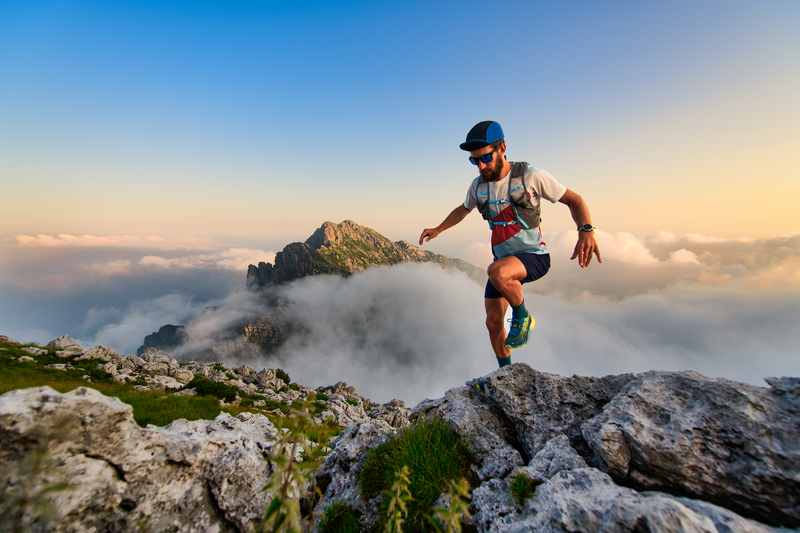
95% of researchers rate our articles as excellent or good
Learn more about the work of our research integrity team to safeguard the quality of each article we publish.
Find out more
MINI REVIEW article
Front. Cell Dev. Biol. , 14 June 2021
Sec. Signaling
Volume 9 - 2021 | https://doi.org/10.3389/fcell.2021.674137
This article is part of the Research Topic The Fertilization Success from the Oocyte's Perspective View all 15 articles
Mammalian females are born with a finite reserve of ovarian follicles, the functional units of the ovary. Building an ovarian follicle involves a complex interaction between multiple cell types, of which the oocyte germ cell and the somatic granulosa cells play a major role. Germ–somatic cell interactions are modulated by factors of different cell origins that influence ovarian development. In early development, failure in correct germ–somatic cell communication can cause abnormalities in ovarian development. These abnormalities can lead to deficient oocyte differentiation, to a diminished ovarian follicle reserve, and consequently to early loss of fertility. However, oocyte–granulosa cell communication is also extremely important for the acquisition of oocyte competence until ovulation. In this paper, we will visit the establishment of follicle reserve, with particular emphasis in germ–somatic cell interactions, and their importance for human fertility.
Mammalian females have a finite reproductive reserve of oocytes, established during fetal life (human) or perinatally (mouse). This reserve is composed of a large number of primordial follicles (PF), in which the oocyte is enclosed by a small number of somatic cells—pre-granulosa cells (Rodrigues et al., 2009; Clarke, 2017). It is during PF formation that the ovarian follicle reserve is established (Pepling and Spradling, 2001; Rodrigues et al., 2009). The oocyte is the essential component of female reproduction and it must undergo growth and development within the environment of an ovarian follicle (Chang et al., 2017). This environment includes bidirectional communication that is absolutely critical for survival of the oocyte and the companion granulosa cells. The oocyte receives nutrients, metabolites, and extrafollicular signals from the surrounding granulosa cells directly influencing its differentiation. The oocyte also signals to the surrounding granulosa cells, inducing their proliferation and survival. This reciprocal communication between oocyte and somatic cells within the follicle is essential to support full oocyte development (Li and Albertini, 2013; Coticchio et al., 2015; McGinnis et al., 2018; review Wang et al., 2017).
Across reproductive life, the initial PF reserve gradually diminishes until it is exhausted, over months (mouse) and decades (human) of attrition (Wang et al., 2017; McGinnis et al., 2018). Unfortunately, 1% of women worldwide suffer early loss of their reserve, a condition termed premature ovarian insufficiency (POI), in which the PF reserve is exhausted before women reach 40 years old (Grive and Freiman, 2015). It has been suggested that POI may be due to a combination of multiple genetic mutations (Bouilly et al., 2015). Therefore, understanding how the follicle reserve is established may shed light on the female reproductive lifespan.
This review will focus on ovarian follicle assembly with particular emphasis on somatic–germ cell communication and its role in establishment of the follicle reserve and, consequently, mammalian female fertility.
