (Supplementary Figure 1). In addition, genes encoding PAPS transporters (SLC35B2 and SLC35B3), a UDP-Xyl and UDP-GlcNAc transporter (SLC35B4), and a UDP-Gal transporter (SLC35A2) were included. PAPSS1 and PAPSS2, CANT1, and IMPAD1/BPNT2 encoding PAPS synthases 1 and 2, calcium-activated nucleotidase 1/UDP-diphosphatase, and 3′-phosphoadenosine 5′-phosphate 3′-phosphatase, respectively, were also listed. Furthermore, genes encoding regulators of GAG biosynthesis such as maintenance of Golgi-resident glycosyltransferases (GOLPH3) (Chang et al., 2013), a Golgi-localized protease (SPPL3) (Kuhn et al., 2015), a transcriptional regulator (ZNF263) (Weiss et al., 2020), and an epigenetic factor (KDM2B) (Weiss et al., 2021) were also added to the list. In total, 66 genes were listed in these categories (Supplementary Table 1).
To evaluate GAG biosynthesis capability in human tissue, we used RNA-Seq data from 37 human tissues, obtained from the Human Protein Atlas (Pontén et al., 2008). TPM is a value that indicates how many transcripts are present in each transcript when there are 1 million total transcripts in the sample (Wagner et al., 2012). The TPM is a method frequently utilized for comparing samples. First, gene expression profiles in each reaction were compared among tissues (Figure 1). The tissues were clustered into two groups by gene expression profiles of GAG biosynthetic reactions. One cluster included the cerebral cortex, thyroid gland, placenta, testis, ovary, endometrium, smooth muscle, cervix/uterine, prostate, fallopian tube, seminal vesicle, adrenal gland, parathyroid gland, spleen, lymph node, skin, lung, urinary bladder, appendix, and gallbladder. This cluster showed relatively higher expression of GAG-related genes, suggesting stronger productive ability of GAGs. In contrast, tissues such as the tonsil, liver, skeletal muscle, pancreas, salivary gland, and heart muscle were clustered together in the same tree because of lower expression of GAG-related genes. Certain digestive organs including the duodenum, small intestine, colon, and rectum were sub-clustered in this group because of the relatively low expression of genes involved in the biosynthesis of HS, CS, and DS. Instead, genes required for KS biosynthesis were expressed in the digestive tissues.
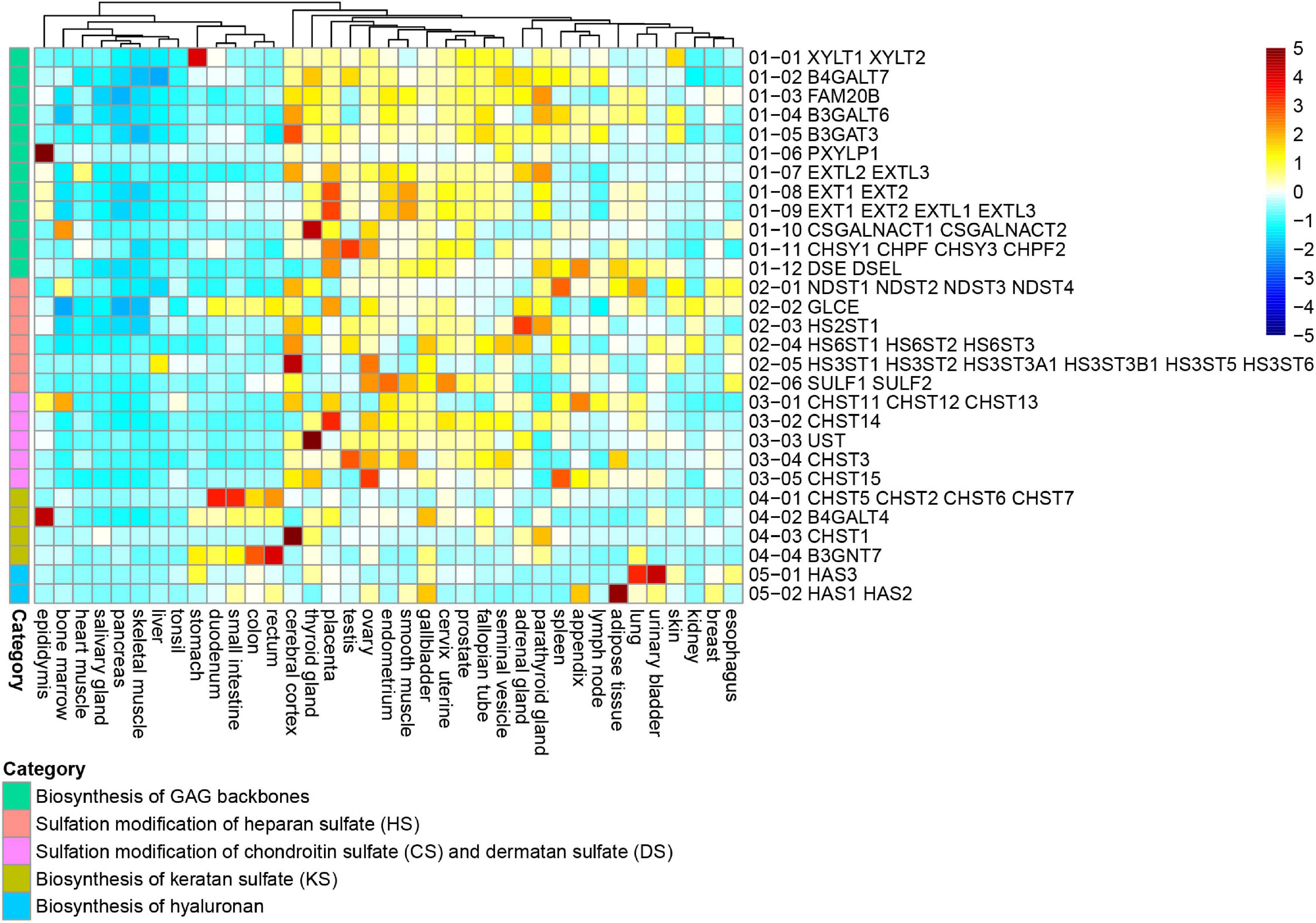
Figure 1. Expression profiles of GAG-related genes in human tissues. Expression data of genes responsible for 29 reactions in five categories including biosynthesis of GAG backbones (green), sulfation of HS (red), sulfation of CS and DS (pink), biosynthesis of KS (ocher), and biosynthesis of HA (blue). Expression data were obtained from 37 human tissues of the Human Protein Atlas. The reaction numbers are correlated with Supplementary Figure 1 and Supplementary Table 1. Transcripts per million transcripts (TPM) values in each of 29 reactions were normalized (z-score) and visualized as a heatmap.
We next examined the Pearson correlation of 66 GAG-related gene expression in 37 human tissues to identify similarities in the expression patterns. In the Pearson correlation heatmap for GAG-related genes, many genes required for the biosynthesis of GAG backbones, including XYLT1, XYLT2, FAM20B, B4GALT7, B3GALT6, B3GAT3, EXT1, EXT2, EXTL2, EXTL3, CHSY1, CHSY3, CHPF, CHPF2, CSGALNACT1, DSE, and DSEL, clustered together (Figure 2 and Supplementary Table 2). The expression patterns of regulator genes for GAG biosynthesis including SPPL3, GOLPH3, and ZNF263, and SLC35B2, SLC35B4, PAPSS1, and IMPAD1, which encode a PAPS transporter, UDP-Xyl/UDP-GlcNAc dual transporter, PAPS synthase 1, and 3′-phosphoadenosine 5′-phosphate 3′-phosphatase, respectively, were also correlated with genes required for biosynthesis of sulfated GAGs in human tissues (Figure 2 and Supplementary Table 2). These findings suggest that expression levels of these genes are regulated by similar mechanisms such as regulation by corresponding signal transductions as well as transcriptional factors. On the other hand, some other genes encoding regulators and transporters were not clustered in the group (Figure 2). For example, the expression patterns of SLC35A2, whose gene product imports UDP-Gal into the lumen of the Golgi apparatus, were different from those of genes categorized as necessary for the biosynthesis of GAG backbones. This is reasonable because UDP-Gal is utilized not only for GAG biosynthesis, but also for biosynthesis of other glycans, such as N-glycans, mucin-type O-glycans, and glycolipid. HAS1 and HAS2, which encode hyaluronan synthase to produce a higher molecular weight form of HA, shared close expression patterns (Figure 2), indicating linkage between gene function and transcriptional regulation. Genes required for sulfation of GAGs were distributed in different trees, although some of them, such as NDST3, HS3ST2, HS3ST3, and HS3ST5, showed correlated expression patterns in normal human tissues (Figure 2 and Supplementary Table 2). It has been reported that several genes such as B3GAT3, EXT2, GLCE, NDST2, HS3ST1, HS3ST6, HS6ST1, and CSGALNACT2 are upregulated by treatment with 4-methylumbelliferyl-β-xyloside, which inhibits the biosynthesis of GAGs (Sasaki et al., 2019). Some of these genes possess enhancer elements named PG stress response elements, PGSE-A and PGSE-B, in their promoter region, which regulate the transcription activation upon Golgi stress caused by PG defects (Sasaki et al., 2019). Among these genes, CSGALNACT2, NDST2, and HS3ST1 were clustered in the Pearson correlation heatmap (Figure 2), suggesting that their expression levels are regulated in a common manner.
