- 1Menzies Health Institute Queensland, Griffith University, Southport, QLD, Australia
- 2Clem Jones Centre for Neurobiology and Stem Cell Research, Griffith University, Nathan, QLD, Australia
- 3Griffith Institute for Drug Discovery, Griffith University, Nathan, QLD, Australia
The central nervous system (CNS) has very limited capacity to regenerate after traumatic injury or disease. In contrast, the peripheral nervous system (PNS) has far greater capacity for regeneration. This difference can be partly attributed to variances in glial-mediated functions, such as axon guidance, structural support, secretion of growth factors and phagocytic activity. Due to their growth-promoting characteristic, transplantation of PNS glia has been trialed for neural repair. After peripheral nerve injuries, Schwann cells (SCs, the main PNS glia) phagocytose myelin debris and attract macrophages to the injury site to aid in debris clearance. One peripheral nerve, the olfactory nerve, is unique in that it continuously regenerates throughout life. The olfactory nerve glia, olfactory ensheathing cells (OECs), are the primary phagocytes within this nerve, continuously clearing axonal debris arising from the normal regeneration of the nerve and after injury. In contrast to SCs, OECs do not appear to attract macrophages. SCs and OECs also respond to and phagocytose bacteria, a function likely critical for tackling microbial invasion of the CNS via peripheral nerves. However, phagocytosis is not always effective; inflammation, aging and/or genetic factors may contribute to compromised phagocytic activity. Here, we highlight the diverse roles of SCs and OECs with the focus on their phagocytic activity under physiological and pathological conditions. We also explore why understanding the contribution of peripheral glia phagocytosis may provide us with translational strategies for achieving axonal regeneration of the injured nervous system and potentially for the treatment of certain neurological diseases.
Introduction
Phagocytosis plays a crucial role in the development, growth and maintenance of both the CNS and the PNS. Some of the key phagocytic events include clearance of apoptotic neurons and cells undergoing necrosis or programmed cell death, as well as clearance of debris arising from pruning of axons and dendrites (Ashwell, 1990; Bishop et al., 2004; Sierra et al., 2010; Chung et al., 2013; Squarzoni et al., 2014; Amaya et al., 2015; Nazareth et al., 2015a, 2020; Mosser et al., 2017). Aging impairs efficient phagocytosis, and diminished phagocytosis has been linked to several CNS pathologies, including neurodegenerative diseases such as Alzheimer’s disease, Parkinson’s disease, multiple sclerosis, and amyotrophic lateral sclerosis (reviewed by Jung and Chung, 2018; Galloway et al., 2019; Tremblay et al., 2019; Valles et al., 2019). Impaired phagocytosis is also a problem after CNS injuries, including spinal cord injury (SCI) and traumatic brain injury (Miklossy and Van der Loos, 1991; George and Griffin, 1994; Becerra et al., 1995; Liu et al., 1995; Buss et al., 2004; Kotter et al., 2006). After peripheral nerve injury, however, removal of cellular and myelin debris is efficient and strongly aids regeneration (Stoll et al., 1989; Fernandezvalle et al., 1995; Liu et al., 1995; Perry et al., 1995; Niemi et al., 2013; Soares et al., 2014). Phagocytosis is also vital for protecting the nervous system from infection, with increased CNS infection rate or severity reported in immunocompromised patients (Nau et al., 2014).
The ability of a phagocyte to remove unwanted, dying, or aberrant cells is a complex and highly dynamic process with extremely precise recognition and degradation at the molecular level and involving multiple receptors and co-receptors. Due to the presence of the blood-brain barrier (BBB) and the glia limitans layer, the immune cell composition differs between the CNS and the PNS. In the past, the CNS was thought to be immunoprivileged, but it is now well known that this is not the case and instead the brain exhibits compartmentalized immune responses (Korin et al., 2017, 2018; Van Hove et al., 2019). With the re-discovery of the meningeal lymphatic system, we now know that these vessels serve as a direct conduit between the CNS and the peripheral immune system (Aspelund et al., 2015; Louveau et al., 2015; Absinta et al., 2017). Under normal physiological conditions, the CNS parenchyma is largely devoid of peripheral immune cells and most of the phagocytic activity is mediated by resident glia (microglia and astrocytes). Microglia are considered the macrophages of the brain and are the key CNS phagocytes. Small populations of T-cells, B-cells, NK-cells and dendritic cells also exist in the CNS, with distinct roles in the compartmentalized immune responses (Korin et al., 2017). The meninges surrounding the CNS also contain circulating immune cells which can enter the CNS after disruption of the BBB (Prinz and Priller, 2017; Papadopoulos et al., 2020).
Similar to the BBB, the peripheral nerves are surrounded by a physiological barrier known as Blood Nerve Barrier (BNB) that separates the peripheral nerve axons from the blood stream. This barrier is similar to the BBB with the exception of the astrocytes that form the glia limitans (Kuczynski, 1980; Fraher et al., 1988; Nugent et al., 1991; Nazareth et al., 2019). The outermost layer of this barrier consists of an epineurium that is mainly made up of collagen fibers, blood vessels and adipocytes (Thomas, 1963; Gamble and Eames, 1964; Ushiki and Ide, 1990; Stolinski, 1995). This encloses the perineurium consisting of collagen fibers and concentric layers of cells called perineurial cells (Shanthaveerappa and Bourne, 1963; Burkel, 1967; Akert et al., 1976). The perineurium in turn envelops the endoneurium consisting of Schwann cell-axonal structures, fibroblasts and resident macrophages (Shanthaveerappa and Bourne, 1963; Bonetti et al., 1993; Reina et al., 2015; Wolbert et al., 2020). After the breakdown of the BNB in the case of an injury or infection, peripheral immune cells (particularly macrophages) are recruited to the peripheral nerves (Perry et al., 1987; Taskinen and Roytta, 1997; Tofaris et al., 2002; Mueller et al., 2003; Beirowski et al., 2004). The peripheral nerve resident macrophages known as endoneurial macrophages, along with the glial cells contribute to the initial phagocytic clearance and aid in nerve-regeneration following injury (Mueller et al., 2003; Wang et al., 2020). However, in the unperturbed PNS, the resident glia are the main phagocytes (Bishop et al., 2004; Wu et al., 2009; Su et al., 2013; Nazareth et al., 2015a). The phagocytic activity of these glia is indispensable for development and normal physiological homeostatic maintenance. Glia are also one of the first responders to PNS injury (Chuah et al., 1995; Perry et al., 1995; Niemi et al., 2013; Su et al., 2013; Nazareth et al., 2015a). A list of phagocytic receptors and co-receptors involved in glia phagocytosis is described in Table 1.
Based on the region of the nervous system they are present in, glia are classified as CNS and PNS glia, with specific roles tailored to their anatomical location, including distinct roles in regeneration and repair. While the PNS can regenerate, unless the injury is large in particular large-gap injuries, i.e., greater than 20 mm (Sulaiman and Gordon, 2000; Lopez-Cebral et al., 2017), regeneration after CNS injury is very limited (Miklossy and Van der Loos, 1991; Kotter et al., 2006; Vargas and Barres, 2007; Lutz and Barres, 2014; Stuve and Zettl, 2014; Mietto et al., 2015). In addition to differences in intrinsic properties of PNS vs. CNS neurons (Fawcett and Verhaagen, 2018), the local environment is important for the differences in regeneration between the CNS and the PNS. In particular, the inflammatory environment (Fitch and Silver, 2008) and the extracellular matrix composition (Barros et al., 2011) make the CNS less growth-permissive than the PNS. The functions of peripheral glia are also considered crucial for the capacity for PNS regeneration. These functions include neurotrophic and physical support, the ability to effectively phagocytose and clear debris, as well as modulation of the inflammatory environment (reviewed by Fregnan et al., 2012; Lutz and Barres, 2014; Barton et al., 2017; Yao et al., 2018; Gilmour et al., 2020).
However, phagocytosis does not only involve clearance of dying and damaged cells, i.e., “self,” but also elimination of “non-self” targets, particularly infectious agents (Vincent et al., 2007; Mariani and Kielian, 2009; Esen and Kielian, 2012; Masaki et al., 2014). The nervous system (both the PNS and CNS) is well protected by the physical and immunological barriers of skin and mucosae, and the CNS is further protected by other barriers including the BBB and glia limitans. There are, however, microbes that are capable of crossing these barriers. The nerves that extend between the nasal cavity and the brain, the trigeminal and olfactory nerves, constitute direct paths by which microbes may reach the CNS, however, phagocytic glia of these nerves are thought to eliminate most infectious agents. Some bacteria, viruses and parasites, however, have been shown to enter and infect the CNS via these nerves (reviewed by Dando et al., 2014; Forrester et al., 2018) and CNS invasion by certain infectious agents has been linked to the development of neurodegenerative diseases (reviewed by Itzhaki et al., 2004; De Chiara et al., 2012; Balin et al., 2018; Dehhaghi et al., 2018). Understanding phagocytosis of pathogens by peripheral glia may enable us to explore ways to protect the nervous system from infection via peripheral cranial nerves.
Here, we provide an overview of the established and emerging knowledge of the phagocytic roles of two key peripheral glial cell types, Schwann cells (SCs) and olfactory ensheathing cells (OECs). We focus on these glia as they are being trialed for cell transplantation therapies due to their regenerative potential and because they are important for protecting the CNS against infection via peripheral nerves. We have previously compared various functions of OECs and SCs that aid neve-regeneration including secretion of growth factors, immunomodulatory properties, and phagocytic ability (Barton et al., 2017; Wright et al., 2018). However, there is a lack of in-depth studies focusing on the phagocytic function of these cells particularly the molecular and cellular mechanisms involved in this intricate process. Hence, we provide a comprehensive discussion about SCs and OEC phagocytosis during development, normal physiological conditions and in pathologies including infection. We also briefly introduce the origin and physiological roles of these glia. Finally, we discuss the implications of modulating the phagocytic behavior of SCs and OECs for the development of therapies.
Glia of the Peripheral Nervous System
The glia of the PNS originate from the neural crest and support peripheral nerves by providing physical and trophic support, myelination and maintenance of homeostasis, both under physiological conditions and after injury (Ledouarin and Smith, 1988; Ledouarin et al., 1991; Buchstaller et al., 2004; Barraud et al., 2010; Suzuki and Osumi, 2015; Perera et al., 2020). The key PNS glia are (1) SCs that populate most peripheral nerves, (2) OECs, which are present in the primary olfactory nervous system (olfactory nerve and outer layer of the olfactory bulb), (3) satellite cells in peripheral ganglia, (4) enteric glia in the intestinal tract.
Schwann cells are the most abundant and well-studied glia of the PNS. SCs and neurons are intimately connected, with symbiotic dependence on each other for growth, maturation and survival. While SCs require signals from axons to undergo differentiation, axons in turn require SCs for trophic and metabolic support, as well as for normal conduction velocity (Bhattacharyya et al., 1994; Jessen et al., 1994; Dong et al., 1995; Gavrilovic et al., 1995; Grinspan et al., 1996; Woodhoo et al., 2004; Voas et al., 2009; Viader et al., 2011; Beirowski et al., 2014; Xiao et al., 2015). SCs also have active roles in aiding repair after injury (Stoll et al., 1989; Fernandezvalle et al., 1995; Perry et al., 1995; Hirata and Kawabuchi, 2002; Arthur-Farraj et al., 2012; Napoli et al., 2012; Weiss et al., 2016).
Olfactory ensheathing cells exhibit many similar characteristics to SCs, but also have distinct roles due to the constant regeneration of the olfactory nerve (Graziadei and Graziadei, 1979; Doucette, 1989, 1990; Mackaysim and Kittel, 1991). Both SCs and OECs respond to injury by phagocytosing and clearing debris, which is important for repair (Su et al., 2013; Nazareth et al., 2015a; Lutz et al., 2017). While SCs participate in the initial clearance of cellular and myelin debris, they also recruit professional phagocytes, including macrophages and neutrophils, by upregulating secretion of a range of inflammatory molecules. The recruited cells then conduct the majority of the phagocytosis to clear the injury site (Fregnan et al., 2012; Hartlehnert et al., 2017; Lindborg et al., 2017). However peripheral nerve injuries in model organisms like Drosophila and zebrafish reveal that macrophage recruitment to the injury site may be independent of SCs and that macrophages might arrive to the site of injury earlier than previously thought (Rosenberg et al., 2012; Soares et al., 2014). Live-cell imaging after in vivo injuries in zebrafish has demonstrated that cells like perineurial glia also participate in the initial debris clean up (Lewis and Kucenas, 2014). After olfactory nerve injury, however, OECs appear to be the main phagocytes and do not recruit macrophages (at least not in large numbers) (Chuah et al., 1995; Su et al., 2013; Nazareth et al., 2015a); in fact, OECs repel macrophages in co-culture (Wright et al., 2020).
Due to their capacity to remove cell debris and to promote neuronal regeneration, both SCs and OECs have been explored as candidates to treat CNS injuries, in particular spinal cord injury, via cell transplantation therapies, with variable outcomes (reviewed by Kocsis and Waxman, 2007; Kanno et al., 2015; Yao et al., 2018; Reshamwala et al., 2019). Transplantation of these glia has also been trialed for improving the rate of PNS regeneration which is ∼1 mm/day (Sulaiman and Gordon, 2000) and for repairing large peripheral nerve injuries (Chen et al., 2006; Radtke et al., 2011; Rodrigues et al., 2012; Xia et al., 2019). In addition to developmental origin, SCs and OECs share many morphological and functional properties. However, they also display distinct differences in their interactions with axons and astrocytes, migratory properties, transcriptomic profile and innate immune functions (Lakatos et al., 2000; Li et al., 2005; Vincent et al., 2005, 2007; Nazareth et al., 2020; Perera et al., 2020). These differences between the two cell types are likely to also influence their therapeutic potential. A better understanding of the functional differences between SCs and OECs will help in the design of better therapeutic strategies that can enhance the neural repair-favorable properties of these glia. One function in particular need of further examination is the phagocytic activity of the cells (Barton et al., 2017; Wright et al., 2018).
SCs: Origin and Physiology
After originating in the neural crest, SCs migrate out with the developing nerves from the neural trunk (Rickmann et al., 1985; Loring and Erickson, 1987; Ledouarin and Smith, 1988). These cells go through three key stages of development: (1) SC precursors, present during early embryonic development [embryonic day (E) 14-15 in rats, E13-14 in mice]; (2) immature SCs, existing during late embryonic development (E15-17 in rats, E13-15 in mice) (Jessen et al., 1994; Dong et al., 1995, 1999; Gavrilovic et al., 1995; Woodhoo et al., 2004); and (3) mature SCs that are present postnatally, of which there are several subtypes [the key subtypes being (i) myelinating SCs, (ii) non-myelinating SCs, and (iii) repair SCs (Figure 1)].
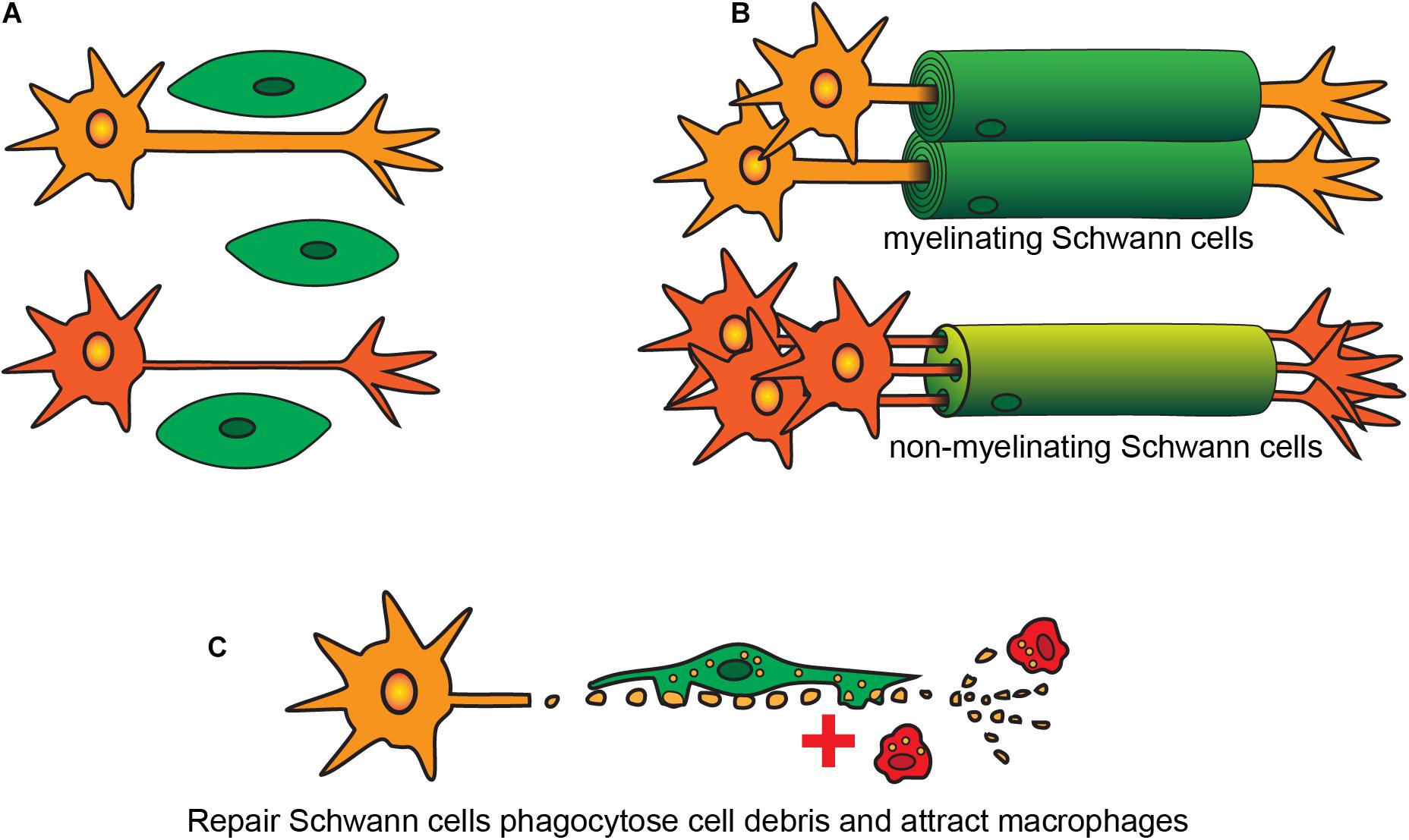
Figure 1. Developmental stages and types of SCs. (A) Immature SCs originate during embryogenesis and interact with different sized axons. (B) SCs become either myelinating or non-myelinating type depending on the size of the axons. (C) After injury both myelinating and non-myelinating SCs revert into a repair phenotype to phagocytose cell debris and to recruit macrophages.
