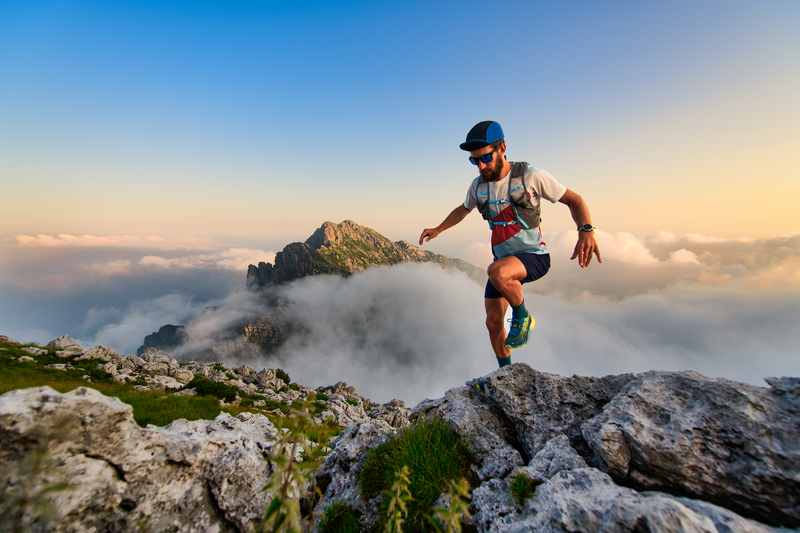
94% of researchers rate our articles as excellent or good
Learn more about the work of our research integrity team to safeguard the quality of each article we publish.
Find out more
BRIEF RESEARCH REPORT article
Front. Cell Dev. Biol. , 22 July 2021
Sec. Stem Cell Research
Volume 9 - 2021 | https://doi.org/10.3389/fcell.2021.667304
Dendritic cells (DC) are professional antigen-presenting cells that develop from hematopoietic stem cells. Different DC subsets exist based on ontogeny, location and function, including the recently identified proinflammatory DC3 subset. DC3 have the prominent activity to polarize CD8+ T cells into CD8+ CD103+ tissue resident T cells. Here we describe human DC3 differentiated from induced pluripotent stem cells (iPS cells). iPS cell-derived DC3 have the gene expression and surface marker make-up of blood DC3 and polarize CD8+ T cells into CD8+ CD103+ tissue-resident memory T cells in vitro. To test the impact of malignant JAK2 V617F mutation on DC3, we differentiated patient-specific iPS cells with JAK2 V617Fhet and JAK2 V617Fhom mutations into JAK2 V617Fhet and JAK2 V617Fhom DC3. The JAK2 V617F mutation enhanced DC3 production and caused a bias toward erythrocytes and megakaryocytes. The patient-specific iPS cell-derived DC3 are expected to allow studying DC3 in human diseases and developing novel therapeutics.
Graphical Abstract. Patient-specific iPS cells with JAK2 V617F mutation differentiate into DC3 dendritic cells, megakaryocytes and red blood cells.
Dendritic cells (DC) develop from hematopoietic stem cells and populate a large array of lymphoid and non-lymphoid tissues in our body. DC play a pivotal role in antigen presentation to induce immunity and immunological tolerance (Guilliams et al., 2014; Mildner and Jung, 2014). DC are classified into two main subsets, classical DC (cDC) and plasmacytoid DC (pDC), based on surface marker expression, function and ontogeny, and cDC are further subdivided into cDC1 and cDC2 (Guilliams et al., 2014; Mildner and Jung, 2014; Collin and Bigley, 2018; Amon et al., 2020). However, DC heterogeneity and in particular cDC2 heterogeneity have been controversial to date. Single cell techniques revealed two subpopulation in cDC2 referred to as DC2 and DC3 (Villani et al., 2017; Brown et al., 2019; Dutertre et al., 2019). DC3 share features with both DC2 and monocytes but are developmentally and functionally different from both DC2 and monocytes (Bourdely et al., 2020; Cytlak et al., 2020). All cDC subsets activate T cells but DC3 have the prominent activity to polarize CD8+ T cells into CD8+ CD103+ tissue-resident memory (TRM) T cells (Bourdely et al., 2020). DC are a scarce cell type in blood and tissues (Granot et al., 2017), which hampers their analysis in mice and man, and their therapeutic application in the human system.
Pluripotent stem cells, including embryonic stem cells (ES cells) and induced pluripotent stem cells (iPS cells), are a particular appealing cell source for studies in developmental biology and disease modeling, and in the human system for regenerative medicine (Rowe and Daley, 2019). Patient and disease-specific iPS cells capture disease-specific and/or associated mutations and this is key to model human diseases in a dish and for compound screening. Human ES cells and iPS cells can be induced to differentiate into hematopoietic cells, often through 3D embryoid body (EB) differentiation (Sturgeon et al., 2014; Ivanovs et al., 2017; Garcia-Alegria et al., 2018), and many blood cell types were generated from human iPS cells, including DC (Ackermann et al., 2015). Frequently, the cytokine cocktails to induce DC development included GM-CSF (Senju et al., 2011; Silk et al., 2012; Cai et al., 2017; Sontag et al., 2017; Sachamitr et al., 2018; Horton et al., 2020), yet DC development is known to depend on Flt3 ligand (Flt3L) (Felker et al., 2010; Mildner and Jung, 2014; Collin and Bigley, 2018; Amon et al., 2020).
The JAK2 V617F mutation is associated with a group of hematopoietic malignancies termed myeloproliferative neoplasms (MPN), including polycythemia vera (PV), essential thrombocythemia (ET) and primary myelofibrosis (PMF) (Vainchenker and Kralovics, 2017). JAK2 V617F causes cytokine-independent constitutive activation of JAK2 kinase and its signaling pathways, thereby leading to aberrant production of red cells, platelets and myeloid cells in MPN. Whether this involves also an aberrant regulation of immune cells, such as DC, and how this might cause and/or contribute to disease is largely unknown.
