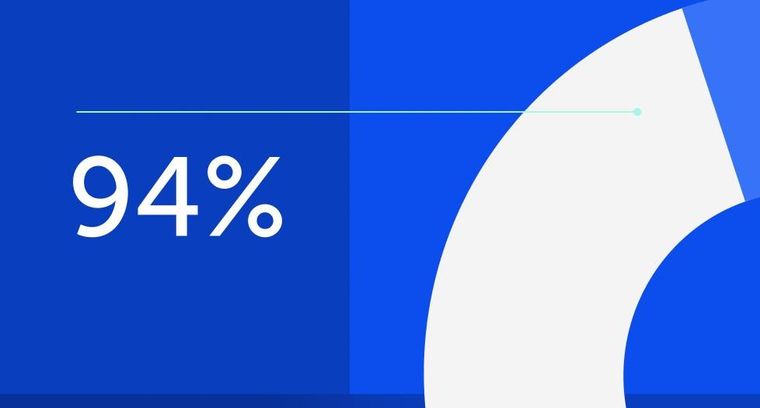
94% of researchers rate our articles as excellent or good
Learn more about the work of our research integrity team to safeguard the quality of each article we publish.
Find out more
REVIEW article
Front. Cell Dev. Biol., 13 May 2021
Sec. Stem Cell Research
Volume 9 - 2021 | https://doi.org/10.3389/fcell.2021.674084
This article is part of the Research TopicMesenchymal Stromal Cell Therapy for Regenerative MedicineView all 38 articles
Bone regeneration is a complex, well-orchestrated process based on the interactions between osteogenesis and angiogenesis, observed in both physiological and pathological situations. However, specific conditions (e.g., bone regeneration in large quantity, immunocompromised regenerative process) require additional support. Tissue engineering offers novel strategies. Bone regeneration requires a cell source, a matrix, growth factors and mechanical stimulation. Regenerative cells, endowed with proliferation and differentiation capacities, aim to recover, maintain, and improve bone functions. Vascularization is mandatory for bone formation, skeletal development, and different osseointegration processes. The latter delivers nutrients, growth factors, oxygen, minerals, etc. The development of mesenchymal stromal cells (MSCs) and endothelial progenitor cells (EPCs) cocultures has shown synergy between the two cell populations. The phenomena of osteogenesis and angiogenesis are intimately intertwined. Thus, cells of the endothelial line indirectly foster osteogenesis, and conversely, MSCs promote angiogenesis through different interaction mechanisms. In addition, various studies have highlighted the importance of the microenvironment via the release of extracellular vesicles (EVs). These EVs stimulate bone regeneration and angiogenesis. In this review, we describe (1) the phenomenon of bone regeneration by different sources of MSCs. We assess (2) the input of EPCs in coculture in bone regeneration and describe their contribution to the osteogenic potential of MSCs. We discuss (3) the interaction mechanisms between MSCs and EPCs in the context of osteogenesis: direct or indirect contact, production of growth factors, and the importance of the microenvironment via the release of EVs.
Bone regeneration is a complex, well-orchestrated process based on the interactions between osteogenesis and angiogenesis (Fröhlich, 2019), observed in both physiological and pathological situations. However, spontaneous bone regeneration could be overwhelmed in several complex clinical conditions (e.g., large bone defect subsequent to trauma, infection, tumor resection and skeletal abnormalities, or compromised regenerative process due to avascular necrosis, atrophic non-unions and osteoporosis) (Dimitriou et al., 2011). Tissue-engineering offers new approaches to foster bone regeneration (Bouland et al., 2020). Bone regeneration requires four elements: a cell source, a scaffold, tissue-inducing factors (signaling factors) and mechanical stimulation (Perez et al., 2018).
Since 2001, when the first three patients were successfully treated for bone defects with expanded autologous bone marrow mesenchymal stromal cells (BM-MSCs) (Quarto et al., 2001), numerous studies have been conducted (Watson et al., 2014; Perez et al., 2018). Different mesenchymal stromal cells (MSCs) sources have been studied in bone tissue engineering in both clinical and preclinical settings (Perez et al., 2018). However, successful bone regeneration relies on vascularization (Rouwkema and Khademhosseini, 2016; Filipowska et al., 2017). Indeed, insufficient vascularization during the initial phase, after in vivo implantation, could lead to insufficient cell integration and death (Pang et al., 2013; Grosso et al., 2017; Fröhlich, 2019). A functionally perfused vascular network also mediates the recruitment of osteoprogenitors, hematopoietic stem cells (HSCs) and immune cells, playing a significant role in tissue regeneration and remodeling (Grosso et al., 2017). Furthermore, vascularization participates in structural and functional reconstruction (Pang et al., 2013).
Different strategies have been undertaken to introduce the vasculature into tissue-engineered constructs, including scaffolds, growth factors, in vitro or in vivo graft prevascularization and coculture (Liu et al., 2015). MSCs and endothelial progenitor cells (EPCs) coculture aims to obtain a synergistic effect in terms of angiogenesis and bone regeneration (Sun et al., 2016). EPCs have been shown by many researchers to be effective in cell-based therapies, improving vascularization for a variety of applications (Atesok et al., 2010). EPCs stimulate angiogenesis and osteogenesis by the secretion of trophic factors (Pang et al., 2013; Jia et al., 2019).
In addition, the therapeutic potential of MSCs, through the production of trophic factors, could modulate the host tissue microenvironment and subsequently facilitate the regenerative process (Li et al., 2012). Recently, Jia et al. (2019) outlined the importance of the microenvironment, particularly extracellular vesicles (EVs), which are considered an important component of cellular paracrine secretion. EVs secreted by EPCs markedly accelerate bone regeneration by stimulating angiogenesis (Jia et al., 2019). In this review, we will first describe the different MSCs sources used to generate bone and analyze relevant clinical studies. We will evaluate the contribution of MSC/EPC coculture to bone regeneration. Finally, we will describe herein the different interactions between MSCs and EPCs. These elements will determine the benefits of MSC/EPC coculture in bone regeneration and the related interactions between these two cell populations.
Mesenchymal stromal cells are multipotent adult stem cells exhibiting multilineage differentiation potential (Squillaro et al., 2016). MSCs are defined by the three following criteria: adherence to plastic, specific surface antigen (Ag) expression, and multipotent differentiation potential, according to the International Society for Cellular Therapy (ISCT) (Figure 1; Dominici et al., 2006). They exhibit great differentiation potential into many different types of tissue lineages, including bone (osteoblasts), cartilage (chondrocytes), muscle (myocytes), and fat (adipocytes). MSCs have been isolated from multiple tissues: bone marrow (BM), skeletal muscle tissue, adipose tissue (AT), synovial membranes, saphenous veins, dental pulp, periodontal ligaments, cervical tissue, Wharton’s jelly, umbilical cords, umbilical cord blood, amniotic fluid, placentae, etc. (Squillaro et al., 2016). In 1995, the first MSCs clinical study used autologous, culture-expanded BM-MSCs in patients with hematological malignancies, demonstrating safety with no reports of adverse events (Koç et al., 2000). Since this first trial, over 3,000 patients enrolled in more than 100 clinical trials have met safety endpoints, with no serious adverse events reported to date (Watson et al., 2014). Since then, MSCs have been studied in numerous clinical studies for representative diseases, including organ transplantation, diabetes, inflammatory, hematological, cardiovascular, auto-immune, cartilage, and bone disease (Squillaro et al., 2016). Clinical studies promoted non-union fracture healing (Hernigou et al., 2015), accelerated fracture healing (Kim et al., 2009) or bone regeneration in bone tissue defect (Pavan Kumar et al., 2016) and other pathological conditions (Manimaran et al., 2014, 2016; Bouland et al., 2020). The clinical applications of the different sources of MSCs are detailed in Table 1.
The BM is the first studied source of MSCs. Several MSC-based cell therapy modalities have been developed with or without cell culturing and with or without matrix. BM-MSCs have significant bone repair and regeneration potential. However, BM-MSC in vitro culture requires time, expensive manufacturing costs, and good manufacturing practice (GMP) facilities, and it presents a contamination risk. These factors render the cells requiring in vitro amplification unsuitable for clinical use. Moreover, during culture, BM-MSCs are exposed to hyperoxic conditions, leading to elevated levels of intracellular reactive oxygen species (ROS) production. Robust assays are required to monitor biological changes that could reduce the efficacy of cell-based therapy. Relative to BM-MSCs, bone marrow mononuclear cells (BM-MNCs) are a heterogeneous cell population that includes MSCs, EPCs, HSCs, and lymphocytes. BM-MNCs can be directly applied bypassing the in vitro expansion process. This can save time, expense and avoid the declining differentiation and migration ability caused by in vitro culture, as well as contamination risk and other uncertain factors, such as genetic aberrations not associated with malignant transformation or in vitro transformed phenotype (Capelli et al., 2014; Du et al., 2020). Recently, Du et al. (2020) compared and observed that fresh BM-MNCs promote more bone regeneration than in vitro expanded BM-MSCs in an animal model. Moreover, the grafts containing the BM-MNCs were better mineralized, with collagen arrangement and microbiomechanical properties similar to those of native tibia (Du et al., 2020). Furthermore, Sun et al. (2009) highlighted that BM-MNCs increase both neovascularization and bone regeneration in an animal model of osteonecrosis of the hip (ONFH) after core decompression.
In 2006, a first patient suffering from compartment syndrome and bone non-union was treated by autologous BM-MNC implantation for therapeutic angiogenesis and subsequent bone regeneration. Four weeks later, angiography showed a marked increase in collateral vessels surrounding the tibial fracture, and union was completed 6 months later. The authors suggested that the increased blood flow helped maintain the peripheral tissue structural and functional integrity and subsequently facilitated bone regeneration (Umemura et al., 2006). Few years later, in a randomized controlled clinical study, Liebergall et al. (2013) assessed that autologous fresh purified CD105+ BM cells (108) injected into a reduced fracture together with platelet-rich plasma (PRP) and allograft demineralized bone matrix significantly reduce time to union. Diabetic patients treated for non-union ankle fractures with BM-MSCs showed a higher rate of successful unions and far fewer complications compared to diabetics receiving iliac crest bone graft as a standard of care. Treatment with percutaneous bone marrow aspirate concentrate (BMAC) promoted non-union healing in 70/86 diabetic patients (82.1%) with a low percentage of complications. In comparison, treatment with bone iliac crest autografts promoted non-union healing in only 53/86 diabetic patients (62.3%). Major complications were assessed in the control group: five amputations, 11 osteonecroses of the fracture wound edge and 17 infections (Hernigou et al., 2015).