In both developing and adult peripheral nerves, myelination and continuous maintenance of myelin architecture around axons is indispensable for conduction of nerve impulses. On interaction with axons, immature SCs undergo numerous phenotypic changes and exit the cell cycle to form myelinating or non-myelinating SCs. Whether SCs that contact axons become myelinating or non-myelinating (the latter also known as Remak cells), depends on the axons they contact (Webster et al., 1973). The process of contacting axons is known as radial sorting, where immature SCs extend their lamellae and contact a bundle of unmyelinated axons. For axons of diameter >1 μm, the SCs establish a 1:1 contact ratio and begin myelination of these large diameter axons (Webster, 1971; Webster et al., 1973; Figure 1), with the myelin consisting of many layers of SC membranes which insulate the axons and increase conduction speed dramatically. Small-diameter axons, such as those of nociceptive neurons, remain unmyelinated and SCs contacting these axons instead become non-myelinating. In some circumstances, the SCs ensheathe multiple small-diameter axons and form an unmyelinated structure called a Remak bundle (Webster et al., 1973). However, in mammals, most non-myelinated axons undergo radial sorting with non-myelinating SCs maintaining a 1:1 contact with the small-caliber axons (Berthold et al., 2005; Sharghi-Namini et al., 2006).
Perisynaptic SCs are non-myelinating SCs present in the distal end of motor nerve terminals in the neuromuscular junctions. Their key roles are similar to those of astrocytes in the CNS, that is, modulation of synaptic activity (Robitaille, 1998; Pinard et al., 2003). After injury, both myelinating and non-myelinating SCs revert into a repair phenotype, which is discussed in the next section.
SC Phagocytosis in Normal Physiological Conditions
Whilst the roles of SCs in neuronal support and myelination have been well studied, their phagocytic roles during development and adulthood are largely unknown. During development, more neurons are born than required, and the neurons also produce more branches than needed for effective connectivity. Excess neurons subsequently undergo apoptosis, which requires rapid clearance to prevent inflammation and antigen presentation (Dekkers et al., 2013). Whilst the contribution of SC-mediated phagocytosis to clearance of these apoptotic bodies is unknown, other peripheral glia phagocytose apoptotic neurons, including satellite glia in the developing peripheral ganglia (Wu et al., 2009) and OECs in the developing olfactory nerve (Nazareth et al., 2015a). Sequencing studies show that SCs possess several phagocytic receptors, including Tyro3-, Mertk-, and Axl- receptors (TAM receptors) and multiple EGF-like-domains-10 (MEGF10) that are upregulated after injury (Napoli et al., 2012; Weiss et al., 2016; Lutz et al., 2017). In the CNS of Drosophila, glia utilize the phagocytic receptor Draper (mammalian orthologue MEGF10), to engulf apoptotic neurons along with pruning of axonal and dendritic debris through various stages of development (Freeman et al., 2003; Awasaki et al., 2006). In rodents, astrocytes and microglia prune synapses and phagocytose apoptotic cells using TAM and MEGF10 receptors, both during development and in adult brains (Chung et al., 2013; Fourgeaud et al., 2016; Iram et al., 2016).Thus, it is likely that SCs can also clear apoptotic corpses and synapses and are not only phagocytic after injury but during normal physiological conditions, in particular during certain developmental stages.
Studies in Drosophila have also provided an insight particularly into somatoseonsory axons in contact with the skin. While glia are the main phagocytes responsible for debris and apoptotic neuron clearance in the developing Drosophila (Freeman et al., 2003; Awasaki and Ito, 2004; Watts et al., 2004), debris generated during dendrite pruning of sensory axons, both during development and after an injury, is phagocytosed by epidermal cells and not peripheral glia or macrophages (Han et al., 2014). Similar findings were also reported in zebrafish where epidermal cells were the primary phagocytes that cleared not only somatosensory axon debris after an injury but also peripheral axons that were misdirected to the skin (Rasmussen et al., 2015). Ablating both macrophages and peripheral glia did not affect debris clearance in this model (Rasmussen et al., 2015). In comparison, studies in the developing mammalian PNS particularly concerning phagocytic clearance are lacking. Identification of the key phagocytes may provide a better understanding of cells involved in maintaining homeostasis in the PNS.
In neuromuscular junctions, perisynaptic SCs are involved in pruning of synapses and remodeling during normal development in mammals (Bishop et al., 2004; Zuo and Bishop, 2008; Monk et al., 2015). The many axons forming connections with muscle cells during prenatal development later retract and remodel their processes (Herrera and Zeng, 2003; Wyatt and Balice-Gordon, 2003) with the retracting axons shedding structures termed axosomes, which contain synaptic organelles (Bishop et al., 2004). Perisynaptic SCs engulf the axosomes, and lysosome-bound axosomes are typically observed within perisynaptic SCs during early postnatal development (Bishop et al., 2004; Song et al., 2008). In addition to engulfing axosomes, perisynaptic SCs (and not macrophages) constitute the main cells responsible for axon pruning at the neuromuscular junction (Bishop et al., 2004; Song et al., 2008; Zuo and Bishop, 2008). Like mammals, synapse remodeling in the neuromuscular junction occurs as a part of Drosophila development. During this process there is generation of “waste” including presynaptic membrane shedding that generates debris and undifferentiated synaptic boutons that fail to mature (Schuster et al., 1996; Ataman et al., 2008; Fuentes-Medel et al., 2009). Unlike mammals, along with peripheral glial present in the neuromuscular junction (Awasaki and Ito, 2004) muscle cells also participate in phagocytosis of debris. Both glia and muscle cells utilize the Drosophila phagocytic receptor, Draper and dCed-6 (mammalian orthologue engulfment adaptor PTB Domain containing-1 (GULP); an adaptor protein) (Fuentes-Medel et al., 2009). These pathways have been previously implicated in the glial cell phagocytosis of apoptotic neurons and debris during CNS development both in insects and mammals (Awasaki and Ito, 2004; Awasaki et al., 2006; Chung et al., 2013; Iram et al., 2016). Interestingly each cell type had a specific target preference, with glia primarily responsible for presynaptic debris engulfment and muscle cells that of synaptic boutons (Fuentes-Medel et al., 2009).
After injury, SCs display both phagocytic and autophagic (“engulfment of self”) behaviors, which are covered in the next section. Whether SCs use both phagocytosis and autophagy to maintain myelin homeostasis during normal development and adulthood is unknown, however, at least autophagy appears to be involved. SCs have been shown to regulate the thickness of myelin sheaths during maturation of peripheral nerves both pre- and postnatally using autophagy (Jang et al., 2015). Transgenic mice, in which the gene encoding a protein required for autophagosome formation (autophagy related protein 7; ATG7), has been selectively knocked out in SCs, display hypermyelination in sciatic nerves resulting in abnormal peripheral nerve function postnatally (Gomez-Sanchez et al., 2015). Thus, SC phagocytosis is crucial during pre- and postnatal development, and potentially also for maintaining certain homeostatic functions in adult life.
SC Phagocytosis in Pathological Conditions and Aging
Peripheral Nerve Injury
Most studies of SC phagocytosis to date have focused on responses to peripheral nerve injury, where they are important for the rapid clearance of cellular and myelin debris (Stoll et al., 1989; Perry et al., 1995; Hirata and Kawabuchi, 2002; Niemi et al., 2013; Gomez-Sanchez et al., 2015; Jang et al., 2016; Lutz et al., 2017). This occurs in a step-by-step process that has been well described, though the intricate mechanisms are still being defined. After peripheral nerve injury, the nerve distal to the site of injury undergoes disintegration, termed Wallerian degeneration, as it was first studied by Augustus Waller in Volney (1851). Destruction of axons occurs due to the initial mechanical trauma and then largely by action of calcium proteases (Touma et al., 2007). The presence of degraded axons, along with the loss of axon-glia contact, causes SCs to re-enter the cell cycle and undergo rapid proliferation (Fernandezvalle et al., 1995; Parkinson et al., 2004; Kobayashi et al., 2012; Yi et al., 2019; Welleford et al., 2020). However, the mechanisms governing this proliferation is different from that observed during development (Kim et al., 2000; Atanasoski et al., 2006). It was earlier thought that after injury, SCs merely undergo de-differentiation and transform from the myelinating/non-myelinating phenotype into an immature phenotype (reviewed by Chen et al., 2007; Jessen and Mirsky, 2008, 2016; Quintes and Brinkmann, 2017). It has, however, been shown that SCs responding to injury undergo reprogramming to down-regulate genes required for myelination and axon support, and instead up-regulate genes involved in repair and regeneration (Nagarajan et al., 2002; Bosse et al., 2006; Arthur-Farraj et al., 2012, 2017; Weiss et al., 2016; Clements et al., 2017).
The transcription factor c-Jun constitutes the main regulator of this process (Arthur-Farraj et al., 2012, 2013; Mirsky et al., 2013; Weiss et al., 2016). These reprogrammed cells constitute a distinct SC subtype – the repair SC (reviewed by Jessen et al., 2015; Jessen and Mirsky, 2016, 2019; Balakrishnan et al., 2021). Repair SCs also undergo morphological changes and are seven to ten-fold longer than immature SCs. The increase in length facilitates the formation of Büngner’s bands, which are “tracks” formed by the repair SCs surrounded by newly formed endoneurial (basement membrane) tubes, along which regenerating axons can extend and reconnect (Gomez-Sanchez et al., 2017).
Importantly, repair SCs also facilitate efficient regeneration by rapidly clearing myelin- and cell debris, by both phagocytosis and autophagy (as the myelin sheets are part of the cells themselves) (Gomez-Sanchez et al., 2015; Jang et al., 2016; Weiss et al., 2016; Lutz et al., 2017). Debris clearance occurs in peripheral nerves around day two post-injury, with around 70-80% debris cleared at 8-10 days after injury (Perry et al., 1987; Stoll et al., 1989; Soares et al., 2014). The role of SCs in debris clearance has been a source of debate over the past century. In the past, some investigators believed that SCs do not help in phagocytosis at all and that infiltrating macrophages play the major role in debris clearance (Liu, 1974; Crang and Blakemore, 1986). Other investigators suggested that this clearance may solely be a SC-mediated event (Nathaniel and Pease, 1963; Satinsky et al., 1964; Fernandezvalle et al., 1995). It is now known that the initial clearance is carried out by SCs, with myelin ovoids detected inside the cells 2 days post-injury (Hirata and Kawabuchi, 2002; Jung et al., 2011). The SCs secrete inflammatory cytokines such as interleukin-6 (IL-6), monocyte chemoattractant protein-1 (MCP-1), tumor necrosis factor-α (TNF-α), and IL-1α (Fregnan et al., 2012) which results in recruitment of macrophages to the site of injury. As hematogenous macrophages enter the injury site later, it is estimated that the initial 40-50% of the debris clearance is performed by SCs along with help from the resident endoneurial macrophages (Perry et al., 1995; Mueller et al., 2003). The exact time of arrival of hematogenous macrophages is unknown. Previous work in rodents reports macrophage arrival at the lesion site almost 2-3 days after injury, with numbers peaking between day 7 and 14 (Perry et al., 1987; Stoll et al., 1989; Taskinen and Roytta, 1997; Bendszus and Stoll, 2003; Mueller et al., 2003). One study reported the presence of infiltrating macrophages as early as 36 h after mouse sciatic nerve axotomy (Beirowski et al., 2004). However, most of these studies have been in fixed samples. In vivo live cell imaging in zebrafish after spinal motor nerve transection showed that macrophages arrive at the lesion site within 1-2 h post-injury (Rosenberg et al., 2012). This occurred prior to axonal degeneration. Further, the authors also indicated that immune cell recruitment in this model was SC independent. Similar results were also observed in peripheral nerve injury in Drosophila (performed by laser ablation of peripheral nerve of fly wing) (Soares et al., 2014). Using live in vivo imaging up to 2 h following injury the authors reported the migration and accumulation of hemocytes (Drosophila equivalent of macrophages) within 30 min post-injury (Soares et al., 2014). These immune cell numbers increased from day 2 to 7 (Soares et al., 2014). Thus live-cell in vivo imaging may provide a greater insight into the complex cell dynamics and response that occurs after a peripheral nerve injury (Rosenberg et al., 2012).
Further the macrophages present during the early stages of nerve injury are pro-inflammatory resembling the M1 phenotype (Peluffo et al., 2015). However, around 7-14 days post-injury the presence of M2 or anti-inflammatory macrophages are detected (Stratton and Shah, 2016). IL-10 is crucial for the switch towards the M2 phenotype. While some IL-10 is produced by SCs and fibroblasts, the majority of this cytokine is produced by the macrophages themselves (George et al., 2004).
The mechanism behind SC debris clearance is not well understood. In the past, some researchers hypothesized that the SCs take up myelin debris and release it back into the extracellular space, to in turn be phagocytosed by macrophages (Odaly and Imaeda, 1967; Liu et al., 1995). Other studies, however, indicated that SCs themselves phagocytose myelin debris, which is digested within lysosomes (Hirata et al., 1999; Hirata and Kawabuchi, 2002). These phagocytic SCs have been likened to macrophages, moving via chemotaxis towards myelin debris, also displaying myelin-recognizing receptors such as galectin-3 (thought to recognize myelin galactolipids) and macrophage markers such as the A1 antigen marker and possibly cluster of differentiation-68 (CD-68) (Oulton and Mezei, 1976; Hirata and Kawabuchi, 2002).
Again, as myelin originates from SC membranes, after injury degraded myelin has been found in autophagosomes (double-membraned vesicles) inside the cells (Thumm and Simons, 2015). Thus, the role of autophagy in the degradation of myelin by SCs has been a key focus in recent research (Gomez-Sanchez et al., 2015; Suzuki et al., 2015; Thumm and Simons, 2015; Jang et al., 2016; Belgrad et al., 2020). Autophagy has been shown to occur particularly during the active demyelination process (Gomez-Sanchez et al., 2015; Suzuki et al., 2015; Jang et al., 2016). It has also been shown that microtubule associated protein light chain 3 II (LC-3II), an indicator of autophagy, is upregulated in SCs after injury, and that autophagosomes containing myelin debris fuse with lysosomes (Gomez-Sanchez et al., 2015; Jang et al., 2016). The autophagic activity was found to be regulated by c-Jun (Gomez-Sanchez et al., 2015; Jang et al., 2016) and pharmacological inhibition of autophagy resulted in substantial inhibition of myelin degradation (Gomez-Sanchez et al., 2015).
In addition to autophagy, SCs have also been revealed to utilize receptor-mediated phagocytosis for myelin debris clearance. In a crush injury model, SCs phagocytosed myelin using two of the TAM (Tyro3, Axl, and Mer) receptors, MERTK and AXL (Lutz et al., 2017); however crush injuries generate more debris than in the commonly used transection models where only autophagy has been reported (Gomez-Sanchez et al., 2015; Jang et al., 2016). Thus, the pathways that SCs use to clear debris may depend on the type and extent of injury. Along with TAM receptors, SCs in damaged nerves also upregulate multiple EGF-like domains 10 (MEGF-10) (Napoli et al., 2012) and low-density lipoprotein receptor related protein-1 (LRP-1) (Flutsch et al., 2015, 2016). Both these receptors mediate phagocytosis of myelin and debris from apoptotic bodies by CNS glia (astrocytes and microglia) after injury in mice (Loov et al., 2012; Ponath et al., 2017; Damisah et al., 2020). Similarly, glia utilize draper (mammalian orthologue MEGF-10), for phagocytosis of debris during Wallerian Denegation after injury in the Drosophila CNS (Hoopfer et al., 2006). Thus, these receptors may also be involved in SC-mediated phagocytosis post-injury.