To investigate the impact of proinflammatory JAK2 V617F signaling on DC we differentiated disease-specific JAK2 V617F iPS cells into DC. Frequently, DC are generated in vitro with GM-CSF, which triggers the JAK2/STAT signaling pathway (Hacker et al., 2003; Hieronymus et al., 2005; Seré et al., 2012; Collin and Bigley, 2018; Zhan et al., 2019) and thus a high dose of GM-CSF stimulation might hide the activity of JAK2 V617F mutation in DC. Here we differentiated human JAK2 V617F iPS cells into DC using Flt3L and IL-7. JAK2 V617F mutation did not affect DC function but enhanced production of DC, erythrocytes and megakaryocytes. Interestingly, the CD1c+ DC obtained exhibited properties of DC3 and polarized CD8+ T cells toward CD8+ CD103+ TRM cells.
Patient-specific iPS cells were obtained by reprogramming peripheral blood mononuclear cells (PBMC) of healthy donor and of two PV patients (referred to as PV1 and PV2) with JAK2 V617F mutation after informed consent (local board reference number EK099/14 and EK206/09) with OCT4, SOX2, c-MYC and KLF4 in CytoTune Sendai virus vectors (Thermo Fisher Scientific) (Sontag et al., 2017). iPS cells with monoallelic and biallelic JAK2 V617F mutation, referred to as JAK2 V617Fhet and JAK2 V617Fhom iPS cells, respectively, and without mutation were isolated and further studied. Patient PV2 exhibited a high JAK2 V617F allele burden (96%) and only JAK2 V617Fhom iPS cell clones were obtained, and thus the isogenic unmutated control was generated by CRISPR/Cas9 editing using the Alt-R CRISPR/Cas9 system (IDT, Coralville, United States).
Briefly, the CRISPR/Cas9 complex (Alt-R HiFi Cas9 nuclease plus gRNA), single-stranded donor template and electroporation enhancer were delivered to cells using the Neon Transfection System and the 100 μl kit (Thermo Fisher Scientific). Before electroporation, iPS cells were treated for 1 h with HDR enhancer (5 μM; IDT, Coralville, United States) and 10 μM Y-27632 (Abcam, Cambridge, United Kingdom). Electroporated cells were seeded on Laminin 521 (Biolamina, Sundbyberg, Sweden) coated plates in StemMACS iPS-Brew XF (Miltenyi Biotec) supplemented with 1x CloneR (Stemcell Technologies, Vancouver, Canada). Genotyping of CRISPR-repaired iPS cell lines was performed by allele-specific PCR targeting the JAK2 V617F mutation. Sequences of crRNA, donor template and allele-specific PCR primers are listed in Supplementary Table 1. Potential off-target genes CREBL2 and COA6 were unaffected (Supplementary Figure 1).
In the Human Pluripotent Stem Cell Registry1 PV1 JAK2 and JAK2 V617Fhet iPS cells are referred as UKAi002-A and UKAi002-B, and PV2 JAK2 and JAK2 V617Fhom iPS cells are referred to as UKAi003-A2 and UKAi003-A, respectively.
Healthy donor iPS cells were obtained by reprogramming PBMC as above and were used as healthy control. Routinely, iPS cells were maintained in StemMACS iPS-Brew XF (Miltenyi Biotec) on 6-well plates coated with Matrigel (Corning).
Differentiation of human iPS cells into hemogenic endothelium (HE) and hematopoietic progenitor cells (HPC) was by spin embryoid bodies (spin EB) protocol (Liu et al., 2015) with some modifications. Briefly, iPS cells were harvested with Accutase and single cell suspension were plated on round-bottom 96 well suspension plates (Greiner) with 4,000 cells/well in serum free medium (SFM) containing 10 ng/ml BMP4 (Miltenyi Biotec), 10 ng/ml bFGF (Peprotech), 10 μM Y-27632 (Abcam), 50 μg/ml L-ascorbic acid (L-AA, Stem Cell Technologies) and 6 μg/ml holo-transferrin (Sigma). SFM was a 1:1 mixture of IMDM and F12 medium (both Thermo Fisher Scientific) containing 0.5% BSA, 2 mM GlutaMAX, 1% chemically defined lipid concentrate (both Thermo Fisher Scientific) and 400 μM 1-thioglycerol (MTG, Sigma). On day 2 fresh culture medium containing BMP4, bFGF, L-AA, holo-transferrin, and 10 ng/ml VEGF (Peprotech) was added.
EB formation was observed after 24–48 h and from day 4 onward half medium change was performed by adding fresh culture medium containing bFGF, VEGF, L-AA, holo-transferrin, and SCF (0.5% supernatant of SCF producing CHO KLS cells) every second day. On day 10–11 EB were harvested by gentle pipetting and 30 EB/well were plated on gelatin-coated 6-well plates in RPMI (Thermo Fisher Scientific), 10% FCS (PAN Biotech) with 25 ng/ml Flt3L (Peprotech), 10 ng/ml IL-7 (Miltenyi Biotec), 2 mM L-Glutamine, and 100 μM 2-Mercaptoethanol (both Thermo Fisher Scientific) referred to as DC culture medium. Four days later fresh DC culture medium was added and on day 8–9 of differentiation suspension and loosely adherent cells were harvested with gentle pipetting and HLA-DR+ cells were isolated by immunomagnetic bead selection (MACS) with HLA-DR microbeads (Miltenyi Biotec) following the manufacture’s instruction. HLA-DR+ cells were resuspended in DC culture medium without or with 1 μg/ml LPS and cultured for 1 day to induce the DC activation. These HLA-DR+ cells were used for further experiments.
Hemogenic endothelium (HE, CD34+ CD31+ CD144+ CD43– CD45– CD73–), hematopoietic progenitor cells (HPC, CD43+ CD34low/−), cells obtained upon DC differentiation and T cells were analyzed by flow cytometry (Supplementary Figures 2A–E). Cells were stained with specific antibodies (Supplementary Table 2) and analyzed on FACS Canto II (BD) as described (Sontag et al., 2017) and data were analyzed with FlowJo software (Tree Star).
For Diff-Quik staining HLA-DR+ cells were centrifuged onto glass slides in Cytospin 4 cytocentrifuge (Thermo Fisher Scientific). Cells were fixed with methanol, stained with Diff-Quik (Medion Dianostics) and mounted with Entellan (Merck).
