- 1ETH Zürich, Zurich, Switzerland
- 2Human Technopole, Milan, Italy
The neocortex is the largest part of the cerebral cortex and a key structure involved in human behavior and cognition. Comparison of neocortex development across mammals reveals that the proliferative capacity of neural stem and progenitor cells and the length of the neurogenic period are essential for regulating neocortex size and complexity, which in turn are thought to be instrumental for the increased cognitive abilities in humans. The domesticated ferret, Mustela putorius furo, is an important animal model in neurodevelopment for its complex postnatal cortical folding, its long period of forebrain development and its accessibility to genetic manipulation in vivo. Here, we discuss the molecular, cellular, and histological features that make this small gyrencephalic carnivore a suitable animal model to study the physiological and pathological mechanisms for the development of an expanded neocortex. We particularly focus on the mechanisms of neural stem cell proliferation, neuronal differentiation, cortical folding, visual system development, and neurodevelopmental pathologies. We further discuss the technological advances that have enabled the genetic manipulation of the ferret in vivo. Finally, we compare the features of neocortex development in the ferret with those of other model organisms.
Introduction
The ferret (Mustela putorius furo) is a small carnivore, domesticated more than 2000 years ago (Davison et al., 1999). There are three principal reasons that have allowed ferret to become a major model organism in developmental neurobiology. First, the ferret is characterized by an expanded and folded neocortex and a diversity of proliferative neural stem and progenitor cells (Kawasaki, 2014; Fernandez et al., 2016; Kalebic et al., 2019). These features are generally accepted to be fundamental for the evolutionary expansion of the neocortex, which in turn is considered to underlie the increased cognitive abilities of humans (Rakic, 2009; Dehay et al., 2015; Fernandez et al., 2016; Sousa et al., 2017; Kalebic and Huttner, 2020). Hence the ferret is used as an animal model to study the evolutionary expansion of the neocortex and those aspects of human brain development that cannot be modeled in organisms with a small brain, such as rodents.
Second, the ferret is born with an immature brain and many of its neurodevelopmental processes, such as cortical folding (gyrification), protract into the first weeks of postnatal life (Barnette et al., 2009; Sawada and Watanabe, 2012). From a practical side, this makes the ferret a more amenable animal model for the study of such processes, than those organisms in which the same processes happen in utero, notably primates. Moreover, as its eyes open only after postnatal day (P) 30, ferret is a suitable model system for studying the early development of the visual system and the role of sensory experience therein (Roy et al., 2018).
Third, the ferret is an established model organism for various human pathological conditions, as it shows similarities not only with the human neurodevelopment, but also with the human immune response (Enkirch and von Messling, 2015). Thus, it has been used to model respiratory and neurological infections, along with neurodevelopmental malformations and brain injury. Finally, ferrets very closely mimic the infection and transmission of SARS-CoV-2, suggesting they could be used as animal models for both the respiratory and neurological aspects of COVID-19 (Kim et al., 2020; Richard et al., 2020; Shi et al., 2020).
The ferret has been used in research for more than 100 years (Yeates, 1911) and it exhibits several features that make it a convenient laboratory animal. These include a large average litter size of eight kits and a fairly short gestation period of 40–42 days (Lindeberg, 2008). Importantly, the ferret is a genetically accessible model system. Its genome has been sequenced (Peng et al., 2014) and several different methods that enable acute genetic manipulation in the embryonic and postnatal brain are available (Borrell, 2010; Kawasaki et al., 2012). Finally, ferret transgenesis has recently been established (Johnson et al., 2018; Yu et al., 2019). In this review we discuss those features of the ferret neurodevelopment that make this animal an important model system for the study of cell biology of neural progenitors, neuronal differentiation and migration, mechanisms underlying gyrification, development of the sensory systems and human pathologies pertinent to the central nervous system.
Neocortex Development
The mammalian neocortex develops from the dorsolateral part of the telencephalon at the rostral-most part of the neural tube (Rakic and Lombroso, 1998; Taverna et al., 2014). At very early stage of neocortical development, the neuroepithelial cells (NECs) surround the central canal and the cerebral ventricles (Taverna et al., 2014). Once cortical neurogenesis starts, NECs give rise to apical radial glia (aRG), the main type of apical progenitors (Taverna et al., 2014; Figure 1). aRG reside in a dense and highly packed pseudostratified germinal layer, the ventricular zone (VZ) (Figure 1). The asymmetric proliferative division of aRG gives rise to the second major class of progenitor cells, basal progenitors (BPs), that will migrate basally to form the subventricular zone (SVZ) (Taverna et al., 2014; Figure 1). In species with an expanded and folded neocortex, such as ferret and human, the SVZ is further divided into inner SVZ (iSVZ) and outer SVZ (oSVZ), with the latter being particularly rich in highly proliferative BPs (Smart et al., 2002; Dehay et al., 2015; Fernandez et al., 2016; Figure 1). Further basally to the SVZ are the transient intermediate zone (IZ), that will form the white matter, the transient subplate (SP) and the cortical plate (CP) (Figure 1), which will form the six-layered neocortical gray matter, a hallmark of the adult mammalian neocortex.
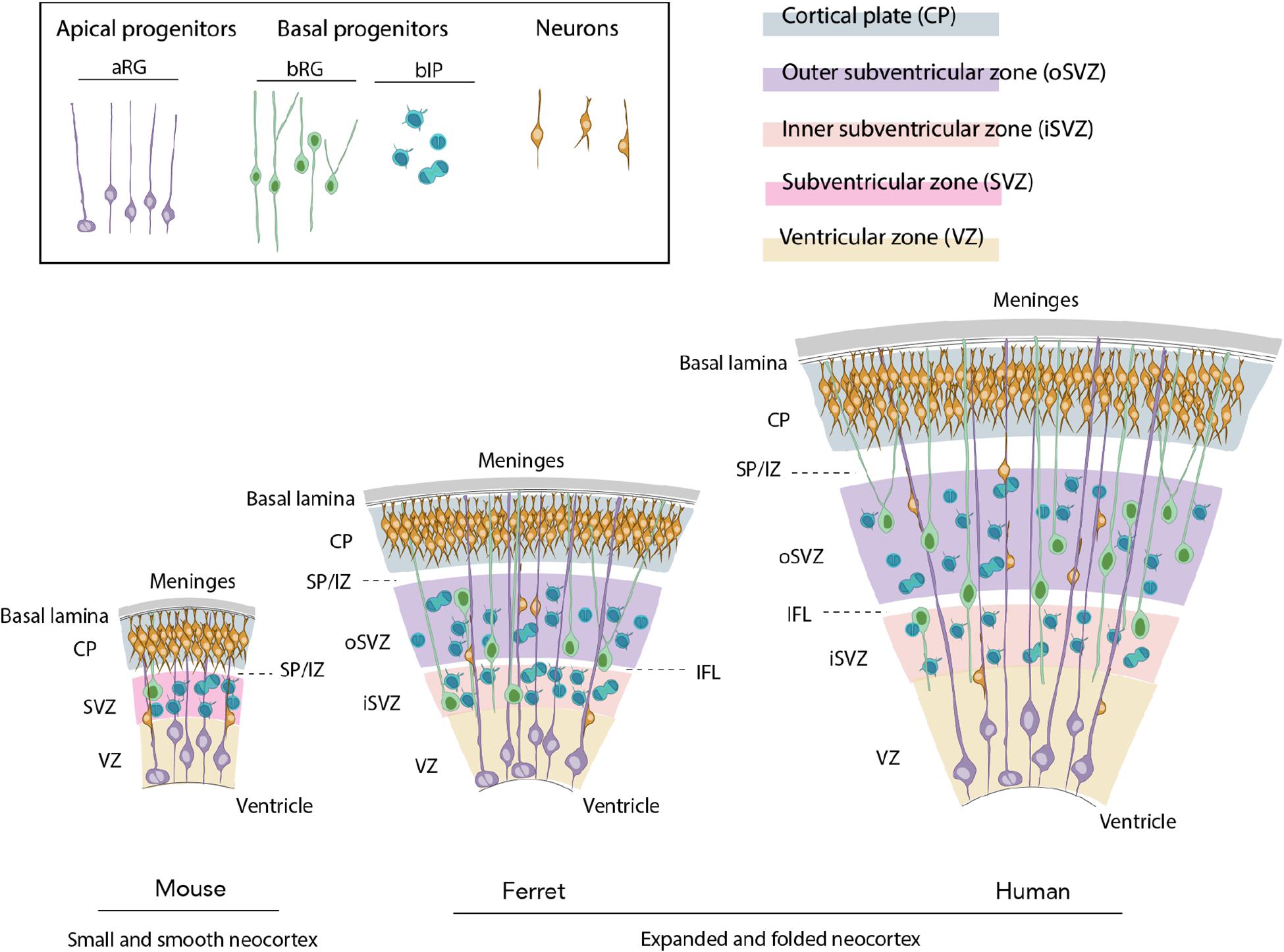
Figure 1. Evolutionary expansion of the neocortex. Ferret and human develop an expanded and folded neocortex, whereas the mouse is characterized by a small and smooth neocortex. The key features of the former are a high abundance of basal progenitors (BP) and particularly basal radial glia (bRG), their morphological heterogeneity, distinction of iSVZ and oSVZ, existence of the inner fiber layer (IFL) and a tangential migration of projection neurons through the germinal zones, intermediate zone (IZ), and subplate (SP) to their final position in the CP.
Newborn projection neurons migrate from the site of their origin in the germinal zones to their final destination in the CP using the radial processes of radial glia (Evsyukova et al., 2013; Buchsbaum and Cappello, 2019; Silva et al., 2019). The mammalian CP is built in an inside-out fashion with the newborn neurons migrating past those already present in the CP to a more superficial (basal) position. Therefore, neocortical layers VI and V, also known as deep layers, are generated first, whereas the layers IV, III, and II, called upper layers, are generated later. The notable exception is the layer I which is situated under the pia and forms first by the Cajal-Retzius pioneer cells (Angevine and Sidman, 1961; Rakic, 1972; Bayer and Altman, 1991; Yamazaki et al., 2004; Molnar et al., 2006; Molyneaux et al., 2007; Franco and Muller, 2013; Lodato and Arlotta, 2015).
Evolutionary Expansion of the Neocortex
Although the neocortex is present in all mammals, its size has been subjected to evolutionary differences within various mammalian orders (Molnar et al., 2006; Krubitzer, 2007; Rakic, 2009; Kaas, 2013; Kalebic et al., 2017). For example, in mouse the neocortex is small and smooth, whereas in human, macaque and ferret, the neocortex underwent an enlargement. The neocortical expansion was disproportionally greater in the tangential axis, which led to the characteristic cortical folding (gyrification), i.e., formation of gyri and sulci, on the basal side of the tissue (Rakic, 2009; Zilles et al., 2013; Fernandez et al., 2016). Thus, gyrification is thought to come as an evolutionary solution to the outstanding growth of the neocortex and necessity to accommodate additional neurons (Zilles et al., 2013; Borrell, 2018; Kroenke and Bayly, 2018). In addition to the increase in surface area, the neocortical expansion concerns also an increase in thickness (Molnar et al., 2006; Rakic, 2009), which is most pronounced in the neocortical layers II and III (also known as supragranular layers). The thickness of those layers is doubled in primates compared to rodents, with carnivores, such as the ferret, showing intermediate characteristics (Hutsler et al., 2005). Moreover, the thickness of the transient SP compared to the CP thickness is increased in primates versus carnivores and in both primates and carnivores compared to rodents (Kostovic and Rakic, 1990; Molnar et al., 2019; Kostovic, 2020). The ferret SP thickens in regions corresponding to future gyri, whereas it becomes thinner in prospective sulci (Smart and McSherry, 1986a,b). The ferret SP finally disappears when gyri reach their maximum development (Smart and McSherry, 1986a,b). In light of this and the fact that the complexity of SP is increased in species with a folded cortex, it has been suggested that the SP is linked to gyrification (Rana et al., 2019).
An increase in neuronal production is thought to be the key factor underlying the evolutionary expansion of the neocortex. Such increase is a consequence of the increased proliferative capacity of neural progenitor cells and the resulting extension of the neurogenic period (Molnar et al., 2006; Rakic, 2009; Borrell and Reillo, 2012; Dehay et al., 2015; Kalebic et al., 2017; Sousa et al., 2017). Among the neural progenitor cells, BPs are widely considered to be instrumental for the increased neuronal production. In species with a small and smooth neocortex, such as mouse, BPs have a low proliferative capacity, typically dividing only once to give rise to two neurons (Haubensak et al., 2004; Miyata et al., 2004; Noctor et al., 2004; Figure 1). Conversely, they have a higher proliferative capacity and are hence more abundant in species with an expanded and folded neocortex, such as ferret and primates (Fietz et al., 2010; Hansen et al., 2010; Reillo et al., 2011; Betizeau et al., 2013; Kalebic et al., 2019; Figure 1).
Basal progenitors can generally be divided into two subclasses: basal intermediate progenitors (bIPs) and basal radial glia (bRG, also known as outer radial glia or oRG), with the latter being particularly instrumental for the neocortical expansion (Fietz and Huttner, 2011; Lui et al., 2011; Borrell and Reillo, 2012; Figure 1). bRG show a significant difference in their proliferative capacities and morphologies across mammals (Kalebic and Huttner, 2020). In mouse, they are scarce and have a low proliferative capacity, whereas in primates and ferret, bRG are more abundant and have a greater proliferative capacity (Fietz and Huttner, 2011; Lui et al., 2011; Borrell and Reillo, 2012; Kalebic et al., 2017). In terms of morphology, mouse bRG are mainly monopolar and contain a single basal process (Shitamukai et al., 2011; Wang et al., 2011) with only a small fraction of cells exhibiting an apically directed process (Wong et al., 2015; Kalebic et al., 2019; Figure 1). bRG in gyrencephalic species were originally also described as monopolar cells with a single basal process (Fietz et al., 2010; Hansen et al., 2010; Reillo et al., 2011). However, further studies revealed larger heterogeneity with the presence of both bipolar cells and monopolar cells with an apically directed process (Betizeau et al., 2013; Reillo et al., 2017; Kalebic et al., 2019; Figure 1). Importantly, bRG with two basal processes together with a presence or absence of an apically directed process have been reported in human and ferret, but not in mouse developing neocortex (Kalebic et al., 2019; Figure 1). BP morphology has been proposed to be an important underlying factor for the evolutionary expansion of the neocortex (Kalebic and Huttner, 2020) as the progenitors containing more processes are more proliferative and enriched in species with an expanded neocortex (Betizeau et al., 2013; Kalebic et al., 2019).
