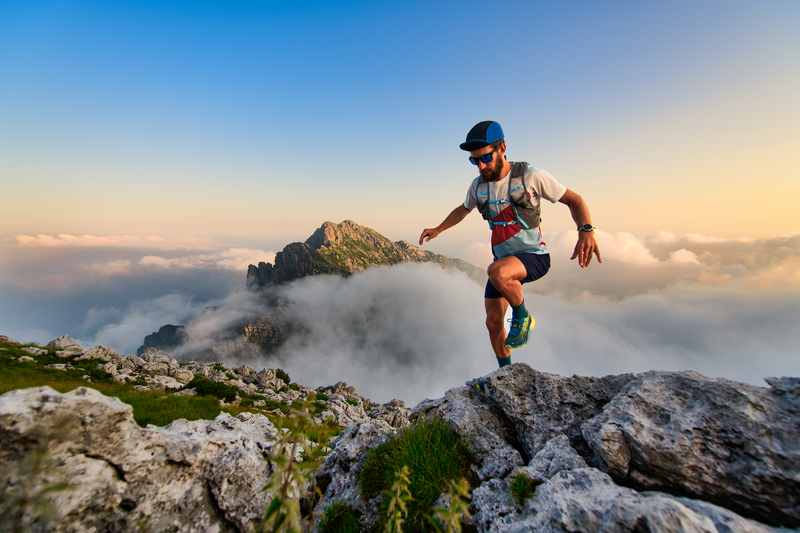
95% of researchers rate our articles as excellent or good
Learn more about the work of our research integrity team to safeguard the quality of each article we publish.
Find out more
REVIEW article
Front. Cell Dev. Biol. , 29 April 2021
Sec. Signaling
Volume 9 - 2021 | https://doi.org/10.3389/fcell.2021.674199
This article is part of the Research Topic Morphogenic Cascades underlying Regeneration and Plasticity after Nervous System Injury View all 8 articles
Tenascin C (TnC) is a glycoprotein highly expressed in the extracellular matrix (ECM) during development and in the adult central nervous system (CNS) in regions of active neurogenesis, where neuron development is a tightly regulated process orchestrated by extracellular matrix components. In addition, newborn cells also communicate with glial cells, astrocytes and microglia, indicating the importance of signal integration in adult neurogenesis. Although TnC has been recognized as an important molecule in the regulation of cell proliferation and migration, complete regulatory pathways still need to be elucidated. In this review we discuss the formation of new neurons in the adult hippocampus and the olfactory system with specific reference to TnC and its regulating functions in this process. Better understanding of the ECM signaling in the niche of the CNS will have significant implications for regenerative therapies.
In contrast to the main form of neural plasticity inferring modifications at the synaptic level, adult neurogenesis confers plasticity through the addition of a population of newly developed neurons with functional synaptic inputs and outputs. Production of new neurons continues throughout life in most invertebrate and vertebrate species even after fetal and early postnatal development has ceased (Cayre et al., 2002). In the adult human brain neurogenesis remains a controversial area of research with evidence both for and against a significant rate of production of new neurons (Eriksson et al., 1998; Spalding et al., 2013; Sorrells et al., 2018). The fact that it is evolutionarily conserved implies that adult neurogenesis is of great functional importance. Nevertheless, adult neurogenesis is restricted to the dentate gyrus (DG) of the hippocampus and the subventricular zone (SVZ) around lateral ventricles (LV) (Altman and Das, 1965; Altman, 1969). Arguably, adult neurogenesis may also happen in other regions (Bernier et al., 2002; Zhao et al., 2003). However, the two established neurogenic niches, the SVZ and the hippocampus, are residential areas for neural stem cells (NSC) where tightly regulated processes of neuronal development are orchestrated by extracellular matrix (ECM) components. In addition, newborn cells also communicate with glia, underlining signal integration in adult neurogenesis.
Tenascin C (TnC) is a member of the tenascin family, comprised of glycoproteins highly expressed in the ECM during development and in the adult central nervous system (CNS) in regions of active neurogenesis (Bartsch et al., 1992; Gates et al., 1995; Ferhat et al., 1996). The term tenascin is derived from the Latin words tenere—to hold, and nasci—to be born, referring to the presence of tenascins in fetal tissue (Chiquet-Ehrismann et al., 1986). Nowadays, it is known that TnC is involved in morphogenic changes during development and tissue remodeling, as well as in cell adhesion and signaling between cells (Jones and Jones, 2000).
In order to understand the functional significance of adult neurogenesis, we need to elucidate the circumstances that modulate the process of addition of newborn neurons into the brain. Most of the research is focused on neurons, while the influence of non-neuronal cells and the ECM are less considered although they both provide stimuli that lead the processes of neurogenesis. Growing body of research points to the importance of glial cells and the ECM in the understanding of cellular plasticity. Thus, in this review we discuss the formation of new neurons in the adult hippocampus and the olfactory system in rodent animal models with specific reference to TnC and its regulating functions in this process. Although direct evidence regarding the role of TnC in processes of adult neurogenesis is lacking, its functions found in other contexts could be extrapolated to adult neurogenesis as potentially important.
New neurons are generated from NSC, a population of self-renewing, multipotent cells. Through the process of self-renewal, a constant pool of dividing cells can be maintained, and the potential of NSC to differentiate into diverse cell types allows for the creation of new neurons and astrocytes. Under physiological conditions NSC can be found in the SVZ surrounding the LV, from where newborn cells migrate to the olfactory bulb (OB), and in the subgranular zone (SGZ) of the DG, where new cells become functionally integrated into existing neuronal circuits. The processes of proliferation, migration and differentiation in the two niches have different spatial and temporal organizations, while the addition of new synapses in an activity dependent manner is under control of neural activity (Ma et al., 2009).
Adult hippocampal neurogenesis is found to be confined to the DG. Namely, local radial glial stem cells in the SGZ undergo proliferation and through asymmetric division give rise to neurons or astrocytes (Kriegstein and Alvarez-Buylla, 2009). The daughter cells originating from the radial glial stem cells are highly proliferative amplifying stem cells, which in turn generates neuroblasts (Gonçalves et al., 2016). New immature neurons show limited migration from the SGZ to the granular cell layer (GCL). Four weeks after proliferation, they begin to express markers of mature neurons (Kempermann et al., 2003). Most of the newborn neurons in the hippocampus differentiate into excitatory granular cells, although there is also a detectable number of newborn interneurons (Liu et al., 2003). After the maturation phase, newborn neurons are indistinguishable from the neighboring mature neurons when characterized by electrical and synaptic properties (Ge et al., 2007).
In contrast to the events during adult hippocampal neurogenesis, newborn cells in the SVZ migrate a long distance to the OB (Alvarez-Buylla and Garcìa-Verdugo, 2002). After proliferation at the stage of neuroblasts, residing cells follow the chain of migration along the rostral migratory stream (RMS) generated by specialized astrocytes. Upon reaching the middle of the OB, cells in the stage of immature neurons detach from the chain and continue to perform radial migration. This is followed by differentiation into two types of interneurons. Most of them differentiate into GABAergic granular neurons, while the rest become periglomerular neurons expressing GABA and/or dopamine as neurotransmitters. Complete integration of newborn neurons into existing circuits requires the establishment of both synaptic inputs and outputs, in order for them to be able to receive and respond to olfactory stimuli (Bonzano et al., 2016).
Matricellular proteins are a group of proteins that are secreted into the ECM but do not have a primary role in the maintenance of its structure (Bornstein, 2009). The term was introduced to explain the diversity of functions of proteins such as TnC. TnC is a glycoprotein present in the ECM as a regulatory protein with different spatial and temporal expression patterns during life. In fact, it is widespread during development, while its expression decreases during life, and remains restricted to plastic areas of active neurogenesis (Gates et al., 1995; Ferhat et al., 1996). In addition, TnC was found to be expressed de novo in lesions and during wound healing (Roll and Faissner, 2019), but also in pathological conditions, such as inflammation, cancer and trauma (Chiquet-Ehrismann and Chiquet, 2003; Midwood et al., 2011). During CNS development TnC is expressed by radial glial cells (Götz et al., 1997), while in the adult CNS, TnC is produced by astrocytes in the SVZ (Kazanis et al., 2007) and granule cells in the hippocampus (Niquet et al., 1995).
In development, disease and regeneration the TnC gene is regulated through cytokines, growth factors and mechanical stimuli (Chiovaro et al., 2015). The expression of TnC during embryogenesis is often associated with morphogenetic changes by mechanisms of segmentation and gene patterning. A number of pro- and anti-inflammatory cytokines has been shown to induce expression of TnC in different cell types, although so far there were no related functional studies. Control of TnC promoter activity is also achieved through upstream regulation by the platelet-derived growth factor and the transforming growth factor-β. Transduction of external mechanical stimuli results in increased TnC expression by way of the activation of the Rho/ROCK signaling cascade that activates the TnC promoter. TnC becomes a functional protein after post-transcriptional and post-translational modifications, such as gene splicing and protein glycosylation (Giblin and Midwood, 2015). Export and incorporation of the suitably modified protein into the ECM is dependent on the ECM composition, while the expression of TnC in different tissues is regulated through proteolytic degradation.