Total RNA was isolated with NucleoSpin RNA Kit (Macherey Nagel) following the manufacturer’s instruction, quantified with NanoDrop (Thermo Fisher Scientific) and subjected to reverse transcription-quantitative polymerase chain reaction (RT-qPCR) analysis. Briefly, total RNA was reverse transcribed with High capacity cDNA Reverse Transcriptase Kit (Thermo Fisher Scientific). Synthesized cDNA was used for qPCR analysis with FAST SYBR Green master mix (Applied BioSystem) on a StepOne Plus device (Applied BioSystem). Expression values were normalized to GAPDH and ΔCt value over 12 was regarded as not-expressed and arbitrarily set to 12. z-scores of −ΔCt mean were calculated for each gene and subjected to hierarchical clustering and representation in heat map format with Morpheus software2. Primers are listed in Supplementary Table 3.
Chemotaxis assay was performed as described (Kurz et al., 2002; Sontag et al., 2017). In brief, transwell inserts (5 μm pore size, Corning) were incubated with culture medium for 1 h (37°C, 5% CO2) to block unspecific binding. In the lower chamber, medium with or without 100 ng/ml CCL19 (Peprotech) was added and then 3–4 × 104 HLA-DR+ cells were added to the upper chamber. After 2 h incubation (37°C, 5% CO2), 104 Dynabeads (15 μm diameter, Dynal polymers) were added to the lower chamber, to allow normalization for variations in the experimental procedure, and cells and beads of the lower chamber were analyzed by flow cytometry as described before (Kurz et al., 2002). The number of migrated cells was determined relative to 104 beads from the ratio of cells/beads analyzed by flow cytometry (Supplementary Figure 2D) and the percentage of migrated cells was calculated relative to the total input number of cells.
MLR was performed as described (Dutertre et al., 2019) with some modifications. PBMC were obtained from healthy donors after informed consent. CD3+ T cells were isolated by MACS with CD3 microbeads (Miltenyi Biotec) and labeled with 5 μM CFSE (Stem Cell Technologies) for 20 min at 37°C. CFSE-labeled CD3+ T cells (105 cells) were mixed with unstimulated or LPS-stimulated HLA-DR+ cells (104 cells) and cultured in 10% KO-serum replacement supplemented IMDM (Thermo Fisher Scientific) for 5 days. CFSE-labeled CD3+ T cells were cultured with 5–10 μg/ml concanavalin A (ConA) or without ConA, to provide positive and negative controls, respectively. Before harvesting the cells, 104 Dynabeads were added and CFSE division and T cell numbers were analyzed by flow cytometry and normalized to beads.
Statistical analyses were performed in Prism 8 (GraphPad) using one-way or two-way ANOVA with Tukey’s multiple comparisons test or uncorrected fisher’s LSD test. Results were considered to be significant at p < 0.05 (∗), p < 0.005 (∗∗), p < 0.001 (∗∗∗), p < 0.0001 (****) with specific comparisons as indicated in respective figure legends.
Human iPS cells were differentiated into HE and HPC in a spin EB protocol (Figure 1A). To establish iPS cell differentiation we employed iPS cells of healthy donor and on day 10 of EB differentiation CD34+ CD43− HE and CD43+ CD34low/− HPC were observed (Figure 1B). EB were harvested and further differentiated into DC with Flt3L and IL-7, thereby following a protocol modified from DC differentiation of cord blood cells (Balan et al., 2018; Laustsen et al., 2018). Monitoring the kinetics of major histocompatibility class II (MHC II) expression by flow cytometry revealed a peak of CD1c+ HLA-DR+ cells on day 8 of differentiation (data not shown). About 40% HLA-DR+ cells were routinely obtained on day 8–9 (Figure 1C) and HLA-DR+ cells were isolated and used for further analyses (Figure 1A).
Figure 1. Human iPS cells differentiate into DC in EB and Flt3L + IL-7 cultures. (A) Schematic representation of iPS cell differentiation into DC. Human iPS cells were differentiated into HE and HPC for 10–11 days in spin EB culture with consecutive supplementation of cytokines as indicated. HE and HPC were then cultured for 8–9 days with Flt3 ligand (Flt3L) and IL-7 to induce DC differentiation (dd 0 to dd 8–9). HLA-DR+ cells were isolated by immunomagnetic bead selection (MACS) and stimulated with 1 μg/ml LPS for 1 day (dd 8–9 + 1). These cells were used for further analysis as indicated. (B) Representative flow cytometry analysis and quantification of HE (CD34+ CD31+ CD144+ CD43– CD45– CD73– cells; blue boxes) and HPC (CD43+ CD34low/− cells) in EB on day 10 of spin EB differentiation. n = 3, line represents mean. (C) Frequency of HLA-DR+ cells on day 8–9 of DC differentiation. n = 6, line represents mean. (D) Representative photomicrographs of unstimulated and LPS-stimulated HLA-DR+ cells in culture (left) and in cytospin preparations stained with Diff-Quik (right). n = 6, scale bars: 100 μm. (E) Gene expression profiling of iPS cells (day 0) and EB on day 2–10 of spin EB differentiation, and unstimulated and LPS-stimulated HLA-DR+ cells on day 8–9 + 1 of DC differentiation by RT-qPCR analysis. Expression values were normalized to GAPDH and ΔCt value over 12 was regarded as not-expressed and arbitrarily set to 12. z-scores of −ΔCt mean calculated for each gene are shown in heat map format (red, high expression; blue, low expression). n = 2. (F,G) Representative flow cytometry analysis and quantification of median fluorescent intensity (MFI) of DC markers on unstimulated and LPS-stimulated CD1c+ HLA-DR+ cells. Blue lines and black lines in histograms represent stained and unstained cells, respectively. MFI values were normalized to unstained cells. (G) n = 2, mean ± SD. (H) Cytokine expression in LPS-stimulated CD1c+ HLA-DR+ cells by RT-qPCR analysis. Unstimulated cells (-LPS) are shown as control. Values were normalized to GAPDH and 2-ΔCt values in log scale were shown. n = 2, mean ± SD. All data shown in (B–H) are from healthy control iPS cells. Data in (D,F) are representative for healthy control and patient PV1 and PV2 iPS cells. n = 2–6.
