- 1Department of Hematology, Zhongnan Hospital of Wuhan University, Wuhan, China
- 2Frontier Science Center for Immunology and Metabolism, Medical Research Institute, Wuhan University, Wuhan, China
Recombination activating genes 1 (Rag1) and Rag2 are expressed in immature lymphocytes and essential for generating the vast repertoire of antigen receptors. Yet, the mechanisms governing the transcription of Rag1 and Rag2 remain to be fully determined, particularly in thymocytes. Combining cDNA microarray and ChIP-seq analysis, we identify Rag1 and Rag2 as novel Notch1 transcriptional targets in acute T-cell lymphoblastic leukemia (T-ALL) cells. We further demonstrate that Notch1 transcriptional complexes directly bind the Rag1 and Rag2 locus in not only T-ALL but also primary double negative (DN) T-cell progenitors. Specifically, dimeric Notch1 transcriptional complexes activate Rag1 and Rag2 through a novel cis-element bearing a sequence-paired site (SPS). In T-ALL and DN cells, dimerization-defective Notch1 causes compromised Rag1 and Rag2 expression; conversely, dimerization-competent Notch1 achieves optimal upregulation of both. Collectively, these results reveal Notch1 dimerization-mediated transcription as one of the mechanisms for activating Rag1 and Rag2 expression in both primary and transformed thymocytes. Our data suggest a new role of Notch1 dimerization in compelling efficient TCRβ rearrangements in DN progenitors during T-cell development.
Introduction
Notch receptors are a family of heterodimeric transmembrane proteins that determine cell fate decisions and growth control. A prime example is that Notch1, one of the four isoforms (Notch1–4), plays an essential role in T-cell development and homeostasis. From a surface protein to a nuclear transcription factor, Notch1 signaling becomes active upon interaction with the Delta and Serrate family of ligands (Delta-like 1, 3, and 4; and Jagged 1 and 2) on juxtaposed cells. This interaction triggers conformational changes of Notch1, allows stepwise proteolytic cleavages, and releases intracellular Notch1 (ICN1). ICN1 then rapidly translocates into the nucleus and forms a transcriptional activation complex with the DNA binding factor RBPJ (also known as CSL) and coactivator of the mastermind family (MAML) (Kopan and Ilagan, 2009). As previously reported, ICN1/RBPJ/MAML complexes dimerize in the nucleus through cooperatively assembling on a specific sequence-paired site (SPS) in the promoter/enhancer of target genes, where the ANK domain within ICN1 mediates the dimer formation (Nam et al., 2007; Arnett et al., 2010).
Notch1 serves as a key regulator required at multiple stages for T-cell progenitor specification and maturation. Notch1 signaling determines T-cell lineage commitment and is essential to generate the early T-cell progenitors (ETP) from multipotent lymphoid progenitors (Sambandam et al., 2005; Tan et al., 2005). Inactivation of Notch1 signaling in hematopoietic progenitors results in a complete failure of T-cell generation, while it induces ectopic B-cell development in the thymus (Pui et al., 1999). Conversely, Notch1 overexpression causes extrathymic T-cell development in bone marrow at the expense of B cells (Radtke et al., 1999). Continuous Notch1 signaling is imperative in lymphocyte developmental progression through ETP, double negative 2 (DN2) and DN3 stages until completion of β-selection, a critical checkpoint where thymocytes successfully assemble pre-T-cell receptors (pre-TCRs) composed of a pre-T-cell antigen receptor α (pTα) chain and a functional TCRβ chain (Radtke et al., 2010). Notch1 expression drastically decreases after β-selection when thymocytes advance to double positive (DP) stage. During the progression, Notch1 provides essential differentiation, proliferation, survival, and metabolic signals, mainly via inducing the expression of downstream targets such as Tcf1 (Weber et al., 2011), Ptcra (Reizis and Leder, 2002), Myc (Weng et al., 2006), Il7r (Gonzales-Garcia et al., 2009), and Hes1 (Tomita et al., 1999; Kaneta et al., 2000). Notch1 dimerization appears essential in driving DN3 thymocytes through the β-selection, in part due to its ability to activate dimer-dependent target genes Ptcra and Myc (Liu et al., 2010).
When aberrantly activated, Notch acts as an oncogene driving acute T-cell lymphoblastic leukemia (T-ALL) (Aster et al., 2011; Liu et al., 2011; Paganin and Ferrando, 2011). Constitutive Notch1 expression in murine hematopoietic progenitors uniformly causes T-ALL (Pear et al., 1996). Moreover, the fact that more than half of the T-ALL patient samples bear gain-of-function Notch1 mutations suggests a central role of Notch1 in leukemogenesis (Weng et al., 2004). Consequently, enhanced Notch1 activity activates a spectrum of downstream targets that mediate leukemogenesis including Myc (Weng et al., 2006), Hes1 (Real et al., 2009), Lef1 (Spaulding et al., 2007), and Deptor (Hu et al., 2017). Unlike wild-type (WT) Notch1, dimer-disrupted allele failed to induce T-ALL in the mouse model, presumably due to the inactivation of dimer-responsive target Myc (Liu et al., 2010). It remains to be determined any additional Notch1 targets sensitive to dimeric Notch1 signaling.
Rag1 and Rag2 encode site-specific endonucleases to create double-stranded breaks (DSB) in the process of V(D)J recombination to constitute the diverse repertoire of T-cell receptors and B-cell immunoglobulin (Ig) receptors in response to diverse exogenous pathogens (Helmink and Sleckman, 2012; Miyazaki et al., 2020). Mice deficient in either Rag1 or Rag2 expression are blocked in T- and B-cell differentiation and exhibit a severe combined immunodeficiency (SCID) phenotype (Mombaerts et al., 1992; Shinkai et al., 1992). Expression of Rag1 and Rag2 is tightly controlled through a variety of DNA cis-elements and trans-factors, frequently in a T- or B-cell-specific manner (Kuo and Schlissel, 2009). Aberrant Rag1/2 activity is linked to oncogenic translocations associated with immature human and mouse B and T leukemia/lymphoma (Zhang et al., 2010).
In the present study, we identified Rag1 and Rag2 as Notch1 downstream targets from the gene expression profiling analysis in murine T-ALL T6E cells. Further mechanistic analyses reveal that ICN1 directly binds the Rag2 locus to activate transcription, where we uncover a novel cis-element specifically recognized by the dimeric ICN1/RBPJ/MAML1 complexes. The Notch1-dimer dependency is not only restricted to T-ALL cells but also observed in DN progenitors, suggesting a new role of Notch1 in the developmental stage prior to β-selection−advancing TCRβ rearrangement. We thereby present a novel mechanism of Notch1-mediated transcriptional activation of Rag1 and Rag2, involved in both T-cell development and leukemia.
Results
Recombination Activation Gene 1 and 2 Are Regulated by Dimeric Notch Signaling
Although the dimerization of Notch1 transcriptional complex is required for T-cell development and leukemogenesis (Liu et al., 2010; Severson et al., 2017), identities and the functional importance of Notch1 dimer-responsive targets remain largely unknown. To disclose novel dimeric Notch1 targets, we reanalyzed a genome-wide gene expression profiling (GSE97465) in a Notch1-dependent murine T-ALL cell line T6E (Severson et al., 2017). In this microarray data, T6E cells were infected with retroviruses expressing empty vector MigR1 with a surrogated GFP marker, MigR1 containing constitutive WT intracellular Notch1 (ICN1) or a dimerization-disrupted mutant ICN1 R1984A (R1984A) (Liu et al., 2010). Candidate genes which were efficiently induced by WT ICN1 but not ICN1 R1984A caught our attention. Filtered gene sets were aligned with fold changes to generate the heatmap of differential genes in response to Notch1 dimer and monomer (Figure 1A). While ICN1 R1984A upregulated many known Notch1 targets, including Dtx1 and Nrarp, it failed to turn on a member of dimer-dependent genes, including previously reported Myc, Hes1, and Deptor (Liu et al., 2010; Hu et al., 2017). Of particular note, Rag1 and Rag2 rank at the top to be most differentially regulated by ICN1 and R1984A (Figure 1A).