In parallel, Hernigou and Beaujean (2002) and Hernigou et al. (2009) published several papers related to ONFH treatment with autologous BMAC with good results. In 2002, 116 patients suffering from ONFH (189 hips) were treated with core decompression and BMAC (Hernigou and Beaujean, 2002). During the follow-up (5–11 years), only 18% (34) of the hips and 20% (22) of the patients, required total hip replacement at a mean of 26 months after decompression and autologous BMAC grafting. In 2009, 342 patients suffering from stage I and II ONFH (534 hips) were treated with core decompression and autologous bone marrow grafting (Hernigou et al., 2009). The patients were followed up from eight to 18 years. Hip replacement was performed in 94/534 cases (17.6%) after collapse. The authors suggested that this procedure should be advised for symptomatic ONFH without collapse. Cai et al. (2014) treated also patients suffering from ONFH. However, the authors injected an association of autologous BM-MNCs and allogeneic umbilical cord mesenchymal stromal cells (UC-MSCs) by arterial perfusion (Cai et al., 2014). The patients were followed for 16.9 months. The Harris scores evaluating pain relief, joint function, walking distance, and image changes were significantly improved (P < 0.05) 3, 6, and 12 months after the procedure. Twenty-eight patients (93.3%) showed hip pain relief, 26 (86.7%) showed improved joint function, and 26 patients (86.7%) benefited from an extended walking distance.
Cella et al. (2011) treated a stage III bisphosphonate-related osteonecrosis of the jaw (BRONJ) with autologous BM-MNCs and PRP. Fifteen months after the procedure, medical imaging assessed bone regeneration and concentric ossification. Soft and hard tissue were assessed during the 30-month uneventful follow-up. Manimaran et al. (2014) treated a case of osteoradionecrosis (ORN) with the association of autologous BMAC, beta-tricalcium phosphate (βTCP) and hydroxyapatite (HA). The treated patient stayed asymptomatic during the follow-up, and moreover, a complete bone remodeling was noticed after 1 year. Pavan Kumar et al. (2016) successfully treated 30 patients with soft or hard tissue defects in the oral and maxillofacial areas with autologous BM-MNCs and observed beneficial effects in bone regeneration and soft tissue wound healing.
Notwithstanding, the regenerative potential of therapies based on expanded BM-MSCs is being tested clinically for the treatment of bone defects, fractures and osteonecrosis (Grayson et al., 2015). Quarto et al. (2001) were the first to report the use of cultured BM-MSCs combined intraoperatively with HA scaffolds to fill large bone defects in three patients. Then, the study of Marcacci et al. (2007) highlighted the long-term durability of bone regeneration achieved by bone engineering. The authors confirmed the use of culture-expanded osteoprogenitor cells in conjunction with porous bioceramics to improve the repair of critical-sized long bone defects. This technique was successfully used by Morishita et al. (2006) in three patients with benign bone tumors. The use of cultured BM-MSCs in association with PRP improved the healing index during lengthening of 51 femurs or tibias compared to 60 controls (Kitoh et al., 2009). A multicenter, randomized, clinical trial reported that autologous cultured osteoblast injection accelerates significantly fracture healing (Kim et al., 2009).
In a randomized trial of 100 patients, Zhao et al. (2012) treated 50 patients suffering from ONFH with culture-expanded autologous BM-MSCs. The authors followed the patients for 5 years. Only two of the 53 BM-MSC-treated hips progressed and underwent vascularized bone grafting. BM-MSCs treatment significantly improved the Harris hip score and decreased the volume of the necrotic lesion. In a European multicenter clinical trial, the surgical implantation of GMP-expanding BM-MSCs in combination with bioceramic granules was feasible and safe for the treatment of fracture non-union and ONFH. Moreover, 26/28 treated patients were radiologically healed at 1 year (Gómez-Barrena et al., 2019).
The AT is another studied source of MSCs. Described first by Zuk et al. (2001) adipose tissue-mesenchymal stromal cells (AT-MSCs) are easily harvested by lipoaspiration in large quantities with minimal discomfort. AT contains a higher stromal cell to volume ratio than BM (Zuk et al., 2002; Mesimäki et al., 2009). The Stromal vascular fraction (SVF) processed from excised adipose tissue, by mechanical or enzymatic isolation, is a heterogeneous cell population containing not only adipose stromal and hematopoietic stem and progenitor cells but also endothelial cells, erythrocytes, fibroblasts, lymphocytes, monocytes/macrophages and pericytes (Zuk et al., 2001; Bourin et al., 2013). AT-MSCs exhibit similar properties to BM-MSCs. However, numerous features distinguish these two cell populations. AT-MSCs display stronger adipogenic, similar chondrogenic and lower osteogenic differentiation potential (Xu et al., 2017). Moreover, AT-MSCs display significant proangiogenic potential in vivo (Rehman et al., 2004). AT-MSCs may play an important role in bone tissue regeneration, and their secretome includes several endocrine and pro-angiogenic factors able to induce bone activity (Paduano et al., 2017).
Lendeckel et al. (2004) and Mesimäki et al. (2009) observed bone regeneration in a clinical setting. Lendeckel et al. (2004) treated a patient suffering from a calvarial defect with a combination of macropore sheets, cancellous bone, adipose-derived mononuclear cells (containing AT-MSCs) and autologous fibrin glue. The computed tomography (CT) scan performed 3 months after the operation showed marked ossification in the defect areas (Lendeckel et al., 2004). Later, Mesimäki et al. (2009) reconstructed a maxillary defect with autologous expanded AT-MSCs and recombinant human (rh) BMP-2 and βTCP granules inside a muscle-free flap. After 36 months of follow-up, the dental implants were osteointegrated, and the bony defect was reconstructed (Mesimäki et al., 2009). Thesleff et al. (2011) assessed bone regeneration using the combination of AT-MSCs and βTCP in four patients with critical-size calvarial defects.
Sándor et al. (2013, 2014) successively published two papers tracking their experience. First, the authors achieved a 10-cm anterior mandibular reconstruction with expanded AT-MSCs, βTCP and rhBMP-2. Histomorphometric analysis showed that the recovered bone core consisted of woven bone (36.7%), osteoids (32.4%), fibrous tissue (23.3%), and residual scaffolds (8.6%) (Sándor et al., 2013). Thereafter, 13 maxillofacial cases were treated with either bioactive glass or βTCP scaffolds seeded with expanded AT-MSCs and, in some cases, with the addition of rhBMP-2. Follow-up time ranged from 12 to 52 months. Successful integration of the construct into the surrounding skeleton was noted in 10 of the 13 cases (Sándor et al., 2014).
AT-MSCs are isolated as part of the aqueous fraction derived from enzymatic digestion of AT. This fraction, known as the SVF, contains AT-MSCs, EPCs, endothelial cells (ECs), macrophages, smooth muscle cells, lymphocytes, pericytes, and adipocytes, among others. SVF displays similar properties to AT-MSCs, such as immunomodulation, anti-inflammation, and angiogenesis, but the distinctive, heterogeneous cellular composition of SVF may be responsible for the better therapeutic outcome observed in different animal studies (Bora and Majumdar, 2017).
Jurgens et al. (2013) noticed in an animal model that expanded AT-MSCs and fresh SVF exhibited similar osteogenic potential. Furthermore, fresh SVF can induce neovascularization through dynamic reassembly of blood endothelial cells (Koh et al., 2011).
In 2015, the first sternum reconstruction using SVF in conjunction with plating techniques was performed. Symptoms improved commensurate with healed areas of non-union 3 months after the operation and still maintained 3 months later. The association of the different cell populations contained in the SVF was suspected to act synergistically (Khalpey et al., 2015). In 2016, eight patients with low-energy proximal humeral fractures were treated with fresh autologous SVF loaded onto ceramic granules within fibrin gel along with standard open reduction and internal fixation (Saxer et al., 2016). Interestingly, the authors suggested that SVF, without expansion or exogenous priming, could spontaneously form bone tissue and vessel structures within a fracture microenvironment. Prins et al. (2016) and Farré-Guash et al. (2018) evaluated bone regeneration for maxillary sinus floor elevation (MSFE) with SVF seeded on either βTCP or biphasic calcium phosphate (BCP) carriers. Bone and osteoid percentages were higher, supported by more mature angiogenesis in the core of the grafted material, in study biopsies (SVF supplemented) than in control biopsies, particularly in βTCP-treated patients (Prins et al., 2016). Furthermore, the vessel distribution was more homogeneous in the study biopsies (Farré-Guash et al., 2018).
In 2016, a patient with an ameloblastoma was cared for with a combination of expanded autologous dental pulp MSCs (DP-MSCs), SVF, PRP, PRF, and βTCP. Bone regeneration was confirmed through medical imaging. The authors observed no recurrence during the one and a half year follow-ups (Manimaran et al., 2016). Two patients suffering from medication-related osteonecrosis of the jaw (MRONJ) were treated with a combination of L-PRF and fresh SVF in a one-step procedure. The follow-up was uneventful, and based on CT scans during the 18 months of follow-up, bone regeneration was noticed (Bouland et al., 2020).
Perinatal derivatives (PnD) consist of birth-associated tissues, cells isolated thereof, and the factors secreted (Silini et al., 2019). The term “perinatal” refers to birth-associated tissues obtained from term placentas and fetal annexes. It refers to amniotic/amnionic membrane, chorionic membrane, chorionic villi, umbilical cord (including Wharton’s jelly), basal plate (including maternal and fetal cells), and amniotic fluid. The term “derivatives” consists of the isolated cells from placental tissues and the factors released by these cells. All the perinatal derivatives-mesenchymal stromal cells (PnD-MSC) present several advantages: a painless and non-invasive harvesting procedure, a low risk of infection and low immunogenicity, and sufficient experience for their clinical application. Furthermore, these MSCs meet the requirements of ethical issues and are very effective at differentiating into osteoblasts (Jäger et al., 2009; Ding et al., 2015). Moreover, UC-MSCs are less differentiated (Zajdel et al., 2017), have higher proliferative potential than adult MSCs (Subramanian et al., 2015) and have higher clonogenic abilities (Laroye et al., 2019) and a slower aging rate (Batsali et al., 2017). Chen et al. (2013) compared the osteogenic properties of BM-MSCs and UC-MSCs in vivo. The authors noticed that similar mineral densities and amounts of bone and vessels were assessed after seeding BM-MSCs or UC-MSCs in an animal calvarial defect model. Hao et al. (2014) observed that intrabone marrow injection of UC-MSCs also promoted new bone formation in a peri-implant defect animal model after immediate implantation. In addition, increased expression of osteogenic genes [alkaline phosphatase (ALP), type 1 collagen and osteocalcin] and a larger trabecular bone area in an osteoporotic animal model were demonstrated after external induction of UC-MSCs (Hendrijantini et al., 2019). In 2017, the first patient suffering from an infected non-union right femoral shaft fracture with significant bone loss was successfully treated with a combination of allogeneic UC-MSCs, bone morphogenetic protein-2 (BMP-2), hydroxyapatite (HA), and mechanical stabilization using the Masquelet technique (Dilogo et al., 2017). Three years later, a case of vertebral defect was successfully treated with expanded UC-MSCs and HA (Rahyussalim et al., 2020).
