- 1School of Life Sciences, Immanuel Kant Baltic Federal University, Kaliningrad, Russia
- 2Department of Psychiatry and Psychotherapy, Charité University Medicine Berlin, Berlin, Germany
Microglia are the resident immune cells of the adult brain that become activated in response to pathogen- or damage-associated stimuli. The acute inflammatory response to injury, stress, or infection comprises the release of cytokines and phagocytosis of damaged cells. Accumulating evidence indicates chronic microglia-mediated inflammation in diseases of the central nervous system, most notably neurodegenerative disorders, that is associated with disease progression. To understand microglia function in pathology, knowledge of microglia communication with their surroundings during normal state and the release of neurotrophins and growth factors in order to maintain homeostasis of neural circuits is of importance. Recent evidence shows that microglia interact with serotonin, the neurotransmitter crucially involved in adult neurogenesis, and known for its role in antidepressant action. In this chapter, we illustrate how microglia contribute to neuroplasticity of the hippocampus and interact with local factors, e.g., BDNF, and external stimuli that promote neurogenesis. We summarize the recent findings on the role of various receptors in microglia-mediated neurotransmission and particularly focus on microglia’s response to serotonin signaling. We review microglia function in neuroinflammation and neurodegeneration and discuss their novel role in antidepressant mechanisms. This synopsis sheds light on microglia in healthy brain and pathology that involves serotonin and may be a potential therapeutic model by which microglia play a crucial role in the maintenance of mood.
Introduction
In the adult brain, microglia are the resident macrophages and, as such, a unique cell population interacting with neurons, astrocytes, oligodendrocytes, and the various signaling molecules. Characterized by Iba-1 and CD11b immunoreactivity (expressed in resting and activated cells; Franco and Fernández-Suárez, 2015), microglia exhibit a diverse, dynamic morphology that allows a quick response to changes in the environment. Under physiological conditions, highly branched microglial cells constantly sense the environment to maintain homeostasis, modulate synapse maturation and connectivity, and regulate neuronal activity (Kettenmann et al., 2011). In the hippocampus, in particular, microglia display a vigilant phenotype (Grabert et al., 2016); they take part in learning-dependent synaptic plasticity and neural network excitability, and release of growth factors and neurotrophins, e.g., brain-derived neurotrophic factor (BDNF) (Parkhurst et al., 2013), involved in memory formation. As part of the limbic system, the hippocampus plays a central role in learning, especially in the encoding and retrieval of episodic and spatial memories (Buzsaki and Moser, 2013). Importantly, microglia contribute to the lifelong generation of new neurons in the hippocampus. Set in the dentate gyrus, neural stem cells (NSCs) retain fate plasticity and respond to a variety of local cues and extrinsic stimuli that foster a neuronal fate. Most of the newly generated cells die before maturation into granule neurons (Dayer et al., 2003) as a strategy balancing cell proliferation vs. cell death. In their role in phagocytosis of damaged cells and debris, recent studies attribute non-activated microglia to the control over the neuronal cell pool by removal of apoptotic progenitor cells (Sierra et al., 2010).
A prominent local component of the neurogenic niche is serotonin (5-HT). Modulating both proliferation and survival of newly generated cells, serotonin is a key regulator of adult neurogenesis (Alenina and Klempin, 2015) and, together with BDNF, is involved in antidepressant mechanisms (Mattson, 2008; Molendijk et al., 2011; Kronenberg et al., 2018a). Accumulating evidence from rodent studies and in vitro modeling indicates that microglia interact with local hormones and neurotransmitters by the expression of various receptors (Pocock and Kettenmann, 2007). Among them are metabotropic glutamate receptors, the chemokine fractalkine receptor (CX3CR1) (Sellner et al., 2016), and various serotonin receptor subtypes, particularly 5-HT2B (Krabbe et al., 2012). Expressed on microglia subpopulations (Kettenmann et al., 2011), receptors’ attraction to neuronal secretion of signaling molecules assists surveillance of the microenvironment (Szepesi et al., 2018).
Upon stimulation, microglia become activated, proliferate, lose their ramified morphology, and display the first innate immune defense (Beynon and Walker, 2012). They rapidly act by secretion of distinctive inflammatory cytokines, e.g., interleukins (ILs), interferons (IFNs), and tumor necrosis factors (TNFs) that in turn modulate the release of neurotransmitters and neurotrophins. Depending on the microenvironment, cytokines function pro- (i.e., IL-1β, IL-6, IL-18, and TNF-α) or anti-inflammatory (i.e., IL-4 and IL-10) (Suzumura, 2013; Franco and Fernández-Suárez, 2015). BDNF exerts primarily anti-inflammatory and neuroprotective effects (Chen et al., 2016). Dysregulation of the immune defense function leads to neuroinflammation and neuronal cell death. Excessive glutamate release is particularly neurotoxic (Lewerenz and Maher, 2015). Microglia-mediated “neuroinflammation” is increasingly recognized to contribute to the development and progression of neurodegenerative diseases and psychiatric disorders. Structural changes in neuroplasticity, altered intrinsic signaling, i.e., of serotonin and BDNF, and impaired neurogenesis are observed in stress-related events, Alzheimer’s disease (AD), or major depression. This review will summarize microglia function on precursor cells in the adult hippocampus, their contribution to neuroplasticity, and modulation by physiologic stimuli. We will synopsize how their behavior is altered upon activation leading to neurodegeneration and will discuss microglia response to serotonin signaling and 5-HT receptor function on microglial cells in vitro and in vivo. We will complete by describing the role of microglia in serotonin-mediated antidepressant action, e.g., in response to the selective serotonin reuptake inhibitor (SSRI) fluoxetine. Key findings are summarized in Table 1.
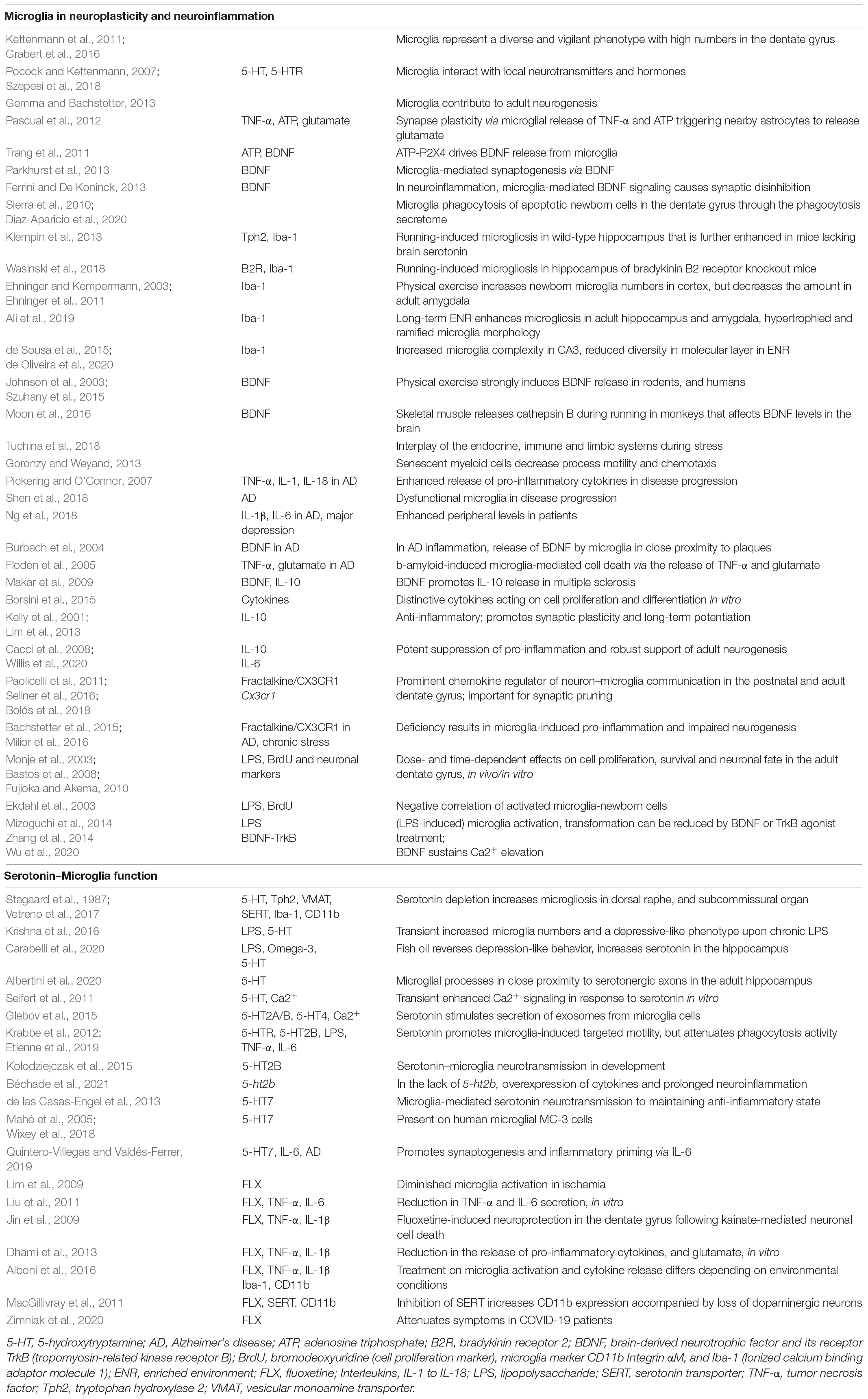
Table 1. Summary of recent findings on microglia function in neuroplasticity and neuro-inflammation in the hippocampus, with focus on serotonin and antidepressant action.