Studies in vertebrates have provided further insight that SCs and macrophages may not be the only cells involved in this process of debris phagocytosis and nerve regeneration. In vivo time-lapse imaging of spinal motor root axon transection (performed by laser axotomy) in zebrafish showed that perineurial glia (the cells forming the perineurium or protective casing surrounding the SC-axon complex in mature peripheral nerves reviewed by Kucenas, 2015), are also actively involved in nerve repair (Lewis and Kucenas, 2014). Previous work in rodents and in vitro data have indicated perineurial glia to be the cells that initiate nerve bridge formation and guide SC migration towards this bridge after injury (Schröder et al., 1993; Parrinello et al., 2010). However, the zebrafish study revealed that along with nerve bridge formation, perineurial glia also played an important role in phagocytosis of debris along with SCs and macrophages (Lewis and Kucenas, 2014). The authors also noted that the glia and macrophages tackled different areas of the injured nerve. After nerve transection the sites both proximal and distal to the site of injury undergo degeneration after which the distal stump undergoes Wallerian Degeneration (Kerschensteiner et al., 2005; Lorenzana et al., 2015). Perineurial glia and SCs were involved in phagocytosis of debris generated at the stumps occurring immediately after transection. Macrophages on the other hand were involved in phagocytosis of debris in the injury gap with additional cells recruited to clear debris generated during Wallerian Degeneration (Lewis and Kucenas, 2014). Thus, a peripheral nerve injury is a complex environment. Utilizing live in vivo imaging system will help understand better the cellular dynamics governing debris clearance.
We can however certainly say that SCs are active phagocytes. Along with in vivo studies discussed above there are numerous in vitro studies from as early as 1945 indicating that SCs can internalize different types of targets, including latex beads, myelin, olfactory axon debris, heat-killed Escherichia coli bacteria and mycobacteria (Weiss and Wang, 1945; Band A.H. et al., 1986; Band H. et al., 1986; Velasquez et al., 2018). These vastly different targets, with a wide range of sizes and surface molecules, can be internalized and processed using a variety of mechanisms. Most studies to date have only focused on internalization, without investigating the capacity and efficiency for degradation and break down (or the timeline required for the different phases of phagocytosis). Regarding debris, the mechanism of uptake is to date mostly unknown, other than that the internalization of debris is actin-mediated and involves the Rho/Rac pathways (Band H. et al., 1986; Velasquez et al., 2018). Omics-profiling of repair SCs has identified several phagocytic and endocytic receptors (such as MERTK and Cathepsin D), along with lysosomal and endosomal markers which likely aid in endocytosis, efferocytosis and myelinophagy, that are expressed by repair SCs after injury (Weiss et al., 2016). We have also recently shown that SCs can phagocytose necrotic bodies by recognizing phosphatidylserine displayed on the surface of the dying cells. We also showed that while these targets are rapidly engulfed, their breakdown is much slower in SCs than in macrophages (Nazareth et al., 2020).
Schwann cells also contain a range of other receptors important for recognition of damage-associated molecular patterns (DAMPs) released by necrotic cells, including Toll-Like receptors (TLRs); TLR2 TLR3, TLR4, TLR7, and receptor for advanced glycation end products (RAGE) (Goethals et al., 2010). A study of SCs challenged with high mobility group box 1 (HMGB1), a DAMP passively released by necrotic cells after peripheral nerve injury, resulted in upregulation of TLR2 and RAGE mRNA in SCs (Man et al., 2015). In addition to up-regulating chemoattractants, human SCs have been shown to participate in antigen presentation via MHC-II (Suzuki et al., 2015; Weiss et al., 2016).
Thus, SCs display a number of phagocytic receptors that are potentially important for phagocytosis of cellular and myelin debris (Weiss et al., 2016; Lutz et al., 2017). SCs are capable of efficiently internalizing and degrading various necrotic targets in vitro (Nazareth et al., 2020), however, this remains to be shown in vivo. Similarly, whether SCs show similar efficiency in the phagocytosis and degradation of apoptotic targets remains to be determined. In spite of their phagocytic ability, after peripheral nerve injuries in vivo SCs enlist the help of various cells including perineurial glia and immune cells to phagocytose debris (Perry et al., 1987; Mueller et al., 2003; Beirowski et al., 2004; Rosenberg et al., 2012; Lewis and Kucenas, 2014; Lindborg et al., 2017).
Peripheral Neuropathies
Some peripheral neuropathies involving SCs include Charcot-Marie-Tooth disease (CMT), Guillain-Barré syndrome, diabetic neuropathy, and neuropathic pain. Peripheral neuropathies have also been reported in the aging population (reviewed by Verdu et al., 2000). Similar to Wallerian degeneration, these conditions are characterized by axonal degradation and demyelination. However, unlike Wallerian degeneration, nerve regeneration and remyelination is impaired either due to excessive degradation of myelin or abnormal myelin clearance (Martini et al., 2013). Thus, it is likely that perturbations of SC-mediated phagocytosis may in some way be involved in the onset and progression of peripheral neuropathies, but this remains to be investigated.
Charcot-Marie-Tooth diseases constitute a group of hereditary disorders and the manifestation of the disease is dependent on inherited genetic mutations (reviewed by Juneja et al., 2019). CMT is classified as demyelinating CMT or axonal CMT, with 80% of the cases belonging to the demyelinating form which is due to the inability of SCs to myelinate or maintain axonal myelin (Berger et al., 2006). As mentioned above, after peripheral nerve injury, both myelinating and non-myelinating SCs revert to a repair phenotype accompanied by upregulation of c-Jun (Arthur-Farraj et al., 2012, 2013). This also drives SC-mediated autophagic clearance of myelin (Gomez-Sanchez et al., 2015; Jang et al., 2016). In later stages of injury, c-Jun expression is down-regulated, and SC differentiation occurs, allowing myelination of newly generated axons (Mirsky et al., 2013; Jessen and Mirsky, 2016; Wagstaff et al., 2017). However, in CMT Type 1A (CMT1A), prolonged elevated levels of c-Jun (Hantke et al., 2014) and LC3B-II (which promotes autophagy) (Lee et al., 2018) have been reported in the SCs surrounding nerves that have not been injured. This dysregulation of SCs autophagy is thought to result in extended Wallerian degeneration, preventing remyelination of axons (Hutton et al., 2011; Lee et al., 2018). Macrophage-mediated phagocytosis of myelin has been shown to contribute to demyelination, particularly in Type 1 CMT mice. Studies on CMT1A, CMT1B and CMT1X mutant mice, have observed an increased number of macrophages near demyelinated nerves exhibiting a “foamy” appearance, due to internalization of myelin from nerves (Kobsar et al., 2005; Groh et al., 2012; Klein et al., 2015; Yuan et al., 2018). It is to date unknown if SCs in CMT also have an elevated phagocytic capacity and if this contributes to demyelination.
Neuropathic pain may arise due to peripheral nerve pathologies and injury. SC responses to PNS injury can play an important role in the progression of pain. Early activation of autophagy in SCs by the pharmacological agent rapamycin has been shown to induce rapid and increased clearance of myelin and axonal debris, promoting nerve regeneration, and reducing chronic pain in rodent pain models (Rangaraju and Notterpek, 2011; Marinelli et al., 2014). Perhaps stimulating SC phagocytosis would have similar therapeutic potential in treating neuropathic pain, however, care must be taken to not produce a strongly pro-inflammatory environment.
Similar to peripheral neuropathies, aging affects several morphological features of peripheral nerves, including loss of myelinated and unmyelinated fibers along with myelination abnormalities (Sakita et al., 2016). Impaired recovery after peripheral nerve injury has also been reported in the elderly population (Verdu et al., 2000). Matching these human studies, SCs in older mice express lower levels of c-Jun with a delay in de-differentiation into the repair SC phenotype after injury than in younger mice (Painter et al., 2014). SCs isolated from aged mice (24 months) also exhibit lower myelin clearance capacity than cells from younger mice, with a 35% decrease in myelin phagocytosis compared to cells isolated from younger animals (Painter et al., 2014). A study investigating peripheral nerve injury in aged rats also showed a decrease in phagocytic ability both in SCs and macrophages. The same study also showed that levels of anti-inflammatory factors such as IL-10, arginase-1, and CCL-2, which normally increase after PNS injury, were decreased in aged rats, potentially correlating with the ability for regeneration and debris clearance (Scheib and Hoke, 2016). Interestingly, one study showed that when sciatic nerves were grafted from old rats into younger rats their myelin clearance improved; conversely, grafting of young sciatic nerves into older rats resulted in accumulation of myelin debris. This indicates that the environment after peripheral nerve injury is crucial for debris clearance (Sakita et al., 2016). Hence, SCs play a crucial role in debris clearance after an injury and reduced phagocytic ability correlating with increasing age may be one of the contributing factors to peripheral nerve abnormalities in the older population.
In summary, SC autophagy and phagocytosis may be intimately related and both crucial for the clearance of myelin-, axon- and cell debris after an injury. It is likely that dysregulation of these functions in SCs contribute to various neuropathies. Determining the cellular and molecular mechanisms behind the autophagic and phagocytic functions of SCs and gaining a deeper understanding of these functions in injury repair, may lead to new effective treatments for peripheral neuropathies.
Peripheral Nerve Infections
Understanding peripheral glia phagocytosis of damaged “self” is vital in the context of injuries and neuropathies. However, an equally important arm of glial phagocytosis is that of “non-self.” Infections can lead to damage of peripheral nerves, resulting in neuropathies. Most of these pathologies are due to immune responses, in particular development of autoimmunity, for example production of antibodies targeting axons or myelin in addition to, or rather than, the infectious agent (reviewed by Neal and Gasque, 2016). However, a few pathogenic bacteria, viruses and protozoans can directly infect SCs, which can result in peripheral nerve damage (Neal and Gasque, 2016) or invasion of the CNS via cranial nerves in which SCs are found, in particular the trigeminal nerve (St John et al., 2016; Duarte et al., 2019; Nazareth et al., 2021). The intranasal branches of this nerve can serve as a direct path to CNS infection by several infectious agents, including Streptococcus pneumoniae, Burkholderia pseudomallei, Chlamydia muridarum, Herpes simplex virus, and Listeria monocytogenes (Shimeld et al., 2001; van Ginkel et al., 2003; St John et al., 2014, 2016; Wei et al., 2020; Nazareth et al., 2021). Thus, understanding SC phagocytosis of “non-self” is important and may lead to novel therapies, as mentioned earlier.
Schwann cells are efficient immune cells displaying several pathogen recognition receptors (PRRs) that they utilize to recognize invading pathogens. Some of these receptors include TLR 1-4, TLR 7, Nod-like receptors, RAGE, C-type lectin receptors, such as cluster of differentiation 209 (CD 209) and mannose receptors (reviewed by Ydens et al., 2013). SCs also display Fcγ receptors (FcγRII and FcγRIII) and components of the classical complement activation pathway, including complement receptor 3 (CR3) (Vedeler et al., 1989, 1990). As macrophages are known to utilize both FcγRs and CR3 to phagocytose infectious agents (Fitzer-Attas et al., 2000; Campagne et al., 2007), it is likely that SCs also utilize these receptors to recognize and engulf microbes. SCs have been also reported to produce a range of cytokines, including interleukins such as IL-6, IL-8, IL-10, IL-23, IFN-β1, IFN-γ, IL-1β, and TNF-α, and chemokines, such as CCL-2,CC-17, CC-19, CXC-11, CXCL-1, MCP-1, MIP-1α, MIP-1β in response to pathogens (Ramesh et al., 2013; Masaki et al., 2014; Dhiman et al., 2019; Nazareth et al., 2021). Secretion of these cyto- and chemokines occurs in parallel with activation of NF-κB and inducible nitric oxide (iNOS) production, in response to pathogenic ligands (Lee et al., 2007). SCs also display MHC molecules and can upregulate and display functionally active MHC class I and II structures when challenged by various pathogens (Samuel et al., 1987; Pereira et al., 1994). Thus, SCs can act as non-professional antigen-presenting cells.
Schwann cells can mount an effective anti-pathogenic immune response to combat infections (Neal and Gasque, 2016), but some microbes instead survive in SCs by manipulating components of the phagocytic pathway. Mycobacterium leprae, the obligate intracellular bacterium that causes leprosy, preferentially infects SCs in the peripheral nerves. There are various structures on M. leprae that bind to receptors on SCs allowing their entry. These include 21-kDa molecule (ML-LBP21) that binds to the α2 laminins on SCs, promoting attachment and entry of the bacteria (Shimoji et al., 1999). M. leprae bacteria also contain phenolic glycolipid 1 (PGL 1) that binds to mannose receptors on SCs, promoting endocytic uptake of the bacteria (Acosta et al., 2018). After binding to SCs, M. leprae modulates host tyrosine kinases, in particular phosphoinositide 3-kinase (PI3K), promoting entry into the cell (Alves et al., 2004). After entry, the bacterium resists endosome processing and maturation by recruiting host cell-derived lipid droplets to phagosomes that contain M. leprae bacteria. The genesis of these lipid droplets within the host cells also further increases SC production of prostaglandin E and IL-10, accompanied by decreased IL-12 and iNOS production (Mattos et al., 2011). Thus, M. leprae suppresses SC immune responses and persists within the cells.
Mycobacterium leprae can infect both myelinating and non-myelinating SCs. Whilst it shows a greater affinity for non-myelinating SCs, infection can lead to severe demyelination of peripheral nerves (Kumar and Sengupta, 2003). M. leprae also takes advantage of the plastic nature of SCs, and after infection re-programs adult SCs into progenitor-like cells. This is accompanied by upregulation of sex determining region Y (SRY) box-2 (Sox-2), seen in early developing neural crest SCs, and downregulation of SRY box-10 (Sox-10), required for SCs differentiation into the myelinating phenotype (Le et al., 2005; Finzsch et al., 2010; Masaki et al., 2013b) – consistent with de-differentiation of the cells. These progenitor-like cells are different from repair SCs, with the progenitor cells expressing both Sox-2 and Sox-10 whilst the repair cells only express Sox-2 (Jessen and Mirsky, 2016). The reprogrammed cells then transfer the bacterium to their neighboring fibroblasts, promoting dissemination within the nerves (Masaki et al., 2013a).
Bacteria that invade the CNS through the trigeminal nerve have been shown to infect SCs in vitro. When SCs were inoculated with S. pneumoniae, the bacteria utilized mannose receptors to the enter cells (Macedo-Ramos et al., 2014). B. pseudomallei, the bacterium that causes melioidosis (including neurological infection), is one of the microbes that can invade the brain after intranasal exposure, via both the trigeminal and the olfactory nerves (St John et al., 2014, 2016). We have found that B. pseudomallei infects a subpopulation of SCs, which then become multinucleated, most probably through the bacterial protein Burkholderia intracellular motility A (BimA), that manipulates the host actin cytoskeleton resulting in cell-cell fusion. However, some cells did not form multinucleated giant cells and contained degraded bacteria (Walkden et al., 2020). Neisseria meningitidis can also infect trigeminal SCs, leading to multinucleation via unknown mechanisms (Delbaz et al., 2020).
Chlamydia pneumoniae has been shown to infect the CNS and linked to late-onset dementia (Balin et al., 1998, 2018; Gerard et al., 2006). Since C. pneumoniae-infected mice rapidly develop olfactory bulb infection after intranasal exposure, the olfactory nerve has for some time been considered a likely path to the CNS for this bacterium (Little et al., 2004, 2014; Boelen et al., 2007). C. muridarum, another Chlamydia species that infects mice, is often used to model C. pneumoniae infections. We recently showed that mice inoculated intranasally with C. muridarum develop CNS infection via both the olfactory and the trigeminal nerves (Nazareth et al., 2021). In vitro, trigeminal SCs were readily infected by C. muridarum, but exhibited more resistance to infection than fibroblasts (Nazareth et al., 2021). Chlamydiae are obligate intracellular bacteria that live inside modified intracellular membrane compartments termed inclusions. The bacteria manipulate the host phagocytic pathway, including the actin cytoskeleton, to induce entry and suppress endosomal-lysosomal components and recruit recycling endosomes. Thus, Chlamydiae can live intracellularly and evade degradation by the phagocytic machinery (reviewed by Gitsels et al., 2019). How Chlamydia bacteria specifically modulate SC phagocytosis to survive intracellularly remains to be investigated.
