- 1Department of Biology, Faculty of Science and Engineering, Konan University, Kobe, Japan
- 2Institute for Integrative Neurobiology, Graduate School of Natural Science, Konan University, Kobe, Japan
- 3Shimoda Marine Research Center, University of Tsukuba, Shimoda, Japan
In vertebrate embryos, dorsal midline tissues, including the notochord, the prechordal plate, and the floor plate, play important roles in patterning of the central nervous system, somites, and endodermal tissues by producing extracellular signaling molecules, such as Sonic hedgehog (Shh). In Ciona, hedgehog.b, one of the two hedgehog genes, is expressed in the floor plate of the embryonic neural tube, while none of the hedgehog genes are expressed in the notochord. We have identified a cis-regulatory region of hedgehog.b that was sufficient to drive a reporter gene expression in the floor plate. The hedgehog.b cis-regulatory region also drove ectopic expression of the reporter gene in the endodermal strand, suggesting that the floor plate and the endodermal strand share a part of their gene regulatory programs. The endodermal strand occupies the same topographic position of the embryo as does the vertebrate hypochord, which consists of a row of single cells lined up immediately ventral to the notochord. The hypochord shares expression of several genes with the floor plate, including Shh and FoxA, and play a role in dorsal aorta development. Whole-embryo single-cell transcriptome analysis identified a number of genes specifically expressed in both the floor plate and the endodermal strand in Ciona tailbud embryos. A Ciona FoxA ortholog FoxA.a is shown to be a candidate transcriptional activator for the midline gene battery. The present findings suggest an ancient evolutionary origin of a common developmental program for the midline structures in Olfactores.
Introduction
The embryonic midline tissues, notochord, and floor plate are signaling centers that pattern vertebrate embryos (Placzek and Briscoe, 2005; Stemple, 2005). The notochord acts as an axial supportive structure and induces the floor plate in the neural tube and patterns somitic mesoderm via Sonic hedgehog (Shh) secretion. The floor plate then patterns the neural tube along the dorso-ventral axis also using the Shh signal. Thus these midline structures are central elements for construction of the vertebrate body plan.
In anamniote embryos, an endodermal rod-shaped structure, hypochord, transiently appears ventral to the notochord (Franz, 1898; Reinhardt, 1904; Gibson, 1910). Development of the hypochord also depends on signals from the notochord (Cleaver and Krieg, 1998). The hypochord was once thought to be simply a supportive structure (Stöhr, 1895; Corbo et al., 1997a) but several lines of evidence suggest that it plays a role in the positioning of the dorsal aorta (Cleaver et al., 1997; Löfberg and Collazo, 1997; Cleaver and Krieg, 1998; Eriksson and Löfberg, 2000; Hogan and Bautch, 2004), and for determination of left–right axis asymmetry (Danos and Yost, 1996; Lohr et al., 1997). Thus, transient midline tissues originating from different germ layers, the floor plate (ectoderm), the notochord (mesoderm), and the hypochord (endoderm), pattern the embryonic structure in vertebrates.
The hypochord shares expression of several genes with the floor plate and the notochord, including Shh and FoxA (Yan et al., 1995; Appel et al., 1999; Dal-Pra et al., 2011; Peyrot et al., 2011). Although their originating germ layers are different, progenitor cells of these midline tissues locate close to one another in the dorsal marginal zone, such as the Spemann organizer in amphibians and the embryonic shield in zebrafish (Shih and Fraser, 1995; Melby et al., 1996; Latimer et al., 2002; Latimer and Appel, 2006; Dal-Pra et al., 2011; Peyrot et al., 2011). These commonalities suggest a tight developmental and evolutionary connection among these midline structures. The notochord is the organ that define the phylum (or superphylum) Chordata, including vertebrates, tunicates, and cephalochordates (Kowalevsky, 1866, 1867; Yasuo and Satoh, 1993; Corbo et al., 1997a, b; Satoh et al., 2014). The ventral midline of the neural tube (nerve cord) in tunicate Ciona embryos expresses homologues of Shh (hedgehog.b) and FoxA (FoxA.a), and has been identified as the floor plate homologue (Corbo et al., 1997a; Takatori et al., 2002; Shi et al., 2009). By contrast, the presence of a hypochord homologue remains obscure in invertebrate chordates, although it has been suggested to be homologous with the epibranchial groove of amphioxus (Klaatsch, 1898) and a similarity between the hypochord and the endodermal strand of Ciona embryos has been pointed out (Corbo et al., 1997a).
Here we provide new evidence that the endodermal strand shares the gene regulatory mechanism with the floor plate in Ciona embryos. Functional analysis of the cis-regulatory region of the floor plate-specific hedgehog.b gene revealed its latent ability to drive transcription in the endodermal strand. Whole-embryo single-cell transcriptome analysis identified a number of genes specifically expressed in both the floor plate and the endodermal strand in Ciona tailbud embryos. These genes and their transcriptional regulation suggest an ancient evolutionary origin of a common developmental program for the midline structures in Olfactores. Our findings also support homology between the vertebrate hypochord and the tunicate endodermal strand.
Results and Discussion
Transcriptional Activation by Cis-Regulatory Regions of Ciona hedgehog.b in the Floor Plate and Hypochord Homologues
Ciona hedgehog.b is expressed in the floor plate, but not in the notochord during embryogenesis (Takatori et al., 2002; Islam et al., 2010; Figures 1A,B). When the 2.6-kb upstream region of hedgehog.b connected with a Kaede reporter (hedgehog.b > kaede) was introduced into Ciona embryos, the expression of Kaede reporter was observed in the floor plate at the mid tailbud stage (Figure 1D). In addition to the expression in the floor plate, “ectopic” Kaede expression was observed in the endodermal strand of some embryos (Figure 1D). In contrast, no Kaede expression was observed in the notochord.
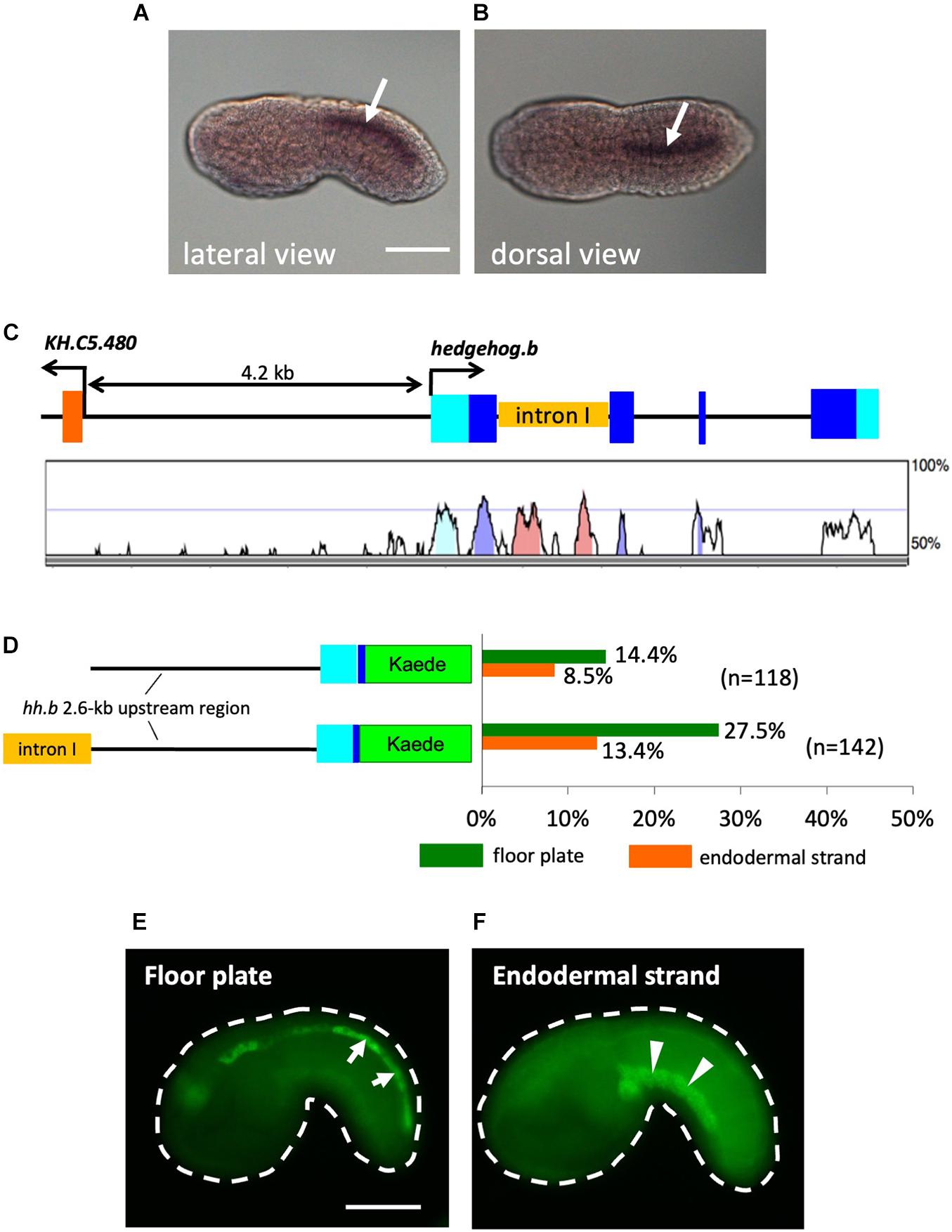
Figure 1. Activity of cis-regulatory regions of Ciona hedgehog.b in the floor plate and the endodermal strand of tail bud embryos. (A,B) Expression pattern of hedgehog.b at the tailbud stage visualized by whole-mount in situ hybridization. Anterior is to the left. Arrows indicate the floor plate (ventral nerve cord). (A) Lateral view. (B) Dorsal view. (C) Genomic organization of the hedgehog.b gene. The exons are indicated by boxes in cyan (untranslated regions) and blue (coding regions). The untranscribed regions and introns are indicated by lines. A peaks-and-valleys graph below the genomic organization diagram is a VISTA profile (Frazer et al., 2004) showing the percent conservation of the nucleotide sequence of each region between C. intestinalis type A and Ciona savignyi. (D) The structure of the Kaede reporter constructs and the reporter expression patterns observed. The left diagrams show schematic structure of each construct. Cyan and blue boxes indicate the 5′ untranslated region and a partial coding region, respectively, of hedgehog.b. The orange box indicates the first intron sequence of hedgehog.b. Bars in the right graph show the percentage of larvae with Kaede expression in a tissue out of all electroporated embryos scored for each construct. Different colors of bars represent expression in different tissues: green, floor plate; orange, endodermal strand. Numbers in parentheses indicate the number of larvae scored for each construct. (E,F) Examples of mid tailbud embryos electroporated with the hedgehog.b(+int) > kaede construct showing Kaede expression in the floor plate (arrows in panel E) and the endodermal strand (arrowheads in panel F). Scale bars, 50 μm.
