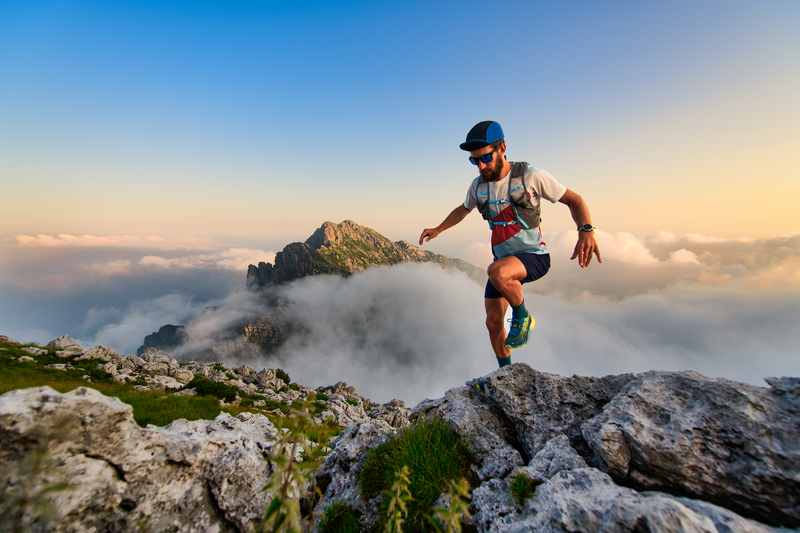
94% of researchers rate our articles as excellent or good
Learn more about the work of our research integrity team to safeguard the quality of each article we publish.
Find out more
REVIEW article
Front. Cell Dev. Biol. , 29 June 2021
Sec. Signaling
Volume 9 - 2021 | https://doi.org/10.3389/fcell.2021.682947
T cell protein tyrosine phosphatase (TCPTP), a vital regulator in glucose metabolism, inflammatory responses, and tumor processes, is increasingly considered a promising target for disease treatments and illness control. This review discusses the structure, substrates and main biological functions of TCPTP, as well as its regulatory effect in glucose metabolism, as an attempt to be referenced for formulating treatment strategies of metabolic disorders. Given the complicated regulation functions in different tissues and organs of TCPTP, the development of drugs inhibiting TCPTP with a higher specificity and a better biocompatibility is recognized as a promising therapeutic strategy for diabetes or obesity. Besides, treatments targeting TCPTP in a specific tissue or organ are suggested to be considerably promising.
Protein tyrosine kinases and phosphatases critically impact numerous biological activities (Denu et al., 1996; Humphrey et al., 2015). TCPTP, also known as protein tyrosine phosphatase non-receptor 2 (PTPN2) refers to a classical non-receptor protein tyrosine phosphatase, which was initially cloned from T-cell cDNA library (Cool et al., 1989; Mosinger et al., 1992; Andersen et al., 2001). TCPTP has two variants, i.e., TC45 and TC48. To be specific, TC45 is located in nuclear, and TC48 is located in endoplasmic reticulum (Bussieres-Marmen et al., 2014). TC45 is expressed in both human and mice, whereas TC48 is expressed only in human. In response to cytokine stimulation, TC45 shuttles from the nucleus to cytoplasm (Tiganis et al., 1999). TCPTP is capable of regulating pathways in glucose metabolism (Gurzov et al., 2014; Sharp et al., 2015; Wiede et al., 2019), inflammation control (Spalinger et al., 2018, 2020; Hsieh et al., 2020; Parlato et al., 2020), cancer progression (Stuible et al., 2008; Manguso et al., 2017; LaFleur et al., 2019; Wiede et al., 2020) and other biological processes by dephosphorylating distinct substrates (Bourdeau et al., 2005), including Janus activated kinase (JAK), signal transducer and activator of transcription (STAT), receptor tyrosine kinases (RTKs) and others. In the present review, the structure, main substrates (JAK/STAT and RTKs), biological functions of TCPTP, as well as its regulatory role in glucose metabolism are summarized, as an attempt to be referenced for developing treatment strategies of metabolic disorders.
Sakaguchi et al. (1992) initially detected Tcptp located on 18p11.2-p11.3 in humans. As impacted by the alternative splicing at the 3′ end of the gene, two isoforms of TCPTP are generated. TC45 composes 387 amino acids translated from ten exons. TC48 consists of 415 amino acids translated from nine exons. According to Figure 1A, exon 9a is identified in TC45 and TC48, while exon 9b only presents in TC48. TC45 is transcribed by exon 9a and exon 10, while TC48 is transcribed by exon 9a + 9b, but without exon 10 (Lorenzen et al., 1995). The exon 9a existing in both isoforms harbors the Nuclear localization signal (NLS) sequence, which can assure the protein located to the nucleus. However, a predominant hydrophobic sequence exists in the 9b exon of TC48. Such a hydrophobic sequence is an NLS inhibitor, capable of preventing TC48 from being located to the nuclear (Lorenzen et al., 1995).
Figure 1. Gene structures and expression profiles of TCPTP. (A) Gene structures of TCPTP (modified according to reference Bussieres-Marmen et al., 2014). Exon 9a is identified in both TC45 and TC48, while exon 9b only presents in TC48. TC45 is transcribed by exon 9a and exon 10, while TC48 is transcribed by exon 9a + 9b, but without exon 10. (B) Expression profiles of TCPTP in different normal tissues. (C) Expression profiles of TCPTP in various normal cells. Data are available on https://www.proteinatlas.org/. NX represents the consensus normalized expression level.
TCPTP is widely distributed in different tissues or cells and we have provided an overview of the mRNA expression files of TCPTP. As shown in Figure 1B, TCPTP is highly expressed in the thymus, pancreas and cerebellum tissues. As shown in Figure 1C, TCPTP is highly expressed in the urothelial cells, monocytes, and cone photoreceptor cells.
TCPTP regulates diverse signaling pathways by dephosphorylating distinct substrates [e.g., Janus activated kinase (JAK), Signal Transducer and Activator of Transcription (STAT), and Receptor Tyrosine Kinases (RTKs)]. This review summarizes the main substrates (JAK/STAT and RTKs) of TCPTP (Figure 2 and Table 1).
Figure 2. Main substrates of TCPTP (modified according to reference Bourdeau et al., 2005). Upon ligand binding, the receptor of IL-2, IL-4, IL-6, IFN-γ, GH, and leptin associated JAKs and STATs become activated. TCPTP can dephosphorylate JAK1 and JAK3, as well as STAT1, STAT3, STAT5, and STAT6 (molecules in blue), whereas it is not identical in different cytokine signaling according to summary of existing literatures. Besides, TCPTP is capable of regulating diverse signaling pathways by dephosphorylating various RTKs (e.g., IR, EGFR, VEGFR, PDGFR, and CSF-1R). TCPTP, T cell protein tyrosine phosphatase; IL-6, interleukin-6; IL-6R, interleukin-6 receptor; IFN-γ, interferon-γ; IFNGR, interferon-γ receptor; Leptin R, leptin receptor; IL-2, interleukin-2; IL-2R, interleukin-2 receptor; GH, growth hormone; GHR, growth hormone receptor; IL-4, interleukin-4; IL-4R, interleukin-4 receptor; JAK, Janus activated kinase; STAT, signal transducer and activator of transcription; RTKs, receptor tyrosine kinases; IR, insulin receptor; EGF, epidermal growth factor; EGFR, epidermal growth factor receptor; VEGF, vascular endothelial growth factor; VEGFR, vascular endothelial growth factor receptor; PDGF, platelet-derived growth factor; PDGFR, platelet-derived growth factor receptor; CSF-1, colony-stimulating factor-1; CSF-1R, colony-stimulating factor-1 receptor.