The prolonged neurogenesis has been suggested to be a consequence of a higher proliferative rate of BPs in animals with an enlarged neocortex. Whereas neurogenesis in mouse lasts ∼9 days, in primates it is 10 times longer and in ferrets it proceeds for ∼5 weeks (Jackson et al., 1989; Noctor et al., 1997; Kornack and Rakic, 1998; Smart et al., 2002; Martinez-Cerdeno et al., 2006). The length of neurogenesis is partially linked to the length of the neural progenitors’ cell cycle, which in mouse is on average 18.5 h (Calegari et al., 2005; Arai et al., 2011), in ferret on average 44 h (Reillo and Borrell, 2012; Turrero Garcia et al., 2015), and in macaque 45 h (Betizeau et al., 2013). A large heterogeneity of ferret neural progenitors is reflected also in the cell cycle duration, with the main difference being the duration of the S-phase (Reillo and Borrell, 2012; Turrero Garcia et al., 2015). The length of the neurogenic period has been proposed to be a particularly important factor of the neocortex expansion within a specific lineage, that is when the neurogenic program is the same (Lewitus et al., 2014). For example, the increase in the length of the neurogenic period has been suggested to be a key factor in the three-fold increase in neocortex size between chimpanzee and human (Lewitus et al., 2014).
Ferret Neocortex Development
Onset and Progression of Neurogenesis
Ferret SP neurons are generated between the embryonic day (E) 20 and E27 (Jackson et al., 1989; Antonini and Shatz, 1990), whereas the neocortical neurogenesis starts approximately at E22 with the production of neurons of layer VI in the parietal lobe (Noctor et al., 1997; Smart et al., 2002; Barnette et al., 2009; Martinez-Cerdeno et al., 2012; Figure 2A). Compared to rodents, neurogenesis in ferrets starts relatively earlier, which is a distinctive trait of all altricial (born in an underdeveloped state) mammals (Workman et al., 2013). As in other mammals, ferret neocortical neurogenesis proceeds in a rostro-lateral to caudal-medial direction due to the transverse neurogenic gradient. For example, by E29 neurons are already populating the CP in areas rostral to the occipital lobe (McSherry and Smart, 1986), whereas neurogenesis in the prospective visual cortex only starts between E30 and E31 (Jackson et al., 1989). The heterochronicity in generation of upper-layer neurons among different cortical areas can be best appreciated by comparing the data on the prospective somatosensory and visual cortices. In the somatosensory area, the production of neurons belonging to layer IV starts at E33 and continues for 3 days, whereas the generation of supragranular neurons covers the last days of gestation, with very few neurons of layer II produced at P1 (Noctor et al., 1997, 1999). The primary visual cortex (A17 or V1) and the visual association area (A18 or V2) have a thicker layer IV, which is reflected by the layer IV neurogenic period lasting 8 days and ending at P1. Moreover, supragranular neurons in these areas are produced almost exclusively postnatally, finishing at P14 (Jackson et al., 1989).
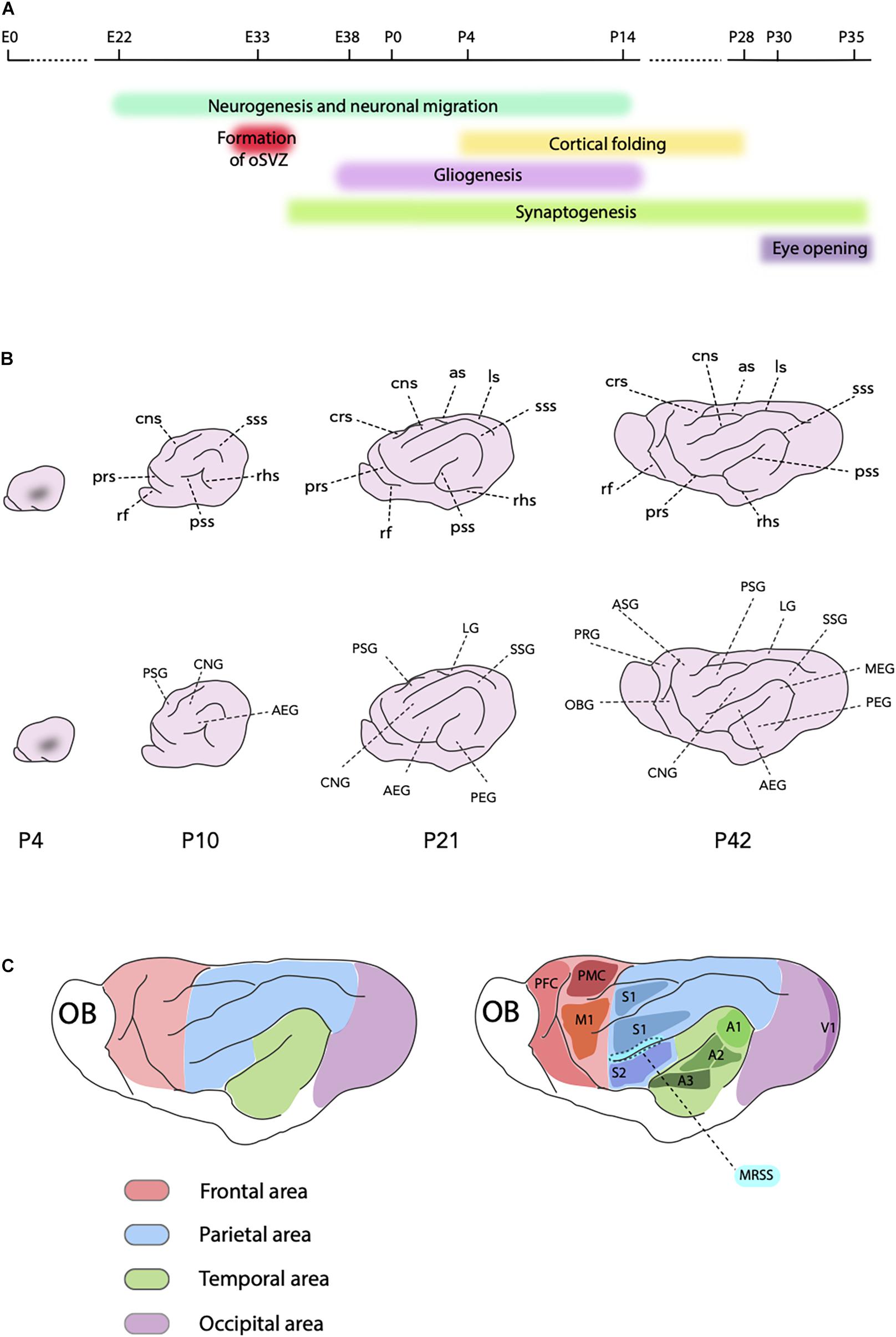
Figure 2. Ferret neocortex development. (A) Schematic timeline showing key processes in the ferret neocortex development and the timing of the eye opening. (B) Scheme of development of the ferret cortical folding from P4, when the first indentation is visible, until P42, the fully mature stage (on all schemes, rostral is left and dorsal is up). Intermediate P10 and P21 stages are also shown. Upper part shows main sulci (in the clockwise order, P42): as, ansinate sulcus; ls, lateral sulcus; sss, suprasylvian sulcus; pss, pseudosylvian sulcus; rhs, rhinal sulcus; prs, presylvian sulcus; rf, rhinal fissure; crs, cruciate sulcus; cns, coronal sulcus. Lower part shows main gyri (in the clockwise order, P42): PSG, posterior sigmoid gyrus; LG, lateral gyrus; SSG, suprasylvian gyrus; MEG, medial ectosylvian gyrus; PEG, posterior ectosylvian gyrus; AEG, anterior ectosylvian gyrus; CNG, coronal gyrus; OBG, orbital gyrus; PRG, proreal gyrus; ASG, anterior sigmoid gyrus. (C) Principal cortical areas in the ferret. Red, frontal area (PFC, prefrontal cortex; PMC, premotor cortex; M1, primary motor cortex); Green, temporal area (A1, primary auditory cortex; A2, secondary auditory cortex; A3, tertiary auditory cortex); Blue, parietal area (S1, primary somatosensory cortex; S2, secondary somatosensory cortex; MRSS, medial rostral suprasylvian area); Violet, occipital area (V1, primary visual cortex or area 17); OB, olfactory bulb.
Neocortex Expansion: oSvz and Bps
During its embryonic development, the ferret contains all the histological and cell biological features required to build an expanded neocortex (Figure 1). First, it has a thick SVZ which splits into the iSVZ and oSVZ at E33 (Reillo and Borrell, 2012; Martinez-Martinez et al., 2016). Second, it has a high abundance of bRG, the progenitor type most prominently implicated in the neocortical expansion (Reillo et al., 2011; Borrell and Reillo, 2012; Kalebic et al., 2019). Third, it contains all the variety of bRG morphotypes that can be found in human (Kalebic et al., 2019). Collectively, these features enable the development of a folded neocortex with expanded supragranular layers. Similarly to human, ferret BPs comprise roughly 50% bIPs and 50% bRG (Fietz et al., 2010; Kalebic et al., 2019). Like in other studied mammals, ferret neurogenic bIPs express the transcription factor Tbr2 and lack processes during mitosis, whereas ferret proliferative bRG are characterized by the expression of Sox2 and Pax6, by the absence of immunoreactivity for Tbr2, and the maintenance of long radial processes during mitosis (Fietz et al., 2010; Reillo et al., 2011; Kalebic et al., 2018, 2019).
Gliogenesis
Ferret gliogenesis starts during the last 4 days of the embryonic development (E38–E41) concomitantly with the peak of neurogenesis (Voigt, 1989; Reillo et al., 2011; Figure 2A). Similar overlap of the neurogenic and gliogenic period is characteristic for the neocortex development of other species with an expanded neocortex, notably macaque and human (Zecevic et al., 2005; Rash et al., 2019). However, in species with a small neocortex, such as mouse, these processes are sequential, that is gliogenesis takes place after neurogenesis (Kriegstein and Alvarez-Buylla, 2009). At E38 ferret neural progenitors start expressing the oligodendrocyte transcription factor 2 (Olig2), marker of oligodendroglial progenitors (Reillo et al., 2011). The abundance of Olig2+ cells increases during the first postnatal week and starts decreasing by P14. The astrocytic marker, glial fibrillary acidic protein (GFAP), starts to be detected at P0 and the abundance of GFAP+ cells peaks during the first two postnatal weeks of life (Voigt, 1989; Reillo et al., 2011). It is important to note that in carnivores, GFAP is expressed by both radial glial cells when they switch to astrogliogenic fate and by mature astrocytes (Voigt, 1989; Reillo et al., 2011).
Neuronal Migration and the End of Neurogenesis
Similar to the onset of neurogenesis, the termination of neuronal production follows rostro-caudal and latero-medial gradients. Whereas in the prospective somatosensory cortex production of neurons is largely completed at birth, in the visual cortex neurogenesis was reported to protract for 2 weeks postnatally (Jackson et al., 1989; Noctor et al., 1997; Figure 2A). Neuronal migration, however, continues postnatally in all brain regions. The majority of prenatally generated neurons reaches their final position in the CP within the first postnatal week, whereas the migration of postnatally generated neurons continues into the second week of life (Jackson et al., 1989; Noctor et al., 1997). This is particularly relevant for the upper-layer neurons, as, for example, in the somatosensory cortex at P1 those neurons are still migrating to their final position (Noctor et al., 1997). Neocortical neurons migrate along the radial processes of radial glia. In lissencephalic species, such as mouse, this migration is radial. In ferret and other gyrencephalic species the neocortical neurons display a tortuous manner of migration which enables tangential dispersion of neurons, that in turn is needed to form the gyri (Gertz and Kriegstein, 2015). At later stages of neocortex development ferret neurons switch from radial to tangential migration, which is accompanied by a sequential use of several different radial glial processes (Gertz and Kriegstein, 2015).
Synaptogenesis
Synaptogenesis in the ferret starts as soon as migrating neurons reach their final position in the CP (Voigt et al., 1993). Synaptophysin, an indicator of synapse formation, first appears in germinal zones of the prospective visual cortex at E34 (Reillo et al., 2017; Figure 2A). Postnatally, synaptophysin levels reduce in germinal zones and increase in the CP concomitantly with the arrival of migrating neurons, suggesting that synapses are formed in a given layer shortly after the cells reach it. As with other aspects of neocortex development, synaptogenesis is characterized by a rostro-caudal and a latero-medial gradients (Voigt et al., 1993; Herrmann, 1996).
Cortical Folding and Morphological Maturation
The ferret is born lissencephalic, that is with a smooth brain, and the folding process takes place during the first month of life (Smart and McSherry, 1986a,b; Neal et al., 2007; Barnette et al., 2009; Sawada and Watanabe, 2012; Figures 2A,B). The first visible indentation appears at P4 (Neal et al., 2007; Sawada and Watanabe, 2012; Figure 2B). At this stage, the ferret shows many characteristics of a predominantly immature brain, as the lateral ventricles are still large and the SVZ is quite pronounced. Hence, this stage of ferret brain development is considered to correspond to the period between the gestation weeks (GW) 16 and 20 of the human fetal development, that is when the primary sulci emerge (Lohmann et al., 1999; Barnette et al., 2009). During the next few postnatal weeks of the ferret development, the lateral ventricles shrink, the SVZ becomes less prominent and the CP thickens (Barnette et al., 2009). These features of the morphological maturation of the ferret brain follow both rostro-caudal, and latero-medial gradients. Moreover, they are similar to, but simpler than the patterns seen in the human brain during the second and third trimester of gestation (Barnette et al., 2009; Sawada and Watanabe, 2012). By P14, all main sulci and gyri are formed, but further maturation of the sulcal indentations and gyral folds occurs over the following 2 weeks (Figure 2B). Gyrification is considered completed by P28 (Sawada and Watanabe, 2012). Nevertheless, the shape of the brain afterward changes remarkably, with the rostral part narrowing and elongating (Barnette et al., 2009; Sawada and Watanabe, 2012).
Cortical Areas
The ferret is an important model organism for studying the development of cortical areas and circuitry. In particular, the ferret gave an important contribution to our understanding of the development and maturation of sensory processing (White and Fitzpatrick, 2007). This is largely due to the fact that the eye opening and the onset of hearing in ferret occur after 1 month of life giving a large postnatal window for studying the sensory development (Lohse et al., 2019). Furthermore, the ferret exhibits certain complex features of brain maturation and neuronal processing that are similar to human and different from rodents. For example, the primary visual cortex in ferrets contains columnar maps of stimulus features, such as orientation selectivity, that are typical of primates (White et al., 2001; White and Fitzpatrick, 2007). In this chapter we outline the contribution of the ferret research to decipher the development of various complex brain functions in the frontal, temporal, parietal and occipital cortical areas.
Frontal Cortex
Ferret frontal cortex lies in the anterior sigmoid, posterior sigmoid, proreal and the orbital gyri at the most rostral part of the brain (Duque and McCormick, 2010; Fritz et al., 2010; Radtke-Schuller et al., 2020; Figure 2C). Contrary to primate frontal lobe, in the ferret there is no recognizable anatomical boundary on the cortical surface that can delimit motor areas from the rest of the frontal cortex. Furthermore, the ferret lacks a clear granular layer, which is used in primates to define the prefrontal cortex. However, the primary motor cortex has been identified in ferret thanks to the large pyramidal cells in layer V that characterize this area (Radtke-Schuller et al., 2020; Figure 2C). The connectivity and neuroanatomy of the ferret frontal cortex has been increasingly of interest to the scientific community for studies regarding cognitive control of sensory processing and attention as well as goal-directed behavior (Fritz et al., 2010; Sellers et al., 2016; Zhou et al., 2016b). Moreover, a resting-state network in the ferret has been reported to resemble the default mode network in primates (Zhou et al., 2016a), which is often disrupted in neurological disorders, such as schizophrenia, autism spectrum disorders and Alzheimer’s disease (Fox and Greicius, 2010). Hence, this finding raises the possibility that the ferret could be used to model such pathological conditions.