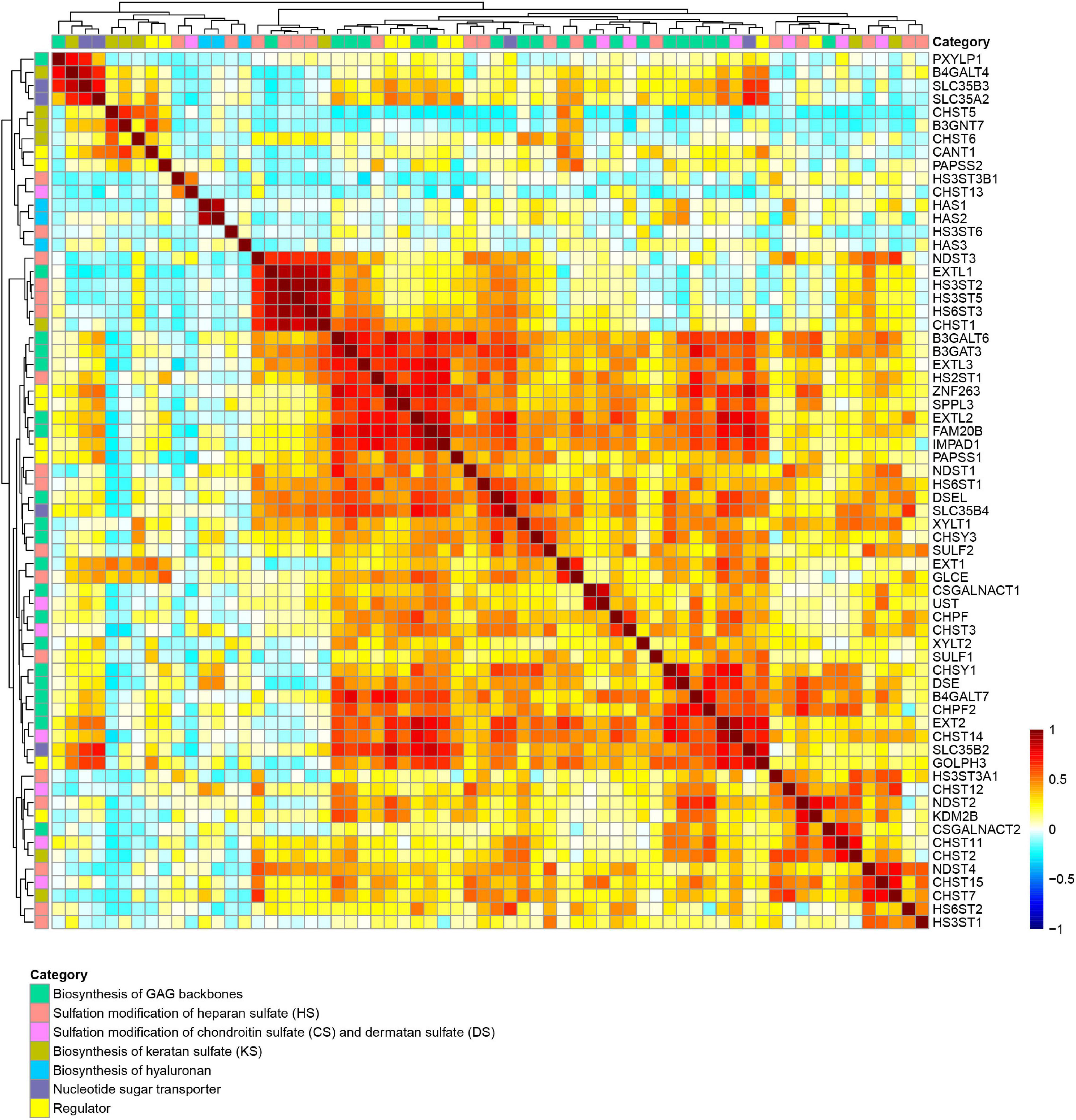
Figure 2. Correlation of expression of GAG-related genes among human tissues. Similarity of expression patterns of 56 genes involved in GAG biosynthesis in 37 human tissues, including biosynthesis of GAG backbones (green), sulfation of HS (red), sulfation of CS and DS (pink), biosynthesis of KS (ocher), biosynthesis of HA (blue), nucleotide sugar transporters (purple), and regulators (yellow) were visualized as a Pearson correlation heatmap. The Pearson correlation values (–1 to 1) are presented as color changes (blue to red).
Prediction of Alterations in GAG Structure in Human Tumor Tissues
Because GAGs are involved in cell adhesion, migration, proliferation, and inflammation, changes in GAG levels can affect tumor development (Sasisekharan et al., 2002; Bishop et al., 2007; Iozzo and Sanderson, 2011; Mizumoto and Sugahara, 2013; Wei et al., 2020). The GlycoMaple tool can compare glycan metabolic pathways between two samples based on gene expression data. The comparison function enables us to reveal underlying changes of PGs or GAGs between normal and tumor tissues. We compared GAG biosynthetic pathways between several primary tumor and normal tissues using RNA-Seq data from two public databases: the Cancer Genome Atlas Program (TCGA) and Genotype-Tissue Expression Project (GTEx) (GTEx Consortium, 2017; Hoadley et al., 2018). TPM values of GAG-related genes were used as expression values, which were obtained from RNA-Seq data of primary tumor and normal tissue samples (brain, pancreas, breast, adrenal glands, and thyroid glands) processed in UCSC Xena (Goldman et al., 2020). Based on these expression values, GAG biosynthetic pathways were then analyzed using GlycoMaple.
Spatio/temporal expression of PGs and biosynthesis of GAGs are critical for establishment and maintenance of fundamental functions of the central nervous system (Karamanos et al., 2018). In brain cancers, various GAG and PG levels have been reported to change (Vitale et al., 2019). The medians of TPM from 662 primary brain tumors and 1,146 normal brain tissues were added into the glycosylation pathways. Pink and green arrows indicate whether expression of the genes (TPM + 1) coding the GAG biosynthetic enzymes were increased more than 1.5-fold and decreased to less than 0.667-fold, respectively, in primary tumors, when compared with the corresponding normal tissues (Figure 3). In primary brain tumor samples, many of the reaction steps in GAG biosynthesis were upregulated (Figure 3). In particular, almost all of the reactions involved in the biosynthesis of GAG backbones were markedly increased (Figure 3A). The expression levels of XYLT1, XYLT2, B4GALT7, B3GALT6, PXYLP1, EXT1, EXT2, EXTL3, CHSY1, CHPF, CHPF2, CSGALNACT1, DSE, and DSEL were increased (Figure 3B). In addition, sulfation of chondroitin and dermatan was predicted to increase in the brain during tumorigenesis, as contributed by CHST3, CHST11, CHST12, CHST14, CHST15, and UST (Figures 3C,D). Besides, the expression levels of several genes encoding core proteins of PG were increased to varying degrees (Supplementary Figure 2A and Supplementary Table 4). It has been reported that various PGs are upregulated in brain cancers. For example, high levels of HA receptors including epican (CD44) were correlated with poor prognosis in cancer patients (Yan and Wang, 2020). RNA levels for fibronectin (FN1), brevican (BCAN), versican (VCAN), perlecan (HSPG2), and several laminins were high in glioblastomas compared with in the normal brain (Dzikowski et al., 2021; von Spreckelsen et al., 2021). A high level of CD74 was expressed in the monocytic subset of immune suppressive myeloid-derived suppressor cells and localized in the tumor microenvironment (Alban et al., 2020). Glypican 2 (GPC2) was selectively expressed in neuroblastoma (Nagarajan et al., 2018). In our analysis, particularly, transcript levels of CD44, BCAN, VCAN, CD74, and GPC2 increased more than five-fold and were the most upregulated PGs in brain tumor tissues, whereas only the expression of GPC5 was downregulated among the PGs (Supplementary Figure 2A and Supplementary Tables 4, 5). This prediction is consistent with previous reports that PGs help drive multiple oncogenic pathways in tumor cells and promote critical tumor–microenvironment interactions in cancer (Iozzo and Sanderson, 2011; Wade et al., 2013; Theocharis et al., 2015; Schaefer et al., 2017; Yan and Wang, 2020). Furthermore, the expression levels of genes involved in the regulation of GAG biosynthesis such as SPPL3, GOLPH3, ZNF263, and KDM2B; in the biosynthesis of a sulfate donor, PAPS synthase encoded by PAPSS1 and PAPSS2; in the transport of PAPS encoded by SLC35B2; and in the catabolism of UDP and 3′-phosphoadenosine 5′-phosphate encoded by CANT1 and IMPAD1, respectively, were also increased by more than 1.5 times in brain tumors compared with normal tissues (Supplementary Figure 2B and Supplementary Table 4). These results suggest that demand for the biosynthesis and sulfation of GAGs changes the expression of regulator genes, which might affect the amount as well as sulfation patterns of GAGs. In contrast, the expression levels of biosynthetic enzymes for HA and KS did not show a definite trend between normal and tumor tissues based upon GlycoMaple predictions (data not shown). This indicates that expression levels of biosynthetic enzymes for HA as well as KS and catabolic enzymes for HA might not significantly change, at least in the brain tumors examined.