TnC is a hexamer, each monomer consisting of the tenascin assembly (TA) domains at the N-terminus, responsible for the assembly of oligomeric proteins, epidermal growth factor-like (EGFL) repeats, fibronectin type III (FN III) domains, and a globular fibrinogen domain at the C-terminus (Figure 1A). Alternative splicing within the FN III domains allows for different isoforms of TnC. Multidomain structure of TnC allows for a variety of interactions with components of the ECM and different types of cells (Midwood et al., 2016). It was first recognized as a molecule that participates in cell adhesion, while later studies suggested that it regulates cell signaling, migration and differentiation (Chiquet-Ehrismann et al., 1986). TnC is engaged in the regulation of cell migration since it is able to perform both adhesive and anti-adhesive interactions (Figure 1B; Faissner and Kruse, 1990; Lochter and Schachner, 1993). TnC is able to influence cell behavior through direct interactions with cell surface receptors, including integrins (Schnapp et al., 1995; Yokosaki et al., 1998), but also with ECM components, fibronectin and chondroitin-sulfate proteoglycans, such as phosphacan (Figure 2; Milev et al., 1997; Jones and Jones, 2000).
Figure 1. The structure of human tenascin C and its involvement in different regulatory pathways. (A) Schematic representation of a TnC monomer composed of N-terminal tenascin assembly (TA) domain, epidermal growth factor-like (EGFL) repeats, two types of fibronectin III (FN III) domains, constitutively expressed domains in TnC variants and alternatively spliced domains, and the C-terminal fibrinogen globe. (B) TnC as a functional hexamer participates in processes of cell proliferation, adhesion, migration, differentiation, cell survival and apoptosis, synaptic functions and in the inflammatory response.
Figure 2. Schematic representation of TnC interactions with cell surface receptors and extracellular matrix components. Signaling from epidermal growth factor (EGF), integrins, Toll-like receptor 4 (TLR4) and Wnt to intracellular effectors such as focal adhesion kinase (FAK), mitogen-activated protein kinase (MAPK), phospholipase C (PLC), leads to changes in gene transcription, resulting in changes of expression of proteins involved in proliferation, adhesion, cell survival/apoptosis, synaptic activity and immune response. TnC performs interactions with components of ECM collagen, fibronectin and chondroitin-sulfate proteoglycans, such as phosphacan, a short form of receptor protein tyrosin phosphatase β.
Although TnC is a crucial regulatory molecule during development and organogenesis, TnC deficient mice are able to develop normally (Saga et al., 1992). However, after morphological and electrophysiological changes in TnC deficient mice have been documented (Evers et al., 2002; Irintchev et al., 2005), it has been demonstrated that TnC deficiency leads to behavioral abnormalities as well (Morellini and Schachner, 2006). Moreover, a detailed study confirmed these results and suggested how TnC interacts with the environment in shaping the behavioral phenotype (Stamenkovic et al., 2017a).
The stem cell niche is a microenvironment where stem cells reside and receive signals that determine their fate. A myriad of signals affects stem cell behavior, including signals from the neighboring cells and the ECM, making cell-to-cell and cell-to-matrix interactions determine whether the cells stay in quiescent state or begin to proliferate. In the SGZ, the thin lamina between the hippocampal hilus and the granular cell layer, proliferating cells are found in clusters around small capillaries (Palmer et al., 2000). Proliferating cells can be labeled with bromodeoxyuridine (BrdU), a synthetic analog of thymidine which incorporates into the DNA during cell division. Cells positive for BrdU, also expressing endothelial markers, were found in this anatomical niche, suggesting that adult neurogenesis actually occurs within the angiogenic niche. In addition, in the SVZ, proliferating cells are located next to blood vessels, while quiescent cells are located adjacent to the epidermal layer lining the ventricles (Tavazoie et al., 2008; Morante-Redolat and Porlan, 2019).
In the adult murine brain along NSC, supporting cells and ECM components, the microenvironment of the neurogenic niche also contains specific proteins, such as TnC and thrombospondin (Garcion et al., 2001; Kazanis et al., 2007; Faissner et al., 2017). As a prominent component of the stem cell niche, TnC guides the processes of NSC development (Chiquet-Ehrismann et al., 2014). NSC have been identified as the main source of their local ECM, since single-cell RNA sequencing of quiescent NSC in the SVZ showed that these cells are rich in mRNA encoding for matrix proteins, while activated NSC or neuroblasts express very few ECM components (Kjell et al., 2020). In addition, proteome analysis showed an enrichment of TnC in the OB and SVZ, compared to the medial side of the lateral ventricle. Assessing the OB, an increase in stiffness was found from the SVZ and RMS toward the OB, implying that cell migration depends on tissue mechanobiology, as it was already known that biochemical and biophysical properties of NSC niche shape adult neurogenesis. Microelastic mapping of the rat DG has shown that ECM in the SGZ, where the population of progenitor cells reside, and the hilus, through which new axons extend to the CA3 region, have lower stiffness than the GCL, wherein differentiated cells migrate (Luque et al., 2016). This was in correspondence with earlier in vitro studies, where it was shown that stiffness regulates NSC differentiation. More precisely, soft ECM promotes NSC differentiation, while stiff ECM suppresses it (Saha et al., 2008). In addition, neurite outgrowth of the differentiating NSC is dependent on the stiffness of the growth medium (Stukel and Willits, 2018). Although it is clear that tissue stiffness correlates with cell density (Koser et al., 2015), niche-specific mechanical properties in both neurogenic areas can also be considered as a consequence of the presence of specific components of the proteome. Nevertheless, more detailed studies concerning the involvement of TnC in the mechanical properties of neurogenic niches could provide valuable information, considering its role in mechanotransduction and control of neurite outgrowth (Chiquet et al., 2007; Andrews et al., 2009).
Cell surface proteins, such as stretch-sensitive ion channels and integrins are able to sense mechanical forces and convert them into biochemical signals. FN III domains of TnC interact with different integrins (Tucker and Chiquet-Ehrismann, 2015) causing a rapid cytoskeleton response to external forces by Rho-dependent actin assembly and contraction (Asparuhova et al., 2009). As a consequence, a change in cell-matrix adhesion changes cell shape and orientation. In this way, the cell can continuously modify its response to external stimuli. Moreover, TnC expression can be induced by mechanical stress such as stretch in fibroblasts (Chiquet et al., 2007). The extensibility of the FN III domains (Oberhauser et al., 1998) imposes elastic properties to the TnC hexamer, directly contributing to tissue elasticity and protection against mechanical stress.
Since mechanical characteristics of the ECM, such as the grade of stiffness and elasticity, are involved in stem cell differentiation by means of affecting lineage commitment, this might be particularly important after tissue injury, where subsequent scar formation might negatively affect the ability of stem cells for repair.
When NSC receive the proper signal, they leave the quiescent state and begin to proliferate. Many extrinsic and intrinsic factors are involved, and TnC has been recognized as an important molecule in the regulation of NSC proliferation, which was first demonstrated in a study by Garcion and coworkers (Garcion et al., 2001). They showed that TnC knockout mice have reduced rates of proliferating cells in the SVZ, suggesting a supportive role of TnC in NSC proliferation. Another role for TnC was proposed by Garwood et al., who suggested the participation of TnC in differentiation of oligodendrocyte precursor cells (Garwood et al., 2004). They noticed a more rapid differentiation of cultured oligodendrocyte precursor cells in the absence of TnC. These two roles could be accomplished through the interaction with Sam68, a protein involved in the Src-like kinase apoptotic pathway. Furthermore, another study by Garcion and coworkers showed that TnC controls the fibroblast growth factor and epidermal growth factor responsiveness and differentiation properties of NSC (Garcion et al., 2004). This study confirmed the inhibitory effect of TnC on neurogenesis, and suggested that TnC deficient neurospheres had higher number of NSC that differentiated into neurons or glia. Moreover, another study showed an increased proliferation of astroglial progenitors in the spinal cord of TnC knockout mice during the embryonic period (Karus et al., 2011). On the other hand, mesenchymal stem cells are able to transdifferentiate into neural linages under specific conditions, and when grown on surface coated by TnC and tenascin R, they differentiate into neurons or oligodendrocytes (Tsai et al., 2014).
Intriguingly, in spite of the importance of TnC during embryonic and early postnatal NSC and neural progenitor proliferation and migration, it was demonstrated that TnC deficiency does not affect the number of NSC nor their progeny, neuroblasts, and periglomerular interneurons, in adult mice (Kazanis et al., 2007). Nevertheless, mice with TnC deficiency showed morphological changes around the LV that were reflected in an increased number of neuroblast clusters along the LV. The authors suggested a network of compensatory ECM molecules in the adult brain that are able to partially compensate for the loss of TnC.