Recently, the study spectrum of dental tissue MSCs has been broadened to bone regeneration. Gronthos et al. (2000) isolated dental pulp MSCs (DP-MSCs) in 2000. This source has the advantage of being easy to harvest (Huang et al., 2009). The DP-MSCs and BM-MSCs have comparable properties. Laino et al. (2005) obtained in vitro living autologous fibrous bone (LAB) tissue after DP-MSC differentiation. After transplantation into immunocompromised rats, lamellar bone with osteocytes within the bone and osteocytes surrounding the trabecula were highlighted. Thereafter, d’Aquino et al. (2007) obtained vascularized bone tissue for the first time. The authors noticed that DP-MSCs synergically differentiate into osteoblasts and endotheliocytes and that flk-1 exerts a pivotal role in coupling osteoblast and endotheliocyte differentiation (d’Aquino et al., 2007). In parallel, MSCs from other dental tissues were identified: exfoliated deciduous tooth stem cells (SHEDs) (Miura et al., 2003), periodontal ligament stem cells (PDLSCs) (Seo et al., 2004), apical papilla stem cells (APSCs) (Sonoyama et al., 2006), dental follicle stem cells (DFSCs) (Morsczeck et al., 2005), and gingival mesenchymal stromal cells (GMSCs) (Mitrano et al., 2010). The relationship between different dental tissue MSCs remains unclear (Huang et al., 2009). All dental tissue MSCs have a similar capacity to differentiate into other cell lineages but are not identical to BM-MSCs (Huang et al., 2009). Different studies noted that SHEDs, DP-MSCs and BM-MSCs have similar osteogenic properties in vivo (Nakajima et al., 2018; Lee et al., 2019). Nevertheless, Lee et al. (2019) observed that BM-MSCs had higher osteogenic differentiation ability in vitro. Manimaran et al. (2014) successfully treated a patient suffering from ORN with the combination of allogeneic expanded DP-MSCs, tricalcium phosphate and PRP after conventional method failure. The 6-months follow-up was uneventful. A periodic orthopantogram (OPG) revealed appreciable bone formation (Manimaran et al., 2014). Two years later, a patient with an ameloblastoma was cared for with the combination of expanded autologous DP-MSCs, SVF, PRP, PRF, and βTCP. The authors observed no recurrence during the one and a half year follow-ups. Bone regeneration was confirmed through medical imaging (Manimaran et al., 2016). Since then, different approaches have been studied to improve bone regeneration using DP-MSCs, such as a specific scaffold (Petridis et al., 2015), the cell sheet technique (Fujii et al., 2018), and inflammation (Tomasello et al., 2017). However, most of the studies are preclinical studies. Petridis et al. (2015) observed bone formation in a rat calvarial defect model using human DPMSCs in a hydrogel scaffold. Tomasello et al. (2017) observed in vitro that inflamed dental tissue-derived MSCs showed a higher proliferative and osteogenic potential. Furthermore, inflammation increases several actin-depolymerizing factors (ADFs) and heat shock proteins (HSPs), playing a role in bone regeneration (Tomasello et al., 2017). To bypass the need for a scaffold, Fujii et al. (2018) regenerated bone through DPMSC cell sheet technology in association with a helioxanthin derivative in vivo.
The following section will describe MSC/EPC coculture in bone regeneration, benefits in angiogenesis and osteogenesis and preclinical applications.
Successful bone regeneration requires neovascularization along with an efficient blood supply (Rouwkema and Khademhosseini, 2016; Wu et al., 2019). Neovascularization is achieved through vasculogenesis and angiogenesis. Vasculogenesis consists of de novo blood vessel formation by the differentiation and assembly of angioblastic progenitor cells during embryogenesis. Postnatal vasculogenesis, defined as the incorporation of circulating EPCs into the microvascular endothelium of newly developing microvessels, plays a major contribution to adult neovascularization. Angiogenesis consists of new blood vessels sprouting from the preexisting vasculature (Rouwkema and Khademhosseini, 2016; Wu et al., 2019).
First isolated by Asahara et al. (1997), EPCs are a population of unipotent progenitors displaying self-renewability, clonogenicity and differentiation capacity that can be easily isolated from peripheral blood-derived from several sources such as BM, spleen, umbilical cord, liver, kidney and other sources (Stolk and van der Geest, 1998; Goerke et al., 2015; Rana et al., 2018). EPCs stimulate angiogenesis and osteogenesis through soluble factor release (BMP-1, 2, 3, 6, 7, and 8, VEGF, TGF-β, etc.) and thus play a significant role in bone formation (Liu et al., 2012; Pang et al., 2013; Jia et al., 2019; Wu et al., 2019). Recent results have highlighted that EPCs increase angiogenesis and osteogenesis by incorporating themselves into newly formed blood vessels, mainly by recruiting resident MSCs and EPCs in the bone forming site (Tamari et al., 2020).
Coculture of MSCs and EPCs aims to obtain a synergistic effect in terms of angiogenesis and bone regeneration (Sun et al., 2016). Usami et al. (2009) observed that coculture of BM-MSC/peripheral blood mononuclear cell-EPCs (PBMC-EPCs) coculture is beneficial for bone tissue engineering. The authors highlighted that even if the soluble factors secreted by EPCs did not facilitate osteogenic differentiation, the newly formed vasculature may enhance bone regeneration. Greater bone formation, more mature trabecular bone formation, and higher neovascularization were highlighted in the coculture (Usami et al., 2009). Positive synergy between BM-MSCs and PBMC-EPCs in vivo translated into improved early vascularization followed by enhanced bone regeneration (Seebach et al., 2010; Zigdon-Giladi et al., 2015). Interestingly, Seebach et al. (2012) noticed in a BM-MSC/PBMC-EPC combination in vivo that EPCs improved vascularization in a bone defect directly through vessel formation and indirectly through the release of growth factors, such as VEGF, recruiting host EPCs. Geuze et al. (2009) observed, in an MSC/EPC coculture in vitro, that both cell types enhanced proliferation. However, BM-MSCs/BM-EPCs at different ratios (1/4;1/1;4/1) did not show a higher bone content than the monoculture of BM-MSCs when keeping the total numbers of seeded cells constant in vivo (Geuze et al., 2009). EPCs and MSCs can be cocultured in vitro on cancellous bone under osteogenic conditions. Early EPCs maintain endothelial differentiation. Coculturing PBMC-EPCs with BM-MSCs stabilizes the latter’s collagen-1α gene expression, which might be beneficial in bone healing (Henrich et al., 2013). Li and Wang (2013) observed that coculture of BM-MSCs and BM-EPCs in direct contact at a ratio of 1/1 can induce upregulation of angiogenic growth factors such as VEGF and IGF-1 and generate a favorable environment for angiogenesis, which in turn favors osteogenesis. 3D cell constructs can be fabricated in vitro by incorporating human umbilical vein endothelial cells (HUVECs) into MSC constructs, promoting the survival rate and osteogenic differentiation of MSCs (Sasaki et al., 2015). Through the use of 3D coculture systems, Genova et al. (2016) showed that endothelial cells (ECs) are able to stimulate the osteodifferentiation of MSCs, enhancing bone production. According to this study, a virtuous loop between MSCs and ECs seems central for the osteogenesis process (Genova et al., 2016). The study of Bidarra et al. (2011) demonstrated that by coculturing MSCs with HUVECs, there was not only an enhancement of osteogenic differentiation but also a significant increase in MSC proliferation. Li et al. (2014) demonstrated that a coculture of peripheral blood (PB)-CD34+ cells and BM-MSCs with HA could more efficiently promote bony regeneration than MSC composites alone in a model of a calvarial critical-size defect in rabbits. To recreate a biological “regenerative” microenvironment, the authors constructed cell sheets mixing MSCs and PB-CD34+. This technology has been proven to be effective for harvesting cells together with their endogenous extracellular matrix so that adhesion molecules on the surface of the cell and cell-cell interactions remain intact (Li et al., 2014). Liang et al. (2016) demonstrated that in vitro coculture of MSCs/EPCs, derived from BMNC, in cell sheets at a ratio of 1/1 promoted osteoblast differentiation. Real-time (RT) PCR revealed that osteogenic-associated gene (Runx2, OCN, and Col-I) and angiogenic-associated gene (VEGF-A and KDR) expression levels were significantly higher in EPC-MSC sheets. Furthermore, in vivo analyses confirmed bone healing regeneration. Quantitative analyses revealed more bone and vessels. The authors suggested that EPCs might provide a local environment favoring MSC osteogenic differentiation (Liang et al., 2016). Wen et al. (2016) established a BM-EPC/BM-MSC indirect coculture system in vitro and in vivo, allowing only soluble factor exchange. The authors demonstrated that BM-MSCs cocultured with BM-EPCs maintained their initial biological properties: no morphological changes, continuous expression of cell genes but negative expression of an endothelial phenotype and pericyte surface markers. Moreover, the authors noticed that the EPC microenvironment positively influenced the proliferative ability of MSCs. Furthermore, the expression of the pluripotency factors OCT4, SOX2, Nanog, and Klf4 (core regulators of cell stemness) was upregulated in coculture as much as the transcription of osteoblastic marker genes (OCN, BSP, and Runx2). EPCs have dynamic roles in maintaining MSC stemness and in regulating their differentiation potential. MSC and EPC combined with fibrin glue (FG) showed improved bone regeneration, with more bone and more cancellous bone with blood vessel structures, when used to repair rat alveolar bone defects compared to monoculture grafts in vivo (Wen et al., 2016).
