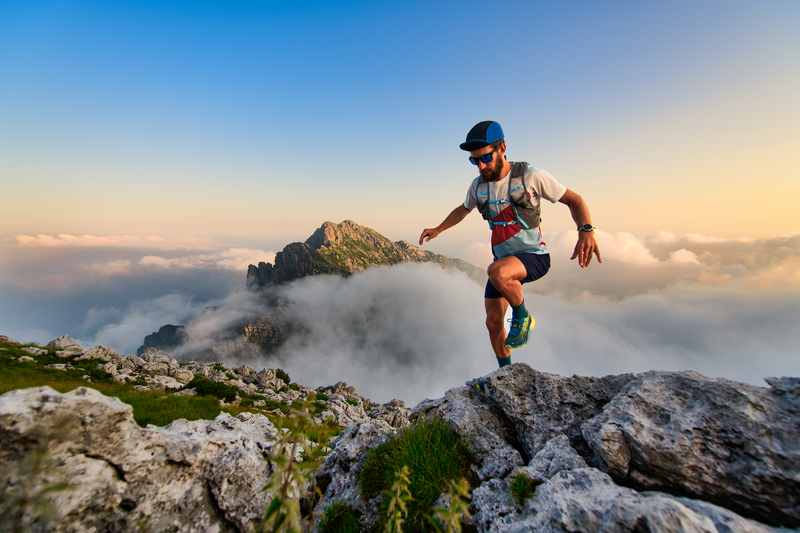
95% of researchers rate our articles as excellent or good
Learn more about the work of our research integrity team to safeguard the quality of each article we publish.
Find out more
REVIEW article
Front. Cell Dev. Biol. , 15 April 2021
Sec. Stem Cell Research
Volume 9 - 2021 | https://doi.org/10.3389/fcell.2021.665412
This article is part of the Research Topic Mesenchymal Stem Cell Senescence and Rejuvenation View all 13 articles
The regenerative and immunomodulatory properties of mesenchymal stem cells (MSCs) have laid a sound foundation for their clinical application in various diseases. However, the clinical efficiency of MSC treatments varies depending on certain cell characteristics. Among these, the roles of cell aging or senescence cannot be excluded. Despite their stemness, evidence of senescence in MSCs has recently gained attention. Many factors may contribute to the senescence of MSCs, including MSC origin (biological niche), donor conditions (age, obesity, diseases, or unknown factors), and culture conditions in vitro. With the rapidly increasing prevalence of diabetes mellitus (DM) and gestational diabetes mellitus (GDM), the effects of hyperglycemia on the senescence of MSCs should be evaluated to improve the application of autologous MSCs. This review aims to present the available data on the senescence of MSCs, its relationship with hyperglycemia, and the strategies to suppress the senescence of MSCs in a hyperglycemic environment.
Mesodermal mesenchymal stem cells (MSCs) have self-renewal and multi-differentiation potential and can ultimately differentiate into osteoblasts, chondrocytes, and adipocytes (Timaner et al., 2020). The regenerative and immunomodulatory properties of MSCs have attracted the attention of researchers and doctors worldwide, because of their potential for cell-based therapy (Ankrum et al., 2014; Kornicka et al., 2018). Strong evidences exist to support the effectiveness of MSC treatments in managing degenerative diseases such as diabetes mellitus, myocardial infarction, liver failure, osteoarthritis, and Alzheimer’s disease (Hunsberger et al., 2016; Packer, 2018; Shi et al., 2018; Wang, 2018). However, the inconsistent results of many clinical trials have hampered their clinical application (Galipeau and Sensébé, 2018). Currently known reasons for these inconsistencies include MSC origin (biological niche), donor characteristics (age, obesity, diseases, or unknown factors), and culture conditions in vitro (Costa et al., 2020). However, the senescence of MSCs has often been overlooked. Autologous MSCs are considered auspicious because patient-derived cells are easily available and do not require sustained immunosuppression (Golpanian et al., 2016), but their senescence due to the personalized microenvironment should be addressed.
Hyperglycemia, oxidative stress, and altered immune reactions are prominent features of the diabetic microenvironment, all of which have been demonstrated to change bone marrow-derived MSC (BMSC) and adipose-derived MSC (AMSC) properties and functions, leading to MSC senescence (Cramer et al., 2010; Mahmoud et al., 2019). Moreover, umbilical cord-derived MSCs (UCMSCs) extracted from obese/diabetic mothers display premature senescence and mitochondrial dysfunction (Kim et al., 2015). With the rapidly increasing prevalence of diabetes and gestational diabetes mellitus, the effects of hyperglycemia on the senescence of MSCs should be addressed for a better application of autologous MSCs. In this review, we summarize published studies on the senescence of MSCs in terms of their definition and hallmarks. Moreover, we review how hyperglycemia drives MSC senescence. Finally, we recommend strategies to suppress MSC senescence in the diabetic microenvironment.
The definition of senescence can be conceptually distinguished from that of aging, with that of senescence emphasizing the cellular level (Hayflick and Moorhead, 1961). Aging is the determining risk factor for most diseases and conditions that limit health span, and seven pillars promote aging, including metabolism, macromolecular damage, epigenetics, inflammation, adaptation to stress, proteostasis and stem cell regeneration. More importantly, these seven pillars are highly intertwined and their interplay plays a crucial role in the aging process (Kennedy et al., 2014). However, “cellular senescence is a cell state triggered by stressful insults and certain physiological processes, characterized by prolonged and generally irreversible cell-cycle arrest with secretory features, macromolecular damage, and altered metabolism” (Gorgoulis et al., 2019). Cellular senescence occurs in various physiological and pathological processes, such as embryogenesis, wound healing, injury, tumor suppression, and aging (Calcinotto et al., 2019). In addition, senescent cells are associated with aging-related diseases, such as type 2 diabetes, atherosclerosis, osteoporosis, glaucoma, and neurodegeneration (He and Sharpless, 2017). The exclusion of senescent cells or compounds that block the senescence-associated secretory phenotype (SASP) has been proposed for the treatment of cancer and aging-related diseases, which might play a major role in extending the health span (Partridge et al., 2020).
Interestingly, MSCs have also been found to be senescent in vivo and in vitro (Lin et al., 2019; Banimohamad-Shotorbani et al., 2020), which might lead to altered biological function and reduced therapeutic effect. Recently, we are seeing increased interest in MSC-based therapy for improving aging-related disorders and autoimmune diseases. Thus, the precise detection of senescent MSCs and elimination or reversion of the phenotype are essential for MSC-based therapy.
MSC senescence can be induced by many intrinsic triggers and oncogenes, as well as physiological and pathological changes in their microenvironment. Based on the nature of the triggers, MSC senescence can be categorized into various types, including replicative senescence, stress-induced senescence, oncogene-induced senescence, and developmental senescence (Zhou et al., 2020). The hallmarks (phenotypic indicators) of MSC senescence vary with the nature of the causes that drive the different types of senescence.