In the mouse model, traditional views hold that oogonia proliferate within a cluster, through a series of incomplete mitosis, due to the speed of cell divisions (Pepling and Spradling, 1998; Rodrigues et al., 2009). These clusters are surrounded by mesonephros-derived somatic cells that form the ovigerous or rete cords, continuous with the surface epithelium of the ovary (Byskov, 1986; McLaren, 2003; Guigon and Magre, 2006). Within the ovigerous cords, at around fetal 15.5 in mice, and 11–12 weeks gestation in humans, oogonia enter into prophase of the first meiotic division and arrest in diplotene stage (van den Hurk and Zhao, 2005; Jones and Pepling, 2013; Grive and Freiman, 2015). Follicular histogenesis is initiated through a process of fragmentation of the ovigerous cords, alignment of epithelial pre-granulosa cells to surround individual primordial oocytes, and the attraction of mesenchymal cells to the follicle’s nascent basement membrane (van den Hurk and Zhao, 2005; Guigon and Magre, 2006). This coordinated interaction, between these different cell types, results in the formation of the lifetime pool of PF, known as the ovarian follicular reserve (Figure 1A).
Figure 1. Ovarian follicle assembly and folliculogenesis. (A) The ovarian follicles are regulated by germ–somatic cell interaction. When this communication is disrupted, follicle assembly is impaired, leading to early follicle reserve loss (Irx3/5; Nobox, Notch2) or inability to acquire meiotic competence (GDF9). (B) Folliculogenesis occurs with oocyte growth, mainly due to a bidirectional communication between the oocyte and granulosa cells through TZPs. If this communication is disrupted, follicle development may arrest at different stages: primordial (Nobox), primary (GDF9/Cx43), or secondary (FSHβ).
This period of PF assembly, and establishment of the follicular reserve is associated with a significant germ cell loss, mostly by apoptosis (Rodrigues et al., 2009; Wang et al., 2020). However, other mechanisms of germ cell loss have been implicated in this process, like autophagy and oocyte shedding through the nascent ovarian epithelium (Rodrigues et al., 2009). Follicle assembly occurs during human’s fetal life, while in the mouse, follicle assembly occurs postnatally (Guigon and Magre, 2006).
The process of cluster breakdown starts in the medullary region of the ovary and progresses to the cortical area with follicle growth (Wang et al., 2017). This movement is supported by two different waves of pre-granulosa cell activation, giving rise to two different classes of PF (Mork et al., 2012; Zheng et al., 2014a). The first wave of pre-granulosa cells contributes to the formation of the first class of PFs in the medulla area and will be the follicles contributing to the onset of puberty (Zheng et al., 2014a, b; Wang et al., 2017), whereas the second wave of differentiating pre-granulosa cells contributes to the formation of the PFs involved in the second class of follicles that will start to grow within the ovarian cortex and support the female reproductive life in its end (Zheng et al., 2014a, b; Wang et al., 2017). Using single-cell RNA sequencing during PF assembly, at E16.5 (mouse) pre-granulosa cell differentiation and second wave initiation, an increase in the expression of stem cell markers Wnt4 and Aldh1a1 was detected, which are essential for retinoic acid biosynthesis (key regulator of meiosis) (Zhang et al., 2018; Wang et al., 2020). Interestingly, it was suggested that instead of meiosis entry, the geographic location of the PF within the ovary may have a greater influence in determining which PF will activate first (Cordeiro et al., 2015). Through design of a triple transgenic mouse for MVH/GDF9/ZP3, the authors observed that the ventral side of the ovary has an earlier onset of meiosis, but the first PF formed and activated were located in the dorsal region (Cordeiro et al., 2015).
Interestingly, two members of the Iroquois homeobox transcription factor gene family (involved in patterning and embryogenesis), Irx3 and Irx5, are fundamental for correct PF formation (Fu et al., 2018). Deleting both factors in mice caused defects in both oocyte and granulosa cells during primordial follicle assembly (Fu et al., 2018). These results show that communication between all the cells involved is essential for proper germ cell cluster breakdown and PF assembly (Fu et al., 2018; Wang et al., 2020).
Cell-to-cell communication is an important driving force during mammalian embryonic development, inducing cell movement, rearrangement, shape changing, etc., of which the ovarian follicle assembly is no exception (Wang et al., 2017). The bidirectional communication between germ cells and somatic cells is fundamental for follicle assembly and adequate follicle environment for acquisition of oocyte competence (Li and Albertini, 2013; Zhang et al., 2018).
The interactions between germ cells (oogonia and oocytes) and somatic cells (granulosa, theca, and stromal cells), vasculature, and extracellular matrix (ECM) are all essential components enabling follicle formation, growth, ovulation, and luteinization. Direct communication between an oocyte and its surrounding companion granulosa cells requires transzonal projections (TZPs) that extend from the granulosa cells closest to the oocyte (corona radiata cells), through the zona pellucida, and reaching all the way to the surface of the oocyte (Albertini et al., 2001; Combelles et al., 2004; review Li and Albertini, 2013). TZPs, containing actin filaments and/or microtubules (Li and Albertini, 2013), connect with the oocyte membrane (oolemma) via intercellular gap junctions and adherens junctions. Thus, the TZPs with open gap junctions provide the basis for germ–somatic cell crosstalk (Li and Albertini, 2013; Clarke, 2017; Wang et al., 2020).
