- 1Institute of Apicultural Research, Chinese Academy of Agricultural Sciences, Beijing, China
- 2ABLife BioBigData Institute, Wuhan, China
- 3Laboratory for Genome Regulation and Human Health, ABLife Inc., Wuhan, China
- 4College of Animal Science, Zhejiang University, Hangzhou, China
- 5Department of Scientific Research, Jilin Province Institute of Apicultural Science, Jilin, China
Embryonic development depends on a highly coordinated shift in transcription programs known as the maternal-to-zygotic transition (MZT). It remains unclear how haploid and diploid embryo coordinate their genomic activation and embryonic development during MZT in haplodiploid animals. Here, we applied a single-embryo RNA-seq approach to characterize the embryonic transcriptome dynamics in haploid males vs. diploid females of the haplodiploid insect honeybee (Apis mellifera). We observed typical zygotic genome activation (ZGA) occurred in three major waves specifically in female honeybee embryos; haploid genome activation was much weaker and occurred later. Strikingly, we also observed three waves of transcriptional activation for thousands of long non-coding transcripts (lncRNA), 73% of which are transcribed from intronic regions and 65% were specific to female honeybee embryos. These findings support a model in which introns encode thousands of lncRNAs that are expressed in a diploid-embryo-specific and ZGA-triggered manner that may have potential functions to regulate gene expression during early embryonic development in the haplodiploid insect honeybee.
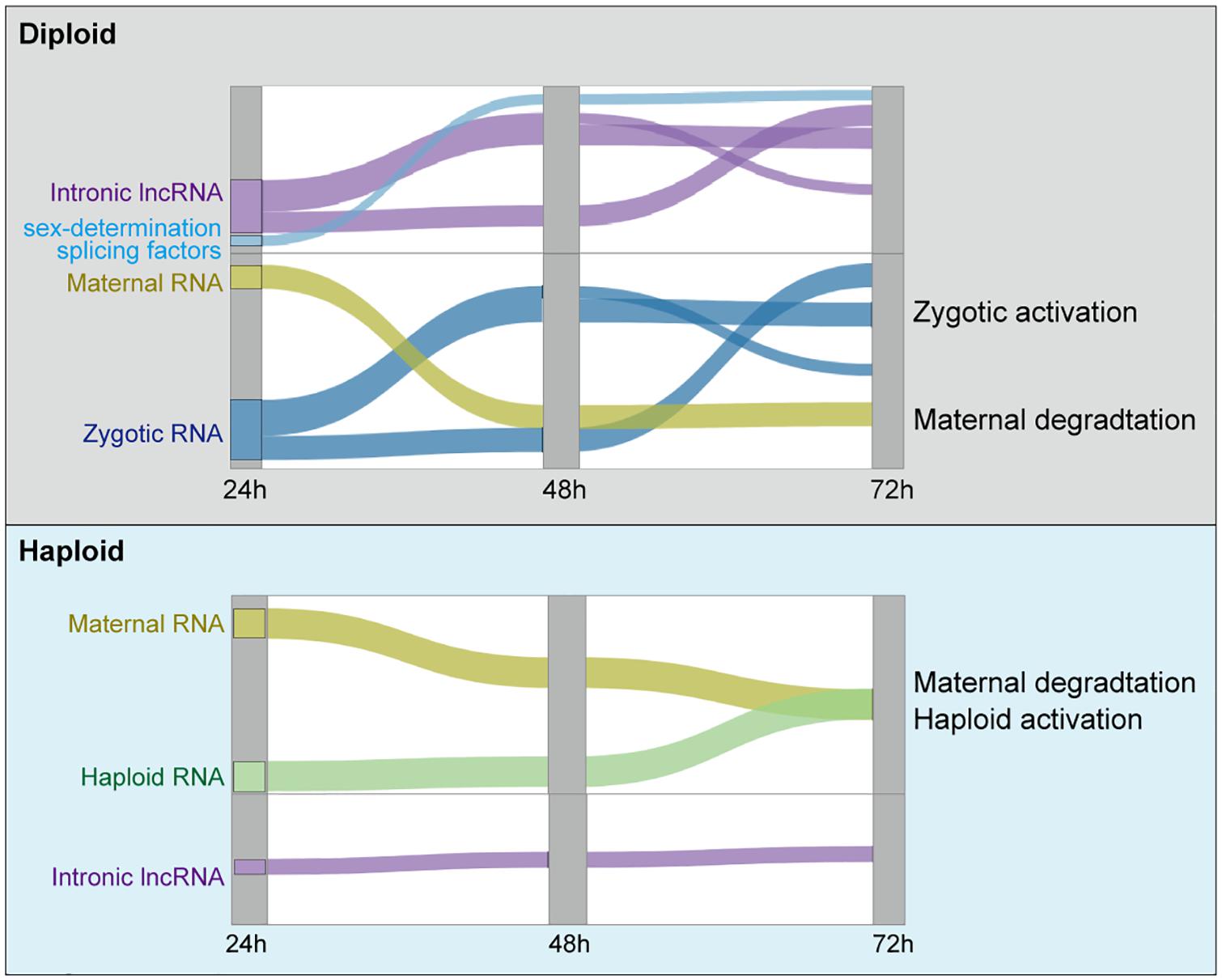
Graphical Abstract. Current working model of the three waves of ZGA in diploid embryos, and the slow maternal degradation in haploid embryos.
Introduction
In metazoans, the early stage of embryonic development following fertilization is instructed by the maternal RNAs and proteins from the female gamete, while the zygotic genome remains quiescent transcriptionally. Subsequently, a process known as zygotic genome activation (ZGA) occurs, and ZGA is known to tightly associated with the degradation of maternal instructions. This transcriptional switch drives the early development of all metazoans, and is known as the maternal-to-zygotic transition (MZT; Tadros and Lipshitz, 2009; Langley et al., 2014; Lee et al., 2014; Despic and Neugebauer, 2018). Recent progresses in single-cell RNA-seq and single-cell imaging in the living embryos of model organisms have advanced our understanding of spatio-temporal transcription and chromatin dynamics during the MZT to unprecedented depths (Briggs et al., 2018; Farrell et al., 2018; Wagner et al., 2018). Notably, current knowledge about ZGA during embryonic development has largely been obtained from studies of fertilized eggs; so, it remains unclear how naturally unfertilized eggs coordinate their genomic activation and embryonic development, as for example in haplodiploid animals like rotifers, spider mites, and honeybees.
About 15–20% of animal species are haplodiploid (Bull, 1983; Hedrick and Parker, 1997). In haplodiploid animals, the fertilized diploid embryos generally develop into females while unfertilized embryos develop into males. Western honeybees (Apis mellifera) are probably the best-studied haplodiploid animals; these well-known social insects are a model for ecological and social behavioral studies and are also of enormous economic importance because of their essential contributions to pollination in agriculture (Robinson et al., 2005; Honeybee Genome Sequencing., 2006; Mattila and Seeley, 2007; Wallberg et al., 2014). Under natural conditions, the queen very precisely deposits two different types of eggs into hexagonal differentially sized cells of a comb: fertilized (female/worker; diploid) eggs are placed into smaller cells, while unfertilized (male/drone; haploid) eggs are placed into larger cells (Ratnieks and Keller, 1998). The embryonic stage of honeybees – lasting about 70 h – starts after the egg is laid (“AEL”) by the queen and ends before the new larvae hatch (Fleig and Sander, 1985, 1986). The developmental times for development from embryos to through the larval and pupal stages before emerging as adults differs markedly for queens (16 days), workers (21 days), and drones (24 days), although there are no phenotypic differences in embryos or throughout the first four larval stages (Bertholf, 1925; Rembold et al., 1980).
It seems to generally be the case in insects that the sex determination occurs based on alternative splicing (AS) regulation of the sex-determination genes (SDGs) (Gempe and Beye, 2011), which have been exemplified in fruit fly and honeybee (Salz, 2011). In the model species Drosophila, transcriptional activation of the sex-determination splicing factor Sex lethal (Sxl) occurs in female embryos but not males (Bell et al., 1988). Sxl controls AS of transformer (tra), which (together with tra2) in turn controls the AS of the doublesex (dsx) in a female-specific manner (Schutt and Nothiger, 2000; Salz and Erickson, 2010). The sex determination mechanism of honeybee (A. mellifera) is partly conserved with Drosophila in AS hierarchy, but have its haplodiploid-specific SDGs. There are two honeybee-specific SDGs – complementary sex determination (csd) and feminizer (fem) – that control the female-specific AS of Am-dsx, thus determining the female developmental pathway in honeybee during embryonic development (Beye et al., 2003; Hasselmann et al., 2008). Both csd and fem encode SR-type splicing factors which are tra homologs (Beye et al., 2003; Hasselmann et al., 2008; Gempe et al., 2009). Two copies of Sxl are present in A. mellifera, but they all have no obvious sex-determining function despite being conserved (Dearden et al., 2006). Am-Tra2 proteins are required to promote female splicing of fem and Am-dsx as well as the male splicing of fem, which are distinct from its function in Drosophila (Nissen et al., 2012). So far, the specificality of haplodiploid animals in sex-determination remains further revealed.
An understanding is emerging that the complexity of the transcriptional landscape in all animals is increased by the presence of long non-coding RNAs (lncRNAs) (Quinn and Chang, 2016). LncRNAs are noncoding RNA sequences of > 200 nucleotides, and these molecules can act as signals, decoys, guides, or scaffolds to regulate the expression of their target genes (Devaux et al., 2015). In addition to the intergenic regions and antisense strands of protein coding genes, intronic regions have been proposed as reservoirs for sequences encoding lncRNAs (Morris and Mattick, 2014; Mattick and Rinn, 2015). For example, the intronic lncRNA CHRF acts as a decoy of miR-489 and thereby functions to stimulate cardiac hypertrophy (Wang et al., 2014). Tadano et al. (2009) reported a honeybee lncRNA, Nb-1, which is encoded by the antisense strand of an intron of another multi-exonic lncRNA; its expression dynamics in worker (female) brains are associated with the division of labor in normal colonies (Tadano et al., 2009). LncRNAs are highly regulated during embryonic development (Batista and Chang, 2013; Fatica and Bozzoni, 2014) as well as in the post-natal brain development, wherein sex-specific expression of lncRNA expression has been observed (Liu et al., 2017). LncRNAs expressed in the promoter region has been reported to influence Sxl expression in Drosophila (Mulvey et al., 2014). The retrotransposon LINE1 specifically expressed in the pre-implantation mouse embryo was recently reported to encode a nuclear RNA regulating transcriptional program specific to the mouse 2-cell embryo (Percharde et al., 2018). LINE RNA represses the DUX-family transcription factors that regulate ZGA in placental mammals (De Iaco et al., 2017). Further, in female mouse cells, Xist encodes a non-coding RNA that initiates the chromosomal silencing process of X inactivation early in embryonic development [at the 4- to 8-cell stage during which ZGA occurs (Wutz et al., 2002; Jeon and Lee, 2011; Chu et al., 2015; McHugh et al., 2015; Wang et al., 2016; Borensztein et al., 2017; da Rocha and Heard, 2017; Sunwoo et al., 2017; Sahakyan et al., 2018]. However, any involvement of lncRNAs in sex-determination and embryogenic development of haplodiploid animals has not been reported yet.
