- Institute of Biochemistry, Christian-Albrechts-University Kiel, Kiel, Germany
Interleukin-6 (IL-6) is the name-giving cytokine of a family of eleven members, including IL-6, CNTF, LIF, and IL-27. IL-6 was first recognized as a B-cell stimulating factor but we now know that the cytokine plays a pivotal role in the orchestration of inflammatory processes as well as in inflammation associated cancer. Moreover, IL-6 is involved in metabolic regulation and it has been shown to be involved in major neural activities such as neuroprotection, which can help to repair and to reduce brain damage. Receptor complexes of all members formed at the plasma membrane contain one or two molecules of the signaling receptor subunit GP130 and the mechanisms of signal transduction are well understood. IL-6 type cytokines can also signal from endomembranes, in particular the endosome, and situations have been reported in which endocytosis of receptor complexes are a prerequisite of intracellular signaling. Moreover, pathogenic GP130 variants were shown to interfere with spatial activation of downstream signals. We here summarize the molecular mechanisms underlying spatial regulation of IL-6 family cytokine signaling and discuss its relevance for pathogenic processes.
Introduction
Interleukin-6 (IL-6) – together with IL-1β and TNFα – is one of the major inflammatory cytokines, which is elevated in most if not all inflammatory states and has also been recognized as a frequent growth factor in many cancers (Grivennikov et al., 2009; Lesina et al., 2011; Garbers et al., 2018; Jones and Jenkins, 2018). IL-6 activity is also an important target of therapy in autoimmune diseases (Kang et al., 2019). The biology of IL-6, which has been cloned 35 years ago (Hirano et al., 1986), is complex and not completely understood (Rose-John, 2018).
IL-6 was originally identified and cloned as a B-cell stimulating factor (Hirano et al., 1986) but it soon turned out that it was identical with hepatocyte stimulating factor (Gauldie et al., 1987), hybridoma growth factor (Brakenhoff et al., 1987) and human interferon beta-2 (Zilberstein et al., 1986), pointing to a pleiotropic spectrum of activities. Now we know that IL-6 plays a prominent role in many inflammatory states and cancer. Moreover, IL-6 has prominent metabolic functions (Wallenius et al., 2002) and is an important factor in neural development (Gadient and Otten, 1994).
Human IL-6 is a four helical glycosylated protein of 184 amino acids (Reif et al., 2021), which shares an overall structural homology with many other cytokines (Spangler et al., 2015). On target cells, IL-6 binds to a membrane-bound IL-6 receptor (IL-6R) and the complex of IL-6 and IL-6R associates with a second receptor subunit called GP130 leading to an onset of intracellular signaling via the janus kinase (JAK)/signal transducer and activator of transcription (STAT), phosphoinositide-3 kinase (PI3K)/AKT kinase and protein tyrosine phosphatase non-receptor type (PTPN) 11/SHP2/mitogen activated protein kinase (MAPK) pathway (Schaper and Rose-John, 2015; Jones and Jenkins, 2018; Rose-John, 2018). Interestingly, GP130 has been recognized to be a subunit of the receptor complexes of IL-11, IL-27, leukemia inhibitory factor (LIF), ciliary neurotrophic factor (CNTF), cardiotrophin like cytokine (CLC), oncostatin M (OSM) and cardiotrophin-1 (CT-1), which together form the IL-6 family of cytokines (Figure 1A; Schaper and Rose-John, 2015; Jones and Jenkins, 2018; Rose-John, 2018).
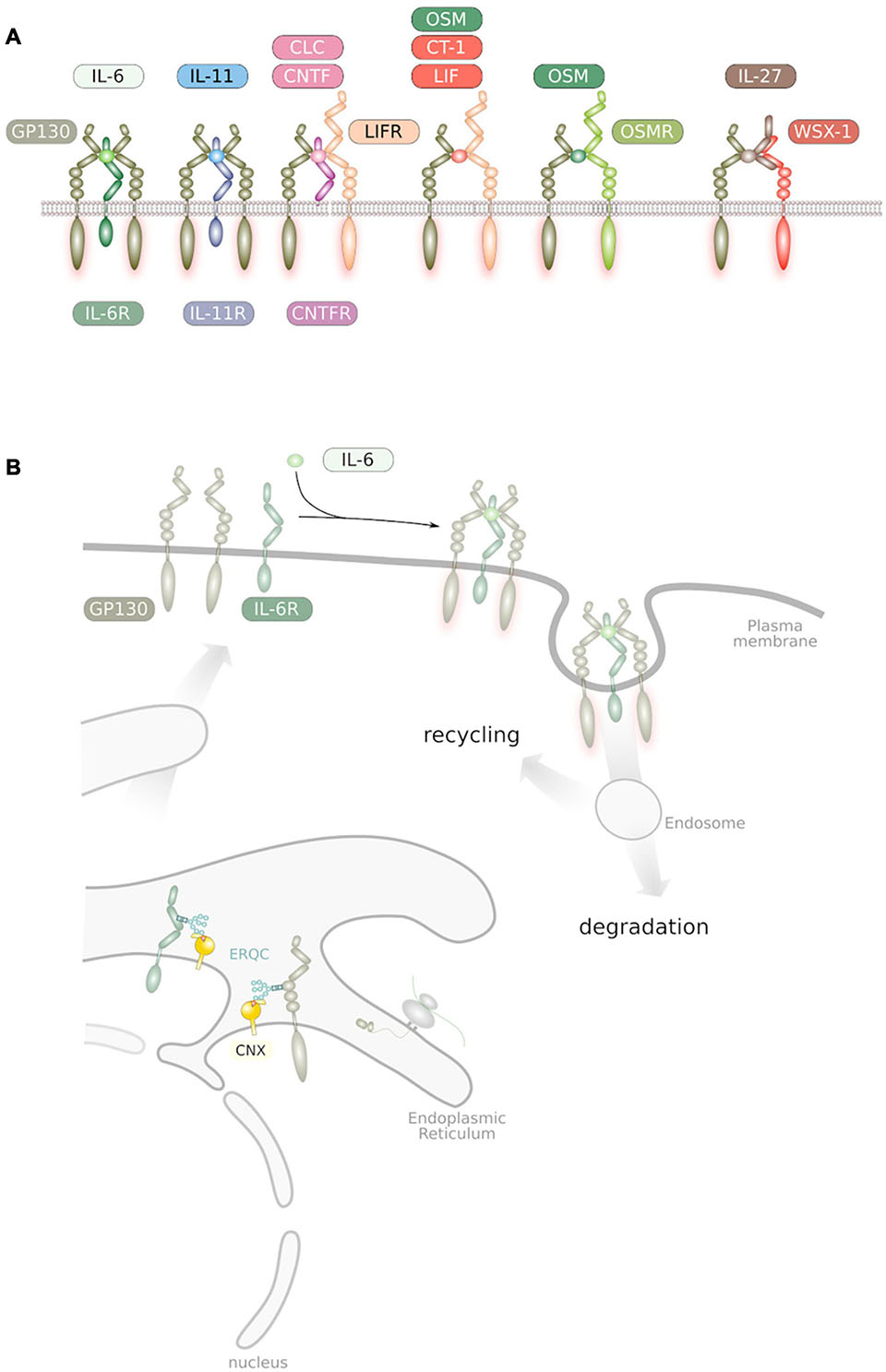
Figure 1. (A) Overview of IL-6 family cytokine receptor complexes. GP130, LIFR, OSMR, and IL-27Rα/WSX-1 are the only receptor subunits of the family that interact with members of the Janus kinase family and are therefore competent for signal transduction (Schmidt-Arras et al., 2021). (B) Trafficking of IL-6 family cytokine receptors. Receptors get synthesized into the endoplasmic reticulum and subsequently glycosylated at Asn residues. These glycans are essential during passage through the ER quality control. Ligand binding at the plasma membrane causes receptor homo/heterodimerization. Internalized receptors are either subjected to degradation or to recycling back to the plasma membrane.
It has been shown that the IL-6R is readily shed from the cell surface of human and murine cells (Müllberg et al., 1993) and is found in human blood (Riethmueller et al., 2017). Interestingly, in humans but not in mice, the soluble IL-6R (sIL-6R) can also be generated by translation from an alternatively spliced mRNA (Lust et al., 1992), although this mechanism accounts only for about 10% of sIL-6R that is found in human blood (Riethmueller et al., 2017). The sIL-6R binds IL-6 (Müllberg et al., 1993) and the complex of IL-6 and sIL-6R stimulates cells, which only express GP130 but no IL-6R (Mackiewicz et al., 1992). Except on mature granulocytes (Wilkinson et al., 2018), IL6ST, encoding GP130, is expressed on all cells of the body. However, expression levels vary. High expression levels were found in the liver, in particular hepatic stellate cells, placenta, breast and lymph node. In leukocytes, IL6ST is in particular expressed in T-cells (Fagerberg et al., 2014; Uhlén et al., 2015). In contrast, IL6R expression is very low in most of the tissues and elevated expression has been found in both types of alveolar cells, hepatocytes, some leukocytes, such as granulocytes, and in the skeletal muscle (Rose-John et al., 1990; Oberg et al., 2006). The ratio of GP130 and alpha receptor such as IL-6R therefore dictates cytokine responsiveness of an individual cell. However, expression data have to be handled with caution as expression levels vary depending on the deposited dataset and expression of IL6ST and IL6R should be validated experimentally in the tissue of interest.
Cells without IL-6R expression are completely unresponsive to IL-6 (Mackiewicz et al., 1992). The mode of signaling via the sIL-6R significantly enlarges the spectrum of target cells of IL-6 and has been called IL-6 trans-signaling (Rose-John and Heinrich, 1994). Similarly, it has been demonstrated in vitro that IL-11 bound to the sIL-11R can stimulate GP130 expressing cells although the in vivo relevance of this process has not yet been elucidated (Lokau et al., 2016). Interestingly, Human Herpes Virus 8 encodes a protein, which shows 25% sequence identity with human IL-6 (Neipel et al., 1997). This viral IL-6 (vIL-6) protein, a soluble protein without transmembrane domain, was shown to directly bind to GP130 without being presented by the human IL-6R (Chow et al., 2001). Therefore, the vIL-6 protein stimulates cells in the absence of IL-6R and therefore shows the same spectrum of target cells as the IL-6/sIL-6R complex via trans-signaling (Molden et al., 1997; Müllberg et al., 2000). In addition to the sIL-6R, which is found in the blood of healthy individuals at concentrations of about 40–80 ng/ml, soluble forms of gp130 are found in the blood at levels of about 400 ng/ml (Garbers et al., 2018; Rose-John, 2018). It is believed that sIL-6R and sgp130 form a buffer for IL-6, which in healthy volunteers is found at 1–5 pg/ml but which rises during inflammatory states by several 100- to 1000-fold (Garbers et al., 2018; Rose-John, 2018).
For a long time, activation of signal proteins by receptor complexes at the plasma membrane were thought to be the only source of downstream signaling. In this monolithic view, internalization of receptor complexes has been solely considered to terminate receptor signaling. However, emerging data suggest that receptor complexes internalized into endosomes can serve as signaling platforms that support sustained intracellular signaling, potentially even with altered signal quality. Here, we summarize current knowledge and discuss the importance of endomembranes, in particular endosomes, for the signal transduction of IL-6 family cytokines.
The Interleukin-6 Family of Cytokines
The IL-6 family of cytokines is defined by the presence of GP130 in their cognate receptor complexes (Figure 1A). IL-6 and IL-11 bind to their specific IL-6R and IL-11R receptor subunits and subsequently associate with a homodimer of GP130 (Figure 1A; Kishimoto, 2005). The cytokines CNTF and CLC interact with the CNTF-R and signal via a heterodimer formed by GP130 and the related protein LIF-R (Kishimoto, 2005). OSM directly binds to GP130 leading to heterodimer formation with LIF-R whereas LIF directly binds to the LIF-R, which heterodimerizes with GP130 (Kishimoto, 2005). OSM can also bind to an alternative receptor complex which is formed by GP130 and the OSM-R (Mosley et al., 1996). IL-27 is a dimeric cytokine formed by the four-helical protein p28 and the soluble cytokine receptor-like protein EBI3, which binds to a heterodimer formed of GP130 and WSX-1 (Pflanz et al., 2002; Figure 1A).
Several designer proteins have been generated to study the biology of IL-6 and the relevance of GP130 signaling. Hyper-IL-6 is a fusion protein of sIL-6R covalently connected to the NH2 terminus of IL-6 by a flexible peptide linker (Fischer et al., 1997). Hyper-IL-6 mimics IL-6 trans-signaling and was used to differentiate between classic- and trans-signaling. Hyper-IL-6 but not IL-6 alone strongly stimulated the expansion of hematopoietic stem cells (Audet et al., 2001) and the survival of sympathetic neurons (März et al., 1998). Smooth muscle cells (Klouche et al., 1999), endothelial cells (Romano et al., 1997), and embryonic stem cells (Humphrey et al., 2004) are only responsive to IL-6 in the presence of sIL-6R. Moreover, it was shown that liver regeneration, which is largely dependent on IL-6 (Cressman et al., 1996) was significantly accelerated in the presence of sIL-6R (Galun et al., 2000; Peters et al., 2000).
While Hyper-IL-6 demonstrated the enormous in vitro and in vivo potential of IL-6 trans-signaling, it did not prove that this signaling mode actually occurred in vivo. Therefore a second designer protein was generated, which was called soluble gp130Fc (sgp130Fc) (Jostock et al., 2001). The sgp130Fc protein consists of the entire extracellular portion of GP130 fused to the Fc portion of a human IgG1 antibody (Jostock et al., 2001). It turned out that sgp130Fc exclusively blocked IL-6 trans-signaling without affecting classic IL-6 signaling via the membrane-bound IL-6R (Jostock et al., 2001). The reason for this specificity was the fact that GP130 shows no measurable affinity for the separate proteins IL-6 or IL-6R. It only binds the complex of IL-6 bound to the IL-6R (Jostock et al., 2001). Therefore, stimulation of cells, which express IL-6R with IL-6 will not be affected by sgp130Fc since IL-6 bound to the membrane-bound IL-6R immediately associates with membrane-bound GP130 and sgp130Fc has no access to the receptor-bound IL-6. In contrast, the complex of IL-6/sIL-6R in solution can just as well bind to membrane-bound GP130 as to sgp130Fc. In the presence of a molar excess of sgp130Fc, IL-6 trans-signaling will be completely blocked (Jostock et al., 2001).
It has been shown that IL-6 trans-signaling is involved in many pathophysiologic states including autoimmunity and cancer (Jones et al., 2011). Recent work demonstrated that specific blockade of IL-6 trans-signaling prevented high-fat diet induced adipose tissue macrophage accumulation (Kraakman et al., 2015) and blocked IL-6 mediated neurodegeneration in a transgenic animal model (Campbell et al., 2014). Recently it was found that IL-6 trans-signaling strongly stimulated repopulation of microglia in the mammalian brain and thereby aided to repair cognitive deficits from brain injury (Willis et al., 2020).