Temporal Cortex
The auditory cortex of the ferret is located in the anterior, medial and posterior ectosylvian gyri (Kelly et al., 1986a; Kowalski et al., 1995; Bizley et al., 2005; Figure 2C). The ferret has become a widely used animal model for investigating the development and plasticity of auditory processing due to two principal reasons (Lohse et al., 2019). First, the onset of hearing in ferrets occurs only at P32 which facilitates the research of certain developmental processes that in human happen in utero (Moore, 1982). Second, ferrets have an audible frequency range that completely overlays with and exceeds the frequency range of humans (Kelly et al., 1986b). Four different tonotopically organized areas, including the primary auditory cortex and the secondary or belt areas, were recognized in the medial and posterior ectosylvian gyri, whereas two fields that are tonotopically not organized were identified in the anterior ectosylvian gyrus (Bizley et al., 2005; Figure 2C). Furthermore, the ferret has become a well-recognized model for studying circuits involved in auditory processing and attention, connecting the primary auditory cortex with the frontal cortex (Fritz et al., 2010). In this context, the rostral ventral posterior auditory field has been identified as a high-order sensory area, homologous to the tertiary (parabelt) area of the primate auditory cortex (Elgueda et al., 2019). Neurons in this area were found to show both changes in auditory responses and encode non-acoustical sound features, such as associated behavioral meaning (Elgueda et al., 2019).
Parietal Cortex
The primary somatosensory cortex in the ferret is well characterized. The part of the somatosensory cortex involved in body representation lies in the posterior sigmoid gyrus, whereas the part involved in the representation of the face is situated in the coronal gyrus (Leclerc et al., 1993; Rice et al., 1993; McLaughlin et al., 1998; Figure 2C). Secondary somatosensory cortex in the ferret is found in the anterior ectosylvian gyrus (Figure 2C). In addition, the medial suprasylvian sulcus contains a higher order somatosensory area with multisensory neurons that are influenced also by auditory stimuli (Keniston et al., 2009; Figure 2C). The most posterior part of the parietal cortex, involved in visual processing, is located in the lateral and suprasylvian gyri (Manger et al., 2002). The posterior suprasylvian area was found to be crucial for motion perception in the ferret (Philipp et al., 2006). The global motion sensitivity integrates motion information across time and space and it is one of the last visual functions to mature. Recent behavioral and electrophysiological data show a relationship between the posterior suprasylvian area and the ability of ferrets to integrate perceptual motion and form, a high-order visual function which is typically studied in primates (Dunn-Weiss et al., 2019). Hence, ferrets are becoming a suitable model not only for the development of the early visual stages, as discussed in the next section, but also for the visual psychophysics and the study of higher-level visual functions (Dunn-Weiss et al., 2019; Danka Mohammed and Khalil, 2020).
Occipital Cortex
The visual cortex is located in the occipital lobe and consists of areas 17–21. Area 17 is the primary visual area, also known as the striate cortex, and it contains a thick layer IV (Rockland, 1985; Figure 2C). The border of area 17 with the area 18, the secondary visual area, is marked by a thinning of layer IV and thickening of layer III. The thinning of layer IV accompanied by a decrease in myelination continues further at the boundary between areas 18 and 19 (Innocenti et al., 2002). Ferrets start opening their eyes after P30 and the full opening is reported to occur only at P35 (Christensson and Garwicz, 2005; Figure 2A). Due to the fact that neuronal specialization, synaptogenesis and other events critical for the development of visual circuits occur postnatally, ferrets became an important model organism for visual cortical development (Jackson et al., 1989; Reillo and Borrell, 2012; Danka Mohammed and Khalil, 2020).
Ferrets have thus been used to study various aspects of the maturation of the visual function, in particular the development of orientation selectivity, direction preference and ocular dominance columns (Katz and Crowley, 2002; White and Fitzpatrick, 2007; Roy et al., 2018; Danka Mohammed and Khalil, 2020). Orientation selectivity is one of the first visual functions to appear in mammals and ferret neurons in the primary visual area exhibit it already at the time of eye opening (Chapman and Stryker, 1993; Chapman et al., 1996; White et al., 2001). The majority of ferret neurons in the visual cortex is also characterized by a more vigorous response to a preferred direction of movement of a visual stimulus (Weliky et al., 1996; Li et al., 2006; Van Hooser et al., 2012). This direction selectivity was found to require visual experience (Li et al., 2006), although recent evidence suggests that there is an early phase of instructive plasticity happening before the eye opening (Roy et al., 2020). The work in ferret has thus made a crucial contribution in addressing the fundamental questions regarding how sensory experience shapes the functional development and maturation of a mammalian brain (Roy et al., 2018).
Techniques for Functional Studies in Ferrets
As the ferret became an established neurodevelopmental model system, different techniques have been developed to manipulate its neocortical development. In this chapter, we review the most commonly used and characterized methodologies along with their respective applications.
Pharmacological Treatments
Different pharmacological treatments have been explored in ferret to study the neocortex development and model various neuropathological conditions. Noctor et al. (1999) applied to ferret a pharmacological protocol to alter neuronal production at precise timepoints during development. The treatment consisted of an intraperitoneal injection of the anti-mitotic drug methylazoxymethanol (Figure 3A). A single injection of methylazoxymethanol inhibits cell division for 24 h and can hence be used to study the birth date of various neuronal subtypes (Noctor et al., 1999, 2001; Palmer et al., 2001) and to model cortical dysplasia in the ferret (Hasling et al., 2003).
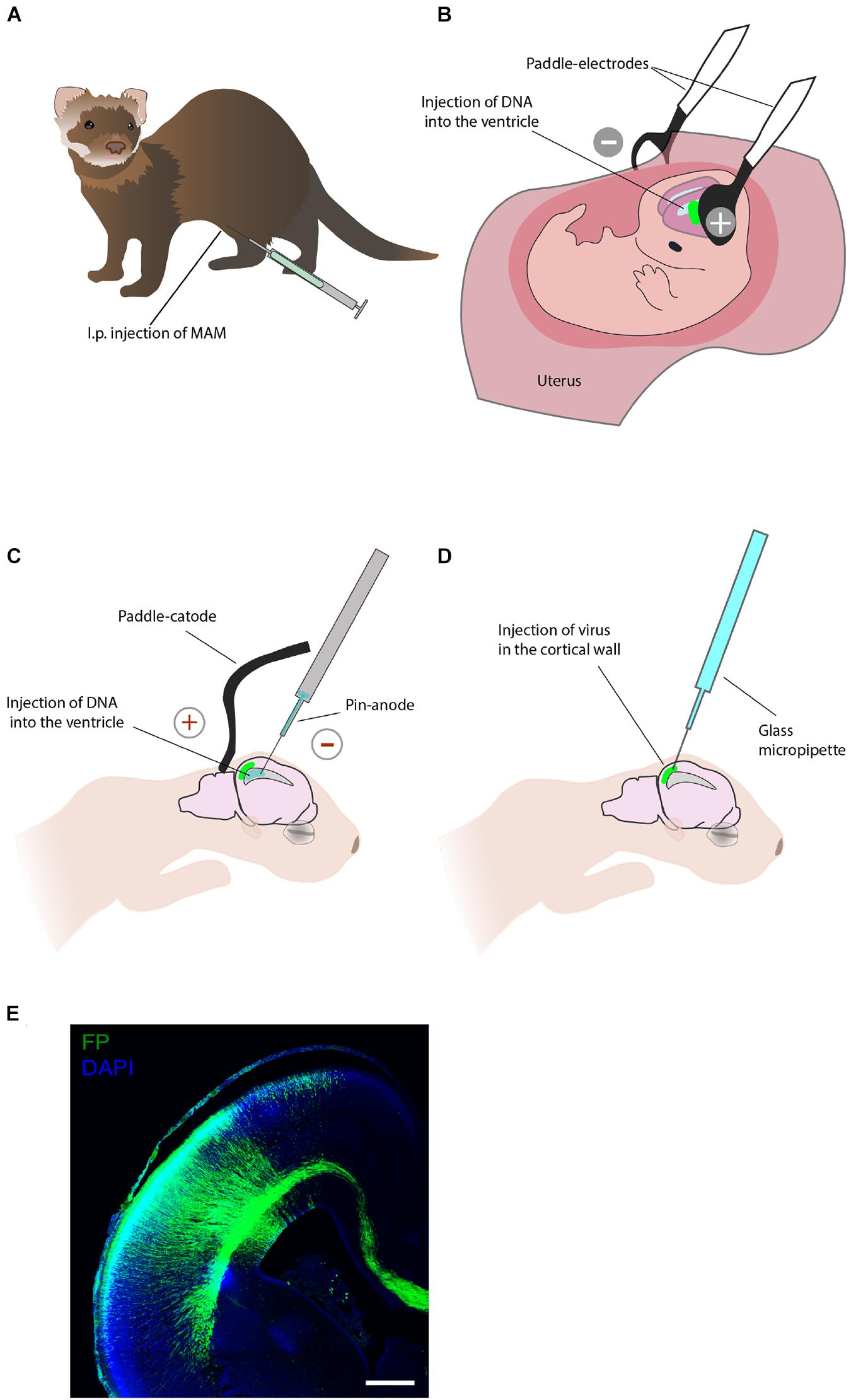
Figure 3. Techniques that allow acute manipulation of the ferret neocortex development in vivo. (A) Pharmacological treatment. Intraperitoneal (i.p.) injection of the anti-mitotic drug methylazoxymethanol (MAM) allows to study neurogenesis and model cortical dysplasia. (B) In utero electroporation enables manipulation of gene expression in neural progenitors and their progeny during embryonic development. (C) Postnatal electroporation during the first week of postnatal life enables studies of neuronal migration. (D) Viral injection at postnatal stages applied to study neural migration. (E) An example of the ferret P0 neocortex that was targeted by in utero electroporation at E33. Immunofluorescence for GFP (green), combined with DAPI staining (blue). Scale bar, 500 μm. This image has been modified from Kalebic et al. (2018).
In Utero Electroporation
In utero electroporation is a key technique for the acute manipulation of gene expression in the embryonic mammalian brain in vivo (Kalebic et al., 2020). This method was first applied to ferrets by Kawasaki and colleagues and it consists of an intraventricular injection of genetic material and subsequent electroporation to permit the entry of delivered molecules to the neuroepithelium (Kawasaki et al., 2012, 2013; Kalebic et al., 2020; Figures 3B,E). In utero electroporation has been widely used in ferrets to study the cell biology of neural progenitors (Kalebic et al., 2019; Kostic et al., 2019; Güven et al., 2020; Matsumoto et al., 2020; Xing et al., 2020), histological features of ferrets neurodevelopment (Martinez-Martinez et al., 2016; Saito et al., 2018), and cortical folding (Toda et al., 2016; Matsumoto et al., 2017b; Shinmyo et al., 2017). Of importance for translational research, in utero electroporation is increasingly being used to express human-specific genes (Kalebic et al., 2018) and to model human neurodevelopmental pathologies in ferrets (Masuda et al., 2015; Matsumoto et al., 2017a). Furthermore, a recent combination of in utero electroporation with the CRISPR/Cas9 technology allows efficient genome editing in the developing ferret neocortex (Shinmyo et al., 2017; Güven et al., 2020; Xing et al., 2020).
Postnatal Electroporation
Due to the fact that the ferret is born with an immature brain, acute genetic manipulation after birth can be used to study the late stages of the neocortex growth and maturation. Notably, this is not possible in the traditional genetically accessible neurodevelopmental models, mouse and rat, where neurogenesis is completed before birth. The postnatal electroporation protocol, established by Borrell and colleagues, has been used on P1 and P6 ferret kits to label various neocortical cells and to manipulate cortical development (Borrell et al., 2006; Borrell, 2010; Reillo et al., 2011; Figure 3C).
In Vivo Viral Injection
Although viral injection is most often used to study neural structure and function at stages when neocortex development is largely completed (Smith and Fitzpatrick, 2016), it also allows local acute labeling of neural cells embryonically and at early postnatal stages (Figure 3D). Retroviral labeling has been used to analyze cell lineages, clonal dispersion and neuronal migration during ferret embryonic and postnatal development (Reid et al., 1997; Ware et al., 1999; Reillo et al., 2017). Adenoviruses have been largely used to study neuronal migration (Borrell et al., 2006; Gertz and Kriegstein, 2015) and dynamics of neural progenitors (Gertz et al., 2014). Moreover, the viral injection can be combined with postnatal electroporation to achieve double labeling by targeting the VZ cells by electroporation, followed by retroviral infection of the cells residing in the oSVZ (Reillo et al., 2011).
Transgenic Ferret Lines
Whereas local, acute genetic manipulation methods have been successfully adapted to ferrets and can now be routinely used, there are only a couple of examples reporting the generation of transgenic ferret lines. Johnson et al. (2018) reported the generation of an Aspm (abnormal spindle-like microcephaly associated) germline knockout ferret line to model human microcephaly. The knockout was achieved by Transcription Activator Like Effector Nucleases (TALEN)-mediated genome editing. A year later, Yu et al. (2019) reported the creation of a transgenic ferret line expressing a dual-fluorescent Cre-reporter system inserted into the ferret ROSA26 intron 1. In this case CRISPR/Cas9-based genome editing was employed.
The Ferret as a Model for Human Pathologies
Owing to the emergence of new techniques, notably those that render the ferret a genetically accessible model organism, it is now possible to use ferrets to study the pathophysiology of various diseases. This is most evident when focusing on neurodevelopmental defects and malformations for which other animal models, such as the mouse, have shown to exhibit limitations. The ferret has been used as a laboratory animal since the beginning of the 20th century, firstly to describe in details its embryology (Yeates, 1911). It was soon shown that ferrets can be a suitable model for respiratory diseases (Smith et al., 1933), which resulted in ferrets becoming one of the leading model systems in this field (Enkirch and von Messling, 2015). Although a great part of the biomedical research involving ferrets focuses on neuroscience and respiratory infections, ferrets have also served as a model for gastrointestinal diseases (Hudson et al., 1992), human lung cancer (Aizawa et al., 2013), and filovirus infections, notably ebolavirus (Cross et al., 2016, 2018).
