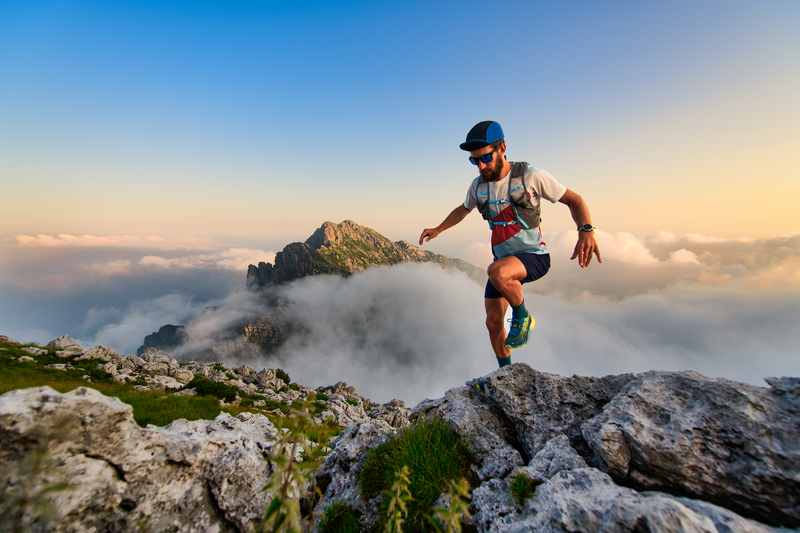
94% of researchers rate our articles as excellent or good
Learn more about the work of our research integrity team to safeguard the quality of each article we publish.
Find out more
REVIEW article
Front. Cell Dev. Biol. , 14 July 2021
Sec. Molecular and Cellular Oncology
Volume 9 - 2021 | https://doi.org/10.3389/fcell.2021.707198
T lymphocytes function as major players in antigen-mediated cytotoxicity and have become powerful tools for exploiting the immune system in tumor elimination. Several types of T cell-based immunotherapies have been prescribed to cancer patients with durable immunological response. Such strategies include immune checkpoint inhibitors, adoptive T cell therapy, cancer vaccines, oncolytic virus, and modulatory cytokines. However, the majority of cancer patients still failed to take the advantage of these kinds of treatments. Currently, extensive attempts are being made to uncover the potential mechanism of immunotherapy resistance, and myeloid-derived suppressor cells (MDSCs) have been identified as one of vital interpretable factors. Here, we discuss the immunosuppressive mechanism of MDSCs and their contributions to failures of T cell-based immunotherapy. Additionally, we summarize combination therapies to ameliorate the efficacy of T cell-based immunotherapy.
Immunotherapy intends to motivate immune cells to fight against tumor cells, instead of targeting tumor cells directly. The main strategies for immunotherapy include strengthening the cytotoxicity of T lymphocytes and eliminating immunosuppression induced by immune suppressive cells or factors. Notably, T cells play irreplaceable roles in the treating process for their participation in recognition of tumor antigens and final combat with tumor cells (Waldman et al., 2020). In addition, it was interpreted that T cells enrichment in tumor sites was associated with favorable responses in immunotherapy (Bruni et al., 2020). Based on this concept, multiple categories of T cell-based immunotherapy are developed and prospered.
Currently, significant strides have been made in T cell-based immunotherapy for cancer treatment since ipilimumab, an anti-cytotoxic T lymphocyte antigen-4 (CTLA-4) antibody, was approved by FDA for use in melanoma treatment. Subsequently, several categories of T cell-based immunotherapy were under active investigations or came into clinical practice (Emens et al., 2017). Such strategies are included: (i) blocking T cells suppressive signals with immune checkpoint inhibitors (ICIs) to emancipate existing T cells; (ii) reinforcing cytotoxic T lymphocytes (CTLs) through adoptive T cell therapy (ACT), such as the administration of tumor-infiltrating lymphocytes (TILs), T cell receptor-modified T (TCR-T) cells and chimeric antigen receptor T (CAR-T) cells; (iii) inducing or generating the release of tumor antigen to stimulate endogenous T lymphocytes by the application of oncolytic virus and cancer vaccines; (iv) potentiating the function of T cells by systematic administration of positive modulatory cytokines, such as interleukin (IL) and interferon (IFN). However, a number of patients quickly relapse after the initial response or fail to show any regression. Only a minority of patients benefit from these therapies (Li X. et al., 2018; Pérez-Ruiz et al., 2020). The good response cancer types include melanoma (Robert et al., 2015; Wolchok et al., 2017) and non-small cell lung cancer (NSCLC) (Antonia et al., 2017; Socinski et al., 2018). Extensive research is currently undergoing to identify determinants of resistance to obtain a superior efficacy in immunotherapy. Various factors are found to be related to the outcome of T cell-based immunotherapy: tumor mutation burden (TMB); antigen-presenting capacity; immunological characteristics of the tumor microenvironment (TME), including infiltration of effector tumor-killing cells and their counterbalance against immunosuppressive network; genetic and epigenetic alterations. In this review, we will pay emphasis on the pillar of immunosuppressive network, myeloid-derived suppressor cells (MDSCs) which often hijack T lymphocytes and abandon their cytotoxic effect on tumor cells (Colligan et al., 2020).
Myeloid-derived suppressor cell is a staple of the immune profile in the TME, which is crucial in cancer genesis, progression, and reaction to therapy (Kumar et al., 2016; Ostrand-Rosenberg and Fenselau, 2018; Tcyganov et al., 2018). In the earliest stage of tumorigenesis, cancer cells always succeed in evading immune surveillance. Once established, tumor cells release a battery of signals that induce the recruitment of immune cells from bone marrow, including MDSCs, resulting in a tumor-promoting milieu. Such is an elegant theory of immunoediting conceptualized by Robert Schreiber, whereby malignant cells, which evolve under selective pressure from immune system, gain the capability of escaping immune recognition and taking advantages of immune system in reverse (Desai et al., 2021). Moreover, therapeutic interventions, including chemotherapy, radiotherapy, and immunotherapy can simultaneously have an effect on the whole process of the TME remodeling. Apart from the direct inhibition of T cells, MDSCs can also foster the genesis and maturation of regulatory T cells (Tregs) (Veglia et al., 2018), tumor-associated macrophages (TAMs) (Ugel et al., 2015) and cancer-associated fibroblasts (CAFs) (Alkasalias et al., 2018), generating a immunosuppressive network (Tesi, 2019). Although a considerable body of original articles and reviews focus on MDSCs, few of them pay attention to their dynamic changes and the influence on T cell-based immunotherapy. Therefore, the present review attempts to figure out the signaling crosstalk to gain a comprehensive understanding of how MDSCs become immunosuppressive and how they counteract the efficacy of T cell-based immunotherapy.
The past 10 years of clinical immunotherapy practice has engendered a deep comprehension of cancer treatment. T cells have become the central focus of arming the immune system to fight against cancer. So far, several types of T cell-based immunotherapies have been applied in clinical practice (Table 1) and nearly all the strategies augment the number of cytotoxic T cells by motivating endogenous T cells or reinfusing externally expanded T cells. A succession of biological elements needs to be fulfilled to achieve successful immunotherapy (Smyth et al., 2016). These elements include immunogenic cancer cell death, efficient antigen presentation or adjuvanticity, and active effector T cells. Notably, absence or low infiltration of cytotoxic lymphocytes was shown to be a critical inadequacy that resulted in non-response to T cell-based immunotherapy (Havel et al., 2019). Theoretically, T cell-based immunotherapies were originally designed to optimize the solution from these aspects. However, only a minority of patients experienced improved survival from these therapies. Numerous studies have been conducted to identify the barriers to a favorable response to T cell-based immunotherapy and multiple challenges have been defined.
Tumor immune phenotype has been identified to forecast the efficiency of immunological tumor elimination. Tumor immune classification has been proposed in recent years, and its potential value in immunotherapy was discussed in the literature. Elaborative evaluation of tumor-infiltrated lymphocytes helps to identify three predominant immune phenotypes: immune-inflamed, immune-excluded, and immune-desert (Ochoa de Olza et al., 2020). Favorable response of T cell-based immunotherapy always occurs in immune-inflamed tumors (Chen and Mellman, 2017). However, in immune-excluded and immune-desert tumors, also known as cold tumors, CD8+ T cells are absent or prevented from effective infiltration. Naturally, the outcome of T cell-based immunotherapy in these tumors is frustrated owing to the lack of contact-dependent cytotoxicity. Biomarkers that are correlated with tumor immune phenotype include programmed death ligand-1 (PD-L1) expression, IFN-γ signature, B cells abundance, and genetic instability, which can be used as predictors in immunotherapy (Kowanetz et al., 2018; Griss et al., 2019).
Heterogeneity of tumor cells reveals the complexity of cancer T cell-based immunotherapy. Malignant tumor is the heterogeneous product of a plethora of genetic alterations. Somatic mutation can produce tumor-specific pipetides which can be recognized by immune system as neoantigens. Also, particular molecular mutations drive the development of targeted therapies with favorable clinical response. However, tumors are as sly as foxes, always utilizing genetic alterations to escape from elimination when targeted. Therefore, although T cell-based immunotherapy has demonstrated active response, the majority of patients will eventually develop resistance to these kinds of therapies. For instance, targeting programmed death-1 (PD-1)/PD-L1 pathway triggered the upregulation of alterative immune checkpoints, such as lymphocyte-activation gene 3 (LAG3) and T cell immunoglobulin domain and mucin domain-3 (TIM-3), resulting in adaptive resistance (Koyama et al., 2016). Usually, high TMB generates antigenicity and activates immune system, making tumors inflamed. High TMB should be a positive biomarker of T cell-based immunotherapy (Samstein et al., 2019). However, recent studies did not value TMB as an effective predictor in PD-1 and CTLA-4 dual blockade therapeutic strategies (Peters et al., 2019a, b). Heterogeneity of tumor cells resulted from persistent bulky mutations may account for the incompetence of TMB in predicting benefit of immunotherapy.
Additionally, negatively regulatory networks can shut down T cell-based immune response. The TME is a dynamic environment composed of the extracellular matrix, blood vessels, signaling molecules, immune components, and malignant cells. Adding to its complicity, treatment-induced biochemical events bring about a confluence of changes. Every immune process could exert influence over the treatment outcome. Monotherapies merely manipulating T cells seem powerless to work out the major impediment that attenuates antitumor immunity. With deeper understanding of the TME and summary on clinical practice, it is consensus that the other therapeutic node, removing immune suppression, cannot be neglected (Smyth et al., 2016; Tesi, 2019).
Among the negatively regulatory networks, cellular components include Tregs, TAMs, CAFs, and MDSCs. They secrete a plethora of cytokines and chemokines, providing hotbed and protector for cancer cells. Notably, MDSCs are acknowledged to directly compromise T cells function, hence their name. Accumulating evidence has suggested the vital role of MDSCs in facilitating an immunosuppressive TME in various cancer types (Parker et al., 2015; Kumar et al., 2016). Numerous immunosuppressive mechanisms have been identified, including soluble mediators, metabolic interactions, and cell-to-cell contact.
Myeloid-derived suppressor cells are a diversified clump of immature myeloid cells with strong immunosuppressive functions. Generally, most MDSCs could fall into two categories, namely: monocytic-MDSCs (M-MDSCs) and polymorphonuclear-MDSCs (PMN-MDSCs, also known as granulocytic-MDSCs, G-MDSCs). In humans, scientists also defined a more immature MDSC as early-stage MDSC (e-MDSC), lacking surface markers for both M-MDSC (CD14+) and PMN-MDSC (CD15+) (Bronte et al., 2016). Under homeostatic conditions, hematopoietic stem cells can polarize towards mature granulocytes, monocytes or dendritic cells (DCs) through common myeloid progenitors (CMPs) and granulocyte-monocyte progenitors. Nevertheless, under pathologic conditions such as chronic infection, cancer or immune-related disease, granulopoiesis is compromised, diverting the mainstay differentiation towards MDSCs (Mackey et al., 2019). Recently, conversion of neutrophils and monocytes to MDSCs has been reported. In particular, CD14+ myeloid cells isolated from melanoma cells have been demonstrated to develop a suppressive activity on T cells when disposed with extracellular vesicles (EVs), thus referred to as EV-MDSCs. Similarly, when exposed to exosomes obtained from chronic lymphocytic leukemia (CLL) cells, monocytes derived from healthy donors displayed the functional characteristics of MDSCs (Bruns et al., 2017). However, Bronte et al. (2016) suggested that human MDSCs generated in vitro should be defined as “MDSC-like” cells. A separate team also identified a monocytic lineage as the precursor of PMN-MDSCs and termed these cells monocyte-like precursors of granulocytes (Mastio et al., 2019). Intriguingly, selective inhibition of monocytic cells had little impact on the genesis of granulocytes in normal mice although it diminished the aggregation of PMN-MDSCs by 50% in tumor-bearing mice. All these findings showed that MDSCs are generated in a complicated hematopoietic network, in which multiple hematopoietic cells have the potential to differentiate into to MDSCs and this process is stringently regulated by factors derived from the TME.
The MDSCs expansion and activation are controlled by multiple TME-derived molecules via several transcriptional pathways. Growth factors such as the stem cell factor (SCF), granulocyte colony-stimulating factor, macrophage colony-stimulating factor, and granulocyte-macrophage colony-stimulating factor (GM-CSF), stimulate myelopoiesis and expansion of MDSCs through JAKs-STAT signaling pathways (Kumar et al., 2016). Among several STAT signaling pathways, STAT3 was the most widely studied and was shown to promote MDSCs proliferation by regulating the expression of proliferation-related genes and apoptosis-related genes, such as bcl-xl, cyclin d, and c-myc (Wang et al., 2020). STAT3 was also reported to induce the production of immunosuppressive factors such as arginase 1 (ARG1) and reactive oxygen spices (ROS) (Wang et al., 2020). In addition, inflammation is another factor affecting the modulation of MDSCs. Inflammation is considered as a companion of cancer and is well involved in nearly all stages of cancer development and the TME sculpture (Greten and Grivennikov, 2019). In the inflammatory milieu, MDSCs are generated to prevent the immune system from overreacting. While get into a cancer-related inflammatory milieu, MDSCs are quickly tamed by cancer cells and transform into a tumor-protective phenotype (Bronte et al., 2016). Inflammatory cytokines, including S100A8/9, adenosine, IFN-γ and IL-6 are responsible for the remodeling of MDSCs by activating toll-like receptor (TLR) signaling pathway (Wang et al., 2020). Furthermore, transforming growth factor-β (TGF-β) and IL-1β were reported to restrain the transcription of human leucocyte antigen-DR (HLA-DR) in monocytes by dampening major histocompatibility complex class II (MHC II) transactivator, converting them to CD14+HLA-DRlo/neg M-MDSCs (Mengos et al., 2019). Additionally, the CCAAT/enhancer binding protein-α (C/EBPα) is an extensively engaged transcription factor that is also imperative for the function of MDSCs (Thevenot et al., 2014). C/EBPα drives the expansion of MDSCs through a cell-surface molecule called the retinoic acid-related orphan receptor C1, during cancer-related inflammation (Strauss et al., 2015). Moreover, the C/EBP homologous protein is an apoptosis-related transcription factor which is stimulated by endoplasmic reticulum (ER) stress and is instrumental in the activation of the IL-6/STAT3 pathway in MDSCs. On the contrary, interferon regulatory factor-8 (IRF8) negatively regulates of the generation of MDSCs, on the account that transgenic overexpression of IRF8 diminished the aggregation of MDSCs and mice with low expression of IRF8 were enriched with MDSC-like cells (Valanparambil et al., 2017).
Additionally, ER stress has emerged as a key mechanism regulating the pathologic activities of MDSCs. MDSCs from tumor-bearing hosts exhibit a greater ER stress response than monocytes or neutrophils from tumor-free hosts (Condamine et al., 2014). Neutrophils derived from healthy donors develop a suppressive capacity when exposed to inducers of ER stress (Condamine et al., 2014). These experimentally induced cells expressed high levels of ARG1, NOS2, and NAPDH oxidase-2 (NOX-2), which are closely associated with immunosuppression. Moreover, ER stress regulates the lifespan of MDSCs, favoring their apoptosis in peripheral tissues and promoting their expansion in bone marrow (Condamine et al., 2014). An additional study showed that thapsigargin-induced ER stress upregulated the level of the lectin-type oxidized LDL receptor 1 (LOX-1) in human neutrophils and promoted their conversion to the immunosuppressive phenotype, which can be interrupted by blocking the inositol-requiring enzyme 1-spliced X-box binding protein-1 pathway (Condamine et al., 2016). Nonetheless, it is still unclear whether LOX-1 is important in the downstream signal transduction to prompt neutrophils to acquire a suppressive potential.
The accumulation of MDSCs in the tumors sites is regulated by chemokines generated from the TME. C-C motif chemokine ligand 2 (CCL2, also called MCP-1) and CCL5 (also called RANTES) are the main chemotactic factors implicated in the migration of M-MDSCs into tumors (Iwamoto et al., 2020). Increased expression of CCL2 is an indicator of the progression of human colorectal cancer while inhibiting CCL2 was reported to reduce the recruitment of MDSCs in colorectal cancer model (Chun et al., 2015). Similarly, PMN-MDSCs are mobilized following the chemotaxis of CXC motif chemokines, including CXC motif chemokine ligand 1 (CXCL1), CXCL2, CXCL5, CXCL8, and CXCL12 (Kumar et al., 2016; Najjar et al., 2017; Li Y.M. et al., 2019). S100A8 and S100A9 proteins are also among the critical driving force in MDSCs recruitment (Song and Struhl, 2021). Notably, PMN-MDSCs are also the sources of S100 proteins, creating a positive feedback loop of MDSCs aggregation (Kowanetz et al., 2010). Evidently, the recruitment of MDSCs involves complicated mechanisms and it occurs according to the type and stage of tumors.
If the immune cells within the TME were divided into two opposing fronts, T cells would be considered as the leaders of the friendly side while MDSCs occupy critical position in the pernicious foe (Tesi, 2019). MDSCs deliver suppression on cytotoxic lymphocytes and innate immune cells. Additionally, they exert their effects on other inhibitory cell populations, forming a powerful immunosuppressive landscape and inhibiting the tumoricidal immune system.