Trypanasoma cruzi, an obligate intracellular protozoan and the causative agent for Chagas disease, can cause both PNS and CNS infections and have particular affinity towards infecting glia (Weinkauf and PereiraPerrin, 2009). T. cruzi contains trans-sialidase parasite-derived neurotrophic factor (PDNF) that is similar to neurotrophin-3 (NT-3). PDNF binds to and activates neurotrophic receptor tyrosine kinase C (TrkC) which allows T. cruzi entry into SCs (Weinkauf and PereiraPerrin, 2009). Phosphorylation of components of the MAPK, Erk1/2 and Akt pathways then occurs, preventing host cell death which allows the parasite to survive within the cells (Chuenkova and PereiraPerrin, 2009).
Thus, SCs can recognize, internalize, and produce an immune response to microbes. However, while SCs are more resistant to infection than many non-professional phagocytes (such as fibroblasts), they appear to be not as efficient phagocytes as macrophages (Nazareth et al., 2021). Intracellular pathogens, in particular, can manipulate SC plasticity and phagocytic pathways to survive within the cells. Understanding the modulation of SCs by pathogens and further manipulation of the intrinsic immune capacity of these cells holds great therapeutic potential to reduce the risk of PNS infections and potentially prevent CNS pathologies.
In summary, SCs display a range of phagocytic receptors that they use to recognize and internalize dying and damaged “self” as well as invading “non-self.” SCs actively participate in the initial debris clearance at a PNS injury site, however, they also recruit macrophages for complete removal of cellular and myelin debris. While studies of SC-mediated phagocytosis of myelin (particularly after injury) is well documented, the role of SC phagocytosis during development and in various peripheral neuropathies has not been investigated in great detail (especially in mammals). Finally, SCs can mount an immune response against infectious agents, but certain microbes can modulate the cells to instead cause infection.
Olfactory Ensheathing Cells (OECs): Origin and Physiology
Olfactory ensheathing cells are the glia of the primary olfactory nervous system, which consists of the olfactory neuroepithelium, olfactory nerve and outer layer (nerve fiber layer, NFL) of the olfactory bulb (Figure 2). OECs are found throughout this system, except within the superficial neuroepithelium. In addition to the main olfactory system, an accessory olfactory system is present in many animals and is located in the dorsal-caudal region of the olfactory bulb. The primary part of the accessory olfactory nervous system is also populated by OECs (reviewed by Mucignat-Caretta et al., 2012). OECs are somewhat similar to non-myelinating SCs in that they ensheathe bundles of non-myelinated axons (Figure 2). However, olfactory nerve bundles are typically larger than the bundles of unmyelinated peripheral axons ensheathed by SCs (Doucette, 1990; Woodhoo and Sommer, 2008).
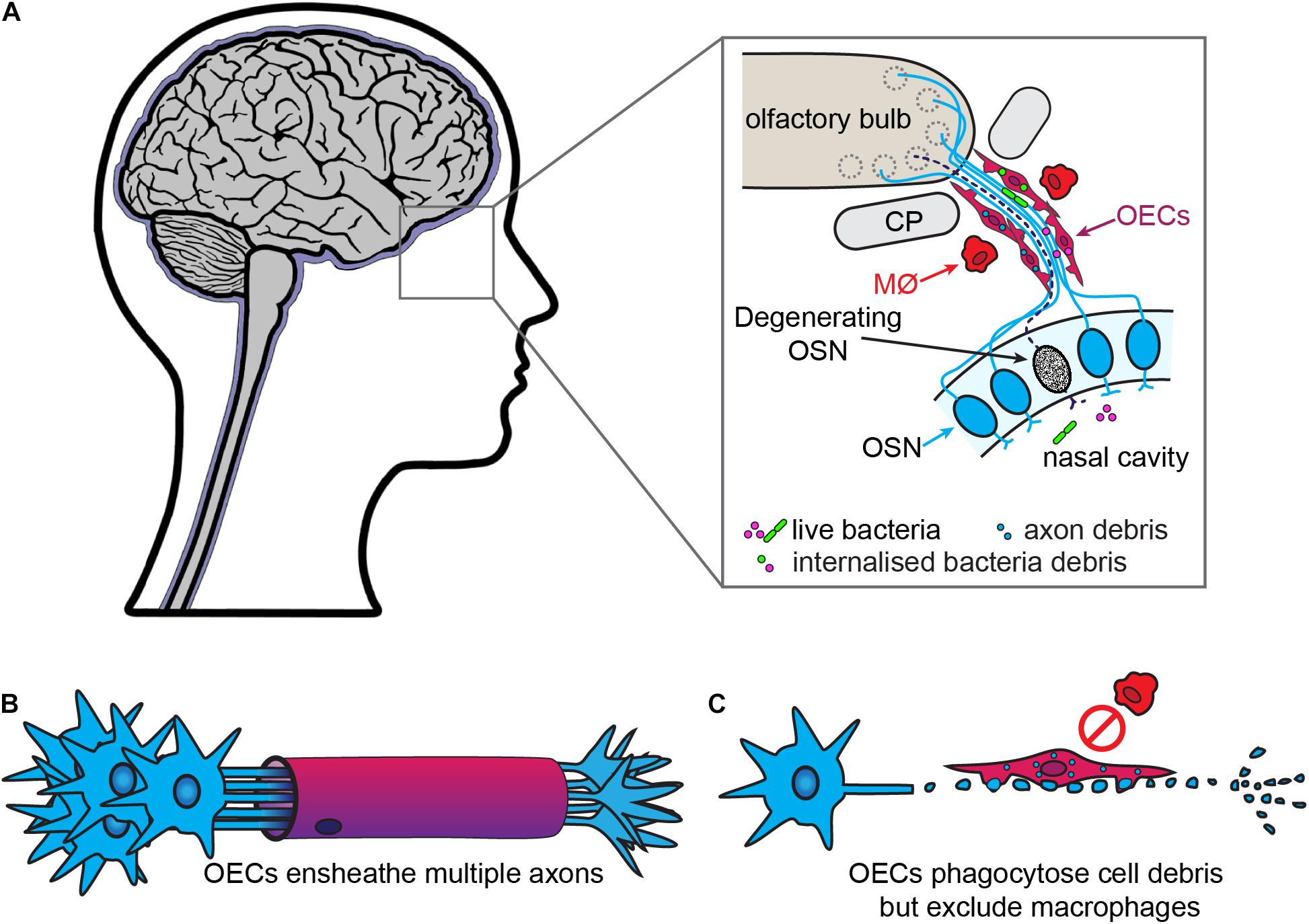
Figure 2. Olfactory ensheathing cells are the primary phagocytes in the olfactory nerve. (A) In the olfactory system, olfactory sensory neurons (OSN, blue) project dendrites into the nasal cavity and extend axons into the olfactory bulb. The bundles of olfactory axons are surrounded by OECs. OSNs are constantly turned over and replaced, with the debris from the degenerating axons (dashed line) phagocytosed by OECs. The OECs also provide a line of defense against bacteria from the nasal cavity which penetrate the olfactory nerve, with OECs engulfing the bacteria. Macrophages (MØ) are largely absent from the olfactory nerve. (B) In contrast to SCs, OECs ensheathe multiple axons and do not myelinate olfactory axons. (C) After injury, OECs phagocytose cell debris but do not recruit macrophages.
In early development, OECs migrate from the neural crest to populate the olfactory placode as early as embryonic day (E9) (in mice) (Katoh et al., 2011; Suzuki and Osumi, 2015), from where they continue to migrate towards the periphery and become part of the olfactory nerve. The olfactory neuroepithelium contains the cell bodies of immature and mature olfactory sensory neurons, basal cells and supporting or sustentacular cells. After differentiation, olfactory sensory neurons start to extend their axons (around E11) into the lamina propria that underlies the olfactory neuroepithelium, where they encounter OECs. As the axons grow towards the emerging olfactory bulb, they are continuously in close contact with OECs (Valverde et al., 1992). Unlike SCs, which accompany developing neurons, OECs migrate ahead of emerging axons (Doucette, 1990; Chuah and Zheng, 1992; Chehrehasa et al., 2010; Ekberg et al., 2012). OECs constitute a structural, tunnel-like support structure for the axons and secrete growth and guidance factors, such as nerve growth factor (NGF), brain derived nerve growth factor (BDNF) and neuregulin (Kafitz and Greer, 1999; Boruch et al., 2001; Woodhall et al., 2001; Lipson et al., 2003; Chung et al., 2004; Feng et al., 2012). Around E12-E13, the developing olfactory nerve bundles fuse with the developing telencephalon and this region then forms the presumptive olfactory bulb (Doucette, 1989) with OECs being limited to the NFL (Valverde et al., 1992). The NFL consists of an outer and an inner layer. OECs located in the outer NFL contribute to the defasciculation of the axons that project into the outer layer of the olfactory bulb, while OECs of the inner NFL mediate sorting and re-fasciculation of these axons into bundles that are projected into specific glomeruli depending on their odorant receptor profile (Mombaerts et al., 1996; Treloar et al., 2002).
These multiple OEC-mediated roles are also important for maintaining normal olfaction both postnatally and during adulthood, as the primary olfactory nervous system is unique in that it regenerates itself during life. When reaching the end of their lifespan, the primary olfactory neurons die, whilst new ones arise from epithelial progenitor cells. In adult animals, olfactory neurons have a lifespan of 1-3 months, with 1-3% of the neurons undergoing apoptosis each day (Graziadei and Graziadei, 1979; Mackaysim and Kittel, 1991). Thus, new olfactory axons are always extending towards the olfactory bulb, with OECs constantly supporting, guiding and sorting these axons (Chuah and Zheng, 1992; Chehrehasa et al., 2010).
Like SCs, OECs are heterogeneous glia, with individual subpopulations exhibiting different properties depending on their anatomical location (reviewed by Ekberg et al., 2012; Ekberg and St John, 2014; Yao et al., 2018). The subtypes of OECs include (i) OECs present in the lamina propria/olfactory nerve, (ii) OECs within the NFL of the olfactory bulb which in turn consists of inner- and outer NFL population and can be even further divided into distinct subpopulations (Windus et al., 2010), and (iii) accessory olfactory bulb OECs. Individual subpopulations of OECs also display differential expression of various molecules in vivo (Ekberg et al., 2012; Ekberg and St John, 2014; Perera et al., 2020) as well as differential behaviors regarding cell-cell/cell-axon interactions and phagocytic activity (Windus et al., 2010; Nazareth et al., 2015b).
OEC Phagocytosis in Normal Physiological Conditions
As in other regions of the nervous system, excess axons and axonal branches that arise during development of the primary olfactory system need to be removed or pruned. In mice, phagocytosis of axon debris by OECs is observed in vivo as early as E14.5, and cultured OECs derived from these mice phagocytose olfactory axon debris in vitro (Nazareth et al., 2015a). The continuous turnover of the olfactory sensory neuron population in adults means that axons of apoptotic neurons are constantly present in the olfactory nerve, leading to large amounts of axon-derived debris. This debris must be removed so newly born neurons can extend their axons into the olfactory nerve and bulb. OECs are the main phagocytes that remove axon debris arising from apoptotic neurons, whilst only very few macrophages are present in olfactory axon bundles (Figure 2; Su et al., 2013; Nazareth et al., 2015a). In addition to debris, OECs can phagocytose bacteria and are likely important for protecting the olfactory nerve against infection by microbes, as part of their normal physiological function as well as in more severe infections (covered in the next section) (Harris et al., 2009; Herbert et al., 2012; Panni et al., 2013; Dando et al., 2014).
Olfactory neurons undergo cell death via apoptosis upon reaching the end of their lifespan, displaying the “eat me” signal phosphatidylserine. In vitro studies have shown that OECs recognize phosphatidylserine on apoptotic neurons via phosphatidylserine receptors prior to engulfment (He et al., 2014; Hao et al., 2017). As discussed earlier, phagocytes usually possess a number of receptors that recognize phosphatidylserine on an apoptotic target; while some receptors directly recognize phosphatidylserine, others require bridging molecules to aid attachment. One such bridging molecule, milk fat globule-EGF factor 8 (MFGE-8), that works with integrin receptors (Hanayama et al., 2002), is expressed by OECs in vitro when apoptotic debris is added to the culture (Li et al., 2017). Normal physiological apoptosis is a “silent” process, in which phagocytic cells engulf apoptotic bodies and secrete anti-inflammatory cytokines (such as IL-10 and TGF-β) (Henson, 2017). Indeed, when phagocytosing apoptotic debris derived from neurons, OECs secrete TGF-β1 which may promote phagocytosis via integrin receptors (Li et al., 2017).
Olfactory ensheathing cells isolated from distinct anatomical locations exhibit different phagocytic capacities both in vivo and in vitro (Nazareth et al., 2015b). One study has shown that acutely isolated OECs from the main olfactory bulb contain more cytoplasmic axon-derived debris than OECs from the accessory bulb. However, after in vitro culture, the opposite occurred (Nazareth et al., 2015b). Perhaps the phagocytic activity of accessory OECs, which are less phagocytic than main OECs in vivo (possibly due to unknown differences in the requirement for phagocytosis in the main versus accessory olfactory nervous system), is more dynamic and susceptible to up-regulation than that of main OECs.
In summary, OECs are efficient and active phagocytes throughout life, including in adulthood. This function differs from that of SCs populating adult peripheral nerves that do not regenerate unless injured. OECs express some key phagocytosis receptors, but may exhibit many more not described to date. Further, different subpopulations of OECs may have different phagocytic abilities. This needs to be further explored especially when determining the best population of OECs to be utilized for transplantation therapies to treat CNS pathologies. Importantly, studies on OEC autophagy are also lacking.
OEC Phagocytosis – Pathological Conditions
Olfactory Nerve Injury
The primary olfactory nervous system is capable of regeneration and repairing itself after most injuries. In animal injury models, both after destruction of the olfactory epithelium (via zinc irrigation or exposure to the drug methimazole) as well as ablation of an olfactory bulb, OECs phagocytose large amounts of debris from degenerated axons (Chuah et al., 1995; Su et al., 2013; Nazareth et al., 2015a). Even in the presence of a small number of invading macrophages after large-scale injury, it is the OECs that contain the vast majority of the internalized debris even after injury (Su et al., 2013; Nazareth et al., 2015a).
Similarly, in the injured Drosophila primary olfactory nervous system, ensheathing glia (Drosophila glia equivalent to OECs), phagocytose debris. This occurs via draper/shark/ced-6 pathway (Doherty et al., 2009). The mammalian orthologues MEGF10/engulfment adaptor PTB Domain containing-1 (GULP-1), have been identified to mediate phagocytosis of apoptotic debris by astrocytes after injury or insult such as ischemia (Hamon et al., 2006; Iram et al., 2016; Morizawa et al., 2017). Whether OECs utilize the MEGF-10/GULP-1 pathway to phagocytose apoptotic debris following injury in mammals is yet to be determined.
In vitro phagocytic assays have also shown that OECs are capable of taking up debris derived from several types of axons (generated by destruction of axons, thus resembling an injury containing both apoptotic and necrotic bodies) as well as myelin debris (He et al., 2014; Nazareth et al., 2015a, 2020; Khankan et al., 2016; Hao et al., 2017). OECs can, like SCs, actively phagocytose and degrade necrotic cells in vitro (also via phosphatidylserine recognition). However, unlike SCs that secrete inflammatory cytokines such as TNF-α and IL-6 post-phagocytosis of these targets, OEC phagocytosis of necrotic bodies does not lead to production of TNF-α, and to only very low levels of IL-6 (Nazareth et al., 2020).
While OECs can effectively engulf and degrade many types of debris in vitro, the mechanisms and receptors involved remain mostly unknown, and the phagocytic activities need to be investigated in vivo. One study has demonstrated that OECs transplanted into the X-irradiated spinal cord are phagocytic. Despite having a different developmental origin than microglia, these OECs were reported to be “microglia-like,” expressing OX42 (CD11), a microglial marker (Lankford et al., 2008). However, in vitro immunolabelling of OECs showed that they do not express this marker under normal physiological conditions, at least not in culture (Hao et al., 2017). In another study, OECs and fibroblasts were transplanted separately into transected mouse spinal cords. Clearance of myelin was significantly more pronounced when OECs were transplanted than after transplantation of fibroblasts (Khankan et al., 2016).
Olfactory Nerves and Infection
The olfactory nerve, like the intranasal branches of the trigeminal nerve, is a direct conduit from the external environment to the CNS, providing a potential entry point for pathogens. Some bacteria, including S. pneumoniae, N. meningitidis, B. pseudomallei, and C. muridarum (van Ginkel et al., 2003; Sjolinder and Jonsson, 2010; St John et al., 2014; Nazareth et al., 2021) and likely also C. pneumoniae (Little et al., 2004, 2014; Boelen et al., 2007), viruses (HSV-1, SARS-CoV2) (Shivkumar et al., 2013; Brann et al., 2020; Meinhardt et al., 2020), and protozoa (N. fowleri) (Jarolim et al., 2000; Moseman, 2020) can enter the CNS via the olfactory nerve. Similar to those that can infect CNS via the trigeminal nerve, most of these infectious agents reached the CNS rapidly (within 24-48 h). With the exception of these microorganisms, OECs are considered to be efficient in defending the CNS against microbes that manage to penetrate the olfactory epithelium (Dando et al., 2014). However, epithelial injury may increase susceptibility to invasion, even with microorganisms that do not typically invade the CNS via this path (Harris et al., 2009; Herbert et al., 2012; Walkden et al., 2020).