Gap junctions are formed by groups of connexin proteins that interlock to form a pore in the cell membrane. The size of the molecules that pass through is regulated by the size of the pore, which depends on the specific gap junctions involved. The primary connexin found in granulosa cells is CX43 (GJA1), although others are also present (Simon et al., 2006; Wang et al., 2020). The primary connexin in the oocyte is CX37 (GJA4) (Simon et al., 1997). The two types of connexins form heterodimeric gap junctions between the TZPs and the oolemma, enabling the necessary cross-communication and passage of critical molecules that support development and survival of both the oocyte and the granulosa cells. Since Cx37 forms one of the smallest pores in the gap junction family, it is the oocyte that regulates the size of molecules that can pass between these two cell types.
Previously, we observed that Cx37 and Cx43 are present in all follicle stages, including primordial (Rodrigues, 2016; Figures 2A–C). We found Cx43 to be impaired in GDF9 and FSHβ knockout mice [Rodrigues, 2016; Figures 2D,E (GDF9) and Figures 2F,G (FSHβ)]. Moreover, in the absence of Cx37 in female mice, the primordial germ cells fail to achieve meiotic competence. In the adult, there is no formation of ovulatory follicles (Simon et al., 1997, 2006), which reinforces the importance of gap junctions in normal ovary function. TZPs are impaired and cell–cell communication is compromised when both Irx3 and Irx5 are disrupted, leading to POI in mice (Fu et al., 2018).
Figure 2. Connexin 43 cell–cell communication regulation in GDF9 and FSHβ knockout mice model. (A) Connexin 37 expression (green dots) in the oocyte of a secondary (white arrows) and primordial (white arrowhead) follicle of a P20 C57 female mouse ovary. Connexin 43 (green dots) distribution in C57 6 days post-birth (P6) (B) and 42 days postnatal (P42) (C), double stained for actin (white). Connexin 43 (red) distribution with double staining for tubulin (green) in GDF9 heterozygous P6 (D) and homozygous (E) female. Connexin 43 (red) distribution with double staining for tubulin (green) in FSHβ P42, in heterozygous (F) and homozygous (G) female. Bars = 20 μm. Image adapted from Rodrigues (2016).
Most of what is known today in mammalian ovarian development results from morphological descriptions of ovaries at different ages in various models and targeted disruption of genes in the mouse models (Roy and Matzuk, 2006). Transgenic animal models have enabled researchers to identify the regulators of ovarian function and to better understand human disorders, in particular those affecting reproduction.
The transforming growth factor β (TGF-β) super family, which, during development, mediates several cell behaviors, including proliferation, differentiation, ECM production, and cell death, includes some members implicated in follicle growth (Pangas and Matzuk, 2004; Sanfins et al., 2018). One member, Growth differentiation factor 9 (GDF9), is a germ cell-specific factor previously identified in growing oocytes (McGrath et al., 1995; Elvin et al., 1999a). The importance of this growth factor during early folliculogenesis was first demonstrated when Dong et al. (1996) inactivated the mouse GDF9 gene. Female mice lacking a functional GDF9 gene are infertile due to the arrest of folliculogenesis at the primary follicle stage (Dong et al., 1996; Elvin et al., 1999a, b; Sanfins et al., 2018; Figures 2D,E). Interestingly, Wang and Roy (2006) discovered that GDF9 was essential for the formation of PF in the hamster. They also found that FSH indirectly regulates oocyte GDF9 gene expression, implying that FSH regulates oocyte gene expression and primordial follicle formation. Recently, GDF9 expression in oocytes at all stages of development including primordial oocytes was confirmed through single-cell RNA sequencing (Wang et al., 2020).
Bone morphogenic protein 15 (BMP15) is another member of the TGF-β gene family. It is an X-linked gene, encoding an oocyte-specific protein (Dube et al., 1998; Sanfins et al., 2018). BMP15 is expressed in oocytes from primary follicles onward (Dube et al., 1998). It is very closely related with GDF9, in both protein structure and gene expression (Yan et al., 2001). In fact, double mutant mice (BMP15–/–GDF9–/–) show early oocyte loss, whereas BMP15 null females are sub-fertile (Yan et al., 2001). The synergy between both factors was demonstrated by improving oocyte quality and granulosa cell regulation with in vitro produced heterodimers GDF9-BMP15 (cumulin; Peng et al., 2013). BMP15 is also involved in regulating gene expression of Cx43, in human granulosa cells (Chang et al., 2017; Sanfins et al., 2018).