Microglia Function in Neuroplasticity—BDNF Signaling and Physiologic Stimuli
Neuroplasticity in the adult hippocampus enables its structure to adapt to environmental challenges and novel experiences by rewiring upon learning and to respond to trauma or injury. Specifically, the discovery that new neurons are continuously generated has stirred hope for new therapeutic strategies to improve cognitive function and to treat neurodegenerative disorders. Microglia contribute to adult neurogenesis and memory formation (Gemma and Bachstetter, 2013). In close proximity to neurons and dendritic spines, microglia control synapse connectivity via secretion of TNF-α and adenosine triphosphate (ATP) that in turn promote astrocyte-mediated neurotransmission (Figure 1; Pascual et al., 2012). Activation of the ATP receptor subtype P2X4 drives BDNF release from microglia, which might display a central pathway in microglia–neuron signaling (Trang et al., 2011). BDNF is crucially involved in neuronal maturation and neurotransmission via binding to tropomyosin-related kinase receptor B (TrkB) located on neurons (Mattson, 2008). Microglial release of BDNF directly affects nearby synapse connectivity and promotes neuronal TrkB phosphorylation that enhances microglia–neuron interplay in learning (Parkhurst et al., 2013). BDNF released by activated microglia alters neuronal excitability by causing synaptic disinhibition (Ferrini and De Koninck, 2013). In their major role in synaptic pruning, microglia actively engulf and remove dysfunctional synapses from neuronal cell bodies in the uninjured brain (Paolicelli et al., 2011). In vicinity to NSCs in the subgranular zone, they remove apoptotic progenitor cells within the first days of cell birth (Sierra et al., 2010) through the phagocytosis secretome (Diaz-Aparicio et al., 2020), thereby balancing synaptogenesis and cell death.
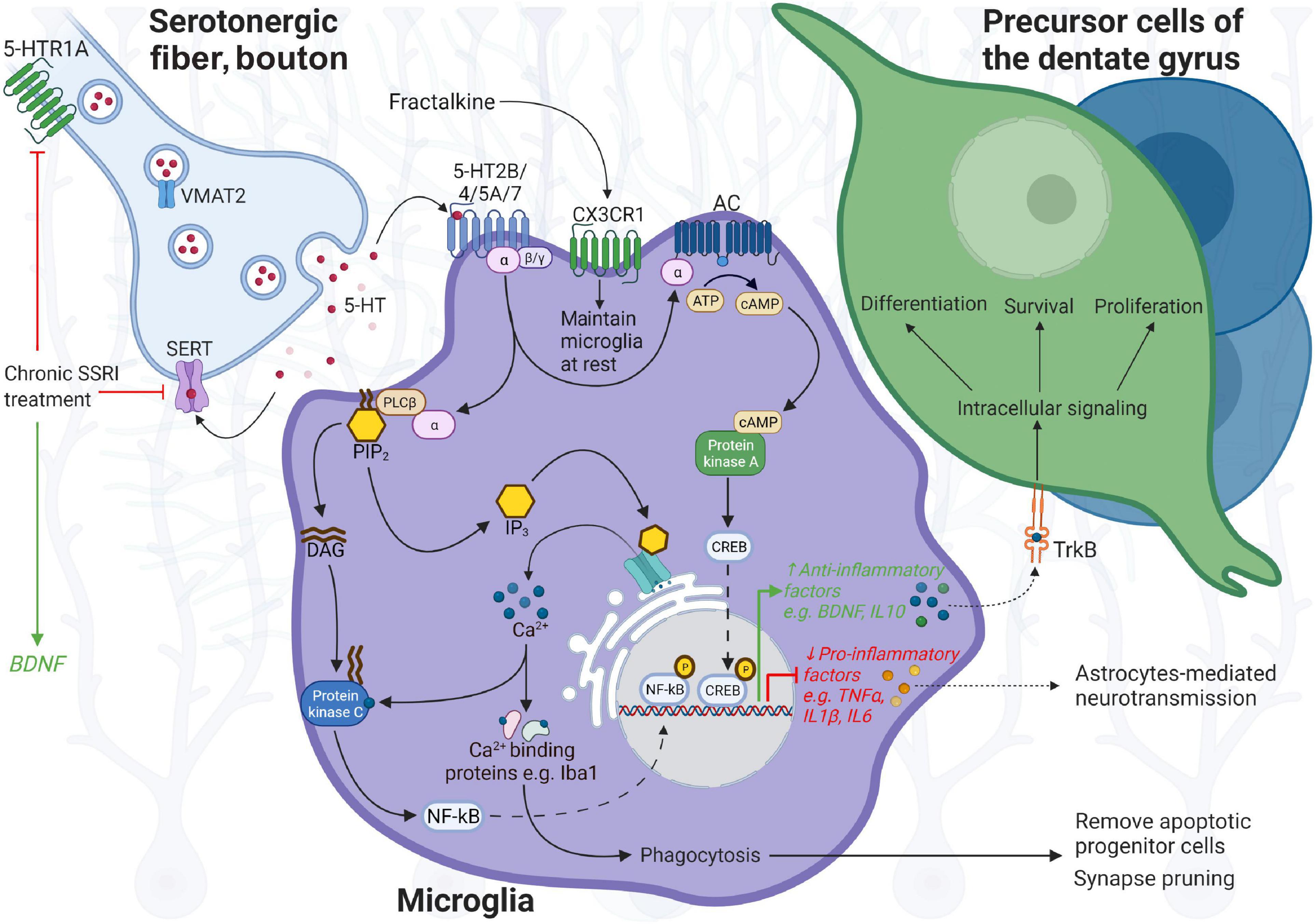
Figure 1. Illustration of microglia function in neuroplasticity of the hippocampus. In close proximity to precursor cells and neurons in the dentate gyrus, resting microglia control the neuronal cell pool by removal of apoptotic progenitor cells and synapse pruning, regulate synaptic plasticity and neural network excitability via ATP, and the release of TNF-α and BDNF, and respond to serotonin (5-HT) neurotransmission. Microglia express 5-HT receptors, most prominently 5-HT2B in response to serotonin, and CX3CR1 in response to neuronal fractalkine/CX3CL1 signaling that allows surveillance of the niche, and communication with neurons to maintain homeostasis. In particular, serotonin neurotransmission can direct microglia function toward neuroprotection or permit the response to inflammation. Dense tracts of serotonergic fibers terminate in the hippocampus. Upon receptor binding, 5-HT2B, coupled to Gαq/G11 protein, activates phospholipase C (PLC), which hydrolyzes phosphatidylinositol-4,5-bisphosphonate (PIP2), and mediates cellular effects through increasing levels of inositol triphosphate (IP3) and diacylglycerol (DAG). IP3 promotes Ca2+ excretion from endoplasmic reticulum, which activates Iba-1 involved in motility and phagocytosis activity of microglia, and is affected by 5-HT2B. IP3-induced Ca2+ release can also stimulate phospholipase C (PLC) (likewise via DAG), activating nuclear factor kappa beta (NF-kB) and in turn controls the expression of pro-inflammatory genes, e.g., TNF-α, IL-1β, and IL-6. Activated by 5-HT4 and 5-HT7 coupled to Gαs, the enzyme adenylate cyclase (AC) synthesizes the second messenger elevating cyclic AMP (cAMP) from ATP that activates protein kinase A (PKA); cAMP response element-binding protein (CREB) then controls transcription of genes involved in the anti-inflammatory response, BDNF or IL-10, exerting effects through their receptors, TrkB and IL-10R, located on precursor cells (TrkB) and neurons. 5-HT5A interactions with Gαi protein inhibit AC and downstream cascades. Upon harmful stimuli, microglia secrete pro-inflammatory cytokines, TNF-α, IL-1β, and IL-6, and actively remove cell debris. In prolonged neuroinflammation, microglia–neuron communication is altered, leading to neurodegeneration and cognitive deficits. In response to SSRIs, targeting SERT and presynaptic 5-HT1A auto-receptors on serotonergic neurons, 5-HT availability is enhanced in the synaptic cleft, which may also modulate BDNF levels. Increased release of pro-inflammatory cytokines may be counter-balanced by increased 5-HT levels upon SERT inhibition through fluoxetine—having anti-inflammatory properties. 5-HT, 5-hydroxytryptamine; BDNF, brain-derived neurotrophic factor and its receptor TrkB (tropomyosin-related kinase receptor B); Iba-1, ionized calcium binding adaptor molecule 1; SERT, serotonin transporter; TNF, tumor necrosis factor; VMAT2, vesicular monoamine transporter 2. BioRender was used to build the image.