During the battle against cancer cells, Tregs and type 2 macrophages betrayed the T cells camp and became accomplices of the MDSCs. When co-incubated with M-MDSCs separated from pancreatic ductal adenocarcinoma, autologous CD4+ T cells could potentiate their differentiation to Foxp3+ Tregs (Siret et al., 2020). Th17 cells were also revealed as a source of Foxp3+ Tregs under the induction of CD14+HLA-DR–/low M-MDSCs via TGF-β and retinoic acid (Hoechst et al., 2011). Furthermore, MDSCs-derived molecules, such as CCL3, CCL4, and CCL5, facilitate the recruitment of CCR5+ Tregs in a mouse model of melanoma (Schlecker et al., 2012). Moreover, a recent preclinical study demonstrated the MDSCs-mediated induction of Tregs occurred in a cell contact manner (Siret et al., 2020). In parallel with mobilization of Tregs, MDSCs can also convert macrophages to type 2 phenotype through releasing increased level of IL-10, thereby promoting tumor progression (Zefferino et al., 2021). More importantly, it was shown that M-MDSCs could directly differentiate into TAMs in a hypoxia inducible factor-1α (HIF-1α) dependent manner (Zefferino et al., 2021). Th17 cells are another group of immunosuppressive cells characterized by the production of IL-17, which cripple T lymphocyte cytotoxicity. MDSCs induce inducible nitric oxide synthase (iNOS) production in T cells, favoring Th17 cells proliferation (Jayakumar and Bothwell, 2019; Dar et al., 2020). Conversely, Tregs were also observed to contribute to the development of MDSCs. Lee et al. (2016) showed that Tregs facilitated the proliferation of MDSCs through the secretion of TGF-β in a colitis model. The study also reported that reduced secretion of TGF-β impaired the expression of ARG1, iNOS, and PD-L1 in M-MDSCs. In a mouse ret melanoma model, Tregs from the tumor site not only inhibited T cells directly but also upregulated PD-L1 expression in MDSCs. Correspondingly, deletion of Tregs using CD25-specific antibodies downregulated the expression of PD-L1, suggesting an interlaced relationship between MDSCs and Tregs (Fujimura et al., 2012). Overall, these findings verified the presence of a powerful negative regulatory network comprising of MDSCs and other inhibitory immune cells, conspiring to abrogate T cell-based antitumor immune response and promote tumor progression (Figure 1).
Figure 1. MDSCs-mediated suppression in T cells. (A) In lymph node, MDSCs promote Treg differentiation and increase FOXP3 expression via TGF-β. T cell proliferation was inhibited due to the increased secretion of reactive oxygen and nitrogen spices. (B) MDSCs inhibit T cell homing by disrupting L-selectin (CD62L) structure and hindering the function of CD44 and CD162, essential in T cell homing. (C) In tumor sites, MDSCs inhibit T cells via immune checkpoint blockade, metabolism deprivation, and suppressive molecules. Moreover, MDSCs induce macrophage differentiation into type 2 macrophage (M2 macrophage). Treg, regulatory T cell; IDO, indoleamine 2,3-dioxygenase.
Myeloid-derived suppressor cells are major sources of reactive oxygen and nitrogen spices in the TME, which are harmful to most cell types including T cells. The production of ROS by NOX-2 during respiratory burst, such as hydrogen peroxide (H2O2), superoxide anions and hydroxyl radicals, was found to be closely related to the eradication of TILs. ROS inhibited T-cell immune responses by restricting interactions between TCR and MHC, whereas ROS inhibitors could reverse the suppressive effect of MDSCs on T cells (Liu et al., 2015). Moreover, elevated ROS levels suppressed their differentiation to mature myeloid cells, enhancing the recruitment of MDSCs and forming a signaling cascade (Adeshakin et al., 2021). Intriguingly, MDSCs were demonstrated to salvage the harmful effect of ROS by expressing the nuclear factor erythroid 2-related factor 2 (Nrf2), an transcription factor mediating cellular antioxidant response (Beury et al., 2016). Another cytotoxic oxynitrite is nitric oxide (NO), which is produced through the catalysis of iNOS. NO was declared to suppress proliferation of T lymphocytes and even prompt their apoptosis. Moreover, its synthesis products with the superoxide anion, peroxynitrite, should be responsible for non-responsiveness of CTLs.
Furthermore, MDSCs deprive T cells of several nutrients such as L-arginine and cysteine which are essential for their metabolism and function (Geiger et al., 2016). Cationic amino-acid transporter-2B works as a mediator in the transportation of L-arginine from the extracellular space into the intracellular space of MDSCs (Cimen Bozkus et al., 2015). A previous study reported that when L-arginine was exhausted by MDSCs, nitration of TCRs and T-cell apoptosis were enhanced through the increased production of IL-10 and prostaglandin E2 (PGE2), leading to impairment T cells activation (Miret et al., 2019). When gathered in MDSCs, L-arginine serves as a substrate in a cascade of synthetic reactions, contributing the immunosuppressive function of MDSCs (Grzywa et al., 2020). For instance, arginases (ARG1 and ARG2) deal with the catabolism of L-arginine to urea and L-ornithine. L-ornithine can further convert to L-proline which is an important immunosuppressive polyamine (Grzywa et al., 2020). Moreover, activated T lymphocytes, together with several Th2 cell-secreted cytokines, including IL-4, IL-5, IL-10, and IL-13 can increase the expression of ARG1 (Grzywa et al., 2020). Upregulation of ARG1 in MDSCs not only trigger T cells inhibition but also promotes extracellular matrix remodeling, favoring tumor growth (Grzywa et al., 2020). However, it was recently demonstrated that the expression of ARG1 is not compulsory in MDSCs-mediated immunosuppression (Bian et al., 2018). The study however emphasized on the importance of cell-to-cell contact in T cells inhibition. Cysteine is another essential amino acid in T cells that was also reported to be deleted by MDSCs, leading to the inhibition of T-cell function (Srivastava et al., 2010). It was shown that depletion of L-arginine and cysteine reduced the synthesis of glutathione (GSH), which could prevent the production of ROS (Srivastava et al., 2010). In addition to amino acid metabolism, alteration of glycolysis and lipid metabolism contributes to MDSCs inhibitory capability, resulting in T cells suppression and tumor progression (Wang et al., 2020). The increased production of lactate augmented the number of MDSCs and fatty acid transport protein 2 was reported to be overexpressed in PMN-MDSCs, which could exert suppressive function by means of arachidonic acid uptake and synthesis of PGE2.
Immunosuppressive mediators are also utilized by MDSCs to induce T cells inhibition. In cancer-bearing hosts, adenosine is excessively produced in the TME. CD39 and CD73 are two critical ectoenzymes that catalyze the generation of adenosine and are mainly expressed on immunosuppressive cells. MDSCs separated from the peripheral blood or tumors of NSCLC patients were reported to express increased levels of CD39 and CD73, under the stimulation of TGF-β in a HIF-1α-dependent way, hence resulting to excessive production of adenosine (Li et al., 2017). Additionally, adenosine was proven to inhibit naïve T cells initiation by blocking the phosphorylation of extracellular regulated protein kinases (ERK), protein kinase B (PKB/Akt) and the zeta-chain-associated protein 70 (Zap70) (Mastelic-Gavillet et al., 2019). The function of activated T cells was also compromised by adenosine, which attenuated the expression of several effector molecules such as CD95L, perforin, IFN-γ, and tumor necrosis factor-α (TNF-α) (Mastelic-Gavillet et al., 2019). Apart from high levels of adenosine, other immunosuppressive cytokines (such as IL-10 and TGF-β) from MDSCs also elicit T cells inhibition. For instance, IL-10 inhibits antigen presentation by impairing the expression of MHC, co-stimulatory signals and cytokines secretion in antigen presenting cells (APCs), hence indirectly suppressing T cells response (Payne et al., 2013). Furthermore, TAMs, TILs, as well as cancer cells are also the source of IL-10 and TGF-β, amplifying their tumor promoting and tumor suppressing activity (Stewart and Smyth, 2011). Therefore, successful treatment requires more specific identification of tumor immune signatures when targeting IL-10 and TGF-β. In addition, PGE2 and indoleamine 2,3-dioxygenase (IDO) were also involved in MDSCs-induced immunosuppression. In a genesis assay of MDSCs, PGE2 was shown to induce the expression of multiple immunosuppressive makers on MDSCs, including IDO, IL-10, and ARG1 (Obermajer and Kalinski, 2012).
Moreover, immune checkpoint molecules were identified to be strong mediators of MDSCs-induced immunosuppression. PD-1/PD-L1 is the mostly studied signal. The binding of PD-L1 on MDSCs and PD-1 on T cells induces T cells anergy and apoptosis (Groth et al., 2019). Moreover, other immune checkpoints are also involved in MDSCs-induced suppression on T cells, including CTLA-4, LAG-3, and TIM-3. These several immune checkpoints complement each other. For instance, the TIM-3/Gal-9 pathway is a critical mechanism for primary or secondary resistance to anti-PD-1 treatment in metastatic NSCLC patients (Limagne et al., 2019). In addition, in patients with high MDSCs levels, ICIs have poorer clinical response. So, whether ICIs could interrupt immune checkpoint-mediated connections between MDSCs and T cells need further investigation.
In addition to the aforementioned mechanisms, MDSCs also impair lymphocytes homing by destroying the structure of CD62L (L-selectin), a lymph node homing receptor, through a disintegrin and metalloprotease 17 (ADAM17) (Ku et al., 2016). The loss of L-selectin was also proved to be correlated with inactivation of CD8+ T cells in lymph nodes (Ku et al., 2016). Further, M-MDSCs could destroy the structure of trafficking-related molecules on T cells in a NO dependent manner, including CD44 and CD162 (Schouppe et al., 2013). CD44 mediates the connection of T cells with extracellular matrix component hyaluronic acid and CD162 is a ligand of selectin P. The destroy of CD44 and CD162 impaired the extravasation and the infiltration of T cells. MDSCs set the myriad of hurdles in T cells trafficking way.
Most studies of immunotherapy concentrated on conventional CD4+ and CD8+ lymphocytes, but unconventional T cells may offer advantages to T cells immunotherapy in cancer treatment, which are also susceptible to MDSCs. The unconventional T cells subsets include natural killer T (NKT) cells, mucosal-associated invariant T (MAIT) cells and γδ T cells (Pellicci et al., 2020). These cells account for approximate 10% of T cells in circulation and a dominant percentage in tissues such as gut mucosa. Conventional T cells functions in a MHC I or MHC II dependent manner, while unconventional T cells interact with MHC class Ib or MHC I like molecules (Godfrey et al., 2018). Activation of type I NKT cells was shown to stimulate the motivation of T cells and NK cells, while the behavior of type II NKT cells was drastically different (Vivier et al., 2012). At the presence of α-GalCer, type I NKT cells could convert MDSCs into stimulatory APCs by producing increased levels of IL-12, IFN-γ, and TNF (Ko et al., 2009). In turn, Zhang et al. (2017) reported that MDSCs could selectively inhibit the production of IFN-γ of NKT cells through membrane-bound TGF-β. However, other two studies revealed that NKT cells were resistant to immunosuppressive effects of MDSCs (Gebremeskel et al., 2015; Horinaka et al., 2016). The quite different observations may be caused by discrepant identifying markers. More sophisticated design is needed to achieve an accurate assessment. γδ T cells also have dual effects on tumors, which is dependent on the characteristics of TME (Li L. et al., 2019). Under normoxic environment, tumor-derived exosomes stimulate the cytotoxic activity of γδ T cells (Li L. et al., 2019). However, oxygen pressure could alter the content of tumor-derived exosomes, which subsequently induced the suppression of MDSCs on γδ T cells (Li L. et al., 2019). Additionally, a subset of Vγ4 γδ T cells are the producer of IL-17, an immune inhibitory cytokine. This subset of Vγ4 γδ T cells enhance the suppressive activity of MDSCs, forming a positive feedback (Ma et al., 2014). The diversity of unconditional T cells and limited identification strategies pose challenge to clearer understanding of their biological characteristics and targeted strategies.
Apart from direct T-cell suppression, MDSCs also exert strong regulatory effects on other tumor inhibitory immune cells, such as NK cells and DCs, hence generating a formidable immunosuppressive force (Ostrand-Rosenberg et al., 2012; Bruno et al., 2019). M-MDSCs obtained from melanoma patients inhibited the cytotoxicity of NK cells through the production of TGF-β (Mao et al., 2014). The same study showed that monocytes treated with PGE2 were activated via the p38MAPK/ERK pathway and consequently secreted elevated levels of TGF-β, resulting in the potent suppression of NK cell activity in vitro. In another study, both CD14+ MDSCs and CD15+ MDSCs from the tumors of head and neck squamous cell carcinoma (HNSCC) patients suppressed NK cells activation (Greene et al., 2020). Moreover, the immunosuppressive ability of CD14+ MDSCs was reversed using TGF-β monoclonal antibodies (mAb) while CD15+ MDSCs were only sensitive to the NOS inhibitor, L-NMMA. In addition to soluble factors, Fc receptors expressed on NK cells were also reported to be critical in MDSCs-induced suppression (Stiff et al., 2018).
Increasing evidence suggests that MDSCs are associated with disrupting the accumulation and function of DCs, although the underlying mechanism is yet to be elucidated. Poschke et al. (2012) demonstrated that purified M-MDSCs extracted from melanoma patients negatively regulated the maturation, trafficking, cytokine production and antigen presentation of DCs. Additionally, in hepatocellular carcinoma model, MDSCs suppressed the capability of DCs to stimulate T lymphocytes and inhibited IL-12 production in DCs through increased IL-10 secretion (Hu et al., 2011). A recent study also demonstrated that MDSCs hindered DCs-mediated antigen presentation, which was dependent on NO-induced STAT1 nitration, and this effect could be reversed by iNOS inhibitors (Markowitz et al., 2017). Moreover, a previous study reported that MDSCs suppressed the generation and antigen presentation of DCs. It also showed that both Notch and STAT3 signaling were required in MDSCs-induced suppression (Wang et al., 2016).
Additionally, MDSCs are involved in B cell-mediated immune responses. A study reported that MDSCs impeded B cells proliferation in vitro through the increased secretion of ARG1 (Wang et al., 2018). After deletion of MDSCs using anti-Gr-1 antibodies, there was an increase in the production of both IL-7 (a B cell stimulator) and B cell-derived IgG, indicating that B cells suffer from MDSCs-induced inhibition. Similar to T cells, Ku et al. (2016) announced that MDSCs also reduced L-selectin levels on B cells through the cell-to-cell contact mechanism, hence disrupting the homing of B cells. Furthermore, it was shown that MDSCs upregulated the expression of PD-L1 and IL-10 on IgA+ B cells via the TNF receptor 2 (Xu et al., 2017). Moreover, MDSCs act as inducers of regulatory B cells, a cluster of immunosuppressive B cells, by delivering IL-10 and TGF-β (Jayakumar and Bothwell, 2019).
In addition to their immunosuppressive capabilities, MDSCs also positively contribute to the immune-independent progression of tumors. They promote the expansion of tumor cells, epithelial-mesenchymal transition (EMT), and enhance stemness by secreting numerous soluble factors, such as IL-10 and TGF-β (Yang et al., 2004; Bruno et al., 2019). Moreover, MDSCs were shown to facilitate metastasis by secreting metalloproteases, such as matrix metallopeptidase 9 (MMP-9), and contribute to pre-metastasis niche formation (Long et al., 2020). When get into a pre-metastasis niche, MDSCs release a large amount of NO, ARG-1, and immunosuppressive factors, altering T cells and NK cells functions and facilitating the recruitment of TAMs and Tregs (Tang et al., 2021). Additionally, MDSCs promote angiogenesis by secreting MMP9 and VEGF (Tang et al., 2021).
Myeloid-derived suppressor cells have been determined in multiple kinds of tumors, such as melanoma, NSCLC, and prostate cancer. Studies on patients payed more attention to MDSCs in peripheral blood, contrary to preclinical studies which largely focused on tumor-infiltrating MDSCs. MDSCs accumulation in circulation always coincides with an advanced tumor burden, stage, grade, and poor prognosis in various types of cancer (Diaz-Montero et al., 2014). For instance, higher percentages (>11%) of circulating CD14+ M-MDSCs independently predicted the risk of death in stage III/IV melanoma patients (Weide et al., 2014). A similar conclusion was also drawn in patients with NSCLC, pancreatic cancer, bladder cancer, gastric cancer, and hepatocellular carcinoma (Chaib et al., 2020).
The MDSCs abundance in circulation was also verified to be correlated with poorer responses to various types of immunotherapy and they shortened the overall survivals (OS) of patients (Gebhardt et al., 2015; Martens et al., 2016). Meyer et al. (2014) reported that low frequencies of M-MDSCs were related to better clinical efficacy in melanoma patients treated with ipilimumab. This finding was consistent with that of another study which reported that high levels of MDSCs dealt with the absence of antigen-specific T lymphocytes, hence engendering limited efficacy of ipilimumab (Weide et al., 2014). Three more studies also reported that lower levels of circulating MDSCs before treatment could be utilized as a predictive marker of favorable reaction to ipilimumab in melanoma patients (Gebhardt et al., 2015; Martens et al., 2016). A similar pattern was also observed in melanoma patients undergoing anti-PD-1 treatment. Fewer pretreatment MDSCs in peripheral blood were associated with better clinical responses and longer survival of melanoma patients treated with nivolumab (Weber et al., 2016). Furthermore, there was an increase in the levels of LOX-1+ PMN-MDSCs (a specific subset of MDSCs) following anti-PD-1 treatment in NSCLC patients with hypo-responsiveness (Kim et al., 2018). LOX-1 was identified as a promising marker for distinguishing between immunosuppressive PMN-MDSCs and neutrophils (Condamine et al., 2016). The accumulation of LOX-1+ PMN-MDSCs in tumor site was also correlated with shorter disease free survival in glioblastoma patients (Chai et al., 2019). These findings therefore indicate that MDSCs always appear to cripple the beneficial effects of immunotherapy by interfering with the function of T cells.
T cells play irreplaceable roles in immunotherapy. Efficient antigen presentation, sufficient activation and expansion, smooth infiltration, and effective cytotoxicity of T cells are indispensable in successful immunotherapy. Given the mechanism of MDSCs-mediated immunosuppression has been widely studied, their effect on clinical response of T cell-based immunotherapy can never be neglected.
Immune checkpoint inhibitors have gained popularity owing to their efficacy in several malignant tumors (Darvin et al., 2018). The PD-1/PD-L1 axis and the CTLA-4/B7 axis are key targets for ICIs and have been widely applied in clinical practice (Tang et al., 2018; Melaiu et al., 2020). Ipilimumab, the first approved anti-CTLA-4 monoclonal antibody, slightly but significantly increased the OS of stage III/IV melanoma patients compared to standard treatments (Hodi et al., 2010). Thereafter, nivolumab and pembrolizumab were approved by the FDA in 2014. The drugs were shown to have durable responses in 40% of patients and conferred an improved OS, compared to chemotherapy and ipilimumab (Robert et al., 2014, 2015). Following this, anti-PD-L1 antibodies, including atezolizumab, avelumab, and durvalumab along with another anti-PD-1 antibody were gradually approved by the FDA, with extensive indications on several types of cancer (Gulley et al., 2017; Hassan et al., 2019). Unfortunately, trials on ipilimumab in several cancer types, such as NSCLC, SCLC, renal cell carcinoma, and prostate cancer did not provide results as satisfactory as those seen in patients with melanoma (Lynch et al., 2012; Reck et al., 2013; Kwon et al., 2014). Additional trials on PD-1/PD-L1 inhibitors, such as atezolizumab, showed little advantage over standard treatment in certain kinds of solid tumors (Powles et al., 2018).