Another oocyte factor, involved in the germ–somatic cell communication is Nobox (Newborn Ovary Homeobox), an oocyte-specific homeobox gene expressed early in development within germ cell clusters, as well as primordial and growing follicles (Suzumori et al., 2002; Rajkovic et al., 2004). In the absence of Nobox, there is an accelerated postnatal oocyte loss (Suzumori et al., 2002). Mutations in the Nobox gene are associated with POI, as well as a delay in germ cell cluster breakdown (Rajkovic et al., 2004; Qin et al., 2007; Lei et al., 2010; Bouilly et al., 2015; Figure 1A).
Although there are more contributors, these oocyte factors are examples of the central importance of oocyte in the bidirectional communication necessary to establish an adequate reserve of ovarian follicles. Most importantly, they illustrate that it is not just one factor, or one cell type involved, but rather a combination of factors and communicating cell types.
Germ cell cluster breakdown is a well-coordinated process and one that demands intercommunication between germ and pre-granulosa cells for efficient PF formation and, with it, establishment of the ovarian follicle reserve (Wang et al., 2020). For example, when Notch 2 is conditionally mutated in granulosa cells, multi-oocyte follicles are formed, thus indicating an impaired germ cluster breakdown. The Notch pathway is an important signaling pathway during embryo development and Notch2 has been reported in both pre-granulosa and granulosa cells (Xu and Gridley, 2013; Wang et al., 2017; Figure 1A). Furthermore, a model of the conditional granulosa cell mutant for Notch2 also showed diminished oocyte apoptosis, increased oocyte survival, but significantly fewer normal PF due to the formation of multi-oocyte follicles (Xu and Gridley, 2013).
As mentioned above, important structures involved in germ–somatic cell interactions are the TZPs, which form gap junctions between the oolemma and the tip of the TPZ extended from the granulosa cell (Simon et al., 2006; Li and Albertini, 2013). The inability to produce pups in the absence of intracellular communication between germ and somatic cells in Cx37 null female mice (Simon et al., 1997), as well as the follicular arrest at primary follicle stage in Cx43 null females (Simon et al., 2006) are significant demonstrations of the importance of this cell–cell communication.
As mentioned previously, loss of IRx3 and IRx5 in female mice caused defects in granulosa cells, particularly in the mis-location of gap junction proteins, resulting in a premature oocyte loss (Fu et al., 2018). Interestingly, Irx5 expression was observed exclusively in granulosa cells while Irx3 alternated between the granulosa and the oocyte during PF assembly (Fu et al., 2018).
It is well known that adult ovary events, such as antral follicle growth, maturation, ovulation, and luteinization are primarily controlled by two gonadotropins: follicle stimulating hormone (FSH) and luteinizing hormone (LH), secreted by the pituitary gland. In turn, the pituitary is controlled by gonadotropin-releasing hormone (GnRH), produced in the hypothalamus (Gougeon, 1996; McGee and Hsueh, 2000). Interestingly, Cx43 expression in granulosa cell is hormonally regulated by both gonadotrophins (FSH and LH) (Granot and Dekel, 1998; Chang et al., 2017; Figures 2A,B,F,G).
FSH receptors (FSHr) are transmembrane G-protein-coupled receptors that localize in granulosa cells in the ovary (Kumar et al., 1997, 1999; Oktay et al., 1997; Burns et al., 2001). Primordial follicle activation and the first phases of follicle development are independent of FSH, as evidenced by the presence of preantral follicles in FSH receptor-null and FSHβ-null female mice (Danilovich et al., 2000; Kumar, 2005). Apparently, loss of FSH receptor or FSHβ did not prevent primordial follicle formation or pre-antral follicle development; however, follicular development was arrested at the preantral stage (Kumar, 2005). There is contradictory evidence for the role of FSH in formation of PF. As mentioned earlier, Wang and Roy (2006) found that FSH activity was essential to PF formation in the hamster, and more recently, it was reported that FSH promotes PF formation in mice through activin stimulation (Lei et al., 2010). This suggests the possibility that other proteins or signaling pathways can compensate for loss of FSH, depending on the research model. Interestingly, 17β-estradiol (E2), which regulates FSH action, was found to inhibit cluster breakdown when added to mouse neonatal ovary cultures (Grive and Freiman, 2015).
Together, oocyte and somatic factors contribute to ovarian follicle reserve establishment and demonstrate how this is a process of cooperation between different cell types within the ovary.
Like PF formation and ovarian follicular reserve assembly, intercellular communication is fundamental for acquisition of oocyte competence (Coticchio et al., 2015; McGinnis et al., 2018).