Novel experiences and external stimuli influence NSC/progenitor behavior, and activity-dependent changes in neuroplasticity occur, including a robust increase in precursor cell proliferation upon running (van Praag et al., 1999; Kronenberg et al., 2003) and cell survival upon exposure to an enriched environment (ENR) (Kempermann and Gage, 1999). The neurogenic regulatory effect of running is mediated through central serotonin (Klempin et al., 2013), with circulatory factors, i.e., the angiotensin-converting enzyme 2 (Klempin et al., 2018) or cathepsin B released by skeletal muscle (Moon et al., 2016) contributing to increased precursor proliferation. Physical exercise increases microgliosis in the dentate gyrus of wild-type mice that is further enhanced in the absence of brain serotonin (Klempin et al., 2013), or in the lack of bradykinin B2 receptor (Wasinski et al., 2018). Physical activity increases the number of newborn microglia in the adult mouse cortex (Ehninger and Kempermann, 2003), while a reduction was observed in the adult amygdala upon running and ENR (Ehninger et al., 2011). While Iba-1 expression is reduced up to 2 months in ENR, long-term ENR conditions enhance microgliosis in adult hippocampus and amygdala accompanied by hypertrophied and ramified microglia morphology (Ali et al., 2019). Microglia proliferation and morphological transformation are characteristics of the vigilant phenotype that allows rapid adaptation to a demanding microenvironment. Thereby, cellular physiology including Ca2+ signaling and highly branched processes supports the sensor ability, while an amoeboid shape with dynamic extensions facilitates cellular locomotion toward the site of neural damage and factor release (Nayak et al., 2014). As a result of positive stimuli, physical exercise, and ENR, altered microglia phenotypes display neuroprotective functions. In ENR, microglia morphology in adult rodent brain shows increased complexity in CA3 (de Sousa et al., 2015) but decreased diversity in the molecular layer (de Oliveira et al., 2020). In animal models for several diseases, physical activity induces anti-inflammatory effects revealed by decreased microglia activation and Iba-1/CD11b expression, a ramified morphology, or normalization in synaptic density in CA3 (Andoh and Koyama, 2020). Exercise also strongly enhances BDNF signaling in mammals that in turn exerts positive cognitive effects (Johnson et al., 2003; Szuhany et al., 2015; Moon et al., 2016). Together, these studies show that microglia contribute to neuroplasticity and synaptic rewiring in the adult hippocampus and respond to physiologic stimuli that could ameliorate from pathologies.
Microglia Function in Neuroinflammation and Neurodegeneration
Neuroplasticity of the adult brain can also be negatively regulated, inducing structural changes and impaired neurogenesis as has been observed in stress-related events (Tuchina et al., 2018), and is associated with age-related cognitive decline and neurodegenerative and psychiatric disorders in humans. Upon inflammation or following infection, an acute immune response comprises the release of pro-inflammatory cytokines and phagocytosis of damaged cells, mainly neurons (Suzumura, 2013). Notably, activation of endogenous microglial cells goes along with migration of blood-derived cells into the brain, such as in ischemia (Kronenberg et al., 2018b). Chronically activated microglia, increased cell density and hyper-ramified morphology (Dubbelaar et al., 2018), and the enhanced release of pro-inflammatory cytokines, e.g., TNF-α, IL-1β, and IL-18, are observed in response to stress, major depression, or AD, leading to disease progression and brain damage (Pickering and O’Connor, 2007). This “hyperactivation of the immune response” might be due to inefficiency in the phagocytosis phenotype of microglia. Indeed, similar characteristics are observed for aging, senescent myeloid cells, where an impaired immune response results from decreased process motility and chemotaxis (Goronzy and Weyand, 2013). Dysfunctional microglia might be a hallmark of late-stage AD development (Shen et al., 2018). In particular, microglial cells are in close proximity to β-amyloid plaques, one of the characteristics of disease progression; their processes engulf β-amyloid that leads to enhanced pro-inflammatory signaling, e.g., of TNF-α inducing cell death and the release of BDNF (Burbach et al., 2004; Floden et al., 2005). Although BDNF is anti-inflammatory and considered as a therapeutic target, increased BDNF signaling might negatively contribute to the aberrant axonal growth in AD in its role as modulator of neuronal and synapse maturation in healthy conditions. However, in an animal model of multiple sclerosis, BDNF promotes IL-10 that reduces clinical severity (Makar et al., 2009). Notably, increased peripheral levels of IL-1β, and of IL-1β and IL-6, but unchanged TNF-α, were reported in patients with AD or major depression, respectively (Ng et al., 2018).
Microglial release of inflammatory factors in the dentate gyrus differentially affects precursor cell proliferation, survival, and differentiation (Borsini et al., 2015). Secretion of anti-inflammatory IL-10 is involved in synaptic plasticity and long-term potentiation (Kelly et al., 2001; Lim et al., 2013) and counteracts the pro-inflammatory phenotype of chronically activated microglia (Cacci et al., 2008). In traumatic brain injury, repopulated microglia can adopt a phenotype that drives repair, specifically by promoting adult neurogenesis via soluble IL-6 receptor (Willis et al., 2020). Increased release of pro-inflammatory cytokines is observed upon decreased microglial CX3CR1 expression in response to fractalkine/CX3CL1 signaling deficiency, which results in a dramatic reduction in adult neurogenesis in chronic stress (Milior et al., 2016) and in AD (Bachstetter et al., 2015). Microglia Cx3cr1 knockout mice display a transient early postnatal increase in synaptogenesis due to deficiency in synaptic pruning in the dentate gyrus (Paolicelli et al., 2011) that is independent of fractalkine signaling (Sellner et al., 2016), accompanied by reduced neuronal maturation of precursor cells and impaired learning and memory in the adult (Bolós et al., 2018). To model systemic inflammation in vitro/in vivo, bacterial lipopolysaccharide (LPS) is administered, resulting in increased microglia density and the release of pro-inflammatory factors IL-1β/IL-6 and TNF-α. A dose- and time-dependent decrease in proliferation and survival of precursor cells is observed in vivo in adult mouse and rat, respectively (Bastos et al., 2008; Fujioka and Akema, 2010), which is accompanied by a depressive-like state (Tang et al., 2016). Thereby, a dramatic reduction in newborn neurons is correlated with an increase in activated microglia (Ekdahl et al., 2003). In vitro experiments reveal that the number of precursor cells adopting a neuronal fate is significantly reduced when co-cultured with activated microglia expressing IL-6, while LPS added directly to precursor cells has no effect on neurogenesis (Monje et al., 2003). A single dose of LPS also significantly decreases the density of p-TrkB and BDNF protein in dentate gyrus and CA3 of young-adult male mice (Zhang et al., 2014). Likewise, an age-related decline in BDNF-TrkB signaling is accompanied by increased microglia activation (Wu et al., 2020). Vice versa, microglia activation, phenotypic transformation, and release of pro-inflammatory cytokines can be reduced by local supplement of BDNF in vivo/in vitro (Wu et al.) or TrkB agonist treatment in vivo (Zhang et al., 2014). BDNF induces sustained elevation of intracellular Ca2+ signaling and inhibits microglial production of nitric oxide (NO) (Mizoguchi et al., 2014).