In this study, we used a single-embryo RNA-seq strategy to explore the transcriptional dynamics of both mRNAs and lncRNAs, with the aim of characterizing the differences between the diploid female and haploid male A. mellifera embryos. We sampled embryos at 24, 48, and 72 h AEL from both larger and smaller cells. We defined three waves of female-specific ZGA of thousands of mRNAs and intronic lncRNAs between 24 and 72 h AEL, wherein the transcriptional activation of csd, fem, and dsx occurred between 24 and 48 h. The haploid genome activation occurs 1 day later, lacking the activation of female-specific SDGs and lncRNAs. Instead, expression of the highly abundant Nb-1 was increased during haploid (male) genome activation. Moreover, intron-retention shows strong sex-specific dynamics during embryonic development.
Materials and Methods
Sample Collection
The honeybee, A. mellifera, colonies were maintained at the Institute of Apicultural Research, Chinese Academy of Agricultural Sciences, Beijing, China, according to standard beekeeping technique. To control the egg laying, a mated queen was confined on an egg-free frame for 3 h before placed back into the hive. Developing Embryos were collected at 24, 48, and 72 h after laying, and 3 embryos (biological replicates) were sampled for each time point. Worker (female) and drone (male) embryos (Supplementary Figure 1B) were collected from the distinct type of frame (Supplementary Figure 1A) laid by the same queen. We sampled two groups of worker (female) and drone (male) embryos laid by two different honeybee queens. Then a total of 36 embryos were collected for single-embryo RNA-seq.
Whole Mount in situ Hybridization
Embryos were fixed in 4% paraformaldehyde for 4 h, dehydrated in methanol, and stored in 100% MeOH at −20°C until use. Samples were rehydrated, pretreated with proteinase K, and hybridized with DIG-labeled RNA probes followed by washing with 2 × SSC/50% formamide three times at 70°C. The signal was detected using an alkaline phosphatase-conjugated anti-DIG antibody (11093274910; Roche). Tissues were incubated in the BM Purple alkaline phosphatase substrate (11442074001; Roche) at 4°C for several hours until the signal developed to the desired extent. Probes for each gene were generated using DIG RNA Labeling Kit (11 175 025 910; Roche). Primers for probe generation were listed in Supplementary Table 4.
Real-Time Quantitative Polymerase Chain Reaction (PCR)
In this study, to elucidate the validity of the RNA-seq data, real-time quantitative PCR (RT-qPCR) was performed for some selected genes, and normalized with an external reference sequence. The same RNA samples for RNA-seq were used for qRT-PCR. The PCR conditions are consisted of denaturing at 95°C for 10 min, 40 cycles of denaturing at 95°C for 15 s, annealing and extension at 60°C for 1 min. PCR amplifications were performed in triplicate for each sample.
Meanwhile, RT-qPCR assay was used to analyze alternative polyadenylation sites (APAs) for tra2 gene. To detect one of the alternative isoforms, one primer is designed in the alternative exon, and an opposing primer is designed in a constitutive exon. To detect the other of the alternative isoform, a boundary-spanning primer for the sequence encompassing the exon–exon junction with the opposing primer in a constitutive exon is used. Relative quantification was achieved by normalization to the amount of internal reference with the 2–ΔΔCt method (Livak and Schmittgen, 2001). We have followed the MIQE guidelines to present RT-qPCR results (Bustin et al., 2009). Primers for qPCR analysis were listed in Supplementary Table 4.
Library Construction
High-quality, full-length cDNA was generated directly from single honeybee embryo by the SMART-Seq v4 Ultra Low Input RNA Kit for Sequencing (Cat. Nos. 634890). cDNA was amplified by LD PCR. RNA can be transcribed by T7 promoter from ds cDNA, RNA was treated with RQ1 DNase (promega) to remove DNA. Quantity of the purified DNA were determined by Qubit. For each sample, 200 ng RNA was used for RNA-seq library preparation. RNAs were iron fragmented at 95°C followed by end repair and 5′ adaptor ligation. Then reverse transcription was performed with RT primer harboring 3′ adaptor sequence and randomized hexamer. The cDNAs were purified and amplified and PCR products corresponding to 200–500 bps were purified, quantified and stored at −80°C until used for sequencing.
For high-throughput sequencing, the libraries were prepared following the manufacturer’s instructions and applied to NextSeq 500 system for 151 nt pair-end sequencing (ABlife Inc.). Six biological replicate RNA-seq samples from two honeybee queens were obtained for each of the three time points.
Raw Data Processing
The raw reads were first removed of their adaptor sequence by cutadapt (Martin, 2011)(v1.8.1). The sense and antisense of t7 promoter were also cut. To remove other contamination during library construction, the left and right most 15 nt bases were also removed. The bases whose quality were lower than 20 were also removed by FASTX-Toolkit (v0.0.14). The reads length less than 16 nt were filtered. Last, the front 65 bp of the reads were kept as cleaned reads. The filtered reads were then mapped to the A. mellifera (Amel_4.5) genome sequence (Honeybee Genome Sequencing., 2006) (Honeybee Genome Sequencing., 2006)1 by TopHat2 with read-edit-dist 4-N 4. We also aligned the filtered reads to the new genome assembly sequence of honey bee (Amel_HAv3) (Wallberg et al., 2019), and found only one percentage improvement of total aligned reads. Because there was no untranslated region (UTR) annotation in new genome assembly, we used Amel_4.5 aligning result and only extracted the reads that were unambiguously aligned to the genome. We then calculated reads number and RPKM value (RPKM represents reads per kilobase and per million) for each gene (Mortazavi et al., 2008).
Measure of Poly-A Tails Length
To measure of poly-A tails length, we used a published method, named pA-finder (Yu et al., 2020). Sequencing reads with poly-A tails were kept for analysis. Preliminary poly-A regions were defined with at least 5A sequences at both sides. Other parameters were the same as described in Yu et al.
Differentially Expressed Genes Analysis
After getting the expression level and reads of all genes in all samples, differentially expressed genes between the paired groups were analyzed by using edgeR (Robinson et al., 2010) embedded in R software. We calculated the significance p-value based on the model of negative binomial distribution for each gene. We also estimated fold changes of gene expression within the edgeR statistical package. The threshold value for Differential Expressed Gene (DEG) has been set as fold change > 2 or < 0.5 and p-value < 0.01.
Alternative Splicing Analysis
The splicing junction (SJ) reads with gaps while aligned to the genome by TopHat2 were obtained. We defined known SJs from all annotated isoforms in honeybee genome annotation and others not matched annotated junction sites are novel SJs. The reads walking through the site and each side of intron boundary region with no less than 8 nt were defined as boundary reads. The junctions located inside the coordinates of annotated genes were regarded as genic SJs, which can be classified into one of the nine types of AS events (ASE). Seven of the canonical ASEs were skipped exons (ES), cassette exon (CE), alternative 5′-splice sites (A5SS), alternative 3′-splice sites (A3SS), mutually exclusive exons (MXE), alternative first exons (AFE or 5′MXE) and alternative last exons (ALE or 3′MXE) according to the models described previously (Wang et al., 2008). AS regulation analysis was performed by running in silico ABL as pipeline as previously described (Xia et al., 2017).
LncRNA Prediction and Direction Identification
To systematically analyze the lncRNA expression pattern, we used a pipeline for lncRNAs identification similar as we previously reported (Liu et al., 2017), which was constructed based on the cufflinks software (Trapnell et al., 2012). All steps of the pipeline have been shown in Supplementary Figure 2C. We calculated coding potential score (CPS) to filter the coding potential transcripts (Kong et al., 2007). When filtering the single exon lncRNAs, we set two thresholds: 1,000 nt to obtain longer single exon lncRNAs, and 500 nt to keep more single exon lncRNAs.
We then used the polyadenylation signal to detect the transcriptional direction of lncRNAs. Firstly, we selected reads whose tail was with more than 10 A or T, allowing 0.1 error rate. Then we aligned the reads longer than 20 nt to the genome sequence. We discarded the aligned reads if their poly (A) tails come from the genomic sequence (internal polyA). The terminal aligned positions of reads were clustered together if they were located within 20 bp on the genome locus, and the clusters were regarded as polyadenylation sites (PASs). We then compared the genomic locations of predicted lncRNAs and PASs. If the PASs were located at the downstream of lncRNAs, the direction of lncRNAs were the same with reference direction, and vice versa. If there were poly (T) sites, the direction of lncRNAs was reversed.
Co-expression Analysis
To fully understand the gene expression pattern during embryogenesis of honeybee, we applied weighted gene co-expression network analysis (WGCNA; Langfelder and Horvath, 2008) to cluster genes that have similar expression pattern with default parameters. All expressed genes were used as input data. Eigengenes for each clustering module was used as the representative expression pattern of genes in each module. To explore the regulatory mode between lncRNAs and their host mRNAs, we calculated the Pearson’s correlation coefficients (PCCs) between them and classified their relation into three class: positive correlated, negative correlated and non-correlated based on the PCCs value.
Drosophila Transcriptome Analysis
We analyzed the transcriptome data of Drosophila from Lott et al. (2011). Transcriptome data were aligned to Drosophila genome (BDGP6.22). Gene annotations were download from Ensembl database (Ensembl release 95). The analysis pipeline was the same as transcriptome data from honeybee. Sex-determination genes were annotated by blast software (Altschul et al., 1990).
Other Statistical Analysis
Grouped by the female and male during the different embryonic development, the RPKM value of all the genes in all samples were used to conduct the principal component analysis (PCA), which was performed by R package prcomp (Ma and Dai, 2011) to show the clustering of samples with the first two components. After normalizing the reads by TPM (tags per million) of each gene in samples, in house-script (sogen) was used for visualization of next-generation sequence data and genomic annotations. The pheatmap package1 in R was used to perform the clustering based on Euclidean distance. To assess the functional enrichment of a given gene set, we aligned the protein sequence of honeybee to the gene ontology (GO) and KEGG databases. Then we used hypergeometric test to calculate the enrichment of a given gene set, and all genes were regarded as background.