Although some evidence about the role of TnC in cell proliferation is contradictory, there are a few regulatory mechanisms of proliferation involving TnC (Figure 2). EGFL repeats of TnC directly interact with the EGF receptor, activating the signaling pathways of phospholipase C, Ras/mitogen activated protein kinase (MAPK) and phosphatidylinositol 3-kinase (PI3)/Akt (Faissner et al., 2017; Hanmin et al., 2020). Proteomics-based research has also shown that TnC can be regulated by the modification of Human Natural Killer-1 (CD57) cells in NSC (Yagi et al., 2010). Namely, post-translational modification of CD57 stimulates TnC-dependent proliferation of NSC via the MAPK pathway. On the other hand, it has been shown that Sam68 is downregulated in cultured NSC in the presence of TnC, while increased expression of this splicing regulator causes a decrease in the proliferation of glial precursors (Moritz et al., 2008). A later study has shown that TnC interacts with lipid rafts in the oligodendrocyte membrane which leads to the inhibition of differentiation of these cells in culture (Czopka et al., 2010). In fact, TnC interferes with phosphorylation of Akt which in turn reduces expression of myelin basic protein and Sam68 in a PI3 kinase-dependent pathway. Also, there are indications that TnC regulates intracellular pathways via modulation of GTPase RhoA and focal adhesion kinase, leading to downstream modification of actin cytoskeleton (Midwood and Schwarzbauer, 2002). TnC disrupts intracellular localization of Rho, disabling its recruitment to the cell membrane. This event has the potential to evoke downstream modification of actin cytoskeleton that ends in the modifications of cell adhesion, contractility, cell cycle progression and gene transcription (Hall, 1998).
Programmed cell death is considered to be a regulatory mechanism for maintaining the rate of experience-dependent adult neurogenesis. A decrease in BrdU-labeled cells in the adult SGZ has been reported, particularly in the first week of their life (Kempermann et al., 2004). The decrease in the number of proliferating BrdU-immunopositive cells is due to the programmed cell death by apoptosis (Biebl et al., 2000). This was later confirmed by Sierra et al. who showed that the majority of the amplifying progenitors undergo cell death by apoptosis during transition to neuroblasts (Sierra et al., 2010). These events take place in the first 4 days of their life and account for most of the decrease in neurogenic rate, this being considered as the main critical period of survival of newborn cells in the adult SGZ. Apoptosis is coupled to microglial phagocytosis, where newborn cells are engulfed by unchallenged microglia (Figure 3), with ramified morphology and low expression of typical inflammatory markers, such as CD11 and CD68, suggesting a non-inflammatory activation pathway. This was supported by the results on microglial transcriptomes that revealed the presence of non-inflammatory mediators in microglia in the developing and adult mouse brain (Bennett et al., 2016). Moreover, phagocytosis is not merely a passive removal of intracellular contents, but it also has an active role in maintaining neurogenesis. Microglia, through its secretome, provides a negative feedback loop, acting as a sensor for local cell death, modulating the balance between proliferation and survival of newborn cells. Thus, Diaz-Apacio et al. used a monolayer of neural progenitor cells derived from neurospheres to model neurogenesis, and allowed them to differentiate in the presence of conditioned medium from control and phagocytic microglia (Diaz-Aparicio et al., 2020). The results showed that the secretome of phagocytic microglia limits neurogenesis through the reduction in the production of neuronal-committed cells. To confirm these results, the hypothesis has been tested in vivo, following the injection of microglia conditioned medium in adult mice. The authors concluded that adult neurogenesis in the hippocampus is under a negative feedback loop provided by microglia. In contrast, a direct role of microglia in the regulation of newly generated neurons in the OB has not been reported.
Figure 3. Schematic representation of glial contribution to the regulation of adult neurogenesis. Astrocytes and microglia secrete growth factors and cytokines that guide the processes of neurogenesis. Microglia participates in the regulation of the neurogenic rate by engulfing apoptotic cells.
A wide range of microglial functions have been shown to be under TnC regulation during early postnatal development, among them cytokine/chemokine secretion, iNOS and COX2 expression, as well as chemotaxis and phagocytosis (Haage et al., 2019). The same study suggested that TnC controls microglial phagocytic activity via histone deacetylase, a protein that has already been linked to microglial functions. Involvement of TnC in microglial functions in CNS disorders has shown that TnC deficient mice have reduced microglial surveillance in the ischemic brain (Manrique-Castano et al., 2020).
In the adult brain, microglia is mostly studied for its role in the immune response, but there have been indications that microglia is involved in adult neurogenesis in a regulatory way, that includes some components of the inflammatory pathway. Brain inflammation has been shown to be detrimental for neurogenesis since activated microglia has a neurotoxic effect on newborn cells through release of IL-1, IL-6, nitric-oxide and reactive oxygen species (Valliéres et al., 2002). A negative correlation has been reported between the number of activated microglia in the rat hippocampus with the number of surviving new neurons (Figure 3; Ekdahl et al., 2003). In addition, the application of a selective inhibitor of microglial activation, minocycline, led to the restoration of hippocampal neurogenesis. There was no evidence that microglia suppresses cell proliferation or neuronal differentiation neither in basal nor in insult-induced neurogenesis.
Expression of TnC in areas of inflammation and tissue damage in inflamed rheumatoid joints suggests its involvement in inflammatory processes. It was shown on mice in vivo that TnC mediates joint inflammation by helping to maintain inflammation, although it does not seem to be involved in its initiation (Midwood et al., 2009). The same study showed that TnC induces synthesis of proinflammatory cytokines in human macrophages by the activation of Toll-like receptor 4 (TLR4). A later study that used experimental sepsis in vivo as an inflammatory model, also reported that TnC mediates synthesis of proinflammatory cytokines in the TLR4 pathway through post-transcriptional control on the micro-RNA level (Piccinini and Midwood, 2012). Mapping of the binding site of TnC showed that this molecule has direct and cooperative interaction with TLR4, but the same binding site was identified as a conserved inflammatory epitope in related proteins (Zuliani-Alvarez et al., 2017), thus confirming that TnC is a part of the TLR4 signaling cascade.
After proliferation and survival, newborn cells at the stage of neuroblasts migrate to their final destinations. Besides the fact that astrocytes secrete growth factors and cytokines (Figure 3), those within the SVZ, RMS and OB express TnC throughout postnatal life, while migrating neuroblasts in the early postnatal subepidermal zone are also enclosed by a TnC-rich layer (Gates et al., 1995; Peretto et al., 2005). This suggests that TnC may play a regulatory role in tangential migration of neuroblasts, which could be attributed to its ability to form repellent interactions with other cell types (Husmann et al., 1995). Nevertheless, when this hypothesis was tested in vivo on TnC deficient mice the chain organization of the migratory pathway was unrelated to TnC deficiency (de Chevigny et al., 2006). In addition, in the SVZ explants culture there was no detectable difference in the rate of migratory neuroblasts between wild-type and TnC deficient mice.
In the SVZ of both postnatal and adult mice neuroblasts interact and modify the surrounding ECM by expressing matrix metalloproteinases (MMP), proteolytic enzymes that use components of ECM as substrates (Bovetti et al., 2007). TnC and other ECM components have been shown to influence neuronal migration in other brain regions through the proteolytic action of MMP (Murase and Horwitz, 2002). It has been reported that TnC is involved in cell migration through the activation of c-Jun N-terminal kinase, a member of the MAPK family, which further on modulates the expression and activity of MMP-9 (Cai et al., 2017). Another study confirmed the involvement of TnC in Wnt signaling (Figure 2), responsible for maintaining the stem cell pool and suppressing aberrant differentiation (Hendaoui et al., 2014).
In vitro migratory assays showed that TnC inhibits the migration of oligodendrocyte progenitor cells by inhibiting Wnt signaling (Kakinuma et al., 2004), which is in congruence with previous in vivo research evaluating the migratory activity of oligodendrocyte precursors in TnC deficient mice (Garcion et al., 2001). On the other hand, another study showed that TnC acts in a dose dependent way to promote the migration of bone marrow stem cells (BMSC) (Ding et al., 2018). Namely, when different concentrations of TnC are added to the medium where murine stem cells are seeded, high concentrations of TnC promote cellular migration. Additionally, it has been suggested that TnC can bind to TLR4 expressed on BMSC, and activate downstream signaling through MAPK, protein kinase B and Wnt signaling pathway. However, further studies are needed to unravel the role of TnC in cellular migration in vivo.
A critical factor for the selection process during the maturation of newborn neurons might be the rate at which newborn neurons are synaptically recruited, resulting in a better chance to survive. The functional importance of newborn neurons relies on their physiological properties. Namely, high excitability of newborn neurons when compared to mature cells, allows them to be more sensitive to synaptic plasticity.
Regulation of synaptic integration of adult-born neurons is shaped by actions of microglia through synaptogenesis and pruning, modulation of perisynaptic structures, and regulation of spine structure and synaptic transmission (Ekdahl, 2012). Microglia depletion in adult mice results in fewer spines with mushroom morphology, leading to overall reduced spine volume, and weaker excitatory synapses in the OB (Wallace et al., 2020). Synaptic transmission may occur in the perisomatic environment undergoing inactivation, degradation or activation of the ECM through the action of MMP-9, a molecule already confirmed to be involved in migration and differentiation of adult neural progenitors in the SVZ (Barkho et al., 2008). Another mechanism of microglial regulation of neuronal activity through remodeling of dendritic spine morphology, including size, shape and motility (Tremblay and Majewska, 2011), results in alterations in actin filaments (Fortin et al., 2012).