Inhibition of IL-6 activity by neutralizing antibodies to the IL-6R has been approved in many countries for the treatment of autoimmune diseases (Tanaka et al., 2014; Kang et al., 2019). The neutralizing antibodies tocilizumab and sarilumab have been approved for the treatment of patients with autoimmune diseases such as Rheumatoid Arthritis (Garbers et al., 2018; Jones and Jenkins, 2018; Kang et al., 2019). Both antibodies block the binding of the ligand IL-6 to the IL-6R (Garbers et al., 2018; Jones and Jenkins, 2018). This helps to avoid a problem seen with neutralizing IL-6 antibodies, which led to enormous accumulation of IL-6 bound to the antibody in the circulation of patients (Lu et al., 1992). In contrast, treatment with neutralizing IL-6R antibodies resulted in only slight elevation of serum IL-6 levels, which were explained by an inhibition of IL-6 internalization via the membrane-bound IL-6R (Nishimoto et al., 2008). However, IL-6R internalization and degradation rates were not altered by the binding of tocilizumab in vitro (Fujimoto et al., 2015). Blockade of IL-6 biologic activity with the help of IL-6R neutralizing antibodies has been approved in many countries. The blockade of IL-6 activity is highly successful and has been shown to be equivalent or superior to the blockade of the cytokine TNFα (Gabay et al., 2013; Burmester et al., 2017). Interestingly, we have shown that specific blockade of IL-6 trans-signaling was as effective as the blockade of global IL-6 activity by a neutralizing antibody indicating that IL-6 trans-signaling represents the pro-inflammatory IL-6 activity (Scheller et al., 2011) whereas IL-6 signaling via the membrane bound IL-6R was rather protective e.g., in the case of bacterial infections (Sodenkamp et al., 2012; Hoge et al., 2013). Having shown that the sgp130Fc protein protected mice in models of inflammatory bowel disease (Atreya et al., 2000; Mitsuyama et al., 2006) we could recently demonstrate the efficacy of the sgp130Fc proteins in patients with Crohn’s disease and ulcerative colitis (Schreiber et al., 2021).
During inflammatory states, IL-6 is secreted by many cell types including myeloid cells, fibroblasts, endothelial cells and T-cells (Kang et al., 2020) and the response to IL-6 stimulation differs between cell types (Jones and Jenkins, 2018). In order to define the cell specific response to IL-6 we have generated a constitutively activated GP130 molecule, which was dimerized by a leucine zipper (Stuhlmann-Laeisz et al., 2006) and inserted this construct, which we termed LGP130, into the ROSA26 locus of mice (Scherger et al., 2019). This mouse model allows to activate GP130 signaling in a cell-autonomous manner in every selected cell type by breeding these mice to appropriate Cre-expressing transgenic mice (Haldar et al., 2007; Scherger et al., 2019). This mouse model allowed us recently to define the interplay of cell-autonomous, activated GP130 in hepatocytes and a systemic innate immune response, which was likely triggered by the hepatic GP130-induced expression of acute phase proteins such as serum amyloid A (Schumacher et al., 2021).
The Life-Cycle of Il-6 Family Receptor Complexes
Expression of IL-6 Receptor Proteins
Response to IL-6 family cytokines is largely determined by the expression of the corresponding receptor complex proteins and it was demonstrated that expression levels of IL6ST, encoding GP130, and LIFR are controlled by epigenetic mechanisms. Inhibition of histone H3 acetylation resulted in elevated expression of IL6ST and LIFR in certain cell types (Blanchard et al., 2002). The promoter region of IL6ST contains several transcription factor binding sites, including those for CCAAT/enhancer binding protein (C/EBP) β, SP1, STAT1/3 (O’Brien and Manolagas, 1997) and NFκB. It is therefore not surprising that IL6ST expression can be induced by several cytokines, including IL-1β, IL-6, IL-10, OSM, and IFNγ (Romas et al., 1996; O’Brien and Manolagas, 1997; Blanchard et al., 2001) that either induce STAT1, STAT3, or NFκB activity. Furthermore, the mitogen-activated protein kinase (MAPK) ERK2 was found to be associated with the IL6ST promoter and enhances IL6ST expression most likely via phosphorylation of the SP1 transcription factor (Bonito et al., 2014). In isolated murine mast cells, IL-10 strongly induced IL6ST expression and subsequently GP130 surface localization and in consequence sensitivity of mast cells toward WSX-1 (Traum et al., 2012). The designer cytokine HyperIL-6 is also able to robustly induce IL6ST expression and subsequent GP130 plasma membrane accumulation in isolated aortic smooth muscle cells (Klouche et al., 1999).
Trafficking of Signal Transducing Receptors
Receptors for IL-6 family cytokines are type I transmembrane proteins. As such they are synthesized into the endoplasmic reticulum (ER), undergo N-linked glycosylation with high-mannose glycan structures and are subject of the ER quality control (Figure 1B; Caramelo and Parodi, 2015). Upon ER exit, glycan structures are modified within the Golgi before receptors traffic to the plasma membrane. Full maturation and trafficking of GP130 to the plasma membrane was demonstrated to occur within one to four hours, depending on the cellular system (Gerhartz et al., 1994; Wang and Fuller, 1995; Schmidt-Arras et al., 2014). GP130 becomes N-glycosylated at several asparagine residues within its extracellular domain (Moritz et al., 2001; Xu et al., 2010). The attached glycans most likely assist in GP130 folding as mutation of N-glycosylation sites largely resulted in localization of GP130 in a perinuclear compartment, most likely the endoplasmic reticulum (Waetzig et al., 2010). Consequently, deficient GP130 glycosylation induced either via mutagenesis or via pharmacological inhibition resulted in impaired cellular sensitivity toward IL-6 family cytokines (Waetzig et al., 2010; Matsuo et al., 2014).
In addition to glycosylation, trafficking of GP130 to the plasma membrane is also hampered by premature intracellular activity of GP130. Activating deletion mutations in IL6ST are found in patients suffering from inflammatory hepatocellular adenoma (IHCA). These deletions vary in size and cluster at the EF loop of domain 2 (Figure 2A) that is part of the cytokine-binding module of GP130 (Rebouissou et al., 2009; Schütt et al., 2013). The surface localization of these constitutively active GP130 variants is largely lowered (Rinis et al., 2014; Schmidt-Arras et al., 2014) due to prolonged association with the lectin-base chaperone calnexin within the ER quality control (Schmidt-Arras et al., 2014) that results in delayed GP130 maturation. Similar observations were made for oncogenic constitutively active receptor tyrosine kinases (Schmidt-Arras and Böhmer, 2020) and suggest that either altered ectodomain conformations or downstream signaling pathways modify receptor processing and trafficking to the plasma membrane.
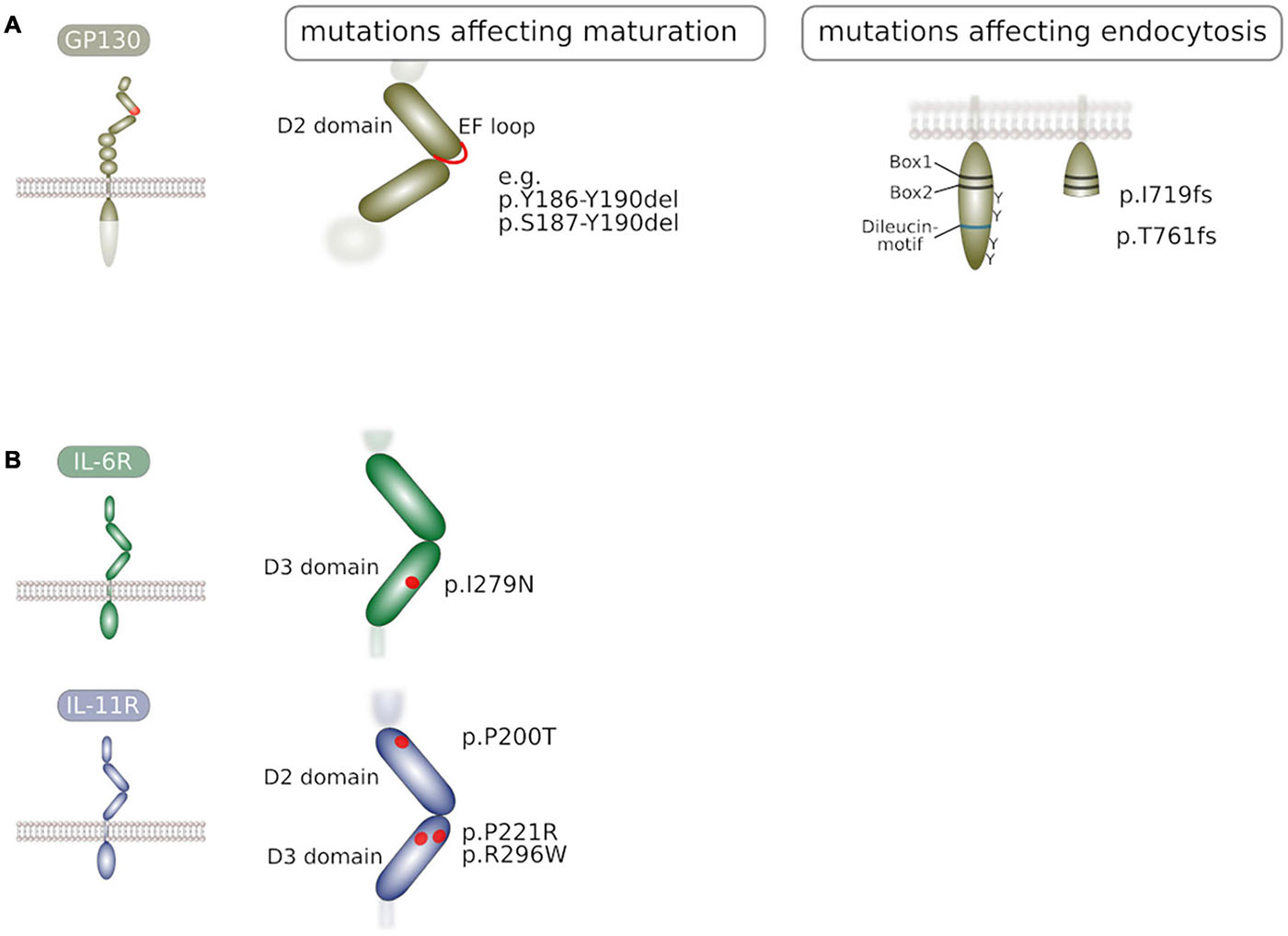
Figure 2. (A) Mutations in GP130 that interfere with receptor maturation or receptor endocytosis. Activating deletion mutations in GP130 were found in inflammatory hepatocellular adenoma and impair GP130 maturation. Frameshift mutations that lead to truncated GP130 molecules devoid tyrosine residues and internalization motif are found in patients. (B) Mutations in the α receptor subunits IL-6R and IL-11R that impair receptor maturation to the plasma membrane. Point mutations in IL-6R are found in patients with a novel immunodeficiency syndrome. Point mutations in IL-11R are found in patients suffering from craniosynostosis. Prolonged residence of these mutants within the ER quality control is likely.
Little is known about biosynthesis and trafficking of the signal-transducing IL-6 family receptor members LIFR and OSMR.
Trafficking of Alpha Receptors
In polarized MDCK cells, the IL-6R is synthesized and transported to the plasma membrane within one hour. During the biosynthetic process, IL-6R becomes N-glycosylated at Asn residues within the extracellular domain (Gerhartz et al., 1994; Riethmueller et al., 2017). IL-6R glycosylation seems to be dispensable for ligand binding and trafficking to the plasma membrane (Riethmueller et al., 2017). In contrast, deletion of the N-terminal Ig-like domain strongly reduces plasma membrane localization of IL-6R most likely due to aberrant receptor maturation (Vollmer et al., 1999). Four hours after synthesis wildtype IL-6R becomes degraded independent of ligand-binding (Gerhartz et al., 1994; Flynn et al., 2021), suggesting that similar to GP130, internalization of IL-6R occurs independent of receptor activity. The short cytoplasmic domain was shown to mediate basolateral sorting in polarized MDCK cells and deletion of the cytoplasmic domain resulted in apical rerouting of IL-6R (Martens et al., 2000). In contrast, the close homolog, IL-11R is present at both, basolateral and apical sides in MDCK cells (Monhasery et al., 2016). Similar to IL-6R, the ectodomain of IL-11R is subjected to N-linked glycosylation. However, in contrast to IL-6R, glycosylation at Asn-194 seems to be essential for the transport to the plasma membrane and substitution of Asn-194 with alanine resulted in predominant localization to the ER (Agthe et al., 2018b).
Aberrant Trafficking of Alpha Receptors Due to Disease-Associated Mutations
Recently, inactivating mutations of IL-6R were identified in two patients suffering from immunodeficiency and an abnormal inflammatory response, associated with eosinophilia and elevated IgE levels (Spencer et al., 2019). Both variants impaired IL-6 signaling while signaling of other IL-6 family cytokines was intact. While one of these variants did not integrate into the plasma membrane due to a premature stop codon, the IL-6R I279N substitution (Figure 2B) resulted in predominant intracellular localization due to impaired trafficking to the surface. It is possible that this variant is entrapped in the ER due to folding defects in the extracellular domain.
Also in IL-11R, loss-of-function mutations (Figure 2B) were identified in patients and found to be associated with craniosynostis, a juvenile disease that causes premature closure of skull sutures (Nieminen et al., 2011; Keupp et al., 2013). Some of these mutations were shown to cause impaired IL-11R trafficking to the plasma membrane thereby impairing cellular susceptibility to IL-11 (Agthe et al., 2018a). Albeit the detailed mechanism of retention has been addressed, it is likely that folding defects in the IL-11R ectodomain result in prolonged association with the ER quality control, resulting in the observed predominant localization to the ER.
Also in LIFR and OSMR loss-of-function mutations were identified. While LIFR mutations were associated with Stüve-Wiedemann syndrome (Dagoneau et al., 2004), a rare disease characterized by skeletal dysplasia, mutations in OSMR were found in patients with primary localized cutaneous amyloidosis (Arita et al., 2008). However, whether these variants have an impact on receptor trafficking has not been addressed yet.
Mechanisms of Internalization
Engulfment into clathrin-coated vesicles is the most common and probably best studied way of receptor internalization and involves the recruitment of clathrin to heterotrimeric adaptor protein (AP) complexes. The reader is referred to recent reviews for further details (Le Roy and Wrana, 2005; Edeling et al., 2006; Briant et al., 2020; Homma et al., 2021). Four different adaptor protein complexes AP1-AP4 that promote formation of clathrin-coated vesicles were identified. AP2 was shown to interact with the cargo either via a YxxΦ motif or a [D/E]XXXL[L/I] ‘acidic di-leucine’ motif. It initially binds to membrane sites with accumulated phosphatidylinositol (4,5)-bisphosphate (PIP2) at the inner leaflet of the membrane (Figure 3A) which leads to a conformational change exposing the cargo binding site (Owen et al., 2004; Edeling et al., 2006). PIP2 also promotes binding of the GTPase dynamin to the plasma membrane that catalyzes pinching off of the endocytic vesicles (Briant et al., 2020).