Briefly, the ovarian follicle reserve is established early in development, and the primordial oocyte, arrested in prophase I of meiosis I, can stay dormant for most of female fertile life. However, periodically, some PFs are recruited to start growing. This follicle growth also initiates oocyte hypertrophy, for storage of macromolecules and organelles in oocyte cytoplasm in order to sustain the first embryonic developmental stages (Li and Albertini, 2013; Clarke, 2017; McGinnis et al., 2018). Following puberty, it is from the pool of growing follicles that some follicles are periodically selected to ovulate a mature oocyte (Coticchio et al., 2015; McGinnis et al., 2018). Communication between oocyte and granulosa cells continues throughout follicle growth, until the LH surge, which triggers the closing of gap junctions and loss of direct communication (Norris et al., 2009). The oocyte nucleus remains arrested at prophase of meiosis I until the LH surge and the closing of gap junctions. This loss of communication triggers changes in oocyte intracellular signaling and the resumption of meiosis, to arrest again at metaphase of meiosis-II (MII) (Rodrigues et al., 2008; McGinnis et al., 2018; Figure 1B).
The stockpiling and follicle growth do not occur if germ–somatic cell interaction is impaired, ultimately leading to female infertility (Li and Albertini, 2013; Clarke, 2017). For example, the GDF9 null mouse ovaries exhibit a reduction of TZPs with altered position, parallel to the oocyte rather than perpendicular, resulting in arrest of follicle development at primary stage and no ovulation (Carabatsos et al., 1998; Sanfins et al., 2018; Figures 1B, 2E,F). In human, GDF9 is essential for cumulus expansion and normal ovulation (Chang et al., 2017). Moreover, a significantly higher number of TZPs were observed in the oocyte–granulosa interface of FSHβ-deficient mice, implying FSH antagonist action toward TPZs in the zona pellucida (Combelles et al., 2004; Clarke, 2017).
This review highlights the importance of germ–somatic cell communication for not only primordial follicle assembly and establishment of the ovarian follicle reserve, but ultimately building a foundation for an optimal female reproductive lifespan.
Although some of these aspects are known, many more are not understood. The future for complete understanding and the possibility of creating solutions to various infertility situations will involve transcriptome analysis and research of uncovered pathways of specific signature genes. Such studies must be complemented with basic cellular research and/or gene disruption. A clear example is the recent uncovering, using RNA sequencing, of specific gene signatures and key pathways for human oocytes and the surrounding granulosa cells. Among these were five different pathways involved in primordial follicle activation, which, if investigated, may shed some light in the treatment of women with POI (Zhang et al., 2018).
PR, DL, LM, and CP contributed to writing of the manuscript. MM and JA contributed to editing and discussion. All authors contributed to the article and approved the submitted version.
This work was partially funded by Fundação para a Ciâcia e Tecnologia (SFRH/BD/6439/2001), Fundação Calouste Gulbenkian (nr78591).
The authors declare that the research was conducted in the absence of any commercial or financial relationships that could be construed as a potential conflict of interest.
Albertini, D. F., Combelles, C. M., Benecchi, E., and Carabatsos, M. J. (2001). Cellular basis for paracrine regulation of ovarian follicle development. Reproduction 121, 647–653. doi: 10.1530/reprod/121.5.647
Bouilly, J., Beau, I., Barraud, S., Bernard, V., Azibi, K., Fagart, J., et al. (2015). Identification of multiple gene mutations accounts for a new genetic architecture of primary ovarian insufficiency. J. Clin. Endocrinol. Metab. 101, 4541–4550. doi: 10.1210/jc.2016-2152
Burns, K. H., Yan, C., Kumar, T. R., and Matzuk, M. M. (2001). Analysis of ovarian gene expression in follicle-stimulating hormone beta knockout mice. Endocrinology 142, 2742–2751. doi: 10.1210/endo.142.7.8279
