- 1Bioimaging Research Hub, Cardiff School of Biosciences, Cardiff University, Wales, United Kingdom
- 2Graduate School of Biomedical Engineering, University of New South Wales, Sydney, NSW, Australia
- 3Raymond Purves Bone and Joint Research Laboratories, Kolling Institute of Medical Research, Royal North Shore Hospital and The Faculty of Medicine and Health, The University of Sydney, St. Leonard’s, NSW, Australia
Chondroitin sulfate (CS) is the most abundant and widely distributed glycosaminoglycan (GAG) in the human body. As a component of proteoglycans (PGs) it has numerous roles in matrix stabilization and cellular regulation. This chapter highlights the roles of CS and CS-PGs in the central and peripheral nervous systems (CNS/PNS). CS has specific cell regulatory roles that control tissue function and homeostasis. The CNS/PNS contains a diverse range of CS-PGs which direct the development of embryonic neural axonal networks, and the responses of neural cell populations in mature tissues to traumatic injury. Following brain trauma and spinal cord injury, a stabilizing CS-PG-rich scar tissue is laid down at the defect site to protect neural tissues, which are amongst the softest tissues of the human body. Unfortunately, the CS concentrated in gliotic scars also inhibits neural outgrowth and functional recovery. CS has well known inhibitory properties over neural behavior, and animal models of CNS/PNS injury have demonstrated that selective degradation of CS using chondroitinase improves neuronal functional recovery. CS-PGs are present diffusely in the CNS but also form denser regions of extracellular matrix termed perineuronal nets which surround neurons. Hyaluronan is immobilized in hyalectan CS-PG aggregates in these perineural structures, which provide neural protection, synapse, and neural plasticity, and have roles in memory and cognitive learning. Despite the generally inhibitory cues delivered by CS-A and CS-C, some CS-PGs containing highly charged CS disaccharides (CS-D, CS-E) or dermatan sulfate (DS) disaccharides that promote neural outgrowth and functional recovery. CS/DS thus has varied cell regulatory properties and structural ECM supportive roles in the CNS/PNS depending on the glycoform present and its location in tissue niches and specific cellular contexts. Studies on the fruit fly, Drosophila melanogaster and the nematode Caenorhabditis elegans have provided insightful information on neural interconnectivity and the role of the ECM and its PGs in neural development and in tissue morphogenesis in a whole organism environment.
Introduction
This chapter highlights the roles of chondroitin sulfate (CS) and dermatan sulfate (DS)-proteoglycans (PGs) in neural biology, heparan sulfate (HS)-PGs were outside the scope of this review and thus are only briefly touched on. However many excellent reviews exist on HS-PGs and their interactions with extracellular matrix (ECM) components in neural development, neural function and potential in neural repair biology (Condomitti and de Wit, 2018; Zhang P. et al., 2018; Roppongi et al., 2020; Xiong et al., 2020; Kamimura and Maeda, 2021; Sakamoto et al., 2021). Roles for HS-PGs in model developmental organisms such as Drosophila melanogaster and Caenorhabditis elegans have also been reviewed (Díaz-Balzac et al., 2014; Blanchette et al., 2017; Kamimura and Maeda, 2017; Saied-Santiago et al., 2017) and the interested reader is referred to these excellent publications for further information.
With the identification of the multiple molecular determinants that provide neuronal connectivity, and with new insights into the modulatory extracellular information regulating axon guidance, neural network and synapse formation, a better understanding of the complexity that neurons face in a living organism is beginning to emerge. Attention is now returning to an ancient regulator of cell-cell interaction: the ECM (Dityatev and Schachner, 2006; Dityatev et al., 2010; Miyata and Kitagawa, 2017; Nicholson and Hrabětová, 2017; Ferrer-Ferrer and Dityatev, 2018; Quraishe et al., 2018; Cope and Gould, 2019; Long and Huttner, 2019; Chelyshev et al., 2020; Jain et al., 2020; Wilson et al., 2020; Carulli and Verhaagen, 2021; Kamimura and Maeda, 2021; Shabani et al., 2021; Su et al., 2021). Among the many matrix components that influence neuronal connectivity, recent studies on the CS-PGs and HS-PGs indicate these ancient molecules form dynamic scaffolds that not only provide a protective environment around cells but are also a source of directive cues that modulate neuronal behavior and synaptic plasticity in tissue development (Haylock-Jacobs et al., 2011; Hayes and Melrose, 2018; Hayes et al., 2018; Karamanos et al., 2018; Hayes and Melrose, 2020a; Shabani et al., 2021).
Roles of GAGs in the Evolution of Life and Electrochemical Properties of Tissues
Glycosaminoglycans (GAGs) and PGs are ancient molecules that evolved over a 500 million year period of invertebrate and vertebrate evolution (Yamada et al., 2011). Natural selection processes ‘chose’ GAGs with molecular recognition, information storage and transfer properties. The PGs that populated the glycocalyx surrounding cells thus had cell instructive properties through their GAG side chains that interacted with morphogens, growth factors, cytokines, cell receptors, cell adhesion molecules and neurotrophic peptides facilitating regulatory roles in embryonic neural development. GAGs also have electro-chemical properties through their sulfate and carboxyl groups that are ionized at physiological pH. GAG-electroconductive gels in the sensory pores of the skin of elasmobranch fish species (i.e., sharks, rays, and skates) have the capacity to detect protons produced by the muscular activity of prey fish species and this equips them with the ability to undergo electro-location to detect prey species, even under highly turbid water conditions where these are not visible (Bellono et al., 2017). Such gels have ultrasensitive proton detection capability, this information is transferred to a sensory nerve interface in the skin pores and then to the brain stem for signal interpretation.
All GAGs have proton detection capability (Josberger et al., 2016; Selberg et al., 2019) and are ancient molecules that were present during the early stages of the evolution of life (Yamada et al., 2011). It has been proposed that proton electrochemical ion gradients across membranes drove cellular metabolism and energy production during early evolution (Lane, 2017). In prokaryotic evolution, GAGs were mainly unsulfated or poorly sulfated; however, when eukaryotic cells evolved, sulfated GAGs predominated. Evolution of membrane polarization became possible in eukaryotic cells and membrane energetics emerged (Wilson and Lin, 1980; Niven and Laughlin, 2008; Dibrova et al., 2012; Lynch and Marinov, 2017). Membrane polarization involves the controlled movement of ions across cell membranes, GAGs had fundamental roles to play in these processes through their proton binding properties. All cells in multicellular organisms utilize membrane polarization when undergoing cell signaling, adhesion, proliferation, migration, and cytokinesis. Some cells such as neurons have developed electrical processes to a high level of precision, and this is the basis of the generation of electrical impulses in neural networks that remotely control cells and tissues in higher animals. Further eukaryotic evolution resulted in the development of a glycocalyx around cells. This contained PGs containing GAG side chains with the ability to instruct cellular behavior. The development of pericellular and extracellular matrices populated by PGs facilitated the development of tissues with variable biophysical properties due to these PGs and their co-operative interactions with structural proteins thus driving specialization with the ECM. Neural networks subsequently evolved to control these tissues of increasing complexity. Neurons are highly energetic cell types that utilize Na(+)/K(+)-ATPase pumps to generate energy. This process also generates chemical and electrical gradients across cell membranes. This membrane polarization process is essential for cell signaling and is aberrantly controlled in neurological diseases. Examination of the ECM PGs that control these neural processes has uncovered valuable therapeutic targets (Soleman et al., 2013; Maćkowiak et al., 2014; De Luca and Papa, 2016; Miyata and Kitagawa, 2016; Yang, 2020; Dityatev et al., 2021).
Glycosaminoglycan were fundamental entities in the formation and regulation of neural networks and tissues and the control of cell behavior during morphogenesis, tissue development and in ECM remodeling in tissue repair (Melrose, 2019b). The sulfation patterns of GAGs have roles in cellular molecular recognition and the regulation of physiological processes (Melrose, 2019b). GAG sulfotransferases and glycosyl transferases in progenitor/stem cell niches support the assembly of GAGs of diverse structure and sulfation patterns and are important in the development of pluripotent stem cell lineages with migratory properties (Stanley, 2016). This allows these cells to participate in tissue development and tissue repair. Sulfate groups are important functional determinants on GAGs. Knock-out sulfotransferase and glycosyl transferase mice have demonstrated the important functional roles of GAGs in tissues (Stanley, 2016). Variable sulfation positions and densities on GAGs convey a range of functional attributes to tissues including an ability to act as electrical conduits to the cell. Sulfate groups are relatively bulky space-filling entities on GAGs, it was pertinent that all spatial orientations and permutations were explored during natural evolutionary selection processes to select GAGs with optimal interactivity. Natural selection forces thus explored many permutations of GAG structural form to optimize cell regulatory capability. Sulfate groups convey interactive molecular recognition and information transfer capability to GAGs and their interactions with growth factors, receptors, morphogens, ECM components, proteases, and protease inhibitory proteins regulate cell signaling processes in tissue morphogenesis and skeletogenesis. Knockout of glycosyl transferases, that are required for GAG assembly, has produced GAG-deficient mice (Stanley, 2016) that have allowed examination of the roles GAGs play in tissue form and function and how these regulate physiological processes in health and disease. The inherent charge transfer and storage properties of GAGs is a “glyco-code” that provides sophisticated cell instructive information (Gabius, 2018; Hayes and Melrose, 2018; Hayes et al., 2018; Kaltner et al., 2019).
CNS/PNS ECM PGs/GAGs, Cellular Regulation, and Neural Tissue Development
As already discussed, GAGs have electrochemical properties equipping them with cell regulatory abilities (Lane, 2017; Selberg et al., 2019). At the individual cell level, voltage gradients occur across cell membranes as so-called, action potentials (Strbak et al., 2016) which form part of the cell signaling and communication machinery of cells, i.e., membrane polarization-depolarization underlying the generation of electrical signaling in neural networks. Proton conductivity is important in many natural cellular processes including oxidative phosphorylation in mitochondria and energy production, uncoupling of membrane potentials during membrane polarization-de-polarization and neural potentiation, as well as in the priming of cells for proliferative events, apoptosis or cell migration (Wilson and Lin, 1980; Vellai et al., 1998; Niven and Laughlin, 2008; Dibrova et al., 2012; Lynch and Marinov, 2017). Electrochemical reactions control cell and tissue polarity and regulate cell behavior, ECM PGs facilitate electrocommunication between cells and their extracellular microenvironments. Cells sense changes in their microenvironments through micromechanical and electrochemical cues from the ECM allowing the cell to maintain a homeostatic tissue compositional balance thus providing optimal tissue functional properties (Guilak et al., 2021; Melrose et al., 2021). GAGs can detect proton gradients and are electroconductive entities that participate in microelectronic events during membrane polarization forming the basis of cell signaling (Wilson and Lin, 1980; Vellai et al., 1998; Niven and Laughlin, 2008; Dibrova et al., 2012; Lynch and Marinov, 2017). Neurons are particularly sensitive to electrostimulation in microelectronic events leading to polarization of the activated neuron cell membrane, however, membrane polarization occurs in all cells to some extent and is the basis of cell signaling during cellular attachment, migration and transmission of signals from cell to cell not only during development but also in neural repair and functional nerve recovery from trauma (Hortobágyi and Maffiuletti, 2011; Hayes and Melrose, 2020b). The GAG components of PGs participate in neurotrophic regulation of cellular movement in the development of neural networks and also in neural repair processes. A diverse collection of neuroregulatory molecules participate in these processes guided by cues from ECM PGs, which are discussed later in this chapter.
The Chondroitin Sulfate and Dermatan Sulfate Components of Neural Proteoglycans
Chondroitin sulfate is the most abundant GAG of the human body and CS side chains are found on a diverse range of PGs. CS is a linear GAG consisting of D-glucuronic acid glycosidically linked to N-acetyl galactosamine to form repeat disaccharides assembled into CS side chains (Zhang, 2010) on PGs up to ∼20 kDa in size (Figure 1). D-glucuronic acid also undergoes epimerization and inversion in structure to form L-iduronic acid in the related GAG, DS also known as CS-B (Figure 2).
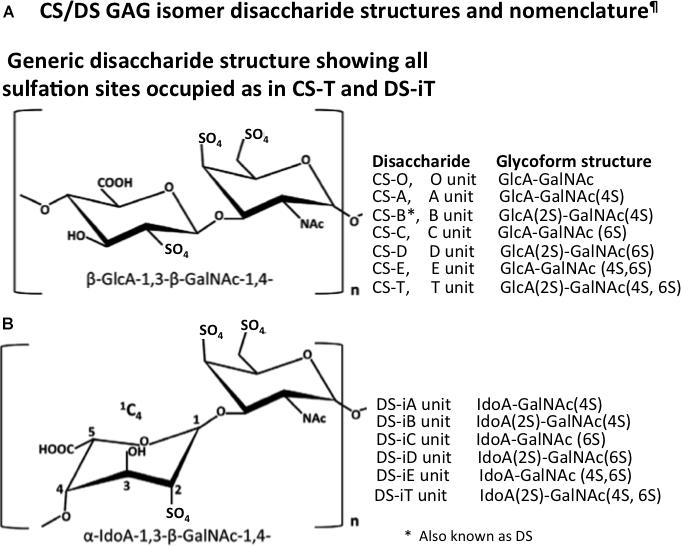
Figure 1. Structure of the CS/DS disaccharides (A) and nomenclature of the CS and DS disaccharide glycoforms (B) as proposed by Malavaki et al. (2008).
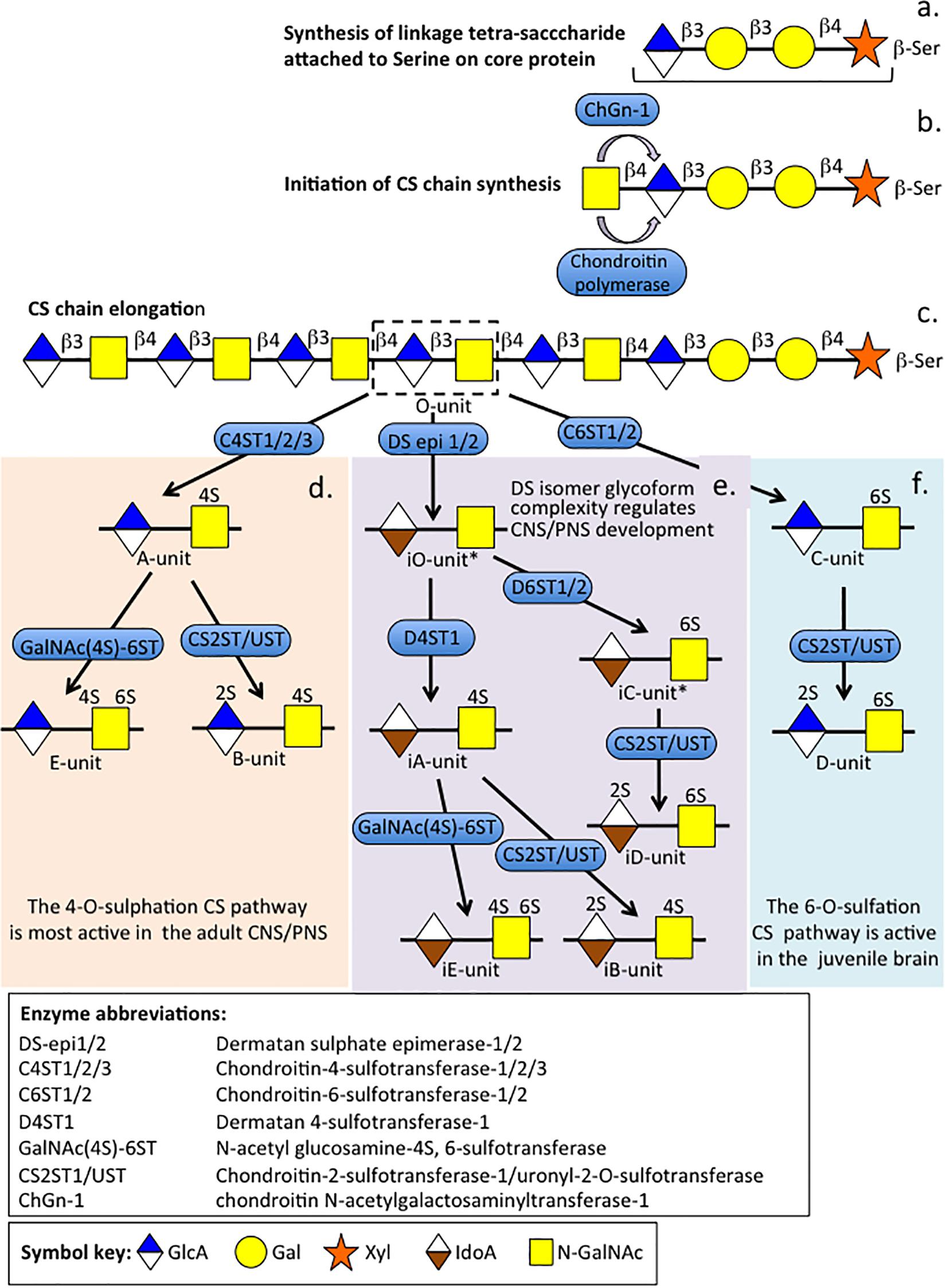
Figure 2. The biosynthesis of CS and DS chains showing their diverse disaccharide glycoforms that are functional in the juvenile and adult CNS/PNS and during the development and repair of the CNS/PNS. Biosynthesis of the tetrasaccharide linker sequence by addition of a xylose residue to a serine residue in the proteoglycan core protein followed by addition of two Galactose and a GlcA residue (a). Initiation of CS chain elongation occurs by addition of a GalNAc residue by chondroitin N-acetylgalactosaminyltransferase-1 or chondroitinpolymerase (b). Elongation of the CS chain occurs by sequential additions of GlcA and GalNAc to the nascent non-reducing terminus by chondroitin polymerases (c). The CS chain is sulfated by chondroitin-4 and 6-sulphotransferases, or the GlcA residue of the O-disaccharide unit is epimerized to IdoA with inversion in structure from a β-D conformation to an α-L conformation followed by a series of sulphotransferases and uronyl-2-sulphotransferase to form the CS-A, CS-B, CS-C, CS-D, CS-E and DS-iA, DS iB, CS-iC, DS-iD, and DS-iE isoforms as shown (d–f). The 4-O-sulfation pathway is most active in the adult brain (d) while various DS isoforms regulate brain development and repair processes (e). The 6-sulfation pathway (f) is most active in the juvenile brain. The DS-iO and DS-iC units have yet to be confirmed. Figure modified from Malmström et al. (2012) and Miyata and Kitagawa (2017).
Chondroitin sulfate is O-sulfated at C-4 or C-6 of the GalNAc, whereas in DS GalNAc is almost exclusively 4-O-sulfated and minor proportions of L-idoA may be O-sulfated at C-2 (Malmström et al., 2012). The conversion of GlcA into IdoA is variable ranging from one to almost 99% conversion of GlcA to IdoA (Malmström et al., 2012). IdoA residues are not regularly distributed along the CS/DS chain and occur in blocks of ≥6 IdoA residues, alternating IdoA/GlcA units, or as isolated IdoA units interspersed within stretches of unmodified GlcA (Tykesson et al., 2018). DS epimerase-1 and dermatan 4-O-sulfotransferase-1 form complexes that generate long epimerized 4-O-sulfated blocks. The presence of idoA in CS/DS alters its properties since a more flexible chain is generated that is more able to explore spatial orientations that maximize binding opportunities with prospective ligands (Ferro et al., 1990). IdoA substituted CS/DS influences cellular properties, such as migration, proliferation, differentiation, angiogenesis and regulates cytokine/growth factor activities (Thelin et al., 2013). CS and DS occur in significant amounts in the brain and have important roles to play in CNS development. DS sulfate epimerase 2 (DS-epi2) is ubiquitously expressed in the infant brain whereas DS epimerase 1 (DS-epi1) expression is faint at all developmental stages (Akatsu et al., 2011). DS-epi2 but not DS-epi1 plays dominant roles in the epimerization of CS/DS and has crucial roles to play in postnatal brain development. CS/DS hybrid chains have roles in the development of the cerebellum with the expression of crucial disulfated CS/DS disaccharides spatiotemporally regulated by specific sulfotransferase enzymes (Mitsunaga et al., 2006). Ubiquitous expression of chondroitin 4-O-sulfotransferase-1 (C4ST-1) and C4ST-2 in the postnatal mouse brain contrasts with dermatan 4-O-sulfotransferase-1 (D4ST-1) and uronyl 2-sulfotransferase (UST) expression which are restricted to the developing cerebellum. The proportions of DS-specific, 4-sulfated IdoA-GalNAc (iA) and 2-sulfated IdoA-GalNAc (iB) produced by sequential D4ST-1 and UST activity has been shown to be highest in CS/DS chains isolated from the developing cerebellum with a 10-fold increase in iB evident. GlcA/IdoA(2-O-sulfate)-GalNAc(6-O-sulfate) (D/iD) and GlcA/IdoA-GalNAc (4,6-O-disulfate) (E/iE) levels, however, decrease to 50 and 30%, respectively, in the developing cerebellum. Thus IdoA-containing iA and iB and D/iD and E/iE units in CS/DS hybrid chains both have important roles to play in the development of the cerebellum and postnatal brain development. The diverse structures that are possible with CS provide multifunctional properties to CS-PGs (Abbott and Nigussie, 2020), with dynamic changes in CS structure providing adaptable regulatory properties to PGs in tissue development and in pathological conditions (Galtrey and Fawcett, 2007). CS-PGs as components of perineuronal nets (PNNs) have neuroprotective properties and regulate neural plasticity and cognitive learning through specific CS mediated interactions (Dyck and Karimi-Abdolrezaee, 2015). PGs are ubiquitous secreted ECM components (Figure 3) that also occur attached to cell surfaces either as transmembrane or glycophosphatidylinositol (GPI)-anchored structures, and intracellularly as granular deposits in some cells (Figures 4, 5). Perlecan is referred to as a HS-PG, however, in many tissues it is a hybrid CS/HS PG and is thus included in this review, particularly in view of its many interesting properties in neural tissues. The chain length of CS, 3D spatial presentation and density of its sulfate groups control its physicochemical and cell interactive and biological properties in tissues through interactions with a diverse range of ligands that regulate many physiological processes (Galtrey and Fawcett, 2007; Dyck and Karimi-Abdolrezaee, 2015). GAGs represent major ECM components of the brain, constituting up to 60% of its mass during early embryonic development and 20% in the adult central nervous system/peripheral nervous system (CNS/PNS). CS substituted PGs are one of the most abundant components of the CNS/PNS. HA is also a major component. HA has a simple structure and is the only non-sulfated GAG, but nevertheless has important biophysical properties which are important in the hydration and compartmentalization of the CNS/PNS. High molecular weight HA is anti-inflammatory, minimizes neuroinflammation and exhibits cell interactive properties that regulate cellular migration, proliferation and differentiation (Sherman et al., 2015). HA is also a component of the sub-ventricular and sub-granular dentate gyrus of the hippocampus which are two regions of the brain containing neuroprogenitor stem cell populations in niches known as fractones (Mercier et al., 2012; Mercier, 2016; Sato et al., 2019).
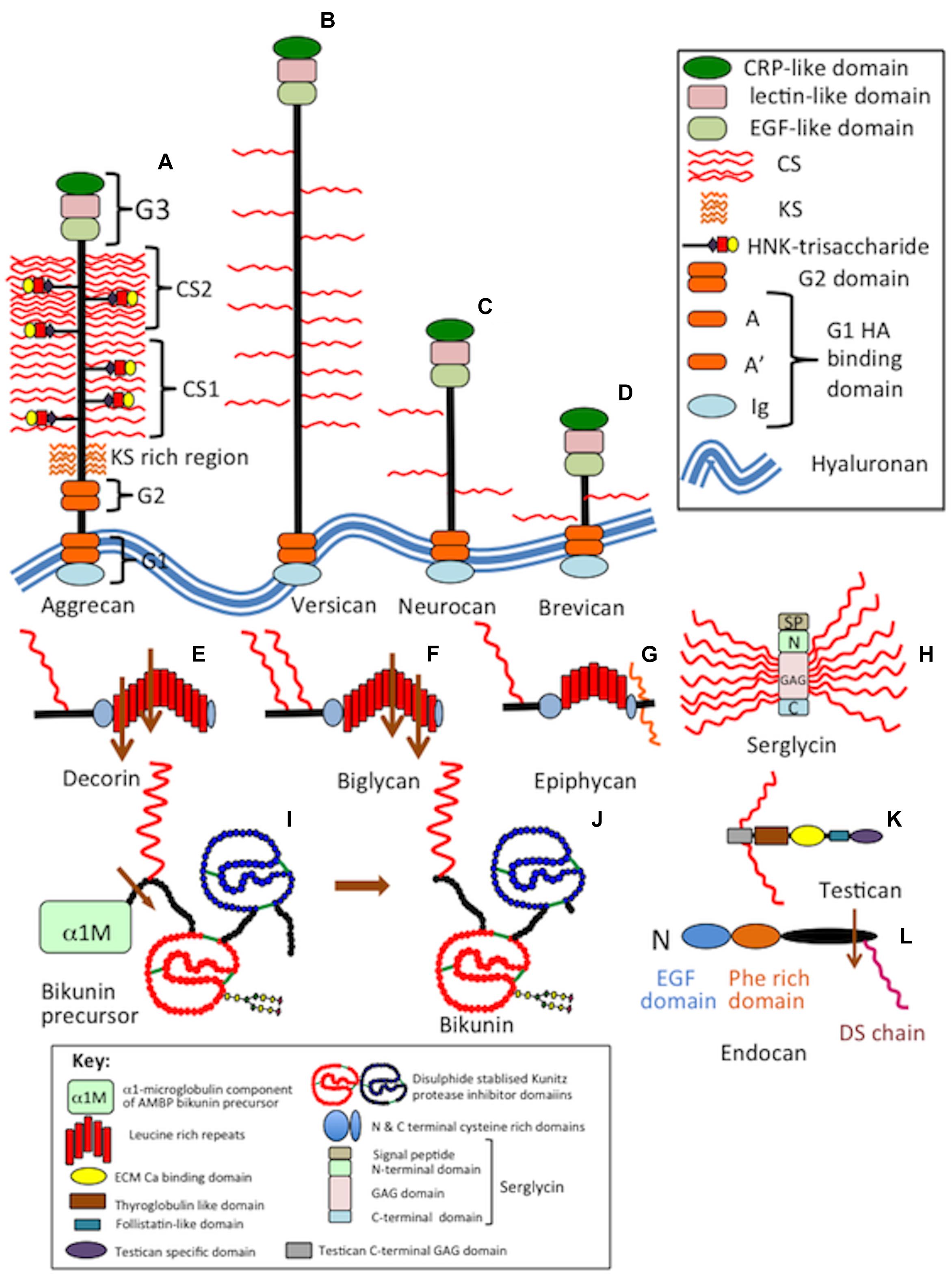
Figure 3. Composite schematic depicting the structural organization of secreted CS-proteoglycans in the CNS/PNS. The lectican proteoglycans aggrecan, versican, neurocan, brevican (A–D), selected members of the small leucine repeat proteoglycans, decorin, biglycan and epiphycan (E–G), serglycin (H), bikunin precursor protein and bikunin (I,J), testican (K) and endocan (L).