Respiratory Infections and Syndromes
The ferret is a well-established animal model to study the symptomatology, transmission and immune response related to respiratory viruses, notably influenza viruses (Zhang et al., 2013; Enkirch and von Messling, 2015; Stittelaar et al., 2016). Moreover, ferrets and other mustelids have been used in studies concerning coronaviruses, since this viral subfamily may cause severe acute respiratory syndrome in mustelids (Chu et al., 2008). Very early in the current COVID-19 pandemic it has been shown that ferrets are highly susceptible to SARS-CoV-2 infection and that they display mild symptoms, such as fever, loss of appetite, occasional coughs, and reduced body activity (Kim et al., 2020; Shi et al., 2020). SARS-CoV-2 can be actively transmitted from infected ferrets to naïve ones through direct contact or by airborne transmission even before the peak of the viral RNA copy number has been reached (Kim et al., 2020; Richard et al., 2020; Shi et al., 2020). These characteristics make the ferret one of the animal models that most closely mimics human infection and transmission (Hobbs and Reid, 2020; Mahdy et al., 2020; Munoz-Fontela et al., 2020).
Because the ferret has major similarities in respiratory tract and lung physiology with that of humans it has been long used as a model organism for cystic fibrosis, a genetic disorder caused by mutations in CFTR (cystic fibrosis transmembrane conductance regulator) and characterized by chronic infection, inflammation, and mucus obstruction (McCarron et al., 2018). Indeed, CFTR-deficient ferrets demonstrated many of the characteristics of human cystic fibrosis disease (Sun et al., 2008, 2010).
Neurological Infections
Recently, the ferret has been used in research involving infection by Zika virus due to the similarity of both the immune responses and certain neurodevelopmental features with those of human, referred to earlier in this review (Hutchinson et al., 2019). Zika virus is neurotropic and an infection during pregnancy in humans can lead to neurodevelopmental malformations, such as microcephaly, i.e. reduced brain size. In ferret these phenotypes were recapitulated with a high variability among embryos suggesting that the ferret can be used as a valuable model to understand the mechanism of the Zika pathology that can lead to a heterogeneous magnitude of symptoms (Hutchinson et al., 2019).
It is becoming increasingly clear that SARS-CoV-2 has both a short- and long-term impact on the central nervous system and various neurological symptoms have already been described (Caporale and Testa, 2021). It is therefore of great importance to search for a suitable animal model that could successfully recapitulate the key phenotypes found in humans (Sanclemente-Alaman et al., 2020). Considering the value of ferrets to model both the respiratory aspects of COVID-19 and certain complex features of the human neurodevelopment, it is an important animal model to evaluate.
Introducing Human-Specific Genomic Changes to Model Human Neurodevelopment
Compared to rodents, the ferret shows some of the key neurodevelopmental features similar to humans, notably an expanded neocortex and gyrencephaly. However, certain neurodevelopmental traits are truly human-specific and they are thought to form a basis for our unparalleled cognitive abilities (Rakic, 2009; Sousa et al., 2017; Ardesch et al., 2019; Pattabiraman et al., 2020). Since various human-specific genomic changes in both coding and non-coding regions are underlying such neurodevelopmental traits, a lot of effort has been made to identify the key changes and examine them functionally in different model systems (Silver, 2016; Dennis et al., 2017; Florio et al., 2018; Pattabiraman et al., 2020; Vaid and Huttner, 2020). The ferret is now becoming an important model organism for testing such human-specific genomic changes in the context of an already expanded brain. For example, the addition of the human-specific ARHGAP11B to the embryonic ferret resulted in a further expansion of its neocortex (Kalebic et al., 2018). This was best reflected in an increase in cell density in the upper layers of the CP, their radial expansion and the tangential expansion of the entire cortex, which are the key hallmarks of evolutionary expansion of the neocortex (Kalebic et al., 2018).
Neurodevelopmental Malformations
Neurodevelopmental malformations, such as microcephaly (reduced brain size), lissencephaly (loss of neocortical folding), polymicrogyria (numerous small neocortical folds), dysplasia (abnormal neocortical lamination), and heterotopias (abnormally positioned cells in periventricular or subcortical regions), are linked to various intellectual or motor disabilities (Romero et al., 2018; Klingler et al., 2021).
Primary microcephaly can have various genetic etiologies and can be linked to many syndromal congenital anomalies (Romero et al., 2018). Many attempts have been made to study primary microcephaly in rodent models, however, due to the small size of their brains, rodents could poorly recapitulate some of the phenotypes found in patients. Therefore, primary microcephaly was the first disease modeled in transgenic ferrets by performing the TALEN-mediated KO of Aspm (abnormal spindle-like microcephaly associated) (Johnson et al., 2018). The Aspm–/– ferrets could successfully recapitulate phenotypic features of the human pathology, such as severe microcephaly, with a striking reduction in brain weight and a decrease in brain surface and thickness (Johnson et al., 2018).
Human patients with classical lissencephaly were often found to have mutations in CDK5 (cyclin-dependent kinase 5) gene (Romero et al., 2018). Studies in Cdk5 KO mice reported various impairments of the brain development, but the link with lissencephaly remained unexplored (Ohshima et al., 1996; Gilmore et al., 1998). In contrast, when Cdk5 was acutely knocked out in the ferret developing neocortex via in utero electroporation, the size of the gyrus in the electroporated area was smaller and the sulcus was shallower, with respect to the contralateral hemisphere (Shinmyo et al., 2017).
Cortical dysplasia is a neurodevelopmental defect caused by impaired neuronal migration and it is associated with epilepsy and mental retardation. The generation of a ferret model for cortical dysplasia was achieved by injection of the anti-mitotic drug methylazoxymethanol (Noctor et al., 1999, 2001; Hasling et al., 2003). The treatment strongly impaired the generation of layer IV neurons and migration of interneurons from the ganglionic eminence (Noctor et al., 1999; Poluch et al., 2008), which could be alleviated by inhibition of GABAA receptors (Abbah and Juliano, 2014).
Thanatophoric dysplasia (TD) is a severe genetic skeletal dysplasia that results from missense mutations in FGFR3 (fibroblast growth factor receptor 3), which cause a constitutive activity of the receptor (Pannier et al., 2009). The cerebral cortex of TD patients shows various abnormalities, of which only megalencephaly (abnormally large brain) could be recapitulated in the mouse model (Lin et al., 2003). A ferret model of TD was generated by in utero electroporation of FGF8, a ligand of the FGFR3, and exhibited a thickened SVZ and an increased number of BPs (Masuda et al., 2015). Importantly TD ferrets could successfully recapitulate other key abnormalities found in human patients, such as polymicrogyria (Masuda et al., 2015), periventricular nodular heterotopia (Matsumoto et al., 2017a), and leptomeningeal glioneuronal heterotopia (Matsumoto et al., 2018).
Hydrocephalus
Hydrocephalus is a condition in which excess cerebrospinal fluid accumulates within the ventricles of the brain. In light of the characteristic postnatal protraction of the ferret neurodevelopment, ferret kits at P14 have been used as a model system for the post-hemorrhagic hydrocephalus, which is associated with premature birth (Di Curzio et al., 2013). To induce the hydrocephalus, ferrets received a kaolin (aluminum silicate hydroxide) injection in the cisterna magna, which led to ventricular dilatation, periventricular white matter damage, astrogliosis, corpus callosum atrophy, thus recapitulating typical human hydrocephalus phenotypes (Di Curzio et al., 2013).
Brain Injury
Ferrets contain a higher white matter content than rodents, similarly to humans (Schwerin et al., 2017). This led to their use as animal models for traumatic brain injury. Using ferrets Lighthall and colleagues developed a method referred to as controlled cortical impact (Lighthall, 1988). This method allows control over biomechanical parameters known to be associated with the traumatic brain injury, such as injury force and velocity and the extent of tissue deformation (Lighthall, 1988; Lighthall et al., 1990; Osier et al., 2015). Recently, controlled cortical impact method in adult ferrets was further optimized and standardized by Schwerin et al. (2017, 2018), generating animal models with transient memory deficits and altered motor skills.
Considering that the degree of maturation of the ferret brain at the moment of birth is similar to that of humans during the last trimester of pregnancy, ferret kits are also used to model preterm and perinatal human brain injury. This model was generated by subjecting the ferret kits to sub-lethal, chronic hypoxia from P10 to P20, which increased astrocytosis and decreased myelination, similar to human patients (Tao et al., 2012).
Discussion
Here we discuss the possibilities and limitations of using the ferret as a model organism for neurodevelopment. We mainly compare ferrets with the other major mammalian model organisms, notably rodents and primates. We particularly focus on the suitability of ferret as an animal model for brain evolution, brain development and neurodevelopmental pathologies.
The Ferret as a Model Organism
A plethora of different factors contributes to determine what makes a good model organism. In the past three decades, the mouse has risen as a principal model organism in many biological disciplines, including developmental neurobiology, largely due to its unparalleled genetic accessibility (Ellenbroek and Youn, 2016). However, in the current era of routine next-generation sequencing and genome editing, this advantage is lost and many historic or emerging model organisms are (re)entering the scene. In their recent review, Matthews and Vosshall (2020) list the ten steps required for an organism to become a model organism, giving the example of the mosquito Aedes aegypti that has become a neurobiological animal model. In light of those steps, we here discuss the advantages and limitations of using the ferret as a neurodevelopmental model organism.
First, the ferret is a highly suitable and interesting model organism for studying brain evolution, brain development and the related pathologies. As discussed in detail in the following sections, ferrets are more than rodents, but less than primates, similar to humans, in most of the above-mentioned aspects. Second, the ferret has been domesticated for more than 2000 years and used as a laboratory animal for more than a 100 years (Yeates, 1911; Davison et al., 1999). Ferrets are relatively small and, compared to non-human primates, the housing and economic cost of their maintenance are lower. Furthermore, similar to rodents, ferrets have a large average litter size of 8 kits per litter, whereas for most primates the litter size is 1 or 2 (Tardif et al., 2003). Third, the ferret genome has been sequenced (Peng et al., 2014). Although it is still poorly annotated, the first attempts have been made and the first transcriptomic datasets have been produced (De Juan Romero et al., 2015). In other methodological aspects, such as validation of antibody reactivity, the ferret is also lagging behind rodents. Fourth, in utero and postnatal electroporations as well as viral injections opened the possibility to introduce genetic manipulation acutely during development (Reid et al., 1997; Borrell et al., 2006; Borrell, 2010; Kawasaki et al., 2012, 2013; Gertz et al., 2014; Kalebic et al., 2020). In this aspect the ferret was the third organism after mouse and rat in which in utero electroporation was achieved. Fifth, transgenic ferrets are now available (Johnson et al., 2018; Yu et al., 2019). Moreover [combining the steps 6–9 from Matthews and Vosshall (2020)] genome editing (Shinmyo et al., 2017), routine gene knock-down (Kostic et al., 2019) and knock-out (Güven et al., 2020; Xing et al., 2020), introduction of precise mutation (Yu et al., 2019), optogenetics (Roy et al., 2016) and other genetic tools important for neuroscience have all been introduced to the ferrets. Finally, interesting questions that can be addressed using the ferret as the model organism are not lacking and include, but are not limited to, the considerations we discuss below.
The Ferret as a Model for the Evolutionary Expansion of the Neocortex
As recently argued by Gilles Laurent, neuroscience has massively benefited from model system diversity and should further embrace comparative and evolutionary approaches in modern brain research (Laurent, 2020). The ferret ideally fits into these considerations by vastly contributing to the evolutionary perspective of the brain. Ferrets and other carnivores diverged from the human lineage earlier than rodents (Bininda-Emonds et al., 2007), yet many of the features of the neocortex expansion are present in the ferret, but not in the mouse. Together with the data collected from other species, this led to a view that the evolutionary expansion of the neocortex happened independently in most mammalian orders, but it has been guided by similar principles (Borrell and Reillo, 2012). This is particularly obvious when looking into neocortical folding, a trait that highly correlates with neocortex expansion (Lewitus et al., 2013; Fernandez et al., 2016). Neocortical folding is present in all mammalian orders (Borrell and Reillo, 2012) and it appears that the Jurassic-era ancestor of all placental mammals already exhibited a folded cortex (O’Leary et al., 2013; Lewitus et al., 2014). In turn this suggests that gyrencephaly was lost and its extent reduced or increased several times independently during mammalian evolution (Kelava et al., 2013).
The enlargement of the neocortex does not concern only its surface area, although this is a dominant trait, but also its thickness (Rakic, 2009; Geschwind and Rakic, 2013). During development, transient SP and germinal zones are thicker in species with an expanded neocortex (Reillo and Borrell, 2012; Dehay et al., 2015; Rana et al., 2019; Kostovic, 2020) and the separation of the oSVZ and iSVZ can be found only in such species (Smart et al., 2002; Dehay et al., 2015). The adult neocortex also exhibits a different thickness, which is on average two-fold greater in primates compared to rodents (Rakic, 2009). This increase is mostly pertinent to the supragranular layers that roughly doubled in primates compared to rodents (Hutsler et al., 2005). Moreover, there is a clear increase in the thickness of the supragranular layers within primates, with humans having 1.5-fold thicker supragranular layers than macaque (Hutsler et al., 2005). Importantly, carnivores exhibit intermediate characteristics and the ferret has supragranular layers that are 2-fold thicker compared to mouse and 2-fold thinner compared to human (Hutsler et al., 2005). The supragranular layers contain neurons that form ipsi- and contra-lateral cortical connections (Lodato and Arlotta, 2015), with the latter thought to play an important role in high-level integrative cortical functions in associative areas (Fame et al., 2011).
Neocortex expansion is characterized by a differential growth of existing neocortical areas as well as the acquisition of novel ones, which in primate brain evolution is particularly relevant in the frontal cortex (Krubitzer and Kaas, 2005; Kaas, 2013). The protomap hypothesis postulates that the final size and pattern of neocortical areas is determined already during development by various molecular gradients that specifically guide and attract afferent systems to appropriate positions where they can interact with specific cells (Rakic, 1988). It has recently been shown that the development of a prospective gyrus vs. a prospective sulcus of the ferret neocortex is also determined by differently expressed genes during embryonic development (De Juan Romero et al., 2015). Interestingly, those differentially expressed genes exhibited a similar expression pattern in fetal human neocortex, but showed no obvious pattern in embryonic mouse neocortex (De Juan Romero et al., 2015).
The Ferret as a Model for Brain Development
Traditionally the greatest value of the ferret as a model system for brain development came from the characteristic protraction of its brain development into the postnatal period. This allowed researchers to study processes in ferret kits that in human and rodents happen in utero. Therefore, thanks to this practical and convenient feature of its neurodevelopment, the ferret rose to one of the principal models for studying cell biology in vivo of neural stem cells, neuronal migration and connectivity. For example, the ferret is a good model to address the maturation of the thalamocortical connectivity in an extra-uterine context (Wess et al., 2017; Lohse et al., 2019). It has recently been shown that SP neurons of the ferret auditory cortex respond to sound at very young ages, even before the opening of the ears, suggesting that the early sound experience can activate subplate circuits before permanent thalamocortical circuits are established (Wess et al., 2017).