Based upon the success of the PD-1/PD-L1 and CTLA-4/B7 blockade, additional immune checkpoints are extensively being explored inpreclinical and clinical investigations (Andrews et al., 2019). LAG3, TIM-3,T cell immunoreceptor with immunoglobulin and the immunoreceptor tyrosine-based inhibitory motif domain (TIGIT) are alternative inhibitory receptors that dampen T cell functions by binding to their ligands (Anderson et al., 2016). LAG3, expressed on T cells, NK cells, plasmacytoid DCs and B cells, is among the most heavily studied targets other than PD-(L)1 and CTLA-4 (Maruhashi et al., 2020). The binding of LAG3 to its ligand, MHC II, causes the suppression of effector T cells, inhibiting their proliferation and effector activities (Huard et al., 1994). Currently, various agents targeting LAG-3 are in the clinical trial either as monotherapies or supplement of anti-PD-(L)1 treatment, but the efficacy is limited (Hong et al., 2018; Maruhashi et al., 2020). TIM-3 is also expressed on several immune cell types, such as T cells, Tregs, DCs, and NK cells, modulating their functions by binding to numerous ligands (Wolf et al., 2020). For instance, binding to galectin 9 induces T cells death and binding to the carcinoembryonic antigen cell adhesion molecule 1 (CEACAM1) appears to induce immune tolerance. In addition, overexpression of TIM-3 predicts poor outcome in multiple cancer types and anti-TIM-3 was shown to reduce suppression on IFN-γ-producing CD8+ T cells (Fourcade et al., 2010; Lu et al., 2017b; Shayan et al., 2017). Early phase clinical trials on the anti-TIM-3 antibody, did not show active data (Curigliano et al., 2019; Harding et al., 2019). TIGIT is an inhibitory receptor of the immunoglobulin superfamily, participating in the restriction of both adaptive and innate immune responses (Andrews et al., 2019; Chauvin and Zarour, 2020). TIGIT is expressed principally on T-cell subsets and NK cells (Joller et al., 2011). However, previous research showed that a single TIGIT blockade had no real benefit in CT26 tumor models while combination therapy with the PD-1/PD-L1 blockade resulted in complete tumor rejection through augmenting of CD8+ T cells proliferation and function (Johnston et al., 2014). Similar results was observed in patients with advanced solid tumors treated with anti-TIGIT antibody, MK-7684, showing 8 partial responses out of 43 individuals in anti-PD1 combination group (33rd Annual Meeting & Pre-Conference Programs of the Society for Immunotherapy of Cancer (SITC, 2018), 2018). In addition to these inhibitory targets, several other “second generation” immune checkpoints are currently under intense investigation, including the V-domain immunoglobulin suppressor of T cell activation (VISTA), B7-H3 (CD276) as well as the B- and T-lymphocyte attenuator (BTLA, CD272) (Andrews et al., 2019). However, given that nearly all these targets came up recently, the preliminary efficacy and safety of related targeting strategies are not known.
While several studies have reported that ICIs have positive clinical outcomes, most patients with advanced cancers, accounting for a significant proportion of cancer-related deaths, have not experienced substantial cancer regressions or improved survival after the treatments (Topalian et al., 2012; Callahan et al., 2018). Only 20% of unselected NSCLC and 40% of melanoma patients respond to PD-1/PD-L1 blockade (Brahmer et al., 2012; Shukuya and Carbone, 2016). Numerous studies targeting LAG-3, TIM-3, TIGIT, VISTA, B7-H3, and BTLA, mainly in early phase, are carrying out, but to little avail. Combination therapy with a conventional strategy or another immunotherapy could be an effective method (Gandhi et al., 2018). However, it is difficult to obtain substantial efficacy due to the non-response or acquired resistance to ICIs.
Several reports have shown that MDSCs promote ICIs resistance. A prospective cohort study of NSCLC patients showed a decreased number of M-MDSCs in the nivolumab-response group whereas the data of non-responders stayed steady. In vitro, researchers found resuscitated secretion IFN-γ of CD8+ T cells under treatment of anti-PD-1, which was impaired by M-MDSCs via the bond of galectin-9 and its receptor TIM-3, indicating that MDSCs can hinder the function of T cells (Wolchok et al., 2017; Limagne et al., 2019). When treated with ipilimumab, responders showed a remarkably lower percentage of M-MDSCs than non-responders (Meyer et al., 2014).
The baseline of MDSCs are associated with ICI response. Weber et al. (2016) found that patients with lower initial M-MDSCs levels in circulation had a better response to nivolumab. This concept was also detected in melanoma patients under nivolumab or ipilimumab treatment (Gibney et al., 2015; Bjoern et al., 2016). Melanoma patients with low baseline MDSC had 34.5% survival benefit compared to patients from higher initial MDSC level group. A computational algorithm was used to analyze the role of peripheral blood mononuclear cells (PBMCs). According to the program, M-MDSC levels were negatively associated with peripheral CD8+ T cell expansion after ipilimumab treatment, and melanoma patients with lower basic M-MDSC levels (less than 14.9%) had a remarkably longer OS (Kitano et al., 2014).
The MDSCs-related factors causing ICIs resistance have also been investigated. In a longitudinal study on nivolumab, PMN-MDSCs were analyzed to decreased significantly in responders while those in non-responders did not show obvious change. In non-responsive patients, factors correlated with MDSCs recruitment and proliferation, including CXCL2, CCL23, C-X3-C motif chemokine ligand 1, and high mobility group box protein 1, were heavily aggregated (Kim et al., 2018). In patients with advanced melanoma, ipilimumab infusion could induce an elevated level of both M-MDSCs and relevant NO production in non-responders (Gebhardt et al., 2015). Furthermore, increased levels of lactate dehydrogenase (LDH) and IL-4Rα+ M-MDSCs after ipilimumab administration were associated with impaired OS in melanoma patients.
Given that the battle against cancer cells takes place mostly in tumor sites, the prevention of T cells infiltration or the dysfunction of infiltrated T cells pose more barriers. In order to figure out factors behind these two kinds of tumor immune inhibition, Jiang and colleagues developed a predictive gene signature, tumor immune dysfunction and exclusion. They demonstrated a correlation between MDSCs profiles and levels of CTLs and that MDSCs signature could predict reaction to anti-PD-1 and anti-CTLA-4 (Jiang et al., 2018). Additionally, myeloid-associated genes, such as cyclooxygenase-2, IL-8, IL-1β, in the tumor were associated with atezolizumab or durvalumab resistance in urothelial bladder cancer patients (Kim et al., 2015). In general, investigations on the role of tumor-derived MDSCs in resistance to ICIs is limited, hindering a clearer cognition on MDSCs and more effective combination immunotherapeutic strategies.
The ACT is another immunotherapy research hotspot. While several strategies modify T cells milieu, ACT directly infuses autologous or allogenic T cells. ACT separates and expands TILs and unmodified cytotoxic cells population from the resected tumor and then transfers them to patients to fight against tumor cells (Rosenberg and Restifo, 2015). TILs have been demonstrated positive data in malignant tumors, such as melanoma (Guedan et al., 2018; Chandran and Klebanoff, 2019). Lymphodepletion incorporation before TILs reinfusion increases response rate, with 20 of 92 patients in complete tumor regression, 19 of which do not relapse 3 years after treatment (Rosenberg et al., 2011). Compared with other modalities of ACT, these results highlight the superiority of pre-existing effector T cells with antitumor activities, which is not eligible in many cancer types (Perica et al., 2015).
T cells from peripheral blood equipped with CARs or TCRs have also revealed a noteworthy effectiveness in hematologic malignancy, with two CAR-T products (axicabtagene ciloleucel and tisagenlecleucel) approved by FDA. The first clinical application of optimized CD19-targeting CAR-T cells in CLL induced a significant response. Clinical trials of CD19-directed CAR T cells have also been conducted in B cell acute lymphoblastic leukemia and lymphoma, with a high complete remission rate (Brentjens et al., 2013; Park et al., 2018; Chong et al., 2021). TCRs-modified T cells targeting specific antigenic peptide-MHC complex expressed mainly in tumor cells are also effective in patients with solid tumors (Angelo et al., 2018). Bispecific T-cell engager (BiTE) is another ACT modality. BiTEs enhance the biological connection of T cells and tumor cells via two scFvs with separate close affinity to CD3 and tumor antigens (Thakur et al., 2018). Blinatumomab (MT103), the first FDA-approved BiTE, specific for CD3 and CD19, is used to treat several hematological malignancies. Actually, achievements of these ACT strategies were gained mostly in hematologic malignancies. Several ACT therapies targeting tumor-specific antigens or stroma-derived structures are under investigation, and only modest efficacy has been achieved in solid tumors. MDSCs-induced immunosuppressive microenvironment posed one of the critical obstinate hurdles.
Several studies have shown that MDSCs play dirty tricks on ACT therapy (Arina and Bronte, 2015; Fultang et al., 2019; Mengos et al., 2019). Besides autologous T cell suppression, MDSCs inhibit both the expansion and function of adoptively transferred T cells. A recent study reported that TILs infusion combined with lymphodepletion significantly increases CD11b+CD15+LOX-1+ PMN-MDSCs, which suppress TILs proliferation and IFN-γ production in melanoma and NSCLC patients (Innamarato et al., 2020). IL-6 was demonstrated to motivate hematopoietic progenitor cells after lymphodepleting. Subsequently, IL-6 and motivated hematopoietic progenitor cells promoted the generation and activation of MDSCs and inhibition of IL-6 enhanced response to ACT in mouse models. A preclinical rodent animal model study showed that CAR-T therapy increases MDSC levels in a GM-CSF-dependent manner, inhibiting the anti-tumor activity of adoptive T cells (Burga et al., 2015). IDO, an intracellular enzyme in MDSCs mediating tryptophan metabolism, hinders CAR-T therapy efficacy via tryptophan metabolites, and a tumor model showed that IDO inhibitor could restore the therapeutic effect (Fan et al., 2017).
Interestingly, while several studies revealed that MDSCs inhibit transferred T cell treatments, an animal experiment showed that MDSCs-co-cultured T cells augment ACT efficacy (Raber et al., 2016). Fewer T cells preconditioned with MDSCs differentiated into effector T cells before adoptive transferring, preserving anti-tumor capability. Inhibition of T cell differentiation is relied on cell-to-cell contact without hindering TCR function or early activation process. A well-designed CD33– CAR-T cells, targeting both CD33+ blast and CD33+ MDSCs, promotes satisfied clinical results. The CD33xCD3 BiTEs studies reported similar results (Jitschin et al., 2018). Moreover, studies have reported that several MDSCs inhibition strategies enhance the anti-tumor effect of ACT therapy. However, the clinical trial outcomes are unknown (Fultang et al., 2019; Li et al., 2020; Sun et al., 2020).
Cancer vaccines and oncolytic virus enhance anti-tumor immune responses. Cancer vaccines contain several products, including immunocompetent cells, proteins, peptides, and nucleic acids, boosting T cell activation in tumors (Hu et al., 2018). The tumor-associated antigen (TAA) and neoantigen, mainly expressed in tumor cells, can be used in vaccine-based therapies (van der Bruggen et al., 2017). In contrast, oncolytic virus, modified viral particles, is an antigen-nonspecific agent used for cancer cell lysis to expose antigens, activating endogenous T cells to initiate cytotoxic responses to cancer cells (Kaufman et al., 2015). FDA has approved a DC vaccine product (sipuleucel T) and an oncolytic virus agent (talimogene laherparepvec) for prostate cancer and melanoma, respectively (Harrington et al., 2016; Kantoff et al., 2010). However, only a few neoantigen and TAAs can generate immune responses, possibly due to the negative selection during T cell development. To date, the clinical performance of cancer vaccines and the oncolytic virus is poor (Macedo et al., 2020).
Favorable outcomes of cancer vaccine and oncolytic virus treatment are dependent on decreased MDSC levels in both tumor models and cancer patients (Poschke et al., 2012; Laborde et al., 2014; Vandenberk et al., 2016). Several preclinical studies and clinical trials testing cancer vaccines and oncolytic virus efficacy have shown that high MDSC levels are related to poor response in cancer patients. Moreover, MDSCs levels increase after treatment (Clements et al., 2015; Keshavarz et al., 2020; Meng et al., 2020), indicating reinforced immunosuppression on anti-tumor immune response. A preclinical study on oncolytic vaccinia showed that increased PGE2 levels promote G-MDSC trafficking, inhibiting immunotherapeutic capability (Hou et al., 2016). Another study showed that the inflammatory component NLRP3, belonging to NOD-like receptor family, is essential in MDSCs-induced immunosuppression (van Deventer et al., 2010). In Nlrp3–/– mice, few MDSCs reached the tumor site, and survival was fourfold in the DC vaccinated group than wild-type mice. However, removing MDSCs with anti-Gr-1 antibody was ineffective in Nlrp3–/– mice, whereas it was effective in wild-type mice, suggesting that NLRP3 is essential in MDSC accumulation. Other studies also showed that MDSCs-derived molecules, including NO and TGF-β, inhibit vaccines and oncolytic virus treatment (Jia et al., 2010; Takaku et al., 2010). When MDSCs were significantly aggregated in the initial generating culture, DCs functions were significantly impaired (Poschke et al., 2012). A clinical trial investigating prophylactic vaccine (NCT02134925) showed high basic levels of circulating MDSCs, accounting for 22 poor responses of the 39 patients (Kimura et al., 2013). Generally, mounting evidence has shown that MDSCs negatively regulate the efficacy of cancer vaccines and oncolytic virus.
Cytokines administration is another strategy of immunotherapy and has been used as an adjuvant to modulate the immune system for robust anti-tumor immunity. T lymphocyte-promoting cytokines, including IL-2 and IFN-α, have gained significant advances. FDA first approved IL-2, an essential T cell growth factor, for cancer treatment. Clinical studies have revealed that it has generated a durable tumor regression in melanoma and renal cell carcinoma patients (Rosenberg, 2014). PEGylated IL-2 (bempegaldesleukin) combined with anti-PD-1 showed a 53% ORR in melanoma patients (Bentebibel et al., 2019). IFN-α is also an FDA-approved cytokine that induces the APC maturation to provide specific antigen presentation and costimulatory factors, triggering T cell activation and enhancing their cytotoxicity. However, IL-2 and other T cell activators have limited clinical utility, owing to their dose-limiting toxicities and facilitative effects on Tregs (Sim and Radvanyi, 2014; Floros and Tarhini, 2015). In recent years, immune-stimulatory cytokines have been mainly used in T cell expansion, promoting adoptive T cell therapies (Rosenberg, 2011).
Therapeutic cytokines are involved in the Th1 immune response, directly activating CTLs or enhancing activity of CTLs-promoting immune cells. However, several studies have shown that they have opposite effects on MDSCs during anti-tumor immune modulation. Alves et al. (2020) reported that there are more G-MDSCs (21.3%) in tyrosine kinase inhibitors (TKIs) and IFN-α combination therapy-treated patients than TKI treated patients (less than 10%), thus a poor immunosuppressive state. IL-2 also shows contradictory effects on PMN-MDSCs. Increased IL-2 levels extend MDSC lifespan in a dose-relevant manner, starting a backfire and impeding better therapeutic efficacy. Besides, augmented activated T cells and their increased GM-CSF production amplify the effect (Bauswein et al., 2018). Another study reported that IL-2 administration prevented the apoptosis of MDSCs and prolonged their survival, augmenting the destructive capability this cluster of cells (Pericle et al., 1994). To date, the effect of cytokines on immunotherapy is unclear. Further investigations are needed for the cytokine efficacy on malignant tumors and their regulation on the TME.
It is necessary to combine T cell-based immunotherapy with MDSCs targeting agents since MDSCs are key players in immunosuppressive TME (Figure 2). Multiple studies have been conducted to investigate the effectiveness of relevant combined approaches (Table 2). In this section, we elaborate the main MDSCs-manipulating strategies employed to reinforce the antitumor activity of T cell-based immunotherapy, through inhibiting expansion and recruitment, promoting differentiation, inhibiting function, inhibiting metabolism, or deleting MDSCs directly.
Figure 2. MDSCs-targeting strategies enhance T cell-based immunotherapy efficacy. ATRA, all-trans-retinoic acid; TKI, tyrosine kinase inhibitor; PDE5, phosphodiesterase-5; HDAC, histone deacetylase; TLRs, toll-like receptors.
Blockers or antagonists of chemoattractants and their receptors could effectively diminish proportion of MDSCs both in circulation and TME, modifying the immunosuppressive microenvironment. The CXCLs-CXCR2 axis is essential in PMN-MDSC recruitment, and blocking the CXCLs-CXCR2 axis pathway inhibits PMN-MDSC aggregation (Highfill et al., 2014; Liao et al., 2019; Horn et al., 2020; Yang et al., 2021). CXCR2+ PMN-MDSCs also increase the expression of inhibitory immune checkpoints, such as PD-1, CTLA-4, and LAG3 on T lymphocytes, promoting T cells anergy (Zhu et al., 2017). Preclinical studies have reported that CXCR2 inhibitors or anti-CXCR2 antibodies alleviate MDSCs-induced immunosuppression, thus improving the effects of anti-PD-1 therapy in rhabdomyosarcoma, pancreatic ductal adenocarcinoma, and colorectal cancer (Steele et al., 2016; Horn et al., 2020). Clinical trials analyzing synergism in CXCR2 inhibitor SX-682 on pembrolizumab or nivolumab are underway (NCT03161431, NCT04477343, and NCT04599140). A human monoclonal antibody that inhibits one of its critical ligands, IL-8, has been used in phase I clinical trial (NCT02536469) (Bilusic et al., 2019) to interrupt CXCR2 pathway activation. Serum IL-8 level significantly reduced two days after anti-IL-8 administration. Anti-IL-8 and nivolumab efficacy is under clinical investigation (NCT03400332 and NCT04123379). CCR2 and CCR5 are essential in M-MDSC recruitment, and CCR5+ MDSCs enriched in tumor sites have a stronger immunosuppressive property. Flores-Toro et al. (2020) reported that CCR2 deficiency or administration of CCR2 antagonist CCX872 promotes efficacy of anti-PD-1 in mouse glioma model. CCX872 inhibits MDSC trafficking, increasing MDSC levels in the bone marrow and reducing their aggregation in the tumor site. The TILs data showed an increased population, elevated IFN-γ secretion, and decreased exhaustion marker expressions. Efficacy of CCR2/CCR5 dual inhibitor combined with nivolumab is under investigation (NCT03496662 and NCT03184870). CSF-1/CSF-1R pathway disruption also inhibits M-MDSCs infiltration, concomitantly influencing TAMs accumulation. Holmgaard et al. (2016) reported that CSF-1R expression on MDSCs is significantly increased after CTLA-4 blockade immunotherapy, with aggravated T cell inhibition. A combination of CTLA-4 blockade and anti-CSF-1R antibody promotes anti-tumor immunity and exacerbates tumor regression (Holmgaard et al., 2016). Additionally, selective CSF-1R targeting using specific inhibitors or antibody attenuates suppressive myeloid cells, and elective CSF-1R targeting combined with PD-1/PD-L1 blockade significantly control tumor growth in mouse neuroblastoma, breast cancer, and colorectal cancer models (Mao et al., 2016; Huang et al., 2020).
All-trans-retinoic acid (ATRA), a member of retinoid family essential in differentiation induction and commonly used in acute promyelocytic leukemia treatment, also inhibits MDSC abundance. ATRA induces MDSC differentiation into macrophages and DCs via an ERK1/2 kinase signaling pathway (Bauer et al., 2018). A phase II clinical study showed that MDSC levels were significantly decreased in SCLC patients treated with combined P53-specific DC vaccine and ATRA compared with the DC vaccine alone (NCT00617409). The P53-specific response had a higher positive rate in the combination group, and granzyme B-positive CD8+ T cell enrichment was only observed in the combination group (Iclozan et al., 2013). Furthermore, ATRA synergistically enhanced ipilimumab efficacy in advanced melanoma patients (NCT02403778) (Tobin et al., 2018). In this study, MDSC levels increased in the ipilimumab monotherapy group and decreased in the combined treatment group. Moreover, HLA-DR+ myeloid cell levels significantly increased, promoting CD8+ T cell production, compared with ipilimumab treatment alone. Traditional chemotherapies inhibit MDSC trafficking and enable them to traffic back. In contrast, ATRA shows advantages by converting MDSCs into more differentiated cells, such as DCs and macrophages, essential in T cell-based immune response.