JAK1 and JAK3 are specific substrates of TCPTP (Kleppe et al., 2010; Luo et al., 2018; Zhang P. et al., 2018). The dephosphorylating sites refer to Y1022 and Y1023 in JAK1, while the dephosphorylating site of JAK3 has not been reported. Bone marrow-derived macrophages from Tcptp–/– mice showed hyper-phosphorylation of JAK1 after the interferon-γ (IFN-γ) treatment (Simoncic et al., 2002). Moreover, TCPTP interacts with JAK1 and JAK3 after activation of interleukin-2 (IL-2) receptor (Simoncic et al., 2002).
First, TCPTP accounts for STAT1 dephosphorylating (ten Hoeve et al., 2002), and it is involved in downregulation of interleukin-6 (IL-6) signaling (Twohig et al., 2019), interleukin-7 (IL-7) signaling (Pike et al., 2017), and IFN-γ signaling (Heinonen et al., 2009). Second, it has been evidenced that TCPTP is capable of dephosphorylating STAT3 at Y705 site (Zhang Y. et al., 2018) and then attenuate IL-6 signaling (Yamamoto et al., 2002), IFN-γ signaling (Scharl et al., 2010a), and leptin signaling (Loh et al., 2011). Third, TCPTP dephosphorylates STAT5 (Y694 in STAT5a and Y699 in STAT5b) and subsequently attenuates IL-2 signaling, growth hormone signaling and leptin signaling that are associated with T cell differentiation, energy regulation, etc. (Yu et al., 2000; Aoki and Matsuda, 2002). Lastly, STAT6 is also a reported substrate for TCPTP. It is evidenced that TCPTP knockdown will increase IL-4-induced STAT6 signaling in B-cell lymphomas (Lu et al., 2005, 2007).
IR refers to a transmembrane protein tyrosine kinase. IR can phosphorylate its downstream substrates [e.g., the Insulin Receptor Substrate-1 (IRS-1)] upon binding insulin (Belfiore et al., 2017). TCPTP can downregulate insulin signaling by dephosphorylating IR (Galic et al., 2003; Tiganis, 2013).
EGFR can be activated by EGF family directly, which has been identified as a specific substrates of TCPTP (Tiganis et al., 1998). As revealed from in-depth studies, the knockdown of TCPTP can facilitate EGFR tyrosine phosphorylation. The residues dephosphorylated by TCPTP are identified as Y992 and Y1068 in Hela cells and human colonic T84 epithelial cells (Mattila et al., 2005; Scharl et al., 2010b).
TCPTP significantly limits the VEGF/VEGFR signaling and consequently preventing excessive angiogenesis. TCPTP dephosphorylates VEGFR in a phosphosite-specific manner and inhibits its kinase activity (Mattila et al., 2008). Baek et al. (2018) reported that epidermal-specific knockout of TCPTP can improve UVB-induced epidermal cell survival by dephosphorylating VEGFR and then regulating VEGFR/JNK signaling.
TCPTP can control the embryo differentiation and fibroblasts proliferation by regulating the PDGF/PDGFR signaling pathway (Persson et al., 2004; Karlsson et al., 2006). Kramer et al. reported that TCPTP knockdown could increase PDGFR phosphorylation at Y751 and Y1021, thereby leading to an enhanced downstream signaling and increased growth rates of fibroblasts (Kramer et al., 2020).
Colony-Stimulating Factor 1 (CSF-1) regulates the survival and differentiation of mononuclear phagocytes by binding with CSF-1R (Stanley and Chitu, 2014). According to Simoncic et al. (2006) TCPTP could negatively regulate the CSF-1/CSF-1R signaling. Zhang et al. (2019) reported that TCPTP could significantly inhibit alveolar bone resorption by dephosphorylating CSF-1R at Y807 site.
According to Figure 3, the manifestations in glucose metabolism, immunoregulation and oncogenesis in Tcptp knockout models are summarized.
Figure 3. TCPTP-mediated biological processes in different tissues and organs. The experimental models, the resulting effects, the tissues or organs and the biological functions of TCPTP are presented from the outside to the inside of the pie chart. OE indicates “overexpression.”
The effect of TCPTP on glucose metabolism varies with tissues. In pancreas, pancreas-specific Tcptp–/– mice exhibits impaired glucose tolerance and Glucose-Stimulated Insulin Secretion (GSIS) when challenged with high fat feeding (Xi et al., 2015). In liver, TCPTP ablation results in enhanced growth hormone signaling, weight gain, insulin resistance and hepatic steatosis sequentially (Gurzov et al., 2015). The fasted blood glucose and hepatic glucose output decrease in Tcptp+/– mice (Fukushima et al., 2010). In adipose, TCPTP overexpression in Epididymal White Adipose Tissue (EWAT) reverses the high Th17/Treg and M1/M2 macrophage ratios significantly, improving insulin resistance of diabetic mice (Li et al., 2018). In muscle, muscle-specific Tcptp deficiency does not impact insulin signaling and glucose homeostasis (Loh et al., 2012). In bone tissue, mice lacking TCPTP in osteoblasts showed an increased bone resorption, osteocalcin bioactivity, and insulin sensitivity (Zee et al., 2012). In brain, TCPTP deletion in Agouti-Related Peptide (AgRP) neurons facilitates insulin sensitivity (Dodd et al., 2018).
Overall, TCPTP can inhibit inflammation. Tcptp–/– mice die soon after birth as impacted by increased numbers of macrophages in spleen and severe systemic inflammatory disease (You-Ten et al., 1997; Heinonen et al., 2004; Scharl et al., 2010a). Spalinger et al. (2020) demonstrated that macrophage-specific TCPTP deficiency increased intestinal permeability via IL-6 release. Besides, Tcptp–/– mice developed B cell deficiency, which was because pre-B cells failed to transit to immature B cells (You-Ten et al., 1997; Doody et al., 2009). For T cells, TCPTP negatively regulates T cell proliferation and activation (Rhee and Veillette, 2012). Hyper-phosphorylation of the activated Lck and reduction of TCR threshold were proved to account for increased T cell activation in Tcptp–/– T cells (Wiede et al., 2011). The infusion of Tcptp–/– CD4+ T cells to colitis mice led to an almost threefold increase of Th1 cell frequency, a twofold increase of Th17 cell frequency and in contrast, a threefold decrease of Tregs frequency (Spalinger et al., 2015).