HLA-DR+ cells showed DC morphology and expression of DC specific genes, such as ITGAX (CD11c), MHCII, IRF4 and IRF8, and the expression of the DC3 genes CD14 and CD163 (Figures 1D,E). We then proceeded to investigate cDC subset specific markers, including CD141 and CLEC9A for cDC1, and CD1c and CD172a for cDC2. We also investigated CD163 and CLEC10A to distinguish DC and monocytes (Heger et al., 2018; Cytlak et al., 2020). The number of HLA-DR+ cells increased with time during DC differentiation and HLA-DR+ cells expressed CD1c and CD14 (Figure 1F and Supplementary Figure 3A). DC showed also expression of CD163, CD172a and CLEC10A, but not CD141 and CLEC9A (Figure 1F and Supplementary Figures 3B, 4A) implying that they are distinct from monocytes and resemble more DC3 than cDC1 or DC2.
DC expressed the co-stimulatory molecules CD40 and CD86, which relates to high HLA-DR expression in these cells, since they were enriched for HLA-DR expression (Figures 1A,F,G). There was no further up-regulation of HLA-DR, CD40, CD83, and CD86 expression upon stimulation with lipopolysaccharide (LPS; Figures 1F,G). DC expressed inflammatory cytokines, including TNFα, IL-6 and IL-8, and there was essentially no further up-regulation upon LPS stimulation with the exception of some up-regulation of IL-6 (Figure 1H). Taken together, human iPS cells were efficiently induced to differentiate into DC with Flt3L and IL-7 and the CD1c+ HLA-DR+ DC obtained exhibited features of DC3.
Next we applied this DC differentiation protocol to disease-specific iPS cells. JAK2 V617F iPS cells from two PV patients (hereafter referred to as PV1 and PV2) with monoallelic and biallelic JAK2 V617F mutation, referred to as JAK2 V617Fhet and JAK2 V617Fhom iPS cells, respectively, and without mutation were studied. Due to the high JAK2 V617F allele burden in patient PV2, only clones with JAK2 V617Fhom mutation were obtained and thus the isogenic unmutated control was generated by CRISPR/Cas9 editing. JAK2 V617Fhet and JAK2 V617Fhom iPS cells and unmutated controls were subjected to spin EB differentiation and CD34+ CD43– HE and CD43+ CD34low/− HPC were obtained after 10–11 days (Figure 2A). Cells were then differentiated into CD1c+ HLA-DR+ cells for 8–9 days. JAK2 V617Fhet and JAK2 V617Fhom iPS cells produced more CD1c+ HLA-DR+ cells than iPS cells without mutation (Figure 2B). The frequency of CD1c+ HLA-DR+ cells for the different V617F genotypes of PV1 and PV2 varied (Supplementary Figure 4B), probably due to patient-specific differences and the increased erythropoiesis, megakaryopoiesis and granulopoiesis observed for these cells (see below Figures 3A,B).
Figure 2. JAK2 V617Fhet and JAK2 V617Fhom iPS cells differentiate into DC. (A) Representative flow cytometry analysis and quantification of HE and HPC (CD34+ CD31+ CD144+ CD43– CD45– CD73– cells and CD43+ CD34low/− cells, respectively) in EB on day 10 of spin EB differentiation. JAK2 V617Fhet and JAK2 V617Fhom om cells of PV patient 1 (PV1) and PV patient 2 (PV2), respectively, and cells of PV1 and PV2 without JAK2 V617F mutation (JAK2) are shown. n = 3, line represents mean; *p < 0.05, two-way ANOVA with Tukey’s multiple comparisons test. (B) The number of HLA-DR+ cells on day 8–9 of DC differentiation. JAK2 genotypes are as in (A). n = 7–10, line represents mean; *p < 0.05, **p < 0.005, one-way ANOVA with uncorrected fisher’s LSD test. (C) Expression of the DC markers CD1c, CD11c, and CD172a, and of CD14 on HLA-DR+ cells was assessed by flow cytometry. Blue lines and black lines in histograms show stained and unstained cells, respectively. (D) Gene expression profiling of unstimulated and LPS-stimulated CD1c+ HLA-DR+ cells on day 8–9 + 1 of DC differentiation by RT-qPCR analysis. Expression values were normalized to GAPDH and z-scores of −ΔCt mean calculated for each gene are shown in heat map format as in Figure 1E. n = 3.
Figure 3. Erythropoiesis and megakaryopoiesis upon differentiation of JAK2 V617Fhom iPS cells. (A) JAK2 V617Fhet and JAK2 V617Fhom iPS cells of patients PV1 and PV2, respectively, were differentiated as in Figure 1A and HLA-DR– cells on day 8–9 of differentiation were analyzed for erythrocytes, megakaryocytes and myeloid cells (granulocytes, monocytes and macrophages) by flow cytometry. Cells without mutation (JAK2) and cells of healthy donor (healthy control) were used as controls. Representative flow cytometry plots are shown. (B) Quantification of flow cytometry data of (A). n = 2–4, mean ± SD; *p < 0.05, **p < 0.005, one-way ANOVA with Tukey’s multiple comparisons test.
Differentiated CD1c+ HLA-DR+ cells of all JAK2 V617F genotypes had the same DC phenotype based on cell morphology, surface maker and gene expression profiling and exhibited DC3 characteristics (Figures 2C,D and Supplementary Figure 4A). DC responded to LPS with augmented expression of proinflammatory and co-stimulatory molecules (Figure 2D and Supplementary Figures 4C,D).
To determine the impact of JAK2 V617F mutation on differentiation into other cell types than DC, HLA-DR– cells of the DC differentiation protocol were analyzed. JAK2 V617Fhom caused a production of CD235a/glycophorin+ and CD42b+ cells (Figures 3A,B), indicative for erythropoiesis and megakaryopoiesis, respectively, which is in line with PV phenotype. A prominent CD14+ CD16+ macrophage population was observed for healthy control and patient PV1 but was essentially absent in patient PV2, again reflecting patient-specific differences.
In summary, the JAK2 V617F mutation enhanced production of CD1c+ HLA-DR+ DC without grossly affecting the DC phenotype and with preserving the DC3 characteristics.