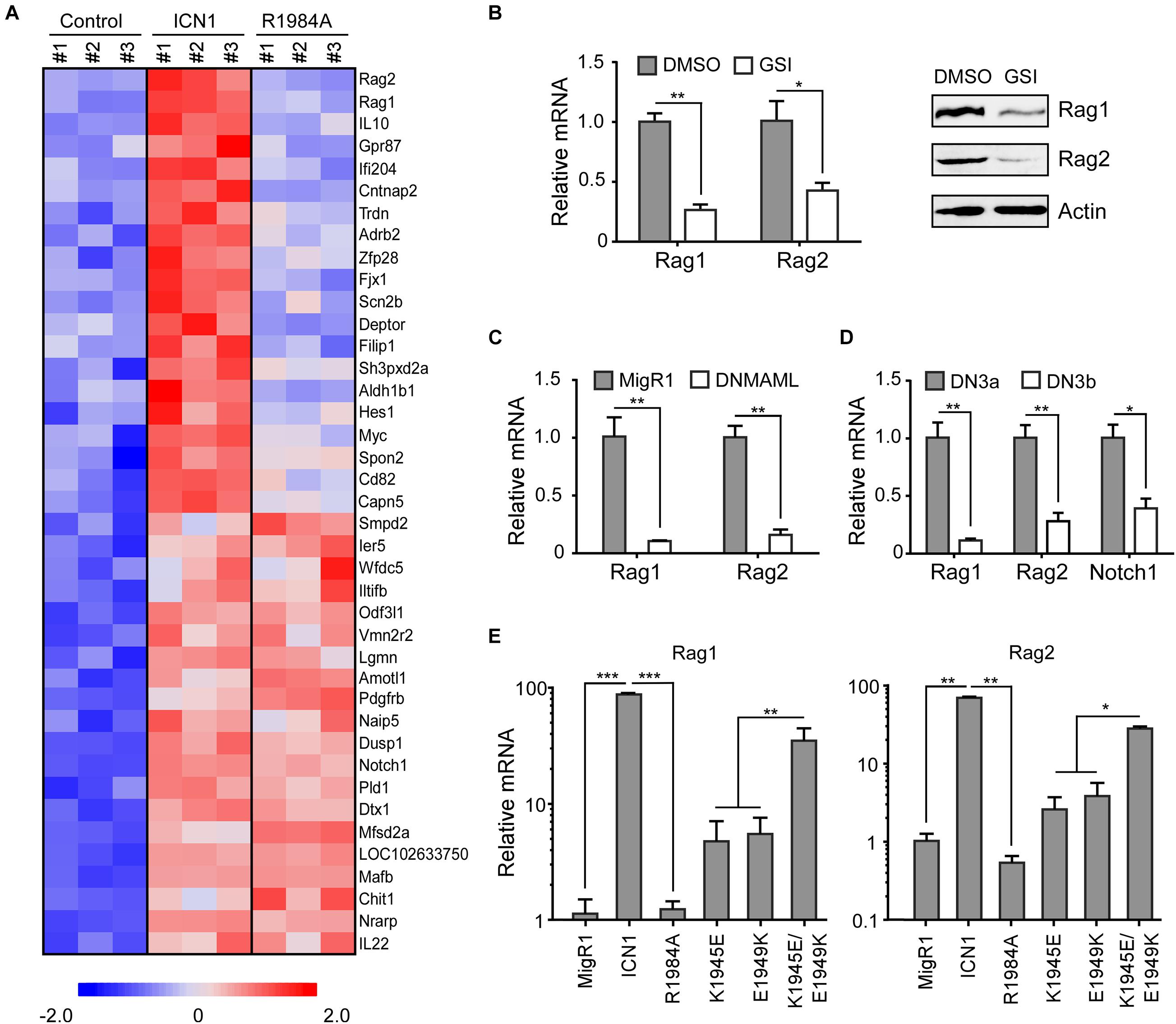
Figure 1. Notch signaling induces Rag1 and Rag2 expression. (A) Identification of Notch1 dimer-dependent target genes. Notch1-dependent genes were determined from a microarray analysis in murine T-ALL T6E cells (GSE97465). Each gene expression is displayed with colored boxes which are scaled in the bottom. ICN1 expression means Notch1 monomer and dimer activation, and R1984A expression denotes only Notch1 monomer activation. Expression data from 20 genes most strictly dependent on the Notch1 dimer and 20 genes equally induced by both Notch1 dimer and monomer are depicted (p < 0.05). (B) T6E cells were treated with JC-18 (1 μM) or DMSO for 24 h. Rag1 and Rag2 mRNA and proteins were detected by real-time PCR (left) and immunoblots (right). (C) T6E cells were transduced with DNMAML1 or empty vector MigR1 surrogated with a GFP marker for 24 h. GFP+ cells were sorted and subjected to mRNA analysis by real-time PCR. (D) DN3a and DN3b cells were isolated from C57BL/6 mice. Rag1 and Rag2 mRNA, along with positive control Notch1 mRNA, were evaluated by real-time PCR. (E) T6E cells were infected by retroviruses expressing MigR1, WT ICN1, or designated dimerization mutants, then subjected to JC-18 treatment for 24 h. Rag1 and Rag2 mRNA levels were examined by real-time PCR. Above all, the expression of Rag1 and Rag2 relative to 18S RNA is shown as the mean of values from triplicate wells ± SD. Representative data from three independent experiments are shown. ***p < 0.001, **p < 0.01, and *p < 0.05.
Since ICN1 dramatically increased the expression of Rag1 and Rag2 as compared with the vector alone, we first confirmed whether their expression depends on Notch1 by analyzing the mRNA levels in various Notch1 inhibitory scenarios. γ-Secreatse inhibitor (GSI) JC-18 treatment, which suppresses Notch activation, in T6E cells resulted in decreased mRNA abundance of Rag1 and Rag2 (Figure 1B, left). Consistently, we detected lower Rag1 and Rag2 protein levels in T6E cells upon Notch inhibition (Figure 1B, right). As a further support, GSI treatment in human T-ALL KOPTK1 and CUTLL1 cells resulted in a significant decrease in mRNA and protein levels of RAG1 and RAG2 (Supplementary Figures 1A,B). We next expressed a dominant negative MAML1 (DNMAML1) to specifically repress the Notch1 pathway and observed sharp decreases of Rag1 and Rag2 as well (Figure 1C). Using primary murine thymocytes, we then investigated a physiological condition whereby Notch1 is downregulated. At the checkpoint of T-cell receptor β-selection, DN3a cells differentiate to DN3b cells, while Notch1 undergoes a dramatic decline upon the completion of TCRβ rearrangement. Correspondingly, Rag1 and Rag2 displayed similar reductions at the transition of DN3a to DN3b (Figure 1D).
The microarray data showed that both Rag1 and Rag2 were activated by ICN1 but not by dimerization-disrupted mutants R1984A (Figure 1A). To validate these data, we took advantage of various ICN1 mutants that are involved in the dimer formation of ICN1/RBPJ/MAML1 complexes on DNA, scoring their effects on Rag1 and Rag2 expression in T6E cells (Liu et al., 2010). Retroviruses expressing empty vector MigR1, or MigR1-ICN1, R1984A, K1945E, E1949K, and K1945E/E1949K were infected to T6E cells, followed by 24 h JC-18 treatment to repress endogenous Notch1 activity. GFP+ cells were sorted to analyze Rag1 and Rag2 mRNA abundance. Consistent with the microarray data, both Rag1 and Rag2 were activated by ICN1 but not by dimerization-disrupted mutant R1984A (Figure 1E). Expression of K1945E or E1949K alone, which breaks the intermolecular salt bridge between Notch1 dimer (Nam et al., 2007), induced moderate Rag1 and Rag2 expression. Notably, the double mutant K1945E/E1949K, which restores electrostatic complementarity and thereby the critical salt bridge essential for dimer formation (Nam et al., 2007), reconstituted the WT ICN1 activity to stimulate the Rag1/2 mRNA expression (Figure 1E). Interestingly, we noticed that WT ICN1 but not dimerization-disrupted mutant R1984A was capable of activating the RAG1/2 mRNA expression in human KOPTK1 and Jurkat cells (Supplementary Figure 1B). Collectively, these data argue that Notch1 dimerization-mediated transcriptional activation is a prominent and conserved mechanism underlying Rag1 and Rag2 regulation in T-ALL cells.
Notch1 Directly Binds the Transcriptional Regulatory Region of Rag2 Gene
To determine if Notch1 transcriptional complex directly binds to and activates the gene expression, we first inspected the Rag1/2 locus from the chromatin immunoprecipitation sequencing (ChIP-seq) data which provide comprehensive Notch1 and RBPJ binding sites in the genome of T6E cells (Wang et al., 2011). Within the Rag locus where Rag1 and Rag2 are juxtaposed with opposite orientation, we detected strong signals of Notch1 and RBPJ binding in the upstream 5′ region of the Rag2 gene (Figure 2A). The tentative binding site locates at ∼2.8 kb from the transcription initiation site of the Rag2 gene and contained a previously characterized Rag2 P4 enhancer (Monroe et al., 1999; Wei et al., 2002). We reasoned that this enhancer region may include key cis-elements responsible for the Rag2 transcription (Figure 2A).