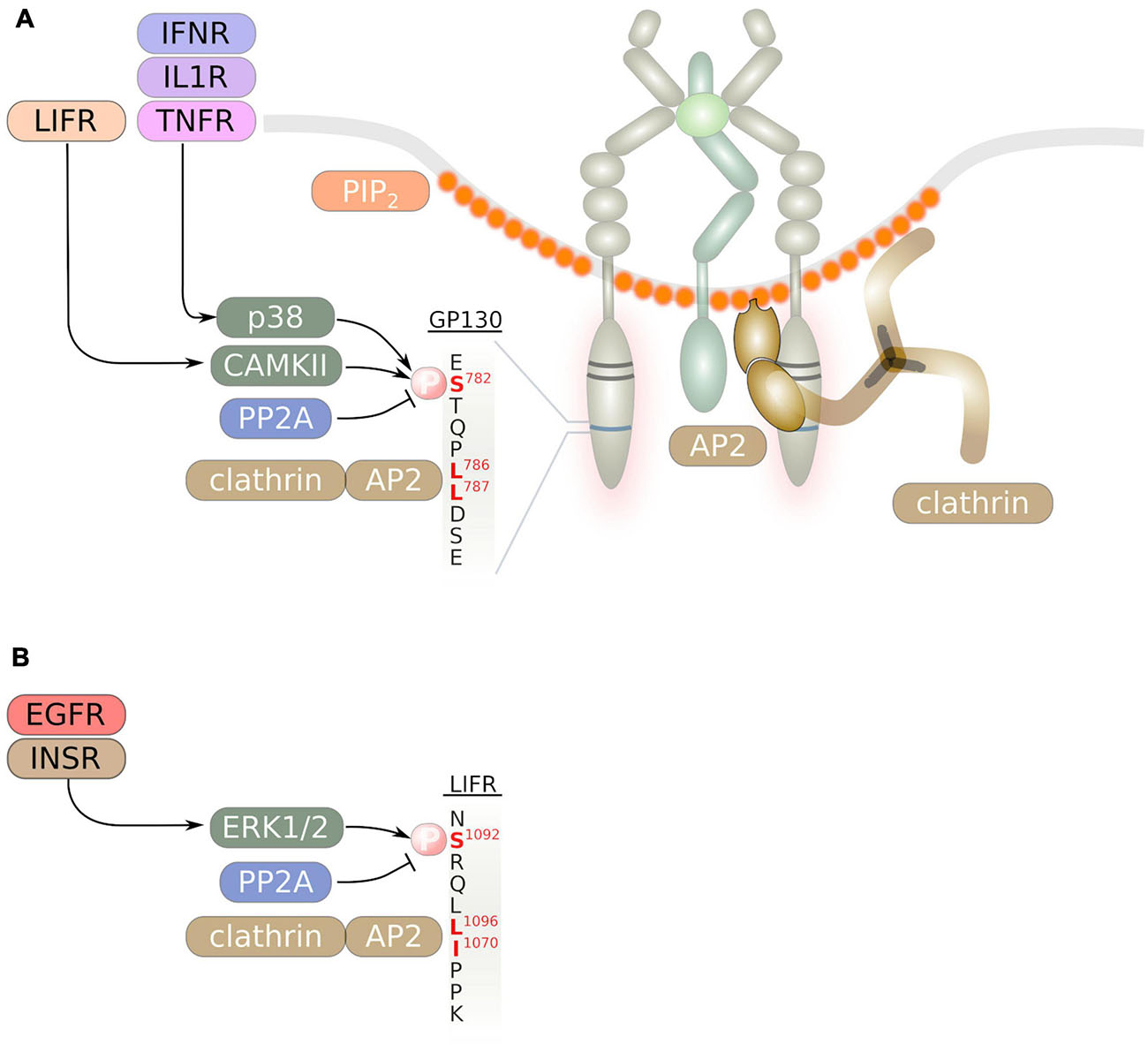
Figure 3. Internalization of IL-6 family signal transducing cytokine receptors occurs via a di-leucin motif. Binding of the adaptor protein complex AP2 to this di-leucin motif and to PIP2 at the inner leaflet of endocytosis-prone membrane areas initiates formation of clathrin-coated vesicles. The di-leucin motifs of GP130 (A) and the LIFR (B) are shown. Serine phosphorylation in close vicinity to the di-leucin motif is mediated by cytoplasmic serine/threonine kinases and enhances receptor internalization.
The C-terminus of GP130 contains a STQPL786L787 di-leucine motif (Figure 3A), that was shown to be essential for efficient internalization of GP130. Substitution of both leucine residues with alanine largely impaired internalization and strongly delayed GP130 degradation (Dittrich et al., 1996). Interestingly, the GP130 di-leucine motif was also shown to mediate basolateral sorting of GP130 in polarized MDCK cells. C-terminal truncation of GP130 led to apical sorting of GP130 (Doumanov et al., 2006). However, little is known if this sorting mechanism also applies in vivo and in human cells. A similar SRQFL1069I1070 di-leucine motif was identified in the C-terminus of LIFR (Figure 3B) that is essential for LIFR internalization (Thiel et al., 1999). GP130 was shown to be constitutively associated with the adaptor protein complex AP-2 (Figure 3A; Thiel et al., 1998). Consequently, internalization of GP130 can be blocked by inhibitors of clathrin or via inhibition of dynamin (Schmidt-Arras et al., 2014; Martinez-Fabregas et al., 2019; Flynn et al., 2021). Consistent with the finding that AP-2 is constitutively associated with GP130, internalization of GP130 was demonstrated to occur independent of ligand-binding or JAK activity (Thiel et al., 1998; Flynn et al., 2021).
A recent report suggested that GP130 internalization kinetics depend on the half live of signaling receptor complexes. In this study, the authors generated IL-6 variants that bind to GP130 independently of IL-6R and with differential affinity toward GP130. Using these variants, the authors demonstrate that the half-life of cytokine/receptor complexes depend on the affinity of the ligand toward GP130. Furthermore, they suggest that long lived cytokine/receptor complexes display enhanced internalization kinetics with localization to EEA1-decorated early endosomes and enhanced degradation kinetics in RPE1 and HeLa cells. The highest internalization rates in this study were found for the HyperIL-6/GP130 complex. This is in stark contrast to previous findings, where HyperIL-6 was found to induce a stable long-lived receptor complex at the plasma membrane of HepG2 cells that displayed reduced internalization kinetics as compared to the IL-6/IL-6R/GP130 complex (Peters et al., 1998). Furthermore, one of the engineered IL-6 variants that had similar GP130 binding affinities as HyperIL-6 displayed a significantly reduced internalization kinetic as compared to HyperIL-6 (Martinez-Fabregas et al., 2019). It is therefore possible that GP130 internalization rates are cell type-dependent and dependent on the type of receptor complex formation.
Albeit Janus kinase activity and therefore GP130 tyrosine phosphorylation is dispensable for GP130 internalization, phosphorylation of Ser-782 that lies in vicinity of the di-leucine motif was demonstrated to enhance GP130 internalization and degradation. A S782A substitution resulted in enhanced cell surface localization of GP130 (Gibson et al., 2000). While LIF stimulation induced GP130 Ser-782 phosphorylation by calmodulin-dependent kinase type (CAMK) II (Gibson et al., 2000, 2005), Ser-782 becomes phosphorylated via p38-activated MAPK-activated protein kinase (MAPKAPK) 2 downstream of the pro-inflammatory cytokines IL-1β, TNF and IFNγ (Radtke et al., 2010; Zha et al., 2017). As a consequence, GP130 downstream signaling is abrogated. Cross-phosphorylation of Ser-782 in GP130 downstream of pro-inflammatory cytokines and subsequent enhanced internalization of GP130 therefore represents a safe-guard mechanism to prevent exacerbated pro-inflammatory signaling.
Similarly, internalization of LIFR is enhanced via ERK1/2-dependent phosphorylation of Ser-1044 that lies upstream of the di-leucine motif (Blanchard et al., 2000). Accordingly, extracellular stimuli such as insulin and EGF enhanced LIFR internalization (Schiemann et al., 1995; Blanchard et al., 2000).
Phosphorylation of GP130 Ser-782 and potentially also of LIFR Ser-1044 is counterbalanced by the serine phosphatase protein phosphatase (PP) 2A and inhibition of PP2A by okadaic acid enhanced degradation of GP130 (Mitsuhashi et al., 2005).
Interestingly, mutations in IL6ST that lead to ligand-independent GP130 activation resulted in differential localization of GP130 to early endosomes, depending on the type of mutation (Schmidt-Arras et al., 2014). Albeit Ser-782 phosphorylation of these variants was not investigated in this study, it is possible that either the amplitude of downstream signaling or receptor ectodomain conformations modulate kinetics and routes of internalization.
Most recently, IL6ST loss-of-function mutations (Figure 2A) were identified in patients suffering from hyper-IgE syndrome (Béziat et al., 2020). These mutations resulted in truncated GP130 variants I719 frameshift (fs) and T761fs lacking STAT3 binding sites and the di-leucin motif. Consequently, these variants displayed largely enhanced plasma membrane localization due to impaired internalization. Interestingly, these GP130 variants exhibited a dominant negative effect over wildtype gp130 in particular on IL-6 and IL-11 signaling. Accordingly, these mutations appeared to be monoallelic in all patients in this study. The authors demonstrated that this effect was at least in part due to sequestration of α receptors. However, it is also plausible that heterodimerization of an inactive variant with wildtype GP130 prolongs the dwell time of wildtype GP130 at the plasma membrane therefore further impairing downstream signaling.
While internalization seems to occur ligand-independently, lysosomal localization of GP130 was enhanced by IL-6 in overexpressing HeLa cells (Flynn et al., 2021). Also in murine CD4+ and CD8+ T-cells, IL-6 stimulation reduced plasma membrane localization of GP130. Accordingly, GP130 was barely detectable in IL-6/sIL-6R double transgenic mice that exhibit constitutive GP130 signaling (Wang et al., 1998), indicating that GP130 phosphorylation and downstream signaling not only enhances internalization but also induces GP130 degradation.
Degradation of GP130 can be mediated by both, lysosome and proteasome. It was demonstrated that the E3 ubiquitin ligase CBL is recruited to GP130 via the associated and tyrosine-phosphorylated PTPase SHP-2. The subsequent trafficking of GP130 to early and then late endosomes is mediated by the sorting protein HGS/Hrs. This protein can engage the ESCRT-0 complex that has been shown to mediate sorting to the endosomal compartment (Vietri et al., 2020). GP130 is subsequently degraded in lysosomes. Deficiency in c-Cbl or HGS results in enhanced and prolonged IL-6 signaling (Tanaka et al., 2008). However, this report did not investigate ligand-independent internalization and degradation.
Independent of ligand-binding IL-6R and IL-11R are endocytosed via clathrin-coated vesicles (Monhasery et al., 2016). Beside internalization, α-receptors can be removed from the cell surface via limited proteolysis (Müllberg et al., 1993; Lokau et al., 2016). The ectodomain of both, IL-6R and IL-11R can be proteolytically processed primarily by membrane-bound proteases of the a disintegrin and metalloprotease (ADAM) family (Zunke and Rose-John, 2017). While this abrogates cytokine signaling in the donor cell, it enables trans-signalling of GP130 in a paracrine fashion on neighboring cells (Peters et al., 2000).
Signal Transduction at Endomembranes
Under physiological conditions, signal transduction of cytokine receptors is initiated at the plasma membrane upon ligand binding. It has become evident that membrane compartmentalization contributes to the regulation of receptor activation. Lipid rafts are dynamic membrane microdomains that are enriched in cholesterol, sphingolipids and GPI-anchored proteins (Le Roy and Wrana, 2005). Due to its hydrophobic nature and its planar and rigid structure, cholesterol favors interaction with saturated lipids with polar headgroups such as sphingolipids yielding the formation of dynamic nanoscale lipid assemblies. Membrane proteins were shown to have sphingolipid binding motifs. It was therefore speculated that protein interaction with “raft lipids” facilitates assembly and functionalization of ordered membrane rafts. Clustering of membrane proteins such as GPI-anchored proteins were shown to promote larger cholesterol-containing spatial and temporal assemblies which are often stabilized by cortical actin (Lingwood and Simons, 2010).
CNTFR but not LIFR and GP130 was shown to reside in the plasma membrane inside lipid rafts in a neuroblastoma cell line. However, upon CNTF but not LIF-stimulation, both, GP130 and LIFR translocated to lipid rafts (Port et al., 2007). In multiple myeloma cells, GP130 was found to be constitutively bound to caveolin-1 that is associated with lipid rafts (Podar et al., 2003). Interestingly, STAT1 and STAT3 were also found to be pre-associated with lipid rafts (Sehgal et al., 2002). Disruption of lipid rafts by methyl-β-cyclodextrin abolished IL-6 induced STAT activation in these cells (Sehgal et al., 2002; Podar et al., 2003), while it did not impair CNTF- or LIF-induced STAT3 phosphorylation in neuroblastoma cells (Port et al., 2007). It can therefore be concluded that residency of GP130 within membrane microdomains and the associated downstream signaling depends on the type of the receptor complex and the cellular context. However, further research is warranted to clarify the mechanisms that regulate sorting of GP130 into different microdomains and its consequences for downstream signaling and biological outcome. Interestingly, also SOCS3 was found to directly bind to caveolin-1 and regulate its stability. Thereby caveolin-1 recruits SOCS3 independent of its SH2 domain. Surprisingly, genetic deficiency of caveolin-1 resulted in enhanced STAT3 phosphorylation upon IL-6 stimulation (Williams et al., 2018). Therefore, caveolin-1 mediates efficient feedback inhibition of STAT3 activation at the plasma membrane.
Signaling of receptor molecules does not terminate at the plasma membrane but can continue throughout the endocytic pathway. There is compelling evidence that receptor tyrosine kinases continue to signal from endomembranes, including the endosomal compartment (Schmidt-Arras and Böhmer, 2020). Also for the IL-6 family cytokine receptors an increasing number of studies demonstrate continuation of signaling from within the endosomal compartment (see below).
The notion that signal emission from endosomes involves ER-endosome contacts stems from the observation that dephosphorylation of several ligand-stimulated receptor tyrosine kinases such as the epidermal growth factor receptor (EGFR), c-Met and the granulocyte colony-stimulating factor receptor (G-CSFR) is mediated by the ER resident protein tyrosine phosphatase (PTP) PTPN1/PTP1B (Ostman and Böhmer, 2001). This has been in particular demonstrated for the EGFR, where internalized EGFR co-localized with PTP1B at ER-endosome contact sites (Haj et al., 2002; Eden et al., 2010). PTP1B was also identified as PTPase for JAK2 and Tyk2 (Myers et al., 2001) and as a regulator of leptin- or G-CSF-induced STAT3 activation (Cheng et al., 2002; Zabolotny et al., 2002; Palande et al., 2011). Downregulation of PTP1B expression resulted in enhanced downstream signaling of GP130, suggesting that inactivation of GP130 signaling also occurs at ER-endosome contact sites (Figure 4A; Fukada and Tonks, 2003). However, experimental evidence for this assumption is still lacking.