The classic phenotype of cellular senescence include cell-cycle arrest, SASP, macromolecular damage, and deregulated metabolic profile (Gorgoulis et al., 2019). Cell-cycle withdrawal is the nature of cellular senescence. An overwhelming amount of studies have demonstrated that SASP (Ozcan et al., 2016) can reinforce senescence and affect other cells through an autocrine and paracrine mechanism (Acosta et al., 2008; Kuilman et al., 2010). Moreover, SASP can induce a local inflammation with compound effects. Both effects are essential for tissue or organ regeneration and reconstruction (Kuilman and Peeper, 2009). This secretome is mainly composed of various extracellular growth factors, including transforming growth factor beta (TGF-β), epidermal growth factor (EGF), platelet derived growth factor, hepatocyte growth factor, and insulin-like growth factor 1-binding proteins, in addition to cytokines/chemokines, receptor decoys, receptor antagonists, and extracellular matrix remodeling proteins (Freund et al., 2010). Among macromolecular damage and deregulated metabolic profile, DNA damage (telomere shortening), chromatin remodeling, autophagy, and senescence-associated epigenetic expression changes (Sengupta and Seto, 2004; Jung et al., 2010; Li et al., 2011; Franzen et al., 2017), mitochondrial dysfunction, and lysosome dysfunction play a major role in MSC senescence.
Compared with common cellular senescence, senescent MSCs have their own specific characteristics, including multipotentiality loss, cell phenotype changes, immunomodulatory property damage, and homing and migration damage (Campisi and d’Adda di Fagagna, 2007). Many studies have shown that the osteogenic differentiation potential of MSCs declines with age (Garcia-Sanchez et al., 2019; Zhou et al., 2019). However, there is no definite conclusion regarding adipogenic differentiation, although most of the studies have demonstrated that the adipogenic differentiation potential of MSCs tends to deteriorates with successive passages under standard expantion conditions (Sugihara et al., 2018). In terms of cell phenotypes, early-passage and late-passage senescent MSCs showed similar levels of identification markers (positive for the markers CD90, CD73, and CD105, negative for the markers CD45, CD34, CD14, CD11b, CD79α, CD19, and HLA-DR) proposed by the International Society for Cellular Therapy, suggesting that their assessment may be restricted only to basic MSC characterization (Yu et al., 2014). In contrast, a number of studies found increased CD295 and CD264 (Madsen et al., 2017) and decreased Stro-1 (Bakopoulou et al., 2017; Redondo et al., 2018), CD106 (vascular cell adhesion protein 1) (Lu et al., 2019) and CD146 (melanoma cell adhesion molecule, MCAM) (Jin et al., 2016) during prolonged culture, suggesting their ability to mark senescent MSCs (Laschober et al., 2009).
A multi-marker, three-step workflow is recommended for identifying senescent cells: “first, assessing senescence-associated beta-galactosidase (SA-β-gal) activity and/or lipofuscin accumulation (SBB or GL13 staining); second, co-staining with other markers frequently observed in (p16INK4A, p21WAF1/Cip1) or absent in (proliferation markers, lamin B1) senescent cells; and third, identifying factors anticipated to be altered in specific senescence contexts” (Gorgoulis et al., 2019). This workflow is applicable to all cell types. Regarding MSCs, analysis of various molecules and biological processes, including cell cycle arrest; DNA damage; and transcriptional, epigenetic, and metabolic changes, has been recommended for senescence detection. The characteristics of senescent MSCs include flattened and enlarged morphology (Kim et al., 2016; Iwasaki et al., 2019), decreased number of colony formation units (Ganguly et al., 2019), increased SA-β-gal activity (Gnani et al., 2019), altered gene and protein expression (p16, p21, p53), shortened or dysfunctional telomeres (Bonab et al., 2006; Guillot et al., 2007), increased 8-oxo-dG or γH2AZ expression (markers of DNA damage), microsatellite instability (marker of genomic instability and deficient DNA repair), and abnormal global methylation. However, specific and unequivocal markers are still lacking, and for reliability, the evaluation of MSC senescence should be based on the assessment of several markers in vitro (Neri and Borzí, 2020).
Type 2 diabetes mellitus (T2DM) is a chronic metabolic disorder that affects an increasing number of people worldwide (Zheng et al., 2018). Current treatment for diabetes failed to maintain long-term blood glucose homeostasis, resulting in insulin secretion deficiency and acute and chronic diabetic complications. Therefore, new approaches for the therapy of T2DM and its chronic complications are of special interest. Currently, cellular therapies involving autologous MSCs, especially those isolated from adipose tissue, constitute a promising treatment for diabetes (Bhansali et al., 2017). Offspring of women with GDM are at high risk of T2DM in adulthood, and MSCs derived from the umbilical cord and placental membrane offer a promising source of autologous MSCs for latent diabetes treatment. However, there are still many obstacles concerning the effectiveness of autologous MSCs isolated from diabetic patients and the effect of the microenvironment that need to be overcome before MSCs can be used for this purpose. A classic feature of senescent MSCs in the hyperglycemic microenvironment is cell-cycle arrest, as confirmed by senescence marker (p16, p53, p21) mRNA and protein expression (Liu J. et al., 2020). Additionally, increased SA-β-galactosidase staining is another important identification marker. Furthermore, senescent MSCs showed decreased proliferation, differentiation alternations, cell phenotype changes and declined migration, angiogenesis, and immunomodulatory capabilities. Though SASP plays a vital role in the common cellular senescence, only few studies have focused on the SASP of senescent MSCs in the hyperglycemic microenvironment, summarized in Figure 1.
Figure 1. The hallmarks of senescent MSCs in the hyperglycemic microenvironment. SASP, senescence-associated secretory phenotype.
Proliferation is the most important characteristic of MSCs. Previous studies have shown that MSCs display lower proliferative capability and higher senescence in a hyperglycemic environment. The earliest study was conducted in 2010, when Cramer et al. showed that elevated glucose levels reduce the proliferative capacity of AMSCs with diabetes and AMSCs without diabetes, but the impact was more notable in AMSCs with diabetes. Similarly, AMSCs from patients with type 2 diabetes showed reduced viability and proliferative potential (Alicka et al., 2019). Intriguingly, incorporation of insulin enhances cell replication, especially in AMSCs without diabetes (Cramer et al., 2010). Another study demonstrated that diabetes mellitus was a significant determinant for forming a higher number of colony-forming units in BMSCs (Neef et al., 2012). Likewise, compared with human UCMSCs obtained from normal pregnant women, UCMSCs obtained from patients with GDM showed declined proliferative activity and increased senescence markers with a higher expression of p16 and p53 (Kim et al., 2015). In animal models, the colony formation capacity of rat adult non-adherent bone marrow MSCs (Na-BM-MSCs) was negatively influenced when cultured in high-glucose-containing medium; however, Na-BM-MSCs seemed to be relatively uninfluenced by aging, suggesting that diabetes, compared to aging, is more associated with reduced numbers and functional viability of MSCs in vivo (Stolzing et al., 2012). Taken together, these studies support the notion that hyperglycemia weaken the proliferation capacity of MSCs regardless of their sources both in vitro and in vivo. More importantly, hyperglycemia has a greater impact on MSC proliferation than does aging and this effect could not be completely restored by adding hypoglycemic agents.
MSCs are characterized by their potent multipotency and do not only have mesodermal differentiation potential but also neurogenic differentiation potential. Current studies on the differentiation potential of senescent MSCs in hyperglycemia are controversial. It has been reported that the adipogenic and osteogenic differentiation capacities of AMSCs in high glucose-treated conditions were comparable to those in low glucose-treated conditions (Cheng et al., 2016). In contrast to this study, Cramer et al showed that that high glucose concentrations reduce the osteogenic and chondrogenic potential of AMSCs (Cramer et al., 2010). Likewise, UCMSCs from patients with GDM displayed significantly lower osteogenic and chondrogenic potential (Kong et al., 2019). Regarding the adipogenic potential of MSC under hyperglycemia conditions, there are some controversial results. Cramer et al. reported that high glucose concentrations enhanced the adipogenic potential of MSCs. Further, when adult human BMSCs were incubated with sera from T2DM patients for 14 days, the expression of adipogenic genes and Oil Red O staining were greatly increased (Moseley et al., 2018). However, GDM-UCMSCs demonstrated a significantly lower adipogenic potential (Kim et al., 2015; Moseley et al., 2018). Furthermore, another study found that BMSCs showed decreased adipogenic differentiation capability under high glucose conditions and that the mRNA expression of peroxisome proliferator activated receptor gamma, CCAAT/enhancer-binding protein alpha, leptin, and adiponectin in BMSCs decreased (Rharass and Lucas, 2019).