The second important feature of ferret neurodevelopment is its similarity to the human, especially when looking into neocortex development. This is most obvious when comparing mouse, ferret and human neocortex (Figure 1) development and distinguishing between the quantitative and qualitative differences across these three species. In quantitative terms, the ferret shows intermediate characteristics in various neurodevelopmental aspects. An example brought in the previous section is that the thickness of supragranular layers in the ferret is an exact intermediate between the mouse and human (Hutsler et al., 2005). In qualitative terms, the ferret shares some neurodevelopmental features with humans that are completely lacking in the mouse. Those include, but are not limited to, the expansion of the SVZ and distinction of the oSVZ (Reillo and Borrell, 2012), abundance of BPs and particularly proliferative bRG (Fietz et al., 2010; Reillo et al., 2011; Kalebic et al., 2019), morphological heterogeneity of bRG (Kalebic et al., 2019), tangential dispersion of migrating neurons (Gertz and Kriegstein, 2015), presence of both inner and outer fiber layers (Saito et al., 2018), and neocortical folding (De Juan Romero et al., 2015; Figure 1). Albeit present in both the human and ferret, most of the features mentioned are quantitatively reduced in the ferret. For example, the ferret neocortex contains all the bRG morphotypes seen in the human, but their relative proportions are different as there are less process-rich bRG in the ferret (Kalebic et al., 2019). Moreover, ferret neocortex is folded, but the gyrencephaly index, i.e. a parameter of the extent of cortical folding, is significantly lower than in humans (Lewitus et al., 2014).
During the neocortical neurogenesis the temporal order of neuronal production follows the well-established inside-out rule. Classical research in the ferret has provided important evidence that the potential of neural progenitors to generate lower vs. upper layer neurons is restricted over time (McConnell and Kaznowski, 1991; Frantz and McConnell, 1996; Desai and McConnell, 2000). Upon heterochronic transplantations, early ferret progenitors, that normally produce lower layer neurons, are capable of producing upper layer neurons, whereas late progenitors appear to be more restricted and can only produce upper layer neurons, even when transplanted into an earlier host environment (McConnell and Kaznowski, 1991; Frantz and McConnell, 1996; Desai and McConnell, 2000). Recent transplantation studies of specific subpopulations of neural progenitors in mouse, however, revealed additional complexity (Oberst et al., 2019; Telley et al., 2019). Notably, mouse apical progenitors, that are generally highly proliferative, were found to be temporally plastic and able to re-enter past neurogenic states. In contrast, mouse BPs, that are highly neurogenic, lacked such plasticity (Oberst et al., 2019). In light of this, it would be important to examine if specific subpopulations of ferret BPs, notably the proliferative bRG and neurogenic bIPs, behave differently upon heterochronic transplantation. Furthermore, recent studies identified selected subpopulations of mouse progenitors (Franco et al., 2012; Garcia-Moreno and Molnar, 2015) that selectively contribute to the production of upper layer neurons and that are restricted in its fate potential even before the onset of neurogenesis. Considering the relative increase in upper layer neurons in ferret vs. mouse, it would be useful to examine if subpopulations of such fate-restricted progenitors are enriched in the ferret.
The Ferret as a Model for Human Neuropathologies
In light of the above-mentioned developmental features, the ferret is considered to be a highly suitable model organism for certain neurodevelopmental pathologies. This primarily refers to neocortical malformations (Klingler et al., 2021), which can be a consequence of impaired progenitor proliferation or neuronal migration (Bizzotto and Francis, 2015; Buchsbaum and Cappello, 2019) and affect neocortex size and shape. Since ferrets exhibit an expanded and folded neocortex, they are suitable for modeling diseases such as microcephaly (Johnson et al., 2018) and lissencephaly (Shinmyo et al., 2017). Considering the important role of ferrets in virology and immunology for modeling human influenzas, it is important to emphasize the role of ferrets for studying the neurotropic effects of viruses such as Zika (Hutchinson et al., 2019) and SARS-CoV-2. Combining the previous application in neurodevelopmental disorders and viral infections, the ferret is becoming an emerging model organism for studying the relationship between the maternal immune activation and neuropsychiatric diseases (Li et al., 2018).
Limitations of the Ferret as a Model Organism for Neurodevelopment
The ferret is a suitable model for studying some of the mechanisms regulating brain development, but it also exhibits certain limitations. Only some molecular and cellular mechanisms involved in neocortex development and the related pathologies are conserved across mammals and this is particularly relevant for the development of complex traits. For example, neocortical folding is thought to arise through an interplay of genetic and mechanical factors (Borrell, 2018; Kroenke and Bayly, 2018), with some of the genetic underpinnings being fairly conserved between ferret and human (Albert and Huttner, 2015; De Juan Romero and Borrell, 2017). In contrast, an extracellular matrix-mediated mechanism that contributes to folding by modulating tissue stiffness was found to operate in human, but not ferret neocortical tissue (Long et al., 2018).
The protracted postnatal period of brain development has traditionally been one of the greatest reasons for using the ferret kits to model the last trimester of human brain development (Barnette et al., 2009), however, it also poses some limitations. The ferret kit is exposed to environmental sensory stimuli that are not present in utero, which can lead to a different development and maturation of cortical sensory areas. An example is the difference in some aspects of maturation of the visual circuitry, which are thought to happen in a hierarchical fashion in humans, but occur in a synchronous manner in ferrets (Danka Mohammed and Khalil, 2020).
Finally, there are ethical considerations to take into account, especially when working with transgenic ferrets. Research involving ferrets is usually subjected to a greater regulatory scrutiny than the one involving mice. In contrast, ferrets could be a suitable replacement for primates when studying developmental mechanisms that are pertinent to all species with an expanded neocortex. Hence, the ethical considerations, along with the space allocation and cost of ferret research, are additional limitations for using ferrets instead of mice, but at the same time provide an advantage for using ferrets as a replacement for primates.
Conclusion
Ferrets are fascinating creatures whose neurodevelopmental features render them an important model organism for brain evolution, development, and related pathologies. In this review we highlight the key characteristics of the developing ferret neocortex and discuss the tools and techniques that enable us to use these animals for revealing the complexity of the mammalian neocortex development and modeling human neurodevelopmental pathologies.
Author Contributions
Both authors listed have made a substantial, direct and intellectual contribution to the work, and approved it for publication.
Conflict of Interest
The authors declare that the research was conducted in the absence of any commercial or financial relationships that could be construed as a potential conflict of interest.
Acknowledgments
We thank Katherine R. Long (King’s College London) for critical reading of the manuscript.
References
Abbah, J., and Juliano, S. L. (2014). Altered migratory behavior of interneurons in a model of cortical dysplasia: the influence of elevated GABAA activity. Cereb. Cortex 24, 2297–2308. doi: 10.1093/cercor/bht073
Aizawa, K., Liu, C., Veeramachaneni, S., Hu, K. Q., Smith, D. E., and Wang, X. D. (2013). Development of ferret as a human lung cancer model by injecting 4-(Nmethyl-N-nitrosamino)-1-(3-pyridyl)-1-butanone (NNK). Lung Cancer 82, 390–396. doi: 10.1016/j.lungcan.2013.09.012
Albert, M., and Huttner, W. B. (2015). Clever space saving-how the cerebral cortex folds. EMBO J. 34, 1845–1847. doi: 10.15252/embj.201591952
Angevine, J. B. J., and Sidman, R. L. (1961). Autoradiographic study of cell migration during histogenesis of cerebral cortex in the mouse. Nature 192, 766–768. doi: 10.1038/192766b0
Antonini, A., and Shatz, C. J. (1990). Relation between putative transmitter phenotypes and connectivity of subplate neurons during cerebral cortical development. Eur. J. Neurosci. 2, 744–761. doi: 10.1111/j.1460-9568.1990.tb00465.x
Arai, Y., Pulvers, J. N., Haffner, C., Schilling, B., Nusslein, I., Calegari, F., et al. (2011). Neural stem and progenitor cells shorten S-phase on commitment to neuron production. Nat. Commun. 2:154. doi: 10.1038/ncomms1155
Ardesch, D. J., Scholtens, L. H., and van den Heuvel, M. P. (2019). The human connectome from an evolutionary perspective. Prog. Brain Res. 250, 129–151. doi: 10.1016/bs.pbr.2019.05.004
Barnette, A. R., Neil, J. J., Kroenke, C. D., Griffith, J. L., Epstein, A. A., Bayly, P. V., et al. (2009). Characterization of brain development in the ferret via MRI. Pediatr. Res. 66, 80–84. doi: 10.1203/PDR.0b013e3181a291d9
Betizeau, M., Cortay, V., Patti, D., Pfister, S., Gautier, E., Bellemin-Meìnard, A., et al. (2013). Precursor diversity and complexity of lineage relationships in the outer subventricular zone of the primate. Neuron 80, 442–457. doi: 10.1016/j.neuron.2013.09.032
Bininda-Emonds, O. R., Cardillo, M., Jones, K. E., MacPhee, R. D., Beck, R. M., Grenyer, R., et al. (2007). The delayed rise of present-day mammals. Nature 446, 507–512. doi: 10.1038/nature05634
Bizley, J. K., Nodal, F. R., Nelken, I., and King, A. J. (2005). Functional organization of ferret auditory cortex. Cereb. Cortex 15, 1637–1653. doi: 10.1093/cercor/bhi042
Bizzotto, S., and Francis, F. (2015). Morphological and functional aspects of progenitors perturbed in cortical malformations. Front. Cell. Neurosci. 9:30. doi: 10.3389/fncel.2015.00030
Borrell, V. (2010). In vivo gene delivery to the postnatal ferret cerebral cortex by DNA electroporation. J. Neurosci. Methods 186, 186–195. doi: 10.1016/j.jneumeth.2009.11.016
Borrell, V. (2018). How cells fold the cerebral cortex. J. Neurosci. 38, 776–783. doi: 10.1523/JNEUROSCI.1106-17.2017
Borrell, V., and Reillo, I. (2012). Emerging roles of neural stem cells in cerebral cortex development and evolution. Dev. Neurobiol. 72, 955–971. doi: 10.1002/dneu.22013
Borrell, V., Kaspar, B. K., Gage, F. H., and Callaway, E. M. (2006). In vivo evidence for radial migration of neurons by long-distance somal translocation in the developing ferret visual cortex. Cereb. Cortex 16, 1571–1583. doi: 10.1093/cercor/bhj094
Buchsbaum, I. Y., and Cappello, S. (2019). Neuronal migration in the CNS during development and disease: insights from in vivo and in vitro models. Development 146:dev163766. doi: 10.1242/dev.163766
Calegari, F., Haubensak, W., Haffner, C., and Huttner, W. B. (2005). Selective lengthening of the cell cycle in the neurogenic subpopulation of neural progenitor cells during mouse brain development. J. Neurosci. 25, 6533–6538. doi: 10.1523/JNEUROSCI.0778-05.2005
Caporale, N., and Testa, G. (2021). COVID-19 lessons from the dish: dissecting CNS manifestations through brain organoids. EMBO J. 40:e107213. doi: 10.15252/embj.2020107213
Chapman, B., and Stryker, M. P. (1993). Development of orientation selectivity in ferret visual cortex and effects of deprivation. J. Neurosci. 13, 5251–5262. doi: 10.1523/JNEUROSCI.13-12-05251.1993
Chapman, B., Stryker, M. P., and Bonhoeffer, T. (1996). Development of orientation preference maps in ferret primary visual cortex. J. Neurosci. 16, 6443–6453. doi: 10.1523/JNEUROSCI.16-20-06443.1996
Christensson, M., and Garwicz, M. (2005). Time course of postnatal motor development in ferrets: ontogenetic and comparative perspectives. Behav. Brain Res. 158, 231–242. doi: 10.1016/j.bbr.2004.09.003
Chu, Y. K., Ali, G. D., Jia, F., Li, Q., Kelvin, D., Couch, R. C., et al. (2008). The SARS-CoV ferret model in an infection-challenge study. Virology 374, 151–163. doi: 10.1016/j.virol.2007.12.032
Cross, R. W., Fenton, K. A., and Geisbert, T. W. (2018). Small animal models of filovirus disease: recent advances and future directions. Expert Opin. Drug Discov. 13, 1027–1040. doi: 10.1080/17460441.2018.1527827
Cross, R. W., Mire, C. E., Borisevich, V., Geisbert, J. B., Fenton, K. A., and Geisbert, T. W. (2016). The domestic ferret (Mustela putorius furo) as a lethal infection model for 3 species of Ebolavirus. J. Infect. Dis. 214, 565–569. doi: 10.1093/infdis/jiw209
Danka Mohammed, C. P., and Khalil, R. (2020). Postnatal development of visual cortical function in the mammalian brain. Front. Syst. Neurosci. 14:29. doi: 10.3389/fnsys.2020.00029
Davison, A., Birks, J. D. S., Griffiths, H. I., Kitchener, A. C., Biggins, D., and Butlin, R. K. (1999). Hybridization and the phylogenetic relationship between polecats and domestic ferrets in Britain. Biol. Conserv. 87, 155–161. doi: 10.1016/S0006-3207(98)00067-6
De Juan Romero, C., and Borrell, V. (2017). Genetic maps and patterns of cerebral cortex folding. Curr. Opin. Cell Biol. 49, 31–37. doi: 10.1016/j.ceb.2017.11.009
De Juan Romero, C., Bruder, C., Tomasello, U., Sanz-Anquela, J. M., and Borrell, V. (2015). Discrete domains of gene expression in germinal layers distinguish the development of gyrencephaly. EMBO J. 34, 1859–1874. doi: 10.15252/embj.201591176
Dehay, C., Kennedy, H., and Kosik, K. S. (2015). The outer subventricular zone and primate-specific cortical complexification. Neuron 85, 683–694. doi: 10.1016/j.neuron.2014.12.060
Dennis, M. Y., Harshman, L., Nelson, B. J., Penn, O., Cantsilieris, S., Huddleston, J., et al. (2017). The evolution and population diversity of human-specific segmental duplications. Nat. Ecol. Evol. 1:69. doi: 10.1038/s41559-016-0069
Desai, A. R., and McConnell, S. K. (2000). Progressive restriction in fate potential by neural progenitors during cerebral cortical development. Development 127, 2863–2872.