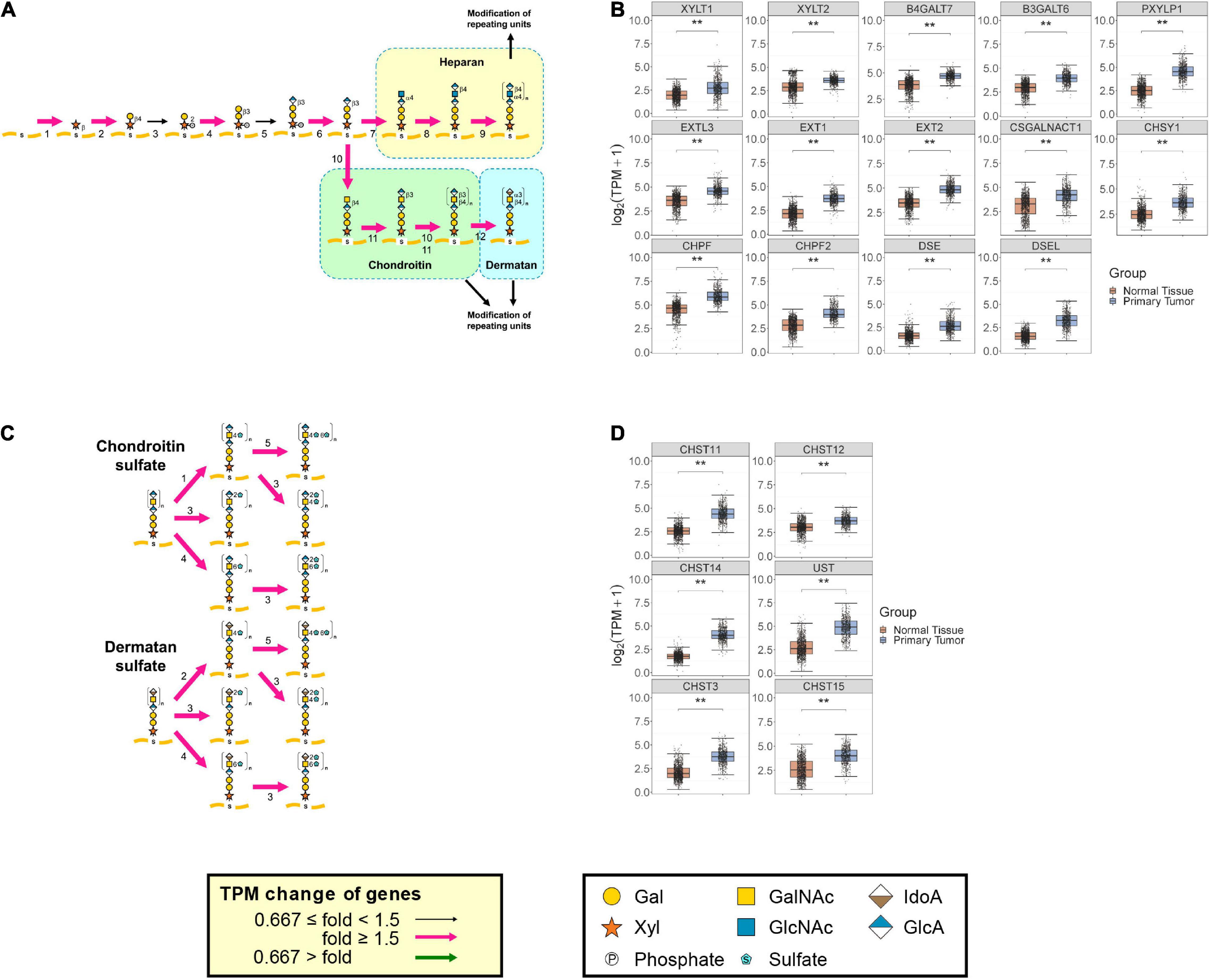
Figure 3. Comparison of expression of GAG biosynthetic enzymes between primary brain tumors and normal tissues. Expression changes of genes responsible for reactions in pathways for biosynthesis of GAG backbones (A) and sulfation of CS and DS (C) between primary brain tumors (N = 662) and normal tissues (N = 1146) were visualized using GlycoMaple. Median TPM values of GAG-related genes in each sample were used for mapping. If several genes overlapped in a reaction, the maximum TPM value among the overlapping genes was used. When several gene products comprised a reaction complex, the minimum TPM value of the subunit genes was used. Fold changes of gene expression whose median TPM + 1 value was increased by >1.5 are shown as pink arrows. Genes that are significantly changed among tumor and normal tissues (Wilcoxon matched-pairs signed rank test; **P < 0.0001) in GAG backbones (B) and sulfation of CS and DS (D) are shown as boxplots. The numbers of each step indicate the enzymes responsible for biosynthesis of GAG backbones (A) and sulfation of CS and DS (B). (A) 1, XYLT1 and XYLT2; 2, B4GALT7; 3, FAM20B; 4, B3GALT6; 5, B3GAT3; 6, PXYLP1; 7, EXTL2 and EXTL3; 8 and 9, EXT1 and EXT2; 10, CSGALNACT1 and CSGALNACT2; 11, CHSY1, CHSY3, CHPF, and CHPF2; 12, DSE and DSEL. (C) 1, CHST11, CHST12, and CHST13; 2, CHST14; 3, UST; 4, CHST3; 5, CHST15.
We also analyzed GAG pathways in pancreatic carcinoma. GAGs are produced at low levels in healthy pancreatic tissue (Theocharis et al., 2000). In contrast, the amounts of GAGs in human pancreatic carcinoma are increased 4-fold, and in particular, show a 22-fold increase of CS and 12-fold increase of HA (Theocharis et al., 2000). The emerging expression of GAGs is considered to be a biological tumor marker for pancreatic tissues. The medians of TPM from 178 primary pancreatic tumors and 165 normal tissues were used to visualize the glycosylation pathways in GlycoMaple. Almost all steps involved in GAG biosynthesis including HS, CS, DS, KS, and HA were expressed in the pancreatic tumor tissues, and increased markedly compared with healthy tissues (Supplementary Figure 3), which was partially consistent with previous reports (Theocharis et al., 2000; Skandalis et al., 2006). It should be noted, however, that the reports showed no changes in the content or molecular size of HS, suggesting that in silico analysis is not always correct. In addition, it was reported that 6-O-sulfated CS on two PGs, versican (VCAN) and decorin (DCN), was upregulated in pancreatic cancer (Skandalis et al., 2006). The VCAN expression level in pancreatic neuroendocrine tumor tissues was found to be higher than in normal pancreatic tissues (Gao et al., 2020). In this study, the RNA-seq data indicated that the expression levels of both CHST3 and CHST15 encoding chondroitin 6-O-sulfotransferase and GalNAc-4-O-sulfate 6-O-sulfotransferase in tumors were increased 4.0 and 3.5 times, respectively, in pancreatic tumors compared with that in normal tissues (Supplementary Figure 3 and Supplementary Table 4). The expression levels of VCAN and DCN were increased 4.8 and 1.5 times, respectively (Supplementary Table 4). Besides, it has been reported that endocan (ESM1) expression in pancreatic neuroendocrine tumor correlates with poor clinical outcomes (Lin et al., 2017). The overexpression of lumican (LUM) and biglycan (BGN) has been reported in pancreatic cancers (Weber et al., 2001; Ishiwata et al., 2007). A high syndecan 1 (SDC1) mRNA level tended to increase the mortality rate due to pancreatic cancer (Wu et al., 2020). In our analysis, expression of all PGs except for neurocan (NCAN), which showed almost no expression, were increased in pancreatic carcinoma (Supplementary Figure 3F). Particularly, VCAN, ESM1, LUM, BGN, and SDC1 were the five most upregulated PGs. Similar to brain tumors, genes encoding transporters and regulators related to GAG biosynthesis were also upregulated in pancreatic tumors (Supplementary Figure 3G and Supplementary Tables 4, 5).
In breast cancer, the DS content has been reported to decrease significantly, whereas the CS content increased in the central area of breast carcinoma tissues compared with fibroadenoma (Olsen et al., 1988). A similar change in GAG levels was observed in prostate cancer, where the DS level decreased and the CS level increased, when compared with normal tissue (De Klerk et al., 1984). GlycoMaple data including expression levels of biosynthetic enzymes for GAGs correlate well with these trends including the amounts of GAGs (Figure 4). In primary breast tumors, there is reduced expression of DSE and DSEL, which are the genes encoding DS epimerase converting GlcA to IdoA, to generate dermatan from the chondroitin precursor chain (Figures 4A,B). In addition, CHPF and CHPF2, whose products are required for the polymerization of chondroitin, were upregulated in breast cancer tissues (Figures 4A,B). Consistent with this result, down- and up-regulations of DSE and CHPF, respectively, were also observed in ductal carcinoma in situ compared with non-malignant breast tissue (Potapenko et al., 2015). It has also been demonstrated that among PG and GAG-related genes, ACAN, VCAN, XYLT2, B3GALT6, CHSY1, CHPF, CHST11, and CHST15 were upregulated in malignant breast cancer tissue, while CHST3 was downregulated (Potapenko et al., 2010). These findings were consistent with our data: expression levels of ACAN, VCAN, B3GALT6, CHPF, CHST11, and CHST15 were increased more than 1.5 times in breast tumors, whereas expression of CHST3 was 3.4-times decreased (Figure 4B, Supplementary Figure 4A, and Supplementary Table 4). In terms of PGs, it has been reported that ESM1 was overexpressed in triple-negative breast cancer cell lines as well as in patient tissues, which is correlated with a poor prognosis (Fernandez-Vega et al., 2013). SDC1 expression was activated, when compared with the very low level of expression in normal breast tissue, while expression of DCN decreased two—five-fold (Eshchenko et al., 2007). BGN was upregulated in human breast cancers, particularly in the tumor stroma compartment, compared with normal mammary glands (Cong et al., 2021). VCAN mRNA levels were upregulated in breast cancer tissues (Takahashi et al., 2012). In contrast, normal breast tissues exhibited high expression levels of GPC3, while the expression was reduced in tumors (Guereno et al., 2020). Despite the fact that HSPG2 expression was correlated with poor patient survival and is considered as a therapeutic target in triple-negative breast cancer (Kalscheuer et al., 2019), the expression levels were two-fold higher in normal breast compared with breast cancer tissues (Jansson et al., 2020). Our analysis also showed changes in expression of those genes: upregulation of SDC1, ESM1, VCAN, and BGN, and downregulation of GPC3, HSPG2, and DCN in breast cancer tissues (Supplementary Figure 4A and Supplementary Tables 4, 5).