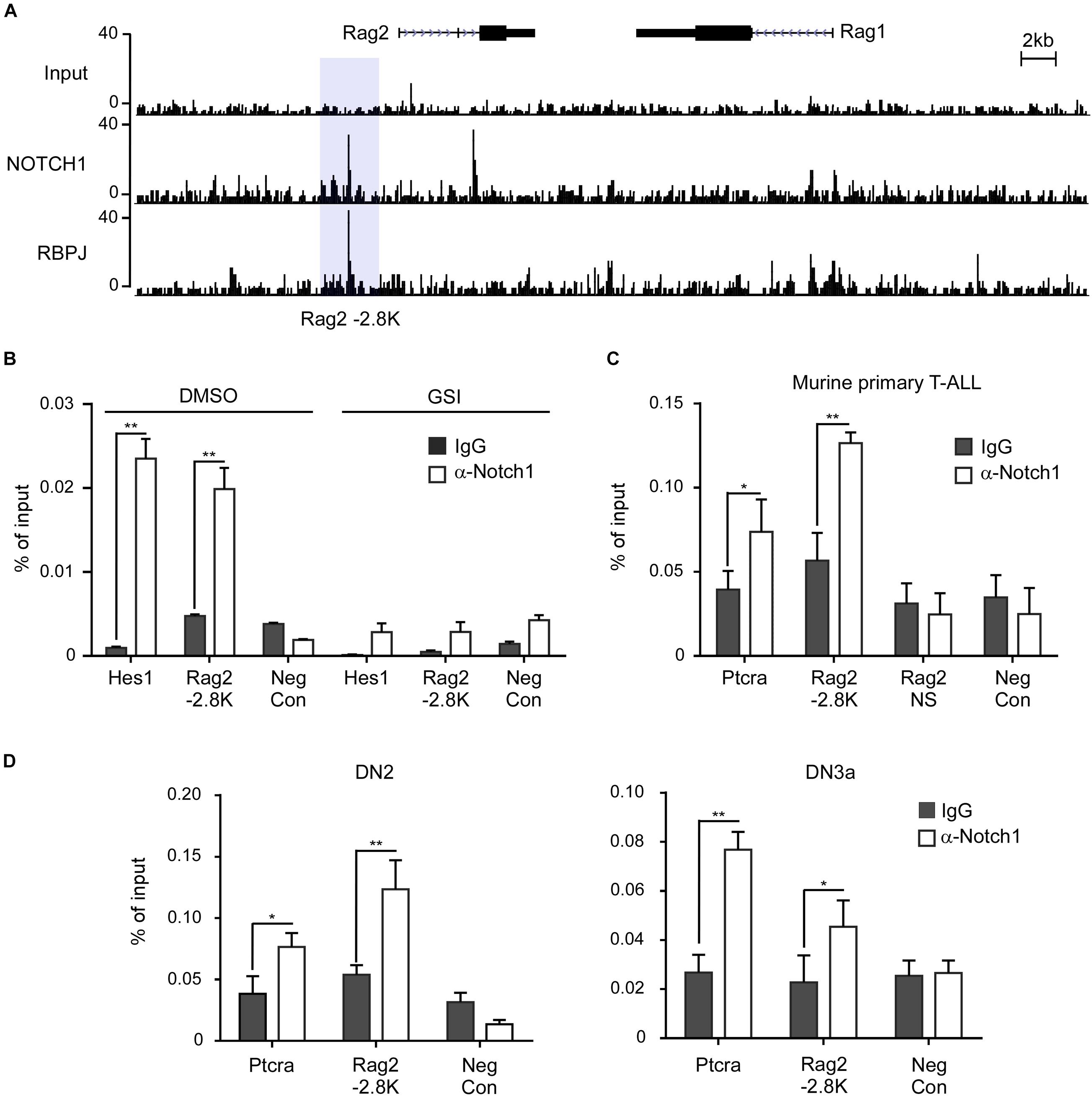
Figure 2. Notch1 directly binds the Rag1/2 locus in T-ALL cells and T-cell progenitors. (A) Analysis of the Rag2 locus in the ChIP-seq data (GSE 29600). The binding signals of Notch1 and RBPJ on the -2.8 kb site upstream of the Rag2 gene are highlighted in light gray. (B–D) Chromatin immunoprecipitation (ChIP) was performed on cross-linked fragmented DNAs prepared from T6E cells treated with DMSO or 1 μM JC-18 for 24 h (B), murine primary T-ALL samples (C), or murine DN2 and DN3a cells (D). Eluted DNAs were then analyzed by qPCR using primers flanking the -2.8 kb site. The amount of DNA amplified from immunoprecipitated DNAs was normalized to that amplified from input DNA. Each sample was prepared from at least two independent experiments and run in triplicates. Rag2 NS, non-specific sequence in Rag2 locus; Neg Con, non-specific sequence in the Nanog gene used as the negative control. **p < 0.01 and *p < 0.05.
ChIP assays were performed to validate Notch1 localization at the 5′ site upstream of Rag2 in T-ALL cells. In T6E cells, Notch1 was found to bind to the Rag2 -2.8 kb, like the well-characterized Notch1 binding site in the Hes1 promoter. DNA regions without RBPJ binding site were included as a negative control (Figure 2B). By inhibiting γ-secretase-mediated proteolytic cleavage of membranous Notch1, GSI treatment decreased the nuclear ICN1 abundance, thus reducing global occupancy of Notch1/RBPJ binding sites in the genome, including that at the Rag2 -2.8 kb site as well as the Hes1 promoter. We further examined the Notch1 occupancy in primary T-ALL cells derived from the Lck-Cre KrasG12D transgenic mice (Chiang et al., 2008). Ptcra, a Notch1 direct target gene encoding the pre-T-cell antigen receptor α (Reizis and Leder, 2002; Liu et al., 2010), was bound by Notch1 at its enhancer region. Similarly, Notch1 bound the Rag2 -2.8 kb site but not a non-specific region in the Rag2 locus, nor a Rag2 irrelevant DNA region bearing no RBPJ binding site shown as a negative control (Figure 2C).
When early T-cell progenitors migrate into the thymus, undergoing stepwise differentiation to mature T cells, Rag1 and Rag2 mRNA start to increase for TCRβ rearrangement. Their levels reach the peak at DN3a stage right prior to β-selection. Whether Notch1 plays a role to induce Rag1 and Rag2 at this developmental stage is not yet clarified. We examined the occupancy of Notch1 on the identified -2.8 kb site in the sorted DN2 and DN3a cells from C57BL/6 mice (Figure 2D). ChIP assays revealed the Notch1 binding at the Rag2 -2.8 kb in both DN2 and DN3a cells, similar to the previously characterized target gene Ptcra. Besides Notch1, RBPJ also occupied the same region (data not shown). In contrast, DNA sequences without RBPJ binding site, as the negative control, were not bound by either Notch1 or RBPJ. Taken together, these results provide strong evidence that Notch1 directly and specifically binds to the regulatory region upstream of Rag2.
We also searched the tentative binding sites of Notch1 and RBPJ on the RAG1/2 locus in human T-ALL cells from previously reported ChIP-seq data (Severson et al., 2017). It appears that the conserved binding sites around -2.8 kb of the Rag2 gene are not present in CUTLL1 cells (Supplementary Figure 2A). Instead, a prominent distal Notch1 binding signal, which was associated with the RBPJ and H3K27ac binding peaks, was detected at 87 kb upstream of the transcription initiation site of the RAG2. Of note, GSI treatment reduced the binding signal intensity of Notch1 and RBPJ. This Notch1 and, mostly likely, RAG2 distal enhancer interaction was validated by conventional ChIP assay (Supplementary Figure 2B). Our findings highly suggest that direct transcriptional activation of RAG1/2 by Notch1 is well conserved between mouse and human, and cis-elements within the -87 kb distal site in human T-ALL cells are responsible for the transcriptional activation.
The Rag2 Gene Locus Contains Paired Binding Sites in Response to Dimeric Notch1 Signaling
In the microarray analysis, Rag1 and Rag2 were shown specifically induced by WT ICN1 but not a dimerization-disrupted mutant R1984A (Figure 1A), suggesting they are Notch1 dimer dependent. Sequence search within the −2.8-kb region revealed a dimeric Notch1 recognizable sequence-paired site (SPS). The paired site consists of two RBPJ binding sites in the head-to-head orientation, separated by 16 nucleotides (Figure 3A). The canonical RBPJ binding site CATGGGAA was considered as a high affinity site, and the other non-canonical site CATGAGAA as a low affinity site based on the previous SPS definition (Arnett et al., 2010).
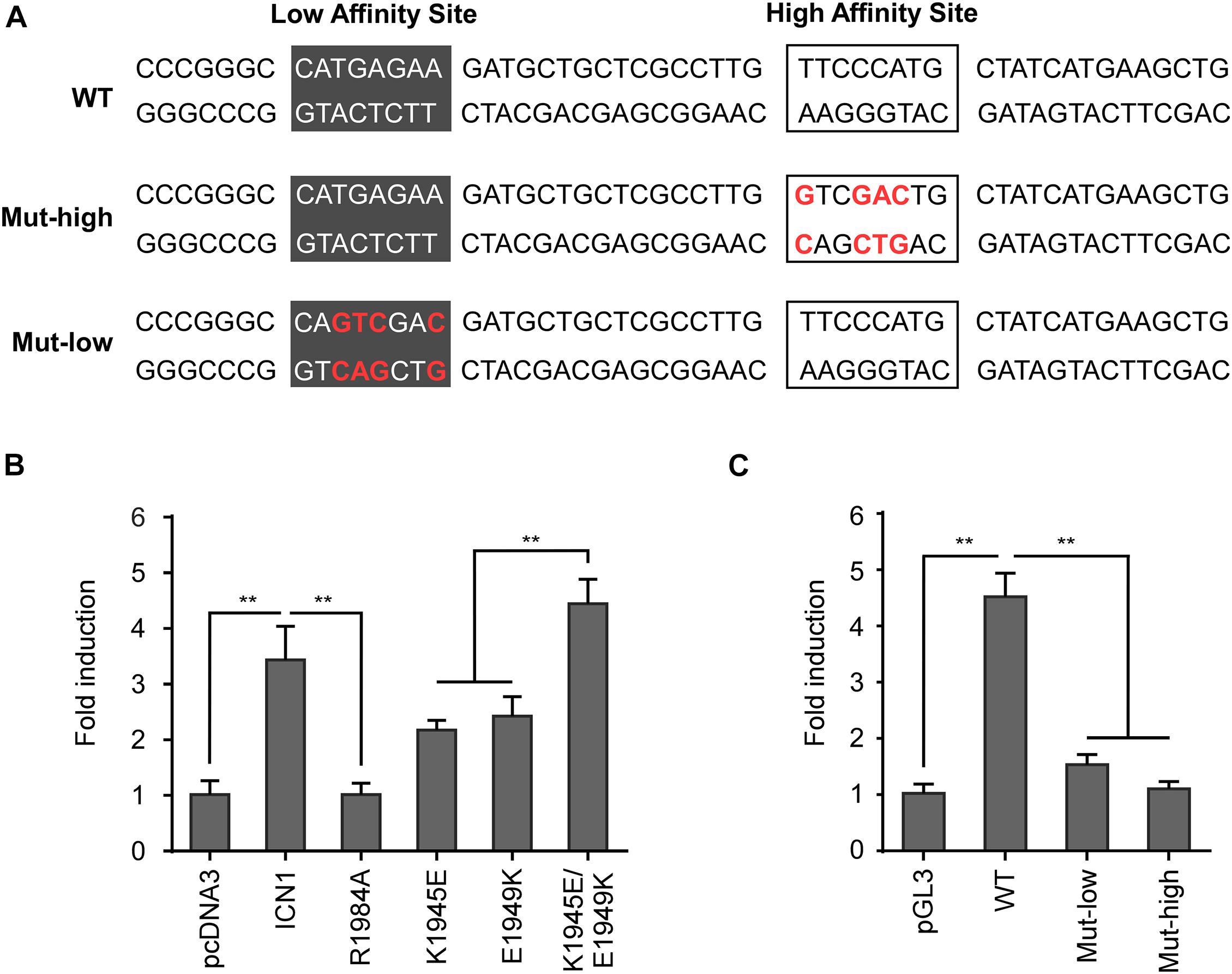
Figure 3. Dimeric Notch1 directly regulates Rag2 transcription. (A) Schematic presentation of the predicted mouse Rag2 SPS. The SPS, within the Rag2 enhancer, consists of a high affinity site (marked by frames) and a low affinity site (marked by dark gray). The high and low affinity sites were mutated as indicated (red), Mut-high and Mut-low, for the following luciferase assays. (B) The Rag2 SPS was constructed to luciferase reporter vector pGL3, then co-transfected with WT ICN1 or indicated mutants. Reporter activities normalized to an empty vector were determined and presented as fold induction. (C) The WT Rag2 SPS or indicated mutants were constructed to luciferase reporter vector pGL3, then co-transfected with WT ICN1. Reporter activities normalized to an empty vector were determined and presented as fold induction. All of the results were shown as the mean of values from triplicate wells ± SD. Data are representative of three independent experiments. ∗∗p < 0.01.