As demonstrated from the generation of epidermal-specific TCPTP-deficient mice, TCPTP suppresses skin tumor formation by down-regulating STAT3 and AKT signaling (Morales et al., 2019). Furthermore, the numbers of skin tumors decrease significantly in epidermal-specific TCPTP-overexpression mice (Kim et al., 2020). TCPTP deletion in hepatocytes promotes non-alcoholic steatohepatitis as well as hepatocellular carcinoma in obese mice (Grohmann et al., 2018). Besides, TCPTP can inhibit oncogenesis of breast cancer (Shields et al., 2013; Veenstra et al., 2019), most of the hematopoietic malignancies (Kleppe et al., 2011; Pike and Tremblay, 2016) and glioblastoma (Navis et al., 2010).
Insulin resistance characterized by insulin signaling pathway defects refers to the key pathological property exhibited by type 2 diabetes (Ono, 2019; Diane et al., 2021; He et al., 2021). Liver, brain, and muscle are the main insulin-sensitive organs (Pomytkin et al., 2018; Tokarz et al., 2018). In liver, insulin-induced phosphatidylinositol 3-kinase (PI3K)/Akt signaling pathway inhibits the expression of key enzymes of gluconeogenesis (Glucose-6-phosphatase and phosphoenolpyruvate carboxykinase) to decrease hepatic glucose production (Saltiel and Kahn, 2001). In TCPTP heterozygous deficiency mice, gluconeogenesis and hepatic glucose output were suppressed, the expression of gluconeogenic genes (glucose-6-phosphatase-alpha and phosphoenolpyruvate carboxykinase 1) were decreased, and the expression of lipogenic genes (sterol regulatory element-binding protein 1c and fatty acid synthase) were increased (Fukushima et al., 2010). According to Figure 4A, IR phosphorylation and PI3K/Akt signaling are up-regulated in hepatocytes derived from Tcptp+/– mice (Fukushima et al., 2010). As shown in Figure 4B, TCPTP is the principal protein tyrosine phosphatase inhibiting insulin signaling in hypothalamus, especially in appetite-suppressing Proopiomelanocortin (POMC) neurons. TCPTP deficiency in POMC neurons can enhance insulin-induced AKT phosphorylation, improve glucose homeostasis, and prevent diet-induced obesity by increasing white adipose tissue browning and energy expenditure (Dodd et al., 2015). TCPTP deficiency in AgRP neurons improves systemic insulin sensitivity by regulating IR signaling, represses hepatic glucose production, and increases Brown Adipose Tissue (BAT) glucose uptake (Dodd et al., 2018). Though TCPTP regulates IR signaling in the liver and hypothalamus neurons, this does not extend to muscle. Muscle-specific lack of TCPTP do not exhibit any insulin sensitivity alterations in gastrocnemius muscle in vivo or in myoblasts in vitro (Loh et al., 2012). Besides, muscle glucose uptake and whole-body glucose tolerance are not impacted by TCPTP deficiency (Loh et al., 2012). What’s more, whole-body glucose homeostasis is also related to osteocalcin activity, which is regulated by insulin signaling in osteoblasts. Osteoblast-specific deletion of TCPTP promotes insulin sensitivity in an osteocalcin-dependent manner (Zee et al., 2012).
Figure 4. Molecular pathways of TCPTP in regulating glucose metabolism in (A) liver, (B) hypothalamus, and (C) pancreas. In liver, TCPTP can dephosphorylate IR and STAT3 to inhibit insulin signaling and IL-6 signaling separately to promote hepatic glucose output. In hypothalamus, TCPTP may act as the principal phosphatase to attenuate insulin and leptin signaling by inhibiting insulin-induced AKT phosphorylation and leptin-induced STAT3 signaling. In pancreas, TCPTP modulates IFN-induced β cell death by dephosphorylating pro-apoptotic protein Bim via JNK1. Furthermore, TCPTP is capable of effectively suppressing the IFN-γ/STAT1 signaling pathway in INS-1E cells. TCPTP, T cell protein tyrosine phosphatase; IL-6, interleukin-6; IL-6R, interleukin-6 receptor; HGP, hepatic glucose production; GH, growth hormone; GHR, growth hormone receptor; IR, insulin receptor; Leptin R, leptin receptor; IFN-γ, interferon-γ; IFNGR, interferon-γ receptor; JAK, Janus activated kinase; STAT, signal transducer and activator of transcription; IRS, insulin receptor substrate; PI3K, phosphatidylinositol 3-kinase; AKT, protein kinase B; JNK1, c-Jun N-terminal kinase 1.
Leptin coordinates feeding, thermogenesis, as well as glucose homeostasis primarily via hypothalamic circuits (Adlanmerini et al., 2021; Montserrat-de la Paz et al., 2021; Perakakis et al., 2021; Pereira et al., 2021). The leptin pathway promotes anorexigenic neuropeptide POMC expression and represses the orexigenic Agouti-Related Peptide (AgRP)/Neuropeptide Y (NPY) expression (Zhang et al., 2015; Santoro et al., 2017). Leptin resistance in hypothalamic neurons plays a key role in exacerbating diet-induced obesity. As shown in Figure 4B, TCPTP is the key protein tyrosine phosphatase inhibiting leptin signaling in hypothalamus. As reported by existing studies, when the expression of hypothalamic TCPTP is up-regulated, the leptin signaling pathway will be attenuated, and the energy expenditure will decrease, thereby causing an increased weight gain (Loh et al., 2011). Hypothalamus-specific Tcptp–/– mice can develop an enhanced leptin sensitivity, decreased food intake, reduced adiposity, and improved glucose metabolism (Dodd et al., 2019). TCPTP deletion in neuronal cells could not only promote the leptin-induced STAT3 signaling but also alter POMC and AgRP expression (Loh et al., 2011). However, TCPTP deletion in POMC neurons alone showed no effects on leptin signaling (Dodd et al., 2015).
Type 1 diabetes mellitus (T1DM) is attributed to pancreatic β cells destruction and insulin production insufficient (Katsarou et al., 2017; Li et al., 2017; Wei et al., 2019; Eizirik et al., 2020; Pang et al., 2020). According to Ounissi-Benkalha and Polychronakos (2008), Sharp et al. (2015), and Wiede et al. (2019), TCPTP is an important locus associated with T1DM. As revealed from in-depth studies, TCPTP can essentially ameliorate β cells from apoptosis caused by virus, dsDNA and IFN-γ (Op de Beeck and Eizirik, 2016). As shown in Figure 4C, TCPTP modulates IFN-induced β cell death by dephosphorylating pro-apoptotic protein Bim via JNK1 (Santin et al., 2011; Marroqui et al., 2014). In addition, siRNA targeting TCPTP exacerbates IFN-γ-induced apoptosis of INS-1E cells while double knockdown of TCPTP and STAT1 protects INS-1E cells against cytokine-induced apoptosis, which indicates that TCPTP can effectively suppress the IFN-γ/STAT1 signaling pathway in pancreatic cells to inhibit inflammation (Moore et al., 2009).
In liver, TCPTP ablation can facilitate growth hormone signaling and weight gain (Gurzov et al., 2015). TCPTP deletion in neuronal cells is capable of elevating the GH-induced STAT5 phosphorylation, decreasing the circulating GH levels, and increasing adiposity (Loh et al., 2011). Given the crosstalk between GH and insulin signaling (Huang et al., 2020), the systemic regulation effect of TCPTP is extremely complex, which requires in-depth investigations.