Serotonin–Microglia Interplay
Serotonin is the most widespread monoamine of the central nervous system, key signaling molecule in neuroplasticity of the hippocampus, and target in antidepressant therapy. Briefly, synthesized in neurons of the brain stem dorsal and median raphe nuclei (DRN, MRN) by the rate-limiting enzyme tryptophan hydroxylase 2, serotonin is packed into synaptic vesicles by the vesicular monoamine transporter (VMAT) 2, and upon release, re-uptake is regulated by the selective serotonin transporter, SERT (Gaspar et al., 2003). Earlier studies on brain serotonin–microglia interaction were done upon stimuli or pharmacological depletion of serotonergic neurons that results in increased microglia density, characterized by Iba-1 and CD11b expression, in DRN (Vetreno et al., 2017), or microgliosis in the subependymal layer of the subcommissural organ in adult rats (Stagaard et al., 1987). Neuroinflammation induced by systemic LPS reduces serotonin levels in the hippocampus that is accompanied by a depressive-like phenotype in rats (Carabelli et al., 2020). Chronic LPS activation only transiently increases microglia numbers and alters striatal and prefrontal serotonin signaling alongside depressive-like behavior (Krishna et al., 2016). Omega-3 administration leads to increased serotonin levels in the hippocampus and reverses the behavioral phenotype (Carabelli et al., 2020). Serotonin fiber pathways project into numerous brain areas and spinal cord. Target areas in the dentate gyrus, precursor cells and neurons, express various 5-HT receptors that control the response from efferent activity at different cell stages within the neuronal lineage (Brezun and Daszuta, 2000; Klempin et al., 2010). Recent studies establish that neurotransmitter receptors are not specific for neurons, but can be found on glial cells, and molecules are detected through diffuse non-synaptic transmission in the extracellular space (Pocock and Kettenmann, 2007). Serotonin, in particular, is released via boutons en passant, and ultrastructure imaging reveals brain serotonin–microglia interplay in the hippocampus with microglia processes in close proximity to serotonergic axons (Albertini et al., 2020). Seven groups (5-HT1–5-HT7) and their subtypes, with 5-HT3 as an exception, belong to the G-protein-coupled receptor family regulating different signaling pathways; almost all of them are expressed on distinct microglia subpopulations (Krabbe et al., 2012; Glebov et al., 2015).
Accumulating evidence attributes 5-HT2B receptor subtype an important role in microglia–neuron communication in rodent brain development (Kolodziejczak et al., 2015), and in microglia-mediated serotonin transmission. In vitro studies reveal enhanced microglia response to injury in acute mouse brain slices (Krabbe et al., 2012), and transiently boosted Ca2+ signaling in cultured resting microglia upon serotonin administration (Seifert et al., 2011). Specifically, activation of 5-HT2B leads to enhanced motility and oriented growths of microglial processes that is important in response to injury but decreases the phagocytosis activity (Krabbe et al., 2012; Etienne et al., 2019). In the lifelong absence of microglial 5-ht2b, peripheral LPS injection causes cytokine overexpression and prolonged neuroinflammation in vivo that goes along with increased morphology transformation and hyper-ramification (Béchade et al., 2021). These studies suggest that serotonin is involved in the alterations of microglial phenotype as is known for peripheral macrophages (de las Casas-Engel et al., 2013). Together with 5-HT2, microglial expression of 5-HT4 is involved in the release of exosomes from microglia that is dependent on elevated cytosolic Ca2+ signaling (Glebov et al., 2015). Microglial secretion of cytokines modulated by serotonin neurotransmission might lead to maintenance of an anti-inflammatory state (de las Casas-Engel et al., 2013); indeed, secretion of pro-inflammatory factors TNF-α or IL-6 was unchanged during LPS stimulation in the presence of serotonin (Krabbe et al., 2012). Functional 5-HT7 receptors are present on human microglial MC-3 cells (Mahé et al., 2005; Wixey et al., 2018). 5-HT7 expressions on both neurons and microglia promote synaptogenesis and induce inflammatory priming via IL-6 production. In an AD animal model, reduced neurotoxicity of β-amyloid was observed in hippocampus upon administration of LP-211, a 5-HT7 agonist (Quintero-Villegas and Valdés-Ferrer, 2019). Collectively, these studies suggest serotonin’s role in keeping microglia in a resting, surveillance, and anti-inflammatory state.
Serotonin–Microglia Interplay Upon Fluoxetine
Dysregulation of serotonin signaling is associated with neurogenic decline, age-related memory loss, and psychiatric disorders. SSRIs increase serotonin neurotransmission targeting SERT and specific 5-HT (auto-) receptors (Descarries and Riad, 2012) that leads to clinical improvement and is linked to a delayed increase in adult neurogenesis as shown in rodents (Malberg et al., 2000; Santarelli et al., 2003). BDNF has been implicated in the pro-neurogenic effects; SSRI-induced increases in serum BDNF have been detected in rodents (Nibuya et al., 1996), and similarly in depressed patients (Molendijk et al., 2011); however, BDNF protein in hippocampus of mice is not elevated (Petermann et al., 2020). Increasing evidence indicates that neurodegenerative diseases and psychiatric disorders are characterized by an immune-inflammatory state and that antidepressants not only improve mood but also possess anti-inflammatory properties. It is suggested that hyperactive microglia and increased pro-inflammatory cytokine levels result in elevated SERT expression as a consequence or interdependency to elevated serotonin levels. SSRIs target SERT function that in addition to inhibiting serotonin re-uptake might activate anti-inflammatory intracellular pathways (Walker, 2013): In LPS-induced primary microglia culture, incubation with serotonin significantly alters TNF-α production (Tynan et al., 2012). Likewise, pre-treatment with five different SSRIs, including fluoxetine, substantially inhibits IL-1β or IL-6 secretion (Liu et al., 2011) and microglial production of TNF-α and NO, with cyclic adenosine monophosphate signaling involved in the regulation of an anti-inflammatory response (Tynan et al., 2012). Co-cultured with cortical neurons, microglial release of the pro-inflammatory factors IL-1β, TNF-α, and glutamate was reduced upon fluoxetine and citalopram (Dhami et al., 2013). In vivo pre-treatment with fluoxetine or paroxetine attenuates LPS-induced increases in TNF-α serum levels (Ohgi et al., 2013). In models of neurodegenerative disease, fluoxetine administration reduces microglia activation in ischemia (Lim et al., 2009) and leads to recovery from kainate-induced cell death in the dentate gyrus (Jin et al., 2009). Depending on environmental challenges, cytokine release in hippocampus is differentially affected by fluoxetine resulting in increased pro-inflammatory IL-1β expression in ENR conditions, but decreased TNF-α production upon stress. However, microglia density and Iba-1/CD11b expression in hippocampus remain unchanged (Alboni et al., 2016). In contrast, in substantia nigra, SERT inhibition by fluoxetine increases microglia activation and CD11b immunoreactivity, leading to loss of dopaminergic neurons (MacGillivray et al., 2011). Together, microglia activity and release of cytokines can be modulated by serotonin neurotransmission, e.g., SERT-mediated clearance of released serotonin upon fluoxetine (Robson et al., 2017) and altered intrinsic cellular signaling. However, whether SERT is expressed on microglia lacks evidence.
