- College of Animal Sciences, Zhejiang Provincial Key Laboratory of Preventive Veterinary Medicine, Institute of Preventive Veterinary Medicine, Zhejiang University, Hangzhou, China
Molting is of great importance for the survival and development of nematodes. Nematode astacins (NAS), a large family of zinc metalloproteases, have been proposed as novel anthelmintic targets due to their multiple roles in biological processes of parasitic nematodes. In this study, we report a well conserved nas-33 gene in nematodes of clade V and elucidate how this gene is involved in the molting process of the free-living nematode Caenorhabditis elegans and the parasitic nematode Haemonchus contortus. A predominant transcription of nas-33 is detected in the larval stages of these worms, particularly in the molting process. Knockdown of this gene results in marked molecular changes of genes involved in cuticle synthesis and ecdysis, compromised shedding of the old cuticle, and reduced worm viability in H. contortus. The crucial role of nas-33 in molting is closely associated with a G protein beta subunit (GPB-1). Suppression of both nas-33 and gpb-1 blocks shedding of the old cuticle, compromises the connection between the cuticle and hypodermis, and leads to an increased number of sick and dead worms, indicating essentiality of this module in nematode development and survival. These findings reveal the functional role of nas-33 in nematode molting process and identify astacins as novel anthelmintic targets for parasitic nematodes of socioeconomic significance.
Introduction
Both free-living and parasitic nematodes develop through four to five larval stages, which are distinguishable by their different size and separated by the temporal shedding of cuticle (i.e., molting). The cuticle is a crucial structure that maintains post-embryonic body shape, acts as an exoskeleton, and permits mobility and elasticity of nematodes (Bird and Bird, 1991; Page and Johnstone, 2007), whereas molting is a series of biological processes including separation of the surface coat from the epidermis (i.e., apolysis), synthesis of a new cuticle during an inactive stage (i.e., lethargus), and shedding of the old cuticle (i.e., ecdysis) (Page, 2001). Proper synthesis of cuticle and regular molting between two life stages are essential for the survival and development of nematodes in the environment or within host animals. In particular, the cuticle of parasitic nematodes is the interface of host-parasite interactions, playing roles in immune recognition and immune evasion within host animals (Maizels, 2013). A wealth of information about the cuticle and the molting process is now available for nematodes, predominantly based on the nematode model organism Caenorhabditis elegans (Politz and Philipp, 1992).
Molecules involved in molting include zinc metalloproteases, leucine amino-peptidases and cysteine proteases (Rogers, 1982; Gamble et al., 1989; Lustigman, 1993). Astacins are a large family of zinc metalloproteases belonging to the M12A family, which was first reported in the crayfish Astacus astacus (Pfleiderer et al., 1967; Mohrlen et al., 2006). In C. elegans, there are 40 genes coding for nematode astacins (nas), representing six subgroups (I, II, III, IV, V, and VI) of astacin-like proteins based on the deduced domain architectures (Mohrlen et al., 2003). These molecules have been reported predominantly expressed in the pharynx, intestine, body wall muscle and hypodermis, with a few of them expressed in neurons and the reproductive tissues of C. elegans (Park et al., 2010). Members of the astacin family exhibit numerous physiological functions in hatching, digestion, peptide processing and pattern formation. In particular, dpy-31 (also known as nas-35), nas-36 and nas-37 have been reported to be involved in the nematode molting process (Maeda et al., 2001; Kamath et al., 2003). Specifically, worms lacking dpy-31/nas-35 showed a dumpy appearance (Novelli et al., 2004, 2006), whereas suppression of nas-36 and nas-37 led to molting defect and temperature-sensitive lethal phenotype (Davis et al., 2004; Suzuki et al., 2004). By contrast, only a few astacin-coding genes have been identified in parasites, such as Brugia malayi (a filarial worm of medical importance), Haemonchus contortus and Teladorsagia circumcincta (highly pathogenic worms of veterinary significance) (Stepek et al., 2010, 2011), and little is known about their functional details in parasitic nematodes. Nonetheless, chemical inhibition of DPY-31/NAS-35 in these parasitic nematodes elicited severe dumpy and immobile phenotypes (France et al., 2015; Stepek et al., 2015), suggesting the possibilities of NAS as drug targets in major parasitic worms of socioeconomic importance. Protease inhibitors that can specifically bind to certain proteases have been used in the therapeutic treatment of parasitic diseases (Shamsi et al., 2016; Deu, 2017). For instance, vinyl sulfone cysteine protease inhibitor K11777 (a substrate-based inhibitor of the gut-associated cathepsin B1 cysteine protease) showed significant efficacy on schistosomiasis in murine model (Abdulla et al., 2007). In addition, screening of inhibitory compounds targeting essential proteases has been an emerging area for Plasmodium falciparum (Sharma et al., 2015; Roy, 2017; Singh et al., 2021). Therefore, a better understanding of the functional roles of nas genes in free-living and parasitic nematodes should underpin the biological understanding of this metalloprotease-coding gene and lay a basis for the discovery of novel interventions.
In this study, we report a zinc metalloprotease coding gene nas-33 that is well conserved in clade V parasitic nematodes, and elucidate the essential roles of this gene in molting process in the free-living nematode C. elegans and the parasitic nematode H. contortus. Novel insights into the essentiality of nas-33, which represents a conserved nematode-specific gene family, should lay a solid foundation for the discovery and development of novel anthelmintics.
Materials and Methods
Nematodes
Caenorhabditis elegans N2 strain was acquired from the Caenorhabditis Genetics Center (CGC), maintained on nematode growth media (NGM) plates at 20°C following the standard protocol (Brenner, 1974). Gravid worms were bleached with hypochlorite solution to collect eggs, which were then incubated in M9 buffer on a rotator for 24 h at 20°C to synchronize all animals at the first larval (L1) stage (Sulston and Hodgkin, 1988). By contrast, H. contortus (ZJ strain; anthelmintic susceptible) were maintained in Hu sheep under a helminth-free condition as described previously (Yan et al., 2014). Adult worms were collected from the abomasa of infected sheep, and eggs were isolated from the uteri of adult female worms, placed on 2% agar plates and cultured at 28°C for 7 days to synchronize all worms at the third larval (L3) stage.
Molecular Cloning and Sequence Analysis
Genomic DNA and total RNA were extracted from the adult worms of H. contortus using a TIANamp Genomic DNA kit (Tiangen Biotech Co., Ltd., Beijing) and the Trizol reagent (Invitrogen, United States), respectively. The first strand cDNA was synthesized using a First Strand cDNA Synthesis Kit (Toyobo Co., Ltd., Japan). Rapid amplification of cDNA ends (RACE) was conducted using the 5′- and 3′-Full RACE kit (Takara Biotechnology Co., Ltd.) to extend a sequence fragment HCISE01811400.t1 in the Sanger database1, a potential nas-33 homolog in H. contortus. PCR products were cloned into a pMD19-T vector and sequenced. Based on the obtained sequence, primers were designed to perform a Genome Walking experiment to acquire the flanking sequences. Primers used were listed in Supplementary Table 1. Functional domain predictions were carried out by searching the predicted amino acid sequences against NCBI2 and InterPro3 databases. Sequence alignment and phylogenetic analyses were performed using MEGA5.
Quantitative Real-Time PCR (qRT-PCR)
Arrested L1s of C. elegans were placed on NGM plates seeded with Escherichia coli strain OP50 and cultured at 20°C. Nematode samples were collected every 2 h until 40 h post incubation for RNA extraction. Transcriptional alteration of Ce-nas-33 during the development was determined by qRT-PCR using SYBR® Green PCR Master Mix (Toyobo, Japan) on a T100 Real-Time PCR System (Bio-Rad, United States). Actin coding gene act-1 was used as an internal control, and cathepsin L-like cysteine protease coding gene cpl-1, nas-37 (apolysis), collagen coding gene col-12 (late lethargus), thioredoxin reductase coding gene trxr-1 or glutathionine reductase coding gene gsr-1 (ecdysis) were selected as markers for molting processes (Hashmi et al., 2002; Davis et al., 2004; Stenvall et al., 2011). Differently, synchronized L3s of H. contortus were used to orally infect sheep, and to collect ∼10,000 eggs, ∼8,000 L1s, 8,000 L2s, 6,000 L3s, 100 L4s and adults for RNA extraction. For each sample, 0.5-1 μg of total RNA was used to prepared cDNA for qRT-PCR performed to determine the transcriptional levels of Hc-nas-33 in different developmental stages of H. contortus. In particular, transcription of Hc-nas-33 in larvae during L1, L1-L2 molting, L2 and L2-L3 molting were measured. 18S rRNA was used as an internal control in H. contortus. Primer sets used can be found in Supplementary Table 1. All experiments were conducted at least three times, and the qRT-PCR data were analyzed using the 2–△Ct method.
RNA Interference (RNAi)
A feeding method was employed to conduct the RNAi assay on H. contortus (Zawadzki et al., 2012). Specific PCR primers were designed to amplify Hc-nas-33 (613–1,569 nt). The PCR products were cloned into the L4440 vector, then transformed into E. coli HT115 strain (DE3) cells. Beta-tubulin isotype-1 coding gene Hc-iso-1 was used as a positive control in H. contortus RNAi assays (Samarasinghe et al., 2011), whereas Bt-cry1Ac from Bacillus thuringiensis (GenBank Accession No. GU322939.1) was used as an “irrelevant” control. Primers used were listed in Supplementary Table 1. Eggs (n ≈ 4,000) of H. contortus were sterilized with antibiotic-antimycotic, washed thoroughly, and incubated with the transformed bacteria at 28°C for 6–10 days. Hatching rate and subsequent larval development of H. contortus were monitored under a microscope on days 1, 3, and 7. On day 3, ∼4,000 larvae were harvested for the extraction of RNA and synthesis of cDNA. Gene knockdown of Hc-nas-33 and associated transcriptional alterations of genes involved in cuticle synthesis (col-12, col-14, cuticle procollagen coding gene dpy-5 and dpy-13), ecdysis (serine/threonine protein kinase coding gene nekl-2, ankyrin repeat and sterile alpha motif domain containing protein coding gene mlt-3 and tropomyosin coding gene lev-11) and remodeling of cuticle-epidermis linkage (muscle attachment abnormal associated gene mua-3 and myotactin coding gene let-805) in RNAi-treated worms were assessed by qRT-PCR.