Toll-like receptors are transmembrane proteins that recognize protein or lipid ligands and activate transcription factors, facilitating the expression of pro-inflammatory factors, such as TNF-α and IL-2 (O’Neill et al., 2013). TLR7/TLR8 activation via TLR7/TLR8 agonist R848 induces M-MDSC differentiation into anti-tumor M1-type macrophages, whereas TLR1/TLR2 activation facilitates the M-MDSC transformation into suppressive M2-type macrophages (Wang et al., 2015; Shayan et al., 2018; Liu et al., 2020). B16 melanoma mouse model showed that R848-loaded b-cyclodextrin nanoparticles (CDNP-R848) improves anti-PD-1 treatment efficacy (Rodell et al., 2018). TLR3/TLR4 activation promotes receptor-interacting protein kinase 3 (RIPK3)-mediated programmed necrosis (Kearney and Martin, 2017). A RIPK3 reduction is associated with MDSC aggregation in colorectal cancer (Yan et al., 2018). Polyinosinic-polycytidylic acid (poly ICLC), TLR3 agonist, decreases MDSC levels, inhibiting their immunosuppressive function (Forghani and Waller, 2015). Additionally, poly ICLC significantly attenuates MDSC aggregation and function when combined with the CAR-T cells, enhancing cytotoxic activity of CAR-T cells via increased IL-2 and IFN-γ production (Forghani and Waller, 2015). TLR9 ligand CpG also blocks MDSC immunosuppression in T cells (Zoglmeier et al., 2011). CpG stimulation reduces Th2 cytokine and increases Th1 cytokine production in MDSCs and more M-MDSCs differentiate into tumoricidal M1 macrophages (Shirota et al., 2012). TLR7, TLR8, and TLR9 co-activation removes large tumors and builds an immune protective line by enhancing the NK cells and CTL infiltration, thus reducing MDSC levels (Zhao et al., 2014).
Signaling cascade-targeting agents compromising MDSCs differentiation, expansion, or function, including JAKs-STATs pathway, PI3K-AKT pathway, is an alternative to T cell-based immunotherapy. MDSCs functions, including immunosuppression and tumor promoting, are well orchestrated by STAT3 pathway. Strategies targeting STAT3 are under active investigation. A mouse liver metastatic tumor model showed that STAT3 inhibition enhanced the anti-tumor efficacy of CAR-T therapy by activating apoptotic signaling pathways and decreasing proliferative signaling pathways in liver-associated MDSCs (Guha et al., 2019). Clinical trials of STAT3 inhibition combined with ICIs are under investigation (NCT02983578 and NCT02499328). PI3K-AKT pathway is also essential in MDSC functions, migration, and metabolism (Martini et al., 2014). PI3Kγ, a PI3K subtype, promotes immunosuppression in malignancies and selectively targeting PI3Kγ with a specific inhibitor IPI-549 inhibits MDSCs-induced immunosuppression, restoring T cells-induced tumoricidal response (Kaneda et al., 2016). A mouse prostate cancer model engineered using signature mutations, BEZ235, a pan-class I PI3K/mTOR inhibitor, combined with immune checkpoint blockade showed a robust synergistic therapeutic efficacy (Lu et al., 2017a).
Several preclinical and clinical studies have shown that the phosphodiesterase-5 (PDE5) inhibitor, used to treat erectile dysfunction, inhibits MDSCs (Weed et al., 2019). A mouse tumor model showed that PDE5 inhibition with tadalafil attenuates MDSC suppressive capabilities by downregulating ARG1 and iNOS expressions, thus increasing T cell infiltration and activation (Yu et al., 2019). In the same study, administration of sildenafil was also approved to potentiate antitumor activity of adoptive therapy. In a clinical study, Tadalafil altered anti-tumor immunity in patients with recurrent HNSCC by downregulating MDSCs and Tregs and increasing cytotoxic CD8+ T cell levels in both peripheral blood and tumor site (Weed et al., 2019). Tadalafil combined with MUC1/polyICLC vaccine also inhibits PD-L1+ macrophage aggregation at the tumor edge, consistent with two clinical trials (NCT00843635 and NCT00894413) (Califano et al., 2015; Weed et al., 2015). However, the efficacy and survival of the trials investigating the role of a PDE5 inhibitor in immune modulation are unknown.
Entinostat, an epigenetic regulator, selectively targeting class I histone deacetylase (HDAC), inhibits MDSC function, reverses immune exclusion, and enhances anti-tumor activity. Entinostat combined with an epigenetic adjuvant, methyltransferase inhibitor 5-azacytidine, alters MDSC trafficking by downregulating CCR2 and CXCR2 expression, inducing MDSC differentiation into an interstitial macrophage-like phenotype (Lu et al., 2020). Murine breast cancer and pancreatic tumor models showed that entinostat combined with anti-PD-1 therapy or anti-CTLA-4 therapy significantly alleviates MDSCs-induced immunosuppression, increases activated granzyme-B-producing CD8+ T effector cell infiltration, significantly improving tumor-free survival (Christmas et al., 2018). A phase II clinical trial (NCT02437136) showed that entinostat combined with pembrolizumab has a favorable response (19%) and 36% clinical benefit rate in 53 progressed melanoma patients (Johnson et al., 2017). Besides, several HDAC inhibitors are being studied (Hashimoto et al., 2020; Kim et al., 2020).
Adenosine also drives tumor progression via various mechanism, including MDSCs-mediated immunosuppression. Abrogating adenosine production by targeting nucleotide-metabolizing enzymes CD73 and CD39, as well as adenosine receptor A2AR seems to be a promising therapeutic strategy (Festag et al., 2020). A clinical study on ovarian cancer patients showed that metformin treatment downregulated the expression and catalytic activity of CD39 and CD73 in both M-MDSCs and PMN-MDSCs via adenosine monophosphate-activated protein kinase α (AMPKα) activation and HIF-1α suppression (Li L. et al., 2018). Besides, the circulating MDSC levels were decreased, and the cytotoxic activity of CD8+ T cells was restored, prolonging the OS of ovarian cancer patients with diabetes (Li L. et al., 2018). Additionally, anti-PD-1 immunotherapy upregulates CD73 level in melanoma patients (Reinhardt et al., 2017). A mouse breast cancer model showed that CD73 specific siRNA-loaded chitosan lactate nanoparticles improve tumor lysate pulsed DC vaccine efficacy. Furthermore, synergism is associated with MDSCs downregulation and T cells upregulation with reduced IL-10 levels and increased IFN-γ secretion (Jadidi-Niaragh et al., 2017). Several preclinical studies have shown that anti-CD73 therapy or A2AR targeting strategies significantly improves ICIs and ACT efficacy compared with monotherapy (Allard et al., 2013; Iannone et al., 2014; Mittal et al., 2014; Beavis et al., 2015; Hay et al., 2016; Reinhardt et al., 2017). Clinical trials evaluating the synergistic effects of CD73-A2AR targeting strategies and T cell-based therapy are underway.
Prostaglandin E2 is an inflammatory factor associated with carcinogenesis and MDSCs induction. COX-1 and COX-2 are key enzymes in PGE2 synthesis. COX-2/PGE2 signaling blockade inhibits MDSCs recruitment and represses MDSCs-induced immunosuppression, causing modified CTL cytotoxicity and enhanced tumoricidal immune response, thus improving therapeutic efficacy. Also, mounting evidence has shown that targeting PGE2 with non-steroidal anti-inflammatory drugs or specific COX-2 inhibitors, such as celecoxib, inhibiting MDSCs, improves immunotherapy outcomes (Veltman et al., 2010; Obermajer et al., 2011). A mouse melanoma model showed that licofelone, a dual COX/5-lipoxygenase (5-LOX) inhibitor, improves therapeutic vaccine efficacy by suppressing Gr-1+CD11b+ MDSC generation and minimizing IL-6 and IL-10 production (Neumann et al., 2016). These studies provide new insights for developing targeted COX-2-mediated PGE2 signaling combined with T cell-based immunotherapies.
Several chemotherapeutic agents reduce MDSCs numbers, and combination strategies with immunotherapy can improve the survival of cancer patients (Draghiciu et al., 2015; Wang et al., 2017). Gemcitabine and 5-fluorouracil (5-FU) are among the most commonly used drugs to eliminate MDSCs in tumor models and cancer patients (Wang et al., 2017). A phase I/II study evaluated the efficacy of gemcitabine combined with pegintron (IFN-α) and p53 synthetic long peptide vaccine in ovarian cancer patients (NCT01639885) (Dijkgraaf et al., 2015). The study showed a significant reduce of MDSCs in gemcitabine group. Further, the combined therapy showed stronger vaccine-induced T-cell responses. In another study, Vincent et al. (2010) indicated that 5-FU selectively inhibits MDSCs, thus promoting T cell-based anti-tumor capability. However, various chemotherapeutic agents have different effects on MDSC modulation. A phase II clinical trial reported that the DC vaccine combined with docetaxel decreases MDSCs, which was an independent prognostic factor of disease-specific survival (NCT01446731) (Kongsted et al., 2017). Nonetheless, the combined therapy showed no significant clinical advantage over docetaxel monotherapy alone, possibly due to the limited included population. Moreover, a spontaneous melanoma model showed that 1 mg/kg paclitaxel administration reduces MDSC levels while 36 mg/kg dosage has no effect (Vincent et al., 2010; Sevko et al., 2013). Conversely, a 175 mg/m2 paclitaxel dose increased circulating MDSC levels (Diaz-Montero et al., 2009). Preconditioning EG7 tumor-bearing mice with a single low dose of doxorubicin or paclitaxel promoted ACT efficacy, with more activated and longer-sustained CD8+ T cells, probably due to MDSC inhibition via suppression of NF-κB and its associated immunosuppressive factors (Hsu et al., 2015). Ongoing clinical trials evaluating the synergistic effect of various chemotherapeutic agents combined with immunotherapy are shown in Table 2. Chemotherapeutic agents influence various cells and only MDSCs-related trials are included. There are many effects of chemotherapeutic agents on MDSC accumulation and various factors should be considered, including optimal drug combinations, administration dosage, intervals, and tumor types and stages.
Immunotherapy combined with TKIs provides an optional choice of standard treatment of several cancers (Hirsch et al., 2020). TKIs are commonly used agents in MDSCs manipulation. To target tyrosine kinases, sunitinib have been applied to patients with oligometastases of various cancer types. The progression-free survival and cause-specific survival were prolonged in sunitinib and radiotherapy combination arm due to the sunitinib-induced M-MDSC reduction and CD4+ and CD8+ T cell enrichment (Chen et al., 2015). However, a phase II clinical trial (NCT01118351) showed no improvement in non-muscle-invasive bladder cancer patients after sunitinib monotherapy regardless of the reversal of MDSCs-mediated immunosuppression (Zahoor et al., 2019). Preclinical or clinical studies have shown that several other TKIs, such as dasatinib and nilotinib, also inhibit MDSCs (Hughes et al., 2017). Notably, apart from MDSCs, TKIs influences other cells. For instance, sorafenib and dasatinib suppress T cells and NK cells in a dose-dependent manner, causing immunocompetence (Martin del Campo et al., 2015). VEGFR-TKI is a group of TKI that can be combined with T cell-based immunotherapy for its active role in angiogenesis and immune modulation. Antiangiogenic agents ameliorate TME by inhibiting immunosuppressive cell infiltration, including MDSCs and Tregs, and increasing recruitment of effector T cells and mature DCs.
High-dose radiation is also a candidate in MDSCs elimination. Radiotherapy is mainstay treatment for localized tumors and isolated metastasis, as well as patients with advanced cancer for palliative treatment. Radiotherapy accelerates immunogenic cell death and induces the release of tumor antigens and a cluster of inflammatory factors, including alarmins, cytokines, and chemokines (Weichselbaum et al., 2017). Certainly, these factors facilitate the infiltration of DCs, T cells, and MDSCs. cGAS-STING pathway is a critical signal in the infiltration of MDSCs and STING agonists alleviated MDSCs-mediated immunosuppression (Liang et al., 2017). The increased infiltration of MDSCs after radiotherapy was mostly witnessed in conventional fractionated radiation. However, ablative and/or hypofractionated radiation resulted the loss of MDSCs, leading to an enhanced antitumor immunity (Filatenkov et al., 2015; Lan et al., 2018). These reversed observations may be correlated with the earlier infiltration of cytotoxic CD8+ T cells (Deng et al., 2014; Filatenkov et al., 2015). When in combination with anti-PD-L1antibody, ablative hypofractionated radiation therapy was more potent for cancer treatment (Lan et al., 2018). Moreover, radiation could upregulate the expression of PD-L1. High-dose ionizing irradiation and PD-L1 blockade synergistically inhibited the infiltration of MDSCs, promoting antitumor immunity (Deng et al., 2014). Generally, radiotherapy is a promising strategy to enhance efficacy of T cell-based immunotherapy for its immune-stimulating capability. High-dose radiation has advantages in MDSCs elimination over conventional radiation.
The last decade has witnessed an evolution in cancer treatment with the progress of immunotherapy. Scientists have expanded the understanding of cancer biology, identifying cytotoxic lymphocytes as a major force to combat against tumor cells. The personalized T cell-based immunotherapy has increasingly been used in cancer patients due to the increasing number of potential clinical trials and FDA-approved therapies. However, only a few patients benefit from these therapies. Mounting evidence suggests that T cell-based immunotherapy efficacy is associated with robust anti-tumor immune response, which is usually damaged in most cases. The fundamental goal of immunotherapy is infiltration of effector cytotoxic T cells. Nevertheless, immunosuppressive cells always work as a vital suppressive force of antitumor immune response. Among these populations, MDSCs play critical roles and pose a challenging to broader-spectrum benefits. Since ICIs are referred to as the “release the brakes” of the immune system, inhibition of MDSCs could act as effective brake pads and this may be an encouraging supplementary strategy for T cell-based immunotherapy. Rational combination therapies seem to be a promising resolution in cancer treatment.
Several therapeutic strategies combining MDSCs targeting strategies and T cell-based immunotherapy have been evaluated. However, most studies have failed, indicating that not all combination strategies synergistically enhance anti-tumor immunity. The immune system is complex, making it difficult to understand predictors. Moreover, MDSCs are a cluster of heterogeneous cells and it is difficult to target myeloid-derived cells due to their diversity, dynamic phenotypes, and functions. Therefore, it is necessary to further study the immunosuppressive network in the TME to reverse MDSCs-induced immunosuppression. To reveal a comprehensive landscape, multiomics and computer-assisted algorithms work more efficiently (Zhou, 2020). These discoveries and innovations will provide us a clear cognition on how to rationally design personalized integrative therapeutic strategies.
HS and KL drafted the manuscript. YN designed all figures and made substantial revision to the original manuscript. XZ and XL checked and modified the manuscript. All authors contributed to the article and approved the submitted version.
This work was supported by National Major Scientific and Technological Special Project for the “Significant New Drugs Development” (No. 2018ZX09201018-013), the National Natural Science Foundation of China (No. 81821002), National Science and Technology Major Projects for Major New Drugs Innovation and Development (No. 2018ZX09733001-004), Sichuan Medical Research Project (No. S15059), Sichuan Science and Technology Program (No. 2021YJ0011), and National Natural Science Foundation of China (No. 81902662).
The authors declare that the research was conducted in the absence of any commercial or financial relationships that could be construed as a potential conflict of interest.
Adeshakin, A. O., Liu, W., Adeshakin, F. O., Afolabi, L. O., Zhang, M., Zhang, G., et al. (2021). Regulation of ROS in myeloid-derived suppressor cells through targeting fatty acid transport protein 2 enhanced anti-PD-L1 tumor immunotherapy. Cell. Immunol. 362:104286. doi: 10.1016/j.cellimm.2021.104286
Alkasalias, T., Moyano-Galceran, L., Arsenian-Henriksson, M., and Lehti, K. (2018). Fibroblasts in the tumor microenvironment: Shield or spear? Int. J. Mol. Sci. 19:1532. doi: 10.3390/ijms19051532
Allard, B., Pommey, S., Smyth, M. J., and Stagg, J. (2013). Targeting CD73 enhances the antitumor activity of anti-PD-1 and Anti-CTLA-4 mAbs. Clin. Cancer Res. 19, 5626–5635. doi: 10.1158/1078-0432.CCR-13-0545
Alves, R., McArdle, S. E. B., Vadakekolathu, J., Gonçalves, A. C., Freitas-Tavares, P., Pereira, A., et al. (2020). Flow cytometry and targeted immune transcriptomics identify distinct profiles in patients with chronic myeloid leukemia receiving tyrosine kinase inhibitors with or without interferon-α. J. Transl. Med. 18:2.
