- Chemical-Biological Department of IISS, Maglie, Italy
I have recently theorized that several similarities exist between the tumor process and embryo development. Starting from an initial cancer stem cell (CSC0), similar to an embryonic stem cell (ESC), after implantation in a niche, primary self-renewing CSCs (CSC1s) would arise, which then generate secondary proliferating CSCs (CSC2s). From these epithelial CSCs, tertiary mesenchymal CSCs (CSC3s) would arise, which, under favorable stereotrophic conditions, by asymmetric proliferation, would generate cancer progenitor cells (CPCs) and then cancer differentiated cells (CDCs), thus giving a defined cell heterogeneity and hierarchy. CSC1s–CSC2s–CSC3s–CPCs–CDCs would constitute a defined “tumor growth module,” able to generate new tumor modules, forming a spherical avascular mass, similar to a tumor sphere. Further growth in situ of this initial tumor would require implantation in the host and vascularization through the overexpression of some aspecific checkpoint molecules, such as CD44, ID, LIF, HSP70, and HLA-G. To expand and spread in the host tissues, this vascularized tumor would then carry on a real growth strategy based on other specific checkpoint factors, such as those contained in the extracellular vesicles (EVs), namely, microRNAs, messenger RNAs, long non-coding RNAs, and integrins. These EV components would be crucial in tumor progression because they can mediate intercellular communications in the surrounding microenvironment and systemically, dictating to recipient cells a new tumor-enslaved phenotype, thus determining pre-metastatic conditions. Moreover, by their induction properties, the EV contents could also frustrate in time the effects of cytolytic tumor therapies, where EVs released by killed CSCs might enter other cancer and non-cancer cells, thus giving chemoresistance, non-CSC/CSC transition (recurrence), and metastasis. Thus, antitumor cytotoxic treatments, “shielded” from the EV-specific checkpoints by suitable adjuvant agents, simultaneously targeting the aforesaid aspecific checkpoints should be necessary for dismantling the hierarchic tumor structure, avoiding recurrence and preventing metastasis.
Introduction
I have recently theorized that several similarities exist between the tumor process and embryo development (Manzo, 2019). Starting from an initial cancer stem cell (i-CSC/CSC0), similar to an embryonic stem cell (ESC) without genomic homeostasis (para-ESC), after implantation in a niche, primary self-renewing cancer stem cells (CSC1s) would arise, corresponding to epiblast cells. CSC1s would then generate secondary proliferating CSCs (CSC2s), equivalent to hypoblast cells. From CSC1s and CSC2s, with an epithelial phenotype, tertiary CSCs (CSC3s) with a mesenchymal phenotype would arise, corresponding to the mesodermal precursors at the primitive streak (PS). Under favorable stereotrophic conditions (normoxia), CSC3s would undergo asymmetric proliferation and pre-differentiation into cancer progenitor cells (CPCs) and then into cancer differentiated cells (CDCs), thus giving a defined cell heterogeneity and hierarchy (Marjanovic et al., 2013; Singh et al., 2015; Bradshaw et al., 2016), mimicking an ectopic rudimentary somito-histo-organogenesis process (Reya et al., 2001; Gibbs, 2009; Ma et al., 2010). In contrast, under unfavorable stereotrophic conditions (hypoxia), CSC3s would delaminate and migrate as quiescent micro-metastases, mimicking embryonic morphogenetic movements and localizing in metastatic niches (Cabrera et al., 2015; Singh et al., 2015; Yang et al., 2018). Here, specific signals, similar to those occurring in the embryonic inductions, would induce an epithelial–mesenchymal transition (EMT)/mesenchymal–epithelial transition (MET) switch (Thiery et al., 2009; Liu et al., 2014), allowing the reversion of quiescent CSC3s into proliferating CSC1s. These cells would be able to generate macro-metastases with the same cell hierarchy as their primary tumors (Marjanovic et al., 2013). Within this proliferation model, CSC1s–CSC2s–CSC3s–CPCs–CDCs would constitute a defined “tumor growth module” with a cord-finger structure (Manzo, 2019, 2020; Figure 1), where it is possible to find well-defined mathematical relationships between CSCs (CSC1s, CSC2s, and CSC3s) and non-CSCs (CPCs and CDCs) at each (n) cell division (Manzo, 2020). A tumor growth module would generate new modules after about 10 division cycles, when the cell number would become presumably too large for survival under unfavorable stereotrophic conditions (Hamilton and Rath, 2019; Manzo, 2020). Such a modular growth process seems to occur also when CSCs, cultured in vitro in the absence of implantation conditions, form solid, round cellular structures with a diameter of about 50–250 μm, named tumor spheres, displaying a modular growth behavior similar to that of avascular tumors in vivo (Johnson et al., 2013; Vinnitsky, 2014). Such tumor growth, occurring by reiterative production of defined cell modules, would generate an initial spherical avascular mass (Figure 2). This might expand until it reaches a diameter of approximately 400 μm since diffusion and the supply of nutrients and oxygen at the core cells are not possible beyond about 200 μm (Hamilton and Rath, 2019). Beyond this limit, avascular tumor growth could occur only with a simultaneous death of the core cells (Hamilton and Rath, 2019). Up to this point, the tumor process would be similar and equivalent to that of a preimplantation blastocyst (Manzo, 2020). Now, further tumor growth would require implantation and vascularization for the oxygen and nutrient supply by the host microenvironment, like in embryo development. In such a way, an avascular tumor might become a vascularized tumor, where, together with nutrients, immune cells also arrive (Figure 3). Therefore, vascular tumor cells need to defend themselves from immune cells for survival, like what a post-implantation semi-allogeneic blastocyst do from maternal immune cells (Yao et al., 2005; Gregori et al., 2015; Manzo, 2019). On the other end, a tumor needs to expand in the host tissues. To this end, it would carry out a real growth strategy based on defined structures, such as extracellular vesicles (EVs) with their contents (Jurj et al., 2020), able to impair the host immune system and induce tumor growth, allowing tumor progression and metastases (Vader et al., 2014; Lin and Yan, 2015, 2019; Han et al., 2019), mimicking ectopic rudimentary organ portions. Now, I intend to point out and analyze crucial factors in the different phases of the cancer process for detecting potential checkpoints to be targeted in therapeutic treatments.
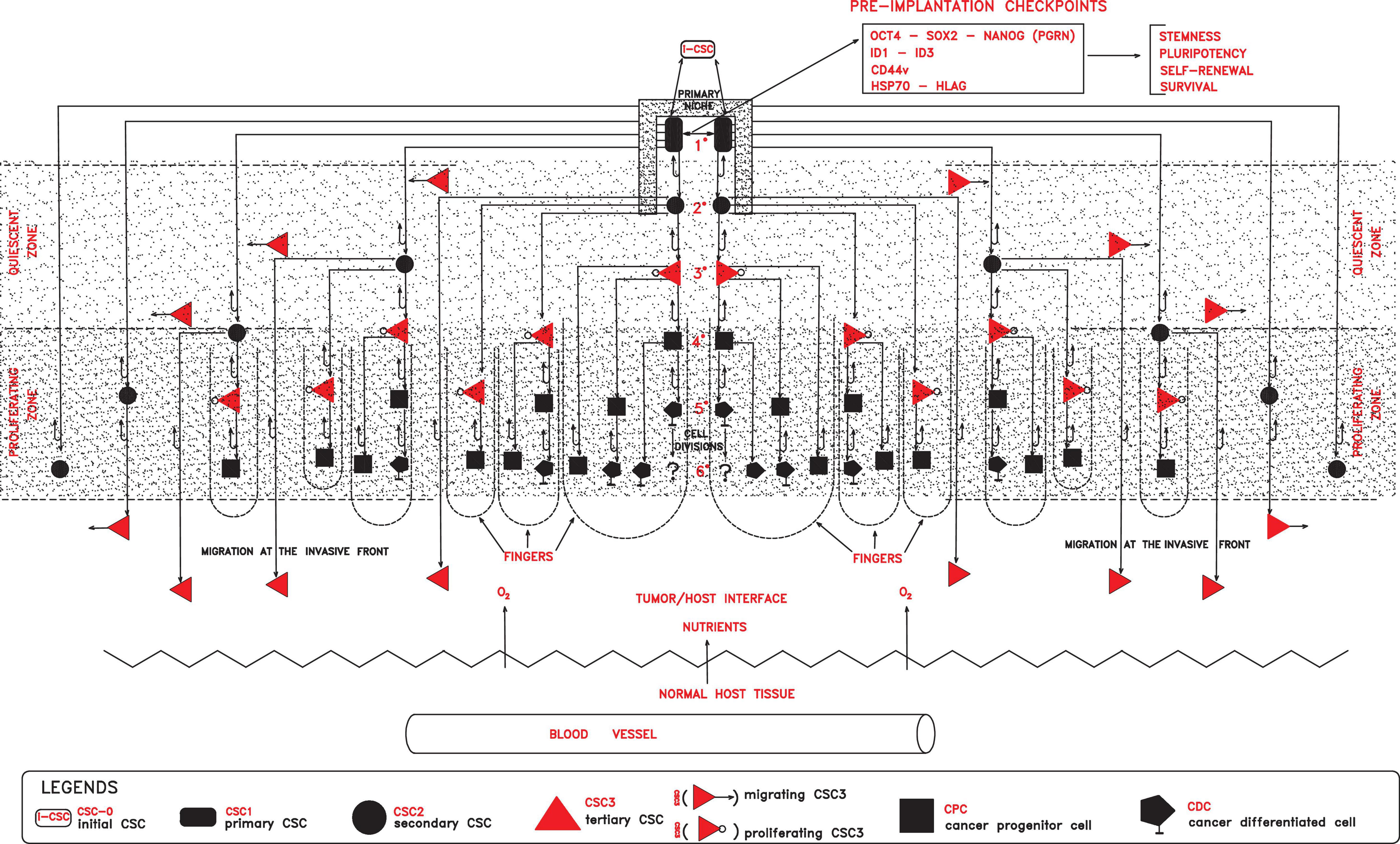
Figure 1. In a primary niche, primary self-renewing cancer stem cells (CSC1s) endowed with stemness properties, due to defined preimplantation checkpoint factors (OCT4, SOX2, NANOG, ID, CD44, HSP70, and HLA-G), would generate progressively secondary proliferating CSCs (CSC2s), tertiary mesenchymal CSCs (CSC3s), cancer progenitor (CPCs), and cancer differentiated (CDCs) cells, globally forming a tumor module where two zones would lie (quiescent and proliferating). In the proliferating zone, more external with normoxia conditions, CSC3s and CPCs would proliferate, generating cell cord-finger structures on the invasive front at the tumor/host interface. On the other hand, in the quiescent zone, more internal with hypoxic conditions, quiescent CSC3s would be induced to migrate peripherally, seeding new local niches in the normal host tissues. All these processes, finally, would result in a tumor module with a defined cell heterogeneity and hierarchy.