IL-6-instigated JAK/STAT3 signaling pathway has emerged as a significant mechanism for decreasing hepatic glucose production by inhibiting the expression of gluconeogenic genes (Inoue et al., 2004). Fukushima et al. (2010) reported that IL-6-induced STAT3 phosphorylation but not JAK1 was enhanced in Tcptp+/– hepatocytes and hepatic glucose output was decreased in Tcptp+/– mice. The results confirm the negative regulatory capacity of TCPTP acting directly on STAT3 in the liver to regulate gluconeogenesis.
IR and leptin signaling downregulation by TCPTP offers the possibility to develop TCPTP inhibitors as potential treatment strategy for T2DM or obesity. Sodium metavanadate refers to the first phosphatase inhibitors to be used clinically to improve human diabetes, which was initially used in 1899 (Heneberg, 2009). However, for the lack of specificity, they could cause unexpected side effects. In 2009, an extremely selective TCPTP inhibitor was synthesized, which showed 200-fold selectivity for TCPTP over other protein tyrosine phosphatases (Zhang et al., 2009). It is noteworthy that Loh et al. confirmed that administration of this inhibitor into cerebral ventricles could enhance leptin-induced STAT3 phosphorylation and energy expenditure in wild type mice (Loh et al., 2011) and up-regulate insulin-induced POMC expression by 2.5-fold (Dodd et al., 2015). Besides, some natural agents (e.g., celastrol) could promote weight loss in diet-induced obesity by inhibiting TCPTP in the hypothalamus (Kyriakou et al., 2018). Furthermore, Dodd et al. (2019) reported that the daily intranasal administration of the glucocorticoid antagonist RU486 down-regulating TCPTP expression could significantly help promote weight loss and improve glucose metabolism in obese mice.
Though the difficulties of TCPTP selectivity have been addressed, major challenges remain in tissues specific to the development of TCPTP inhibitors. This is of particularly importance for the role of TCPTP in hematopoietic development, immunoregulation and oncogenesis. The in situ delivery and sustained release of drugs targeting TCPTP in specific organs or tissues may be a promising development direction.
On the whole, given the role of TCPTP in glucose metabolism by regulating insulin, leptin and other signaling pathways, this review considers that the development of specific and effective TCPTP inhibitors for individual cells, tissues or organs is a promising therapeutic strategy for diabetes or obesity. Moreover, the in situ delivery and sustained release systems of TCPTP inhibitors in specific tissues should be investigated in subsequent studies.
YW and SL drafted the initial manuscript. TJ and YF made substantial contributions to the acquisition of data. XX and DZ critically reviewed it for important intellectual content. All authors gave the final approval of the version to be published.
This work was supported by the National Natural Science Foundation of China (Nos. 82071148 and 82001055), the Construction Engineering Special Fund of Taishan Scholars (No. TS201511106), the Key Project of Chinese National Programs for Research and Development (No. 2016YFC1102705), the Shandong Provincial Natural Science Foundation (No. ZR2020QH158), the Fundamental Research Funds of Shandong University (No. 2018GN024), the China Postdoctoral Science Foundation (No. 2019M662371), and the Youth Scientific Research Funds of School of Stomatology, Shandong University (No. 2019QNJJ03).
The authors declare that the research was conducted in the absence of any commercial or financial relationships that could be construed as a potential conflict of interest.
Adlanmerini, M., Nguyen, H. C., Krusen, B. M., Teng, C. W., Geisler, C. E., Peed, L. C., et al. (2021). Hypothalamic rev-erb nuclear receptors control diurnal food intake and leptin sensitivity in diet-induced obese mice. J. Clin. Invest. 131:e140424. doi: 10.1172/jci140424
Andersen, J. N., Mortensen, O. H., Peters, G. H., Drake, P. G., Iversen, L. F., Olsen, O. H., et al. (2001). Structural and evolutionary relationships among protein tyrosine phosphatase domains. Mol. Cell. Biol. 21, 7117–7136. doi: 10.1128/mcb.21.21.7117-7136.2001
Aoki, N., and Matsuda, T. (2002). A nuclear protein tyrosine phosphatase tc-ptp is a potential negative regulator of the prl-mediated signaling pathway: dephosphorylation and deactivation of signal transducer and activator of transcription 5a and 5b by tc-ptp in nucleus. Mol. Endocrinol. 16, 58–69. doi: 10.1210/mend.16.1.0761
Baek, M., Kim, M., Lim, J. S., Morales, L. D., Hernandez, J., Mummidi, S., et al. (2018). Epidermal-specific deletion of tc-ptp promotes uvb-induced epidermal cell survival through the regulation of flk-1/jnk signaling. Cell Death Dis. 9, 730–730. doi: 10.1038/s41419-018-0781-9
Belfiore, A., Malaguarnera, R., Vella, V., Lawrence, M. C., Sciacca, L., Frasca, F., et al. (2017). Insulin receptor isoforms in physiology and disease: an updated view. Endocr. Rev. 38, 379–431. doi: 10.1210/er.2017-00073
Bourdeau, A., Dube, N., and Tremblay, M. L. (2005). Cytoplasmic protein tyrosine phosphatases, regulation and function: the roles of ptp1b and tc-ptp. Curr. Opin. Cell Biol. 17, 203–209. doi: 10.1016/j.ceb.2005.02.001
Bussieres-Marmen, S., Hutchins, A. P., Schirbel, A., Rebert, N., Tiganis, T., Fiocchi, C., et al. (2014). Characterization of ptpn2 and its use as a biomarker. Methods 65, 239–246. doi: 10.1016/j.ymeth.2013.08.020
Cool, D. E., Tonks, N. K., Charbonneau, H., Walsh, K. A., Fischer, E. H., and Krebs, E. G. (1989). Cdna isolated from a human t-cell library encodes a member of the protein-tyrosine-phosphatase family. Proc. Natl. Acad. Sci. U. S. A. 86, 5257–5261. doi: 10.1073/pnas.86.14.5257
Denu, J. M., Stuckey, J. A., Saper, M. A., and Dixon, J. E. (1996). Form and function in protein dephosphorylation. Cell 87, 361–364. doi: 10.1016/s0092-8674(00)81356-2