Interestingly, when cultured in an appropriate induction medium, both AMSCs from diabetic donors (dAMSCs) and from non-diabetic donors (nAMSCs) exhibited potential for trans-differentiation into neuron-like cells via a reactive oxygen species (ROS)-mediated mechanism (Cheng et al., 2016). Exposure to GDM may cause irreversible senescence and stress damage to endogenous amniotic MSCs; however, chorionic MSCs from GDM mothers can be efficiently reprogrammed into insulin-secreting cells and have a therapeutic potential comparable to that of chorionic MSCs from healthy mothers. Specifically, chorionic MSCs from both healthy and GDM mothers exhibited increased pancreatic transcription factor expression in parallel with retinoic acid, activin A, glucagon-like peptide-1, EGF, and other chemical components and could generate functional insulin-producing cells with betacellulin-sensitive insulin expression (Chen L. et al., 2020).
Overall, these data suggest that hyperglycemia plays a pivotal role in the differentiation process of MSCs, and this process depends on the different sources of MSC. In general, hyperglycemia weakens the osteogenic and chondrogenic differentiation capability, but interestingly, enhances trans-differentiation into neuron-like cells and insulin-secreting cells. However, there are no uncontested results regarding the adipogenic differentiation. This inconsistency may be due to the age of MSC donors, different passages of MSCs, and the extent and duration of hyperglycemia.
Markers for identification and stemness are major areas of interest within the field of MSC senescence under hyperglycemic conditions. It has been reported that the expression of cell surface markers in dAMSCs and nAMSCs was similar to that of MSCs (Cheng et al., 2016). Moreover, senescent human UCMSCs, also express comparable levels of CD73, CD90, CD105, and marker proteins (Kim et al., 2015). Additionally, when cultured in high and normal glucose conditions, MSCs in the endosteal niche lining compact bone were not altered, demonstrating the presence of analogous MSC (CD73/CD105/CD90), embryonic (Slug and Snail), and multipotency (CD146) markers throughout the extended culture (Al-Qarakhli et al., 2019).
However, MSCs under hyperglycemia showed negligible pluripotency (Nanog, Oct4) and hematopoietic (CD34/CD45) markers (Al-Qarakhli et al., 2019). Recently, one study showed that stemness markers were significantly lower in insulin-controlled GDM mothers-UCMSCs and diet-controlled GDM mothers-UCMSCs than in normal UCMSCs (Kong et al., 2019). Another study using a systems biology approach identified widespread downregulation of MSC markers in the subcutaneous adipose tissue of diabetic rats (Ferrer-Lorente et al., 2014). Likewise, nucleus pulpous-derived MSCs cultured with high glucose showed decreased expression of stemness genes as well as decreased mRNA and protein expression of silent information regulator protein 1 (SIRT1), SIRT6, glucose transporter 1, and hypoxia inducible factor-1α (HIF-1α), but enhanced cell senescence, cell apoptosis, and caspase-3 expression (Liu Y. et al., 2020). Contrary to these data, Cheng et al. reported that dAMSCs or high-glucose treated nAMSCs presented higher expression of the pluripotent markers Sox-2, Oct-4, and Nanog, and this effect was related to ROS-mediated Akt attenuation. With antioxidant treatment, high glucose-treated nAMSCs exhibited enhanced cell growth but no stemness enhancement (Cheng et al., 2016). This rather contradictory result may be due to individual difference in MSC donors. Collectively, these studies indicate that hyperglycemia has a little effect on the identification markers, but decreases the stemness of MSCs in most cases.
In addition to the above features, MSCs in hyperglycemic conditions show decreased immunomodulatory properties (Montanucci et al., 2016), angiogenesis (Xiao et al., 2020), and migration (Liu Y. et al., 2020), but increased SASP and insulin resistance. SASP is mainly composed of various extracellular growth factors, cytokines/chemokines and receptor antagonists. It is well known that the proliferation and cytokine secretion (TGF-β and VEGF) of MSCs are inhibited after long-term culture even in MSCs derived from young donors (Baek et al., 2018). One study showed that aging and metabolic changes in diabetes also modified the MSC cytokine production abilities (Kondo et al., 2015). Moreover, another study showed that AMSCs from T2DM patients exhibited lower adiponectin, VEGF, and chemokine ligand-12 secretion, but demonstrated an overproduction of leptin (Alicka et al., 2019). Interestingly, data also showed that a high glucose environment causes prominent disparities in the expression of genes involved in insulin resistance, such as resistin and adiponectin between nAMSCs and dAMSCs. Some changes in the expression of these genes were permanent in dAMSCs when treated with insulin (Cramer et al., 2010). Overall, the evidence presented in this section suggests hyperglycemia adversely affect the biological function of MSC which is critical for their therapeutic effect.
Cell-cycle withdrawal is the core feature of MSC senescence. High glucose results in a significant decline in the number of cells in the G0/G1 phases and an increase in cells in the S and G2/M phases, suggesting that high glucose induces cell cycle withdrawal of MSCs. Besides, cell cycle markers (p16, p21, p27, and p53) were surprisingly upregulated in insulin-controlled GDM mothers-UCMSCs and diet-controlled GDM mothers-UCMSCs compared to normal UCMSCs (Kong et al., 2019). The increase in the expression of all these cell cycle markers results in increased levels of cyclin-dependent kinase inhibitors (CKD) which actuate entry into senescence by triggering Rb to block cell cycle progression, summarized in Figure 2. Apart from this classical pathway, studies have shown that phosphorylation of p38 mitogen-activated protein kinase (MAPK) in MSCs was increased under high glucose conditions without alterations of total p38 MAPK levels. The study also revealed that the p38 MAPK inhibitor SB203580 can alleviate the phosphorylation of p38 MAPK (Qi et al., 2019). In the next sections, brief discussions have been presented on the mechanisms of senescent MSCs under hyperglycemic conditions from the aspects of mitochondria dysfunction, microRNAs, telomerase and epigenetics, summarized in Figure 2.
Mitochondrial dysfunction is a vital factor during MSC senescence in the hyperglycemic microenvironment and has been extensively investigated. A previous study showed that GDM-UCMSCs displayed significantly declined mitochondrial activity and low expression of the mitochondrial function regulatory genes COX1, PGC-1a, ND2, ND9, and TFAM (Kim et al., 2015). Similarly, another study demonstrated that the gene expression of antioxidant enzymes and that of mitochondrial function genes (NADH dehydrogenase subunit 2, mitochondrial transcription factor A (TFAM), peroxisome proliferator-activated receptor γ coactivator-1alpha, and NADH dehydrogenase (ubiquinone) 1β-subcomplex) were obviously lower in UCMSCs from GDM mothers than in UCMSCs from healthy mothers (Kong et al., 2019). Additionally, AMSCs from type 2 diabetes patients exhibit a senescence phenotype and mitochondrial dysfunction because of excessive oxidative stress (Alicka et al., 2019). Moreover, high glucose-treated nASCs exhibited declined cell migration and higher intracellular ROS than did low glucose-treated cells. This high glucose-induced biological reaction is associated with ROS-mediated Akt attenuation (Cheng et al., 2016). Consistent with previous studies, Rharass et al. showed that intracellular and extracellular ROS concentrations were significantly increased by 50% under high glucose exposure, paralleled by increased mRNA levels of the H2O2 generating enzyme NADPH oxidase 4 (Rharass and Lucas, 2019). All of the studies reviewed here support the notion that hyperglycemia drive mitochondrial dysfunction through the ROS pathway which is fundamental to the senescence of MSCs.