Anderson, A. C., Joller, N., and Kuchroo, V. K. (2016). Lag-3, Tim-3, and TIGIT: co-inhibitory receptors with specialized functions in immune regulation. Immunity 44, 989–1004. doi: 10.1016/j.immuni.2016.05.001
Andrews, L. P., Yano, H., and Vignali, D. A. A. (2019). Inhibitory receptors and ligands beyond PD-1, PD-L1 and CTLA-4: breakthroughs or backups. Nat. Immunol. 20, 1425–1434. doi: 10.1038/s41590-019-0512-0
Angelo, S. P., Melchiori, L., Merchant, M. S., Bernstein, D., Glod, J., Kaplan, R., et al. (2018). Antitumor activity associated with prolonged persistence of adoptively transferred NY-ESO-1 c259T cells in Synovial Sarcoma. Cancer Discov. 8, 944–957. doi: 10.1158/2159-8290.CD-17-1417
Antonia, S. J., Villegas, A., Daniel, D., Vicente, D., Murakami, S., Hui, R., et al. (2017). Durvalumab after chemoradiotherapy in stage III non–small-cell lung cancer. N. Engl. J. Med. 377, 1919–1929. doi: 10.1056/NEJMoa1709937
Arina, A., and Bronte, V. (2015). Myeloid-derived suppressor cell impact on endogenous and adoptively transferred T cells. Curr. Opin. Immunol. 33, 120–125. doi: 10.1016/j.coi.2015.02.006
Bauer, R., Udonta, F., Wroblewski, M., Ben-Batalla, I., Santos, I. M., Taverna, F., et al. (2018). Blockade of myeloid-derived suppressor cell expansion with all-trans retinoic acid increases the efficacy of antiangiogenic therapy. Cancer Res. 78, 3220–3232. doi: 10.1158/0008-5472.can-17-3415
Bauswein, M., Singh, A., Ralhan, A., Neri, D., Fuchs, K., Blanz, K. D., et al. (2018). Human T cells modulate myeloid-derived suppressor cells through a TNF-α-mediated mechanism. Immunol. Lett. 202, 31–37. doi: 10.1016/j.imlet.2018.07.010
Beavis, P. A., Milenkovski, N., Henderson, M. A., John, L. B., Allard, B., Loi, S., et al. (2015). Adenosine receptor 2A blockade increases the efficacy of anti–PD-1 through enhanced antitumor T-cell responses. Cancer Immunol. Res. 3, 506–517. doi: 10.1158/2326-6066.CIR-14-0211
Bentebibel, S.-E., Hurwitz, M. E., Bernatchez, C., Haymaker, C., Hudgens, C. W., Kluger, H. M., et al. (2019). A first-in-human study and biomarker analysis of NKTR-214, a novel IL2Rβγ-biased cytokine, in patients with advanced or metastatic solid tumors. Cancer Discov. 9, 711–721. doi: 10.1158/2159-8290.CD-18-1495
Beury, D. W., Carter, K. A., Nelson, C., Sinha, P., Hanson, E., Nyandjo, M., et al. (2016). Myeloid-derived suppressor cell survival and function are regulated by the transcription factor Nrf2. J. Immunol. 196, 3470–3478. doi: 10.4049/jimmunol.1501785
Bian, Z., Abdelaal, A. M., Shi, L., Liang, H., Xiong, L., Kidder, K., et al. (2018). Arginase-1 is neither constitutively expressed in nor required for myeloid-derived suppressor cell-mediated inhibition of T-cell proliferation. Eur. J. Immunol. 48, 1046–1058. doi: 10.1002/eji.201747355
Bilusic, M., Heery, C. R., Collins, J. M., Donahue, R. N., Palena, C., Madan, R. A., et al. (2019). Phase I trial of HuMax-IL8 (BMS-986253), an anti-IL-8 monoclonal antibody, in patients with metastatic or unresectable solid tumors. J. Immunother. Cancer 7, 240. doi: 10.1186/s40425-019-0706-x
Bjoern, J., Juul Nitschke, N., Zeeberg Iversen, T., Schmidt, H., Fode, K., and Svane, I. M. (2016). Immunological correlates of treatment and response in stage IV malignant melanoma patients treated with Ipilimumab. Oncoimmunology 5:e1100788. doi: 10.1080/2162402X.2015.1100788
Brahmer, J. R., Tykodi, S. S., Chow, L. Q. M., Hwu, W.-J., Topalian, S. L., Hwu, P., et al. (2012). Safety and activity of Anti–PD-L1 antibody in patients with advanced cancer. N. Engl. J. Med. 366, 2455–2465. doi: 10.1056/NEJMoa1200694
Brentjens, R. J., Davila, M. L., Riviere, I., Park, J., Wang, X., Cowell, L. G., et al. (2013). CD19-targeted T cells rapidly induce molecular remissions in adults with chemotherapy-refractory acute lymphoblastic leukemia. Sci. Transl. Med. 5:177ra138. doi: 10.1126/scitranslmed.3005930
Bronte, V., Brandau, S., Chen, S.-H., Colombo, M. P., Frey, A. B., Greten, T. F., et al. (2016). Recommendations for myeloid-derived suppressor cell nomenclature and characterization standards. Nat. Commun. 7:12150. doi: 10.1038/ncomms12150
Bruni, D., Angell, H. K., and Galon, J. (2020). The immune contexture and Immunoscore in cancer prognosis and therapeutic efficacy. Nat. Rev. Cancer 20, 662–680. doi: 10.1038/s41568-020-0285-7
Bruno, A., Mortara, L., Baci, D., Noonan, D. M., and Albini, A. (2019). Myeloid derived suppressor cells interactions with natural killer cells and pro-angiogenic activities: roles in tumor progression. Front. Immunol. 10:771. doi: 10.3389/fimmu.2019.00771
Bruns, H., Böttcher, M., Qorraj, M., Fabri, M., Jitschin, S., Dindorf, J., et al. (2017). CLL-cell-mediated MDSC induction by exosomal miR-155 transfer is disrupted by vitamin D. Leukemia 31, 985–988. doi: 10.1038/leu.2016.378
Burga, R. A., Thorn, M., Point, G. R., Guha, P., Nguyen, C. T., Licata, L. A., et al. (2015). Liver myeloid-derived suppressor cells expand in response to liver metastases in mice and inhibit the anti-tumor efficacy of anti-CEA CAR-T. Cancer Immunol. Immunother. 64, 817–829. doi: 10.1007/s00262-015-1692-6
Califano, J. A., Khan, Z., Noonan, K. A., Rudraraju, L., Zhang, Z., Wang, H., et al. (2015). Tadalafil augments tumor specific immunity in patients with head and neck squamous cell carcinoma. Clin. Cancer Res. 21, 30–38. doi: 10.1158/1078-0432.CCR-14-1716
Callahan, M. K., Kluger, H., Postow, M. A., Segal, N. H., Lesokhin, A., Atkins, M. B., et al. (2018). Nivolumab plus Ipilimumab in patients with advanced melanoma: updated survival, response, and safety data in a phase I dose-escalation study. J. Clin. Oncol. 36, 391–398. doi: 10.1200/JCO.2017.72.2850
Chai, E., Zhang, L., and Li, C. (2019). LOX-1+ PMN-MDSC enhances immune suppression which promotes glioblastoma multiforme progression. Cancer Manage. Res. 11, 7307–7315. doi: 10.2147/CMAR.S210545
Chaib, M., Chauhan, S. C., and Makowski, L. (2020). Friend or Foe? Recent strategies to target myeloid cells in cancer. Front. Cell Dev. Biol. 8:351. doi: 10.3389/fcell.2020.00351
Chandran, S. S., and Klebanoff, C. A. (2019). T cell receptor-based cancer immunotherapy: emerging efficacy and pathways of resistance. Immunol. Rev. 290, 127–147. doi: 10.1111/imr.12772
Chauvin, J.-M., and Zarour, H. M. (2020). TIGIT in cancer immunotherapy. J. Immunother. Cancer 8:e000957. doi: 10.1136/jitc-2020-000957
Chen, D. S., and Mellman, I. (2017). Elements of cancer immunity and the cancer–immune set point. Nature 541, 321–330. doi: 10.1038/nature21349
Chen, H.-M., Ma, G., Gildener-Leapman, N., Eisenstein, S., Coakley, B. A., Ozao, J., et al. (2015). Myeloid-derived suppressor cells as an immune parameter in patients with concurrent Sunitinib and Stereotactic body radiotherapy. Clin. Cancer Res. 21, 4073–4085. doi: 10.1158/1078-0432.CCR-14-2742
Chong, E. A., Ruella, M., and Schuster, S. J. (2021). Five-year outcomes for refractory B-cell lymphomas with CAR T-cell therapy. N. Engl. J. Med. 384, 673–674. doi: 10.1056/NEJMc2030164
Christmas, B. J., Rafie, C. I., Hopkins, A. C., Scott, B. A., Ma, H. S., Cruz, K. A., et al. (2018). Entinostat converts immune-resistant breast and pancreatic cancers into checkpoint-responsive tumors by reprogramming tumor-infiltrating MDSCs. Cancer Immunol. Res. 6, 1561–1577. doi: 10.1158/2326-6066.CIR-18-0070
Chun, E., Lavoie, S., Michaud, M., Gallini, C. A., Kim, J., Soucy, G., et al. (2015). CCL2 promotes colorectal carcinogenesis by enhancing polymorphonuclear myeloid-derived suppressor cell population and function. Cell Rep. 12, 244–257. doi: 10.1016/j.celrep.2015.06.024
Cimen Bozkus, C., Elzey, B. D., Crist, S. A., Ellies, L. G., and Ratliff, T. L. (2015). Expression of cationic amino acid transporter 2 is required for myeloid-derived suppressor cell–mediated control of T cell immunity. J. Immunol. 195, 5237–5250. doi: 10.4049/jimmunol.1500959
Clements, D. R., Sterea, A. M., Kim, Y., Helson, E., Dean, C. A., Nunokawa, A., et al. (2015). Newly recruited CD11b+, GR-1+, Ly6C(high) myeloid cells augment tumor-associated immunosuppression immediately following the therapeutic administration of oncolytic reovirus. J. Immunol. 194, 4397–4412. doi: 10.4049/jimmunol.1402132
Colligan, S. H., Tzetzo, S. L., and Abrams, S. I. (2020). Myeloid-driven mechanisms as barriers to antitumor CD8+ T cell activity. Mol. Immunol. 118, 165–173. doi: 10.1016/j.molimm.2019.12.012
Condamine, T., Dominguez, G. A., Youn, J.-I., Kossenkov, A. V., Mony, S., Alicea-Torres, K., et al. (2016). Lectin-type oxidized LDL receptor-1 distinguishes population of human polymorphonuclear myeloid-derived suppressor cells in cancer patients. Sci. Immunol. 1:aaf8943. doi: 10.1126/sciimmunol.aaf8943
Condamine, T., Kumar, V., Ramachandran, I. R., Youn, J.-I., Celis, E., Finnberg, N., et al. (2014). ER stress regulates myeloid-derived suppressor cell fate through TRAIL-R–mediated apoptosis. J. Clin. Invest. 124, 2626–2639. doi: 10.1172/JCI74056
Curigliano, G., Gelderblom, H., Mach, N., Doi, T., Tai, W. M. D., Forde, P., et al. (2019). Abstract CT183: phase (Ph) I/II study of MBG453± spartalizumab (PDR001) in patients (pts) with advanced malignancies. Cancer Res. 79(13 Suppl.):CT183. doi: 10.1158/1538-7445.AM2019-CT183
Dar, A. A., Patil, R. S., Pradhan, T. N., Chaukar, D. A., D’Cruz, A. K., and Chiplunkar, S. V. (2020). Myeloid-derived suppressor cells impede T cell functionality and promote Th17 differentiation in oral squamous cell carcinoma. Cancer Immunol. Immunother. 69, 1071–1086. doi: 10.1007/s00262-020-02523-w
Darvin, P., Toor, S. M., Sasidharan Nair, V., and Elkord, E. (2018). Immune checkpoint inhibitors: recent progress and potential biomarkers. Exp. Mol. Med. 50, 1–11. doi: 10.1038/s12276-018-0191-1
Deng, L., Liang, H., Burnette, B., Beckett, M., Darga, T., Weichselbaum, R. R., et al. (2014). Irradiation and anti-PD-L1 treatment synergistically promote antitumor immunity in mice. J. Clin. Invest. 124, 687–695. doi: 10.1172/JCI67313
Desai, R., Coxon, A. T., and Dunn, G. P. (2021). Therapeutic applications of the cancer immunoediting hypothesis. Semin. Cancer Biol. doi: 10.1016/j.semcancer.2021.03.002 [Epub ahead of print].
Diaz-Montero, C. M., Finke, J., and Montero, A. J. (2014). Myeloid-derived suppressor cells in cancer: therapeutic, predictive, and prognostic implications. Semin. Oncol. 41, 174–184. doi: 10.1053/j.seminoncol.2014.02.003
Diaz-Montero, C. M., Salem, M. L., Nishimura, M. I., Garrett-Mayer, E., Cole, D. J., and Montero, A. J. (2009). Increased circulating myeloid-derived suppressor cells correlate with clinical cancer stage, metastatic tumor burden, and doxorubicin-cyclophosphamide chemotherapy. Cancer Immunol. Immunother. 58, 49–59. doi: 10.1007/s00262-008-0523-4
Dijkgraaf, E. M., Santegoets, S. J. A. M., Reyners, A. K. L., Goedemans, R., Nijman, H. W., van Poelgeest, M. I. E., et al. (2015). A phase 1/2 study combining gemcitabine, Pegintron and p53 SLP vaccine in patients with platinum-resistant ovarian cancer. Oncotarget 6, 32228–32243. doi: 10.18632/oncotarget.4772
Draghiciu, O., Lubbers, J., Nijman, H. W., and Daemen, T. (2015). Myeloid derived suppressor cells-An overview of combat strategies to increase immunotherapy efficacy. Oncoimmunology 4:e954829. doi: 10.4161/21624011.2014.954829
Emens, L. A., Ascierto, P. A., Darcy, P. K., Demaria, S., Eggermont, A. M. M., Redmond, W. L., et al. (2017). Cancer immunotherapy: opportunities and challenges in the rapidly evolving clinical landscape. Eur. J. Cancer 81, 116–129. doi: 10.1016/j.ejca.2017.01.035
Fan, F., Zhao, W., Liu, J., He, A., Chen, Y., Cao, X., et al. (2017). Durable remissions with BCMA-specific chimeric antigen receptor (CAR)-modified T cells in patients with refractory/relapsed multiple myeloma. J. Clin. Oncol 35:LBA3001. doi: 10.1200/JCO.2017.35.18_suppl.LBA3001
Festag, J., Thelemann, T., Schell, M., Raith, S., Michel, S., Jaschinski, F., et al. (2020). Preventing ATP Degradation by ASO-mediated knockdown of CD39 and CD73 results in A2aR-independent rescue of T cell proliferation. Mol. Ther. Nucleic Acids 21, 656–669. doi: 10.1016/j.omtn.2020.06.020
Filatenkov, A., Baker, J., Mueller, A. M. S., Kenkel, J., Ahn, G. O., Dutt, S., et al. (2015). Ablative tumor radiation can change the tumor immune cell microenvironment to induce durable complete remissions. Clin. Cancer Res. 21, 3727–3739. doi: 10.1158/1078-0432.CCR-14-2824
Flores-Toro, J. A., Luo, D., Gopinath, A., Sarkisian, M. R., Campbell, J. J., Charo, I. F., et al. (2020). CCR2 inhibition reduces tumor myeloid cells and unmasks a checkpoint inhibitor effect to slow progression of resistant murine gliomas. Proc. Natl. Acad. Sci. U.S.A. 117, 1129–1138. doi: 10.1073/pnas.1910856117
Floros, T., and Tarhini, A. A. (2015). Anticancer cytokines: biology and clinical effects of interferon-α2, Interleukin (IL)-2, IL-15, IL-21, and IL-12. Semin. Oncol. 42, 539–548. doi: 10.1053/j.seminoncol.2015.05.015
Forghani, P., and Waller, E. K. (2015). Poly (I: C) modulates the immunosuppressive activity of myeloid-derived suppressor cells in a murine model of breast cancer. Breast Cancer Res. Treat. 153, 21–30. doi: 10.1007/s10549-015-3508-y
Fourcade, J., Sun, Z., Benallaoua, M., Guillaume, P., Luescher, I. F., Sander, C., et al. (2010). Upregulation of Tim-3 and PD-1 expression is associated with tumor antigen–specific CD8+ T cell dysfunction in melanoma patients. J. Exp. Med. 207, 2175–2186. doi: 10.1084/jem.20100637
Fujimura, T., Ring, S., Umansky, V., Mahnke, K., and Enk, A. H. (2012). Regulatory T cells stimulate B7-H1 expression in myeloid-derived suppressor cells in ret melanomas. J. Invest. Dermatol. 132, 1239–1246. doi: 10.1038/jid.2011.416
Fultang, L., Panetti, S., Ng, M., Collins, P., Graef, S., Rizkalla, N., et al. (2019). MDSC targeting with Gemtuzumab ozogamicin restores T cell immunity and immunotherapy against cancers. EBioMedicine 47, 235–246. doi: 10.1016/j.ebiom.2019.08.025
Gandhi, L., Rodríguez-Abreu, D., Gadgeel, S., Esteban, E., Felip, E., De Angelis, F., et al. (2018). Pembrolizumab plus chemotherapy in metastatic non–small-cell lung cancer. N. Engl. J. Med. 378, 2078–2092. doi: 10.1056/NEJMoa1801005
Gebhardt, C., Sevko, A., Jiang, H., Lichtenberger, R., Reith, M., Tarnanidis, K., et al. (2015). Myeloid cells and related chronic inflammatory factors as novel predictive markers in melanoma treatment with Ipilimumab. Clin. Cancer Res. 21, 5453–5459. doi: 10.1158/1078-0432.CCR-15-0676
Gebremeskel, S., Clattenburg, D. R., Slauenwhite, D., Lobert, L., and Johnston, B. (2015). Natural killer T cell activation overcomes immunosuppression to enhance clearance of postsurgical breast cancer metastasis in mice. Oncoimmunology 4:e995562. doi: 10.1080/2162402X.2014.995562
Geiger, R., Rieckmann, J. C., Wolf, T., Basso, C., Feng, Y., Fuhrer, T., et al. (2016). L-arginine modulates T cell metabolism and enhances survival and anti-tumor activity. Cell 167, 829–842.e13. doi: 10.1016/j.cell.2016.09.031
Gibney, G. T., Kudchadkar, R. R., DeConti, R. C., Thebeau, M. S., Czupryn, M. P., Tetteh, L., et al. (2015). Safety, correlative markers, and clinical results of adjuvant nivolumab in combination with vaccine in resected high-risk metastatic Melanoma. Clin. Cancer Res. 21, 712–720. doi: 10.1158/1078-0432.CCR-14-2468
Godfrey, D. I., Le Nours, J., Andrews, D. M., Uldrich, A. P., and Rossjohn, J. (2018). Unconventional T cell targets for cancer immunotherapy. Immunity 48, 453–473. doi: 10.1016/j.immuni.2018.03.009
Greene, S., Robbins, Y., Mydlarz, W. K., Huynh, A. P., Schmitt, N. C., Friedman, J., et al. (2020). Inhibition of MDSC trafficking with SX-682, a CXCR1/2 inhibitor, enhances NK-cell immunotherapy in head and neck cancer models. Clin. Cancer Res. 26, 1420–1431. doi: 10.1158/1078-0432.CCR-19-2625
Greten, F. R., and Grivennikov, S. I. (2019). Inflammation and cancer: triggers, mechanisms, and consequences. Immunity 51, 27–41. doi: 10.1016/j.immuni.2019.06.025
Griss, J., Bauer, W., Wagner, C., Simon, M., Chen, M., Grabmeier-Pfistershammer, K., et al. (2019). B cells sustain inflammation and predict response to immune checkpoint blockade in human melanoma. Nat. Commun. 10:4186. doi: 10.1038/s41467-019-12160-2
Groth, C., Hu, X., Weber, R., Fleming, V., Altevogt, P., Utikal, J., et al. (2019). Immunosuppression mediated by myeloid-derived suppressor cells (MDSCs) during tumour progression. Br. J. Cancer 120, 16–25. doi: 10.1038/s41416-018-0333-1
Grzywa, T. M., Sosnowska, A., Matryba, P., Rydzynska, Z., Jasinski, M., Nowis, D., et al. (2020). Myeloid cell-derived arginase in cancer immune response. Front. Immunol. 11:938. doi: 10.3389/fimmu.2020.00938
Guedan, S., Ruella, M., and June, C. H. (2018). Emerging cellular therapies for cancer. Annu. Rev. Immunol. 37, 145–171. doi: 10.1146/annurev-immunol-042718-041407
Guha, P., Gardell, J., Darpolor, J., Cunetta, M., Lima, M., Miller, G., et al. (2019). STAT3 inhibition induces Bax-dependent apoptosis in liver tumor myeloid-derived suppressor cells. Oncogene 38, 533–548. doi: 10.1038/s41388-018-0449-z
Gulley, J. L., Rajan, A., Spigel, D. R., Iannotti, N., Chandler, J., Wong, D. J. L., et al. (2017). Avelumab for patients with previously treated metastatic or recurrent non-small-cell lung cancer (JAVELIN Solid Tumor): dose-expansion cohort of a multicentre, open-label, phase 1b trial. Lancet Oncol. 18, 599–610. doi: 10.1016/S1470-2045(17)30240-1
Harding, J. J., Patnaik, A., Moreno, V., Stein, M., Jankowska, A. M., Velez de Mendizabal, N., et al. (2019). A phase Ia/Ib study of an anti-TIM-3 antibody (LY3321367) monotherapy or in combination with an anti-PD-L1 antibody (LY3300054): interim safety, efficacy, and pharmacokinetic findings in advanced cancers. J. Clin. Oncol. 37:12.