Diane, A., Abunada, H., Khattab, N., Md Moin, A. S., Butler, A. E., and Dehbi, M. (2021). Role of the dnaj/hsp40 family in the pathogenesis of insulin resistance and type 2 diabetes. Ageing Res. Rev. 67:101313. doi: 10.1016/j.arr.2021.101313
Dodd, G. T., Decherf, S., Loh, K., Simonds, S. E., Wiede, F., Balland, E., et al. (2015). Leptin and insulin act on pomc neurons to promote the browning of white fat. Cell 160, 88–104. doi: 10.1016/j.cell.2014.12.022
Dodd, G. T., Lee-Young, R. S., Bruning, J. C., and Tiganis, T. (2018). Tcptp regulates insulin signaling in agrp neurons to coordinate glucose metabolism with feeding. Diabetes 67, 1246–1257. doi: 10.2337/db17-1485
Dodd, G. T., Xirouchaki, C. E., Eramo, M., Mitchell, C. A., Andrews, Z. B., Henry, B. A., et al. (2019). Intranasal targeting of hypothalamic ptp1b and tcptp reinstates leptin and insulin sensitivity and promotes weight loss in obesity. Cell Rep. 28, 2905–2922.e5. doi: 10.1016/j.celrep.2019.08.019
Doody, K. M., Bourdeau, A., and Tremblay, M. L. (2009). T-cell protein tyrosine phosphatase is a key regulator in immune cell signaling: lessons from the knockout mouse model and implications in human disease. Immunol. Rev. 228, 325–341. doi: 10.1111/j.1600-065X.2008.00743.x
Eizirik, D. L., Pasquali, L., and Cnop, M. (2020). Pancreatic β-cells in type 1 and type 2 diabetes mellitus: different pathways to failure. Nat. Rev. Endocrinol. 16, 349–362. doi: 10.1038/s41574-020-0355-7
Fukushima, A., Loh, K., Galic, S., Fam, B., Shields, B., Wiede, F., et al. (2010). T-cell protein tyrosine phosphatase attenuates stat3 and insulin signaling in the liver to regulate gluconeogenesis. Diabetes 59, 1906–1914. doi: 10.2337/db09-1365
Galic, S., Klingler-Hoffmann, M., Fodero-Tavoletti, M. T., Puryer, M. A., Meng, T. C., Tonks, N. K., et al. (2003). Regulation of insulin receptor signaling by the protein tyrosine phosphatase tcptp. Mol. Cell. Biol. 23, 2096–2108. doi: 10.1128/mcb.23.6.2096-2108.2003
Grohmann, M., Wiede, F., Dodd, G. T., Gurzov, E. N., Ooi, G. J., Butt, T., et al. (2018). Obesity drives stat-1-dependent nash and stat-3-dependent hcc. Cell 175, 1289–1306.e20. doi: 10.1016/j.cell.2018.09.053
Gurzov, E. N., Stanley, W. J., Brodnicki, T. C., and Thomas, H. E. (2015). Protein tyrosine phosphatases: molecular switches in metabolism and diabetes. Trends Endocrinol. Metab. 26, 30–39. doi: 10.1016/j.tem.2014.10.004
Gurzov, E. N., Tran, M., Fernandez-Rojo, M. A., Merry, T. L., Zhang, X., Xu, Y., et al. (2014). Hepatic oxidative stress promotes insulin-stat-5 signaling and obesity by inactivating protein tyrosine phosphatase n2. Cell Metab. 20, 85–102. doi: 10.1016/j.cmet.2014.05.011
He, F., Huang, Y., Song, Z., Zhou, H. J., Zhang, H., Perry, R. J., et al. (2021). Mitophagy-mediated adipose inflammation contributes to type 2 diabetes with hepatic insulin resistance. J. Exp. Med. 218:e20201416. doi: 10.1084/jem.20201416
Heinonen, K. M., Bourdeau, A., Doody, K. M., and Tremblay, M. L. (2009). Protein tyrosine phosphatases ptp-1b and tc-ptp play nonredundant roles in macrophage development and ifn-gamma signaling. Proc. Natl. Acad. Sci. U. S. A. 106, 9368–9372. doi: 10.1073/pnas.0812109106
Heinonen, K. M., Nestel, F. P., Newell, E. W., Charette, G., Seemayer, T. A., Tremblay, M. L., et al. (2004). T-cell protein tyrosine phosphatase deletion results in progressive systemic inflammatory disease. Blood 103, 3457–3464. doi: 10.1182/blood-2003-09-3153
Heneberg, P. (2009). Use of protein tyrosine phosphatase inhibitors as promising targeted therapeutic drugs. Curr. Med. Chem. 16, 706–733. doi: 10.2174/092986709787458407
Hsieh, W. C., Svensson, M. N., Zoccheddu, M., Tremblay, M. L., Sakaguchi, S., Stanford, S. M., et al. (2020). Ptpn2 links colonic and joint inflammation in experimental autoimmune arthritis. JCI Insight 5:e141868. doi: 10.1172/jci.insight.141868
Huang, Z., Huang, L., Waters, M. J., and Chen, C. (2020). Insulin and growth hormone balance: Implications for obesity. Trends Endocrinol. Metab. 31, 642–654. doi: 10.1016/j.tem.2020.04.005
Humphrey, S. J., James, D. E., and Mann, M. (2015). Protein phosphorylation: a major switch mechanism for metabolic regulation. Trends Endocrinol. Metab. 26, 676–687. doi: 10.1016/j.tem.2015.09.013
Inoue, H., Ogawa, W., Ozaki, M., Haga, S., Matsumoto, M., Furukawa, K., et al. (2004). Role of stat-3 in regulation of hepatic gluconeogenic genes and carbohydrate metabolism in vivo. Nat. Med. 10, 168–174. doi: 10.1038/nm980
Karlsson, S., Kowanetz, K., Sandin, A., Persson, C., Ostman, A., Heldin, C.-H., et al. (2006). Loss of t-cell protein tyrosine phosphatase induces recycling of the platelet-derived growth factor (pdgf) beta-receptor but not the pdgf alpha-receptor. Mol. Biol. Cell 17, 4846–4855. doi: 10.1091/mbc.e06-04-0306
Katsarou, A., Gudbjörnsdottir, S., Rawshani, A., Dabelea, D., Bonifacio, E., Anderson, B. J., et al. (2017). Type 1 diabetes mellitus. Nat. Rev. Dis. Primers 3:17016. doi: 10.1038/nrdp.2017.16
Kim, M., Morales, L. D., Lee, C. J., Olivarez, S. A., Kim, W. J., Hernandez, J., et al. (2020). Overexpression of tc-ptp in murine epidermis attenuates skin tumor formation. Oncogene 39, 4241–4256. doi: 10.1038/s41388-020-1282-8
Kleppe, M., Lahortiga, I., El Chaar, T., De Keersmaecker, K., Mentens, N., Graux, C., et al. (2010). Deletion of the protein tyrosine phosphatase gene ptpn2 in t-cell acute lymphoblastic leukemia. Nat. Genet. 42, 530–535. doi: 10.1038/ng.587
Kleppe, M., Soulier, J., Asnafi, V., Mentens, N., Hornakova, T., Knoops, L., et al. (2011). Ptpn2 negatively regulates oncogenic jak1 in t-cell acute lymphoblastic leukemia. Blood 117, 7090–7098. doi: 10.1182/blood-2010-10-314286
Kramer, F., Dernedde, J., Mezheyeuski, A., Tauber, R., Micke, P., and Kappert, K. (2020). Platelet-derived growth factor receptor beta activation and regulation in murine myelofibrosis. Haematologica 105, 2083–2094. doi: 10.3324/haematol.2019.226332