Migration in response to chemokine gradient and T cell activation represent important DC properties. To this end, CD1c+ HLA-DR+ DC were activated with LPS or left untreated and subjected to chemotaxis toward CCL19. Both JAK2 V617Fhom DC and DC without mutation effectively migrated toward CCL19 chemokine (Figure 4A and Supplementary Figure 5A). JAK2 V617Fhom DC and unmutated controls also effectively induced CD4 and CD8 T cell proliferation (Figure 4B). DC3 polarize CD8+ T cells into CD8+ CD103+ TRM cells (Bourdely et al., 2020), and thus we explored CD103 expression in proliferated T cells. JAK2 V617Fhom DC and unmutated DC induced CD103 expression in CD8 T cells, indicating polarization toward CD8+ CD103+ TRM cells (Figure 4B). There was no CD103 expression in unstimulated T cells or T cells stimulated with concanavalin A (ConA). This T cell polarization is known to depend on TGFβ (Bourdely et al., 2020) and we note that the iPS cell-derived DC studied here show abundant expression of TGFβ (Supplementary Figure 5B).
Figure 4. JAK2 V617Fhom DC migrate toward CCL19 chemokine and induce polarization of CD8+ T cells toward CD8+ CD103+ tissue resident T cells. (A) DC obtained from JAK2 V617Fhom iPS cells (patient PV2) and the respective iPS cells without mutation (JAK2) migrate toward CCL19 chemokine. CD1c+ HLA-DR+ cells of day 8–9 of DC differentiation were treated with LPS (1 μg/ml, 24 h) or left untreated and subjected to CCL19 chemotaxis assay. Migrated cells in response to CCL19 were measured by flow cytometry and the percentage of migrated cells is shown. n = 3, line represents mean; *p < 0.05, **p < 0.005, two-way ANOVA with uncorrected fisher’s LSD test. (B) DC obtained from patient PV2 iPS cells as in (A) and from healthy donor iPS cells (healthy control) induce CD4+ and CD8+ T cell proliferation in MLR assays. CD1c+ HLA-DR+ cells on day 8–9 of DC differentiation were stimulated with LPS (1 μg/ml, 24 h) and co-cultured with CFSE labeled T cells for 5 days and CD4+ and CD8+ T cell proliferation was determined by flow cytometry. Representative flow cytometry analysis and quantification of percentage and number of dividing CD4+, CD8+, and/or CD103+ T cells are shown. ConA-stimulated and unstimulated T cells, positive and negative control, respectively. n = 2–3, independent experiments and independent healthy donors; line represents mean; *p < 0.05, **p < 0.005, ***p < 0.001, one-way ANOVA with Tukey’s multiple comparisons test vs. unstimulated T cells or as indicated.
Taken together, the JAK2 V617Fhom DC and the unmutated and healthy control DC obtained here are fully competent in chemotaxis toward CCL19 and in CD4 and CD8 T cell activation. In addition their ability of polarizing CD8+ T cells toward CD8+ CD103+ TRM cells qualifies them as DC3.
DC3 represent a novel CD1c+ DC subset recently identified by single cell analysis (Villani et al., 2017; Dutertre et al., 2019). DC3 are unique in their capacity to polarize CD8+ T cells into CD8+ CD103+ TRM cells (Bourdely et al., 2020). Here we describe a DC differentiation system for human DC3 from iPS cells. These iPS cell-derived DC3 have the gene expression and surface marker make-up of blood DC3 and polarize CD8+ T cells into CD8+ CD103+ TRM cells in vitro.
DC3 are within the DC landscape positioned between monocytes and DC2, but resemble more DC2 than monocytes. Additionally, DC3 are proposed to directly develop from macrophage/DC progenitor (MDP) along an IRF8low trajectory and independently of the IRF8high common DC progenitor (CDP) trajectory, which gives rise to cDC1, DC2 and pDC (Cytlak et al., 2020).
iPS cell differentiation in EB protocols into HE and HPC, and then further into mature hematopoietic cells is known to resemble yolk-sac hematopoiesis (Ivanovs et al., 2017; Lee et al., 2018), where only unspecific DC precursors are found and no further DC subsets are distinguished (Popescu et al., 2019). In these protocols myeloid cells, such as primitive macrophages (Takata et al., 2017; Lee et al., 2018), mast cells (Kovarova et al., 2010) and primitive megakaryocytes (Liu et al., 2015), develop rather than definitive lymphocytes (Sturgeon et al., 2014). Thus, in our iPS cell differentiation this myeloid bias in concert with the cytokines Flt3L and IL-7 might favor DC3 differentiation rather than development of other lineages.
Upon DC3 differentiation several DC lineage determining transcription factors, such as IRF4, IRF8 and PU.1, were upregulated. Yet the differentiation conditions applied apparently did not support executing bona fide cDC1, DC2 and pDC development. Additionally, whether the DC3 obtained exhibit a primitive imprint as remnant of yolk-sac hematopoiesis is an open question. Thus, an efficient execution of cDC1, DC2 and pDC development might require more definitive hematopoietic progenitors and/or higher IRF8 expression (Sontag et al., 2017; Cytlak et al., 2020).
Whether DC3 development dependents on Flt3L or GM-CSF is controversial (Dutertre et al., 2019; Bourdely et al., 2020). Initial data indicate that in our iPS cell differentiation system GM-CSF caused development of granulocytes and macrophages rather than DC3 (data not shown). In addition, JAK2 V617Fhom iPS cells showed a bias toward erythropoiesis and megakaryopoiesis in line with the PV patient phenotype. These data indicate that the differentiation system employed here allows development into further hematopoietic lineages. Interestingly, the JAK2 V617F mutation increased DC3 numbers but did not impact on the DC3 functions analyzed so far.
Finally, patients with systemic lupus erythematosus (SLE) show accumulated DC3 in blood, which correlates with SLE disease activity index (Dutertre et al., 2019). In breast cancer patients DC3 infiltration and frequencies of CD8+ CD103+ TRM cells correlate, which is related to a protective prognosis (Bourdely et al., 2020). Thus, the novel iPS cell differentiation system for DC3 developed here stands as a valuable tool for studying DC3 in human disease and for developing novel therapeutic strategies, such as pharmacologically targeting DC3 in disease.