Bone tissue engineering aims to replace the use of autologous bone grafts, the actual gold standard. Nau et al. (2018) demonstrated in an animal model that an induced membrane filled with BM-MNCs or EPCs derived from the spleen and MSCs seeded on βTCP supports bone defect healing to a similar degree as transplanted syngeneic bone. Thus, cell therapy approaches might be feasible to reduce the use of syngeneic bone grafts during the application of the induced membrane technique (Nau et al., 2018). Currently, their combination is studied in different clinical applications, such as reconstruction of bone defects after radiotherapy or maxillary sinus augmentation. BM-MSC/BM-EPC sheets promote bone healing in irradiated rats. BM-EPCs improved the osteogenic differentiation of BM-MSC sheets and enhanced ectopic bone formation (Liu et al., 2018). BM-MSC/BM-EPC coculture on bio-Oss significantly enhanced adhesion and ALP activity in vitro. Their association with maxillary sinus augmentation resulted in significantly greater bone formation (height, compressive strength, bone volume density, trabecular thickness, and trabecular number and a significantly lower trabecular separation) in vivo (Li et al., 2020).
Besides, BM-MSC, AT-MSCs could be an interesting source of MSCs, considering their proangiogenic properties, high resistance to hypoxia, and ease of isolation (Wang et al., 2020). The AT-MSC/BM-EPC combination increases osteogenesis and angiogenesis-related gene expression in vitro. The results of animal experiments demonstrated that the AT-MSC/BM-EPC association accelerates critical-sized bone defect repair (He et al., 2020). Coculture of AT-MSC sheet-BM-EPCs generated more bone and cartilage. The newly formed tissue was denser, and the vascular lumen structures were more mature. Intramembranous and enchondral ossification processes coexist (Wang et al., 2020). Hayrapetyan et al. (2016) noticed that ECs showed a positive effect on osteogenic differentiation and mineralization in both direct and indirect culture systems in AT-MSC/HUVEC coculture. However, HUVECs had no effect on AT-MSCs proliferation. Direct coculture of AT-MSCs with HUVECs seems to be more effective than indirect coculture, probably due to simultaneous direct cell–cell contact and actions of secreted soluble molecules. AT-MSCs accounted for only 50% of the total cells in the AT-MSC/HUVEC coculture but achieved equal levels of mineralization compared to the AT-MSC monoculture (Hayrapetyan et al., 2016).
Endothelial progenitor cells and MSCs are thus commonly used to promote vessel formation and osteoblastic differentiation in tissue engineering. However, the underlying mechanisms of vessel formation and osteoblastic differentiation remain unclear. We will further describe the different interactions cell-cell, cell-matrix and cell-soluble factors coexisting between MSCs and EPCs.
Bone formation requires intimate cooperation between osteoprogenitors and endothelial cells (Grellier et al., 2009b). Intercellular interactions include cell–cell, cell–matrix, and cell–soluble factors. Grellier et al. (2009a) suggested the coexistence of three communication pathways: gap junctions, adherence and tight junctions, and diffusible factor secretion activating specific receptors on target cells (Figure 2).
Figure 2. Illustration of the different types of interaction between mesenchymal stromal cells and endothelial progenitor cells: gap junctions, adherence junctions, soluble factors, and extracellular vesicles.
Villars et al. (2000) showed that BM-MSCs express and synthesize VEGF. HUVEC-conditioned medium has a proliferative effect, and early osteoblastic marker levels increase when these cells are cocultured with HUVECs only in direct contact. Interestingly, the authors highlighted that differentiation was not modulated by VEGF alone. Direct contact between HUVECs and bone cell progenitors was mandatory (Villars et al., 2000). This signaling involves different heterotypic connections requiring adhesion molecules or gap junctions, such as Cx43 (Villars et al., 2002). Communication through Cx43 gap junctions increases the expression of early osteoblastic differentiation markers by osteoprogenitors. Cx43 expression in ECs and BM-MSCs contributes to the regulation of gene expression in osteoblastic cells, but the mechanisms remain elusive. Guillotin et al. (2004) reported that cell cooperation exists between ECs derived from large or small vessels and those derived from cord blood and osteoprogenitor cells. In accordance with their previous work, the authors suggested that ECs may support initial osteoblastic proliferation but do not alter the ability of osteoblasts to produce extracellular mineralizing matrix (ECM) (Guillotin et al., 2008). Direct contact during HUVEC/human osteoprogenitor (HOP) coculture results in cell rearrangement, giving rise to tubular-like networks promoted by soluble factors. VEGF alone could not affect EC migration under these conditions. However, VEGF seems to play some role in coculture osteoblastic differentiation (Grellier et al., 2009b).
(N)-cadherin is widely expressed in multiple tissues and participates in heterotypic contacts between different cell types. (N)-cadherin is expressed by both osteoblasts and ECs (Grellier et al., 2009a). Li et al. (2010) observed that (N)-cadherin expression was significantly upregulated in human BM-MSC and HUVEC cocultures. (N)-cadherin was concentrated in the cocultured human BM-MSC membrane but distributed within the cytoplasm of monocultured human BM-MSCs, indicating that cell–cell adhesion was improved in cocultured cells (Li et al., 2010). In addition, more beta-catenin was found to translocate into the cocultured cell nuclei, and more T cell factor-1 (TCF-1) was detected. Finally, the mRNA levels of early osteoblastic markers, including alkaline phosphatase (ALP) and type I collagen (Col-I), were significantly upregulated and subsequently increased (N)-cadherin expression. Interestingly, a link between (N)-cadherin and Cx43 has been suggested to underscore this effect (Bidarra et al., 2011).
Paracrine signaling requires diffusible factors spreading through the ECM. For Ern et al. (2010) the scarcity of MSC and EC contacts in a coculture suggests the influence of growth factor-mediated cell interactions. Wang et al. (1997) proposed bilateral osteoendothelial communication involving soluble factors such as VEGF. MSCs and ECs produce VEGF, which is able to modulate the growth and differentiation of both cell types (Wang et al., 1997; Mayer et al., 2005). Growth factors produced by ECs, including endothelin-1 (ET-1), insulin-like growth factor (IGF), and bone morphogenetic protein-2 (BMP-2), can modulate MSC osteogenic differentiation through interactions with specific membrane receptors (Grellier et al., 2009b). ET-1 is a vasoconstrictor secreted by ECs; it is involved in the regulation of craniofacial development and MSC osteogenic differentiation. Tsai et al. (2015) demonstrated that ECs regulate MSC functions through the secretion of ET-1 and AKT signaling. In response to VEGF, ECs can produce IGF-1, which in turn induces an upregulation of ALP in osteoblasts (Wang et al., 1997). The role of BMP-2 in coculture was highlighted by Kaigler et al. (2005). The inhibition of BMP-2 expression by ECs results in a significant decrease in osteogenic differentiation of BM-MSCs cocultured with ECs. bFGF can act on both ECs and MSCs, activating EC proliferation and inducing ALP and Col-1 in osteoprogenitors (Distler et al., 2003; Marie, 2003). These interactive effects may be mediated by the MAPK/ERK signaling pathway. The ECM components also support the interactions between ECs and osteoprogenitors. ECM produced by HUVECs increases the expression of ALP in osteoblasts (Lampert et al., 2016). TGF-β is expressed by ECs and osteoprogenitors and is sequestered in the ECM. After its release from the ECM, cell migration of both cell types is induced to recruit cells to the bone healing site (Wang et al., 2008).
Extracellular vesicles released by almost any cell have an important role in cell-to-cell communication. EVs are generally classified into exosomes (EXOs), 30–100 nm in diameter, initially derived from endosomes as intraluminal vesicles, and microvesicles (MVs), 50–1000 nm in diameter, generated by outward budding and fission of the plasma membrane (van Niel et al., 2018). Recently, it has been proposed that the beneficial paracrine effects observed after MSC therapy might be mediated, at least in part, by EVs. EVs can be incorporated into cells via endocytosis or phagocytosis, leading to transfer of their contents (proteins, lipids, DNA, RNA, and mitochondria). Among these constituents, microRNAs are small non-coding RNA molecules that have a prominent role in gene regulation and biological functions. Both MSC-EV release and content are modified by environmental conditions (Gorgun et al., 2020). Xie et al. (2017) observed that MSC-derived EVs promote dose-dependent HUVEC proliferation, migration and tube formation. In vitro experiments showed that MSC-derived EVs had no major effect on the proliferation, apoptosis or osteogenesis of MSCs, indicating that EV-modified scaffolds promote bone regeneration mainly by accelerating vascularization (Xie et al., 2017). Qin and Zhang (2017) reported the EPC communication and regulation of BM-MSCs through EPC-derived EVs. In this study, EPC-EVs increased MSC proliferation and inhibited their osteoblastic differentiation (Qin and Zhang, 2017). In contrast, Jia et al. (2019) in an animal model, observed that EPC-EVs accelerate osteogenesis and bone consolidation during distraction osteogenesis by stimulating angiogenesis. More trabecular mature bone and less fibrous or cartilaginous tissues were discovered after EPC or EPC-EV treatment (Jia et al., 2019). Interestingly, EPC-EVs enhanced the proliferation, migration and angiogenic capacity of HUVECs through exosomal miR-126. In addition, EPC-Exos increase the expression of angiogenesis-related genes (VEGFa, bFGF, TGFβ1, and ANG) in HUVECs (Xu et al., 2018).
Bone regeneration is a complex phenomenon involving a cell source, a scaffold, tissue-inducing factors (signaling factors) and mechanical stimulation. Preclinical and clinical studies have demonstrated that MSCs have added value in bone regeneration. Different sources of MSCs, expanded or not, are being explored. However, the great variability in terms of MSC source, dose, methodology and outcome measures renders direct comparison of the studies difficult, and studies require standardization.
Nevertheless, vascularization remains a key component. MSC and EPC coculture fosters not only osteogenesis but also angiogenesis with positive synergy. The EPCs enable and hasten osteogenesis. Knowing their precocious action in the differentiation process, EPCs are considered osteoinductive mediators. However, research still needs to optimize the knowledge of different elements, such as the cell population ratio. Cell-to-cell contact, ECM and soluble factors collaborate toward bone regeneration. Notwithstanding, the exact interaction mechanisms between these two cell populations remain unexplained. Recent studies have shown that the microenvironment participates in bone regeneration through EV production. The latter transport elements such as microRNAs, playing a key role in bone formation. Thorough studies should be conducted to better understand the role of each cell population and the nature of their interactions for bone regeneration.
CB, PP, DD, IL, DB, LL, and NM: conceptualization. CB, FC, and LL: writing. CB, PP, DD, IL, DB, LL, and NM: editing. All authors read and agreed to the published version of the manuscript.