To functionally validate the putative SPS, we scored various dimerization mutants in a luciferase reporter assay. The putative Rag2 SPS was constructed upstream of a luciferase reporter and co-expressed with WT ICN1 or mutants. As expected, WT ICN1 induced a robust activation of the Rag2 reporter gene compared with the empty vector. In contrast, R1984A resulted in little reporter activity. The other two dimerization-incompetent mutants K1945E and E1949K, impairing the intermolecular connection between Notch1 dimer, also resulted in a considerable decrease of the luciferase activity. As expected, the double mutant in cis K1945E/E1949K, capable of reconstituting dimeric ICN1, rescued the reporter expression (Figure 3B). These data together indicate that Notch1 dimerization is required to activate Rag2 SPS-mediated transcription.
To directly confirm that Rag2 SPS is a functional cis-element required for Notch1-mediated transcriptional activation, we mutated the high affinity binding site or the low affinity site individually to examine the Rag2 SPS reporter gene activity. Previous reports suggest that both sites are required for cooperatively assembling dimeric complexes on DNA (Liu et al., 2010). Whereas ICN1 achieves full induction on WT SPS, mutations on either high affinity or low affinity site failed to induce the reporter gene expression (Figure 3C). Taken together, our results provide strong evidence that the identified murine Rag2 SPS is a bona fide specific and functional cis-element, bound by Notch1 transcriptional complex and required for Rag2 transcriptional activation. In human T-ALL cells, we noticed a highly conserved NOTCH1/RBPJ binding motif “GTGGGAA,” a canonical high affinity site, within the distal RAG2 regulatory DNA sequence, whereas a functional SPS site remained uncertain (Supplementary Figure 2C). It seems that cis-elements required for RAG1/2 transcriptional activation are not well-conserved between human and mouse, despite the analogous mechanism of Notch1-mediated transcriptional activation.
Although no SPS was revealed yet on the Rag1 transcriptional regulatory region, both endogenous Rag1 and Rag2 appeared to be regulated by Notch1 dimerization (Figure 1E), raising a question why Rag1 is sensitive to Notch1 dimer. Given Rag1 and Rag2 are coordinately regulated at the transcriptional level and asymmetrically disposed elements are mostly found at the 5′ of Rag2 gene (Kuo and Schlissel, 2009), the identified SPS is most likely one of the key cis-elements responsible for transcriptional activation of both recombination activating genes in vivo.
Enforced Dimeric Notch1 Expression in Hematopoietic Progenitors Induces Rapid Rag1/2 Expression
Co-expression of Rag1 and Rag2 during thymocyte development is essential for the assembly of functional genes encoding the TCR by recognizing and cleaving conserved recombination signal sequences located adjacent to clusters of V, D, and J gene segments (Zhang et al., 2006; Bosticardo et al., 2021). The mechanisms responsible for the lymphoid-specific and developmental stage-specific regulation of Rag1 and Rag2 expression are poorly understood. To determine if Rag1 and Rag2 were regulated by Notch1 dimerization in thymocyte development, we exploited NG-BAC mice, a Rag2 reporter strain in which a bacterial artificial chromosome (BAC) transgene expresses a GFP in place of Rag2, to investigate Rag2 transcription (Yu et al., 1999). Hematopoietic progenitors (Lin–Sca+c-Kit+, LSK cells) derived from NG-BAC mice were purified and transduced with retroviruses expressing the NGFR vector alone, ICN1, or the dimerization-disrupted mutant R1984A (Figure 4A). Transduced cells were subjected to in vitro co-culture with murine OP9 stromal cells. The Rag2 expression, reflected by GFP fluorescence, was analyzed. As early as 48 h post-transduction, Rag2 expression was detected in approximately 10% in ICN1 expressing (NGFR+) cells, whereas very few, if any, GFP+ cells were seen in the vector control cells (Figures 4B,C). Again, R1984A-transduced cells failed to induce Rag2 expression in this time frame, suggesting Rag2 expression is much more sensitive to Notch1 dimerization during thymocyte development.
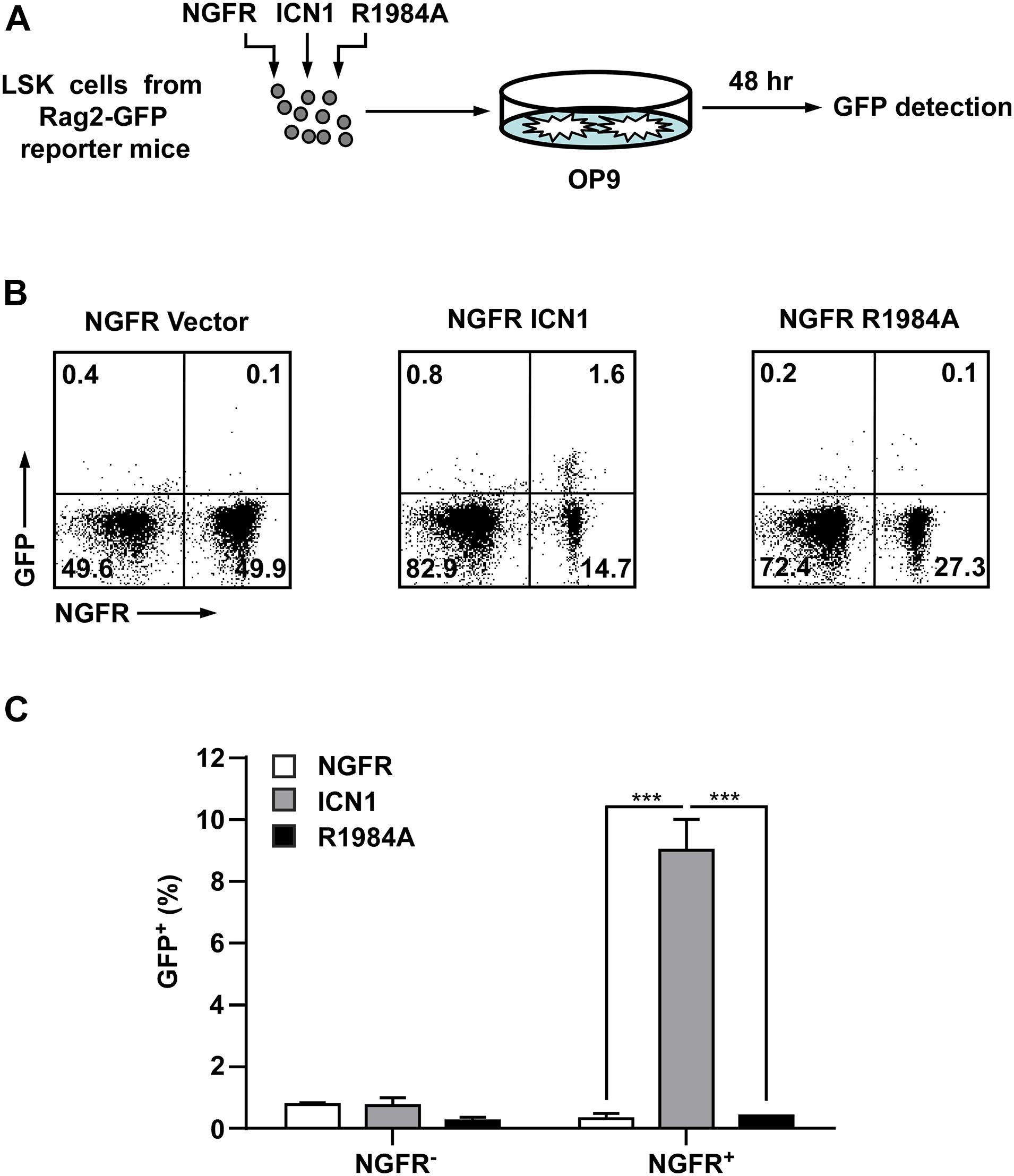
Figure 4. Enforced expression of dimeric Notch1 activates the Rag2 reporter expression in hematopoietic progenitors. (A) Schematic presentation of the experimental procedure. GFP– LSK (Lin–Sca+c-Kit+) cells derived from RAG2-GFP reporter mice (NG-BAC) were transduced with viruses expressing ICN1 or R1984A with NGFR as surrogated marker. The infected cells were co-cultured with stromal cells OP9 for 48 h and then measured the GFP+ percentage as an indication of Rag2 expression. (B) Flow cytometry analysis of GFP and NGFR-PE expressions in hematopoietic cells recovered from OP9 culture. (C) A histogram presentation of GFP+ cell percentages in NGFR-negative or NGFR-positive populations in panel (B). ***p < 0.001.