Harrington, K. J., Andtbacka, R. H., Collichio, F., Downey, G., Chen, L., Szabo, Z., et al. (2016). Efficacy and safety of talimogene laherparepvec versus granulocyte-macrophage colony-stimulating factor in patients with stage IIIB/C and IVM1a melanoma: subanalysis of the Phase III OPTiM trial. Onco Targets Ther. 9, 7081–7093. doi: 10.2147/OTT.S115245
Hashimoto, A., Fukumoto, T., Zhang, R., and Gabrilovich, D. (2020). Selective targeting of different populations of myeloid-derived suppressor cells by histone deacetylase inhibitors. Cancer Immunol. Immunother. 69, 1929–1936. doi: 10.1007/s00262-020-02588-7
Hassan, R., Thomas, A., Nemunaitis, J. J., Patel, M. R., Bennouna, J., Chen, F. L., et al. (2019). Efficacy and safety of Avelumab treatment in patients with advanced unresectable mesothelioma: phase 1b results From the JAVELIN solid tumor trial. JAMA Oncol. 5, 351–357. doi: 10.1001/jamaoncol.2018.5428
Havel, J. J., Chowell, D., and Chan, T. A. (2019). The evolving landscape of biomarkers for checkpoint inhibitor immunotherapy. Nat. Rev. Cancer 19, 133–150. doi: 10.1038/s41568-019-0116-x
Hay, C. M., Sult, E., Huang, Q., Mulgrew, K., Fuhrmann, S. R., McGlinchey, K. A., et al. (2016). Targeting CD73 in the tumor microenvironment with MEDI9447. Oncoimmunology 5:e1208875. doi: 10.1080/2162402X.2016.1208875
Highfill, S. L., Cui, Y., Giles, A. J., Smith, J. P., Zhang, H., Morse, E., et al. (2014). Disruption of CXCR2-mediated MDSC tumor trafficking enhances anti-PD1 efficacy. Sci. Transl. Med. 6:237ra267. doi: 10.1126/scitranslmed.3007974
Hirsch, L., Flippot, R., Escudier, B., and Albiges, L. (2020). Immunomodulatory roles of VEGF pathway inhibitors in renal cell carcinoma. Drugs 80, 1169–1181. doi: 10.1007/s40265-020-01327-7
Hodi, F. S., O’Day, S. J., McDermott, D. F., Weber, R. W., Sosman, J. A., Haanen, J. B., et al. (2010). Improved survival with Ipilimumab in patients with metastatic melanoma. N. Engl. J. Med. 363, 711–723. doi: 10.1056/NEJMoa1003466
Hoechst, B., Gamrekelashvili, J., Manns, M. P., Greten, T. F., and Korangy, F. (2011). Plasticity of human Th17 cells and iTregs is orchestrated by different subsets of myeloid cells. Blood 117, 6532–6541. doi: 10.1182/blood-2010-11-317321
Holmgaard, R. B., Brachfeld, A., Gasmi, B., Jones, D. R., Mattar, M., Doman, T., et al. (2016). Timing of CSF-1/CSF-1R signaling blockade is critical to improving responses to CTLA-4 based immunotherapy. Oncoimmunology 5, e1151595. doi: 10.1080/2162402X.2016.1151595
Hong, D. S., Schoffski, P., Calvo, A., Sarantopoulos, J., Ochoa De Olza, M., Carvajal, R. D., et al. (2018). Phase I/II study of LAG525 ± spartalizumab (PDR001) in patients (pts) with advanced malignancies. J. Clin. Oncol. 36:3012. doi: 10.1200/JCO.2018.36.15_suppl.3012
Horinaka, A., Sakurai, D., Ihara, F., Makita, Y., Kunii, N., Motohashi, S., et al. (2016). Invariant NKT cells are resistant to circulating CD15+ myeloid-derived suppressor cells in patients with head and neck cancer. Cancer Sci. 107, 207–216. doi: 10.1111/cas.12866
Horn, L. A., Riskin, J., Hempel, H. A., Fousek, K., Lind, H., Hamilton, D. H., et al. (2020). Simultaneous inhibition of CXCR1/2, TGF-β, and PD-L1 remodels the tumor and its microenvironment to drive antitumor immunity. J. Immunother. Cancer 8:e000326. doi: 10.1136/jitc-2019-000326
Hou, W., Sampath, P., Rojas, J. J., and Thorne, S. H. (2016). Oncolytic Virus-mediated targeting of PGE2 in the tumor alters the immune status and sensitizes established and resistant tumors to immunotherapy. Cancer Cell 30, 108–119. doi: 10.1016/j.ccell.2016.05.012
Hsu, F.-T., Chen, T.-C., Chuang, H.-Y., Chang, Y.-F., and Hwang, J.-J. (2015). Enhancement of adoptive T cell transfer with single low dose pretreatment of doxorubicin or paclitaxel in mice. Oncotarget 6, 44134–44150. doi: 10.18632/oncotarget.6628
Hu, C.-E., Gan, J., Zhang, R.-D., Cheng, Y.-R., and Huang, G.-J. (2011). Up-regulated myeloid-derived suppressor cell contributes to hepatocellular carcinoma development by impairing dendritic cell function. Scand. J. Gastroenterol. 46, 156–164. doi: 10.3109/00365521.2010.516450
Hu, Z., Ott, P. A., and Wu, C. J. (2018). Towards personalized, tumour-specific, therapeutic vaccines for cancer. Nat. Rev. Immunol. 18, 168–182. doi: 10.1038/nri.2017.131
Huang, S., Zhou, N., Zhao, L., Gimple, R. C., Ahn, Y. H., Zhang, P., et al. (2020). Pharmacological activation of estrogen receptor beta overcomes tumor resistance to immune checkpoint blockade therapy. iScience 23:101458. doi: 10.1016/j.isci.2020.101458
Huard, B., Tournier, M., Hercend, T., Triebel, F., and Faure, F. (1994). Lymphocyte-activation gene 3/major histocompatibility complex class II interaction modulates the antigenic response of CD4+ T lymphocytes. Eur. J. Immunol. 24, 3216–3221. doi: 10.1002/eji.1830241246
Hughes, A., Clarson, J., Tang, C., Vidovic, L., White, D. L., Hughes, T. P., et al. (2017). CML patients with deep molecular responses to TKI have restored immune effectors and decreased PD-1 and immune suppressors. Blood 129, 1166–1176. doi: 10.1182/blood-2016-10-745992
Iannone, R., Miele, L., Maiolino, P., Pinto, A., and Morello, S. (2014). Adenosine limits the therapeutic effectiveness of anti-CTLA4 mAb in a mouse melanoma model. Am. J. Cancer Res. 4, 172–181.
Iclozan, C., Antonia, S., Chiappori, A., Chen, D.-T., and Gabrilovich, D. (2013). Therapeutic regulation of myeloid-derived suppressor cells and immune response to cancer vaccine in patients with extensive stage small cell lung cancer. Cancer Immunol. Immunother. 62, 909–918. doi: 10.1007/s00262-013-1396-8
Innamarato, P., Kodumudi, K., Asby, S., Schachner, B., Hall, M., Mackay, A., et al. (2020). Reactive myelopoiesis triggered by lymphodepleting chemotherapy limits the efficacy of adoptive T cell therapy. Mol. Ther. 28, 2252–2270. doi: 10.1016/j.ymthe.2020.06.025
Iwamoto, H., Izumi, K., and Mizokami, A. (2020). Is the C-C Motif Ligand 2–C-C chemokine receptor 2 axis a promising target for cancer therapy and diagnosis? Int. J. Mol. Sci. 21:9328. doi: 10.3390/ijms21239328
Jadidi-Niaragh, F., Atyabi, F., Rastegari, A., Kheshtchin, N., Arab, S., Hassannia, H., et al. (2017). CD73 specific siRNA loaded chitosan lactate nanoparticles potentiate the antitumor effect of a dendritic cell vaccine in 4T1 breast cancer bearing mice. J. Control. Release 246, 46–59. doi: 10.1016/j.jconrel.2016.12.012
Jayakumar, A., and Bothwell, A. L. M. (2019). Functional diversity of myeloid-derived suppressor cells: the multitasking hydra of cancer. J. Immunol. 203, 1095–1103. doi: 10.4049/jimmunol.1900500
Jia, W., Jackson-Cook, C., and Graf, M. R. (2010). Tumor-infiltrating, myeloid-derived suppressor cells inhibit T cell activity by nitric oxide production in an intracranial rat glioma+vaccination model. J. Neuroimmunol. 223, 20–30. doi: 10.1016/j.jneuroim.2010.03.011
Jiang, P., Gu, S., Pan, D., Fu, J., Sahu, A., Hu, X., et al. (2018). Signatures of T cell dysfunction and exclusion predict cancer immunotherapy response. Nat. Med. 24, 1550–1558. doi: 10.1038/s41591-018-0136-1
Jitschin, R., Saul, D., Braun, M., Tohumeken, S., Völkl, S., Kischel, R., et al. (2018). CD33/CD3-bispecific T-cell engaging (BiTE®) antibody construct targets monocytic AML myeloid-derived suppressor cells. J. Immunother. Cancer 6, 116. doi: 10.1186/s40425-018-0432-9
Johnson, M. L., Gonzalez, R., Opyrchal, M., Gabrilovich, D., Ordentlich, P., Brouwer, S., et al. (2017). ENCORE 601: a phase II study of entinostat (ENT) in combination with pembrolizumab (PEMBRO) in patients with melanoma. J. Clin. Oncol 35:9529. doi: 10.1200/JCO.2017.35.15_suppl.9529
Johnston, R. J., Comps-Agrar, L., Hackney, J., Yu, X., Huseni, M., Yang, Y., et al. (2014). The Immunoreceptor TIGIT Regulates antitumor and antiviral CD8 (+) T cell effector function. Cancer Cell 26, 923–937. doi: 10.1016/j.ccell.2014.10.018
Joller, N., Hafler, J. P., Brynedal, B., Kassam, N., Spoerl, S., Levin, S. D., et al. (2011). Cutting edge: TIGIT Has T cell-intrinsic inhibitory functions. J. Immunol. 186, 1338–1342. doi: 10.4049/jimmunol.1003081
Kaneda, M. M., Messer, K. S., Ralainirina, N., Li, H., Leem, C. J., Gorjestani, S., et al. (2016). PI3Kγ is a molecular switch that controls immune suppression. Nature 539, 437–442. doi: 10.1038/nature19834
Kantoff, P. W., Higano, C. S., Shore, N. D., Berger, E. R., Small, E. J., Penson, D. F., et al. (2010). Sipuleucel-T immunotherapy for castration-resistant prostate cancer. N. Engl. J. Med. 363, 411–422. doi: 10.1056/NEJMoa1001294
Kaufman, H. L., Kohlhapp, F. J., and Zloza, A. (2015). Oncolytic viruses: a new class of immunotherapy drugs. Nat. Rev. Drug Discov. 14, 642–662. doi: 10.1038/nrd4663
Kearney, C. J., and Martin, S. J. (2017). An inflammatory perspective on necroptosis. Mol. Cell 65, 965–973. doi: 10.1016/j.molcel.2017.02.024
Keshavarz, M., Ebrahimzadeh, M. S., Miri, S. M., Dianat-Moghadam, H., Ghorbanhosseini, S. S., Mohebbi, S. R., et al. (2020). Oncolytic Newcastle disease virus delivered by Mesenchymal stem cells-engineered system enhances the therapeutic effects altering tumor microenvironment. Virol. J. 17:64. doi: 10.1186/s12985-020-01326-w
Kim, H. R., Park, S.-M., Seo, S.-U., Jung, I., Yoon, H. I., Gabrilovich, D. I., et al. (2018). The ratio of peripheral regulatory T Cells to Lox-1+ Polymorphonuclear myeloid-derived suppressor cells predicts the early response to Anti–PD-1 therapy in patients with non–small cell lung cancer. Am. J. Respir. Crit. Care Med. 199, 243–246. doi: 10.1164/rccm.201808-1502LE
Kim, J. W., Bellmunt, J., Powles, T., Loriot, Y., Vogelzang, N. J., Cruz Zambrano, C., et al. (2015). Clinical activity, safety, and biomarkers of MPDL3280A in metastatic urothelial bladder cancer: additional analysis from phase IA study. J. Clin. Oncol. 33:297. doi: 10.1200/jco.2015.33.7_suppl.297
Kim, Y.-D., Park, S.-M., Ha, H. C., Lee, A. R., Won, H., Cha, H., et al. (2020). HDAC Inhibitor, CG-745, enhances the anti-cancer effect of anti-pd-1 immune checkpoint inhibitor by modulation of the immune microenvironment. J. Cancer 11, 4059–4072. doi: 10.7150/jca.44622
Kimura, T., McKolanis, J. R., Dzubinski, L. A., Islam, K., Potter, D. M., Salazar, A. M., et al. (2013). MUC1 vaccine for individuals with advanced adenoma of the colon: a cancer immunoprevention feasibility study. Cancer Prev. Res. 6, 18–26. doi: 10.1158/1940-6207.CAPR-12-0275
Kitano, S., Postow, M. A., Ziegler, C. G. K., Kuk, D., Panageas, K. S., Cortez, C., et al. (2014). Computational algorithm-driven evaluation of monocytic myeloid-derived suppressor cell frequency for prediction of clinical outcomes. Cancer Immunol. Res. 2, 812–821. doi: 10.1158/2326-6066.CIR-14-0013
Ko, H.-J., Lee, J.-M., Kim, Y.-J., Kim, Y.-S., Lee, K.-A., and Kang, C.-Y. (2009). Immunosuppressive myeloid-derived suppressor cells can be converted into immunogenic APCs with the help of activated NKT Cells: an alternative cell-based antitumor vaccine. J. Immunol. 182, 1818–1828. doi: 10.4049/jimmunol.0802430
Kongsted, P., Borch, T. H., Ellebaek, E., Iversen, T. Z., Andersen, R., and Met, Ö, et al. (2017). Dendritic cell vaccination in combination with docetaxel for patients with metastatic castration-resistant prostate cancer: a randomized phase II study. Cytotherapy 19, 500–513. doi: 10.1016/j.jcyt.2017.01.007
Kowanetz, M., Wu, X., Lee, J., Tan, M., Hagenbeek, T., Qu, X., et al. (2010). Granulocyte-colony stimulating factor promotes lung metastasis through mobilization of Ly6G+Ly6C+ granulocytes. Proc. Natl. Acad. Sci. U.S.A. 107, 21248–21255. doi: 10.1073/pnas.1015855107
Kowanetz, M., Zou, W., Gettinger, S. N., Koeppen, H., Kockx, M., Schmid, P., et al. (2018). Differential regulation of PD-L1 expression by immune and tumor cells in NSCLC and the response to treatment with atezolizumab (anti–PD-L1). Proc. Natl. Acad. Sci. U.S.A. 115, E10119–E10126. doi: 10.1073/pnas.1802166115
Koyama, S., Akbay, E. A., Li, Y. Y., Herter-Sprie, G. S., Buczkowski, K. A., Richards, W. G., et al. (2016). Adaptive resistance to therapeutic PD-1 blockade is associated with upregulation of alternative immune checkpoints. Nat. Commun. 7:10501. doi: 10.1038/ncomms10501
Ku, A. W., Muhitch, J. B., Powers, C. A., Diehl, M., Kim, M., Fisher, D. T., et al. (2016). Tumor-induced MDSC act via remote control to inhibit L-selectin-dependent adaptive immunity in lymph nodes. eLife 5:e17375. doi: 10.7554/eLife.17375
Kumar, V., Patel, S., Tcyganov, E., and Gabrilovich, D. I. (2016). The nature of myeloid-derived suppressor cells in the tumor microenvironment. Trends Immunol. 37, 208–220. doi: 10.1016/j.it.2016.01.004
Kwon, E. D., Drake, C. G., Scher, H. I., Fizazi, K., Bossi, A., van den Eertwegh, A. J. M., et al. (2014). Ipilimumab versus placebo after radiotherapy in patients with metastatic castration-resistant prostate cancer that had progressed after docetaxel chemotherapy (CA184-043): a multicentre, randomised, double-blind, phase 3 trial. Lancet Oncol. 15, 700–712. doi: 10.1016/S1470-2045(14)70189-5
Laborde, R. R., Lin, Y., Gustafson, M. P., Bulur, P. A., and Dietz, A. B. (2014). Cancer vaccines in the world of immune suppressive monocytes (CD14(+)HLA-DR(lo/neg) Cells): the gateway to improved responses. Front. Immunol. 5:147. doi: 10.3389/fimmu.2014.00147
Lan, J., Li, R., Yin, L.-M., Deng, L., Gui, J., Chen, B.-Q., et al. (2018). Targeting myeloid-derived suppressor cells and programmed death Ligand 1 confers therapeutic advantage of ablative hypofractionated radiation therapy compared with conventional fractionated radiation therapy. Int. J. Radiat. Oncol. Biol. Phys. 101, 74–87. doi: 10.1016/j.ijrobp.2018.01.071
Lee, C.-R., Kwak, Y., Yang, T., Han, J. H., Park, S.-H., Ye, M. B., et al. (2016). Myeloid-derived suppressor cells are controlled by regulatory T cells via TGF-β during Murine Colitis. Cell Rep. 17, 3219–3232. doi: 10.1016/j.celrep.2016.11.062
Li, H., Ding, J., Lu, M., Liu, H., Miao, Y., Li, L., et al. (2020). CAIX-specific CAR-T cells and sunitinib show synergistic effects against metastatic renal cancer models. J. Immunother 43, 16–28.