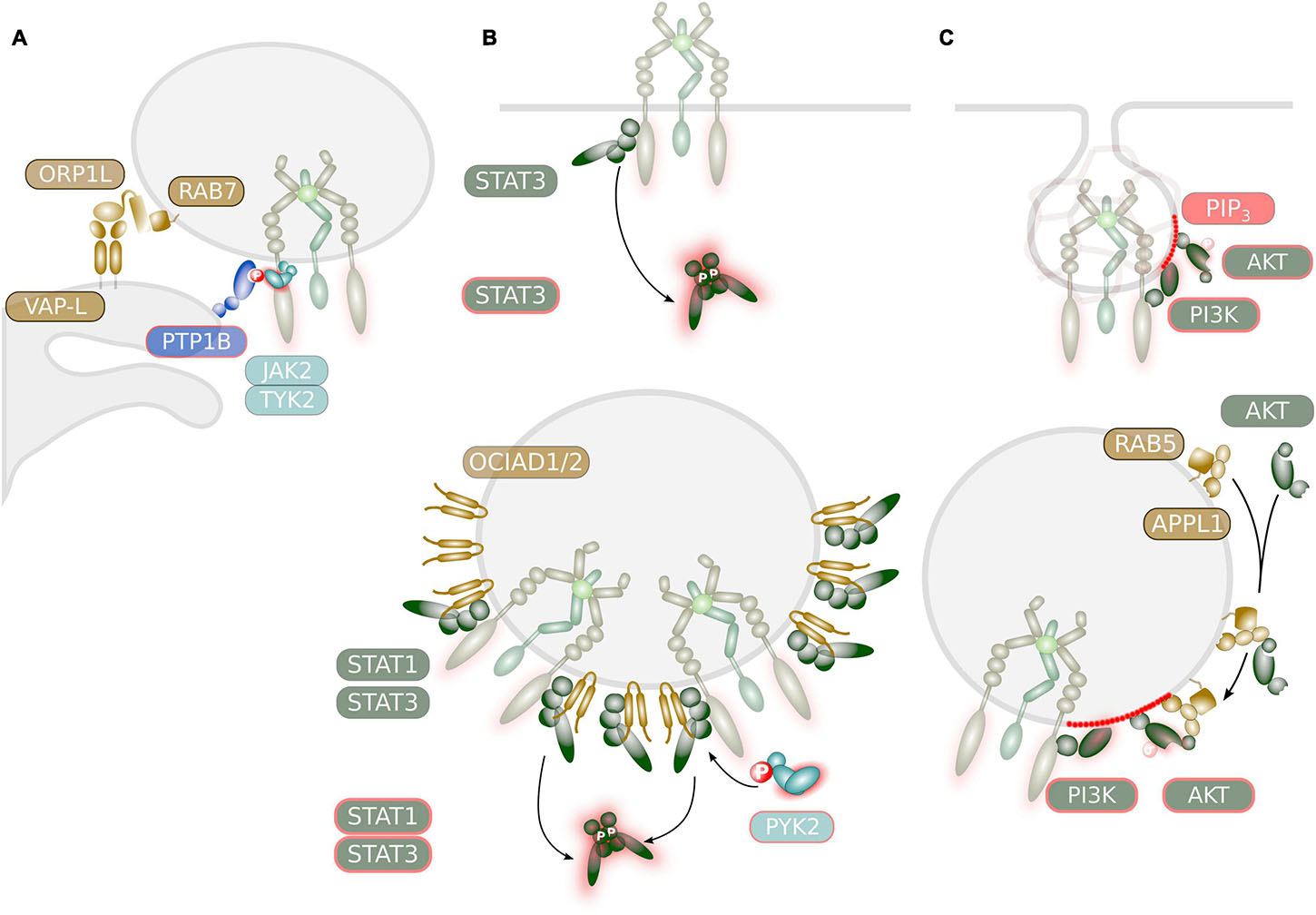
Figure 4. Potential mechanisms regulating signaling of IL-6 family cytokine receptors at endosomes. (A) ER-contact sites might regulate JAK activity. The ER-resident PTPase PTPN1/PTP1B was demonstrated to deactivate STAT signaling through dephosphorylation of JAK2 or TYK2. This might occur at ER-endosome contact sites as was demonstrated for the EGFR. These contact sites are mediated via interaction of the integral ER protein VAP-L and the endosomal associated protein ORP1L which is a RAB7 effector. (B) Selective and sustainable STAT activation at endosomal membranes. STAT proteins with high receptor affinity are readily activated at the plasma membrane. Activation of lower affinity STAT proteins might depend on endosomal localization of the receptor. STAT activation by IL-6 family cytokines at endosomes might be facilitated by the endosome-associated protein OCIAD1/ASRIJ and OCAID2. Recruitment of PYK2 to endosomes might prolong STAT activation as was demonstrated for the EGFR and HGFR/MET. (C) Compartment-specific AKT activation. The generation of PIP3 occurs in two waves: a first short peak at the plasma membrane and a second peak during clathrin-mediated endocytosis. Recruitment of PI3K isoforms to endosomes is mediated via RAB5. Activation of AKT by IL-6 family cytokine might be facilitated by the endosome-associated adaptor protein APPL1 that helps to recruit inactive AKT.
ER-endosome contact sites are established during the maturation of the endosome and it is thought that the majority of late endosomal (LE) vesicles are in contact with the ER (Raiborg et al., 2015). ER-endosome contact sites are established through interaction of the integral ER protein vesicle-associated protein (VAP) A with endosomal membrane-associated proteins. Among those, the cholesterol-sensing protein ORP1L is recruited to the late endosome via direct interaction with the small GTPase RAB7 (Figure 4A; Raiborg et al., 2015).
Activation of STAT Proteins
Recruitment and activation of STAT proteins is a key signaling event in IL-6 family cytokine receptors (Heinrich et al., 2003; Rose-John, 2018) and plasma membrane recruitment of STAT3 to the activated IL-6 receptor complex has been observed (Shah et al., 2006).
However, there are multiple indications that STAT activation can occur from endosomes and might be initiated after cytokine receptor internalization. A small fraction of STAT3 proteins were found to be constitutively associated with early endosomes (Shah et al., 2006) and it is therefore conceivable that internalized receptors can activate STAT proteins in endosomes “en passant.” The endosomal proteins OCIA domain-containing protein (OCIAD) 1/ASRIJ and OCIAD2 are members of the ovarian carcinoma immunoreactive antigen (OCIA) protein family. Both, OCIAD1/ASRIJ and OCIAD2 directly interact with STAT3 at endosomal vesicles (Figure 4B; Sinha et al., 2013, 2018). While OCIAD1 facilitates STAT3 phosphorylation (Sinha et al., 2013) OCIAD2 is essential for STAT3 activation (Sinha et al., 2018).
Activation of STAT1 and 3 downstream of the receptor tyrosine kinases EGFR, platelet-derived growth factor receptor (PDGFR)β and hepatocyte growth factor receptor (HGFR/MET) was observed to occur exclusively from endosomes (Bild et al., 2002; Kermorgant and Parker, 2008; Sadowski et al., 2013; Parks and Ceresa, 2014; Jastrzębski et al., 2017). Interestingly signaling outcome for HGFR/MET differs depending on the localization of endosomal vesicles (Ménard et al., 2014), further suggesting that contact of endosomal vesicles to the ER modulates receptor signaling. Endosomal STAT3 activation by EGFR and HGFR/MET is further enhanced through the recruitment of the cytoplasmic tyrosine kinase PYK2 to early endosomes, representing a positive feedback loop to sustain endosomal STAT3 activation and to promote epithelial-to-mesenchymal transition (EMT) and therefore tumor invasiveness (Verma et al., 2015).
There is strong evidence that IL-6-mediated STAT3 phosphorylation requires endosomal localization of GP130. Upon IL-6 stimulation, a large fraction of STAT3 is recruited to endosomal vesicles (Xu et al., 2007; German et al., 2011) and inhibition of clathrin-mediated endocytosis impairs GP130-induced STAT3 phosphorylation (Xu et al., 2007; Schmidt-Arras et al., 2014). Also STAT3 activation through co-trafficking of IL-6/IL-6R/GP130 complexes in intracellular compartments of dendritic cells occurred at endosomes (Verboogen et al., 2018). Along this line, inhibition of PP2A by okadaic acid blunted STAT3 activation downstream of IL-6 stimulation and correlated with reduced internalization and proteasomal degradation of GP130 (Mitsuhashi et al., 2005). GP130 was also found to activate PYK2, which might contribute to sustained endosomal activation of STAT3 (Figure 4B; Schaeffer et al., 2001). In contrast, inhibition of clathrin-mediated endocytosis did not block OSM-induced STAT3 activation (Kermorgant and Parker, 2008).
Therefore, it is not absolutely evident if STAT proteins are primarily activated from the endosome or from the plasma membrane. There is evidence that receptor complex stability, receptor phosphorylation/dephosphorylation kinetics, and affinity of STATs toward phosphorylated receptor chains determines localization of STAT activation. As an example, while STAT3 activation by OSM occurred rapidly and independent of internalization, STAT3 phosphorylation downstream of HGFR/MET occurred exclusively in endosomes at a later time point after stimulation. This observation correlated with phospho-STAT3 signal strength: while OSM induces strong STAT3 phosphorylation, phospho-STAT3 signals induced HGFR/MET are rather weak (Kermorgant and Parker, 2008). Furthermore, the decision on which particular STAT protein becomes activated seems to be spatially regulated.
These observations might be explained in part by the affinity of STAT SH2 domains toward a phosphorylated tyrosine residue in the receptor complex. Due to the different nature of their SH2-domains, STAT proteins possess differential affinity for phosphorylated tyrosine residues at receptor molecules. While STAT3 can bind to multiple phosphotyrosine residues in GP130, the binding of STAT1 is more restricted (Heinrich et al., 2003). In addition, STAT1 and STAT3 bind with different affinities to phosphorylated GP130 (Wiederkehr-Adam et al., 2003). As a consequence, STAT1 and STAT3 compete in particular for phosphorylated Tyr-905 and to a lower extent for phosphorylated Tyr-915 in GP130 (Heinrich et al., 2003; Martinez-Fabregas et al., 2019).
A very recent report used engineered IL-6 variants with variable affinity for GP130 that where independent of IL-6R binding. The affinity of ligands correlated with receptor complex dwell times and with internalization rates. As a consequence, short lived receptor complexes induced a high phospho-STAT3 to phospho-STAT1 ratio. Activation of STAT1 was lowered in intermediate affinity receptor complexes but not low or high affinity complexes, when clathrin-mediated endocytosis was impaired (Martinez-Fabregas et al., 2019). This suggests that internalization of lower affinity complexes enhances ligand-receptor dwell time and enables the low-affinity binder STAT1 to get activated at endosomes. Unfortunately, the impact of internalization on IL-6- or HyperIL-6-mediated STAT1 phosphorylation was not addressed in this study.
Activation of the Ras/MAPK Pathway
Similar to receptor tyrosine kinases, upon ligand-stimulation at the plasma membrane, cytokine receptors induce several signal transduction modules including the activation of the small membrane-bound GTPase Ras and downstream activation of the mitogen activated protein kinase (MAPK) cascade.
There has been agreement that activation of the small GTPase RAS occurs at the plasma membrane. However, there is a growing number of reports demonstrating that among the four different RAS isoforms N-RAS, H-RAS, KRAS4A and K-RAS4B, N- and H-RAS are also localized to endomembranes, including the endosomes (Fehrenbacher et al., 2009). Activation of RAS isoforms at endomembranes can vary in amplitude (Aran and Prior, 2013) and have different biological outcomes (Daniels et al., 2006; Matallanas et al., 2006). As an example, activation of ERK downstream of EGFR activation initiates at the plasma membrane but continues in endosomes, where the signal strength is even increased (Anastasi et al., 2013).
IL-6 family receptors activate RAS and MAPK cascade by two different mechanisms. Phosphorylation of Tyr-759 in GP130 and Tyr-974 in LIFR, respectively, leads to the recruitment of tyrosine-protein phosphatase non-receptor type (PTPN) 11/SHP2. Subsequent phosphorylation of PTPN11/SHP2 creates a binding site for GRB2/SOS and subsequent immediate activation of RAS/MAPK pathway (Schiemann et al., 1997). In contrast, phosphorylation of Tyr-861 in OSMR recruits the adaptor protein SHC that gets phosphorylated and bound to GRB2/SOS (Hermanns et al., 2000). PTPN11/SHP2 can dephosphorylate GP130 (Lehmann et al., 2003), however its role as feedback inhibitor is under debate (Dittrich et al., 2012). However, GP130 Y759F substitution or genetic deficiency of PTPN11/SHP2 results in hyperactivation of STAT3 (Tebbutt et al., 2002; Jenkins et al., 2005; Bard-Chapeau et al., 2011). Interestingly, inactivation of SHP2 catalytic activity by NADPH oxidase (NOX) 1/4-induced ROS production downstream of the PDGFR occurred on early endosomes. While it is known that GP130 can activate the small GTPase Rac1 (Arulanandam et al., 2010), little is known if this also leads to NOX activation and ROS production.
Inhibition of dynamin-mediated internalization enhanced ERK phosphorylation after short term stimulation with IL-6 (Schmidt-Arras et al., 2014), indicating that endocytosis blunts the initial phase of GP130-mediated RAS/MAPK activation at the plasma membrane. Sustained RAS/MAPK activation downstream of GP130 needs the recruitment of the multi-site docking protein GAB1 to GP130 via PTPN11/SHP2 (Bongartz et al., 2019). Interestingly, sustained RAS/MAPK activation downstream of the EGFR is also mediated via GAB1 and recruitment of GAB1 to EGFR occurred at early endosomes (Kostenko et al., 2006). It is therefore likely, that sustained RAS/MAPK activation via GAB1 downstream of GP130 also occurs at endosomes. Further studies are warranted to dissect GP130-dependent spatial RAS/MAPK activation, resulting differences in signal quality and its biological consequences.
The PI3Kinase Pathway
Beside STAT protein activation, activity of phosphoinositide-3 kinase (PI3K) and phosphorylation of phosphoinositol 4,5,-bisphosphate (PIP2) to generate the second messenger phosphatidylinositol 3,4,5,-trisphosphate (PIP3) is important for the biological effects of IL-6 type cytokines, in particular GP130. As such, PI3K was shown to be essential for GP130-induced pro-inflammatory signaling in endothelial cells (Morris et al., 2008; Suzuki et al., 2011; Zegeye et al., 2018), or tumorigenesis (Heinrich et al., 2003; Thiem et al., 2013; Werner-Klein et al., 2020).