Byskov, A. G. (1986). Differentiation of mammalian embryonic gonad. Physiol. Rev. 66, 71–117. doi: 10.1152/physrev.1986.66.1.71
Carabatsos, M. J., Elvin, J., Matzuk, M. M., and Albertini, D. F. (1998). Characterization of oocyte and follicle development in growth differentiation factor-9-deficient mice. Dev. Biol. 204, 373–384. doi: 10.1006/dbio.1998.9087
Chang, H.-M., Qiao, J., and Leung, P. C. K. (2017). Oocyte-somatic cell interaction in the human ovary-novel role of bone morphogenetic proteins and growth differentiation factors. Hum. Reprod. Update 23, 1–18. doi: 10.1093/humupd/dmw039
Clarke, H. J. (2017). Regulation of germ cell development by intracellular signaling in the mammalian ovarian follicle. WIREs Dev. Biol. 7:e294. doi: 10.1002/wdev.294
Combelles, C. M., Carabatsos, M. J., Kumar, T. R., Matzuk, M. M., and Albertini, D. F. (2004). Hormonal control of somatic cell oocyte interactions during ovarian follicle development. Mol. Reprod. Dev. 69, 347–355. doi: 10.1002/mrd.20128
Cordeiro, M. H., Kim, S.-Y., Ebbert, K., Duncan, F. E., Ramalho-Santos, J., and Woodruff, T. K. (2015). Geography of follicle formation in the embryonic mouse ovary impacts activation pattern during the first wave of folliculogenesis. Biol. Reprod. 93:88. doi: 10.1095/biolreprod.115.131227
Coticchio, G., Del Canto, M., Renzini, M. M., Guglielmo, M. C., Brambillasca, F., Turchi, D., et al. (2015). Ooocyte maturation: gamete-somatic cell interactions, meiotic resumption, cytoskeletal dynamics and cytoplasmic reorganization. Hum. Reprod. Update 21, 427–454. doi: 10.1093/humupd/dmv011
Danilovich, N., Babu, P. S., Xing, W., Gerdes, M., Krishnamurthy, H., and Sairam, M. R. (2000). Estrogen deficiency, obesity, and skeletal abnormalities in follicle-stimulating hormone receptor knockout (FORKO) female mice. Endocrinology 141, 4295–4308. doi: 10.1210/endo.141.11.7765
Dong, J., Albertini, D. F., Nishimori, K., Kumar, T. R., Lu, N., and Matzuk, M. M. (1996). Growth differentiation factor-9 is required during early ovarian folliculogenesis. Nature 383, 531–535. doi: 10.1038/383531a0
Dube, J. L., Wang, P., Elvin, J., Lyons, K. M., Celeste, A. J., and Matzuk, M. M. (1998). The bone morphogenetic protein 15 gene is X-linked and expressed in oocytes. Mol. Endocrinol. 12, 1809–1817. doi: 10.1210/mend.12.12.0206
Elvin, J. A., Clark, A. T., Wang, P., Wolfman, N. M., and Matzuk, M. M. (1999a). Paracrine actions of growth differentiation factor-9 in the mammalian ovary. Mol. Endocrinol. 13, 1035–1048. doi: 10.1210/mend.13.6.0310
Elvin, J. A., Yan, C., Wang, P., Nishimori, K., and Matzuk, M. M. (1999b). Molecular characterization of the follicle defects in the growth differentiation factor 9-deficient ovary. Mol. Endocrinol. 13, 1018–1034. doi: 10.1210/mend.13.6.0309
Fu, A., Oberholtzer, S. M., Bagheri-Fam, S., Rastetler, R. H., Holdreith, C., Caceres, V. L., et al. (2018). Dynamic expression patterns of Irx3 and Irx5 during germline nest breakdown and primordial follicle formation promote follicle survival in mouse ovaries. PLoS Genet. 14:e1007488. doi: 10.1371/journal.pgen.1007488
Gougeon, A. (1996). Regulation of ovarian follicular development in primates: facts and hypotheses. Endocr Rev 17, 121–155. doi: 10.1210/edrv-17-2-121
Granot, I., and Dekel, N. (1998). Celle-to-cell communication in the ovarian follicle: development and hormonal regulation of the expression of connexin43. Hum. Reprod. 13, 85–97. doi: 10.1093/humrep/13.suppl_4.85
Grive, K. J., and Freiman, R. N. (2015). the development origins of mammalian ovarian reserve. Devlopment 142, 2554–2563. doi: 10.1242/dev.125211
Guigon, C. J., and Magre, S. (2006). Contribution of germ cells to the differentiation and maturation of the ovary: insights from models of germ cell depletion. Biol. Reprod. 74, 450–458. doi: 10.1095/biolreprod.105.047134
Jones, R. L., and Pepling, M. E. (2013). Role of the antiapoptotic proteins BCL2 and MCL1 in the neonatal mouse ovary. Biol. Reprod. 2:46.