In the adult hippocampus, glutamatergic synapses onto newborn cells are characterized by an increased long-term potentiation (LTP) with a decreased induction threshold (Lledo et al., 2006). This was confirmed with single cell recordings in the hippocampal slices of adult rats, where immature granule cells in the DG were found to have lower LTP threshold compared to more mature neurons (Wang et al., 2000). Adult-born olfactory interneurons undergo different synaptic modifications compared with pre-existing mature interneurons (Nissant et al., 2009). A transient expression of LTP, and post-tetanic potentiation, were found exclusively in adult-born granule cells, not in pre-existing ones, making newborn granule cells particularly sensitive to synaptic plasticity. Thus, potentiation of the synaptic strength onto granule cells could increase feedforward inhibition onto olfactory bulb interneuron outputs, promoting efficient coding of information (Rinberg and Gelperin, 2006). Therefore, in both neurogenic regions, the ability of newborn neurons to balance excitatory/inhibitory inputs and outputs is crucial for their integration in existing circuits.
TnC has a prominent role in synaptic functions (Strekalova et al., 2002) and modulation of cellular behavior, since it is involved in the induction of LTP, possibly through the modulation of L-type voltage-dependent Ca2+ channels (Evers et al., 2002). It has also been reported that TnC has a prominent role in fine tuning of the balance between excitation and inhibition (Stamenkovic et al., 2017b). Namely, it was shown in TnC-deficient mice that the exposure to enriched environment (EE) induces lower expression of excitatory markers and a higher density of inhibitory terminals as compared to wild-type mice. An in vitro study in a three-dimensional nanofiber gel with a TnC derived peptide, showed extensive neurite outgrowth and increased expression of neuronal marker when compared to two-dimensional cultures (Sever et al., 2018). It is thus, plausible to emphasize that TnC may play an indirect role in neuronal differentiation and synaptic integration.
The effects of tenascin C on the above cellular processes (depicted in sections “Cell Proliferation,” “Survival of Newborn Cells,” and “Functional Integration of New Neurons”) are summed up in Table 1.
Table 1. Effects of Tenascin C on processes of proliferation, survival, migration and differentiation.
Adult neurogenesis is a process considered as a type of cellular plasticity, an important mechanism for the regeneration of the CNS. The fact that the birth of new neurons in the adult rodent brain is conserved in the OB and the hippocampus, structures with high degree of structural and functional plasticity that are involved in storage and encoding of the sensory/memory information, qualifies these neurogenic brain areas for important roles in the physiological adaptation to new stimuli. A number of computational studies suggest theoretical roles of adult hippocampal neurogenesis, such as the increase of hippocampal memory capacity (Becker, 2005), reduction of interference between new and old memories, encoding of new memories (Wiskott et al., 2006), and the encoding of time in memories (Aimone et al., 2009). OB neurogenesis has not been as extensively studied computationally as hippocampal neurogenesis, but an in vivo study suggested that new neurons in their critical period of integration are required for the encoding of learning of odor related experience (Forest et al., 2019).
Impact of adult neurogenesis on cognitive functions is a matter of great complexity that needs to take a variety of factors into consideration, along with their interconnection and interplay. Moreover, all the functions of the hippocampus and the OB should be considered, hence a term of neurogenic niche has been introduced in order to explain the downstream influence of neurogenesis on behavior (Eisch and Petrik, 2012). This hypothesis could be supported by behavioral studies, that suggested a possible role of adult hippocampal neurogenesis in pattern separation (Clelland et al., 2009) and in memory consolidation (Kitamura et al., 2009). Moreover, manipulation of sensory input by odor deprivation or enrichment caused a decrease or increase, respectively, in survival of newborn interneurons (Bovetti et al., 2009). Another study that used odor enrichment as a paradigm, showed that treatment of mice with different fragrances resulted in an increased tyrosine-hydroxylase positive dopaminergic cell population, without changes in calretinin- and calbindin-positive neurons (Bonzano et al., 2014).
Dynamic regulation of different stages of adult neurogenesis in the hippocampus and the OB, including proliferation, survival, migration and integration, is under control of both intrinsic and extrinsic factors (Table 2). Environmental stimuli through exposure to an EE contributes to the survival of new granule neurons in the SGZ, without affecting the SVZ (Brown et al., 2003). Physical activity such as running, increases cell proliferation and survival of newborn neurons in the SGZ (Van Praag et al., 2005). On the other hand, enriched odor exposure increases proliferation in the SVZ, while the neurogenesis in the SGZ remains unaffected (Rochefort et al., 2002). Moreover, odor enrichment drives increased integration of newborn dopaminergic neurons (Bonzano et al., 2014).
Local environment, although providing signals for proper cellular development in physiological conditions, has a crucial role in keeping tissue homeostasis after injury or in pathological conditions. In the hippocampus of rats after focal cerebral ischemia an increased number of proliferating cells has been reported, but on the other hand, survival of newborn neurons has been reduced (Takasawa et al., 2002). In the experimental model of global cerebral ischemia, neurogenesis persists in the SVZ up to 1 year after the insult (Sekeljic et al., 2012). In fact, this study documented an increased number of proliferating cells and colocalization of markers of microglial activation and neurons. A proposed explanation delt with a beneficial role of microglia in chronic postischemic phase, supported by the findings of Ziv and colleagues, who suggested the microglia-T cell crosstalk to be a promoter for cell proliferation in the SVZ (Ziv et al., 2006). The same study has also put immune control over maintenance of adult neurogenesis in context of EE, suggesting that T cells are required for spatial learning and memory.
EE has been shown beneficial for many animal models of CNS disorders, such as Alzheimer’s and Parkinson’s disease, epilepsy or stroke (Young et al., 1999; Bezard et al., 2003; Jankowsky et al., 2005). Since NSC represent a constant source of cellular material for regenerative processes in the brain after injury, enhanced neurogenesis after exposure to EE could be additionally beneficial for disorders characterized by a reduced cell number.
It has been shown that aging has a detrimental effect on neurogenesis, with a decreasing rate of neurogenesis in later stages of life (Kuhn et al., 1996; Enwere et al., 2004). The process of aging does not only affect stem cells, but also their microenvironment. Namely, with the accumulation of stress-induced damage, such as induced by reactive oxygen species, local signaling pathways become dysregulated. Since decreased neurogenesis occurs in neurodegenerative disorders such as Alzheimer’s disease and depression (Rodríguez and Verkhratsky, 2011), while increased neurogenesis occurs in epilepsy (Jessberger and Parent, 2015), it can be concluded that this process is only beneficial within a physiologically adaptive range. Beyond a critical level of neurogenesis, the networks experience noise as a consequence of overexcitability. Considering all the conditions, it is not yet clear whether disrupted neurogenesis has a causative role in these disorders or a symptomatic one. A balance between the degenerative processes and the control of inflammation with neurogenesis could contribute to survival after global ischemia or in Alzheimer’s type dementia.
Thanks to the pleiotropic role of TnC in the CNS diseases, such as tumors, vascular and neurodegenerative diseases, this glycoprotein may be a candidate for clinical application (Hanmin et al., 2020). TnC has been recognized as another molecule involved in chronic inflammation in Alzheimer’s disease since its gene transcription is significantly increased in both cultured microglia after amyloid β (Aβ) peptide challenge, as well as in the brain of Alzheimer’s disease mouse model. In addition, TnC deficient mice have reduced proinflammatory but enhanced anti-inflammatory activity (Xie et al., 2013). TnC is upregulated in many human pathologies, and although it has been most extensively studied in the context of cancer, its immunomodulatory role also associates it with inflammatory diseases (Marzeda and Midwood, 2018). In adult human brains of AD patients, TnC was detected in diffused extracellular deposits in cortical gray matter, surrounding cored Aβ plaques (Mi et al., 2016). In addition, TnC plaques were associated with reactive astrocytes, microglia and Tau containing neurites. Furthermore, TnC was reported as a plasma biomarker of AD, since it is significantly increased in blood of AD patients (Soares et al., 2012). The beneficial effect of TnC on Alzheimer’s disease pathogenesis implicates this ECM glycoprotein as a new therapeutic target.
As discussed here, TnC is considered as an important molecule for tissue repair and regeneration by participating in intracellular pathways that can lead to cell proliferation, survival and motility. Further studies need to integrate the knowledge about the role of TnC in adult neurogenic niches. Better understanding of the ECM signaling in the niche of the adult CNS will have significant implications for regenerative therapies. Strategies to enhance repair by endogenous stem cells or to replace lost cells by transplantation of exogenous stem cells could be improved by manipulations that mimic niche signals and therefore promote stem cell survival, proliferation and differentiation.
MT, PA, and VS were engaged in manuscript conceptualization. PA provided resources. MT was engaged in writing original draft preparation. PA and VS did writing—review and editing. All authors contributed to the article and approved the submitted version.
This work was supported by the Ministry of education, science and technological development of the Republic of Serbia, contract no. 451-03-9/2021-14/200178.