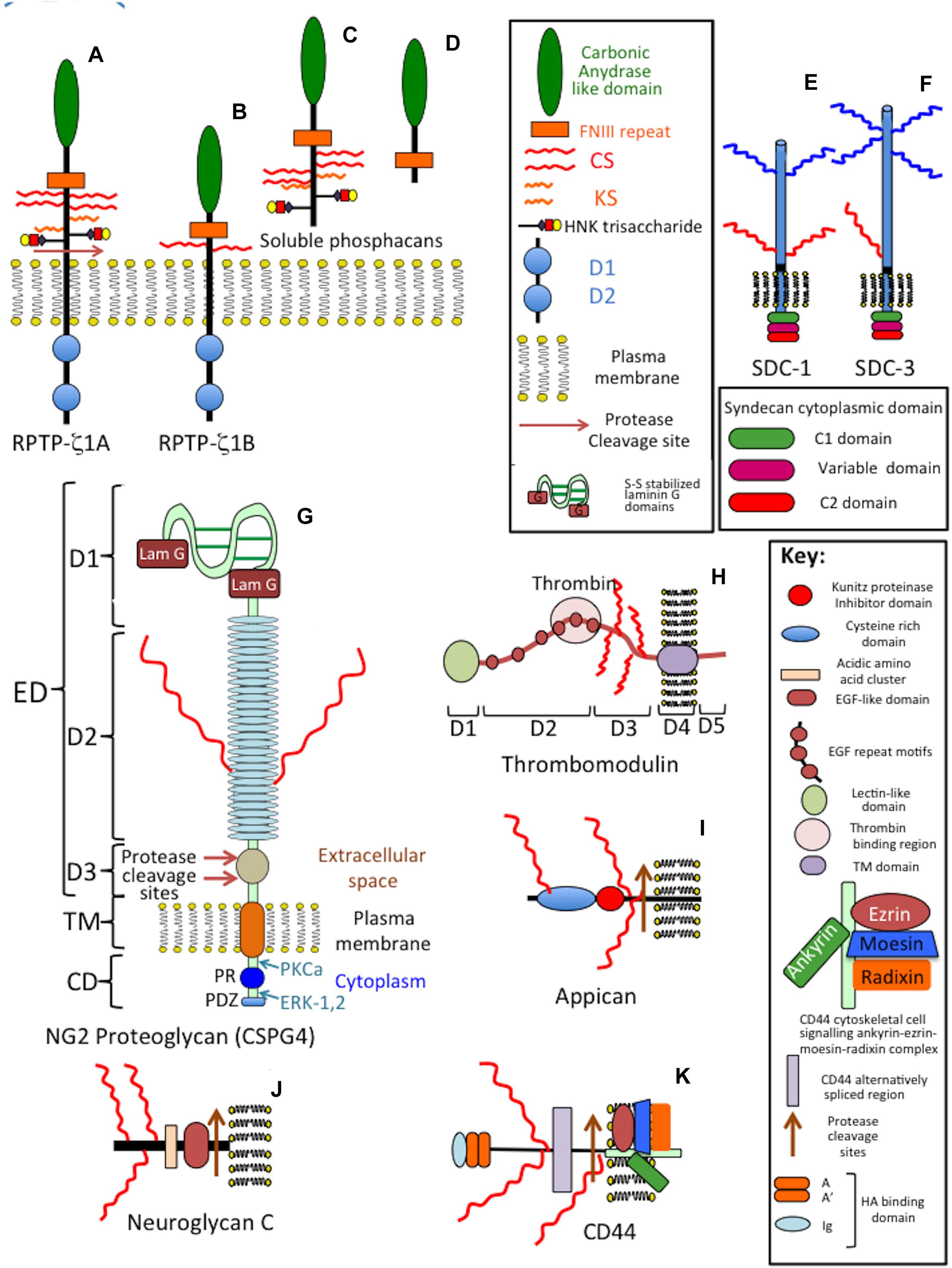
Figure 4. Composite schematic depicting the structural organization of cell membrane attached CS-proteoglycans in the CNS/PNS. Phosphacan precursor, receptor protein tyrosine phosphatase (RPTP)-1A and 1B forms (A,B) and soluble phosphacan released by proteolysis of (A) as shown (C). A non-glycanated truncated phosphacan variant has also been described (D). Syndecan-1 (SDC-1) (E) and syndecan-3 (SDC-3) (F). The transmembrane form of NG2 proteoglycan (CSPG4) (G) and its soluble form released from cells by protease cleavage close to the cell membrane (H). The soluble form of CSPG4 becomes lodged in the ECM through interactions mediated by its LamG N-terminal motifs and by interactions of its central cysteine-rich domain with type IV and VI collagen. Thrombomodulin showing its terminal lectin-like domain, six EGF repeat modules, thrombin binding region which allows it to act as an anti-coagulant in the brain microvasculature and transmembrane cell attachment domain (I). Appican (J), neuroglycan-C (K), and the HA-receptor CD-44 (L) with protease cleavage sites that results in the generation of soluble forms of these proteoglycans. The CS-E chains of appican bind strongly to the growth factors midkine and pleiotrophin, neuroglycan-C binds to ephrin cell surface receptors resulting in induction of cell signaling mediated by the ephrin cytoplasmic regions while sCD44 binds to HA in the ECM through its disulphide stabilized A, A′ and Ig folds. Several CAMs also act as CS-PG receptors through interaction with the CS side chains of these PGs (see Figure 7).
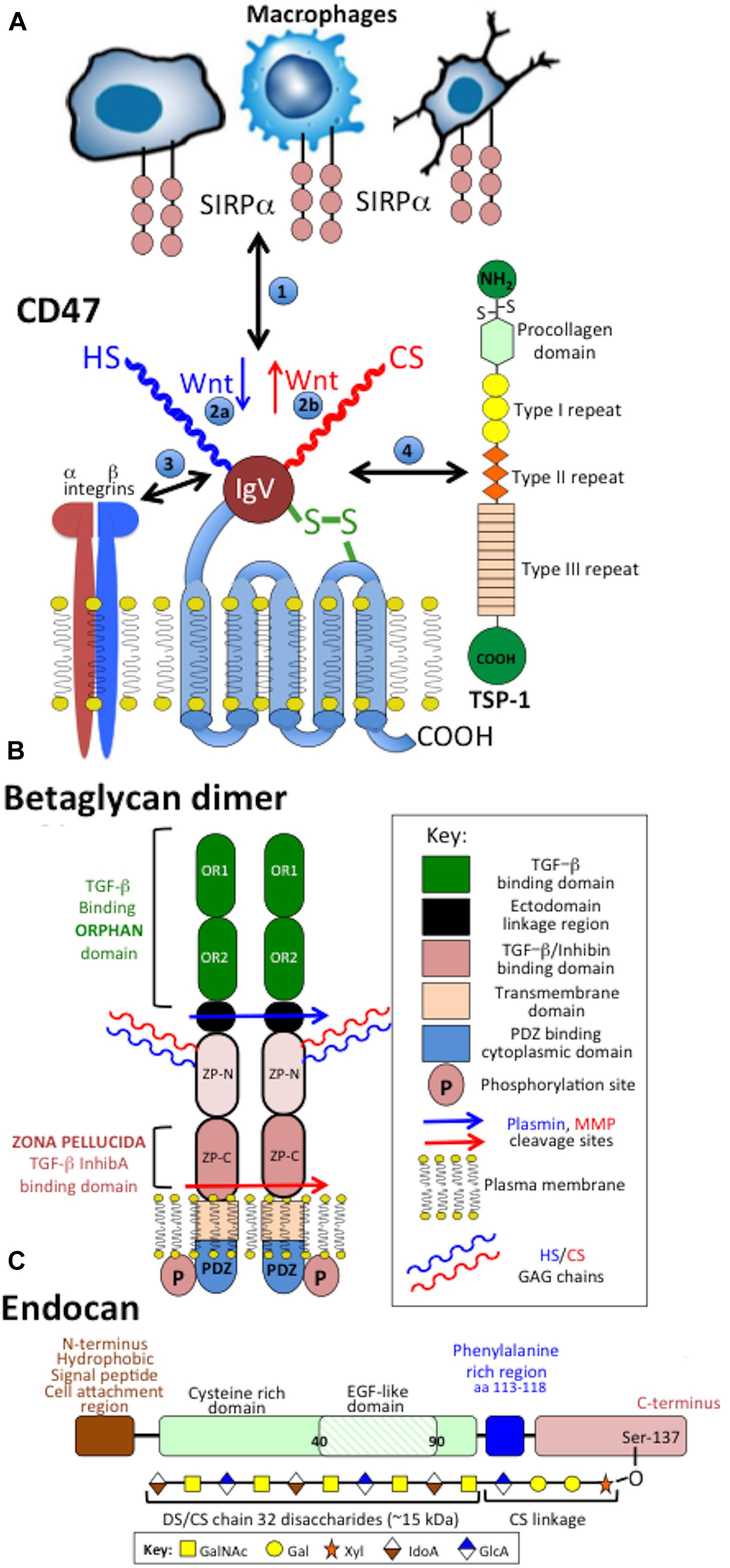
Figure 5. Schematic depictions of the structural organization of the transmembrane CS-PG receptors CD47 showing its interactions with macrophages, integrins and thrombospondin-1 (TSP-1) (A), betaglycan dimer (B) and endocan (C). Betaglycan forms an active dimer on the cell surface. Protease cleavage sites are indicated which release fragments of the betaglycan ecto-domain. Endocan has an EGF-like and a phenylalanine rich domain and a single CS/DS GAG chain attached to its C-terminus.
Developmental Animal Models Used to Examine Neural Development and Regulation in a Whole Organism Environment
Studies on the fruit fly, D. melanogaster (FitzGerald et al., 2006; Nishihara, 2010; Losada-Perez et al., 2016; Davis et al., 2019) and the nematode C. elegans (White et al., 1976; Mulcahy et al., 2018; Schafer, 2018; Kovács et al., 2020; White, 2020) have provided insightful information on the role of the ECM and some of its specific components in neural development and tissue morphogenesis in a whole organism environment. White et al. (1986) undertook the first complete mapping study of the nematode’s nervous system using manual reconstruction of serial electron micrographs, to characterize the morphology of each of the 302 neurons in the adult nematode and their interconnected chemical and electrical synapses. This study is an invaluable guide on the C. elegans neural network and has significantly influenced studies on behavioral neurobiology and network science. The nervous system of C. elegans has a total of 302 invariantly organized neurons, that have been grouped into 118 categories. Neurons in C. elegans have simple morphologies displaying few, if any, branches and are generally highly connected through local synaptic connections with neighboring neurons, ∼ 5000 chemical synapses, 2000 neuromuscular junctions and 600 gap junctions have been identified in C. elegans. The specific patterns and functional properties of electrical synapses of the C. elegans nervous system have been systematically examined through a genome- and nervous-system-wide analysis of the expression patterns of the invertebrate electrical synapse constituents, the innexins (Hu, 2007; Güiza et al., 2018; Bhattacharya et al., 2019). Innexins are transmembrane proteins that form gap junctions in invertebrates. Highly complex expression patterns are evident throughout the nervous system, and when animals enter an insulin-dependent arrest stage due to exposure to harsh environmental conditions, termed the dauer stage, C. elegans larvae undergo Dauer arrest at the second molt (Cassada and Russell, 1975). Many insights into the signaling pathways and the molecular mechanisms that govern this developmental transition have been uncovered in the last decade. Dauer pheromone the major physiologic signal that promotes Dauer arrest, has been purified, identified, and synthesized and the vast majority of Dauer regulatory genes are now known (Jeong et al., 2005). Dauer pheromone (daumone, ascr#1) is the first C. elegans pheromone identified. Electrical synapse remodeling is responsible for the altered locomotory and chemosensory behavior of Dauer affected neurons (Starich et al., 2009). These neurons are regulated by a mechanism that involves FoxO transcription factors that mediate dynamic innexin expression plasticity in a neuron-type- and environment-specific manner (Hobert, 2010; Amran et al., 2021). C. elegans is a useful model for the examination of developmental processes regulated by ECM components (Hobert, 2010; Amran et al., 2021). HSPGs and chondroitin proteoglycans (CPGs) both have prominent roles to play in developmental processes in C. elegans (Saied-Santiago et al., 2017). Most ECM molecules in C. elegans are conserved and are homologues to mammalian proteins, however, fewer ECM protein isoforms are present and these exhibit less redundancy compared to in mammalian tissues. Furthermore, mutations in C. elegans produce comparable developmental defects to those evident in mammals. The small size, short lifespan of 3–4 days and defined neural interconnectivity of the C. elegans nervous system facilitate its use in systematic functional studies that have yielded valuable insights into neuronal differentiation (Chisholm and Jin, 2005). This information is also relevant to more complex neural systems since the basic cellular machinery of synaptic transmission is highly conserved across species. In C. elegans, 719 out of ∼20,000 genes (∼4%) of its genome encode matrisome proteins, including 181 collagens, 35 glycoproteins, 10 proteoglycans, and 493 matrisome-associated proteins, 173 out of the 181 collagen genes are unique to nematodes and are predicted to encode cuticular collagens (Teuscher et al., 2019).
Comparative genomic studies on human and C. elegans has identified orthologous genes in C. elegans that have comparable regulatory properties to human genes and demonstrated mechanisms relevant to human biology. C. elegans represents an experimental system that can be used in genetic approaches to address biological questions relevant to human development, physiology, and disease. Genetic based phenotype screens have identified C. elegans genes homologous to human disease-associated genes, and fundamental properties about their roles and mechanisms of action in pathological human tissues. Reverse genetic methods developed in C. elegans have expanded the repertoire of genetic approaches to examine the roles of specific genes in human disease processes. These methods have the ability to phenocopy loss-of-function mutations by feeding worms bacteria expressing double-stranded RNA, RNAi. Studies in C. elegans have also facilitated genome-wide screens or screens specifically targeting human disease genes and the large-scale generation of deletion or point mutations for functional genetic studies (Kim et al., 2018).
The Drosophila matrisome consists of 641 genes, 27 are homologs/orthologs to human core matrisome genes, 219 are homologs/orthologs to mammalian matrisome genes and the remaining 395 are genes are specific to Drosophila (Davis et al., 2019). Comparative genomic analyses have been undertaken between human and Drosophila genomes and core promoter regions. Although fruit flies have a genome that is 25 times smaller than the human genome, many fruit fly genes have comparable genes in humans that control the same biological functions. Twelve fruit fly genome sequences are available in the FlyBase database1, a collaboration of Harvard, Cambridge, Mass.; Indiana, Bloomington; and the University of Cambridge, United Kingdom. The fruit fly genome sequences are also available from NIH’s National Center for Biotechnology Information2, European Molecular Biology laboratory’s Nucleotide Sequence Database, EMBL-Bank3, and DNA Data Bank of Japan, DDBJ4 (FitzGerald et al., 2006).
As found in higher organisms, PGs have important roles in cellular regulation critical to the development of metazoan organisms. C. elegans, however, produces a large amount of non-sulfated chondroitin (Chn) in addition to a small amount of low sulfation CS. Until recently, C. elegans was known to express nine Chn/CS-PGs dissimilar to vertebrate CS-PGs. A recent glycoproteomic study identified 15 additional CPGs in C. elegans and three of these were homologous to human proteins, thus selected CS-PG core proteins appear conserved throughout evolution (Noborn and Larson, 2021). Bioinformatic analysis of primary amino acid sequence data identified a broad range of functional domains in these C. elegans PGs, thus specific PG core protein mediated functional properties appeared to have evolved early in metazoan evolution.
Drosophila melanogaster has also proved to be a useful model for the investigation of developmental GAG functions in vivo confirming in vitro findings. HS and CS GAG side chains of PGs are structurally conserved between Drosophila and mammals, including humans. Mutant and RNAi fruit flies show that HS-PGs and CS-PGs play key roles in the regulation of developmental signaling pathways involving FGF, Wingless (Wg)/Wnt, Hedgehog, and Decapentaplegic (Dpp, a ligand of the TGFβ superfamily). Glycosyl transferases, sulfotransferases, sugar-nucleotide transporters including 3′-phosphoadenosine 5′-phosphosulfate (PAPS) transporters, all have important roles to play in GAG biosynthesis and the functional status of PGs in neuronal development and maintenance (Nishihara, 2010). It should be noted, however, that the major non-sulfated Chn in C. elegans has crucial roles in embryonic cell and tissue development and tissue morphogenesis. Since Chn is present on mammalian PG core proteins along with CS no studies have been possible to specifically target the functional roles of Chn in these PGs, however, insights into the biological properties of Chn in mammals can be gleaned from the use of Chn as a drug in the treatment of osteoarthritis (OA) (Singh et al., 2015). A meta-analysis of 43 reviews which analyzed the use of Chn for the treatment of OA and alleviation of joint pain yielded moderate to inconclusive results. However, Chn elicited a significant improvement in the anti-inflammatory profile of synoviocytes and chondrocytes in an OA model analyzed by multiplex and Western blot analysis. Chn significantly decreased the levels of several pro-inflammatory cytokines (IL-1β, IL-5, 6, 7, 9, 15, 17), anti-inflammatory cytokines (IL-4, IL-10), chemokines (IL-8, MCAF, MIP-1a, MIP-1b, RANTES) in synovial fluid samples and decreased expression of the OA biomarkers MyD88 and MMP-13 (Vassallo et al., 2021). Evidence therefore exists that Chn displays anti-inflammatory properties in mammalian tissues. However in C. elegans where Chn predominates over CS the biological properties of Chn have more profound effects on neural biology. A number of studies have demonstrated the fundamental biological roles played by Chn, CS, and DS, attached to the core proteins of cell surface and ECM PGs (Sugahara et al., 2003). PGs decorated with CS, DS, or HS have diverse roles in growth factor, morphogen and cytokine-mediated cell signaling through cellular receptors that play critical roles in the development of the CNS (Melrose et al., 2021). As discussed later in this chapter, these functions of PGs are closely associated with GAG sulfation patterns. Surprisingly, non-sulfated Chn is indispensable in the morphogenesis and cell division of C. elegans, as revealed by RNA interference experiments of the recently cloned chondroitin synthase gene and by the analysis of squashed vulva (sqv) gene mutants. It should be noted that while orthologous forms of human perlecan/HS-PG2 exist in C. elegans (UNC-52) and D. melanogaster (Trol) these have a different modular structure to human perlecan and are devoid of domain-I and thus they lack HS substitution (Celestrin et al., 2018). Thus the interactive properties provided by the HS chains of human perlecan do not occur in these orthologs and the interactive properties conveyed by these form of perlecan is due to modular components in their core proteins (Condomitti and de Wit, 2018; Martinez et al., 2018).
The Instructional Properties of CS- and HS-PGs in Neural Development and Tissue Morphogenesis in Caenorhabditis elegans
Blocking of chondroitin synthesis results in cell proliferative defects in early embryogenesis in C. elegans and leads to early embryonic death (Mizuguchi et al., 2003). Mutations in eight sqv genes in C. elegans causes defects in embryonic cytokinesis and in vulval morphogenesis during postembryonic development. Sqv-1, 2, 3, 4, 6, 7, 8 control CS and HS biosynthesis, while sqv-5 encodes a bifunctional glycosyltransferase responsible for the biosynthesis of Chn, but not HS (Hwang et al., 2003a, b). Sqv mutations in C. elegans have lethal consequences due to disruption in cell proliferation and the lack of the formation of an extracellular space between the egg and the eggshell, apparently due to disruption in the normal GAG containing ECM structures. Cloning and characterization of the sqv-2 and -6 genes showed that sqv-6 encoded a protein similar to human xylosyltransferase, while sqv-2 encoded a protein similar to human galactosyltransferase II. SQV-6 and SQV-2 proteins act in concert with other SQV proteins to catalyze the stepwise formation of the PG core protein linkage tetrasaccharide GlcAβ1,3Galβ1, 3Galβ1,4Xylβ-O-(Ser), common to CS and HS (Hwang et al., 2003a, b). This linkage tetrasaccharide acts as an acceptor molecule for the assembly of the CS and HS chains. Chain elongation is initiated by the addition of GlcNAc or GalNAc, with the former addition resulting in the biosynthesis of HS chains by sequential additions of GlcA and GlcNAc, while if GalNAc is the initial sugar added to the acceptor group this results in the synthesis of CS chains. These GAG chains are sulphated in a later biosynthetic stage at various positions on GlcNAc or GalNAc by specific sulfotransferases. GlcA can also be epimerised to IdoA and sulphated at O-2 in HS chains. HS chains can be sulphated at multiple positions. CS sulfation is an important functional determinant in the regulation mammalian neural tissue development and repair, however, in C. elegans and D. melanogaster the Chn chains are not sulphated but nevertheless have essential roles to play in early embryonic development and tissue morphogenesis in later developmental stages.
Vertebrates produce multiple CS-PGs with important roles in development and the mechanical performance of tissues. The Chn chains in C. elegans are not sulfated, but nevertheless they still play essential roles in embryonic development and vulval morphogenesis (Olson et al., 2006). C. elegans Chn PG core proteins, do not share sequence similarities with PGs from D. melanogaster or Hydra vulgaris. The C. elegans CPG-1 and CPG-2 PGs are expressed during embryonic development and bind chitin, which may have a structural role to play in the egg (Olson et al., 2006). Chitin is a widespread polymer in nature and is a polymer composed of N-Acetyl glucosamine. Chitin is a primary component of fungal cell walls, the exoskeletons of arthropods such as crustaceans and insects and the scales of fish. Depletion of CPG-1/CPG-2 results in multinucleated single-cell embryos in C. elegans, this is also observed with depletion of the SQV-5 chondroitin synthase protein, Chn chains of CPG1/CPG2 play essential roles in cytokinesis. This is achieved through regulation of GAG biosynthetic enzymes. C. elegans microRNA mir-79, an ortholog of mammalian miR-9, controls sugar-chain homeostasis by targeting two proteins in the PG GAG biosynthetic pathway: a chondroitin synthase (SQV-5; squashed vulva-5) and a uridine 5′-diphosphate-sugar transporter (SQV-7). Loss of mir-79 causes neurodevelopmental defects through dysregulation of SQV-5 and SQV-7. This results in a partial shutdown of HS biosynthesis that effects the LON-2/glypican pathway and disrupts neuronal migration. MicroRNA thus represents a regulatory axis that maintains PG homeostasis. Sqv genes 1–8 control the invagination of vulval epithelial cells, normal oocyte formation and embryogenesis. Sequencing of sqv-3, sqv-7, and sqv-8 genes indicated potential roles for the proteins they encode in glycolipid or glycoprotein biosynthesis. sqv-3, -7, and -8 affect the biosynthesis of GAGs and the bioactivity of PGs establishing their essential roles in tissue morphogenesis and pattern development in C. elegans (Bulik et al., 2000).
Sulfation of PG GAG side chains has critical roles to play in the cell regulatory properties of PGs and their roles in many essential physiological processes. Sulfation reactions involves activated sulfate, and the universal sulfate donor 3′-phosphoadenosine 5′-phosphosulfate (PAPS). In animals, PAPS is synthesized from ATP and inorganic sulfate by PAPS synthase, genetic defects in PAPS synthase 2, one of two PAPS synthase isozymes, causes dwarfism. In order to better understand the developmental role of sulfation in tissue PGs, a C. elegans PAPS synthase-homologous gene, pps-1 has been cloned and the depleted expression of its product, PPS-1 examined (Dejima et al., 2006). PPS-1 protein exhibits specific roles in the formation of PAPS in vitro. Disruption of the pps-1 gene by RNAi methods causes widespread developmental tissue defects, a decrease in GAG sulfation in the pps-1 null mutant exhibits larval lethality. Sulfation is essential for normal growth and the integrity of the epidermis in C. elegans and has been used as a model to demonstrate the role of HS modifications in a defined biological process. Genetic analyses suggest that syndecan/sdn-1 and HS 6-O-sulfotransferase, hst-6, function in a signaling pathway and glypican/lon-2 and HS 2-O-sulfotransferase, hst-2, function in a parallel pathway. HS modifications may be part regulated at the level of tissue expression of genes encoding for HS-PGs and HS modifying enzymes. There is a delicate balance in such HS modifications that may deleteriously effect cell migration, HS is a critical regulator of cell signaling in normal development and disease. HS-PGs have roles in the structural organization of neurochemical synapses, involving interactions with the core protein as well as the HS side chains (Cizeron et al., 2021). Specific modifications to HS contribute to a sugar code which provides specificity to synaptic interactions. SDN-1 is a unique C. elegans syndecan ortholog found in synaptic junctions. 3-O sulfation of SDN-1 maintains the ECM protein punctin/MADD-4/ (MAP kinase-activating death domain protein) that defines the synaptic domains, however, in mammals 3-O sulfation is a rare modification in HS despite the seven HS modifying enzymes that can produce 3-O sulfation.
Punctin/MADD-4, a member of the ADAMTSL ECM protein family, is a synaptic organizer in C. elegans. MADD is an enzyme encoded by the MADD gene. The Ig-like domain of MADD is the primary determinant for N-MADD-4B interactions with NLG-1 in vitro (Platsaki et al., 2020). At GABAergic neuromuscular junctions, the short isoform MADD-4B binds the ectodomain of neuroligin (NLG-1), which is also a postsynaptic organizer of inhibitory synapses (Tu et al., 2015). Proteolysis of MADD-4B generates N-MADD-4B, which contains four thrombospondin domains and one Ig-like domain that bind NLG-1 (Maro et al., 2015). A second processing event eliminates the C-terminal Ig-like domain of N-MADD-4B and its ability to bind NLG-1. The death domain of the type 1 tumor necrosis factor receptor (TNFR1) mediates the downstream effects of TNF. MADD interacts with TNFR1 residues and is a component of the TNFR1 signaling complex. The MADD death domain stimulates ERK and c-JUN N-terminal kinase MAP kinases inducing phosphorylation of cytosolic phospholipase A2. Thus, MADD links TNFR1, MAP kinase activation and arachidonic acid release, which may explain the pleiotropic effects of TNF.
Growth cones facilitate the repair of damaged neural tissue by promoting axon regeneration, syndecan, is required for growth cone function during axon regeneration in C. elegans (Edwards and Hammarlund, 2014; Gopal et al., 2016, 2021). In the absence of syndecan, regenerating growth cones are unstable and they collapse, impeding regrowth to target cells. Syndecan has two distinct functions during axon regeneration: (i) axon guidance requiring its HS-dependent expression outside the nervous system (ii) intrinsic growth cone stabilization mediated by the SDC core protein independently of HS.