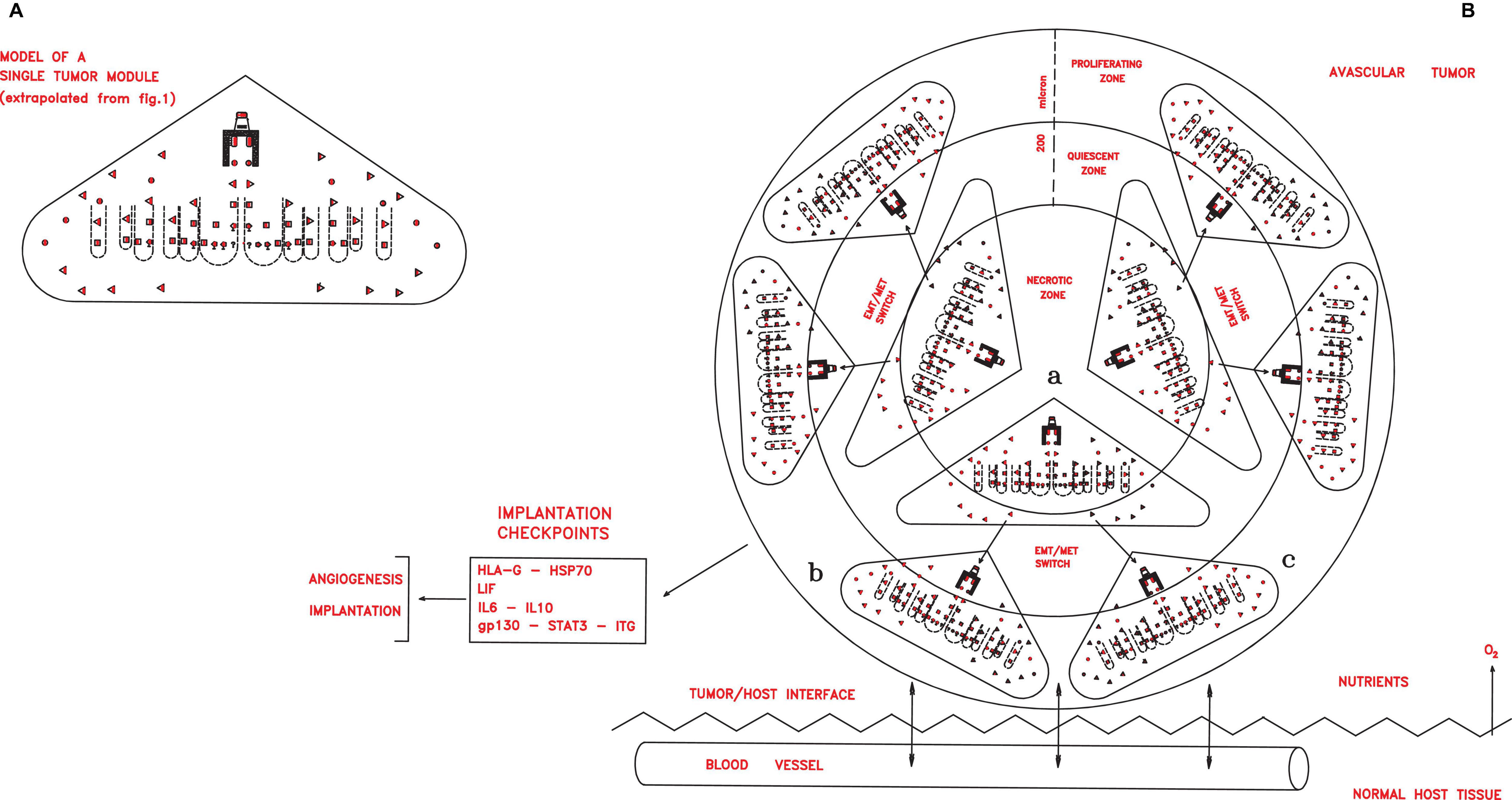
Figure 2. (A) A simplified scheme of a single tumor module, obtained from Figure 1 extrapolating only the symbols depicting the different cancer cells shown in the legends of the Figure 1. In an avascular tumor (B), initial tumor modules (a), located in the central zone, would generate new tumor modules (b,c) in a spherical structure of about 400 microns. Since the nutrient diffusion limits are about 200 μm, further growth would imply cell death in the central zone, while in the peripheral zone growth could occur through neoangiogenesis and vascularization, which allow tumor implantation in the host tissues, thanks to defined checkpoint factors (HLA-G, HSP70, IL-6, and gp130–STAT3–ITG).
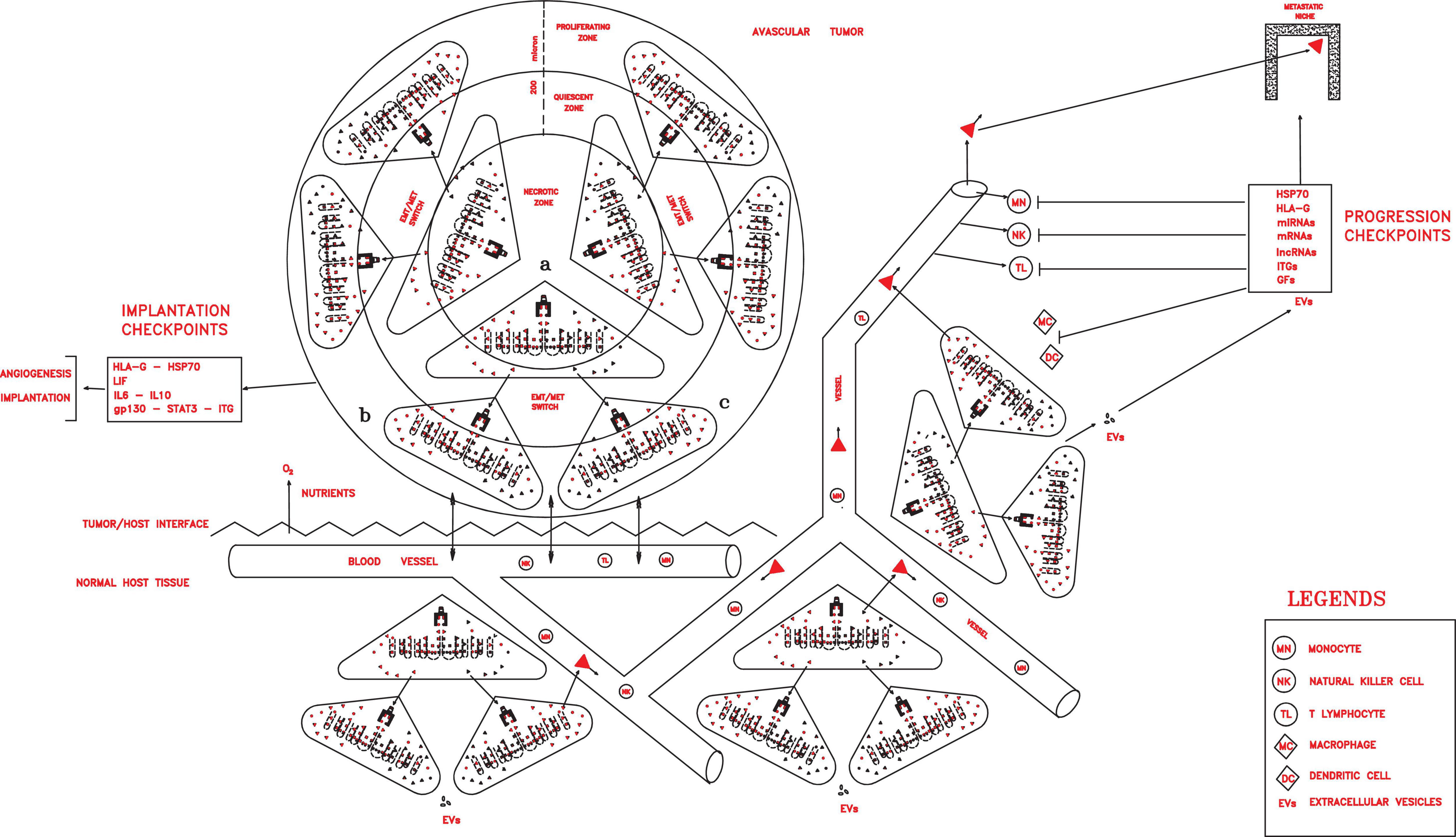
Figure 3. Once implanted and vascularized, a tumor could grow rapidly, invading host tissues, spreading in the circulation, and installing in distant pre-metastatic niches, thanks to progression checkpoint factors (HLA-G, HSP70, miRNAs, mRNAs, lncRNAs, ITGs, and GFs) released in extracellular vesicles (exosomes in particular) by cancer stem cells (CSCs) and cancer progenitor cells (CPCs) and able to impair all immune cells.
Theoretical Checkpoints in the Different Phases of the Cancer Process
In the cancer process, three stages could be distinguished: preimplantation avascular tumor, implantation vascularized tumor, and progressing metastatic tumor.
Avascular Tumors (Preimplantation Tumors)
In this first phase, molecular checkpoints playing a major role could be factors able to confer to cells of an initial tumor persistent stemness, self-renewal, pluripotency, survival, and apoptosis inhibition, such as OCT4, NANOG, SOX2, STAT3, CD44, ID, HLA-G, and HSP70 factors (Figure 1).
OCT4, SOX2, and NANOG
OCT4, SOX2, and NANOG are well known to constitute a sort of molecular engine in both embryogenesis and cancer genesis, regulating the so-called pluripotency gene regulatory network (PGRN), sustaining stemness, pluripotency, self-renewal, and reprogramming (Festuccia et al., 2013).
CD44
This factor is a cell surface protein constituting a signal platform that regulates the expressions of genes related to proliferation, migration, survival, and invasion (Williams et al., 2013; Yan et al., 2015). The CD44 variant isoforms (CD44v3, CD44v6, CD44v8, etc.), absent in normal tissues, seem to be restricted to aggressive tumors and have crucial roles in the regulation of stemness, self-renewal, tumor initiation, metastasis, and chemoresistance (Zeilstra et al., 2014; Chanmee et al., 2015). They are expressed both in epithelial (ALDH1+CD44+Ki67+, hypothetical CSC1s) and mesenchymal (ALDH1–CD44+Ki67–, hypothetical CSC3s) CSCs (Liu et al., 2014; Manzo, 2019). CD44v isoforms are critical during EMT in cancer progression (Xu et al., 2015; Yan et al., 2015; Chen C. et al., 2018). The CD44/STAT3 complex induces both epithelial (proliferation) and mesenchymal (migration) features (Yan et al., 2015), and a transforming growth factor beta (TGF-β)-induced CD44high/ID1high expression occurs in glioma-initiating cells (Anido et al., 2010). Moreover, a positive feedback couples Ras activation and CD44v (Cheng et al., 2006; Krishnamachary et al., 2012). Thus, CD44v could be a sort of molecular trigger of a direct reprogramming (Su et al., 2011) in the EMT/MET switch of quiescent CSC3s to self-renewing CSC1s.
ID1 and ID3
ID1 and ID3 are expressed only in embryonic and cancer cells, but not in most adult tissues. ID1 proteins have multiple roles in several processes, such as in the implantation of CSCs (CSC1s) in primary and metastatic niches, apoptosis inhibition, survival and growth, angiogenesis, and chemoresistance (Lyden et al., 1999; Ling et al., 2006; Niola et al., 2012; Nair et al., 2014). ID1 proteins, in association with ubiquitous E proteins, prevent CSCs from differentiating (Ling et al., 2006), thus determining the crucial “blocking event” that would confer to CSC1s persistent stemness, with self-renewal and pluripotency capacities, reiteratively feeding the tumor. ID1 and ID3 would be necessary for TIC (tumor-initiating cell) functions in the genesis of both primary tumors and metastases, sustaining proliferation via p21 (Gupta et al., 2007; O’Brien et al., 2012). ID1 and ID3 are required for angiogenesis and the vascularization of tumor xenografts (Lyden et al., 1999; Teo et al., 2020) necessary for macro-metastases development.
HSP70s and HLA-Gs
These proteins are precociously expressed in embryogenesis and cancer genesis, but only transiently during mitosis (70-kDa heat shock proteins, HSP70s) or in a few defined organs (human leukocyte antigen G, HLA-Gs) in adults (Stangl et al., 2011; Tilburgs et al., 2015). In preimplantation embryos and initial avascular tumors, they would constitute protection and survival systems, both by preventing apoptosis (HSP70) (Samali and Cotter, 1996) and defending from adverse host microenvironments (HSP70 and membrane HLA-G1), or also a system for invading and colonizing the host tissues (soluble HLA-G5) (Rouas-Freiss et al., 2007; Sheu and Shih, 2010). HLA-Gs are expressed in embryonic and tumor mesenchymal cells (CSC3s) and in progenitor cells (CPCs) (Yen et al., 2009). A small amount of HSP70s is necessary for preimplantation embryogenesis (Luft and Dix, 1999). HSP70s can be expressed on the cell surface or exported in the circulation (Shu and Huang, 2008). Highly metastatic tumors, but not their primary counterparts, express membrane HSP70s. HSP70+ tumors actively release exosomes with an HSP70+ surface (Stangl et al., 2011), which might act in tumor protection, survival, and spread. During embryo development, HLA-Gs orchestrate the early interaction of human trophoblasts with the maternal niche for implantation (Gregori et al., 2015); after implantation, HLA-Gs are expressed in the endothelial cells of developing vessels, mesenchymal cells, and progenitor cells (Hunt et al., 2005; Yao et al., 2005; Verloes et al., 2011). Tumor and mesenchymal cells secrete HLA-Gs in EVs (Yen et al., 2009; Burrello et al., 2016; Rebmann et al., 2016). HLA-G expression has been shown in many cancer types, both in primary and metastatic tumors, mainly as soluble HLA-G5, but also associated with EVs (Rebmann et al., 2003, 2016). HLA-G expression is induced by hypoxia via HIF-1a (Rebmann et al., 2003) and is upregulated by interleukin (IL)-10 with an autocatalytic feedback (Urosevic et al., 2001, 2002). HLA-G induces IL-6 production (Urosevic et al., 2002) and, thus, the activation of the gp130–JAK–STAT3 pathway, regulating proliferation, migration, invasion, and angiogenesis, namely, tumor progression and metastasis (Kamran et al., 2013). Notably, HLA-G expression is also induced and upregulated by chemotherapeutic agents (Rouas-Freiss et al., 2003; Yan et al., 2005).