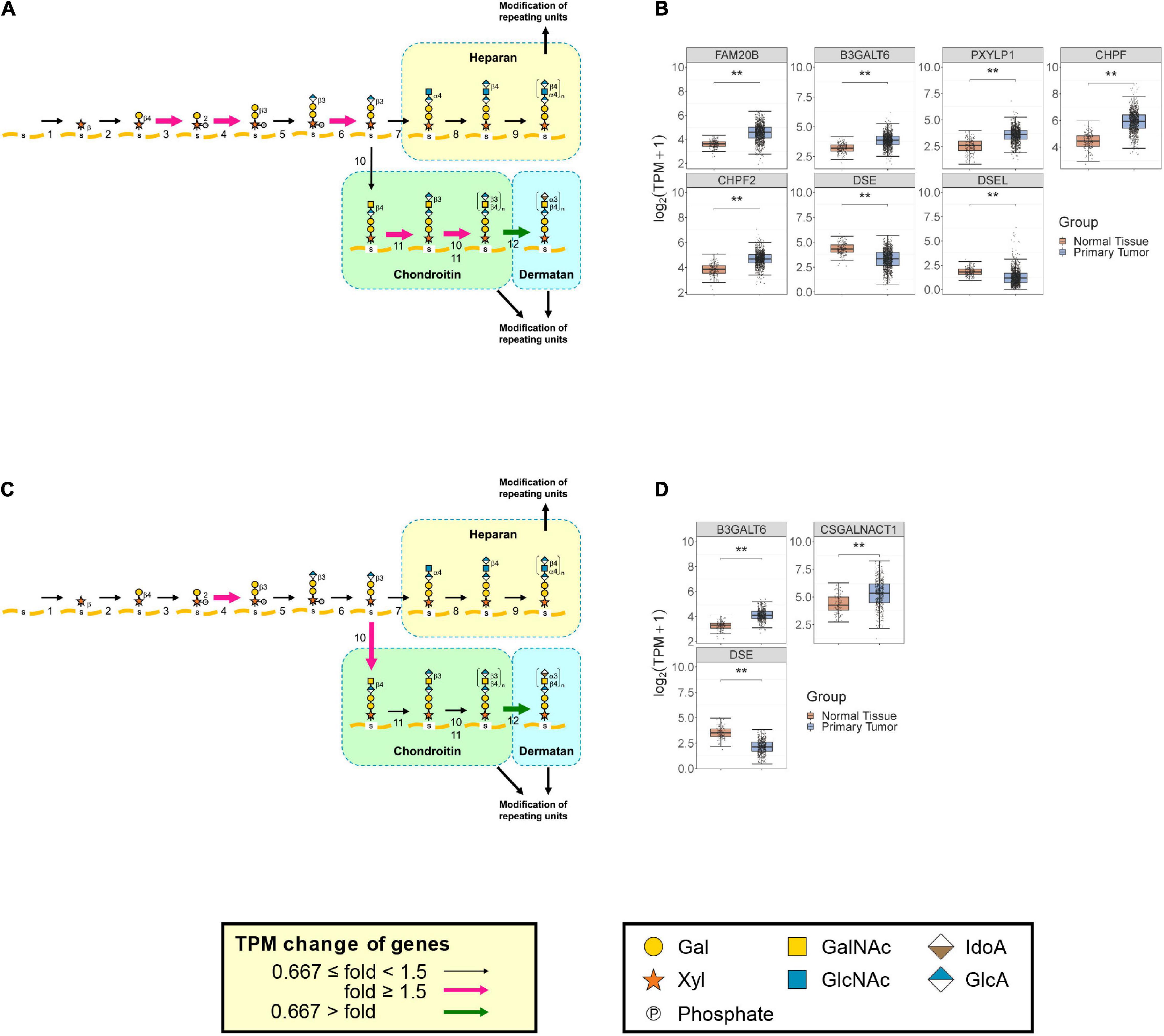
Figure 4. Comparison of expression of GAG biosynthetic enzymes between breast and prostate primary tumors and normal tissues. Expression changes of genes responsible for biosynthetic pathways for GAG backbones between primary breast tumors (N = 1092) and normal tissues (N = 179) (A) or primary prostate tumors (N = 495) and normal tissues (N = 100) (C) were visualized using GlycoMaple, as described in Figure 3. Fold changes of gene expression whose median TPM + 1 value was increased by >1.5 and decreased by <0.667 are shown as pink and green arrows, respectively. GAG core biosynthesis genes that were significantly changed among tumor and normal tissues (Wilcoxon matched-pairs signed rank test; **P < 0.0001) in breast (B) and prostate (D) are shown as boxplots. The numbers of each step are referred to in the legend of Figure 3.
In primary prostate tumors, DSE expression was decreased to less than half (Figures 4C,D). In addition, a greater than two-fold increase was found in CSGALNACT1, whose product initiates the biosynthesis of the disaccharide region of the chondroitin chain (Figures 4C,D). These results are consistent with the findings of decreased DS and increased CS in pancreatic cancerous tissues (De Klerk et al., 1984), suggesting that GlycoMaple is a powerful tool for estimating changes of GAGs in diseased tissues. In terms of PGs, it has been reported that the expression of fibromodulin (FMOD) in malignant prostate tissues is significantly upregulated compared with that in benign tissues (Bettin et al., 2016). A significant decrease in tissue DCN expression is associated with tumor progression and metastasis in certain types of cancer including prostate cancer (Rezaie et al., 2020). The GPC2 mRNA expression level was utilized to predict survival associated with prostate cancer (Xu et al., 2018). In our analysis, upregulations of neuroglycan C (CSPG5), bamacan (SMC3), and collagen type IX alpha2 (COL9A2) and downregulations of GPC1, GPC2, HSPG2, CSPG4, and DCN were detected (Supplementary Figure 4B and Supplementary Table 5).
Finally, we estimated GAG changes in the adrenal and thyroid glands during tumorization. GlycoMaple analysis predicted decreased levels of CS and DS, because of the downregulation of CSGALNACT1 and DSE in both the adrenal gland and thyroid gland tumors compared with that in normal tissues (Supplementary Figure 5). To date, there has been no report that GAG levels are changed in those tissues during tumorization. Therefore, it is worth examining the amounts of CS and DS in tumor tissues.
Concluding Remarks
In this study, we analyzed the expression levels of GAG biosynthetic enzymes as well as PGs in various normal and tumor tissues. GAG biosynthetic levels differed, depending upon the tissue functions and requirements. Analyses of the amount and sulfation pattern of GAGs are not easy to perform and require relatively abundant starting materials. The estimation of glycan structures based on gene expression data could be performed easily from small amounts of samples, and is useful to discover properties of cells of interest and obtain clues to structural changes among cell types. We applied GlycoMaple analysis to visualize the expression of genes involved in GAG biosynthesis and PG levels and to estimate glycan changes based on gene expression. Comprehensive analysis using gene expression levels in human tissues revealed new findings showing that expression of some genes required for GAG biosynthesis are regulated in a similar manner. For example, expression of genes required for the biosynthesis of GAG backbones including CS, DS, HS, and HA may be similarly regulated. GlycoMaple estimation showed that GAG biosynthetic patterns and core proteins of PGs were markedly changed during tumor progression in some tissues, which correlated with previous research. The process of CS formation would be upregulated during the formation of many tumor types (Berto et al., 2001; Sakko et al., 2008; Svensson et al., 2011). However, there are several limitations in GlycoMaple analysis (Huang et al., 2021). First, estimations of GAG changes in tumors using GlycoMaple, are just predictions based on expression changes of genes related to GAG biosynthesis. Second, the estimation of glycans from GlycoMaple is not quantitative. Therefore, after identifying potential target pathways from these estimations, it is essential to validate GAG as well as PG levels using biochemical analyses. Nonetheless, GlycoMaple could provide a ‘bird’s eye view’ of glycosylation pathways in cells/tissues of interest and clues for focusing on altered glycosylation pathways between diseased and normal tissues. Changes in glycosylation patterns during tumor progression were widely observed. In the future, GlycoMaple analysis could contribute to the development of biomarkers and clinical diagnostics using transcriptional data from clinical patient samples.
Data Availability Statement
The original contributions generated for this study are included in the article/Supplementary Material, further inquiries can be directed to the corresponding author.
Author Contributions
MF and Y-FH conceptualized and designed the study and wrote a draft of the manuscript. Y-FH conducted analyses and validated the results. SM strengthened the background of GAG. All authors checked and edited the manuscript.