Because the reporter expression was only observed in a relatively small proportion of transfected embryos and the ectopic expression in the endodermal strand was observed (Figure 1D), we thought that additional cis-regulatory sequences might be present outside of the 2.6-kb upstream region. Comparative genomics between Ciona intestinalis type A and Ciona savignyi revealed that the first intron of hedgehog.b contains highly conserved non-coding regions, which could be candidates for such additional cis-regulatory sequences (Figure 1C). To test this possibility, we placed the first intron sequence upstream of the 2.6-kb genomic region in the hedgehog.b > kaede construct and examined Kaede reporter expression in embryos transfected with this DNA construct (Figure 1D). As expected, higher frequency of Kaede expression in the floor plate was observed (Figures 1D,E). However, the reporter expression in the endodermal strand also remained (Figures 1D,F). The endodermal strand is a caudal midline structure that lies immediately ventral to the notochord and its homology with the vertebrate hypochord has been proposed (Corbo et al., 1997a). Thus, the cis-regulatory regions of Ciona hedgehog.b can activate transcription in the floor plate and hypochord homologues. This observation further prompted us to test an idea that the floor plate and the endodermal strand share a developmental program including the transcriptional machinery.
Single-Cell Transcriptomic Analysis Revealed a Gene Battery Shared Among the Midline Tissues
To further investigate the shared developmental program between the floor plate and the endodermal strand, we compared gene expression profiles between these tissues by whole-embryo single-cell transcriptomics at the mid tailbud stage (Table 1 and Figure 2; Horie T. et al., 2018; Horie R. et al., 2018; Cao et al., 2019). Whole-embryo single-cell transcriptome data clearly revealed that hedgehog.b is expressed in the floor plate but not expressed in any other tissues, including the notochord and the endodermal strand (Figure 2B). Among the top 20 genes highly expressed in the endodermal strand, 8 genes were shown to be significantly enriched (p < 0.05) in the floor plate (Table 1). Of these, five genes were highly enriched (p < 0.001) in the floor plate (Table 1 and Figure 2). These genes include fz4 (gene model ID KH.C6.162) encoding a Frizzled4 receptor, foxA.a (KH.C11.313) encoding a FoxA transcription factor, KH.C2.442 encoding a solute carrier family 1 protein, KH.C5.232 encoding a tissue inhibitor of metalloproteinases 4, and KH.C4.230 encoding a calponin/transgelin family protein (transgelin-related.b). Interestingly, four of these genes (fz4, foxA.a, KH.C5.23, and KH.C4.230) are also expressed in the notochord (Figures 2D–G). The expression pattern of foxA.a is consistent with the previously reported whole-mount in situ hybridization (Corbo et al., 1997a). These genes may constitute a gene battery co-regulated in the midline tissues at the mid tailbud stage.
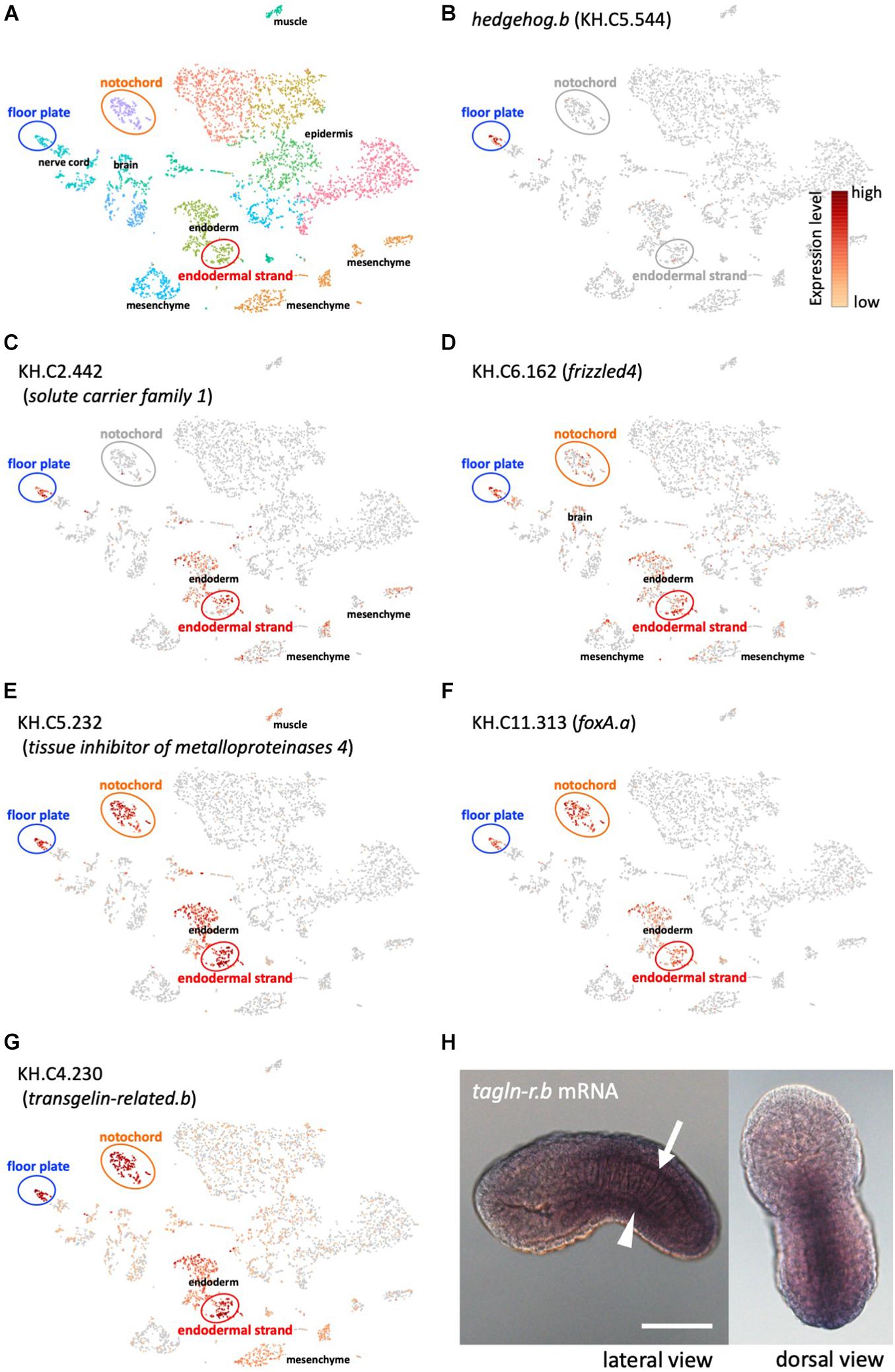
Figure 2. Whole-embryo single-cell RNA-seq analysis of midline tissue-specific genes. (A) A t-distributed stochastic neighbor embedding (t-SNE) projection map of mid-tailbud stage embryos obtained in a previous study (Horie T. et al., 2018). Each dot corresponds to the transcriptome of a single cell, and cells possessing similar transcriptome profiles map near each other. The major tissue types in tailbud-stage embryos were identified. Identification of tissue types is based on the expression of known marker genes as previously described (Horie T. et al., 2018). Clusters of cells corresponding to the floor plate, the notochord, and the endodermal strand are encircled. (B) The t-SNE projection map highlighting hedgehog.b-expressing cells (red dots) in the floor plate. (C–G) The t-SNE projection map showing the expression profiles of representative genes that are specifically expressed in both the floor plate and the endodermal strand (Table 1). Red and orange dots indicate cells expressing KH.C2.442 (C), KH.C6.162 (frizzled4, D), KH.C5.232 (E), KH.C11.313 (foxA.a, F), and KH.C4.230 (tagln-r.b, G). (H) Expression pattern of tagln-r.b at the tailbud stage visualized by whole-mount in situ hybridization. The arrow and the arrowhead indicate the floor plate and the endodermal strand, respectively. Scale bar, 50 μm.