The raw data supporting the conclusions of this article will be made available by the authors, without undue reservation.
The studies involving human participants were reviewed and approved by RWTH Aachen University Faculty of Medicine Ethics Board, reference numbers EK099/14 and EK206/09. The patients/participants provided their written informed consent to participate in this study.
TS: conception and design, experiments, collection and assembly of data, data analysis and interpretation, figure preparation and manuscript writing. MT, JB, and KO: experiments, collection and/or assembly of iPS cell data, data analysis and interpretation. NF and KG: experiments, collection and/or assembly of DC data, data analysis, interpretation and figure preparation. CK and SS: establishment of patient iPS cells. KS, SK, TB and NC: data analysis and interpretation. YT: conception and design. MZ: conception and design, data analysis and interpretation and manuscript writing. All authors approved the final version of the manuscript for submission.
MT was funded by CAPES-Alexander von Humboldt postdoctoral fellowship (99999.001703/2014-05) and donation by U. Lehmann. This work was supported in part by a collaborative grant (CRU344) of the Deutsche Forschungsgemeinschaft (German Research Foundation) to MZ (ZE432/10-1), NC (CH1509/1-1), SK (KO2155/7-1), and TB (BR1782/5-1), by a grant of IZKF Aachen to KS and SK (O1-2) and MZ and NC (O1-4), and by StemCellFactory III funds of the Ministry of Culture and Science of the German State of North Rhine-Westphalia and the European Regional Development Fund (EFRE), D sseldorf, Germany, to MZ.
The authors declare that the research was conducted in the absence of any commercial or financial relationships that could be construed as a potential conflict of interest.
We acknowledge the support of the Interdisciplinary Center for Clinical Research Aachen (IZKF Aachen) FACS Core Facility for cell sorting and the expert administrative assistance of E. Mierau.
The Supplementary Material for this article can be found online at: https://www.frontiersin.org/articles/10.3389/fcell.2021.667304/full#supplementary-material
Supplementary Figure 1 | DNA sequence of potential CRISPR/Cas9 off-target genes CREBL2 and COA6. The potential CRISPR/Cas9 off-target genes cAMP responsive element binding protein like 2 (CREBL2) and cytochrome C oxidase assembly factor 6 (COA6) were analyzed by DNA sequencing (A,B, respectively) and no off-target effects were found. CRISPR/Cas9 gRNA sequence targeting JAK2 (light orange box); PAM sequence (light green box).
Supplementary Figure 2 | Gating strategies for flow cytometry analysis. (A) Gating strategy for CD34+ CD31+ CD144+ CD43– CD45– CD73– HE (blue boxes) and CD43+ CD34low/− HPC in Figures 1B,2A. (B) Gating strategy for HLA-DR+ DC and the DC subsets cDC1 (CD141 and CLEC9A) and cDC2 (CD1c and CD172a) and for the co-stimulatory molecules CD40, CD83 and CD86 in Figures 1C,F,G, 2B,C and Supplementary Figures 4B–D. (C) Gating strategy for CD235a/glycophorin A+ erythrocytes, CD42b+ megakaryocytes and CD14+, CD16+, and CD66b+ myeloid cells (monocytes, makrophages and granulocytes, respectively) in Figures 3A,B. (D) Gating strategy for DC migration toward CCL19 chemokine in Figure 4A and Supplementary Figure 5A. (E) Gating strategy for CD4+ and CD8+ T cells and for CD103 in T cell activation assays in Figure 4B.
Supplementary Figure 3 | (A) Kinetics of emerging HLA-DR+ CD1c+ CD14+ cells of healthy donor during DC differentiation at days 4, 8 and 12 by flow cytometry. (B) Representative flow cytometry analysis of CD163 and CLEC10A on HLA-DR+ CD1c+ CD14+ cells of healthy donor on days 7–8 of DC differentiation. Control, unstained cells. n = 2.
Supplementary Figure 4 | (A) Representative flow cytometry analysis of CD141 and CLEC9A on HLA-DR+ cells on day 8–9 of DC differentiation. JAK2 genotypes of patients PV1 and PV2, and healthy donor (healthy control) are as in Figure 3A. Blue lines and black lines in histograms represent stained and unstained cells, respectively. n = 2–3. (B) The percentage of HLA-DR+ cells on day 8–9 of DC differentiation for JAK2 V617Fhet and JAK2 V617Fhom cells of patients PV1 and PV2, and for cells without mutation (JAK2). n = 7–10, line represents mean; ∗p < 0.05, ∗∗p < 0.005, ****p < 0.0001, one-way ANOVA with uncorrected fisher’s LSD test. (C) Representative flow cytometry analysis of CD40, CD83, and CD86 on unstimulated and LPS-stimulated CD1c+ HLA-DR+ cells on day 8–9 + 1 of DC differentiation. JAK2 genotypes of patients PV1 and PV2 are as in (B). n = 3. (D) Expression of DC activation markers CD40, CD83 and CD86 on unstimulated and LPS-stimulated CD1c+ HLA-DR+ cells of (C). MFI values were normalized to unstained cells. JAK2 genotypes of patients PV1 and PV2 are as in (B). n = 3, mean ± SD; ∗p < 0.05, two-way ANOVA with uncorrected fisher’s LSD test between unstimulated and LPS-stimulated cells for each JAK2 V617F genotype or as indicated.
Supplementary Figure 5 | (A) Chemotaxis assay toward CCL19 of JAK2 V617Fhom and JAK2 control CD1c+ HLA-DR+ cells as in Figure 4A. Numbers of migrated cells were determined by flow cytometry and the fold change to the cell number without CCL19 is shown. n = 3, mean ± SD; ns, not significant. (B) TGFβ1 expression in unstimulated and LPS-stimulated HLA-DR+ cells of Supplementary Figure 4C determined by RT-qPCR analysis. Values were normalized to GAPDH and 2–ΔCt values were shown. n = 3, mean ± SD; ∗p < 0.05, ∗∗p < 0.005, one-way ANOVA with uncorrected fisher’s LSD test between unstimulated and LPS-stimulated cells for the various JAK2 V617F genotypes as indicated.