The Instructional Properties of CS- and HS-Proteoglycans in Neural Development and Tissue Morphogenesis in D. melanogaster
Blocking Chn synthesis results in defects in cytokinesis and embryogenic development in D. melanogaster leading to early embryonic death. This demonstrates the essential developmental roles Chn plays in Drosophila embryonic cytokinesis and cell division. Drosophila has proved to be a useful model for the investigation of developmental GAG functions in vivo confirming in vitro findings with GAGs (FitzGerald et al., 2006; Nishihara, 2010). HS and CS GAG side chains of PGs are structurally conserved between Drosophila and mammals, including humans. CS sulfation is an important functional determinant in the regulation of mammalian neural tissue development and repair; however, in C. elegans and D. melanogaster the Chn chains are not sulfated but, nevertheless, have essential roles to play in early embryonic development and tissue morphogenesis in later developmental stages.
Windpipe (Wdp) is a novel CS-PG recently identified in Drosophila. Wdp is a single-pass transmembrane protein with leucine-rich repeat (LRR) motifs and has three extracellular CS chain attachment sites (Takemura et al., 2020). Wdp modulates the Hedgehog (Hh) cell signaling pathway. In the wing disk, overexpression of Wdp inhibits Hh signaling an effect that is dependent on its CS chains and protein interactive LRR motifs. The JAK/STAT (Janus Kinase/Signal Transducer and Activator of Transcription) signaling pathway regulates adult stem cell activities and is essential for the maintenance of intestinal homeostasis in Drosophila. Wdp interaction with the receptor Domeless (Dome) promotes its internalization and lysosomal degradation. Wdp thus acts as a negative feedback regulator of JAK/STAT cell signaling and is a novel regulatory component of JAK/STAT signaling in Drosophila adult intestines (Ren et al., 2015).
Glycosaminoglycans such as HS and CS have roles in intercellular signaling thus disruptions of genes encoding enzymes that mediate GAG biosynthesis have severe consequences in Drosophila and mice. Mutations in the Drosophila gene sugarless, encoding a uridine diphosphate (UDP)-glucose dehydrogenase, impairs developmental signaling through the Wnt family member Wingless, and FGF and Hedgehog signaling pathways. Undersulfated and oversulfated CS chains are implicated in neural development, cloning of a chondroitin synthase homolog in Caenorhabditis elegans and depletion in Chn expression results in defects in cytokinesis in early embryogenesis and early embryonic death demonstrating the essential role Chn plays in early developmental processes (Mizuguchi et al., 2003).
Drosophila has an NG2 homologue called kon-tiki (kon), that promotes CNS repair (Losada-Perez et al., 2016). Crush injury upregulates kon expression and induces glial cell proliferation and differentiation by activating glial genes and prospero (pros). Negative feedback loops with Notch and Pros allow Kon to drive the homeostatic regulation of neuronal repair with modulation of Kon levels in glia, potentially preventing or promoting CNS repair (Losada-Perez et al., 2016). The interplay between Kon, Notch, and Pros is therefore essential in neural repair in Drosophila. Prospero homeobox protein-1 is encoded by the PROX1 gene in humans. This pan-neural protein has essential roles to play in the proper differentiation of neuronal lineages and in the expression of genes in the Drosophila CNS. Prospero is a sequence-specific DNA-binding protein that can act as a transcription factor through interaction with homeodomain proteins to differentially modulate their DNA-binding properties (Hassan et al., 1997; Yousef and Matthews, 2005). Functional interactions between Prospero and homeodomain proteins is supported by observations showing that Prospero, together with the homeodomain protein, Deformed, are required for proper regulation of a Deformed-dependent neural-specific transcriptional enhancer (Hassan et al., 1997). The DNA-binding and homeodomain protein-interactive properties of Prospero are localized in its highly conserved C-terminal region.
Syndecan (Sdc) is a conserved transmembrane HS-PG bearing CS chains on its ectodomain. In vertebrates, this extracellular domain of Sdc is shed and acts as a soluble effector of cellular communication events, and the Sdc cytoplasmic domain participates in intracellular signaling needed to maintain epithelial integrity. In Drosophila, Sdc has been shown to be necessary for Slit signaling-dependent axonal guidance during CNS development (Chanana et al., 2009). Sdc acts in a cell-autonomous manner in Slit-receiving cells and that its membrane-anchored extracellular domain is sufficient to mediate Slit signaling. The HS-PG Dally-like protein (Dlp), which lacks CS on its extracellular domain, can only partially substitute for Sdc function but its activity is not restricted to the Slit target cells. Sdc and Dlp act in a cooperative but non-redundant manner in neural tissues with Dlp transferring Slit from its site of expression to the target cells, where it interacts with CS-modified Sdc.
Caenorhabditis elegans Netrins and Neural Development
UNCoordinated-6 (UNC-6) was the first C. elegans member of the netrin family that was discovered (Krahn et al., 2019). UNC-6 shares homology to human netrin-1, and is a key signaling molecule in the regulation of directional axonal migration in nematodes (Krahn et al., 2019). Similar to netrin-1, UNC-6 interacts with multiple receptors to guide axonal migration (Moore et al., 2007; Rajasekharan and Kennedy, 2009; Ogura et al., 2012). Netrin is a key guidance protein regulating the orientation of axonal growth during neural network formation in C. elegans. LON-2/glypican, modulates UNC-6/netrin signaling through interactions with the UNC-40/DCC (deleted in colorectal carcinoma) receptor (Yang et al., 2014). LON-2 expressed on the cell surface in the intestine and hypodermis in C. elegans and in D. melanogaster promotes growth factor binding in several developmental processes, negatively regulating the TGF-β receptor signaling pathway and BMP-like signaling that regulates tissue growth and body length. N-terminal sequencing of the signal peptide of LON-2, identified a 14 cysteine domain of functional importance, SGXG GAG attachment site and C-terminal GPI anchor site showing that LON-2 is a member of the glypican family (Eisenhaber et al., 2000; Gumienny et al., 2007). The other C. elegans glypican, gpn-1, has no significant effect on the body size (Blanchette et al., 2015). Null mutations in C. elegans genes encoding HS biosynthetic enzymes that process the HS side chains of LON-2, significantly reduce body size. hse-5, hst-2, and hst-6 encode C. elegans counterparts to mammalian glucuronyl C5-epimerase, 2 O-sulfotransferase, and 6 O-sulfotransferase, respectively. This demonstrates the important functional role HS plays in neural development in C. elegans and the importance of the HS sulfation patterns for this activity (Bülow and Hobert, 2004; Gysi et al., 2013; Díaz-Balzac et al., 2014; Saied-Santiago et al., 2017). HS chains of HS-PGs carry multiple structural modifications due to sulfation and epimerization of GlcA that influence their ligand binding properties. This is why HS-PGs have such diverse effects in tissue and axonal development. The core proteins of C. elegans SDN-1 and glypican/LON-2 and HS modifying enzymes thus both have roles in axonal guidance through interactions with UNC-6/Netrin (Rhiner et al., 2005). C. elegans SDN-1/syndecan control of neural migration and axonal guidance also occurs through regulation of Slit/Robo signaling in parallel with C5-epimerase HSE-5, and/or the 2O-sulfotransferase HST-2 activity, which provide distinct regulatory HS modification patterns on SDN-1.
Mammalian Neuronal Proteoglycans
Neural PGs occur as large and small extracellular, cell surface and intracellular components. The salient features of neural PGs are summarized in Table 1 and their structural organizations are shown schematically in Figures 3–5.
Roles for the CS-Rich Lectican PG Family in Perineuronal Net Structures
The lectican family of neural PGs have similar structures to aggrecan but do not contain keratan sulfate (KS) or a G2 globular domain. Furthermore, their molecular dimensions are smaller due to shorter core proteins and less extensive distributions of CS side-chains (Yamaguchi, 2000). Lectican PGs occur as diffuse ECM components and as dense PNN structures attached to HA through interactions with lectican N-terminal HA-binding regions. This aggregate is stabilized by tenascin-R and Bral-1 (Hyaluronan and Proteoglycan Link Protein 2; HAPLN2). The form of aggrecan found in brain differs from that of cartilage aggrecan in that it contains less KS chains, and its CS chains are less densely distributed along the CS1 and CS2 core protein regions (Hayes and Melrose, 2020a). Some CS chains in neural aggrecan are replaced by HNK-1 trisaccharide which also attaches to the same core protein linkage tetrasaccharide as CS. Once the HNK-1 trisaccharide is assembled chain elongation ceases resulting in a reduction in CS chain density but introduces cell interactive properties. Neural aggrecan guides neural crest progenitor cell migration during embryonic neurogenesis and formation of the neural tube and notochord (Hayes and Melrose, 2020a). Preclinical spinal cord injury (SCI) and traumatic brain injury (TBI) animal model studies demonstrate that the enzymatic degradation of CS-PGs from gliotic scars using chondroitinase ABC improves neuronal functional recovery (Bradbury and Carter, 2011; Cheng et al., 2015; Muir et al., 2019). Endogenous degradation of the core protein of CS-PGs by ADAMTS-4 also improves neuronal functional recovery (Tauchi et al., 2012). While the CS-A and CS-C side chains of the lecticans inhibit neural repair, not all CS-PGs inhibit axonal re-growth (Mencio et al., 2021). PGs containing over-sulfated CS-B, and CS-E promote neurite outgrowth and functional recovery (Bovolenta and Fernaud-Espinosa, 2000). The EGF-like motif in the G3 domain of the lecticans has also been shown to regulate cell migration and tissue repair (Aguirre et al., 2007; Du et al., 2010). Overexpression of human EGFR in CNP (hEGFR) mice accelerates remyelination and functional recovery following focal demyelination. Progenitor cells over-expressing NG2 PG also improve re-myelination through EGFR mediated cell signaling (Keirstead and Blakemore, 1999; Aguirre et al., 2007). PNNs surrounding the soma and dendrites of a number of neuronal cell types are prevalent during neural development and maturation (Carulli and Verhaagen, 2021). A similar structure, the perinodal ECM surrounds the axonal nodes of Ranvier and appear after re-myelination, acting as a protective ion-diffusion barrier (Bekku and Oohashi, 2019; Fawcett et al., 2019). Perinodal structures in the Nodes of Ranvier also contain PNN components such as brevican and versican V2 (Bekku et al., 2009; Dours-Zimmermann et al., 2009).
Perineuronal net are variably distributed in the brain, the somatosensory frontal lobes of the cerebral cortex have a particularly high density of PNNs, however, they are sparsely distributed in the sub-ventricular and sub-granular dentate gyrus of the hippocampus. These regions contain neuro-progenitor stem cell niches termed fractones (Mercier and Arikawa-Hirasawa, 2012; Sato et al., 2019). Abnormal PNN formation impacts on neural development and may result in degenerative synaptic pathology in schizophrenia (Pantazopoulos et al., 2021), bipolar disorder, major depression, and autism spectrum disorders (Sorg et al., 2016). CS-PGs in PNNs control synaptic plasticity, and have roles in memory in the aging brain, deterioration of PNNs contribute to the age-dependent decline in brain function. Recent work has revealed the importance of PNNs in the control of CNS plasticity. Digestion, blocking or removal of PNNs impedes functional recovery after a variety of CNS lesions. Deficient PNN numbers are implicated in a number of psychiatric disorders and suggested as therapeutic targets in their treatment (Dityatev et al., 2021). Incorrect assembly of PNNs or degradation of PNN components by excessive MMP activity can lead to the development of epilepsy (Rankin-Gee et al., 2015; Dubey et al., 2017; Mencio et al., 2021). Deficient levels of HA in PNN structures promote epilepsy and spontaneous convulsions in animal models (Perkins et al., 2017). The CS-PGs of PNNs have important functional roles to play in perisynaptic structures that prevent the development of Alzheimer’s disease (AD) (Morawski et al., 2012), cortical regions with abundant levels of ECM CS-PGs are less affected by degenerative features associated with the development of AD (Bruckner et al., 1999). PNNs also have important roles to play in Schizophrenia and Bipolar disorder (Berretta, 2012; Mauney et al., 2013). In unaffected individuals, the density of PNNs in the prefrontal cortex increases during pre-puberty and early adolescence. However, in patients with schizophrenia, a 70% reduction in PNN numbers in the prefrontal cortex has been observed (Mauney et al., 2013). The organization and function of PNNs is also disturbed in bipolar disorder (Gandal et al., 2018). Stem cells have been administered to promote recovery of normal PNN structure in an attempt to reverse these debilitating conditions (Forostyak et al., 2014). With an appreciation of PNNs and their important contributions to synaptic stability (Miyata et al., 2018), plasticity, memory and cognitive learning in normal brain tissues this has led to the identification of abnormalities in PNN assembly or expression of PNN components associated with particular neurodegerative conditions (Yamaguchi, 2000; Wen et al., 2018). Thus PNNs have become a therapeutic focus in the treatment of these conditions (Dityatev et al., 2006, 2010, 2021).
Neural Proteoglycans
Aggrecan
In the CNS/PNS, aggrecan core protein contains KS, HNK-1 trisaccharide and CS side chains (Hayes and Melrose, 2020a) that convey unique tissue-specific functional properties (Figure 3A). Aggrecan’s ability to form macro-aggregates with HA provides water imbibing, space-filling and matrix stabilizing properties to the PNS/CNS ECM and in brain establishes ionic gradients and microcompartments important for the optimal activity of neural cell populations.
The ability of the aggrecan core protein to assemble CS and KS chains at high density provides its well-known water-imbibing properties. Specific arrangements of GAG chains on aggrecan are functional determinant providing unique tissue context-dependent regulatory properties over neural cell populations. The aggrecan core protein KS and CS side chains and N-linked oligosaccharides all display neurite outgrowth-inhibitory activity (Hering et al., 2020). The cell mediatory properties of aggrecan’s GAGs thus convey diverse regulatory roles in tissue development and in neuroprotective matrix stabilization of PNNs. Variation in the sulfation position and density on the CS side chains can influence morphogen and growth factor binding relevant to tissue development (Reichsman et al., 1996; Nandini and Sugahara, 2006; Nadanaka et al., 2008; Whalen et al., 2013; Mizumoto et al., 2015).
Versican
Versican is a large member of the lectican family (Yamaguchi, 2000) with a 400 kDa core protein modestly substituted with CS side chains (Figure 3B). Versican occurs as four alternatively spliced isoforms, VO, V1, V2, V3 (Yamaguchi, 2000). Versican was named after its versatile roles as a cell instructional and ECM organizational functional PG in tissue development, cell migration, adhesion, proliferation, and differentiation. Versican V1 promotes neuritogenesis (Wu et al., 2004). Versican interacts with HA through its G1 globular domain, C-type lectin G3 motifs interact with tenascin-R to stabilize HA-versican macro-aggregates (Bignami et al., 1993) and with HNK-1-substituted cell adhesion proteins (Bignami et al., 1993), HNK-1 glycolipids (Miura et al., 2001), and sulfated GAGs (Miura et al., 1999). Free G1, G3 versican domains released by proteases have regulatory properties in cell adhesion, proliferation, apoptosis, migration, angiogenesis, invasion, and metastasis. Versican G3 domain regulates neurite growth and synaptic transmission of hippocampal neurons by activating EGFR (Xiang et al., 2006). NgR2 interacts with versican G3 suppressing axonal plasticity (Bäumer et al., 2014) and has a dominant-negative effect on astrocytoma cell proliferation (Wu et al., 2001). An 80 kDa N-terminal matricryptic fragment of versican (versikine) generated by ADAMTS-4 (a disintegrin and metalloproteinase with thrombospondin motifs) cleavage acts as an alarmin in the innate immune response (Yamada et al., 2011). Interactions between myeloma stromal and myeloid cells generates versikine, a DAMP (damage-associated molecular pattern) that may facilitate immune sensing of myeloma tumors (Hope et al., 2016). Versikine also occurs during connective tissue remodeling during embryonic development (Nandadasa et al., 2014). Versican V2 is highly expressed in the adult brain (Schmalfeldt et al., 1998), promotes angiogenesis (Yang and Yee, 2013), and interactions with neurons (Horii-Hayashi et al., 2008). Versican V1 induces neural differentiation and neuritogenesis (Wu et al., 2004). Versican isoforms are differentially distributed in gliomas, medulloblastomas, schwannomas, neurofibromas, and meningiomas. Versican V2 is the major isoform found in gliomas. Versican V0 and V1 are found in all tissues, Versican V3 is found in all tissues except medulloblastomas.
Neurocan
Neurocan has a widespread distribution in the CNS/PNS and is a component of PNNs (Schmidt et al., 2020) and regulates synaptic signaling (Sullivan et al., 2018). Neurocan (Figure 3C) has roles in neurodegenerative disorders (Lin et al., 2021). β-amyloid increases neurocan expression in astrocytes through Sox9 influencing the development of AD. Mutations in the neurocan gene predispose to bipolar disorder and schizophrenia (Mühleisen et al., 2012; Raum et al., 2015). Neurocan regulates neural migration and axonal development in the cerebral cortex influencing the folding of the occipital and pre-frontal lobes and an increased probability of developing schizophrenia (Schultz et al., 2014).
Brevican
Brevican is the smallest lectican CS-PG family member (Figure 3D) present in PNNs in some cases, but aggrecan and versican are the principal lecticans in PNNs (Yamaguchi, 1996). Brain-enriched hyaluronan-binding protein (BEHAB) is an N-terminally cleaved (Matthews et al., 2000) bioactive fragment of brevican that is dramatically increased in human gliomas (Nutt et al., 2001; Viapiano et al., 2008) where it promotes glial cell motility and the aggressiveness of gliomas (Yamaguchi, 1996; Gary and Hockfield, 2000; Nutt et al., 2001; Viapiano et al., 2008; Giamanco and Matthews, 2020).
Other Non-Lectican Large Neural Proteoglycans
Phosphacan/Receptor Protein Tyrosine Phosphatase-Zeta (RPTP-ζ)
A cell membrane bound precursor form of phosphacan (RPTP-ζ) (Figures 4A,B) is processed by proteases to release a soluble PG ectodomain (Figure 4C) called phosphacan (Chow et al., 2008), truncated and non-GAG substituted forms of phosphacan have also been described (Figure 4D) with neurite outgrowth promoting activity (Fujikawa et al., 2017). This property is thus due to the core protein in some phosphacan species while neurite outgrowth activity may also be conveyed by GAG components, such as oversulfated CS-B and CS-E, in other phosphacan glycoforms (Dobbertin et al., 2003; Hikino et al., 2003). Phosphacan populations bearing KS and HNK-1 have also been described as well as the more common CS-glycanated form (Melrose, 2019b). Phosphacan promotes PNN formation (Eill et al., 2020). RPTP-ζphosphacan contain extracellular carbonic anhydrase (CAH) and fibronectin type III repeat domains, which foster protein–protein interactions (Milev et al., 1994; Lamprianou et al., 2011). A truncated 90 kDa phosphacan form is not a PG, but is substituted with the HNK-1 trisaccharide which facilitates interactive properties with a number of cell adhesion and ECM molecules (Garwood et al., 2003). Phosphacan promotes neuron–glial interactions, neuronal differentiation, myelination, and axonal repair. The CAH carbonic anhydrase domain of phosphacan promotes protein–protein recognition, induces cell adhesion, neurite outgrowth of primary neurons, and differentiation of neuroblastoma cells (Adamsky et al., 2001); contactin is a phosphacan neuronal receptor that regulates neural development and axonal repair.
NG2 Proteoglycan/CSPG4
CSPG4 modular transmembrane CS-PG also occurs as a soluble protease generated form (Schäfer and Tegeder, 2018; Figure 4G). CSPG4 is expressed by oligodendrocyte precursor cells (OPCs), NG2 glia (Butt et al., 2019), pericytes (Girolamo et al., 2013), activated astrocytes in damaged neural tissues (Anderson et al., 2016) and fibroblasts and macrophages associated with the meninges (Tamburini et al., 2019). NG2/CSPG4 is the largest complex macromolecule of the neuron surfaceome (Tamburini et al., 2019). OPCs are sensitive to electrophysiological stimulation through synaptic interactions that induce cellular proliferation and tissue repair.
The 290 kDa ectodomain of CSPG4 is released from OPCs by ADAM10 (α-secretase) (Moransard et al., 2011; Clarke et al., 2012; Huang et al., 2014) and are a major source of neural CSPG4 (Jones et al., 2002). Neurons, astrocytes, and microglial cells do not express CSPG4. Glioblastoma cells (Moransard et al., 2011; Huang et al., 2014), endothelial cells and pericytes in gliotic scars express CSPG4 (Jones et al., 2002; McTigue et al., 2006). NG2 PG binds type V and VI collagen through its central non-globular domain (Clarke et al., 2012; Huang et al., 2014) and with integrins (Sakry and Trotter, 2016). C-terminal LamG domains of NG2 interact with BM components and are crucial for formation of synaptic neuroligin-neurexin complexes and glial cell signaling (Jeong et al., 2017) and also interact with matriglycan-dystroglycan, perlecan, agrin and type XVIII collagen to localize NG2PG in motor neuron endplates in the neuromuscular junction (NMJ) (Walimbe et al., 2020).
Betaglycan
Betaglycan homo-dimeric transmembrane (TM) CS/HS PG (Mythreye and Blobe, 2009; Bilandzic and Stenvers, 2011) contains inhibin, FGF-2, Wnt, and TGF-β binding sites (Boyd et al., 1990; Segarini, 1991; Massagué et al., 1992; Sandbrink et al., 1996; Miyazono, 1997; Lewis et al., 2000; Gray et al., 2001; Kim et al., 2019; Bernard et al., 2020; Figure 5B). The HS chains of betaglycan bind FGF-2. Wnt signaling is regulated independently of TGF-β (Jenkins et al., 2018). HS inhibits Wnt signaling, while CS promotes Wnt signaling (Jenkins et al., 2016, 2018). Betaglycan N-and O- linked oligosaccharides and GAG chains, modulate betaglycan’s growth factor-mediated, vascular and cancer cell migratory properties (Pantazaka and Papadimitriou, 2014) and Inhibin A and B binding (Makanji et al., 2007). Fragments of betaglycan released by plasmin and MMPs act as circulating antagonists to normal betaglycan interactions. Inhibin/activin subunits and betaglycan are co-localized in the human brain (MacConell et al., 2002; Miller et al., 2012). Betaglycan-FGF-2 mediate neural proliferation and differentiation in neuroblastoma (Knelson et al., 2013). TGF-β also enhances glioma migration and invasion. TGF-β TbetaR I-III signaling phosphorylates Sma and MAD-related protein (SMAD), soluble TbetaR-I-III antagonize this process (Naumann et al., 2008). TGF-β enhances adult neurogenesis in the sub-ventricular zone (SVZ) and supports pro-neurogenic roles for TGF-β (Battista et al., 2006; Mathieu et al., 2010). Activins and inhibins, stimulate or inhibit secretion of FSH and the differentiation, proliferation and function of many cell types (Vale et al., 2004). Activin receptors highly expressed in neuronal cells, and activin mRNA are upregulated by neuronal activity. Models of TBI display enhanced activin A expression exacerbated by hypoxic/ischemic injury, mechanical irritation, and chemical damage (Florio et al., 2007). FGF-2 is neuroprotective and prevents apoptosis by strengthening anti-apoptotic pathways promoting neurogenesis in the adult hippocampus by upregulation of activin A activity (Woodruff, 1998; Alzheimer and Werner, 2002; Florio et al., 2007).
The Small Neural Proteoglycans
Neuroglycan C
Neuroglycan C is a part time (Oohira et al., 2004) brain specific TM (Watanabe et al., 1995; Yasuda et al., 1998; Shuo et al., 2004) 150 kDa CS-PG (Figure 4J) with a 120 kDa core protein that can also be shed by MMPs (Shuo et al., 2007), GAG-free forms of CSPG5 have also been described. Neuroglycan C, is a novel member of the neuregulin family (Kinugasa et al., 2004), interacting with pleiotropin (Nakanishi et al., 2010) producing neurite outgrowth-promoting activity mediated by phosphatidylinositol 3-kinase and protein kinase C (Nakanishi et al., 2006). CSPG5 forms peri-synaptic structures in the postnatal adult rat cortex (Jüttner et al., 2013). Impaired CSPG5 properties are evident in schizophrenia (So et al., 2010). Alternatively spliced forms have been identified in the human brain, recombinant CSPG5 induces phosphorylation of Erb2 and Erb3 and induces proliferation of neocorticol neurons (Kinugasa et al., 2004; Nakanishi et al., 2006). The neurite outgrowth promoting activity of neuroglycan C resides in its EGF and acidic amino acid domains (Nakanishi et al., 2006).
Syndecans
Syndecan transmembrane HS/CS-PGs (Figures 4E,F) modulate cell adhesion, cell–cell interactions and ligand-receptor interactions that regulate neural plasticity, promote neural growth and development (Couchman et al., 2015; Gopal et al., 2021). Sdc-3 and Sdc-4 are found throughout the nervous system and have roles in motor neuron development (Liu et al., 2020), Slit/Robo signaling and guidance of axonal development (Steigemann et al., 2004). Sdc3 is a co-receptor for Heparin-Binding Growth-Associated Molecule (HB-GAM)/midkine-induced neurite outgrowth in perinatal rat brain neurons. HB-GAM acts as a local, synaptic factor that promotes presynaptic and postsynaptic differentiation during neural development. Sdc3 also has roles in adult neuronal synaptic plasticity in the hippocampus in rat models following injury and regulates the neuronal internodal axonal ECM during re-myelination in growth, remodeling and repair (Steigemann et al., 2004). Sdc3 and Sdc4 promote functional recovery of neural tissues re-organizing sodium and potassium channels (Steigemann et al., 2004). Oligodendrocytes are sensitive to electro-stimulation, and this maintains their membrane polarization required for the promotion of axonal repair processes. Sdc1 is upregulated by neurons following TBI and SCI (Murakami et al., 2015). Sdc1 and Sdc3 knockdown in dorsal root ganglia (DRG) neurons induces short neurite extensions suggesting roles in nerve regeneration, synaptic formation and plasticity (Akita et al., 2004; Steigemann et al., 2004). Syndecans shed from the cell surface by MMPs, act as soluble growth factor co-receptors that regulate cell migration acting antagonistically with cell surface syndecans competing for FGF and VEGF binding (Gopal et al., 2021) and interact with integrins potentially influencing cellular behavior, adhesion, spreading, migration, proliferation, tissue morphogenesis and pathogenetic tissue changes (Couchman et al., 2015).
Decorin
Decorin (Figure 3E) regulates cellular survival, migratory, proliferative and angiogenic signaling and collagen fibril formation, sequesters TGF-β and antagonizes receptor tyrosine kinase family members, including EGFR and IGF-IR (Schönherr et al., 2005; Iozzo et al., 2011; Neill et al., 2012). MayDay, a ∼12 kDa N-terminal chemotactic factor, generated by macrophage-induced MMP-12 cleavage of decorin, recruits mesenchymal stem cells (MSCs) to damaged tissue regions in vitro and in vivo, promoting tissue repair (Dempsey et al., 2020). In situ hybridization (ISH) has localized decorin in areas of microvascular proliferation within gliomas and may be a therapeutic target in anti-angiogenic therapy (Patel et al., 2020) or approaches targeting TGF-β activity in tumors (Birch et al., 2020). Decorin protects neuronal tissue from the damaging effects of anti-oxidants and neuroinflammation following TBI by inactivation and has anti-tumor activity by inhibiting glioma cell migration (Yao et al., 2016). Decorin inhibits TGF-β activity, fibrous scar formation in neural tissues following trauma.