For further analysis, we adopted KH.C4.230 as a model to investigate transcriptional regulation in the midline tissues because its expression level is relatively high and the enriched expression in the floor plate, the notochord, and the endodermal strand is strongly supported by the single-cell transcriptomic analysis (p-values, 3.96E-09, 1.49E-14, and 4.52E-08, respectively). KH.C4.230 encodes a protein belonging to the calponin/transgelin family. Calponins and transgelins are actin-associated proteins highly conserved from yeast to mammals (Prinjha et al., 1994; Goodman et al., 2003). We named KH.C4.230 as transgelin-related.b (tagln-r.b) based on the sequence similarity and genomic arrangement (Figure 3). Whole-mount in situ hybridization confirmed that tagln-r.b is expressed in the floor plate, the notochord, and the endodermal strand (Figure 2H).
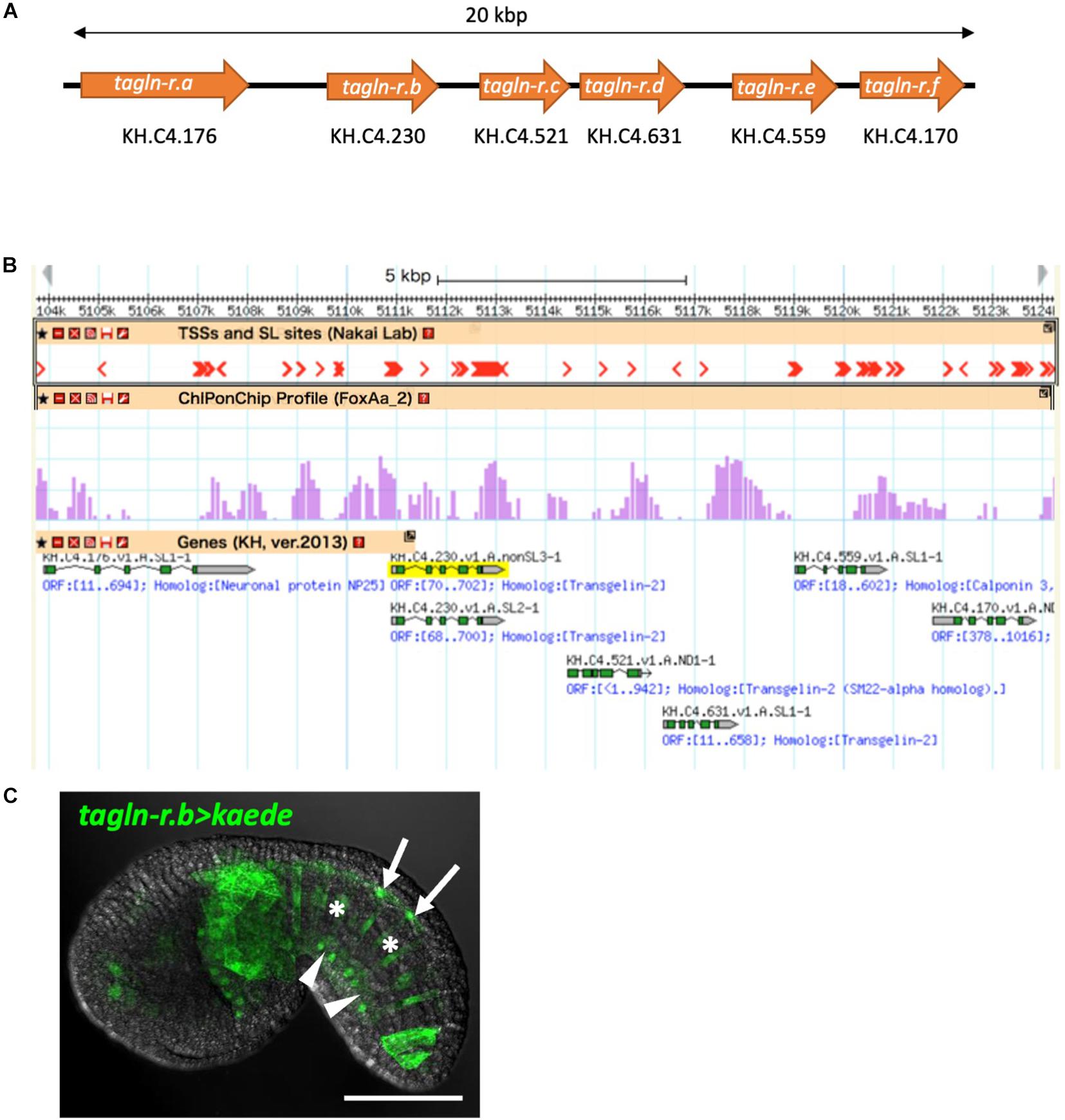
Figure 3. The calponin/transgelin family gene cluster in the Ciona genome. (A) Schematic diagram of the gene cluster. Six genes, tagln-r.a, tagln-r.b, tagln-r.c, tagln-r.d, tagln-r.e, and tagln-r.f, each encoding a calponin/transgelin family protein are clustered in a 20-kb genomic region. (B) The transcriptional landscape of the tagln-r loci. Transcription start sites (TSSs) and spliced leader (SL) trans-splicing sites (Yokomori et al., 2016) and FoxA.a binding sites determined by ChIP-on-chip analysis (Kubo et al., 2010) were mapped on the Ghost Genome Browser (Satou et al., 2005, 2008). (C) Localization of Kaede reporter expressed under the control of the upstream cis-regulatory region of tagln-r.b. Arrows, arrowheads, and asterisks indicate the floor plate, the endodermal strand, and the notochord, respectively. Scale bar, 50 μm.
In the genome of C. intestinalis type A, tagln-r.b is clustered in tandem with five other calponin/transgelin family genes within a 20-kb genomic region (Figure 3A). Whole-embryo single-cell transcriptome and high-throughput in situ hybridization data in the Ghost database (Satou et al., 2005) indicate that at least three of these tagln-r genes (tagln-r.c, tagln-r.d, and tagln-r.e) are also specifically expressed in the floor plate, the notochord, and the endodermal strand (Supplementary Figure 1; spatial expression patterns of tagln-r.e can be found at http://ghost.zool.kyoto-u.ac.jp/cgi-bin/photogetkh.cgi?inkey=CLSTR02020). Thus the clustered tagln-r genes are likely to be co-regulated as a member of the gene battery above mentioned.
The Role of FoxA.a as a Common Transcriptional Activator for the Midline Gene Battery
Because the expression profile of foxA.a (Figure 2F) was very similar to that of tagln-r.b (Figure 2G), FoxA.a seemed to be a good candidate for a common transcriptional activator in the midline tissues. To test this possibility, we examined distribution of the FoxA.a binding sites in the upstream of the putative transcription start sites of each of the clustered tagln-r genes using a set of ChIP-on-chip data of FoxA.a (Kubo et al., 2010). As expected, FoxA.a binding sites are enriched in the 5′ flanking region of each tagln-r gene (Figure 3B). To analyze the transcriptional regulatory mechanism of tagln-r.b, its 2.8-kb upstream region was connected with the coding sequence of Kaede (Figure 4A) and introduced into Ciona embryos. The tagln-r.b > kaede DNA construct recapitulated the endogenous expression pattern of tagln-r.b; it was expressed in the floor plate, the notochord, and the endodermal strand (Figure 3C), suggesting that the 2.8-kb upstream region contains cis-regulatory sequences sufficient for transcription in the midline tissues.