MicroRNAs (miRNAs) and lncRNAs are small non-coding RNAs that regulate gene expression, playing a pivotal role in diverse biological processes, including MSC senescence. One study showed that AMSCs isolated from diabetes patients or cultured in a medium containing glycation end products (AGEs) showed decreased stem cell activity, differentiation potential, and angiogenesis effect. Moreover, the expression of miRNA-1248 was reduced, paralleled by increased expression of CBP/p300-interacting transactivator with ED-rich tail 2 (CITED2), an inhibitor of HIF-1α, which influences growth factors that promote cellular proliferation, angiogenesis, and wound healing. Thus, the authors of the study concluded that glucolipotoxicity impaired the effectiveness of AMSCs through the miR-1248/CITED2/HIF-1α pathway (Xiao et al., 2020). As previously mentioned, several studies have shown that hyperglycemia reduces the osteogenic potential of MSCs. Recently, one study explored the core molecular mechanism and found that stearoyl-coenzyme A desaturase-1 (SCD1) expression was downregulated in T2DM patients, and decreased SCD1 reduced the osteogenic differentiation of BMSCs by activating the miR-203a/FOS and miR-1908/EXO1 regulatory pathways (Chen Y.S. et al., 2020). In addition, a previous study found obvious differences in the expression of miRNAs involved in cell proliferation (miR-146a-5p, miR-16-5p, and miR-145-5p), together with miRNA and genes responsible for insulin sensitivity and glucose homeostasis (miR-17-5p, miR-24-3p, 140-3p, SIRT1, TGFβ, HIF-1α, LIN28, and FOXO1). More importantly, the authors observed a similar correspondence between miR-16-5p, miR-17-5p, miR-24-3p, 140-3p, miR-145-5p, and miR-146a-5p expression in the extracellular vesicle fraction. Apart from miRNAs, lncRNAs are also involved in the MSC senescence triggered by hyperglycemia, and it has been shown that high glucose inhibits osteogenic differentiation of MSCs through the lncRNA AK028326/CXCL13 pathway, revealing new molecular mechanism of many osteogenesis-related diseases, especially in patients with diabetes mellitus (Cao et al., 2016).
The telomere is a region of repetitive nucleotide sequences at each end of a chromatid, which protects the end of the chromosome from deterioration. Telomerase attrition is one of the hallmarks of cellular senescence. One study showed that telomerase was significantly lower in UCMSCs from GDM mothers than in UCMSCs from normal mothers (Kong et al., 2019). Moreover, the expression of the positive telomere maintenance marker (rTERT, TR) in MSCs in the endosteal niche lining compact bone was downregulated under high glucose conditions. Interestingly, telomere length is altered throughout in vitro expansion, with hyperglycemia markedly decreasing telomere lengths at PD50 and PD200 (Al-Qarakhli et al., 2019). Together, these studies recognize telomere attrition as one of the mechanisms of senescent MSCs in the hyperglycemic microenvironment.
Epigenetics is one of the pillars of aging and MSC senescence which can be detected by epigenetic modifications. One study showed that cardiac mesenchymal cells from patients with T2DM were characterized by premature cellular senescence, reduced proliferation, decreased differentiation potential, and decreased phosphorylation at histone H3 serine 10. Global histone code profiling of cardiac MSCs rom patients with T2DM demonstrated that acetylation of histone H3 lysine 9 (H3K9Ac) and lysine 14 (H3K14Ac) was reduced, while the trimethylation of H3K9Ac and lysine 27 was greatly increased. Moreover, DNA CpG island hypermethylation was detected at the promoter of genes involved in genomic stability and cell growth control. These results reveal that epigenetic changes may be an essential factor in MSC senescence in hyperglycemia (Vecellio et al., 2014).
MSCs from streptozotocin-induced diabetic rats displayed decreased proliferation and osteogenic differentiation potential, but increased senescence and apoptosis, and these effects appeared to be mediated by increased AGEs and an jincrease in the receptor for AGEs (Stolzing et al., 2010). Diabetic BMSCs showed inhibited osteogenesis and muscle ARNT-like protein 1 (BMAL1) expression, and over-expression of BMAL1 could recover BMSC osteogenesis in T2DM partly by reducing GSK-3β expression to trigger the Wnt/β-catenin pathway (Li et al., 2017).
Cellular senescence has been proven to be reversible. At present, the most promising drugs for this purpose include rapamycin, senolytics, and metformin (Partridge et al., 2020). For senescent MSCs, in addition to drugs, genetic modification, microRNA treatment and preconditioning modification are important approaches for rejuvenation (Neves et al., 2017; Ocansey et al., 2020). Currently, strategies to block the senescence of MSCs in hyperglycemic conditions include drugs (metformin, resveratrol, cytokines, and histone acetylase activator) and preconditioning modification (hypoxia), summarized in Figure 3. Besides, various in vitro experiments demonstrated that good glucose control could alleviate the senescence of MSCs, suggesting that blood glucose control should be advised to prevent or limit senescence of MSCs in patients with diabetes. More importantly, for MSCs from normal or diabetic donors, the appropriate glucose control in the MSC expansion process and in the transplantation process is essential for the clinical efficiency of MSC treatments (Lo et al., 2011).
Figure 3. Strategies to suppress senescence of MSCs in the hyperglycemic microenvironment. SCD1, stearoyl-coenzyme A desaturase-1; bFGF, basic fibroblast growth factor; SPV106, histone acetylase activator pentadecylidenemalonate 1b.
Metformin is widely prescribed for T2DM; interestingly, recent studies have suggested an important role for metformin in mitigating aging. After treatment with metformin, AMSCs isolated from mice showed reduced cellular senescence and decreased ROS but increased proliferative potential and osteogenic differentiation potential and declined adipogenic differentiation (Marycz et al., 2016). Resveratrol, a small polyphenol, has emerged as a potential therapy due to its anticancer and anti-inflammatory properties. In diabetic animal models, AMSCs preconditioned with resveratrol showed increased stem cell function and better effects (Chen et al., 2019). One of the mechanisms is that resveratrol increases the expression of the survival marker p-Akt, resulting in enhanced AMSC viability (Cheng et al., 2016). It is well known that the proliferation and cytokine secretion (TGF-β and VEGF) of MSCs are inhibited after long-term culture even in MSCs when derived from young donors (Baek et al., 2018). However, this situation could be changed by the addition of substance P, suggesting that substance P could block the loss of the therapeutic potential of MSCs by preserving their proliferative and paracrine potential. Moreover, another study found that basic fibroblast growth factor promotes the proliferation and inhibits the apoptosis of AMSCs isolated from patients with T2DM by reducing cellular oxidative stress (Nawrocka et al., 2017). Similarly, when pretreated with a mixture of Stromal cell-derived factor-1 alpha and bFGF, insulin-producing cells differentiated from AMSCs showed maximally alleviated senescence, apoptosis, and cell damage. Interestingly, these AMSCs demonstrated enhanced release of insulin, increased cell proliferation and upregulation of insulin 1, insulin 2, Ngn3, Nkx6.2, and Pdx1when triggered by hyperglycemia. Epigenetics, as one of the pillars of MSC senescence, has been studied for searching targets to suppress the senescence of MSCs. For example, the histone acetylase activator pentadecylidenemalonate 1b (SPV106) has been shown to alleviate cellular senescence and recover the regenerative and multi-differentiation potential of cardiac MSCs by restoring normal amounts of H3K9Ac and H3K14Ac and reducing DNA CpG hypermethylation (Vecellio et al., 2014). In addition, hypoxic preconditioning was able to enhance the regeneration potential of aging bone marrow MSCs into pancreaticβ-cells in streptozotocin-induced type-1 diabetic mice by altering gene expression levels of certain growth factors (Waseem et al., 2016). The underlying molecular mechanism might be related to hypoxia, which induces different regulation patterns in many cell cycle checkpoint genes such as HIF-1a, ataxia telangiectasia mutated, and ataxia telangiectasia and Rad3 related p53, p21, p27, and p21 (Sharma and Bhonde, 2015).