Funding
This work was supported by grants from the National Natural Science Foundation of China 32071278 and 31770853 (MF), the Program of Introducing Talents of Discipline to Universities 111-2-06, and the International Joint Research Laboratory for Investigation of Glycoprotein Biosynthesis at Jiangnan University. This work was also supported by a Grant-in-Aid for Scientific Research (C) from the Japan Society for the Promotion of Science (#19K07054) (SM).
Conflict of Interest
The authors declare that the research was conducted in the absence of any commercial or financial relationships that could be construed as a potential conflict of interest.
Publisher’s Note
All claims expressed in this article are solely those of the authors and do not necessarily represent those of their affiliated organizations, or those of the publisher, the editors and the reviewers. Any product that may be evaluated in this article, or claim that may be made by its manufacturer, is not guaranteed or endorsed by the publisher.
Acknowledgments
We thank Rachel James, from Liwen Bianji, Edanz Group China, for editing the English text of a draft of this manuscript.
Supplementary Material
The Supplementary Material for this article can be found online at: https://www.frontiersin.org/articles/10.3389/fcell.2021.709018/full#supplementary-material
Footnotes
References
Abbruzzese, F., Basoli, F., Costantini, M., Giannitelli, S. M., Gori, M., Mozetic, P., et al. (2017). Hyaluronan: an overview. J. Biol. Regul. Homeost. Agents 31(4 Suppl. 2), 9–22.
Aikawa, J., and Esko, J. D. (1999). Molecular cloning and expression of a third member of the heparan sulfate/heparin glcnac N-deacetylase/N-sulfotransferase family. J. Biol. Chem. 274, 2690–2695. doi: 10.1074/jbc.274.5.2690
Aikawa, J., Grobe, K., Tsujimoto, M., and Esko, J. D. (2001). Multiple isozymes of heparan sulfate/heparin glcnac N-deacetylase/GlcN N-sulfotransferase: structure and activity of the fourth member, NDST4. J. Biol. Chem. 276, 5876–5882. doi: 10.1074/jbc.M009606200
Akama, T. O., Misra, A. K., Hindsgaul, O., and Fukuda, M. N. (2002). Enzymatic synthesis in vitro of the disulfated disaccharide unit of corneal keratan sulfate. J. Biol. Chem. 277, 42505–42513. doi: 10.1074/jbc.M207412200
Akama, T. O., Nakayama, J., Nishida, K., Hiraoka, N., Suzuki, M., McAuliffe, J., et al. (2001). Human corneal GlcNac 6-O-sulfotransferase and mouse intestinal GlcNac 6-O-sulfotransferase both produce keratan sulfate. J. Biol. Chem. 276, 16271–16278. doi: 10.1074/jbc.M009995200
Alban, T. J., Bayik, D., Otvos, B., Rabljenovic, A., Leng, L., Jia-Shiun, L., et al. (2020). Glioblastoma myeloid-derived suppressor cell subsets express differential macrophage migration inhibitory factor receptor profiles that can be targeted to reduce immune suppression. Front. Immunol. 11:1191. doi: 10.3389/fimmu.2020.01191
Almeida, R., Levery, S. B., Mandel, U., Kresse, H., Schwientek, T., Bennett, E. P., et al. (1999). Cloning and expression of a proteoglycan UDP-galactose: beta-xylose β1,4-galactosyltransferase I. A seventh member of the human β4-galactosyltransferase gene family. J. Biol. Chem. 274, 26165–26171. doi: 10.1074/jbc.274.37.26165
Baghy, K., Tatrai, P., Regos, E., and Kovalszky, I. (2016). Proteoglycans in liver cancer. World J. Gastroenterol. 22, 379–393. doi: 10.3748/wjg.v22.i1.379
Bai, X., Zhou, D., Brown, J. R., Crawford, B. E., Hennet, T., and Esko, J. D. (2001). Biosynthesis of the linkage region of glycosaminoglycans: cloning and activity of galactosyltransferase II, the sixth member of the β1,3-galactosyltransferase family (β3GalT6). J. Biol. Chem. 276, 48189–48195. doi: 10.1074/jbc.M107339200
Berto, A. G., Oba, S. M., Michelacci, Y. M., and Sampaio, L. O. (2001). Galactosaminoglycans from normal myometrium and leiomyoma. Braz. J. Med. Biol. Res. 34, 633–637. doi: 10.1590/s0100-879x2001000500011
Bettin, A., Reyes, I., and Reyes, N. (2016). Gene expression profiling of prostate cancer-associated genes identifies fibromodulin as potential novel biomarker for prostate cancer. Int. J. Biol. Markers 31, e153–e162. doi: 10.5301/jbm.5000184
Bishop, J. R., Schuksz, M., and Esko, J. D. (2007). Heparan sulphate proteoglycans fine-tune mammalian physiology. Nature 446, 1030–1037. doi: 10.1038/nature05817
Bülow, H. E., and Hobert, O. (2006). The molecular diversity of glycosaminoglycans shapes animal development. Annu. Rev. Cell Dev. Biol. 22, 375–407. doi: 10.1146/annurev.cellbio.22.010605.093433
Caterson, B., and Melrose, J. (2018). Keratan sulfate, a complex glycosaminoglycan with unique functional capability. Glycobiology 28, 182–206. doi: 10.1093/glycob/cwy003
Chang, W. L., Chang, C. W., Chang, Y. Y., Sung, H. H., Lin, M. D., Chang, S. C., et al. (2013). The Drosophila GOLPH3 homolog regulates the biosynthesis of heparan sulfate proteoglycans by modulating the retrograde trafficking of exostosins. Development 140, 2798–2807. doi: 10.1242/dev.087171
Cong, L., Maishi, N., Annan, D. A., Young, M. F., Morimoto, H., Morimoto, M., et al. (2021). Inhibition of stromal biglycan promotes normalization of the tumor microenvironment and enhances chemotherapeutic efficacy. Breast Cancer Res. 23:51. doi: 10.1186/s13058-021-01423-w
De Klerk, D. P., Lee, D. V., and Human, H. J. (1984). Glycosaminoglycans of human prostatic cancer. J. Urol. 131, 1008–1012. doi: 10.1016/s0022-5347(17)50750-8
Dzikowski, L., Mirzaei, R., Sarkar, S., Kumar, M., Bose, P., Bellail, A., et al. (2021). Fibrinogen in the glioblastoma microenvironment contributes to the invasiveness of brain tumor-initiating cells. Brain Pathol. (in press). e12947. doi: 10.1111/bpa.12947
Eriksson, I., Sandbäck, D., Ek, B., Lindahl, U., and Kjellén, L. (1994). cDNA cloning and sequencing of mouse mastocytoma glucosaminyl N-deacetylase/N-sulfotransferase, an enzyme involved in the biosynthesis of heparin. J. Biol. Chem. 269, 10438–10443. doi: 10.1016/s0021-9258(17)34079-6
Eshchenko, T. Y., Rykova, V. I., Chernakov, A. E., Sidorov, S. V., and Grigorieva, E. V. (2007). Expression of different proteoglycans in human breast tumors. Biochemistry 72, 1016–1020. doi: 10.1134/s0006297907090143
Esko, J. D., and Selleck, S. B. (2002). Order out of chaos: assembly of ligand binding sites in heparan sulfate. Annu. Rev. Biochem. 71, 435–471. doi: 10.1146/annurev.biochem.71.110601.135458
Fernandez-Vega, I., Garcia, O., Crespo, A., Castanon, S., Menendez, P., Astudillo, A., et al. (2013). Specific genes involved in synthesis and editing of heparan sulfate proteoglycans show altered expression patterns in breast cancer. BMC Cancer 13:24. doi: 10.1186/1471-2407-13-24
Fransson, L.-A., Cheng, F., Yoshida, K., Heinegård, D., Malmström, A., and Schmidtchen, A. (1993). “Patterns of epimerization and sulphation in dermatan sulphate chains,” in Dermatan Sulphate Proteoglycans: Chemistry, Biology, Chemical Pathology, ed. J. E. Scott (London: Portland Press), 11–25.
Fukuta, M., Inazawa, J., Torii, T., Tsuzuki, K., Shimada, E., and Habuchi, O. (1997). Molecular cloning and characterization of human keratan sulfate Gal-6-sulfotransferase. J. Biol. Chem. 272, 32321–32328. doi: 10.1074/jbc.272.51.32321
Gao, H., Cheng, Y., Chen, Y., Luo, F., Shao, Y., Sun, Z., et al. (2020). The expression of versican and its role in pancreatic neuroendocrine tumors. Pancreatology 20, 142–147. doi: 10.1016/j.pan.2019.11.009
Goldman, M. J., Craft, B., Hastie, M., Repecka, K., McDade, F., Kamath, A., et al. (2020). Visualizing and interpreting cancer genomics data via the Xena platform. Nat. Biotechnol. 38, 675–678. doi: 10.1038/s41587-020-0546-8
Götting, C., Kuhn, J., Zahn, R., Brinkmann, T., and Kleesiek, K. (2000). Molecular cloning and expression of human UDP-D-xylose: proteoglycan core protein β-D-xylosyltransferase and its first isoform XT-II. J. Mol. Biol. 304, 517–528. doi: 10.1006/jmbi.2000.4261
GTEx Consortium (2017). Genetic effects on gene expression across human tissues. Nature 550, 204–213. doi: 10.1038/nature24277
Guereno, M., Delgado Pastore, M., Lugones, A. C., Cercato, M., Todaro, L., Urtreger, A., et al. (2020). Glypican-3 (GPC3) inhibits metastasis development promoting dormancy in breast cancer cells by p38 MAPK pathway activation. Eur. J. Cell Biol. 99:151096. doi: 10.1016/j.ejcb.2020.151096
Häcker, U., Nybakken, K., and Perrimon, N. (2005). Heparan sulphate proteoglycans: the sweet side of development. Nat. Rev. Mol. Cell Biol. 6, 530–541. doi: 10.1038/nrm1681
Hascall, V. C. (2019). The journey of hyaluronan research in the journal of biological chemistry. J. Biol. Chem. 294, 1690–1696. doi: 10.1074/jbc.TM118.005836
Hascall, V., and Esko, J. D. (2015). “Hyaluronan,” in Essentials of Glycobiology, eds A. Varki, R. D. Cummings, J. D. Esko, P. Stanley, G. W. Hart, M. Aebi, et al. (Cold Spring Harbor, NY: Cold Spring Harbor Laboratory Press), 197–206.