An overreaction of the immune system, a “cytokine storm” (Ragab et al., 2020) is also associated with the pathophysiology following SARS-CoV-2 infection that might contribute to long-term neurological impairments. Preliminary results reveal that fluoxetine treatment specifically decreases viral protein expression in COVID-19 patients (Zimniak et al., 2020). Thus, SSRI treatment with anti-inflammatory effects given early might prevent both severe progression of the disease and chronic despair.
Discussion
Over the past few years, it has become apparent that endogenous microglia of the adult brain take part in neuroplasticity of the hippocampus by controlling the neuronal cell pool, regulating synaptic plasticity in learning via release of TNF-α and BDNF, and responding to physical exercise (Table 1). Resting microglia express 5-HT2B and CX3CR1, constantly survey the niche’s microenvironment, and communicate with neurons to maintain homeostasis. In particular, serotonin neurotransmission can direct microglia function toward neuroprotection, or permit the response to inflammation (Pocock and Kettenmann, 2007). Upon harmful stimuli, microglia perform an innate immune response; secrete pro-inflammatory cytokines TNF-α, IL-1β, and IL-6; and actively remove cell debris, similar to peripheral macrophages. When toxic molecules are removed from the nervous tissue, microglia become “alternatively activated”, change their phenotype to anti-inflammatory (IL-10), and start restoring homeostasis (Lobo-Silva et al., 2016). However, in chronic diseases, neuron–microglia communication is somewhat altered, causing a prolonged inflammatory state, leading to impaired chemotaxis and phagocytosis. Hyperactivation of the immune response also impairs survival and differentiation of progenitor cells, which, together with impaired serotonin and BDNF signaling, are characteristics of major depression. With SSRIs such as fluoxetine targeting both signaling pathways and, in addition, enabling an anti-inflammatory response, microglia might display an add-on therapeutic target to improve psychiatric disorders, cognitive decline, or viral-induced neurological deficits. Nonetheless, considering the various factors involved and the vast heterogeneity of human microglial cells (Böttcher et al., 2019), there is a long road ahead.
Author Contributions
AT, OT, and FK have equally contributed to designed and wrote the manuscript. All authors contributed to the article and approved the submitted version.
Funding
The 5 Top 100 Russian Academic Excellence Project (to AT and OT) and Rahel Hirsch Fellowship (to FK) supported this work.
Conflict of Interest
The authors declare that the research was conducted in the absence of any commercial or financial relationships that could be construed as a potential conflict of interest.
References
Albertini, G., Etienne, F., and Roumier, A. (2020). Regulation of microglia by neuromodulators: modulations in major and minor modes. Neurosci. Lett. 733:135000. doi: 10.1016/j.neulet.2020.135000
Alboni, S., Poggini, S., Garofalo, S., Milior, G., El Hajj, H., Lecours, C., et al. (2016). Fluoxetine treatment affects the inflammatory response and microglial function according to the quality of the living environment. Brain Behav. Immun. 58, 261–271. doi: 10.1016/j.bbi.2016.07.155
Alenina, N., and Klempin, F. (2015). The role of serotonin in adult hippocampal neurogenesis. Behav. Brain Res. 277, 49–57. doi: 10.1016/j.bbr.2014.07.038
Ali, S., Liu, X., Queen, N. J., Patel, R. S., Wilkins, R. K., Mo, X., et al. (2019). Long-term environmental enrichment affects microglial morphology in middle age mice. Aging 11, 2388–2402. doi: 10.18632/aging.101923
Andoh, M., and Koyama, R. (2020). Exercise, microglia, and beyond – workout to communicate with microglia. Neural. Regen. Res. 15, 2029–2030. doi: 10.4103/1673-5374.282241
Bachstetter, A. D., Van Eldik, L. J., Schmitt, F. A., Neltner, J. H., Ighodaro, E. T., Webster, S. J., et al. (2015). Disease-related microglia heterogeneity in the hippocampus of Alzheimer’s disease, dementia with Lewy bodies, and hippocampal sclerosis of aging. Acta Neuropathol. Commun. 3:32.
Bastos, G. N., Moriya, T., Inui, F., Katura, T., and Nakahata, N. (2008). Involvement of cyclooxygenase-2 in lipopolysaccharide-induced impairment of the newborn cell survival in the adult mouse dentate gyrus. Neuroscience 155, 454–462. doi: 10.1016/j.neuroscience.2008.06.020
Béchade, C., D’Andrea, I., Etienne, F., Verdonk, F., Moutkine, I., Banas, S. M., et al. (2021). The serotonin 2B receptor is required in neonatal microglia to limit neuroinflammation and sickness behavior in adulthood. Glia 69, 638–654. doi: 10.1002/glia.23918
Beynon, S. B., and Walker, F. R. (2012). Microglial activation in the injured and healthy brain: what are we really talking about? Practical and theoretical issues associated with the measurement of changes in microglial morphology. Neuroscience 225, 162–171. doi: 10.1016/j.neuroscience.2012.07.029
Bolós, M., Perea, J. R., Terreros-Roncal, J., Pallas-Bazarra, N., Jurado-Arjona, J., Ávila, J., et al. (2018). Absence of microglial CX3CR1 impairs the synaptic integration of adult-born hippocampal granule neurons. Brain Behav. Immun. 68, 76–89. doi: 10.1016/j.bbi.2017.10.002
Borsini, A., Zunszain, P. A., Thuret, S., and Pariante, C. M. (2015). The role of inflammatory cytokines as key modulators of neurogenesis. Trends Neurosci. 38, 145–157. doi: 10.1016/j.tins.2014.12.006
Böttcher, C., Schlickeiser, S., Sneeboer, M. A. M., Kunkel, D., Knop, A., Paza, E., et al. (2019). Human microglia regional heterogeneity and phenotypes determined by multiplexed single-cell mass cytometry. Nat. Neurosci. 22, 78–90. doi: 10.1038/s41593-018-0290-2
Brezun, J. M., and Daszuta, A. (2000). Serotonergic reinnervation reverses lesion-induced decreases in PSA-NCAM labeling and proliferation of hippocampal cells in adult rats. Hippocampus 10, 37–46. doi: 10.1002/(sici)1098-1063(2000)10:1<37::aid-hipo4>3.0.co;2-c
Burbach, G. J., Hellweg, R., Haas, C. A., Del Turco, D., Deicke, U., Abramowski, D., et al. (2004). Induction of brain-derived neurotrophic factor in plaque-associated glial cells of aged APP23 transgenic mice. J. Neurosci. 24, 2421–2430. doi: 10.1523/jneurosci.5599-03.2004
Buzsaki, G., and Moser, E. I. (2013). Memory, navigation and theta rhythm in the hippocampal-entorhinal system. Nat. Neurosci. 16, 130–138. doi: 10.1038/nn.3304