Li, J., Wang, L., Chen, X., Li, L., Li, Y., Ping, Y., et al. (2017). CD39/CD73 upregulation on myeloid-derived suppressor cells via TGF-β-mTOR-HIF-1 signaling in patients with non-small cell lung cancer. Oncoimmunology 6:e1320011. doi: 10.1080/2162402X.2017.1320011
Li, L., Cao, B., Liang, X., Lu, S., Luo, H., Wang, Z., et al. (2019). Microenvironmental oxygen pressure orchestrates an anti- and pro-tumoral γδ T cell equilibrium via tumor-derived exosomes. Oncogene 38, 2830–2843. doi: 10.1038/s41388-018-0627-z
Li, L., Wang, L., Li, J., Fan, Z., Yang, L., Zhang, Z., et al. (2018). Metformin-induced reduction of CD39 and CD73 blocks myeloid-derived suppressor cell activity in patients with ovarian cancer. Cancer Res. 78, 1779–1791. doi: 10.1158/0008-5472.CAN-17-2460
Li, X., Shao, C., Shi, Y., and Han, W. (2018). Lessons learned from the blockade of immune checkpoints in cancer immunotherapy. J. Hematol. Oncol. 11:31. doi: 10.1186/s13045-018-0578-4
Li, Y.-M., Liu, Z.-Y., Wang, J.-C., Yu, J.-M., Li, Z.-C., Yang, H.-J., et al. (2019). Receptor-interacting protein Kinase 3 deficiency recruits myeloid-derived suppressor cells to hepatocellular carcinoma through the chemokine (C-X-C Motif) Ligand 1–Chemokine (C-X-C Motif) Receptor 2 Axis. Hepatology 70, 1564–1581. doi: 10.1002/hep.30676
Liang, H., Deng, L., Hou, Y., Meng, X., Huang, X., Rao, E., et al. (2017). Host STING-dependent MDSC mobilization drives extrinsic radiation resistance. Nat. Commun. 8:1736. doi: 10.1038/s41467-017-01566-5
Liao, W., Overman, M. J., Boutin, A. T., Shang, X., Zhao, D., Dey, P., et al. (2019). KRAS-IRF2 axis drives immune suppression and immune therapy resistance in colorectal cancer. Cancer Cell 35, 559–572.e7. doi: 10.1016/j.ccell.2019.02.008
Limagne, E., Richard, C., Thibaudin, M., Fumet, J.-D., Truntzer, C., Lagrange, A., et al. (2019). Tim-3/galectin-9 pathway and mMDSC control primary and secondary resistances to PD-1 blockade in lung cancer patients. Oncoimmunology 8:e1564505. doi: 10.1080/2162402X.2018.1564505
Liu, Y., Wei, J., Guo, G., and Zhou, J. (2015). Norepinephrine-induced myeloid-derived suppressor cells block T-cell responses via generation of reactive oxygen species. Immunopharmacol. Immunotoxicol. 37, 359–365. doi: 10.3109/08923973.2015.1059442
Liu, Z., Xie, Y., Xiong, Y., Liu, S., Qiu, C., Zhu, Z., et al. (2020). TLR 7/8 agonist reverses oxaliplatin resistance in colorectal cancer via directing the myeloid-derived suppressor cells to tumoricidal M1-macrophages. Cancer Lett. 469, 173–185. doi: 10.1016/j.canlet.2019.10.020
Long, Y., Lu, Z., Xu, S., Li, M., Wang, X., Zhang, Z., et al. (2020). Self-delivery micellar nanoparticles prevent premetastatic niche formation by interfering with the early recruitment and vascular destruction of granulocytic myeloid-derived suppressor cells. Nano Lett. 20, 2219–2229. doi: 10.1021/acs.nanolett.9b03883
Lu, X., Horner, J. W., Paul, E., Shang, X., Troncoso, P., Deng, P., et al. (2017a). Effective combinatorial immunotherapy for castration-resistant prostate cancer. Nature 543, 728–732. doi: 10.1038/nature21676
Lu, X., Yang, L., Yao, D., Wu, X., Li, J., Liu, X., et al. (2017b). Tumor antigen-specific CD8+ T cells are negatively regulated by PD-1 and Tim-3 in human gastric cancer. Cell. Immunol. 313, 43–51. doi: 10.1016/j.cellimm.2017.01.001
Lu, Z., Zou, J., Li, S., Topper, M. J., Tao, Y., Zhang, H., et al. (2020). Epigenetic therapy inhibits metastases by disrupting premetastatic niches. Nature 579, 284–290. doi: 10.1038/s41586-020-2054-x
Lynch, T. J., Bondarenko, I., Luft, A., Serwatowski, P., Barlesi, F., Chacko, R., et al. (2012). Ipilimumab in combination with paclitaxel and carboplatin as first-line treatment in Stage IIIB/IV non–small-cell lung cancer: results from a randomized, double-blind, multicenter phase II study. J. Clin. Oncol. 30, 2046–2054. doi: 10.1200/JCO.2011.38.4032
Ma, S., Cheng, Q., Cai, Y., Gong, H., Wu, Y., Yu, X., et al. (2014). IL-17A produced by γδ T cells promotes tumor growth in hepatocellular carcinoma. Cancer Res. 74, 1969–1982. doi: 10.1158/0008-5472.CAN-13-2534
Macedo, N., Miller, D. M., Haq, R., and Kaufman, H. L. (2020). Clinical landscape of oncolytic virus research in 2020. J. Immunother. Cancer 8:e001486. doi: 10.1136/jitc-2020-001486
Mackey, J. B. G., Coffelt, S. B., and Carlin, L. M. (2019). Neutrophil maturity in cancer. Front. Immunol. 10:1912. doi: 10.3389/fimmu.2019.01912
Mao, Y., Eissler, N., Blanc, K. L., Johnsen, J. I., Kogner, P., and Kiessling, R. (2016). Targeting suppressive myeloid cells potentiates checkpoint inhibitors to control spontaneous neuroblastoma. Clin. Cancer Res. 22, 3849–3859. doi: 10.1158/1078-0432.CCR-15-1912
Mao, Y., Sarhan, D., Steven, A., Seliger, B., Kiessling, R., and Lundqvist, A. (2014). Inhibition of tumor-derived prostaglandin-E2 blocks the induction of myeloid-derived suppressor cells and recovers natural killer cell activity. Clin. Cancer Res. 20, 4096–4106. doi: 10.1158/1078-0432.CCR-14-0635
Markowitz, J., Wang, J., Vangundy, Z., You, J., Yildiz, V., Yu, L., et al. (2017). Nitric oxide mediated inhibition of antigen presentation from DCs to CD4+ T cells in cancer and measurement of STAT1 nitration. Sci. Rep. 7:15424. doi: 10.1038/s41598-017-14970-0
Martens, A., Wistuba-Hamprecht, K., Foppen, M. G., Yuan, J., Postow, M. A., Wong, P., et al. (2016). Baseline peripheral blood biomarkers associated with clinical outcome of advanced melanoma patients treated with Ipilimumab. Clin. Cancer Res. 22, 2908–2918. doi: 10.1158/1078-0432.CCR-15-2412
Martin del Campo, S. E., Levine, K. M., Mundy-Bosse, B. L., Grignol, V. P., Fairchild, E. T., Campbell, A. R., et al. (2015). The Raf Kinase Inhibitor Sorafenib Inhibits JAK–STAT signal transduction in human immune cells. J. Immunol. 195, 1995–2005. doi: 10.4049/jimmunol.1400084
Martini, M., De Santis, M. C., Braccini, L., Gulluni, F., and Hirsch, E. (2014). PI3K/AKT signaling pathway and cancer: an updated review. Ann. Med. 46, 372–383. doi: 10.3109/07853890.2014.912836
Maruhashi, T., Sugiura, D., Okazaki, I.-M., and Okazaki, T. (2020). LAG-3: from molecular functions to clinical applications. J. Immunother. Cancer 8:e001014. doi: 10.1136/jitc-2020-001014
Mastelic-Gavillet, B., Navarro Rodrigo, B., Décombaz, L., Wang, H., Ercolano, G., Ahmed, R., et al. (2019). Adenosine mediates functional and metabolic suppression of peripheral and tumor-infiltrating CD8 + T cells. J. Immunother. Cancer 7:257. doi: 10.1186/s40425-019-0719-5
Mastio, J., Condamine, T., Dominguez, G., Kossenkov, A. V., Donthireddy, L., Veglia, F., et al. (2019). Identification of monocyte-like precursors of granulocytes in cancer as a mechanism for accumulation of PMN-MDSCs. J. Exp. Med. 216, 2150–2169. doi: 10.1084/jem.20181952
Melaiu, O., Lucarini, V., Giovannoni, R., Fruci, D., and Gemignani, F. (2020). News on immune checkpoint inhibitors as immunotherapy strategies in adult and pediatric solid tumors. Semin. Cancer Biol. doi: 10.1016/j.semcancer.2020.07.001 [Epub ahead of print].
Meng, G., Li, B., Chen, A., Zheng, M., Xu, T., Zhang, H., et al. (2020). Targeting aerobic glycolysis by dichloroacetate improves Newcastle disease virus-mediated viro-immunotherapy in hepatocellular carcinoma. Br. J. Cancer 122, 111–120. doi: 10.1038/s41416-019-0639-7
Mengos, A. E., Gastineau, D. A., and Gustafson, M. P. (2019). The CD14(+)HLA-DR(lo/neg) Monocyte: an immunosuppressive phenotype that restrains responses to cancer immunotherapy. Front. Immunol. 10:1147. doi: 10.3389/fimmu.2019.01147
Meyer, C., Cagnon, L., Costa-Nunes, C. M., Baumgaertner, P., Montandon, N., Leyvraz, L., et al. (2014). Frequencies of circulating MDSC correlate with clinical outcome of melanoma patients treated with ipilimumab. Cancer Immunol. Immunother. 63, 247–257. doi: 10.1007/s00262-013-1508-5
Miret, J. J., Kirschmeier, P., Koyama, S., Zhu, M., Li, Y. Y., Naito, Y., et al. (2019). Suppression of myeloid cell arginase activity leads to therapeutic response in a NSCLC mouse model by activating anti-tumor immunity. J. Immunother. Cancer 7:32. doi: 10.1186/s40425-019-0504-5
Mittal, D., Young, A., Stannard, K., Yong, M., Teng, M. W. L., Allard, B., et al. (2014). Antimetastatic effects of blocking PD-1 and the adenosine A2A receptor. Cancer Res. 74, 3652–3658. doi: 10.1158/0008-5472.CAN-14-0957
Najjar, Y. G., Rayman, P., Jia, X., Pavicic, P. G., Rini, B. I., Tannenbaum, C., et al. (2017). Myeloid-derived suppressor cell subset accumulation in renal cell carcinoma parenchyma is associated with intratumoral expression of IL1β, IL8, CXCL5, and Mip-1α. Clin. Cancer Res. 23, 2346–2355. doi: 10.1158/1078-0432.CCR-15-1823
Neumann, S., Shirley, S. A., Kemp, R. A., and Hook, S. M. (2016). Improved antitumor activity of a therapeutic melanoma vaccine through the use of the dual COX-2/5-LO inhibitor licofelone. Front. Immunol. 7:537. doi: 10.3389/fimmu.2016.00537
Obermajer, N., and Kalinski, P. (2012). Generation of myeloid-derived suppressor cells using prostaglandin E2. Transplant. Res. 1:15. doi: 10.1186/2047-1440-1-15
Obermajer, N., Muthuswamy, R., Odunsi, K., Edwards, R. P., and Kalinski, P. (2011). PGE(2)-induced CXCL12 production and CXCR4 expression controls the accumulation of human MDSCs in ovarian cancer environment. Cancer Res. 71, 7463–7470. doi: 10.1158/0008-5472.CAN-11-2449
Ochoa de Olza, M., Navarro Rodrigo, B., Zimmermann, S., and Coukos, G. (2020). Turning up the heat on non-immunoreactive tumours: opportunities for clinical development. Lancet Oncol. 21, e419–e430. doi: 10.1016/S1470-2045(20)30234-5
O’Neill, L. A. J., Golenbock, D., and Bowie, A. G. (2013). The history of Toll-like receptors — redefining innate immunity. Nat. Rev. Immunol. 13, 453–460. doi: 10.1038/nri3446
Ostrand-Rosenberg, S., and Fenselau, C. (2018). Myeloid-derived suppressor cells: immune-suppressive cells that impair antitumor immunity and are sculpted by their environment. J. Immunol. 200, 422–431. doi: 10.4049/jimmunol.1701019
Ostrand-Rosenberg, S., Sinha, P., Beury, D. W., and Clements, V. K. (2012). Cross-talk between myeloid-derived suppressor cells (MDSC), macrophages, and dendritic cells enhances tumor-induced immune suppression. Semin. Cancer Biol. 22, 275–281. doi: 10.1016/j.semcancer.2012.01.011
Park, J. H., Rivière, I., Gonen, M., Wang, X., Sénéchal, B., Curran, K. J., et al. (2018). Long-term follow-up of CD19 CAR therapy in acute lymphoblastic leukemia. N. Engl. J. Med. 378, 449–459. doi: 10.1056/NEJMoa1709919
Parker, K. H., Beury, D. W., and Ostrand-Rosenberg, S. (2015). “Chapter Three - myeloid-derived suppressor cells: critical cells driving immune suppression in the tumor microenvironment,” in Advances in Cancer Research, Vol. 128, eds X.-Y. Wang and P. B. Fisher (Cambridge, MA: Academic Press), 95–139.
Payne, N. L., Sun, G., McDonald, C., Moussa, L., Emerson-Webber, A., Loisel-Meyer, S., et al. (2013). Human adipose-derived mesenchymal stem cells engineered to secrete IL-10 inhibit APC function and limit CNS autoimmunity. Brain Behav. Immun. 30, 103–114. doi: 10.1016/j.bbi.2013.01.079
Pellicci, D. G., Koay, H.-F., and Berzins, S. P. (2020). Thymic development of unconventional T cells: how NKT cells, MAIT cells and γδ T cells emerge. Nat. Rev. Immunol. 20, 756–770. doi: 10.1038/s41577-020-0345-y
Pérez-Ruiz, E., Melero, I., Kopecka, J., Sarmento-Ribeiro, A. B., García-Aranda, M., and De Las Rivas, J. (2020). Cancer immunotherapy resistance based on immune checkpoints inhibitors: targets, biomarkers, and remedies. Drug Resist. Updat. 53:100718. doi: 10.1016/j.drup.2020.100718
Perica, K., Varela, J. C., Oelke, M., and Schneck, J. (2015). Adoptive T cell immunotherapy for cancer. Rambam Maimonides Med. J. 6:e0004. doi: 10.5041/RMMJ.10179
Pericle, F., Liu, J. H., Diaz, J. I., Blanchard, D. K., Wei, S., Forni, G., et al. (1994). Interleukin-2 prevention of apoptosis in human neutrophils. Eur. J. Immunol. 24, 440–444.
Peters, S., Cho, B. C., Reinmuth, N., Lee, K. H., Luft, A., Ahn, M.-J., et al. (2019a). Abstract CT074: tumor mutational burden (TMB) as a biomarker of survival in metastatic non-small cell lung cancer (mNSCLC): blood and tissue TMB analysis from MYSTIC, a Phase III study of first-line durvalumab ± tremelimumab vs chemotherapy. Cancer Res. 79(13 Suppl.), CT074. doi: 10.1158/1538-7445.AM2019-CT074
Peters, S., Ramalingam, S. S., Paz-Ares, L., Bernabe Caro, R., Zurawski, B., Kim, S. W., et al. (2019b). LBA4_PR - Nivolumab (NIVO) + low-dose ipilimumab (IPI) vs platinum-doublet chemotherapy (chemo) as first-line (1L) treatment (tx) for advanced non-small cell lung cancer (NSCLC): CheckMate 227 part 1 final analysis. Ann. Oncol. 30, v913–v914. doi: 10.1093/annonc/mdz394.075
Poschke, I., Mao, Y., Adamson, L., Salazar-Onfray, F., Masucci, G., and Kiessling, R. (2012). Myeloid-derived suppressor cells impair the quality of dendritic cell vaccines. Cancer Immunol. Immunother. 61, 827–838. doi: 10.1007/s00262-011-1143-y
Powles, T., Durán, I., van der Heijden, M. S., Loriot, Y., Vogelzang, N. J., De Giorgi, U., et al. (2018). Atezolizumab versus chemotherapy in patients with platinum-treated locally advanced or metastatic urothelial carcinoma (IMvigor211): a multicentre, open-label, phase 3 randomised controlled trial. Lancet 391, 748–757. doi: 10.1016/S0140-6736(17)33297-X
Raber, P. L., Sierra, R. A., Thevenot, P. T., Shuzhong, Z., Wyczechowska, D. D., Kumai, T., et al. (2016). T cells conditioned with MDSC show an increased anti-tumor activity after adoptive T cell based immunotherapy. Oncotarget 7, 17565–17578. doi: 10.18632/oncotarget.8197
Reck, M., Bondarenko, I., Luft, A., Serwatowski, P., Barlesi, F., Chacko, R., et al. (2013). Ipilimumab in combination with paclitaxel and carboplatin as first-line therapy in extensive-disease-small-cell lung cancer: results from a randomized, double-blind, multicenter phase 2 trial†. Ann. Oncol. 24, 75–83. doi: 10.1093/annonc/mds213
Reinhardt, J., Landsberg, J., Schmid-Burgk, J. L., Ramis, B. B., Bald, T., Glodde, N., et al. (2017). MAPK signaling and inflammation link melanoma phenotype switching to induction of CD73 during immunotherapy. Cancer Res. 77, 4697–4709. doi: 10.1158/0008-5472.CAN-17-0395
Robert, C., Long, G. V., Brady, B., Dutriaux, C., Maio, M., Mortier, L., et al. (2014). Nivolumab in previously untreated melanoma without BRAF mutation. N. Engl. J. Med. 372, 320–330. doi: 10.1056/NEJMoa1412082
Robert, C., Schachter, J., Long, G. V., Arance, A., Grob, J. J., Mortier, L., et al. (2015). Pembrolizumab versus Ipilimumab in advanced melanoma. N. Engl. J. Med. 372, 2521–2532. doi: 10.1056/NEJMoa1503093
Rodell, C. B., Arlauckas, S. P., Cuccarese, M. F., Garris, C. S., Li, R., Ahmed, M. S., et al. (2018). TLR7/8-agonist-loaded nanoparticles promote the polarization of tumour-associated macrophages to enhance cancer immunotherapy. Nat. Biomed. Eng. 2, 578–588. doi: 10.1038/s41551-018-0236-8
Rosenberg, S. A. (2011). Cell transfer immunotherapy for metastatic solid cancer–what clinicians need to know. Nat. Rev. Clin. Oncol. 8, 577–585. doi: 10.1038/nrclinonc.2011.116
Rosenberg, S. A. (2014). IL-2: the first effective immunotherapy for human cancer. J. Immunol. 192, 5451–5458. doi: 10.4049/jimmunol.1490019
Rosenberg, S. A., and Restifo, N. P. (2015). Adoptive cell transfer as personalized immunotherapy for human cancer. Science 348, 62–68. doi: 10.1126/science.aaa4967
Rosenberg, S. A., Yang, J. C., Sherry, R. M., Kammula, U. S., Hughes, M. S., Phan, G. Q., et al. (2011). Durable complete responses in heavily pretreated patients with metastatic melanoma using T-cell transfer immunotherapy. Clin. Cancer Res. 17, 4550–4557. doi: 10.1158/1078-0432.CCR-11-0116
Samstein, R. M., Lee, C.-H., Shoushtari, A. N., Hellmann, M. D., Shen, R., Janjigian, Y. Y., et al. (2019). Tumor mutational load predicts survival after immunotherapy across multiple cancer types. Nat. Genet. 51, 202–206. doi: 10.1038/s41588-018-0312-8
Schlecker, E., Stojanovic, A., Eisen, C., Quack, C., Falk, C. S., Umansky, V., et al. (2012). Tumor-infiltrating monocytic myeloid-derived suppressor cells mediate CCR5-dependent recruitment of regulatory T cells favoring tumor growth. J. Immunol. 189, 5602–5611. doi: 10.4049/jimmunol.1201018