Yeast Two-Hybrid Screening
Total RNA was isolated from the L3s of H. contortus to construct a cDNA library, which was then transformed into the Y187 yeast strain. The full-length nas-33 cDNA was amplified and subcloned into pGBKT7, then transformed into yeast strain Y2H Gold. The NAS33 protein was used as a bait to screen the yeast cDNA library according to the manufacturer’s user guide (Matchmaker® Gold Yeast Two-Hybrid System User Manual). Clones grown on the SD/-Leu/-Trp/-His/-Ade plates were confirmed by further selection and used to extract plasmids for sequencing inserts. Among the candidate genes after library screening, one insert was predicted to encode a 321 amino acid polypeptide that shares homology with the guanine nucleotide-binding protein subunit beta-1, which was renamed here as Hc-gpb-1.
In vitro Pull-Down Assay
Bait protein (GST-fused Hc-NAS-33) was expressed using a Bac-to-Bac Baculovirus Expression System, immobilized with GST beads at 4°C for 4 h and then washed by phosphate buffer saline (PBS) containing 1% Triton X-100. Potential prey protein (HA-tagged Hc-GPB-1) was produced in HEK 293T cells transfected with pGEX-4t-1-Hc-gpb-1. Immobilized bait protein was incubated with 300 μl cell lysates containing HA-tagged protein at 4°C for 2 h, followed by washing with PBS. Protein-protein interaction complex was eluted, resuspended in 40 μl 2 × SDS loading buffer, boiled and separated by SDS-PAGE. Anti-GST and Anti-HA antibodies were used to analyze the interaction of Hc-NAS-33 and Hc-GPB-1.
Co-immunoprecipitation (Co-IP)
HA-tagged Hc-NAS-33 and FLAG-tagged Hc-GPB-1 proteins were prepared from transfected HEK 293T cells, then incubated with anti-FLAG agarose at 4°C for 2 h. After that, the proteins were washed with immunoprecipitation buffer (136.89 mM NaCl, 2.67 mM KCl, 8.1 mM Na2HPO4, 1.76 mM KH2PO4 and 0.5% Tween 20) for eight times, mixed with 50 μl 5 × SDS loading buffer and boiled for 10 min. Protein samples were separated by SDS-PAGE, and subjected to Western Blot analysis. Anti-HA and anti-FLAG antibodies were used to detect fused proteins Hc-NAS-33 and Hc-GPB-1, respectively. Co-IP of Hc-NAS-33 and Hc-GPB-1 with exchanged tags was also performed.
Co-localization
Promoters of Ce-nas-33 (sequence between K04E7.4 and Ce-nas-33 start codon) and Ce-gpb-1 (sequence between F44E5.14 and Ce-gpb-1 start codon), as well as sequences 2,000 nt upstream Hc-nas-33 and Hc-gpb-1 were used to drive gene expression of Hc-nas-33 and Hc-gpb-1 in C. elegans, respectively. Plasmid expressing Hc-NAS-33-GFP (pPD95_77-Cep-Hc-nas33) was constructed by inserting the promoter of Ce-nas-33 cloned from C. elegans DNA and Hc-nas-33 full-length cDNA into the germline expression vector pPD95_77 via BamH I and Kpn I restriction site successively. As the same, the promoter of Ce-gpb-1 was cloned into pPD95_77 at BamH I restriction site and Hc-gpb-1-mCherry overlapped sequence was inserted afterward to obtain plasmid expressing Hc-GPB-1-mCherry (pPD95_77-Cep-Hc-gpb1-mCherry). Recombinant plasmids were microinjected into the gonads of young adult worms as described previously (Mello et al., 1991), together with pRF4 plasmids (50 ng/μl) introducing mutant allele of rol-6 gene. F2 larvae with a roller phenotype were selected to examine the expression patterns of fusion proteins in the transgenic worms using a fluorescent microscope (Zeiss LSM 780). In addition, Hc-nas-33 and Hc-gpb-1 was cloned into C2-EGFP vector and pcDNA3 (+)-mCherry vector, respectively. Recombinant plasmids were co-transfected into the human embryonic kidney 293T (HEK 293T) cell line using Lipofectamine 2000 (Invitrogen). Transfected cells were further cultured at 37°C in 5% CO2 for 24–48 h, then stained with DAPI for 30 min at room temperature. GFP and mCherry expression in HEK 293T cells were analyzed using a fluorescent microscope (Zeiss LSM 780).
Transmission Electron Microscopy (TEM)
About 2000 RNAi-treated and -untreated worms were collected on day 5, washed in physiological saline and fixed in 2.5% glutaraldehyde and 1% Triton X-100 and 0.1 M sodium phosphate buffer at 4°C for 7 days. Fixed samples were mounted into agar blocks and postfixed in 1% OsO4 and 0.1 M sodium phosphate buffer for 2 h, and further processed for dehydration and infiltration. Processed specimens were placed in Eppendorf tubes containing Spurr resin and heated at 70°C overnight, then sectioned and stained with uranyl acetate for 5 min and alkaline for 10 min. TEM scanning was performed using a Hitachi Model H-7650 TEM microscope. Micrographs (n = 4) captured at the middle of worms were selected for analysis. Thickness of the worm cuticle was measured using the ImageJ.
Developmental and Survival Assay
Separate (Hc-nas-33 or Hc-gpb-1) and simultaneous (Hc-nas-33 + Hc-gpb-1) RNAi assays were conducted on the early larval stage of H. contortus using the feeding method described above. Gene knockdown analyses were performed to estimate the transcriptional association of these two genes in the treated worms, using qRT-PCR as described above. Primer sets used were listed in Supplementary Table 1. Developmental and survival variations of treated worms were assessed in terms of the morphology, molting and morbidity (i.e., sickness and death) of free-living L1s, L2s, and L3s (infective) of H. contortus. In brief, three sub-samples of 200 μl culture medium (containing about 200 larvae) were taken from the culturing system and transferred into 6-well culture plate on days 3 and 7. The treated larvae were examined by microscopy, in aspects of larval development and survival. Experiments were repeated for three times on different days.
Statistical Analysis
Data were presented as mean ± standard deviation (SD). Statistical analyses of gene transcription, cuticle thickness and larval development were carried out by Student’s t-test with the GraphPad Prism 8 (GraphPad Software, United States). P < 0.05 was considered as statistically significant difference.
Results
nas-33 Is Relatively Conserved in Free-Living and Parasitic Nematodes
Apart from C. elegans, orthologs of nas-33 were commonly identified in nematodes of clade V, including the free-living Pristionchus pacificus and the parasitic Ancylostoma caninum, A. ceylanicum, and H. contortus. Specifically, the cDNA-confirmed Hc-nas-33 was 1714 bp (GenBank accession No. MT891116) with a 66-bp 5′ UTR and a 79-bp 3′ UTR (Supplementary Figure 1), which encoded a protein containing a ZnMc-astacin_like domain, an EGF domain and a CUB domain (Figure 1A). Notably, there was a missing thrombospondin type-1 (TSP1) repeat in the deduced Hc-NAS-33, compared with the domain architecture of Ce-NAS-33 (Figure 1A). The alignment of protein sequence with other nematode homologs showed a relatively high similarity within the predicted domains (Supplementary Figure 2).