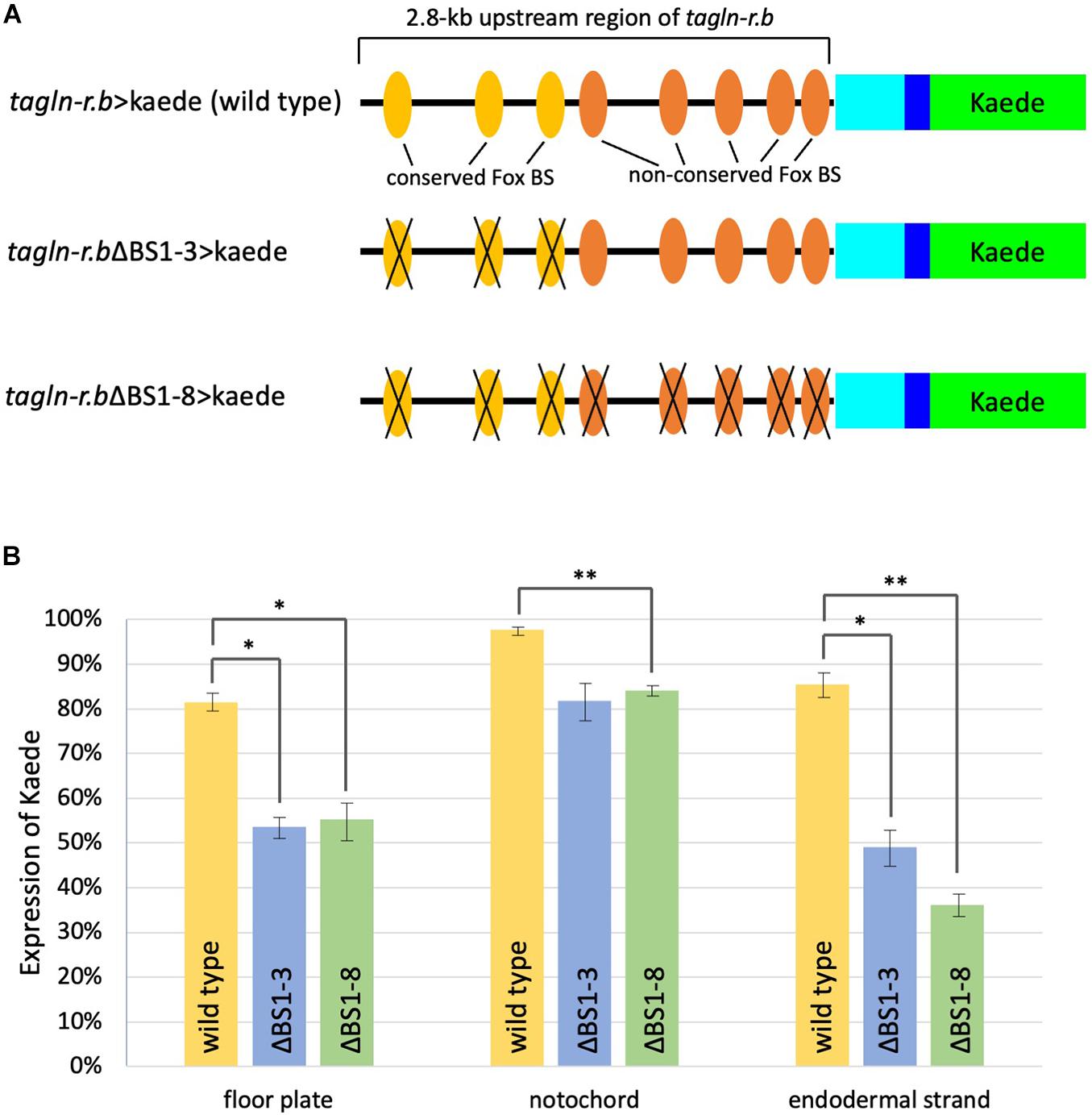
Figure 4. Functional analysis of putative Fox binding sites (BSs) in the cis-regulatory region of tagln-r.b. (A) Schematic diagram of the Kaede reporter constructs. Cyan and blue boxes indicate the 5′ untranslated region and a partial coding region, respectively, of tagln-r.b. Green boxes indicate the coding sequence of Kaede. Substitution mutations were introduced into putative Fox BSs. Colored ovals indicate the putative Fox BSs and black crosses indicate mutated BSs. (B) Expression of the Kaede reporter in the midline tissues of tailbud embryos. Localization of Kaede was detected by immunofluorescence staining in mid tailbud (12 hpf) embryos that developed from fertilized eggs electroporated with tagln-r.b > kaede fusion constructs. Vertical bars indicate the percentage of Kaede-positive embryos. Error bars represent SEM from three independent experiments. The total number of embryos scored for each construct was 172 for tagln-r.b > kaede (wild-type), 152 for tagln-r.bΔBS1-3 > kaede, and 147 for tagln-r.bΔBS1-8 > kaede. Statistical analysis was carried out using the standard Student t-test (∗∗P < 0.01, ∗P < 0.05).
The 2.8-kb upstream region of tagln-r.b contains eight putative Fox binding sites (Figure 4A). Among these sites, three distal sites [binding sites (BS) 1–3] are conserved between C. intestinalis type A and Ciona savignyi, whereas five proximal sites (BS4-8) are not conserved. To test functional importance of putative Fox BSs, three conserved sites (BS1-3) or all eight BSs (BS1-8) were mutated in the tagln-r.b > kaede construct (Figure 4A) and the reporter expression was examined in the mid tailbud embryos transfected with these DNA constructs (Figure 4B). When only the conserved sites were mutated (ΔBS1-3), the reporter expression was significantly reduced in the floor plate and the endodermal strand, whereas it was moderately reduced in the notochord. Additional mutations in the non-conserved BSs (BS4-8) did not further decrease the reporter expression in each tissue. These results suggest that a Fox transcription factor, presumably FoxA.a, serves as a transcriptional activator of tagln-r.b in the midline tissues via direct interaction with the upstream region. Our observation also suggests that a greater contribution of FoxA.a to transcriptional activation of tagln-r.b in the floor plate and the endodermal strand than in the notochord. Because disruption of all Fox BSs in the cis-regulatory region of tagln-r.b had only slightly reduced the reporter expression in the notochord (Figure 4), it is plausible that Brachyury is the main activator for tagln-r.b in the notochord.
Among 29 Fox transcription factors identified in C. intestinalis type A (Imai et al., 2004; Satou et al., 2005), FoxA.a is the most plausible candidate as the transcription factor that interacts with Fox BSs in the upstream region of tagln-r.b for three reasons. First, as mentioned above, the ChIP-on-chip data demonstrated FoxA.a binding to the upstream region of tagln-r.b (Kubo et al., 2010). Second, expression patterns of foxA.a and tagln-r.b are similar to each other. Third, none of the other Fox family genes show similar expression patterns (Imai et al., 2004). In a strict sense, however, the present analysis does not exclude the possibility that a Fox transcription factor other than FoxA.a is involved in the transcriptional activation of tagln-r.b. To further assess the role of FoxA.a in tagln-r.b expression in the midline tissues, functional manipulations of FoxA.a, such as overexpression of wild-type and a repressor form and tissue-specific knockdown, will be required in future studies.
Disruption of all Fox BSs in the cis-regulatory region of tagln-r.b did not completely abolished the reporter expression in the floor plate and the endodermal strand (Figure 4). This suggests that other transcription factors are involved in transactivation of tagln-r.b. Future identification of transcription factors that interacts with the cis-regulatory region of tagln-r.b will contribute to the elucidation of the gene regulatory networks for the development of the floor plate and the endodermal strand.
Developmental Roles of the Endodermal Strand in Ciona Embryos
The hypochord, transient rod-like structure situated under the notochord, is first described in embryos of elasmobranchs (Leydig, 1852). Many morphological studies on this structure were reported in embryos of lampreys, fishes, and amphibians in the late 19th and early 20th centuries (Hatta, 1893; Franz, 1898; Klaatsch, 1898; Reinhardt, 1904; Gibson, 1910). Since then, however, the hypochord has been neglected by researchers for many years, and its function remains elusive. An inductive role in the formation of the dorsal aorta has been proposed (Cleaver et al., 1997; Löfberg and Collazo, 1997; Cleaver and Krieg, 1998; Eriksson and Löfberg, 2000). Although it is uncertain whether the hypochord has a structural counterpart in embryos of higher vertebrates, a similar inductive role of the dorsal endoderm in blood vessel patterning has been proposed in avian embryos (Hogan and Bautch, 2004).
The only function of the endodermal strand known to date is its role as the precursor of the adult intestine (Hirano and Nishida, 2000; Nakazawa et al., 2013). The similarity between the hypochord and the endodermal strand prompted us to ask whether the Ciona endodermal strand has an inductive role similar to that of the vertebrate hypochord. In vertebrate embryos, the blood vessel precursor angioblasts migrate toward the hypochord or dorsal endoderm to form the dorsal aorta (Cleaver and Krieg, 1998; Eriksson and Löfberg, 2000; Hogan and Bautch, 2004). To test whether similar cell migration occurs in Ciona embryos, we labeled trunk mesenchyme cells with the photoconvertible fluorescent protein Kaede (Ando et al., 2002) and fluorescence emitted by Kaede was converted from green to red by irradiation with 405-nm violet light at 10 hpf. The kaede transgene was expressed using an upstream regulatory region of Ciona pax2/5/8.a, which could drive the reporter gene expression in trunk mesenchyme cells. These embryos were analyzed by time-laps imaging from late tailbud (12 hpf) to larval (24.5 hpf) stages (Figure 5). Some of the Kaede-labeled mesenchyme cells were shown to migrate into the tail along the endodermal strand (Figure 5 and Supplementary Video 1). The Ciona endodermal strand may exert an inductive cue for the migratory mesenchyme cells, suggesting a functional similarity between the vertebrate hypochord and the Ciona endodermal strand.