MSCs are promising cells for the treatment of regenerative and immunomodulatory diseases. Various studies have shown that hyperglycemia may induce MSC senescence and diminish crucial functions of MSCs, such as cell proliferation, differentiation capacity, angiogenesis and immunomodulatory capability, which remarkably restrict their therapeutic efficiency. The appropriate use of MSCs for clinical applications demands a general knowledge of the MSC senescence process. Additionally, approaches that generate large populations of MSCs in vitro without affecting their regenerative or immunomodulatory properties need to be established. In this review we discussed the relevant literature to date and suggested possible methods to improve therapeutic efficacy through regulating specific factors or the hyperglycemia microenvironment associated with MSC senescence.
Unlike BMSCs and AMSCs, UCMSCs have a painless collection process, faster self-renewal properties and lower immunogenicity. UCMSCs are attractive autologous or allogenic agents for the treatment of cancer and aging-related diseases (Ding et al., 2015). However, gestational diabetes is progressively prevalent and predicted to influence more than 20 million livebirths (about one in six) worldwide (Saravanan, 2020). Thus, considerably more work will need to be done to investigate the senescence of UCMSCs in the hyperglycemic environment. Besides, uniform experimental studies to elucidate the molecular basis and signaling pathways regulating senescence in MSCs in a diabetic microenvironment are urgently required, especially in the field of mitochondrial dysfunction and epigenetics. These mechanistic studies would contribute to discovering new strategy to suppress MSCs senescence in the hyperglycemic microenvironment. Furthermore, studies involving the detection of the senescence of MSCs in DM-related chronic complications such as diabetic nephropathy, diabetic neuropathy, and diabetic retinopathy will be beneficial. New specific assays to identify senescent MSCs to predict the safety and potency of autologous MSCs in DM or GDM and new strategies to suppress the senescence of MSCs should be developed. Data from these studies would facilitate accurate evaluation of the efficacy of the treatment and provide more precise inclusion and exclusion criteria for the proper selection of patient groups with diabetes that would best benefit from the treatment.
MY searched literature and drafted the manuscript. YZ and HY searched literature. XL provided ideas and revised the manuscript. All authors read and approved the final manuscript.
The authors declare that the research was conducted in the absence of any commercial or financial relationships that could be construed as a potential conflict of interest.
All figures have been created with biorender.com.
Acosta, J. C., O’Loghlen, A., Banito, A., Guijarro, M. V., Augert, A., Raguz, S., et al. (2008). Chemokine signaling via the CXCR2 receptor reinforces senescence. Cell 133, 1006–1018. doi: 10.1016/j.cell.2008.03.038
Alicka, M., Major, P., Wysocki, M., and Marycz, K. (2019). Adipose-Derived mesenchymal stem cells isolated from patients with Type 2 diabetes show reduced “Stemness” through an altered secretome profile, impaired anti-oxidative protection, and mitochondrial dynamics deterioration. J. Clin. Med. 8:765. doi: 10.3390/jcm8060765
Al-Qarakhli, A. M. A., Yusop, N., Waddington, R. J., and Moseley, R. (2019). Effects of high glucose conditions on the expansion and differentiation capabilities of mesenchymal stromal cells derived from rat endosteal niche. BMC Mol. Cell Biol. 20:51. doi: 10.1186/s12860-019-0235-y
Ankrum, J. A., Ong, J. F., and Karp, J. M. (2014). Mesenchymal stem cells: immune evasive, not immune privileged. Nat. Biotech. 32, 252–260. doi: 10.1038/nbt.2816
Baek, S. M., Son, Y., and Hong, H. S. (2018). Substance P blocks the impairment of paracrine potential of MSC due to long term culture. Mol. Cell Toxicol. 14, 283–290. doi: 10.1007/s13273-018-0031-3
Bakopoulou, A., Apatzidou, D., Aggelidou, E., Gousopoulou, E., Leyhausen, G., Volk, J., et al. (2017). Isolation and prolonged expansion of oral mesenchymal stem cells under clinical-grade, GMP-compliant conditions differentially affects “stemness” properties. Stem Cell Res. Ther. 8:247. doi: 10.1186/s13287-017-0705-0
Banimohamad-Shotorbani, B., Kahroba, H., Sadeghzadeh, H., Wilson, D. M. 3rd, Maadi, H., Samadi, N., et al. (2020). DNA damage repair response in mesenchymal stromal cells: from cellular senescence and aging to apoptosis and differentiation ability. Ageing Res. Rev. 62:101125. doi: 10.1016/j.arr.2020.101125
Bhansali, S., Dutta, P., Kumar, V., Yadav, M. K., Jain, A., Mudaliar, S., et al. (2017). Efficacy of autologous bone marrow-derived mesenchymal stem cell and mononuclear cell transplantation in type 2 diabetes mellitus: a randomized, placebo-controlled comparative study. Stem Cells Dev. 26, 471–481. doi: 10.1089/scd.2016.0275
Bonab, M. M., Alimoghaddam, K., Talebian, F., Ghaffari, S. H., Ghavamzadeh, A., and Nikbin, B. (2006). Aging of mesenchymal stem cell in vitro. BMC Cell Biol. 7:14. doi: 10.1186/1471-2121-7-14
Calcinotto, A., Kohli, J., Zagato, E., Pellegrini, L., Demaria, M., and Alimonti, A. (2019). Cellular senescence: aging, cancer, and injury. Physiol. Rev. 99, 1047–1078. doi: 10.1152/physrev.00020.2018
Campisi, J., and d’Adda di Fagagna, F. (2007). Cellular senescence: when bad things happen to good cells. Nat. Rev. Mol. Cell Biol. 8, 729–740. doi: 10.1038/nrm2233
Cao, B., Liu, N., and Wang, W. (2016). High glucose prevents osteogenic differentiation of mesenchymal stem cells via lncRNA AK028326/CXCL13 pathway. Biomed. Pharmacother.=Biomed. Pharmacother. 84, 544–551. doi: 10.1016/j.biopha.2016.09.058
Chen, L., Forsyth, N. R., and Wu, P. (2020). Chorionic and amniotic placental membrane-derived stem cells, from gestational diabetic women, have distinct insulin secreting cell differentiation capacities. J. Tissue Eng. Regen. Med. 14, 243–256. doi: 10.1002/term.2988
Chen, Y. S., Kang, X. R., Zhou, Z. H., Yang, J., Xin, Q., Ying, C. T., et al. (2020). MiR-1908/EXO1 and MiR-203a/FOS, regulated by scd1, are associated with fracture risk and bone health in postmenopausal diabetic women. Aging 12, 9549–9584. doi: 10.18632/aging.103227