Ackermann, M., Liebhaber, S., Klusmann, J., and Lachmann, N. (2015). Lost in translation: pluripotent stem cell-derived hematopoiesis. EMBO Mol. Med. 7, 1388–1402. doi: 10.15252/emmm.201505301
Amon, L., Lehmann, C. H. K., Heger, L., Heidkamp, G. F., and Dudziak, D. (2020). The ontogenetic path of human dendritic cells. Mol. Immunol. 120, 122–129. doi: 10.1016/j.molimm.2020.02.010
Balan, S., Arnold-Schrauf, C., Abbas, A., Couespel, N., Savoret, J., Imperatore, F., et al. (2018). Large-scale human dendritic cell differentiation revealing Notch-dependent lineage bifurcation and heterogeneity. Cell Rep. 24, 1902–1915.e6. doi: 10.1016/j.celrep.2018.07.033
Bourdely, P., Anselmi, G., Vaivode, K., Ramos, R. N., Missolo-Koussou, Y., Hidalgo, S., et al. (2020). Transcriptional and functional analysis of CD1c+ human dendritic cells identifies a CD163+ subset priming CD8+CD103+ T cells. Immunity 53, 335–352.e8. doi: 10.1016/j.immuni.2020.06.002
Brown, C. C., Gudjonson, H., Pritykin, Y., Deep, D., Lavallée, V. P., Mendoza, A., et al. (2019). Transcriptional basis of mouse and human dendritic cell heterogeneity. Cell 179, 846–863.e24. doi: 10.1016/j.cell.2019.09.035
Cai, S., Hou, J., Fujino, M., Zhang, Q., Ichimaru, N., Takahara, S., et al. (2017). iPSC-derived regulatory dendritic cells inhibit allograft rejection by generating alloantigen-specific regulatory T cells. Stem Cell Reports 8, 1174–1189. doi: 10.1016/j.stemcr.2017.03.020
Collin, M., and Bigley, V. (2018). Human dendritic cell subsets: an update. Immunology 154, 3–20. doi: 10.1111/imm.12888
Cytlak, U., Resteu, A., Pagan, S., Green, K., Milne, P., Maisuria, S., et al. (2020). Differential IRF8 transcription factor requirement defines two pathways of dendritic cell development in humans. Immunity 53, 353–370.e8. doi: 10.1016/j.immuni.2020.07.003
Dutertre, C. A., Becht, E., Irac, S. E., Khalilnezhad, A., Narang, V., Khalilnezhad, S., et al. (2019). Single-cell analysis of human mononuclear phagocytes reveals subset-defining markers and identifies circulating inflammatory dendritic cells. Immunity 51, 573–589.e8. doi: 10.1016/j.immuni.2019.08.008
Felker, P., Seré, K., Lin, Q., Becker, C., Hristov, M., Hieronymus, T., et al. (2010). TGF-β1 accelerates dendritic cell differentiation from common dendritic cell progenitors and directs subset specification toward conventional dendritic cells. J. Immunol. 185, 5326–5335. doi: 10.4049/jimmunol.0903950
Garcia-Alegria, E., Menegatti, S., Fadlullah, M. Z. H., Menendez, P., Lacaud, G., and Kouskoff, V. (2018). Early human hemogenic endothelium generates primitive and definitive hematopoiesis in vitro. Stem Cell Reports 11, 1061–1074. doi: 10.1016/j.stemcr.2018.09.013
Granot, T., Senda, T., Carpenter, D. J., Matsuoka, N., Weiner, J., Gordon, C. L., et al. (2017). Dendritic cells display subset and tissue-specific maturation dynamics over human life. Immunity 46, 504–515. doi: 10.1016/j.immuni.2017.02.019
Guilliams, M., Ginhoux, F., Jakubzick, C., Naik, S. H., Onai, N., Schraml, B. U., et al. (2014). Dendritic cells, monocytes and macrophages: a unified nomenclature based on ontogeny. Nat. Rev. Immunol. 14, 571–578. doi: 10.1038/nri3712
Hacker, C., Kirsch, R. D., Ju, X. S., Hieronymus, T., Gust, T. C., Kuhl, C., et al. (2003). Transcriptional profiling identifies Id2 function in dendritic cell development. Nat. Immunol. 4, 380–386. doi: 10.1038/ni903
Heger, L., Balk, S., Lühr, J. J., Heidkamp, G. F., Lehmann, C. H. K., Hatscher, L., et al. (2018). CLEC10A is a specific marker for human CD1c+ dendritic cells and enhances their toll-like receptor 7/8-induced cytokine secretion. Front. Immunol. 9:744. doi: 10.3389/fimmu.2018.00744
Hieronymus, T., Gust, T. C., Kirsch, R. D., Jorgas, T., Blendinger, G., Goncharenko, M., et al. (2005). Progressive and controlled development of mouse dendritic cells from Flt3 + CD11b + progenitors in vitro. J. Immunol. 174, 2552–2562. doi: 10.4049/jimmunol.174.5.2552
Horton, C., Davies, T. J., Lahiri, P., Sachamitr, P., and Fairchild, P. J. (2020). Induced pluripotent stem cells reprogrammed from primary dendritic cells provide an abundant source of immunostimulatory dendritic cells for use in immunotherapy. Stem Cells 38, 67–79. doi: 10.1002/stem.3095
Ivanovs, A., Rybtsov, S., Ng, E. S., Stanley, E. G., Elefanty, A. G., and Medvinsky, A. (2017). Human haematopoietic stem cell development: From the embryo to the dish. Development 144, 2323–2337. doi: 10.1242/dev.134866
Kovarova, M., Latour, A. M., Chason, K. D., Tilley, S. L., and Koller, B. H. (2010). Human embryonic stem cells: a source of mast cells for the study of allergic and inflammatory diseases. Blood 115, 3695–3703. doi: 10.1182/blood-2009-08-237206
Kurz, S. M., Diebold, S. S., Hieronymus, T., Gust, T. C., Bartunek, P., Sachs, M., et al. (2002). The impact of c-met/scatter factor receptor on dendritic cell migration. Eur. J. Immunol. 32, 1832–1838. doi: 10.1002/1521-4141(200207)32:7<1832::AID-IMMU1832<3.0.CO;2-2
Laustsen, A., Bak, R. O., Krapp, C., Kjær, L., Egedahl, J. H., Petersen, C. C., et al. (2018). Interferon priming is essential for human CD34+ cell-derived plasmacytoid dendritic cell maturation and function. Nat. Commun. 9:3525. doi: 10.1038/s41467-018-05816-y