Cacci, E., Ajmone-Cat, M. A., Anelli, T., Biagioni, S., and Minghetti, L. (2008). In vitro neuronal and glial differentiation from embryonic or adult neural precursor cells are differently affected by chronic or acute activation of microglia. Glia 56, 412–425. doi: 10.1002/glia.20616
Carabelli, B., Delattre, A. M., Waltrick, A. P. F., Araújo, G., Suchecki, D., Machado, R. B., et al. (2020). Fish-oil supplementation decreases Indoleamine-2,3-Dioxygenase expression and increases hippocampal serotonin levels in the LPS depression model. Behav. Brain Res. 390:112675. doi: 10.1016/j.bbr.2020.112675
Chen, J. J., Wang, T., An, C. D., Jiang, C. Y., Zhao, J., and Li, S. (2016). Brain-derived neurotrophic factor: a mediator of inflammation-associated neurogenesis in Alzheimer’s disease. Rev. Neurosci. 27, 793–811. doi: 10.1515/revneuro-2016-0017
Dayer, A. G., Ford, A. A., Cleaver, K. M., Yassaee, M., and Cameron, H. A. (2003). Short-term and long-term survival of new neurons in the rat dentate gyrus. J. Comp. Neurol. 460, 563–572. doi: 10.1002/cne.10675
de las Casas-Engel, M., Domínguez-Soto, A., Sierra-Filardi, E., Bragado, R., Nieto, C., Puig-Kroger, A., et al. (2013). Serotonin skews human macrophage polarization through HTR2B and HTR7. J. Immunol. 190, 2301–2310. doi: 10.4049/jimmunol.1201133
de Oliveira, T. C. G., Carvalho-Paulo, D., de Lima, C. M., de Oliveira, R. B., Santos Filho, C., Diniz, D. G., et al. (2020). Long-term environmental enrichment reduces microglia morphological diversity of the molecular layer of dentate gyrus. Eur. J. Neurosci. 52, 4081–4099. doi: 10.1111/ejn.14920
de Sousa, A. A., Dos Reis, R. R., de Lima, C. M., de Oliveira, M. A., Fernandes, T. N., Gomes, G. F., et al. (2015). Three-dimensional morphometric analysis of microglial changes in a mouse model of virus encephalitis: age and environmental influences. Eur. J. Neurosci. 42, 2036–2050. doi: 10.1111/ejn.12951
Descarries, L., and Riad, M. (2012). Effects of the antidepressant fluoxetine on the subcellular localization of 5-HT1A receptors and SERT. Philos. Trans. R. Soc. Lond. B Biol. Sci. 367, 2416–2425. doi: 10.1098/rstb.2011.0361
Dhami, K. S., Churchward, M. A., Baker, G. B., and Todd, K. G. (2013). Fluoxetine and citalopram decrease microglial release of glutamate and D-serine to promote cortical neuronal viability following ischemic insult. Mol. Cell. Neurosci. 56, 365–374. doi: 10.1016/j.mcn.2013.07.006
Diaz-Aparicio, I., Paris, I., Sierra-Torre, V., Plaza-Zabala, A., Rodríguez-Iglesias, N., Márquez-Ropero, M., et al. (2020). Microglia actively remodel adult hippocampal neurogenesis through the phagocytosis secretome. J. Neurosci. 40, 1453–1482. doi: 10.1523/jneurosci.0993-19.2019
Dubbelaar, M. L., Kracht, L., Eggen, B. J. L., and Boddeke, E. (2018). The kaleidoscope of microglialphenotypes. Front. Immunol. 9:1753. doi: 10.3389/fimmu.2018.01753
Ehninger, D., and Kempermann, G. (2003). Regional effects of wheel running and environmental enrichment on cell genesis and microglia proliferation in the adult murine neocortex. Cereb. Cortex 13, 845–851. doi: 10.1093/cercor/13.8.845
Ehninger, D., Wang, L. P., Klempin, F., Romer, B., Kettenmann, H., and Kempermann, G. (2011). Enriched environment and physical activity reduce microglia and influence the fate of NG2 cells in the amygdala of adult mice. Cell Tissue Res. 345, 69–86. doi: 10.1007/s00441-011-1200-z
Ekdahl, C. T., Claasen, J. H., Bonde, S., Kokaia, Z., and Lindvall, O. (2003). Inflammation is detrimental for neurogenesis in adult brain. Proc. Natl. Acad. Sci. U. S. A. 100, 13632–13637.
Etienne, F., Mastrolia, V., Maroteaux, L., Girault, J. A., Gervasi, N., and Roumier, A. (2019). Two-photon imaging of microglial processes’ attraction toward ATP or serotonin in acute brain slices. J. Vis. Exp. 143:e58788.
Ferrini, F., and De Koninck, Y. (2013). Microglia control neuronal network excitability via BDNF signalling. Neural Plast. 2013:429815.
Floden, A. M., Li, S., and Combs, C. K. (2005). Beta-amyloid-stimulated microglia induce neuron death via synergistic stimulation of tumor necrosis factor alpha and NMDA receptors. J. Neurosci. 25, 2566–2575. doi: 10.1523/jneurosci.4998-04.2005
Franco, R., and Fernández-Suárez, D. (2015). Alternatively activated microglia and macrophages in the central nervous system. Prog. Neurobiol. 131, 65–86. doi: 10.1016/j.pneurobio.2015.05.003
Fujioka, H., and Akema, T. (2010). Lipopolysaccharide acutely inhibits proliferation of neural precursor cells in the dentate gyrus in adult rats. Brain Res. 1352, 35–42. doi: 10.1016/j.brainres.2010.07.032
Gaspar, P., Cases, O., and Maroteaux, L. (2003). The developmental role of serotonin: news from mouse molecular genetics. Nat. Rev. Neurosci. 4, 1002–1012. doi: 10.1038/nrn1256
Gemma, C., and Bachstetter, A. D. (2013). The role of microglia in adult hippocampal neurogenesis. Front. Cell. Neurosci. 7:229. doi: 10.3389/fncel.2013.00229
Glebov, K., Löchner, M., Jabs, R., Lau, T., Merkel, O., Schloss, P., et al. (2015). Serotonin stimulates secretion of exosomes from microglia cells. Glia 63, 626–634. doi: 10.1002/glia.22772
Goronzy, J. J., and Weyand, C. M. (2013). Understanding immunosenescence to improve responses to vaccines. Nat. Immun. 14, 428–436. doi: 10.1038/ni.2588
Grabert, K., Michoel, T., Karavolos, M. H., Clohisey, S., Baillie, J. K., Stevens, M. P., et al. (2016). Microglial brain region-dependent diversity and selective regional sensitivities to aging. Nat. Neurosci. 19, 504–516. doi: 10.1038/nn.4222
Jin, Y., Lim, C. M., Kim, S. W., Park, J. Y., Seo, J. S., Han, P. L., et al. (2009). Fluoxetine attenuates kainic acid-induced neuronal cell death in the mouse hippocampus. Brain Res. 1281, 108–116. doi: 10.1016/j.brainres.2009.04.053
Johnson, R. A., Rhodes, J. S., Jeffrey, S. L., Garland, T. Jr., and Mitchell, G. S. (2003). Hippocampal brain-derived neurotrophic factor but not neurotrophin-3 increases more in mice selected for increased voluntary wheel running. Neuroscience 121, 1–7. doi: 10.1016/s0306-4522(03)00422-6
Kelly, A., Lynch, A., Vereker, E., Nolan, Y., Queenan, P., Whittaker, E., et al. (2001). The anti-inflammatory cytokine, interleukin (IL)-10, blocks the inhibitory effect of IL-1 beta on long term potentiation. A role for JNK. J. Biol. Chem. 276, 45564–45572. doi: 10.1074/jbc.m108757200
Kempermann, G., and Gage, F. H. (1999). Experience-dependent regulation of adult hippocampal neurogenesis: effects of long-term stimulation and stimulus withdrawal. Hippocampus 9, 321–332. doi: 10.1002/(sici)1098-1063(1999)9:3<321::aid-hipo11>3.0.co;2-c
Kettenmann, H., Hanisch, U. K., Noda, M., and Verkhratsky, A. (2011). Physiology of microglia. Physiol. Rev. 91, 461–553.