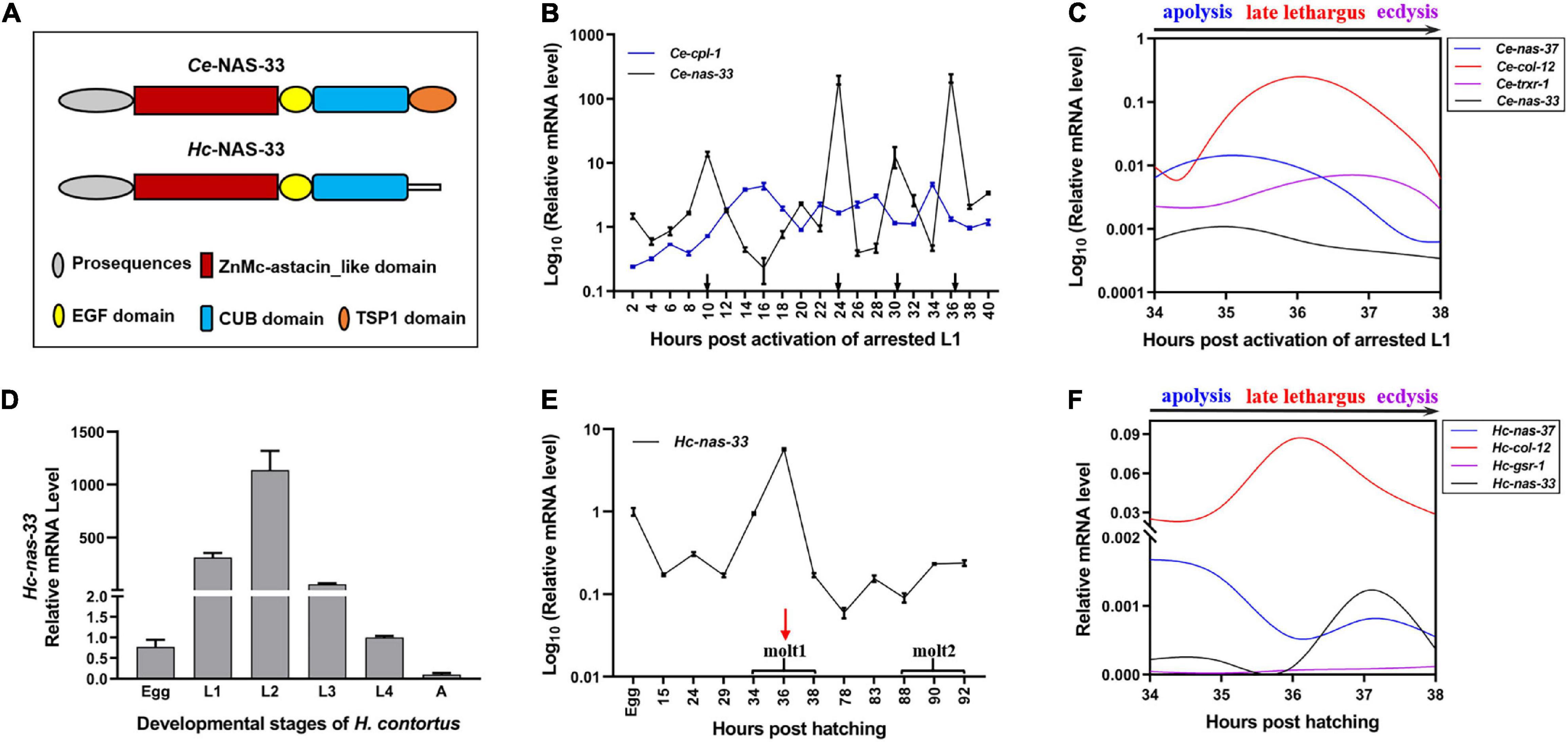
Figure 1. Developmental transcription analysis of nas-33 in Caenorhabditis elegans and Haemonchus contortus. (A) Schematic diagram for domain architectures of Ce-NAS-33 and Hc-NAS-33. (B) Ce-nas-33 shows a tightly regulated transcriptional pattern across four molting periods (black arrows) of C. elegans. (C) Transcriptional alterations of marker genes nas-37, col-12, trxr-1 for apolysis, late lethargus and ecdysis, and nas-33 during the molting of C. elegans. (D) Transcription levels of Hc-nas-33 in different development stages of H. contortus. (E) Hc-nas-33 shows a transcriptional peak during the first molting of H. contortus. (F) Transcriptional alterations of marker genes nas-37, col-12, gsr-1 for apolysis, late lethargus and ecdysis, and nas-33 during the first molting of H. contortus. Error bars indicate mean ± standard deviation (SD). A Non-linear Curve Fit is performed to indicate the dynamic transcriptional changes of marker genes involved in the molting processes.
nas-33 Is Highly Expressed During Late Lethargus
Developmental transcription analyses showed predominant gene expression of nas-33 in the larval (i.e., L1, L2, L3, and L4) stages of both C. elegans and H. contortus (Figures 1B,D). Specifically, four transcriptional peaks were identified for Ce-nas-33 across the development from the activated L1 stage to the adult stage of C. elegans (Figure 1B). In particular, Ce-nas-33 appeared to play a role in the late lethargus phase, with reference to the transcriptions of marker genes (i.e., nas-37, col-12, and trxr-1) for molting process (i.e., apolysis, late lethargus and ecdysis) (Figure 1C). A transcriptional peak was also found during the L1-L2 molting of H. contortus (Figure 1E). By contrast, unlike the peaked transcriptional level of nas-33 between apolysis and late lethargus steps in C. elegans, Hc-nas-33 appeared to be highly transcribed between late lethargus and ecdysis processes in H. contortus, which were defined based on the transcriptions of marker genes nas-37, col-12, and gsr-1 (Figure 1F).
Knockdown of nas-33 Leads to Molting Defects in H. contortus
Compared with the untreated worms, two layers of cuticles were observed in the nas-33 RNAi-treated larvae of H. contortus (Figure 2A). To confirm the association between phenotypic change and RNAi-mediated knockdown of nas-33 in H. contortus, transcriptional levels of nas-33 and iso-1 (positive control) were measured by qRT-PCR. Both of the two genes showed a significant (P < 0.05) decrease in the RNAi-treated larvae (Figure 2B), with the thickness of the L2 cuticle significantly (P < 0.001) thinner than that of untreated larvae (Figure 2C). In addition, successful gene knockdown of nas-33 led to marked transcriptional alterations of genes involved in the molting process of H. contortus. Specifically, lower transcriptional level of nas-33 (P < 0.01) in the RNAi-treated worms was linked to significant downregulation of four genes col-12 (P < 0.05), col-14 (P < 0.01), dpy-5 (P < 0.001), and dpy-13 (P < 0.01) involved in cuticle synthesis, three genes nekl-2 (P < 0.01), mlt-3 (P < 0.01), and lev-11 (P < 0.001) involved in ecdysis, and one gene mua-3 (P < 0.05) involved in junction remodeling (Figure 2D). In addition, suppression of nas-33 significantly compromised the molting process of L1s in H. contortus. Particularly, gene knockdown of Hc-nas-33 resulted in obvious molting defects (e.g., failure of shedding the old cuticle and corset phenotype), compared with negative control (Figure 3).
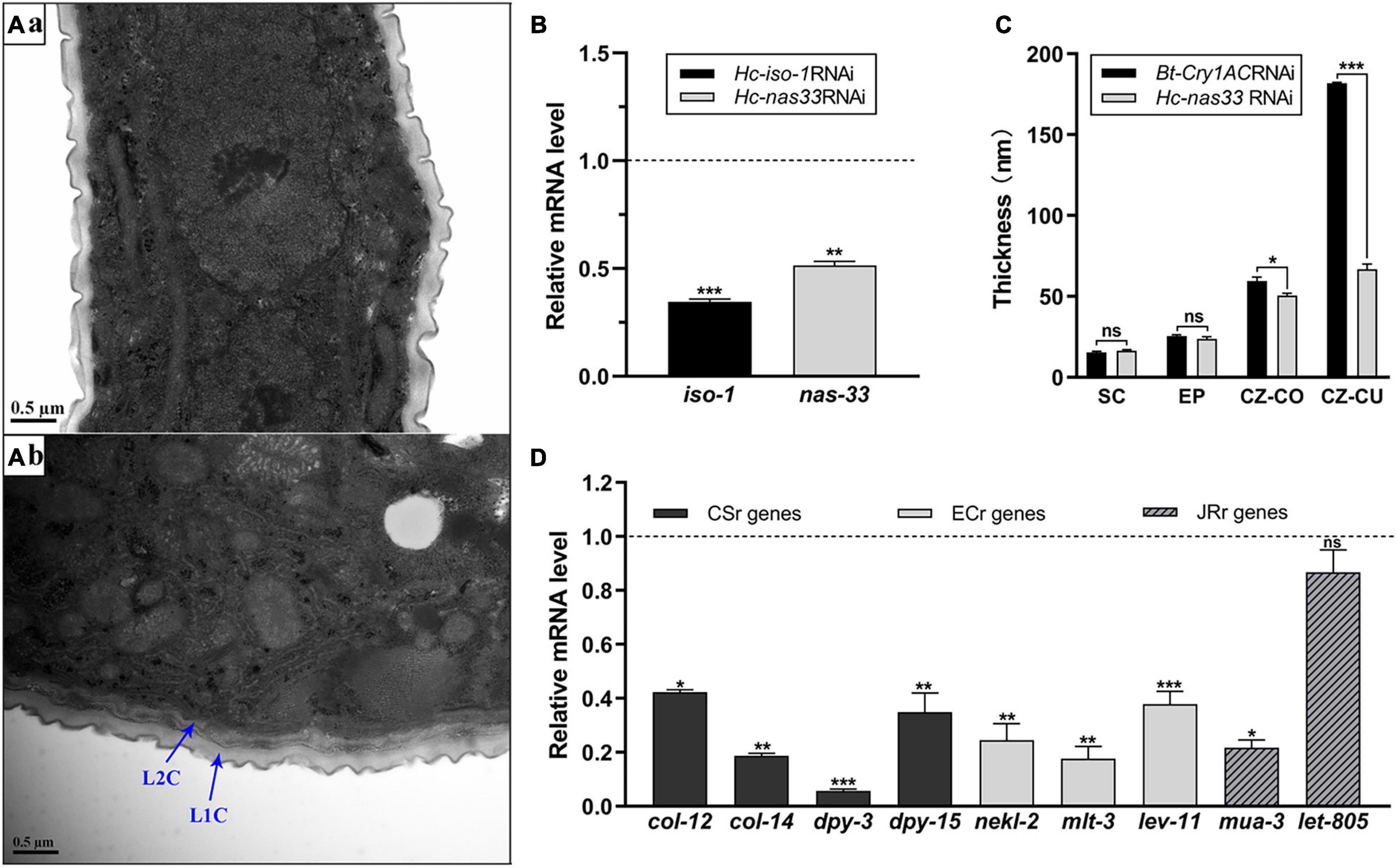
Figure 2. Structural and transcriptional analyses of RNA interference-treated Haemonchus contortus. (A) Transmission electron microscopy of Bt-Cry1AC RNAi-treated (a) and nas-33 RNAi-treated (b) worms. Scale bar: 0.5 μm. L1C: L1 cuticle; L2C: L2 cuticle. (B) Gene knockdown analysis of iso-1 (positive control) and nas-33 in RNAi-treated worms, with reference to the transcriptions of these genes in the untreated worms. (C) Cuticle thickness (measured by ImageJ) of the second larval stage of H. contortus after RNAi. SC, surface coat; EP, epicuticle; CZ-CO, collagen rich layer of cortical zone; CZ-CU, cuticlin rich layer of cortical zone. (D) Relative transcriptional changes of molting related genes in RNAi-treated worms, compared with that in untreated worms. CSr genes, cuticle synthesis related genes (col-12, col-14, dpy-3, dpy-15); ECr genes, ecdysis related genes (nekl-2, mlt-3, lev-11); JRr genes, junction remodeling related genes (mua-3, let-805). Student’s t-test is used for the statistical analysis between treated and untreated worms. *P < 0.05; **P < 0.01; ***P < 0.001; ns, no significance. Dotted line represents the transcriptional level in negative control worms.