To further determine the role of Notch1 dimerization in the activation of Rag1 and Rag2 during T-cell fate specification, we purified hematopoietic stem and progenitor cells derived from C57BL/6 mice and transduced them with retroviruses expressing the MigR1 vector, ICN1, or the dimerization-disrupted mutant R1984A, then seeded them on murine OP9 stromal cells for 96 h. GFP+ Thy1+ T-lineage cells were then sorted to analyze the mRNA expression of Rag1/2 and Ptcra (Figure 5A). As shown in Figures 5B–D, mRNA levels of Rag1 and Rag2 were markedly elevated in cells transduced with WT ICN1 as compared with empty vector control, while the expression of R1984A resulted in minimal induction of both genes (Figures 5B,C). As a well-characterized dimeric Notch1 target required for thymocyte development, Ptcra was selectively activated by WT ICN1 but not R1984A (Figure 5D). These data therefore argue the requirement of Notch1 dimerization in activating Rag1/2 expression during T-cell specification.
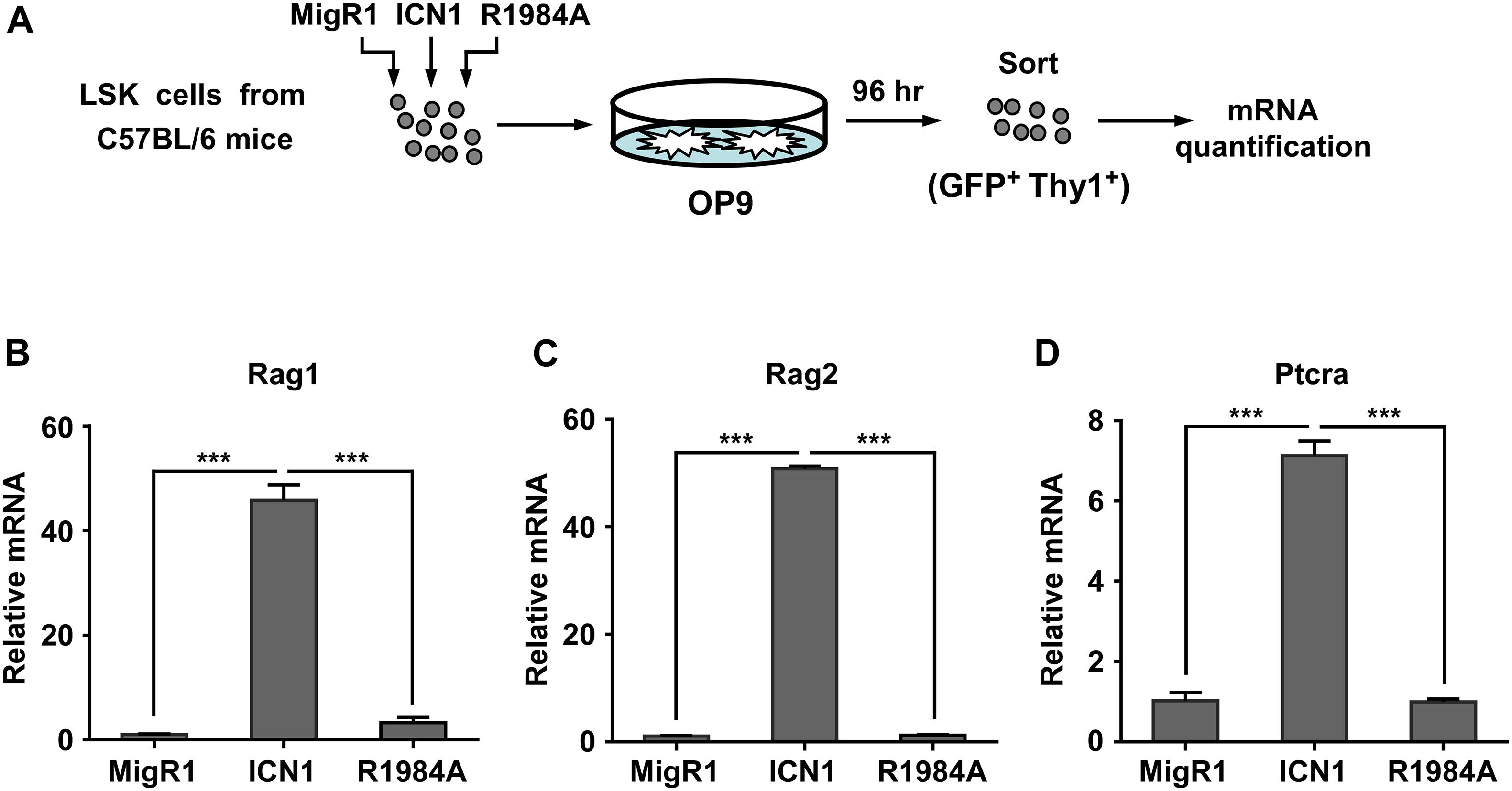
Figure 5. Dimeric Notch1 activates Rag1 and Rag2 during thymocyte development. (A) Schematic presentation of the experimental procedure. LSK cells derived from C57BL/6 mice were transduced with retroviruses expressing the MigR1 vector, ICN1, or R1984A with GFP as a marker. After transduction, the infected cells were co-cultured with murine OP9 stromal cells for 96 h. GFP+ Thy1+ T-lineage cells were sorted for mRNA quantification. (B–D) qRT-PCR analysis showing the mRNA expression of Rag1 (B), Rag2 (C), and Ptcra (D) in sorted cells. ***p < 0.001.
Discussion
The recombination activating genes 1 and 2 are crucial endonucleases to initiate V(D)J recombination in immature lymphocytes and, when overly activated, also implicated in lymphoid malignancies. Yet, transcriptional control of Rag1 and Rag2 is not completely understood. In this report, we demonstrate Notch1 activates Rag1 and Rag2 expression in both murine and human T cells. We identify a novel cis-element in murine T cells, which bears a dimeric Notch1 recognition site SPS and is required for Notch1-mediated transcriptional activation. Dimeric Notch1 enables the optimal expression of Rag1 and Rag2 in both T-ALL and T-cell progenitors. Hence, the present study manifests a prominent role of Notch1 in transcriptional activation of Rag1 and Rag2 in both T-cell development and leukemogenesis.
Notch1 transcriptional complex develops dimer in the nucleus on DNA sequence bearing SPS, such as the promoter of Hes1, a classical Notch1 target (Nam et al., 2007). Yet, the conventional SPS is not commonly detected in the genome. The hypothesis of SPS composition of one canonical site and one cryptic site was later confirmed in the enhancer of Ptcra and Hes5 (Arnett et al., 2010; Liu et al., 2010). Using the matrix developed in the Blacklow Lab to predict the potential dimeric Notch1-responsive element (Arnett et al., 2010), we identified a tentative SPS proximal to the Rag2 enhancer in murine thymocytes. Luciferase reporter assays with the site-specific mutagenesis demonstrate that the cis-element SPS is a bona fide dimeric Notch1-responsive site. Although residing on the Rag2 transcription regulatory region, Rag1 and Rag2 appear dependent on dimeric Notch1 for full activation in T-ALL cells. Since inversely orientated Rag1 and Rag2 are coordinately regulated at the transcriptional level with major cis-elements identified on the Rag2 side (Yu et al., 1999), our newly revealed SPS is most likely the DNA sequence essential for transcriptional activation of both Rag1 and Rag2 in murine T cells. Therefore, we not only give another example that one canonical site and one cryptic site constitute a functional SPS, but also provide a novel pair of cis-element (SPS) and trans-factor (dimeric Notch1) to dictate the transcription of Rag1 and Rag2.
RAG2 has been shown as a functionally important downstream effector of Notch1 in mediating T-ALL development. T-ALL onsets were significantly delayed or impaired when ICN1 expression was enforced in the Rag2–/– bone marrow, which was reversed upon expression of the TCRβ transgene (Allman et al., 2001; Campese et al., 2006). The outcome suggests a critical synergistic effect of Notch1 and pre-TCR signaling to initiate T-ALL. In addition, it remains possible that Notch1-induced aberrant RAG1/2 expression creates unnecessary DSBs, eliciting inappropriate translocations or inducing DNA damages that ordinate with Notch1 to amplify oncogenic signaling and drive further neoplastic progression. For example, RAG endonuclease-elicited Notch1 truncations are revealed in almost all murine T-ALL (Tsuji et al., 2004; Ashworth et al., 2010). Likewise, in human T-ALL, there is a RAG-mediated SIL/SCL rearrangement in a Notch-dependent T-ALL line SIL-ALL, resulting in a remarkable increase of SCL (TAL1) expression (Aplan et al., 1992). We and others have shown that inhibition of Notch signaling results in Rag1 and Rag2 downregulation, manifesting an important correlation of Notch1 and Rag1/2 and also implying a role of Rag1/2 in Notch1-induced T-ALL (Riz et al., 2010; Kourtis et al., 2018). It is reasonable to speculate a new role of Notch1 in affecting genome instability via inducing Rag1/2 expression. Another interesting finding is that depletion of RAG1 or RAG2 elicits T-ALL cell growth inhibition (data not shown), suggesting that these recombination activation genes may have other tumor-promoting roles in cells already transformed.