Olfactory ensheathing cells cultured in vitro produce an immune response to bacterial lipopolysaccharide (LPS) and various pathogen associated molecular patterns (PAMPs). OECs also contain TLR-2, TLR-4 and mannose receptors that may aid in recognizing and responding to various pathogenic components (Vincent et al., 2007; Carvalho et al., 2013). OECs challenged with E. coli in vitro can endocytose the bacteria, resulting in an inflammatory response, with NF-κB translocation, cytokine growth-regulated oncogene (Gro) and iNOS production (Harris et al., 2009; Panni et al., 2013). OECs display a chemoattraction to heat-killed E. coli, which they recognize via TLR-4, and are capable of degrading them by phagocytosis (Leung et al., 2008). However, it has not been documented that OECs actually degrade live E. coli. OECs also respond to Staphylococcus aureus infection both in vivo and in vitro with an inflammatory response including secretion of IL-6, TNF-α, NF-κB, and iNOS (Harris et al., 2009; Herbert et al., 2012).
In vitro studies have shown that OECs can respond to those bacteria that can invade the olfactory nerve, but that the response is not enough to eliminate the bacteria. Upon challenge with B. pseudomallei, cultured OECs can rapidly kill ∼90% of the bacteria, as well as produce a range of cytokines/chemokines (Dando et al., 2016). B. pseudomallei can, however, also survive inside some OECs, and like B. pseudomallei infection of trigeminal SCs, this can lead to the formation of multinucleated cells (Walkden et al., 2020). S. pneumoniae bacteria can be recognized and internalized by OECs after binding to mannose receptors on the cells (Macedo-Ramos et al., 2011; Carvalho et al., 2013), but cannot be degraded by the cells (Macedo-Ramos et al., 2016). One study showed that S. pneumoniae suppresses the OEC-mediated immune response by downregulating iNOS production and secretion of growth factors such as NT-3, BDNF and GDNF, which can affect general glial health and function, and thus immune functions (Ruiz-Mendoza et al., 2016). The same study also indicated that S. pneumoniae-infected OECs can secrete factors that contribute to microglial apoptosis (Ruiz-Mendoza et al., 2016). Whilst not yet studied in vivo, it is possible that that repression of microglial responses could aid infection of the olfactory bulb (and subsequently the rest of the CNS). A recent study showed that OECs can respond to and restrict, but not eliminate, intracellular growth of C. muridarum. In fact, OECs responded to these bacteria in a similar manner to macrophages with secretion of a plethora of cyto- and chemokines; the immune response was overall stronger than for trigeminal SCs (Nazareth et al., 2021). Chlamydiae bacteria have previously been widely reported to survive and persist for very long periods in professional phagocytes, in particular in macrophages (reviewed by Chen et al., 2019; Wong et al., 2019). Whether C. muridarum can persist in OECs in the long-term and if this this may contribute to infection of the CNS remains to be investigated.
In summary, OECs are the main phagocytes in the olfactory system and play an active role in phagocytic clearance of axon debris throughout life, as well as after an injury. Also, being situated near the external environment in the nasal cavity, OECs are equipped to protect against microbial challenges. Certain infectious agents can manipulate the phagocytic pathway of the cells and survive intracellularly, similarly to those that can resist killing by SCs (Macedo-Ramos et al., 2011, 2016; Mutso et al., 2020). However, much is lacking regarding our knowledge of the molecular mechanisms involved in OEC-mediated phagocytosis; studies in transgenic mice lacking key phagocytic receptors are particularly warranted. Identifying and understanding the differences between physiological phagocytosis and infection-driven phagocytosis will help identify ways to protect the brain from pathogens that may invade the CNS via the olfactory nerve, as previously discussed for trigeminal nerve infection.
Discussion
Key Differences Between SC- and OEC- Mediated Phagocytosis
Over the years there has been a debate about which peripheral glia, SCs or OECs, are more suitable to treat CNS pathologies. An ideal candidate to treat nervous system injury would need to be an efficient phagocyte, capable of clearing cellular and myelin debris without production of adverse inflammatory response. In addition, it would also require modulating the existent inflammatory environment and continue secreting nerve growth factors to help regeneration. Hence it is important to discuss how SCs and OECs may differ in these aspects as it may enable to decide on which cell type may possess the best therapeutic potential.
In the past, OECs and SCs were thought to be relatively similar (Wewetzer et al., 2002; Ulrich et al., 2014); OECs were even called “olfactory SCs.” However, studies over the last two decades have revealed that the two cell types are unique and have clearly distinct genetic profiles (Franssen et al., 2008; Perera et al., 2020). Gene ontology studies comparing transcriptomes of the two cell types showed that cultured OECs display a higher level of expression of genes related to tissue repair and regeneration in particular those required for phagocytosis and degradation of targets (Vincent et al., 2005; Franssen et al., 2008). Similarly, OECs have been shown to be more efficient phagocytes than SCs of necrotic/myelin debris in vitro (Nazareth et al., 2020). When challenged with various infectious agents and pathogen-derived ligands in vitro OECs have been found less prone to infection, and produce a stronger immune response compared to SCs (Vincent et al., 2007; Walkden et al., 2020; Nazareth et al., 2021).
Schwann cell phagocytosis in peripheral nerves appears to occur on a significant scale only in pathological conditions. While SCs participate in the initial debris removal of the injured nerve, other cells like perineurial glia and resident macrophages may also be involved in this process (Mueller et al., 2003; Lewis and Kucenas, 2014; Wang et al., 2020). Further they produce a range of pro-inflammatory mediators that recruit immune cells, particularly macrophages, to aid in debris clearing (Dubovy et al., 2014; Hartlehnert et al., 2017). Unlike SCs, OECs are a part of a constantly regenerating nerve, requiring rapid and efficient phagocytosis of axons from apoptotic neurons to prevent inflammation. OECs are the key cells performing this function without recruiting macrophages (Chehrehasa et al., 2014; Nazareth et al., 2015a; Murtaza et al., 2019). Whilst the mechanisms behind this key difference between OECs and SCs is mostly unknown, macrophage migration inhibitory factor (MIF), which is secreted by both OECs and macrophages, mediates segregation between the two cell types at least in vitro (Wright et al., 2020).
Inflammation is in general considered to hamper neural regeneration. OECs can produce a range of pro-inflammatory cytokines and chemokines both after injury and infection, but simultaneously promote rather than inhibit nervous system regeneration (Pastrana et al., 2006; Vincent et al., 2007; Franssen et al., 2008; Roet et al., 2011). One reason for this may be that OECs challenged with various inflammatory stimuli continue proliferating while promoting neurite outgrowth, with an increased phagocytic activity (Lankford et al., 2008; He et al., 2014; Roet and Verhaagen, 2014; Hao et al., 2017). Another reason may be that SCs can lose their plasticity when present in a chronically inflamed environment (Joshi et al., 2016) and this may affect their ability to perform phagocytosis and aid regeneration post-transplantation. However, the specific milieu at a CNS injury site, especially in the chronic stages, is a very complex inflamed environment, with reactive astrocytes, invading immune cells, growth-inhibitory factors, necrotic cells and large amounts of debris. It is unknown if both OECs and SCs continue to be efficient phagocytes in this complex environment, whilst also promoting regeneration. While phagocytosis of apoptotic targets is a silent event, phagocytosis of necrotic and myelin debris is usually followed by production of pro-inflammatory cytokines (Nazareth et al., 2020). For therapeutic purposes, however, it is critical that a strong pro-inflammatory response does not occur (in particular after transplantation into CNS injury sites, which are already hostile and pro-inflammatory). OECs been suggested to be immunomodulatory and/or in fact decrease inflammation in the host tissue (Lopez-Vales et al., 2004; Huo et al., 2012; Khankan et al., 2016; Xie et al., 2019; Zhang et al., 2021). One study showed that whilst SCs may have had some beneficial effects on the number of invading macrophages, they were unable to modulate the overall inflammatory environment at the CNS injury site (Pearse et al., 2018), but more studies on SCs are required. Thus, we need to increase our understanding of the mechanisms involved in both OEC and SC-mediated phagocytosis.
As OECs and SCs may have different but still complementary phagocytic abilities, co-transplantation of both cells may be an interesting option. Few studies have to date investigated co-transplantation of OECs and SCs to treat nervous system injuries (Ramón-Cueto et al., 1998; Takami et al., 2002; Pearse et al., 2004; Fouad et al., 2005; Au et al., 2007; You et al., 2011; Sun et al., 2013; Chen et al., 2014; Zhang et al., 2017), however the outcomes have been variable. One study looking at transplantation of either SCs, OECs or combination treatment into rat thoracic spinal cord after a moderate contusion injury reported that all three treatment groups presented a decrease in cavitation at the injury site and increase in number of myelinated axons (Takami et al., 2002). However, the number of myelinated axons were higher in SC or combination group than OECs alone. There was also a significant recovery in locomotor function in the SC alone treatment than the other two groups, thus indicating that the SC alone treatment produced best outcomes post-transplantation (Takami et al., 2002). However, interestingly there was an increase in accumulation of reactive astrocytes in the SC containing groups (Takami et al., 2002). In contrast, two other groups reported no difference between SCs only, OEC only and combination treatment (Pearse et al., 2004; Chen et al., 2014). A clinical study in patients with chronic complete SCI reported functional recovery in all groups receiving cell treatments (SCs, OECs, or co-transplantation) compared to no treatment group (Chen et al., 2014). However, no differences in recovery were observed between the three groups receiving glia transplantation. Similarly, in a rat model of thoracic contusive injury, while nerve growth was observed after transplantation of the two peripheral glia and in the co-transplantation group, no differences were reported amongst the three groups (Pearse et al., 2004). However, the authors also reported that locomotor recovery was greatly enhanced when methylprednisolone (a corticosteroid) and IL-10 were administered prior transplantation of glia (Pearse et al., 2004). Similar outcomes were also reported in complete spinal transection studies in adult rats. While cell grafts consisting of OEC and SC bridges improved forelimb and hindlimb movement along with an increase in number of myelinated axons and serotonergic fibers than non-treated controls, supplementing cell grafts with chondroitinase improved overall outcomes (Fouad et al., 2005). On the other hand, some studies have reported improved outcomes in nervous system injury models with combination treatment with SCs and OECs than either cell alone. One PNS study showed that co-transplantation promoted both axonal regeneration and functional repair after sciatic nerve injury in rats compared to transplantation of either cell type alone (You et al., 2011). Similarly, a CNS study showed that co-transplantation of OEC and SCs into the contused rat spinal cord resulted in better modulation of the inflammatory response (lower numbers of reactive astrocytes, reduced infiltration by immune cells and shift in microglia/macrophages to more anti-inflammatory phenotypes), along with improved motor function (Zhang et al., 2017). The authors also report greater distribution of glia at the site of injury when combined. This is not surprising as previous studies have reported than introducing OECs into an injured spinal cord increased migration of SCs along with improved axonal regeneration (Ramón-Cueto et al., 1998; Au et al., 2007). However, none of these co-transplantation studies have investigated the phagocytic clearance of cellular and myelin debris in this combination therapy. As we know that rapid clearance of debris is crucial for repair following nervous system injuries, an understanding of this crucial function will help us achieve the best outcomes from a potential co-transplantation therapy.
Stimulating Phagocytosis of Peripheral Glia to Treat Neural Injuries
Stimulating phagocytosis of endogenous or transplanted peripheral glia in nerve injuries may aid the overall process of regeneration and recovery (Wright et al., 2018). Several animal studies have shown that stimulating SC clearance of myelin debris can improve outcomes after injury. For example, supplying NGF after sciatic nerve crush injury in mice enhanced SC uptake and degradation of myelin both via autophagy and phagocytosis, leading to improved nerve regeneration (Li et al., 2020). Application of pharmacological agents such as rapamycin (a lipophilic macrolide drug which promotes SC autophagy) (Huang et al., 2016), antineoplastic drug Epothilone B (Zhou et al., 2020), as well natural compounds such as curcumin (Liu et al., 2016; Zhao et al., 2017), and resveratrol (Zhang J.Y. et al., 2020) have altered SC autophagic flux to enhance clearance of myelin and improve myelination. Specific enhancement of SC autophagy has also been explored to treat various peripheral neuropathies. Administration of rapamycin in mice during early stages of Wallerian degeneration increased SC autophagic flux which promoted myelin compaction, which occurred along with reduced inflammatory responses. This aided long-lasting analgesic effects and prevented development of chronic pain (Marinelli et al., 2014). Peripheral neuropathies are also characterized by either abnormal protein expression or mis-folding of proteins which aggregate within SCs. One such protein is peripheral myelin promoter 22 (PMP 22) that has been reported to be mis-expressed and aggregated within SCs especially in type 1 CMT (van Paassen et al., 2019). Treatment of SCs with rapamycin (Rangaraju and Notterpek, 2011), suberoylanilide hydroxamic acid (SAHA), 17-allylamino-17-demethoxy-geldanamycin (17-AAG) or clonazepam (Watanabe et al., 2010) facilitated autophagy, resulting in breakdown and disposal of these aggregated unfolded proteins in vitro. However, in vivo studies in a mouse model of CMT1A linked with PMP22 aggregation showed that although rapamycin promoted peripheral nerve myelination it did not improve the overall neuromuscular function in these animals (Nicks et al., 2014). The field would, however, strongly benefit from more studies directed towards stimulating SC phagocytosis rather than just autophagy for therapeutic purposes.
Several studies have to date identified compounds that can stimulate OEC phagocytosis (as well as autophagy – a topic which remains to be studied in itself). We, along with laboratories around the world, have identified various agents that can increase OEC phagocytosis, including the natural products 2-methoxy-1,4-naphthoquinone (Chen et al., 2020), the serrulatane diterpenoids 3-acetoxy-7,8-dihydroxyserrulat-14-en-19-oic acid and 3,7,8-trihydroxyserrulat-14-en-19-oic acid (Chen et al., 2018), curcumin (Velasquez et al., 2014); curcumin in combination with E. coli-derived LPS (Hao et al., 2017), and anti-inflammatory cytokine TGF-β (Li et al., 2017). In vitro injury assays (neurons co-cultured with OECs accompanied by neuronal debris addition), showed that increased OEC clearance of debris due to application of stimuli (LPS + curcumin Hao et al., 2017 or TGF-βLi et al., 2017) promoted neuronal survival. A recent study in a rat spinal cord injury model reported that transplanted OECs that had been pre-treated with curcumin led to better functional recovery and axonal regrowth than transplantation with control OECs (Guo et al., 2020). The stimulated cells secreted larger amounts of growth factors in vivo along with improved immunomodulation of the injury site. The same study also reported that the OECs stimulated with curcumin exhibited increased phosphatidylserine receptor expression (Guo et al., 2020), suggesting that increased phagocytic activity may have aided the overall therapeutic outcomes. Thus, stimulating glial phagocytosis may improve neural repair therapies.
Stimulating Peripheral Glia to Clear Pathogens
Peripheral nerves can allow microbes to enter the CNS, bypassing the BBB and the blood- cerebrospinal fluid barrier. The ability to infect and survive within glia are suggested to be a key strategy by which certain infectious agents can gain access to these nerves (Dando et al., 2014), but further mechanistic and in vivo studies are needed, which can reveal drug targets. In particular, the phagocytic phase in which glia degrade microorganisms needs attention. We also need to understand specific risk factors of CNS infections via cranial nerves, such as epithelial injury and long-term use of antibiotics, which may promote persistent infection. Another important research topic is to determine how infections can re-activate after being latent or dormant in the nervous system. It is well known that bacteria can persist within phagocytic cells to later escape and cause infection under favorable conditions (Bastidas et al., 2013), but very little is known regarding this phenomenon in glia. We also need to understand the long-term consequences of nervous system infection, in which glia have important roles. These may include neurodegeneration; for example, the connection between late-onset dementia and (1) infectious agents (including both C. pneumoniae and HSV-1) and (2) genetic factors such as the apolipoprotein ε4 (ApoEε4) allele, which is associated with impaired glial phagocytosis, is becoming increasingly evident (Itzhaki et al., 2016; Balin et al., 2018; Woods et al., 2020).