Di Curzio, D. L., Buist, R. J., and Del Bigio, M. R. (2013). Reduced subventricular zone proliferation and white matter damage in juvenile ferrets with kaolin-induced hydrocephalus. Exp. Neurol. 248, 112–128. doi: 10.1016/j.expneurol.2013.06.004
Dunn-Weiss, E., Nummela, S. U., Lempel, A. A., Law, J. M., Ledley, J., Salvino, P., et al. (2019). Visual motion and form integration in the behaving Ferret. eNeuro 6:ENEURO.0228-19.2019. doi: 10.1523/ENEURO.0228-19.2019
Duque, A., and McCormick, D. A. (2010). Circuit-based localization of ferret prefrontal cortex. Cereb. Cortex 20, 1020–1036. doi: 10.1093/cercor/bhp164
Elgueda, D., Duque, D., Radtke-Schuller, S., Yin, P., David, S. V., Shamma, S. A., et al. (2019). State-dependent encoding of sound and behavioral meaning in a tertiary region of the ferret auditory cortex. Nat. Neurosci. 22, 447–459. doi: 10.1038/s41593-018-0317-8
Ellenbroek, B., and Youn, J. (2016). Rodent models in neuroscience research: is it a rat race? Dis. Model. Mech. 9, 1079–1087. doi: 10.1242/dmm.026120
Enkirch, T., and von Messling, V. (2015). Ferret models of viral pathogenesis. Virology 479-480, 259–270. doi: 10.1016/j.virol.2015.03.017
Evsyukova, I., Plestant, C., and Anton, E. S. (2013). Integrative mechanisms of oriented neuronal migration in the developing brain. Annu. Rev. Cell Dev. Biol. 29, 299–353. doi: 10.1146/annurev-cellbio-101512-122400
Fame, R. M., MacDonald, J. L., and Macklis, J. D. (2011). Development, specification, and diversity of callosal projection neurons. Trends Neurosci. 34, 41–50. doi: 10.1016/j.tins.2010.10.002
Fernandez, V., Llinares-Benadero, C., and Borrell, V. (2016). Cerebral cortex expansion and folding: what have we learned? EMBO J. 35, 1021–1044. doi: 10.15252/embj.201593701
Fietz, S. A., and Huttner, W. B. (2011). Cortical progenitor expansion, self-renewal and neurogenesis-a polarized perspective. Curr. Opin. Neurobiol. 21, 23–35. doi: 10.1016/j.conb.2010.10.002
Fietz, S. A., Kelava, I., Vogt, J., Wilsch-Brauninger, M., Stenzel, D., Fish, J. L., et al. (2010). OSVZ progenitors of human and ferret neocortex are epithelial-like and expand by integrin signaling. Nat. Neurosci. 13, 690–699. doi: 10.1038/nn.2553
Florio, M., Heide, M., Pinson, A., Brandl, H., Albert, M., Winkler, S., et al. (2018). Evolution and cell-type specificity of human-specific genes preferentially expressed in progenitors of fetal neocortex. eLife 7:e32332. doi: 10.7554/eLife.32332
Fox, M. D., and Greicius, M. (2010). Clinical applications of resting state functional connectivity. Front. Syst. Neurosci. 4:19. doi: 10.3389/fnsys.2010.00019
Franco, S. J., and Muller, U. (2013). Shaping our minds: stem and progenitor cell diversity in the mammalian neocortex. Neuron 77, 19–34. doi: 10.1016/j.neuron.2012.12.022
Franco, S. J., Gil-Sanz, C., Martinez-Garay, I., Espinosa, A., Harkins-Perry, S. R., Ramos, C., et al. (2012). Fate-restricted neural progenitors in the mammalian cerebral cortex. Science 337, 746–749. doi: 10.1126/science.1223616
Frantz, G. D., and McConnell, S. K. (1996). Restriction of late cerebral cortical progenitors to an upper-layer fate. Neuron 17, 55–61. doi: 10.1016/S0896-6273(00)80280-9
Fritz, J. B., David, S. V., Radtke-Schuller, S., Yin, P., and Shamma, S. A. (2010). Adaptive, behaviorally gated, persistent encoding of task-relevant auditory information in ferret frontal cortex. Nat. Neurosci. 13, 1011–1019. doi: 10.1038/nn.2598
Garcia-Moreno, F., and Molnar, Z. (2015). Subset of early radial glial progenitors that contribute to the development of callosal neurons is absent from avian brain. Proc. Natl. Acad. Sci. U.S.A. 112, E5058–E5067. doi: 10.1073/pnas.1506377112
Gertz, C. C., and Kriegstein, A. R. (2015). Neuronal migration dynamics in the developing ferret cortex. J. Neurosci. 35, 14307–14315. doi: 10.1523/JNEUROSCI.2198-15.2015
Gertz, C. C., Lui, J. H., Lamonica, B. E., Wang, X., and Kriegstein, A. R. (2014). Diverse behaviors of outer radial glia in developing ferret and human cortex. J. Neurosci. 34, 2559–2570. doi: 10.1523/JNEUROSCI.2645-13.2014
Geschwind, D. H., and Rakic, P. (2013). Cortical evolution: judge the brain by its cover. Neuron 80, 633–647. doi: 10.1016/j.neuron.2013.10.045
Gilmore, E. C., Ohshima, T., Goffinet, A. M., Kulkarni, A. B., and Herrup, K. (1998). Cyclin-dependent kinase 5-deficient mice demonstrate novel developmental arrest in cerebral cortex. J. Neurosci. 18, 6370–6377. doi: 10.1523/JNEUROSCI.18-16-06370.1998
Güven, A., Kalebic, N., Long, K. R., Florio, M., Vaid, S., Brandl, H., et al. (2020). Extracellular matrix-inducing Sox9 promotes both basal progenitor proliferation and gliogenesis in developing neocortex. eLife 9:e49808. doi: 10.7554/eLife.49808
Hansen, D. V., Lui, J. H., Parker, P. R., and Kriegstein, A. R. (2010). Neurogenic radial glia in the outer subventricular zone of human neocortex. Nature 464, 554–561. doi: 10.1038/nature08845
Hasling, T. A., Gierdalski, M., Jablonska, B., and Juliano, S. L. (2003). A radialization factor in normal cortical plate restores disorganized radial glia and disrupted migration in a model of cortical dysplasia. Eur. J. Neurosci. 17, 467–480. doi: 10.1046/j.1460-9568.2003.02468.x
Haubensak, W., Attardo, A., Denk, W., and Huttner, W. B. (2004). Neurons arise in the basal neuroepithelium of the early mammalian telencephalon: a major site of neurogenesis. Proc. Natl. Acad. Sci. U.S.A. 101, 3196–3201. doi: 10.1073/pnas.0308600100
Herrmann, K. (1996). Differential distribution of AMPA receptors and glutamate during pre- and postnatal development in the visual cortex of ferrets. J. Comp. Neurol. 375, 1–17. doi: 10.1002/(SICI)1096-9861(19961104)375:1<1::AID-CNE1>3.0.CO;2-7
Hobbs, E. C., and Reid, T. J. (2020). Animals and SARS-CoV-2: species susceptibility and viral transmission in experimental and natural conditions, and the potential implications for community transmission. Transbound. Emerg. Dis. [Epub ahead of print]. doi: 10.1111/tbed.13885
Hudson, M., Piasecki, C., Sankey, E. A., Sim, R., Wakefield, A. J., More, L. J., et al. (1992). A ferret model of acute multifocal gastrointestinal infarction. Gastroenterology 102, 1591–1596. doi: 10.1016/0016-5085(92)91718-J
Hutchinson, E. B., Chatterjee, M., Reyes, L., Djankpa, F. T., Valiant, W. G., Dardzinski, B., et al. (2019). The effect of Zika virus infection in the ferret. J. Comp. Neurol. 527, 1706–1719. doi: 10.1002/cne.24640
Hutsler, J. J., Lee, D. G., and Porter, K. K. (2005). Comparative analysis of cortical layering and supragranular layer enlargement in rodent carnivore and primate species. Brain Res. 1052, 71–81. doi: 10.1016/j.brainres.2005.06.015
Innocenti, G. M., Manger, P. R., Masiello, I., Colin, I., and Tettoni, L. (2002). Architecture and callosal connections of visual areas 17, 18, 19 and 21 in the ferret (Mustela putorius). Cereb. Cortex 12, 411–422. doi: 10.1093/cercor/12.4.411
Jackson, C. A., Peduzzi, J. D., and Hickey, T. L. (1989). Visual cortex development in the ferret. I. Genesis and migration of visual cortical neurons. J. Neurosci. 9, 1242–1253. doi: 10.1523/JNEUROSCI.09-04-01242.1989
Johnson, M. B., Sun, X., Kodani, A., Borges-Monroy, R., Girskis, K. M., Ryu, S. C., et al. (2018). Aspm knockout ferret reveals an evolutionary mechanism governing cerebral cortical size. Nature 556, 370–375. doi: 10.1038/s41586-018-0035-0
Kaas, J. H. (2013). The evolution of brains from early mammals to humans. Wiley Interdiscip. Rev. Cogn. Sci. 4, 33–45. doi: 10.1002/wcs.1206
Kalebic, N., and Huttner, W. B. (2020). Basal progenitor morphology and neocortex evolution. Trends Neurosci. 43, 843–853. doi: 10.1016/j.tins.2020.07.009
Kalebic, N., Gilardi, C., Albert, M., Namba, T., Long, K. R., Kostic, M., et al. (2018). Human-specific ARHGAP11B induces hallmarks of neocortical expansion in developing ferret neocortex. eLife 7:e41241. doi: 10.7554/eLife.41241
Kalebic, N., Gilardi, C., Stepien, B., Wilsch-Brauninger, M., Long, K. R., Namba, T., et al. (2019). Neocortical expansion due to increased proliferation of basal progenitors is linked to changes in their morphology. Cell Stem Cell 24, 535–550.e9. doi: 10.1016/j.stem.2019.02.017
Kalebic, N., Langen, B., Helppi, J., Kawasaki, H., and Huttner, W. B. (2020). In vivo targeting of neural progenitor cells in ferret neocortex by in utero electroporation. J. Vis. Exp. 159:e61171. doi: 10.3791/61171
Kalebic, N., Long, K., and Huttner, W. B. (2017). “Neocortex expansion in development and evolution: the cell biology of neural stem and progenitor cells and the impact of human-specific gene expression,” in Evolution of nervous systems 2e, ed. J. Kaas (Oxford: Elsevier), 73–89. doi: 10.1016/B978-0-12-804042-3.00136-6
Katz, L. C., and Crowley, J. C. (2002). Development of cortical circuits: lessons from ocular dominance columns. Nat. Rev. Neurosci. 3, 34–42. doi: 10.1038/nrn703
Kawasaki, H. (2014). Molecular investigations of the brain of higher mammals using gyrencephalic carnivore ferrets. Neurosci. Res. 86, 59–65. doi: 10.1016/j.neures.2014.06.006
Kawasaki, H., Iwai, L., and Tanno, K. (2012). Rapid and efficient genetic manipulation of gyrencephalic carnivores using in utero electroporation. Mol. Brain 5:24. doi: 10.1186/1756-6606-5-24
Kawasaki, H., Toda, T., and Tanno, K. (2013). In vivo genetic manipulation of cortical progenitors in gyrencephalic carnivores using in utero electroporation. Biol. Open 2, 95–100. doi: 10.1242/bio.20123160
Kelava, I., Lewitus, E., and Huttner, W. B. (2013). The secondary loss of gyrencephaly as an example of evolutionary phenotypical reversal. Front. Neuroanat. 7:16. doi: 10.3389/fnana.2013.00016
Kelly, J. B., Judge, P. W., and Phillips, D. P. (1986a). Representation of the cochlea in primary auditory cortex of the ferret (Mustela putorius). Hear. Res. 24, 111–115. doi: 10.1016/0378-5955(86)90054-7
Kelly, J. B., Kavanagh, G. L., and Dalton, J. C. (1986b). Hearing in the ferret (Mustela putorius): thresholds for pure tone detection. Hear. Res. 24, 269–275. doi: 10.1016/0378-5955(86)90025-0
Keniston, L. P., Allman, B. L., Meredith, M. A., and Clemo, H. R. (2009). Somatosensory and multisensory properties of the medial bank of the ferret rostral suprasylvian sulcus. Exp. Brain Res. 196, 239–251. doi: 10.1007/s00221-009-1843-0
Kim, Y. I., Kim, S. G., Kim, S. M., Kim, E. H., Park, S. J., Yu, K. M., et al. (2020). Infection and rapid transmission of SARS-CoV-2 in ferrets. Cell Host Microbe 27, 704–709.e2. doi: 10.1016/j.chom.2020.03.023
Klingler, E., Francis, F., Jabaudon, D., and Cappello, S. (2021). Mapping the molecular and cellular complexity of cortical malformations. Science 371:eaba4517. doi: 10.1126/science.aba4517
Kornack, D. R., and Rakic, P. (1998). Changes in cell-cycle kinetics during the development and evolution of primate neocortex. Proc. Natl. Acad. Sci. U.S.A. 95, 1242–1246. doi: 10.1073/pnas.95.3.1242
Kostic, M., Paridaen, J., Long, K. R., Kalebic, N., Langen, B., Grubling, N., et al. (2019). YAP activity is necessary and sufficient for basal progenitor abundance and proliferation in the developing neocortex. Cell Rep. 27, 1103–1118.e6. doi: 10.1016/j.celrep.2019.03.091
Kostovic, I. (2020). The enigmatic fetal subplate compartment forms an early tangential cortical nexus and provides the framework for construction of cortical connectivity. Prog. Neurobiol. 194:101883. doi: 10.1016/j.pneurobio.2020.101883
Kostovic, I., and Rakic, P. (1990). Developmental history of the transient subplate zone in the visual and somatosensory cortex of the macaque monkey and human brain. J. Comp. Neurol. 297, 441–470. doi: 10.1002/cne.902970309
Kowalski, N., Versnel, H., and Shamma, S. A. (1995). Comparison of responses in the anterior and primary auditory fields of the ferret cortex. J. Neurophysiol. 73, 1513–1523. doi: 10.1152/jn.1995.73.4.1513
Kriegstein, A., and Alvarez-Buylla, A. (2009). The glial nature of embryonic and adult neural stem cells. Annu. Rev. Neurosci. 32, 149–184. doi: 10.1146/annurev.neuro.051508.135600
Kroenke, C. D., and Bayly, P. V. (2018). How forces fold the cerebral cortex. J. Neurosci. 38, 767–775. doi: 10.1523/JNEUROSCI.1105-17.2017
Krubitzer, L. (2007). The magnificent compromise: cortical field evolution in mammals. Neuron 56, 201–208. doi: 10.1016/j.neuron.2007.10.002
Krubitzer, L., and Kaas, J. (2005). The evolution of the neocortex in mammals: How is phenotypic diversity generated? Curr. Opin. Neurobiol. 15, 444–453. doi: 10.1016/j.conb.2005.07.003