It is common knowledge that generation of PIP3 occurs during clathrin-mediated receptor endocytosis due to RAB5-mediated recruitment of PI3K (Zerial and McBride, 2001; Sato et al., 2003). Generation of PIP3 occurs in two waves due to differential recruitment of PIP3 phosphatases to endocytic vesicles. Recruitment of PI3K to the plasma membrane induces the occurrence of a short peak of PIP3 accumulation, which is rapidly terminated by the PIP3 phosphatase SHIP2 just before the clathrin-coated endocytic vesicles pinch off the membrane (Figure 4C). Upon dissociation of the clathrin coat, receptor-associated PI3K induces a second peak of PIP3. Endocytic vesicles can fuse with a myriad of different types of endosomal vesicles. One type of vesicles is decorated with the adaptor protein, phosphotyrosine interaction, PH domain, and leucine zipper containing (APPL) 1 (Naguib, 2016). APPL1 is a RAB5 effector protein that directly interacts with protein kinase B (PKB)/AKT (Mitsuuchi et al., 1999) and determines its substrate specificity (Schenck et al., 2008). As such, APPL1 was demonstrated to facilitate AKT activation downstream of the EGFR (Jones et al., 2006) and the insulin receptor (Saito et al., 2007) on endosomal vesicles (Figure 4C). While recruitment of class I PI3Kα to activated receptor tyrosine kinases at endomembranes is mediated via microtubule-associated protein (MAP) 4 and microtubules (Thapa et al., 2020), the liver-specific class II PI3K-C2γ directly interacts with RAB5 to mediate AKT activation downstream of hepatic insulin signaling (Braccini et al., 2015). Albeit APPL1 strongly interacts with PI3K in thymic T cells, APPL1 is dispensable for AKT activation in these cells (Tan et al., 2010, 2016). In contrast, HGFR/MET-induced AKT activation in fibroblasts depends on APPL1 (Tan et al., 2016).
Unfortunately, only little is known about spatial regulation of PI3K signaling upon IL-6 family receptor activation. Given its dominant expression in T-cells and hepatocytes, it is possible that GP130 employs RAB5 and APPL1 for PI3K activation and endosomal membranes. There are indeed some indications that GP130 induces PIP3-dependent signaling from endosomes. In response to IL-6 stimulation, recruitment of PI3K regulatory subunit p85 to GP130 occurs via phosphorylated GAB1 (Heinrich et al., 2003). As outlined above, it is likely that GAB1 is recruited to GP130 at endosomes. Furthermore, activation of the mammalian target of rapamycin complex (mTORC) by GP130 requires PI3K activity (Thiem et al., 2013) and mTORC1 and 2 were found to be associated with endosomal vesicles and the lysosome (Ögmundsdóttir et al., 2012; Ebner et al., 2017; Marat et al., 2017). While accumulation of plasma membrane PIP3 is linked to cellular migration (Devreotes and Horwitz, 2015; Yan et al., 2021), was mTORC activation shown to mediate autophagy and survival (Kim and Guan, 2019). It is therefore conceivable that differential spatial activation of PI3K downstream of GP130 has differential biological outcomes. Hence, detailed temporal and spatial analysis of GP130-induced PI3K signaling is highly warranted, in particular dependent on the cellular context.
Conclusion and Perspectives
The concept of spatial regulation of intracellular signaling has only recently emerged. While data on spatial signaling of receptor tyrosine kinases is increasing, only little is known about compartmentalization of cytokine signaling, in particular for IL-6 family cytokine receptors and warrants further investigation. Given the fact that biological outcome is dependent on compartment-specific signaling (Daniels et al., 2006; Matallanas et al., 2006; Martinez-Fabregas et al., 2019), a more detailed knowledge on the mechanisms of spatial regulation of cytokine receptor signaling would open up a new avenue for therapeutics design. In this respect, it is not only interesting to answer the question e.g., on the spatial activation of STAT proteins but to investigate if compartment-specific signals alter signal quality. As an example, kinetics of STAT activation might not only determine nuclear shuttling rates but as a direct consequence could alter on-off rates on STAT-responsive promoters and therefore regulate expression of STAT-responsive genes in a specific manner. A recent report has demonstrated that fine-tuning of STAT3 resident time at STAT-responsive genes indeed alters gene expression (Martinez-Fabregas et al., 2020). The design of cytokine variants that favor particular compartment-specific receptor signaling would be an elegant way for the design of future therapeutics.
Targeting of the artificial LGP130 variant to different subcellular compartments would allow to identify GP130-initiated, compartment-specific signaling pathways independent of ligand stimulation and without the need of receptor endocytosis. When inserted into the ROSA26 locus, expression of these variants could be initiated in a Cre-/Flp-recombinase-dependent and cell type-specific manner. This would allow analysis of the impact of compartment-specific signaling modules on biological outcomes of a particular cell type.
In combination with the aforementioned novel designer cytokines we would be able to fine-tune GP130 signaling in preclinical disease models and open up the avenue for novel therapeutics.
Author Contributions
DS-A and SR-J conceived, wrote, and edited the manuscript. DS-A generated artwork. Both authors contributed to the article and approved the submitted version.
Funding
This work was supported by the Deutsche Forschungsgemeinschaft (DFG), Bonn [grant number SFB841, project C1 to DS-A and SR-J, grant number SFB877, project A1 to SR-J] and the Cluster of Excellence ‘Precision Medicine in Chronic Inflammation’ to SR-J.
Conflict of Interest
The authors declare that the research was conducted in the absence of any commercial or financial relationships that could be construed as a potential conflict of interest.
References
Agthe, M., Brügge, J., Garbers, Y., Wandel, M., Kespohl, B., Arnold, P., et al. (2018a). Mutations in craniosynostosis patients cause defective interleukin-11 receptor maturation and drive craniosynostosis-like disease in mice. Cell Rep. 25, 10–18.e5.
Agthe, M., Garbers, Y., Grötzinger, J., and Garbers, C. (2018b). Two N-Linked glycans differentially control maturation, trafficking and proteolysis, but not activity of the IL-11 Receptor. Cell. Physiol. Biochem. 45, 2071–2085. doi: 10.1159/000488044
Anastasi, S., Alemà, S., and Segatto, O. (2013). “Regulation of epidermal growth factor receptor signaling by endocytosis in normal and malignant cells,” in Vesicle Trafficking in Cancer, eds Y. Yarden and G. Tarcic (New York, NY: Springer).
Aran, V., and Prior, I. A. (2013). Compartmentalized Ras signaling differentially contributes to phenotypic outputs. Cell. Signal. 25, 1748–1753. doi: 10.1016/j.cellsig.2013.05.004
Arita, K., South, A. P., Hans-Filho, G., Sakuma, T. H., Lai-Cheong, J., Clements, S., et al. (2008). Oncostatin M receptor-beta mutations underlie familial primary localized cutaneous amyloidosis. Am. J. Hum. Genet. 82, 73–80. doi: 10.1016/j.ajhg.2007.09.002
Arulanandam, R., Geletu, M., Feracci, H., and Raptis, L. (2010). Activated Rac1 requires gp130 for Stat3 activation, cell proliferation and migration. Exp. Cell. Res. 316, 875–886. doi: 10.1016/j.yexcr.2009.10.017
Atreya, R., Mudter, J., Finotto, S., Müllberg, J., Jostock, T., Wirtz, S., et al. (2000). Blockade of interleukin 6 trans signaling suppresses T-cell resistance against apoptosis in chronic intestinal inflammation: evidence in crohn disease and experimental colitis in vivo. Nat. Med. 6, 583–588. doi: 10.1038/75068
Audet, J., Miller, C. L., Rose-John, S., Piret, J. M., and Eaves, C. J. (2001). Distinct role of gp130 activation in promoting self-renewal divisions by mitogenically stimulated murine hematopoietic stem cells. Proc. Natl. Acad. Sci. U.S.A. 98, 1757–1762. doi: 10.1073/pnas.98.4.1757
Bard-Chapeau, E., Li, S., Ding, J., Zhang, S., Zhu, H., Princen, F., et al. (2011). Ptpn11/Shp2 acts as a tumor suppressor in hepatocellular carcinogenesis. Cancer Cell 19, 629–639. doi: 10.1016/j.ccr.2011.03.023
Béziat, V., Tavernier, S. J., Chen, Y.-H., Ma, C. S., Materna, M., Laurence, A., et al. (2020). Dominant-negative mutations in human IL6ST underlie hyper-IgE syndrome. J. Exp. Med. 217:e2019180405272020c.
Bild, A. H., Turkson, J., and Jove, R. (2002). Cytoplasmic transport of Stat3 by receptor-mediated endocytosis. EMBO J. 21, 3255–3263. doi: 10.1093/emboj/cdf351
Blanchard, F., Duplomb, L., Wang, Y., Robledo, O., Kinzie, E., Pitard, V., et al. (2000). Stimulation of leukemia inhibitory factor receptor degradation by extracellular signal-regulated kinase. J. Biol. Chem. 275, 28793–28801. doi: 10.1074/jbc.m003986200
Blanchard, F., Kinzie, E., Wang, Y., Duplomb, L., Godard, A., Held, W. A., et al. (2002). FR901228, an inhibitor of histone deacetylases, increases the cellular responsiveness to IL-6 type cytokines by enhancing the expression of receptor proteins. Oncogene 21, 6264–6277. doi: 10.1038/sj.onc.1205777
Blanchard, F., Wang, Y., Kinzie, E., Duplomb, L., Godard, A., and Baumann, H. (2001). Oncostatin M regulates the synthesis and turnover of gp130, leukemia inhibitory factor receptor alpha, and oncostatin M receptor beta by distinct mechanisms. J. Biol. Chem. 276, 47038–47045. doi: 10.1074/jbc.m107971200
Bongartz, H., Gille, K., Hessenkemper, W., Mandel, K., Lewitzky, M., Feller, S. M., et al. (2019). The multi-site docking protein Grb2-associated binder 1 (Gab1) enhances interleukin-6-induced MAPK-pathway activation in an SHP2-, Grb2-, and time-dependent manner. Cell Commun. Signal. 17:135.
Bonito, N. A., Drechsler, J., Stoecker, S., Carmo, C. R., Seckl, M. J., Hermanns, H. M., et al. (2014). Control of gp130 expression by the mitogen-activated protein kinase ERK2. Oncogene 33, 2255–2263. doi: 10.1038/onc.2013.159
Braccini, L., Ciraolo, E., Campa, C. C., Perino, A., Longo, D. L., Tibolla, G., et al. (2015). PI3K-C2γ is a Rab5 effector selectively controlling endosomal Akt2 activation downstream of insulin signalling. Nat. Commun. 6:7400.
Brakenhoff, J. P., de Groot, E. R., Evers, R. F., Pannekoek, H., and Aarden, L. A. (1987). Molecular cloning and expression of hybridoma growth factor in Escherichia coli. J. Immunol. 139, 4116–4121.
Briant, K., Redlingshöfer, L., and Brodsky, F. M. (2020). Clathrin’s life beyond 40: connecting biochemistry with physiology and disease. Curr. Opin. Cell Biol. 65, 141–149. doi: 10.1016/j.ceb.2020.06.004
Burmester, G. R., Lin, Y., Patel, R., van Adelsberg, J., Mangan, E. K., Graham, N. M. H., et al. (2017). Efficacy and safety of sarilumab monotherapy versus adalimumab monotherapy for the treatment of patients with active rheumatoid arthritis (MONARCH): a randomised, double-blind, parallel-group phase III trial. Ann. Rheum. Dis. 76, 840–847. doi: 10.1136/annrheumdis-2016-210310
Campbell, I. L., Erta, M., Lim, S. L., Frausto, R., May, U., Rose-John, S., et al. (2014). Trans-signaling is a dominant mechanism for the pathogenic actions of interleukin-6 in the brain. J. Neurosci. 34, 2503–2513. doi: 10.1523/jneurosci.2830-13.2014
Caramelo, J. J., and Parodi, A. J. (2015). A sweet code for glycoprotein folding. FEBS Lett. 589, 3379–3387. doi: 10.1016/j.febslet.2015.07.021
Cheng, A., Uetani, N., Simoncic, P. D., Chaubey, V. P., Lee-Loy, A., McGlade, C. J., et al. (2002). Attenuation of leptin action and regulation of obesity by protein tyrosine phosphatase 1B. Dev. Cell 2, 497–503. doi: 10.1016/s1534-5807(02)00149-1
Chow, D., He, X., Snow, A. L., Rose-John, S., and Garcia, K. C. (2001). Structure of an extracellular gp130 cytokine receptor signaling complex. Science 291, 2150–2155. doi: 10.1126/science.1058308
Cressman, D. E., Greenbaum, L. E., DeAngelis, R. A., Ciliberto, G., Furth, E. E., Poli, V., et al. (1996). Liver failure and defective hepatocyte regeneration in interleukin-6-deficient mice. Science 274, 1379–1383. doi: 10.1126/science.274.5291.1379
Dagoneau, N., Scheffer, D., Huber, C., Al-Gazali, L. I., Di Rocco, M., Godard, A., et al. (2004). Null leukemia inhibitory factor receptor (LIFR) mutations in Stuve-Wiedemann/Schwartz-Jampel type 2 syndrome. Am. J. Hum. Genet. 74, 298–305. doi: 10.1086/381715
Daniels, M. A., Teixeiro, E., Gill, J., Hausmann, B., Roubaty, D., Holmberg, K., et al. (2006). Thymic selection threshold defined by compartmentalization of Ras/MAPK signalling. Nature 444, 724–729. doi: 10.1038/nature05269
Devreotes, P., and Horwitz, A. R. (2015). Signaling networks that regulate cell migration. Cold Spring Harb. Perspect. Biol. 7:a005959. doi: 10.1101/cshperspect.a005959
Dittrich, A., Quaiser, T., Khouri, C., Görtz, D., Mönnigmann, M., and Schaper, F. (2012). Model-driven experimental analysis of the function of SHP-2 in IL-6-induced Jak/STAT signaling. Mol. Biosyst. 8, 2119–2134. doi: 10.1039/c2mb05488d
Dittrich, E., Haft, C. R., Muys, L., Heinrich, P. C., and Graeve, L. (1996). A di-leucine motif and an upstream serine in the interleukin-6 (IL-6) signal transducer gp130 mediate ligand-induced endocytosis and down-regulation of the IL-6 receptor. J. Biol. Chem. 271, 5487–5494. doi: 10.1074/jbc.271.10.5487
Doumanov, J. A., Daubrawa, M., Unden, H., and Graeve, L. (2006). Identification of a basolateral sorting signal within the cytoplasmic domain of the interleukin-6 signal transducer gp130. Cell. Signal. 18, 1140–1146. doi: 10.1016/j.cellsig.2005.09.006
Ebner, M., Sinkovics, B., Szczygieł, M., Ribeiro, D. W., and Yudushkin, I. (2017). Localization of mTORC2 activity inside cells. J. Cell Biol. 216, 343–353.