CB was funded as a Clinical Master Specialist Ph.D. fellow by “Fonds de la Recherche Scientifique” (FNRS) (40001548). This work was supported by “Les Amis de l’Institut Jules Bordet” and “l’Association d’aide à la recherche Médicale André Vésale.”
The authors declare that the research was conducted in the absence of any commercial or financial relationships that could be construed as a potential conflict of interest.
Asahara, T., Murohara, T., Sullivan, A., Silver, M., van der Zee, R., Li, T., et al. (1997). Isolation of putative progenitor endothelial cells for angiogenesis. Science 275, 964–967. doi: 10.1126/science.275.5302.964
Atesok, K., Li, R., Stewart, D. J., and Schemitsch, E. H. (2010). Endothelial progenitor cells promote fracture healing in a segmental bone defect model. J. Orthop. Res. 28, 1007–1014. doi: 10.1002/jor.21083
Batsali, A. K., Pontikoglou, C., Koutroulakis, D., Pavlaki, K. I., Damianaki, A., Mavroudi, I., et al. (2017). Differential expression of cell cycle and WNT pathway-related genes accounts for differences in the growth and differentiation potential of Wharton’s jelly and bone marrow-derived mesenchymal stem cells. Stem Cell Res. Ther. 8:102.
Bidarra, S. J., Barrias, C. C., Barbosa, M. A., Soares, R., Amédée, J., and Granja, P. L. (2011). Phenotypic and proliferative modulation of human mesenchymal stem cells via crosstalk with endothelial cells. Stem Cell Res. 7, 186–197. doi: 10.1016/j.scr.2011.05.006
Bora, P., and Majumdar, A. S. (2017). Adipose tissue-derived stromal vascular fraction in regenerative medicine: a brief review on biology and translation. Stem Cell Res. Ther. 8:145.
Bouland, C., Meuleman, N., Widelec, J., Keiani-Mothlagh, K., Voisin, C., Lagneaux, L., et al. (2020). Case reports of medication-related osteonecrosis of the jaw (MRONJ) treated with uncultured stromal vascular fraction and L-PRF. J. Stomatol. Oral. Maxillofac. Surg. 2020, S2468–S7855.
Bourin, P., Bunnell, B. A., Casteilla, L., Dominici, M., Katz, A. J., March, K. L., et al. (2013). Stromal cells from the adipose tissue-derived stromal vascular fraction and culture expanded adipose tissue-derived stromal/stem cells: a joint statement of the International Federation for Adipose Therapeutics and Science (IFATS) and the International Society for Cellular Therapy (ISCT). Cytotherapy 15, 641–648. doi: 10.1016/j.jcyt.2013.02.006
Cai, J., Wu, Z., Huang, L., Chen, J., Wu, C., Wang, S., et al. (2014). Cotransplantation of bone marrow mononuclear cells and umbilical cord mesenchymal stem cells in avascular necrosis of the femoral head. Transplant Proc. 46, 151–155. doi: 10.1016/j.transproceed.2013.06.021
Capelli, C., Pedrini, O., Cassina, G., Spinelli, O., Salmoiraghi, S., Golay, J., et al. (2014). Frequent occurrence of non-malignant genetic alterations in clinical grade mesenchymal stromal cells expanded for cell therapy protocols. Haematologica 99, e94–e97.
Cella, L., Oppici, A., Arbasi, M., Moretto, M., Piepoli, M., Vallisa, D., et al. (2011). Autologous bone marrow stem cell intralesional transplantation repairing bisphosphonate related osteonecrosis of the jaw. Head Face Med. 7:16.
Chen, W., Liu, J., Manuchehrabadi, N., Weir, M. D., Zhu, Z., and Xu, H. H. (2013). Umbilical cord and bone marrow mesenchymal stem cell seeding on macroporous calcium phosphate for bone regeneration in rat cranial defects. Biomaterials 34, 9917–9925. doi: 10.1016/j.biomaterials.2013.09.002
d’Aquino, R., Graziano, A., Sampaolesi, M., Laino, G., Pirozzi, G., De Rosa, A., et al. (2007). Human postnatal dental pulp cells co-differentiate into osteoblasts and endotheliocytes: a pivotal synergy leading to adult bone tissue formation. Cell Death Differ. 14, 1162–1171. doi: 10.1038/sj.cdd.4402121
Dilogo, I. H., Primaputra, M. R. A., Pawitan, J. A., and Liem, I. K. (2017). Modified Masquelet technique using allogeneic umbilical cord-derived mesenchymal stem cells for infected non-union femoral shaft fracture with a 12-cm bone defect: A case report. Int. J. Surg. Case Rep. 34, 11–16. doi: 10.1016/j.ijscr.2017.03.002
Dimitriou, R., Jones, E., McGonagle, D., and Giannoudis, P. V. (2011). Bone regeneration: current concepts and future directions. BMC Med. 9:66.
Ding, D. C., Chang, Y. H., Shyu, W. C., and Lin, S. Z. (2015). Human umbilical cord mesenchymal stem cells: a new era for stem cell therapy. Cell Transplant. 24, 339–347. doi: 10.3727/096368915x686841
Distler, J. H. W., Hirth, A., Kurowska-Stolarska, M., Gay, R. E., Gay, S., and Distler, O. (2003). Angiogenic and angiostatic factors in the molecular control of angiogenesis. Q. J. Nucl. Med. 47, 149–161.
Dominici, M., Le Blanc, K., Mueller, I., Slaper-Cortenbach, I., Marini, F., Krause, D., et al. (2006). Minimal criteria for defining multipotent mesenchymal stromal cells. The international society for cellular therapy position statement. Cytotherapy 8, 315–317. doi: 10.1080/14653240600855905
Du, F., Wang, Q., Ouyang, L., Wu, H., Yang, Z., Fu, X., et al. (2020). Comparison of concentrated fresh mononuclear cells and cultured mesenchymal stem cells from bone marrow for bone regeneration. Stem Cells Transl. Med. 2020:19.
Ern, C., Krump-Konvalinkova, V., Docheva, D., Schindler, S., Rossmann, O., Böcker, W., et al. (2010). Interactions of human endothelial and multipotent mesenchymal stem cells in cocultures. Open Biomed. Eng. J. 4, 190–198. doi: 10.2174/1874120701004010190
Farré-Guash, E., Bravenboer, N., Helder, M. N., Schulten, E. A. J. M., Ten Bruggenkate, C. M., and Klein-Nulend, J. (2018). Blood Vessel Formation and Bone Regeneration Potential of the Stromal Vascular Fraction Seeded on a Calcium Phosphate Scaffold in the Human Maxillary Sinus Floor Elevation Model. Materials 2018:11.
Filipowska, J., Tomaszewski, K. A., Niedźwiedzki, L., Walocha, J. A., and Niedźwiedzki, T. (2017). The role of vasculature in bone development, regeneration and proper systemic functioning. Angiogenesis 20, 291–302. doi: 10.1007/s10456-017-9541-1
Fröhlich, L. F. (2019). MicroRNAs at the Interface between Osteogenesis and Angiogenesis as Targets for Bone Regeneration. Cells 8:121. doi: 10.3390/cells8020121
Fujii, Y., Kawase-Koga, Y., Hojo, H., Yano, F., Sato, M., Chung, U. I., et al. (2018). Bone regeneration by human dental pulp stem cells using a helioxanthin derivative and cell-sheet technology. Stem Cell Res. Ther. 9:24.
Genova, T., Munaron, L., Carossa, S., and Mussano, F. (2016). Overcoming physical constraints in bone engineering: ‘the importance of being vascularized’. J. Biomater. Appl. 30, 940–951. doi: 10.1177/0885328215616749
Geuze, R. E., Wegman, F., Oner, F. C., Dhert, W. J., and Alblas, J. (2009). Influence of endothelial progenitor cells and platelet gel on tissue-engineered bone ectopically in goats. Tissue Eng. Part A 15, 3669–3677. doi: 10.1089/ten.tea.2009.0289
Goerke, S. M., Obermeyer, J., Plaha, J., Stark, G. B., and Finkenzeller, G. (2015). Endothelial progenitor cells from peripheral blood support bone regeneration by provoking an angiogenic response. Microvasc. Res. 98, 40–47. doi: 10.1016/j.mvr.2014.12.001
Gómez-Barrena, E., Rosset, P., Gebhard, F., Hernigou, P., Baldini, N., Rouard, H., et al. (2019). Feasibility and safety of treating non-unions in tibia, femur and humerus with autologous, expanded, bone marrow-derived mesenchymal stromal cells associated with biphasic calcium phosphate biomaterials in a multicentric, non-comparative trial. Biomaterials 196, 100–108. doi: 10.1016/j.biomaterials.2018.03.033
Gorgun, C., Ceresa, D., Lesage, R., Villa, F., Reverberi, D., Balbi, C., et al. (2020). Dissecting the effects of preconditioning with inflammatory cytokines and hypoxia on the angiogenic potential of mesenchymal stromal cell (MSC)-derived soluble proteins and extracellular vesicles (EVs). Biomaterials 269:120633. doi: 10.1016/j.biomaterials.2020.120633
Grayson, W. L., Bunnell, B. A., Martin, E., Frazier, T., Hung, B. P., and Gimble, J. M. (2015). Stromal cells and stem cells in clinical bone regeneration. Nat. Rev. Endocrinol. 11, 140–150.
Grellier, M., Bordenave, L., and Amedee, J. (2009a). Cell-to-cell communication between osteogenic and endothelial lineages implications for tissue engineering. Trends Biotechnol. 27, 562–571. doi: 10.1016/j.tibtech.2009.07.001
Grellier, M., Ferreira-Tojais, N., Bourget, C., Bareille, R., Guillemot, F., and Amédée, J. (2009b). Role of vascular endothelial growth factor in the communication between human osteoprogenitors and endothelial cells. J. Cell Biochem. 106, 390–398. doi: 10.1002/jcb.22018
Gronthos, S., Mankani, M., Brahim, J., Robey, P. G., and Shi, S. (2000). Postnatal human dental pulp stem cells (DPSCs) in vitro and in vivo. Proc. Natl. Acad. Sci. USA 97, 13625–13630. doi: 10.1073/pnas.240309797
Grosso, A., Burger, M. G., Lunger, A., Schaefer, D. J., Banfi, A., and Di Maggio, N. (2017). It Takes Two to Tango: Coupling of Angiogenesis and Osteogenesis for Bone Regeneration. Front. Bioeng. Biotechnol. 5:68.