The authors declare that the research was conducted in the absence of any commercial or financial relationships that could be construed as a potential conflict of interest.
We thank Andrej Korenić for review and editing.
Aimone, J. B., Wiles, J., and Gage, F. H. (2009). Computational influence of adult neurogenesis on memory encoding. Neuron 61, 187–202. doi: 10.1016/j.neuron.2008.11.026
Altman, J. (1969). Autoradiographic and histological studies of postnatal neurogenesis. III. dating the time of production and onset of differentiation of cerebellar microneurons in rats. J. Comp. Neurol. 136, 269–293. doi: 10.1002/cne.901360303
Altman, J., and Das, G. D. (1965). Autoradiographic and histological evidence of postnatal hippocampal neurogenesis in rats. J. Comp. Neurol. 124, 319–335. doi: 10.1002/cne.901240303
Alvarez-Buylla, A., and Garcìa-Verdugo, J. M. (2002). Neurogenesis in adult subventricular zone. J. Neurosci. 22, 629–634. doi: 10.1523/JNEUROSCI.22-03-00629.2002
Andrews, M. R., Czvitkovich, S., Dassie, E., Vogelaar, C. F., Faissner, A., Blits, B., et al. (2009). α9 integrin promotes neurite outgrowth on tenascin-C and enhances sensory axon regeneration. J. Neurosci. 29, 5546–5557. doi: 10.1523/JNEUROSCI.0759-09.2009
Asparuhova, M. B., Gelman, L., and Chiquet, M. (2009). Role of the actin cytoskeleton in tuning cellular responses to external mechanical stress. Scand. J. Med. Sci. Sports 19, 490–499. doi: 10.1111/j.1600-0838.2009.00928.x
Barkho, B. Z., Munoz, A. E., Li, X., Li, L., Cunningham, L. A., and Zhao, X. (2008). Endogenous matrix metalloproteinase (MMP)-3 and MMP-9 promote the differentiation and migration of adult neural progenitor cells in response to chemokines. Stem Cells 26, 3139–3149. doi: 10.1634/stemcells.2008-0519
Bartsch, S., Bartsch, U., Dijrries, U., and Ekblom, P. (1992). Expression cortex of tenascin in the developing and adult cerebellar cortex. J. Neurosci. 12, 736–749.
Becker, S. (2005). A computational principle for hippocampal learning and neurogenesis. Hippocampus 15, 722–738. doi: 10.1002/hipo.20095
Bennett, M. L., Bennett, F. C., Liddelow, S. A., Ajami, B., Zamanian, J. L., Fernhoff, N. B., et al. (2016). New tools for studying microglia in the mouse and human CNS. Proc. Natl. Acad. Sci. U S A. 113, E1738–E1746. doi: 10.1073/pnas.1525528113
Bernier, P. J., Bédard, A., Vinet, J., Lévesque, M., and Parent, A. (2002). Newly generated neurons in the amygdala and adjoining cortex of adult primates. Proc. Natl. Acad. Sci. U S A. 99, 11464–11469. doi: 10.1073/pnas.172403999
Bezard, E., Dovero, S., Belin, D., Duconger, S., Jackson-Lewis, V., Przedborski, S., et al. (2003). Enriched environment confers resistance to 1-Methyl-4-Phenyl-1,2,3,6-Tetrahydropyridine and cocaine: involvement of dopamine transporter and trophic factors. J. Neurosci. 23, 10999–11007. doi: 10.1523/jneurosci.23-35-10999.2003
Biebl, M., Cooper, C. M., Winkler, J., and Kuhn, H. G. (2000). Analysis of neurogenesis and programmed cell death reveals a self- renewing capacity in the adult rat brain. Neurosci. Lett. 291, 17–20. doi: 10.1016/S0304-3940(00)01368-9
Bonzano, S., Bovetti, S., Fasolo, A., Peretto, P., and De Marchis, S. (2014). Odour enrichment increases adult-born dopaminergic neurons in the mouse olfactory bulb. Eur. J. Neurosci. 40, 3450–3457. doi: 10.1111/ejn.12724
Bonzano, S., Bovetti, S., Gendusa, C., Peretto, P., and De Marchis, S. (2016). Adult born olfactory bulb dopaminergic interneurons: molecular determinants and experience-dependent plasticity. Front. Neurosci. 10:189. doi: 10.3389/fnins.2016.00189
Bornstein, P. (2009). Matricellular proteins: an overview. J. Cell Commun. Signal. 3, 163–165. doi: 10.1007/s12079-009-0069-z
Bovetti, S., Bovolin, P., Perroteau, I., and Puche, A. C. (2007). Subventricular zone-derived neuroblast migration to the olfactory bulb is modulated by matrix remodelling. Eur. J. Neurosci. 25, 2021–2033. doi: 10.1111/j.1460-9568.2007.05441.x
Bovetti, S., Veyrac, A., Peretto, P., Fasolo, A., and de Marchis, S. (2009). Olfactory enrichment influences adult neurogenesis modulating GAD67 and plasticity-related molecules expression in newborn cells of the olfactory bulb. PLoS One 4:e6359. doi: 10.1371/journal.pone.0006359
Brown, J., Cooper-Kuhn, C. M., Kempermann, G., Van Praag, H., Winkler, J., Gage, F. H., et al. (2003). Enriched environment and physical activity stimulate hippocampal but not olfactory bulb neurogenesis. Eur. J. Neurosci. 17, 2042–2046. doi: 10.1046/j.1460-9568.2003.02647.x
Cai, J., Du, S., Wang, H., Xin, B., Wang, J., Shen, W., et al. (2017). Tenascin-C induces migration and invasion through JNK/c-Jun signalling in pancreatic cancer. Oncotarget 8, 74406–74422. doi: 10.18632/oncotarget.20160
Cayre, M., Malaterre, J., Scotto-Lomassese, S., Strambi, C., and Strambi, A. (2002). The common properties of neurogenesis in the adult brain: from invertebrates to vertebrates. Comp. Biochem. Physiol. -B Biochem. Mol. Biol. 132, 1–15. doi: 10.1016/S1096-4959(01)00525-5
Chiovaro, F., Chiquet-Ehrismann, R., and Chiquet, M. (2015). Transcriptional regulation of tenascin genes. Cell Adhesion Migrat. 9, 34–47. doi: 10.1080/19336918.2015.1008333
Chiquet, M., Tunç-Civelek, V., and Sarasa-Renedo, A. (2007). Gene regulation by mechanotransduction in fibroblasts. Appl. Physiol. Nutrit. Metab. 32, 967–973. doi: 10.1139/H07-053
Chiquet-Ehrismann, R., and Chiquet, M. (2003). Tenascins: regulation and putative functions during pathological stress. J. Pathol. 200, 488–499. doi: 10.1002/path.1415
Chiquet-Ehrismann, R., Mackie, E. J., Pearson, C. A., and Sakakura, T. (1986). Tenascin: an extracellular matrix protein involved in tissue interactions during fetal development and oncogenesis. Cell 47, 131–139. doi: 10.1016/0092-8674(86)90374-0
Chiquet-Ehrismann, R., Orend, G., Chiquet, M., Tucker, R. P., and Midwood, K. S. (2014). Tenascins in stem cell niches. Matrix Biol. 37, 112–123. doi: 10.1016/j.matbio.2014.01.007
Clelland, C. D., Choi, M., Romberg, C., Clemenson, G. D., Fragniere, A., Tyers, P., et al. (2009). A functional role for adult hippocampal neurogenesis in spatial pattern separation. Science 325, 210–213. doi: 10.1126/science.1173215
Czopka, T., Von Holst, A., Ffrench-Constant, C., and Faissner, A. (2010). Regulatory mechanisms that mediate tenascin C-dependent inhibition of oligodendrocyte precursor differentiation. J. Neurosci. 30, 12310–12322. doi: 10.1523/JNEUROSCI.4957-09.2010
de Chevigny, A., Lemasson, M., Saghatelyan, A., Sibbe, M., Schachner, M., and Lledo, P. M. (2006). Delayed onset of odor detection in neonatal mice lacking tenascin-C. Mol. Cell. Neurosci. 32, 174–186. doi: 10.1016/j.mcn.2006.04.002