Edeling, M. A., Smith, C., and Owen, D. (2006). Life of a clathrin coat: insights from clathrin and AP structures. Nat. Rev. Mol. Cell Biol. 7, 32–44. doi: 10.1038/nrm1786
Eden, E. R., White, I. J., Tsapara, A., and Futter, C. E. (2010). Membrane contacts between endosomes and ER provide sites for PTP1B-epidermal growth factor receptor interaction. Nat. Cell Biol. 12, 267–272. doi: 10.1038/ncb2026
Fagerberg, L., Hallström, B. M., Oksvold, P., Kampf, C., Djureinovic, D., Odeberg, J., et al. (2014). Analysis of the human tissue-specific expression by genome-wide integration of transcriptomics and antibody-based proteomics. Mol. Cell. Proteom. 13, 397–406. doi: 10.1074/mcp.m113.035600
Fehrenbacher, N., Bar-Sagi, D., and Philips, M. (2009). Ras/MAPK signaling from endomembranes. Mol. Oncol. 3, 297–307. doi: 10.1016/j.molonc.2009.06.004
Fischer, M., Goldschmitt, J., Peschel, C., Brakenhoff, J. P., Kallen, K. J., Wollmer, A., et al. (1997). I. A bioactive designer cytokine for human hematopoietic progenitor cell expansion. Nat. Biotechnol. 15, 142–145. doi: 10.1038/nbt0297-142
Flynn, C. M., Kespohl, B., Daunke, T., Garbers, Y., Düsterhöft, S., Rose-John, S., et al. (2021). Interleukin-6 controls recycling and degradation, but not internalization of its receptors. J. Biol. Chem. 296:100434. doi: 10.1016/j.jbc.2021.100434
Fujimoto, K., Ida, H., Hirota, Y., Ishigai, M., Amano, J., and Tanaka, Y. (2015). Intracellular dynamics and fate of a humanized anti-interleukin-6 receptor monoclonal antibody, Tocilizumab. Mol. Pharmacol. 88, 660–675. doi: 10.1124/mol.115.099184
Fukada, T., and Tonks, N. K. (2003). Identification of YB-1 as a regulator of PTP1B expression: implications for regulation of insulin and cytokine signaling. EMBO J. 22, 479–493. doi: 10.1093/emboj/cdg067
Gabay, C., Emery, P., van Vollenhoven, R., Dikranian, A., Alten, R., Pavelka, K., et al. (2013). Tocilizumab monotherapy versus adalimumab monotherapy for treatment of rheumatoid arthritis (ADACTA): a randomised, double-blind, controlled phase 4 trial. Lancet 381, 1541–1550. doi: 10.1016/s0140-6736(13)60250-0
Gadient, R. A., and Otten, U. (1994). Expression of interleukin-6 (IL-6) and interleukin-6 receptor (IL-6R) mRNAs in rat brain during postnatal development. Brain Res. 637, 10–14. doi: 10.1016/0006-8993(94)91211-4
Galun, E., Zeira, E., Pappo, O., Peters, M., and Rose-John, S. (2000). Liver regeneration induced by a designer human IL-6/sIL-6R fusion protein reverses severe hepatocellular injury. FASEB J. 14, 1979–1987. doi: 10.1096/fj.99-0913com
Garbers, C., Heink, S., Korn, T., and Rose-John, S. (2018). Interleukin-6: designing specific therapeutics for a complex cytokine. Nat. Rev. Drug Discov. 17, 395–412. doi: 10.1038/nrd.2018.45
Gauldie, J., Richards, C., Harnish, D., Lansdorp, P., and Baumann, H. (1987). Interferon beta 2/B-cell stimulatory factor type 2 shares identity with monocyte-derived hepatocyte-stimulating factor and regulates the major acute phase protein response in liver cells. Proc. Natl. Acad. Sci. U.S.A. 84, 7251–7255. doi: 10.1073/pnas.84.20.7251
Gerhartz, C., Dittrich, E., Stoyan, T., Rose-John, S., Yasukawa, K., Heinrich, P. C., et al. (1994). Biosynthesis and half-life of the interleukin-6 receptor and its signal transducer gp130. Eur. J. Biochem. 223, 265–274. doi: 10.1111/j.1432-1033.1994.tb18991.x
German, C. L., Sauer, B. M., and Howe, C. L. (2011). The STAT3 beacon: IL-6 recurrently activates STAT 3 from endosomal structures. Exp. Cell. Res. 317, 1955–1969. doi: 10.1016/j.yexcr.2011.05.009
Gibson, R. M., Laszlo, G. S., and Nathanson, N. M. (2005). Calmodulin-dependent protein kinases phosphorylate gp130 at the serine-based dileucine internalization motif. Biochim. Biophys. Acta 1714, 56–62. doi: 10.1016/j.bbamem.2005.05.014
Gibson, R. M., Schiemann, W. P., Prichard, L. B., Reno, J. M., Ericsson, L. H., and Nathanson, N. M. (2000). Phosphorylation of human gp130 at Ser-782 adjacent to the Di-leucine internalization motif. Effects on expression and signaling. J. Biol. Chem. 275, 22574–22582. doi: 10.1074/jbc.m907658199
Grivennikov, S., Karin, E., Terzic, J., Mucida, D., Yu, G.-Y., Vallabhapurapu, S., et al. (2009). IL-6 and Stat3 are required for survival of intestinal epithelial cells and development of colitis-associated cancer. Cancer Cell 15, 103–113. doi: 10.1016/j.ccr.2009.01.001
Haj, F. G., Verveer, P. J., Squire, A., Neel, B. G., and Bastiaens, P. I. H. (2002). Imaging sites of receptor dephosphorylation by PTP1B on the surface of the endoplasmic reticulum. Science 295, 1708–1711. doi: 10.1126/science.1067566
Haldar, M., Hancock, J. D., Coffin, C. M., Lessnick, S. L., and Capecchi, M. R. (2007). A conditional mouse model of synovial sarcoma: insights into a myogenic origin. Cancer Cell 11, 375–388. doi: 10.1016/j.ccr.2007.01.016
Heinrich, P. C., Behrmann, I., Haan, S., Hermanns, H. M., Müller-Newen, G., and Schaper, F. (2003). Principles of interleukin (IL)-6-type cytokine signalling and its regulation. Biochemistry 374, 1–20. doi: 10.1042/bj20030407
Hermanns, H. M., Radtke, S., Schaper, F., Heinrich, P. C., and Behrmann, I. (2000). Non-redundant signal transduction of interleukin-6-type cytokines. The adapter protein Shc is specifically recruited to rhe oncostatin M receptor. J. Biol. Chem. 275, 40742–40748. doi: 10.1074/jbc.m005408200
Hirano, T., Yasukawa, K., Harada, H., Taga, T., Watanabe, Y., Matsuda, T., et al. (1986). Complementary DNA for a novel human interleukin (BSF-2) that induces B lymphocytes to produce immunoglobulin. Nature 324, 73–76. doi: 10.1038/324073a0
Hoge, J., Yan, I., Jänner, N., Schumacher, V., Chalaris, A., Steinmetz, O. M., et al. (2013). IL-6 controls the innate immune response against Listeria monocytogenes via classical IL-6 signaling. J. Immunol. 190, 703–711. doi: 10.4049/jimmunol.1201044
Homma, Y., Hiragi, S., and Fukuda, M. (2021). Rab family of small GTPases: an updated view on their regulation and functions. FEBS J. 288, 36–55. doi: 10.1111/febs.15453
Humphrey, R. K., Beattie, G. M., Lopez, A. D., Bucay, N., King, C. C., Firpo, M. T., et al. (2004). Maintenance of pluripotency in human embryonic stem cells is STAT3 independent. Stem Cells 22, 522–530. doi: 10.1634/stemcells.22-4-522
Jastrzębski, K., Zdżalik-Bielecka, D., Mamińska, A., Kalaidzidis, Y., Hellberg, C., and Miaczynska, M. (2017). Multiple routes of endocytic internalization of PDGFRβ contribute to PDGF-induced STAT3 signaling. J. Cell Sci. 130, 577–589. doi: 10.1242/jcs.191213
Jenkins, B. J., Grail, D., Nheu, T., Najdovska, M., Wang, B., Waring, P., et al. (2005). Hyperactivation of Stat3 in gp130 mutant mice promotes gastric hyperproliferation and desensitizes TGF-beta signaling. Nat. Med. 11, 845–852. doi: 10.1038/nm1282
Jones, R. B., Gordus, A., Krall, J. A., and MacBeath, G. (2006). A quantitative protein interaction network for the ErbB receptors using protein microarrays. Nature 439, 168–174. doi: 10.1038/nature04177
Jones, S. A., and Jenkins, B. J. (2018). Recent insights into targeting the IL-6 cytokine family in inflammatory diseases and cancer. Nat. Rev. Immunol. 18, 773–789. doi: 10.1038/s41577-018-0066-7
Jones, S. A., Scheller, J., and Rose-John, S. (2011). Therapeutic strategies for the clinical blockade of IL-6/gp130 signaling. J. Clin. Invest. 121, 3375–3383. doi: 10.1172/jci57158
Jostock, T., Müllberg, J., Ozbek, S., Atreya, R., Blinn, G., Voltz, N., et al. (2001). Soluble gp130 is the natural inhibitor of soluble interleukin-6 receptor transsignaling responses. Eur. J. Biochem. 268, 160–167. doi: 10.1046/j.1432-1327.2001.01867.x
Kang, S., Narazaki, M., Metwally, H., and Kishimoto, T. (2020). Historical overview of the interleukin-6 family cytokine. J. Exp. Med. 217:e20190347.
Kang, S., Tanaka, T., Narazaki, M., and Kishimoto, T. (2019). Targeting interleukin-6 signaling in clinic. Immunity 50, 1007–1023. doi: 10.1016/j.immuni.2019.03.026
Kermorgant, S., and Parker, P. J. (2008). Receptor trafficking controls weak signal delivery: a strategy used by c-Met for STAT3 nuclear accumulation. J. Cell Biol. 182, 855–863. doi: 10.1083/jcb.200806076
Keupp, K., Li, Y., Vargel, I., Hoischen, A., Richardson, R., Neveling, K., et al. (2013). Mutations in the interleukin receptor IL11RA cause autosomal recessive Crouzon-like craniosynostosis. Mol. Genet. Genom. Med. 1, 223–237. doi: 10.1002/mgg3.28
Kim, J., and Guan, K.-L. (2019). mTOR as a central hub of nutrient signalling and cell growth. Nat. Cell Biol. 21, 63–71. doi: 10.1038/s41556-018-0205-1
Kishimoto, T. (2005). Interleukin-6: from basic science to medicine–40 years in immunology. Annu. Rev. Immunol. 23, 1–21. doi: 10.1146/annurev.immunol.23.021704.115806
Klouche, M., Bhakdi, S., Hemmes, M., and Rose-John, S. (1999). Novel path to activation of vascular smooth muscle cells: up-regulation of gp130 creates an autocrine activation loop by IL-6 and its soluble receptor. J. Immunol. 163, 4583–4589.
Kostenko, O., Tsacoumangos, A., Crooks, D., Kil, S. J., and Carlin, C. (2006). Gab1 signaling is regulated by EGF receptor sorting in early endosomes. Oncogene 25, 6604–6617. doi: 10.1038/sj.onc.1209675
Kraakman, M. J., Kammoun, H. L., Allen, T. L., Deswaerte, V., Henstridge, D. C., Estevez, E., et al. (2015). Blocking IL-6 trans-signaling prevents high-fat diet-induced adipose tissue macrophage recruitment but does not improve insulin resistance. Cell Metab. 21, 403–416. doi: 10.1016/j.cmet.2015.02.006
Le Roy, C., and Wrana, J. L. (2005). Clathrin- and non-clathrin-mediated endocytic regulation of cell signalling. Nat. Rev. Mol. Cell Biol. 6, 112–126. doi: 10.1038/nrm1571
Lehmann, U., Schmitz, J., Weissenbach, M., Sobota, R. M., Hortner, M., Friederichs, K., et al. (2003). SHP2 and SOCS3 contribute to Tyr-759-dependent attenuation of interleukin-6 signaling through gp130. J. Biol. Chem. 278, 661–671. doi: 10.1074/jbc.m210552200
Lesina, M., Kurkowski, M. U., Ludes, K., Rose-John, S., Treiber, M., Klöppel, G., et al. (2011). Stat3/Socs3 activation by IL-6 transsignaling promotes progression of pancreatic intraepithelial neoplasia and development of pancreatic cancer. Cancer Cell 19, 456–469. doi: 10.1016/j.ccr.2011.03.009
Lingwood, D., and Simons, K. (2010). Lipid rafts as a membrane-organizing principle. Science 327, 46–50. doi: 10.1126/science.1174621
Lokau, J., Nitz, R., Agthe, M., Monhasery, N., Aparicio-Siegmund, S., Schumacher, N., et al. (2016). Proteolytic cleavage governs interleukin-11 Trans-signaling. Cell Rep. 14, 1761–1773. doi: 10.1016/j.celrep.2016.01.053
Lu, Z. Y., Brochier, J., Wijdenes, J., Brailly, H., Bataille, R., and Klein, B. (1992). High amounts of circulating interleukin (IL)-6 in the form of monomeric immune complexes during anti-IL-6 therapy. Towards a new methodology for measuring overall cytokine production in human in vivo. Eur. J. Immunol. 22, 2819–2824. doi: 10.1002/eji.1830221110
Lust, J. A., Donovan, K. A., Kline, M. P., Greipp, P. R., Kyle, R. A., and Maihle, N. J. (1992). Isolation of an mRNA encoding a soluble form of the human interleukin-6 receptor. Cytokine 4, 96–100. doi: 10.1016/1043-4666(92)90043-q
Mackiewicz, A., Schooltink, H., Heinrich, P. C., and Rose-John, S. (1992). Complex of soluble human IL-6-receptor/IL-6 up-regulates expression of acute-phase proteins. J. Immunol. 149, 2021–2027.
Marat, A. L., Wallroth, A., Lo, W.-T., Müller, R., Norata, G. D., Falasca, M., et al. (2017). mTORC1 activity repression by late endosomal phosphatidylinositol 3,4-bisphosphate. Science 356, 968–972. doi: 10.1126/science.aaf8310
Martens, A. S., Bode, J. G., Heinrich, P. C., and Graeve, L. (2000). The cytoplasmic domain of the interleukin-6 receptor gp80 mediates its basolateral sorting in polarized madin-darby canine kidney cells. J. Cell Sci. 113(Pt 20), 3593–3602. doi: 10.1242/jcs.113.20.3593
Martinez-Fabregas, J., Wang, L., Pohler, E., Cozzani, A., Wilmes, S., Kazemian, M., et al. (2020). CDK8 Fine-Tunes IL-6 transcriptional activities by limiting STAT3 resident time at the gene loci. Cell Rep. 33:108545. doi: 10.1016/j.celrep.2020.108545
Martinez-Fabregas, J., Wilmes, S., Wang, L., Hafer, M., Pohler, E., Lokau, J., et al. (2019). Kinetics of cytokine receptor trafficking determine signaling and functional selectivity. eLife 8:e49314.