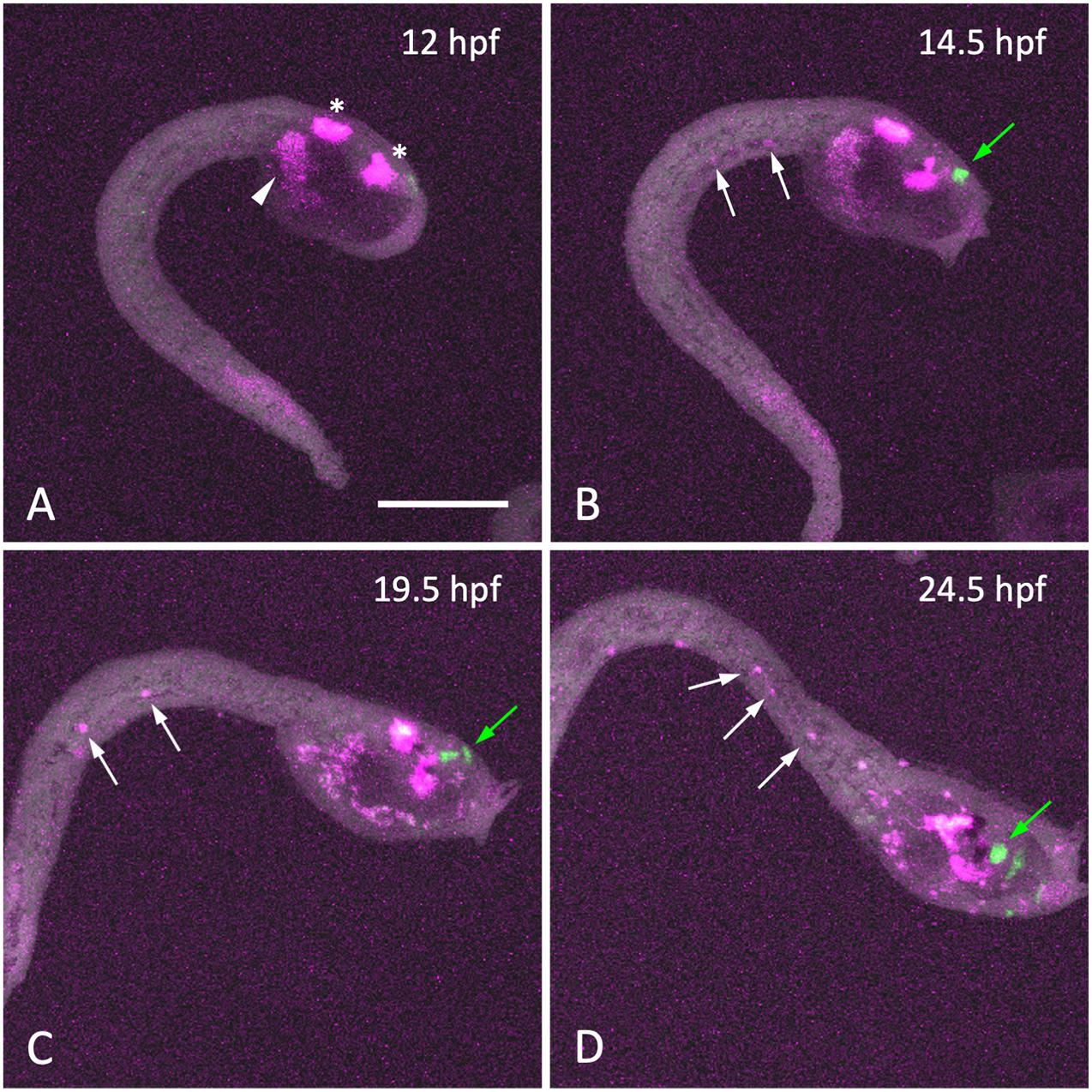
Figure 5. Migration of mesenchyme cells along the endodermal strand during larval development. (A–D) Time-lapse fluorescent images of a late-tailbud embryo expressing Kaede under the control of the cis-regulatory region of Pax2/5/8.a at the time indicated. Kaede fluorescence was photo-converted from green to red (shown in magenta) by 405-nm laser irradiation at 12 hpf (A). At 12 hpf, photo-converted Kaede fluorescence was observed in the central nervous system (asterisks) and mesenchyme cells (arrowhead) in the trunk region, whereas no cells were labeled in the tail region. As development proceeded (B–D), a few cells labeled with photoconverted-Kaede appeared in the tail region and posteriorly migrated along the endodermal strand (white arrows). Cells synthesized Kaede after photo-conversion were labeled with green fluorescence (green arrows in panels B–D). Scale bar, 100 μm.
The top 10 predominantly expressed genes in the endodermal strand include genes encoding extracellular ligands and receptors, including SLIT and NTRK-like protein (KH.C4.693), secreted frizzled-related protein (KH.C1.520), and frizzled receptor (KH.C6.162) (Table 1). Expression of these genes suggests an active interaction between the endodermal strand and other tissues. In zebrafish, the hypochord expresses the frzb/sfrp3 gene that encodes a secreted frizzled-related protein (Thisse et al., 2001; Tendeng and Houart, 2006), showing a further similarity between the endodermal strand and the hypochord. Functional analysis of these genes may give insights into the role of the endodermal strand in Ciona embryos.
Conserved Developmental Programs for Midline Tissues in Olfactores
The present findings, along with a number of previous studies, illustrate common features and the difference of midline development between vertebrates and tunicates (Figure 6). The gene regulatory network for notochord development in ascidian embryos has been extensively studied (Imai et al., 2006; Hotta et al., 2008; Passamaneck et al., 2009; Kubo et al., 2010; José-Edwards et al., 2015; Reeves et al., 2021). Brachyury is a key specifier gene for the notochord formation. FoxA.a is an upstream activator of Brachyury (Imai et al., 2006; Hotta et al., 2008; Kubo et al., 2010), but it also directly activates notochord-specific genes (Passamaneck et al., 2009; José-Edwards et al., 2015; Reeves et al., 2021).
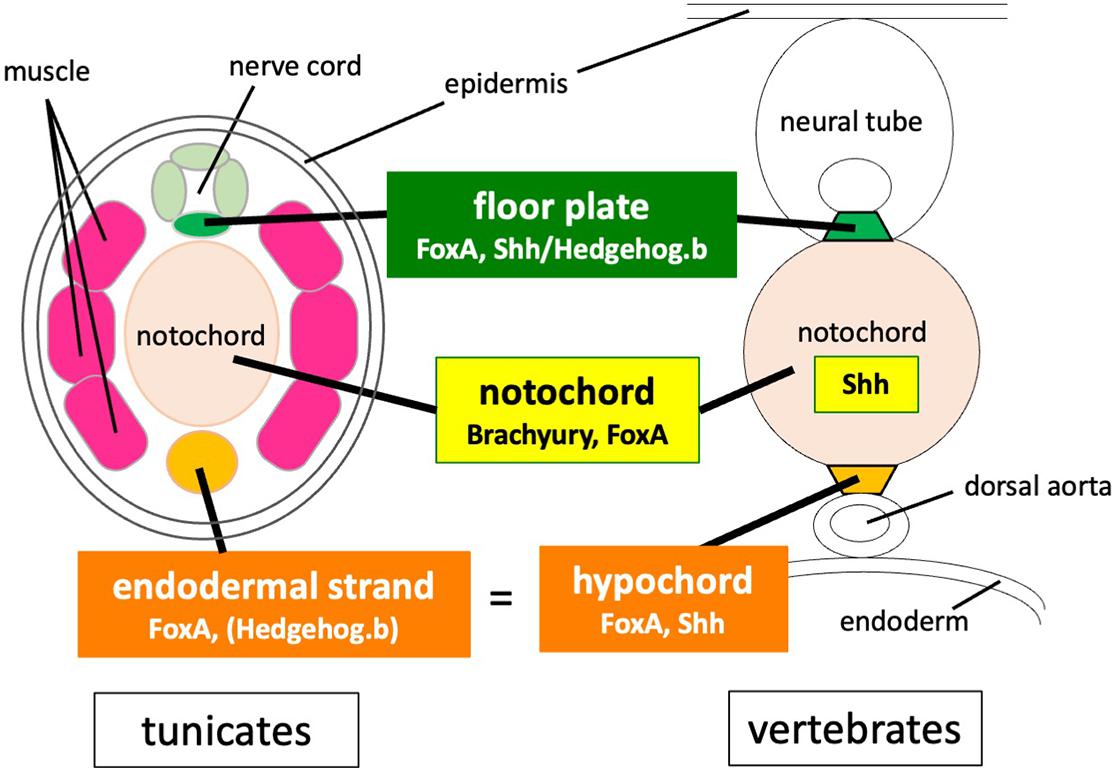
Figure 6. Comparison of developmental programs for midline tissues between tunicates and vertebrates. Co-expression of Brachyury and FoxA family transcription factors is required for notochord development both in vertebrates and tunicates. Both the notochord and the floor plate secrete Shh in vertebrate embryos, whereas hedgehog genes are not expressed in the notochord of tunicate embryos. FoxA and Shh/hedgehog.b are co-expressed in the floor plate of both vertebrates and tunicates. FoxA and Shh are also co-expressed in the hypochord precursor. The tunicate endodermal strand expresses FoxA.a and has a latent transactivation potential of hedgehog.b.
Co-expression of Brachyury and FoxA family transcription factors is required for notochord development both in vertebrates and tunicates (Herrmann and Kispert, 1994; Teillet et al., 1998; Friedman and Kaestner, 2006; Imai et al., 2006; Hotta et al., 2008; Passamaneck et al., 2009; José-Edwards et al., 2015). The notochord patterns the central nervous system, somitic mesoderm, and dorsal endoderm by secreting Shh in vertebrate embryos, whereas hedgehog genes are not expressed in the notochord of tunicate embryos (Takatori et al., 2002). FoxA and Shh/Hedgehog.b are co-expressed in the floor plate of both vertebrates and tunicates (Tessier-Lavigne et al., 1988; Placzek et al., 1990a, b; Takatori et al., 2002; Imai et al., 2009; Dal-Pra et al., 2011; Peyrot et al., 2011). FoxA and Shh are also co-expressed in the hypochord or its primordium (Ruiz i Altaba, 1998; Dal-Pra et al., 2011; Peyrot et al., 2011). The tunicate endodermal strand expresses FoxA.a (Corbo et al., 1997a) and has a latent transactivation potential of hedgehog.b as shown in this study.