Laurent, G. (2020). On the value of model diversity in neuroscience. Nat. Rev. Neurosci. 21, 395–396. doi: 10.1038/s41583-020-0323-1
Leclerc, S. S., Rice, F. L., Dykes, R. W., Pourmoghadam, K., and Gomez, C. M. (1993). Electrophysiological examination of the representation of the face in the suprasylvian gyrus of the ferret: a correlative study with cytoarchitecture. Somatosens. Mot. Res. 10, 133–159. doi: 10.3109/08990229309028829
Lewitus, E., Kelava, I., and Huttner, W. B. (2013). Conical expansion of the outer subventricular zone and the role of neocortical folding in evolution and development. Front. Hum. Neurosci. 7:424. doi: 10.3389/fnhum.2013.00424
Lewitus, E., Kelava, I., Kalinka, A. T., Tomancak, P., and Huttner, W. B. (2014). An adaptive threshold in mammalian neocortical evolution. PLoS Biol. 12:e1002000. doi: 10.1371/journal.pbio.1002000
Li, Y., Dugyala, S. R., Ptacek, T. S., Gilmore, J. H., and Frohlich, F. (2018). Maternal immune activation alters adult behavior, gut microbiome and juvenile brain oscillations in ferrets. eNeuro 5:ENEURO.0313-18.2018. doi: 10.1523/ENEURO.0313-18.2018
Li, Y., Fitzpatrick, D., and White, L. E. (2006). The development of direction selectivity in ferret visual cortex requires early visual experience. Nat. Neurosci. 9, 676–681. doi: 10.1038/nn1684
Lighthall, J. W. (1988). Controlled cortical impact: a new experimental brain injury model. J. Neurotrauma 5, 1–15. doi: 10.1089/neu.1988.5.1
Lighthall, J. W., Goshgarian, H. G., and Pinderski, C. R. (1990). Characterization of axonal injury produced by controlled cortical impact. J. Neurotrauma 7, 65–76. doi: 10.1089/neu.1990.7.65
Lin, T., Sandusky, S. B., Xue, H., Fishbein, K. W., Spencer, R. G., Rao, M. S., et al. (2003). A central nervous system specific mouse model for thanatophoric dysplasia type II. Hum. Mol. Genet. 12, 2863–2871. doi: 10.1093/hmg/ddg309
Lindeberg, H. (2008). Reproduction of the female ferret (Mustela putorius furo). Reprod. Domest. Anim. 43(Suppl. 2), 150–156. doi: 10.1111/j.1439-0531.2008.01155.x
Lodato, S., and Arlotta, P. (2015). Generating neuronal diversity in the mammalian cerebral cortex. Annu. Rev. Cell Dev. Biol. 31, 699–720. doi: 10.1146/annurev-cellbio-100814-125353
Lohmann, G., von Cramon, D. Y., and Steinmetz, H. (1999). Sulcal variability of twins. Cereb. Cortex 9, 754–763. doi: 10.1093/cercor/9.7.754
Lohse, M., Bajo, V. M., and King, A. J. (2019). Development, organization and plasticity of auditory circuits: lessons from a cherished colleague. Eur. J. Neurosci. 49, 990–1004. doi: 10.1111/ejn.13979
Long, K. R., Newland, B., Florio, M., Kalebic, N., Langen, B., Kolterer, A., et al. (2018). Extracellular matrix components HAPLN1, Lumican, and Collagen I cause hyaluronic acid-dependent folding of the developing human neocortex. Neuron 99, 702–719. doi: 10.1016/j.neuron.2018.07.013
Lui, J. H., Hansen, D. V., and Kriegstein, A. R. (2011). Development and evolution of the human neocortex. Cell 146, 18–36. doi: 10.1016/j.cell.2011.06.030
Mahdy, M. A. A., Younis, W., and Ewaida, Z. (2020). An overview of SARS-CoV-2 and animal infection. Front. Vet. Sci. 7:596391. doi: 10.3389/fvets.2020.596391
Manger, P. R., Masiello, I., and Innocenti, G. M. (2002). Areal organization of the posterior parietal cortex of the ferret (Mustela putorius). Cereb. Cortex 12, 1280–1297. doi: 10.1093/cercor/12.12.1280
Martinez-Cerdeno, V., Cunningham, C. L., Camacho, J., Antczak, J. L., Prakash, A. N., Cziep, M. E., et al. (2012). Comparative analysis of the subventricular zone in rat, ferret and macaque: evidence for an outer subventricular zone in rodents. PLoS One 7:e30178. doi: 10.1371/journal.pone.0030178
Martinez-Cerdeno, V., Noctor, S. C., and Kriegstein, A. R. (2006). The role of intermediate progenitor cells in the evolutionary expansion of the cerebral cortex. Cereb. Cortex 16(Suppl. 1), i152–i161. doi: 10.1093/cercor/bhk017
Martinez-Martinez, M. A., De Juan Romero, C., Fernandez, V., Cardenas, A., Götz, M., and Borrell, V. (2016). A restricted period for formation of outer subventricular zone defined by Cdh1 and Trnp1 levels. Nat. Commun. 7:11812. doi: 10.1038/ncomms11812
Masuda, K., Toda, T., Shinmyo, Y., Ebisu, H., Hoshiba, Y., Wakimoto, M., et al. (2015). Pathophysiological analyses of cortical malformation using gyrencephalic mammals. Sci. Rep. 5:15370. doi: 10.1038/srep15370
Matsumoto, N., Hoshiba, Y., Morita, K., Uda, N., Hirota, M., Minamikawa, M., et al. (2017a). Pathophysiological analyses of periventricular nodular heterotopia using gyrencephalic mammals. Hum. Mol. Genet. 26, 1173–1181. doi: 10.1093/hmg/ddx038
Matsumoto, N., Kobayashi, N., Uda, N., Hirota, M., and Kawasaki, H. (2018). Pathophysiological analyses of leptomeningeal heterotopia using gyrencephalic mammals. Hum. Mol. Genet. 27, 985–991. doi: 10.1093/hmg/ddy014
Matsumoto, N., Shinmyo, Y., Ichikawa, Y., and Kawasaki, H. (2017b). Gyrification of the cerebral cortex requires FGF signaling in the mammalian brain. eLife 6:e29285. doi: 10.7554/eLife.29285
Matsumoto, N., Tanaka, S., Horiike, T., Shinmyo, Y., and Kawasaki, H. (2020). A discrete subtype of neural progenitor crucial for cortical folding in the gyrencephalic mammalian brain. eLife 9:e54873. doi: 10.7554/eLife.54873
Matthews, B. J., and Vosshall, L. B. (2020). How to turn an organism into a model organism in 10 ‘easy’ steps. J. Exp. Biol. 223(Pt Suppl. 1):jeb218198. doi: 10.1242/jeb.218198
McCarron, A., Donnelley, M., and Parsons, D. (2018). Airway disease phenotypes in animal models of cystic fibrosis. Respir. Res. 19:54. doi: 10.1186/s12931-018-0750-y
McConnell, S. K., and Kaznowski, C. E. (1991). Cell cycle dependence of laminar determination in developing neocortex. Science 254, 282–285. doi: 10.1126/science.1925583
McLaughlin, D. F., Sonty, R. V., and Juliano, S. L. (1998). Organization of the forepaw representation in ferret somatosensory cortex. Somatosens. Mot. Res. 15, 253–268. doi: 10.1080/08990229870673
McSherry, G. M., and Smart, I. H. (1986). Cell production gradients in the developing ferret isocortex. J. Anat. 144, 1–14.
Miyata, T., Kawaguchi, A., Saito, K., Kawano, M., Muto, T., and Ogawa, M. (2004). Asymmetric production of surface-dividing and non-surface-dividing cortical progenitor cells. Development 131, 3133–3145. doi: 10.1242/dev.01173
Molnar, Z., Clowry, G. J., Sestan, N., Alzu’bi, A., Bakken, T., Hevner, R. F., et al. (2019). New insights into the development of the human cerebral cortex. J. Anat. 235, 432–451. doi: 10.1111/joa.13055
Molnar, Z., Metin, C., Stoykova, A., Tarabykin, V., Price, D. J., Francis, F., et al. (2006). Comparative aspects of cerebral cortical development. Eur. J. Neurosci. 23, 921–934. doi: 10.1111/j.1460-9568.2006.04611.x
Molyneaux, B. J., Arlotta, P., Menezes, J. R., and Macklis, J. D. (2007). Neuronal subtype specification in the cerebral cortex. Nat. Rev. Neurosci. 8, 427–437. doi: 10.1038/nrn2151
Moore, D. R. (1982). Late onset of hearing in the ferret. Brain Res. 253, 309–311. doi: 10.1016/0006-8993(82)90698-9
Munoz-Fontela, C., Dowling, W. E., Funnell, S. G. P., Gsell, P. S., Riveros-Balta, A. X., Albrecht, R. A., et al. (2020). Animal models for COVID-19. Nature 586, 509–515. doi: 10.1038/s41586-020-2787-6
Neal, J., Takahashi, M., Silva, M., Tiao, G., Walsh, C. A., and Sheen, V. L. (2007). Insights into the gyrification of developing ferret brain by magnetic resonance imaging. J. Anat. 210, 66–77. doi: 10.1111/j.1469-7580.2006.00674.x
Noctor, S. C., Martinez-Cerdeno, V., Ivic, L., and Kriegstein, A. R. (2004). Cortical neurons arise in symmetric and asymmetric division zones and migrate through specific phases. Nat. Neurosci. 7, 136–144. doi: 10.1038/nn1172
Noctor, S. C., Palmer, S. L., Hasling, T., and Juliano, S. L. (1999). Interference with the development of early generated neocortex results in disruption of radial glia and abnormal formation of neocortical layers. Cereb. Cortex 9, 121–136. doi: 10.1093/cercor/9.2.121
Noctor, S. C., Palmer, S. L., McLaughlin, D. F., and Juliano, S. L. (2001). Disruption of layers 3 and 4 during development results in altered thalamocortical projections in ferret somatosensory cortex. J. Neurosci. 21, 3184–3195. doi: 10.1523/JNEUROSCI.21-09-03184.2001
Noctor, S. C., Scholnicoff, N. J., and Juliano, S. L. (1997). Histogenesis of ferret somatosensory cortex. J. Comp. Neurol. 387, 179–193. doi: 10.1002/(SICI)1096-9861(19971020)387:2<179::AID-CNE2>3.0.CO;2-Y
Oberst, P., Fievre, S., Baumann, N., Concetti, C., Bartolini, G., and Jabaudon, D. (2019). Temporal plasticity of apical progenitors in the developing mouse neocortex. Nature 573, 370–374. doi: 10.1038/s41586-019-1515-6
Ohshima, T., Ward, J. M., Huh, C. G., Longenecker, G., Veeranna, Pant, H. C., et al. (1996). Targeted disruption of the cyclin-dependent kinase 5 gene results in abnormal corticogenesis, neuronal pathology and perinatal death. Proc. Natl. Acad. Sci. U.S.A. 93, 11173–11178. doi: 10.1073/pnas.93.20.11173
O’Leary, M. A., Bloch, J. I., Flynn, J. J., Gaudin, T. J., Giallombardo, A., Giannini, N. P., et al. (2013). The placental mammal ancestor and the post-K-Pg radiation of placentals. Science 339, 662–667. doi: 10.1126/science.1229237
Osier, N. D., Korpon, J. R., and Dixon, C. E. (2015). “Controlled cortical impact model,” in Brain Neurotrauma: Molecular, Neuropsychological, and Rehabilitation Aspects, ed. F. H., Kobeissy (Boca Raton, FL: CRC Press).
Palmer, S. L., Noctor, S. C., Jablonska, B., and Juliano, S. L. (2001). Laminar specific alterations of thalamocortical projections in organotypic cultures following layer 4 disruption in ferret somatosensory cortex. Eur. J. Neurosci. 13, 1559–1571. doi: 10.1046/j.0953-816x.2001.01519.x
Pannier, S., Martinovic, J., Heuertz, S., Delezoide, A. L., Munnich, A., Schibler, L., et al. (2009). Thanatophoric dysplasia caused by double missense FGFR3 mutations. Am. J. Med. Genet. A 149A, 1296–1301. doi: 10.1002/ajmg.a.32880
Pattabiraman, K., Muchnik, S. K., and Sestan, N. (2020). The evolution of the human brain and disease susceptibility. Curr. Opin. Genet. Dev. 65, 91–97. doi: 10.1016/j.gde.2020.05.004
Peng, X., Alfoldi, J., Gori, K., Eisfeld, A. J., Tyler, S. R., Tisoncik-Go, J., et al. (2014). The draft genome sequence of the ferret (Mustela putorius furo) facilitates study of human respiratory disease. Nat. Biotechnol. 32, 1250–1255. doi: 10.1038/nbt.3079
Philipp, R., Distler, C., and Hoffmann, K. P. (2006). A motion-sensitive area in ferret extrastriate visual cortex: an analysis in pigmented and albino animals. Cereb. Cortex 16, 779–790. doi: 10.1093/cercor/bhj022
Poluch, S., Jablonska, B., and Juliano, S. L. (2008). Alteration of interneuron migration in a ferret model of cortical dysplasia. Cereb. Cortex 18, 78–92. doi: 10.1093/cercor/bhm032
Radtke-Schuller, S., Town, S. M., Yin, P., Elgueda, D., Schuller, G., Bizley, J. K., et al. (2020). Dorsal prefrontal and premotor cortex of the ferret as defined by distinctive patterns of thalamo-cortical projections. Brain Struct. Funct. 225, 1643–1667. doi: 10.1007/s00429-020-02086-7
Rakic, P. (1972). Mode of cell migration to the superficial layers of fetal monkey neocortex. J. Comp. Neurol. 145, 61–83. doi: 10.1002/cne.901450105
Rakic, P. (1988). Specification of cerebral cortical areas. Science 241, 170–176. doi: 10.1126/science.3291116
Rakic, P. (2009). Evolution of the neocortex: a perspective from developmental biology. Nat. Rev. Neurosci. 10, 724–735. doi: 10.1038/nrn2719
Rakic, P., and Lombroso, P. J. (1998). Development of the cerebral cortex: I. Forming the cortical structure. J. Am. Acad. Child Adolesc. Psychiatry 37, 116–117. doi: 10.1097/00004583-199801000-00026
Rana, S., Shishegar, R., Quezada, S., Johnston, L., Walker, D. W., and Tolcos, M. (2019). The Subplate: A potential driver of cortical folding? Cereb. Cortex 29, 4697–4708. doi: 10.1093/cercor/bhz003
Rash, B. G., Duque, A., Morozov, Y. M., Arellano, J. I., Micali, N., and Rakic, P. (2019). Gliogenesis in the outer subventricular zone promotes enlargement and gyrification of the primate cerebrum. Proc. Natl. Acad. Sci. U.S.A. 116, 7089–7094. doi: 10.1073/pnas.1822169116
Reid, C. B., Tavazoie, S. F., and Walsh, C. A. (1997). Clonal dispersion and evidence for asymmetric cell division in ferret cortex. Development 124, 2441–2450.