Diaz-Aparicio, I., Paris, I., Sierra-Torre, V., Plaza-Zabala, A., Rodríguez-Iglesias, N., Márquez-Ropero, M., et al. (2020). Microglia actively remodel adult hippocampal neurogenesis through the phagocytosis secretome. J. Neurosci. 40, 1453–1482. doi: 10.1523/JNEUROSCI.0993-19.2019
Ding, H., Jin, M., Liu, D. A. I., Wang, S., and Zhang, J. (2018). Tenascin - C promotes the migration of bone marrow stem cells via toll - like receptor 4 - mediated signaling pathways: MAPK. AKT and Wnt. Mol. Med. Rep. 17, 7603–7610. doi: 10.3892/mmr.2018.8855
Eisch, A. J., and Petrik, D. (2012). Depression and hippocampal neurogenesis: a road to remission? Science 338, 72–75. doi: 10.1126/science.1222941
Ekdahl, C. T. (2012). Microglial activation-tuning and pruning adult neurogenesis. Front. Pharmacol. 3:41. doi: 10.3389/fphar.2012.00041
Ekdahl, C. T., Claasen, J.-H., Bonde, S., Kokaia, Z., and Lindvall, O. (2003). Inflammation is detrimental for neurogenesis in adult brain. Proc. Natl. Acad. Sci. 100, 13632–13637. doi: 10.1073/pnas.2234031100
Enwere, E., Shingo, T., Gregg, C., Fujikawa, H., Ohta, S., and Weiss, S. (2004). Aging results in reduced epidermal growth factor receptor signaling, diminished olfactory neurogenesis, and deficits in fine olfactory discrimination. J. Neurosci. 24, 8354–8365. doi: 10.1523/JNEUROSCI.2751-04.2004
Eriksson, P. S., Perfilieva, E., Björk-Eriksson, T., Alborn, A. M., Nordborg, C., Peterson, D. A., et al. (1998). Neurogenesis in the adult human hippocampus. Nat. Med. 4, 1313–1317. doi: 10.1038/3305
Evers, M. R., Salmen, B., Bukalo, O., Rollenhagen, A., Bösl, M. R., Morellini, F., et al. (2002). Impairment of L-type Ca2+ channel-dependent forms of hippocampal synaptic plasticity in mice deficient in the extracellular matrix glycoprotein tenascin-C. J. Neurosci. 22, 7177–7194. doi: 10.1523/jneurosci.22-16-07177.2002
Faissner, A., and Kruse, J. (1990). J1/tenascin is a repulsive substrate for central nervous system neurons. Neuron 5, 627–637. doi: 10.1016/0896-6273(90)90217-4
Faissner, A., Roll, L., and Theocharidis, U. (2017). Tenascin-C in the matrisome of neural stem and progenitor cells. Mol. Cell. Neurosci. 81, 22–31. doi: 10.1016/j.mcn.2016.11.003
Ferhat, L., Chevassus, Au Louis, N., Jorquera, I., Niquet, J., Khrestchatisky, M., et al. (1996). Transient increase of tenascin-C in immature hippocampus: astroglial and neuronal expression. J. Neurocytol. 25, 53–66. doi: 10.1007/BF02284785
Forest, J., Moreno, M., Cavelius, M., Chalençon, L., Ziessel, A., Sacquet, J., et al. (2019). Short-term availability of adult-born neurons for memory encoding. Nat. Commun. 10:5609. doi: 10.1038/s41467-019-13521-7
Fortin, D. A., Srivastava, T., and Soderling, T. R. (2012). Structural modulation of dendritic spines during synaptic plasticity. Neuroscientist 18, 326–341. doi: 10.1177/1073858411407206
Garcion, E., Faissner, A., and Ffrench-Constant, C. (2001). Knockout mice reveal a contribution of the extracellular matrix molecule tenascin-C to neural precursor proliferation and migration. Development 128, 2485–2496.
Garcion, E., Halilagic, A., Faissner, A., and ffrench-Constant, C. (2004). Generation of an environmental niche for neural stem cell development bythe extracellular matrix molecule tenascin C. Development 131, 3423–3432. doi: 10.1242/dev.01202
Garwood, J., Garcion, E., Dobbertin, A., Heck, N., Calco, V., Ffrench-Constant, C., et al. (2004). The extracellular matrix glycoprotein Tenascin-C is expressed by oligodendrocyte precursor cells and required for the regulation of maturation rate, survival and responsiveness to platelet-derived growth factor. Eur. J. Neurosci. 20, 2524–2540. doi: 10.1111/j.1460-9568.2004.03727.x
Gates, M. A., Thomas, L. B., Howard, E. M., Laywell, E. D., Sajin, B., Faissner, A., et al. (1995). Cell and molecular analysis of the developing and adult mouse subventricular zone of the cerebral hemispheres. J. Comp. Neurol. 266, 249–266.
Ge, S., Pradhan, D. A., Ming, G. L., and Song, H. (2007). GABA sets the tempo for activity-dependent adult neurogenesis. Trends Neurosci. 30, 1–8. doi: 10.1016/j.tins.2006.11.001
Giblin, S. P., and Midwood, K. S. (2015). Tenascin-C: form versus function. Cell Adhesion Migrat. 9, 48–82. doi: 10.4161/19336918.2014.987587
Gonçalves, J. T., Schafer, S. T., and Gage, F. H. (2016). Adult neurogenesis in the hippocampus: from stem cells to behavior. Cell 167, 897–914. doi: 10.1016/j.cell.2016.10.021
Götz, M., Bolz, J., Joester, A., and Faissner, A. (1997). Tenascin-C synthesis and influence on axonal growth during rat cortical development. Eur. J. Neurosci. 9, 496–506. doi: 10.1111/j.1460-9568.1997.tb01627.x
Haage, V., Elmadany, N., Roll, L., Faissner, A., Gutmann, D. H., Semtner, M., et al. (2019). Tenascin C regulates multiple microglial functions involving TLR4 signaling and HDAC1. Brain Behav. Immun. 81, 470–483. doi: 10.1016/j.bbi.2019.06.047
Hall, A. (1998). Rho GTPases and the actin cytoskeleton. Science 279, 509–514. doi: 10.1126/science.279.5350.509
Hanmin, C., Xiangyue, Z., Lenahan, C., Ling, W., Yibo, O., and Yue, H. (2020). Pleiotropic role of Tenascin-C in central nervous system diseases: from basic to clinical applications. Front. Neurol. 11:576230. doi: 10.3389/fneur.2020.576230
Hendaoui, I., Tucker, R. P., Zingg, D., Bichet, S., Schittny, J., and Chiquet-Ehrismann, R. (2014). Tenascin-C is required for normal Wnt/β-catenin signaling in the whisker follicle stem cell niche. Matrix Biol. 40, 46–53. doi: 10.1016/j.matbio.2014.08.017
Husmann, K., Carbonetto, S., and Schachner, M. (1995). Distinct sites on Tenascin-C mediate repellent or adhesive interactions with different neuronal cell types. Cell Adhesion Commun. 3, 293–310. doi: 10.3109/15419069509081015
Irintchev, A., Rollenhagen, A., Troncoso, E., Kiss, J. Z., and Schachner, M. (2005). Structural and functional aberrations in the cerebral cortex of tenascin-C deficient mice. Cereb. Cortex 15, 950–962. doi: 10.1093/cercor/bhh195
Jankowsky, J. L., Melnikova, T., Fadale, D. J., Xu, G. M., Slunt, H. H., Gonzales, V., et al. (2005). Environmental enrichment mitigates cognitive deficits in a mouse model of Alzheimer’s disease. J. Neurosci. 25, 5217–5224. doi: 10.1523/JNEUROSCI.5080-04.2005
Jessberger, S., and Parent, J. M. (2015). Epilepsy and adult neurogenesis. Cold Spring Harb. Perspect. Biol. 7:a020677. doi: 10.1101/cshperspect.a020677
Jones, P. L., and Jones, F. S. (2000). Tenascin-C in development and disease: gene regulation and cell function. Matrix Biol. 19, 581–596. doi: 10.1016/S0945-053X(00)00106-2
Kakinuma, Y., Saito, F., Osawa, S., and Miura, M. (2004). A mechanism of impaired mobility of oligodendrocyte progenitor cells by tenascin C through modification of wnt signaling. FEBS Lett. 568, 60–64. doi: 10.1016/j.febslet.2004.05.022