Guillotin, B., Bareille, R., Bourget, C., Bordenave, L., and Amédée, J. (2008). Interaction between human umbilical vein endothelial cells and human osteoprogenitors triggers pleiotropic effect that may support osteoblastic function. Bone 42, 1080–1091. doi: 10.1016/j.bone.2008.01.025
Guillotin, B., Bourget, C., Remy-Zolgadri, M., Bareille, R., Fernandez, P., Conrad, V., et al. (2004). Human primary endothelial cells stimulate human osteoprogenitor cell differentiation. Cell Physiol. Biochem. 14, 325–332. doi: 10.1159/000080342
Hao, P. J., Wang, Z. G., Xu, Q. C., Xu, S., Li, Z. R., Yang, P. S., et al. (2014). Effect of umbilical cord mesenchymal stem cell in peri-implant bone defect after immediate implant: an experiment study in beagle dogs. Int. J. Clin. Exp. Pathol. 7, 8271–8278.
Hayrapetyan, A., Surjandi, S., Lemsom, E. E. P. J., Wolters, M. M. M. W., Jansen, J. A., and van den Beucken, J. J. J. P. (2016). Coculture effects on the osteogenic differentiation of human mesenchymal stromal cells. Tissue Eng. Regen. Med. 13, 713–723.
He, Y., Lin, S., Ao, Q., and He, X. (2020). The co-culture of ASCs and EPCs promotes vascularized bone regeneration in critical-sized bone defects of cranial bone in rats. Stem Cell Res. Ther. 11:338.
Hendrijantini, N., Hartono, P., Ari, M. D. A., and Rantan, F. A. (2019). Human Umbilical Cord Mesenchymal Stem-Cell Therapy to Increase the Density of Osteoporotic Mandibular Bone. Eur. J. Dent. 13, 58–63.
Henrich, D., Wilhelm, K., Warzecha, J., Frank, J., Barker, J., Marzi, I., et al. (2013). Human endothelial-like differentiated precursor cells maintain their endothelial characteristics when cocultured with mesenchymal stem cell and seeded onto human cancellous bone. Mediators Inflamm. 2013:364591.
Hernigou, P., and Beaujean, F. (2002). Treatment of osteonecrosis with autologous bone marrow grafting. Clin. Orthop. Relat. Res. 405, 14–23. doi: 10.1097/00003086-200212000-00003
Hernigou, P., Guissou, I., Homma, Y., Poignard, A., Chevallier, C., Rouard, H., et al. (2015). Percutaneous injection of bone marrow mesenchymal stem cells for ankle non-unions decreases complications in patients with diabetes. Int. Orthop. 39, 1639–1643. doi: 10.1007/s00264-015-2738-2
Hernigou, P., Poignard, A., Zilber, S., and Rouard, H. (2009). Cell therapy of hip osteonecrosis with autologous bone marrow grafting. Indian J. Orthop. 43, 40–45. doi: 10.4103/0019-5413.45322
Huang, G. T., Gronthos, S., and Shi, S. (2009). Mesenchymal stem cells derived from dental tissues vs. those from other sources: their biology and role in regenerative medicine. J. Dent Res. 88, 792–806. doi: 10.1177/0022034509340867
Jäger, M., Zilkens, C., Bittersohl, B., and Rüdiger Krauspe, R. (2009). Cord blood – an alternative source for bone regeneration. Stem Cell Rev. Rep. 5, 266–277. doi: 10.1007/s12015-009-9083-z
Jia, Y., Zhu, Y., Qiu, S., Xu, J., and Chai, Y. (2019). Exosomes secreted by endothelial progenitor cells accelerate bone regeneration during distraction osteogenesis by stimulating angiogenesis. Stem Cell Res. Ther. 10:12.
Jurgens, W. J. F. M., Kroeze, R. J., Zandieh-Doulabi, B., van Dijk, A., Renders, G. A. P., Smit, T. H., et al. (2013). One-step surgical procedure for the treatment of osteochondral defects with adipose-derived stem cells in a caprine knee defect: a pilot study. Biores Open Access. 2, 315–325. doi: 10.1089/biores.2013.0024
Kaigler, D., Krebsbach, P. H., West, E. R., Horger, K., Huang, Y. C., and Mooney, D. J. (2005). Endothelial cell modulation of bone marrow stromal cell osteogenic potential. FASEB J. 19, 665–667.
Khalpey, Z., Marsh, K. M., Ferng, A., Riaz, I. B., Hemphill, C., Johnson, K., et al. (2015). First in Man: Sternal Reconstruction with Autologous Stem Cells. ASAIO J. 61, e31–e32.
Kim, S. J., Shin, Y. W., Yang, K. H., Kim, S. B., Yoo, M. J., Han, S. K., et al. (2009). A multi-center, randomized, clinical study to compare the effect and safety of autologous cultured osteoblast (Ossron) injection to treat fractures. BMC Musculoskelet Disord. 10:20.
Kitoh, H., Kawasumi, M., Kaneko, H., and Ishiguro, N. (2009). Differential effects of culture-expanded bone marrow cells on the regeneration of bone between the femoral and the tibial lengthenings. J. Pediatr Orthop. 29, 643–649. doi: 10.1097/bpo.0b013e3181b2afb2
Koç, O. N., Gerson, S. L., Cooper, B. W., Dyhouse, S. M., Haynesworth, S. E., Caplan, A. L., et al. (2000). Rapid hematopoietic recovery after coinfusion of autologous-blood stem cells and culture-expanded marrow mesenchymal stem cells in advanced breast cancer patients receiving high-dose chemotherapy. J. Clin. Oncol. 18, 307–316. doi: 10.1200/jco.2000.18.2.307
Koh, Y. J., Koh, B. I., Kim, H., Joo, H. J., Jin, H. K., Jeon, J., et al. (2011). Stromal vascular fraction from adipose tissue forms profound vascular network through the dynamic reassembly of blood endothelial cells. Arterioscler. Thromb. Vasc. Biol. 31, 1141–1150. doi: 10.1161/atvbaha.110.218206
Laino, G., d’Aquino, R., Graziano, A., Lanza, V., Carinci, F., Naro, F., et al. (2005). A New Population of Human Adult Dental Pulp Stem Cells: A Useful Source of Living Autologous Fibrous Bone Tissue (LAB). J. Bone Miner. Res. 20, 1394–1402. doi: 10.1359/jbmr.050325
Lampert, F. M., Simunovic, F., Finkenzeller, G., Pfeifer, D., Stark, G. B., Winninger, O., et al. (2016). Transcriptomic Changes in Osteoblasts Following Endothelial Cell-Cocultivation Suggest a Role of Extracellular Matrix in Cellular Interaction. J. Cell Biochem. 117, 1869–1879. doi: 10.1002/jcb.25486
Laroye, C., Gauthier, M., Antonot, H., Decot, V., Reppel, L., and Bensoussan, D. (2019). Mesenchymal Stem/Stromal Cell Production Compliant with Good Manufacturing Practice: Comparison between Bone Marrow, the Gold Standard Adult Source, and Wharton’s Jelly, an Extraembryonic Source. J. Clin. Med. 8:2207. doi: 10.3390/jcm8122207
Lee, Y. C., Chan, Y. H., Hsieh, S. C., Lew, W. Z., and Feng, S. W. (2019). Comparing the Osteogenic Potentials and Bone Regeneration Capacities of Bone Marrow and Dental Pulp Mesenchymal Stem Cells in a Rabbit Calvarial Bone Defect Model. Int. J. Mol. Sci. 20:5015. doi: 10.3390/ijms20205015
Lendeckel, S., Jödicke, A., Christophis, P., Heidinger, K., Wolff, J., Fraser, J. K., et al. (2004). Autologous stem cells (adipose) and fibrin glue used to treat widespread traumatic calvarial defects: case report. J. Craniomaxillofac. Surg. 32, 370–373. doi: 10.1016/j.jcms.2004.06.002
Li, F., Whyte, N., and Niyibizi, C. (2012). Differentiating multipotent mesenchymal stromal cells generate factors that exert paracrine activities on exogenous MSCs: Implications for paracrine activities in bone regeneration. Biochem. Biophys. Res. Commun. 426, 475–479. doi: 10.1016/j.bbrc.2012.08.095
Li, G., Wang, X., Cao, J., Ju, Z., Ma, D., Liu, Y., et al. (2014). Coculture of peripheral blood CD34+ cell and mesenchymal stem cell sheets increase the formation of bone in calvarial critical-size defects in rabbits. Br. J. Oral. Maxillofac. Surg. 52, 134–139. doi: 10.1016/j.bjoms.2013.10.004
Li, H., Daculsi, R., Grellier, M., Bareille, R., Bourget, C., and Amedee, J. (2010). Role of neural-cadherin in early osteoblastic differentiation of human bone marrow stromal cells cocultured with human umbilical vein endothelial cells. Am. J. Physiol. Cell Physiol. 299, C422–C430.
Li, Q., and Wang, Z. (2013). Influence of mesenchymal stem cells with endothelial progenitor cells in co-culture on osteogenesis and angiogenesis: an in vitro study. Arch. Med. Res. 44, 504–513. doi: 10.1016/j.arcmed.2013.09.009
Li, Q., Yu, T., Wang, F., Liu, X., and Wang, Z. (2020). Endothelial progenitor cells with stem cells enhance osteogenic efficacy. Am. J. Transl. Res. 12, 2409–2424.
Liang, Y., Wen, L., Shang, F., Wu, J., Sui, K., and Ding, Y. (2016). Endothelial progenitors enhanced the osteogenic capacities of mesenchymal stem cells in vitro and in a rat alveolar bone defect model. Arch. Oral. Biol. 68, 123–130. doi: 10.1016/j.archoralbio.2016.04.007
Liebergall, M., Schroeder, J., Mosheiff, R., Gazit, Z., Yoram, Z., Rasooly, L., et al. (2013). Stem cell-based therapy for prevention of delayed fracture union: a randomized and prospective preliminary study. Mol. Ther. 21, 1631–1638. doi: 10.1038/mt.2013.109
Liu, H., Jiao, Y., Zhou, W., Bai, S., Feng, Z., Dong, Y., et al. (2018). Endothelial progenitor cells improve the therapeutic effect of mesenchymal stem cell sheets on irradiated bone defect repair in a rat model. J. Transl. Med. 16:137.