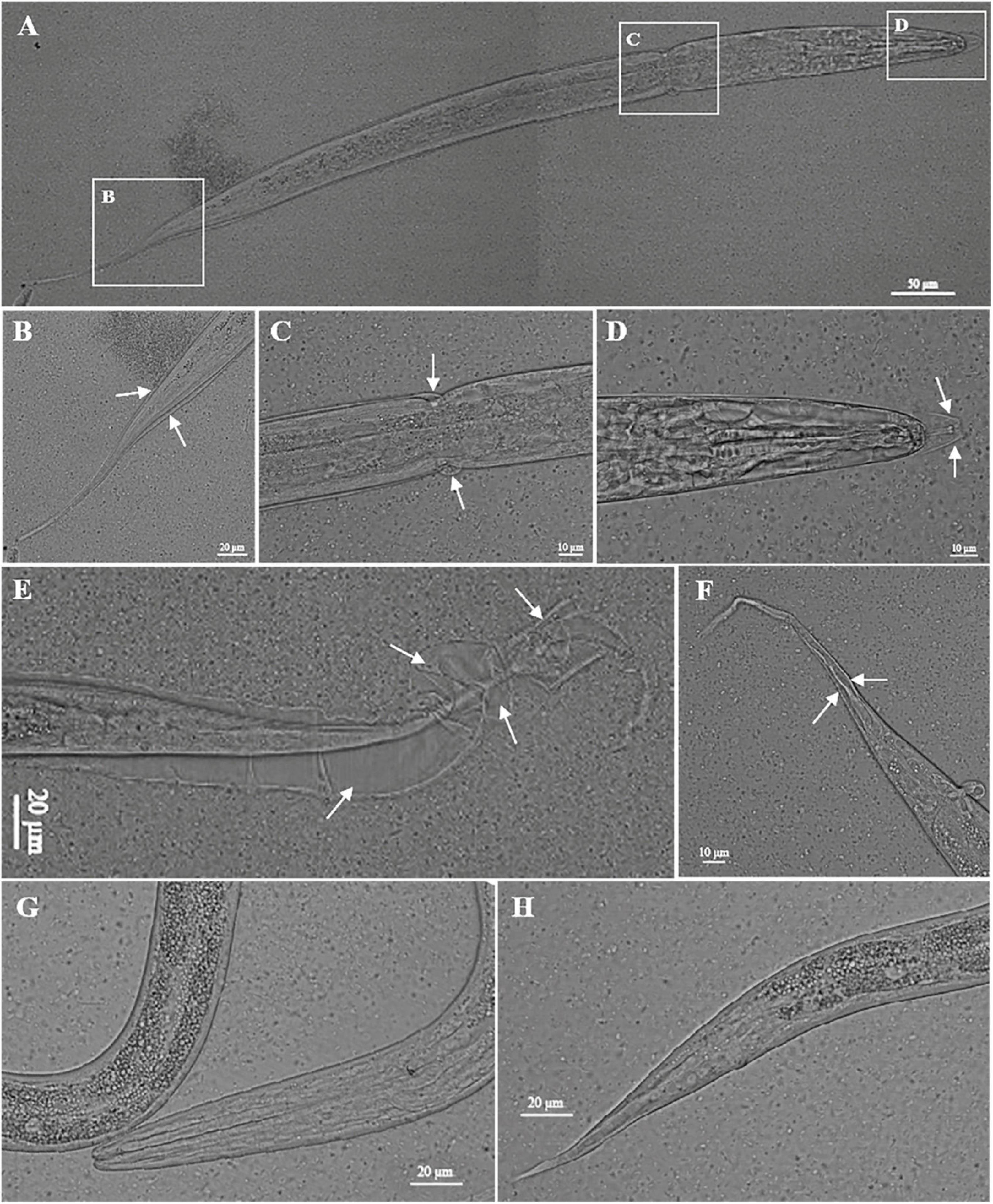
Figure 3. Knockdown of nas-33 leads to molting defects in Haemonchus contortus. (A–D) Defects in the first molting of H. contortus led to larva death. (E,F) Defects in the first molting of H. contortus resulted in attachment of the old cuticle to the second stage larva. Cuticles failed to be shed are marked with white arrows. (G,H) Phenotype of untreated larvae of H. contortus.
Hc-NAS-33 Interacts With a G Protein Subunit in vitro and in vivo
By screening the yeast two-hybrid cDNA library of H. contortus, several proteins were identified as candidates interacting with Hc-NAS-33, including a guanine nucleotide-binding protein subunit beta-1 (GPB-1). The interaction between Hc-NAS-33 and Hc-GPB-1 was verified by the GST pull-down assay in vitro (Figure 4A), and confirmed with the co-IP assay in vivo (Figure 4). In addition, protein expression analyses of Hc-NAS-33 and Hc-GPB-1 in both HEK 293T cells and tissues of C. elegans to some extent showed a similar protein distribution. In cells, Hc-NAS-33 was consistently colocalized with Hc-GPB-1 in the cytoplasm (Figure 4B), whereas in worms, scattered co-localization of Hc-NAS-33 and Hc-GPB-1 was observed in the intestine of adult worms but not in the pharynx area (Figure 5). Low efficiency and no activity were observed for the possible promoter sequences of Hc-nas-33 and Hc-gpb-1. Driven by promoters of Ce-nas-33 and Ce-gpb-1, heterologous protein expression of Hc-NAS-33 and Hc-GPB-1 were achieved in C. elegans, and confirmed by western blot (Supplementary Figure 3). Tissue distribution of Hc-GPB-1 is mostly consistent with the sites of Ce-gpb-1 promoter activity in worms, whereas interestingly, a discrepancy between Ce-nas-33 promoter activity and Hc-NAS-33 protein distribution was identified (Supplementary Figure 4).
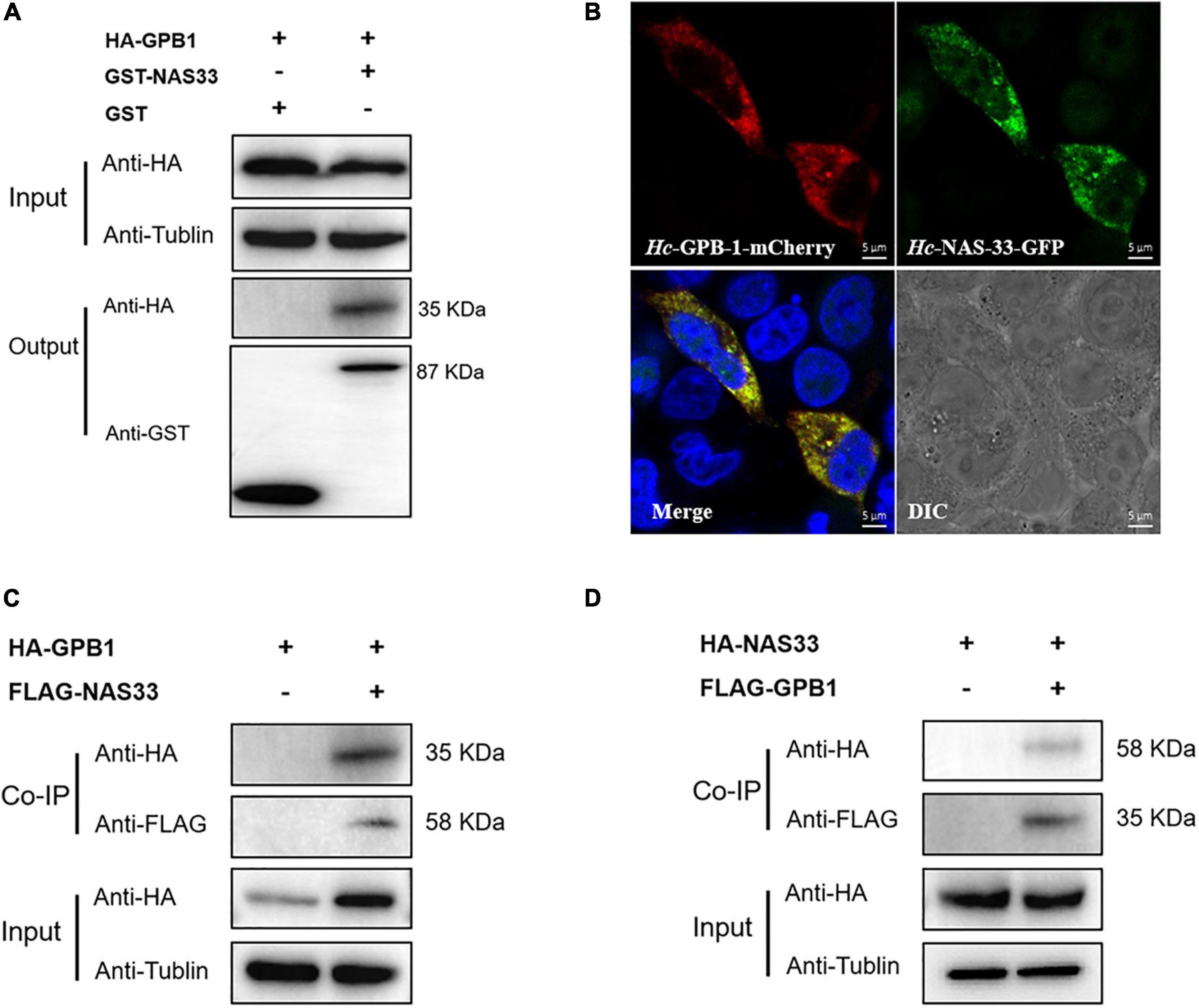
Figure 4. Hc-NAS-33 interacts with Hc-GPB-1 in vitro and in vivo. (A) Pull-down assay showing the interaction of Hc-NAS-33 and Hc-GPB-1. (B) Co-localization of Hc-NAS-33 and Hc-GPB-1 in HEK 293T cells. Scale bar: 5 μm. (C,D) Co-IP assay verifying the interaction of Hc-NAS-33 and Hc-GPB-1 in vivo.