Karus, M., Denecke, B., Ffrench-Constant, C., Wiese, S., and Faissner, A. (2011). The extracellular matrix molecule tenascin C modulates expression levels and territories of key patterning genes during spinal cord astrocyte specification. Development 138, 5321–5331. doi: 10.1242/dev.067413
Kazanis, I., Belhadi, A., Faissner, A., and ffrench-Constant, C. (2007). The adult mouse subependymal zone regenerates efficiently in the absence of Tenascin-C. J. Neurosci. 27, 13991–13996. doi: 10.1523/jneurosci.3279-07.2007
Kempermann, G., Gast, D., Kronenberg, G., Yamaguchi, M., and Gage, F. H. (2003). Early determination and long-term persistence of adult-generated new neurons in the hippocampus of mice. Development 130, 391–399. doi: 10.1242/dev.00203
Kempermann, G., Jessberger, S., Steiner, B., and Kronenberg, G. (2004). Milestones of neuronal development in the adult hippocampus. Trends Neurosci. 27, 447–452. doi: 10.1016/j.tins.2004.05.013
Kitamura, T., Saitoh, Y., Takashima, N., Murayama, A., Niibori, Y., Ageta, H., et al. (2009). Adult neurogenesis modulates the hippocampus-dependent period of associative fear memory. Cell 139, 814–827. doi: 10.1016/j.cell.2009.10.020
Kjell, J., Fischer-Sternjak, J., Thompson, A. J., Friess, C., Sticco, M. J., Salinas, F., et al. (2020). Defining the adult neural stem cell niche proteome identifies key regulators of adult neurogenesis. Cell Stem Cell 26, 277–293.e8. doi: 10.1016/j.stem.2020.01.002
Koser, D. E., Moeendarbary, E., Hanne, J., Kuerten, S., and Franze, K. (2015). CNS cell distribution and axon orientation determine local spinal cord mechanical properties. Biophys. J. 108, 2137–2147. doi: 10.1016/j.bpj.2015.03.039
Kriegstein, A., and Alvarez-Buylla, A. (2009). The glial nature of embryonic and adult neural stem cells. Annual Rev. Neurosci. 32, 149–184. doi: 10.1146/annurev.neuro.051508.135600
Kuhn, H. G., Dickinson-Anson, H., and Gage, F. H. (1996). Neurogenesis in the dentate gyrus of the adult rat: age-related decrease of neuronal progenitor proliferation. J. Neurosci. 16, 2027–2033. doi: 10.1523/jneurosci.16-06-02027.1996
Liu, S. H., Wang, J., Zhu, D. Y., Fu, Y. P., Lukowiak, K., and Lu, Y. M. (2003). Generation of functional inhibitory neurons in the adult rat hippocampus. J. Neurosci. 23, 732–736. doi: 10.1523/jneurosci.23-03-00732.2003
Lledo, P. M., Alonso, M., and Grubb, M. S. (2006). Adult neurogenesis and functional plasticity in neuronal circuits. Nat. Rev. Neurosci. 7, 179–193. doi: 10.1038/nrn1867
Lochter, A., and Schachner, M. (1993). Tenascin and extracellular matrix glycoproteins: from promotion to polarization of neurite growth in vitro. J. Neurosci. 13, 3986–4000. doi: 10.1523/jneurosci.13-09-03986.1993
Luque, T., Kang, M. S., Schaffer, D. V., and Kumar, S. (2016). Microelastic mapping of the rat dentate gyrus. Royal Soc. Open Sci. 3:150702. doi: 10.1098/rsos.150702
Ma, D. K., Kim, W. R., Ming, G., and Song, H. (2009). Activity-dependent extrinsic regulation of adult olfactory bulb and hippocampal neurogenesis. Annals N. Y. Acad. Sci. 1170, 664–673. doi: 10.1111/j.1749-6632.2009.04373.x
Manrique-Castano, D., Dzyubenko, E., Borbor, M., Vasileiadou, P., Kleinschnitz, C., Roll, L., et al. (2020). Tenascin-C preserves microglia surveillance and restricts leukocyte and, more specifically, T cell infiltration of the ischemic brain. Brain Behav. Immun. 91, 639–648. doi: 10.1016/j.bbi.2020.10.016
Marzeda, A. M., and Midwood, K. S. (2018). Internal affairs: tenascin-C as a clinically relevant, endogenous driver of innate immunity. J. Histochem. Cytochem. 66, 289–304. doi: 10.1369/0022155418757443
Mi, Z., Halfter, W., Abrahamson, E. E., Klunk, W. E., Mathis, C. A., Mufson, E. J., et al. (2016). Tenascin-C is associated with cored amyloid-b plaques in Alzheimer disease and pathology burdened cognitively normal elderly. J. Neuropathol. Exp. Neurol. 75, 868–876. doi: 10.1093/jnen/nlw062
Midwood, K., Sacre, S., Piccinini, A. M., Inglis, J., Trebaul, A., Chan, E., et al. (2009). Tenascin-C is an endogenous activator of Toll-like receptor 4 that is essential for maintaining inflammation in arthritic joint disease. Nat. Med. 15, 774–780. doi: 10.1038/nm.1987
Midwood, K. S., Chiquet, M., Tucker, R. P., and Orend, G. (2016). Tenascin-C at a glance. J. Cell Sci. 129, 4321–4327. doi: 10.1242/jcs.190546
Midwood, K. S., Hussenet, T., Langlois, B., and Orend, G. (2011). Advances in tenascin-C biology. Cell. Mol. Life Sci. 68, 3175–3199. doi: 10.1007/s00018-011-0783-6
Midwood, K. S., and Schwarzbauer, J. E. (2002). Tenascin-C modulates matrix contraction via focal adhesion kinase– and rho-mediated signaling pathways. Mol. Biol. Cell 13, 3601–3613. doi: 10.1091/mbc.e02-05-0292
Milev, P., Fischer, D., Haring, M., Schulthess, T., Margolis, R. K., Chiquet- Ehrismann, R., et al. (1997). The fibrinogen-like globe of tenascin-C mediates its interactions with neurocan and phosphacan/protein-tyrosine phosphatase-ζ/β. J. Biol. Chem. 272, 15501–15509. doi: 10.1074/jbc.272.24.15501
Morante-Redolat, J. M., and Porlan, E. (2019). Neural stem cell regulation by adhesion molecules within the subependymal niche. Front. Cell Dev. Biol. 7:102. doi: 10.3389/fcell.2019.00102
Morellini, F., and Schachner, M. (2006). Enhanced novelty-induced activity, reduced anxiety, delayed resynchronization to daylight reversal and weaker muscle strength in tenascin-C-deficient mice. Eur. J. Neurosci. 23, 1255–1268. doi: 10.1111/j.1460-9568.2006.04657.x
Moritz, S., Lehmann, S., Faissner, A., and von Holst, A. (2008). An induction gene trap screen in neural stem cells reveals an instructive function of the niche and identifies the splicing regulator Sam68 as a Tenascin-C-Regulated target gene. Stem Cells 26, 2321–2331. doi: 10.1634/stemcells.2007-1095
Murase, S. I., and Horwitz, A. F. (2002). Deleted in colorectal carcinoma and differentially expressed integrins mediate the directional migration of neural precursors in the rostral migratory stream. J. Neurosci. 22, 3568–3579. doi: 10.1523/jneurosci.22-09-03568.2002
Niquet, J., Jorquera, I., Faissner, A., Ben-Ari, Y., and Represa, A. (1995). Gliosis and axonal sprouting in the hippocampus of epileptic rats are associated with an increase of tenascin-C immunoreactivity. J. Neurocytol. 24, 611–624. doi: 10.1007/BF01257376
Nissant, A., Bardy, C., Katagiri, H., Murray, K., and Lledo, P. M. (2009). Adult neurogenesis promotes synaptic plasticity in the olfactory bulb. Nat. Neurosci. 12, 728–730. doi: 10.1038/nn.2298
Oberhauser, A. F., Marszalek, P. E., Erickson, H. P., and Fernandez, J. M. (1998). The molecular elasticity of the extracellular matrix protein tenascin. Nature 393, 181–185. doi: 10.1038/30270
Palmer, T. D., Willhoite, A. R., and Gage, F. H. (2000). Vascular niche for adult hippocampal neurogenesis. J. Comp. Neurol. 425, 479–494.