Vascular Tumors (Implanted Tumors)
In this second phase, the major molecular checkpoints might be factors able to confer to cancer cells implantation properties in primary local niches and angiogenesis for vascularization, such as ID1, ID3, leukemia inhibitory factor and its receptor (LIF/LIFr), IL-6/IL-6r, IL-10, gp130, Janus kinase (JAK), signal transducer and activator of transcription 3 (STAT3), HSP70, and HLA-G factors (Figure 2). LIF/LIFr would have an autocrine/paracrine function in embryo implantation (Cullinam et al., 1996) by activating and regulating the gp130–JAK–STAT3, AKT, and ERK1–2 MAPK signal pathways that induce the expression of integrin a5b1, realizing implantation, endothelial proliferation, and subsequent angiogenesis–vascularization (Cheng et al., 2001; Sherwin et al., 2002; Park et al., 2003). LIF–gp130–STAT3 pathway activation is also linked to IL-6 and IL-10, as well as to HLA-G that interacts with these factors (Urosevic and Dummer, 2003; Yue et al., 2015). In LIF knockout mice and in “in vitro” fertilized human embryos lacking HLA-G5, implantation does not occur (Fuzzi et al., 2002). In many cancer types, including melanomas, skin, kidney, prostate, and pancreatic cancers, a LIF signal is expressed at high levels, inducing an autocrine/paracrine cell proliferation, like in embryo implantation (Cullinam et al., 1996; Kellokumpu-Lehtinen et al., 1996). The LIF amount secreted by a tumor seems to regulate cancer genesis (Guo et al., 2015). In solid tumors, LIF expression is induced by hypoxia via HIF-2a and by TGF-β (Yue et al., 2015). A high LIFr expression is crucial for tumor implantation: analysis of 90 nevi and 441 melanomas shows that LIFr expression is low for all nevus stages, starts to increase in dysplastic nevi, becomes higher in implanted melanomas, and is highest in metastatic melanomas (Guo et al., 2015). Thus, LIF/LIFr, together with interacting IL-6/IL-6r, IL-10, and HLA-G, would be crucial for implantation, autocrine/paracrine growth, the vascularization of primary tumors via gp130–JAK–STAT3 pathways, as well as for genesis of mesenchymal metastasizing cells (Yan et al., 2015).
Progressing Tumors (Metastatic Tumors)
In this tumor phase, many different molecular factors and structures could constitute crucial checkpoints of the cancer process, which are able to favor local growth and angiogenesis and confer properties of invasion and migration, protection and escape from immune surveillance, detection and invasion of specific pre-metastatic niches, and the capacity of dictating tumor conditions in the surrounding microenvironment. Such factors could include CD44v, ID1, ID3, HSP70, HLA-G, LIF/LIFr, IL-6, IL-10, and EVs with their contents, such as exosomal integrins (ITGs), microRNAs (miRNAs), messenger RNAs (mRNAs), long non-coding RNAs (lncRNAs), and growth factors (GFs) (Figure 3).
Essentially, three types of EVs are released by tumor cells, marked on the basis of their size: (a) exosomes, (b) microvesicles, and (c) apoptotic bodies. Exosomes are released constitutively and/or upon cell activation and hypoxia induction, have a size of about 40–150 nm in diameter, and have an endocellular origin from early endosomes. Exosomes enter the recipient cells by an inverse mechanism of endocytosis within endosomes, from which they release their contents in the recipient cell cytoplasm (Vader et al., 2014). Microvesicles have a size of about 50–2,000 nm in diameter, have a membrane origin, are released like the exosomes, and enter the recipient cells by direct membrane fusion, direct endocytosis, or after interaction of their ligands with specific cell surface receptors (Vader et al., 2014). Apoptotic bodies have a size of about 50–50,000 nm, are formed by random blebbing of the plasma membrane, are released by apoptotic tumor cells, and may contain nuclear fragments with DNA and histones, as well as fragments of cytoplasmic organelles, which they can transfer also to normal cells, leading to the development of a full tumorigenic potential (Vader et al., 2014). EVs contain nucleic acids (DNA fragments, oncogenes, mRNAs, miRNAs, and lncRNAs), lipids, proteins (oncoproteins, tetraspanins, Rab GTPases, HSPs, HLA-Gs, and lectins), surface intercellular adhesion molecules (ICAM and integrins A and B), GFs (TGF-β, TNF-α, FGF2, and VEGF), selectins, cytokines (IL-6 and IL-8), and metalloproteases (Dilsiz, 2020; Jurj et al., 2020). The contents of EVs found in body fluids are closely related to the status of the producing cells, of which they can be biomarkers (Vader et al., 2014) and be recognized by or transferred to other cells in a selective manner, thus influencing the phenotype and functions of the recipient cells. In cancer patients, the quantity of circulating EVs seems to be higher than that in healthy subjects and has been found to correlate with poor prognosis (Kim et al., 2003; Vader et al., 2014). Depending on the tumor type and location, EVs can be isolated from plasma, serum, urine, body fluids, and even saliva (Vader et al., 2014). Tumor cells utilize EVs for dictating a defined tumor functional phenotype to surrounding cells (Naito et al., 2017; Jurj et al., 2020). Recent data show that tumor EVs contain molecules for intercellular communications (da Silva Nardi et al., 2016; Rebmann et al., 2016) that act on and impair recipient immune cells, favoring tumor initiation, growth, angiogenesis, immune surveillance, evasion, EMT, invasion, metastasis, and chemoresistance (Sheu and Shih, 2010; Kosaka, 2016). EVs (exosomes in particular) perform distinct roles during each of the sequential steps in the pre-metastatic niche evolution, namely, vascular leakiness, stromal cell education at organ-tropic sites, bone marrow-derived cell education and recruitment (Hoshino et al., 2015). Exosomes, with their contents, play roles in the sequential steps of the whole tumor process, from the primary tumor site modulation (such as the induction of angiogenesis, EMT, and immune suppression) to organ-specific metastasis homing, involving integrins, miRNAs, GFs, and growth factor receptors (GF-Rs) (Li et al., 2019). Exosomes interact with pre-metastatic niches, inducing angiogenesis, immune modulation, and reprogramming (Grange et al., 2011). Such an induced reprogramming activity by EVs could also be responsible for the conversion of non-CSCs into CSCs upon radio-chemotherapeutic treatments, which, in destroying CSCs, likely spread the EVs of these both in the tumor microenvironment and systemically (Chen et al., 2017; Ozawa et al., 2018; Keklikoglou et al., 2019; Lu et al., 2020). Moreover, exosomes help metastatic circulating cells in escaping immune surveillance and surviving in the blood circulation (Li et al., 2019). EVs from highly metastatic tumor cells have been shown to carry significantly different cargoes than do EVs from poorly metastatic cells (Rana et al., 2013; Vader et al., 2014). Exosomes reflect the status of donor cells, such as the hypoxic status of glioma cells, where they mediate the hypoxia-dependent activation of vascular cells during tumor development (Kucharzewska et al., 2013; Vader et al., 2014; Han et al., 2019).
Embryonic Inductions and Tumor Inductions
Tumor progression necessarily requires the control and the overexploitation of the host surrounding microenvironment. To this end, tumor cells can dictate tumor functional phenotypes to surrounding cells through molecules for intercellular communications, such as HLA-G and exosomal miRNAs, as embryonic cells also do (da Silva Nardi et al., 2016; Rebmann et al., 2016; Dilsiz, 2020; Pillay et al., 2020). These molecules could induce such phenotypes by activating or silencing defined genic systems in the recipient cells.
Embryonic Inductions and Histo-Organogenesis
The above inductive process could occur through EV contents (Sheu and Shih, 2010; da Silva Nardi et al., 2016; Rebmann et al., 2016; Naito et al., 2017; Jurj et al., 2020; Pillay et al., 2020) and be similar to the embryonic inductions occurring between mesenchymal and epithelial cells from early gastrula to organogenesis, during which the embryo structures progressively develop (Balinsky, 1970). It is known that embryonic inductions require a direct contact between mesenchymal and epithelial cells (Balinsky, 1970), as described in the SCID mouse model, between meta-nephric mesenchymal (MM) cells and ureteric bud (UB) epithelial progenitor cells in a three-dimensional co-culture, allowing for a direct cell–cell contact (Ratajczak et al., 2006; Valadi et al., 2007; Velagapudi et al., 2012). Here, a well-orchestrated series of reciprocal inductive events leads to the progressive formation of different structures of an early simple nephrogenesis (Velagapudi et al., 2012). Factors known to induce MM cells are present in UB cell-conditioned media and include several GFs, such as epidermal growth factor (EGF), TGF-α, basic fibroblast growth factor (bFGF), bone morphogenetic protein 7 (BMP7), hepatocyte growth factor (HGF), that could be indicated as “epithelial inductors.” Whereas, factors able to induce UB cells are present in MM cell-conditioned media and include the glial cell line-derived neurotrophic factor (GDNF), HGF, and extracellular matrix (ECM) proteins (collagen, fibronectin, and laminin), which could be indicated as “mesenchymal inductors” (Balinsky, 1970; Ratajczak et al., 2006; Velagapudi et al., 2012), with HGF as both an epithelial and mesenchymal inductor. These reciprocal inductions could trigger, in both epithelial and mesenchymal cells, a progressive consequent activation and/or silencing of defined genes, thus realizing a specific “inductive gene chain” (IGC) that finally confers to each cell of the generated progeny a distinct phenotype, resulting in a defined histological and physiological cell hierarchy in the histo-organogenesis process. The terminal IGC of each cell type would then be preserved by a defined genic homeostasis through genetic, epigenetic, and microenvironment signals.
Tumor Inductions and Metastasis
Now, I hypothesize that such a type of intercellular induction could also occur in the cancer progression between mesenchymal CSCs (CSC3s) and surrounding cells, both tumor cells (CPCs and CDCs) and normal host cells (fibroblasts, macrophages, epithelial, and endothelial cells), through the EV contents of CSCs and CPCs, dictating new tumor-associated phenotypes. The induced cells would reciprocally supply their epithelial inductors to mesenchymal CSCs for generating oligopotent CPCs that, actively proliferating, would generate abundant different CDCs, thus determining a defined cell hierarchy and the histopathological features of a tumor. This process of consequential reciprocal inductions would occur through defined “on/off” switches, according to the genic program (IGC) of the origin cancer cell (CSC0). The level of realization of a defined IGC within the induced cells of a tumor would be responsible for their differentiation degree and, thus, for the different malignancy levels. I think that a crucial mechanism for the inductions in tumor progression is based on the cargoes of EVs (exosomes in particular) (Sheu and Shih, 2010; da Silva Nardi et al., 2016; Rebmann et al., 2016; Naito et al., 2017; Jurj et al., 2020; Pillay et al., 2020). In the recipient cancer and normal cells, exosomes deliver their contents modulating cell signaling pathways. Indeed, their repertoire of different components seems to constitute just a molecular machinery suitable for realizing cell induction processes for the growth and development of metastases, as equivalent to rudiments of organ portions (Velagapudi et al., 2012; Jurj et al., 2020). Cancer cells can produce about 10 times more exosomes than do normal cells. Released in the surrounding tumor microenvironment, exosomes have important roles in tumor initiation, progression, immunosuppression, neovascularization, metastasis, and drug resistance. Tumor cell-released exosomes can be taken up by the surrounding cells and travel through biological fluids, such as the blood, urine, and saliva (Dilsiz, 2020). Thus, cancer-associated EVs might exert systemic effects through the transfer of their cargoes, resulting in the reprogramming of recipient cells (stromal cells, immune cells, and bone marrow-derived cells) in the surrounding tumor microenvironment (Han et al., 2019). I believe that all this would reflect the induction phenomena in embryo development from the gastrula to the organogenesis phase (Balinsky, 1970), where direct cell-to-cell interactions between mesenchymal and epithelial cells occur and are indispensable for the pleiotropic development of defined biological structures.