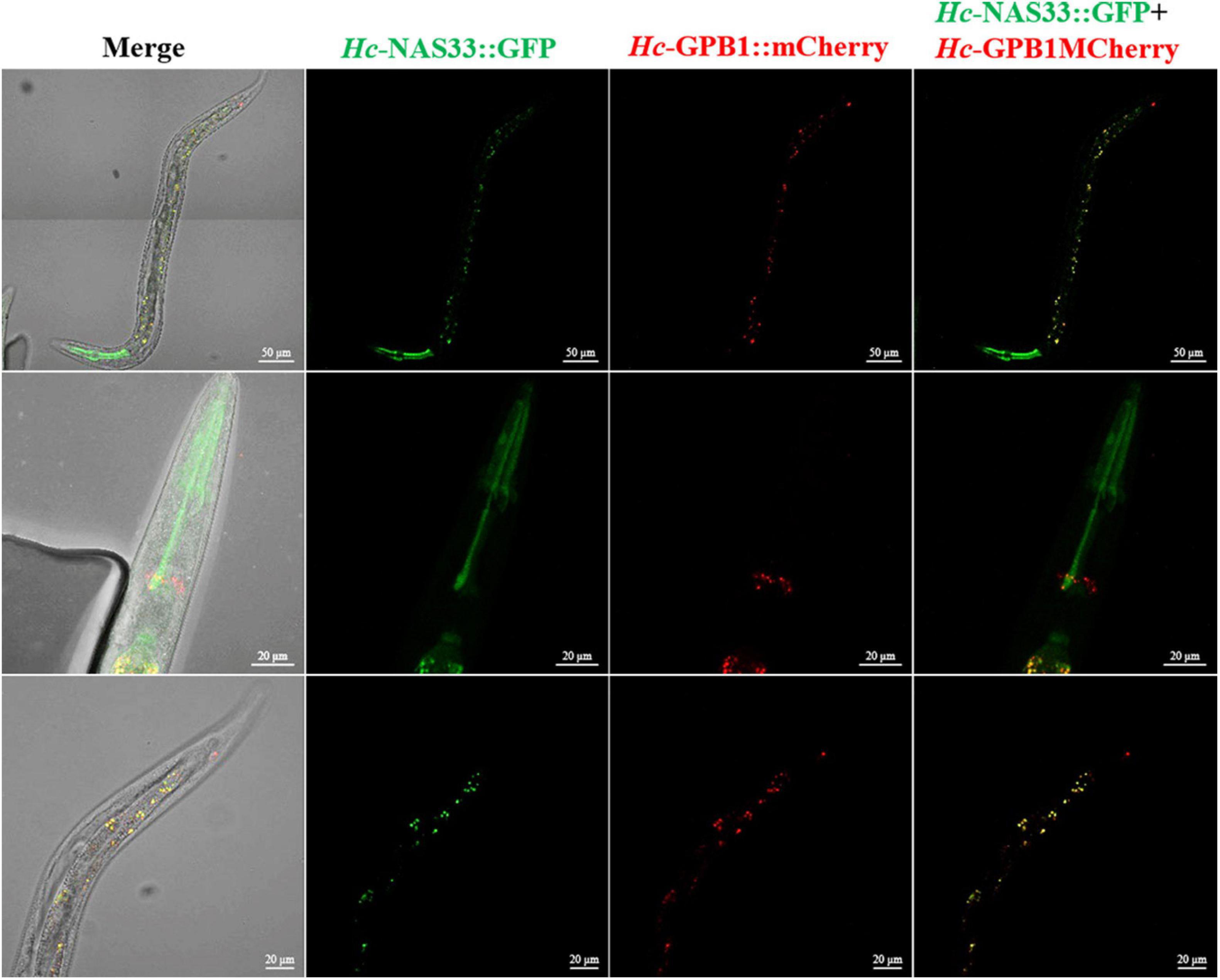
Figure 5. Co-localization of Hc-NAS-33 and Hc-GPB-1 in C. elegans. GFP, green fluorescent protein; mCherry, monmer cherry fluorescent protein.
Knockdown of NAS-33 and GPB-1 Blocked Development and Survival of Infective Larvae
In particular, suppression of Hc-nas-33 and Hc-gpb-1 led to obvious molting defects in treated H. contortus (Figure 6). Specifically, in nas-33 RNAi-treated worms, both old and new cuticles were found closely attached to the hypodermis (Figure 6B). In gpb-1 RNAi-treated worms, only one layer of cuticle was observed, with a loose connection to the hypodermis (Figure 6C). In nas-33 and gpb-1 RNAi-treated worms, both two layers of the cuticle and a loose connection to the hypodermis were identified (Figure 6D). In particular, compared with negative control, independent RNAi of gpb-1 was linked to a significant (P < 0.05) upregulation of nas-33 in treated worms, whereas knockdown of nas-33 resulted in significant (P < 0.05) downregulation of gpb-1 in H. contortus. Notably, simultaneous RNAi of nas-33 and gpb-1 significantly enhanced the knockdown efficacies of both nas-33 (P < 0.05) and gpb-1 (P < 0.0001) (Figure 7A).
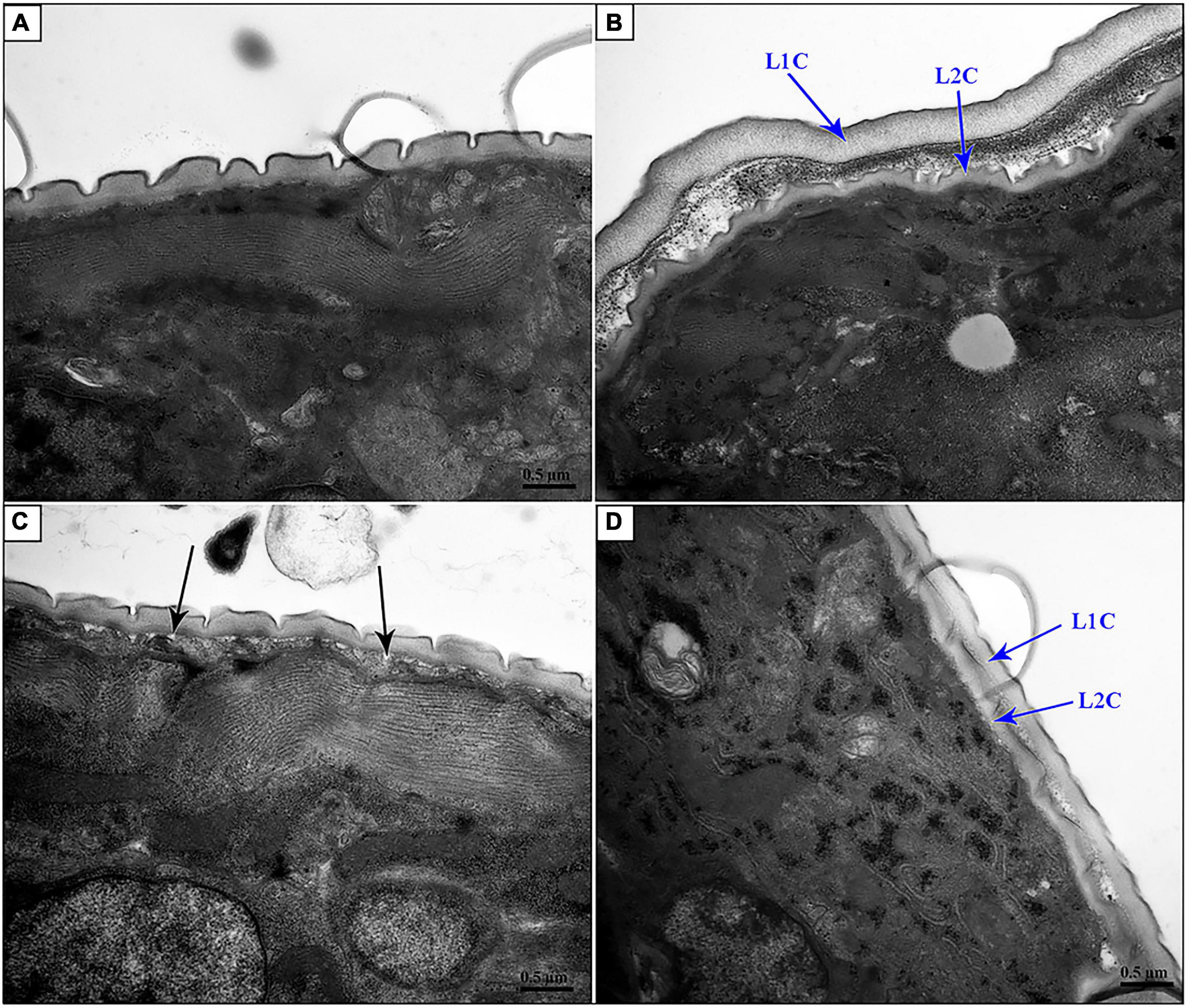
Figure 6. Transmission electron microscopy of nas-33 and gpb-1 RNA interference-treated Haemonchus contortus. (A) Cuticle structure of Bt-Cry1AC RNAi-treated worm. (B) Two layers of cuticles of nas-33 RNAi-treated worm. (C) Loose connection between cuticle and epidermis in gpb-1 RNAi-treated worm. (D) Two layers of cuticles and loose connection between cuticle and epidermis of nas-33 and gpb-1 RNAi-treated worm. L1C, L1 cuticle; L2C, L2 cuticle. Black arrows point to the loose connections between cuticle and epidermis. Scale bar: 0.5 μm.