In conclusion, the present study suggests that the floor plate and the hypochord homologue of Ciona embryos share a gene battery, which is regulated by a common transcription activator FoxA.a. The FoxA transcription factor seems to be a key regulator for midline development both in ascidians and vertebrates. The endodermal strand may have an inductive role for a novel population of migratory trunk cells, which further reveals a common feature shared between the endodermal strand and the hypochord. Altogether, the present findings suggest an ancient origin of a common developmental program for and common developmental roles of the midline structures in Olfactores.
Materials and Methods
Ciona Adults and Embryos
Mature adults of C. intestinalis type A (also called Ciona robusta) were provided by the Maizuru Fisheries Research Station of Kyoto University and by the Misaki Marine Biological Station of the University of Tokyo through the National Bio-Resource Project of the Ministry of Education, Culture, Sports, Science and Technology of Japan (MEXT), and were maintained in indoor tanks of artificial seawater (ASW) (Marine Art BR; Tomita Pharmaceutical, Tokushima, Japan) at 18°C. The adults were also collected from the pond on the Fukae campus of Kobe University, Kobe, Japan and from the fishing harbor in Murotsu, Hyogo, Japan. Eggs and sperm were obtained surgically from the gonoducts, and the eggs were fertilized in vitro. After insemination, the embryos were raised in ASW containing 50 μg/ml streptomycin sulfate (S6501; Sigma-Aldrich, St. Louis, MO, United States) at 18°C.
Whole-Mount in situ Hybridization
The cDNA clones for hedgehog.b (Gene Collection ID R1CiGC41g11) and tagln-r.b (Gene Collection ID R1CiGC29n19) were obtained from the Ciona Gene Collection release 1 (Satou et al., 2002) and used as the templates to synthesize probes. To linearize the plasmid DNA for probe synthesis, cDNA clones were digested with XbaI (for hedgehog.b) or EcoRI (for tagln-r.b). Antisense RNA probes were synthesized with T7 RNA polymerase by using a DIG RNA Labeling Kit (Sigma-Aldrich, St. Louis, MI, United States). Ciona intestinalis type A embryos were fixed at the early tailbud stage in 4% paraformaldehyde in 0.1 M MOPS (pH 7.5) and 0.5 M NaCl at 4°C for 16 h, prior to storage in 80% ethanol at −30°C. Whole-mount in situ hybridization was carried out as described (Oonuma and Kusakabe, 2019).
Preparation of Reporter Constructs and Electroporation
To make the hedgehog.b > kaede plasmid, the 2.6-kb upstream region of Ciona hedgehog.b (Gene Model ID KH.C5.544) was amplified from the genomic DNA of C. intestinalis type A by PCR using a pair of nucleotide primers (5′-ATCTGCAGGGTTTGATGCACAGCAAC-3′ and 5′-ATGGAT CCCCTGACCCGCATGATATGAC-3′), digested with PstI and BamHI, and then inserted into the PstI/BamHI sites of the pSP-Kaede vector (Hozumi et al., 2010). To make the hedgehog.b(+int) > kaede construct, the first intron sequence of hedgehog.b was amplified from the genomic DNA using a pair of nucleotide primers (5′-TTCTCGAGGCAGCAGTATGTGCCAC-3′ and 5′-CCCTGCA GCCATCCCAAGCTTCGATAAC-3′), digested with XhoI and PstI, and then inserted into the XhoI/PstI sites of the hedgehog.b > kaede plasmid. The tagln-r.b > kaede plasmid was made by inserting the 2.8-kb upstream region of Ciona tagln-r.b (Gene Model ID KH.C4.230) into the pSP-Kaede plasmid using an In-Fusion HD Cloning kit (Takara Bio, Japan). The 2.8-kb upstream region of tagln-r.b was amplified from the genomic DNA by PCR using a pair of nucleotide primers (5′-AAACTCGAGTCACACGAATTAAGCAAAGC-3′ and 5′-TTTTTCTCGTTGCGCCAT-3′). To generate mutant constructs, tagln-r.bΔBS1-3 > kaede and tagln-r.bΔBS1-8 > kaede, putative Fox binding sites (RYAAAYA; Chen et al., 2016) were mutagenized by the PCR-based method as previously described (Oonuma and Kusakabe, 2019). Oligonucleotide primers used for the mutagenesis of fox binding sites (BS1-8) were: 5′-GGTACGgcccaAAGCAGGAATTTTAATAGCAGT-3′ and 5′ -CTGCTTtgggcCGTACCTTTACCTTACTGGGTGG-3′ for BS1; 5′-GCTTCTcgggtTCTTGCCAAATAAGGCGA-3′ and 5′-GCAA GAacccgAGAAGCACGAAGCAAATTC-3′ for BS2; 5′-AACT GTTtgggtTCTTGGGCGAGCTAAGC-3′ and 5′-CCCAAGAac ccaAACAGTTTCATTGAAAGAGCC-3′ for BS3; 5′-GCAAA AGacccgATTCGTGCGACGGATTC-3′ and 5′-CACGAATcgggt CTTTTGCTCTCCCATGCA-3′ for BS4; 5′-CCTAGATcgggcTC GTACAACAGTTTGACGTAAGTTC-3′ and 5′-TGTACGAgc ccgATCTAGGCGTATTTCCACACG-3′ for BS5; 5′-TGTTATG gcccgACTCCATTCGTTCAACTTTCTAGA-3′ and 5′-GGAGT cgggcCATAACACCATACCTGTCGCGCG-3′ for BS6; 5′-GCGTTTtgggcCGTTTGATTGATAAATGTACGTAAGAGA-3′ and 5′-CAAACGgcccaAAACGCATTTAAAAGCCAGTT-3′ for BS7; 5′-CCTCATAGacccaAGCGAATCCATTGTCAAGTC-3′ and 5′-ATTCGCTtgggtCTATGAGGAGTATAGGCGAGGTG-3′ for BS8. To make the pax2/5/8.a > kaede plasmid, the 4.4-kb upstream region of Ciona pax2/5/8.a (Gene Model ID KH.S545.1/KH.S1363.2; Imai et al., 2004) was amplified from the genomic DNA of C. intestinalis type A by PCR using a pair of nucleotide primers (5′-CGACTCTAGAGGATCCGTGATTGTTACGTGG-3′ and 5′-TGGGGATCAGCAATGGATCCCCTTGCGGCC-3′), digested with BamHI, and then inserted into the BamHI site of the pSP-Kaede vector. Plasmid DNA constructs were electroporated into fertilized Ciona eggs as described by Corbo et al. (1997b).
Immunofluorescence Staining
Immunofluorescent staining was carried out according to the method described by Nishitsuji et al. (2012). To visualize the localization of Kaede, a rabbit anti-Kaede polyclonal antibody (PM012; Medical & Biological Laboratories, Nagoya, Japan; for Kaede) was diluted 1:1000 in 10% goat serum in T-PBS (0.1% Triton X-100 in PBS) and used as the primary antibody. The secondary antibody was an Alexa Fluor 488-conjugated anti-rabbit IgG (A11008; Thermo Fisher Scientific, Waltham, MA, United States). Fluorescent images were obtained by using a laser scanning confocal microscope (FV1200 IX83; Olympus, Tokyo, Japan). Confocal images were collected at 1-μm intervals in the z-axis.
Whole-Embryo Single-Cell Transcriptomic Analysis
A published single-cell transcriptome dataset of mid-tailbud embryos obtained using the 10x Genomics Chromium system (Horie T. et al., 2018; Horie R. et al., 2018; Cao et al., 2019) was used to analyze expression profiles of genes in the midline tissues. The dataset is available through GEO (GSE120035): https://www.ncbi.nlm.nih.gov/geo/query/acc.cgi?acc=GSE120035. The t-distributed stochastic neighbor embedding (t-SNE) analysis was performed using the Loupe Cell Browser 3.1.1 software (10x Genomics, Pleasanton, CA, United States). The processed data in a Loupe Cell Browser file (.cloupe) is available through the Mendeley data repository: http://dx.doi.org/10.17632/n4pxpr28cb.1. Differentially expressed genes were identified and ranked by statistical significance as previously described (Horie T. et al., 2018).
Time-Lapse Live Imaging and Photo-Conversion of Kaede
Embryos electroporated with pax2/5/8.a > kaede were reared in ASW and mounted on a glass slide with ASW containing 1.5% methylcellulose at 10 hpf. Photoconversion of Kaede was performed as described (Oonuma et al., 2016). Fluorescent images were taken every 15 min for 12.5 h at 18°C by using a laser scanning confocal microscope (FV1200 IX83; Olympus, Japan). Confocal images were collected at 1-μm intervals in the z-axis.
Data Availability Statement
The original contributions presented in the study are included in the article/Supplementary Materials, further inquiries can be directed to the corresponding author.
Author Contributions
TK conceived the project and wrote the manuscript. KO, KS, TH, and TK designed the experiments. KO, MY, NM, NO, MM, ST, HS, EN, YH, and KS performed the experiments. TH, ST, MS, and TK analyzed the single-cell RNA-seq data. TH provided the essential materials. KO, NO, and TK analyzed and interpreted the data. KO, TH, and TK edited the manuscript. All authors reviewed the manuscript.