Results
Activation of Developmental Transcription Programs Differs Between Diploid Female and Haploid Male Embryos
A previous study of seven transcriptomes from honeybee (A. mellifera) within 24 h AEL only revealed zygotic activation of a small number of genes (Pires et al., 2016). To study ZGA dynamics in honeybee, we here used single-embryo RNA-seq to explore the transcriptional landscape and differences between diploid female and haploid male honeybee embryos from 24 to 72 h AEL. The single-embryo RNA-seq data we analyzed from the developing Drosophila embryos for mitotic cycles 10–14, thereby collectively covering the embryonic stages after cleavage up until the completion of cellularization.
Our single-embryo RNA-seq approach was designed to study the transcriptional dynamics of both mRNAs and lncRNAs in male and female honeybee embryos (Figure 1A) (see section “Materials and Methods”). A total of 36 single-embryo RNA-seq transcriptomes were generated from embryos laid by 2 queens (P1 and P2). Among the 18 transcriptomes from each of the two queens, three female embryos (F) were collected from the female cells and three male embryos (M) from the male cells at each time point of 24, 48, and 72 h AEL (Supplementary Figures 1A,B). The sequencing depth and expression profiles of the 36 single-embryo RNA-seq transcriptomes are summarized in Supplementary Tables 1, 2, respectively. In each sample, around 73–89% of honeybee genes were detected (RPKM > 0.1). Gene expression was normalized using a dividing size factor by the DESeq method (Anders and Huber, 2010). The 75th percentile levels of genes were identical between samples after normalization (Supplementary Figure 1C), similar with previously published single-embryo RNA-seq data from Drosophila (Lott et al., 2011) (Supplementary Figure 1D). Almost all of the currently known lncRNAs (99.26%, 2412/2434) and most of the annotated protein coding genes (98.23%,10436/10623) were expressed at least in one honeybee embryonic sample (Supplementary Figures 1E,F), indicating that single-embryo RNA-seq data from the developing honeybee embryos was of sufficient quality to enable further study of their mRNA and lncRNA transcriptomic dynamics. We analyzed the dynamics of the polyA-tails of embryonic transcripts by counting the A numbers of reads aligned to the end of transcripts (Yu et al., 2020), demonstrating that the poly(A) tail lengths of male embryos increased during embryonic development while those of female embryos did not change (Figures 1B–D).
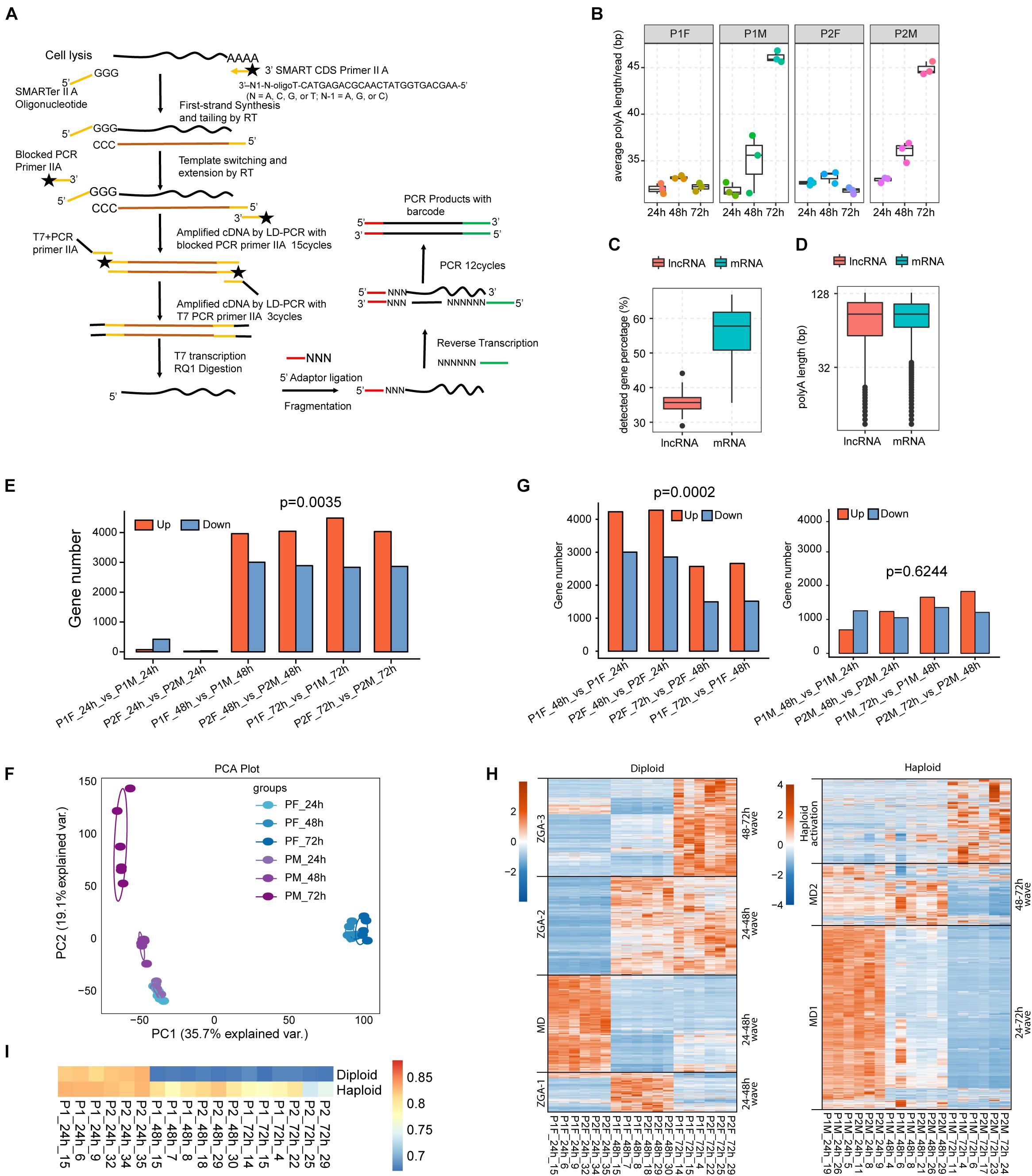
Figure 1. The difference in the developmental transcription program activation between diploid female and haploid male embryos. (A) Schematic plot showing the workflow of single-embryonic library construction. (B) Box plot of average poly (A) length showing that the poly (A) length of male embryos increased during embryonic development (p-value < 0.001, one way ANOVA test for both datasets), while that of female kept constant (p-value > 0.01, one way ANOVA test for both datasets). (C) Box plot showing that the percentage of lncRNAs with poly (A) tails was lower than that of mRNAs (p-value < 0.0001, t-test). (D) Box plot of the poly (A) length showing that there was no significant difference of average poly (A) length (middle line in box plot) between lncRNAs and mRNAs (p-value > 0.05, t-test). (E) Barplot of DEGs between male and female embryos. More up-regulated DEGs were observed in female embryos after 24 h (up-regulated DEGs number vs. down-regulated DEGs number, p-value = 0.0035, t-test). (F) PCA analysis showing the divergent gene expression pattern between male and female embryos after 24 h. (G) Barplot of DEGs between adjacent time points for female (left) and male (right) embryos, respectively. More up-regulated DEGs were observed in female embryos during development (up-regulated DEGs number vs. down-regulated DEGs number, p-value = 0.0002, t-test). (H) Hierarchical clustering heatmap showing the expression waves of all DEGs detected in this study. Male and female samples were separated for the plotting. Expression waves were separated by black lines. The dendrogram was not shown. MD: maternal degradation. (I) The Pearson’s correlation coefficients (PCCs) was shown by heatmap for each of the single-embryonic samples with the published oocyte sample (Pires et al., 2016).
Principal component analysis, PCCs, and DEG analysis based on the edgeR method (Robinson et al., 2010) using FDR < 0.05 and |log2FC| > 1 were performed to explore major transcriptional trends during honeybee embryonic development (Figures 1E,F and Supplementary Figures 2A,B). The transcriptomes of all 12 embryos, 6 for each sex, at 24 h AEL were closely clustered, and they were highly distinct from all the other embryos; the latter transcriptomes for both female and male embryos subsequently exhibited tremendous divergence in their expression patterns (Figure 1F and Supplementary Figures 2A,B). Diploid female embryos (both 48 and 72 h) clustered and were well-separated by the first component (35.7% explained variation), while the haploid male embryos were separated by the second principal component (19.1% explained variation) (Figure 1F).
DEG analysis revealed that more genes were significantly upregulated than downregulated between 48 and 24 h, and between 72 and 48 h (p-value = 0.0002, t-test), and the similar results were observed for two independent sample groups (n = 2) laid by two different queens (Figure 1G, left panel). These findings are consistent with strong transcriptional activation during female embryonic development. Hierarchical clustering analysis revealed three waves of female-specific activation: that proceeded from 24 to 48 h AEL (Wave 1, 916 genes), from 24 to 72 h AEL (Wave 2, 2,731 genes), and from 48 to 72 h AEL (Wave 3, 2,649 genes) (Figure 1H, left panel). Wave 1 is characterized by a strong activation between 24 and 48 h AEL, which was then re-silenced after 48 h AEL. In contrast, the activation in Wave 2 lasted until 72 h AEL. The activation in Wave 3 primarily occurred between 48 and 72 h AEL (Figure 1H, left panel).
In male embryos between 48 and 24 h AEL, there were about 2 × more differentially expressed downregulated genes (569) than upregulated genes (Figure 1G, right panel), supporting the idea of maternal repression rather than a “haploid activation” (Figure 1G, right panel). Moreover, the downregulation of maternal RNA occurred more slowly in haploid than in diploid embryos (Figures 1F,I). The poly(A) tail lengths of female embryos (Figures 1B–D) were also consistent with slower maternal degradation. There was a single activation wave in the haploid embryos that occurred between 48 to 72 h AEL (Figure 1H, right panel).
A Majority of the lncRNAs Expressed During Embryonic Development Are Intronic
Before we profiled the expression dynamics of embryonic honeybee lncRNAs, we decided to perform a de novo lncRNA prediction from the 36 single-embryo transcriptomes. We here adopted a cufflinks-based lncRNA identification procedure similar to a previous report (Liu et al., 2017) (Supplementary Figure 2C). We thusly identified 902 multi-exonic lncRNAs and 4,191 candidate single-exonic lncRNA with a minimum length of 1,000-nt. Most of the single-exonic lncRNAs (91.9%) were derived from the intronic regions, and 51.1% of multi-exonic lncRNAs were from the intronic regions. Note that most of the annotated lncRNAs from honeybees reported in previous studies are multi-exonic; only 36.7% were reported to be from intronic regions (Figure 2A).