Kyriakou, E., Schmidt, S., Dodd, G. T., Pfuhlmann, K., Simonds, S. E., Lenhart, D., et al. (2018). Celastrol promotes weight loss in diet-induced obesity by inhibiting the protein tyrosine phosphatases ptp1b and tcptp in the hypothalamus. J. Med. Chem. 61, 11144–11157. doi: 10.1021/acs.jmedchem.8b01224
LaFleur, M. W., Nguyen, T. H., Coxe, M. A., Miller, B. C., Yates, K. B., Gillis, J. E., et al. (2019). Ptpn2 regulates the generation of exhausted cd8(+) t cell subpopulations and restrains tumor immunity. Nat. Immunol. 20, 1335–1347. doi: 10.1038/s41590-019-0480-4
Li, W., Huang, E., and Gao, S. (2017). Type 1 diabetes mellitus and cognitive impairments: a systematic review. J. Alzheimers Dis. 57, 29–36. doi: 10.3233/jad-161250
Li, Y., Zhou, H. M., Wang, F., Han, L., Liu, M. H., Li, Y. H., et al. (2018). Overexpression of ptpn2 in visceral adipose tissue ameliorated atherosclerosis via t cells polarization shift in diabetic apoe(-/-) mice. Cell. Physiol. Biochem. 46, 118–132. doi: 10.1159/000488415
Loh, K., Fukushima, A., Zhang, X., Galic, S., Briggs, D., and Enriori, et al. (2011). Elevated hypothalamic tcptp in obesity contributes to cellular leptin resistance. Cell Metab. 14, 684–699. doi: 10.1016/j.cmet.2011.09.011
Loh, K., Merry, T. L., Galic, S., Wu, B. J., Watt, M. J., Zhang, S., et al. (2012). T cell protein tyrosine phosphatase (tcptp) deficiency in muscle does not alter insulin signalling and glucose homeostasis in mice. Diabetologia 55, 468–478. doi: 10.1007/s00125-011-2386-z
Lorenzen, J. A., Dadabay, C. Y., and Fischer, E. H. (1995). Cooh-terminal sequence motifs target the t cell protein tyrosine phosphatase to the er and nucleus. J. Cell Biol. 131, 631–643. doi: 10.1083/jcb.131.3.631
Lu, X., Chen, J., Sasmono, R. T., Hsi, E. D., Sarosiek, K. A., Tiganis, T., et al. (2007). T-cell protein tyrosine phosphatase, distinctively expressed in activated-b-cell-like diffuse large b-cell lymphomas, is the nuclear phosphatase of stat6. Mol. Cell. Biol. 27, 2166–2179. doi: 10.1128/mcb.01234-06
Lu, X., Nechushtan, H., Ding, F., Rosado, M. F., Singal, R., Alizadeh, A. A., et al. (2005). Distinct il-4-induced gene expression, proliferation, and intracellular signaling in germinal center b-cell-like and activated b-cell-like diffuse large-cell lymphomas. Blood 105, 2924–2932. doi: 10.1182/blood-2004-10-3820
Luo, N., Formisano, L., Gonzalez-Ericsson, P. I., Sanchez, V., Dean, P. T., Opalenik, S. R., et al. (2018). Melanoma response to anti-pd-l1 immunotherapy requires jak1 signaling, but not jak2. Oncoimmunology 7:e1438106. doi: 10.1080/2162402X.2018.1438106
Manguso, R. T., Pope, H. W., Zimmer, M. D., Brown, F. D., Yates, K. B., Miller, B. C., et al. (2017). In vivo crispr screening identifies ptpn2 as a cancer immunotherapy target. Nature 547, 413–418. doi: 10.1038/nature23270
Marroqui, L., Santin, I., Dos Santos, R. S., Marselli, L., Marchetti, P., and Eizirik, D. L. (2014). Bach2, a candidate risk gene for type 1 diabetes, regulates apoptosis in pancreatic beta-cells via jnk1 modulation and crosstalk with the candidate gene ptpn2. Diabetes 63, 2516–2527. doi: 10.2337/db13-1443
Mattila, E., Auvinen, K., Salmi, M., and Ivaska, J. (2008). The protein tyrosine phosphatase tcptp controls vegfr2 signalling. J. Cell Sci. 121, 3570–3580. doi: 10.1242/jcs.031898
Mattila, E., Pellinen, T., Nevo, J., Vuoriluoto, K., Arjonen, A., and Ivaska, J. (2005). Negative regulation of egfr signalling through integrin-alpha1beta1-mediated activation of protein tyrosine phosphatase tcptp. Nat. Cell Biol. 7, 78–85. doi: 10.1038/ncb1209
Montserrat-de la Paz, S., Pérez-Pérez, A., Vilariño-García, T., Jiménez-Cortegana, C., Muriana, F. J. G., Millán-Linares, M. C., et al. (2021). Nutritional modulation of leptin expression and leptin action in obesity and obesity-associated complications. J. Nutr. Biochem. 89:108561. doi: 10.1016/j.jnutbio.2020.108561
Moore, F., Colli, M. L., Cnop, M., Esteve, M. I., Cardozo, A. K., Cunha, D. A., et al. (2009). Ptpn2, a candidate gene for type 1 diabetes, modulates interferon-gamma-induced pancreatic beta-cell apoptosis. Diabetes 58, 1283–1291. doi: 10.2337/db08-1510
Morales, L. D., Archbold, A. K., Olivarez, S., Slaga, T. J., DiGiovanni, J., and Kim, D. J. (2019). The role of t-cell protein tyrosine phosphatase in epithelial carcinogenesis. Mol. Carcinog. 58, 1640–1647. doi: 10.1002/mc.23078
Mosinger, B. Jr., Tillmann, U., Westphal, H., and Tremblay, M. L. (1992). Cloning and characterization of a mouse cdna encoding a cytoplasmic protein-tyrosine-phosphatase. Proc. Natl. Acad. Sci. U. S. A. 89, 499–503. doi: 10.1073/pnas.89.2.499
Navis, A. C., van den Eijnden, M., Schepens, J. T. G., Hooft van Huijsduijnen, R., Wesseling, P., et al. (2010). Protein tyrosine phosphatases in glioma biology. Acta Neuropathol. 119, 157–175. doi: 10.1007/s00401-009-0614-0
Ono, H. (2019). Molecular mechanisms of hypothalamic insulin resistance. Int. J. Mol. Sci. 20:1317. doi: 10.3390/ijms20061317
Op de Beeck, A., and Eizirik, D. L. (2016). Viral infections in type 1 diabetes mellitus–why the β cells? Nat. Rev. Endocrinol. 12, 263–273. doi: 10.1038/nrendo.2016.30
Ounissi-Benkalha, H., and Polychronakos, C. (2008). The molecular genetics of type 1 diabetes: new genes and emerging mechanisms. Trends Mol. Med. 14, 268–275. doi: 10.1016/j.molmed.2008.04.002
Pang, H., Luo, S., Huang, G., Xia, Y., Xie, Z., and Zhou, Z. (2020). Advances in knowledge of candidate genes acting at the beta-cell level in the pathogenesis of t1dm. Front. Endocrinol. 11:119. doi: 10.3389/fendo.2020.00119
Parlato, M., Nian, Q., Charbit-Henrion, F., Ruemmele, F. M., Rodrigues-Lima, F., and Cerf-Bensussan, N. (2020). Loss-of-function mutation in ptpn2 causes aberrant activation of jak signaling via stat and very early onset intestinal inflammation. Gastroenterology 159, 1968–1971. doi: 10.1053/j.gastro.2020.07.040