Liu, Y., Chan, J. K., and Teoh, S. H. (2015). Review of vascularised bone tissue-engineering strategies with a focus on co-culture systems. J. Tissue Eng. Regen. Med. 9, 85–105. doi: 10.1002/term.1617
Liu, Y., Teoh, S. H., Chong, M. S., Lee, E. S., Mattar, C. N., Randhawa, N. K., et al. (2012). Vasculogenic and osteogenesis-enhancing potential of human umbilical cord blood endothelial colony-forming cells. Stem Cells 30, 1911–1924. doi: 10.1002/stem.1164
Manimaran, K., Sankaranarayanan, S., Ravi, V. R., Elangovan, S., Chandramohan, M., and Mahendra Perumal, S. (2014). Treatment of osteoradionecrosis of mandible with bone marrow concentrate and with dental pulp stem cells. Ann. Maxillofac. Surg. 4, 189–192. doi: 10.4103/2231-0746.147130
Manimaran, K., Sharma, R., Sankaranarayanan, S., and Mahendra Perumal, S. (2016). Regeneration of mandibular ameloblastoma defect with the help of autologous dental pulp stem cells and buccal pad of fat stromal vascular fraction. Ann. Maxillofac. Surg. 6, 97–100. doi: 10.4103/2231-0746.186128
Marcacci, M., Kon, E., Moukhachev, V., Lavroukov, A., Kutepov, S., Quarto, R., et al. (2007). Stem cells associated with macroporous bioceramics for long bone repair: 6- to 7-year outcome of a pilot clinical study. Tissue Eng. 13, 947–955. doi: 10.1089/ten.2006.0271
Marie, P. J. (2003). Fibroblast growth factor signaling controlling osteoblast differentiation. Gene 316, 23–32. doi: 10.1016/s0378-1119(03)00748-0
Mayer, H., Bertram, H., Lindenmaier, W., Korff, T., Weber, H., and Weich, H. (2005). Vascular endothelial growth factor (VEGF-A) expression in human mesenchymal stem cells: autocrine and paracrine role on osteoblastic and endothelial differentiation. J. Cell Biochem. 95, 827–839. doi: 10.1002/jcb.20462
Mesimäki, K., Lindroos, B., Törnwall, J., Mauno, J., Lindqvist, C., Kontio, R., et al. (2009). Novel maxillary reconstruction with ectopic bone formation by GMP adipose stem cells. Int. J. Oral. Maxillofac. Surg. 38, 201–209. doi: 10.1016/j.ijom.2009.01.001
Mitrano, T. I., Grob, M. S., Carrión, F., Nova-Lamperti, E., Luz, P. A., Fierro, F. S., et al. (2010). Culture and characterization of mesenchymal stem cells from human gingival tissue. J. Periodontol. 81, 917–925. doi: 10.1902/jop.2010.090566
Miura, M., Gronthos, S., Zhao, M., Lu, B., Fisher, L. W., Robey, P. G., et al. (2003). SHED: stem cells from human exfoliated deciduous teeth. Proc. Natl. Acad. Sci. USA. 100, 5807–5812.
Morishita, T., Honoki, K., Ohgushi, H., Kotobuki, N., Matsushima, A., and Takakura, Y. (2006). Tissue engineering approach to the treatment of bone tumors: three cases of cultured bone grafts derived from patients’ mesenchymal stem cells. Artif. Organs. 30, 115–118. doi: 10.1111/j.1525-1594.2006.00190.x
Morsczeck, C., Götz, W., Schierholz, J., Zeilhofer, F., Kühn, U., Möhl, C., et al. (2005). Isolation of precursor cells (PCs) from human dental follicle of wisdom teeth. Matrix Biol. 24, 155–165. doi: 10.1016/j.matbio.2004.12.004
Nakajima, K., Kunimatsu, R., Ando, K., Ando, T., Hayashi, Y., Kihara, T., et al. (2018). Comparison of the bone regeneration ability between stem cells from human exfoliated deciduous teeth, human dental pulp stem cells and human bone marrow mesenchymal stem cells. Biochem. Biophys. Res. Commun. 497, 876–882. doi: 10.1016/j.bbrc.2018.02.156
Nau, C., Simon, S., Schaible, A., Seebach, C., Schröder, K., Marzi, I., et al. (2018). Influence of the induced membrane filled with syngeneic bone and regenerative cells on bone healing in a critical size defect model of the rat’s femur. Injury 49, 1721–1731. doi: 10.1016/j.injury.2018.06.041
Paduano, F., Marrelli, M., Amantea, M., Rengo, C., Rengo, S., Goldberg, M., et al. (2017). Adipose Tissue as a Strategic Source of Mesenchymal Stem Cells in Bone Regeneration: A Topical Review on the Most Promising Craniomaxillofacial Applications. Int. J. Mol. Sci. 18:2140. doi: 10.3390/ijms18102140
Pang, H., Wu, X. H., Fu, S. L., Luo, F., Zhang, Z. H., Hou, T. Y., et al. (2013). Prevascularisation with endothelial progenitor cells improved restoration of the architectural and functional properties of newly formed bone for bone reconstruction. Int. Orthop. 37, 753–759. doi: 10.1007/s00264-012-1751-y
Pavan Kumar, B., Ram Mohan, S., Mohan, A. P., Jeevan Kumar, K. A., and Yashwanth Yadav, B. (2016). Versatility of Pleuripotent Undifferentiated Stem Cells Aspirated from Bone Marrow and its Applications in Oral and Maxillofacial Surgery. J. Maxillofac. Oral Surg. 15, 1–11. doi: 10.1007/s12663-015-0793-2
Perez, J. R., Kouroupis, D., Li, D. J., Best, T. M., Kaplan, L., and Correa, D. (2018). Tissue Engineering and Cell-Based Therapies for Fractures and Bone Defects. Front. Bioeng. Biotechnol. 6:105.
Petridis, X., Diamanti, E., Trigas, G. C. H., Kalyvas, D., and Kitraki, E. (2015). Bone regeneration in critical-size calvarial defects using human dental pulp cells in an extracellular matrix-based scaffold. J. Craniomaxillofac. Surg. 43, 483–490. doi: 10.1016/j.jcms.2015.02.003
Prins, H. J., Schulten, E. A., Ten Bruggenkate, C. M., Klein-Nulend, J., and Helder, M. N. (2016). Bone Regeneration Using the Freshly Isolated Autologous Stromal Vascular Fraction of Adipose Tissue in Combination With Calcium Phosphate Ceramics. Stem Cells Transl. Med. 5, 1362–1374. doi: 10.5966/sctm.2015-0369
Qin, Y., and Zhang, C. (2017). Endothelial progenitor cell-derived extracellular vesicle-meditated cell-to-cell communication regulates the proliferation and osteoblastic differentiation of bone mesenchymal stromal cells. Mol. Med. Rep. 16, 7018–7024. doi: 10.3892/mmr.2017.7403
Quarto, R., Mastrogiacomo, M., Cancedda, R., Kutepov, S. M., Mukhachev, V., Lavroukov, A., et al. (2001). Repair of Large Bone Defects with the Use of Autologous Bone Marrow Stromal Cells. N. Engl. J. Med. 344, 385–386. doi: 10.1056/nejm200102013440516
Rahyussalim, A. J., Ivansyah, M. D., Nugroho, A., Wikanjaya, R., Canintika, A. F., and Kurniawati, T. (2020). Vertebral body defects treated with umbilical-cord mesenchymal stem cells combined with hydroxyapatite scaffolds: The first case report. Int. J. Surg. Case Rep. 66, 304–308. doi: 10.1016/j.ijscr.2019.12.002
Rana, D., Kumar, A., and Sharma, S. (2018). Endothelial Progenitor Cells as Molecular Targets in Vascular Senescence and Repair. Curr. Stem. Cell Res. Ther. 13, 438–446. doi: 10.2174/1574888x13666180502100620
Rehman, J., Traktuev, D., Li, J., Merfeld-Clauss, S., and Temm-Grove, C. J. (2004). Secretion of angiogenic and antiapoptotic factors by human adipose stromal cells. Circulation 109, 1292–1298. doi: 10.1161/01.cir.0000121425.42966.f1
Rouwkema, J., and Khademhosseini, A. (2016). Vascularization and Angiogenesis in Tissue Engineering: Beyond Creating Static Networks. Trends Biotechnol. 34, 733–745. doi: 10.1016/j.tibtech.2016.03.002
Sándor, G. K., Numminen, J., Wolff, J., Thesleff, T., Miettinen, A., Tuovinen, V. J., et al. (2014). Adipose stem cells used to reconstruct 13 cases with cranio-maxillofacial hard-tissue defects. Stem Cells Transl. Med. 3, 530–540. doi: 10.5966/sctm.2013-0173
Sándor, G. K., Tuovinen, V. J., Wolff, J., Patrikoski, M., Jokinen, J., and Nieminen, E. (2013). Adipose stem cell tissue-engineered construct used to treat large anterior mandibular defect: a case report and review of the clinical application of good manufacturing practice-level adipose stem cells for bone regeneration. J. Oral. Maxillofac. Surg. 71, 938–950. doi: 10.1016/j.joms.2012.11.014
Sasaki, J. I., Hashimoto, M., Yamaguchi, S., Itoh, Y., Yoshimoto, I., Matsumoto, T., et al. (2015). Fabrication of Biomimetic Bone Tissue Using Mesenchymal Stem Cell-Derived Three-Dimensional Constructs Incorporating Endothelial Cells. PLoS One. 10:e0129266. doi: 10.1371/journal.pone.0129266
Saxer, F., Scherberich, A., Todorov, A., Studer, P., Miot, S., Schreiner, S., et al. (2016). Implantation of Stromal Vascular Fraction Progenitors at Bone Fracture Sites: From a Rat Model to a First-in-Man Study. Stem Cells. 34, 2956–2966. doi: 10.1002/stem.2478
Seebach, C., Henrich, D., Kähling, C., Wilhelm, K., Tami, A. E., Alini, M., et al. (2010). Endothelial progenitor cells and mesenchymal stem cells seeded onto beta-TCP granules enhance early vascularization and bone healing in a critical-sized bone defect in rats. Tissue Eng. Part A. 16, 1961–1970. doi: 10.1089/ten.tea.2009.0715
Seebach, C., Henrich, D., Wilhelm, K., Barker, J. H., and Marzi, I. (2012). Endothelial progenitor cells improve directly and indirectly early vascularization of mesenchymal stem cell-driven bone regeneration in a critical bone defect in rats. Cell Transplant. 21, 1667–1677. doi: 10.3727/096368912x638937
Seo, B. M., Miura, M., Gronthos, S., Bartold, P. M., Batouli, S., Brahim, J., et al. (2004). Investigation of multipotent postnatal stem cells from human periodontal ligament. Lancet 364, 149–155. doi: 10.1016/s0140-6736(04)16627-0
Silini, A. R., Masserdotti, A., Papait, A., and Parolini, O. (2019). Shaping the Future of Perinatal Cells: Lessons From the Past and Interpretations of the Present. Front. Bioeng. Biotechnol. 7:75.