Kumar, T. R. (2005). What have we learned about gonadotropin function from gonadotropin subunit and receptor knockout mice? Reproduction 130, 293–302. doi: 10.1530/rep.1.00660
Kumar, T. R., Palapattu, G., Wang, P., Woodruff, T. K., Boime, I., Byrne, M. C., et al. (1999). Transgenic models to study gonadotropin function: the role of follicle-stimulating hormone in gonadal growth and tumorigenesis. Mol. Endocrinol. 13, 851–865. doi: 10.1210/mend.13.6.0297
Kumar, T. R., Wang, Y., Lu, N., and Matzuk, M. M. (1997). Follicle stimulating hormone is required for ovarian follicle maturation but not male fertility. Nat. Genet. 15, 201–204. doi: 10.1038/ng0297-201
Lei, L., Jin, S., Mayo, K. E., and Woodruff, T. K. (2010). The interactions between the stimulatory effect of follicle-stimulating hormone and the inhibitory effect of estrogen on mouse primordial folliculogenesis. Biol. Reprod. 82, 13–22. doi: 10.1095/biolreprod.109.077404
Li, R., and Albertini, D. F. (2013). The road to maturation: somatic cell interaction and self-organization of the mammalian oocyte. Nat. Rev. Mol. Cell Biol. 14, 141–152. doi: 10.1038/nrm3531
McGee, E. A., and Hsueh, A. J. (2000). Initial and cyclic recruitment of ovarian follicles. Endocr. Rev. 21, 200–214. doi: 10.1210/edrv.21.2.0394
McGinnis, L. K., Rodrigues, P., and Limback, D. (2018). “Structural aspects of oocytes maturation,” in Encyclopedia of Reproduction, 2nd Edn, Vol. 3, ed. S. Michael (Amsterdam: Elsevier Inc), 176–182. doi: 10.1016/B978-0-12-801238-3.64445-8
McGrath, S. A., Esquela, A. F., and Lee, S. J. (1995). Oocyte-specific expression of growth / differentiation factor-9. Mol Endocrinol 9, 131–136. doi: 10.1210/me.9.1.131
McLaren, A. (2003). Primordial germ cells in the mouse. Dev. Biol. 262, 1–15. doi: 10.1016/s0012-1606(03)00214-8
Mork, L., Maatouk, D. M., McMahon, J. A., Guo, J. J., Zhang, P., McMahon, A. P., et al. (2012). Temporal differences in granulosa cell specification in the ovary reflect distinct follicle fates in mice. Biol. Reprod. 86:37.
Norris, N. P., Ratzan, W. J., Freudzon, M., Mehlmann, L. M., Krall, J., Movsesian, M. A., et al. (2009). Cyclic GMP from the surrounding somatic cells regulates cyclic AMP and meiosis in the mouse oocyte. Development 136, 1869–1878. doi: 10.1242/dev.035238
Oktay, K., Briggs, D., and Gosden, R. G. (1997). Ontogeny of follicle-stimulating hormone receptor gene expression in isolated human ovarian follicles. J. Clin. Endocrinol. Metab. 82, 3748–3751. doi: 10.1210/jc.82.11.3748
Pangas, S. A., and Matzuk, M. M. (2004). Genetic models for transforming growth factor beta superfamily signaling in ovarian follicle development. Mol. Cell. Endocrinol. 225, 83–91. doi: 10.1016/j.mce.2004.02.017
Peng, J., Li, Q., Wigglesworth, K., Rangarajan, A., Kattamuri, C., Peterson, R. T., et al. (2013). Growth differentiation factor 9:bone morphogenetic protein 15 heterodimers are potent regulators of ovarian functions. Proc. Natl. Acad. Sci. U.S.A. 110, E776–E785. doi: 10.1073/pnas.1218020110
Pepling, M. E., and Spradling, A. C. (1998). Female mouse germ cells form synchronously dividing cysts. Development 125, 3323–3328.
Pepling, M. E., and Spradling, A. C. (2001). Mouse ovarian germ cell cyst undergo programmed breakdown to form primordial follicles. Dev. Biol. 2345, 339–351. doi: 10.1006/dbio.2001.0269
Qin, Y., Choi, Y., Zhao, H., Simpson, J. L., Chen, Z. J., and Rajkovic, A. (2007). NOBOX homeobox mutation causes premature ovarian failure. Am. J. Hum. Genet. 81, 576–581. doi: 10.1086/519496
Rajkovic, A., Pangas, S. A., Ballow, D., Suzumori, N., and Matzuk, M. M. (2004). NOBOX deficiency disrupts early folliculogenesis and oocyte-specific gene expression. Science 305, 1157–1159. doi: 10.1126/science.1099755
Rodrigues, P. (2016). Revisiting Germ Cell Dynamics and Ovarian Follicle Assembly in the Mouse Model. Ph.D. thesis. Lisbon: Lisbon University Press.