Biglycan
Biglycan (Figure 3F) is synthesized by astrocytes (Koops et al., 1996) and immune cells (Mohan et al., 2010) and has neurotrophic activity, stimulates glial cell proliferation (Kikuchi et al., 2000) and neuronal cell survival (Koops et al., 1996). It is part of the proteome of the normal human retrobulbar optic nerve (Zhang et al., 2016) and is massively upregulated around gliotic scars following trauma (Stichel et al., 1995). NF-κB upregulates biglycan, protecting human neuroblastoma cells from nitric oxide (NO)-induced cell death by inhibiting AMPK-mTOR mediated autophagy and intracellular reactive oxygen species (ROS) production from mitochondrial oxidative bursts (Wang et al., 2015), targeting Erk1/2 and p38 signaling pathways to prevent NO-induced neuronal cell apoptosis (Chen et al., 2020). Biglycan regulates neuroinflammation (Xie et al., 2020) through M1 microglial cell activation in the early stages of subarachnoid hemorrhage, targeting Erk1/2 and p38 signaling pathways (Chen et al., 2018). Biglycan binds to Notch-3 and accumulates in cerebral autosomal dominant arteriopathy with subcortical infarcts and leukoencephalopathy (CADASIL) (Zhang X. et al., 2015). Transcriptomic profiling of the hypothalamus and hippocampus, supports a central regulatory role for biglycan (bgn) in molecular pathways linking metabolic events with the immune response, and neuronal plasticity (Ying et al., 2018). Transcriptomic profiling of hypothalamus, hippocampus, and liver supports regulatory roles for Bgn in molecular pathways involved in metabolism, the immune response, and neuronal plasticity (Ying et al., 2018).
Epiphycan
The synaptic poles of inner hair cells of the cochlea have audio-sensory properties and are surrounded by basket-like ECM structures with similar roles to the PNNs of neurons in the CNS (Sonntag et al., 2015). Epiphycan (Figure 3G) and aggrecan are cochlear components and of the gel-filled tectorial membrane which detects auditory signals and transmits these to sensory hair cells (Melrose, 2019a). Epiphycan is expressed by cochlear supporting cells and is necessary for normal hearing. Epiphycan mRNA is abundantly expressed in the cochlea in the organ of Corti of neonatal and adult mice. The cochlea of epiphycan knockout (KO) mice display a normal morphology, however, the auditory brain-stem response is altered since epiphycan is necessary for normal auditory function (Hanada et al., 2017). These PNN like structures surround high function neuron types which respond to signals received from inner sensory hair cells, transducing audio signals into mechanical stimuli and receptor-mediated action potentials which are sent to spiral ganglion neurons (Sonntag et al., 2015). These neuron types operate at very high discharge rates and efficiently convey signals to the auditory brainstem for further processing. The hearing loss evident in cartilage matrix deficiency (CMD) mice is related to aggrecan deficiency in the cochlea (Melrose, 2019a).
Appican
Two variants of the related amyloid precursor-like protein 2 (APLP2) carry single CS-E side chains, which bind midkine and pleiotrophin (Thinakaran and Sisodia, 1994; Shioi et al., 1995; Thinakaran et al., 1995). Multiple splice variants of amyloid-beta precursor protein (APP) (Figure 4I) and APLP2 arise from alternative splicing of three exons in APP and two exons in APLP2 (Kitaguchi et al., 1988; Ponte et al., 1988). The CS attachment site on APP/APLP2 is located adjacent to the membrane-spanning domain through deletion of 18 (APP) or 12 (APLP2) amino acids (Thinakaran et al., 1995). Splice variants also occur lacking CS side chains. APP and APLP are widely distributed in the CNS/PNS, APP is expressed by glial cells in the CNS/PNS.
Bikunin/ITI
Bikunin (inter-α-trypsin inhibitor light chain) is synthesized by neurons (Chen et al., 2016), occurring as a tissue form and small circulating PG containing a single CS chain (Figure 3J). Bikunin displays anti-inflammatory, anti-protease, anti-microbial, anti-viral properties and also functions as a growth factor (Fries and Blom, 2000; Lord et al., 2020). Bikunin is expressed in brain tissue (Takano et al., 1999; Kim et al., 2020) and accumulates in brain tumors. Bikunin CS chains contain embedded disulfated CS-D motifs (Lord et al., 2013, 2020). A related Kunitz protease inhibitor, placental bikunin (hepatocyte growth factor activator inhibitor type-2) has been reported to inhibit glioblastoma tumor invasion (Hamasuna et al., 2001), however, this is a dissimilar protein to serum bikunin. Traumatic impact to the brain and spinal cord can release nuclear components such as histone H1 into the circulation or cerebrospinal fluid (CSF). Histone H1 has neuro-stimulatory effects and activates the innate immune response in the CNS mediated by microglial cells (Gilthorpe et al., 2013). This promotes neural cell survival, up-regulates major histocompatibility complex (MHC) class II antigen expression and is a powerful microglial chemoattractant. Release of histone H1 from the degenerative CNS drives a positive immune response (Gilthorpe et al., 2013) but can also be cytotoxic. Plasma immune tolerance induction (ITI) neutralizes the cytotoxic effects of histone H1, decreasing histone-induced platelet aggregation (Chaaban et al., 2015) through complexation of the histone with the negatively charged CS GAG chains of ITI (Chaaban et al., 2015). Hypoxic-ischemic encephalopathy predisposes infants to long-term cognitive decline impacting on life quality and healthcare resources (Chen et al., 2019). ITI regulates neonatal inflammation, decreases damage to brain tissues (Chen et al., 2019) and neuronal cell death, attenuates glial responses and leucocyte invasion with long-term beneficial effects in neonatal models of brain injury (Koehn et al., 2020).
Serglycin
Serglycin (Figure 3H) is a small intracellular PG present in secretory granules of hemopoietic and endothelial cells (Kolset and Tveit, 2008) with regulatory properties over immune cells (Kolset and Pejler, 2011). It also promotes the development and aggressiveness of many tumor types including glioblastoma and is a glioblastoma biomarker (Roy et al., 2017; Manou et al., 2020). Suppression of serglycin in LN-18 shSRGN mutant cells results in retarded glioma proliferation, migration and invasive potential (Manou et al., 2020). Serglycin expression is elevated in astrocyte-glioma co-cultures. Astrocytes promote glioblastoma growth and is a potential glioma therapeutic target (Mega et al., 2020).
Endocan
Endocan (Figures 3L, 5C) is a small endothelial cell surface DS-PG found in cerebral blood vessels and is a small circulating PG in the blood stream (Frahm et al., 2013). Human umbilical vein endothelial cells (HUVECs) produce a truncated, alternatively spliced form of endocan which is neither glycosylated or secreted (Tsai et al., 2002). Circulating PGs are relatively rare; examples include endocan, bikunin, and macrophage colony stimulating factor-1 (Aitkenhead et al., 2002; Zhao et al., 2004). Endocan shares no homologies with other ECM PGs (De Freitas and Lassalle, 2015), does not contain LRRs or C-type lectin domains. Endocan, endothelial cell specific molecule-1 (ESM-1) encoded by the ESM-1 gene is an atypical DS-PG, with a single DS chain and distinctive structural and functional properties (Xing et al., 2016; Sun et al., 2019). Endocan is expressed by endothelial cells, regulated by proinflammatory pro-angiogenic molecules, has matrix-binding properties and is a marker of endothelial cell activation. TNF-α, IL-1, TGF-β1, FGF-2, and VEGF-2 induce endocan expression in vitro, IFN-γ inhibits TNF-α induced upregulation of endocan (Scherpereel et al., 2003). Endocan is associated with neuroinflamation in highly vascularized tumors in meningiomas, gliomas and lung cancer (Maurage et al., 2009) and with new blood vessel development in glioma (Maurage et al., 2009), pituitary adenoma, renal cell carcinoma, pediatric brain injury (Lele et al., 2019) and is a biomarker of cerebral damage (Morleo et al., 2019). Endocan expression is upregulated in human cytomegaloviral infection which increases glioma development in brain tissues (Scherpereel et al., 2003; Xing et al., 2016) leading to its suggestion as a therapeutic target in glioma (Atukeren et al., 2016). Endocan binds to lymphocytes and monocytes through high affinity interactions with integrin CD11a/CD18 lymphocyte function associated antigen-1 (LFA-1). A protease cleaved form of endocan (p14) antagonizes these interactions (Gaudet et al., 2020). Endocan promotes adhesion of monocytes and endothelial cells (Sun et al., 2019). The DS chains of endocan bind and activate hepatocyte growth factor (HGF) in vitro (Lyon et al., 1998; Mythreye and Blobe, 2009), L- and P-Selectins, fibronectin, chemokines, cytokines, RANTES, Stromal Cell-Derived Factor-1β (SDF-1β), IL-8, monocyte chemoattractant protein-1 (MCP-1), IFN-γ, and platelet factor-4 (PF-4), midkine, pleiotrophin, FGF-2, and FGF-7 (Sarrazin et al., 2006).
Testican
Testican-1 and -2 are CS/HS PGs (Figure 3K), of the BM-40/SPARC/osteonectin family of extracellular calcium-binding proteins consisting of a signal peptide, a follistatin-like domain, a central Ca2+-binding domain, a thyroglobulin-like domain, and a C-terminal GAG attachment region. Testican-1 and 2 are expressed by multiple neuronal cell types in olfactory bulb, cerebral cortex, thalamus, hippocampus, cerebellum, and medulla (Marr et al., 2000). Neuronal testican-1 is upregulated following brain trauma (Iseki et al., 2011) and is also expressed by activated astrocytes. Testican-1 modulates neuronal attachment and MMP activation (Bocock et al., 2003; Edgell et al., 2004), inhibits membrane type MMPs and cathepsin-L but not cathepsin-B. Testican-1 contains a single thyropin domain highly homologous to domains in cysteine proteinase inhibitors, the CS chains of testican-1 are essential for inhibition of cathepsin-L. Testican-2 is a HS/CS PG, has GAG-substituted and GAG-free forms that inhibit neurite extension, regulating neuronal growth and development (Schnepp et al., 2005). Testican-2 abrogates the inhibition of metallothionein-1(MT1)-MMP- or MT3-MMP-mediated pro-MMP-2 activation by testican-1 (Nakada et al., 2003). Testican-3, a HS-PG exclusive to brain tissues suppresses MT1-MMP mediated activation of MMP-2 and tumor invasion (Hartmann et al., 2013).
Thrombomodulin (CD141)
Thrombomodulin (Figure 4H) inhibits thrombin as part of the anticoagulant protein C-system in the endothelium, is anti-inflammatory and promotes barrier-stabilization. Thrombomodulin is a protective factor in the brain during ischemic stroke, enhancing vessel post-ischemic recovery in the blood brain barrier. Thrombin’s physiological roles in the brain stabilize normal brain function in synaptic transmission and plasticity through direct or indirect activation of Protease-Activated Receptor-1 (PAR1) and has neuroprotective roles in neurological diseases (Krenzlin et al., 2016).
Cluster of Differentiation 44
Cluster of differentiation 44 (CD44), a major transmembrane glycoprotein HA receptor (Figure 4K) in the CNS/PNS, has roles in cell division, migration, adhesion, and cell–cell and cell–ECM signaling (Dzwonek and Wilczynski, 2015). Alternatively spliced CD44v3 is a CS-PG, 20 isoforms of CD44 have been reported associated with several kinds of tumors. CD44 expression is highly dynamic and transitions between different isoforms during tumor development (Lah et al., 2020). In glioma, CD44 and integrins attach the cell to ECM forming focal adhesion complexes and generate traction forces that facilitate cell spreading, essential in the cell migratory machinery in glioma cell invasion (Mooney et al., 2016). In the normal brain, CD44 is a major HA receptor that interacts with osteopontin, collagens, and MMPs stabilisating and remodeling the CNS/PNS ECM (Dzwonek and Wilczynski, 2015). HA is highly interactive through CD44, conveying cell instructional cues, and ECM stabilization, hydration and space-filling properties thus maintaining tissue compartmentalisation, ionic gradients and niches important in the metabolism of neural cell populations (Sherman et al., 2015; Peters and Sherman, 2020), including neural progenitor stem cell niches (Preston and Sherman, 2011).
Cluster of Differentiation 47
Cluster of differentiation 47 (CD47) (Figure 5A), originally named integrin-associated protein (IAP) is a receptor for thrombospondin-1 (TSP-1) regulates cellular migration, proliferation, and the survival of vascular cells, in innate and adaptive immune regulation (Barclay and Van den Berg, 2014; Murata et al., 2014). TSP-1 acts via CD47 to inhibit NO signaling in the vascular system supporting blood pressure by regulation limiting endothelial nitric oxide synthase (eNOS) activation and endothelial-dependent vasorelaxation. CD47 is a ligand for signal regulatory protein α (SIRPα), also known as SHPS-1/BIT/CD172a). The CD47-SIRPα signaling system is a cell-cell communication system (Zhang H. et al., 2015; Matlung et al., 2017; Weiskopf, 2017). CD47-SIRPα interactions have been termed an innate immune checkpoint in macrophages (Li et al., 2021). Blockade of anti-phagocytic CD47-SIRPα interactions using humanized antibodies to CD47 (Hu5F9-G4) has yielded promising results in preclinical studies of a number of human malignancies including pediatric brain tumors: medulloblastoma, atypical teratoid rhabdoid tumors, primitive neuroectodermal tumor, pediatric glioblastoma, and diffuse intrinsic pontine glioma (Gholamin et al., 2017) and accelerates the clearance of hematomas in experimental intraventricular hemorrhage (Ye et al., 2021). Thus by targeting the immunological checkpoint complex CD47-SIRPα, the development of glioblastoma can be inhibited, the function of phagocytic, dendritic and T-lymphocytes enhanced and the efficiency of tumor cell removal improved by innate and adaptive immune responses (Hutter et al., 2019; Hu et al., 2020; Kuo et al., 2020; Zhang et al., 2020).
Astrochondrin
Some specialized CNS proteins such as astrochondrin, a cell surface CS-PG of astrocytes carry L2/HNK-1 and L5 carbohydrate structures interactive with ECM components such as laminin and type IV collagen this may facilitate interaction of astrocyte foot processes with the brain microvasculature and meningeal membranes (Streit et al., 1993).
Photomedin
Photomedin is another brain-specific glycoprotein of the eye neuroepithelium that interacts with CS-E (Furutani et al., 2005). Photomedin is a member of the olfactomedin protein family and has regulatory roles in axonal growth and differentiation of sensory cilia in the neural epithelium.
FORSE-1 (Forebrain-Surface-Embryonic) Proteoglycan
FORSE-1 contains LeX-carbohydrate, stage specific embryonic antigen-1 (SSEA-1) or CD15, terminal Galβ(1-4)Fucα(1-3)GlcNAc-R oligosaccharide epitopes with roles in embryonic neural development (Allendoerfer et al., 1995, 1999; Kelly et al., 2019).
CS-PGs Regulation of Neuronal Cell Signaling
Neural cell populations including astrocytes, oligodendrocytes, neurons, endothelial cells and pericytes of the brain microvasculature and microglial cells synthesize a range of CS-PGs that interact with a variety of cell surface molecules and receptors (Figure 6). These modulate cellular processes that control CNS/PNS function and repair (Djerbal et al., 2017; Figure 7). CS-PGs contribute to the structural integrity and compartmentalisation of the brain ECM and have important organizational functional roles in brain regions (Dityatev et al., 2010). CS-PGs operate at multiple functional levels involving interactions with growth factors, receptors, adhesion molecules, neural guidance proteins and ECM proteins (Djerbal et al., 2017). Transmembrane CS-PGs are active during cell-cell crosstalk, they may also be secreted or released from the cell surface by proteases to act remotely from their cells of origin and may antagonize normal transmembrane PG interactions (Figure 7F). CS-PGs are not uniformly distributed in the CNS/PNS but occur concentrated in neural growth cones and PNNs strategically positioned to control processes occurring at the cell-tissue interface (Shimbo et al., 2013; Sugitani et al., 2020). Growth cone receptor protein tyrosine phosphatases (RPTPs) bind with high affinity to CS-PGs, this controls axonal growth and provides guidance cues during regeneration, plasticity and neuronal development and in repair responses (Djerbal et al., 2017). CS-PGs attached to RPTP members can also exert repulsive guidance cues and inhibit neuritogenesis (Figure 6A). Lectican PG family members (neurocan, brevican, versican, aggrecan) are diffusely distributed in the CNS ECM and are also components of the denser PNNs. The CS component of CS-PGs vary in composition with tissue development. During embryonic development CS-C is a predominant isoform while CS-A is more abundant in adult neural tissues (Figure 2). The sulphation patterns and charge density are important functional determinants of CS glycoforms. The more highly charged CS-E, B and D are components of PNN PGs, CS-A and CS-C are diffusely distributed in the ECM distant from PNN structures (Djerbal et al., 2017). The chemo-repellent semaphorin 3A (SEMA3A), a component of PNNs (Battistini and Tamagnone, 2016; Fard and Tamagnone, 2020), interacts with CS-E and B but not CS-D, thus such interactions are not purely mediated by charge; saccharide sequences in CS chains also determine the interactive properties of CS-PGs and their core proteins also contain ECM interactive modules. Selective binding of midkine (MK) and brain-derived neurotrophic factor (BDNF) to CS-E of some CS-PGs leads to neurite outgrowth. The Nogo receptors NgR1 and NgR3 bind to Nogo and inhibit neurite outgrowth. NgR1 and NgR3 also bind specifically to CS-B, CS-D, and CS-E with high affinity, and this inhibits neurite outgrowth. Cell adhesion molecules are operative in cell–cell and cell–ECM interactions that regulate tissue integrity, cellular communication and cellular migration during CNS development and repair following trauma and are evident as pathological functional changes in neurological disorders. Neural cell adhesion molecule (NCAM) in particular, has specific roles in the promotion of neurite outgrowth of motor neurons that improves locomotor functional recovery following SCI. NCAM and neuroglia cell adhesion molecule (NgCAM) bind with high affinity to the CS-PG phosphacan, reducing neurite outgrowth and adhesion. Chondroitinase ABC moderately reduces phosphacan-NCAM binding showing this interaction is mainly mediated through phosphacan core protein interactions (Figure 7). Neurocan binding to NCAM and NgCAM also inhibits neurite outgrowth but unlike phosphacan, chondroitinase ABC abrogates this, showing that neurocan-NCAM interactions are mediated through CS. NCAM and NgCAM act as receptors for phosphacan and neurocan. Contactin-1 is a further GPI anchored cell adhesion molecule (CAM) that facilitates axonal growth and dendritic interactions that promote neurogenesis, CS-E binds contactin-1 with significant affinity and promotes neural growth. Thus, CS-PG binding to contactin-1 can modulate contactin-1 interactions that normally mediate cell-cell interaction (Figure 7F). CS-PG5 (neuroglycan C) forms peri-synaptic matrix assemblies that regulate neuronal synaptic activity in the cerebral cortex of rats (Pintér et al., 2020).
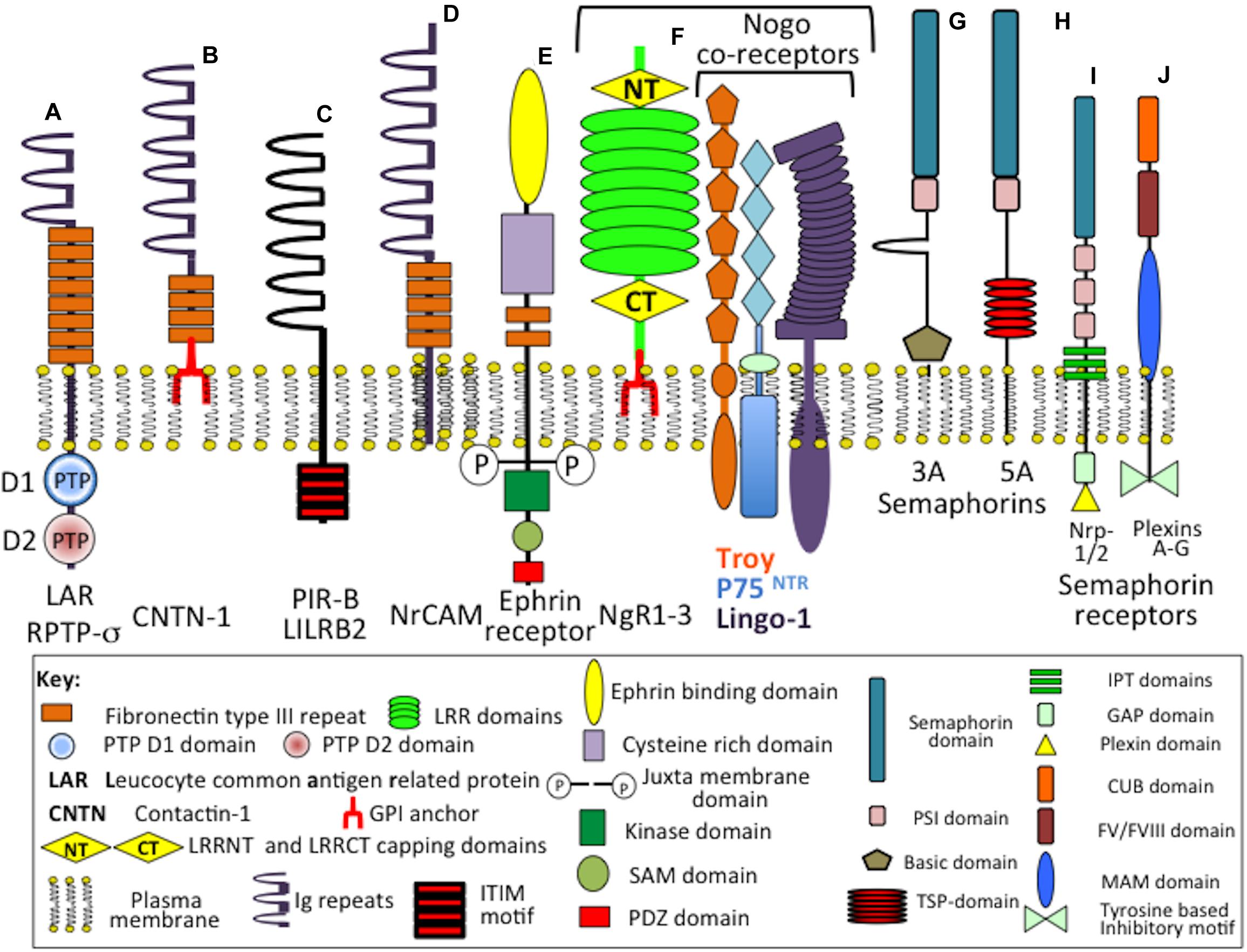
Figure 6. Schematic depiction of the modular structures of diverse cell surface molecules expressed by neural cells that bind CS-proteoglycans. The fibronectin-III and Ig repeat transmembrane protein LAR (Leukocyte common antigen-related receptor protein tyrosine phosphatase (A) and homologous contactin-1 (CNTN-1) (B) Murine paired immunoglobulin receptor B (PirB) and its human ortholog leukocyte immunoglobulin-like receptor B2 (LILRB2) (C), neural cell adhesion molecule (NrCAM) (D). The ephrin receptor (E), Nogo and its co-receptors (F), semaphorins 3A (G), 5A (H) and the neuropilin (I) and neuroplexin receptors (J).
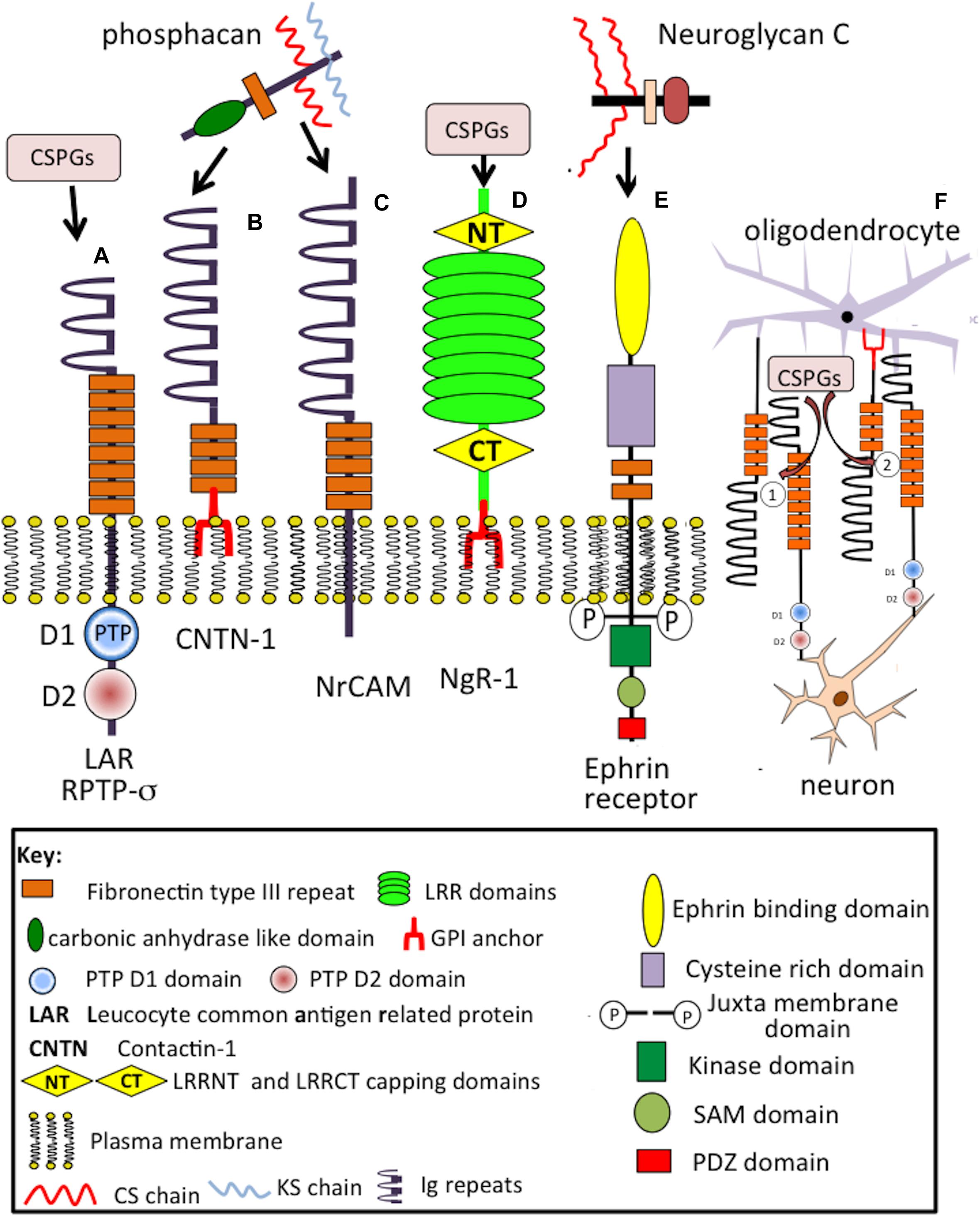
Figure 7. Schematic representation of the structural organization of neural cell adhesion molecules that act as CS-PG receptors (A–C), and the neural NgR1 (D) and ephrin receptors (E) where binding of CS-PGs results in an inhibitory signal to the neuron. Ephrin acts as a receptor for Neuroglycan-C, while phosphacan binds to Contactin-1 (B) and NrCAM (C). LAR acts as a receptor for several CS-PGs. Oligodendrocyte-neuronal communication mediated by LAR-NrCAM (Condomitti and de Wit, 2018) or LAR-contactin-1 interactions (Zhang P. et al., 2018) can be regulated by competitive binding of CS-PGs to these cell surface components (F).