Properties and Roles of EV Contents in Cancer
Integrins
Adhesion and ECM molecules, such as integrins, tenascin, and periostin, were shown to promote metastases of spreading cancer cells (Weaver et al., 1997; Oskarsson et al., 2011; Fukuda et al., 2015; Hoshino et al., 2015). On tumor-derived exosomes, a specific repertoire of ITGs has been detected, which dictates their adhesion to specific cell types and ECM molecules in particular organs. ITGs expressed on the exosome surface could be specific for a defined tumor kind: for example, the exosomal ITGs a6b4 and a6b1 are associated with lung metastases, while ITG a1b5 is linked to liver metastases (Hoshino et al., 2015; Sung et al., 2015; Li et al., 2019). ITGs might determine organ-specific metastatic sites, both controlling directional cell movements through tissues in association with fibronectin (Sung et al., 2015) and preparing pre-metastatic niches through the ITG-mediated fusion of exosomes with organ-specific resident cells, as well as likely reactivating stemness genes (OCT4, SOX2, NANOG, and KFL4) via S100 factors (Lu et al., 2020). Thus, tumor exosome ITGs might determine organotropic metastasis through sequential mechanisms for the organ-specific homing process (Valadi et al., 2007; Hoshino et al., 2015). For example, exosomes expressing ITG avb5 specifically bind Kupffer cells in the liver, while exosomes expressing ITGs a6b4 and a6b1 bind lung resident fibroblasts and epithelial cells (Hoshino et al., 2015). This specific organotropism and the related uptake of defined exosome ITGs promote, in the target cells, the upregulation of different pro-inflammatory and pro-migratory S100 genes in lung fibroblasts and Kupffer cells, but not in lung epithelial cells. Since S100A4 regulates lung metastasis and is controlled by ITG a6b4 (Hoshino et al., 2015), it has been suggested that this ITG activates the S100A4–Src axis in lung fibroblasts during the pre-metastatic niche formation (Hoshino et al., 2015). Moreover, since S100A10 facilitates OCT4-mediated breast cancer stemness (Lu et al., 2020), exosome ITGs could not only promote specific organotropic adhesion but also trigger the signaling pathways for stemness and reprogramming, as well as for inflammatory responses in target organs (Hoshino et al., 2015). Notably, exosomes secreted by a certain tumor are sufficient to redirect the organotropism of metastases of a different tumor type, normally unable to metastasize a particular organ (Hoshino et al., 2015). The exosomal ITG expression profiles of plasma exosomes isolated from cancer patients could be used for predicting sites of future metastasis and for developing diagnostic tests and therapeutic tools (Hoshino et al., 2015). After the ITG-mediated organotropic incorporation of tumor exosomes in recipient cells, ITGs have been shown to upregulate the expressions of several S100 genes related to cell migration and pro-inflammation (Hoshino et al., 2015). Now, since many different ITGs and S100 genes exist, I think that they, together with other factors, such as exosomal miRNAs, lncRNAs, and mRNAs, could constitute or be part of a cell-specific inductive machinery finally activating the IGC of a recipient cell at a defined genic level (Hoshino et al., 2015; Lu et al., 2020) related to the IGC of the tumor donor cell, thus determining the differentiation degree of the recipient cell.
miRNAs
MicroRNAs represent the major class of small (20–22 nt), single-strand, non-coding RNA molecules. They are known to be fundamental regulators of gene expression in cancer cells, mainly as negative regulators of mRNA translation by binding to its complementary sequences (about 6–8 nt) into the 5′ or the 3′ region, thus leading to the degradation of specific target mRNAs or to the inhibition of their translation at a posttranscriptional level (Dilsiz, 2020). It is believed that miRNAs control about 60% of all the protein-coding genes in humans through miRNA–mRNA regulatory relationships, where many different miRNAs are often required to target a single mRNA molecule by a mechanism recognizing complementary sequences, with subsequent mRNA degradation or translation repression (Dilsiz, 2020). miRNAs are transferred to target recipient cells by exosomes, which protect them from degradation until their entry into target cells. Since cancer cells produce a high variety of exo-miRNAS that promote tumor proliferation, angiogenesis, and migration, these onco-miRNAs may be good biomarkers for many types of cancer, providing information about the identity of the type of cells releasing them and about their target cells (Dilsiz, 2020). Moreover, miRNAs in exosomes derived from leukemia cells (miRNA17–22 cluster) are involved in the migration and maturation of endothelial cells for cancer angiogenesis (Umezu et al., 2013; Jurj et al., 2020). Exo-miRNAs and other RNAs are also responsible for the activation or suppression of the innate and adaptive immune systems, such as miRNAs that act as TRL (Toll-like receptor) ligands in different types of cancer, stimulating their progression (Fabbri et al., 2012; Jurj et al., 2020). The exosomes of glioma stem cells with overexpression of miRNA-21 can be delivered to endothelial cells, thus stimulating neoangiogenesis, as exosomes from renal CSCs also do (Grange et al., 2011; Jurj et al., 2020). Exosome-specific miRNAs transferred from chemoresistant cancer cells in sensitive recipient cells can confer horizontal resistance through the modulation of drug-induced apoptosis, signaling pathways, and gene expression (Chen W. X. et al., 2014; Mao et al., 2016; Qin et al., 2017; Jurj et al., 2020). In breast cancer cells, exosomes contain different miRNAs that can modify the expression profiles of specific target genes, such as p27 by miRNA-24, as well as the chemoresistance by miRNA-5p (Mao et al., 2016; Jurj et al., 2020). Thus, miRNAs from tumor EVs might have several roles, such as silencing the genic program activities of recipient cells by repressing the translation of their mRNAs (Vader et al., 2014; Dilsiz, 2020) as well as inducing reprogramming at a genic level related to that of the donor cell (Kucharzewska et al., 2013), favoring angiogenesis for tumor progression and transferring horizontal chemoresistance.
mRNAs
Messenger RNAs from tumor EVs would have the function of being translated into donor proteins (Ratajczak et al., 2006; Valadi et al., 2007; Vader et al., 2014) able to reactivate in recipient cells a genic program for inducing a new phenotype, with properties related to the status of the donor (Kucharzewska et al., 2013; Han et al., 2019). In effect, the mRNAs contained in microvesicles released by activated macrophages reflect specific phenotypes of the classically activated pro-inflammatory M1 or, alternatively, activated anti-inflammatory M2 macrophages (Garzetti et al., 2014; Jurj et al., 2020), thus indicating that the cell phenotype can be dictated by mRNAs transferred in recipient cells. Microvesicle mRNAs from colorectal cancer cells also promote the proliferation of endothelial cells (Hong et al., 2009).
LIF, IL-6, and GFs
LIF, IL-6, GFs, and other factors could have the role of stimulating recipient cell growth and angiogenesis (Hood et al., 2009; Li et al., 2019), as well as of interacting with specific membrane receptors on target cells, thus activating defined endocellular pathways, such as the IL-6–gp130–JAK–STAT3 pathway. CSC-specific signaling proteins (β-catenin), specific surface receptors (CD133 and CD44), stem cell factors (OCT4), functional enzymes (ALDH), and transcription factors (TFs), which are activators of cell pathways all exported in exosomes, can mediate tumor stroma modulations by cancer cells and vice versa (Pavlides et al., 2009; Boelens et al., 2014; Richards et al., 2017; Jurj et al., 2020).
HSP70s and HLA-Gs
HSP70s and membrane HLA-G1–4 on tumor cell would have a role of defense in the direct contact with immune [T lymphocytes (TLs) and natural killer (NK)] cells, neutralizing their cytotoxic activities or even inducing their apoptosis (Rouas-Freiss et al., 2003; Chalmin et al., 2010). HSP70s and soluble HLA-G5–7 released with tumor cell exosomes could favor and consent the invasion and colonization of host tissues in a systemic way, blocking all the components of the host immune system and enslaving other host cells for their ends (Rouas-Freiss et al., 2003; Sheu and Shih, 2010). The above series of events associated with EV contents clearly indicates that, in tumor progression, a real strategy exists for realizing an expansion–invasion–colonization process through cell inductions, which recalls those during embryo development. In this process, EVs and their contents could really be the crucial checkpoints in tumor progression since they can mediate and dictate the communications within the microenvironment both at the interface tumor/host tissues (Milane et al., 2015) and at a systemic level. In this strategy, each EV factor could carry on defined roles: defense from immune cells (HLA-Gs and HSP70s), detection of and adhesion to organ-specific pre-metastatic niches (ITGs), expression of oncoproteins in recipient cells (mRNAs), silencing and or activation of recipient cell genes (miRNAs and lncRNAs), and angiogenesis and growth (HLA-Gs, IL-6, LIF, and GFs).
Current and Prospective Anticancer Strategies
During cancer development, different checkpoints could constitute potential therapeutic targets in the various phases of the process.
Aspecific Targets
The following targets would be checkpoints theoretically associated with the development of different tumor types.
ID1, ID3, and CD44v
Inhibition of ID1 expression suppresses invasion and metastases in aggressive salivary and breast cancer (Fong et al., 2003; Murase et al., 2016). ID protein inhibition by a peptide aptamer induces cell cycle arrest and apoptosis in ovarian cancer cells (Mern et al., 2010a), and the inactivation of ID1 genes induces sensitivity of prostate cancer cells to chemotherapeutic drugs (Wong et al., 2008). Co-suppression of ID1 and ID3 significantly reduces proliferation, invasiveness, anchorage-independent growth, and angiogenesis and increases apoptosis in small-cell lung cancer (Chen D. et al., 2014); moreover, targeting ID1 and ID3 reduces the formation of peritoneal metastases by gastric cancer cells (Tsuchiya et al., 2005). Targeting ID1 and ID3 by a specific peptide aptamer induces E-box promoter activity, cell cycle arrest, and apoptosis in breast cancer cells (Mern et al., 2010b). Thus, targeting ID1 and ID3 could prevent growth, angiogenesis, and progression in primary avascular tumors and initial metastatic lesions. Knockdown of CD44 induces the differentiation of breast CSCs (Pham et al., 2011). CD44v isoforms are promising targets for the elimination of CSCs (Jin et al., 2006; Orian-Rousseau and Ponta, 2015; Yan et al., 2015). The inhibition of CD44v3 and CD44v6 by the A5G27 peptide copolymer blocks tumor invasion and metastatic colonization (Zaiden et al., 2017). Thus, targeting CD44v could exert synergic effects with ID1/ID3 targeting to block the stemness and migration of CSCs.
LIF/LIFr, IL-6, HLA-Gs, and HSP70s
LIFr knockdown inhibits the migration of melanoma cells in wound-healing tests (Guo et al., 2015). Neutralizing antibodies (Abs) knock down the activity or expression of LIF and reduce in vitro the stem cell-like properties of murine slow-growing CSCs (American association for cancer research, 2012). The conformational anti-HLA-G monoclonal antibody (mAbs) 87G, as well as IL-2, IL-12, and IL-15, restores the NK activity drastically inhibited by HLA-Gs (Tilburgs et al., 2015). HSP70s could be an immune therapeutic target in a wide spectrum of tumor types, and cmHsp70.1 mAbs can significantly reduce the bulk of mHSP70 + CT26 mouse colon tumors (Stangl et al., 2011). Thus, targeting the above factors could prevent the implantation of initial avascular tumors, their vascularization, and subsequent progression.
Specific Targets
These targets would be checkpoints theoretically associated with a defined tumor type or even to a single tumor.
EVs
Extracellular vesicles are recently becoming an emerging target in cancer therapy, and currently, several clinical trials using exosome-based cancer therapy are ongoing (Vader et al., 2014; Abak et al., 2018; Chulpanova et al., 2018). The origin and concentration of circulating microparticles differ according to the type and evolution of cancers (Kucharzewska et al., 2013; Mege et al., 2016; Jurj et al., 2020). As natural carriers for diverse bioactive cargoes, EVs are potential vehicles for the delivery of many forms of therapeutic substances, including mRNAs, miRNAs, lncRNAs, proteins, and drugs (doxorubicin, paclitaxel, curcumin, and acridine orange) (Pascucci et al., 2014; Srivastava et al., 2016; Wang J. et al., 2016; Bunggulawa et al., 2018; Han et al., 2019). Exosomes can be isolated from a patient’s fluids and, after suitable modification, transferred to the same patient for a targeted cancer therapy. Studies have reported a significant higher efficacy of drugs loaded into exosomes when compared with free drugs and can cross biological barriers, even the blood–brain barrier (Yang et al., 2015; Dilsiz, 2020). One exosome-based therapeutic strategy is the inhibition of onco-miRNAs by the delivery of antagonist tumor-suppressive complementary miRNAs, injected either systemically or locally into the tumor (Dilsiz, 2020). Systemically injected exosomes targeted to the EGF receptor (EGFR) deliver antitumor miRNAs to breast cancer cells (Ohno et al., 2013; Jurj et al., 2020). Exosomes can be used as nanoparticles for suppressing the tumor growth and angiogenesis in gastric cancer by delivering HGF siRNAs (Zhang et al., 2018). Another original therapeutic strategy seems to be the removal of exosomes from circulation by extracorporeal hemofiltration or the prevention of the fusion and uptake of exosomes by target cancer cells (Marleau et al., 2012; Dilsiz, 2020; Jurj et al., 2020). On the other hand, exosomes can interfere with the activity of immunotherapeutic agents, such as therapeutic antibodies, which, a few hours after administration, result in approximately one-third to one-half bound to target cell exosomes (Battke et al., 2011). Human tumor-derived exosomes downmodulate NKG2D expression and inhibit the binding of Abs with tumor cells, reducing the antibody-dependent cellular cytotoxicity (ADCC) (Clayton et al., 2008; Ashiru et al., 2010; Battke et al., 2011). Similarly, tumor-derived exosomes participate in chemotherapeutic resistance by exporting certain drugs from cisplatin-resistant ovarian cancer cells (Safaei et al., 2005), thus impairing the endocellular activity of the drug.