Klempin, F., Babu, H., De Pietri Tonelli, D., Alarcon, E., Fabel, K., and Kempermann, G. (2010). Oppositional effects of serotonin receptors 5-HT1a, 2, and 2c in the regulation of adult hippocampal neurogenesis. Front. Mol. Neurosci. 3:14. doi: 10.3389/fnmol.2010.00014
Klempin, F., Beis, D., Mosienko, V., Kempermann, G., Bader, M., and Alenina, N. (2013). Serotonin is required for exercise-induced adult hippocampal neurogenesis. J. Neurosci. 33, 8270–8275. doi: 10.1523/jneurosci.5855-12.2013
Klempin, F., Mosienko, V., Matthes, S., Villela, D. C., Todiras, M., Penninger, J. M., et al. (2018). Depletion of angiotensin-converting enzyme 2 reduces brain serotonin and impairs the running-induced neurogenic response. Cell. Mol. Life Sci. 75, 3625–3634. doi: 10.1007/s00018-018-2815-y
Kolodziejczak, M., Béchade, C., Gervasi, N., Irinopoulou, T., Banas, S. M., Cordier, C., et al. (2015). Serotonin modulates developmental microglia via 5-HT2B receptors: potential implication during synaptic refinement of retinogeniculate projections. ACS Chem. Neurosci. 6, 1219–1230. doi: 10.1021/cn5003489
Krabbe, G., Matyash, V., Pannasch, U., Mamer, L., Boddeke, H. W., and Kettenmann, H. (2012). Activation of serotonin receptors promotes microglial injury-induced motility but attenuates phagocytic activity. Brain Behav. Immun. 26, 419–428. doi: 10.1016/j.bbi.2011.12.002
Krishna, S., Dodd, C. A., and Filipov, N. M. (2016). Behavioral and monoamine perturbations in adult male mice with chronic inflammation induced by repeated peripheral lipopolysaccharide administration. Behav. Brain Res. 302, 279–290. doi: 10.1016/j.bbr.2016.01.038
Kronenberg, G., Petermann, M., Dormann, C., Bader, M., Gass, P., Hellweg, R., et al. (2018a). Brain serotonin critically contributes to the biological effects of electroconvulsive seizures. Eur. Arch. Psychiatry Clin. Neurosci. 268, 861–864. doi: 10.1007/s00406-018-0924-0
Kronenberg, G., Reuter, K., Steiner, B., Brandt, M. D., Jessberger, S., Yamaguchi, M., et al. (2003). Subpopulations of proliferating cells of the adult hippocampus respond differently to physiologic neurogenic stimuli. J. Comp. Neurol. 467, 455–463. doi: 10.1002/cne.10945
Kronenberg, G., Uhlemann, R., Richter, N., Klempin, F., Wegner, S., Staerck, L., et al. (2018b). Distinguishing features of microglia- and monocyte-derived macrophages after stroke. Acta Neuropathol. 135, 551–568. doi: 10.1007/s00401-017-1795-6
Lewerenz, J., and Maher, P. (2015). Chronic glutamate toxicity in neurodegenerative diseases-what is the evidence? Front. Neurosci. 9:469. doi: 10.3389/fnins.2015.00469
Lim, C. M., Kim, S. W., Park, J. Y., Kim, C., Yoon, S. H., and Lee, J. K. (2009). Fluoxetine affords robust neuroprotection in the postischemic brain via its anti-inflammatory effect. J. Neurosci. Res. 87, 1037–1045. doi: 10.1002/jnr.21899
Lim, S. H., Park, E., You, B., Jung, Y., Park, A. R., Park, S. G., et al. (2013). Neuronal synapse formation induced by microglia and interleukin 10. PLoS One 8:e81218. doi: 10.1371/journal.pone.0081218
Liu, D., Wang, Z., Liu, S., Wang, F., Zhao, S., and Hao, A. (2011). Anti-inflammatory effects of fluoxetine in lipopolysaccharide(LPS)-stimulated microglial cells. Neuropharmacology 61, 592–599. doi: 10.1016/j.neuropharm.2011.04.033
Lobo-Silva, D., Carriche, G. M., Castro, A. G., Roque, S., and Saraiva, M. (2016). Balancing the immune response in the brain: IL-10 and its regulation. J. Neuroinflamm. 13:297.
MacGillivray, L., Reynolds, K. B., Sickand, M., Rosebush, P. I., and Mazurek, M. F. (2011). Inhibition of the serotonin transporter induces microglial activation and downregulation of dopaminergic neurons in the substantia nigra. Synapse 65, 1166–1172. doi: 10.1002/syn.20954
Mahé, C., Loetscher, E., Dev, K. K., Bobirnac, I., Otten, U., and Schoeffter, P. (2005). Serotonin 5-HT7 receptors coupled to induction of interleukin-6 in human microglial MC-3 cells. Neuropharmacology 49, 40–47. doi: 10.1016/j.neuropharm.2005.01.025
Makar, T. K., Bever, C. T., Singh, I. S., Royal, W., Sahu, S. N., Sura, T. P., et al. (2009). Brain-derived neurotrophic factor gene delivery in an animal model of multiple sclerosis using bone marrow stem cells as a vehicle. J. Neuroimmunol. 210, 40–51. doi: 10.1016/j.jneuroim.2009.02.017
Malberg, J. E., Eisch, A. J., Nestler, E. J., and Duman, R. S. (2000). Chronic antidepressant treatment increases neurogenesis in adult rat hippocampus. J. Neurosci. 20, 9104–9110. doi: 10.1523/jneurosci.20-24-09104.2000
Mattson, M. P. (2008). Glutamate and neurotrophic factors in neuronal plasticity and disease. Ann. N. Y. Acad. Sci. 1144, 97–112. doi: 10.1196/annals.1418.005
Milior, G., Lecours, C., Samson, L., Bisht, K., Poggini, S., Pagani, F., et al. (2016). Fractalkine receptor deficiency impairs microglial and neuronal responsiveness to chronic stress. Brain Behav. Immun. 55, 114–125. doi: 10.1016/j.bbi.2015.07.024
Mizoguchi, Y., Kato, T. A., Seki, Y., Ohgidani, M., Sagata, N., Horikawa, H., et al. (2014). Brain-derived neurotrophic factor (BDNF) induces sustained intracellular Ca2+ elevation through the up-regulation of surface transient receptor potential 3 (TRPC3) channels in rodent microglia. J. Biol. Chem. 289, 18549–18555. doi: 10.1074/jbc.m114.555334
Molendijk, M. L., Bus, B. A., Spinhoven, P., Penninx, B. W., Kenis, G., Prickaerts, J., et al. (2011). Serum levels of brain-derived neurotrophic factor in major depressive disorder: state-trait issues, clinical features and pharmacological treatment. Mol. Psychiatry 16, 1088–1095. doi: 10.1038/mp.2010.98
Monje, M. L., Toda, H., and Palmer, T. D. (2003). Inflammatory blockade restores adult hippocampal neurogenesis. Science 302, 1760–1765. doi: 10.1126/science.1088417
Moon, H. Y., Becke, A., Berron, D., Becker, B., Sah, N., Benoni, G., et al. (2016). Running-induced systemic cathepsin B secretion is associated with memory function. Cell Metab. 24, 332–340. doi: 10.1016/j.cmet.2016.05.025
Nayak, D., Roth, T. L., and McGavern, D. B. (2014). Microglia development and function. Annu. Rev. Immunol. 32, 367–402.
Ng, A., Tam, W. W., Zhang, M. W., Ho, C. S., Husain, S. F., McIntyre, R. S., et al. (2018). IL-1β, IL-6, TNF- α and CRP in elderly patients with depression or alzheimer’s disease: systematic review and meta-analysis. Sci. Rep. 8:12050.
Nibuya, M., Nestler, E. J., and Duman, R. S. (1996). Chronic antidepressant administration increases the expression of cAMP response element binding protein (CREB) in rat hippocampus. J. Neurosci. 16, 2365–2372. doi: 10.1523/jneurosci.16-07-02365.1996
Ohgi, Y., Futamura, T., Kikuchi, T., and Hashimoto, K. (2013). Effects of antidepressants on alternations in serum cytokines and depressive-like behavior in mice after lipopolysaccharide administration. Pharmacol. Biochem. Behav. 103, 853–859. doi: 10.1016/j.pbb.2012.12.003
Paolicelli, R. C., Bolasco, G., Pagani, F., Maggi, L., Scianni, M., Panzanelli, P., et al. (2011). Synaptic pruning by microglia is necessary for normal brain development. Science 333, 1456–1458. doi: 10.1126/science.1202529
Parkhurst, C. N., Yang, G., Ninan, I., Savas, J. N., Yates, J. R. III, Lafaille, J. J., et al. (2013). Microglia promote learning-dependent synapse formation through brain-derived neurotrophic factor. Cell 155, 1596–1609. doi: 10.1016/j.cell.2013.11.030
Pascual, O., Ben Achour, S., Rostaing, P., Triller, A., and Bessis, A. (2012). Microglia activation triggers astrocyte-mediated modulation of excitatory neurotransmission. Proc. Natl. Acad. Sci. U. S. A. 109, E197–E205.