The semaphorins are a family of guidance proteins of embryonic peripheral nerve projections and have roles in synaptogenesis and the maintenance of neural interconnections in adulthood thus maintaining cerebral homeostasis. SEMA3A is upregulated following CNS injury, causes growth cone collapse by signaling through synaptic neuropilin-1 (Nrp-1) and plexin receptors and is also known as collapsin-1. SEMA3A in PNNs can modulate synaptic morphology and function (Goshima et al., 2002; Mecollari et al., 2014; Battistini and Tamagnone, 2016; Alto and Terman, 2017; Fard and Tamagnone, 2020). SEMA3A interacts with CS-E with high affinity and neuropilin-1 (Nrp-1) in SEMA3A-Nrp-plexin signaling complexes to potently inhibit neural sprouting following SCI, and also inhibits neural growth factor (NGF) (Mecollari et al., 2014; Fard and Tamagnone, 2020) (Figure 6). Compared to SEMA3A, SEMA5A is less well studied, but also has important functional roles in CNS development and response to injury (Conrad et al., 2010). SEMA5A contains a cluster of thrombospondin (TSP) repeats which promote neural outgrowth. CS-PGs interact with this TSP repeat region producing a neuro-repulsive response whereas HS-PGs produce a neuro-attractive response. Thus, CS has important SEMA5A regulatory properties. A proteomic surface plasmon resonance and microarray study by Conrad et al. (2010), showed that interactions with neurotrophic factors was not confined to the highly charged CS-E glycoforms and that significant interactions also occurred between CS-A and Sema 3E, Sema 6B and ephrin A3 (Conrad et al., 2010).
Conclusion
This chapter has shown the impressive diversity in CS-PG form and function in the CNS/PNS. CS-PGs undertake many essential roles in neural tissues through the provision of a functional ECM for the many different cell populations resident in the CNS/PNS. The intricacies of some of the cell regulatory properties conveyed by CS-PGs have been illustrated, as has the complex interplay between distinct neural cell populations in the maintenance of CNS/PNS tissue function and homeostasis. Aberrations in the assembly of the ECM through processing defects in component CS-PGs can have serious functional consequences in brain tissues and can lead to neurodegenerative diseases. Such defects underline the fundamental importance of the ECM in normal tissue function and the potential of CS-PGs as promising therapeutic targets for future treatment of many of these neurodegenerative conditions.
Author Contributions
JM obtained the funding, conceptualized the study, and wrote the initial draft of the manuscript. AH assisted in manuscript writing and editing and had intellectual input into data interpretation. Both authors approved the final version of the manuscript.
Funding
This study was supported by the NHMRC Project Grant 512167.
Conflict of Interest
The authors declare that the research was conducted in the absence of any commercial or financial relationships that could be construed as a potential conflict of interest.
Publisher’s Note
All claims expressed in this article are solely those of the authors and do not necessarily represent those of their affiliated organizations, or those of the publisher, the editors and the reviewers. Any product that may be evaluated in this article, or claim that may be made by its manufacturer, is not guaranteed or endorsed by the publisher.
Glossary
AD, Alzheimer’s disease; ADAM, A disintegrin and metalloproteinase domain-containing protein; ADAMTSL, A disintegrin and metalloproteinase with thrombospondin motifs; AMPK, 5′ adenosine monophosphate-activated protein kinase; APLP2, amyloid precursor-like protein 2; APP, amyloid-beta precursor protein; BBB, blood brain barrier; BDNF, brain-derived neurotrophic factor; BEHAB, brain-enriched hyaluronan-binding protein; Bgn, biglycan; BMP, bone morphogenic protein; C4ST-1, chondroitin 4-O-sulfotransferase-1; C4ST-2, Chondroitin 4-O-sulfotransferase-2; CADASIL, cerebral autosomal dominant arteriopathy with subcortical infarcts and leukoencephalopathy; CAM, cell adhesion molecule; CD44, cluster of differentiation 44; CD47, cluster of differentiation 47; Chn, chondroitin; CMD, cartilage matrix deficiency; CNP, 2′,3′-cyclic nucleotide 3′-phosphodiesterase; CNS, central nervous system; CPG, chondroitin proteoglycans; CS, chondroitin sulfate; CSF, cerebrospinal fluid; CS-PG, chondroitin sulfate proteoglycan; D/iD, GlcA/IdoA(2-O-sulfate)-GalNAc(6-O-sulfate); D4ST-1, dermatan 4-O-sulfotransferase-1; DAMP, damage-associated molecular pattern; DCC, deleted in colorectal carcinoma; DDBJ, DNA Data Bank of Japan; Dlp, Dally-like protein; Dome, domeless; Dpp, decapentaplegic, a ligand of the TGFβ superfamily; DRG, dorsal root ganglion/ganglia; DS, dermatan sulfate; DS-PG, dermatan sulfate proteoglycan; E/iE, GlcA/IdoA-GalNAc (4,6-O-disulfate); ECM, extracellular matrix; EGF(R), epidermal growth factor (Receptor); eNOS, endothelial nitric oxide synthase; ESM-1, endothelial cell specific molecule-1; FGF, fibroblast growth factor; FORSE-1, forebrain surface embryonic-1; FSH, follicle-stimulating hormone; GPI, glycophosphatidylinositol; HA, hyaluronic acid; HAPLN2, hyaluronan and proteoglycan link protein 2; HB-GAM, heparin-binding growth-associated molecule; hEGFR, human epidermal growth factor receptor; HGF, hepatocyte growth factor; Hh, Hedgehog; HNK-1, human natural killer-1; HS, heparan sulfate; HS-PG, heparan sulfate proteoglycan; HSPG2, heparan sulfate proteoglycan 2 (perlecan); Hst, heparan sulfate sulfotransferase; HUVECs, human umbilical vein endothelial cells; iA, 4-sulfated IdoA-GalNAc; IAP, integrin-associated protein; iB, 2-sulfated IdoA-GalNAc; IFN-γ, interferon-γ; IGF-IR, insulin-like growth factor-1 receptor; IL, interleukin; ISH, in situ hybridization; ITI, immune tolerance induction; JAK/STAT, Janus kinase/signal transducer and activator of transcription; KO, knockout; Kon, Kon-tiki (NG2 homolog); KS, keratan sulfate; LFA-1, lymphocyte function associated antigen-1; LRR, leucine-rich repeat; MADD, muscle arm development defective; MAP, mitogen-activated protein; MCAF, monocyte chemotactic and activating factor; MCP-1, monocyte chemoattractant protein-1; MHC, major histocompatibility complex; MIP, macrophage inflammatory protein; MK, midkine; MMP, matrix metalloproteinase; MSC, mesenchymal stem cell; MT-1, metallothionein-1; mTOR, mechanistic target of rapamycin; NCAM, neural cell adhesion molecule; NF-κB, nuclear factor Kappa-light-chain-enhancer of activated B cells; NG2, neuron-glial antigen 2; NgCAM, neuroglia cell adhesion molecule; NGF, neural growth factor; NgR2, Nogo-66 Receptor Homolog2; NLG, neuroligin; NMJ, neuromuscular junction; NO, nitric oxide; Nrp-1, neuropilin 1; OA, osteoarthritis; OPC, oligodendrocyte precursor cells; PAP, 3′-phosphoadenosine 5′-phosphosulfate; PAPS, 3′-phosphoadenosine 5′-phosphosulfate; PAR1, protease-activated receptor-1, PG, proteoglycan; PNN, perineuronal net; PNS, peripheral nervous system; Pros, prospero; RANTES, regulated upon activation, normal T cell expressed and secreted; RNAi, ribonucleic acid interference; ROS, reactive oxygen species; RPTPs, receptor protein tyrosine phosphatases; RPTP-ζ, receptor protein tyrosine phosphatase-ζ; SCI, spinal cord injury; Sdc, syndecan; SDF-1β, stromal cell-derived factor-1β; SDN-1, syndecan homolog found in Caenorhabditis elegans; SEMA3A, semaphorin 3A; SEMA5A, semaphorin 5A; SIRPα, signal regulatory protein α; SMAD, Sma and MAD-related protein; SOX9, SRY-box transcription factor 9; Sqv, squashed vulva gene; SSEA-1, stage specific embryonic antigen-1; SVZ, subventricular zone; TBI, traumatic brain injury; TGF-β, transforming growth factor-β; TM, transmembrane; TNFR, tumor necrosis factor receptor; TNF-α, tumor necrosis factor-α; TSP-1, thrombospondin-1; UDP, uridine diphosphate; UNC, uncoordinated; UST, uronyl 2-sulfotransferase; VEGF, vascular endothelial growth factor; Wdp, windpipe; Wg, wingless.
Footnotes
- ^ http://flybase.bio.indiana.edu
- ^ www.ncbi.nlm.nih.gov
- ^ www.ebi.ac.uk/embl/index.html
- ^ www.ddbj.nig.ac.jp
References
Abbott, L., and Nigussie, F. (2020). Adult neurogenesis in the mammalian dentate gyrus. Anat. Histol. Embryol. 49, 3–16. doi: 10.1111/ahe.12496
Adamsky, K., Schilling, J., Garwood, J., Faissner, A., and Peles, E. (2001). Glial tumor cell adhesion is mediated by binding of the FNIII domain of receptor protein tyrosine phosphatase beta (RPTPbeta) to tenascin C. Oncogene 20, 609–618. doi: 10.1038/sj.onc.1204119
Aguirre, A., Dupree, J. L., Mangin, J. M., and Gallo, V. (2007). A functional role for EGFR signaling in myelination and remyelination. Nat. Neurosci. 10, 990–1002. doi: 10.1038/nn1938
Aitkenhead, M., Wang, S. J., Nakatsu, M. N., Mestas, J., Heard, C., and Hughes, C. C. (2002). Identification of endothelial cell genes expressed in an in vitro model of angiogenesis: induction of ESM-1, (beta)ig-h3, and NrCAM. Microvasc. Res. 63, 159–171. doi: 10.1006/mvre.2001.2380
Akatsu, C., Mizumoto, S., Kaneiwa, T., Maccarana, M., Malmström, A., Yamada, S., et al. (2011). Dermatan sulfate epimerase 2 is the predominant isozyme in the formation of the chondroitin sulfate/dermatan sulfate hybrid structure in postnatal developing mouse brain. Glycobiology 21, 565–574. doi: 10.1093/glycob/cwq208
Akita, K., Toda, M., Hosoki, Y., Inoue, M., Fushiki, S., Oohira, A., et al. (2004). Heparan sulphate proteoglycans interact with neurocan and promote neurite outgrowth from cerebellar granule cells. Biochem. J. 383, 129–138. doi: 10.1042/bj20040585
Allendoerfer, K., Durairaj, A., Matthews, G. A., and Patterson, P. H. (1999). Morphological domains of Lewis-X/FORSE-1 immunolabeling in the embryonic neural tube are due to developmental regulation of cell surface carbohydrate expression. Dev. Biol. 211, 208–219. doi: 10.1006/dbio.1999.9308
Allendoerfer, K., Magnani, J. L., and Patterson, P. H. (1995). FORSE-1, an antibody that labels regionally restricted subpopulations of progenitor cells in the embryonic central nervous system, recognizes the Le(x) carbohydrate on a proteoglycan and two glycolipid antigens. Mol. Cell. Neurosci. 6, 361–395.
Alto, L., and Terman, J. R. (2017). Semaphorins and their signaling mechanisms. Methods Mol. Biol. 1493, 1–25. doi: 10.1007/978-0-387-70956-7_1
Alzheimer, C., and Werner, S. (2002). Fibroblast growth factors and neuroprotection. Adv. Exp. Med. Biol. 513, 335–351. doi: 10.1007/978-1-4615-0123-7_12
Amenta, A., Creely, H. E., Mercado, M. L., Hagiwara, H., McKechnie, B. A., Lechner, B. E., et al. (2012). Biglycan is an extracellular MuSK binding protein important for synapse stability. J. Neurosci. 32, 2324–2334. doi: 10.1523/jneurosci.4610-11.2012
Amran, A., Pigatto, L., Pocock, R., and Gopal, S. (2021). Functions of the extracellular matrix in development: lessons from Caenorhabditis elegans. Cell. Signal. 84:110006. doi: 10.1016/j.cellsig.2021.110006
Anderson, M., Burda, J. E., Ren, Y., Ao, Y., O’Shea, T. M., Kawaguchi, R., et al. (2016). Astrocyte scar formation aids central nervous system axon regeneration. Nature 532, 195–200. doi: 10.1038/nature17623
Atukeren, P., Kunbaz, A., Turk, O., Kemerdere, R., Ulu, M. O., Turkmen Inanir, N., et al. (2016). Expressions of endocan in patients with meningiomas and gliomas. Dis. Markers 2016:7157039.
Barclay, A., and Van den Berg, T. K. (2014). The interaction between signal regulatory protein alpha (SIRPα) and CD47: structure, function, and therapeutic target. Annu. Rev. Immunol. 32, 25–50. doi: 10.1146/annurev-immunol-032713-120142
Battista, D., Ferrari, C. C., Gage, F. H., and Pitossi, F. J. (2006). Neurogenic niche modulation by activated microglia: transforming growth factor beta increases neurogenesis in the adult dentate gyrus. Eur. J. Neurosci. 23, 83–93. doi: 10.1111/j.1460-9568.2005.04539.x
Battistini, C., and Tamagnone, I. (2016). Transmembrane semaphorins, forward and reverse signaling: have a look both ways. Cell. Mol. Life Sci. 73, 1609–1622. doi: 10.1007/s00018-016-2137-x
Bäumer, B., Kurz, A., Borrie, S. C., Sickinger, S., Dours-Zimmermann, M. T., Zimmermann, D. R., et al. (2014). Nogo receptor homolog NgR2 expressed in sensory DRG neurons controls epidermal innervation by interaction with Versican. J. Neurosci. 34, 1633–1646. doi: 10.1523/jneurosci.3094-13.2014
Bedoui, Y., Neal, J. W., and Gasque, P. (2018). The neuro-immune-regulators (NIREGs) promote tissue resilience; a vital component of the host’s defense strategy against neuroinflammation. J. Neuroimmune Pharmacol. 13, 309–329. doi: 10.1007/s11481-018-9793-6
Bekku, Y., and Oohashi, T. (2019). Under the ECM dome: the physiological role of the perinodal extracellular matrix as an ion diffusion barrier. Adv. Exp. Med. Biol. 1190, 107–122. doi: 10.1007/978-981-32-9636-7_8
Bekku, Y., Rauch, U., Ninomiya, Y., and Oohashi, T. (2009). Brevican distinctively assembles extracellular components at the large diameter nodes of Ranvier in the CNS. J. Neurochem. 108, 1266–1276. doi: 10.1111/j.1471-4159.2009.05873.x
Bellono, N. W., Leitch, D. B., and Julius, D. (2017). Molecular basis of ancestral vertebrate electroreception. Nature 543, 391–396. doi: 10.1038/nature21401
Bernard, D., Smith, C. L., and Brûlé, E. (2020). A tale of two proteins: betaglycan, IGSF1, and the continuing search for the inhibin B receptor. Trends Endocrinol. Metab. 31, 37–45. doi: 10.1016/j.tem.2019.08.014
Berretta, S. (2012). Extracellular matrix abnormalities in schizophrenia. Neuropharmacology 62, 1584–1597. doi: 10.1016/j.neuropharm.2011.08.010
Bhattacharya, A., Aghayeva, U., Berghoff, E. G., and Hobert, O. (2019). Plasticity of the electrical connectome of C. elegans. Cell 176, 1174–1189.e16.
Bignami, A., Hosley, M., and Dahl, D. (1993). Hyaluronic acid and hyaluronic acid-binding proteins in brain extracellular matrix. Anat. Embryol. (Berl.) 188, 419–433.
Bilandzic, M., and Stenvers, K. L. (2011). Betaglycan: a multifunctional accessory. Mol. Cell. Endocrinol. 339, 180–189. doi: 10.1016/j.mce.2011.04.014
Birch, J., Coull, B. J., Spender, L. C., Watt, C., Willison, A., Syed, N., et al. (2020). Multifaceted transforming growth factor-beta (TGFβ) signalling in glioblastoma. Cell. Signal. 72:109638. doi: 10.1016/j.cellsig.2020.109638
Blanchette, C., Perrat, P. N., Thackeray, A., and Bénard, C. Y. (2015). Glypican is a modulator of netrin-mediated axon guidance. PLoS Biol. 13:e1002183. doi: 10.1371/journal.pbio.1002183
Blanchette, C., Thackeray, A., Perrat, P. N., Hekimi, S., and Bénard, C. Y. (2017). Functional requirements for heparan sulfate biosynthesis in morphogenesis and nervous system development in C. elegans. PLoS Genet. 13:e1006525. doi: 10.1371/journal.pgen.1006525
Bocock, J., Edgell, C. J., Marr, H. S., and Erickson, A. H. (2003). Human proteoglycan testican-1 inhibits the lysosomal cysteine protease cathepsin L. Eur. J. Biochem. 270, 4008–4015. doi: 10.1046/j.1432-1033.2003.03789.x
Bovolenta, P., and Fernaud-Espinosa, I. (2000). Nervous system proteoglycans as modulators of neurite outgrowth. Prog. Neurobiol. 61, 113–132. doi: 10.1016/s0301-0082(99)00044-1
Boyd, F., Cheifetz, S., Andres, J., Laiho, M., and Massagué, J. (1990). Transforming growth factor-beta receptors and binding proteoglycans. J. Cell Sci. 13, 131–138. doi: 10.1242/jcs.1990.supplement_13.12
Bradbury, E. J., and Carter, L. M. (2011). Manipulating the glial scar: chondroitinase ABC as a therapy for spinal cord injury. Brain Res. Bull. 84, 306–316. doi: 10.1016/j.brainresbull.2010.06.015
Bruckner, G. H. D., Härtig, W., Drlicek, M., Arendt, T., and Brauer, K. (1999). Cortical areas abundant in extracellular matrix chondroitin sulphate proteoglycans are less affected by cytoskeletal changes in Alzheimer’s disease. Neuroscience 92, 791–805. doi: 10.1016/s0306-4522(99)00071-8
Bulik, D., Wei, G., Toyoda, H., Kinoshita-Toyoda, A., Waldrip, W. R., Esko, J. D., et al. (2000). sqv-3, -7, and -8, a set of genes affecting morphogenesis in Caenorhabditis elegans, encode enzymes required for glycosaminoglycan biosynthesis. Proc. Natl. Acad. Sci. U.S.A. 97, 10838–10843. doi: 10.1073/pnas.97.20.10838
Bülow, H., and Hobert, O. (2004). Differential sulfations and epimerization define heparan sulfate specificity in nervous system development. Neuron 41, 723–736. doi: 10.1016/s0896-6273(04)00084-4
Butt, A., Papanikolaou, M., and Rivera, A. (2019). Physiology of oligodendroglia. Adv. Exp. Med. Biol. 1175, 117–128. doi: 10.1007/978-981-13-9913-8_5
Carulli, D., and Verhaagen, J. (2021). An extracellular perspective on CNS maturation: perineuronal nets and the control of plasticity. Int. J. Mol. Sci. 22:2434. doi: 10.3390/ijms22052434
Cassada, R., and Russell, R. L. (1975). The dauerlarva, a post-embryonic developmental variant of the nematode Caenorhabditis elegans. Dev. Biol. 46, 326–342. doi: 10.1016/0012-1606(75)90109-8
Celestrin, K., Díaz-Balzac, C. A., Tang, L. T. H., Ackley, B. D., and Bülow, H. E. (2018). Four specific immunoglobulin domains in UNC-52/Perlecan function with NID-1/Nidogen during dendrite morphogenesis in Caenorhabditis elegans. Development 145:dev158881.
Chaaban, H., Keshari, R. S., Silasi-Mansat, R., Popescu, N. I., Mehta-D’Souza, P., Lim, Y. P., et al. (2015). Inter-α inhibitor protein and its associated glycosaminoglycans protect against histone-induced injury. Blood 125, 2286–2296. doi: 10.1182/blood-2014-06-582759
Chanana, B., Steigemann, P., Jäckle, H., and Vorbrüggen, G. (2009). Reception of Slit requires only the chondroitin-sulphate-modified extracellular domain of Syndecan at the target cell surface. Proc. Natl. Acad. Sci. U.S.A. 106, 11984–11988. doi: 10.1073/pnas.0901148106
Chelyshev, Y. A., Kabdesh, I. M., and Mukhamedshina, Y. O. (2020). Extracellular matrix in neural plasticity and regeneration. Cell. Mol. Neurobiol. doi: 10.1007/s10571-020-00986-0
Chen, S., Guo, D., Lei, B., Bi, J., and Yang, H. (2020). Biglycan protects human neuroblastoma cells from nitric oxide-induced death by inhibiting AMPK-mTOR mediated autophagy and intracellular ROS level. Biotechnol. Lett. 42, 657–668. doi: 10.1007/s10529-020-02818-z
Chen, S., Guo, D., Zhang, W., Xie, Y., Yang, H., Cheng, B., et al. (2018). Biglycan, a nitric oxide-downregulated proteoglycan, prevents nitric oxide-induced neuronal cell apoptosis via targeting Erk1/2 and p38 signaling pathways. J. Mol. Neurosci. 66, 68–76. doi: 10.1007/s12031-018-1151-x
Chen, X., Nakada, S., Donahue, J. E., Chen, R. H., Tucker, R., Qiu, J., et al. (2019). Neuroprotective effects of inter-alpha inhibitor proteins after hypoxic-ischemic brain injury in neonatal rats. Exp. Neurol. 317, 244–259. doi: 10.1016/j.expneurol.2019.03.013
Chen, X., Rivard, L., Naqvi, S., Nakada, S., Padbury, J. F., Sanchez-Esteban, J., et al. (2016). Expression and localization of Inter-alpha Inhibitors in rodent brain. Neuroscience 324, 69–81. doi: 10.1016/j.neuroscience.2016.03.009
Cheng, C., Lin, C. T., Lee, M. J., Tsai, M. J., Huang, W. H., Huang, M. C., et al. (2015). Local delivery of high-dose chondroitinase ABC in the sub-acute stage promotes axonal outgrowth and functional recovery after complete spinal cord transection. PLoS One 10:e0138705. doi: 10.1371/journal.pone.0138705
Chisholm, A., and Jin, Y. (2005). Neuronal differentiation in C. elegans. Curr. Opin. Cell Biol. 17, 682–689. doi: 10.1016/j.ceb.2005.10.004
Cho, J., Chak, K., Andreone, B. J., Wooley, J. R., and Kolodkin, A. L. (2012). The extracellular matrix proteoglycan perlecan facilitates transmembrane semaphorin-mediated repulsive guidance. Genes Dev. 26, 2222–2235. doi: 10.1101/gad.193136.112
Chow, J., Fujikawa, A., Shimizu, H., Suzuki, R., and Noda, M. (2008). Metalloproteinase- and gamma-secretase-mediated cleavage of protein-tyrosine phosphatase receptor type Z. J. Biol. Chem. 283, 30879–30889. doi: 10.1074/jbc.m802976200
Cizeron, M., Granger, L., Bülow, H. E., and Bessereau, J. L. (2021). Specific heparan sulfate modifications stabilize the synaptic organizer MADD-4/Punctin at C. elegans neuromuscular junctions. Genetics iyab073. doi: 10.1093/genetics/iyab073
Clarke, L., Young, K. M., Hamilton, N. B., Li, H., Richardson, W. D., and Attwell, D. (2012). Properties and fate of oligodendrocyte progenitor cells in the corpus callosum, motor cortex, and piriform cortex of the mouse. J. Neurosci. 32, 8173–8185. doi: 10.1523/jneurosci.0928-12.2012
Condomitti, G., and de Wit, J. (2018). Heparan sulfate proteoglycans as emerging players in synaptic specificity. Front. Mol. Neurosci. 11:14. doi: 10.3389/fnmol.2018.00014
Conrad, A. H., Zhang, Y., Tasheva, E. S., and Conrad, G. W. (2010). Proteomic analysis of potential keratan sulfate, chondroitin sulfate a, and hyaluronic acid molecular interactions. Invest. Ophthalmol. Vis. Sci. 51, 4500–4515. doi: 10.1167/iovs.09-4914
Cope, E., and Gould, E. (2019). Adult neurogenesis, glia, and the extracellular matrix. Cell Stem Cell 24, 690–705. doi: 10.1016/j.stem.2019.03.023
Couchman, J., Gopal, S., Lim, H. C., Nørgaard, S., and Multhaupt, H. A. (2015). Fell-muir lecture: syndecans: from peripheral coreceptors to mainstream regulators of cell behaviour. Int. J. Exp. Pathol. 96, 1–10. doi: 10.1111/iep.12112
Davis, M., Horne-Badovinac, S., and Naba, A. (2019). In-silico definition of the Drosophila melanogaster matrisome. Matrix Biol. Plus 4:100015. doi: 10.1016/j.mbplus.2019.100015
De Luca, C., and Papa, M. (2016). Looking inside the matrix: perineuronal nets in plasticity, maladaptive plasticity and neurological disorders. Neurochem. Res. 41, 1507–1515. doi: 10.1007/s11064-016-1876-2
Dejima, K., Seko, A., Yamashita, K., Gengyo-Ando, K., Mitani, S., Izumikawa, T., et al. (2006). Essential roles of 3′-phosphoadenosine 5′-phosphosulfate synthase in embryonic and larval development of the nematode Caenorhabditis elegans. J. Biol. Chem. 281, 11431–11440. doi: 10.1074/jbc.m601509200
Dempsey, S., Miller, C. H., Schueler, J., Veale, R. W. F., Day, D. J., and May, B. C. H. (2020). A novel chemotactic factor derived from the extracellular matrix protein decorin recruits mesenchymal stromal cells in vitro and in vivo. PLoS One 15:e0235784. doi: 10.1371/journal.pone.0235784
Díaz-Balzac, C., Lázaro-Peña, M. I., Tecle, E., Gomez, N., and Bülow, H. E. (2014). Complex cooperative functions of heparan sulfate proteoglycans shape nervous system development in Caenorhabditis elegans. G3 (Bethesda) 4, 1859–1870. doi: 10.1534/g3.114.012591
Dibrova, D., Chudetsky, M. Y., Galperin, M. Y., Koonin, E. V., and Mulkidjanian, A. Y. (2012). The role of energy in the emergence of biology from chemistry. Orig. Life Evol. Biosph. 42:5.