Lee, C. Z. W., Kozaki, T., and Ginhoux, F. (2018). Studying tissue macrophages in vitro: are iPSC-derived cells the answer? Nat. Rev. Immunol. 18, 716–725. doi: 10.1038/s41577-018-0054-y
Liu, Y., Wang, Y., Gao, Y., Forbes, J. A., Qayyum, R., Becker, L., et al. (2015). Efficient generation of megakaryocytes from human induced pluripotent stem cells using food and drug administration-approved pharmacological reagents. Stem Cells Transl. Med. 4, 309–319. doi: 10.5966/sctm.2014-0183
Mildner, A., and Jung, S. (2014). Development and function of dendritic cell subsets. Immunity 40, 642–656. doi: 10.1016/j.immuni.2014.04.016
Popescu, D. M., Botting, R. A., Stephenson, E., Green, K., Webb, S., Jardine, L., et al. (2019). Decoding human fetal liver haematopoiesis. Nature 574, 365–371. doi: 10.1038/s41586-019-1652-y
Rowe, R. G., and Daley, G. Q. (2019). Induced pluripotent stem cells in disease modelling and drug discovery. Nat. Rev. Genet. 20, 377–388. doi: 10.1038/s41576-019-0100-z
Sachamitr, P., Leishman, A. J., Davies, T. J., and Fairchild, P. J. (2018). Directed differentiation of human induced pluripotent stem cells into dendritic cells displaying tolerogenic properties and resembling the CD141+ subset. Front. Immunol. 8:1935. doi: 10.3389/fimmu.2017.01935
Senju, S., Haruta, M., Matsumura, K., Matsunaga, Y., Fukushima, S., Ikeda, T., et al. (2011). Generation of dendritic cells and macrophages from human induced pluripotent stem cells aiming at cell therapy. Gene Ther. 18, 874–883. doi: 10.1038/gt.2011.22
Seré, K. M., Lin, Q., Felker, P., Rehage, N., Klisch, T., Ortseifer, I., et al. (2012). Dendritic cell lineage commitment is instructed by distinct cytokine signals. Eur. J. Cell Biol. 91, 515–523. doi: 10.1016/j.ejcb.2011.09.007
Silk, K. M., Silk, J. D., Ichiryu, N., Davies, T. J., Nolan, K. F., Leishman, A. J., et al. (2012). Cross-presentation of tumour antigens by human induced pluripotent stem cell-derived CD141 XCR1 dendritic cells. Gene Ther. 19, 1035–1040. doi: 10.1038/gt.2011.177
Sontag, S., Förster, M., Qin, J., Wanek, P., Mitzka, S., Schüler, H. M., et al. (2017). Modelling IRF8 deficient human hematopoiesis and dendritic cell development with engineered iPS cells. Stem Cells 35, 898–908. doi: 10.1002/stem.2565
Sturgeon, C. M., Ditadi, A., Awong, G., Kennedy, M., and Keller, G. (2014). Wnt signaling controls the specification of definitive and primitive hematopoiesis from human pluripotent stem cells. Nat. Biotechnol. 32, 554–561. doi: 10.1038/nbt.2915
Takata, K., Kozaki, T., Lee, C. Z. W., Thion, M. S., Otsuka, M., Lim, S., et al. (2017). Induced-pluripotent-stem-cell-derived primitive macrophages provide a platform for modeling tissue-resident macrophage differentiation and function. Immunity 47, 183–198.e6. doi: 10.1016/j.immuni.2017.06.017
Vainchenker, W., and Kralovics, R. (2017). Genetic basis and molecular pathophysiology of classical myeloproliferative neoplasms. Blood 129, 667–679. doi: 10.1182/blood-2016-10-695940
Villani, A. C., Satija, R., Reynolds, G., Sarkizova, S., Shekhar, K., Fletcher, J., et al. (2017). Single-cell RNA-seq reveals new types of human blood dendritic cells, monocytes, and progenitors. Science 356:eaah4573. doi: 10.1126/science.aah4573
Keywords: induced pluripotent stem cell, iPS cells, hematopoiesis, dendritic cells, DC3, JAK2, JAK2 V617F
Citation: Satoh T, Toledo MAS, Boehnke J, Olschok K, Flosdorf N, Götz K, Küstermann C, Sontag S, Seré K, Koschmieder S, Brümmendorf TH, Chatain N, Tagawa YI and Zenke M (2021) Human DC3 Antigen Presenting Dendritic Cells From Induced Pluripotent Stem Cells. Front. Cell Dev. Biol. 9:667304. doi: 10.3389/fcell.2021.667304
Received: 12 February 2021; Accepted: 30 June 2021;
Published: 22 July 2021.
Edited by:
Valerie Kouskoff, The University of Manchester, United KingdomReviewed by:
Songjie Cai, Brigham and Women’s Hospital and Harvard Medical School, United StatesCopyright © 2021 Satoh, Toledo, Boehnke, Olschok, Flosdorf, Götz, Küstermann, Sontag, Seré, Koschmieder, Brümmendorf, Chatain, Tagawa and Zenke. This is an open-access article distributed under the terms of the Creative Commons Attribution License (CC BY). The use, distribution or reproduction in other forums is permitted, provided the original author(s) and the copyright owner(s) are credited and that the original publication in this journal is cited, in accordance with accepted academic practice. No use, distribution or reproduction is permitted which does not comply with these terms.
*Correspondence: Martin Zenke, bWFydGluLnplbmtlQHJ3dGgtYWFjaGVuLmRl
Disclaimer: All claims expressed in this article are solely those of the authors and do not necessarily represent those of their affiliated organizations, or those of the publisher, the editors and the reviewers. Any product that may be evaluated in this article or claim that may be made by its manufacturer is not guaranteed or endorsed by the publisher.
Research integrity at Frontiers
Learn more about the work of our research integrity team to safeguard the quality of each article we publish.