ITGs
When uptaken in specific organs, tumor-derived exosomes prepare the pre-metastatic niche through distinct expression patterns of the ITGs associated with the metastases of defined organs. Targeting exosomal ITGs may effectively block organ-specific metastasis; targeting ITG a6b4, the exosome uptake and metastases in lungs decrease, as well as targeting ITG avb5 in the liver. Exosomal ITGs and exosome-inducible S100 molecules can constitute targets for an anti-metastatic combination therapy (Hoshino et al., 2015). ITG a6b4 controls the expression of genes associated with cell motility, invasion, and metastasis, including S100A4/metastasin (Grum-Schwensen et al., 2005; Chen et al., 2009; Kim et al., 2009; Lukanidin and Sleeman, 2012; Hoshino et al., 2015). In mice lacking the S100A4 gene, tumor development and metastasis formation are suppressed (Grum-Schwensen et al., 2005; Lukanidin and Sleeman, 2012; Hoshino et al., 2015). Silencing of S100A10 blocks the chemotherapy-induced enrichment of breast CSCs, impairs tumor initiation, and delays recurrence (Pillay et al., 2020). In three-dimensional culture and in vivo, reversion of the malignant phenotype of human breast cancer cells occurs by ITG blocking antibodies (Weaver et al., 1997; Hoshino et al., 2015).
miRNAs
Besides potential diagnostic and prognostic biomarkers in cancer monitoring, exo-miRNAs can be used in therapeutic strategies, such as the inhibition of onco-miRNA expression by the delivery of antagonist tumor-suppressor miRNAs: oligonucleotides complementary to the sequences of the targeted onco-miRNAs, loaded into exosomes, can be delivered both systemically and by local injections in the tumor bulk (Dilsiz, 2020). Tumor-suppressor miRNAs, loaded in exosomes and delivered, can inhibit pro-angiogenic mRNAs or knockdown specific genes for inhibiting tumor growth (Dilsiz, 2020); the exosome-formed synthetic miRNA-143 transferred to osteosarcoma cells inhibits their migration (Shimbo et al., 2014; Jurj et al., 2020). Notably, consistent with the para-embryonic nature of cancer, miRNAs have been shown in pregnancy, which may provide insights into a possible cure for cancer (Pillay et al., 2020). Cancer cell exosomes depend on cell surface heparan sulfate proteoglycans for their internalization and functional activity (Christianson et al., 2013; Vader et al., 2014), and heparin blocks EV transfer between donor and recipient cells (Atai et al., 2013; Vader et al., 2014).
Overall, ID1, ID3, CD44, LIF/LIFr, HLA-G, and HSP70 could be crucial aspecific targets mainly in primary tumor development, whereas exosome mRNAs, miRNAs, lncRNAs, ITGs, and related S100 factors might be important specific targets for blocking tumor progression and recurrence as well as for inhibiting organotropic metastasis locations.
Discussion and Proposals: “Tumor Checkpoint Profiles” for “Shielded” Cancer Treatments
Final Considerations
One first consideration about the aforesaid targets is that the non-specific checkpoints (ID1, ID3, CD44v, LIF/LIFr, HSP70, and HLA-G) would be theoretically associated with the development of different tumor types, while the specific checkpoints (miRNAs, lncRNAs, mRNAs, ITGs, and S100s) could be associated with a defined tumor type or, perhaps, to a single tumor. Thus, since these specific factors are circulating in biological fluids (Dilsiz, 2020) and reflect the status of tumor donor cells (Kucharzewska et al., 2013; Han et al., 2019), it would be important to detect and identify the different EV factors from tumors of the same kind, with similar differentiation degree and molecular features, for knowing whether common crucial characterizing checkpoints exist and act in the induction and maintenance of a specific phenotype in the CSCs and non-CSCs of similar tumors. To this end, it might be useful to collect, in a systematic manner (Table 1), all the data about each tumor in a sort of “cancer checkpoint hub” (Mege et al., 2016) in order to create a “molecular checkpoint profile” of each tumor type. This might allow characterizing the different tumor types, knowing their evolution, and detecting eventual common specific and aspecific targets suitable for aimed tumor treatments. In such a direction, it could be relevant to explore, for example, the potential properties of the ITG a2b1 as a potential marker and driver of all cancer metastasis types, protection from which could avoid all metastatic events (Hoshino et al., 2015).
Targeting the tumor cells immunologically with mAbs binding the complement (ADCC), or with other cytolytic immune mechanisms (NK cells and cytotoxic TLs), could result unfavorably (Wieckowski et al., 2009; Chen X. et al., 2018; Lin and Yan, 2019) since EVs released from killed tumor cells would spread in the microenvironment and in circulation, transferring their pathogenetic components to other cells, which might be induced to convert from non-CSCs into CSCs (Chen et al., 2017; Lu et al., 2020), enslaved to tumor ends (Sheu and Shih, 2010; Vader et al., 2014; Crange et al., 2015; Chen X. et al., 2018), or even killed by apoptosis (Lindman et al., 2006; Wieckowski et al., 2009).
Chemo- or radiotherapeutic treatments also could have the aforesaid unfavorable effects (Yan et al., 2005; Chen et al., 2017; Lu et al., 2020) since they might spread the cellular contents of the killed tumor cells, in particular EVs, which would enter other cancer and non-cancer cells, leading to the recurrence of the disease in time, as, in general, really occurs with these therapeutic treatments. In such a direction, it would be interesting to explore the blocking effect of heparin on the EV transfer between donor and recipient cells (Atai et al., 2013; Christianson et al., 2013) as a potential anti-EV molecular shield in cytotoxic cancer treatments.
Removal of immunosuppressive exosomes from the patient circulation by extracorporeal hemofiltration has been suggested and carried out as a therapeutic adjuvant in cancer treatment (Marleau et al., 2012). This idea appears to be good since such a technique could eliminate a likely crucial mechanism of tumor relapse and metastasis, in accordance with the above considerations.
The tumor bulk hierarchic structure, for which I suggested a modular organization of different cancer cell populations (Figures 1, 3) (Manzo, 2020), also might be a crucial problem to be considered in cytotoxic therapeutic strategies.
“Shielded” Cytolytic Cancer Treatments
The emerging data about the induction properties of EVs and their contents in tumor progression and metastasis now allow a better view of the whole cancer process, where exosome contents appear to be crucial specific checkpoints in tumor immune evasion, recurrence, and metastasis. In this scenario, it seems very important to consider that any destruction of CSCs, by surgery or radio-, chemo-, or immunotherapy, could inevitably lead to disease recurrence and metastasis because of the induction of stemness by EV contents (Yan et al., 2005; Chen et al., 2017; Lu et al., 2020). Therefore, it would be indispensable to carry out cytotoxic antitumor treatments under protection from this activity, on the basis of the molecular checkpoint profile of a defined tumor type. To this end, the following general multistep therapeutic strategy could be proposed for back-dismantling the tumor hierarchic histological structure, preventing recurrence and metastasis:
Step 1: Depletion of non-proliferative differentiated tumor cells (CDCs) by conventional chemotherapeutic agents (Wang T. et al., 2016; Roy et al., 2018) and/or radiotherapy, killing different bulk cancer cells. Since non-specific chemotherapies or radiotherapy, besides CDCs, also kill random CSCs, releasing exosomal factors with stemness induction properties (Chen et al., 2017), these cytolytic treatments should be effectuated under protection from whole exosomes (for example by hemofiltration or heparin) (Atai et al., 2013; Christianson et al., 2013) and free exosomal factors (for example by anti-HLA-G/HSP70/ITG/S100 mAbs or by miRNAs targeting onco-RNAs) (Weaver et al., 1997; Stangl et al., 2011; Hoshino et al., 2015; Tilburgs et al., 2015; Dilsiz, 2020) to avoid immune system impairment, non-CSC/CSC transition (recurrence), and pre-metastatic niche induction (metastasis).
Step 2: Depletion of actively proliferating tumor cells (CSC2s and CPCs) by cell cycle-independent apoptotic drugs, like alkylating agents (Wang T. et al., 2016; Roy et al., 2018), yet under a protected approach, as in step 1.
Step 3: Elimination of slow- and non-proliferating CSCs (CSC1s and CSC3s), always under a shielded approach, like in step 1, by anti-CSC chemotherapeutic agents (Wang T. et al., 2016; Roy et al., 2018), mi-Abs or aptamers specifically targeting ID1, ID3, CD44, and LIF/LIFr (Heap et al., 2005; Pecak et al., 2020), or also in a natural way by NK cells that preferentially target tumor cells with a CSC phenotype (Ames et al., 2015), now easily accessible in the tumor site, after the depletion of CSC2s, CPCs, and CDCs.
Data Availability Statement
The original contributions presented in the study are included in the article/supplementary material, further inquiries can be directed to the corresponding author/s.
Author Contributions
The author confirms being the sole contributor of this work and has approved it for publication.
Conflict of Interest
The author declares that the research was conducted in the absence of any commercial or financial relationships that could be construed as a potential conflict of interest.
References
Abak, A., Abhari, A., and Rahimzadeh, S. (2018). Exosomes in cancer: small vesicular transporters for cancer progression and metastasis, biomarkers in cancer therapeutics. Peer J 6:e4763. doi: 10.7717/peerj.4763
American association for cancer research (2012). Leukemia Inhibitory Factor May be a Promising Target Against Pancreatic Cancer. Philadelphia: American association for cancer research.
Ames, E., Canter, R. J., Grossenbacher, S. K., Mac, S., Chen, M., Smith, R. C., et al. (2015). NK cells preferentially target tumor cells with a cancer stem cell phenotype. J. Immunol. 195, 4010–4019. doi: 10.4049/jimmunol.1500447
Anido, J., Saez-Borderias, A., Gonzalez-Junca, A., Rodon, L., Folch, G., Carmona, M. A., et al. (2010). TGF-beta receptor inhibitors target the CD44(high)/Id1(high) glioma-initiating cell population in human glioblastoma. Cancer Cell 18, 655–668. doi: 10.1016/j.ccr.2010.10.023
Ashiru, O., Boutet, P., Fernandez-Messina, L., Aguera-Gonzalez, S., Skepper, J. N., Vales-Gomez, M., et al. (2010). Natural killer cell cytotoxicity is suppressed by exposure to the human NKG2D ligand MICA∗008 that is shed by tumor cells in exosomes. Cancer Res. 70, 481–489. doi: 10.1158/0008-5472.can-09-1688
Atai, N. A., Balaj, L., van Veen, H., Breakefield, X. O., Jarzyna, P. A., van Noorden, C. J. F., et al. (2013). Heparin blocks transfer of extracellular vesicles between donor and recipient cells. J. Neurooncol. 115, 343–351. doi: 10.1007/s11060-013-1235-y
Balinsky, B. (1970). Introduzione alla Embriologia. Italian version of ‘An introduction to Embryology’. Bologna: Zanichelli SpA, 215–239.
Battke, C., Ruiss, R., Welsch, U., Wimberger, P., Lang, S., Jochum, S., et al. (2011). Tumour exosomes inhibit binding of tumour-reactive antibodies to tumour cells and reduce ADCC. Cancer Immunol. Immunother. 60, 639–648. doi: 10.1007/s00262-011-0979-5
Boelens, M. C., Wu, T. J., Nabet, B. Y., Xu, B., Qiu, Y., Yoon, T., et al. (2014). Exosome transfer from stromal to breast cancer cells regulates therapy resistance pathways. Cell 159, 499–513. doi: 10.1016/j.cell.2014.09.051
Bradshaw, A., Wickremsekera, A., Tan, S. T., Peng, L., Davis, P. F., and Itinteang, T. (2016). Cancer stem cell hierarchy in glioblastoma multiforme. Front. Surg. 3:21. doi: 10.3389/fsurg.2016.00021
Bunggulawa, E. J., Wang, W., Yin, T., Wang, N., Durkan, C., Wang, Y., et al. (2018). Recent advancements in the use of exosomes as drug delivery systems. J. Nanobiotechnol. 16:81.