Our data also suggest that Notch1 activates Rag2 in T-cell progenitors. Previous publications reported that inhibition of Notch1 impairs VDJβ rearrangement and TCRβ expression (Wolfer et al., 2002; Maillard et al., 2006). Although not yet demonstrated, the reduced TCRβ may result from the decreased Rag2 expression in the absence of Notch1. Moreover, overexpression of ICN1 but not R1984A in hematopoietic progenitors led to rapid upregulation of Rag1/2, suggesting the role of dimeric Notch1 in upregulating Rag1/2 in physiological conditions. However, whether Notch1 serves a primary role in activating Rag1/2 expression prior to β-selection awaits further investigation. Since Notch1 expression decreases rapidly after β-selection, upregulation of Rag1/2 for TCRα rearrangement is likely exerted by other transcriptional factors. Interestingly, when mice harboring Notch1 dimerization mutants were examined for the impacts of dimeric deficiency on multiorgan development, mature T-cell subsets appeared normal, despite the total number of thymocytes declined to some extent (Kobia et al., 2020). Functional validations of mature T cells in these Notch1 dimeric deficient mice are yet to be determined. Despite the paradoxical findings, we here provide compelling evidence that the transcriptional activation mediated by canonical Notch1 signaling pathway is one of the prominent mechanisms to orchestrate optimal Rag1/2 expression in T-cell progenitors during development and transformation.
Materials and Methods
Mice
C57BL/6 mice were obtained from Beijing Vital River Laboratory Animal Technology Co., Ltd., Beijing, China. NG-BAC (RAG2-GFP) transgenic reporter mice were as described (Yu et al., 1999). All animal experiments were performed under animal ethical regulations, and the study protocol was approved by the Institutional Animal Care and Use Committee of Wuhan University.
Cell Culture
Murine T-ALL cell line T6E and human T-ALL cell lines KOPTK1, Jurkat, and CUTLL1 were kindly provided by Dr. Warren Pear (University of Pennsylvania, United States). The 293T cell line was purchased from the American Type Culture Collection (ATCC, Manassas, VA, United States). T-ALL cell lines were grown in complete RPMI-1640 (Gibco, Waltham, MA, United States) supplemented with 10% fetal bovine serum (FBS, Gemini Bio, West Sacramento, CA, United States), 1% penicillin/streptomycin (Hyclone, Logan, UT, United States), 1% non-essential amino acids (Gibco), 2 mM L-glutamine (Sigma, St. Louis, MO, United States), 1 mM sodium pyruvate (Sigma), and 100 μM β-mercaptoethanol (Sigma). The 293T cell was maintained in Dulbecco’s modified Eagle’s medium (DMEM, Gibco) containing 10% FBS (Gibco) and 1% penicillin/streptomycin (Hyclone). All cell lines were authenticated using short tandem repeat (STR) analysis, cultured for fewer than 2 months after resuscitation, and tested for mycoplasma contamination once a month using PCR assay.
Reagents and Plasmids
γ-Secretase inhibitor JC-18 was a generous gift from Yue-Ming Li (Searfoss et al., 2003), and compound E was purchased from MedChemExpress (Monmouth Junction, NJ, United States). The retroviral constructs MigR1-ICN1 and MigR1-DNMAML1 (13–74)-GFP were as described (Weng et al., 2003), and MigR1-R1984A, NGFR-ICN1, and NGFR-R1984A were illustrated as previously reported (Liu et al., 2010). In the luciferase reporter assay, the murine DNA fragment containing SPS in Rag2 transcriptional regulatory region was cloned into the pGL3 promoter vector. The forward primer is GCATATGGTACCTCGCATCCTTTTCTACTTCC and the reverse primer is ATATCTCGAGAGGAGGAGCACCACCA GTTT. The high and low affinity binding sites were subjected to mutation using QuickChange site-specific mutagenesis kit (Stratagene, San Diego, CA, United States). Primers to mutate the high affinity site are GATGCTGCTCGCCTTGGTCGACTGCTATCATGAAGCTG (forward) and CAGCTTCATGATAGCAGTCGACCAAGG CGAGCAGCATC (reverse); primers to mutate the low affinity site are GCCTATCCCCGGGCCAGTCGACGATGCTGC TCGCCTTG (forward) and CAAGGCGAGCAGCATC GTCGACTGGCCCGGGGATAGGC (reverse). All constructs were validated by DNA sequence.
qRT-PCR
Total RNA was prepared using TRIzol (Invitrogen, Waltham, MA, United States), followed by concentration and purity determination; 1 μg of random primed total RNAs was reverse-transcribed with SuperScript II (Invitrogen) according to the instructions of the manufacturer. Primer sequences for real-time PCR were as follows: murine Rag1—forward, CAACGAGCACTGAAACTCCA; reverse, CCCGTCACTCTTGAAACGAT; murine Rag2—forward, ATTCCTGCTACCTCCCACCT; reverse, CGGAAAGCTCAT TGTTTGGT; human RAG1—forward, GCAGATGAGT CTGACCACGA; reverse, CTTGAAAGTCCGGAGAATGC; and human RAG2—forward, CAAACCCGCCATGATCTACT; reverse, TTGCTTCCTGCTGACAGATG. Primers used for Ptcra and 18s rRNA were as described (Liu et al., 2010). All the primers for SYBR green detection were used at a final concentration of 0.4 μM. Real-time amplification in FAST SYBR Green Master Mix (Bio-Rad, Hercules, CA, United States) was performed on CFX Connect Real-Time PCR System (Bio-Rad). Relative expression of the mRNA was calculated by the 2–ΔΔCt method and normalized to 18s rRNA.
Retroviral Transduction and OP9 Co-culture
High-titer retroviruses were produced as described (Chiang et al., 2008). For retroviral transduction of hematopoietic stem progenitor cells (HSPCs), LSK (Lin–Sca+c-Kit+) cells were purified from 6- to 8-week-old C57BL/6 or NG-BAC mice. Retroviral supernatants were added into wells coated with 20 mg/ml RetroNectin (Takara, Tokyo, Japan) and incubated for 4–6 h at 37°C before washing with PBS. Purified LSK cells were suspended in the stimulation cocktails (DMEM, 1% penicillin–streptomycin, 15% FBS, 2 mM L-glutamate, 10 ng/ml mIL-3, 10 ng/ml mIL-6, 20 ng/ml mSCF, and 20 ng/ml mFlt3L) (Liu et al., 2010) and then added to virus-bound RetroNectin-coated plates. After transduction, the infected LSK cells were plated onto fresh OP9 monolayers at 106 cells/well in six-well plates. All co-cultures were performed in the presence of 1 ng/ml mIL-7 and 5 ng/ml hFlt3L within the OP9 culture medium (αMEM supplemented with 20% FBS and 1% penicillin–streptomycin) (Wong et al., 2012). For LSK cells derived from NG-BAC mice, transduced cells were analyzed by flow cytometry using PE anti-NGFR (BD Pharmingen, San Diego, CA, United States) and GFP fluorescence signals. For LSK cells derived from C57BL/6 mice, transduced T lineage cells (GFP+ Thy1+) were sorted for qRT-PCR analysis.
Chromatin Immunoprecipitation
The ChIP assay and the subsequent quantitative PCR were performed following the protocol as described (Weng et al., 2006). Briefly, T6E or CUTLL1 cells were treated with DMSO or GSI (1 μM compound E) for 24 h prior to fixation. After fixation for 10 min in 1% paraformaldehyde at room temperature, cells were washed, lysed, and sonicated to shear DNA between 200 and 600 bp in length. Antiserum specific for Notch1 TAD domain (Wu et al., 2000) or normal rabbit IgG was used for immunoprecipitating associated DNA, which was subjected to qPCR using the SYBR system on CFX Connect Real-Time PCR System (Bio-Rad). The primers used are specified as follows: murine hes1 promoter—forward, CGTGTCTCTTCCTCCCAT; reverse, CCAGGACCAAGGAGAGAGGT; murine Rag2 −2.8 kb enhancer—forward, AGCTCCCCACTACCCGAGTCA; reverse, GCTGGTCCACCTTTGTTCTCTAA; Nanog negative control—forward, GGCTGCCTCTCCTCGCCCT; reverse, GTGCACACAGCTGGGCCTGA; and human RAG2 −87 kb enhancer—forward, TAACTGTCAGCTGGCCACAA; reverse, TGGTCTGTCTTGCTCACCTG. Each sample was independently prepared at least two times and run in triplicate. The input DNA was defined as an aliquot of sheared chromatin resulting from crude lysate and was used to normalize the sample to the amount of chromatin added to each ChIP reaction.
Purification of Double Negative Thymocytes
CD4–CD8– DN thymocytes were negatively selected with anti-CD4 and anti-CD8 MACS beads according to the protocol of the manufacturer (Miltenyi Biotec, Auburn, CA, United States). After staining with antibodies against lineage (Lin) markers B220, CD19, CD11b, Gr1, CD11c, NK1.1, Ter119, CD3ε, CD8α, CD8β, TCRβ, and TCRγδ (BD Pharmingen), DN2 thymocytes (c-KithiCD25hiLin–) were purified based on staining with anti-c-Kit and anti-CD25 antibodies (BD Pharmingen) by cell sorting on FACSAria (BD Biosciences) and analyzed on the LSR-II (BD Biosciences). DN3a (c-Kit–/loCD25hiLin–CD27lo) cells were purified by sorting after staining with anti-CD27 antibody (eBioscience, San Diego, CA, United States).