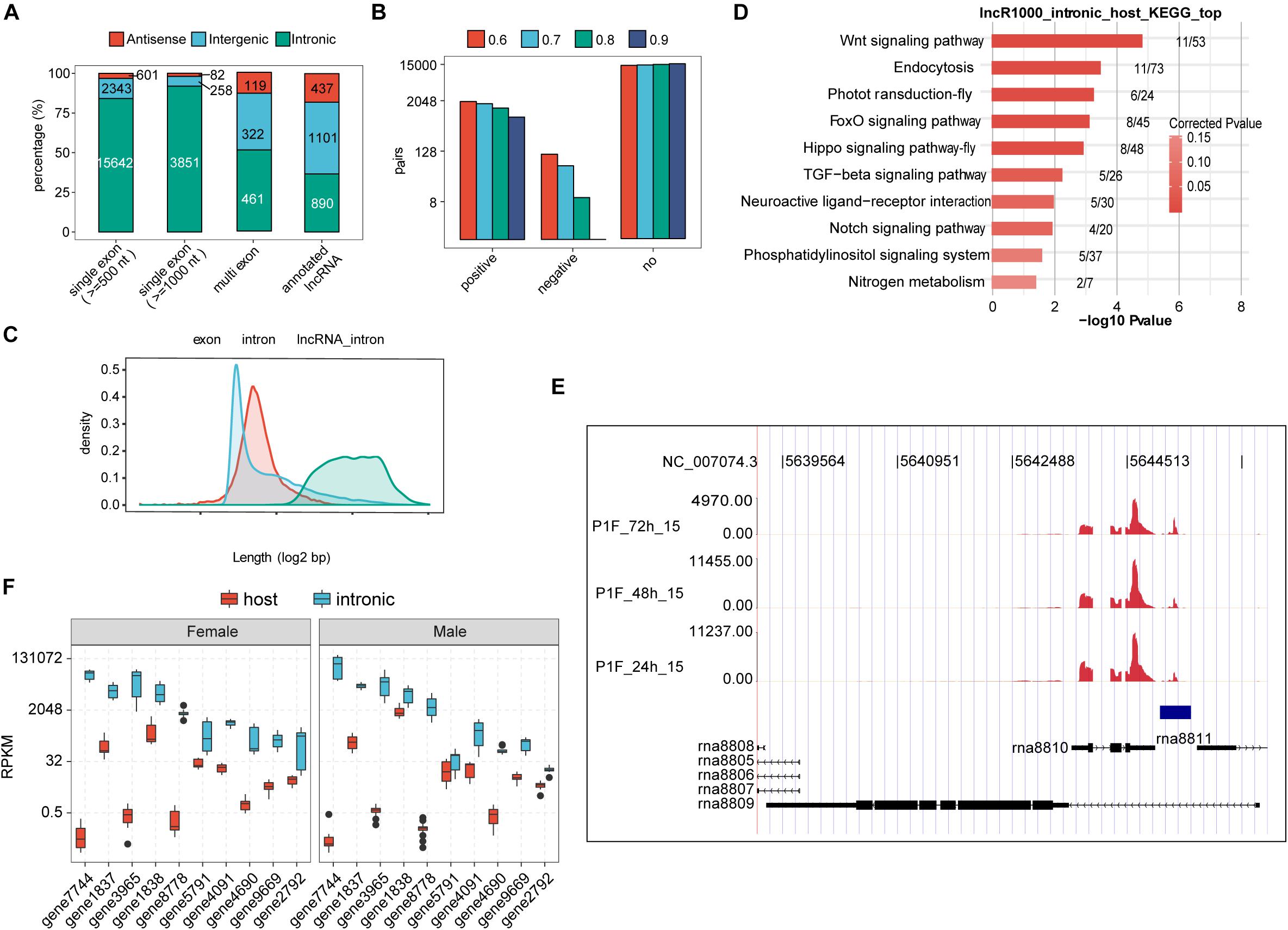
Figure 2. Embryonic honeybee lncRNAs are dominantly intronic. (A) Bar plot showing the genomic locus distribution of the newly identified and previously annotated lncRNAs in honeybee. Single exonic lncRNAs were selected with two thresholds (1,000 and 500-nt). The genomic distribution of the newly identified single-exonic, multiple-exonic lncRNAs and the annotated lncRNAs is shown. (B) Bar plot showing the number of co-expressed pairs between intronic lncRNAs and their host mRNAs by PCC calculation. Four PCC thresholds were shown in the figure. No indicated the pairs that were not satisfied with the threshold. (C) Density of the length distribution of exons and introns of honeybee. Introns containing lncRNAs (500-nt threshold for single exon lncRNAs) were plotted separately. The length density distribution was generated by density function in R. (D) The top 10 functional enriched KEGG pathways of genes encoding intronic lncRNAs (1,000-nt threshold for single exon lncRNAs). (E) Reads density plot showing the prevalent transcription in intronic region. Blue frame indicates the newly predicted transcript in the intronic region of rna8809. The annotated intronic lncRNAs rna8810 and rna8811 were transcribed extensively as well. (F) Box plot showing the higher expression levels of intronic transcripts than their host exonic mRNAs in both female and male embryos.
Interestingly, the previously reported lncRNA Nb-1, whose expression dynamics in worker brains was associated with the division of labor in normal colonies, is 599-nt in length, single-exonic, and located in the intronic region of an annotated multi-exonic lncRNA (LOC102654021) (Tadano et al., 2009). Seeking to identify lncRNAs with similar characteristics to Nb-1 lncRNA, we retained all of the predicted single-exonic lncRNA genes longer than 500-nt; 18,568 single-exonic lncRNAs, including Nb-1, were thusly obtained (Figure 2A). Notably, the same criteria resulted in a dramatically smaller number of novel Drosophila embryonic lncRNAs (Supplementary Figure 2D). Most of the annotated Drosophila lncRNAs were single exonic, and generally smaller than 1,000-nt in length (Supplementary Figure 2E). Our results thus indicate that Drosophila embryos expressed an order of magnitude fewer lncRNAs than do honeybee embryos.
To validate the authenticity of predicted lncRNAs and investigate the relationship between lncRNAs and their host gene, we further analyzed the poly(A) tail sequences at the 3′-end to determine the lncRNA direction (Supplementary Figure 2F). Because sequences with poly (A) tail were only a small fraction of total aligned reads, we thus only determined the transcription direction of 1,382 (7.4%) lncRNAs. Among these, 1,159 were intronic lncRNAs, and 1,143 were from protein-coding genes. Interestingly, 37.2% (310) were expressed from the antisense direction of a protein coding gene (Supplementary Table 3), suggesting an independent expression manner of these lncRNAs. Based on gene annotation of honeybee, half of the genome sequences are intronic regions (Supplementary Figure 2G), with a large population (27,602, 14.47%) longer than 2,000 nt. The majority of honeybee lncRNAs expressed during embryonic development were from long introns (Figure 2C). Expression correlation analysis showed that the expression of most (over 85%) intronic lncRNAs was not correlated with the expression of their host genes (Figure 2B and Supplementary Figure 3A), indicating their independent transcription from their host genes. By dividing single exon lncRNAs into different classes according to their expression, we found the number of higher expressed lncRNAs was not decreased but increased in female samples (Supplementary Figure 3B). Notably, the host gene containing intronic lncRNAs in honeybee embryos were highly enriched in developmental-control related signaling pathways (Figure 2D). Among the top-10 genes whose introns harboring mostly expressed lncRNAs, four encode neuron-related functions, including Neurexin 1 (gene3965), neuromusculin (gene8778), discs large 1 (gene9669), and an RNA binding protein RBFox1 (A2bp1, gene2792). The expression from these 10-top host gene exons was about one-to-six orders of magnitude lower that from their intronic RNA regions (Figures 2E,F).
Honeybee lncRNAs Are Extensively Activated in Female but Not Male Embryos
We then profiled the expression dynamics of embryonic honeybee lncRNAs. We observed that the detected number of lncRNAs was increased during the developmental course in female embryos but decreased in male embryos (Figure 3A). This large sex-difference was not observed in Drosophila (Figure 3B). PCCs and PCA analyses of the expression profiles of lncRNAs showed that their expression patterns in female honeybee embryos at 24, 48, and 72 h AEL were highly divergent. In contrast, the global lncRNA expression patterns in male embryos were strikingly similar (Figure 3C and Supplementary Figure 2C). Heatmap clustering also revealed the globally-activated expression of honeybee lncRNAs specifically in female embryos, although maternally repressed lncRNAs were similar in both female and male embryos (Figure 3D). Importantly, there were three obvious waves of lncRNA activation for 24–48, 24–72, and 48–72 h, just like what we observed in the mRNA data for diploid females (Figures 3D, left, 1H, left). Statistics of the lncRNAs specifically activated in zygotic female embryos showed that 72.7% of them were transcribed from intronic regions and 64.6% were specifically expressed in female embryos (Figure 3E).