Burrello, J., Monticone, S., Gai, C., Gomez, Y., Kholia, S., and Camussi, G. (2016). Stem cell-derived extracellular vesicles and immune- modulation. Front. Cell Dev. Biol. 4:83. doi: 10.3389/fcell.2016.00083
Cabrera, M. C., Hollingsworth, R. E., and Hurt, E. M. (2015). Cancer stem cell plasticity and tumor hierarchy. World J. Stem Cells 7, 27–36. doi: 10.4252/wjsc.v7.i1.27
Chalmin, F., Ladoire, S., Mignot, G., Vincent, J., Bruchard, M., Remi-Martin, J. P., et al. (2010). Membrane-associated Hsp72 from tumor-derived exosomes mediates STAT3-dependent immunosuppressive function of mouse and human myeloid-derived suppressor cells. J. Clin. Invest. 120, 457–471. doi: 10.1172/JCI40483
Chanmee, T., Ontong, P., Kimata, K., and Itano, N. (2015). Key roles of hyaluronan and its CD44 receptor in the stemness and survival of cancer stem cells. Front. Oncol. 10:180. doi: 10.3389/fonc.2015.00180
Chen, C., Zhao, S., Karnad, A., and Freeman, J. W. (2018). The biology and role of CD44 in cancer progression: therapeutic implications. J. Hematol. Oncol. 11:64. doi: 10.1186/s13045-018-0605-5
Chen, D., Forootan, S. S., Gosney, J. R., Forootan, F. S., and Ke, Y. (2014). Increased expression of ID1 and ID3 promotes tumorigenicity by enhancing angiogenesis and suppressing apoptosis in small cell lung cancer. Genes Cancer 5, 212–225. doi: 10.18632/genesandcancer.20
Chen, M., Sinha, M., Luxon, B. A., Bresnick, A. R., and O’Connor, K. L. (2009). Integrin alpha6beta4 controls the expression of genes associated with cell motility, invasion, and metastasis, including S100A4/metastasin. J. Biol. Chem. 284, 1484–1494. doi: 10.1074/jbc.m803997200
Chen, W. X., Liu, X. M., Lv, M. M., Chen, L., Zhao, J. H., Zhong, S. L., et al. (2014). Exosomes from drug-resistant breast cancer cells transmit chemoresistance by a horizontal transferof microRNAs. PLoS One 9:e95240. doi: 10.1371/journal.pone.0095240
Chen, X., Liao, R., Li, D., and Sun, J. (2017). Induced cancer stem cells generated by radiochemotherapy and their therapeutic implications. Oncotarget 8, 17301–17312. doi: 10.18632/oncotarget.14230
Chen, X., Zhou, J., Li, X., Wang, X., Lin, Y., and Wang, X. (2018). Exosomes derived from hypoxic epithelial ovarian cancer cells deliver microRNAs to macrophages and elicit a tumor-p romoted phenotype. Cancer Lett. 435, 80–91. doi: 10.1016/j.canlet.2018.08.001
Cheng, C., Yaffe, M. B., and Sharp, P. A. (2006). A positive feedback loop couples Ras activation and CD44 alternative splicing. Genes Dev. 2006, 1715–1720. doi: 10.1101/gad.1430906
Cheng, J. G., Chen, J. R., Hernandez, L., Alvord, W. G., and Stewart, C. L. (2001). Dual control of LIF expression and LIF receptor function regulate Stat3 activation at the onset of uterine receptivity and embryo implantation. PNAS 98, 8680–8685. doi: 10.1073/pnas.151180898
Christianson, H. C., Svensson, K. J., van Kuppevelt, T. H., Li, J. P., and Belting, M. (2013). Cancer cell exosomes depend on cell-surface heparan sulfate proteoglycans for their internalization and functional activity. Proc. Natl. Acad. Sci. U.S.A. 110, 17380–17385. doi: 10.1073/pnas.1304266110
Chulpanova, D. S., Kitaeva, K. V., James, V., Rizvanov, A. A., and Solovyeva, V. V. (2018). Therapeutic prospects of extracellular vesicles in cancer treatment. Front. Immunol. 9:1534. doi: 10.3389/fimmu.2018.01534
Clayton, A., Mitchell, J. P., Court, J., Linnane, S., Mason, M. D., and Tabi, Z. (2008). Human tumor-derived exosomes down-modulate NKG2D expression. J. Immunol. 180, 7249–7258. doi: 10.4049/jimmunol.180.11.7249
Crange, C., Tapparo, M., Tritta, S., Deregibus, M. C., Battaglia, A., Gontero, P., et al. (2015). Role of HLA-G and extracellular vesicles in renal cancer stem cell-induced inhibition of dendritic cell differentiation. CMC Cancer 15:1009.
Cullinam, E. B., Abbondanzo, S. J., Anderson, P. S., Pollard, J. W., Lessey, B. A., and Stewart, C. L. (1996). Leukemia inhibitory factor (LIF) and LIF receptor expression in human endometrium suggests a potential autocrine/paracrine function in regulating embryo implantation. Proc. Natl. Acad. Sci. U.S.A. 93, 3115–3120. doi: 10.1073/pnas.93.7.3115
da Silva Nardi, F., Konig, L., Wagner, B., Giebel, B., Santos Manvailer, L. F., and Rebmann, V. (2016). Soluble monomers, dimers and HLA-G-expressing extracellular vesicles: the three dimensions of structural complexity to use HLA-G as a clinical biomarker. HLAG Immune Response Genet. 88, 77–86. doi: 10.1111/tan.12844
Dilsiz, N. (2020). Role of exosomes and exosomal microRNAs in cancer. Future Sci. OA 6:FSO465. doi: 10.2144/fsoa-2019-0116
Fabbri, M., Paone, A., Calore, F., Galli, R., Gaudio, E., Santhanam, R., et al. (2012). MicroRNAs bind to Toll-like receptors to induce prometastatic inflammatory response. Proc. Natl. Acad. Sci. U.S.A. 109, E2110–E2116.
Festuccia, N., Osorno, R., Wilson, V., and Chambers, I. (2013). The role of pluripotency gene regulatory network components in mediating transitions between pluripotent cell states. Curr. Opin. Genet. Dev. 23, 504–511. doi: 10.1016/j.gde.2013.06.003
Fong, S., Itahana, Y., Sumida, T., Singh, J., Coppe, J. P., Liu, Y., et al. (2003). Id-1 as a molecular target in therapy for breast cancer cell invasion and metastasis. Proc. Natl. Acad. Sci. U.S.A. 100, 13543–13548. doi: 10.1073/pnas.2230238100
Fukuda, K., Sugihara, E., Ohta, S., Izuhara, K., Funakoshi, T., Amagai, M., et al. (2015). Periostin is a key niche component for wound metastasis of melanoma. PLoS One 10:e0129704. doi: 10.1371/journal.pone.0129704
Fuzzi, B., Rizzo, R., Criscuoli, L., Noci, I., Melchiorri, L., Scarselli, B., et al. (2002). HLA-G expression in early embryos is a fundamental prerequisite for the obtainment of pregnancy. Eur. J. Immunol. 32, 311–315. doi: 10.1002/1521-4141(200202)32:2<311::aid-immu311>3.0.co;2-8
Garzetti, L., Menon, R., Finardi, A., Bergami, A., Sica, A., Martino, G., et al. (2014). Activated macrophages release microvesicles containing polarized M1 or M2 mRNAs. J. Leukoc. Biol. 95, 817–825. doi: 10.1189/jlb.0913485
Gibbs, C. P. (2009). Cancer Stem Cells in Sarcoma. V6N4 ESUN Editorial. Ossining, NY: Liddy Shriver sarcoma initiative.
Grange, C., Tapparo, M., Collino, F., Vitillo, L., Damasco, C., Deregibus, M. C., et al. (2011). Microvesicles Released from Human Renal Cancer Stem Cells Stimulate Angiogenesis and Formation of Lung Premetastatic Niche. Cancer Res. 71, 5346–5356. doi: 10.1158/0008-5472.can-11-0241
Gregori, S., Amodio, G., Quattrone, F., and Panina-Bordignon, P. (2015). HLA-G orchestrates the early interaction of human trophoblasts with the maternal niche. Front. Immunol. 30:128. doi: 10.3389/fimmu.2015.00128
Grum-Schwensen, B., Klingelhofer, J., Berg, C. H., El-Naaman, C., Grigorian, M., Lukanidin, E., et al. (2005). Suppression of tumor development and metastasis formation in mice lacking the S100A4(mts1) gene. Cancer Res. 65, 3772–3780. doi: 10.1158/0008-5472.can-04-4510
Guo, H., Cheng, Y., Martinka, M., and McElwee, K. (2015). High LIFr expression stimulates melanoma cell migration and is associated with unfavorable prognosis in melanoma. Pncotarget 6, 25484–25498. doi: 10.18632/oncotarget.4688
Gupta, G. P., Perk, J., Acharyya, S., de Candia, P., Vivek, Mittal V, Todorova-Manova, K., et al. (2007). ID genes mediate tumor reinitiation during breast cancer lung metastasis. Proc. Natl. Acad. Sci. U.S.A. 194, 19506–19511. doi: 10.1073/pnas.0709185104
Hamilton, G., and Rath, B. (2019). Role of circulating tumor cell spheroids in drug resistance. Cancer Drug Resist. 2, 762–772. doi: 10.20517/cdr.2019.47
Han, L., Lam, E. W.-F., and Sun, Y. (2019). Extracellular vesicles in the tumor micro-environment: old stories, but new tales. Mol. Cancer 77:59.
Heap, C. J., Wang, Y., Pinheiro, T. J., Reading, S. A., Jennings, K. R., and Dimmock, N. J. (2005). Analysis of a 17-aminoacid residue, virus- neutralizing microantibody. J. Gen. Virol. 86(Pt 6), 1791–1800. doi: 10.1099/vir.08o812-0
Hong, B. S., Cho, J. H., Kim, H., Choi, E. J., Rho, S., Kim, J., et al. (2009). Colorectal cancer cell-derived microvesicles are enriched in cell cycle-related mRNAs that promote proliferation of endothelial cells. BMC Genomics 10:556. doi: 10.1186/1471-2164-10-556
Hood, J. L., Pan, H., Lanza, G. M., Wickline, S. A., and Consortium for Translational Research in Advanced Imaging and Nanomedicine (C-TRAIN) (2009). Paracrine induction of endothelium by tumor exosomes. Lab. Invest. 89, 1317–1328. doi: 10.1O38/labinvest.20O9.94
Hoshino, A., Costa-Silva, B., Shen, T. L., Rodrigues, G., Hashimoto, A., Tesic Mark, M., et al. (2015). Tumour exosome integrins determine organotropic metastasis. Nature 527, 329–335. doi: 10.1038/nature15756
Hunt, J. S., Petroff, M. G., McIntire, R. H., and Ober, C. (2005). HLA-G and immune tolerance in pregnancy. JASEBJ 19, 681–693. doi: 10.1096/fj.04-2078rev
Jin, L., Hope, K. J., Zhai, Q., Smadja-Joffe, F., and Dick, J. E. (2006). Targeting of CD44 eradicates human acute myeloid leukemic stem cells. Nat. Med. 12, 1167–1174. doi: 10.1038/nm1483
Johnson, S., Chen, H., and Lo, P. K. (2013). In vitro tumor-sphere formation assays. Bio Protocol 3:e325. doi: 10.21769/Bio-Protoc.3
Jurj, A., Zanoaga, O., Braicu, C., Lazar, V., Tomuleasa, C., Irimie, A., et al. (2020). A comprehensive picture of extracellular vesicles and their contents. Molecular transfer to cancer cells. Cancer 12:298. doi: 10.3390/cancers12020298
Kamran, M. Z., Patil, P., and Gude, R. P. (2013). Role of STAT3 in cancer metastasis and advances. Biomed. Res. Int. 2013:421821. doi: 10.1155/2013/421821
Keklikoglou, I., Cianciaruso, C., Guc, E., Squadrito, M. L., Spring, L. M., Tazzyman, S., et al. (2019). Chemotherapy elicits pro-metastatic extracellular vesicles in breast cancer models. Bat. Cell Biol. 21, 190–202. doi: 10.1038/s41556-018-0256-3
Kellokumpu-Lehtinen, P., Talpaz, M., Harris, D., Van, Q., Kurzrock, R., and Estrow, Z. (1996). Leukemia inhibitory factor stimulates breast, kidney and prostate cancer cell proliferation by paracrine and autocrine pathways. Int. J. Cancer 66, 515–519. doi: 10.1002/(sici)1097-0215(19960516)66:4<515::aid-ijc15>3.0.co;2-6
Kim, H. K., Song, K. S., Park, Y. S., Kang, Y. H., Lee, Y. J., Lee, K. R., et al. (2003). Elevated levels of circulating platelet microparticles, VEGF, IL-6 and RANTES in patients with gastric cancer: possible role of a metastasis predictor. Eur. J. Cancer 2003, 184–191. doi: 10.1016/s0959-8049(02)00596-8
Kim, T. H., Kim, H. I., Soung, Y. H., Shaw, L. A., and Chung, J. (2009). Integrin (alpha6beta4) signals through Src to increase expression of S100A4, a metastasis-promoting factor: implications for cancer cell invasion. Mol. Cancer Res. 7, 1605–1612. doi: 10.1158/1541-7786.mcr-09-0102
Kosaka, N. (2016). Decoding the secret of cancer by means of extracellular vesicles. J. Clin. Med. 5:E22. doi: 10.3390/jcm5020022
Krishnamachary, B., Penet, M. F., Nimmagadda, S., Mironchik, Y., Raman, V., Solaiyappan, M., et al. (2012). Hypoxia regulates CD44 and its variant isoforms through HIF-1a in triple negative breast cancer. PLoS One 7:e44078. doi: 10.1371/journal.pone.0044078
Kucharzewska, P., Christianson, H. C., Welch, J. E., Svensson, K. J., Fredlund, E., Ringner, M., et al. (2013). Exosomes reflect the hypoxic status of glioma cells and mediate hypoxia dependent activation of vascular cells during tumor development. Proc. Natl. Acad. Sci. U.S.A. 110, 7312–7317. doi: 10.1073/pnas.1220998110
Li, K., Chen, Y., Li, A., Tan, C., and Liu, X. (2019). Exosomes play roles in sequential processes of tumor metastasis. Int. J. Cancer 144, 1486–1495. doi: 10.1002/ijc.31774
Lin, A., and Yan, W. H. (2015). Human leukocyte antigen-G (HLA-G) expression in cancers: roles in immune evasion, metastasis and target for therapy. Mol. Med. 21, 782–791. doi: 10.2119/molmed.2015.00083
Lin, A., and Yan, W. H. (2019). Intercellular transfer of HLA-G: its potential in cancer immunology. Clin. Transl. Immunol. 8:e1077.