Perakakis, N., Farr, O. M., and Mantzoros, C. S. (2021). Leptin in leanness and obesity: jacc state-of-the-art review. J. Am. Coll. Cardiol. 77, 745–760. doi: 10.1016/j.jacc.2020.11.069
Pereira, S., Cline, D. L., Glavas, M. M., Covey, S. D., and Kieffer, T. J. (2021). Tissue-specific effects of leptin on glucose and lipid metabolism. Endocr. Rev. 42, 1–28. doi: 10.1210/endrev/bnaa027
Persson, C., Sävenhed, C., Bourdeau, A., Tremblay, M. L., Markova, B., Böhmer, F. D., et al. (2004). Site-selective regulation of platelet-derived growth factor beta receptor tyrosine phosphorylation by t-cell protein tyrosine phosphatase. Mol. Cell. Biol. 24, 2190–2201. doi: 10.1128/mcb.24.5.2190-2201.2004
Pike, K. A., Hatzihristidis, T., Bussieres-Marmen, S., Robert, F., Desai, N., Miranda-Saavedra, D., et al. (2017). Tc-ptp regulates the il-7 transcriptional response during murine early t cell development. Sci. Rep. 7:13275. doi: 10.1038/s41598-017-13673-w
Pike, K. A., and Tremblay, M. L. (2016). Tc-ptp and ptp1b: regulating jak-stat signaling, controlling lymphoid malignancies. Cytokine 82, 52–57. doi: 10.1016/j.cyto.2015.12.025
Pomytkin, I., Costa-Nunes, J. P., Strekalova, T., Kasatkin, V., Veniaminova, E., Demchenko, A., et al. (2018). Insulin receptor in the brain: mechanisms of activation and the role in the cns pathology and treatment. CNS Neurosci. Ther. 24, 763–774. doi: 10.1111/cns.12866
Rhee, I., and Veillette, A. (2012). Protein tyrosine phosphatases in lymphocyte activation and autoimmunity. Nat. Immunol. 13, 439–447. doi: 10.1038/ni.2246
Sakaguchi, A. Y., Sylvia, V. L., Martinez, L., Smith, E. A., Han, E. S., Lalley, P. A., et al. (1992). Assignment of tyrosine-specific t-cell phosphatase to conserved syntenic groups on human chromosome 18 and mouse chromosome 18. Genomics 12, 151–154. doi: 10.1016/0888-7543(92)90418-r
Saltiel, A. R., and Kahn, C. R. (2001). Insulin signalling and the regulation of glucose and lipid metabolism. Nature 414, 799–806. doi: 10.1038/414799a
Santin, I., Moore, F., Colli, M. L., Gurzov, E. N., Marselli, L., Marchetti, P., et al. (2011). Ptpn2, a candidate gene for type 1 diabetes, modulates pancreatic β-cell apoptosis via regulation of the bh3-only protein bim. Diabetes 60, 3279–3288. doi: 10.2337/db11-0758
Santoro, A., Campolo, M., Liu, C., Sesaki, H., Meli, R., Liu, Z. W., et al. (2017). Drp1 suppresses leptin and glucose sensing of pomc neurons. Cell Metab. 25, 647–660. doi: 10.1016/j.cmet.2017.01.003
Scharl, M., Hruz, P., and McCole, D. F. (2010a). Protein tyrosine phosphatase non-receptor type 2 regulates ifn-gamma-induced cytokine signaling in thp-1 monocytes. Inflamm. Bowel Dis. 16, 2055–2064. doi: 10.1002/ibd.21325
Scharl, M., Rudenko, I., and McCole, D. F. (2010b). Loss of protein tyrosine phosphatase n2 potentiates epidermal growth factor suppression of intestinal epithelial chloride secretion. Am. J. Physiol. Gastrointest. Liver Physiol. 299, G935–G945. doi: 10.1152/ajpgi.00106.2010
Sharp, R. C., Abdulrahim, M., Naser, E. S., and Naser, S. A. (2015). Genetic variations of ptpn2 and ptpn22: role in the pathogenesis of type 1 diabetes and crohn’s disease. Front. Cell Infect. Microbiol. 5:95. doi: 10.3389/fcimb.2015.00095
Shields, B. J., Wiede, F., Gurzov, E. N., Wee, K., Hauser, C., Zhu, H. J., et al. (2013). Tcptp regulates sfk and stat3 signaling and is lost in triple-negative breast cancers. Mol. Cell. Biol. 33, 557–570. doi: 10.1128/mcb.01016-12
Simoncic, P. D., Bourdeau, A., Lee-Loy, A., Rohrschneider, L. R., Tremblay, M. L., Stanley, E. R., et al. (2006). T-cell protein tyrosine phosphatase (tcptp) is a negative regulator of colony-stimulating factor 1 signaling and macrophage differentiation. Mol. Cell. Biol. 26, 4149–4160. doi: 10.1128/MCB.01932-05
Simoncic, P. D., Lee-Loy, A., Barber, D. L., Tremblay, M. L., and McGlade, C. J. (2002). The t cell protein tyrosine phosphatase is a negative regulator of janus family kinases 1 and 3. Curr. Biol. 12, 446–453. doi: 10.1016/s0960-9822(02)00697-8
Spalinger, M. R., Kasper, S., Chassard, C., Raselli, T., Frey-Wagner, I., Gottier, C., et al. (2015). Ptpn2 controls differentiation of cd4(+) t cells and limits intestinal inflammation and intestinal dysbiosis. Mucosal Immunol. 8, 918–929. doi: 10.1038/mi.2014.122
Spalinger, M. R., Manzini, R., Hering, L., Riggs, J. B., Gottier, C., Lang, S., et al. (2018). Ptpn2 regulates inflammasome activation and controls onset of intestinal inflammation and colon cancer. Cell Rep. 22, 1835–1848. doi: 10.1016/j.celrep.2018.01.052
Spalinger, M. R., Sayoc-Becerra, A., Santos, A. N., Shawki, A., Canale, V., Krishnan, M., et al. (2020). Ptpn2 regulates interactions between macrophages and intestinal epithelial cells to promote intestinal barrier function. Gastroenterology 159, 1763–1777. doi: 10.1053/j.gastro.2020.07.004
Stanley, E. R., and Chitu, V. (2014). Csf-1 receptor signaling in myeloid cells. Cold Spring Harb. Perspect. Biol. 6:a021857. doi: 10.1101/cshperspect.a021857
Stuible, M., Doody, K. M., and Tremblay, M. L. (2008). Ptp1b and tc-ptp: regulators of transformation and tumorigenesis. Cancer Metastasis Rev. 27, 215–230. doi: 10.1007/s10555-008-9115-1
ten Hoeve, J., de Jesus Ibarra-Sanchez, M., Fu, Y., Zhu, W., Tremblay, M., David, M., et al. (2002). Identification of a nuclear stat1 protein tyrosine phosphatase. Mol. Cell. Biol. 22, 5662–5668. doi: 10.1128/mcb.22.16.5662-5668.2002
Tiganis, T. (2013). Ptp1b and tcptp–nonredundant phosphatases in insulin signaling and glucose homeostasis. FEBS J. 280, 445–458. doi: 10.1111/j.1742-4658.2012.08563.x
Tiganis, T., Bennett, A. M., Ravichandran, K. S., and Tonks, N. K. (1998). Epidermal growth factor receptor and the adaptor protein p52shc are specific substrates of t-cell protein tyrosine phosphatase. Mol. Cell. Biol. 18, 1622–1634. doi: 10.1128/mcb.18.3.1622