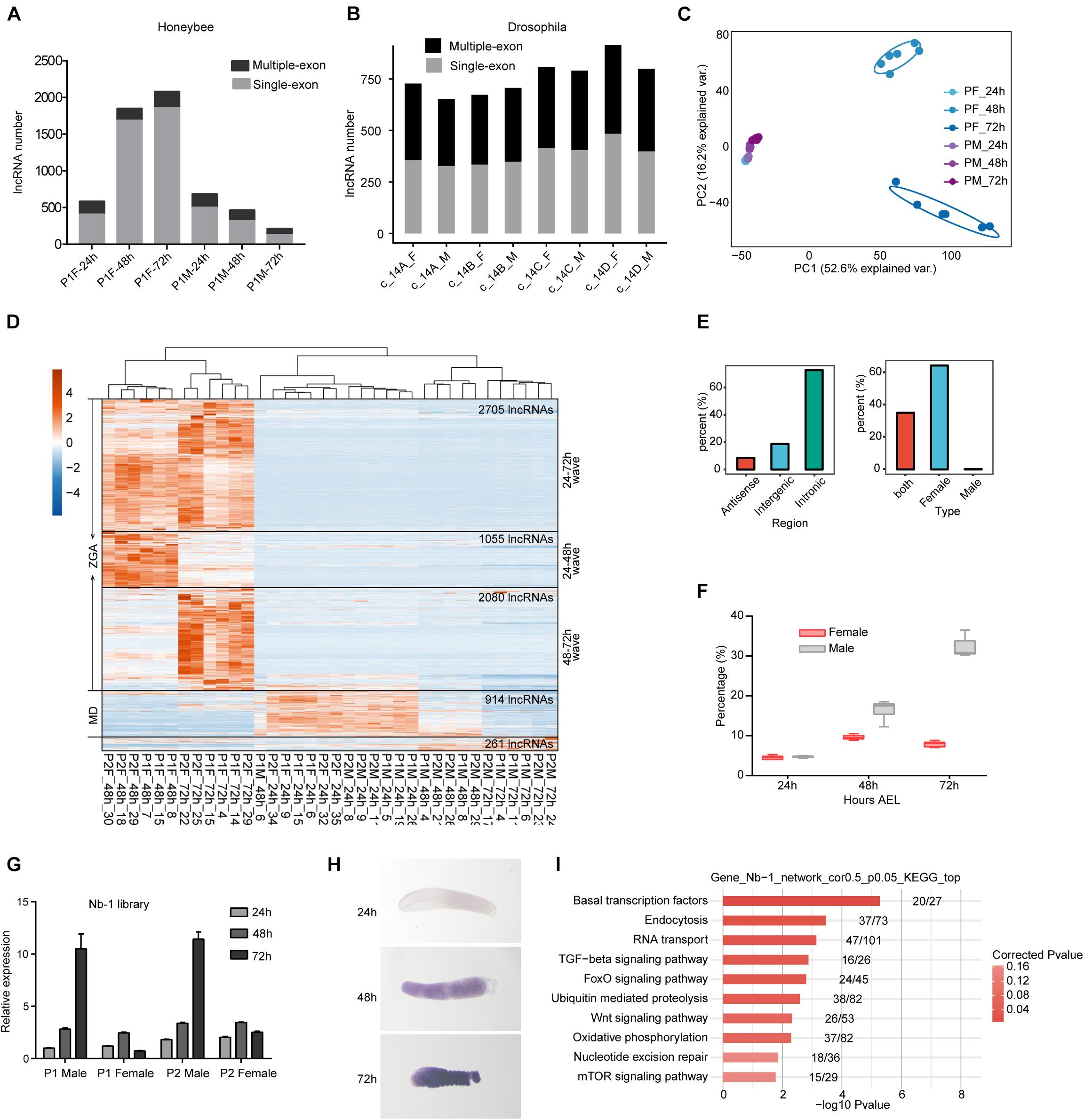
Figure 3. LncRNAs are extensively activated during the embryonic development of honeybee female embryos. (A) The numbers of detected lncRNAs (1,000-nt threshold for single exon lncRNAs) were increased in female embryos, and decreased in male embryos. In each sample group, we randomly selected 20 million mapped reads from each sample to perform lncRNAs prediction pipeline. Results of 18 samples from one queen were shown. The results of other 18 samples were similar. (B) The numbers of detected lncRNAs were not obviously changed in female and male embryos of fruit fly. The same analysis method was executed as in panel (A). (C) PCA analysis showing the divergent lncRNA expression pattern specifically for female embryos after 24 h (1,000-nt threshold for single exon lncRNAs). (D) Hierarchical clustering heatmap showing the zygotic expression waves of lncRNAs in female embryos (1,000-nt threshold for single exon lncRNAs). (E) The percentage of intronic lncRNAs (left) and female specific lncRNAs (right) among the zygotic activated lncRNAs shown in panel (D). (F) Boxplot showing the increased percentage of Nb-1 reads relative to the total reads aligned to gene regions in honeybee female and male embryos. (G) RT-qPCR validation of Nb-1 expression showing high consistence with the sequenced libraries. (H) In situ hybridization experiment validated the robust increase of Nb-1 expression in male embryos. (I) Bar plot showing the top ten enriched KEGG pathways for genes whose expressions were correlated with Nb-1 (p-value < 0.05 and PCCs > 0.5).
The most highly expressed lncRNAs in both female and male was Nb-1 lncRNA, which occupied as high as 36.49% of the total reads mapped to the previously annotated gene regions (Figure 3F). Strikingly, Nb-1 expression was increased in both sexes at 48 h AEL; however, its expression increased robustly and specifically in male embryos at 72 h AEL (Figure 3F). We validated the male-specific increase in the expression of Nb-1 by both qPCR and in situ hybridization (ISH) experiments (Figures 3G,H). Although Nb-1 expression was highly regulated, expression of its host gene was consistently low (Supplementary Figure 3C). To predict potential targets of lncRNA Nb-1, we used co-expression network analysis, and revealed a negative correlation between Nb-1 and 95% of its co-expressed genes (p-value < 0.05 and | PCCs| > 0.5). The co-expressed genes showed enrichment for basal transcription factors, endocytosis, RNA transport, as well as for several developmental-control signaling pathways (Figure 3I), implying the potential functions of Nb-1 in embryonic development of honeybee.
The Dynamics of Embryonic Intron Splicing Are Associated With the Expression of Known Sex-Determination Splicing Factors Like csd, fem, tra2, and Sxl
Our observation of a large population of intronic lncRNAs, in both sense and antisense strands, suggests that there may be interplay between intron splicing and lncRNA expression. Interestingly, global splicing overview revealed that splicing efficiency was relatively constant in diploid females but was time-dependently reduced in haploid males during embryonic development (Figure 4A). Further, the detected AS events were constant in females, but substantially decreased in male embryos, and did so in a time-dependent manner (Figure 4B). The large difference in the AS activity between female and male embryos was coincident with the activation of thousands of lncRNAs in female but not male embryos.
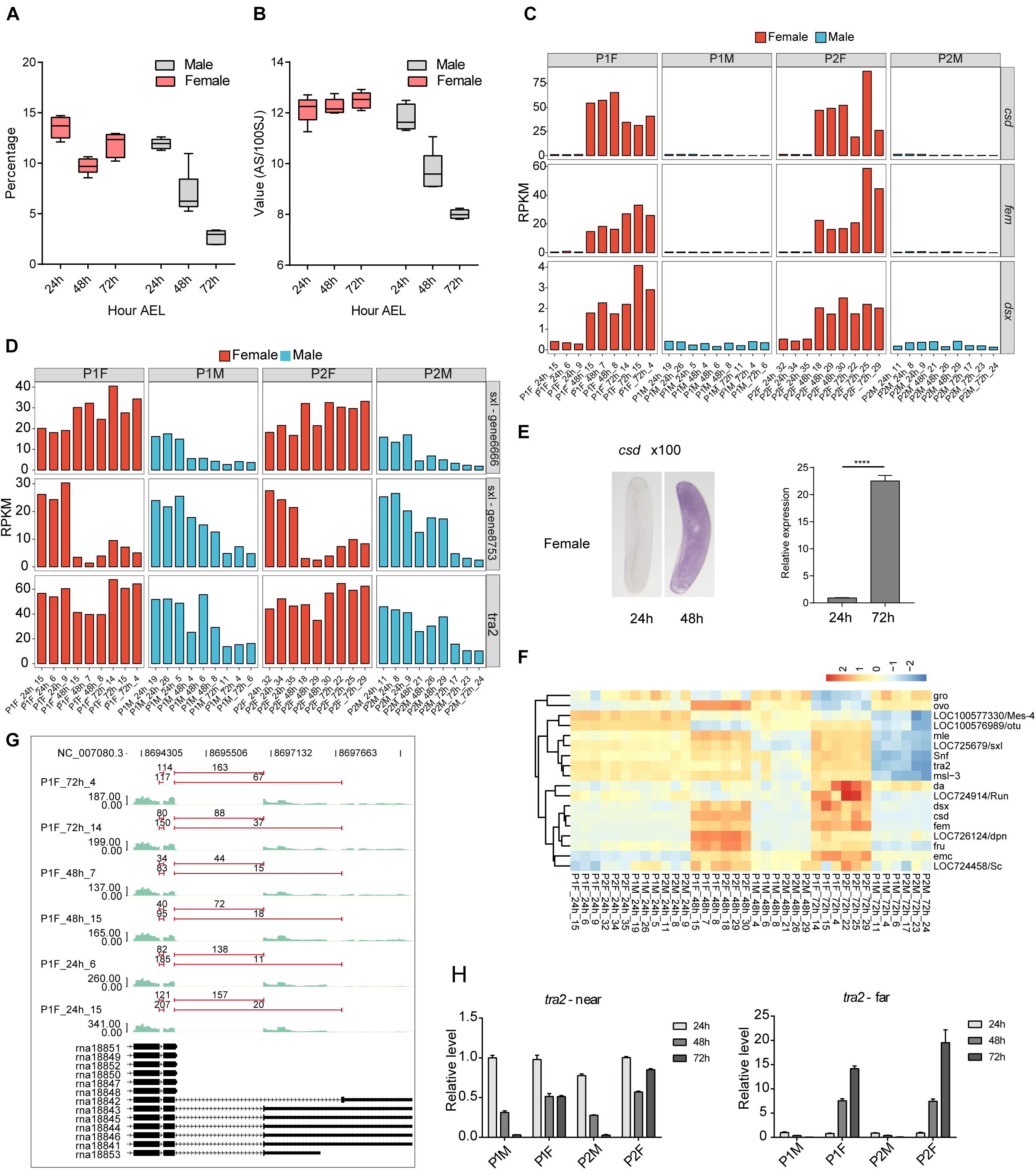
Figure 4. Alternative splicing (AS) is regulated in a sex-specific pattern during honeybee embryonic development. (A) Boxplot showing the percentage of spliced reads among total uniquely mapped reads for each group. (B) Boxplot showing the detection efficiency of AS events in female and male eggs. We used the detected ASEs number per 100 SJs as the detection efficiency. (C) Bar plot showing the time-dependent expression dynamics of Sxl, tra, and dsx in male and female embryos. (D) Bar plot showing the time-dependent expression dynamics of tra2 and two copies of Sxl in male and female embryos. (E) ISH and RT-qPCR validation results of the increased expression level of csd gene after 24 h AEL in female embryos. The embryos were amplified by 100 times, and the purple color represented the intensity signal of csd (ISH, left). (F) Hierarchical clustering heatmap of the expression level of SDGs revealed two types of expression patterns: maternal expressed (upper cluster) and zygotic activated (lower cluster) (G) Reads density plot showing the APA regulation of tra2 transcripts in female embryos. The numbers above the red line indicate the counts of splicing junction reads. (H) Bar plot showed the RT-qPCR validation of APA usage for tra2 transcripts. Left panel showing the proximal PAS signal, and right panel for distal PAS signal.
We here compared our honeybee data to a published single-embryo transcriptomes of fruit fly (see section “Materials and Methods”) to delineate any links between the expression of SDGs and the expression or AS dynamics of mRNAs and lncRNAs at each of the embryonic developmental stages. In Drosophila, we noted that Sxl expression was low in both males and females at mitotic cycle 10–13, but was robustly activated at the 14th mitotic cycle specific in females (Supplementary Figure 3D), indicative of a strong sex-specific zygotic activation. Expression of tra2 showed no sex-specificity, showing a time-dependent maternal degradation in both sexes of diploid embryos (Supplementary Figure 3D). Expression of dsx was at a consistently very low level, yet zygotic activation occurred in both sexes of diploid embryos from mitotic cycle 11–14 in a time-dependent manner (Supplementary Figure 3D).