Petermann, M., Kronenberg, G., Mosienko, V., Bader, M., Alenina, N., Hellweg, R., et al. (2020). Alterations in BDNF protein concentrations in the hippocampus do not explain the pro-neurogenic effect of citalopram on adult neurogenesis. Pharmacopsychiatry [Epub ahead of print]
Pickering, M., and O’Connor, J. J. (2007). Pro-inflammatory cytokines and their effects in the dentate gyrus. Prog. Brain Res. 163, 339–354. doi: 10.1016/s0079-6123(07)63020-9
Pocock, J. M., and Kettenmann, H. (2007). Neurotransmitter receptors on microglia. Trends Neurosci. 30, 527–535. doi: 10.1016/j.tins.2007.07.007
Quintero-Villegas, A., and Valdés-Ferrer, S. I. (2019). Role of 5-HT(7) receptors in the immune system in health and disease. Mol. Med. 26:2.
Ragab, D., Salah Eldin, H., Taeimah, M., Khattab, R., and Salem, R. (2020). The COVID-19 cytokine storm; what we know so far. Front. Immunol. 11:1446. doi: 10.3389/fimmu.2020.01446
Robson, M. J., Quinlan, M. A., and Blakely, R. D. (2017). Immune system activation and depression: roles of serotonin in the central nervous system and periphery. ACS Chem. Neurosci. 8, 932–942. doi: 10.1021/acschemneuro.6b00412
Santarelli, L., Saxe, M., Gross, C., Surget, A., Battaglia, F., Dulawa, S., et al. (2003). Requirement of hippocampal neurogenesis for the behavioral effects of antidepressants. Science 301, 805–809. doi: 10.1126/science.1083328
Seifert, S., Pannell, M., Uckert, W., Färber, K., and Kettenmann, H. (2011). Transmitter- and hormone-activated Ca(2+) responses in adult microglia/brain macrophages in situ recorded after viral transduction of a recombinant Ca(2+) sensor. Cell Calcium 49, 365–375. doi: 10.1016/j.ceca.2011.03.005
Sellner, S., Paricio-Montesinos, R., Spieß, A., Masuch, A., Erny, D., Harsan, L. A., et al. (2016). Microglial CX3CR1 promotes adult neurogenesis by inhibiting Sirt 1/p65 signaling independent of CX3CL1. Acta Neuropathol. Commun. 4:102.
Shen, Z., Bao, X., and Wang, R. (2018). Clinical PET imaging of microglial activation: implications for microglial therapeutics in alzheimer’s disease. Front. Aging Neurosci. 10:314. doi: 10.3389/fnagi.2018.00314
Sierra, A., Encinas, J. M., Deudero, J. J., Chancey, J. H., Enikolopov, G., Overstreet-Wadiche, L. S., et al. (2010). Microglia shape adult hippocampal neurogenesis through apoptosis-coupled phagocytosis. Cell Stem Cell 7, 483–495. doi: 10.1016/j.stem.2010.08.014
Stagaard, M., Balslev, Y., Lundberg, J. J., and Møllgård, K. (1987). Microglia in the hypendyma of the rat subcommissural organ following brain lesion with serotonin neurotoxin. J. Neurocytol. 16, 131–142. doi: 10.1007/bf02456704
Suzumura, A. (2013). Neuron-microglia interaction in neuroinflammation. Curr. Protein Pept. Sci. 14, 16–20. doi: 10.2174/1389203711314010004
Szepesi, Z., Manouchehrian, O., Bachiller, S., and Deierborg, T. (2018). Bidirectional microglia-neuron communication in health and disease. Front. Cell. Neurosci. 12:323. doi: 10.3389/fncel.2018.00323
Szuhany, K. L., Bugatti, M., and Otto, M. W. (2015). A meta-analytic review of the effects of exercise on brain-derived neurotrophic factor. J. Psychiatr. Res. 60, 56–64. doi: 10.1016/j.jpsychires.2014.10.003
Tang, M. M., Lin, W. J., Pan, Y. Q., Guan, X. T., and Li, Y. C. (2016). Hippocampal neurogenesis dysfunction linked to depressive-like behaviors in a neuroinflammation induced model of depression. Physiol. Behav. 161, 166–173. doi: 10.1016/j.physbeh.2016.04.034
Trang, T., Beggs, S., and Salter, M. W. (2011). Brain-derived neurotrophic factor from microglia: a molecular substrate for neuropathic pain. Neuron Glia Biol. 7, 99–108. doi: 10.1017/s1740925x12000087
Tuchina, O., Sidorova, M., Turkin, A., Shvaiko, D., Shalaginova, I., and Vakolyuk, I. A. (2018). Molecular mechanisms of neuroinflammation initiation and development in a model of posttraumatic stress disorder. Genes Cells XIII 47–55.
Tynan, R. J., Weidenhofer, J., Hinwood, M., Cairns, M. J., Day, T. A., and Walker, F. R. (2012). A comparative examination of the anti-inflammatory effects of SSRI and SNRI antidepressants on LPS stimulated microglia. Brain Behav. Immun. 26, 469–479. doi: 10.1016/j.bbi.2011.12.011
van Praag, H., Kempermann, G., and Gage, F. H. (1999). Running increases cell proliferation and neurogenesis in the adult mouse dentate gyrus. Nat. Neurosci. 2, 266–270. doi: 10.1038/6368
Vetreno, R. P., Patel, Y., Patel, U., Walter, T. J., and Crews, F. T. (2017). Adolescent intermittent ethanol reduces serotonin expression in the adult raphe nucleus and upregulates innate immune expression that is prevented by exercise. Brain Behav. Immun. 60, 333–345. doi: 10.1016/j.bbi.2016.09.018
Walker, F. R. (2013). A critical review of the mechanism of action for the selective serotonin reuptake inhibitors: do these drugs possess anti-inflammatory properties and how relevant is this in the treatment of depression? Neuropharmacology 67, 304–317. doi: 10.1016/j.neuropharm.2012.10.002
Wasinski, F., Batista, R. O., Bader, M., Araujo, R. C., and Klempin, F. (2018). Bradykinin B2 receptor is essential to running-induced cell proliferation in the adult mouse hippocampus. Brain struct. Funct. 223, 3901–3907. doi: 10.1007/s00429-018-1711-4
Willis, E. F., MacDonald, K. P. A., Nguyen, Q. H., Garrido, A. L., Gillespie, E. R., Harley, S. B. R., et al. (2020). Repopulating microglia promote brain repair in an IL-6-dependent manner. Cell 180, 833–846.e16.
Wixey, J. A., Reinebrant, H. E., Chand, K. K., and Buller, K. M. (2018). Disruption to the 5-HT(7) receptor following hypoxia-ischemia in the immature rodent brain. Neurochem. Res. 43, 711–720. doi: 10.1007/s11064-018-2473-3
Wu, S. Y., Pan, B. S., Tsai, S. F., Chiang, Y. T., Huang, B. M., Mo, F. E., et al. (2020). BDNF reverses aging-related microglial activation. J. Neuroinflamm. 17:210.
Zhang, J. C., Wu, J., Fujita, Y., Yao, W., Ren, Q., Yang, C., et al. (2014). Antidepressant effects of TrkB ligands on depression-like behavior and dendritic changes in mice after inflammation. Int. J. Neuropsychopharmacol. 18:pyu077.
Keywords: microglia, serotonin, hippocampus, BDNF, fluoxetine, neuroinflammation
Citation: Turkin A, Tuchina O and Klempin F (2021) Microglia Function on Precursor Cells in the Adult Hippocampus and Their Responsiveness to Serotonin Signaling. Front. Cell Dev. Biol. 9:665739. doi: 10.3389/fcell.2021.665739
Received: 08 February 2021; Accepted: 12 April 2021;
Published: 24 May 2021.
Edited by:
Carmen Castro, University of Cádiz, SpainReviewed by:
Akira Monji, Saga University, JapanRita Gaspar, Coimbra Institute for Biomedical Imaging and Translational Research (CIBIT), Portugal
Copyright © 2021 Turkin, Tuchina and Klempin. This is an open-access article distributed under the terms of the Creative Commons Attribution License (CC BY). The use, distribution or reproduction in other forums is permitted, provided the original author(s) and the copyright owner(s) are credited and that the original publication in this journal is cited, in accordance with accepted academic practice. No use, distribution or reproduction is permitted which does not comply with these terms.
*Correspondence: Friederike Klempin, ZnJpZWRlcmlrZS5rbGVtcGluQGNoYXJpdGUuZGU=