Lindman, A., Dowden, A., and Zavazava, N. (2006). Soluble HLA-G molecules induce apoptosis in natural killer cells. Am. J. Reprod. Immunol. 56, 68–76. doi: 10.1111/j.1600-0897.2006.00395.x
Ling, M. T., Wang, X., Zhang, X., and Wong, Y. C. (2006). The multiple roles of Id-1 in cancer progression. Differentiation 74, 481–487. doi: 10.1111/j.1432-0436.2006.00083.x
Liu, S., Cong, Y., Wang, D., Sun, Y., Deng, L., Liu, Y., et al. (2014). Breast cancer stem cells transition between epithelial and mesenchymal states reflective of their normal counterparts. Stem Cell Rep. 2:7891. doi: 10.1016/j.stemcr.2013.11.009
Lu, H., Xie, Y., Tran, L., Lan, J., Yang, Y., Murugan, N. L., et al. (2020). Chemotherapy-induced S100A10 recruits KDM6A to facilitate OCT4-mediated breast cancer stemness. J. Clin. Invest. 130, 4607–4623. doi: 10.1172/JCI138577
Luft, J. C., and Dix, D. J. (1999). HSP70 expression and function during embryogenesis. Cell Stress Chaperones 4, 162–170. doi: 10.1379/1466-1268(1999)004<0162:heafde>2.3.co;2
Lukanidin, E., and Sleeman, J. P. (2012). Building the niche: the role of the S100 proteins in metastatic growth. Semin. Cancer Biol. 22, 216–225. doi: 10.1016/j.semcancer.2012.02.006
Lyden, D., Young, A. Z., Zagzag, D., Yan, W., Gerald, W., O’Reilly, R., et al. (1999). Id1and Id3 are required for neurogenesis, angiogenesis and vascularization of tumor xenografts. Nature 401, 670–677. doi: 10.1038/44334
Ma, Y., Zhang, P., Wang, F., Yang, J., Yang, Z., and Qin, H. (2010). The relationship between early embryo development and tumourigenesis. J. Cell. Mol. Med. 14, 2697–2701. doi: 10.1111/j.1582-4934.2010.01191.x
Manzo, G. (2019). Similarities between embryo development and cancer process suggest new strategies for research and therapy of tumors: a new point of view. Front. Cell Dev. Biol. 7:20. doi: 10.3389/fcell.2019.00020
Manzo, G. (2020). Defined mathematical relationships among cancer cells suggest modular growth in tumor progression and highlight developmental features consistent with a para-embryonic nature of cancer. Front. Cell Dev. Biol. 8:804. doi: 10.3389/fcell.2020.00804
Mao, L., Li, J., Chen, W. X., Cai, Y. Q., Yu, D. D., Zhong, S. L., et al. (2016). Exosomes decrease sensitivity of breast cancer cells to adriamycin by delivering microRNAs. Tumor Biol. 37, 5247–5256. doi: 10.1007/s13277-015-4402-2
Marjanovic, N. D., Weinberg, R. A., and Chaffer, C. L. (2013). Cell plasticity and heterogeneity in cancer. Clin. Chem. 59, 168–179. doi: 10.1373/clinchem.2012.184655
Marleau, A. M., Chen, C.-S., Joyce, J. A., and Tullis, R. H. (2012). Exosome removal as a therapeutic adjuvant in cancer. J. Transl. Med. 10:134. doi: 10.1186/1479-5876-10-134
Mege, D., Panicot-Dubois, L., Ouaissi, M., Robert, S., Sielezneff, I., Sastre, B., et al. (2016). The origin and concentration of circulating microparticles differ according to cancer type and evolution: a prospective single-center study. Int. J. Cancer 138, 939–948. doi: 10.1002/ijc.29837
Mern, D. S., Hasskarl, J., and Burwinkel, B. (2010a). Inhibition of Id proteins by a peptide aptamer induces cell-cycle arrest and apoptosis in ovarian cancer cells. Br. J. Cancer 103, 1237–1244. doi: 10.1038/sj.bjc.6605897
Mern, D. S., Hoppe-Seyler, K., Hoppe-Seyler, F., Hasskarl, J., and Burwinkel, B. (2010b). Targeting Id1 and Id3 by a specific peptide aptamer induces E-box promoter activity, cell cycle arrest, and apoptosis in breast cancer cells. Breast Cancer Res. Treat. 124, 623–633. doi: 10.1007/s10549-010-0810-6
Milane, L., Singh, A., Mattheolabakis, G., Suresh, M., and Amiji, M. M. (2015). Exosome mediated communication within the tumor microenvironment. J. Control. Release 219, 278–294. doi: 10.1016/j.jconrel.2015.06.029
Murase, R., Sumida, T., Kawamura, R., Onishi-Ishikawa, A., Hamakawa, H., McAllister, S. D., et al. (2016). Suppression of invasion and metastasis in aggressive salivary cancer cells through targeted inhibition of ID1 gene expression. Cancer Lett. 377, 11–16. doi: 10.1016/j.canlet.2016.04.021
Nair, R., Teo, W. S., Mittal, V., and Swarbrik, A. (2014). ID proteins regulate diverse aspects of cancer progression and provide novel therapeutic opportunities. Mol. Ther. 22, 1407–1415. doi: 10.1038/mt.2014.83
Naito, Y., Yoshioka, Y., Yamamoto, Y., and Ochiya, T. (2017). How cancer cells dictate their microenvironment: present roles of extracellular vesicles. Cell Mol. Life Sci. 74, 697–713. doi: 10.1007/s00018-016-2346-3
Niola, F., Zhao, X., Singh, D., Castano, A., Sullivan, R., Lauria, M., et al. (2012). Id proteins synchronize stemness and anchorage to the niche of neural stem cells. Nat. Cell Biol. 14, 477–487. doi: 10.1038/ncb2490
O’Brien, C. A., Kreso, A., Ryan, P., Hermans, K. G., Gibson, L., Wang, Y., et al. (2012). ID1 and ID3 regulate the self-renewal capacity of human colon cancer-initiating cells through p21. Cancer Cell 21, 777–792. doi: 10.1016/j.ccr.2012.04.036
Ohno, S., Takanashi, M., Sudo, K., Ueda, S., Ishikawa, A., Matsuyama, N., et al. (2013). Systemically injected exosomes targeted to EGFR deliver antitumor microRNA to breast cancer cells. Mol. Ther. 21, 185–191. doi: 10.1038/mt.2012.180
Orian-Rousseau, V., and Ponta, H. (2015). Perspectives of CD44 targeting therapies. Arch. Toxicol. 89, 3–14. doi: 10.1007/s00204-014-1424-2
Oskarsson, T., Acharyya, S., Zhang, X. F., Vanharanta, S., Tavazoie, S. F., Morris, P. G., et al. (2011). Breast cancer cells produce tenascin C as a metastatic niche component to colonize the lungs. Nat. Med. 17, 867–874. doi: 10.1038/nm.2379
Ozawa, P. M. M., Alkhilaiwi, F., Cavalli, I. J., Malheiros, D., de Souza Fonseca Ribeiro, E. M., and Cavalli, L. R. (2018). Extracellular vesicles from triple-negative breast cancer cells promote proliferation and drug resistance in non-tumorigenic breast cells. Breast Cancer Res. Treat. 2018, 713–723. doi: 10.1007/s10549-018-4925-5
Park, J. I., Strock, C. J., Ball, D. W., and Nelkin, B. D. (2003). The Ras/Raf/MEK/extracellular signal-regulated kinase pathway induces autocrine-paracrine growth inhibition via the leukemia inhibitory factor/JAK/STAT pathway. Mol. Cell Biol. 23, 543–554. doi: 10.1128/mcb.23.2.543-554.2003
Pascucci, L., Cocce, V., Bonomi, A., Ami, D., Ceccarelli, P., Ciusani, E., et al. (2014). Paclitaxel is incorporated by mesenchymal stromal cells and released in exosomes that inhibit in vitro tumor growth: a new approach for drug delivery. J. Control. Release 192, 262–270. doi: 10.1016/j.jconrel.2014.07.042
Pavlides, S., Whitaker-Menezes, D., Castello-Cros, R., Flomenberg, N., Witkiewicz, A. K., Frank, P. G., et al. (2009). The reverse Warburg effect: aerobic glycolysis in cancer associated fibroblasts and the tumor stroma. Cell Cycle 8, 3984–4001. doi: 10.4161/cc.8.23.10238
Pecak, A., Skalniak, L., Pels, K., Ksiazek, M., Madej, M., Krzemien, D., et al. (2020). Anti-CD44 DNA aptamers selectively target cancer cells. Nucleic Acid Ther. 30:833. doi: 10.1089/nat.2019.0833
Pham, P. V., Phan, N. L., Nguyen, N. T., Truong, N. H., Duong, T. T., Le, D. V., et al. (2011). Differentiation of breast cancer stem cells by knockdown of CD44: promising differentiation therapy. Transl. Med. 9:209. doi: 10.1186/1479-5876-9-209
Pillay, P., Moodley, K., Vatish, M., and Moodley, J. (2020). Exosome miRNAs in pregnancy provide insight into a possible cure for cancer. Int. J. Mol. Sci. 21:5384. doi: 10.3390/ijms21155384
Qin, X., Yu, S., Zhou, L., Shi, M., Hu, Y., Xu, X., et al. (2017). Cisplatin-resistant lung cancer cell-derived exosomes increase cisplatin resistance of recipient cells in exosomal miR-100-5p-dependent manner. Int. J. Nanomed. 12, 3721–3733. doi: 10.2147/ijn.s131516
Rana, S., Malinowska, K., and Zoller, M. (2013). Exosomal tumor microRNA modulates premetastatic organ cells. Neoplasia 15, 281–295. doi: 10.1593/neo122010
Ratajczak, J., Miekus, K., Kucia, M., Zhang, J., Reca, R., Dvorak, P., et al. (2006). Embryonic stem cell-derived microvesicles reprogram hematopoietic progenitors: evidence for horizontal transfer of mRNA and protein delivery. Leukemia 20, 847–856. doi: 10.1038/sj.leu.2404132
Rebmann, V., Konig, L., Nardi Fda, S., Wagner, B., Manvailer, L. F., and Horn, P. A. (2016). The potential of HLA-G-bearing extracellular vesicles as a future element in HLA-G immune biology. Front. Immunol. 4:173. doi: 10.3389/fimmu.2016.00173
Rebmann, V., Regel, J., Stolke, D., and Grosse-Wilde, H. (2003). Secretion of sHLA-G molecules in malignancies. Semin. Cancer Biol. 13, 371–377. doi: 10.1016/S1044-579X(03)00028-2
Reya, T., Morrison, S. J., Clarke, M. F., and Weissman, I. L. (2001). Stem cells, cancer and cancer stem cells. Nature 414, 105–111.