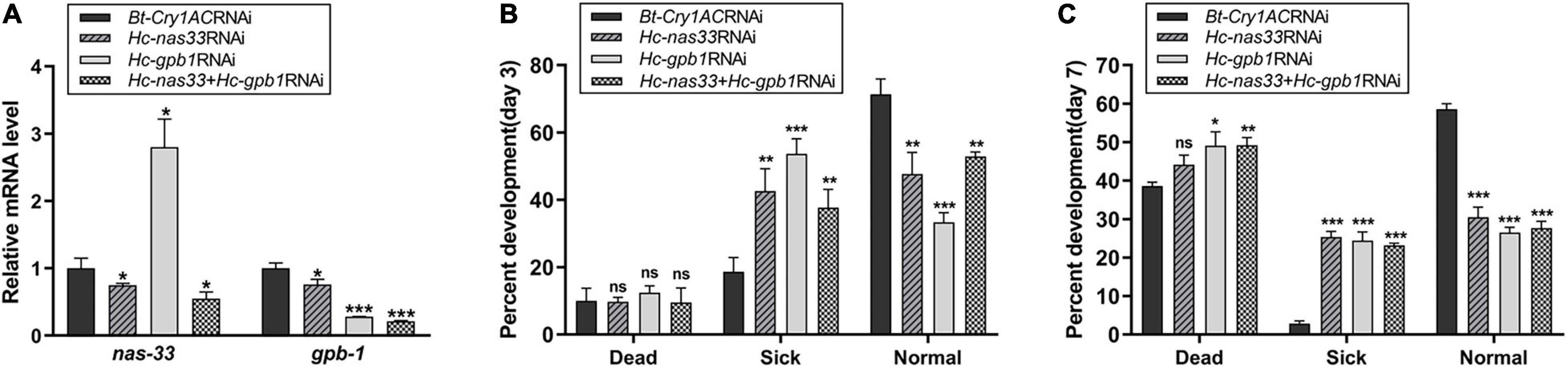
Figure 7. Effects of nas-33 and gpb-1 RNA interference on the development and survival of Haemonchus contortus. (A) Influences of nas-33 or gpb-1 RNAi on the relative mRNA levels (normalized by the transcriptional level of Bt-Cry1AC) of nas-33 and gpb-1, respectively. (B) Influences of separate (Hc-nas-33 or Hc-gpb-1) and simultaneous (Hc-nas-33 + Hc-gpb-1) RNAi on the development and survival of H. contortus on day 3. (C) Influences of separate (Hc-nas-33 or Hc-gpb-1) and simultaneous (Hc-nas-33 + Hc-gpb-1) RNAi on the development and survival of H. contortus on day 7. Sick phenotype includes developmental delay, decreased mobility, abnormality and molting defect. Student’s t-test is used for the statistical analysis between treated and negative control. *P < 0.05; **P < 0.01; ***P < 0.001; ns, no significance.
Defects in larval molting resulted in developmental and survival variations in treated H. contortus (Figures 7B,C). In particular, simultaneous knockdown of nas-33 and gpb-1 resulted in delayed larval development, decreased mobility and sickness. Compared with negative control, increased number (∼40%) of sick larvae (P < 0.01) and decreased healthy larvae (P < 0.01) were found in RNAi-treated groups on day 3 (Figure 7B), and increased number (∼50%) of dead larvae (including the free-living L2s and the infective L3s) and decreased number of healthy larvae (P < 0.001) in treated groups on day 7 (Figure 7C). No significant difference was found between independent and simultaneous RNAi of nas-33 and gpb-1, in terms of phenotypic changes.
Discussion
In this study, we report an essential zinc metalloprotease NAS-33 in nematode species. Our findings elucidated that this metalloprotease is likely to play a role in the larval molting process of the free-living C. elegans and the parasitic H. contortus. In particular, Hc-NAS-33 interacts with Hc-GPB-1 to control shedding of the old cuticle and remodeling of the connection between the new cuticle and the hypodermis of worms. Suppression of the Hc-NAS-33-GPB-1 module resulted in molting defects and a moderate lethal phenotype, suggesting the essentiality of nas genes in nematodes.
The astacin-like protein coding gene nas-33 is relatively conserved in free-living and parasitic nematodes. In C. elegans, nas-35, -36, and -37 encode astacins of subgroup V (NAS-33 to -38) which have the N-terminal astacin-like, C-terminal EGF (epidermal growth factor), CUB (C1r/C1s, embryonic sea urchin protein Uegf, Bmp-1) and TSP1 domains in order, implying an essential role of astacins of subgroup V in the molting, survival and development of nematodes. Compared with the domain architecture of Ce-NAS-33, Hc-NAS-33 lacks a TSP1 domain. This domain is usually found in extracellular matrix proteins (Zhang et al., 2020) and a number of proteins involved in the complement pathway (Patthy, 1988). In particular, the thrombospondin type 1 repeat containing proteins ADAMTS (a disintegrin-like and metalloprotease domain) have been proved to be principal mediators of ECM destruction (Apte and Parks, 2015; Liu et al., 2015). In nematodes, cuticle components are synthesized, secreted and modified in the extracellular matrix, and TSP1 of Ce-NAS-33 might function in the modification and arrangement of cuticle proteins during late lethargus. However, it is still not clear whether the difference in NAS-33 protein sequence is associated with the unique life cycle and living conditions of parasitic nematodes.
The gene nas-33 appears to play a role in the molting processes of H. contortus. First, predominant transcription of nas-33 in larval stages indicated that this gene might play roles in larvae development and survival. In C. elegans, genes involved in molting (apolysis, late lethargus and ecdysis) usually have a dynamic expression pattern (Hendriks et al., 2014; Turek and Bringmann, 2014). For instance, higher mRNA levels of Ce-nas-33 were detected in the apolysis and late lethargus stages. However, apart from body-size changes, no significant difference related to molting was observed in C. elegans after knockdown of Ce-nas-33 (Supplementary Figure 5), which might be explained by functional redundancy of nas genes in this free-living nematode. Interestingly, it was found that the mRNA level of Hc-nas-33 was higher in the late lethargus and early ecdysis (based on the transcription of marker genes nas-37, col-12 and gsr-1) in H. contortus, which is different from that of Ce-nas-33 in C. elegans, indicating subtle functional differences in molting between these two species. Second, downregulation of collagen-associated genes (col-12, col-14, dpy-5, and dpy-13; Johnstone and Barry, 1996; Mcmahon et al., 2003; Page and Johnstone, 2007) in RNAi-treated worms suggests that Hc-nas-33 is required in the synthesis of cuticle structure elements (Cox et al., 1981; Page, 2001), which is further confirmed by the change of cortical zone thickness. In addition, it was reported that nekl-2 and mlt-3 genes involved in nekl-mlt kinase network (Yochem et al., 2015; Lazetic and Fay, 2017), and lev-11 involved in muscle contraction spinning and flipping behavior (Frand et al., 2005; Barnes et al., 2018; Watabe et al., 2018) are essential for molting in C. elegans. Reduced expression of nekl-2, mlt-3 and lev-11 were observed in the Hc-nas-33RNAi experiment, indicating that Hc-nas-33 might be involved in ecdysis via muscle contraction regulation. These findings indicate that Hc-nas-33 and associated metalloproteases might be a target for reducing the population of H. contortus infected larvae, suggesting a possible approach to the prevention of haemonchosis, although it warrants further investigations.
Hc-NAS-33 and Hc-GPB-1 are required for cuticle synthesis and cuticle-epidermis linkage remodeling. This statement can be supported by the decreased thickness of cortical zone in Hc-nas-33 RNAi-treated worms and decreased thickness of both epicuticle and cortical zone in gpb-1 RNAi-treated worms (Supplementary Figure 6A). These results indicate that Hc-NAS-33 and Hc-GPB-1 are related to protein components synthesis, with Hc-GPB-1 likely required for lipid and glycolipid synthesis during the molting process. Additionally, it has been demonstrated that mua-3 and let-805/myotactin are component of hemidesmosome-like structures (HDLSs) through which the epidermis and cuticle are attached to each other. MUA-3 may help to link collagens in the basal zone to the epidermal cytoskeleton (Bercher et al., 2001), and LET-805 may guide the remodeling of basement membrane attachments during molting (Hresko et al., 1999). In the current work, silencing of Hc-nas-33 led to a predominant downregulation of mua-3, whereas knockdown of Hc-gpb-1 was linked to let-805 (Supplementary Figure 6B), suggesting their roles in both cuticle synthesis and the remodeling of basement membrane attachments.
Apart from molting, NAS-33 and GPB-1 might play additional roles in nematode species. This is because that the two proteins were partially co-localized in worms. Little has been reported on other functional roles of NAS-33, although it might play a role in embryogenesis or hatching as transcription of this gene was detected in H. contortus eggs. By contrast, GPB-1 expression has been observed in the neurons, hypodermal seam cells, gonad and vulva in both larval and adult stages of C. elegans. Such expression pattern is consistent with the phenotypes including abnormalities in early embryogenesis, sterility and abnormalities in the germ line caused by GPB-1 depletion (Zwaal et al., 1996). Additionally, the distribution of GPB-1 at the cell membrane is dynamic and asymmetric during the division of one-cell stage C. elegans embryos (Thyagarajan et al., 2011). However, these functional roles of NAS-33 or GPB-1 in H. contortus and associated parasitic nematodes warrants further investigations.
In conclusion, we identified an essential astacin protein NAS-33 in nematode species. Suppression of this protein and associated G protein subunit resulted in molting defect and death of infective larvae of a highly pathogenic strongylid nematode. Our work provides comprehensive insights into the essentiality of nas gene family in nematode molting and survival, and thereby lays a foundation for the discovery of potential targets for the prevention of parasitic diseases of socioeconomic significance.
Data Availability Statement
The raw data supporting the conclusions of this article will be made available by the authors, without undue reservation.
Ethics Statement
The animal study was reviewed and approved by the Experimental Animal Ethics Committee, Zhejiang University.