Luciferase Reporter Gene Assays
Empty pcDNA3 or pcDNA3 expressing ICN1 (or indicated mutants) was transiently co-transfected in triplicate into human 293T cells with firefly luciferase pGL3-promoter vector pGL3 or driven by elements containing the Rag2 SPS (or mutants). Cells were transfected using FuGENE 6 Transfection Reagent (Promega, Madison, WI, United States) with 0.8 μg of firefly luciferase reporter constructs, 0.1 μg of expression vectors, and 0.1 μg of Renilla luciferase expression vector. Luciferase activities were measured 24 h later using the Promega Dual Luciferase kit (Promega). Firefly luciferase activities were normalized with Renilla luciferase control values. Relative activity from the empty vector lysate was set arbitrarily to a value of 1.
Flow Cytometry
Cells transduced with NGFR or NGFR-ICN1 and NGFR-R1984A were stained with the PE anti-NGFR (BD Pharmingen) in FACS buffer [1 × DPBS, 10 mM HEPES, 0.02% NaN3, 0.2% BSA (w/v)] on ice in the presence of rat and mouse IgG (Sigma-Aldrich, St. Louis, MO, United States) for 20 min, then washed and resuspended in FACS buffer. Acquisition was performed on LSRII (BD Biosciences). Dead cells and doublets were excluded based on FSC and SSC characteristics. Data were analyzed with FlowJo software (Tree Star, Ashland, OR, United States).
Data Availability Statement
The raw data supporting the conclusions of this article will be made available by the authors, without undue reservation.
Ethics Statement
The animal study was reviewed and approved by Institutional Animal Care and Use Committee of Wuhan University.
Author Contributions
All authors listed have made a substantial, direct and intellectual contribution to the work, and approved it for publication.
Funding
This work was supported by the National Natural Science Foundation of China (81770177 and 81970152 to HL and 81830084 to GQ) and the Fundamental Research Funds for the Central Universities (2042020kf0208 to HL).
Conflict of Interest
The authors declare that the research was conducted in the absence of any commercial or financial relationships that could be construed as a potential conflict of interest.
Acknowledgments
We would like to thank Warren Pear (University of Pennsylvania, United States) and Avinash Bhandoola (National Institute of Health, United States) for conceptual contribution and technique support.
Supplementary Material
The Supplementary Material for this article can be found online at: https://www.frontiersin.org/articles/10.3389/fcell.2021.703338/full#supplementary-material
References
Allman, D., Karnell, F. G., Punt, J. A., Bakkour, S., Xu, L., Myung, P., et al. (2001). Separation of Notch1 promoted lineage commitment and expansion/transformation in developing T cells. J. Exp. Med. 194, 99–106. doi: 10.1084/jem.194.1.99
Aplan, P. D., Lombardi, D. P., Reaman, G. H., Sather, H. N., Hammond, G. D., and Kirsch, I. R. (1992). Involvement of the putative hematopoietic transcription factor SCL in T-cell acute lymphoblastic leukemia. Blood 79, 1327–1333. doi: 10.1182/blood.v79.5.1327.bloodjournal7951327
Arnett, K. L., Hass, M., McArthur, D. G., Ilagan, M. X., Aster, J. C., Kopan, R., et al. (2010). Structural and mechanistic insights into cooperative assembly of dimeric Notch transcription complexes. Nat. Struct. Mol. Biol. 17, 1312–1317. doi: 10.1038/nsmb.1938
Ashworth, T. D., Pear, W. S., Chiang, M. Y., Blacklow, S. C., Mastio, J., Xu, L., et al. (2010). Deletion-based mechanisms of Notch1 activation in T-ALL: key roles for RAG recombinase and a conserved internal translational start site in Notch1. Blood 116, 5455–5464. doi: 10.1182/blood-2010-05-286328
Aster, J. C., Blacklow, S. C., and Pear, W. S. (2011). Notch signalling in T-cell lymphoblastic leukaemia/lymphoma and other haematological malignancies. J. Pathol. 223, 262–273.
Bosticardo, M., Pala, F., and Notarangelo, L. D. (2021). RAG deficiencies: recent advances in disease pathogenesis and novel therapeutic approaches. Eur. J. Immunol. 51, 1028–1038. doi: 10.1002/eji.202048880
Campese, A. F., Garbe, A. I., Zhang, F., Grassi, F., Screpanti, I., and von Boehmer, H. (2006). Notch1-dependent lymphomagenesis is assisted by but does not essentially require pre-TCR signaling. Blood 108, 305–310. doi: 10.1182/blood-2006-01-0143
Chiang, M. Y., Xu, L., Shestova, O., Histen, G., L’heureux, S., Romany, C., et al. (2008). Leukemia-associated NOTCH1 alleles are weak tumor initiators but accelerate K-ras-initiated leukemia. J. Clin. Invest. 118, 3181–3194. doi: 10.1172/jci35090
Gonzales-Garcia, S., García-Peydró, M., Martin-Gayo, E., Ballestar, E., Esteller, M., Bornstein, R., et al. (2009). CSL-MAML-dependent Notch1 signaling controls T lineage-specific IL-7Ralpha gene expression in early human thymopoiesis and leukemia. J. Exp. Med. 206, 779–791. doi: 10.1084/jem.20081922
Helmink, B. A., and Sleckman, B. P. (2012). The response to and repair of RAG-mediated DNA double-strand breaks. Annu. Rev. Immunol. 30, 175–202. doi: 10.1146/annurev-immunol-030409-101320
Hu, Y., Su, H., Liu, C., Wang, Z., Huang, L., Wang, Q., et al. (2017). DEPTOR is a direct NOTCH1 target that promotes cell proliferation and survival in T-cell leukemia. Oncogene 36, 1038–1047. doi: 10.1038/onc.2016.275
Kaneta, M., Osawa, M., Sudo, K., Nakauchi, H., Farr, A. G., and Takahama, Y. (2000). A role for pref-1 and HES-1 in thymocyte development. J. Immunol. 164, 256–264. doi: 10.4049/jimmunol.164.1.256
Kobia, F. M., Preusse, K., Dai, Q., Weaver, N., Hass, M. R., Chaturvedi, P., et al. (2020). Notch dimerization and gene dosage are important for normal heart development, intestinal stem cell maintenance, and splenic marginal zone B-cell homeostasis during mite infestation. PLoS Biol. 18:e3000850. doi: 10.1371/journal.pbio.3000850
Kopan, R., and Ilagan, M. X. (2009). The canonical Notch signaling pathway: unfolding the activation mechanism. Cell 137, 216–233. doi: 10.1016/j.cell.2009.03.045
Kourtis, N., Lazaris, C., Hockemeyer, K., Balandrán, J. C., Jimenez, A. R., Mullenders, J., et al. (2018). Oncogenic hijacking of the stress response machinery in T cell acute lymphoblastic leukemia. Nat. Med. 24, 1157–1166. doi: 10.1038/s41591-018-0105-8
Kuo, T. C., and Schlissel, M. S. (2009). Mechanisms controlling expression of the RAG locus during lymphocyte development. Curr. Opin. Immunol. 21, 173–178. doi: 10.1016/j.coi.2009.03.008
Liu, H., Chi, A. W., Arnett, K. L., Chiang, M. Y., Xu, L., Shestova, O., et al. (2010). Notch dimerization is required for leukemogenesis and T-cell development. Genes Dev. 24, 2395–2407. doi: 10.1101/gad.1975210
Liu, H., Chiang, M. Y., and Pear, W. S. (2011). Critical roles of NOTCH1 in acute T-cell lymphoblastic leukemia. Int. J. Hematol. 94, 118–125. doi: 10.1007/s12185-011-0899-3
Maillard, I., Tu, L., Sambandam, A., Yashiro-Ohtani, Y., Millholland, J., Keeshan, K., et al. (2006). The requirement for Notch signaling at the beta-selection checkpoint in vivo is absolute and independent of the pre-T cell receptor. J. Exp. Med. 203, 2239–2245. doi: 10.1084/jem.20061020
Miyazaki, K., Watanabe, H., Yoshikawa, G., Chen, K., Hidaka, R., Aitani, Y., et al. (2020). The transcription factor E2A activates multiple enhancers that drive Rag expression in developing T and B cells. Sci. Immunol. 5:eabb1455. doi: 10.1126/sciimmunol.abb1455
Mombaerts, P., Iacomini, J., Johnson, R. S., Herrup, K., Tonegawa, S., and Papaioannou, V. E. (1992). RAG-1-deficient mice have no mature B and T lymphocytes. Cell 68, 869–877. doi: 10.1016/0092-8674(92)90030-g
Monroe, R. J., Chen, F., Ferrini, R., Davidson, L., and Alt, F. W. (1999). RAG2 is regulated differentially in B and T cells by elements 5’ of the promoter. Proc. Natl. Acad. Sci. U.S.A. 96, 12713–12718. doi: 10.1073/pnas.96.22.12713
Nam, Y., Sliz, P., Pear, W. S., Aster, J. C., and Blacklow, S. C. (2007). Cooperative assembly of higher-order Notch complexes functions as a switch to induce transcription. Proc. Natl. Acad. Sci. U.S.A. 104, 2103–2108. doi: 10.1073/pnas.0611092104