Hashimoto, Y., Orellana, A., Gil, G., and Hirschberg, C. B. (1992). Molecular cloning and expression of rat liver N-heparan sulfate sulfotransferase. J. Biol. Chem. 267, 15744–15750. doi: 10.1016/s0021-9258(19)49598-7
Hoadley, K. A., Yau, C., Hinoue, T., Wolf, D. M., Lazar, A. J., Drill, E., et al. (2018). Cell-of-origin patterns dominate the molecular classification of 10,000 tumors from 33 types of cancer. Cell 173, 291–304. doi: 10.1016/j.cell.2018.03.022
Huang, Y. F., Aoki, K., Akase, S., Ishihara, M., Liu, Y. S., Yang, G., et al. (2021). Global mapping of glycosylation pathways in human-derived cells. Dev. Cell 56, 1195–1209.e7. doi: 10.1016/j.devcel.2021.02.023
Iozzo, R. V. (1998). Matrix proteoglycans: from molecular design to cellular function. Annu. Rev. Biochem. 67, 609–652. doi: 10.1146/annurev.biochem.67.1.609
Iozzo, R. V., and Sanderson, R. D. (2011). Proteoglycans in cancer biology, tumor microenvironment and angiogenesis. J. Cell. Mol. Med. 15, 1013–1031. doi: 10.1111/j.1582-4934.2010.01236.x
Ishiwata, T., Cho, K., Kawahara, K., Yamamoto, T., Fujiwara, Y., Uchida, E., et al. (2007). Role of lumican in cancer cells and adjacent stromal tissues in human pancreatic cancer. Oncol. Rep. 18, 537–543.
Itano, N., and Kimata, K. (2002). Mammalian hyaluronan synthases. IUBMB Life 54, 195–199. doi: 10.1080/15216540214929
Itano, N., Sawai, T., Yoshida, M., Lenas, P., Yamada, Y., Imagawa, M., et al. (1999). Three isoforms of mammalian hyaluronan synthases have distinct enzymatic properties. J. Biol. Chem. 274, 25085–25092. doi: 10.1074/jbc.274.35.25085
Izumikawa, T. (2019). Regulatory mechanism of 2-O-phosphorylation of xylose in the glycosaminoglycan-linkage region of the tetrasaccharide. Trend Glycosci. Glycotechnol. 31, E57–E62. doi: 10.4052/tigg.1955.2E
Izumikawa, T., Koike, T., Shiozawa, S., Sugahara, K., Tamura, J., and Kitagawa, H. (2008). Identification of chondroitin sulfate glucuronyltransferase as chondroitin synthase-3 involved in chondroitin polymerization: chondroitin polymerization is achieved by multiple enzyme complexes consisting of chondroitin synthase family members. J. Biol. Chem. 283, 11396–11406. doi: 10.1074/jbc.M707549200
Izumikawa, T., Uyama, T., Okuura, Y., Sugahara, K., and Kitagawa, H. (2007). Involvement of chondroitin sulfate synthase-3 (chondroitin synthase-2) in chondroitin polymerization through its interaction with chondroitin synthase-1 or chondroitin-polymerizing factor. Biochem. J. 403, 545–552. doi: 10.1042/BJ20061876
Jansson, M., Billing, O., Herdenberg, C., Lundin, C., Tolockiene, E., Nazemroaya, A., et al. (2020). Expression and circulating levels of perlecan in breast cancer – implications for oestrogen dependent stromal remodeling. J. Mammary Gland Biol. Neoplasia 25, 69–77. doi: 10.1007/s10911-020-09447-2
Jiang, D., Liang, J., and Noble, P. W. (2011). Hyaluronan as an immune regulator in human diseases. Physiol. Rev. 91, 221–264. doi: 10.1152/physrev.00052.2009
Kalscheuer, S., Khanna, V., Kim, H., Li, S., Sachdev, D., DeCarlo, A., et al. (2019). Discovery of HSPG2 (Perlecan) as a therapeutic target in triple negative breast cancer. Sci. Rep. 9:12492. doi: 10.1038/s41598-019-48993-6
Kam, T., and Alexander, M. (2014). Drug-induced immune thrombocytopenia. J. Pharm. Pract. 27, 430–439. doi: 10.1177/0897190014546099
Kamiyama, S., Sasaki, N., Goda, E., Ui-Tei, K., Saigo, K., Narimatsu, H., et al. (2006). Molecular cloning and characterization of a novel 3′-phosphoadenosine 5′-phosphosulfate transporter, PAPST2. J. Biol. Chem. 281, 10945–10953. doi: 10.1074/jbc.M508991200
Kamiyama, S., Suda, T., Ueda, R., Suzuki, M., Okubo, R., Kikuchi, N., et al. (2003). Molecular cloning and identification of 3′-phosphoadenosine 5′-phosphosulfate transporter. J. Biol. Chem. 278, 25958–25963. doi: 10.1074/jbc.M302439200
Karamanos, N. K., Piperigkou, Z., Theocharis, A. D., Watanabe, H., Franchi, M., Baud, S., et al. (2018). Proteoglycan chemical diversity drives multifunctional cell regulation and therapeutics. Chem. Rev. 118, 9152–9232. doi: 10.1021/acs.chemrev.8b00354
Kim, B. T., Kitagawa, H., Tamura, J., Saito, T., Kusche-Gullberg, M., Lindahl, U., et al. (2001). Human tumor suppressor EXT gene family members EXTL1 and EXTL3 encode α1,4-N-acetylglucosaminyltransferases that likely are involved in heparan sulfate/heparin biosynthesis. Proc. Natl. Acad. Sci. U.S.A. 98, 7176–7181. doi: 10.1073/pnas.131188498
Kim, B. T., Kitagawa, H., Tanaka, J., Tamura, J., and Sugahara, K. (2003). In vitro heparan sulfate polymerization: crucial roles of core protein moieties of primer substrates in addition to the EXT1-EXT2 interaction. J. Biol. Chem. 278, 41618–41623. doi: 10.1074/jbc.M304831200
Kitagawa, H., Izumikawa, T., Uyama, T., and Sugahara, K. (2003). Molecular cloning of a chondroitin polymerizing factor that cooperates with chondroitin synthase for chondroitin polymerization. J. Biol. Chem. 278, 23666–23671. doi: 10.1074/jbc.m302493200
Kitagawa, H., Shimakawa, H., and Sugahara, K. (1999). The tumor suppressor EXT-like gene EXTL2 encodes an α1, 4-N-acetylhexosaminyltransferase that transfers N-acetylgalactosamine and N-acetylglucosamine to the common glycosaminoglycan-protein linkage region. The key enzyme for the chain initiation of heparan sulfate. J. Biol. Chem. 274, 13933–13937. doi: 10.1074/jbc.274.20.13933
Kitagawa, H., Tone, Y., Tamura, J., Neumann, K. W., Ogawa, T., Oka, S., et al. (1998). Molecular cloning and expression of glucuronyltransferase I involved in the biosynthesis of the glycosaminoglycan-protein linkage region of proteoglycans. J. Biol. Chem. 273, 6615–6618. doi: 10.1074/jbc.273.12.6615
Kitagawa, H., Uyama, T., and Sugahara, K. (2001). Molecular cloning and expression of a human chondroitin synthase. J. Biol. Chem. 276, 38721–38726. doi: 10.1074/jbc.M106871200
Kitayama, K., Hayashida, Y., Nishida, K., and Akama, T. O. (2007). Enzymes responsible for synthesis of corneal keratan sulfate glycosaminoglycans. J. Biol. Chem. 282, 30085–30096. doi: 10.1074/jbc.M703695200
Kjellen, L., and Lindahl, U. (1991). Proteoglycans: structures and interactions. Annu. Rev. Biochem. 60, 443–475. doi: 10.1146/annurev.bi.60.070191.002303
Kjellen, L., and Lindahl, U. (2018). Specificity of glycosaminoglycan-protein interactions. Curr. Opin. Struct. Biol. 50, 101–108. doi: 10.1016/j.sbi.2017.12.011
Koike, T., Izumikawa, T., Sato, B., and Kitagawa, H. (2014). Identification of phosphatase that dephosphorylates xylose in the glycosaminoglycan-protein linkage region of proteoglycans. J. Biol. Chem. 289, 6695–6708. doi: 10.1074/jbc.m113.520536
Koike, T., Izumikawa, T., Tamura, J.-I., and Kitagawa, H. (2009). FAM20B is a kinase that phosphorylates xylose in the glycosaminoglycan–protein linkage region. Biochem. J. 421, 157–162. doi: 10.1042/bj20090474
Kuhn, P. H., Voss, M., Haug-Kroper, M., Schroder, B., Schepers, U., Brase, S., et al. (2015). Secretome analysis identifies novel signal Peptide peptidase-like 3 (Sppl3) substrates and reveals a role of Sppl3 in multiple Golgi glycosylation pathways. Mol. Cell. Proteomics 14, 1584–1598. doi: 10.1074/mcp.M115.048298
Kusche-Gullberg, M., and Kjellen, L. (2003). Sulfotransferases in glycosaminoglycan biosynthesis. Curr. Opin. Struct. Biol. 13, 605–611. doi: 10.1016/j.sbi.2003.08.002
Lamari, F. N., and Karamanos, N. K. (2006). Structure of chondroitin sulfate. Adv. Pharmacol. 53, 33–48. doi: 10.1016/S1054-3589(05)53003-5
Li, J.-P., Hagner-McWhirter, Å, Kjellén, L., Palgi, J., Jalkanen, M., and Lindahl, U. (1997). Biosynthesis of heparin/heparan sulfate: cdna cloning and expression of D-glucuronyl C5-epimerase from bovine lung. J. Biol. Chem. 272, 28158–28163. doi: 10.1074/jbc.272.44.28158
Liang, J., Jiang, D., and Noble, P. W. (2016). Hyaluronan as a therapeutic target in human diseases. Adv. Drug Deliv. Rev. 97, 186–203. doi: 10.1016/j.addr.2015.10.017
Lidholt, K., Kjellen, L., and Lindahl, U. (1989). Biosynthesis of heparin. Relationship between the polymerization and sulphation processes. Biochem. J. 261, 999–1007. doi: 10.1042/bj2610999
Lin, L. Y., Yeh, Y. C., Chu, C. H., Won, J. G. S., Shyr, Y. M., Chao, Y., et al. (2017). Endocan expression is correlated with poor progression-free survival in patients with pancreatic neuroendocrine tumors. Medicine 96:e8262. doi: 10.1097/MD.0000000000008262
Lind, T., Tufaro, F., McCormick, C., Lindahl, U., and Lidholt, K. (1998). The putative tumor suppressors EXT1 and EXT2 are glycosyltransferases required for the biosynthesis of heparan sulfate. J. Biol. Chem. 273, 26265–26268. doi: 10.1074/jbc.273.41.26265
Lindahl, U. (1989). “Biosynthesis of heparin and related polysaccharides,” in Heparin, eds D. A. Lane and U. Lindahl (London: Edward Arnold), 159–189.