In honeybee embryos, csd, fem, and dsx showed very low expression at 24 h AEL, yet the expression of all three of these genes was strongly activated in diploids from 24 to 48 h AEL, which did not occur in haploids (Figure 4C). ISH and qPCR confirmed the induced expression of csd in females (Figure 4E). The expression of the other two aforementioned sex-determination splicing factors (tra2 and Sxl) was high in 24 h embryos and oocytes (Figure 4D and Supplementary Figure 3E), indicative of their maternal expression. We found evidence for the maternal degradation of transcript from one copy of Sxl gene (gene8753) in diploid honeybee embryos and for all the tra2 and Sxl genes in the haploid embryos. Note that the expression of the tra2 gene and the one Sxl copy (gene6666) was constantly high throughout embryonic development in female (Figure 4D). This robust transcriptional activation of the female-specific splicing factors csd and fem, viewed alongside the constantly high levels of tra2 and Sxl in female embryos (but not male embryos) from 24 to 48 h AEL, together support the findings that highly efficient splicing occurs, specifically in females, throughout embryonic development.
We also examined the expression levels of all 18 genes previously reported to function in sex-determination (Sanchez, 2008; Salz, 2011). Clustering analysis of their expression profiles revealed two distinct groups: one group appeared strongly influenced by maternal expression while the other seemed to be controlled by the female-specific activation waves (Figure 4F). To further verify this transcriptional profile, we re-analyzed our RNA-seq data in the context of a previously published proteome dataset from the embryos of A. mellifera that were collected at similar time-points during embryogenesis (Fang et al., 2015) and found that our data included mRNA transcripts corresponding to 98.48% of the proteins identified in that dataset (Supplementary Figure 3F). Notably in the proteome dataset, three SDG proteins – Gro, Snf, and an Sxl homolog (gene6666) – were detected at all stages of male and female embryos (Supplementary Figure 3G). However, the Tra2 protein was specifically detected at 48 and 72 h AEL only in female embryos, supporting the result from our single embryo RNA-seq.
Alternative splicing regulation of SDGs is known to be key regulatory influence during sex determination (Sanchez, 2008; Salz, 2011; Haussmann et al., 2016), so we detected AS events for the csd, fem, dsx and other SDGs in female embryos and found that splicing isoforms that were consistent with previous reports (Hasselmann et al., 2008; Gempe et al., 2009) (Supplementary Figure 4 and Supplementary Table 5). There are multiple AS events observed among these SDGs: csd contain 8 types of ASEs, including MXE, alternative 3′ splice site (A3SS), and intron retention events (IR); we only observed two IR events of fem in our data; dsx had ASEs of IR and alternative 5′ splice site (A5SSA) (Supplementary Table 5). The fruitless (fru) gene were reported functioning in the sex-specific neuronal differentiation of Drosophila (Ryner et al., 1996; Demir and Dickson, 2005). We also found the fru homolog in honeybee, but no obvious AS differentiation was observed between female and male embryos. We found 16 ASEs in fru, including the types of MXE, A5SSA, A3SS, and exon skipping (ES) (Supplementary Table 5). AS of Sxl and tra2 were similar in both female and male embryos (Supplementary Figure 4). However, female and male embryos differed significantly at their respective AS products with the last exon of tra2, which was present as two mRNA isoforms with APAs (Figure 4G, p-value = 0.003, Fisher’s exact test). At 24 h AEL, both female and male embryos primarily used the proximal 3′ss and polyadenylation site, leading to the dominant form of shorter 3′UTR. At 48 and 72 h AEL stages, the usage of the distal 3′ss and polyadenylation site in female embryos was significantly increased, but remained largely unchanged in male embryos (Figure 4G). RT-qPCR experiments validated the APA events as well as the specific usage of the APA site in female embryos (Figure 4H).
Discussion
Based on our results, we propose a model in which the inactivation of the extra set of chromosomes carried by diploid female honeybees may be controlled influenced by ZGA-specific lncRNA activation during embryonic development. Haploid male embryonic development lacks such a zygotic activation program; moreover, transcriptional activation is weak in haploids, and maternal degradation occurs more slowly than in diploids, which is also observed in Drosophila embryos (Bashirullah et al., 1999), suggesting a possible similar mechanism of RNA degradation in Drosophila and honeybee. During diploid embryonic development, at least three waves of ZGA occur, with the expression of thousands of protein coding genes. The ZGA-activated expression of sex-determination splicing factors (csd, fem, tra2, and Sxl) occurs in the early wave. Importantly, we also found that the ZGA program simultaneously occurred with the expression of thousands of intronic lncRNAs. Based on the regulatory functions of lncRNAs in many species (Morris and Mattick, 2014; Statello et al., 2021), One possible explanation is that these lncRNAs may function to participate in ZGA program in the diploids. Given the lack of ZGA program in haploids, it is consistent that we did not observe zygotic activated lncRNA expression. This model suggests a unique dose compensation mechanism for haplodiploid animals that involves over a thousand lncRNAs which may apparently involve in the expression regulation of genes during the ZGA program (Graphical Abstract).
During embryonic development, MZT controls a coordinated cascade of genetically encoded events (Lee et al., 2014). In fertilized female honeybee embryos, we found that the maternal transcripts are globally degraded from 24 to 48 h AEL, and robust ZGA in honeybee occurs in waves throughout the late blastoderm and gastrulation stages ranging from 24 to 72 h (Nelson, 1915; Fleig and Sander, 1985), which resembles the previously reported ZGA program in Drosophila (Pritchard and Schubiger, 1996). The process of sex determination in Drosophila is known to be controlled by the transcriptional activation of the Sxl splicing factor in female embryos (Schutt and Nothiger, 2000) and Sxl-initiated short splicing regulation cascade composed of three genes Sxl, tra2, and dsx (Sanchez, 2008; Gempe et al., 2009; Salz, 2011; Bopp et al., 2014; Zhang et al., 2014). From our data, we observed a more complex splicing regulation network comprising Sxl, tra2, csd, fem, and dsx in honeybee embryos, all of which are under ZGA program control in female embryos. Additionally, we found that tra2 expression may be controlled via a previously unknown AS-coupled APA. Taken together, it is likely that honeybee and Drosophila use evolutionally divergent mechanisms to control dsx expression. We propose that the ZGA-activated expressions of sex-determining splicing factors in the early wave may function to support the high splicing efficiency and the intronic lncRNA activation that we observed in diploid embryos.
The involvement of lncRNAs in regulating early embryonic development is conserved among animals (Fatica and Bozzoni, 2014). Xist/XIST RNA is directly involved in the repression of chromatin formation; Xist is specifically expressed in early female mouse and human embryonic cells to orchestrate X-chromosome inactivation (XCI) (da Rocha and Heard, 2017; Sahakyan et al., 2018). In agreement with a role for lncRNAs in chromosome dose compensation, we here demonstrate that the transcription of thousands of lncRNAs is specifically and temporally observed in female honeybee during embryonic development, implying lncRNA activation may be controlled by the same ZGA program and has the potential to regulate thousands of protein-coding genes.
Our study provides evidence that different genome activation programs are exerted in female vs. male embryos that illustrates that these programs affect very large populations of both mRNAs and lncRNAs. lncRNAs are known to function in establishing cell epigenetic states that control cell fate specification during early embryonic development (Burton and Torres-Padilla, 2014). For example, some lncRNAs regulate the expression of HOX genes, and the latter are transcription factors highly conserved and specify the development embryo body plans (Barber and Rastegar, 2010; Mallo and Alonso, 2013; Fatica and Bozzoni, 2014). A recent study showed the potential contribution of epigenetic differences to sex-biased gene expression in adult males and females of two haplodiploid animals (Wang et al., 2015). It is thus conceivable that the prevalence of sex-specific lncRNA expression during honeybee embryonic development may contribute to the establishment of epigenetic states for different cell fate specification programs that control their later developmental paths toward queens, workers, and drones.
We found here that honeybee introns apparently encode thousands of lncRNAs, and the prevalent transcriptional activation of these intronic lncRNAs during ZGA in female honeybee embryos implies a model in which the female-specific lncRNAs may activate zygotic genome transcription and regulate the gene expression in honeybee. Further studies on the epigenetic level and interaction between lncRNAs and genomic DNA regions of different genes in developing embryos and during other developmental stages of honeybee and other haplodiploid animals could be conducted to explore the lncRNA interaction profiles and mechanisms (Wang et al., 2015; Grath and Parsch, 2016). The zygotic activation of female-specific intronic lncRNAs is supported by the constantly high splicing efficiency and the activation of sex-determining splicing factors specifically in female embryos. The lack of the ZGA during blastoderm formation in haploid males apparently thusly escapes the activation of the female-specific lncRNAs and its attendant lncRNA-mediated gene expression. The insights gained from our study illuminate a new path with many intriguing questions that can be addressed experimentally and can thereby help decipher both the haploid genome activation and the unique female-specific lncRNA mechanisms underlying chromosome silencing and ZGA in these genetically and behaviorally fascinating social insects.
Data Availability Statement
The raw sequencing data has been deposited in GEO and are accessible through accession numbers GSE101741 for all RNA-seq sequencing reads.
Author Contributions
LW, YiZ, HZ, and MW conceived the project. MW, XX, FY, and LZ designed and performed the experiments. DC, HZ, YuZ, MW, LZ, CC, and YiZ analyzed the data. XX, LW, QN, and SW contributed to the reagents, materials, and analysis tools. YiZ, LW, DC, MW, and HZ wrote the manuscript. All authors contributed to the article and approved the submitted version.
Funding
This study was supported by the Agricultural Science & Technology Innovation Program (talent training programs, Grant No. 20150815) and the Chinese Academy of Agricultural Sciences, and the National Natural Science Foundation of China (Grant No. 31602016). This study was also supported by ABLife Inc., Wuhan (Grant No. ABL2015-09007).
Conflict of Interest
DC, FY, YuZ, CC, and YiZ were employed by the company ABLife Inc.
The remaining authors declare that the research was conducted in the absence of any commercial or financial relationships that could be construed as a potential conflict of interest.
Publisher’s Note
All claims expressed in this article are solely those of the authors and do not necessarily represent those of their affiliated organizations, or those of the publisher, the editors and the reviewers. Any product that may be evaluated in this article, or claim that may be made by its manufacturer, is not guaranteed or endorsed by the publisher.