Paganin, M., and Ferrando, A. (2011). Molecular pathogenesis and targeted therapies for NOTCH1-induced T-cell acute lymphoblastic leukemia. Blood Rev. 25, 83–90. doi: 10.1016/j.blre.2010.09.004
Pear, W. S., Aster, J. C., Scott, M. L., Hasserjian, R. P., Soffer, B., Sklar, J., et al. (1996). Exclusive development of T cell neoplasms in mice transplanted with bone marrow expressing activated Notch alleles. J. Exp. Med. 183, 2283–2291. doi: 10.1084/jem.183.5.2283
Pui, J. C., Allman, D., Xu, L., DeRocco, S., Karnell, F. G., Bakkour, S., et al. (1999). Notch1 expression in early lymphopoiesis influences B versus T lineage determination. Immunity 11, 299–308. doi: 10.1016/s1074-7613(00)80105-3
Radtke, F., Fasnacht, N., and Macdonald, H. R. (2010). Notch signaling in the immune system. Immunity 32, 14–27. doi: 10.1016/j.immuni.2010.01.004
Radtke, F., Wilson, A., Stark, G., Bauer, M., van Meerwijk, J., MacDonald, H. R., et al. (1999). Deficient T cell fate specification in mice with an induced inactivation of Notch1. Immunity 10, 547–558. doi: 10.1016/s1074-7613(00)80054-0
Real, P. J., Tosello, V., Palomero, T., Castillo, M., Hernando, E., de Stanchina, E., et al. (2009). Gamma-secretase inhibitors reverse glucocorticoid resistance in T cell acute lymphoblastic leukemia. Nat. Med. 15, 50–58. doi: 10.1038/nm.1900
Reizis, B., and Leder, P. (2002). Direct induction of T lymphocyte-specific gene expression by the mammalian Notch signaling pathway. Genes Dev. 16, 295–300. doi: 10.1101/gad.960702
Riz, I., Hawley, T. S., Luu, T. V., Lee, N. H., and Hawley, R. G. (2010). TLX1 and NOTCH coregulate transcription in T cell acute lymphoblastic leukemia cells. Mol. Cancer 9:181. doi: 10.1186/1476-4598-9-181
Sambandam, A., Maillard, I., Zediak, V. P., Xu, L., Gerstein, R. M., Aster, J. C., et al. (2005). Notch signaling controls the generation and differentiation of early T lineage progenitors. Nat. Immunol. 6, 663–670. doi: 10.1038/ni1216
Searfoss, G. H., Jordan, W. H., Calligaro, D. O., Galbreath, E. J., Schirtzinger, L. M., Berridge, B. R., et al. (2003). Adipsin, a biomarker of gastrointestinal toxicity mediated by a functional gamma-secretase inhibitor. J. Biol. Chem. 278, 46107–46116. doi: 10.1074/jbc.m307757200
Severson, E., Arnett, K. L., Wang, H. F., Zang, C., Taing, L., Liu, H., et al. (2017). Genome-wide identification and characterization of Notch transcription complex-binding sequence-paired sites in leukemia cells. Sci. Signal. 10:eaag1598. doi: 10.1126/scisignal.aag1598
Shinkai, Y., Rathbun, G., Lam, K. P., Oltz, E. M., Stewart, V., Mendelsohn, M., et al. (1992). RAG-2-deficient mice lack mature lymphocytes owing to inability to initiate V(D)J rearrangement. Cell 68, 855–867. doi: 10.1016/0092-8674(92)90029-c
Spaulding, C., Reschly, E. J., Zagort, D. E., Yashiro-Ohtani, Y., Beverly, L. J., Capobianco, A., et al. (2007). Notch1 co-opts lymphoid enhancer factor 1 for survival of murine T-cell lymphomas. Blood 110, 2650–2658. doi: 10.1182/blood-2007-04-084202
Tan, J. B., Visan, I., Yuan, J. S., and Guidos, C. J. (2005). Requirement for Notch1 signals at sequential early stages of intrathymic T cell development. Nat. Immunol. 6, 671–679. doi: 10.1038/ni1217
Tomita, K., Hattori, M., Nakamura, E., Nakanishi, S., Minato, N., and Kageyama, R. (1999). The bHLH gene Hes1 is essential for expansion of early T cell precursors. Genes Dev. 13, 1203–1210. doi: 10.1101/gad.13.9.1203
Tsuji, H., Ishii-Ohba, H., Katsube, T., Ukai, H., Aizawa, S., Doi, M., et al. (2004). Involvement of illegitimate V(D)J recombination or microhomology-mediated nonhomologous end-joining in the formation of intragenic deletions of the Notch1 gene in mouse thymic lymphomas. Cancer Res. 64, 8882–8890. doi: 10.1158/0008-5472.can-03-1163
Wang, H., Zou, J., Zhao, B., Johannsen, E., Ashworth, T., Wong, H., et al. (2011). Genome-wide analysis reveals conserved and divergent features of Notch1/RBPJ binding in human and murine T-lymphoblastic leukemia cells. Proc. Natl. Acad. Sci. U.S.A. 108, 14908–14913. doi: 10.1073/pnas.1109023108
Weber, B. N., Chi, A. W., Chavez, A., Yashiro-Ohtani, Y., Yang, Q., Shestova, O., et al. (2011). A critical role for TCF-1 in T-lineage specification and differentiation. Nature 476, 63–68. doi: 10.1038/nature10279
Wei, X. C., Kishi, H., Jin, Z. X., Zhao, W. P., Kondo, S., Matsuda, T., et al. (2002). Characterization of chromatin structure and enhancer elements for murine recombination activating gene-2. J. Immunol. 169, 873–881. doi: 10.4049/jimmunol.169.2.873
Weng, A. P., Ferrando, A. A., Lee, W., Morris, J. P. IV, Silverman, L. B., Sanchez-Irizarry, C., et al. (2004). Activating mutations of NOTCH1 in human T cell acute lymphoblastic leukemia. Science 306, 269–271. doi: 10.1126/science.1102160
Weng, A. P., Millholland, J. M., Yashiro-Ohtani, Y., Arcangeli, M. L., Lau, A., Wai, C., et al. (2006). c-Myc is an important direct target of Notch1 in T-cell acute lymphoblastic leukemia/lymphoma. Genes Dev. 20, 2096–2109. doi: 10.1101/gad.1450406
Weng, A. P., Nam, Y., Wolfe, M. S., Pear, W. S., Griffin, J. D., Blacklow, S. C., et al. (2003). Growth suppression of pre-T acute lymphoblastic leukemia cells by inhibition of notch signaling. Mol. Cell Biol. 23, 655–664. doi: 10.1128/mcb.23.2.655-664.2003
Wolfer, A., Wilson, A., Nemir, M., MacDonald, H. R., and Radtke, F. (2002). Inactivation of Notch1 impairs VDJbeta rearrangement and allows pre-TCR-independent survival of early alpha beta Lineage Thymocytes. Immunity 16, 869–879. doi: 10.1016/s1074-7613(02)00330-8
Wong, G. W., Knowles, G. C., Mak, T. W., Ferrando, A. A., and Zuniga-Pflucker, J. C. (2012). HES1 opposes a PTEN-dependent check on survival, differentiation, and proliferation of TCRbeta-selected mouse thymocytes. Blood 120, 1439–1448. doi: 10.1182/blood-2011-12-395319
Wu, L., Aster, J. C., Blacklow, S. C., Lake, R., Artavanis-Tsakonas, S., and Griffin, J. D. (2000). MAML1, a human homologue of Drosophila mastermind, is a transcriptional co-activator for NOTCH receptors. Nat. Genet. 26, 484–489. doi: 10.1038/82644
Yu, W., Misulovin, Z., Suh, H., Hardy, R. R., Jankovic, M., Yannoutsos, N., et al. (1999). Coordinate regulation of RAG1 and RAG2 by cell type-specific DNA elements 5’ of RAG2. Science 285, 1080–1084. doi: 10.1126/science.285.5430.1080
Zhang, F., Thomas, L. R., Oltz, E. M., and Aune, T. M. (2006). Control of thymocyte development and recombination-activating gene expression by the zinc finger protein Zfp608. Nat. Immunol. 7, 1309–1316. doi: 10.1038/ni1397
Keywords: Notch1 dimerization, Recombination activating genes, T-cell acute lymphoblastic leukemia, Double negative thymocyte, T-cell development
Citation: Dong Y, Guo H, Wang D, Tu R, Qing G and Liu H (2021) Genome-Wide Analysis Identifies Rag1 and Rag2 as Novel Notch1 Transcriptional Targets in Thymocytes. Front. Cell Dev. Biol. 9:703338. doi: 10.3389/fcell.2021.703338
Received: 30 April 2021; Accepted: 15 June 2021;
Published: 12 July 2021.
Edited by:
Binghui Li, Capital Medical University, ChinaReviewed by:
Bo Li, Sun Yat-sen University, ChinaPeng Li, Guangzhou Institutes of Biomedicine and Health, Chinese Academy of Sciences (CAS), China
Copyright © 2021 Dong, Guo, Wang, Tu, Qing and Liu. This is an open-access article distributed under the terms of the Creative Commons Attribution License (CC BY). The use, distribution or reproduction in other forums is permitted, provided the original author(s) and the copyright owner(s) are credited and that the original publication in this journal is cited, in accordance with accepted academic practice. No use, distribution or reproduction is permitted which does not comply with these terms.
*Correspondence: Guoliang Qing, cWluZ2d1b2xpYW5nQHdodS5lZHUuY24=; Hudan Liu, aHVkYW5saXVAd2h1LmVkdS5jbg==
†These authors have contributed equally to this work