Author Contributions
YH, XC, and AD designed the research. YH, JW, and DT conducted the experiments. YY, FW, and HZ provided regents. JZ and JW analyzed the data. YH and GM wrote the manuscript. XC, GM, and AD offered advice during the research and provided financial support. All authors read and approved the final manuscript.
Funding
This project was supported by grants from the National Key Basic Research Program of China (973 program), Grant/Award no. 2015CB150300, the National Key Research and Development Program of China, Grant/Award no. 2017YFD0501203, and the National Natural Science Foundation of China (NSFC), Grant/Award no. 32002304.
Conflict of Interest
The authors declare that the research was conducted in the absence of any commercial or financial relationships that could be construed as a potential conflict of interest.
Acknowledgments
We would like to thank Li Xie and his colleagues (Bio-ultrastructure Analysis Laboratory of Analysis Center of Agrobiology and Environmental Sciences, Zhejiang University) for their assistance with the transmission electron microscopy analysis. We thank Min Hu (State Key Laboratory of Agricultural Microbiology, College of Veterinary Medicine, Huazhong Agricultural University) for providing Bt-Cry1AC plasmid. We are grateful to Caiyong Chen (MOE Key Laboratory of Biosystems Homeostasis and Protection, College of Life Sciences, Zhejiang University) for providing the pPD95_67 vector.
Supplementary Material
The Supplementary Material for this article can be found online at: https://www.frontiersin.org/articles/10.3389/fcell.2021.695003/full#supplementary-material
Supplementary Figure 1 | Isolation of Hc-nas-33 DNA and cDNA from Haemonchus contortus. (A) 5′- RACE. (B) 3′- RACE. (C) 5′- Genome Walking. (D) 3′- Genome Walking. (E) Cloning of Hc-nas-33 full length coding sequence by overlapping PCR. 1, 1–213 nucleotide sequence of Hc-nas-33; 2, 207–1,569 nucleotide sequence of Hc-nas-33; 3, full length coding sequence of Hc-nas-33. SP, specific primer; M, DNA marker.
Supplementary Figure 2 | Characterization of Hc-nas-33 in Haemonchus contortus. (A) Schematic diagram of Hc-nas-33 gene structure (GenBank accession No. MT891117). Black blocks represent exons and gray blocks represent non-coding 5′- and 3′-untranslated region (UTR) sequence. (B) Functional domain prediction and alignment of amino acid sequence of Hc-NAS-33 and homologs in other nematode species.
Supplementary Figure 3 | Western blotting of Hc-NAS-33 and Hc-GPB-1 expressed in transgenic worms. M, standard protein marker.
Supplementary Figure 4 | Promoter activity analysis of pCe-nas-33, pHc-nas-33, and pCe-gpb-1 in Caenorhabditis elegans. (A–C) Massive expression of pCe-nas-33:GFP in the epidermis of C. elegans. (D,E) Weak expression of pHc-nas-33:GFP in several intestine cells. (F,G) Specific expression of pCe-gpb-1:mCherry in the head and tail of C. elegans.
Supplementary Figure 5 | Effects of Ce-nas-33 knockdown on Caenorhabditis elegans. (A) Development of vulva showed no significant difference after Ce-nas-33 RNAi compared with negative control (Bt-Cry1AC). (B,C) Morphological changes (body width and body length) at 36 and 48 h after Ce-nas-33 RNAi treatment. Student’s t-test is used for the statistical analysis between treated and negative control. ∗∗∗P < 0.001.
Supplementary Figure 6 | Analysis of cuticle thickness and junction remodeling related genes after gpb-1 RNA interference. (A) Cuticle thickness (measured by ImageJ) changes of the second stage larva after gpb-1 RNAi. SC, surface coat; EP, epicuticle; CZ-CO, collagen rich layer of cortical zone; CZ-CU, cuticlin rich layer of cortical zone. (B) Influences of gpb-1 RNAi on the transcription of junction remodeling related genes (mua-3 and let-805). Student’s t-test is used for the statistical analysis between treated and negative control. ∗∗P < 0.01; ∗∗∗P < 0.001; ns, no significance.
Supplementary Table 1 | Information on primer sets used in this study.
Footnotes
- ^ https://www.sanger.ac.uk
- ^ https://www.ncbi.nlm.nih.gov/Structure/cdd/wrpsb.cgi
- ^ http://www.ebi.ac.uk/interpro/scan.html
References
Abdulla, M., Lim, K., Sajid, M., Mckerrow, J. H., and Caffrey, C. R. (2007). Schistosomiasis mansoni: novel chemotherapy using a cysteine protease inhibitor. PLoS Med. 4:130–138. doi: 10.1371/journal.pmed.0040014
Apte, S. S., and Parks, W. C. (2015). Metalloproteinases: a parade of functions in matrix biology and an outlook for the future. Matrix Biol. 44–46, 1–6. doi: 10.1016/j.matbio.2015.04.005
Barnes, D. E., Watabe, E., Ono, K., Kwak, E., Kuroyanagi, H., and Ono, S. (2018). Tropomyosin isoforms differentially affect muscle contractility in the head and body regions of the nematode Caenorhabditis elegans. Mol. Biol. Cell. 29, 1075–1088. doi: 10.1091/mbc.E17-03-0152
Bercher, M., Wahl, J., Vogel, B. E., Lu, C., Hedgecock, E. M., Hall, D. H., et al. (2001). mua-3, a gene required for mechanical tissue integrity in Caenorhabditis elegans, encodes a novel transmembrane protein of epithelial attachment complexes. J. Cell Biol. 154, 415–426. doi: 10.1083/jcb.200103035
Bird, A. F., and Bird, J. (1991). The Structure of Nematodes. California, CA: San Diego Academic Press, 1–316.
Cox, G. N., Kusch, M., and Edgar, R. S. (1981). Cuticle of Caenorhabditis elegans – its isolation and partial characterization. J. Cell Biol. 90, 7–17. doi: 10.1083/jcb.90.1.7
Davis, M. W., Birnie, A. J., Chan, A. C., Page, A. P., and Jorgensen, E. M. (2004). A conserved metalloprotease mediates ecdysis in Caenorhabditis elegans. Development 131, 6001–6008. doi: 10.1242/dev.01454
Deu, E. (2017). Proteases as antimalarial targets: strategies for genetic, chemical, and therapeutic validation. FEBS J. 284, 2604–2628. doi: 10.1111/febs.14130
France, D. J., Stepek, G., Houston, D. R., Williams, L., Mccormack, G., Walkinshaw, M. D., et al. (2015). Identification and activity of inhibitors of the essential nematode-specific metalloprotease DPY-31. Bioorg. Med. Chem. Lett. 25, 5752–5755. doi: 10.1016/j.bmcl.2015.10.077
Frand, A. R., Russel, S., and Ruvkun, G. (2005). Functional genomic analysis of C. elegans molting. PLoS Biol. 3:e312. doi: 10.1371/journal.pbio.0030312
Gamble, H. R., Purcell, J. P., and Fetterer, R. H. (1989). Purification of a 44 kilodalton protease which mediates the ecdysis of infective Haemonchus contortus larvae. Mol. Biochem. Parasit. 33, 49–58.
Hashmi, S., Britton, C., Liu, J., Guiliano, D. B., Oksov, Y., and Lustigman, S. (2002). Cathepsin L is essential for embryogenesis and development of Caenorhabditis elegans. J. Biol. Chem. 277, 3477–3486. doi: 10.1074/jbc.M106117200
Hendriks, G., Gaidatzis, D., Aeschimann, F., and Grosshans, H. (2014). Extensive oscillatory gene expression during C. elegans larval development. Mol. Cell 53, 380–392. doi: 10.1016/j.molcel.2013.12.013
Hresko, M. C., Schriefer, L. A., Shrimankar, P., and Waterston, R. H. (1999). Myotactin, a novel hypodermal protein involved in muscle-cell adhesion in Caenorhabditis elegans. J. Cell Biol. 146, 659–672. doi: 10.1083/jcb.146.3.659
Johnstone, I. L., and Barry, J. D. (1996). Temporal reiteration of a precise gene expression pattern during nematode development. EMBO J. 15, 3633–3639. doi: 10.1002/j.1460-2075.1996.tb00732.x
Kamath, R. S., Fraser, A. G., Dong, Y., Poulin, G., Durbin, R., Gotta, M., et al. (2003). Systematic functional analysis of the Caenorhabditis elegans genome using RNAi. Nature 421, 231–237. doi: 10.1038/nature01278
Lazetic, V., and Fay, D. S. (2017). Conserved ankyrin repeat proteins and their NIMA kinase partners regulate extracellular matrix remodeling and intracellular trafficking in Caenorhabditis elegans. Genetics 205, 273–293. doi: 10.1534/genetics.116.194464
Liu, P., Yan, S., Chen, M., Chen, A., Yao, D., Xu, X., et al. (2015). Effects of baicalin on collagen I and collagen III expression in pulmonary arteries of rats with hypoxic pulmonary hypertension. Int. J. Mol. Med. 35, 901–908. doi: 10.3892/ijmm.2015.2110
Lustigman, S. (1993). Molting, enzymes and new targets for chemotherapy of Onchocerca volvulus. Parasitol. Today 9, 294–297. doi: 10.1016/0169-4758(93)90128-3
Maeda, I., Kohara, Y., Yamamoto, M., and Sugimoto, A. (2001). Large-scale analysis of gene function in Caenorhabditis elegans by high-throughput RNAi. Curr. Biol. 11, 171–176.