März, P., Cheng, J. G., Gadient, R. A., Patterson, P. H., Stoyan, T., Otten, U., et al. (1998). Sympathetic neurons can produce and respond to interleukin 6. Proc. Natl. Acad. Sci. U.S.A. 95, 3251–3256. doi: 10.1073/pnas.95.6.3251
Matallanas, D., Sanz-Moreno, V., Arozarena, I., Calvo, F., Agudo-Ibáñez, L., Santos, E., et al. (2006). Distinct utilization of effectors and biological outcomes resulting from site-specific Ras activation: Ras functions in lipid rafts and Golgi complex are dispensable for proliferation and transformation. Mol. Cell. Biol. 26, 100–116. doi: 10.1128/mcb.26.1.100-116.2006
Matsuo, R., Morihara, H., Mohri, T., Murasawa, S., Takewaki, K., Nakayama, H., et al. (2014). The inhibition of N-glycosylation of glycoprotein 130 molecule abolishes STAT3 activation by IL-6 family cytokines in cultured cardiac myocytes. PLoS One 9:e111097. doi: 10.1371/journal.pone.0111097
Ménard, L., Parker, P. J., and Kermorgant, S. (2014). Receptor tyrosine kinase c-Met controls the cytoskeleton from different endosomes via different pathways. Nat. Commun. 5:3907.
Mitsuhashi, S., Shima, H., Tanuma, N., Sasa, S., Onoe, K., Ubukata, M., et al. (2005). Protein phosphatase type 2A, PP2A, is involved in degradation of gp130. Mol. Cell. Biochem. 269, 183–187. doi: 10.1007/s11010-005-3089-x
Mitsuuchi, Y., Johnson, S. W., Sonoda, G., Tanno, S., Golemis, E. A., and Testa, J. R. (1999). Identification of a chromosome 3p14.3-21.1 gene, APPL, encoding an adaptor molecule that interacts with the oncoprotein-serine/threonine kinase AKT2. Oncogene 18, 4891–4898. doi: 10.1038/sj.onc.1203080
Mitsuyama, K., Matsumoto, S., Rose-John, S., Suzuki, A., Hara, T., Tomiyasu, N., et al. (2006). STAT3 activation via interleukin 6 trans-signalling contributes to ileitis in SAMP1/Yit mice. Gut 55, 1263–1269. doi: 10.1136/gut.2005.079343
Molden, J., Chang, Y., You, Y., Moore, P. S., and Goldsmith, M. A. (1997). A Kaposi’s sarcoma-associated herpesvirus-encoded cytokine homolog (vIL-6) activates signaling through the shared gp130 receptor subunit. J. Biol. Chem. 272, 19625–19631. doi: 10.1074/jbc.272.31.19625
Monhasery, N., Moll, J., Cuman, C., Franke, M., Lamertz, L., Nitz, R., et al. (2016). Transcytosis of IL-11 and apical redirection of gp130 Is Mediated by IL-11α receptor. Cell Rep. 16, 1067–1081. doi: 10.1016/j.celrep.2016.06.062
Moritz, R. L., Hall, N. E., Connolly, L. M., and Simpson, R. J. (2001). Determination of the disulfide structure and N-glycosylation sites of the extracellular domain of the human signal transducer gp130. J. Biol. Chem. 276, 8244–8253. doi: 10.1074/jbc.m009979200
Morris, V. A., Punjabi, A. S., and Lagunoff, M. (2008). Activation of Akt through gp130 receptor signaling is required for Kaposi’s sarcoma-associated herpesvirus-induced lymphatic reprogramming of endothelial cells. J. Virol. 82, 8771–8779. doi: 10.1128/jvi.00766-08
Mosley, B., De Imus, C., Friend, D., Boiani, N., Thoma, B., Park, L. S., et al. (1996). Dual oncostatin M (OSM) receptors. Cloning and characterization of an alternative signaling subunit conferring OSM-specific receptor activation. J. Biol. Chem. 271, 32635–32643. doi: 10.1074/jbc.271.51.32635
Müllberg, J., Geib, T., Jostock, T., Hoischen, S. H., Vollmer, P., Voltz, N., et al. (2000). IL-6 receptor independent stimulation of human gp130 by viral IL-6. J. Immunol. 164, 4672–4677. doi: 10.4049/jimmunol.164.9.4672
Müllberg, J., Schooltink, H., Stoyan, T., Günther, M., Graeve, L., Buse, G., et al. (1993). The soluble interleukin-6 receptor is generated by shedding. Eur. J. Immunol. 23, 473–480. doi: 10.1002/eji.1830230226
Myers, M. P., Andersen, J. N., Cheng, A., Tremblay, M. L., Horvath, C. M., Parisien, J. P., et al. (2001). TYK2 and JAK2 are substrates of protein-tyrosine phosphatase 1B. J. Biol. Chem. 276, 47771–47774. doi: 10.1074/jbc.c100583200
Naguib, A. (2016). Following the trail of lipids: Signals initiated by PI3K function at multiple cellular membranes. Sci. Signal. 9:re4. doi: 10.1126/scisignal.aad7885
Neipel, F., Albrecht, J. C., Ensser, A., Huang, Y. Q., Li, J. J., Friedman-Kien, A. E., et al. (1997). Human herpesvirus 8 encodes a homolog of interleukin-6. J. Virol. 71, 839–842. doi: 10.1128/jvi.71.1.839-842.1997
Nieminen, P., Morgan, N. V., Fenwick, A. L., Parmanen, S., Veistinen, L., Mikkola, M. L., et al. (2011). Inactivation of IL11 signaling causes craniosynostosis, delayed tooth eruption, and supernumerary teeth. Am. J. Hum. Genet. 89, 67–81. doi: 10.1016/j.ajhg.2011.05.024
Nishimoto, N., Terao, K., Mima, T., Nakahara, H., Takagi, N., and Kakehi, T. (2008). Mechanisms and pathologic significances in increase in serum interleukin-6 (IL-6) and soluble IL-6 receptor after administration of an anti-IL-6 receptor antibody, tocilizumab, in patients with rheumatoid arthritis and Castleman disease. Blood 112, 3959–3964. doi: 10.1182/blood-2008-05-155846
Oberg, H.-H., Wesch, D., Grüssel, S., Rose-John, S., and Kabelitz, D. (2006). Differential expression of CD126 and CD130 mediates different STAT-3 phosphorylation in CD4+CD25- and CD25high regulatory T cells. Int. Immunol. 18, 555–563. doi: 10.1093/intimm/dxh396
O’Brien, C. A., and Manolagas, S. C. (1997). Isolation and Characterization of the Human gp130 Promoter: regulation by STATS. J. Biol. Chem. 272, 15003–15010. doi: 10.1074/jbc.272.23.15003
Ögmundsdóttir, M. H., Heublein, S., Kazi, S., Reynolds, B., Visvalingam, S. M., Shaw, M. K., et al. (2012). Proton-assisted amino acid transporter PAT1 complexes with Rag GTPases and activates TORC1 on late endosomal and lysosomal membranes. PLoS One 7:e36616. doi: 10.1371/journal.pone.0036616
Ostman, A., and Böhmer, F. D. (2001). Regulation of receptor tyrosine kinase signaling by protein tyrosine phosphatases. Trends Cell Biol. 11, 258–266. doi: 10.1016/s0962-8924(01)01990-0
Owen, D. J., Collins, B. M., and Evans, P. R. (2004). Adaptors for clathrin coats: structure and function. Annu. Rev. Cell Dev. Biol. 20, 153–191. doi: 10.1146/annurev.cellbio.20.010403.104543
Palande, K., Roovers, O., Gits, J., Verwijmeren, C., Iuchi, Y., Fujii, J., et al. (2011). Peroxiredoxin-controlled G-CSF signalling at the endoplasmic reticulum-early endosome interface. J. Cell Sci. 124, 3695–3705. doi: 10.1242/jcs.089656
Parks, E. E., and Ceresa, B. P. (2014). Cell surface epidermal growth factor receptors increase Src and c-Cbl activity and receptor ubiquitylation. J. Biol. Chem. 289, 25537–25545. doi: 10.1074/jbc.m114.579581
Peters, M., Blinn, G., Jostock, T., Schirmacher, P., Meyer Zum Büschenfelde, K. H., Galle, P. R., et al. (2000). Combined interleukin 6 and soluble interleukin 6 receptor accelerates murine liver regeneration. Gastroenterology 119, 1663–1671. doi: 10.1053/gast.2000.20236
Peters, M., Blinn, G., Solem, F., Fischer, M., Meyer zum Büschenfelde, K. H., and Rose-John, S. (1998). In vivo and in vitro activities of the gp130-stimulating designer cytokine Hyper-IL-6. J. Immunol. 161, 3575–3581.
Pflanz, S., Timans, J. C., Cheung, J., Rosales, R., Kanzler, H., Gilbert, J., et al. (2002). IL-27, a heterodimeric cytokine composed of EBI3 and p28 protein, induces proliferation of naive CD4+ T cells. Immunity 16, 779–790. doi: 10.1016/s1074-7613(02)00324-2
Podar, K., Tai, Y.-T., Cole, C. E., Hideshima, T., Sattler, M., Hamblin, A., et al. (2003). Essential role of caveolae in interleukin-6- and insulin-like growth factor I-triggered Akt-1-mediated survival of multiple myeloma cells. J. Biol. Chem. 278, 5794–5801. doi: 10.1074/jbc.m208636200
Port, M. D., Gibson, R. M., and Nathanson, N. M. (2007). Differential stimulation-induced receptor localization in lipid rafts for interleukin-6 family cytokines signaling through the gp130/leukemia inhibitory factor receptor complex. J. Neurochem. 101, 782–793. doi: 10.1111/j.1471-4159.2007.04471.x
Radtke, S., Wüller, S., Yang, X.-P., Lippok, B. E., Mütze, B., Mais, C., et al. (2010). Cross-regulation of cytokine signalling: pro-inflammatory cytokines restrict IL-6 signalling through receptor internalisation and degradation. J. Cell Sci. 123, 947–959. doi: 10.1242/jcs.065326
Raiborg, C., Wenzel, E. M., and Stenmark, H. (2015). ER-endosome contact sites: molecular compositions and functions. EMBO J. 34, 1848–1858. doi: 10.15252/embj.201591481
Rebouissou, S., Amessou, M., Couchy, G., Poussin, K., Imbeaud, S., Pilati, C., et al. (2009). Frequent in-frame somatic deletions activate gp130 in inflammatory hepatocellular tumours. Nature 457, 200–204. doi: 10.1038/nature07475
Reif, A., Lam, K., Weidler, S., Lott, M., Boos, I., Lokau, J., et al. (2021). Natural Glycoforms of Human Interleukin 6 show atypical plasma clearance. Angew. Chem. Int. Edn. doi: 10.1002/anie.202101496 [Epub ahead of print].
Riethmueller, S., Somasundaram, P., Ehlers, J. C., Hung, C.-W., Flynn, C. M., Lokau, J., et al. (2017). Proteolytic origin of the soluble human IL-6R in vivo and a decisive role of N-Glycosylation. PLoS Biol. 15:e2000080. doi: 10.1371/journal.pbio.2000080
Rinis, N., Küster, A., Schmitz-Van de Leur, H., Mohr, A., and Müller-Newen, G. (2014). Intracellular signaling prevents effective blockade of oncogenic gp130 mutants by neutralizing antibodies. Cell Commun. Signal. 12:14. doi: 10.1186/1478-811x-12-14
Romano, M., Sironi, M., Toniatti, C., Polentarutti, N., Fruscella, P., Ghezzi, P., et al. (1997). Role of IL-6 and its soluble receptor in induction of chemokines and leukocyte recruitment. Immunity 6, 315–325. doi: 10.1016/s1074-7613(00)80334-9
Romas, E., Udagawa, N., Zhou, H., Tamura, T., Saito, M., Taga, T., et al. (1996). The role of gp130-mediated signals in osteoclast development: regulation of interleukin 11 production by osteoblasts and distribution of its receptor in bone marrow cultures. J. Exp. Med. 183, 2581–2591. doi: 10.1084/jem.183.6.2581
Rose-John, S., and Heinrich, P. C. (1994). Soluble receptors for cytokines and growth factors: generation and biological function. Biochem. J. 300(Pt 2), 281–290. doi: 10.1042/bj3000281
Rose-John, S., Schooltink, H., Lenz, D., Hipp, E., Dufhues, G., Schmitz, H., et al. (1990). Studies on the structure and regulation of the human hepatic interleukin-6 receptor. Eur. J. Biochem. 190, 79–83. doi: 10.1111/j.1432-1033.1990.tb15548.x
Sadowski, Ł, Jastrzȩbski, K., Kalaidzidis, Y., Heldin, C.-H., Hellberg, C., and Miaczynska, M. (2013). Dynamin inhibitors impair endocytosis and mitogenic signaling of PDGF. Traffic 14, 725–736. doi: 10.1111/tra.12061
Saito, T., Jones, C. C., Huang, S., Czech, M. P., and Pilch, P. F. (2007). The interaction of Akt with APPL1 is required for insulin-stimulated Glut4 translocation. J. Biol. Chem. 282, 32280–32287. doi: 10.1074/jbc.m704150200
Sato, M., Ueda, Y., Takagi, T., and Umezawa, Y. (2003). Production of PtdInsP3 at endomembranes is triggered by receptor endocytosis. Nat. Cell Biol. 5, 1016–1022. doi: 10.1038/ncb1054
Schaeffer, M., Schneiderbauer, M., Weidler, S., Tavares, R., Warmuth, M., de Vos, G., et al. (2001). Signaling through a novel domain of gp130 mediates cell proliferation and activation of Hck and Erk kinases. Mol. Cell. Biol. 21, 8068–8081. doi: 10.1128/mcb.21.23.8068-8081.2001
Schaper, F., and Rose-John, S. (2015). Interleukin-6: biology, signaling and strategies of blockade. Cytokine Growth Fact. Rev. 26, 475–487. doi: 10.1016/j.cytogfr.2015.07.004
Scheller, J., Chalaris, A., Schmidt-Arras, D., and Rose-John, S. (2011). The pro- and anti-inflammatory properties of the cytokine interleukin-6. Biochim. Biophys. Acta 1813, 878–888.
Schenck, A., Goto-Silva, L., Collinet, C., Rhinn, M., Giner, A., Habermann, B., et al. (2008). The endosomal protein Appl1 mediates Akt substrate specificity and cell survival in vertebrate development. Cell 133, 486–497. doi: 10.1016/j.cell.2008.02.044
Scherger, A. K., Al-Maarri, M., Maurer, H. C., Schick, M., Maurer, S., Öllinger, R., et al. (2019). Activated gp130 signaling selectively targets B cell differentiation to induce mature lymphoma and plasmacytoma. JCI Insight 4:e128435.