Tiganis, T., Kemp, B. E., and Tonks, N. K. (1999). The protein-tyrosine phosphatase tcptp regulates epidermal growth factor receptor-mediated and phosphatidylinositol 3-kinase-dependent signaling. J. Biol. Chem. 274, 27768–27775. doi: 10.1074/jbc.274.39.27768
Tokarz, V. L., MacDonald, P. E., and Klip, A. (2018). The cell biology of systemic insulin function. J. Cell Biol. 217, 2273–2289. doi: 10.1083/jcb.201802095
Twohig, J. P., Cardus Figueras, A., Andrews, R., Wiede, F., Cossins, B. C., Derrac Soria, A., et al. (2019). Activation of naïve cd4+ t cells re-tunes stat1 signaling to deliver unique cytokine responses in memory cd4+ t cells. Nat. Immunol. 20, 458–470. doi: 10.1038/s41590-019-0350-0
Veenstra, C., Karlsson, E., Mirwani, S. M., Nordenskjöld, B., Fornander, T., Pérez-Tenorio, G., et al. (2019). The effects of ptpn2 loss on cell signalling and clinical outcome in relation to breast cancer subtype. J. Cancer Res. Clin. Oncol. 145, 1845–1856. doi: 10.1007/s00432-019-02918-y
Wei, W., Ehlerding, E. B., Lan, X., Luo, Q. Y., and Cai, W. (2019). Molecular imaging of β-cells: diabetes and beyond. Adv. Drug Deliv. Rev. 139, 16–31. doi: 10.1016/j.addr.2018.06.022
Wiede, F., Brodnicki, T. C., Goh, P. K., Leong, Y. A., Jones, G. W., Yu, D., et al. (2019). T-cell-specific ptpn2 deficiency in nod mice accelerates the development of type 1 diabetes and autoimmune comorbidities. Diabetes 68, 1251–1266. doi: 10.2337/db18-1362
Wiede, F., Lu, K.-H., Du, X., Liang, S., Hochheiser, K., Dodd, G. T., et al. (2020). Ptpn2 phosphatase deletion in t cells promotes anti-tumour immunity and car t-cell efficacy in solid tumours. EMBO J. 39:e103637. doi: 10.15252/embj.2019103637
Wiede, F., Shields, B. J., Chew, S. H., Kyparissoudis, K., van Vliet, C., Galic, S., et al. (2011). T cell protein tyrosine phosphatase attenuates t cell signaling to maintain tolerance in mice. J. Clin. Invest. 121, 4758–4774. doi: 10.1172/JCI59492
Xi, Y., Liu, S., Bettaieb, A., Matsuo, K., Matsuo, I., Hosein, E., et al. (2015). Pancreatic t cell protein-tyrosine phosphatase deficiency affects beta cell function in mice. Diabetologia 58, 122–131. doi: 10.1007/s00125-014-3413-7
Yamamoto, T., Sekine, Y., Kashima, K., Kubota, A., Sato, N., Aoki, N., et al. (2002). The nuclear isoform of protein-tyrosine phosphatase tc-ptp regulates interleukin-6-mediated signaling pathway through stat3 dephosphorylation. Biochem. Biophys. Res. Commun. 297, 811–817. doi: 10.1016/s0006-291x(02)02291-x
You-Ten, K. E., Muise, E. S., Itié, A., Michaliszyn, E., Wagner, J., Jothy, S., et al. (1997). Impaired bone marrow microenvironment and immune function in t cell protein tyrosine phosphatase-deficient mice. J. Exp. Med. 186, 683–693. doi: 10.1084/jem.186.5.683
Yu, C. L., Jin, Y. J., and Burakoff, S. J. (2000). Cytosolic tyrosine dephosphorylation of stat5. Potential role of shp-2 in stat5 regulation. J. Biol. Chem. 275, 599–604. doi: 10.1074/jbc.275.1.599
Zee, T., Settembre, C., Levine, R. L., and Karsenty, G. (2012). T-cell protein tyrosine phosphatase regulates bone resorption and whole-body insulin sensitivity through its expression in osteoblasts. Mol. Cell. Biol. 32, 1080–1088. doi: 10.1128/MCB.06279-11
Zhang, D., Jiang, Y., Song, D., Zhu, Z., Zhou, C., Dai, L., et al. (2019). Tyrosine-protein phosphatase non-receptor type 2 inhibits alveolar bone resorption in diabetic periodontitis via dephosphorylating csf1 receptor. J. Cell. Mol. Med. 23, 6690–6699. doi: 10.1111/jcmm.14545
Zhang, P., Zhang, W., Zhang, D., Wang, M., Aprecio, R., Ji, N., et al. (2018). 25-hydroxyvitamin d3 -enhanced ptpn2 positively regulates periodontal inflammation through the jak/stat pathway in human oral keratinocytes and a mouse model of type 2 diabetes mellitus. J. Periodontal Res. 53, 467–477. doi: 10.1111/jre.12535
Zhang, S., Chen, L., Luo, Y., Gunawan, A., Lawrence, D. S., and Zhang, Z. Y. (2009). Acquisition of a potent and selective tc-ptp inhibitor via a stepwise fluorophore-tagged combinatorial synthesis and screening strategy. J. Am. Chem. Soc. 131, 13072–13079. doi: 10.1021/ja903733z
Zhang, Y., Ding, H., Wang, X., and Ye, S. D. (2018). Modulation of stat3 phosphorylation by ptpn2 inhibits naive pluripotency of embryonic stem cells. FEBS Lett. 592, 2227–2237. doi: 10.1002/1873-3468.13112
Keywords: T cell protein tyrosine phosphatase, protein tyrosine phosphatase non-receptor 2, glucose metabolism, insulin signaling pathway, leptin signaling pathway
Citation: Wang Y-n, Liu S, Jia T, Feng Y, Xu X and Zhang D (2021) T Cell Protein Tyrosine Phosphatase in Glucose Metabolism. Front. Cell Dev. Biol. 9:682947. doi: 10.3389/fcell.2021.682947
Received: 19 March 2021; Accepted: 09 June 2021;
Published: 29 June 2021.
Edited by:
Andrea Graziani, University of Turin, ItalyReviewed by:
Alex Von Kriegsheim, University of Edinburgh, United KingdomCopyright © 2021 Wang, Liu, Jia, Feng, Xu and Zhang. This is an open-access article distributed under the terms of the Creative Commons Attribution License (CC BY). The use, distribution or reproduction in other forums is permitted, provided the original author(s) and the copyright owner(s) are credited and that the original publication in this journal is cited, in accordance with accepted academic practice. No use, distribution or reproduction is permitted which does not comply with these terms.
*Correspondence: Dongjiao Zhang, ZGp6aGFuZzExMDlAMTYzLmNvbQ==
†These authors have contributed equally to this work and share first authorship
Disclaimer: All claims expressed in this article are solely those of the authors and do not necessarily represent those of their affiliated organizations, or those of the publisher, the editors and the reviewers. Any product that may be evaluated in this article or claim that may be made by its manufacturer is not guaranteed or endorsed by the publisher.
Research integrity at Frontiers
Learn more about the work of our research integrity team to safeguard the quality of each article we publish.