Dityatev, A., and Schachner, M. (2006). The extracellular matrix and synapses. Cell Tissue Res. 326, 647–654. doi: 10.1007/s00441-006-0217-1
Dityatev, A., Frischknecht, R., and Seidenbecher, C. I. (2006). Extracellular matrix and synaptic functions. Results Probl. Cell Differ. 43, 69–97. doi: 10.1007/400_025
Dityatev, A., Seidenbecher, C. I., and Schachner, M. (2010). Compartmentalization from the outside: the extracellular matrix and functional microdomains in the brain. Trends Neurosci. 33, 503–512. doi: 10.1016/j.tins.2010.08.003
Dityatev, A., Seidenbecher, C., and Morawski, M. (2021). Brain extracellular matrix: an upcoming target in neurological and psychiatric disorders. Eur J Neurosci. 12, 3807–3810. doi: 10.1111/ejn.15336
Djerbal, L., Lortat-Jacob, H., and Kwok, J. (2017). Chondroitin sulfates and their binding molecules in the central nervous system. Glycoconj. J. 34, 363–376. doi: 10.1007/s10719-017-9761-z
Dobbertin, A., Rhodes, K. E., Garwood, J., Properzi, F., Heck, N., Rogers, J. H., et al. (2003). Regulation of RPTPbeta/phosphacan expression and glycosaminoglycan epitopes in injured brain and cytokine-treated glia. Mol. Cell. Neurosci. 24, 951–971. doi: 10.1016/s1044-7431(03)00257-4
Dours-Zimmermann, M., Maurer, K., Rauch, U., Stoffel, W., Fässler, R., and Zimmermann, D. R. (2009). Versican V2 assembles the extracellular matrix surrounding the nodes of ranvier in the CNS. J. Neurosci. 29, 7731–7742. doi: 10.1523/jneurosci.4158-08.2009
Du, W., Yang, B. B., Shatseva, T. A., Yang, B. L., Deng, Z., Shan, S. W., et al. (2010). Versican G3 promotes mouse mammary tumor cell growth, migration, and metastasis by influencing EGF receptor signaling. PLoS One 5:e13828. doi: 10.1371/journal.pone.0013828
Dubey, D., McRae, P. A., Rankin-Gee, E. K., Baranov, E., Wandrey, L., Rogers, S., et al. (2017). Increased metalloproteinase activity in the hippocampus following status epilepticus. Epilepsy Res. 132, 50–58. doi: 10.1016/j.eplepsyres.2017.02.021
Dyck, S., and Karimi-Abdolrezaee, S. (2015). Chondroitin sulfate proteoglycans: key modulators in the developing and pathologic central nervous system. Exp. Neurol. 269, 169–187. doi: 10.1016/j.expneurol.2015.04.006
Dzwonek, J., and Wilczynski, G. M. (2015). CD44: molecular interactions, signaling and functions in the nervous system. Front. Cell. Neurosci. 9:175. doi: 10.3389/fncel.2015.00175
Edgell, C., BaSalamah, M. A., and Marr, H. S. (2004). Testican-1: a differentially expressed proteoglycan with protease inhibiting activities. Int. Rev. Cytol. 236, 101–122. doi: 10.1016/s0074-7696(04)36003-1
Edwards, T., and Hammarlund, M. (2014). Syndecan promotes axon regeneration by stabilizing growth cone migration. Cell Rep. 8, 272–283. doi: 10.1016/j.celrep.2014.06.008
Eill, G. J., Sinha, A., Morawski, M., Viapiano, M. S., and Matthews, R. T. (2020). The protein tyrosine phosphatase RPTPzeta/phosphacan is critical for perineuronal net structure. J. Biol. Chem. 295, 955–968. doi: 10.1074/jbc.ra119.010830
Eisenhaber, B., Bork, P., Yuan, Y., Löffler, G., and Eisenhaber, F. (2000). Automated annotation of GPI anchor sites: case study C. elegans. Trends Biochem. Sci. 25, 340–341. doi: 10.1016/s0968-0004(00)01601-7
Faissner, A., Heck, N., Dobbertin, A., and Garwood, J. (2006). DSD-1-proteoglycan/phosphacan and receptor protein tyrosine phosphatase-beta isoforms during development and regeneration of neural tissues. Adv. Exp. Med. Biol. 557, 25–53. doi: 10.1007/0-387-30128-3_3
Fard, D., and Tamagnone, L. (2020). Semaphorins in health and disease. Cytokine Growth Factor Rev. 57, 55–63. doi: 10.1016/j.cytogfr.2020.05.006
Fawcett, J., Oohashi, T., and Pizzorusso, T. (2019). The roles of perineuronal nets and the perinodal extracellular matrix in neuronal function. Nat. Rev. Neurosci. 20, 451–465. doi: 10.1038/s41583-019-0196-3
Ferrer-Ferrer, M., and Dityatev, A. (2018). Shaping synapses by the neural extracellular matrix. Front. Neuroanat. 12:40. doi: 10.3389/fnana.2018.00040
Ferro, D., Provasoli, A., Ragazzi, M., Casu, B., Torri, G., Bossennec, V., et al. (1990). Conformer populations of L-iduronic acid residues in glycosaminoglycan sequences. Carbohydr. Res. 195, 157–167. doi: 10.1016/0008-6215(90)84164-p
FitzGerald, P., Sturgill, D., Shyakhtenko, A., Oliver, B., and Vinson, C. (2006). Comparative genomics of Drosophila and human core promoters. Genome Biol. 7:R53.
Florio, P., Gazzolo, D., Luisi, S., and Petraglia, F. (2007). Activin A in brain injury. Adv. Clin. Chem. 43, 117–130. doi: 10.1016/s0065-2423(06)43004-3
Forostyak, S. H. A., Turnovcova, K., Svitil, P., Jendelova, P., and Sykova, E. (2014). Intrathecal delivery of mesenchymal stromal cells protects the structure of altered perineuronal nets in SOD1 rats and amends the course of ALS. Stem Cells 32, 3163–3172. doi: 10.1002/stem.1812
Frahm, K., Nash, C. P., and Tobet, S. A. (2013). Endocan immunoreactivity in the mouse brain: method for identifying nonfunctional blood vessels. J. Immunol. Methods 398-399, 27–32. doi: 10.1016/j.jim.2013.09.005
Fries, E., and Blom, A. M. (2000). Bikunin not just a plasma proteinase inhibitor. Int. J. Biochem. Cell Biol. 32, 125–137. doi: 10.1016/s1357-2725(99)00125-9
Fujikawa, A., Chow, J. P. H., Matsumoto, M., Suzuki, R., Kuboyama, K., Yamamoto, N., et al. (2017). Identification of novel splicing variants of protein tyrosine phosphatase receptor type Z. J. Biochem. 162, 381–390. doi: 10.1093/jb/mvx042
Furutani, Y., Manabe, R., Tsutsui, K., Yamada, T., Sugimoto, N., Fukuda, S., et al. (2005). Identification and characterization of photomedins: novel olfactomedin-domain-containing proteins with chondroitin sulphate-E-binding activity. Biochem. J. 389, 675–684. doi: 10.1042/bj20050120
Gabius, H. (2018). The sugar code: why glycans are so important. Biosystems 164, 102–111. doi: 10.1016/j.biosystems.2017.07.003
Galtrey, C., and Fawcett, J. W. (2007). The role of chondroitin sulfate proteoglycans in regeneration and plasticity in the central nervous system. Brain Res. Rev. 54, 1–18. doi: 10.1016/j.brainresrev.2006.09.006
Gandal, M., Zhang, P., Hadjimichael, E., Walker, R. L., Chen, C., Liu, S., et al. (2018). Transcriptome-wide isoform-level dysregulation in ASD, schizophrenia, and bipolar disorder. Science 362:eaat8127. doi: 10.1126/science.aat8127
Garwood, J., Heck, N., Reichardt, F., and Faissner, A. (2003). Phosphacan short isoform, a novel non-proteoglycan variant of phosphacan/receptor protein tyrosine phosphatase-beta, interacts with neuronal receptors and promotes neurite outgrowth. J. Biol. Chem. 278, 24164–24173. doi: 10.1074/jbc.m211721200
Gary, S., and Hockfield, S. (2000). BEHAB/brevican: an extracellular matrix component associated with invasive glioma. Clin. Neurosurg. 47, 72–82.
Gaudet, A., Portier, L., Mathieu, D., Hureau, M., Tsicopoulos, A., Lassalle, P., et al. (2020). Cleaved endocan acts as a biologic competitor of endocan in the control of ICAM-1-dependent leukocyte diapedesis. J. Leukoc. Biol. 107, 833–841. doi: 10.1002/jlb.3ab0320-612rr
Gholamin, S., Mitra, S. S., Feroze, A. H., Liu, J., Kahn, S. A., Zhang, M., et al. (2017). Disrupting the CD47-SIRPα anti-phagocytic axis by a humanized anti-CD47 antibody is an efficacious treatment for malignant pediatric brain tumors. Sci. Transl. Med. 9:eaaf2968. doi: 10.1126/scitranslmed.aaf2968
Giamanco, K., and Matthews, R. T. (2020). The role of BEHAB/Brevican in the tumor microenvironment: mediating glioma cell invasion and motility. Adv. Exp. Med. Biol. 1272, 117–132. doi: 10.1007/978-3-030-48457-6_7
Gilthorpe, J., Oozeer, F., Nash, J., Calvo, M., Bennett, D. L., Lumsden, A., et al. (2013). Extracellular histone H1 is neurotoxic and drives a pro-inflammatory response in microglia. F1000 Res. 2:148. doi: 10.12688/f1000research.2-148.v1
Girolamo, F., Dallatomasina, A., Rizzi, M., Errede, M., Walchli, T., Mucignat, M. T., et al. (2013). Diversified expression of NG2/CSPG4 isoforms in glioblastoma and human foetal brain identifies pericyte subsets. PLoS One 8:e84883. doi: 10.1371/journal.pone.0084883
Gooi, H., Feizi, T., Kapadia, A., Knowles, B. B., Solter, D., and Evans, M. J. (1981). Stage-specific embryonic antigen involves alpha 1 goes to 3 fucosylated type 2 blood group chains. Nature 292, 156–158. doi: 10.1038/292156a0
Gopal, S., Arokiasamy, S., Pataki, C., Whiteford, J. R., and Couchman, J. R. (2021). Syndecan receptors: pericellular regulators in development and inflammatory disease. Open Biol. 11:200377.
Gopal, S., Couchman, J., and Pocock, R. (2016). Redefining the role of syndecans in C. elegans biology. Worm 5:e1142042. doi: 10.1080/21624054.2016.1142042
Goshima, Y., Ito, T., Sasaki, Y., and Nakamura, F. (2002). Semaphorins as signals for cell repulsion and invasion. J. Clin. Invest. 109, 993–998. doi: 10.1172/jci0215467
Gray, P., Bilezikjian, L. M., and Vale, W. (2001). Antagonism of activin by inhibin and inhibin receptors: a functional role for betaglycan-glycan. Mol. Cell. Endocrinol. 180, 47–53. doi: 10.1016/s0303-7207(01)00515-9
Guilak, F., Hayes, A. J., and Melrose, J. (2021). Perlecan in pericellular mechanosensory cell-matrix communication, extracellular matrix stabilisation and mechanoregulation of load-bearing connective tissues. Int. J. Mol. Sci. 22:2716. doi: 10.3390/ijms22052716
Güiza, J., Barría, I., Sáez, J. C., and Vega, J. L. (2018). Innexins: expression, regulation, and functions. Front. Physiol. 9:1414. doi: 10.3389/fphys.2018.01414
Gumienny, T., MacNeil, L. T., Wang, H., de Bono, M., Wrana, J. L., and Padgett, R. W. (2007). Glypican LON-2 is a conserved negative regulator of BMP-like signaling in Caenorhabditis elegans. Curr. Biol. 17, 159–164. doi: 10.1016/j.cub.2006.11.065
Gysi, S., Rhiner, C., Flibotte, S., Moerman, D. G., and Hengartner, M. O. (2013). A network of HSPG core proteins and HS modifying enzymes regulates netrin-dependent guidance of D-type motor neurons in Caenorhabditis elegans. PLoS One 8:e74908. doi: 10.1371/journal.pone.0074908
Hakomori, S., Nudelman, E., Levery, S., Solter, D., and Knowles, B. B. (1981). The hapten structure of a developmentally regulated glycolipid antigen (SSEA-1) isolated from human erythrocytes and adenocarcinoma: a preliminary note. Biochem. Biophys. Res. Commun. 100, 1578–1586. doi: 10.1016/0006-291x(81)90699-9
Hamasuna, R., Kataoka, H., Meng, J. Y., Itoh, H., Moriyama, T., Wakisaka, S., et al. (2001). Reduced expression of hepatocyte growth factor activator inhibitor type-2/placental bikunin (HAI-2/PB) in human glioblastomas: implication for anti-invasive role of HAI-2/PB in glioblastoma cells. Int. J. Cancer 93, 339–345. doi: 10.1002/ijc.1349
Hanada, Y., Nakamura, Y., Ishida, Y., Takimoto, Y., Taniguchi, M., Ozono, Y., et al. (2017). Epiphycan is specifically expressed in cochlear supporting cells and is necessary for normal hearing. Biochem. Biophys. Res. Commun. 492, 379–385. doi: 10.1016/j.bbrc.2017.08.092
Hartmann, U., Hülsmann, H., Seul, J., Röll, S., Midani, H., Breloy, I., et al. (2013). Testican-3: a brain-specific proteoglycan member of the BM-40/SPARC/osteonectin family. J. Neurochem. 125, 399–409. doi: 10.1111/jnc.12212
Hassan, B., Li, L., Bremer, K. A., Chang, W., Pinsonneault, J., and Vaessin, H. (1997). Prospero is a panneural transcription factor that modulates homeodomain protein activity. Proc. Natl. Acad. Sci. U.S.A. 94, 10991–10996. doi: 10.1073/pnas.94.20.10991
Hayes, A., and Melrose, J. (2018). Glycans and glycosaminoglycans in neurobiology: key regulators of neuronal cell function and fate. Biochem. J. 475, 2511–2545. doi: 10.1042/bcj20180283
Hayes, A., and Melrose, J. (2020a). Aggrecan, the primary weight-bearing cartilage proteoglycan, has context-dependent, cell-directive properties in embryonic development and neurogenesis: aggrecan glycan side chain modifications convey interactive biodiversity. Biomolecules 10:E1244.
Hayes, A., and Melrose, J. (2020b). Electro-stimulation, a promising therapeutic treatment modality for tissue repair: emerging roles of sulfated glycosaminoglycans as electro-regulatory mediators of intrinsic repair processes. Adv. Ther. 3:2000151. doi: 10.1002/adtp.202000151
Hayes, A., Sugahara, K., Farrugia, B., Whitelock, J. M., Caterson, B., and Melrose, J. (2018). Biodiversity of CS-proteoglycan sulphation motifs: chemical messenger recognition modules with roles in information transfer, control of cellular behaviour and tissue morphogenesis. Biochem. J. 475, 587–620. doi: 10.1042/bcj20170820
Haylock-Jacobs, S., Keough, M. B., Lau, L., and Yong, V. W. (2011). Chondroitin sulphate proteoglycans: extracellular matrix proteins that regulate immunity of the central nervous system. Autoimmun. Rev. 10, 766–772. doi: 10.1016/j.autrev.2011.05.019
Hering, T., Beller, J. A., Calulot, C. M., and Snow, D. M. (2020). Contributions of chondroitin sulfate, keratan sulfate and N-linked oligosaccharides to inhibition of neurite outgrowth by aggrecan. Biology (Basel) 9:E29.
Hikino, M., Mikami, T., Faissner, A., Vilela-Silva, A. C., Pavão, M. S., and Sugahara, K. (2003). Oversulfated dermatan sulfate exhibits neurite outgrowth-promoting activity toward embryonic mouse hippocampal neurons: implications of dermatan sulfate in neuritogenesis in the brain. J. Biol. Chem. 278, 43744–43754. doi: 10.1074/jbc.m308169200
Hobert, O. (2010). “Neurogenesis in the nematode Caenorhabditis elegans,” in WormBook, ed. The C. elegans Research Community (New York, NY: WormBook). doi: 10.1895/wormbook.1.12.2
Hope, C., Foulcer, S., Jagodinsky, J., Chen, S. X., Jensen, J. L., Patel, S., et al. (2016). Immunoregulatory roles of versican proteolysis in the myeloma microenvironment. Blood 128, 680–685. doi: 10.1182/blood-2016-03-705780
Horii-Hayashi, N., Okuda, H., Tatsumi, K., Ishizaka, S., Yoshikawa, M., and Wanaka, A. (2008). Localization of chondroitin sulfate proteoglycan versican in adult brain with special reference to large projection neurons. Cell Tissue Res. 334, 163–177. doi: 10.1007/s00441-008-0698-1
Hortobágyi, T., and Maffiuletti, N. A. (2011). Neural adaptations to electrical stimulation strength training. Eur. J. Appl. Physiol. 111, 2439–2449. doi: 10.1007/s00421-011-2012-2
Hu, J., Xiao, Q., Dong, M., Guo, D., Wu, X., and Wang, B. (2020). Glioblastoma immunotherapy targeting the innate immune checkpoint CD47-SIRPα Axis. Front. Immunol. 11:593219. doi: 10.3389/fimmu.2020.593219
Hu, P. J. (2007). “Dauer,” in WormBook, ed. The C. elegans Research Community (New York, NY: WormBook). doi: 10.1895/wormbook.1.144.1
Huang, W., Zhao, N., Bai, X., Karram, K., Trotter, J., Goebbels, S., et al. (2014). Novel NG2-CreERT2 knock-in mice demonstrate heterogeneous differentiation potential of NG2 glia during development. Glia 62, 896–913. doi: 10.1002/glia.22648
Hutter, G., Theruvath, J., Graef, C. M., Zhang, M., Schoen, M. K., Manz, E. M., et al. (2019). Microglia are effector cells of CD47-SIRPα antiphagocytic axis disruption against glioblastoma. Proc. Natl. Acad. Sci. U.S.A. 116, 997–1006. doi: 10.1073/pnas.1721434116
Hwang, H., Olson, S. K., Brown, J. R., Esko, J. D., and Horvitz, H. R. (2003a). The Caenorhabditis elegans genes sqv-2 and sqv-6, which are required for vulval morphogenesis, encode glycosaminoglycan galactosyltransferase II and xylosyltransferase. J. Biol. Chem. 278, 11735–11738. doi: 10.1074/jbc.c200518200
Hwang, H., Olson, S. K., Esko, J. D., and Horvitz, H. R. (2003b). Caenorhabditis elegans early embryogenesis and vulval morphogenesis require chondroitin biosynthesis. Nature 423, 439–443. doi: 10.1038/nature01634
Iozzo, R., Buraschi, S., Genua, M., Xu, S. Q., Solomides, C. C., Peiper, S. C., et al. (2011). Decorin antagonizes IGF receptor I (IGF-IR) function by interfering with IGF-IR activity and attenuating downstream signaling. J. Biol. Chem. 286, 34712–34721. doi: 10.1074/jbc.m111.262766
Iseki, K., Hagino, S., Zhang, Y., Mori, T., Sato, N., Yokoya, S., et al. (2011). Altered expression pattern of testican-1 mRNA after brain injury. Biomed. Res. 32, 373–378. doi: 10.2220/biomedres.32.373
Islam, S., and Watanabe, H. (2020). Versican: a dynamic regulator of the extracellular matrix. J. Histochem. Cytochem. 68, 763–775. doi: 10.1369/0022155420953922
Jain, D., Mattiassi, S., Goh, E. L., and Yim, E. K. F. (2020). Extracellular matrix and biomimetic engineering microenvironment for neuronal differentiation. Neural Regen. Res. 15, 573–585. doi: 10.4103/1673-5374.266907
Jenkins, L., Horst, B., Lancaster, C. L., and Mythreye, K. (2018). Dually modified transmembrane proteoglycans in development and disease. Cytokine Growth Factor Rev. 39, 124–136. doi: 10.1016/j.cytogfr.2017.12.003
Jenkins, L., Singh, P., Varadaraj, A., Lee, N. Y., Shah, S., Flores, H. V., et al. (2016). Altering the proteoglycan state of transforming growth factor β Type III receptor (TβRIII)/betaglycan modulates canonical Wnt/β-catenin signaling. J. Biol. Chem. 291, 25716–25728. doi: 10.1074/jbc.m116.748624
Jeong, J., Paskus, J. D., and Roche, K. W. (2017). Posttranslational modifications of neuroligins regulate neuronal and glial signaling. Curr. Opin. Neurobiol. 45, 130–138. doi: 10.1016/j.conb.2017.05.017
Jeong, P.-Y., Jung, M., Yim, Y.-H., Kim, H., Park, M., Hong, E., et al. (2005). Chemical structure and biological activity of the Caenorhabditis elegans dauer-inducing pheromone. Nature 433, 541–545. doi: 10.1038/nature03201
Johnson, H., Rosenberg, L., Choi, H. U., Garza, S., Höök, M., and Neame, P. J. (1997). Characterization of epiphycan, a small proteoglycan with a leucine-rich repeat core protein. J. Biol. Chem. 272, 18709–18717. doi: 10.1074/jbc.272.30.18709
Jones, L., Yamaguchi, Y., Stallcup, W. B., and Tuszynski, M. H. (2002). NG2 is a major chondroitin sulfate proteoglycan produced after spinal cord injury and is expressed by macrophages and oligodendrocyte progenitors. J. Neurosci. 22, 2792–2803. doi: 10.1523/jneurosci.22-07-02792.2002
Josberger, E., Hassanzadeh, P., Deng, Y., Sohn, J., Rego, M. J., Amemiya, C. T., et al. (2016). Proton conductivity in ampullae of Lorenzini jelly. Sci. Adv. 2:e1600112. doi: 10.1126/sciadv.1600112
Jüttner, R., Montag, D., Craveiro, R. B., Babich, A., Vetter, P., and Rathjen, F. G. (2013). Impaired presynaptic function and elimination of synapses at premature stages during postnatal development of the cerebellum in the absence of CALEB (CSPG5/neuroglycan C). Eur. J. Neurosci. 38, 3270–3280. doi: 10.1111/ejn.12313
Kali, A., and Shetty, K. S. (2014). Endocan: a novel circulating proteoglycan. Indian J. Pharmacol. 46, 579–583. doi: 10.4103/0253-7613.144891
Kaltner, H., Abad-Rodríguez, J., Corfield, A. P., Kopitz, J., and Gabius, H. J. (2019). The sugar code: letters and vocabulary, writers, editors and readers and biosignificance of functional glycan-lectin pairing. Biochem. J. 476, 2623–2655. doi: 10.1042/bcj20170853
Kamimura, K., and Maeda, N. (2017). Heparan sulfate proteoglycans in Drosophila neuromuscular development. Biochim. Biophys. Acta Gen. Subj. 1861, 2442–2446. doi: 10.1016/j.bbagen.2017.06.015
Kamimura, K., and Maeda, N. (2021). Glypicans and heparan sulfate in synaptic development, neural plasticity, and neurological disorders. Front. Neural Circuits 15:595596. doi: 10.3389/fncir.2021.595596
Karamanos, N., Piperigkou, Z., Theocharis, A. D., Watanabe, H., Franchi, M., Baud, S., et al. (2018). Proteoglycan chemical diversity drives multifunctional cell regulation and therapeutics. Chem. Rev. 118, 9152–9232. doi: 10.1021/acs.chemrev.8b00354
Keirstead, H., and Blakemore, W. F. (1999). The role of oligodendrocytes and oligodendrocyte progenitors in CNS remyelination. Adv. Exp. Med. Biol. 468, 183–197. doi: 10.1007/978-1-4615-4685-6_15
Kelly, A., O’Malley, A., Redha, M., O’Keeffe, G. W., and Barry, D. S. (2019). The distribution of the proteoglycan FORSE-1 in the developing mouse central nervous system. J. Anat. 234, 216–226. doi: 10.1111/joa.12907
Kerever, A., Mercier, F., Nonaka, R., de Vega, S., Oda, Y., Zalc, B., et al. (2014). Perlecan is required for FGF-2 signaling in the neural stem cell niche. Stem Cell Res. 12, 492–505. doi: 10.1016/j.scr.2013.12.009
Kiani, C., Chen, L., Wu, Y. J., Yee, A. J., and Yang, B. B. (2002). Structure and function of aggrecan. Cell Res. 12, 19–32. doi: 10.1038/sj.cr.7290106
Kikuchi, A., Tomoyasu, H., Kido, I., Takahashi, K., Tanaka, A., Nonaka, I., et al. (2000). Haemopoietic biglycan produced by brain cells stimulates growth of microglial cells. J. Neuroimmunol. 106, 78–86. doi: 10.1016/s0165-5728(99)00258-1
Kim, B., De La Monte, S., Hovanesian, V., Patra, A., Chen, X., Chen, R. H., et al. (2020). Ontogeny of inter-alpha inhibitor protein (IAIP) expression in human brain. J. Neurosci. Res. 98, 869–887. doi: 10.1002/jnr.24565
Kim, S., Henen, M. A., and Hinck, A. P. (2019). Structural biology of betaglycan and endoglin, membrane-bound co-receptors of the TGF-beta family. Exp. Biol. Med. (Maywood) 244, 1547–1558. doi: 10.1177/1535370219881160
Kim, W., Underwood, R. S., Greenwald, I., and Shaye, D. D. (2018). OrthoList 2: a new comparative genomic analysis of human and Caenorhabditis elegans genes. Genetics 210, 445–461. doi: 10.1534/genetics.118.301307
Kinugasa, Y., Ishiguro, H., Tokita, Y., Oohira, A., Ohmoto, H., and Higashiyama, S. (2004). Neuroglycan C, a novel member of the neuregulin family. Biochem. Biophys. Res. Commun. 321, 1045–1049. doi: 10.1016/j.bbrc.2004.07.066
Kitaguchi, N., Takahashi, Y., Tokushima, Y., Shiojiri, S., and Ito, H. (1988). Novel precursor of Alzheimer’s disease amyloid protein shows protease inhibitory activity. Nature 331, 530–532. doi: 10.1038/331530a0
Knelson, E., Gaviglio, A. L., Tewari, A. K., Armstrong, M. B., Mythreye, K., and Blobe, G. C. (2013). Type III TGF-β receptor promotes FGF2-mediated neuronal differentiation in neuroblastoma. J. Clin. Invest. 123, 4786–4798. doi: 10.1172/jci69657
Koehn, L., Chen, X., Logsdon, A. F., Lim, Y. P., and Stonestreet, B. S. (2020). novel neuroprotective agents to treat neonatal hypoxic-ischemic encephalopathy: inter-alpha inhibitor proteins. Int. J. Mol. Sci. 21:9193. doi: 10.3390/ijms21239193
Kolset, S. O., and Pejler, G. (2011). Serglycin: a structural and functional chameleon with wide impact on immune cells. J. Immunol. 187, 4927–4933. doi: 10.4049/jimmunol.1100806
Kolset, S., and Tveit, H. (2008). Serglycin–structure and biology. Cell. Mol. Life Sci. 65, 1073–1085. doi: 10.1007/s00018-007-7455-6
Koops, A., Kappler, J., Junghans, U., Kuhn, G., Kresse, H., and Müller, H. W. (1996). Cultured astrocytes express biglycan, a chondroitin/dermatan sulfate proteoglycan supporting the survival of neocortical neurons. Brain Res. Mol. Brain Res. 41, 65–73. doi: 10.1016/0169-328x(96)00067-8
Kovács, I., Barabási, D. L., and Barabási, A. L. (2020). Uncovering the genetic blueprint of the C. elegans nervous system. Proc. Natl. Acad. Sci. U.S.A. 117, 33570–33577. doi: 10.1073/pnas.2009093117
Krahn, N., Meier, M., Reuten, R., Koch, M., Stetefeld, J., and Patel, T. R. (2019). Solution structure of C. elegans UNC-6: a nematode paralogue of the axon guidance protein Netrin-1. Biophys. J. 116, 2121–2130. doi: 10.1016/j.bpj.2019.04.033
Krenzlin, H., Lorenz, V., Danckwardt, S., Kempski, O., and Alessandri, B. (2016). The importance of thrombin in cerebral injury and disease. Int. J. Mol. Sci. 17:84. doi: 10.3390/ijms17010084
Kuo, T., Chen, A., Harrabi, O., Sockolosky, J. T., Zhang, A., Sangalang, E., et al. (2020). Targeting the myeloid checkpoint receptor SIRPα potentiates innate and adaptive immune responses to promote anti-tumor activity. J. Hematol. Oncol. 13:160.