Richards, K. E., Zeleniak, A. E., Fishel, M. L., Wu, J., Littlepage, L. E., and Hill, R. (2017). Cancer-associated fibroblast exosomes regulate survival and proliferation of pancreatic cancer cells. Oncogene 36, 1770–1778. doi: 10.1038/onc.2016.353
Rouas-Freiss, N., Moreau, P., Menier, C., and Carosella, E. D. (2003). HLA-G in cancer: a way to turn off the immune system. Semin. Cancer Biol. 13, 325–336. doi: 10.1016/s1044-579x(03)00023-3
Rouas-Freiss, N., Moreau, P., Menier, C., LeMaoult, J., and Carosella, E. D. (2007). Expression of tolerogenic HLA-G molecules in cancer prevents antitumor responses. Semin. Cancer Biol. 17, 413–421. doi: 10.1016/j.semcancer.2007.07.003
Roy, A., Sarker, S., Upadhyay, Pal, A., Adhikary, A., Ray, M., et al. (2018). Methylglyoxal at metronomic doses sensitizes breast cancer cells to doxorubicin and cisplatin causing synergistic induction of programmed cell death and inhibition of stemness. Biochem. Pharmacol. 156, 322–339. doi: 10.1016/j.bcp.2018.08.041
Safaei, R., Larson, B. J., Cheng, T. C., Gibson, M. A., Otani, S., Naerdemann, W., et al. (2005). Abnormal lysosomal trafficking and enhanced exosomal export of cisplatin in drug-resistant human ovarian carcinoma cells. Mol. Cancer Ther. 4, 1595–1604. doi: 10.1158/1535-7163.mct-05-0102
Samali, A., and Cotter, T. G. (1996). Heat shock proteins increase resistance to apoptosis. Exp. Cell Res. 223, 163–170. doi: 10.1006/excr.1996.0070
Sherwin, J. R. A., Smith, S. K., Wilson, A., and Sharkey, A. M. (2002). Soluble gp130 is up-regulated in the implantation window and shows altered secretion in patients with primary unexplained infertility. J. Clin. Endocrinol. Metab. 87, 3953–3960. doi: 10.1210/jcem.87.8.8766
Sheu, J., and Shih, I. E. (2010). HLA-G and immune evasion in cancer cells. Med. Assoc. 109, 248–257. doi: 10.1016/S0929-6646(10)60050-2
Shimbo, K., Miyaki, S., Ishitobi, H., Kato, Y., Kubo, T., Shimose, S., et al. (2014). Exosome-formed synthetic microRNA-143 is transferred to osteosarcoma cells and inhibits their migration. Biochem. Biophys. Res. Commun. 445, 381–387. doi: 10.1016/j.bbrc.2014.02.007
Shu, C. W., and Huang, C. M. (2008). HSP70s: from tumor transformation to cancer therapy. Clin. Med. Oncol. 2, 335–345.
Singh, A. K., Arya, R. K., Maheshwari, S., Singh, A., Meena, S., Pandey, P., et al. (2015). Tumor heterogeneity and cancer stem cell paradigm: updates in concept, controversies and clinical relevance. Int. J. Cancer 136, 1991–2000. doi: 10.1002/ijc.28804
Srivastava, A., Amreddy, N., Babu, A., Panneerselvam, J., Mehta, M., Muralidharan, R., et al. (2016). Nanosomes carrying doxorubicin exhibit potent anticancer activity against human lung cancer cells. Sci. Rep. 6:38541.
Stangl, S., Gehrmann, M., Riegger, J., Kuhs, K., Riederer, I., Sievert, W., et al. (2011). Targeting membrane heat-shock protein 70 (HSP70) on tumors by cmHSP70.1 antibody. PNAS 108, 733–738.
Su, Y. J., Lai, H. M., Chang, Y. W., Chen, J. Y., and Lee, J. L. (2011). Direct reprogramming of stem cell properties in colon cancer cells by CD44. EMBO J. 30, 3186–3199. doi: 10.1038/emboj.2011.211
Sung, B. H., Ketova, T., Hoshino, D., Zijlstra, A., and Weaver, A. M. (2015). Directional cell movement through tissues is controlled by exosome secretion. Nat. Commun. 6:7164.
Teo, W. S., Holliday, H., Karthikeyan, N., Cazet, A. S., Roden, D. L., Harvey, K., et al. (2020). ID proteins promote a CSC phenotype in mouse model of triple negative breast cancer via negative regulation of Robo1. Front. Cell Dev. Biol. 8:552. doi: 10.3389/fcell.2020.00552
Thiery, J. P., Acloque, H., Huang, R. J. Y., and Nieto, M. A. (2009). Epithelial-mesenchymal transitions in development and disease. Cell 139, 871–890. doi: 10.1016/j.cell.2009.11.007
Tilburgs, T., Evans, J. H., Crespo, A. C., and Strominger, J. L. (2015). The HLA-G cycle provides for both NK tolerance and immunity at the maternal-fetal interface. PNAS 112, 13312–13317. doi: 10.1073/pnas.1517724112
Tsuchiya, T., Okaji, Y., Tsuno, N. H., Sakurai, D., Tsukiya, N., Kawai, K., et al. (2005). Targeting ID1 and ID3 inhibits peritoneal metastasis of gastric cancer. Cancer Sci. 96, 784–790. doi: 10.1111/j.1349-7006.2005.00113.x
Umezu, T., Ohyashiki, K., Kuroda, M., and Ohyashiki, J. H. (2013). Leukemia cell to endothelial cell communication via exosomal miRNAs. Oncogene 32, 2747–2755. doi: 10.1038/onc.2012.295
Urosevic, M., and Dummer, R. (2003). HLA-G and IL-10 expression in human cancer–different stories with the same message. Semin. Cancer Biol. 13, 337–342. doi: 10.1016/s1044-579x(03)00024-5
Urosevic, M., Kurrer, M. O., Kamarashev, J., Mueller, B., Weder, W., Burg, G., et al. (2001). Human leukocyte antigen G up-regulation in lung cancer associates with high-grade histology, human leukocyte antigen class I loss and interleukin-10 production. Am. J. Pathol. 159, 817–824. doi: 10.1016/s0002-9440(10)61756-7
Urosevic, M., Willers, J., Mueller, B., Kempf, W., Burg, G., and Dummer, R. (2002). HLA-G protein up-regulation in primary cutaneous lymphomas is associated with interleukin-10 expression in large cell T-cell lymphomas and indolent B-cell lymphomas. Blood 99, 609–617. doi: 10.1182/blood.v99.2.609
Vader, P., Breakefield, X. O., and Wood, M. J. A. (2014). Extracellular vesicles: emerging targets for cancer therapy. Trends Mol. Med. 20, 385–393. doi: 10.1016/j.molmed.2014.03.002
Valadi, H., Ekstrom, K., Bossios, A., Sjostrand, M., Lee, J. J., and Lotvall, J. O. (2007). Exosome-mediated transfer of mRNAs and microRNAs is a novel mechanism of genetic exchange between cells. Nat. Cell Biol. 9, 654–659. doi: 10.1038/ncb1596
Velagapudi, C., Nilsson, R.-P., Lee, M. J., Burns, H. S., Ricono, M. J., Arar, M., et al. (2012). Reciprocal induction of simple organogenesis by mouse kidney progenitor cells in three-dimensional co-culture. Am. J. Pathol. 180, 819–838. doi: 10.1016/j.ajpath.2011.11.002
Verloes, A., Van de Velde, H., LeMaoult, J., Mateizel, I., Cauffmann, G., Horn, P. A., et al. (2011). HLA-G expression in human embryonic stem cells and preimplantation embryos. J. Immunol. 186, 2663–2671. doi: 10.4049/jimmunol.1001081
Vinnitsky, V. (2014). The development of a malignant tumor is due to a desperate asexual self-cloning process in which cancer stem cells develop the ability to mimic the genetic program of germline cells. Intrinsically Disord. Proteins 2:e29997. doi: 10.4161/idp.29997
Wang, J., Zheng, Y., and Zhao, M. (2016). Exosome-based cancer therapy: implication for targeting cancer stem cells. Front. Pharmacol. 7:533. doi: 10.3389/fpharm.2016.00533
Wang, T., Narayanaswamy, R., Ren, H., and Torchilin, V. P. (2016). Combination therapy targeting both cancer stem-like cells and bulk tumor cells for improved efficacy of breast cancer treatment. Cancer Biol. Ther. 17, 698–707. doi: 10.1080/15384047.2016.1190488
Weaver, V. M., Petersen, O. W., Wang, F., Larabell, C. A., Briand, P., Damsky, C., et al. (1997). Reversion of the malignant phenotype of human breast cells in three-dimensional culture and in vivo by integrin blocking antibodies. J. Cell Biol. 137, 231–245. doi: 10.1083/jcb.137.1.2
Wieckowski, E. U., Visus, C., Szainjk, M., Szczepanski, M. J., Storkus, W. J., and Whiteside, T. L. (2009). Tumor-derived microvesicles promote regulatory T cell expansion and induce apoptosis in tumor-reactive activated CD8+ T lymphocytes. J. Immunol. 183, 3720–3730. doi: 10.4049/jimmunol.0900970
Williams, K., Motiani, K., Giridhar, P. V., and Kasper, S. (2013). CD44 integrates signaling in normal stem cell, cancer stem cell and (pre)metastatic niches. Exp. Biol. Med. 13, 238324–238338.
Wong, Y. C., Zhang, X. M., Ling, M. T., and Wang, X. H. (2008). Inactivation of ID-1 gene induces sensitivity of prostate cancer cells to chemotherapeutic drugs. Adv. Exp. Med. Biol. 617, 565–572. doi: 10.1007/978-0-387-69080-3_58
Xu, H., Tian, Y., Yuan, X., Wu, H., Liu, Q., Pestell, R. G., et al. (2015). The role of CD44 in epithelial-mesenchymal transition and cancer development. Onco Targets Ther. 8, 3783–3792. doi: 10.2147/OTTS95470
Yan, W. H., Alin, A. F., Chang, C. C., and Ferrone, S. (2005). Induction of HLA-G expression in a melanoma cell line OCM-1Afollowing the treatment with 5-aza-2’-deoxycytidine. Cell Res. 15, 523–531. doi: 10.1038/sj.cr.7290376
Yan, Y., Zuo, X., and Wei, D. (2015). Concise review: emerging role of CD44 in cancer stem cells: a promising biomarker and therapeutic target. Stem Cells Transl. Med. 4, 1033–1043. doi: 10.5966/sctm.0048
Yang, T., Martin, P., Fogarty, B., Brown, A., Schurman, K., Phipps, R., et al. (2015). Exosome delivered anticancer drugs across the blood-brain barrier for brain cancer therapy in Danio rerio. Pharm. Res. 32, 2003–2014. doi: 10.1007/s11095-014-1593-y
Yang, X., Liang, X., Zheng, M., and Tang, Y. (2018). Cellular phenotype plasticity in cancer dormancy and metastasis. Front. Oncol. 8:505. doi: 10.3389/fonc.2018.00505
Yao, Y. Q., Barlow, D. H., and Sargent, I. L. (2005). Differential expression of alternatively spliced transcripts of HLA-G in human preimplantation embryos and inner cell masses. J. Immunol. 15, 8379–8385. doi: 10.4049/jimmunol.175.12.8379
Yen, B. L., Chang, C. J., Liu, K. J., Chen, Y. C., Hu, H. I., Bai, C. H., et al. (2009). Human embryonic stem cell-derived mesenchymal progenitors possess strong immunosuppressive effects toward natural killer cells as well as T lymphocytes. Stem Cells 27, 451–456. doi: 10.1634/stemcells.2008-0390
Yue, X., Wu, L., and Hu, W. (2015). The regulation of leukemia inhibitory factor. Cancer Cell Microenviron. 2:e877.
Zaiden, M., Feinshtein, V., and David, A. (2017). Inhibition of CD44v3 and CD44v6 function blocks tumor invasion and metastatic colonization. J. Control. Release 257, 10–20. doi: 10.1016/j.jconrel.2017.01.021
Zeilstra, J., Joosten, S. P., van Andel, H., Tolg, C., Berns, A., Snoek, M., et al. (2014). Stem cell CD44v isoforms promote intestinal cancer formation in Apc (min) mice downstream of WNT signaling. Oncogene 33, 665–670. doi: 10.1038/onc.2012.611
Keywords: cancer stem cell, molecular checkpoints, extracellular vesicles, embryo, therapy
Citation: Manzo G (2021) Specific and Aspecific Molecular Checkpoints as Potential Targets for Dismantling Tumor Hierarchy and Preventing Relapse and Metastasis Through Shielded Cytolytic Treatments. Front. Cell Dev. Biol. 9:665321. doi: 10.3389/fcell.2021.665321
Received: 08 February 2021; Accepted: 17 May 2021;
Published: 06 July 2021.
Edited by:
Giuseppe Palma, Istituto Nazionale Tumori Fondazione G. Pascale (IRCCS), ItalyReviewed by:
Benedetta Bussolati, University of Turin, ItalyFederica Collino, University of Padua, Italy
Copyright © 2021 Manzo. This is an open-access article distributed under the terms of the Creative Commons Attribution License (CC BY). The use, distribution or reproduction in other forums is permitted, provided the original author(s) and the copyright owner(s) are credited and that the original publication in this journal is cited, in accordance with accepted academic practice. No use, distribution or reproduction is permitted which does not comply with these terms.
*Correspondence: Giovanni Manzo, Z2lvdmFubmltYW56b2J0ckBnbWFpbC5jb20=
†Retired