Concluding Remarks
Glia of the peripheral nervous system are strongly phagocytic cells during development, in the adult and after injury or infection. Yet, depending on their location the glia have different functions and engulf both “self” and “non-self” targets. In the developing and adult CNS, glia play an important role in the phagocytic clearance of various targets. However, in the PNS under physiological conditions not much is known about the role of SC phagocytosis in the maintenance of homoeostasis, particularly in mammals. In the healthy adult, damage to most peripheral nerves is limited and the need for phagocytosis by SCs is low. In contrast, the olfactory nerve is constantly being replenished and is also subjected to microbe invasion; thus, OECs are required to constantly phagocytose cell debris and be ready to respond to infectious agents. After injury, SCs clear cellular and myelin debris, however they enlist the help of other cells including perineurial glia and recruit macrophages to aid clearance and repair. On the other hand, OECs are largely responsible for phagocytosis and do not appear to recruit macrophages. While both SCs and OECs have a capacity for phagocytosis, the mechanisms that govern this process are largely unknown. Identifying the molecular and cellular mechanisms that drive phagocytosis of various targets by peripheral glial will then allow us to exploit this function. Stimulating SCs and OECs to clear cellular and myelin debris will help improve repair after injury or will aid better infection prevention/treatments. Understanding the differences in the phagocytic characteristics between SCs and OECs is also important for development of transplantation therapies to repair neural injuries as it may be possible to exploit key benefits of each cell type. Overall, increasing our understanding of glial phagocytosis may lead to the design of therapies to treat injuries and diseases of the nervous system.
Author Contributions
LN performed the literature search and drafted the manuscript. JE provided the overall supervision. All authors reviewed and edited the manuscript.
Funding
This work was funded by a Menzies Health Institute Queensland grant and a GODA Foundation grant to JE, and a Clem Jones Foundation Grant to JS and JE. LN was supported by the Australian Government Research Training Program scholarship.
Conflict of Interest
The authors declare that the research was conducted in the absence of any commercial or financial relationships that could be construed as a potential conflict of interest.
References
Absinta, M., Ha, S. K., Nair, G., Sati, P., Luciano, N. J., Palisoc, M., et al. (2017). Human and nonhuman primate meninges harbor lymphatic vessels that can be visualized noninvasively by mri. Elife 6:29738. doi: 10.7554/eLife.29738
Acosta, C. C. D., Dias, A. A., Rosa, T., Batista-Silva, L. R., Rosa, P. S., Toledo-Pinto, T. G., et al. (2018). Pgl i expression in live bacteria allows activation of a cd206/ppar gamma cross-talk that may contribute to successful mycobacterium leprae colonization of peripheral nerves. PLos Pathog. 14:1007151. doi: 10.1371/journal.ppat.1007151
Akert, K., Sandri, C., Weibel, E. R., Peper, K., and Moor, H. (1976). The fine structure of the perineural endothelium. Cell Tissue Res. 165, 281–295. doi: 10.1007/bf00222433
Alves, L., Lima, L. D. M., Maeda, E. D. S., Carvalho, L., Holy, J., Sarno, E. N., et al. (2004). Mycobacterium leprae infection of human schwann cells depends on selective host kinases and pathogen-modulated endocytic pathways. Fems Microbiol. Lett. 238, 429–437. doi: 10.1016/j.femsle.2004.08.007
Amaya, D. A., Wegner, M., Stolt, C. C., Chehrehasa, F., Ekberg, J. A., and St John, J. A. (2015). Radial glia phagocytose axonal debris from degenerating overextending axons in the developing olfactory bulb. J. Comp. Neurol. 523, 183–196. doi: 10.1002/cne.23665
Arthur-Farraj, P. J., Latouche, M., Wilton, D. K., Quintes, S., Chabrol, E., Banerjee, A., et al. (2012). C-jun reprograms schwann cells of injured nerves to generate a repair cell essential for regeneration. Neuron 75, 633–647. doi: 10.1016/j.neuron.2012.06.021
Arthur-Farraj, P. J., Morgan, C. C., Adamowicz, M., Gomez-Sanchez, J. A., Fazal, S. V., Beucher, A., et al. (2017). Changes in the coding and non-coding transcriptome and DNA methylome that define the schwann cell repair phenotype after nerve injury. Cell Rep. 20, 2719–2734. doi: 10.1016/j.celrep.2017.08.064
Arthur-Farraj, P., Latouche, M., Wilton, D. K., Quintes, S., Chabrol, E., Woodhoo, A., et al. (2013). Schwann cell c-jun is essential for successful nerve regeneration. J. Periph. Nerv. Syst. 18, 7–7.
Ashwell, K. (1990). Microglia and cell-death in the developing mouse cerebellum. Dev. Brain Res. 55, 219–230. doi: 10.1016/0165-3806(90)90203-b
Aspelund, A., Antila, S., Proulx, S. T., Karlsen, T. V., Karaman, S., Detmar, M., et al. (2015). A dural lymphatic vascular system that drains brain interstitial fluid and macromolecules. J. Exp. Med. 212, 991–999. doi: 10.1084/jem.20142290
Ataman, B., Ashley, J., Gorczyca, M., Ramachandran, P., Fouquet, W., Sigrist, S. J., et al. (2008). Rapid activity-dependent modifications in synaptic structure and function require bidirectional wnt signaling. Neuron 57, 705–718. doi: 10.1016/j.neuron.2008.01.026
Atanasoski, S., Scherer, S. S., Sirkowski, E., Leone, D., Garratt, A. N., Birchmeier, C., et al. (2006). Erbb2 signaling in schwann cells is mostly dispensable for maintenance of myelinated peripheral nerves and proliferation of adult schwann cells after injury. J. Neurosci. 26, 2124–2131. doi: 10.1523/jneurosci.4594-05.2006
Au, E., Richter, M. W., Vincent, A. J., Tetzlaff, W., Aebersold, R., Sage, E. H., et al. (2007). Sparc from olfactory ensheathing cells stimulates schwann cells to promote neurite outgrowth and enhances spinal cord repair. J. Neurosci. 27, 7208–7221. doi: 10.1523/jneurosci.0509-07.2007
Awasaki, T., and Ito, K. (2004). Engulfing action of glial cells is required for programmed axon pruning during drosophila metamorphosis. Curr. Biol. 14, 668–677. doi: 10.1016/j.cub.2004.04.001
Awasaki, T., Tatsumi, R., Takahashi, K., Arai, K., Nakanishi, Y., Ueda, R., et al. (2006). Essential role of the apoptotic cell engulfment genes draper and ced-6 in programmed axon pruning during drosophila metamorphosis. Neuron 50, 855–867. doi: 10.1016/j.neuron.2006.04.027
Baetas-da-Cruz, W., Alves, L., Pessolani, M. C. V., Barbosa, H. S., Regnier-Vigouroux, A., Corte-Real, S., et al. (2009). Schwann cells express the macrophage mannose receptor and mhc class ii. Do they have a role in antigen presentation? J. Periph. Nerv. Syst. 14, 84–92. doi: 10.1111/j.1529-8027.2009.00217.x
Balakrishnan, A., Belfiore, L., Chu, T.-H., Fleming, T., Midha, R., Biernaskie, J., et al. (2021). Insights into the role and potential of schwann cells for peripheral nerve repair from studies of development and injury. Front. Mol. Neurosci. 13:e608442. doi: 10.3389/fnmol.2020.608442
Balin, B. J., Gerard, H. C., Arking, E. J., Appelt, D. M., Branigan, P. J., Abrams, J. T., et al. (1998). Identification and localization of chlamydia pneumoniae in the alzheimer’s brain. Med. Microbiol. Immunol. 187, 23–42. doi: 10.1007/s004300050071
Balin, B. J., Hammond, C. J., Little, C. S., Hingley, S. T., Al-Atrache, Z., Appelt, D. M., et al. (2018). Chlamydia pneumoniae: an etiologic agent for late-onset dementia. Front. Aging Neurosci. 10:302. doi: 10.3389/fnagi.2018.00302
Band, A. H., Chitamber, S. D., Bhattacharya, A., and Talwar, G. P. (1986). Mechanism of phagocytosis of mycobacteria by schwann cells and their comparison with macrophages. Int. J. Lepr. Other. Mycobact. Dis. 54, 294–299.
Band, H., Bhattacharya, A., and Talwar, G. P. (1986). Mechanism of phagocytosis by schwann cells. J. Neurol. Sci. 75, 113–119.
Barraud, P., Seferiadis, A. A., Tyson, L. D., Zwart, M. F., Szabo-Rogers, H. L., Ruhrberg, C., et al. (2010). Neural crest origin of olfactory ensheathing glia. Proc. Natl. Acad. Sci. U.S.A. 107, 21040–21045. doi: 10.1073/pnas.1012248107
Barros, C. S., Franco, S. J., and Muller, U. (2011). Extracellular matrix: Functions in the nervous system. Cold Spring Harb. Perspect. Biol. 3:a005108. doi: 10.1101/cshperspect.a005108
Barton, M. J., John, J. S., Clarke, M., Wright, A., and Ekberg, J. (2017). The glia response after peripheral nerve injury: a comparison between schwann cells and olfactory ensheathing cells and their uses for neural regenerative therapies. Int. J. Mol. Sci. 18:e18020287. doi: 10.3390/ijms18020287
Bastidas, R. J., Elwell, C. A., Engel, J. N., and Valdivia, R. H. (2013). Chlamydial intracellular survival strategies. Cold Spring Harb. Perspect. Med. 3:20. doi: 10.1101/cshperspect.a010256
Becerra, J. L., Puckett, W. R., Hiester, E. D., Quencer, R. M., Marcillo, A. E., Post, M. J., et al. (1995). Mr-pathologic comparisons of wallerian degeneration in spinal cord injury. AJNR Am. J. Neuroradiol. 16, 125–133.
Beirowski, B., Babetto, E., Golden, J. P., Chen, Y. J., Yang, K., Gross, R. W., et al. (2014). Metabolic regulator lkb1 is crucial for schwann cell-mediated axon maintenance. Nat. Neurosci. 17, 1351–1361. doi: 10.1038/nn.3809
Beirowski, B., Berek, L., Adalbert, R., Wagner, D., Grumme, D. S., Addicks, K., et al. (2004). Quantitative and qualitative analysis of wallerian degeneration using restricted axonal labelling in yfp-h mice. J. Neurosci. Methods 134, 23–35. doi: 10.1016/j.jneumeth.2003.10.016
Belgrad, J., De Pace, R., and Fields, R. D. (2020). Autophagy in myelinating glia. J. Neurosci. 40, 256–266. doi: 10.1523/JNEUROSCI.1066-19.2019
Bendszus, M., and Stoll, G. (2003). Caught in the act: In vivo mapping of macrophage infiltration in nerve injury by magnetic resonance imaging. J. Neurosci. 23, 10892–10896.
Berger, P., Niemann, A., and Suter, U. (2006). Schwann cells and the pathogenesis of inherited motor and sensory neuropathies (charcot-marie-tooth disease). Glia 54, 243–257. doi: 10.1002/glia.20386
Berthold, C. H., Fraher, J. P., King, R. H. M., and Rydmark, M. (2005). “Chapter 3 – microscopic anatomy of the peripheral nervous system,” in Peripheral Neuropathy (Fourth Edition), eds P. J. Dyck and P. K. Thomas (Philadelphia, PA: W.B. Saunders), 35–91.
Bhattacharyya, A., Brackenbury, R., and Ratner, N. (1994). Axons arrest the migration of schwann cell precursors. Development 120, 1411–1420.
Bishop, D. L., Misgeld, T., Walsh, M. K., Gan, W. B., and Lichtman, J. W. (2004). Axon branch removal at developing synapses by axosome shedding. Neuron 44, 651–661. doi: 10.1016/j.neuron.2004.10.026
Boelen, E., Stassen, F. R. M., van der Ven, A., Lemmens, M. A. M., Steinbusch, H. P. J., Bruggeman, C. A., et al. (2007). Detection of amyloid beta aggregates in the brain of balb/c mice after chlamydia pneumoniae infection. Acta Neuropathol. 114, 255–261. doi: 10.1007/s00401-007-0252-3
Boivin, A., Pineau, I., Barrette, B., Filali, M., Vallieres, N., Rivest, S., et al. (2007). Toll-like receptor signaling is critical for wallerian degeneration and functional recovery after peripheral nerve injury. J. Neurosci. 27, 12565–12576. doi: 10.1523/jneurosci.3027-07.2007
Bonetti, B., Monaco, S., Giannini, C., Ferrari, S., Zanusso, G., and Rizzuto, N. (1993). Human peripheral nerve macrophages in normal and pathological conditions. J. Neurol. Sci. 118, 158–168. doi: 10.1016/0022-510x(93)90105-8
Boruch, A. V., Conners, J. J., Pipitone, M., Deadwyler, G., Storer, P. D., Devries, G. H., et al. (2001). Neurotrophic and migratory properties of an olfactory ensheathing cell line. Glia 33, 225–229. doi: 10.1002/1098-1136(200103)33:3<225::Aid-glia1021<3.3.Co;2-p
Bosse, F., Hasenpusch-Theil, K., Kury, P., and Muller, H. W. (2006). Gene expression profiling reveals that peripheral nerve regeneration is a consequence of both novel injury-dependent and reactivated developmental processes. J. Neurochem. 96, 1441–1457. doi: 10.1111/j.1471-4159.2005.03635.x
Bowman, C. C., Rasley, A., Tranguch, S. L., and Marriott, I. (2003). Cultured astrocytes express toll-like receptors for bacterial products. Glia 43, 281–291. doi: 10.1002/glia.10256
Brann, D. H., Tsukahara, T., Weinreb, C., Lipovsek, M., Van den Berge, K., Gong, B. Y., et al. (2020). Non-neuronal expression of sars-cov-2 entry genes in the olfactory system suggests mechanisms underlying covid-19-associated anosmia. Sci. Adv. 6:5801. doi: 10.1126/sciadv.abc5801
Buchstaller, J., Sommer, L., Bodmer, M., Hoffmann, R., Suter, U., and Mantei, N. (2004). Efficient isolation and gene expression profiling of small numbers of neural crest stem cells and developing schwann cells. J. Neurosci. 24, 2357–2365. doi: 10.1523/jneurosci.4083-03.2004
Burkel, W. E. (1967). The histological fine structure of perineurium. Anat. Rec. 158, 177–189. doi: 10.1002/ar.1091580207
Buss, A., Brook, G. A., Kakulas, B., Martin, D., Franzen, R., Schoenen, J., et al. (2004). Gradual loss of myelin and formation of an astrocytic scar during wallerian degeneration in the human spinal cord. Brain 127(Pt 1), 34–44. doi: 10.1093/brain/awh001
Campagne, M. V., Wiesmann, C., and Brown, E. J. (2007). Macrophage complement receptors and pathogen clearance. Cell. Microbiol. 9, 2095–2102. doi: 10.1111/j.1462-5822.2007.00981.x
Carvalho, L. A., Nobrega, A. F., Soares, I. D. P., Carvalho, S. L., Allodi, S., Baetas-da-Cruz, W., et al. (2013). The mannose receptor is expressed by olfactory ensheathing cells in the rat olfactory bulb. J. Neurosci. Res. 91, 1572–1580. doi: 10.1002/jnr.23285
Chauhan, P., Hu, S., Sheng, W. S., Prasad, S., and Lokensgard, J. R. (2017). Modulation of microglial cell fcγ receptor expression following viral brain infection. Sci. Rep. 7:41889. doi: 10.1038/srep41889
Chauhan, V. S., Sterka, D. G., Furr, S. R., Young, A. B., and Marriott, I. (2009). Nod2 plays an important role in the inflammatory responses of microglia and astrocytes to bacterial cns pathogens. Glia 57, 414–423. doi: 10.1002/glia.20770
Chehrehasa, F., Ekberg, J. A. K., and St John, J. A. (2014). A novel method using intranasal delivery of edu demonstrates that accessory olfactory ensheathing cells respond to injury by proliferation. Neurosci. Lett. 563, 90–95. doi: 10.1016/j.neulet.2014.01.043
Chehrehasa, F., Windus, L. C. E., Ekberg, J. A. K., Scott, S. E., Amaya, D., Mackay-Sim, A., et al. (2010). Olfactory glia enhance neonatal axon regeneration. Mol. Cell. Neurosci. 45, 277–288. doi: 10.1016/j.mcn.2010.07.002
Chen, H. L., Wen, Y. T., and Li, Z. Y. (2019). Clear victory for chlamydia: the subversion of host innate immunity. Front. Microbiol. 10:1412. doi: 10.3389/fmicb.2019.01412
Chen, L., Huang, H., Xi, H., Zhang, F., Liu, Y., Chen, D., et al. (2014). A prospective randomized double-blind clinical trial using a combination of olfactory ensheathing cells and schwann cells for the treatment of chronic complete spinal cord injuries. Cell Trans. 23(Suppl. 1), S35–S44. doi: 10.3727/096368914x685014
Chen, M. H., Chen, P. R., Chen, M. H., Hsieh, S. T., Huang, J. S., and Lin, F. H. (2006). Gelatin-tricalcium phosphate membrane modified with ngf and cultured schwann cells for peripheral nerve repair: a tissue engineering approach. Biomed. Eng. Appl. Basis Commun. 18, 47–54. doi: 10.4015/s1016237206000105
Chen, M., Vial, M. L., Gee, L., Davis, R. A., St John, J. A., and Ekberg, J. A. K. (2020). The plant natural product 2-methoxy-1,4-naphthoquinone stimulates therapeutic neural repair properties of olfactory ensheathing cells. Sci. Rep. 10:951. doi: 10.1038/s41598-020-57793-2
Chen, M., Vial, M. L., Velasquez, J. T., Ekberg, J. A. K., Davis, R. A., and St John, J. A. (2018). The serrulatane diterpenoid natural products rad288 and rad289 stimulate properties of olfactory ensheathing cells useful for neural repair therapies. Sci. Rep. 8:10240. doi: 10.1038/s41598-018-28551-2
Chen, Z.-L., Yu, W.-M., and Strickland, S. (2007). Peripheral regeneration. Ann. Rev. Neurosci. 30, 209–233. doi: 10.1146/annurev.neuro.30.051606.094337
Choucair-Jaafar, N., Laporte, V., Levy, R., Poindron, P., Lombard, Y., and Gies, J. P. (2011). Complement receptor 3 (cd11b/cd18) is implicated in the elimination of beta-amyloid peptides. Fundamen. Clin. Pharmacol. 25, 115–122. doi: 10.1111/j.1472-8206.2010.00811.x
Choudhury, M. E., Miyanishi, K., Takeda, H., Islam, A., Matsuoka, N., Kubo, M., et al. (2020). Phagocytic elimination of synapses by microglia during sleep. Glia 68, 44–52. doi: 10.1002/glia.23698
Chuah, M. I., and Zheng, D. R. (1992). The human primary olfactory pathway-fine-structural and cytochmical aspects during development and in adults. Micros. Res. Techn. 23, 76–85. doi: 10.1002/jemt.1070230107
Chuah, M. I., Tennent, R., and Jacobs, I. (1995). Response of olfactory schwann-cells to intranasal zinc-sulfate irrigation. J. Neurosci. Res. 42, 470–478. doi: 10.1002/jnr.490420405
Chuenkova, M. V., and PereiraPerrin, M. (2009). Trypanosoma cruzi targets akt in host cells as an intracellular antiapoptotic strategy. Sci. Signal. 2:374. doi: 10.1126/scisignal.2000374
Chung, R. S., Woodhouse, A., Fung, S., Dickson, T. C., West, A. K., Vickers, J. C., et al. (2004). Olfactory ensheathing cells promote neurite sprouting of injured axons in vitro by direct cellular contact and secretion of soluble factors. Cell. Mol. Life Sci. 61, 1238–1245. doi: 10.1007/s00018-004-4026-y
Chung, W. S., Clarke, L. E., Wang, G. X., Stafford, B. K., Sher, A., Chakraborty, C., et al. (2013). Astrocytes mediate synapse elimination through megf10 and mertk pathways. Nature 504:394. doi: 10.1038/nature12776
Clements, M. P., Byrne, E., Guerrero, L. F. C., Cattin, A. L., Zakka, L., Ashraf, A., et al. (2017). The wound microenvironment reprograms schwann cells to invasive mesenchymal-like cells to drive peripheral nerve regeneration. Neuron 96:98. doi: 10.1016/j.neuron.2017.09.008
Crang, A. J., and Blakemore, W. F. (1986). Observations on wallerian degeneration in explant cultures of cat sciatic-nerve. J. Neurocytol. 15, 471–482. doi: 10.1007/bf01611730
Crehan, H., Hardy, J., and Pocock, J. (2012). Microglia, alzheimer’s disease, and complement. Int. J. Alzheimer Dis. 2012:983640. doi: 10.1155/2012/983640
Crehan, H., Hardy, J., and Pocock, J. (2013). Blockage of cr1 prevents activation of rodent microglia. Neurobiol. Dis. 54, 139–149. doi: 10.1016/j.nbd.2013.02.003
Damisah, E. C., Hill, R. A., Rai, A., Chen, F. Y., Rothlin, C. V., Ghosh, S., et al. (2020). Astrocytes and microglia play orchestrated roles and respect phagocytic territories during neuronal corpse removal in vivo. Sci. Adv. 6:eaba3239. doi: 10.1126/sciadv.aba3239
Dando, S. J., Ipe, D. S., Batzloff, M., Sullivan, M. J., Crossman, D. K., Crowley, M., et al. (2016). Burkholderia pseudomallei capsule exacerbates respiratory melioidosis but does not afford protection against antimicrobial signaling or bacterial killing in human olfactory ensheathing cells. Infect. Immun. 84, 1941–1956. doi: 10.1128/iai.01546-15
Dando, S. J., Mackay-Sim, A., Norton, R., Currie, B. J., St John, J. A., Ekberg, J. A. K., et al. (2014). Pathogens penetrating the central nervous system: Infection pathways and the cellular and molecular mechanisms of invasion. Clin. Microbiol. Rev. 27, 691–726. doi: 10.1128/cmr.00118-13
Dashiell, S. M., Vanguri, P., and Koski, C. L. (1997). Dibutyryl cyclic amp and inflammatory cytokines mediate c3 expression in schwann cells. Glia 20, 308–321.