Lah, T., Novak, M., and Breznik, B. (2020). Brain malignancies: glioblastoma and brain metastases. Semin. Cancer Biol. 60, 262–273. doi: 10.1016/j.semcancer.2019.10.010
Lamprianou, S., Chatzopoulou, E., Thomas, J. L., Bouyain, S., and Harroch, S. (2011). A complex between contactin-1 and the protein tyrosine phosphatase PTPRZ controls the development of oligodendrocyte precursor cells. Proc. Natl. Acad. Sci. U.S.A. 108, 17498–17503. doi: 10.1073/pnas.1108774108
Lane, N. (2017). Proton gradients at the origin of life. Bioessays 39:1600217. doi: 10.1002/bies.201600217
Lee, S., Zhang, X., and Wang, M. M. (2014). Vascular accumulation of the small leucine-rich proteoglycan decorin in CADASIL. Neuroreport 25, 1059–1063. doi: 10.1097/wnr.0000000000000230
Lele, A., Alunpipatthanachai, B., Qiu, Q., Clark-Bell, C., Watanitanon, A., Moore, A., et al. (2019). Plasma levels, temporal trends and clinical associations between biomarkers of inflammation and vascular homeostasis after pediatric traumatic brain injury. Dev. Neurosci. 41, 177–192. doi: 10.1159/000502276
Lewis, K., Gray, P. C., Blount, A. L., MacConell, L. A., Wiater, E., Bilezikjian, L. M., et al. (2000). Betaglycan binds inhibin and can mediate functional antagonism of activin signalling. Nature 404, 411–414. doi: 10.1038/35006129
Li, Z., Li, Y., Gao, J., Fu, Y., Hua, P., Jing, Y., et al. (2021). The role of CD47-SIRPα immune checkpoint in tumor immune evasion and innate immunotherapy. Life Sci. 273, 779150.
Lin, J. Z., Duan, M. R., Lin, N., and Zhao, W. J. (2021). The emerging role of the chondroitin sulfate proteoglycan family in neurodegenerative diseases. Rev. Neurosci. doi: 10.1515/revneuro-2020-0146
Liu, C., Kuo, Y. C., Wang, C. Y., Hsu, C. C., Ho, Y. J., Chiang, Y. C., et al. (2020). Syndecan-3 contributes to the regulation of the microenvironment at the node of Ranvier following end-to side neurorrhaphy: sodium image analysis. Histochem. Cell Biol. 155, 355–367. doi: 10.1007/s00418-020-01936-z
Long, K., and Huttner, W. B. (2019). How the extracellular matrix shapes neural development. Open Biol. 9:180216. doi: 10.1098/rsob.180216
Lord, M., Day, A. J., Youssef, P., Zhuo, L., Watanabe, H., Caterson, B., et al. (2013). Sulfation of the bikunin chondroitin sulfate chain determines heavy chain. hyaluronan complex formation. J. Biol. Chem. 288, 22930–22941. doi: 10.1074/jbc.m112.404186
Lord, M., Melrose, J., Day, A. J., and Whitelock, J. M. (2020). The inter-α-trypsin inhibitor family: versatile molecules in biology and pathology. J. Histochem. Cytochem. 68, 907–927. doi: 10.1369/0022155420940067
Losada-Perez, M., Harrison, N., and Hidalgo, A. (2016). Molecular mechanism of central nervous system repair by the Drosophila NG2 homologue kon-tiki. J. Cell Biol. 214, 587–601. doi: 10.1083/jcb.201603054
Lynch, M., and Marinov, G. K. (2017). Membranes, energetics, and evolution across the prokaryote-eukaryote divide. Elife 6:e20437.
Lyon, M., Deakin, J. A., Rahmoune, H., Fernig, D. G., Nakamura, T., and Gallagher, J. T. (1998). Hepatocyte growth factor/scatter factor binds with high affinity to dermatan sulfate. J. Biol. Chem. 273, 271–278. doi: 10.1074/jbc.273.1.271
MacConell, L., Leal, A. M., and Vale, W. W. (2002). The distribution of betaglycan protein and mRNA in rat brain, pituitary, and gonads: implications for a role for betaglycan in inhibin-mediated reproductive functions. Endocrinology 143, 1066–1075. doi: 10.1210/endo.143.3.8707
Maćkowiak, M., Mordalska, P., and Wędzony, K. (2014). Neuroligins, synapse balance and neuropsychiatric disorders. Pharmacol. Rep. 66, 830–835. doi: 10.1016/j.pharep.2014.04.011
Makanji, Y., Harrison, C. A., Stanton, P. G., Krishna, R., and Robertson, D. M. (2007). Inhibin A and B in vitro bioactivities are modified by their degree of glycosylation and their affinities to betaglycan. Endocrinology 148, 2309–2316. doi: 10.1210/en.2006-1612
Malavaki, C., Mizumoto, S., Karamanos, N., and Sugahara, K. (2008). Recent advances in the structural study of functional chondroitin sulfate and dermatan sulfate in health and disease. Connect. Tissue Res. 49, 133–139. doi: 10.1080/03008200802148546
Malmström, A., Bartolini, B., Thelin, M. A., Pacheco, B., and Maccarana, M. (2012). Iduronic acid in chondroitin/dermatan sulfate: biosynthesis and biological function. J. Histochem. Cytochem. 60, 916–925. doi: 10.1369/0022155412459857
Manou, D., Bouris, P., Kletsas, D., Götte, M., Greve, B., Moustakas, A., et al. (2020). Serglycin activates pro-tumorigenic signaling and controls glioblastoma cell stemness, differentiation and invasive potential. Matrix Biol. Plus 6–7:100033. doi: 10.1016/j.mbplus.2020.100033
Margolis, R., and Margolis, R. K. (1994). Aggrecan-versican-neurocan family proteoglycans. Methods Enzymol. 245, 105–126. doi: 10.1016/0076-6879(94)45008-0
Margolis, R., Rauch, U., Maurel, P., and Margolis, R. U. (1996). Neurocan and phosphacan: two major nervous tissue-specific chondroitin sulfate proteoglycans. Perspect. Dev. Neurobiol. 3, 273–290.
Maro, G., Gao, S., Olechwier, A. M., Hung, W. L., Liu, M., Özkan, E., et al. (2015). MADD-4/punctin and neurexin organize C. elegans GABAergic postsynapses through neuroligin. Neuron 86, 1420–1432. doi: 10.1016/j.neuron.2015.05.015
Marr, H., Basalamah, M. A., Bouldin, T. W., Duncan, A. W., and Edgell, C. J. (2000). Distribution of testican expression in human brain. Cell Tissue Res. 302, 139–144. doi: 10.1007/s004410000277
Martinez, J., Dhawan, A., and Farach-Carson, M. C. (2018). Modular proteoglycan perlecan/HSPG2: mutations, phenotypes, and functions. Genes (Basel) 9:556. doi: 10.3390/genes9110556
Massagué, J., Andres, J., Attisano, L., Cheifetz, S., López-Casillas, F., Ohtsuki, M., et al. (1992). TGF-beta receptors. Mol. Reprod. Dev. 32, 99–104.
Mathieu, P., Piantanida, A. P., and Pitossi, F. (2010). Chronic expression of transforming growth factor-beta enhances adult neurogenesis. Neuroimmunomodulation 17, 200–201. doi: 10.1159/000258723
Matlung, H., Szilagyi, K., Barclay, N. A., and van den Berg, T. K. (2017). The CD47-SIRPα signaling axis as an innate immune checkpoint in cancer. Immunol. Rev. 276, 145–164. doi: 10.1111/imr.12527
Matthews, R., Gary, S. C., Zerillo, C., Pratta, M., Solomon, K., Arner, E. C., et al. (2000). Brain-enriched hyaluronan binding (BEHAB)/brevican cleavage in a glioma cell line is mediated by a disintegrin and metalloproteinase with thrombospondin motifs (ADAMTS) family member. J. Biol. Chem. 275, 22695–22703. doi: 10.1074/jbc.m909764199
Mauney, S., Athanas, K. M., Pantazopoulos, H., Shaskan, N., Passeri, E., Berretta, S., et al. (2013). Developmental pattern of perineuronal nets in the human prefrontal cortex and their deficit in schizophrenia. Biol. Psychiatry 74, 427–435. doi: 10.1016/j.biopsych.2013.05.007
Maurage, C., Adam, E., Minéo, J. F., Sarrazin, S., Debunne, M., Siminski, R. M., et al. (2009). Endocan expression and localization in human glioblastomas. J. Neuropathol. Exp. Neurol. 68, 633–641. doi: 10.1097/nen.0b013e3181a52a7f
McTigue, D., Tripathi, R., and Wei, P. (2006). NG2 colocalizes with axons and is expressed by a mixed cell population in spinal cord lesions. J. Neuropathol. Exp. Neurol. 65, 406–420. doi: 10.1097/01.jnen.0000218447.32320.52
Mecollari, V., Nieuwenhuis, B., and Verhaagen, J. (2014). A perspective on the role of class III semaphorin signaling in central nervous system trauma. Front. Cell. Neurosci. 8:328. doi: 10.3389/fncel.2014.00328
Mega, A., Hartmark Nilsen, M., Leiss, L. W., Tobin, N. P., Miletic, H., Sleire, L., et al. (2020). Astrocytes enhance glioblastoma growth. Glia 68, 316–327. doi: 10.1002/glia.23718
Melrose, J. (2019a). Functional consequences of keratan sulfate sulfation in electrosensory tissues and in neuronal regulation. Adv. Biosyst. 3:e1800327.
Melrose, J. (2019b). Keratan sulfate (KS)-proteoglycans and neuronal regulation in health and disease: the importance of KS-glycodynamics and interactive capability with neuroregulatory ligands. J. Neurochem. 149, 170–194. doi: 10.1111/jnc.14652
Melrose, J., Hayes, A. J., and Bix, G. (2021). The CNS/PNS extracellular matrix provides instructive guidance cues to neural cells and neuroregulatory proteins in neural development and repair. Int. J. Mol. Sci. 22:5583. doi: 10.3390/ijms22115583
Mencio, C., Hussein, R. K., Yu, P., and Geller, H. M. (2021). The role of chondroitin sulfate proteoglycans in nervous system development. J. Histochem. Cytochem. 69, 61–80. doi: 10.1369/0022155420959147
Mercier, F. (2016). Fractones: extracellular matrix niche controlling stem cell fate and growth factor activity in the brain in health and disease. Cell. Mol. Life Sci. 73, 4661–4674. doi: 10.1007/s00018-016-2314-y
Mercier, F., and Arikawa-Hirasawa, E. (2012). Heparan sulfate niche for cell proliferation in the adult brain. Neurosci. Lett. 510, 67–72. doi: 10.1016/j.neulet.2011.12.046
Mercier, F., Kwon, Y. C., and Douet, V. (2012). Hippocampus/amygdala alterations, loss of heparan sulfates, fractones and ventricle wall reduction in adult BTBR T+ tf/J mice, animal model for autism. Neurosci. Lett. 506, 208–213. doi: 10.1016/j.neulet.2011.11.007
Milev, P., Friedlander, D. R., Sakurai, T., Karthikeyan, L., Flad, M., Margolis, R. K., et al. (1994). Interactions of the chondroitin sulfate proteoglycan phosphacan, the extracellular domain of a receptor-type protein tyrosine phosphatase, with neurons, glia, and neural cell adhesion molecules. J. Cell Biol. 127, 1703–1715. doi: 10.1083/jcb.127.6.1703
Miller, M., Lambert-Messerlian, G. M., Eklund, E. E., Heath, N. L., Donahue, J. E., and Stopa, E. G. (2012). Expression of inhibin/activin proteins and receptors in the human hypothalamus and basal forebrain. J. Neuroendocrinol. 24, 962–972. doi: 10.1111/j.1365-2826.2012.02289.x
Mitsunaga, C., Mikami, T., Mizumoto, S., Fukuda, J., and Sugahara, K. (2006). Chondroitin sulfate/dermatan sulfate hybrid chains in the development of cerebellum. Spatiotemporal regulation of the expression of critical disulfated disaccharides by specific sulfotransferases. J. Biol. Chem. 281, 18942–18952. doi: 10.1074/jbc.m510870200
Miura, R., Aspberg, A., Ethell, I. M., Hagihara, K., Schnaar, R. L., Ruoslahti, E., et al. (1999). The proteoglycan lectin domain binds sulfated cell surface glycolipids and promotes cell adhesion. J. Biol. Chem. 274, 11431–11438. doi: 10.1074/jbc.274.16.11431
Miura, R., Ethell, I. M., and Yamaguchi, Y. (2001). Carbohydrate-protein interactions between HNK-1-reactive sulfoglucuronyl glycolipids and the proteoglycan lectin domain mediate neuronal cell adhesion and neurite outgrowth. J. Neurochem. 76, 413–424. doi: 10.1046/j.1471-4159.2001.00042.x
Miyata, S., and Kitagawa, H. (2016). Chondroitin sulfate and neuronal disorders. Front. Biosci. (Landmark Ed.) 21:1330–1340. doi: 10.2741/4460
Miyata, S., and Kitagawa, H. (2017). Formation and remodeling of the brain extracellular matrix in neural plasticity: roles of chondroitin sulfate and hyaluronan. Biochim. Biophys. Acta Gen. Subj. 1861, 2420–2434. doi: 10.1016/j.bbagen.2017.06.010
Miyata, S., Nadanaka, S., Igarashi, M., and Kitagawa, H. (2018). Structural variation of chondroitin sulfate chains contributes to the molecular heterogeneity of perineuronal nets. Front. Integr. Neurosci. 12:3. doi: 10.3389/fnint.2018.00003
Mizuguchi, S., Uyama, T., Kitagawa, H., Nomura, K. H., Dejima, K., Gengyo-Ando, K., et al. (2003). Chondroitin proteoglycans are involved in cell division of Caenorhabditis elegans. Nature 423, 443–448. doi: 10.1038/nature01635
Mizumoto, S., Yamada, S., and Sugahara, K. (2015). Molecular interactions between chondroitin-dermatan sulfate and growth factors/receptors/matrix proteins. Curr. Opin. Struct. Biol. 34, 35–42. doi: 10.1016/j.sbi.2015.06.004
Mohan, H., Krumbholz, M., Sharma, R., Eisele, S., Junker, A., Sixt, M., et al. (2010). Extracellular matrix in multiple sclerosis lesions: fibrillar collagens, biglycan and decorin are upregulated and associated with infiltrating immune cells. Brain Pathol. 20, 966–975.
Mooney, K., Choy, W., Sidhu, S., Pelargos, P., Bui, T. T., Voth, B., et al. (2016). The role of CD44 in glioblastoma multiforme. J. Clin. Neurosci. 34, 1–5. doi: 10.1016/j.jocn.2016.05.012
Moore, S., Tessier-Lavigne, M., and Kennedy, T. E. (2007). Netrins and their receptors. Adv. Exp. Med. Biol. 621, 17–31. doi: 10.1007/978-0-387-76715-4_2
Moransard, M., Dann, A., Staszewski, O., Fontana, A., Prinz, M., and Suter, T. (2011). NG2 expressed by macrophages and oligodendrocyte precursor cells is dispensable in experimental autoimmune encephalomyelitis. Brain 134, 1315–1330. doi: 10.1093/brain/awr070
Morawski, M. B. G., Jäger, C., Seeger, G., Matthews, R. T., and Arendt, T. (2012). Involvement of perineuronal and perisynaptic extracellular matrix in Alzheimer’s disease neuropathology. Brain Pathol. 22, 547–561. doi: 10.1111/j.1750-3639.2011.00557.x
Morleo, B., Teresinski, G., Rousseau, G., Tse, R., Tettamanti, C., Augsburger, M., et al. (2019). Biomarkers of cerebral damage in fatal hypothermia: preliminary results. Am. J. Forensic. Med. Pathol. 40, 242–245. doi: 10.1097/paf.0000000000000484
Mühleisen, T., Mattheisen, M., Strohmaier, J., Degenhardt, F., Priebe, L., Schultz, C. C., et al. (2012). Association between schizophrenia and common variation in neurocan (NCAN), a genetic risk factor for bipolar disorder. Schizophr. Res. 138, 69–73. doi: 10.1016/j.schres.2012.03.007
Muir, E., De Winter, F., Verhaagen, J., and Fawcett, J. (2019). Recent advances in the therapeutic uses of chondroitinase ABC. Exp. Neurol. 321:113032. doi: 10.1016/j.expneurol.2019.113032
Mulcahy, B., Witvliet, D., Holmyard, D., Mitchell, J., Chisholm, A. D., Meirovitch, Y., et al. (2018). A pipeline for volume electron microscopy of the Caenorhabditis elegans nervous system. Front Neural Circuits 12:94. doi: 10.3389/fncir.2018.00094
Murakami, K., Tanaka, T., Bando, Y., and Yoshida, S. (2015). Nerve injury induces the expression of syndecan-1 heparan sulfate proteoglycan in primary sensory neurons. Neuroscience 300, 338–350. doi: 10.1016/j.neuroscience.2015.05.033
Murata, Y., Kotani, T., Ohnishi, H., and Matozaki, T. (2014). The CD47-SIRPα signalling system: its physiological roles and therapeutic application. J. Biochem. 155, 335–344. doi: 10.1093/jb/mvu017
Mythreye, K., and Blobe, G. C. (2009). Proteoglycan signaling co-receptors: roles in cell adhesion, migration and invasion. Cell. Signal. 21, 1548–1558. doi: 10.1016/j.cellsig.2009.05.001
Nadanaka, S., Ishida, M., Ikegami, M., and Kitagawa, H. (2008). Chondroitin 4-O-sulfotransferase-1 modulates Wnt-3a signaling through control of E disaccharide expression of chondroitin sulfate. J. Biol. Chem. 283, 27333–27343. doi: 10.1074/jbc.m802997200
Nakada, M., Miyamori, H., Yamashita, J., and Sato, H. (2003). Testican 2 abrogates inhibition of membrane-type matrix metalloproteinases by other testican family proteins. Cancer Res. 63, 3364–3369.
Nakanishi, K., Aono, S., Hirano, K., Kuroda, Y., Ida, M., Tokita, Y., et al. (2006). Identification of neurite outgrowth-promoting domains of neuroglycan C, a brain-specific chondroitin sulfate proteoglycan, and involvement of phosphatidylinositol 3-kinase and protein kinase C signaling pathways in neuritogenesis. J. Biol. Chem. 281, 24970–24978. doi: 10.1074/jbc.m601498200
Nakanishi, K., Tokita, Y., Aono, S., Ida, M., Matsui, F., Higashi, Y., et al. (2010). Neuroglycan C, a brain-specific chondroitin sulfate proteoglycan, interacts with pleiotrophin, a heparin-binding growth factor. Neurochem. Res. 35, 1131–1137. doi: 10.1007/s11064-010-0164-9
Nandadasa, S., Foulcer, S., and Apte, S. S. (2014). The multiple, complex roles of versican and its proteolytic turnover by ADAMTS proteases during embryogenesis. Matrix Biol. 35, 34–41. doi: 10.1016/j.matbio.2014.01.005
Nandini, C., and Sugahara, K. (2006). Role of the sulfation pattern of chondroitin sulfate in its biological activities and in the binding of growth factors. Adv. Pharmacol. 53, 253–279. doi: 10.1016/s1054-3589(05)53012-6
Nastase, M., Young, M. F., and Schaefer, L. (2012). Biglycan: a multivalent proteoglycan providing structure and signals. J. Histochem. Cytochem. 60, 963–975. doi: 10.1369/0022155412456380
Naumann, U., Maass, P., Gleske, A. K., Aulwurm, S., Weller, M., and Eisele, G. (2008). Glioma gene therapy with soluble transforming growth factor-beta receptors II and III. Int. J. Oncol. 33, 759–765.
Neill, T., Schaefer, L., and Iozzo, R. V. (2012). Decorin: a guardian from the matrix. Am. J. Pathol. 181, 380–387.
Nicholson, C., and Hrabětová, S. (2017). Brain extracellular space: the final frontier of neuroscience. Biophys. J. 113, 2133–2142. doi: 10.1016/j.bpj.2017.06.052
Nishihara, S. (2010). Glycosyltransferases and transporters that contribute to proteoglycan synthesis in Drosophila: identification and functional analyses using the heritable and inducible RNAi system. Methods Enzymol. 480, 323–351. doi: 10.1016/s0076-6879(10)80015-1
Niven, J., and Laughlin, S. B. (2008). Energy limitation as a selective pressure on the evolution of sensory systems. J. Exp. Biol. 211, 1792–1804. doi: 10.1242/jeb.017574
Noborn, F., and Larson, G. (2021). Characterization of C. elegans chondroitin proteoglycans and their large functional and structural heterogeneity; evolutionary aspects on structural differences between humans and the nematode. Adv. Exp. Med. Biol. 21, 155–170. doi: 10.1007/5584_2020_485
Nutt, C., Zerillo, C. A., Kelly, G. M., and Hockfield, S. (2001). Brain enriched hyaluronan binding (BEHAB)/brevican increases aggressiveness of CNS-1 gliomas in Lewis rats. Cancer Res. 61, 7056–7059.
Ogura, K., Asakura, T., and Goshima, Y. (2012). Localization mechanisms of the axon guidance molecule UNC-6/Netrin and its receptors, UNC-5 and UNC-40, in Caenorhabditis elegans. Dev. Growth Differ. 54, 390–397. doi: 10.1111/j.1440-169x.2012.01349.x
Olson, S., Bishop, J. R., Yates, J. R., Oegema, K., and Esko, J. D. (2006). Identification of novel chondroitin proteoglycans in Caenorhabditis elegans: embryonic cell division depends on CPG-1 and CPG-2. J. Cell Biol. 173, 985–994. doi: 10.1083/jcb.200603003
Oohira, A., Shuo, T., Tokita, Y., Nakanishi, K., and Aono, S. (2004). Neuroglycan C, a brain-specific part-time proteoglycan, with a particular multidomain structure. Glycoconj. J. 21, 53–57. doi: 10.1023/b:glyc.0000043748.90896.83
Pangalos, M. N., Shioi, J., Efthimiopoulos, S., Wu, A., and Robakis, N. K. (1996). Characterization of appican, the chondroitin sulfate proteoglycan form of the Alzheimer amyloid precursor protein. Neurodegeneration 5, 445–451. doi: 10.1006/neur.1996.0061
Pantazaka, E., and Papadimitriou, E. (2014). Chondroitin sulfate-cell membrane effectors as regulators of growth factor-mediated vascular and cancer cell migration. Biochim. Biophys. Acta 1840, 2643–2650. doi: 10.1016/j.bbagen.2014.01.009
Pantazopoulos, H., Katsel, P., Haroutunian, V., Chelini, G., Klengel, T., and Berretta, S. (2021). Molecular signature of extracellular matrix pathology in schizophrenia. Eur. J. Neurosci. 12, 3960–3987. doi: 10.1111/ejn.15009
Patel, K., Yao, J., Raymond, C., Yong, W., Everson, R., Liau, L. M., et al. (2020). Decorin expression is associated with predictive diffusion MR phenotypes of anti-VEGF efficacy in glioblastoma. Sci. Rep. 10:14819.
Perkins, K., Arranz, A. M., Yamaguchi, Y., and Hrabetova, S. (2017). Brain extracellular space, hyaluronan, and the prevention of epileptic seizures. Rev. Neurosci. 28, 869–892.
Peters, A., and Sherman, L. S. (2020). Diverse roles for hyaluronan and hyaluronan receptors in the developing and adult nervous system. Int. J. Mol. Sci. 21:5988. doi: 10.3390/ijms21175988
Pintér, A., Hevesi, Z., Zahola, P., Alpár, A., and Hanics, J. (2020). Chondroitin sulfate proteoglycan-5 forms perisynaptic matrix assemblies in the adult rat cortex. Cell. Signal. 74:109710. doi: 10.1016/j.cellsig.2020.109710
Platsaki, S., Zhou, X., Pinan-Lucarré, B., Delauzun, V., Tu, H., Mansuelle, P., et al. (2020). The Ig-like domain of Punctin/MADD-4 is the primary determinant for interaction with the ectodomain of neuroligin NLG-1. J. Biol. Chem. 295, 16267–16279. doi: 10.1074/jbc.ra120.014591
Ponte, P., Gonzalez-DeWhitt, P., Schilling, J., Miller, J., Hsu, D., Greenberg, B., et al. (1988). A new A4 amyloid mRNA contains a domain homologous to serine proteinase inhibitors. Nature 331, 525–527. doi: 10.1038/331525a0
Preston, M., and Sherman, L. S. (2011). Neural stem cell niches: roles for the hyaluronan-based extracellular matrix. Front. Biosci. (Schol. Ed.) 3:1165–1179. doi: 10.2741/218
Quraishe, S., Forbes, L. H., and Andrews, M. R. (2018). The extracellular environment of the CNS: influence on plasticity, sprouting, and axonal regeneration after spinal cord injury. Neural Plast. 2018:2952386.