Rodrigues, P., Limback, D., McGinnis, L. K., Plancha, C. E., and Albertini, D. F. (2009). Multiple mechanisms of germ cell loss in the perinatal mouse ovary. Reproduction 137, 709–720. doi: 10.1530/rep-08-0203
Rodrigues, P., Limback, D., McGinnis, L. K., Plancha, C. E., and Albertini, D. F. (2008). Oogenesis: prospects and challenges for the future. J. Cell. Physiol. 216, 355–365. doi: 10.1002/jcp.21473
Roy, A., and Matzuk, M. M. (2006). Deconstructing mammalian reproduction: using knockouts to define fertility pathways. Reproduction 131, 207–219. doi: 10.1530/rep.1.00530
Sanfins, A., Rodrigues, P., and Albertini, D. F. (2018). GDF-9 and BMP-15 direct the follicle symphony. J. Assist. Reprod. Genet. 35, 1741–1717. doi: 10.1007/s10815-018-1268-4
Simon, A. M., Chen, H., and Jackson, C. L. (2006). Cx37 and Cx43 localize to zona pellucida in mouse ovarian follicles. Cell Commun. Adhes. 13, 61–77. doi: 10.1080/15419060600631748
Simon, A. M., Goodenough, D. A., Li, E., and Paul, D. L. (1997). Female infertility in mice lacking connexin 37. Nature 385, 525–529. doi: 10.1038/385525a0
Suzumori, N., Yan, C., Matzuk, M. M., and Rajkovic, A. (2002). Nobox is a homeobox-encoding gene preferentially expressed in primordial and growing oocytes. Mech. Dev. 111, 137–141. doi: 10.1016/s0925-4773(01)00620-7
van den Hurk, R., and Zhao, J. (2005). Formation of mammalian oocytes and their growth, differentiation and maturation within ovarian follicles. Theriogenology 63, 1717–1751. doi: 10.1016/j.theriogenology.2004.08.005
Wang, C. A., and Roy, S. K. (2006). Expression of growth differentiation factor 9 in the oocytes is essential for development of primordial follicles in the hamster ovary. Endocrinology 147, 1725–1734. doi: 10.1210/en.2005-1208
Wang, C., Zhou, B., and Xia, G. (2017). Mechanisms controlling germline cyst breakdown and primordial follicle formation. Cell Mol. Life Sci. 74, 2547–2566. doi: 10.1007/s00018-017-2480-6
Wang, J.-J., Ge, W., Zhai, Q.-Y., Liu, J.-C., Sun, X.-W., Liu, W.-X., et al. (2020). Single-cell transcriptome landscape of ovarian cell during follicle assembly in mice. PLoS Biol. 18:e3001025. doi: 10.1371/journal.pbio.3001025
Xu, J., and Gridley, T. (2013). Notch2 is required in somatic cells for breakdown of ovarian germ-cell nest and formation of primordial follicles. BMC Biol. 11:13. doi: 10.1186/1741-7007-11-13
Yan, C., Wang, P., DeMayo, J., DeMayo, F. J., Elvin, J. A., Carino, C., et al. (2001). Synergistic roles of bone morphogenic protein 15 and growth differentiation factor 9 in ovarian function. Mol. Endocrinol. 15, 854–866. doi: 10.1210/mend.15.6.0662
Zhang, Y., Yan, Z., Qin, Q., Nisenblat, V., Chang, H.-M., Yu, Y., et al. (2018). Transcriptome landscape of human folliculogenesis reveals oocyte and granulosa cell interactions. Mol. Cell 72, 1021–1034. doi: 10.1016/j.molcel.2018.10.029
Zheng, W., Zhang, H., and Liu, K. (2014b). The two classes of primordial follicles in the mouse ovary: their development, physiological functions and implications for future research. Mol. Hum. Reprod. 20, 286–292. doi: 10.1093/molhr/gau007
Keywords: primordial follicle, follicle reserve, germ–somatic cells interaction, germ cell factor, somatic cell factor
Citation: Rodrigues P, Limback D, McGinnis L, Marques M, Aibar J and Plancha CE (2021) Germ–Somatic Cell Interactions Are Involved in Establishing the Follicle Reserve in Mammals. Front. Cell Dev. Biol. 9:674137. doi: 10.3389/fcell.2021.674137
Received: 28 February 2021; Accepted: 11 May 2021;
Published: 14 June 2021.
Edited by:
Rafael A. Fissore, University of Massachusetts Amherst, United StatesReviewed by:
Karin Lykke-Hartmann, Aarhus University, DenmarkCopyright © 2021 Rodrigues, Limback, McGinnis, Marques, Aibar and Plancha. This is an open-access article distributed under the terms of the Creative Commons Attribution License (CC BY). The use, distribution or reproduction in other forums is permitted, provided the original author(s) and the copyright owner(s) are credited and that the original publication in this journal is cited, in accordance with accepted academic practice. No use, distribution or reproduction is permitted which does not comply with these terms.
*Correspondence: Patrícia Rodrigues, cGF0cmljaWEucm9kcmlndWVzQHVsdXNvZm9uYS5wdA==; cGF0cmljaWEucm9kcmlndWVzQGNlbWVhcmUucHQ=
Disclaimer: All claims expressed in this article are solely those of the authors and do not necessarily represent those of their affiliated organizations, or those of the publisher, the editors and the reviewers. Any product that may be evaluated in this article or claim that may be made by its manufacturer is not guaranteed or endorsed by the publisher.
Research integrity at Frontiers
Learn more about the work of our research integrity team to safeguard the quality of each article we publish.