De Chiara, G., Marcocci, M. E., Sgarbanti, R., Civitelli, L., Ripoli, C., Piacentini, R., et al. (2012). Infectious agents and neurodegeneration. Mol. Neurobiol. 46, 614–638. doi: 10.1007/s12035-012-8320-7
De Simone, R., Antonietta-Cat, M., Nicolini, A., and Minghetti, L. (2002). Expression of phosphatidylserine receptor and down-regulation of pro-inflammatory molecule production by its natural ligand in rat microglial cultures. J. Neuropathol. Exp. Neurol. 61, 237–244. doi: 10.1093/jnen/61.3.237
Dehhaghi, M., Panahi, H. K. S., and Guillemin, G. J. (2018). Microorganisms’ footprint in neurodegenerative diseases. Front. Cell. Neurosci. 12:466. doi: 10.3389/fncel.2018.00466
Dekkers, M. P. J., Nikoletopoulou, V., and Barde, Y. A. (2013). Death of developing neurons: new insights and implications for connectivity. J. Cell Biol. 203, 385–393. doi: 10.1083/jcb.201306136
Delbaz, A., Chen, M., Jen, F. E. C., Schulz, B. L., Gorse, A. D., Jennings, M. P., et al. (2020). Neisseria meningitidis induces pathology-associated cellular and molecular changes in trigeminal schwann cells. Infect. Immun. 88:955. doi: 10.1128/iai.00955-19
Dhiman, G., Abraham, R., and Griffin, D. E. (2019). Human schwann cells are susceptible to infection with zika and yellow fever viruses, but not dengue virus. Sci. Rep. 9:46389. doi: 10.1038/s41598-019-46389-0
Doherty, J., Logan, M. A., Tasdemir, O. E., and Freeman, M. R. (2009). Ensheathing glia function as phagocytes in the adult drosophila brain. J. Neurosci. 29, 4768–4781. doi: 10.1523/jneurosci.5951-08.2009
Dong, Z. P., Sinanan, A., Parkinson, D., Parmantier, E., Mirsky, R., and Jessen, K. R. (1999). Schwann cell development in embryonic mouse nerves. J. Neurosci. Res. 56, 334–348.
Dong, Z., Brennan, A., Liu, N., Yarden, Y., Lefkowitz, G., Mirsky, R., et al. (1995). Neu differentiation factor is a neuron-glia signal and regulates survival, proiferration and maturation of rat schwann-cell precursors. Neuron 15, 585–596. doi: 10.1016/0896-6273(95)90147-7
Doucette, R. (1989). Development of the nerve-fiber layer in the olfactory-bulb of mouse embryos. J. Comparat. Neurol. 285, 514–527. doi: 10.1002/cne.902850407
Doucette, R. (1990). Glial influences on axonal growth in the primary olfactory system. Glia 3, 433–449. doi: 10.1002/glia.440030602
Duarte, L. F., Farias, M. A., Alvarez, D. M., Bueno, S. M., Riedel, C. A., and Gonzalez, P. A. (2019). Herpes simplex virus type 1 infection of the central nervous system: insights into proposed interrelationships with neurodegenerative disorders. Front. Cell. Neurosci. 13:46. doi: 10.3389/fncel.2019.00046
Dubovy, P., Klusakova, I., and Svizenska, I. H. (2014). Inflammatory profiling of schwann cells in contact with growing axons distal to nerve injury. Biomed. Res. Int. 4:691041. doi: 10.1155/2014/691041
Ekberg, J. A. K., Amaya, D., Mackay-Sim, A., and St John, J. A. (2012). The migration of olfactory ensheathing cells during development and regeneration. Neurosignals 20, 147–158. doi: 10.1159/000330895
Ekberg, J. A. K., and St John, J. A. (2014). Crucial roles for olfactory ensheathing cells and olfactory mucosal cells in the repair of damaged neural tracts. Anatom. Record Adv. Integrat. Anat. Evol. Biol. 297, 121–128. doi: 10.1002/ar.22803
Esen, N., and Kielian, T. (2012). “Contribution of astrocytes to cns immunity roles of pattern recognition receptors (prrs),” in Astrocytes: Wiring the Brain, eds E. Scemes and D. C. Spray, 107–136. (Boca Raton, USA: Frontiers in Neuroscience, CRC Press; Taylor & Francis Group).
Farina, C., Aloisi, F., and Meinl, E. (2007). Astrocytes are active players in cerebral innate immunity. Trends Immunol. 28, 138–145. doi: 10.1016/j.it.2007.01.005
Fawcett, J. W., and Verhaagen, J. (2018). Intrinsic determinants of axon regeneration. Dev. Neurobiol. 78, 890–897. doi: 10.1002/dneu.22637
Feng, S. J., Zhuang, M. H., and Wu, R. (2012). Secretion of nerve growth factor, brain-derived neurotrophic factor, and glial cell-line derived neurotrophic factor in co-culture of four cell types in cerebrospinal fluid-containing medium. Neural Reg. Res. 7, 2907–2914. doi: 10.3969/j.issn.1673-5374.2012.36.008
Fernandezvalle, C., Bunge, R. P., and Bunge, M. B. (1995). Schwann cells degrade myelin and proliferrate in absence of macrophages-evidence from in vitro studies of wallerian degeneration. J. Neurocytol. 24, 667–679. doi: 10.1007/bf01179817
Finzsch, M., Schreiner, S., Kichko, T., Reeh, P., Tamm, E. R., Bosl, M. R., et al. (2010). Sox10 is required for schwann cell identity and progression beyond the immature schwann cell stage. J. Cell Biol. 189, 701–712. doi: 10.1083/jcb.200912142
Fitch, M. T., and Silver, J. (2008). Cns injury, glial scars, and inflammation: Inhibitory extracellular matrices and regeneration failure. Exp. Neurol. 209, 294–301. doi: 10.1016/j.expneurol.2007.05.014
Fitzer-Attas, C. J., Lowry, M., Crowley, M. T., Finn, A. J., Meng, F. Y., DeFranco, A. L., et al. (2000). Fc gamma receptor-mediated phagocytosis in macrophages lacking the src family tyrosine kinases hck, fgr, and lyn. J. Exp. Med. 191, 669–681. doi: 10.1084/jem.191.4.669
Flutsch, A., Henry, K., Gonias, S. L., and Campana, W. M. (2015). C-jun is activated by ldl receptor-related protein-1 (lrp1) in schwann cells. Glia 63, E120–E121.
Flutsch, A., Henry, K., Mantuano, E., Lam, M. S., Shibayama, M., Takahashi, K., et al. (2016). Evidence that ldl receptor-related protein 1 acts as an early injury detection receptor and activates c-jun in schwann cells. Neuroreport 27, 1305–1311. doi: 10.1097/wnr.0000000000000691
Forrester, J. V., McMenamin, P. G., and Dando, S. J. (2018). Cns infection and immune privilege. Nat. Rev. Neurosci. 19, 655–671. doi: 10.1038/s41583-018-0070-8
Fouad, K., Schnell, L., Bunge, M. B., Schwab, M. E., Liebscher, T., and Pearse, D. D. (2005). Combining schwann cell bridges and olfactory-ensheathing glia grafts with chondroitinase promotes locomotor recovery after complete transection of the spinal cord. J. Neurosci. 25, 1169–1178. doi: 10.1523/jneurosci.3562-04.2005
Fourgeaud, L., Traves, P. G., Tufail, Y., Leal-Bailey, H., Lew, E. D., Burrola, P. G., et al. (2016). Tam receptors regulate multiple features of microglial physiology. Nature 532, 240. doi: 10.1038/nature17630
Fraher, J. P., Smiddy, P. F., and Osullivan, V. R. (1988). The central peripheral transitional regions of cranial nerves-oculomotor nerve. J. Anat. 161, 103–113.
Franssen, E. H. P., De Bree, F. M., Essing, A. H. W., Ramon-Cueto, A., and Verhaagen, J. (2008). Comparative gene expression profiling of olfactory ensheathing glia and schwann cells indicates distinct tissue repair characteristics of olfactory ensheathing glia. Glia 56, 1285–1298. doi: 10.1002/glia.20697
Freeman, M. R., Delrow, J., Kim, J., Johnson, E., and Doe, C. Q. (2003). Unwrapping glial biology: Gcm target genes regulating glial development, diversification, and function. Neuron 38, 567–580. doi: 10.1016/s0896-6273(03)00289-7
Fregnan, F., Muratori, L., Simoes, A. R., Giacobini-Robecchi, M. G., and Raimondo, S. (2012). Role of inflammatory cytokines in peripheral nerve injury. Neural Reg. Res. 7, 2259–2266. doi: 10.3969/j.issn.1673-5374.2012.29.003
Fricker, M., Neher, J. J., Zhao, J. W., Thery, C., Tolkovsky, A. M., and Brown, G. C. (2012). Mfg-e8 mediates primary phagocytosis of viable neurons during neuroinflammation. J. Neurosci. 32, 2657–2666. doi: 10.1523/jneurosci.4837-11.2012
Fuentes-Medel, Y., Logan, M. A., Ashley, J., Ataman, B., Budnik, V., and Freeman, M. R. (2009). Glia and muscle sculpt neuromuscular arbors by engulfing destabilized synaptic boutons and shed presynaptic debris. PLoS Biol. 7:e1000184. doi: 10.1371/journal.pbio.1000184
Fuller, J. P., Stavenhagen, J. B., and Teeling, J. L. (2014). New roles for fc receptors in neurodegeneration-the impact on lmmunotherapy for alzheimer’s disease. Front. Neurosci. 8:e00235. doi: 10.3389/fnins.2014.00235
Galloway, D. A., Phillips, A. E. M., Owen, D. R. J., and Moore, C. S. (2019). Phagocytosis in the brain: Homeostasis and disease. Front. Immunol. 10:790. doi: 10.3389/fimmu.2019.00790
Gamble, H. J., and Eames, R. A. (1964). An electron microscope study of the connective tissues of human peripheral nerve. J. Anat. 98(Pt 4), 655–663.
Gasque, P., and Morgan, B. P. (1996). Complement regulatory protein expression by a human oligodendrocyte cell line: Cytokine regulation and comparison with astrocytes. Immunology 89, 338–347. doi: 10.1046/j.1365-2567.1996.d01-756.x
Gaultier, A., Wu, X. H., Le Moan, N., Takimoto, S., Mukandala, G., Akassoglou, K., et al. (2009). Low-density lipoprotein receptor-related protein 1 is an essential receptor for myelin phagocytosis. J. Cell Sci. 122, 1155–1162. doi: 10.1242/jcs.040717
Gavrilovic, J., Brennan, A., Mirsky, R., and Jessen, K. R. (1995). Fibroblast growth-factors and insulin growth factors combine to promote survival of rat schwann cell precursors wihtout induction of DNA synthesis. Eur. J. Neurosci. 7, 77–85. doi: 10.1111/j.1460-9568.1995.tb01022.x
George, A., Kleinschnitz, C., Zelenka, M., Brinkhoff, J., Stoll, G., and Sommer, C. (2004). Wallerian degeneration after crush or chronic constriction injury of rodent sciatic nerve is associated with a depletion of endoneurial interleukin-10 protein. Exp. Neurol. 188, 187–191. doi: 10.1016/j.expneurol.2004.02.011
George, R., and Griffin, J. W. (1994). Delayed macrophage responses and myelin clearance during wallerian degeneration in the central nervous system: the dorsal radiculotomy model. Exp. Neurol. 129, 225–236. doi: 10.1006/exnr.1994.1164
Gerard, H. C., Dreses-Werringloer, U., Wildt, K. S., Deka, S., Oszust, C., Balin, B. J., et al. (2006). Chlamydophila (chlamydia) pneumoniae in the alzheimer’s brain. Fems Immunol. Med. Microbiol. 48, 355–366. doi: 10.1111/j.1574-695X.2006.00154.x
Gilmour, A. D., Reshamwala, R., Wright, A. A., Ekberg, J. A. K., and St John, J. A. (2020). Optimizing olfactory ensheathing cell transplantation for spinal cord injury repair. J. Neurotr. 37, 817–829. doi: 10.1089/neu.2019.6939
Gitsels, A., Sanders, N., and Vanrompay, D. (2019). Chlamydial infection from outside to inside. Front. Microbiol. 10:2329. doi: 10.3389/fmicb.2019.02329
Goethals, S., Ydens, E., Timmerman, V., and Janssens, S. (2010). Toll-like receptor expression in the peripheral nerve. Glia 58, 1701–1709. doi: 10.1002/glia.21041
Gomez-Sanchez, J. A., Carty, L., Iruarrizaga-Lejarreta, M., Palomo-Irigoyen, M., Varela-Rey, M., Griffith, M., et al. (2015). Schwann cell autophagy, myelinophagy, initiates myelin clearance from injured nerves. J. Cell Biol. 210, 153–168. doi: 10.1083/jcb.201503019
Gomez-Sanchez, J. A., Pilch, K. S., van der Lans, M., Fazal, S. V., Benito, C., Wagstaff, L. J., et al. (2017). After nerve injury, lineage tracing shows that myelin and remak schwann cells elongate extensively and branch to form repair schwann cells, which shorten radically on remyelination. J. Neurosci. 37, 9086–9099. doi: 10.1523/jneurosci.1453-17.2017
Graziadei, G. A. M., and Graziadei, P. P. C. (1979). Neurogenesis and neuron regeneration in the olfactory system of mammals. 2. Degeneration and reconstitution of the olfactory sensory neurons after axotomy. J. Neurocytol. 8, 197–213.