Funding
This study was supported in part by Grants-in-Aid for Scientific Research from JSPS to TK (16H04724 and 19H03213), KO (17K15130 and 19K16150), and TH (16K07433 and 19H03204). This study was also supported in part by research grants from the Takeda Science Foundation and the Hirao Taro Foundation of KONAN GAKUEN for Academic Research.
Conflict of Interest
The authors declare that the research was conducted in the absence of any commercial or financial relationships that could be construed as a potential conflict of interest.
Acknowledgments
We thank the National BioResource Project of MEXT and all members of the Maizuru Fisheries Research Station and Yutaka Satou Lab of Kyoto University and the Misaki Marine Biological Station of the University of Tokyo for providing us with C. intestinalis adults. We also thank the Graduate School of Maritime Sciences, Kobe University for generously allowing us to collect C. intestinalis on the campus.
Supplementary Material
The Supplementary Material for this article can be found online at: https://www.frontiersin.org/articles/10.3389/fcell.2021.704367/full#supplementary-material
Supplementary Video 1 | Time-lapse of an embryo showing migration of mesenchyme cells labeled with photo-converted Kaede shown in Figure 5.
References
Ando, R., Hama, H., Yamamoto-Hino, M., Mizuno, H., and Miyawaki, A. (2002). An optical marker based on the UV-induced green-to-red photoconversion of a fluorescent protein. Proc. Natl. Acad. Sci. U.S.A. 99, 12651–12656. doi: 10.1073/pnas.202320599
Appel, B., Fritz, A., Westerfield, M., Grunwald, D. J., Eisen, J. S., and Riley, B. B. (1999). Delta-mediated specification of midline cell fates in zebrafish embryos. Curr. Biol. 9, 247–256. doi: 10.1016/s0960-9822(99)80113-4
Cao, C., Lemaire, L. A., Wang, W., Yoon, P. H., Choi, Y. A., Parsons, L. R., et al. (2019). Comprehensive single-cell transcriptome lineages of a proto-vertebrate. Nature 571, 349–354. doi: 10.1038/s41586-019-1385-y
Chen, X., Ji, Z., Webber, A., and Sharrocks, A. D. (2016). Genome-wide binding studies reveal DNA binding specificity mechanisms and functional interplay amongst Forkhead transcription factors. Nucleic Acids Res. 44, 1566–1578. doi: 10.1093/nar/gkv1120
Cleaver, O., and Krieg, P. A. (1998). VEGF mediates angioblast migration during development of the dorsal aorta in Xenopus. Development 125, 3905–3914. doi: 10.1242/dev.125.19.3905
Cleaver, O., Tonissen, K. F., Saha, M. S., and Krieg, P. A. (1997). Neovascularization of the Xenopus embryo. Dev. Dyn. 210, 66–77.
Corbo, J. C., Erives, A., Di Gregorio, A., Chang, A., and Levine, M. (1997a). Dorsovental patterning of the vertebrate neural tube is conserved in a protochordate. Development 124, 2335–2344. doi: 10.1242/dev.124.12.2335
Corbo, J. C., Levine, M., and Zeller, R. W. (1997b). Characterization of a notochord-specific enhancer from the Brachyury promoter region of the ascidian, Ciona intestinalis. Development 124, 589–602. doi: 10.1242/dev.124.3.589
Dal-Pra, S., Thisse, C., and Thisse, B. (2011). FoxA transcription factors are essential for the development of dorsal axial structures. Dev. Biol. 350, 484–495. doi: 10.1016/j.ydbio.2010.12.018
Danos, M. C., and Yost, H. J. (1996). Role of notochord in specification of cardiac left-right orientation in zebrafish and Xenopus. Dev. Biol. 177, 96–103. doi: 10.1006/dbio.1996.0148
Eriksson, J., and Löfberg, J. (2000). Development of the hypochord and dorsal aorta in the zebrafish embryo (Danio rerio). J. Morphol. 244, 167–176. doi: 10.1002/(sici)1097-4687(200006)244:3<167::aid-jmor2>3.0.co;2-j
Franz, K. (1898). Über die entwicklung von hypochorda und Ligamentum longitudinale ventrale bei Teleostiern. Morphol. Jahrb. 25, 143–155.
Frazer, K. A., Pachter, L., Poliakov, A., Rubin, E. M., and Dubchak, I. (2004). VISTA: computational tools for comparative genomics. Nucleic Acids Res. 32, W273–W279.
Friedman, J. R., and Kaestner, K. H. (2006). The Foxa family of transcription factors in development and metabolism. Cell Mol. Life Sci. 63, 2317–2328. doi: 10.1007/s00018-006-6095-6
Gibson, W. T. (1910). The development of the hypochord in Raia batis; with a note upon the occurrence of the epibranchial groove in amniote embryos. Anat. Anz. 35, 407–428.
Goodman, A., Goode, B. L., Matsudaira, P., and Fink, G. R. (2003). The Saccharomyces cerevisiae calponin/transgelin homolog Scp1 functions with fimbrin to regulate stability and organization of the actin cytoskeleton. Mol. Biol. Cell 14, 2617–2629. doi: 10.1091/mbc.e03-01-0028
Hatta, S. (1893). On the formation of the germinal layers in Petromyzon. J. Coll. Sci. Imp. Univ. Jpn. 5, 129–147.
Hirano, T., and Nishida, H. (2000). Developmental fates of larval tissues after metamorphosis in the ascidian, Halocynthia roretzi. II. Origin of endodermal tissues of the juvenile. Dev. Genes Evol. 210, 55–63. doi: 10.1007/s004270050011
Hogan, K. A., and Bautch, V. L. (2004). Blood vessel patterning at the embryonic midline. Curr. Top. Dev. Biol. 62, 55–85. doi: 10.1016/s0070-2153(04)62003-5
Horie, R., Hazbun, A., Chen, K., Cao, C., Levine, M., and Horie, T. (2018). Shared evolutionary origin of vertebrate neural crest and cranial placodes. Nature 560, 228–232. doi: 10.1038/s41586-018-0385-7
Horie, T., Horie, R., Chen, K., Cao, C., Nakagawa, M., Kusakabe, T. G., et al. (2018). Regulatory cocktail for dopaminergic neurons in a protovertebrate identified by whole-embryo single-cell transcriptomics. Genes Dev. 32, 1297–1302. doi: 10.1101/gad.317669.118
Hotta, K., Takahashi, H., Satoh, N., and Gojobori, T. (2008). Brachyury-downstream gene sets in a chordate, Ciona intestinalis: integrating notochord specification, morphogenesis and chordate evolution. Evol. Dev. 10, 37–51. doi: 10.1111/j.1525-142x.2007.00212.x
Hozumi, A., Kawai, N., Yoshida, R., Ogura, Y., Ohta, N., Satake, H., et al. (2010). Efficient transposition of a single Minos transposon copy in the genome of the ascidian Ciona intestinalis with a transgenic line expressing transposase in eggs. Dev. Dyn. 239, 1076–1088. doi: 10.1002/dvdy.22254
Imai, K. S., Hino, K., Yagi, K., Satoh, N., and Satou, Y. (2004). Gene expression profiles of transcription factors and signaling molecules in the ascidian embryo: towards a comprehensive understanding of gene networks. Development 131, 4047–4058. doi: 10.1242/dev.01270
Imai, K. S., Levine, M., Satoh, N., and Satou, Y. (2006). Regulatory blueprint for a chordate embryo. Science 312, 1183–1187. doi: 10.1126/science.1123404
Imai, K. S., Stolfi, A., Levine, M., and Satou, Y. (2009). Gene regulatory networks underlying the compartmentalization of the Ciona central nervous system. Development 136, 285–293. doi: 10.1242/dev.026419
Islam, A. F. M. T., Moly, P. K., Miyamoto, Y., and Kusakabe, T. G. (2010). Distinctive expression patterns of Hedgehog pathway genes in the Ciona intestinalis. Zool. Sci. 27, 84–90. doi: 10.2108/zsj.27.84
José-Edwards, D. S., Oda-Ishii, I., Kugler, J. E., Passamaneck, Y. J., Katikala, L., Nibu, Y., et al. (2015). Brachyury, Foxa2 and the cis-regulatory origins of the notochord. PLoS Genet. 11:e1005730. doi: 10.1371/journal.pgen.1005730
Klaatsch, H. (1898). Zur Frage nach der morphologiscen Bedeutung der Hypochorda. Morphol. Jahrb. 25, 156–169.
Kowalevsky, A. (1866). Entwicklungsgeschichte der einfachen Ascidien. Mem. l’Acad. St. Petersbourg. 7, 11–19.
Kowalevsky, A. (1867). Entwicklungsgeschichte des Amphioxus lanceolatus. Mem. l’Acad. St. Petersbourg 11, 1–17.
Kubo, A., Suzuki, N., Yuan, X., Nakai, K., Satoh, N., Imai, K. S., et al. (2010). Genomic cis-regulatory networks in the early Ciona intestinalis embryo. Development 137, 1613–1623. doi: 10.1242/dev.046789
Latimer, A. J., and Appel, B. (2006). Notch signaling regulates midline cell specification and proliferation in zebrafish. Dev. Biol. 298, 392–402. doi: 10.1016/j.ydbio.2006.05.039
Latimer, A. J., Dong, X., Markov, Y., and Appel, B. (2002). Delta-Notch signaling induces hypochord development in zebrafish. Development 129, 2555–2563. doi: 10.1242/dev.129.11.2555
Leydig, F. (1852). Beträge zur Mikroskopischen Anatomie und Entwicklungsgeschichte der rochen und Haie. Leipzig: Wilhelm Engelmann.