Chen, T. S., Kuo, C. H., Day, C. H., Pan, L. F., Chen, R. J., Chen, B. C., et al. (2019). Resveratrol increases stem cell function in the treatment of damaged pancreas. J. Cell Physiol. 234, 20443–20452. doi: 10.1002/jcp.28646
Cheng, N. C., Hsieh, T. Y., Lai, H. S., and Young, T. H. (2016). High glucose-induced reactive oxygen species generation promotes stemness in human adipose-derived stem cells. Cytotherapy 18, 371–383. doi: 10.1016/j.jcyt.2015.11.012
Costa, L. A., Eiro, N., Fraile, M., Gonzalez, L. O., Saá, J., Garcia-Portabella, P., et al. (2020). Functional heterogeneity of mesenchymal stem cells from natural niches to culture conditions: implications for further clinical uses. Cell. Mol. Life Sci. 78, 447–467. doi: 10.1007/s00018-020-03600-0
Cramer, C., Freisinger, E., Jones, R. K., Slakey, D. P., Dupin, C. L., Newsome, E. R., et al. (2010). Persistent high glucose concentrations alter the regenerative potential of mesenchymal stem cells. Stem Cells Dev. 19, 1875–1884. doi: 10.1089/scd.2010.0009
Ding, D. C., Chang, Y. H., Shyu, W. C., and Lin, S. Z. (2015). Human umbilical cord mesenchymal stem cells: a new era for stem cell therapy. Cell Transplant. 24, 339–347. doi: 10.3727/096368915x686841
Ferrer-Lorente, R., Bejar, M. T., Tous, M., Vilahur, G., and Badimon, L. (2014). Systems biology approach to identify alterations in the stem cell reservoir of subcutaneous adipose tissue in a rat model of diabetes: effects on differentiation potential and function. Diabetologia 57, 246–256. doi: 10.1007/s00125-013-3081-z
Franzen, J., Zirkel, A., Blake, J., Rath, B., Benes, V., Papantonis, A., et al. (2017). Senescence-associated DNA methylation is stochastically acquired in subpopulations of mesenchymal stem cells. Aging Cell 16, 183–191. doi: 10.1111/acel.12544
Freund, A., Orjalo, A. V., Desprez, P. Y., and Campisi, J. (2010). Inflammatory networks during cellular senescence: causes and consequences. Trends Mol. Med. 16, 238–246. doi: 10.1016/j.molmed.2010.03.003
Galipeau, J., and Sensébé, L. (2018). Mesenchymal stromal cells: clinical challenges and therapeutic opportunities. Cell Stem Cell 22, 824–833. doi: 10.1016/j.stem.2018.05.004
Ganguly, P., El-Jawhari, J. J., Burska, A. N., Ponchel, F., Giannoudis, P. V., and Jones, E. A. (2019). The analysis of in vivo aging in human bone marrow mesenchymal stromal cells using colony-forming unit-fibroblast assay and the CD45(low)CD271(+) phenotype. Stem Cells Int. 2019:5197983. doi: 10.1155/2019/5197983
Garcia-Sanchez, D., Fernandez, D., Rodriguez-Rey, J. C., and Perez-Campo, F. M. (2019). Enhancing survival, engraftment, and osteogenic potential of mesenchymal stem cells. World J. Stem Cells 11, 748–763. doi: 10.4252/wjsc.v11.i10.748
Gnani, D., Crippa, S., Della Volpe, L., Rossella, V., Conti, A., Lettera, E., et al. (2019). An early-senescence state in aged mesenchymal stromal cells contributes to hematopoietic stem and progenitor cell clonogenic impairment through the activation of a pro-inflammatory program. Aging Cell 18:e12933. doi: 10.1111/acel.12933
Golpanian, S., Wolf, A., Hatzistergos, K. E., and Hare, J. M. (2016). Rebuilding the damaged heart: mesenchymal stem cells, cell-based therapy, and engineered heart tissue. Physiol. Rev. 96, 1127–1168. doi: 10.1152/physrev.00019.2015
Gorgoulis, V., Adams, P. D., Alimonti, A., Bennett, D. C., Bischof, O., Bishop, C., et al. (2019). Cellular senescence: defining a path forward. Cell 179, 813–827. doi: 10.1016/j.cell.2019.10.005
Guillot, P. V., Gotherstrom, C., Chan, J., Kurata, H., and Fisk, N. M. (2007). Human first-trimester fetal MSC express pluripotency markers and grow faster and have longer telomeres than adult MSC. Stem Cells (Dayton, Ohio) 25, 646–654. doi: 10.1634/stemcells.2006-0208
Hayflick, L., and Moorhead, P. S. (1961). The serial cultivation of human diploid cell strains. Exp. Cell Res. 25, 585–621. doi: 10.1016/0014-4827(61)90192-6
He, S., and Sharpless, N. E. (2017). Senescence in health and disease. Cell 169, 1000–1011. doi: 10.1016/j.cell.2017.05.015
Hunsberger, J. G., Rao, M., Kurtzberg, J., Bulte, J. W. M., Atala, A., LaFerla, F. M., et al. (2016). Accelerating stem cell trials for Alzheimer’s disease. Lancet Neurol. 15, 219–230. doi: 10.1016/s1474-4422(15)00332-4
Iwasaki, K., Komaki, M., Akazawa, K., Nagata, M., Yokoyama, N., Watabe, T., et al. (2019). Spontaneous differentiation of periodontal ligament stem cells into myofibroblast during ex vivo expansion. J. Cell Physiol. 234, 20377–20391. doi: 10.1002/jcp.28639
Jin, H. J., Kwon, J. H., Kim, M., Bae, Y. K., Choi, S. J., Oh, W., et al. (2016). Downregulation of melanoma cell adhesion molecule (MCAM/CD146) accelerates cellular senescence in human umbilical cord blood-derived mesenchymal stem cells. Stem Cells Transl. Med. 5, 427–439. doi: 10.5966/sctm.2015-0109
Jung, J.-W., Lee, S., Seo, M.-S., Park, S.-B., Kurtz, A., Kang, S.-K., et al. (2010). Histone deacetylase controls adult stem cell aging by balancing the expression of polycomb genes and jumonji domain containing 3. Cell. Mol. Life Sci. 67, 1165–1176. doi: 10.1007/s00018-009-0242-9
Kennedy, B. K., Berger, S. L., Brunet, A., Campisi, J., Cuervo, A. M., Epel, E. S., et al. (2014). Geroscience: linking aging to chronic disease. Cell 159, 709–713. doi: 10.1016/j.cell.2014.10.039
Kim, J., Piao, Y., Pak, Y. K., Chung, D., Han, Y. M., Hong, J. S., et al. (2015). Umbilical cord mesenchymal stromal cells affected by gestational diabetes mellitus display premature aging and mitochondrial dysfunction. Stem Cells Dev. 24, 575–586. doi: 10.1089/scd.2014.0349
Kim, J. H., Shin, S. H., Li, T. Z., and Suh, H. (2016). Influence of in vitro biomimicked stem cell ‘niche’ for regulation of proliferation and differentiation of human bone marrow-derived mesenchymal stem cells to myocardial phenotypes: serum starvation without aid of chemical agents and prevention of spontaneous stem cell transformation enhanced by the matrix environment. J. Tissue Eng. Regen. Med. 10, E1–E13. doi: 10.1002/term.1754