Acknowledgments
We thank Xiaoying Wang, Jinglin Gao, and Tianbin Wang for assistance with honey bee experiments; Kai Wang, Suzhen Qi, and Fuliang Hu for technical support. We thank Chao Cheng and Yaxun Wei in ABLife Inc., for their professional assistance in graphing and dealing with the raw reads filtering. We also thank Xiaotian Luo and Han Yu in ABLife Inc. for their experimental assistance. We are very grateful to John Snyder for his professional editing on the language and discussion of the structure of this manuscript.
Supplementary Material
The Supplementary Material for this article can be found online at: https://www.frontiersin.org/articles/10.3389/fcell.2021.690167/full#supplementary-material
Footnotes
References
Altschul, S. F., Gish, W., Miller, W., Myers, E. W., and Lipman, D. J. (1990). Basic local alignment search tool. J. Mol. Biol. 215, 403–410. doi: 10.1016/s0022-2836(05)80360-2
Anders, S., and Huber, W. (2010). Differential expression analysis for sequence count data. Genome Biol. 11:R106.
Barber, B. A., and Rastegar, M. (2010). Epigenetic control of Hox genes during neurogenesis, development, and disease. Ann. Anat. 192, 261–274. doi: 10.1016/j.aanat.2010.07.009
Bashirullah, A., Halsell, S. R., Cooperstock, R. L., Kloc, M., Karaiskakis, A., Fisher, W. W., et al. (1999). Joint action of two RNA degradation pathways controls the timing of maternal transcript elimination at the midblastula transition in Drosophila melanogaster. Embo J. 18, 2610–2620. doi: 10.1093/emboj/18.9.2610
Batista, P. J., and Chang, H. Y. (2013). Long noncoding RNAs: cellular address codes in development and disease. Cell 152, 1298–1307. doi: 10.1016/j.cell.2013.02.012
Bell, L. R., Maine, E. M., Schedl, P., and Cline, T. W. (1988). Sex-lethal, a Drosophila sex determination switch gene, exhibits sex-specific RNA splicing and sequence similarity to RNA binding proteins. Cell 55, 1037–1046. doi: 10.1016/0092-8674(88)90248-6
Bertholf, L. M. (1925). The moults of the honeybee. J. Econ. Entomol. 18, 380–384. doi: 10.1093/jee/18.2.380
Beye, M., Hasselmann, M., Fondrk, M. K., Page, R. E., and Omholt, S. W. (2003). The gene csd is the primary signal for sexual development in the honeybee and encodes an SR-type protein. Cell 114, 419–429. doi: 10.1016/s0092-8674(03)00606-8
Bopp, D., Saccone, G., and Beye, M. (2014). Sex determination in insects: variations on a common theme. Sex Dev. 8, 20–28. doi: 10.1159/000356458
Borensztein, M., Syx, L., Ancelin, K., Diabangouaya, P., Picard, C., Liu, T., et al. (2017). Xist-dependent imprinted X inactivation and the early developmental consequences of its failure. Nat. Struct. Mol. Biol. 24, 226–233. doi: 10.1038/nsmb.3365
Briggs, J. A., Weinreb, C., Wagner, D. E., Megason, S., Peshkin, L., Kirschner, M. W., et al. (2018). The dynamics of gene expression in vertebrate embryogenesis at single-cell resolution. Science 360, eaar5780. doi: 10.1126/science.aar5780
Bull, J. J. (1983). Evolution of Sex Determining Mechanisms. San Francisco CA: The Benjamin/Cummings Publishing Company, Inc.
Burton, A., and Torres-Padilla, M. E. (2014). Chromatin dynamics in the regulation of cell fate allocation during early embryogenesis. Nat. Rev. Mol. Cell Biol. 15, 723–734. doi: 10.1038/nrm3885
Bustin, S. A., Benes, V., Garson, J. A., Hellemans, J., Huggett, J., Kubista, M., et al. (2009). The MIQE guidelines: minimum Information for Publication of quantitative real-time PCR experiments. Clin. Chem. 55, 611–622. doi: 10.1373/clinchem.2008.112797
Chu, C., Zhang, Q. C., Da Rocha, S. T., Flynn, R. A., Bharadwaj, M., Calabrese, J. M., et al. (2015). Systematic discovery of Xist RNA binding proteins. Cell 161, 404–416. doi: 10.1016/j.cell.2015.03.025
da Rocha, S. T., and Heard, E. (2017). Novel players in X inactivation: insights into Xist-mediated gene silencing and chromosome conformation. Nat. Struct. Mol. Biol. 24, 197–204. doi: 10.1038/nsmb.3370
De Iaco, A., Planet, E., Coluccio, A., Verp, S., Duc, J., and Trono, D. (2017). DUX-family transcription factors regulate zygotic genome activation in placental mammals. Nat. Genet. 49, 941–945. doi: 10.1038/ng.3858
Dearden, P. K., Wilson, M. J., Sablan, L., Osborne, P. W., Havler, M., Mcnaughton, E., et al. (2006). Patterns of conservation and change in honey bee developmental genes. Genome Res. 16, 1376–1384. doi: 10.1101/gr.5108606
Demir, E., and Dickson, B. J. (2005). fruitless splicing specifies male courtship behavior in Drosophila. Cell 121, 785–794. doi: 10.1016/j.cell.2005.04.027
Despic, V., and Neugebauer, K. M. (2018). RNA tales – how embryos read and discard messages from mom. J. Cell Sci. 131:jcs201996.
Devaux, Y., Zangrando, J., Schroen, B., Creemers, E. E., Pedrazzini, T., Chang, C. P., et al. (2015). Long noncoding RNAs in cardiac development and ageing. Nat. Rev. Cardiol. 12, 415–425. doi: 10.1038/nrcardio.2015.55
Fang, Y., Feng, M., Han, B., Qi, Y., Hu, H., Fan, P., et al. (2015). Proteome analysis unravels mechanism underling the embryogenesis of the honeybee drone and its divergence with the worker (Apis mellifera lingustica). J. Proteome Res. 14, 4059–4071. doi: 10.1021/acs.jproteome.5b00625
Farrell, J. A., Wang, Y., Riesenfeld, S. J., Shekhar, K., Regev, A., and Schier, A. F. (2018). Single-cell reconstruction of developmental trajectories during zebrafish embryogenesis. Science 360:eaar3131. doi: 10.1126/science.aar3131
Fatica, A., and Bozzoni, I. (2014). Long non-coding RNAs: new players in cell differentiation and development. Nat. Rev. Genet. 15, 7–21. doi: 10.1038/nrg3606
Fleig, R., and Sander, K. (1985). Blastoderm development in honey bee embryogenesis as seen in the scanning electron microscope. Int. J. Invertebr. Reprod. 8, 279–286. doi: 10.1080/01688170.1985.10510156
Fleig, R., and Sander, K. (1986). Embryogenesis of the honeybee Apis mellifera l. (hymenoptera : apidae): an sem study. Int. J. Insect Morphol. Embryol. 15, 449–462. doi: 10.1016/0020-7322(86)90037-1
Gempe, T., and Beye, M. (2011). Function and evolution of sex determination mechanisms, genes and pathways in insects. Bioessays 33, 52–60. doi: 10.1002/bies.201000043
Gempe, T., Hasselmann, M., Schiott, M., Hause, G., Otte, M., and Beye, M. (2009). Sex determination in honeybees: two separate mechanisms induce and maintain the female pathway. PLoS Biol. 7:e1000222. doi: 10.1371/journal.pbio.1000222
Hasselmann, M., Gempe, T., Schiøtt, M., Nunes-Silva, C. G., Otte, M., and Beye, M. (2008). Evidence for the evolutionary nascence of a novel sex determination pathway in honeybees. Nature 454, 519–522. doi: 10.1038/nature07052
Haussmann, I. U., Bodi, Z., Sanchez-Moran, E., Mongan, N. P., Archer, N., Fray, R. G., et al. (2016). m6A potentiates Sxl alternative pre-mRNA splicing for robust Drosophila sex determination. Nature 540, 301–304. doi: 10.1038/nature20577
Hedrick, P. W., and Parker, J. D. (1997). Evolutionary genetics and genetic variation of haplodiploids and X-linked genes. Annu. Rev. Ecol. Syst. 28, 55–83. doi: 10.1146/annurev.ecolsys.28.1.55
Honeybee Genome Sequencing. (2006). Insights into social insects from the genome of the honeybee Apis mellifera. Nature 443, 931–949. doi: 10.1038/nature05260
Jeon, Y., and Lee, J. T. (2011). YY1 tethers Xist RNA to the inactive X nucleation center. Cell 146, 119–133. doi: 10.1016/j.cell.2011.06.026
Kong, L., Zhang, Y., Ye, Z. Q., Liu, X. Q., Zhao, S. Q., Wei, L., et al. (2007). CPC: assess the protein-coding potential of transcripts using sequence features and support vector machine. Nucleic Acids Res. 35, W345–W349.
Langfelder, P., and Horvath, S. (2008). WGCNA: an R package for weighted correlation network analysis. BMC Bioinformatics 9:559.