Sonoyama, W., Liu, Y., Fang, D., Yamaza, T., Seo, B. M., Zhang, C., et al. (2006). Mesenchymal stem cell-mediated functional tooth regeneration in swine. PLoS One 1:e79. doi: 10.1371/journal.pone.0000079
Squillaro, T., Peluso, G., and Galderisi, U. (2016). Clinical Trials With Mesenchymal Stem Cells: An Update. Cell Transplant. 25, 829–848. doi: 10.3727/096368915x689622
Stolk, L. M., and van der Geest, S. (1998). Plasma concentrations after a clomipramine intoxication. J. Anal. Toxicol. 22, 612–613. doi: 10.1093/jat/22.7.612
Subramanian, A., Fong, C. Y., Biswas, A., and Bongso, A. (2015). Comparative Characterization of Cells from the Various Compartments of the Human Umbilical Cord Shows that the Wharton’s Jelly Compartment Provides the Best Source of Clinically Utilizable Mesenchymal Stem Cells. PLoS One 10:e0127992. doi: 10.1371/journal.pone.0127992
Sun, K., Zhou, Z., Ju, X., Zhou, Y., Lan, J., Chen, D., et al. (2016). Combined transplantation of mesenchymal stem cells and endothelial progenitor cells for tissue engineering: a systematic review and meta-analysis. Stem Cell Res. Ther. 7:151.
Sun, Y., Feng, Y., and Zhang, C. (2009). The effect of bone marrow mononuclear cells on vascularization and bone regeneration in steroid-induced osteonecrosis of the femoral head. Joint Bone Spine 76, 685–690. doi: 10.1016/j.jbspin.2009.04.002
Tamari, T., Kawar-Jaraisy, R., Doppelt, O., Giladi, B., Sabbah, N., and Zigdon-Giladi, H. (2020). The Paracrine Role of Endothelial Cells in Bone Formation via CXCR4/SDF-1 Pathway. Cells 9:1325. doi: 10.3390/cells9061325
Thesleff, T., Lehtimäki, K., Niskakangas, T., Mannerström, B., Miettinen, S., Suuronen, R., et al. (2011). Cranioplasty with adipose-derived stem cells and biomaterial: a novel method for cranial reconstruction. Neurosurgery 68, 1535–1540. doi: 10.1227/neu.0b013e31820ee24e
Tomasello, L., Mauceri, R., Coppola, A., Pitrone, M., Pizzo, G., Campisi, G., et al. (2017). Mesenchymal stem cells derived from inflamed dental pulpal and gingival tissue: a potential application for bone formation. Stem Cell Res. Ther. 8:179.
Tsai, T. L., Wang, B., Squire, M. W., Guo, L. W., and Li, W. J. (2015). Endothelial cells direct human mesenchymal stem cells for osteo- and chondro-lineage differentiation through endothelin-1 and AKT signaling. Stem Cell Res. Ther. 6:88.
Umemura, T., Nishioka, K., Igarashi, A., Kato, Y., Ochi, M., Chayama, K., et al. (2006). Autologous bone marrow mononuclear cell implantation induces angiogenesis and bone regeneration in a patient with compartment syndrome. Circ. J. 70, 1362–1364. doi: 10.1253/circj.70.1362
Usami, K., Mizuno, H., Okada, K., Narita, Y., Aoki, M., Kondo, T., et al. (2009). Composite implantation of mesenchymal stem cells with endothelial progenitor cells enhances tissue-engineered bone formation. J. Biomed. Mater. Res. A. 90, 730–741. doi: 10.1002/jbm.a.32142
van Niel, G., D’Angelo, G., and Raposo, G. (2018). Shedding light on the cell biology of extracellular vesicles. Nat. Rev. Mol. Cell Biol. 19, 213–228. doi: 10.1038/nrm.2017.125
Villars, F., Bordenave, L., Bareille, R., and Amédée, J. (2000). Effect of human endothelial cells on human bone marrow stromal cell phenotype: role of VEGF? J. Cell Biochem. 79, 672–685. doi: 10.1002/1097-4644(20001215)79:4<672::aid-jcb150>3.0.co;2-2
Villars, F., Guillotin, B., Amédée, T., Dutoya, S., Bordenave, L., Bareille, R., et al. (2002). Effect of HUVEC on human osteoprogenitor cell differentiation needs heterotypic gap junction communication. Am. J. Physiol. Cell Physiol. 282, C775–C785.
Wang, D. S., Miura, M., Demura, H., and Sato, K. (1997). Anabolic effects of 1,25-dihydroxyvitamin D3 on osteoblasts are enhanced by vascular endothelial growth factor produced by osteoblasts and by growth factors produced by endothelial cells. Endocrinology 138, 2953–2962. doi: 10.1210/endo.138.7.5275
Wang, X. J., Dong, Z., Zhong, X. H., Shi, R. Z., Huang, S. H., Lou, Y., et al. (2008). Transforming growth factor-beta1 enhanced vascular endothelial growth factor synthesis in mesenchymal stem cells. Biochem. Biophys. Res. Commun. 365, 548–554. doi: 10.1016/j.bbrc.2007.11.014
Wang, Z., Han, L., Sun, T., Wang, W., Li, X., and Wu, B. (2020). Osteogenic and angiogenic lineage differentiated adipose-derived stem cells for bone regeneration of calvarial defects in rabbits. J. Biomed. Mater. Res. 2020:8.
Watson, L., Elliman, S. J., and Coleman, C. M. (2014). From isolation to implantation: a concise review of mesenchymal stem cell therapy in bone fracture repair. Stem Cell Res. Ther. 5:51. doi: 10.1186/scrt439
Wen, L., Wang, Y., Wen, N., Yuan, G., Wen, M., Zhang, L., et al. (2016). Role of Endothelial Progenitor Cells in Maintaining Stemness and Enhancing Differentiation of Mesenchymal Stem Cells by Indirect Cell-Cell Interaction. Stem Cells Dev. 25, 123–138. doi: 10.1089/scd.2015.0049
Wu, V., Helder, M. N., Bravenboer, N., Ten Bruggenkate, C. M., Jin, J., Klein-Nulend, J., et al. (2019). Bone Tissue Regeneration in the Oral and Maxillofacial Region: A Review on the Application of Stem Cells and New Strategies to Improve Vascularization. Stem. Cells Int. 2019:6279721.
Xie, H., Wang, Z., Zhang, L., Lei, Q., Zhao, A., Wang, H., et al. (2017). Extracellular Vesicle-functionalized Decalcified Bone Matrix Scaffolds with Enhanced Pro-angiogenic and Pro-bone Regeneration Activities. Sci. Rep. 7:45622.
Xu, L., Liu, Y., Sun, Y., Wang, B., Xiong, Y., Lin, W., et al. (2017). Tissue source determines the differentiation potentials of mesenchymal stem cells: a comparative study of human mesenchymal stem cells from bone marrow and adipose tissue. Stem Cell Res. Ther. 8:275.
Xu, W., Zilong, L., Lijuan, H., Wenyu, G., and Lei, Z. (2018). Exosomes derived from endothelial progenitor cells ameliorate acute lung injury by transferring miR-126. Exp. Cell Res. 370, 13–23. doi: 10.1016/j.yexcr.2018.06.003
Zajdel, A., Kałucka, M., Kokoszka-Mikołaj, E., and Wilczok, A. (2017). Osteogenic differentiation of human mesenchymal stem cells from adipose tissue and Wharton’s jelly of the umbilical cord. Acta Biochim. Pol. 64, 365–369.
Zhao, D., Cui, D., Wang, B., Tian, F., Guo, L., Yang, L., et al. (2012). Treatment of early stage osteonecrosis of the femoral head with autologous implantation of bone marrow-derived and cultured mesenchymal stem cells. Bone 50, 325–330. doi: 10.1016/j.bone.2011.11.002
Zigdon-Giladi, H., Bick, T., Lewinson, D., and Machtei, E. E. (2015). Co-transplantation of endothelial progenitor cells and mesenchymal stem cells promote neovascularization and bone regeneration. Clin. Implant Dent. Relat. Res. 17, 353–359. doi: 10.1111/cid.12104
Zuk, P. A., Zhu, M., Ashjian, P., De Ugarte, D. A., Huang, J. I., Mizuno, H., et al. (2002). Human adipose tissue is a source of multipotent stem cells. Mol. Biol. Cell 13, 4279–4295.
Keywords: bone regeneration, endothelial progenitor cell (EPC), mesenchymal stromal cell (MSC), cross-talk, review
Citation: Bouland C, Philippart P, Dequanter D, Corrillon F, Loeb I, Bron D, Lagneaux L and Meuleman N (2021) Cross-Talk Between Mesenchymal Stromal Cells (MSCs) and Endothelial Progenitor Cells (EPCs) in Bone Regeneration. Front. Cell Dev. Biol. 9:674084. doi: 10.3389/fcell.2021.674084
Received: 28 February 2021; Accepted: 23 April 2021;
Published: 13 May 2021.
Edited by:
Guido Moll, Charité – Universitätsmedizin Berlin, GermanyReviewed by:
Ornella Parolini, Catholic University of the Sacred Heart Rome, ItalyCopyright © 2021 Bouland, Philippart, Dequanter, Corrillon, Loeb, Bron, Lagneaux and Meuleman. This is an open-access article distributed under the terms of the Creative Commons Attribution License (CC BY). The use, distribution or reproduction in other forums is permitted, provided the original author(s) and the copyright owner(s) are credited and that the original publication in this journal is cited, in accordance with accepted academic practice. No use, distribution or reproduction is permitted which does not comply with these terms.
*Correspondence: Cyril Bouland, Y3lyaWwuYm91bGFuZEB1bGIuYmU=
Disclaimer: All claims expressed in this article are solely those of the authors and do not necessarily represent those of their affiliated organizations, or those of the publisher, the editors and the reviewers. Any product that may be evaluated in this article or claim that may be made by its manufacturer is not guaranteed or endorsed by the publisher.
Research integrity at Frontiers
Learn more about the work of our research integrity team to safeguard the quality of each article we publish.