Rajasekharan, S., and Kennedy, T. E. (2009). The netrin protein family. Genome Biol. 10:239. doi: 10.1186/gb-2009-10-9-239
Rankin-Gee, E., McRae, P. A., Baranov, E., Rogers, S., Wandrey, L., and Porter, B. E. (2015). Perineuronal net degradation in epilepsy. Epilepsia 56, 1124–4433. doi: 10.1111/epi.13026
Rauch, U., Feng, K., and Zhou, X. H. (2001). Neurocan: a brain chondroitin sulfate proteoglycan. Cell. Mol. Life Sci. 58, 1842–1856. doi: 10.1007/pl00000822
Raum, H., Dietsche, B., Nagels, A., Witt, S. H., Rietschel, M., Kircher, T., et al. (2015). A genome-wide supported psychiatric risk variant in NCAN influences brain function and cognitive performance in healthy subjects. Hum. Brain Mapp. 36, 378–390. doi: 10.1002/hbm.22635
Reichsman, F., Smith, L., and Cumberledge, S. (1996). Glycosaminoglycans can modulate extracellular localization of the wingless protein and promote signal transduction. J. Cell Biol. 135, 809–827.
Ren, W., Zhang, Y., Li, M., Wu, L., Wang, G., Baeg, G. H., et al. (2015). Windpipe controls Drosophila intestinal homeostasis by regulating JAK/STAT pathway via promoting receptor endocytosis and lysosomal degradation. PLoS Genet. 11:e1005180. doi: 10.1371/journal.pgen.1005180
Rhiner, C., Gysi, S., Fröhli, E., Hengartner, M. O., and Hajnal, A. (2005). Syndecan regulates cell migration and axon guidance in C. elegans. Development 132, 4621–4633. doi: 10.1242/dev.02042
Roppongi, R., Dhume, S. H., Padmanabhan, N., Silwal, P., Zahra, N., Karimi, B., et al. (2020). LRRTMs organize synapses through differential engagement of neurexin and PTPσ. Neuron 106, 108–125.e112.
Roy, A., Attarha, S., Weishaupt, H., Edqvist, P. H., Swartling, F. J., Bergqvist, M., et al. (2017). Serglycin as a potential biomarker for glioma: association of serglycin expression, extent of mast cell recruitment and glioblastoma progression. Oncotarget 8, 24815–24827. doi: 10.18632/oncotarget.15820
Saied-Santiago, K., Townley, R. A., Attonito, J. D., da Cunha, D. S., Díaz-Balzac, C. A., Tecle, E., et al. (2017). Coordination of heparan sulfate proteoglycans with Wnt signaling to control cellular migrations and positioning in Caenorhabditis elegans. Genetics 206, 1951–1967. doi: 10.1534/genetics.116.198739
Sakamoto, K., Ozaki, T., Suzuki, Y., and Kadomatsu, K. (2021). Type IIa RPTPs and glycans: roles in axon regeneration and synaptogenesis. Int. J. Mol. Sci. 22:5524. doi: 10.3390/ijms22115524
Sakry, D., and Trotter, J. (2016). The role of the NG2 proteoglycan in OPC and CNS network function. Brain Res. 1638, 161–166. doi: 10.1016/j.brainres.2015.06.003
Sandbrink, R., Masters, C. L., and Beyreuther, K. (1996). APP gene family. Alternative splicing generates functionally related isoforms. Ann. N. Y. Acad. Sci. 777, 281–287. doi: 10.1111/j.1749-6632.1996.tb34433.x
Sarrazin, S., Adam, E., Lyon, M., Depontieu, F., Motte, V., Landolfi, C., et al. (2006). Endocan or endothelial cell specific molecule-1 (ESM-1): a potential novel endothelial cell marker and a new target for cancer therapy. Biochim. Biophys. Acta 1765, 25–37. doi: 10.1016/j.bbcan.2005.08.004
Sato, Y., Kiyozumi, D., Futaki, S., Nakano, I., Shimono, C., Kaneko, N., et al. (2019). Ventricular-subventricular zone fractones are speckled basement membranes that function as a neural stem cell niche. Mol. Biol. Cell 30, 56–68. doi: 10.1091/mbc.e18-05-0286
Schäfer, M., and Tegeder, I. (2018). NG2/CSPG4 and progranulin in the posttraumatic glial scar. Matrix Biol. 6, 571–588. doi: 10.1016/j.matbio.2017.10.002
Schafer, W. (2018). The worm connectome: back to the future. Trends Neurosci. 41, 763–765. doi: 10.1016/j.tins.2018.09.002
Scherpereel, A., Gentina, T., Grigoriu, B., Sénéchal, S., Janin, A., Tsicopoulos, A., et al. (2003). Overexpression of endocan induces tumor formation. Cancer Res. 63, 6084–6089.
Schmalfeldt, M., Dours-Zimmermann, M. T., Winterhalter, K. H., and Zimmermann, D. R. (1998). Versican V2 is a major extracellular matrix component of the mature bovine brain. J. Biol. Chem. 273, 15758–15764. doi: 10.1074/jbc.273.25.15758
Schmidt, S., Arendt, T., Morawski, M., and Sonntag, M. (2020). Neurocan contributes to perineuronal net development. Neuroscience 442, 69–86. doi: 10.1016/j.neuroscience.2020.06.040
Schmitt, M. (2016). Versican vs versikine: tolerance vs attack. Blood 128, 612–613. doi: 10.1182/blood-2016-06-721092
Schnepp, A., Komp Lindgren, P., Hülsmann, H., Kröger, S., Paulsson, M., and Hartmann, U. (2005). Mouse testican-2. Expression, glycosylation, and effects on neurite outgrowth. J. Biol. Chem. 280, 1274–1280.
Schönherr, E., Sunderkötter, C., Iozzo, R. V., and Schaefer, L. (2005). Decorin, a novel player in the insulin-like growth factor system. J. Biol. Chem. 280, 15767–15772. doi: 10.1074/jbc.m500451200
Schultz, C., Mühleisen, T. W., Nenadic, I., Koch, K., Wagner, G., Schachtzabel, C., et al. (2014). Common variation in NCAN, a risk factor for bipolar disorder and schizophrenia, influences local cortical folding in schizophrenia. Psychol. Med. 44, 811–820. doi: 10.1017/s0033291713001414
Selberg, J., Jia, M., and Rolandi, M. (2019). Proton conductivity of glycosaminoglycans. PLoS One. 14:e0202713. doi: 10.1371/journal.pone.0202713
Shabani, Z., Ghadiri, T., Karimipour, M., Sadigh-Eteghad, S., Mahmoudi, J., Mehrad, H., et al. (2021). Modulatory properties of extracellular matrix glycosaminoglycans and proteoglycans on neural stem cells behavior: highlights on regenerative potential and bioactivity. Int. J. Biol. Macromol. 171, 366–381. doi: 10.1016/j.ijbiomac.2021.01.006
Sherman, L., Matsumoto, S., Su, W., Srivastava, T., and Back, S. A. (2015). Hyaluronan synthesis, catabolism, and signaling in neurodegenerative diseases. Int. J. Cell Biol. 2015:368584.
Shimbo, M., Ando, S., Sugiura, N., Kimata, K., and Ichijo, H. (2013). Moderate repulsive effects of E-unit-containing chondroitin sulfate (CSE) on behavior of retinal growth cones. Brain Res. 1491, 34–43. doi: 10.1016/j.brainres.2012.11.011
Shioi, J., Pangalos, M. N., Ripellino, J. A., Vassilacopoulou, D., Mytilineou, C., Margolis, R. U., et al. (1995). The Alzheimer amyloid precursor proteoglycan (appican) is present in brain and is produced by astrocytes but not by neurons in primary neural cultures. J. Biol. Chem. 270, 11839–11844. doi: 10.1074/jbc.270.20.11839
Shuo, T., Aono, S., Matsui, F., Tokita, Y., Maeda, H., Shimada, K., et al. (2004). Developmental changes in the biochemical and immunological characters of the carbohydrate moiety of neuroglycan C, a brain-specific chondroitin sulfate proteoglycan. Glycoconj. J. 20, 267–278. doi: 10.1023/b:glyc.0000025821.22618.33
Shuo, T., Aono, S., Nakanishi, K., Tokita, Y., Kuroda, Y., Ida, M., et al. (2007). Ectodomain shedding of neuroglycan C, a brain-specific chondroitin sulfate proteoglycan, by TIMP-2- and TIMP-3-sensitive proteolysis. J. Neurochem. 102, 1561–1568. doi: 10.1111/j.1471-4159.2007.04658.x
Singh, J., Noorbaloochi, S., MacDonald, R., and Maxwell, L. J. (2015). Chondroitin for osteoarthritis. Cochrane Database Syst. Rev. 1:CD005614.
So, H., Fong, P. Y., Chen, R. Y., Hui, T. C., Ng, M. Y., Cherny, S. S., et al. (2010). Identification of neuroglycan C and interacting partners as potential susceptibility genes for schizophrenia in a Southern Chinese population. Am. J. Med. Genet. B Neuropsychiatr. Genet. 153B, 103–113.
Soleman, S., Filippov, M. A., Dityatev, A., and Fawcett, J. W. (2013). Targeting the neural extracellular matrix in neurological disorders. Neuroscience 253, 194–213. doi: 10.1016/j.neuroscience.2013.08.050
Sonntag, M., Blosa, M., Schmidt, S., Rübsamen, R., and Morawski, M. (2015). Perineuronal nets in the auditory system. Hear Res. 329, 21–32. doi: 10.1016/j.heares.2014.12.012
Sorg, B., Berretta, S., Blacktop, J. M., Fawcett, J. W., Kitagawa, H., Kwok, J. C., et al. (2016). Casting a wide net: role of perineuronal nets in neural plasticity. J. Neurosci. 36, 11459–11468. doi: 10.1523/jneurosci.2351-16.2016
Stanley, P. (2016). What have we learned from glycosyltransferase knockouts in mice? J. Mol. Biol. 428, 3166–3182. doi: 10.1016/j.jmb.2016.03.025
Starich, T., Xu, J., Skerrett, I. M., Nicholson, B. J., and Shaw, J. E. (2009). Interactions between innexins UNC-7 and UNC-9 mediate electrical synapse specificity in the Caenorhabditis elegans locomotory nervous system. Neural Dev. 11:16. doi: 10.1186/1749-8104-4-16
Steigemann, P., Molitor, A., Fellert, S., Jäckle, H., and Vorbrüggen, G. (2004). Heparan sulfate proteoglycan syndecan promotes axonal and myotube guidance by slit/robo signaling. Curr. Biol. 14, 225–230. doi: 10.1016/j.cub.2004.01.006
Stichel, C., Kappler, J., Junghans, U., Koops, A., Kresse, H., and Müller, H. W. (1995). Differential expression of the small chondroitin/dermatan sulfate proteoglycans decorin and biglycan after injury of the adult rat brain. Brain Res. 704, 263–274. doi: 10.1016/0006-8993(95)01131-5
Strbak, O., Kanuchova, Z., and Krafcik, A. (2016). Proton gradients as a key physical factor in the evolution of the forced transport mechanism across the lipid membrane. Orig. Life Evol. Biosph. 46, 523–531. doi: 10.1007/s11084-016-9496-z
Streit, A., Nolte, C., Rásony, T., and Schachner, M. (1993). Interaction of astrochondrin with extracellular matrix components and its involvement in astrocyte process formation and cerebellar granule cell migration. J. Cell Biol. 120, 799–814. doi: 10.1083/jcb.120.3.799
Su, M., Soomro, S. H., Jie, J., and Fu, H. (2021). Effects of the extracellular matrix on myelin development and regeneration in the central nervous system. Tissue Cell 69:101444. doi: 10.1016/j.tice.2020.101444
Sugahara, K., Mikami, T., Uyama, T., Mizuguchi, S., Nomura, K., and Kitagawa, H. (2003). Recent advances in the structural biology of chondroitin sulfate and dermatan sulfate. Curr. Opin. Struct. Biol. 13, 612–620. doi: 10.1016/j.sbi.2003.09.011
Sugitani, K., Egorova, D., Mizumoto, S., Nishio, S., Yamada, S., Kitagawa, H., et al. (2020). Hyaluronan degradation and release of a hyaluronan-aggrecan complex from perineuronal nets in the aged mouse brain. Biochim. Biophys. Acta Gen. Subj. 1865:129804. doi: 10.1016/j.bbagen.2020.129804
Sullivan, C., Gotthard, I., Wyatt, E. V., Bongu, S., Mohan, V., Weinberg, R. J., et al. (2018). Perineuronal net protein neurocan inhibits NCAM/EphA3 repellent signaling in GABAergic interneurons. Sci. Rep. 8:6143.
Sun, H., Zhang, H., Li, K., Wu, H., Zhan, X., Fang, F., et al. (2019). ESM-1 promotes adhesion between monocytes and endothelial cells under intermittent hypoxia. J. Cell Physiol. 234, 1512–1521. doi: 10.1002/jcp.27016
Takano, M., Mori, Y., Shiraki, H., Horie, M., Okamoto, H., Narahara, M., et al. (1999). Detection of bikunin mRNA in limited portions of rat brain. Life Sci. 65, 757–762. doi: 10.1016/s0024-3205(99)00302-1
Takemura, M., Noborn, F., Nilsson, J., Bowden, N., Nakato, E., Baker, S., et al. (2020). Chondroitin sulfate proteoglycan Windpipe modulates Hedgehog signaling in Drosophila. Mol. Biol. Cell 31, 813–824. doi: 10.1091/mbc.e19-06-0327
Tamburini, E., Dallatomasina, A., Quartararo, J., Cortelazzi, B., Mangieri, D., Lazzaretti, M., et al. (2019). Structural deciphering of the NG2/CSPG4 proteoglycan multifunctionality. FASEB J. 33, 3112–3128. doi: 10.1096/fj.201801670r
Tauchi, R., Imagama, S., Natori, T., Ohgomori, T., Muramoto, A., Shinjo, R., et al. (2012). The endogenous proteoglycan-degrading enzyme ADAMTS-4 promotes functional recovery after spinal cord injury. J. Neuroinflammation 9:53.
Teuscher, A., Jongsma, E., Davis, M. N., Statzer, C., Gebauer, J. M., Naba, A., et al. (2019). The in-silico characterization of the Caenorhabditis elegans matrisome and proposal of a novel collagen classification. Matrix Biol. Plus 1:100001. doi: 10.1016/j.mbplus.2018.11.001
Thelin, M., Bartolini, B., Axelsson, J., Gustafsson, R., Tykesson, E., Pera, E., et al. (2013). Biological functions of iduronic acid in chondroitin/dermatan sulfate. FEBS J. 280, 2431–2446. doi: 10.1111/febs.12214
Thinakaran, G., and Sisodia, S. S. (1994). Amyloid precursor-like protein 2 (APLP2) is modified by the addition of chondroitin sulfate glycosaminoglycan at a single site. J. Biol. Chem. 269, 22099–22104. doi: 10.1016/s0021-9258(17)31761-1
Thinakaran, G., Slunt, H. H., and Sisodia, S. S. (1995). Novel regulation of chondroitin sulfate glycosaminoglycan modification of amyloid precursor protein and its homologue, APLP2. J. Biol. Chem. 270, 16522–16525. doi: 10.1074/jbc.270.28.16522
Tsai, J., Zhang, J., Minami, T., Voland, C., Zhao, S., Yi, X., et al. (2002). Cloning and characterization of the human lung endothelial-cell-specific molecule-1 promoter. J. Vasc. Res. 39, 148–159. doi: 10.1159/000057763
Tu, H., Pinan-Lucarré, B., Ji, T., Jospin, M., and Bessereau, J. L. (2015). C. elegans punctin clusters GABA(A) receptors via neuroligin binding and UNC-40/DCC recruitment. Neuron 86, 1407–1419. doi: 10.1016/j.neuron.2015.05.013
Tykesson, E., Hassinen, A., Zielinska, K., Thelin, M. A., Frati, G., Ellervik, U., et al. (2018). Dermatan sulfate epimerase 1 and dermatan 4-O-sulfotransferase 1 form complexes that generate long epimerized 4-O-sulfated blocks. J. Biol. Chem. 293, 13725–13735. doi: 10.1074/jbc.ra118.003875
Vale, W., Wiater, E., Gray, P., Harrison, C., Bilezikjian, L., and Choe, S. (2004). Activins and inhibins and their signaling. Ann. N. Y. Acad. Sci. 1038, 142–147.
Vassallo, V., Stellavato, A., Cimini, D., Pirozzi, A. V. A., Alfano, A., Cammarota, M., et al. (2021). Unsulfated biotechnological chondroitin by itself as well as in combination with high molecular weight hyaluronan improves the inflammation profile in osteoarthritis in vitro model. J. Cell Biochem. doi: 10.1002/jcb.29907
Vellai, T., Takács, K., and Vida, G. (1998). A new aspect to the origin and evolution of eukaryotes. J. Mol. Evol. 46, 499–507. doi: 10.1007/pl00006331
Viapiano, M., Hockfield, S., and Matthews, R. T. (2008). BEHAB/brevican requires ADAMTS-mediated proteolytic cleavage to promote glioma invasion. J. Neurooncol. 88, 261–272. doi: 10.1007/s11060-008-9575-8
Walimbe, A., Okuma, H., Joseph, S., Yang, T., Yonekawa, T., Hord, J. M., et al. (2020). POMK regulates dystroglycan function via LARGE1-mediated elongation of matriglycan. Elife 9:e61388.
Wang, L., Cheng, B. F., Yang, H. J., Wang, M., and Feng, Z. W. (2015). NF-κB protects human neuroblastoma cells from nitric oxide-induced apoptosis through upregulating biglycan. Am. J. Transl. Res. 7, 1541–1552.
Watanabe, E., Maeda, N., Matsui, F., Kushima, Y., Noda, M., and Oohira, A. (1995). Neuroglycan C, a novel membrane-spanning chondroitin sulfate proteoglycan that is restricted to the brain. J. Biol. Chem. 270, 26876–26882. doi: 10.1074/jbc.270.45.26876
Weiskopf, K. (2017). Cancer immunotherapy targeting the CD47/SIRPα axis. Eur. J. Cancer 76, 100–109. doi: 10.1016/j.ejca.2017.02.013
Wen, T., Binder, D. K., Ethell, I. M., and Razak, K. A. (2018). The perineuronal ‘safety’ net? Perineuronal net abnormalities in neurological disorders. Front. Mol. Neurosci. 11:270. doi: 10.3389/fnmol.2018.00270
Whalen, D., Malinauskas, T., Gilbert, R. J., and Siebold, C. (2013). Structural insights into proteoglycan-shaped Hedgehog signaling. Proc. Natl. Acad. Sci. U.S.A. 110, 16420–16425. doi: 10.1073/pnas.1310097110
White, J., Southgate, E., Thomson, J. N., and Brenner, S. (1976). Factors which determine connectivity in the nervous system of C. elegans. Cold Spring Harb. Symp. Quant. Biol. 48:633640.
White, J., Southgate, E., Thomson, J. N., and Brenner, S. (1986). The structure of the nervous system of the nematode Caenorhabditis elegans. Philos. Trans. R. Soc. Lond. B Biol. Sci. 314, 1–340. doi: 10.1098/rstb.1986.0056
Wigley, R., Hamilton, N., Nishiyama, A., Kirchhoff, F., and Butt, A. M. (2007). Morphological and physiological interactions of NG2-glia with astrocytes and neurons. J. Anat. 10, 661–670. doi: 10.1111/j.1469-7580.2007.00729.x
Wilson, E., Knudson, W., and Newell-Litwa, K. (2020). Hyaluronan regulates synapse formation and function in developing neural networks. Sci. Rep. 10:16459.
Wilson, T., and Lin, E. C. (1980). Evolution of membrane bioenergetics. J. Supramol. Struct. 13, 421–446. doi: 10.1002/jss.400130403
Woodruff, T. (1998). Regulation of cellular and system function by activin. Biochem. Pharmacol. 55, 953–963. doi: 10.1016/s0006-2952(97)00477-2
Wu, Y., Sheng, W., Chen, L., Dong, H., Lee, V., Lu, F., et al. (2004). Versican V1 isoform induces neuronal differentiation and promotes neurite outgrowth. Mol. Biol. Cell 15, 2093–2104. doi: 10.1091/mbc.e03-09-0667
Wu, Y., Zhang, Y., Cao, L., Chen, L., Lee, V., Zheng, P. S., et al. (2001). Identification of the motif in versican G3 domain that plays a dominant-negative effect on astrocytoma cell proliferation through inhibiting versican secretion and binding. J. Biol. Chem. 276, 14178–14186. doi: 10.1074/jbc.m100618200
Xiang, Y., Dong, H., Wan, Y., Li, J., Yee, A., Yang, B. B., et al. (2006). Versican G3 domain regulates neurite growth and synaptic transmission of hippocampal neurons by activation of epidermal growth factor receptor. J. Biol. Chem. 281, 19358–19368. doi: 10.1074/jbc.m512980200
Xie, Y., Peng, J., Pang, J., Guo, K., Zhang, L., Yin, S., et al. (2020). Biglycan regulates neuroinflammation by promoting M1 microglial activation in early brain injury after experimental subarachnoid hemorrhage. J. Neurochem. 152, 368–380. doi: 10.1111/jnc.14926
Xing, Y., Wang, Y., Wang, S., Wang, X., Fan, D., Zhou, D., et al. (2016). Human cytomegalovirus infection contributes to glioma disease progression via upregulating endocan expression. Transl. Res. 177, 103–126.
Xiong, A., Spyrou, A., and Forsberg-Nilsson, K. (2020). Involvement of heparan sulfate and heparanase in neural development and pathogenesis of brain tumors. Adv. Exp. Med. Biol. 1221, 365–403. doi: 10.1007/978-3-030-34521-1_14
Yamada, S., Sugahara, K., and Ozbek, S. (2011). Evolution of glycosaminoglycans: comparative biochemical study. Commun. Integr. Biol. 4, 150–158. doi: 10.4161/cib.4.2.14547
Yamaguchi, Y. (1996). Brevican: a major proteoglycan in adult brain. Perspect. Dev. Neurobiol. 3, 307–317.
Yamaguchi, Y. (2000). Lecticans: organizers of the brain extracellular matrix. Cell. Mol. Life Sci. 57, 276–289. doi: 10.1007/pl00000690
Yang, W., and Yee, A. J. (2013). Versican V2 isoform enhances angiogenesis by regulating endothelial cell activities and fibronectin expression. FEBS Lett. 587, 185–192. doi: 10.1016/j.febslet.2012.11.023
Yang, X. (2020). Chondroitin sulfate proteoglycans: key modulators of neuronal plasticity, long-term memory, neurodegenerative, and psychiatric disorders. Rev. Neurosci. 31, 555–568. doi: 10.1515/revneuro-2019-0117
Yang, Y., Lee, W. S., Tang, X., and Wadsworth, W. G. (2014). Extracellular matrix regulates UNC-6 (netrin) axon guidance by controlling the direction of intracellular UNC-40 (DCC) outgrowth activity. PLoS One 9:e97258. doi: 10.1371/journal.pone.0097258
Yao, T., Zhang, C. G., Gong, M. T., Zhang, M., Wang, L., and Ding, W. (2016). Decorin-mediated inhibition of the migration of U87MG glioma cells involves activation of autophagy and suppression of TGF-β signaling. FEBS Open Bio 31, 707–719. doi: 10.1002/2211-5463.12076
Yasuda, Y., Tokita, Y., Aono, S., Matsui, F., Ono, T., Sonta, S., et al. (1998). Cloning and chromosomal mapping of the human gene of neuroglycan C (NGC), a neural transmembrane chondroitin sulfate proteoglycan with an EGF module. Neurosci. Res. 32, 313–322. doi: 10.1016/s0168-0102(98)00098-4
Ye, F., Hua, Y., Keep, R. F., Xi, G., and Garton, H. J. L. (2021). CD47 blocking antibody accelerates hematoma clearance and alleviates hydrocephalus after experimental intraventricular hemorrhage. Neurobiol. Dis. 155:105384. doi: 10.1016/j.nbd.2021.105384
Ying, Z., Byun, H. R., Meng, Q., Noble, E., Zhang, G., Yang, X., et al. (2018). Biglycan gene connects metabolic dysfunction with brain disorder. Biochim. Biophys. Acta Mol. Basis Dis. 1864, 3679–3687. doi: 10.1016/j.bbadis.2018.10.002
Yousef, M., and Matthews, B. W. (2005). Structural basis of Prospero-DNA interaction: implications for transcription regulation in developing cells. Structure 13, 601–607. doi: 10.1016/j.str.2005.01.023
Zhang, H., Li, F., Yang, Y., Chen, J., and Hu, X. (2015). SIRP/CD47 signaling in neurological disorders. Brain Res. 1623, 74–80. doi: 10.1016/j.brainres.2015.03.012
Zhang, L. (2010). Glycosaminoglycan (GAG) biosynthesis and GAG-binding proteins. Prog. Mol. Biol. Transl. Sci. 93, 1–17. doi: 10.1016/s1877-1173(10)93001-9
Zhang, P., Karani, R., Turner, R. L., Dufresne, C., Ferri, S., Van Eyk, J. E., et al. (2016). The proteome of normal human retrobulbar optic nerve and sclera. Proteomics 16, 2592–2596. doi: 10.1002/pmic.201600229
Zhang, P., Lu, H., Peixoto, R. T., Pines, M. K., Ge, Y., Oku, S., et al. (2018). Heparan sulfate organizes neuronal synapses through neurexin partnerships. Cell 174, 1450–1464.e23.
Zhang, W., Ge, Y., Cheng, Q., Zhang, Q., Fang, L., and Zheng, J. (2018). Decorin is a pivotal effector in the extracellular matrix and tumour microenvironment. Oncotarget 9, 5480–5491. doi: 10.18632/oncotarget.23869
Zhang, W., Huang, Q., Xiao, W., Zhao, Y., Pi, J., Xu, H., et al. (2020). Advances in anti-tumor treatments targeting the CD47/SIRPα axis. Front. Immunol. 11:18. doi: 10.3389/fimmu.2020.00018
Zhang, X., Lee, S. J., Young, M. F., and Wang, M. M. (2015). The small leucine-rich proteoglycan BGN accumulates in CADASIL and binds to NOTCH3. Transl. Stroke Res. 6, 148–155. doi: 10.1007/s12975-014-0379-1
Keywords: chondroitin sulfate, dermatan sulfate, proteoglycans, lecticans, neuroregulation, neural repair
Citation: Hayes AJ and Melrose J (2021) Neural Tissue Homeostasis and Repair Is Regulated via CS and DS Proteoglycan Motifs. Front. Cell Dev. Biol. 9:696640. doi: 10.3389/fcell.2021.696640
Received: 19 April 2021; Accepted: 13 July 2021;
Published: 02 August 2021.
Edited by:
Jessica C. F. Kwok, University of Leeds, United KingdomReviewed by:
Jun Zhou, German Cancer Research Center (DKFZ), GermanyReyna Hernandez-Benitez, Salk Institute for Biological Studies, United States
Copyright © 2021 Hayes and Melrose. This is an open-access article distributed under the terms of the Creative Commons Attribution License (CC BY). The use, distribution or reproduction in other forums is permitted, provided the original author(s) and the copyright owner(s) are credited and that the original publication in this journal is cited, in accordance with accepted academic practice. No use, distribution or reproduction is permitted which does not comply with these terms.
*Correspondence: James Melrose, amFtZXMubWVscm9zZUBzeWRuZXkuZWR1LmF1; orcid.org/0000-0001-9237-0524