Kondo, M., Kamiya, H., Himeno, T., Naruse, K., Nakashima, E., Watarai, A., et al. (2015). Therapeutic efficacy of bone marrow-derived mononuclear cells in diabetic polyneuropathy is impaired with aging or diabetes. J. Diabetes Investig. 6, 140–149. doi: 10.1111/jdi.12272
Kong, C. M., Subramanian, A., Biswas, A., Stunkel, W., Chong, Y. S., Bongso, A., et al. (2019). Changes in stemness properties, differentiation potential, oxidative stress, senescence and mitochondrial function in wharton’s jelly stem cells of umbilical cords of mothers with gestational diabetes mellitus. Stem Cell Rev. Rep. 15, 415–426. doi: 10.1007/s12015-019-9872-y
Kornicka, K., Houston, J., and Marycz, K. (2018). Dysfunction of mesenchymal stem cells isolated from metabolic syndrome and type 2 diabetic patients as result of oxidative stress and autophagy may limit their potential therapeutic use. Stem Cell Rev. Rep. 14, 337–345. doi: 10.1007/s12015-018-9809-x
Kuilman, T., Michaloglou, C., Mooi, W. J., and Peeper, D. S. (2010). The essence of senescence. Genes Dev. 24, 2463–2479. doi: 10.1101/gad.1971610
Kuilman, T., and Peeper, D. S. (2009). Senescence-messaging secretome: sms-ing cellular stress. Nat. Rev. Cancer 9, 81–94. doi: 10.1038/nrc2560
Laschober, G. T., Brunauer, R., Jamnig, A., Fehrer, C., Greiderer, B., and Lepperdinger, G. (2009). Leptin receptor/CD295 is upregulated on primary human mesenchymal stem cells of advancing biological age and distinctly marks the subpopulation of dying cells. Exp. Gerontol. 44, 57–62. doi: 10.1016/j.exger.2008.05.013
Li, X., Liu, N., Wang, Y., Liu, J., Shi, H., Qu, Z., et al. (2017). Brain and muscle aryl hydrocarbon receptor nuclear translocator-like protein-1 cooperates with glycogen synthase kinase-3beta to regulate osteogenesis of bone-marrow mesenchymal stem cells in type 2 diabetes. Mol. Cell. Endocrinol. 440, 93–105. doi: 10.1016/j.mce.2016.10.001
Li, Z., Liu, C., Xie, Z., Song, P., Zhao, R. C. H., Guo, L., et al. (2011). Epigenetic dysregulation in mesenchymal stem cell aging and spontaneous differentiation. PLoS One 6:e20526. doi: 10.1371/journal.pone.0020526
Lin, H., Sohn, J., Shen, H., Langhans, M. T., and Tuan, R. S. (2019). Bone marrow mesenchymal stem cells: aging and tissue engineering applications to enhance bone healing. Biomaterials 203, 96–110. doi: 10.1016/j.biomaterials.2018.06.026
Liu, J., Ding, Y., Liu, Z., and Liang, X. (2020a). Senescence in mesenchymal stem cells: functional alterations, molecular mechanisms, and rejuvenation strategies. Front. Cell Dev. Biol. 8:258. doi: 10.3389/fcell.2020.00258
Liu, Y., Li, Y., Nan, L. P., Wang, F., Zhou, S. F., Wang, J. C., et al. (2020b). The effect of high glucose on the biological characteristics of nucleus pulposus-derived mesenchymal stem cells. Cell Biochem. Funct. 38, 130–140. doi: 10.1002/cbf.3441
Lo, T., Ho, J. H., Yang, M. H., and Lee, O. K. (2011). Glucose reduction prevents replicative senescence and increases mitochondrial respiration in human mesenchymal stem cells. Cell Transplan. 20, 813–825. doi: 10.3727/096368910x539100
Lu, S. H., Ge, M., You, Y. H., Huo, J., Liang, H. Y., Yu, W. Y., et al. (2019). [Comparison of the biological functions between human bone marrow derived CD106 (+)mesenchymal stem cells and CD106 (-) Subgroup]. Zhongguo Yi Xue Ke Xue Yuan Xue Bao. Acta Academiae Medicinae Sinicae 41, 443–451. doi: 10.3881/j.issn.1000-503X.10887
Madsen, S. D., Russell, K. C., Tucker, H. A., Glowacki, J., Bunnell, B. A., and O’Connor, K. C. (2017). Decoy TRAIL receptor CD264: a cell surface marker of cellular aging for human bone marrow-derived mesenchymal stem cells. Stem Cell Res. Ther. 8:201. doi: 10.1186/s13287-017-0649-4
Mahmoud, M., Abu-Shahba, N., Azmy, O., and El-Badri, N. (2019). Impact of diabetes mellitus on human mesenchymal stromal cell biology and functionality: implications for autologous transplantation. Stem Cell Rev. Rep. 15, 194–217. doi: 10.1007/s12015-018-9869-y
Marycz, K., Tomaszewski, K. A., Kornicka, K., Henry, B. M., Wroński, S., Tarasiuk, J., et al. (2016). Metformin decreases reactive oxygen species, enhances osteogenic properties of adipose-derived multipotent mesenchymal stem cells in vitro, and increases bone density in vivo. Oxidative Med. Cell. Longevity 2016:9785890. doi: 10.1155/2016/9785890
Montanucci, P., Pescara, T., Pennoni, I., Alunno, A., Bistoni, O., Torlone, E., et al. (2016). Functional profiles of human umbilical cord-derived adult mesenchymal stem cells in obese/diabetic versus healthy women. Curr. Diabetes Rev. [Online ahead of print] doi: 10.2174/1573399812666160629102910
Moseley, K. F., Doyle, M. E., and Jan De Beur, S. M. (2018). Diabetic serum from older women increases adipogenic differentiation in mesenchymal stem cells. Endocrine Res. 43, 155–165. doi: 10.1080/07435800.2018.1441868
Nawrocka, D., Kornicka, K., Szydlarska, J., and Marycz, K. (2017). Basic fibroblast growth factor inhibits apoptosis and promotes proliferation of adipose-derived mesenchymal stromal cells isolated from patients with type 2 diabetes by reducing cellular oxidative stress. Oxidative Med. Cell. Longevity 2017:3027109. doi: 10.1155/2017/3027109
Neef, K., Choi, Y. H., Weichel, A., Rahmanian, P. B., Liakopoulos, O. J., Stamm, C., et al. (2012). The influence of cardiovascular risk factors on bone marrow mesenchymal stromal cell fitness. Cytotherapy 14, 670–678. doi: 10.3109/14653249.2012.663483
Neri, S., and Borzí, R. M. (2020). Molecular mechanisms contributing to mesenchymal stromal cell aging. Biomolecules 10:30. doi: 10.3390/biom10020340
Neves, J., Sousa-Victor, P., and Jasper, H. (2017). Rejuvenating strategies for stem cell-based therapies in aging. Cell Stem Cell 20, 161–175. doi: 10.1016/j.stem.2017.01.008
Ocansey, D. K. W., Pei, B., Yan, Y., Qian, H., Zhang, X., Xu, W., et al. (2020). Improved therapeutics of modified mesenchymal stem cells: an update. J. Transl. Med. 18:42. doi: 10.1186/s12967-020-02234-x
Ozcan, S., Alessio, N., Acar, M. B., Mert, E., Omerli, F., Peluso, G., et al. (2016). Unbiased analysis of senescence associated secretory phenotype (SASP) to identify common components following different genotoxic stresses. Aging 8, 1316–1329. doi: 10.18632/aging.100971