Schouppe, E., Mommer, C., Movahedi, K., Laoui, D., Morias, Y., Gysemans, C., et al. (2013). Tumor-induced myeloid-derived suppressor cell subsets exert either inhibitory or stimulatory effects on distinct CD8+ T-cell activation events. Eur. J. Immunol. 43, 2930–2942. doi: 10.1002/eji.201343349
Sevko, A., Michels, T., Vrohlings, M., Umansky, L., Beckhove, P., Kato, M., et al. (2013). Antitumor effect of paclitaxel is mediated by inhibition of myeloid-derived suppressor cells and chronic inflammation in the spontaneous melanoma model. J. Immunol. 190, 2464–2471. doi: 10.4049/jimmunol.1202781
Shayan, G., Kansy, B. A., Gibson, S. P., Srivastava, R. M., Bryan, J. K., Bauman, J. E., et al. (2018). Phase Ib study of immune biomarker modulation with neoadjuvant cetuximab and TLR8 stimulation in head and neck cancer to overcome suppressive myeloid signals. Clin. Cancer Res. 24, 62–72. doi: 10.1158/1078-0432.CCR-17-0357
Shayan, G., Srivastava, R., Li, J., Schmitt, N., Kane, L. P., and Ferris, R. L. (2017). Adaptive resistance to anti-PD1 therapy by Tim-3 upregulation is mediated by the PI3K-Akt pathway in head and neck cancer. Oncoimmunology 6:e1261779. doi: 10.1080/2162402X.2016.1261779
Shirota, Y., Shirota, H., and Klinman, D. M. (2012). Intratumoral injection of CpG oligonucleotides induces the differentiation and reduces the immunosuppressive activity of myeloid-derived suppressor cells. J. Immunol. 188, 1592–1599. doi: 10.4049/jimmunol.1101304
Shukuya, T., and Carbone, D. P. (2016). Predictive markers for the efficacy of Anti-PD-1/PD-L1 antibodies in lung cancer. J. Thorac. Oncol. 11, 976–988. doi: 10.1016/j.jtho.2016.02.015
Sim, G. C., and Radvanyi, L. (2014). The IL-2 cytokine family in cancer immunotherapy. Cytokine Growth Factor Rev. 25, 377–390. doi: 10.1016/j.cytogfr.2014.07.018
Siret, C., Collignon, A., Silvy, F., Robert, S., Cheyrol, T., André, P., et al. (2020). Deciphering the crosstalk between myeloid-derived suppressor cells and regulatory T cells in pancreatic Ductal Adenocarcinoma. Front. Immunol. 10:3070. doi: 10.3389/fimmu.2019.03070
SITC (2018). 33rd annual meeting & pre-conference programs of the Society For Immunotherapy of Cancer (SITC 2018). J. Immunother. Cancer 6:115. doi: 10.1186/s40425-018-0423-x
Smyth, M. J., Ngiow, S. F., Ribas, A., and Teng, M. W. L. (2016). Combination cancer immunotherapies tailored to the tumour microenvironment. Nat. Rev. Clin. Oncol. 13, 143–158. doi: 10.1038/nrclinonc.2015.209
Socinski, M. A., Jotte, R. M., Cappuzzo, F., Orlandi, F., Stroyakovskiy, D., Nogami, N., et al. (2018). Atezolizumab for first-line treatment of Metastatic Nonsquamous NSCLC. N. Engl. J. Med. 378, 2288–2301. doi: 10.1056/NEJMoa1716948
Song, R., and Struhl, K. (2021). S100A8/S100A9 cytokine acts as a transcriptional coactivator during breast cellular transformation. Sci. Adv. 7:eabe5357. doi: 10.1126/sciadv.abe5357
Srivastava, M. K., Sinha, P., Clements, V. K., Rodriguez, P., and Ostrand-Rosenberg, S. (2010). Myeloid-derived suppressor cells inhibit T-cell activation by depleting cystine and cysteine. Cancer Res. 70, 68–77. doi: 10.1158/0008-5472.CAN-09-2587
Steele, C. W., Karim, S. A., Leach, J. D. G., Bailey, P., Upstill-Goddard, R., Rishi, L., et al. (2016). CXCR2 inhibition profoundly suppresses metastases and augments immunotherapy in pancreatic Ductal Adenocarcinoma. Cancer Cell 29, 832–845. doi: 10.1016/j.ccell.2016.04.014
Stewart, T. J., and Smyth, M. J. (2011). Improving cancer immunotherapy by targeting tumor-induced immune suppression. Cancer Metastasis Rev. 30, 125–140. doi: 10.1007/s10555-011-9280-5
Stiff, A., Trikha, P., Mundy-Bosse, B., McMichael, E., Mace, T. A., Benner, B., et al. (2018). Nitric oxide production by myeloid-derived suppressor cells plays a role in impairing Fc receptor–mediated natural killer cell function. Clin. Cancer Res. 24, 1891–1904. doi: 10.1158/1078-0432.CCR-17-0691
Strauss, L., Sangaletti, S., Consonni, F. M., Szebeni, G., Morlacchi, S., Totaro, M. G., et al. (2015). RORC1 regulates tumor-promoting “Emergency” Granulo-Monocytopoiesis. Cancer Cell 28, 253–269. doi: 10.1016/j.ccell.2015.07.006
Sun, R., Luo, H., Su, J., Di, S., Zhou, M., Shi, B., et al. (2020). Olaparib suppresses MDSC Recruitment via SDF1α/CXCR4 axis to improve the anti-tumor efficacy of CAR-T cells on breast cancer in mice. Mol. Ther. 29, 60–74. doi: 10.1016/j.ymthe.2020.09.034
Takaku, S., Terabe, M., Ambrosino, E., Peng, J., Lonning, S., McPherson, J. M., et al. (2010). Blockade of TGF-beta enhances tumor vaccine efficacy mediated by CD8(+) T cells. Int. J. Cancer 126, 1666–1674. doi: 10.1002/ijc.24961
Tang, F., Tie, Y., Hong, W., Wei, Y., Tu, C., and Wei, X. (2021). Targeting myeloid-derived suppressor cells for Premetastatic niche disruption after tumor resection. Ann. Surg. Oncol. 28, 4030–4048. doi: 10.1245/s10434-020-09371-z
Tang, J., Yu, J. X., Hubbard-Lucey, V. M., Neftelinov, S. T., Hodge, J. P., and Lin, Y. (2018). The clinical trial landscape for PD1/PDL1 immune checkpoint inhibitors. Nat. Rev. Drug Discov. 17, 854–855. doi: 10.1038/nrd.2018.210
Tcyganov, E., Mastio, J., Chen, E., and Gabrilovich, D. I. (2018). Plasticity of myeloid-derived suppressor cells in cancer. Curr. Opin. Immunol. 51, 76–82. doi: 10.1016/j.coi.2018.03.009
Tesi, R. J. (2019). MDSC; the most important cell you have never heard of. Trends Pharmacol. Sci. 40, 4–7. doi: 10.1016/j.tips.2018.10.008
Thakur, A., Huang, M., and Lum, L. G. (2018). Bispecific antibody based therapeutics: strengths and challenges. Blood Rev. 32, 339–347. doi: 10.1016/j.blre.2018.02.004
Thevenot, P. T., Sierra, R. A., Raber, P. L., Al-Khami, A. A., Trillo-Tinoco, J., Zarreii, P., et al. (2014). The stress-response sensor chop regulates the function and accumulation of myeloid-derived suppressor cells in tumors. Immunity 41, 389–401. doi: 10.1016/j.immuni.2014.08.015
Tobin, R. P., Jordan, K. R., Robinson, W. A., Davis, D., Borges, V. F., Gonzalez, R., et al. (2018). Targeting myeloid-derived suppressor cells using all-trans retinoic acid in melanoma patients treated with Ipilimumab. Int. Immunopharmacol. 63, 282–291. doi: 10.1016/j.intimp.2018.08.007
Topalian, S. L., Hodi, F. S., Brahmer, J. R., Gettinger, S. N., Smith, D. C., McDermott, D. F., et al. (2012). Safety, activity, and immune correlates of anti–PD-1 Antibody in Cancer. N. Engl. J. Med. 366, 2443–2454. doi: 10.1056/NEJMoa1200690
Ugel, S., De Sanctis, F., Mandruzzato, S., and Bronte, V. (2015). Tumor-induced myeloid deviation: when myeloid-derived suppressor cells meet tumor-associated macrophages. J. Clin. Invest. 125, 3365–3376. doi: 10.1172/JCI80006
Valanparambil, R. M., Tam, M., Gros, P.-P., Auger, J.-P., Segura, M., Gros, P., et al. (2017). IRF-8 regulates expansion of myeloid-derived suppressor cells and Foxp3+ regulatory T cells and modulates Th2 immune responses to gastrointestinal nematode infection. PLoS Pathog. 13:e1006647. doi: 10.1371/journal.ppat.1006647
van der Bruggen, P., Traversari, C., Chomez, P., Lurquin, C., De Plaen, E., Van den Eynde, B. J., et al. (2017). A gene encoding an antigen recognized by cytolytic T lymphocytes on a human melanoma. J. Immunol. 178, 2617–2621.
van Deventer, H. W., Burgents, J. E., Wu, Q. P., Woodford, R.-M. T., Brickey, W. J., Allen, I. C., et al. (2010). The inflammasome component Nlrp3 Impairs antitumor vaccine by enhancing the accumulation of tumor-associated myeloid-derived suppressor cells. Cancer Res. 70, 10161–10169. doi: 10.1158/0008-5472.CAN-10-1921
Vandenberk, L., Garg, A. D., Verschuere, T., Koks, C., Belmans, J., Beullens, M., et al. (2016). Irradiation of necrotic cancer cells, employed for pulsing dendritic cells (DCs), potentiates DC vaccine-induced antitumor immunity against high-grade glioma. Oncoimmunology 5:e1083669. doi: 10.1080/2162402X.2015.1083669
Veglia, F., Perego, M., and Gabrilovich, D. (2018). Myeloid-derived suppressor cells coming of age. Nat. Immunol. 19, 108–119. doi: 10.1038/s41590-017-0022-x
Veltman, J. D., Lambers, M. E. H., van Nimwegen, M., Hendriks, R. W., Hoogsteden, H. C., Aerts, J. G. J. V., et al. (2010). COX-2 inhibition improves immunotherapy and is associated with decreased numbers of myeloid-derived suppressor cells in mesothelioma. Celecoxib influences MDSC function. BMC Cancer 10:464. doi: 10.1186/1471-2407-10-464
Vincent, J., mignot, g., chalmin, f., ladoire, s., bruchard, m., chevriaux, a., et al. (2010). 5-fluorouracil selectively kills tumor-associated myeloid-derived suppressor cells resulting in enhanced T cell–dependent antitumor immunity. Cancer Res. 70, 3052–3061. doi: 10.1158/0008-5472.CAN-09-3690
Vivier, E., Ugolini, S., Blaise, D., Chabannon, C., and Brossay, L. (2012). Targeting natural killer cells and natural killer T cells in cancer. Nat. Rev. Immunol. 12, 239–252. doi: 10.1038/nri3174
Waldman, A. D., Fritz, J. M., and Lenardo, M. J. (2020). A guide to cancer immunotherapy: from T cell basic science to clinical practice. Nat. Rev. Immunol. 20, 651–668. doi: 10.1038/s41577-020-0306-5
Wang, J., Shirota, Y., Bayik, D., Shirota, H., Tross, D., Gulley, J. L., et al. (2015). Effect of TLR agonists on the differentiation and function of human monocytic myeloid-derived suppressor cells. J. Immunol. 194, 4215–4221. doi: 10.4049/jimmunol.1402004
Wang, S.-H., Lu, Q.-Y., Guo, Y.-H., Song, Y.-Y., Liu, P.-J., and Wang, Y.-C. (2016). The blockage of Notch signalling promoted the generation of polymorphonuclear myeloid-derived suppressor cells with lower immunosuppression. Eur. J. Cancer 68, 90–105. doi: 10.1016/j.ejca.2016.08.019
Wang, Y., Jia, A., Bi, Y., Wang, Y., and Liu, G. (2020). Metabolic regulation of myeloid-derived suppressor cell function in cancer. Cells 9:1011. doi: 10.3390/cells9041011
Wang, Y., Schafer, C. C., Hough, K. P., Tousif, S., Duncan, S. R., Kearney, J. F., et al. (2018). Myeloid-derived suppressor cells impair B cell responses in lung cancer through IL-7 and STAT5. J. Immunol. 201, 278–295. doi: 10.4049/jimmunol.1701069
Wang, Z., Till, B., and Gao, Q. (2017). Chemotherapeutic agent-mediated elimination of myeloid-derived suppressor cells. Oncoimmunology 6:e1331807. doi: 10.1080/2162402X.2017.1331807
Weber, J., Gibney, G., Kudchadkar, R., Yu, B., Cheng, P., Martinez, A. J., et al. (2016). Phase I/II study of metastatic melanoma patients treated with Nivolumab Who had progressed after Ipilimumab. Cancer Immunol. Res. 4, 345–353. doi: 10.1158/2326-6066.CIR-15-0193
Weed, D. T., Vella, J. L., Reis, I. M., De la fuente, A. C., Gomez, C., Sargi, Z., et al. (2015). Tadalafil reduces myeloid-derived suppressor cells and regulatory T cells and promotes tumor immunity in patients with head and neck squamous cell carcinoma. Clin. Cancer Res. 21, 39–48. doi: 10.1158/1078-0432.CCR-14-1711
Weed, D. T., Zilio, S., Reis, I. M., Sargi, Z., Abouyared, M., Gomez-Fernandez, C. R., et al. (2019). The reversal of immune exclusion mediated by Tadalafil and an anti-tumor vaccine also induces PDL1 upregulation in recurrent head and neck squamous cell carcinoma: interim analysis of a Phase I clinical trial. Front. Immunol. 10:1206. doi: 10.3389/fimmu.2019.01206
Weichselbaum, R. R., Liang, H., Deng, L., and Fu, Y.-X. (2017). Radiotherapy and immunotherapy: A beneficial liaison? Nat. Rev. Clin. Oncol. 14, 365–379. doi: 10.1038/nrclinonc.2016.211
Weide, B., Martens, A., Zelba, H., Stutz, C., Derhovanessian, E., Di Giacomo, A. M., et al. (2014). Myeloid-derived suppressor cells predict survival of patients with advanced melanoma: comparison with regulatory T Cells and NY-ESO-1- or Melan-A–specific T cells. Clin. Cancer Res. 20, 1601–1609. doi: 10.1158/1078-0432.CCR-13-2508
Wolchok, J. D., Chiarion-Sileni, V., Gonzalez, R., Rutkowski, P., Grob, J.-J., Cowey, C. L., et al. (2017). Overall survival with combined nivolumab and ipilimumab in advanced melanoma. N. Engl. J. Med. 377, 1345–1356. doi: 10.1056/NEJMoa1709684
Wolf, Y., Anderson, A. C., and Kuchroo, V. K. (2020). TIM3 comes of age as an inhibitory receptor. Nat. Rev. Immunol. 20, 173–185. doi: 10.1038/s41577-019-0224-6
Xu, X., Meng, Q., Erben, U., Wang, P., Glauben, R., Kühl, A. A., et al. (2017). Myeloid-derived suppressor cells promote B-cell production of IgA in a TNFR2-dependent manner. Cell. Mol. Immunol. 14, 597–606. doi: 10.1038/cmi.2015.103
Yan, G., Zhao, H., Zhang, Q., Zhou, Y., Wu, L., Lei, J., et al. (2018). A RIPK3-PGE 2 circuit mediates myeloid-derived suppressor cell–potentiated colorectal carcinogenesis. Cancer Res. 78, 5586–5599. doi: 10.1158/0008-5472.CAN-17-3962
Yang, J., Yan, C., Vilgelm, A. E., Chen, S.-C., Ayers, G. D., Johnson, C. A., et al. (2021). Targeted Deletion of CXCR2 in myeloid cells alters the tumor immune environment to improve antitumor immunity. Cancer Immunol. Res. 9, 200–213. doi: 10.1158/2326-6066.CIR-20-0312
Yang, L., DeBusk, L. M., Fukuda, K., Fingleton, B., Green-Jarvis, B., Shyr, Y., et al. (2004). Expansion of myeloid immune suppressor Gr+CD11b+ cells in tumor-bearing host directly promotes tumor angiogenesis. Cancer Cell 6, 409–421. doi: 10.1016/j.ccr.2004.08.031
Yu, S. J., Ma, C., Heinrich, B., Brown, Z. J., Sandhu, M., Zhang, Q., et al. (2019). Targeting the crosstalk between cytokine-induced killer cells and myeloid-derived suppressor cells in hepatocellular carcinoma. J. Hepatol. 70, 449–457. doi: 10.1016/j.jhep.2018.10.040
Zahoor, H., Mir, M. C., Barata, P. C., Stephenson, A. J., Campbell, S. C., Fergany, A., et al. (2019). Phase II trial of continuous treatment with sunitinib in patients with high-risk (BCG-refractory) non-muscle invasive bladder cancer. Invest. New Drugs 37, 1231–1238. doi: 10.1007/s10637-018-00716-w
Zefferino, R., Piccoli, C., Di Gioia, S., Capitanio, N., and Conese, M. (2021). How Cells communicate with each other in the tumor microenvironment: suggestions to design novel therapeutic strategies in cancer disease. Int. J. Mol. Sci. 22:2550. doi: 10.3390/ijms22052550
Zhang, H., Li, Z., Wang, L., Tian, G., Tian, J., Yang, Z., et al. (2017). Critical role of myeloid-derived suppressor cells in tumor-induced liver immune suppression through inhibition of NKT cell function. Front. Immunol. 8:129. doi: 10.3389/fimmu.2017.00129
Zhao, B. G., Vasilakos, J. P., Tross, D., Smirnov, D., and Klinman, D. M. (2014). Combination therapy targeting toll like receptors 7, 8 and 9 eliminates large established tumors. J. Immunother. Cancer 2:12. doi: 10.1186/2051-1426-2-12
Zhou, S. (2020). Multiomics kaleidoscope to visualize cancer hallmarks. Genome Biol. 21:264. doi: 10.1186/s13059-020-02176-z
Zhu, H., Gu, Y., Xue, Y., Yuan, M., Cao, X., and Liu, Q. (2017). CXCR2(+) MDSCs promote breast cancer progression by inducing EMT and activated T cell exhaustion. Oncotarget 8, 114554–114567. doi: 10.18632/oncotarget.23020
Keywords: myeloid-derived suppressor cells, T cell-based immunotherapy, combination therapy, immune checkpoint inhibitors, adoptive T cell therapy
Citation: Shi H, Li K, Ni Y, Liang X and Zhao X (2021) Myeloid-Derived Suppressor Cells: Implications in the Resistance of Malignant Tumors to T Cell-Based Immunotherapy. Front. Cell Dev. Biol. 9:707198. doi: 10.3389/fcell.2021.707198
Received: 09 May 2021; Accepted: 21 June 2021;
Published: 14 July 2021.
Edited by:
George S. Karagiannis, Albert Einstein College of Medicine, United StatesReviewed by:
Xin Lu, University of Notre Dame, United StatesCopyright © 2021 Shi, Li, Ni, Liang and Zhao. This is an open-access article distributed under the terms of the Creative Commons Attribution License (CC BY). The use, distribution or reproduction in other forums is permitted, provided the original author(s) and the copyright owner(s) are credited and that the original publication in this journal is cited, in accordance with accepted academic practice. No use, distribution or reproduction is permitted which does not comply with these terms.
*Correspondence: Xia Zhao, eGlhLXpoYW9AMTI2LmNvbQ==
†These authors have contributed equally to this work and share first authorship
Disclaimer: All claims expressed in this article are solely those of the authors and do not necessarily represent those of their affiliated organizations, or those of the publisher, the editors and the reviewers. Any product that may be evaluated in this article or claim that may be made by its manufacturer is not guaranteed or endorsed by the publisher.
Research integrity at Frontiers
Learn more about the work of our research integrity team to safeguard the quality of each article we publish.