Löfberg, J., and Collazo, A. (1997). Hypochord, an enigmatic embryonic structure: study of the axolotl embryo. J. Morphol. 232, 57–66. doi: 10.1002/(sici)1097-4687(199704)232:1<57::aid-jmor3>3.0.co;2-l
Lohr, J. L., Danos, M. C., and Yost, H. J. (1997). Left-right asymmetry of a nodal-related gene is regulated by dorsoanterior midline structures during Xenopus development. Development 124, 1465–1472. doi: 10.1242/dev.124.8.1465
Melby, A. E., Warga, R. M., and Kimmel, C. B. (1996). Specification of cell fates at the dorsal margin of the zebrafish gastrula. Development 122, 2225–2237. doi: 10.1242/dev.122.7.2225
Nakazawa, K., Yamazawa, T., Moriyama, Y., Ogura, Y., Kawai, N., Sasakura, Y., et al. (2013). Formation of the digestive tract in Ciona intestinalis includes two distinct morphogenic processes between its anterior and posterior parts. Dev. Dyn. 242, 1172–1183. doi: 10.1002/dvdy.24009
Nishitsuji, K., Horie, T., Ichinose, A., Sasakura, Y., Yasuo, H., and Kusakabe, T. G. (2012). Cell lineage and cis-regulation for a unique GABAergic/glycinergic neuron type in the larval nerve cord of the ascidian Ciona intestinalis. Dev. Growth Differ. 54, 177–186. doi: 10.1111/j.1440-169x.2011.01319.x
Oonuma, K., and Kusakabe, T. G. (2019). Spatio-temporal regulation of Rx and mitotic patterns shape the eye-cup of the photoreceptor cells in Ciona. Dev. Biol. 445, 245–255. doi: 10.1016/j.ydbio.2018.11.011
Oonuma, K., Tanaka, M., Nishitsuji, K., Kato, Y., Shimai, K., and Kusakabe, T. G. (2016). Revised lineage of larval photoreceptor cells in Ciona reveals archetypal collaboration between neural tube and neural crest in sensory organ formation. Dev. Biol. 420, 178–185. doi: 10.1016/j.ydbio.2016.10.014
Passamaneck, Y. J., Katikala, L., Perrone, L., Dunn, M. P., Oda-Ishii, I., and Di Gregorio, A. (2009). Direct activation of a notochord cis-regulatory module by Brachyury and FoxA in the ascidian Ciona intestinalis. Development 136, 3679–3689. doi: 10.1242/dev.038141
Peyrot, S. M., Wallingford, J. B., and Harland, R. M. (2011). A revised model of Xenopus dorsal midline development: differential and separable requirements for Notch and Shh signaling. Dev. Biol. 352, 254–266. doi: 10.1016/j.ydbio.2011.01.021
Placzek, M., and Briscoe, J. (2005). The floor plate: multiple cells, multiple signals. Nat. Rev. Neurosci. 6, 230–240. doi: 10.1038/nrn1628
Placzek, M., Tessier-Lavigne, M., Jessell, T., and Dodd, J. (1990a). Orientation of commissural axons in vitro in response to a floor plate-derived chemoattractant. Development 110, 19–30. doi: 10.1242/dev.110.1.19
Placzek, M., Tessier-Lavigne, M., Yamada, T., Jessell, T., and Dodd, J. (1990b). Mesodermal control of neural cell identity: floor plate induction by the notochord. Science 250, 985–988. doi: 10.1126/science.2237443
Prinjha, R. K., Shapland, C. E., Hsuan, J. J., Totty, N. F., Mason, I. J., and Lawson, D. (1994). Cloning and sequencing of cDNAs encoding the actin cross-linking protein transgelin defines a new family of actin-associated proteins. Cell Motil. Cytoskeleton 28, 243–255. doi: 10.1002/cm.970280307
Reeves, W. M., Shimai, K., Winkley, K. M., and Veeman, M. T. (2021). Brachyury controls Ciona notochord fate as part of a feed-forward network. Development 148:dev195230.
Ruiz i Altaba, A. (1998). Combinatorial Gli gene function in floor plate and neuronal inductions by Sonic hedgehog. Development 125, 2203–2212. doi: 10.1242/dev.125.12.2203
Satoh, N., Rokhsar, D., and Nishikawa, T. (2014). Chordate evolution and the three-phylum system. Proc. R. Soc. B 281:20141729. doi: 10.1098/rspb.2014.1729
Satou, Y., Kawashima, T., Shoguchi, E., Nakayama, A., and Satoh, N. (2005). An integrated database of the ascidian, Ciona intestinalis: towards functional genomics. Zool. Sci. 22, 837–843. doi: 10.2108/zsj.22.837
Satou, Y., Mineta, K., Ogasawara, M., Sasakura, Y., Shoguchi, E., Ueno, K., et al. (2008). Improved genome assembly and evidence-based global gene model set for the chordate Ciona intestinalis: new insight into intron and operon populations. Genome Biol. 9:R152.
Satou, Y., Yamada, L., Mochizuki, Y., Takatori, N., Kawashima, T., Sasaki, A., et al. (2002). A cDNA resource from the basal chordate Ciona intestinalis. Genesis 33, 153–154.
Shi, W., Peyrot, S. M., Munro, E., and Levine, M. (2009). FGF3 in the floor plate directs notochord convergent extension in the Ciona tadpole. Development 136, 23–28. doi: 10.1242/dev.029157
Shih, J., and Fraser, S. E. (1995). Distribution of tissue progenitors within the shield region of the zebrafish gastrula. Development 121, 2755–2765. doi: 10.1242/dev.121.9.2755
Stemple, D. L. (2005). Structure and function of the notochord: an essential organ for chordate development. Development 132, 2503–2512. doi: 10.1242/dev.01812
Stöhr, P. (1895). Über die Entwicklung der Hypochorda und des dorsalen Pankreas bei Rana temporaria. Morphol. Jahrb. 23, 123–141.
Takatori, N., Satou, Y., and Satoh, N. (2002). Expression of hedgehog genes in Ciona intestinalis embryos. Mech. Dev. 116, 235–238. doi: 10.1016/s0925-4773(02)00150-8
Teillet, M.-A., Lapointe, F., and Le Douarin, N. M. (1998). The relationships between notochord and floor plate in vertebrate development revisited. Proc. Natl. Acad. Sci. USA 95, 11738–11738.
Tendeng, C., and Houart, C. (2006). Cloning and embryonic expression of five distinct sfrp genes in the zebrafish Danio rerio. Gene Expr. Patterns 6, 761–771. doi: 10.1016/j.modgep.2006.01.006
Tessier-Lavigne, M., Placzek, M., Lumsden, A. G. S., Dodd, J., and Jessell, T. M. (1988). Chemotropic guidance of developing axons in the mammalian central nervous system. Nature 336, 775–778. doi: 10.1038/336775a0
Thisse, B., Pflumio, S., Fürthauer, M., Loppin, B., Heyer, V., Degrave, A., et al. (2001). Expression of the Zebrafish Genome During Embryogenesis. (NIH RO1 RR15402-01). ZFIN Direct Data Submission. Available online at: https://zfin.org/ZDB-PUB-010810-1. (accessed May 21, 2021).
Yan, Y. L., Hatta, K., Riggleman, B., and Postlethwait, J. H. (1995). Expression of a type II collagen gene in the zebrafish embryonic axis. Dev. Dyn. 203, 363–376. doi: 10.1002/aja.1002030308
Yasuo, H., and Satoh, N. (1993). Function of vertebrate T gene. Nature 364, 582–583. doi: 10.1038/364582b0
Keywords: ascidian, Sonic hedgehog, floor plate, endodermal strand, Ciona intestinalis sp. A, notochord, FoxA transcription factors, hypochord
Citation: Oonuma K, Yamamoto M, Moritsugu N, Okawa N, Mukai M, Sotani M, Tsunemi S, Sugimoto H, Nakagome E, Hasegawa Y, Shimai K, Horie T and Kusakabe TG (2021) Evolution of Developmental Programs for the Midline Structures in Chordates: Insights From Gene Regulation in the Floor Plate and Hypochord Homologues of Ciona Embryos. Front. Cell Dev. Biol. 9:704367. doi: 10.3389/fcell.2021.704367
Received: 02 May 2021; Accepted: 25 May 2021;
Published: 21 June 2021.
Edited by:
Salvatore D’Aniello, Stazione Zoologica Anton Dohrn, ItalyReviewed by:
Honoo Satake, Suntory Foundation for Life Sciences, JapanAlberto Stolfi, Georgia Institute of Technology, United States
Copyright © 2021 Oonuma, Yamamoto, Moritsugu, Okawa, Mukai, Sotani, Tsunemi, Sugimoto, Nakagome, Hasegawa, Shimai, Horie and Kusakabe. This is an open-access article distributed under the terms of the Creative Commons Attribution License (CC BY). The use, distribution or reproduction in other forums is permitted, provided the original author(s) and the copyright owner(s) are credited and that the original publication in this journal is cited, in accordance with accepted academic practice. No use, distribution or reproduction is permitted which does not comply with these terms.
*Correspondence: Takehiro G. Kusakabe, dGdrQGtvbmFuLXUuYWMuanA=