Packer, M. (2018). The Alchemist’s nightmare: might mesenchymal stem cells that are recruited to repair the injured heart be transformed into fibroblasts rather than cardiomyocytes? Circulation 137, 2068–2073. doi: 10.1161/circulationaha.117.032190
Partridge, L., Fuentealba, M., and Kennedy, B. K. (2020). The quest to slow ageing through drug discovery. Nat. Rev. Drug Discov. 19, 513–532. doi: 10.1038/s41573-020-0067-7
Qi, L., Wang, R., Shi, Q., Yuan, M., Jin, M., and Li, D. (2019). Umbilical cord mesenchymal stem cell conditioned medium restored the expression of collagen II and aggrecan in nucleus pulposus mesenchymal stem cells exposed to high glucose. J. Bone Mineral Metab. 37, 455–466. doi: 10.1007/s00774-018-0953-9
Redondo, J., Sarkar, P., Kemp, K., Virgo, P. F., Pawade, J., Norton, A., et al. (2018). Reduced cellularity of bone marrow in multiple sclerosis with decreased MSC expansion potential and premature ageing in vitro. Multiple Sclerosis (Houndmills, Basingstoke, England) 24, 919–931. doi: 10.1177/1352458517711276
Rharass, T., and Lucas, S. (2019). High glucose level impairs human mature bone marrow adipocyte function through increased ros production. Front. Endocrinol. 10:607. doi: 10.3389/fendo.2019.00607
Saravanan, P. (2020). Gestational diabetes: opportunities for improving maternal and child health. Lancet. Diabetes Endocrinol. 8, 793–800. doi: 10.1016/s2213-8587(20)30161-3
Sengupta, N., and Seto, E. (2004). Regulation of histone deacetylase activities. J. Cell. Biochem. 93, 57–67. doi: 10.1002/jcb.20179
Sharma, S., and Bhonde, R. (2015). Mesenchymal stromal cells are genetically stable under a hostile in vivo-like scenario as revealed by in vitro micronucleus test. Cytotherapy 17, 1384–1395. doi: 10.1016/j.jcyt.2015.07.004
Shi, Y., Wang, Y., Li, Q., Liu, K., Hou, J., Shao, C., et al. (2018). Immunoregulatory mechanisms of mesenchymal stem and stromal cells in inflammatory diseases. Nat. Rev. Nephrol. 14, 493–507. doi: 10.1038/s41581-018-0023-5
Stolzing, A., Bauer, E., and Scutt, A. (2012). Suspension cultures of bone-marrow-derived mesenchymal stem cells: effects of donor age and glucose level. Stem Cells Dev. 21, 2718–2723. doi: 10.1089/scd.2011.0406
Stolzing, A., Sellers, D., Llewelyn, O., and Scutt, A. (2010). Diabetes induced changes in rat mesenchymal stem cells. Cells Tissues Organs 191, 453–465. doi: 10.1159/000281826
Sugihara, H., Teramoto, N., Yamanouchi, K., Matsuwaki, T., and Nishihara, M. (2018). Oxidative stress-mediated senescence in mesenchymal progenitor cells causes the loss of their fibro/adipogenic potential and abrogates myoblast fusion. Aging 10, 747–763. doi: 10.18632/aging.101425
Timaner, M., Tsai, K. K., and Shaked, Y. (2020). The multifaceted role of mesenchymal stem cells in cancer. Semin. Cancer Biol. 60, 225–237. doi: 10.1016/j.semcancer.2019.06.003
Vecellio, M., Spallotta, F., Nanni, S., Colussi, C., Cencioni, C., Derlet, A., et al. (2014). The histone acetylase activator pentadecylidenemalonate 1b rescues proliferation and differentiation in the human cardiac mesenchymal cells of type 2 diabetic patients. Diabetes 63, 2132–2147. doi: 10.2337/db13-0731
Wang, L. (2018). Mesenchymal stromal cells in treatment of acute-on-chronic liver failure. Hepatology (Baltimore, Md.) 67, 2056–2057. doi: 10.1002/hep.29670
Waseem, M., Khan, I., Iqbal, H., Eijaz, S., Usman, S., Ahmed, N., et al. (2016). Hypoxic preconditioning improves the therapeutic potential of aging bone marrow mesenchymal stem cells in streptozotocin-induced type-1 diabetic mice. Cell. Reprogram. 18, 344–355. doi: 10.1089/cell.2016.0002
Xiao, S., Zhang, D., Liu, Z., Jin, W., Huang, G., Wei, Z., et al. (2020). Diabetes-induced glucolipotoxicity impairs wound healing ability of adipose-derived stem cells-through the miR-1248/CITED2/HIF-1α pathway. Aging 12, 6947–6965. doi: 10.18632/aging.103053
Yu, Y., Park, Y. S., Kim, H. S., Kim, H. Y., Jin, Y. M., Jung, S. C., et al. (2014). Characterization of long-term in vitro culture-related alterations of human tonsil-derived mesenchymal stem cells: role for CCN1 in replicative senescence-associated increase in osteogenic differentiation. J. Anatomy 225, 510–518. doi: 10.1111/joa.12229
Zheng, Y., Ley, S. H., and Hu, F. B. (2018). Global aetiology and epidemiology of type 2 diabetes mellitus and its complications. Nat. Rev. Endocrinol. 14, 88–98. doi: 10.1038/nrendo.2017.151
Zhou, T., Yan, Y., Zhao, C., Xu, Y., Wang, Q., and Xu, N. (2019). Resveratrol improves osteogenic differentiation of senescent bone mesenchymal stem cells through inhibiting endogenous reactive oxygen species production via AMPK activation. Redox Rep.: Commun. Free Radical Res. 24, 62–69. doi: 10.1080/13510002.2019.1658376
Keywords: mesenchymal stem cells, senescence, hyperglycemia, diabetes mettitus, mitochondrial dysfunction
Citation: Yin M, Zhang Y, Yu H and Li X (2021) Role of Hyperglycemia in the Senescence of Mesenchymal Stem Cells. Front. Cell Dev. Biol. 9:665412. doi: 10.3389/fcell.2021.665412
Received: 08 February 2021; Accepted: 24 March 2021;
Published: 15 April 2021.
Edited by:
Mark Hamrick, Augusta University, United StatesReviewed by:
Philippe Bourin, Independent Researcher, Toulouse, FranceCopyright © 2021 Yin, Zhang, Yu and Li. This is an open-access article distributed under the terms of the Creative Commons Attribution License (CC BY). The use, distribution or reproduction in other forums is permitted, provided the original author(s) and the copyright owner(s) are credited and that the original publication in this journal is cited, in accordance with accepted academic practice. No use, distribution or reproduction is permitted which does not comply with these terms.
*Correspondence: Xia Li, bGl4aWFAY3N1LmVkdS5jbg==
Disclaimer: All claims expressed in this article are solely those of the authors and do not necessarily represent those of their affiliated organizations, or those of the publisher, the editors and the reviewers. Any product that may be evaluated in this article or claim that may be made by its manufacturer is not guaranteed or endorsed by the publisher.
Research integrity at Frontiers
Learn more about the work of our research integrity team to safeguard the quality of each article we publish.