Langley, A. R., Smith, J. C., Stemple, D. L., and Harvey, S. A. (2014). New insights into the maternal to zygotic transition. Development 141, 3834–3841. doi: 10.1242/dev.102368
Lee, M. T., Bonneau, A. R., and Giraldez, A. J. (2014). Zygotic genome activation during the maternal-to-zygotic transition. Annu. Rev. Cell Dev. Biol. 30, 581–613. doi: 10.1146/annurev-cellbio-100913-013027
Liu, S., Wang, Z., Chen, D., Zhang, B., Tian, R., Wu, J., et al. (2017). Annotation and cluster analysis of spatiotemporal- and sex-related lncRNA expression in Rhesus macaque brain. Genome Res. 27, 1608–1620. doi: 10.1101/gr.217463.116
Livak, K. J., and Schmittgen, T. D. (2001). Analysis of relative gene expression data using real-time quantitative PCR and the 2(T)(-Delta Delta C) method. Methods 25, 402–408. doi: 10.1006/meth.2001.1262
Lott, S. E., Villalta, J. E., Schroth, G. P., Luo, S., Tonkin, L. A., and Eisen, M. B. (2011). Noncanonical compensation of zygotic X transcription in early Drosophila melanogaster development revealed through single-embryo RNA-seq. PLoS Biol. 9:e1000590. doi: 10.1371/journal.pbio.1000590
Ma, S., and Dai, Y. (2011). Principal component analysis based methods in bioinformatics studies. Brief Bioinform. 12, 714–722. doi: 10.1093/bib/bbq090
Mallo, M., and Alonso, C. R. (2013). The regulation of Hox gene expression during animal development. Development 140, 3951–3963. doi: 10.1242/dev.068346
Martin, M. (2011). Cutadapt removes adapter sequences from high-throughput sequencing reads. EMBnet J. 17, 10–12. doi: 10.14806/ej.17.1.200
Mattick, J. S., and Rinn, J. L. (2015). Discovery and annotation of long noncoding RNAs. Nat. Struct. Mol. Biol. 22, 5–7. doi: 10.1038/nsmb.2942
Mattila, H. R., and Seeley, T. D. (2007). Genetic diversity in honey bee colonies enhances productivity and fitness. Science 317, 362–364. doi: 10.1126/science.1143046
McHugh, C. A., Chen, C. K., Chow, A., Surka, C. F., Tran, C., Mcdonel, P., et al. (2015). The Xist lncRNA interacts directly with SHARP to silence transcription through HDAC3. Nature 521, 232–236. doi: 10.1038/nature14443
Morris, K. V., and Mattick, J. S. (2014). The rise of regulatory RNA. Nat. Rev. Genet. 15, 423–437. doi: 10.1038/nrg3722
Mortazavi, A., Williams, B. A., Mccue, K., Schaeffer, L., and Wold, B. (2008). Mapping and quantifying mammalian transcriptomes by RNA-Seq. Nat. Methods 5, 621–628. doi: 10.1038/nmeth.1226
Mulvey, B. B., Olcese, U., Cabrera, J. R., and Horabin, J. I. (2014). An interactive network of long non-coding RNAs facilitates the Drosophila sex determination decision. Biochim. Biophys. Acta 1839, 773–784. doi: 10.1016/j.bbagrm.2014.06.007
Nissen, I., Müller, M., and Beye, M. (2012). The Am-tra2 gene is an essential regulator of female splice regulation at two levels of the sex determination hierarchy of the honeybee. Genetics 192, 1015–1026. doi: 10.1534/genetics.112.143925
Percharde, M., Lin, C. J., Yin, Y., Guan, J., Peixoto, G. A., Bulut-Karslioglu, A., et al. (2018). A LINE1-nucleolin partnership regulates early development and ESC identity. Cell 174:e319.
Pires, C. V., Freitas, F. C., Cristino, A. S., Dearden, P. K., and Simoes, Z. L. (2016). Transcriptome analysis of honeybee (Apis Mellifera) haploid and diploid embryos reveals early zygotic transcription during cleavage. PLoS One 11:e0146447. doi: 10.1371/journal.pone.0146447
Pritchard, D. K., and Schubiger, G. (1996). Activation of transcription in Drosophila embryos is a gradual process mediated by the nucleocytoplasmic ratio. Genes Dev. 10, 1131–1142. doi: 10.1101/gad.10.9.1131
Quinn, J. J., and Chang, H. Y. (2016). Unique features of long non-coding RNA biogenesis and function. Nat. Rev. Genet. 17, 47–62. doi: 10.1038/nrg.2015.10
Ratnieks, F. L. W., and Keller, L. (1998). Queen control of egg fertilization in the honey bee. Behav. Ecol. Sociobiol. 44, 57–61. doi: 10.1007/s002650050514
Rembold, H., Kremer, J. P., and Ulrich, G. M. (1980). Characterization of postembryonic developmental stages of the female castes of the honey bee, Apis mellifera L. Apidologie 11, 29–38. doi: 10.1051/apido:19800104
Robinson, G. E., Grozinger, C. M., and Whitfield, C. W. (2005). Sociogenomics: social life in molecular terms. Nat. Rev. Genet. 6, 257–270. doi: 10.1038/nrg1575
Robinson, M. D., Mccarthy, D. J., and Smyth, G. K. (2010). edgeR: a Bioconductor package for differential expression analysis of digital gene expression data. Bioinformatics 26, 139–140. doi: 10.1093/bioinformatics/btp616
Ryner, L. C., Goodwin, S. F., Castrillon, D. H., Anand, A., Villella, A., Baker, B. S., et al. (1996). Control of male sexual behavior and sexual orientation in Drosophila by the fruitless gene. Cell 87, 1079–1089. doi: 10.1016/s0092-8674(00)81802-4
Sahakyan, A., Yang, Y., and Plath, K. (2018). The role of xist in X-chromosome dosage compensation. Trends Cell Biol. 28, 999–1013. doi: 10.1016/j.tcb.2018.05.005
Salz, H. K. (2011). Sex determination in insects: a binary decision based on alternative splicing. Curr. Opin. Genet. Dev. 21, 395–400. doi: 10.1016/j.gde.2011.03.001
Salz, H. K., and Erickson, J. W. (2010). Sex determination in Drosophila: the view from the top. Fly (Austin) 4, 60–70. doi: 10.4161/fly.4.1.11277
Sanchez, L. (2008). Sex-determining mechanisms in insects. Int. J. Dev. Biol. 52, 837–856. doi: 10.1387/ijdb.072396ls
Schutt, C., and Nothiger, R. (2000). Structure, function and evolution of sex-determining systems in dipteran insects. Development 127, 667–677. doi: 10.1242/dev.127.4.667
Statello, L., Guo, C. J., Chen, L. L., and Huarte, M. (2021). Gene regulation by long non-coding RNAs and its biological functions. Nat. Rev. Mol. Cell Biol. 22, 96–118.
Sunwoo, H., Colognori, D., Froberg, J. E., Jeon, Y., and Lee, J. T. (2017). Repeat E anchors Xist RNA to the inactive X chromosomal compartment through CDKN1A-interacting protein (CIZ1). Proc. Natl. Acad. Sci. U.S.A. 114, 10654–10659. doi: 10.1073/pnas.1711206114
Tadano, H., Yamazaki, Y., Takeuchi, H., and Kubo, T. (2009). Age- and division-of-labour-dependent differential expression of a novel non-coding RNA, Nb-1, in the brain of worker honeybees, Apis mellifera L. Insect Mol. Biol. 18, 715–726. doi: 10.1111/j.1365-2583.2009.00911.x
Tadros, W., and Lipshitz, H. D. (2009). The maternal-to-zygotic transition: a play in two acts. Development 136, 3033–3042. doi: 10.1242/dev.033183
Trapnell, C., Roberts, A., Goff, L., Pertea, G., Kim, D., Kelley, D. R., et al. (2012). Differential gene and transcript expression analysis of RNA-seq experiments with TopHat and Cufflinks. Nat. Protoc. 7, 562–578. doi: 10.1038/nprot.2012.016
Wagner, D. E., Weinreb, C., Collins, Z. M., Briggs, J. A., Megason, S. G., and Klein, A. M. (2018). Single-cell mapping of gene expression landscapes and lineage in the zebrafish embryo. Science 360, 981–987. doi: 10.1126/science.aar4362
Wallberg, A., Bunikis, I., Pettersson, O. V., Mosbech, M. B., Childers, A. K., Evans, J. D., et al. (2019). A hybrid de novo genome assembly of the honeybee, Apis mellifera, with chromosome-length scaffolds. BMC Genomics 20:275.
Wallberg, A., Han, F., Wellhagen, G., Dahle, B., Kawata, M., Haddad, N., et al. (2014). A worldwide survey of genome sequence variation provides insight into the evolutionary history of the honeybee Apis mellifera. Nat. Genet. 46, 1081–1088. doi: 10.1038/ng.3077
Wang, E. T., Sandberg, R., Luo, S., Khrebtukova, I., Zhang, L., Mayr, C., et al. (2008). Alternative isoform regulation in human tissue transcriptomes. Nature 456, 470–476. doi: 10.1038/nature07509
Wang, F., Shin, J., Shea, J. M., Yu, J., Boskovic, A., Byron, M., et al. (2016). Regulation of X-linked gene expression during early mouse development by Rlim. Elife 5:e19127.
Wang, K., Liu, F., Zhou, L. Y., Long, B., Yuan, S. M., Wang, Y., et al. (2014). The long noncoding RNA CHRF regulates cardiac hypertrophy by targeting miR-489. Circ. Res. 114, 1377–1388. doi: 10.1161/circresaha.114.302476
Wang, X., Werren, J. H., and Clark, A. G. (2015). Genetic and epigenetic architecture of sex-biased expression in the jewel wasps Nasonia vitripennis and giraulti. Proc. Natl. Acad. Sci. U.S.A. 112, E3545–E3554.
Wutz, A., Rasmussen, T. P., and Jaenisch, R. (2002). Chromosomal silencing and localization are mediated by different domains of Xist RNA. Nat. Genet. 30, 167–174. doi: 10.1038/ng820
Xia, H., Chen, D., Wu, Q., Wu, G., Zhou, Y., Zhang, Y., et al. (2017). CELF1 preferentially binds to exon-intron boundary and regulates alternative splicing in HeLa cells. Biochim. Biophys. Acta 1860, 911–921. doi: 10.1016/j.bbagrm.2017.07.004
Yu, F. Y., Zhang, Y., Cheng, C., Wang, W. Q., Zhou, Z. S., Rang, W. L., et al. (2020). Poly(A)-seq: a method for direct sequencing and analysis of the transcriptomic poly(A)-tails. PLoS One 15:e0234696. doi: 10.1371/journal.pone.0234696
Keywords: embryonic development, haplodiploid honeybee, long non-coding RNAs, sex determination, zygotic genome activation
Citation: Wang M, Chen D, Zheng H, Zhao L, Xue X, Yu F, Zhang Y, Cheng C, Niu Q, Wang S, Zhang Y and Wu L (2021) Sex-Specific Development in Haplodiploid Honeybee Is Controlled by the Female-Embryo-Specific Activation of Thousands of Intronic LncRNAs. Front. Cell Dev. Biol. 9:690167. doi: 10.3389/fcell.2021.690167
Received: 02 April 2021; Accepted: 12 July 2021;
Published: 06 August 2021.
Edited by:
Li Cai, Rutgers, The State University of New Jersey – Busch Campus, United StatesReviewed by:
Akira Nakamura, Kumamoto University, JapanMontserrat Corominas, University of Barcelona, Spain
Copyright © 2021 Wang, Chen, Zheng, Zhao, Xue, Yu, Zhang, Cheng, Niu, Wang, Zhang and Wu. This is an open-access article distributed under the terms of the Creative Commons Attribution License (CC BY). The use, distribution or reproduction in other forums is permitted, provided the original author(s) and the copyright owner(s) are credited and that the original publication in this journal is cited, in accordance with accepted academic practice. No use, distribution or reproduction is permitted which does not comply with these terms.
*Correspondence: Liming Wu, YXBpc3d1QDEyNi5jb20=; Yi Zhang, eWl6aGFuZ0BhYmxpZmUuY2M=; Qingsheng Niu, MTM5NDMyMzM2NjNAMTYzLmNvbQ==
†These authors have contributed equally to this work and share first authorship