Maizels, R. M. (2013). Toxocara canis: molecular basis of immune recognition and evasion. Vet. Parasitol. 193, 365–374. doi: 10.1016/j.vetpar.2012.12.032
Mcmahon, L., Muriel, J. M., Roberts, B., Quinn, M., and Johnstone, I. L. (2003). Two sets of interacting collagens form functionally distinct substructures within a Caenorhabditis elegans extracellular matrix. Mol. Biol. Cell 14, 1366–1378. doi: 10.1091/mbc.E02-08-0479
Mello, C. C., Kramer, J. M., Stinchcomb, D., and Ambros, V. (1991). Efficient gene-transfer in C. elegans extrachromosomal maintenance and integration of transforming sequences. EMBO J. 10, 3959–3970. doi: 10.1002/j.1460-2075.1991.tb04966.x
Mohrlen, F., Hutter, H., and Zwilling, R. (2003). The astacin protein family in Caenorhabditis elegans. Eur. J. Biochem. 270, 4909–4920. doi: 10.1046/j.1432-1033.2003.03891.x
Mohrlen, F., Maniura, M., Plickert, G., Frohme, M., and Frank, U. (2006). Evolution of astacin-like metalloproteases in animals and their function in development. Evol. Dev. 8, 223–231. doi: 10.1111/j.1525-142X.2006.00092.x
Novelli, J., Ahmed, S., and Hodgkin, J. (2004). Gene interactions in Caenorhabditis elegans define DPY-31 as a candidate procollagen C-proteinase and SQT-3/ROL-4 as its predicted major target. Genetics 168, 1259–1273. doi: 10.1534/genetics.104.027953
Novelli, J., Page, A. P., and Hodgkin, J. (2006). The C terminus of collagen SQT-3 has complex and essential functions in nematode collagen assembly. Genetics 172, 2253–2267. doi: 10.1534/genetics.105.053637
Page, A. P. (2001). “The nematode cuticle: synthesis, modification and mutants”, in Parasitic Nematodes: Molecular Biology, Biochemistry and Immunology, ed. M. W. Kennedy, and W. Harnett (Oxon, NY: CABI Publishing), 167–193. doi: 10.1079/9780851994239.0167
Page, A. P., and Johnstone, I. L. (2007). “The cuticle”, in WormBook, The C. elegans Research Community, WormBook. doi: 10.1895/wormbook.1.138.1
Park, J., Pan, J., Moehrlen, F., Schupp, M., Johnsen, R., Baillie, D. L., et al. (2010). Characterization of the astacin family of metalloproteases in C. elegans. BMC Dev. Biol. 10:14. doi: 10.1186/1471-213X-10-14
Patthy, L. (1988). Detecting distant homologies of mosaic proteins – analysis of the sequences of thrombomodulin, thrombospondin complement components C9, C8-alpha and C8-beta, vitronectin and plasma-cell membrane glycoprotein PC-1. J. Mol. Biol. 202, 689–696. doi: 10.1016/0022-2836(88)90550-5
Pfleiderer, G., Zwilling, R., and Sonneborn, H. H. (1967). Zur evolution der endopeptidasen .3. eine protease vom molekulargewicht 11000 und eine trypsinahnliche fraktion aud astacus fluviatilis fabr. Hoppe Seylers Z. Physiol. Chem. 348:1319. doi: 10.1515/bchm2.1967.348.1.1319
Politz, S. M., and Philipp, M. (1992). Caenorhabditis elegans as a model for parasitic nematodes – a focus on the cuticle. Parasitol. Today 8, 6–12. doi: 10.1016/0169-4758(92)90302-I
Rogers, W. P. (1982). Enzymes in the exsheathing fluid of nematodes and their biological significance. Int. J. Parasitol. 12, 495–502. doi: 10.1016/0020-7519(82)90043-1
Roy, K. K. (2017). Targeting the active sites of malarial proteases for antimalarial drug discovery: approaches, progress and challenges. Int. J. Antimicrob. Agents 50, 287–302. doi: 10.1016/j.ijantimicag.2017.04.006
Samarasinghe, B., Knox, D. P., and Britton, C. (2011). Factors affecting susceptibility to RNA interference in Haemonchus contortus and in vivo silencing of an H11 aminopeptidase gene. Int. J. Parasitol. 41, 51–59. doi: 10.1016/j.ijpara.2010.07.005
Shamsi, T. N., Parveen, R., and Fatima, S. (2016). Characterization, biomedical and agricultural applications of protease inhibitors: a review. Int. J. Biol. Macromol. 91, 1120–1133. doi: 10.1016/j.ijbiomac.2016.02.069
Sharma, R. K., Younis, Y., Mugumbate, G., Njoroge, M., Gut, J., Rosenthal, P. J., et al. (2015). Synthesis and structure-activity-relationship studies of thiazolidinediones as antiplasmodial inhibitors of the Plasmodium falciparum cysteine protease falcipain-2. Eur. J. Med. Chem. 90, 507–518. doi: 10.1016/j.ejmech.2014.11.061
Singh, A., Kalamuddin, M., Maqbool, M., Mohmmed, A., Malhotra, P., and Hoda, N. (2021). Quinoline carboxamide core moiety-based compounds inhibit P. falciparum falcipain-2: design, synthesis and antimalarial efficacy studies. Bioorg. Chem. 108:104514. doi: 10.1016/j.bioorg.2020.104514
Stenvall, J., Fierro-Gonzalez, J. C., Swoboda, P., Saamarthy, K., Cheng, Q., Cacho-Valadez, B., et al. (2011). Selenoprotein TRXR-1 and GSR-1 are essential for removal of old cuticle during molting in Caenorhabditis elegans. Proc. Natl. Acad. Sci. U. S. A. 108, 1064–1069. doi: 10.1073/pnas.1006328108
Stepek, G., Mccormack, G., Birnie, A. J., and Page, A. P. (2011). The astacin metalloprotease moulting enzyme NAS-36 is required for normal cuticle ecdysis in free-living and parasitic nematodes. Parasitology 138, 237–248. doi: 10.1017/S0031182010001113
Stepek, G., Mccormack, G., and Page, A. P. (2010). Collagen processing and cuticle formation is catalysed by the astacin metalloprotease DPY-31 in free-living and parasitic nematodes. Int. J. Parasitol. 40, 533–542. doi: 10.1016/j.ijpara.2009.10.007
Stepek, G., Mccormack, G., Winter, A. D., and Page, A. P. (2015). A highly conserved, inhibitable astacin metalloprotease from Teladorsagia circumcincta is required for cuticle formation and nematode development. Int. J. Parasitol. 45, 345–355. doi: 10.1016/j.ijpara.2015.01.004
Sulston, J., and Hodgkin, J. (1988). The nematode Caenorhabditis elegans. Methods Cold Spring Harbor Monograph Series 17, 587–606.
Suzuki, M., Sagoh, N., Iwasaki, H., Inoue, H., and Takahashi, K. (2004). Metalloproteases with EGF, CUB, and thrombospondin-1 domains function in molting of Caenorhabditis elegans. Biol. Chem. 385, 565–568. doi: 10.1515/BC.2004.069
Thyagarajan, K., Afshar, K., and Goenczy, P. (2011). Polarity mediates asymmetric trafficking of the G beta heterotrimeric G-protein subunit GPB-1 in C. elegans embryos. Development 138, 2773–2782. doi: 10.1242/dev.063354
Turek, M., and Bringmann, H. (2014). Gene expression changes of caenorhabditis elegans larvae during molting and sleep-like lethargus. PLoS One 9:e113269. doi: 10.1371/journal.pone.0113269
Watabe, E., Ono, S., and Kuroyanagi, H. (2018). Alternative splicing of the Caenorhabditis elegans lev-11 tropomyosin gene is regulated in a tissue-specific manner. Cytoskeleton 75, 427–436. doi: 10.1002/cm.21489
Yan, B., Guo, X., Zhou, Q., Yang, Y., Chen, X., Sun, W., et al. (2014). Hc-fau, a novel gene regulating diapause in the nematode parasite Haemonchus contortus. Int. J. Parasitol. 44, 775–786. doi: 10.1016/j.ijpara.2014.05.011
Yochem, J., Lazetic, V., Bell, L., Chen, L., and Fay, D. (2015). C. elegans NIMA-related kinases NEKL-2 and NEKL-3 are required for the completion of molting. Dev. Biol. 398, 255–266. doi: 10.1016/j.ydbio.2014.12.008
Zawadzki, J. L., Kotze, A. C., Fritz, J. A., Johnson, N. M., Hemsworth, J. E., Hines, B. M., et al. (2012). Silencing of essential genes by RNA interference in Haemonchus contortus. Parasitology 139, 613–629. doi: 10.1017/S0031182012000121
Zhang, R., Kumra, H., and Reinhardt, D. P. (2020). Quantification of extracellular matrix fiber systems related to ADAMTS proteins. Methods Mol. Biol. 2043:237–250.
Keywords: nematode astacin, nas-33, gpb-1, molting, anthelmintic target
Citation: Huang Y, Wu J, Chen X, Tong D, Zhou J, Wu F, Zhang H, Yang Y, Ma G and Du A (2021) A Zinc Metalloprotease nas-33 Is Required for Molting and Survival in Parasitic Nematode Haemonchus contortus. Front. Cell Dev. Biol. 9:695003. doi: 10.3389/fcell.2021.695003
Received: 14 April 2021; Accepted: 17 June 2021;
Published: 13 July 2021.
Edited by:
Qingfeng Zhang, Tongji University, ChinaReviewed by:
Wesley Lyeverton Correia Ribeiro, Federal University of Ceara, BrazilChris Li, City College of New York (CUNY), United States
Copyright © 2021 Huang, Wu, Chen, Tong, Zhou, Wu, Zhang, Yang, Ma and Du. This is an open-access article distributed under the terms of the Creative Commons Attribution License (CC BY). The use, distribution or reproduction in other forums is permitted, provided the original author(s) and the copyright owner(s) are credited and that the original publication in this journal is cited, in accordance with accepted academic practice. No use, distribution or reproduction is permitted which does not comply with these terms.
*Correspondence: Guangxu Ma, Z3htYTFAemp1LmVkdS5jbg==; Aifang Du, YWZkdUB6anUuZWR1LmNu