Lindahl, U., and Rodén, L. (1972). “Carbohydrate-protein linkages in proteoglycans of animal, plant and bacterial origin,” in Glycoproteins: Their Composition, Structure and Function, ed. A. Gottschalk (Amsterdam: Elsevier), 491–517.
Lindahl, U., Couchman, J., Kimata, K., and Esko, J. D. (2015). “Proteoglycans and sulfated glycosaminoglycans,” in Essentials of Glycobiology, eds A. Varki, R. D. Cummings, J. D. Esko, P. Stanley, G. W. Hart, M. Aebi, et al. (Cold Spring Harbor, NY: Cold Spring Harbor Laboratory Press), 207–221.
Maccarana, M., Olander, B., Malmström, J., Tiedemann, K., Aebersold, R., Lindahl, U., et al. (2006). Biosynthesis of dermatan sulfate: chondroitin-glucuronate C5-epimerase is identical to SART2. J. Biol. Chem. 281, 11560–11568. doi: 10.1074/jbc.M513373200
McCormick, C., Leduc, Y., Martindale, D., Mattison, K., Esford, L. E., Dyer, A. P., et al. (1998). The putative tumour suppressor EXT1 alters the expression of cell-surface heparan sulfate. Nat. Genet. 19, 158–161. doi: 10.1038/514
Mizumoto, S., and Sugahara, K. (2013). Glycosaminoglycans are functional ligands for receptor for advanced glycation end-products in tumors. FEBS J. 280, 2462–2470. doi: 10.1111/febs.12156
Mizumoto, S., Yamada, S., and Sugahara, K. (2015). Molecular interactions between chondroitin–dermatan sulfate and growth factors/receptors/matrix proteins. Curr. Opin. Struct. Biol. 34, 35–42. doi: 10.1016/j.sbi.2015.06.004
Morla, S. (2019). Glycosaminoglycans and glycosaminoglycan mimetics in cancer and inflammation. Int. J. Mol. Sci. 20:1963. doi: 10.3390/ijms20081963
Nagarajan, A., Malvi, P., and Wajapeyee, N. (2018). Heparan sulfate and heparan sulfate proteoglycans in cancer initiation and progression. Front. Endocrinol. 9:483. doi: 10.3389/fendo.2018.00483
Narentuya, Takeda-Uchimura, Y., Foyez, T., Zhang, Z., Akama, T. O., Yagi, H., et al. (2019). GlcNAc6ST3 is a keratan sulfate sulfotransferase for the protein-tyrosine phosphatase PTPRZ in the adult brain. Sci. Rep. 9:4387. doi: 10.1038/s41598-019-40901-2
Neill, T., Schaefer, L., and Iozzo, R. V. (2015). Oncosuppressive functions of decorin. Mol. Cell. Oncol. 2:e975645. doi: 10.4161/23723556.2014.975645
Okajima, T., Yoshida, K., Kondo, T., and Furukawa, K. (1999). Human homolog of Caenorhabditis elegans sqv-3 gene is galactosyltransferase I involved in the biosynthesis of the glycosaminoglycan-protein linkage region of proteoglycans. J. Biol. Chem. 274, 22915–22918. doi: 10.1074/jbc.274.33.22915
Olsen, E. B., Trier, K., Eldov, K., and Ammitzboll, T. (1988). Glycosaminoglycans in human breast cancer. Acta Obstet. Gynecol. Scand. 67, 539–542. doi: 10.3109/00016348809029866
Pacheco, B., Malmström, A., and Maccarana, M. (2009). Two dermatan sulfate epimerases form iduronic acid domains in dermatan sulfate. J. Biol. Chem. 284, 9788–9795. doi: 10.1074/jbc.M809339200
Pönighaus, C., Ambrosius, M., Casanova, J., Prante, C., Kuhn, J., Esko, J., et al. (2007). Human xylosyltransferase II is involved in the biosynthesis of the uniform tetrasaccharide linkage region in chondroitin sulfate and heparan sulfate proteoglycans. J. Biol. Chem. 282, 5201–5206. doi: 10.1074/jbc.M611665200
Pontén, F., Jirström, K., and Uhlen, M. (2008). The human protein atlas—a tool for pathology. J. Pathol. 216, 387–393. doi: 10.1002/path.2440
Potapenko, I. O., Haakensen, V. D., Luders, T., Helland, A., Bukholm, I., Sorlie, T., et al. (2010). Glycan gene expression signatures in normal and malignant breast tissue; possible role in diagnosis and progression. Mol. Oncol. 4, 98–118. doi: 10.1016/j.molonc.2009.12.001
Potapenko, I. O., Luders, T., Russnes, H. G., Helland, A., Sorlie, T., Kristensen, V. N., et al. (2015). Glycan-related gene expression signatures in breast cancer subtypes; relation to survival. Mol. Oncol. 9, 861–876. doi: 10.1016/j.molonc.2014.12.013
Presto, J., Thuveson, M., Carlsson, P., Busse, M., Wile ìn, M., Eriksson, I., et al. (2008). Heparan sulfate biosynthesis enzymes EXT1 and EXT2 affect NDST1 expression and heparan sulfate sulfation. Proc. Natl. Acad. Sci. U.S.A. 105, 4751–4756. doi: 10.1073/pnas.0705807105
Prydz, K. (2015). Determinants of glycosaminoglycan (GAG) structure. Biomolecules 5, 2003–2022. doi: 10.3390/biom5032003
Rezaie, R., Falakian, Z., Mazloomzadeh, S., Ayati, M., Morakabati, A., Teimouri Dastjerdan, M. R., et al. (2020). While urine and plasma decorin remain unchanged in prostate cancer, prostatic tissue decorin has a prognostic value. Iran. Biomed. J 24, 229–235. doi: 10.29252/ibj.24.4.229
Rodén, L. (1980). “Structure and metabolism of connective tissue proteoglycans,” in The Biochemistry of Glycoproteins and Proteoglycans, ed. W. J. Lennarz (New York: Plenum Press), 267–371. doi: 10.1007/978-1-4684-1006-8_7
Rodén, L., Ananth, S., Campbell, P., Curenton, T., Ekborg, G., Manzella, S., et al. (1992). “Heparin—an introduction,” in Heparin and Related Polysaccharides, eds D. A. LaneIngemar, I. Björk, and U. Lindahl (New York: Plenum Press), 1–20. doi: 10.1007/978-1-4899-2444-5_1
Sakko, A. J., Butler, M. S., Byers, S., Reinboth, B. J., Stahl, J., Kench, J. G., et al. (2008). Immunohistochemical level of unsulfated chondroitin disaccharides in the cancer stroma is an independent predictor of prostate cancer relapse. Cancer Epidemiol. Biomarkers Prev. 17, 2488–2497. doi: 10.1158/1055-9965.EPI-08-0204
Sarrazin, S., Lamanna, W. C., and Esko, J. D. (2011). Heparan sulfate proteoglycans. Cold Spring Harb. Perspect. Biol. 3:a004952.