Reillo, I., and Borrell, V. (2012). Germinal zones in the developing cerebral cortex of ferret: ontogeny, cell cycle kinetics, and diversity of progenitors. Cereb. Cortex 22, 2039–2054. doi: 10.1093/cercor/bhr284
Reillo, I., de Juan Romero, C., Cardenas, A., Clasca, F., Martinez-Martinez, M. A., and Borrell, V. (2017). A complex code of extrinsic influences on cortical progenitor cells of higher mammals. Cereb. Cortex 27, 4586–4606. doi: 10.1093/cercor/bhx171
Reillo, I., de Juan Romero, C., Garcia-Cabezas, M. A., and Borrell, V. (2011). A role for intermediate radial glia in the tangential expansion of the mammalian cerebral cortex. Cereb. Cortex 21, 1674–1694. doi: 10.1093/cercor/bhq238
Rice, F. L., Gomez, C. M., Leclerc, S. S., Dykes, R. W., Moon, J. S., and Pourmoghadam, K. (1993). Cytoarchitecture of the ferret suprasylvian gyrus correlated with areas containing multiunit responses elicited by stimulation of the face. Somatosens. Mot. Res. 10, 161–188. doi: 10.3109/08990229309028830
Richard, M., Kok, A., de Meulder, D., Bestebroer, T. M., Lamers, M. M., Okba, N. M. A., et al. (2020). SARS-CoV-2 is transmitted via contact and via the air between ferrets. Nat. Commun. 11:3496. doi: 10.1038/s41467-020-17367-2
Rockland, K. S. (1985). Anatomical organization of primary visual cortex (area 17) in the ferret. J. Comp. Neurol. 241, 225–236. doi: 10.1002/cne.902410209
Romero, D. M., Bahi-Buisson, N., and Francis, F. (2018). Genetics and mechanisms leading to human cortical malformations. Semin. Cell Dev. Biol. 76, 33–75. doi: 10.1016/j.semcdb.2017.09.031
Roy, A., Christie, I. K., Escobar, G. M., Osik, J. J., Popovic, M., Ritter, N. J., et al. (2018). Does experience provide a permissive or instructive influence on the development of direction selectivity in visual cortex? Neural Dev. 13:16. doi: 10.1186/s13064-018-0113-x
Roy, A., Osik, J. J., Ritter, N. J., Wang, S., Shaw, J. T., Fiser, J., et al. (2016). Optogenetic spatial and temporal control of cortical circuits on a columnar scale. J. Neurophysiol. 115, 1043–1062. doi: 10.1152/jn.00960.2015
Roy, A., Wang, S., Meschede-Krasa, B., Breffle, J., and Van Hooser, S. D. (2020). An early phase of instructive plasticity before the typical onset of sensory experience. Nat. Commun. 11:11. doi: 10.1038/s41467-019-13872-1
Saito, K., Mizuguchi, K., Horiike, T., Dinh Duong, T. A., Shinmyo, Y., and Kawasaki, H. (2018). Characterization of the inner and outer fiber layers in the developing cerebral cortex of gyrencephalic ferrets. Cereb. Cortex 29, 4303–4311. doi: 10.1093/cercor/bhy312
Sanclemente-Alaman, I., Moreno-Jimenez, L., Benito-Martin, M. S., Canales-Aguirre, A., Matias-Guiu, J. A., Matias-Guiu, J., et al. (2020). Experimental models for the study of central nervous system infection by SARS-CoV-2. Front. Immunol. 11:2163. doi: 10.3389/fimmu.2020.02163
Sawada, K., and Watanabe, M. (2012). Development of cerebral sulci and gyri in ferrets (Mustela putorius). Congenit. Anom. 52, 168–175. doi: 10.1111/j.1741-4520.2012.00372.x
Schwerin, S. C., Chatterjee, M., Imam-Fulani, A. O., Radomski, K. L., Hutchinson, E. B., Pierpaoli, C. M., et al. (2018). Progression of histopathological and behavioral abnormalities following mild traumatic brain injury in the male ferret. J. Neurosci. Res. 96, 556–572. doi: 10.1002/jnr.24218
Schwerin, S. C., Hutchinson, E. B., Radomski, K. L., Ngalula, K. P., Pierpaoli, C. M., and Juliano, S. L. (2017). Establishing the ferret as a gyrencephalic animal model of traumatic brain injury: optimization of controlled cortical impact procedures. J. Neurosci. Methods 285, 82–96. doi: 10.1016/j.jneumeth.2017.05.010
Sellers, K. K., Yu, C., Zhou, Z. C., Stitt, I., Li, Y., Radtke-Schuller, S., et al. (2016). Oscillatory dynamics in the frontoparietal attention network during sustained attention in the ferret. Cell Rep. 16, 2864–2874. doi: 10.1016/j.celrep.2016.08.055
Shi, J., Wen, Z., Zhong, G., Yang, H., Wang, C., Huang, B., et al. (2020). Susceptibility of ferrets, cats, dogs, and other domesticated animals to SARS-coronavirus 2. Science 368, 1016–1020. doi: 10.1126/science.abb7015
Shinmyo, Y., Terashita, Y., Dinh Duong, T. A., Horiike, T., Kawasumi, M., Hosomichi, K., et al. (2017). Folding of the cerebral cortex requires Cdk5 in upper-layer neurons in gyrencephalic mammals. Cell Rep. 20, 2131–2143. doi: 10.1016/j.celrep.2017.08.024
Shitamukai, A., Konno, D., and Matsuzaki, F. (2011). Oblique radial glial divisions in the developing mouse neocortex induce self-renewing progenitors outside the germinal zone that resemble primate outer subventricular zone progenitors. J. Neurosci. 31, 3683–3695. doi: 10.1523/JNEUROSCI.4773-10.2011
Silva, C. G., Peyre, E., and Nguyen, L. (2019). Cell migration promotes dynamic cellular interactions to control cerebral cortex morphogenesis. Nat. Rev. Neurosci. 20, 318–329. doi: 10.1038/s41583-019-0148-y
Silver, D. L. (2016). Genomic divergence and brain evolution: how regulatory DNA influences development of the cerebral cortex. Bioessays 38, 162–171. doi: 10.1002/bies.201500108
Smart, I. H., and McSherry, G. M. (1986a). Gyrus formation in the cerebral cortex in the ferret. I. Description of the external changes. J. Anat. 146, 141–152.
Smart, I. H., and McSherry, G. M. (1986b). Gyrus formation in the cerebral cortex of the ferret. II. Description of the internal histological changes. J. Anat. 147, 27–43.
Smart, I. H., Dehay, C., Giroud, P., Berland, M., and Kennedy, H. (2002). Unique morphological features of the proliferative zones and postmitotic compartments of the neural epithelium giving rise to striate and extrastriate cortex in the monkey. Cereb. Cortex 12, 37–53. doi: 10.1093/cercor/12.1.37
Smith, G. B., and Fitzpatrick, D. (2016). Viral injection and cranial window implantation for in vivo two-photon imaging. Methods Mol. Biol. 1474, 171–185. doi: 10.1007/978-1-4939-6352-2_10
Smith, W., Andrewes, C. H., and Laidlaw, P. P. (1933). A virus obtained from influenza patients. Lancet 8, 66–68. doi: 10.1016/S0140-6736(00)78541-2
Sousa, A. M. M., Meyer, K. A., Santpere, G., Gulden, F. O., and Sestan, N. (2017). Evolution of the human nervous system function, structure, and development. Cell 170, 226–247. doi: 10.1016/j.cell.2017.06.036
Stittelaar, K. J., de Waal, L., van Amerongen, G., Veldhuis Kroeze, E. J., Fraaij, P. L., van Baalen, C. A., et al. (2016). Ferrets as a novel animal model for studying human respiratory syncytial virus infections in immunocompetent and immunocompromised hosts. Viruses 8:168. doi: 10.3390/v8060168
Sun, X., Sui, H., Fisher, J. T., Yan, Z., Liu, X., Cho, H. J., et al. (2010). Disease phenotype of a ferret CFTR-knockout model of cystic fibrosis. J. Clin. Invest. 120, 3149–3160. doi: 10.1172/JCI43052
Sun, X., Yan, Z., Yi, Y., Li, Z., Lei, D., Rogers, C. S., et al. (2008). Adeno-associated virus-targeted disruption of the CFTR gene in cloned ferrets. J. Clin. Invest. 118, 1578–1583. doi: 10.1172/JCI34599
Tao, J. D., Barnette, A. R., Griffith, J. L., Neil, J. J., and Inder, T. E. (2012). Histopathologic correlation with diffusion tensor imaging after chronic hypoxia in the immature ferret. Pediatr. Res. 71, 192–198. doi: 10.1038/pr.2011.32
Tardif, S. D., Smucny, D. A., Abbott, D. H., Mansfield, K., Schultz-Darken, N., and Yamamoto, M. E. (2003). Reproduction in captive common marmosets (Callithrix jacchus). Comp. Med. 53, 364–368.
Taverna, E., Götz, M., and Huttner, W. B. (2014). The cell biology of neurogenesis: toward an understanding of the development and evolution of the neocortex. Annu. Rev. Cell Dev. Biol. 30, 465–502. doi: 10.1146/annurev-cellbio-101011-155801
Telley, L., Agirman, G., Prados, J., Amberg, N., Fievre, S., Oberst, P., et al. (2019). Temporal patterning of apical progenitors and their daughter neurons in the developing neocortex. Science 364:eaav2522. doi: 10.1126/science.aav2522
Toda, T., Shinmyo, Y., Dinh Duong, T. A., Masuda, K., and Kawasaki, H. (2016). An essential role of SVZ progenitors in cortical folding in gyrencephalic mammals. Sci. Rep. 6:29578. doi: 10.1038/srep29578
Turrero Garcia, M., Chang, Y., Arai, Y., and Huttner, W. B. (2015). S-phase duration is the main target of cell cycle regulation in neural progenitors of developing ferret neocortex. J. Comp. Neurol. 524, 456–470. doi: 10.1002/cne.23801
Vaid, S., and Huttner, W. B. (2020). Transcriptional regulators and human-specific/primate-specific genes in neocortical neurogenesis. Int. J. Mol. Sci. 21:4614. doi: 10.3390/ijms21134614
Van Hooser, S. D., Li, Y., Christensson, M., Smith, G. B., White, L. E., and Fitzpatrick, D. (2012). Initial neighborhood biases and the quality of motion stimulation jointly influence the rapid emergence of direction preference in visual cortex. J. Neurosci. 32, 7258–7266. doi: 10.1523/JNEUROSCI.0230-12.2012
Voigt, T. (1989). Development of glial cells in the cerebral wall of ferrets: direct tracing of their transformation from radial glia into astrocytes. J. Comp. Neurol. 289, 74–88. doi: 10.1002/cne.902890106
Voigt, T., De Lima, A. D., and Beckmann, M. (1993). Synaptophysin immunohistochemistry reveals inside-out pattern of early synaptogenesis in ferret cerebral cortex. J. Comp. Neurol. 330, 48–64. doi: 10.1002/cne.903300105
Wang, X., Tsai, J. W., LaMonica, B., and Kriegstein, A. R. (2011). A new subtype of progenitor cell in the mouse embryonic neocortex. Nat. Neurosci. 14, 555–561. doi: 10.1038/nn.2807
Ware, M. L., Tavazoie, S. F., Reid, C. B., and Walsh, C. A. (1999). Coexistence of widespread clones and large radial clones in early embryonic ferret cortex. Cereb. Cortex 9, 636–645. doi: 10.1093/cercor/9.6.636
Weliky, M., Bosking, W. H., and Fitzpatrick, D. (1996). A systematic map of direction preference in primary visual cortex. Nature 379, 725–728. doi: 10.1038/379725a0
Wess, J. M., Isaiah, A., Watkins, P. V., and Kanold, P. O. (2017). Subplate neurons are the first cortical neurons to respond to sensory stimuli. Proc. Natl. Acad. Sci. U.S.A. 114, 12602–12607. doi: 10.1073/pnas.1710793114
White, L. E., and Fitzpatrick, D. (2007). Vision and cortical map development. Neuron 56, 327–338. doi: 10.1016/j.neuron.2007.10.011
White, L. E., Coppola, D. M., and Fitzpatrick, D. (2001). The contribution of sensory experience to the maturation of orientation selectivity in ferret visual cortex. Nature 411, 1049–1052. doi: 10.1038/35082568
Wong, F. K., Fei, J. F., Mora-Bermudez, F., Taverna, E., Haffner, C., Fu, J., et al. (2015). Sustained Pax6 expression generates primate-like basal radial glia in developing mouse neocortex. PLoS Biol. 13:e1002217. doi: 10.1371/journal.pbio.1002217
Workman, A. D., Charvet, C. J., Clancy, B., Darlington, R. B., and Finlay, B. L. (2013). Modeling transformations of neurodevelopmental sequences across mammalian species. J. Neurosci. 33, 7368–7383. doi: 10.1523/JNEUROSCI.5746-12.2013
Xing, L., Kalebic, N., Namba, T., Vaid, S., Wimberger, P., and Huttner, W. B. (2020). Serotonin receptor 2A activation promotes evolutionarily relevant basal progenitor proliferation in the developing neocortex. Neuron 108, 1113–1129.e6. doi: 10.1016/j.neuron.2020.09.034
Yamazaki, H., Sekiguchi, M., Takamatsu, M., Tanabe, Y., and Nakanishi, S. (2004). Distinct ontogenic and regional expressions of newly identified Cajal-Retzius cell-specific genes during neocorticogenesis. Proc. Natl. Acad. Sci. U.S.A. 101, 14509–14514. doi: 10.1073/pnas.0406295101
Yu, M., Sun, X., Tyler, S. R., Liang, B., Swatek, A. M., Lynch, T. J., et al. (2019). Highly efficient transgenesis in ferrets using CRISPR/Cas9-mediated homology-independent insertion at the ROSA26 locus. Sci. Rep. 9:1971. doi: 10.1038/s41598-018-37192-4
Zecevic, N., Chen, Y., and Filipovic, R. (2005). Contributions of cortical subventricular zone to the development of the human cerebral cortex. J. Comp. Neurol. 491, 109–122. doi: 10.1002/cne.20714
Zhang, Q., Shi, J., Deng, G., Guo, J., Zeng, X., He, X., et al. (2013). H7N9 influenza viruses are transmissible in ferrets by respiratory droplet. Science 341, 410–414. doi: 10.1126/science.1240532
Zhou, Z. C., Salzwedel, A. P., Radtke-Schuller, S., Li, Y., Sellers, K. K., Gilmore, J. H., et al. (2016a). Resting state network topology of the ferret brain. Neuroimage 143, 70–81. doi: 10.1016/j.neuroimage.2016.09.003
Zhou, Z. C., Yu, C., Sellers, K. K., and Frohlich, F. (2016b). Dorso-lateral frontal cortex of the ferret encodes perceptual difficulty during visual discrimination. Sci. Rep. 6:23568. doi: 10.1038/srep23568
Keywords: ferret (Mustela putorius furo), neocortex, brain development, brain evolution, cortical folding, neural stem/progenitor cells
Citation: Gilardi C and Kalebic N (2021) The Ferret as a Model System for Neocortex Development and Evolution. Front. Cell Dev. Biol. 9:661759. doi: 10.3389/fcell.2021.661759
Received: 31 January 2021; Accepted: 01 April 2021;
Published: 29 April 2021.
Edited by:
Benedetta Artegiani, Princess Maxima Center for Pediatric Oncology, NetherlandsReviewed by:
Fernando Garcia-Moreno, Achucarro Basque Center for Neuroscience, SpainSimon Hippenmeyer, Institute of Science and Technology Austria (IST Austria), Austria
Copyright © 2021 Gilardi and Kalebic. This is an open-access article distributed under the terms of the Creative Commons Attribution License (CC BY). The use, distribution or reproduction in other forums is permitted, provided the original author(s) and the copyright owner(s) are credited and that the original publication in this journal is cited, in accordance with accepted academic practice. No use, distribution or reproduction is permitted which does not comply with these terms.
*Correspondence: Nereo Kalebic, bmVyZW8ua2FsZWJpY0BmaHQub3Jn