Schiemann, W. P., Bartoe, J. L., and Nathanson, N. M. (1997). Box 3-independent Signaling Mechanisms are involved in leukemia inhibitory factor receptor α- and gp130-mediated Stimulation of Mitogen-activated Protein Kinase. Evidence for participation of multiple signaling pathways which converge at ras. J. Biol. Chem. 272, 16631–16636. doi: 10.1074/jbc.272.26.16631
Schiemann, W. P., Graves, L. M., Baumann, H., Morella, K. K., Gearing, D. P., Nielsen, M. D., et al. (1995). Phosphorylation of the human leukemia inhibitory factor (LIF) receptor by mitogen-activated protein kinase and the regulation of LIF receptor function by heterologous receptor activation. Proc. Natl. Acad. U.S.A. 92, 5361–5365. doi: 10.1073/pnas.92.12.5361
Schmidt-Arras, D., and Böhmer, F.-D. (2020). Mislocalisation of activated receptor tyrosine kinases - challenges for cancer therapy. Trends Mol. Med. 26, 833–847. doi: 10.1016/j.molmed.2020.06.002
Schmidt-Arras, D., Galun, E., and Rose-John, S. (2021). The two facets of gp130 signalling in liver tumorigenesis. Semin. Immunopathol. doi: 10.1007/s00281-021-00861-0 [Epub ahead of print].
Schmidt-Arras, D., Müller, M., Stevanovic, M., Horn, S., Schütt, A., Bergmann, J., et al. (2014). Oncogenic deletion mutants of gp130 signal from intracellular compartments. J. Cell Sci. 127, 341–353. doi: 10.1242/jcs.130294
Schreiber, S., Aden, K., Bernardes, J. P., Conrad, C., Tran, F., Höper, H., et al. (2021). Therapeutic IL-6 trans-signalling inhibition by olamkicept (sgp130Fc) in patients with active inflammatory bowel disease. Gastroenterology doi: 10.1053/j.gastro.2021.02.062 [Epub ahead of print].
Schumacher, N., Yan, K., Gandraß, M., Müller, M., Krisp, C., Häsler, R., et al. (2021). Cell-autonomous hepatocyte-specific GP130 signaling is sufficient to trigger a robust innate immune response in mice. J. Hepatol. 74, 407–418. doi: 10.1016/j.jhep.2020.09.021
Schütt, A., Zacharias, M., Schneider, N., Horn, S., Grötzinger, J., Rose-John, S., et al. (2013). gp130 activation is regulated by D2-D3 interdomain connectivity. Biochem. J. 450, 487–496. doi: 10.1042/bj20121660
Sehgal, P. B., Guo, G. G., Shah, M., Kumar, V., and Patel, K. (2002). Cytokine Signaling: STATS in plasma membrane rafts. J. Biol. Chem. 277, 12067–12074.
Shah, M., Patel, K., Mukhopadhyay, S., Xu, F., Guo, G., and Sehgal, P. B. (2006). Membrane-associated STAT3 and PY-STAT3 in the cytoplasm. J. Biol. Chem. 281, 7302–7308. doi: 10.1074/jbc.m508527200
Sinha, A., Khadilkar, R. J., Vinay, K. S., Roychowdhury Sinha, A., and Inamdar, M. S. (2013). Conserved regulation of the Jak/STAT pathway by the endosomal protein asrij maintains stem cell potency. Cell Rep. 4, 649–658. doi: 10.1016/j.celrep.2013.07.029
Sinha, S., Bheemsetty, V. A., and Inamdar, M. S. (2018). A double helical motif in OCIAD2 is essential for its localization, interactions and STAT3 activation. Sci. Rep. 8:7362.
Sodenkamp, J., Waetzig, G. H., Scheller, J., Seegert, D., Grötzinger, J., Rose-John, S., et al. (2012). Therapeutic targeting of interleukin-6 trans-signaling does not affect the outcome of experimental tuberculosis. Immunobiology 217, 996–1004. doi: 10.1016/j.imbio.2012.01.015
Spangler, J. B., Moraga, I., Mendoza, J. L., and Garcia, K. C. (2015). Insights into cytokine-receptor interactions from cytokine engineering. Annu. Rev. Immunol. 33, 139–167. doi: 10.1146/annurev-immunol-032713-120211
Spencer, S., Köstel Bal, S., Egner, W., Lango Allen, H., Raza, S. I., Ma, C. A., et al. (2019). Loss of the interleukin-6 receptor causes immunodeficiency, atopy, and abnormal inflammatory responses. J. Exp. Med. 216, 1986–1998. doi: 10.1084/jem.20190344
Stuhlmann-Laeisz, C., Lang, S., Chalaris, A., Krzysztof, P., Enge, S., Eichler, J., et al. (2006). Forced dimerization of gp130 leads to constitutive STAT3 activation, cytokine-independent growth, and blockade of differentiation of embryonic stem cells. Mol. Biol. Cell 17, 2986–2995. doi: 10.1091/mbc.e05-12-1129
Suzuki, T., Yoshinaga, N., and Tanabe, S. (2011). Interleukin-6 (IL-6) regulates claudin-2 expression and tight junction permeability in intestinal epithelium. J. Biol. Chem. 286, 31263–31271. doi: 10.1074/jbc.m111.238147
Tan, Y., Xin, X., Coffey, F. J., Wiest, D. L., Dong, L. Q., and Testa, J. R. (2016). Appl1 and Appl2 are expendable for mouse development but are essential for HGF-Induced Akt activation and migration in mouse embryonic fibroblasts. J. Cell. Physiol. 231, 1142–1150. doi: 10.1002/jcp.25211
Tan, Y., You, H., Coffey, F. J., Wiest, D. L., and Testa, J. R. (2010). Appl1 is dispensable for Akt signaling in vivo and mouse T-cell development. Genesis 48, 531–539. doi: 10.1002/dvg.20657
Tanaka, T., Narazaki, M., Ogata, A., and Kishimoto, T. (2014). A new era for the treatment of inflammatory autoimmune diseases by interleukin-6 blockade strategy. Semin. Immunol. 26, 88–96. doi: 10.1016/j.smim.2014.01.009
Tanaka, Y., Tanaka, N., Saeki, Y., Tanaka, K., Murakami, M., Hirano, T., et al. (2008). c-Cbl-dependent monoubiquitination and lysosomal degradation of gp130. Mol. Cell. Biol. 28, 4805–4818. doi: 10.1128/mcb.01784-07
Tebbutt, N. C., Giraud, A. S., Inglese, M., Jenkins, B., Waring, P., Clay, F. J., et al. (2002). Reciprocal regulation of gastrointestinal homeostasis by SHP2 and STAT-mediated trefoil gene activation in gp130 mutant mice. Nat. Med. 8, 1089–1097. doi: 10.1038/nm763
Thapa, N., Chen, M., Horn, H. T., Choi, S., Wen, T., and Anderson, R. A. (2020). Phosphatidylinositol-3-OH kinase signalling is spatially organized at endosomal compartments by microtubule-associated protein 4. Nat. Cell Biol. 22, 1357–1370. doi: 10.1038/s41556-020-00596-4
Thiel, S., Behrmann, I., Timmermann, A., Dahmen, H., Müller-Newen, G., Schaper, F., et al. (1999). Identification of a Leu-lle internalization motif within the cytoplasmic domain of the leukaemia inhibitory factor receptor. Biochem. J. 339(Pt 1), 15–19. doi: 10.1042/0264-6021:3390015
Thiel, S., Dahmen, H., Martens, A., Müller-Newen, G., Schaper, F., Heinrich, P. C., et al. (1998). Constitutive internalization and association with adaptor protein-2 of the interleukin-6 signal transducer gp130. FEBS Lett. 441, 231–234. doi: 10.1016/s0014-5793(98)01559-2
Thiem, S., Pierce, T. P., Palmieri, M., Putoczki, T. L., Buchert, M., Preaudet, A., et al. (2013). mTORC1 inhibition restricts inflammation-associated gastrointestinal tumorigenesis in mice. J. Clin. Invest. 123, 767–781.
Traum, D., Timothee, P., Silver, J., Rose-John, S., Ernst, M., and LaRosa, D. F. (2012). IL-10-induced gp130 expression in mouse mast cells permits IL-6 trans-signaling. J. Leukocyte Biol. 91, 427–435. doi: 10.1189/jlb.0411209
Uhlén, M., Fagerberg, L., Hallström, B. M., Lindskog, C., Oksvold, P., Mardinoglu, A., et al. (2015). Tissue-based map of the human proteome. Science 347:1260419.
Verboogen, D. R. J., Revelo, N. H., Ter Beest, M., and van den Bogaart, G. (2018). Interleukin-6 secretion is limited by self-signaling in endosomes. J. Mol. Cell. Biol. 11, 144–157. doi: 10.1093/jmcb/mjy038
Verma, N., Keinan, O., Selitrennik, M., Karn, T., Filipits, M., and Lev, S. (2015). PYK2 sustains endosomal-derived receptor signalling and enhances epithelial-to-mesenchymal transition. Nat. Commun. 6:6064.
Vietri, M., Radulovic, M., and Stenmark, H. (2020). The many functions of ESCRTs. Nat. Rev. Mol. Cell Biol. 21, 25–42. doi: 10.1038/s41580-019-0177-4
Vollmer, P., Oppmann, B., Voltz, N., Fischer, M., and Rose-John, S. (1999). A role for the immunoglobulin-like domain of the human IL-6 receptor. Eur. J. Biochem. 263, 438–446. doi: 10.1046/j.1432-1327.1999.00511.x
Waetzig, G. H., Chalaris, A., Rosenstiel, P., Suthaus, J., Holland, C., Karl, N., et al. (2010). N-linked glycosylation is essential for the stability but not the signaling function of the interleukin-6 signal transducer glycoprotein 130. J. Biol. Chem. 285, 1781–1789. doi: 10.1074/jbc.m109.075952
Wallenius, V., Wallenius, K., Ahrén, B., Rudling, M., Carlsten, H., Dickson, S. L., et al. (2002). Interleukin-6-deficient mice develop mature-onset obesity. Nat. Med. 8, 75–79. doi: 10.1038/nm0102-75
Wang, X. J., Taga, T., Yoshida, K., Saito, M., Kishimoto, T., and Kikutani, H. (1998). gp130, the cytokine common signal-transducer of interleukin-6 cytokine family, is downregulated in T cells in vivo by interleukin-6. Blood 91, 3308–3314. doi: 10.1182/blood.v91.9.3308
Wang, Y., and Fuller, G. M. (1995). Biosynthetic and glycosylation events of the IL-6 receptor beta-subunit, gp130. J. Cell. Biochem. 57, 610–618. doi: 10.1002/jcb.240570405
Werner-Klein, M., Grujovic, A., Irlbeck, C., Obradović, M., Hoffmann, M., Koerkel-Qu, H., et al. (2020). Interleukin-6 trans-signaling is a candidate mechanism to drive progression of human DCCs during clinical latency. Nat. Commun. 11:4977.
Wiederkehr-Adam, M., Ernst, P., Müller, K., Bieck, E., Gombert, F. O., Ottl, J., et al. (2003). Characterization of phosphopeptide motifs specific for the Src homology 2 domains of signal transducer and activator of transcription 1 (STAT1) and STAT3. J. Biol. Chem. 278, 16117–16128. doi: 10.1074/jbc.m300261200
Wilkinson, A. N., Gartlan, K. H., Kelly, G., Samson, L. D., Olver, S. D., Avery, J., et al. (2018). Granulocytes Are Unresponsive to IL-6 Due to an Absence of gp130. J. Immunol. 200, 3547–3555. doi: 10.4049/jimmunol.1701191
Williams, J. J. L., Alotaiq, N., Mullen, W., Burchmore, R., Liu, L., Baillie, G. S., et al. (2018). Interaction of suppressor of cytokine signalling 3 with cavin-1 links SOCS3 function and cavin-1 stability. Nat. Commun. 9:168.
Willis, E. F., MacDonald, K. P. A., Nguyen, Q. H., Garrido, A. L., Gillespie, E. R., Harley, S. B. R., et al. (2020). Repopulating microglia promote brain repair in an IL-6-dependent manner. Cell 180, 833–846.e16.
Xu, F., Mukhopadhyay, S., and Sehgal, P. B. (2007). Live cell imaging of interleukin-6-induced targeting of “transcription factor” STAT3 to sequestering endosomes in the cytoplasm. Am. J. Physiol. Cell Physiol. 293, C1374–C1382.
Xu, Y., Kershaw, N. J., Luo, C. S., Soo, P., Pocock, M. J., Czabotar, P. E., et al. (2010). Crystal structure of the entire ectodomain of gp130: insights into the molecular assembly of the tall cytokine receptor complexes. J. Biol. Chem. 285, 21214–21218.
Yan, L., Tsujita, K., Fujita, Y., and Itoh, T. (2021). PTEN is required for the migration and invasion of Ras-transformed MDCK cells. FEBS Lett. doi: 10.1002/1873-3468.14052 [Epub ahead of print].
Zabolotny, J. M., Bence-Hanulec, K. K., Stricker-Krongrad, A., Haj, F., Wang, Y., Minokoshi, Y., et al. (2002). PTP1B regulates leptin signal transduction in vivo. Dev. Cell 2, 489–495. doi: 10.1016/s1534-5807(02)00148-x
Zegeye, M. M., Lindkvist, M., Fälker, K., Kumawat, A. K., Paramel, G., Grenegård, M., et al. (2018). Activation of the JAK/STAT3 and PI3K/AKT pathways are crucial for IL-6 trans-signaling-mediated pro-inflammatory response in human vascular endothelial cells. Cell Commun. Signal. 16:55.
Zerial, M., and McBride, H. (2001). Rab proteins as membrane organizers. Nat. Rev. Mol. Cell Biol. 2, 107–117. doi: 10.1038/35052055
Zha, Z., Bucher, F., Nejatfard, A., Zheng, T., Zhang, H., Yea, K., et al. (2017). Interferon-γ is a master checkpoint regulator of cytokine-induced differentiation. Proc. Natl. Acad. Sci. U.S.A. 114, E6867–E6874.
Zilberstein, A., Ruggieri, R., Korn, J. H., and Revel, M. (1986). Structure and expression of cDNA and genes for human interferon-beta-2, a distinct species inducible by growth-stimulatory cytokines. EMBO J. 5, 2529–2537. doi: 10.1002/j.1460-2075.1986.tb04531.x
Keywords: IL-6, IL-11, GP130, cytokine, endosome, signal transduction, inflammation
Citation: Schmidt-Arras D and Rose-John S (2021) Endosomes as Signaling Platforms for IL-6 Family Cytokine Receptors. Front. Cell Dev. Biol. 9:688314. doi: 10.3389/fcell.2021.688314
Received: 30 March 2021; Accepted: 28 April 2021;
Published: 01 June 2021.
Edited by:
Geert Van Den Bogaart, University of Groningen, NetherlandsReviewed by:
Jennifer L. Stow, The University of Queensland, AustraliaPaige Lacy, University of Alberta, Canada
Harry Warner, University of Groningen, Netherlands
Copyright © 2021 Schmidt-Arras and Rose-John. This is an open-access article distributed under the terms of the Creative Commons Attribution License (CC BY). The use, distribution or reproduction in other forums is permitted, provided the original author(s) and the copyright owner(s) are credited and that the original publication in this journal is cited, in accordance with accepted academic practice. No use, distribution or reproduction is permitted which does not comply with these terms.
*Correspondence: Dirk Schmidt-Arras, ZGFycmFzQGJpb2NoZW0udW5pLWtpZWwuZGU=; Stefan Rose-John, cm9zZWpvaG5AYmlvY2hlbS51bmkta2llbC5kZQ==