Sasaki, K., Komori, R., Taniguchi, M., Shimaoka, A., Midori, S., Yamamoto, M., et al. (2019). PGSE is a novel enhancer regulating the proteoglycan pathway of the mammalian Golgi stress response. Cell Struct. Funct. 44, 1–19. doi: 10.1247/csf.18031
Sasisekharan, R., Shriver, Z., Venkataraman, G., and Narayanasami, U. (2002). Roles of heparan-sulphate glycosaminoglycans in cancer. Nat. Rev. Cancer 2, 521–528. doi: 10.1038/nrc842
Schaefer, L., Tredup, C., Gubbiotti, M. A., and Iozzo, R. V. (2017). Proteoglycan neofunctions: regulation of inflammation and autophagy in cancer biology. FEBS J. 284, 10–26. doi: 10.1111/febs.13963
Seko, A., and Yamashita, K. (2004). β1, 3-N-acetylglucosaminyltransferase-7 (β3Gn-T7) acts efficiently on keratan sulfate-related glycans. FEBS Lett. 556, 216–220. doi: 10.1016/s0014-5793(03)01440-6
Seko, A., Dohmae, N., Takio, K., and Yamashita, K. (2003). β1,4-Galactosyltransferase (β4GalT)-IV is specific for GlcNAc 6-O-sulfate. β4GalT-IV acts on keratan sulfate-related glycans and a precursor glycan of 6-sulfosialyl-Lewis X. J. Biol. Chem. 278, 9150–9158. doi: 10.1074/jbc.m211480200
Skandalis, S. S., Kletsas, D., Kyriakopoulou, D., Stavropoulos, M., and Theocharis, D. A. (2006). The greatly increased amounts of accumulated versican and decorin with specific post-translational modifications may be closely associated with the malignant phenotype of pancreatic cancer. Biochim. Biophys. Acta 1760, 1217–1225. doi: 10.1016/j.bbagen.2006.03.021
Stuhlsatz, H. W., Keller, R., Becker, G., Oeben, M., Lennartz, L., Fischer, D. C., et al. (1989). “Structure of keratan sulphate proteoglycans: core proteins, linkage regions, carbohydrate chains,” in Keratan Sulphate Proteoglycans: Chemistry, Biology, Chemical Pathology, eds H. Greiling and J. E. Scott (London: Portland Press), 1–11.
Sugahara, K., and Kitagawa, H. (2000). Recent advances in the study of the biosynthesis and functions of sulfated glycosaminoglycans. Curr. Opin. Struct. Biol. 10, 518–527. doi: 10.1016/s0959-440x(00)00125-1
Svensson, K. J., Christianson, H. C., Kucharzewska, P., Fagerstrom, V., Lundstedt, L., Borgquist, S., et al. (2011). Chondroitin sulfate expression predicts poor outcome in breast cancer. Int. J. Oncol. 39, 1421–1428. doi: 10.3892/ijo.2011.1164
Takahashi, Y., Kuwabara, H., Yoneda, M., Isogai, Z., Tanigawa, N., and Shibayama, Y. (2012). Versican G1 and G3 domains are upregulated and latent transforming growth factor-beta binding protein-4 is downregulated in breast cancer stroma. Breast Cancer 19, 46–53. doi: 10.1007/s12282-011-0264-7
Thelin, M. A., Bartolini, B., Axelsson, J., Gustafsson, R., Tykesson, E., Pera, E., et al. (2013). Biological functions of iduronic acid in chondroitin/dermatan sulfate. FEBS J. 280, 2431–2446. doi: 10.1111/febs.12214
Theocharis, A. D., Skandalis, S. S., Neill, T., Multhaupt, H. A., Hubo, M., Frey, H., et al. (2015). Insights into the key roles of proteoglycans in breast cancer biology and translational medicine. Biochim. Biophys. Acta 1855, 276–300. doi: 10.1016/j.bbcan.2015.03.006
Theocharis, A. D., Tsara, M. E., Papageorgacopoulou, N., Karavias, D. D., and Theocharis, D. A. (2000). Pancreatic carcinoma is characterized by elevated content of hyaluronan and chondroitin sulfate with altered disaccharide composition. Biochim. Biophys. Acta 1502, 201–206. doi: 10.1016/s0925-4439(00)00051-x
Uyama, T., Kitagawa, H., Tamura, J., and Sugahara, K. (2002). Molecular cloning and expression of human chondroitin N-acetylgalactosaminyltransferase: the key enzyme for chain initiation and elongation of chondroitin/dermatan sulfate on the protein linkage region tetrasaccharide shared by heparin/heparan sulfate. J. Biol. Chem. 277, 8841–8846. doi: 10.1074/jbc.M111434200
Uyama, T., Kitagawa, H., Tanaka, J., Tamura, J., Ogawa, T., and Sugahara, K. (2003). Molecular cloning and expression of a second chondroitin N-acetylgalactosaminyltransferase involved in the initiation and elongation of chondroitin/dermatan sulfate. J. Biol. Chem. 278, 3072–3078. doi: 10.1074/jbc.M209446200
Venkatachalam, K. (2003). Human 3′-phosphoadenosine 5′-phosphosulfate (PAPS) synthase: biochemistry, molecular biology and genetic deficiency. IUBMB Life 55, 1–11. doi: 10.1080/1521654031000072148
Vitale, D., Kumar Katakam, S., Greve, B., Jang, B., Oh, E. S., Alaniz, L., et al. (2019). Proteoglycans and glycosaminoglycans as regulators of cancer stem cell function and therapeutic resistance. FEBS J. 286, 2870–2882. doi: 10.1111/febs.14967
von Spreckelsen, N., Fadzen, C. M., Hartrampf, N., Ghotmi, Y., Wolfe, J. M., Dubey, S., et al. (2021). Targeting glioblastoma using a novel peptide specific to a deglycosylated isoform of brevican. Adv. Ther. 4:2000244. doi: 10.1002/adtp.202000244
Wade, A., Robinson, A. E., Engler, J. R., Petritsch, C., James, C. D., and Phillips, J. J. (2013). Proteoglycans and their roles in brain cancer. FEBS J. 280, 2399–2417. doi: 10.1111/febs.12109
Wagner, G. P., Kin, K., and Lynch, V. J. (2012). Measurement of mRNA abundance using RNA-seq data: RPKM measure is inconsistent among samples. Theory Biosci. 131, 281–285. doi: 10.1007/s12064-012-0162-3
Weber, C. K., Sommer, G., Michl, P., Fensterer, H., Weimer, M., Gansauge, F., et al. (2001). Biglycan is overexpressed in pancreatic cancer and induces G1-arrest in pancreatic cancer cell lines. Gastroenterology 121, 657–667. doi: 10.1053/gast.2001.27222
Wei, J., Hu, M., Huang, K., Lin, S., and Du, H. (2020). Roles of proteoglycans and glycosaminoglycans in cancer development and progression. Int. J. Mol. Sci. 21:5983. doi: 10.3390/ijms21175983
Weiss, R. J., Spahn, P. N., Chiang, A. W. T., Liu, Q., Li, J., Hamill, K. M., et al. (2021). Genome-wide screens uncover KDM2B as a modifier of protein binding to heparan sulfate. Nat. Chem. Biol. 17, 684–692. doi: 10.1038/s41589-021-00776-9
Weiss, R. J., Spahn, P. N., Toledo, A. G., Chiang, A. W. T., Kellman, B. P., Li, J., et al. (2020). ZNF263 is a transcriptional regulator of heparin and heparan sulfate biosynthesis. Proc. Natl. Acad. Sci. U.S.A. 117, 9311–9317. doi: 10.1073/pnas.1920880117
Wu, Y., Huang, H., Fervers, B., and Lu, L. (2020). Syndecan-1 and KRAS gene expression signature associates with patient survival in pancreatic cancer. Pancreas 49, 1187–1194. doi: 10.1097/MPA.0000000000001654
Xu, D., and Esko, J. D. (2014). Demystifying heparan sulfate–protein interactions. Annu. Rev. Biochem. 83, 129–157. doi: 10.1146/annurev-biochem-060713-035314
Xu, N., Wu, Y. P., Yin, H. B., Xue, X. Y., and Gou, X. (2018). Molecular network-based identification of competing endogenous RNAs and mRNA signatures that predict survival in prostate cancer. J. Transl. Med. 16:274. doi: 10.1186/s12967-018-1637-x
Keywords: dermatan sulfate (DS), chondroitin sulfate (CS), glycosaminoglycan (GAG), GlycoMaple, heparan sulfate (HS), hyaluronan (HA), keratan sulfate (KS), proteoglycan (PG)
Citation: Huang Y-F, Mizumoto S and Fujita M (2021) Novel Insight Into Glycosaminoglycan Biosynthesis Based on Gene Expression Profiles. Front. Cell Dev. Biol. 9:709018. doi: 10.3389/fcell.2021.709018
Received: 13 May 2021; Accepted: 18 August 2021;
Published: 06 September 2021.
Edited by:
Ayano Satoh, Okayama University, JapanReviewed by:
Kristian Prydz, University of Oslo, NorwayNikos Karamanos, University of Patras, Greece
Copyright © 2021 Huang, Mizumoto and Fujita. This is an open-access article distributed under the terms of the Creative Commons Attribution License (CC BY). The use, distribution or reproduction in other forums is permitted, provided the original author(s) and the copyright owner(s) are credited and that the original publication in this journal is cited, in accordance with accepted academic practice. No use, distribution or reproduction is permitted which does not comply with these terms.
*Correspondence: Morihisa Fujita, ZnVqaXRhQGppYW5nbmFuLmVkdS5jbg==