Peretto, P., Giachino, C., Aimar, P., Fasolo, A., and Bonfanti, L. (2005). Chain formation and glial tube assembly in the shift from neonatal to adult subventricular zone of the rodent forebrain. J. Comp. Neurol. 487, 407–427. doi: 10.1002/cne.20576
Piccinini, A. M., and Midwood, K. S. (2012). Endogenous control of immunity against infection: tenascin-C regulates TLR4-Mediated inflammation via MicroRNA-155. Cell Rep. 2, 914–926. doi: 10.1016/j.celrep.2012.09.005
Rinberg, D., and Gelperin, A. (2006). Olfactory neuronal dynamics in behaving animals. Sem. Cell Dev. Biol. 17, 454–461. doi: 10.1016/j.semcdb.2006.04.009
Rochefort, C., Gheusi, G., Vincent, J. D., and Lledo, P. M. (2002). Enriched odor exposure increases the number of newborn neurons in the adult olfactory bulb and improves odor memory. J. Neurosci. 22, 2679–2689. doi: 10.1523/jneurosci.22-07-02679.2002
Rodríguez, J. J., and Verkhratsky, A. (2011). Neurogenesis in Alzheimer’s disease. J. Anatomy 219, 78–89. doi: 10.1111/j.1469-7580.2011.01343.x
Roll, L., and Faissner, A. (2019). Tenascins in CNS lesions. Sem. Cell Dev. Biol. 89, 118–124. doi: 10.1016/j.semcdb.2018.09.012
Saga, Y., Yagi, T., Ikawa, Y., Sakakura, T., and Aizawa, S. (1992). Mice develop normally without tenascin. Genes Dev. 6, 1821–1831. doi: 10.1101/gad.6.10.1821
Saha, K., Keung, A. J., Irwin, E. F., Li, Y., Little, L., Schaffer, D. V., et al. (2008). Substrate modulus directs neural stem cell behavior. Biophys. J. 95, 4426–4438. doi: 10.1529/biophysj.108.132217
Schnapp, L. M., Hatch, N., Ramos, D. M., Klimanskaya, I. V., Sheppard, D., and Pytela, R. (1995). The human integrin α8β1 functions as a receptor for tenascin, fibronectin, and vitronectin. J. Biol. Chem. 270, 23196–23202. doi: 10.1074/jbc.270.39.23196
Sekeljic, V., Bataveljic, D., Stamenkovic, S., Ułamek, M., Jabłoński, M., Radenovic, L., et al. (2012). Cellular markers of neuroinflammation and neurogenesis after ischemic brain injury in the long-term survival rat model. Brain Structure Funct. 217, 411–420. doi: 10.1007/s00429-011-0336-7
Sever, M., Gunay, G., Guler, M. O., and Tekinay, A. B. (2018). Tenascin-C derived signaling induces neuronal differentiation in a three-dimensional peptide nanofiber gel. Biomater. Sci. 6, 1859–1868. doi: 10.1039/c7bm00850c
Sierra, A., Encinas, J. M., Deudero, J. J. P., Chancey, J. H., Enikolopov, G., Overstreet-wadiche, L. S., et al. (2010). Microglia shape adult hippocampal neurogenesis through apoptosis-coupled phagocytosis. Stem Cell 7, 483–495. doi: 10.1016/j.stem.2010.08.014
Soares, H. D., Potter, W. Z., Pickering, E., Kuhn, M., Immermann, F. W., Shera, D. M., et al. (2012). Plasma biomarkers associated with the apolipoprotein E genotype and Alzheimer disease. Arch. Neurol. 69, 1310–1317. doi: 10.1001/archneurol.2012.1070
Sorrells, S. F., Paredes, M. F., Cebrian-silla, A., Qi, D., Kelley, K. W., James, D., et al. (2018). Human hippocampal neurogenesis drops sharply. Nature 555, 377–381. doi: 10.1038/nature25975.Human
Spalding, K. L., Bergmann, O., Alkass, K., Bernard, S., Salehpour, M., Huttner, H. B., et al. (2013). XDynamics of hippocampal neurogenesis in adult humans. Cell 153:1219. doi: 10.1016/j.cell.2013.05.002
Stamenkovic, V., Milenkovic, I., Galjak, N., Todorovic, V., and Andjus, P. (2017a). Enriched environment alters the behavioral profile of tenascin-C deficient mice. Behav. Brain Res. 331, 241–253. doi: 10.1016/j.bbr.2017.05.047
Stamenkovic, V., Stamenkovic, S., Jaworski, T., Gawlak, M., Jovanovic, M., Jakovcevski, I., et al. (2017b). The extracellular matrix glycoprotein tenascin-C and matrix metalloproteinases modify cerebellar structural plasticity by exposure to an enriched environment. Brain Structure Funct. 222, 393–415. doi: 10.1007/s00429-016-1224-y
Strekalova, T., Sun, M., Sibbe, M., Evers, M., Dityatev, A., Gass, P., et al. (2002). Fibronectin domains of extracellular matrix molecule tenascin-C modulate hippocampal learning and synaptic plasticity. Mol. Cell. Neurosci. 21, 173–187. doi: 10.1006/mcne.2002.1172
Stukel, J. M., and Willits, R. K. (2018). The interplay of peptide affinity and scaffold stiffness on neuronal differentiation of neural stem cells. Biomed. Mater. 13:024102. doi: 10.1088/1748-605X/aa9a4b
Takasawa, K. I., Kitagawa, K., Yagita, Y., Sasaki, T., Tanaka, S., Matsushita, K., et al. (2002). Increased proliferation of neural progenitor cells but reduced survival of newborn cells in the contralateral hippocampus after focal cerebral ischemia in rats. J. Cereb. Blood Flow Metab. 22, 299–307. doi: 10.1097/00004647-200203000-00007
Tavazoie, M., Van der Veken, L., Silva-Vargas, V., Louissaint, M., Colonna, L., Zaidi, B., et al. (2008). A specialized vascular niche for adult neural stem cells. Cell Stem Cell 3, 279–288. doi: 10.1016/j.stem.2008.07.025
Tremblay, M. È, and Majewska, A. K. (2011). A role for microglia in synaptic plasticity? Commun. Integrat. Biol. 4, 220–222. doi: 10.4161/cib.4.2.14506
Tsai, H. L., Chiu, W. T., Fang, C. L., Hwang, S. M., Renshaw, P. F., and Lai, W. F. T. (2014). Different forms of tenascin-C with tenascin-r regulate neural differentiation in bone marrow-derived human mesenchymal stem cells. Tissue Eng. - Part A 20, 1908–1921. doi: 10.1089/ten.tea.2013.0188
Tucker, R. P., and Chiquet-Ehrismann, R. (2015). Tenascin-C: its functions as an integrin ligand. Int. J. Biochem. Cell Biol. 65, 165–168. doi: 10.1016/j.biocel.2015.06.003
Valliéres, L., Campbell, I. L., Gage, F. H., and Sawchenko, P. E. (2002). Reduced hippocampal neurogenesis in adult transgenic mice with chronic astrocytic production of interleukin-6. J. Neurosci. 22, 486–492. doi: 10.1523/jneurosci.22-02-00486.2002
Van Praag, H., Shubert, T., Zhao, C., and Gage, F. H. (2005). Exercise enhances learning and hippocampal neurogenesis in aged mice. J. Neurosci. 25, 8680–8685. doi: 10.1523/JNEUROSCI.1731-05.2005
Wallace, J., Lord, J., Dissing-Olesen, L., Stevens, B., and Murthy, V. N. (2020). Microglial depletion disrupts normal functional development of adult-born neurons in the olfactory bulb. eLife 9:e50531. doi: 10.7554/eLife.50531
Wang, S., Scott, B. W., and Wojtowicz, J. M. (2000). Heterogenous properties of dentate granule neurons in the adult rat. J. Neurobiol. 42, 248–257.
Wiskott, L., Rasch, M. J., and Kempermann, G. (2006). A functional hypothesis for adult hippocampal neurogenesis: avoidance of catastrophic interference in the dentate gyrus. Hippocampus 16, 329–343. doi: 10.1002/hipo.20167
Xie, K., Liu, Y., Hao, W., Walter, S., Penke, B., Hartmann, T., et al. (2013). Tenascin-C deficiency ameliorates Alzheimer’s disease-related pathology in mice. Neurobiol. Aging 34, 2389–2398. doi: 10.1016/j.neurobiolaging.2013.04.013
Yagi, H., Yanagisawa, M., Suzuki, Y., Nakatani, Y., Ariga, T., Kato, K., et al. (2010). HNK-1 epitope-carrying tenascin-C spliced variant regulates the proliferation of mouse embryonic neural stem cells. J. Biol. Chem. 285, 37293–37301. doi: 10.1074/jbc.M110.157081
Yokosaki, Y., Matsuura, N., Higashiyama, S., Murakami, I., Obara, M., Yamakido, M., et al. (1998). Identification of the ligand binding site for the integrin α9β1 in the third fibronectin type III repeat of tenascin-C. J. Biol. Chem. 273, 11423–11428. doi: 10.1074/jbc.273.19.11423
Young, D., Lawlor, P. A., Leone, P., Dragunow, M., and During, M. J. (1999). Environmental enrichment inhibits spontaneous apoptosis, prevents seizures and is neuroprotective. Nat. Med. 5, 448–453. doi: 10.1038/7449
Zhao, M., Momma, S., Delfani, K., Carlén, M., Cassidy, R. M., Johansson, C. B., et al. (2003). Evidence for neurogenesis in the adult mammalian substantia nigra. Proc. Natl. Acad. Sci. U S A. 100, 7925–7930. doi: 10.1073/pnas.1131955100
Ziv, Y., Ron, N., Butovsky, O., Landa, G., Sudai, E., Greenberg, N., et al. (2006). Immune cells contribute to the maintenance of neurogenesis and spatial learning abilities in adulthood. Nat. Neurosci. 9, 268–275. doi: 10.1038/nn1629
Keywords: tenascin C, adult neurogenesis, subgranular zone, subventricular zone, astrocyte, microglia, stem cell migration
Citation: Tucić M, Stamenković V and Andjus P (2021) The Extracellular Matrix Glycoprotein Tenascin C and Adult Neurogenesis. Front. Cell Dev. Biol. 9:674199. doi: 10.3389/fcell.2021.674199
Received: 28 February 2021; Accepted: 09 April 2021;
Published: 29 April 2021.
Edited by:
Irina Ivanova Stoyanova, Medical University of Varna, BulgariaReviewed by:
Lars Roll, Ruhr University Bochum, GermanyCopyright © 2021 Tucić, Stamenković and Andjus. This is an open-access article distributed under the terms of the Creative Commons Attribution License (CC BY). The use, distribution or reproduction in other forums is permitted, provided the original author(s) and the copyright owner(s) are credited and that the original publication in this journal is cited, in accordance with accepted academic practice. No use, distribution or reproduction is permitted which does not comply with these terms.
*Correspondence: Pavle Andjus, cGFuZGp1c0BiaW8uYmcuYWMucnM=
Disclaimer: All claims expressed in this article are solely those of the authors and do not necessarily represent those of their affiliated organizations, or those of the publisher, the editors and the reviewers. Any product that may be evaluated in this article or claim that may be made by its manufacturer is not guaranteed or endorsed by the publisher.
Research integrity at Frontiers
Learn more about the work of our research integrity team to safeguard the quality of each article we publish.