Griffiths, M. R., Gasque, P., and Neal, J. W. (2009). The multiple roles of the innate immune system in the regulation of apoptosis and inflammation in the brain. J. Neuropathol. Exp. Neurol. 68, 217–226. doi: 10.1097/NEN.0b013e3181996688
Grinspan, J. B., Marchionni, M. A., Reeves, M., Coulaloglou, M., and Scherer, S. S. (1996). Axonal interactions regulate schwann cell apoptosis in developing peripheral nerve: Neuregulin receptors and the role of neuregulins. J. Neurosci. 16, 6107–6118.
Groh, J., Weis, J., Zieger, H., Stanley, E. R., Heuer, H., and Martini, R. (2012). Colony-stimulating factor-1 mediates macrophage-related neural damage in a model for charcot-marie-tooth disease type 1x. Brain 135, 88–104. doi: 10.1093/brain/awr283
Guo, J. B., Cao, G. H., Yang, G. Q., Zhang, Y. M., Wang, Y. K., Song, W., et al. (2020). Transplantation of activated olfactory ensheathing cells by curcumin strengthens regeneration and recovery of function after spinal cord injury in rats. Cytotherapy 22, 301–312. doi: 10.1016/j.jcyt.2020.03.002
Hamon, Y., Trompier, D., Ma, Z., Venegas, V., Pophillat, M., Mignotte, V., et al. (2006). Cooperation between engulfment receptors: the case of abca1 and megf10. PLoS One 1:120. doi: 10.1371/journal.pone.0000120
Han, C., Song, Y. Q., Xiao, H., Wang, D. N., Franc, N. C., Jan, L. Y., et al. (2014). Epidermal cells are the primary phagocytes in the fragmentation and clearance of degenerating dendrites in drosophila. Neuron 81, 544–560. doi: 10.1016/j.neuron.2013.11.021
Hanayama, R., Tanaka, M., Miwa, K., Shinohara, A., Iwamatsu, A., and Nagata, S. (2002). Identification of a factor that links apoptotic cells to phagocytes. Nature 417, 182–187. doi: 10.1038/417182a
Hantke, J., Carty, L., Wagstaff, L. J., Turmaine, M., Wilton, D. K., Quintes, S., et al. (2014). C-jun activation in schwann cells protects against loss of sensory axons in inherited neuropathy. Brain 137, 2922–2937. doi: 10.1093/brain/awu257
Hao, D. J., Liu, C. C., Zhang, L. L., Chen, B., Zhang, Q., Zhang, R., et al. (2017). Lipopolysaccharide and curcumin co-stimulation potentiates olfactory ensheathing cell phagocytosis via enhancing their activation. Neurotherapeutics 14, 502–518. doi: 10.1007/s13311-016-0485-8
Harris, J. A., West, A. K., and Chuah, M. I. (2009). Olfactory ensheathing cells: Nitric oxide production and innate immunity. Glia 57, 1848–1857. doi: 10.1002/glia.20899
Hartlehnert, M., Derksen, A., Hagenacker, T., Kindermann, D., Schafers, M., Pawlak, M., et al. (2017). Schwann cells promote post-traumatic nerve inflammation and neuropathic pain through mhc class ii. Sci. Rep. 7:12744. doi: 10.1038/s41598-017-12744-2
He, B. R., Xie, S. T., Wu, M. M., Hao, D. J., and Yang, H. (2014). Phagocytic removal of neuronal debris by olfactory ensheathing cells enhances neuronal survival and neurite outgrowth via p38mapk activity. Mol. Neurobiol. 49, 1501–1512. doi: 10.1007/s12035-013-8588-2
Healy, L. M., Perron, G., Won, S. Y., Michell-Robinson, M. A., Rezk, A., Ludwin, S. K., et al. (2016). Mertk is a functional regulator of myelin phagocytosis by human myeloid cells. J. Immunol. 196, 3375–3384. doi: 10.4049/jimmunol.1502562
Henson, P. M. (2017). Cell removal: efferocytosis. Annu. Rev. Cell. Dev. Biol. 33, 127–144. doi: 10.1146/annurev-cellbio-111315-125315
Herbert, R. P., Harris, J., Chong, K. P., Chapman, J., West, A. K., and Chuah, M. I. (2012). Cytokines and olfactory bulb microglia in response to bacterial challenge in the compromised primary olfactory pathway. J. Neuroinflamm. 9:109. doi: 10.1186/1742-2094-9-109
Herrera, A. A., and Zeng, Y. (2003). Activity-dependent switch from synapse formation to synapse elimination during development of neuromuscular junctions. J. Neurocytol. 32, 817–833. doi: 10.1023/B:NEUR.0000020626.29900.fb
Hirata, K., and Kawabuchi, M. (2002). Myelin phagocytosis by macrophages and nonmacrophages during wallerian degeneration. Microsc. Res. Tech. 57, 541–547. doi: 10.1002/jemt.10108
Hirata, K., Mitoma, H., Ueno, N., He, J. W., and Kawabuchi, M. (1999). Differential response of macrophage subpopulations to myelin degradation in the injured rat sciatic nerve. J. Neurocytol. 28, 685–695. doi: 10.1023/a:1007012916530
Hoopfer, E. D., McLaughlin, T., Watts, R. J., Schuldiner, O., O’Leary, D. D., and Luo, L. (2006). Wlds protection distinguishes axon degeneration following injury from naturally occurring developmental pruning. Neuron 50, 883–895. doi: 10.1016/j.neuron.2006.05.013
Huang, H. C., Chen, L., Zhang, H. X., Li, S. F., Liu, P., Zhao, T. Y., et al. (2016). Autophagy promotes peripheral nerve regeneration and motor recovery following sciatic nerve crush injury in rats. J. Mol. Neurosci. 58, 416–423. doi: 10.1007/s12031-015-0672-9
Huo, S. J., Li, Y. C., Xie, J., Li, Y., Raisman, G., Zeng, Y. X., et al. (2012). Transplanted olfactory ensheathing cells reduce retinal degeneration in royal college of surgeons rats. Curr. Eye Res. 37, 749–758. doi: 10.3109/02713683.2012.697972
Hutton, E. J., Carty, L., Laura, M., Houlden, H., Lunn, M. P. T., Brandner, S., et al. (2011). C-jun expression in human neuropathies: a pilot study. J. Periph. Nerv. Syst. 16, 295–303. doi: 10.1111/j.1529-8027.2011.00360.x
Iram, T., Ramirez-Ortiz, Z., Byrne, M. H., Coleman, U. A., Kingery, N. D., Means, T. K., et al. (2016). Megf10 is a receptor for c1q that mediates clearance of apoptotic cells by astrocytes. J. Neurosci. 36, 5185–5192. doi: 10.1523/jneurosci.3850-15.2016
Itzhaki, R. E., Wozniak, M. A., Appelt, D. M., and Balin, B. (2004). Infiltration of the brain by pathogens causes alzheimer’s disease. Neurobiol. Aging 25, 619–627. doi: 10.1016/j.neurobiolaging.2003.12.021
Itzhaki, R. F., Lathe, R., Balin, B. J., Ball, M. J., Bearer, E. L., Braak, H., et al. (2016). Microbes and alzheimer’s disease. J. Alzheimers Dis. 51, 979–984. doi: 10.3233/JAD-160152
Jack, C. S., Arbour, N., Manusow, J., Montgrain, V., Blain, M., McCrea, E., et al. (2005). Tlr signaling tailors innate immune responses in human microglia and astrocytes. J. Immunol. 175, 4320–4330. doi: 10.4049/jimmunol.175.7.4320
Jang, S. Y., Shin, Y. K., Park, S. Y., Park, J. Y., Lee, H. J., Yoo, Y. H., et al. (2016). Autophagic myelin destruction by schwann cells during wallerian degeneration and segmental demyelination. Glia 64, 730–742. doi: 10.1002/glia.22957
Jang, S. Y., Shin, Y. K., Park, S. Y., Park, J. Y., Rha, S. H., Kim, J. K., et al. (2015). Autophagy is involved in the reduction of myelinating schwann cell cytoplasm during myelin maturation of the peripheral nerve. PLoS One 10:e0116624. doi: 10.1371/journal.pone.0116624
Jarolim, K. L., McCosh, J. K., Howard, M. J., and John, D. T. (2000). A light microscopy study of the migration of naegleria fowleri from the nasal submucosa to the central nervous system during the early stage of primary amebic meningoencephalitis in mice. J. Parasitol. 86, 50–55.
Jessen, K. R., and Mirsky, R. (2008). Negative regulation of myelination: Relevance for development, injury, and demyelinating disease. Glia 56, 1552–1565. doi: 10.1002/glia.20761
Jessen, K. R., and Mirsky, R. (2016). The repair schwann cell and its function in regenerating nerves. J. Physiol. 594, 3521–3531. doi: 10.1113/JP270874
Jessen, K. R., and Mirsky, R. (2019). The success and failure of the schwann cell response to nerve injury. Front. Cell. Neurosci. 13:33. doi: 10.3389/fncel.2019.00033
Jessen, K. R., Brennan, A., Morgan, L., Mirsky, R., Kent, A., Hashimoto, Y., et al. (1994). The schwann-cell precursor and its fate-a study of cell-death and differentiation during gliogenesis in rat embryonic nerves. Neuron 12, 509–527. doi: 10.1016/0896-6273(94)90209-7
Jessen, K. R., Mirsky, R., and Lloyd, A. C. (2015). Schwann cells: Development and role in nerve repair. Cold Spring Harb. Perspect. Biol. 7:a020487. doi: 10.1101/cshperspect.a020487
Jones, R. S., Minogue, A. M., Connor, T. J., and Lynch, M. A. (2013). Amyloid-beta-induced astrocytic phagocytosis is mediated by cd36, cd47 and rage. J. Neuroimm. Pharmacol. 8, 301–311. doi: 10.1007/s11481-012-9427-3
Joshi, A. R., Holtmann, L., Bobylev, I., Schneider, C., Ritter, C., Weis, J., et al. (2016). Loss of schwann cell plasticity in chronic inflammatory demyelinating polyneuropathy (cidp). J. Neuroinflamm. 13:255. doi: 10.1186/s12974-016-0711-7
Juneja, M., Burns, J., Saporta, M. A., and Timmerman, V. (2019). Challenges in modelling the charcot-marie-tooth neuropathies for therapy development. J. Neurol. Neurosurg. Psychiatry 90, 58–67. doi: 10.1136/jnnp-2018-318834
Jung, J., Cai, W., Lee, H. K., Pellegatta, M., Shin, Y. K., Jang, S. Y., et al. (2011). Actin polymerization is essential for myelin sheath fragmentation during wallerian degeneration. J. Neurosci. 31, 2009–2015. doi: 10.1523/jneurosci.4537-10.2011
Jung, Y. J., and Chung, W. S. (2018). Phagocytic roles of glial cells in healthy and diseased brains. Biomol. Therap. 26, 350–357. doi: 10.4062/biomolther.2017.133
Kafitz, K. W., and Greer, C. A. (1999). Olfactory ensheathing cells promote neurite extension from embryonic olfactory receptor cells in vitro. Glia 25, 99–110. doi: 10.1002/(sici)1098-1136(19990115)25:2<99::Aid-glia1<3.3.Co;2-m
Kanno, H., Pearse, D. D., Ozawa, H., Itoi, E., and Bunge, M. B. (2015). Schwann cell transplantation for spinal cord injury repair: Its significant therapeutic potential and prospectus. Rev. Neurosci. 26, 121–128. doi: 10.1515/revneuro-2014-0068
Katoh, H., Shibata, S., Fukuda, K., Sato, M., Satoh, E., Nagoshi, N., et al. (2011). The dual origin of the peripheral olfactory system: placode and neural crest. Mol. Brain 4:34. doi: 10.1186/1756-6606-4-34
Kaur, C., Too, H. F., and Ling, E. A. (2004). Phagocytosis of escherichia coli by amoeboid microglial cells in the developing brain. Acta Neuropathol. 107, 204–208. doi: 10.1007/s00401-003-0798-7
Kerschensteiner, M., Schwab, M. E., Lichtman, J. W., and Misgeld, T. (2005). In vivo imaging of axonal degeneration and regeneration in the injured spinal cord. Nat. Med. 11, 572–577. doi: 10.1038/nm1229
Khankan, R. R., Griffis, K. G., Haggerty-Skeans, J. R., Zhong, H., Roy, R. R., Edgerton, V. R., et al. (2016). Olfactory ensheathing cell transplantation after a complete spinal cord transection mediates neuroprotective and immunomodulatory mechanisms to facilitate regeneration. J. Neurosci. 36, 6269–6286. doi: 10.1523/jneurosci.0085-16.2016
Kim, H. A., Pomeroy, S. L., Whoriskey, W., Pawlitzky, I., Benowitz, L. I., Sicinski, P., et al. (2000). A developmentally regulated switch directs regenerative growth of schwann cells through cyclin d1. Neuron 26, 405–416. doi: 10.1016/s0896-6273(00)81173-3
Klein, D., Groh, J., Weishaupt, A., and Martini, R. (2015). Endogenous antibodies contribute to macrophage-mediated demyelination in a mouse model for cmt1b. J. Neuroinflamm. 12:49. doi: 10.1186/s12974-015-0267-y
Kobayashi, M., Ishibashi, S., Tomimitsu, H., Yokota, T., and Mizusawa, H. (2012). Proliferating immature schwann cells contribute to nerve regeneration after ischemic peripheral nerve injury. J. Neuropathol. Exp. Neurol. 71, 511–519. doi: 10.1097/NEN.0b013e318257fe7b
Kobsar, I., Hasenpusch-Theil, K., Wessig, C., Muller, H. W., and Martini, R. (2005). Evidence for macrophage-mediated myelin disruption in an animal model for charcot-marie-tooth neuropathy type 1a. J. Neurosci. Res. 81, 857–864. doi: 10.1002/jnr.20601
Kocsis, J. D., and Waxman, S. G. (2007). Schwann cells and their precursors for repair of central nervous system myelin. Brain 130, 1978–1980. doi: 10.1093/brain/awm161
Koenigsknecht, J., and Landreth, G. (2004). Microglial phagocytosis of fibrillar beta-amyloid through a beta(1) integrin-dependent mechanism. J. Neurosci. 24, 9838–9846. doi: 10.1523/jneurosci.2557-04.2004
Konishi, H., Okamoto, T., Hara, Y., Komine, O., Tamada, H., Maeda, M., et al. (2020). Astrocytic phagocytosis is a compensatory mechanism for microglial dysfunction. Embo J. 39:464. doi: 10.15252/embj.2020104464
Korin, B., Ben-Shaanan, T. L., Schiller, M., Dubovik, T., Azulay-Debby, H., Boshnak, N. T., et al. (2017). High-dimensional, single-cell characterization of the brain’s immune compartment. Nat. Neurosci. 20:1300. doi: 10.1038/nn.4610
Korin, B., Dubovik, T., and Rolls, A. (2018). Mass cytometry analysis of immune cells in the brain. Nat. Protoc. 13, 377–391. doi: 10.1038/nprot.2017.155
Kotter, M. R., Li, W. W., Zhao, C., and Franklin, R. J. (2006). Myelin impairs cns remyelination by inhibiting oligodendrocyte precursor cell differentiation. J. Neurosci. 26, 328–332. doi: 10.1523/jneurosci.2615-05.2006
Kucenas, S. (2015). Perineurial glia. Cold Spring Harb. Perspect. Biol. 7:a020511. doi: 10.1101/cshperspect.a020511
Kumar, V., and Sengupta, U. (2003). Ultrastructural study of schwann cells and endothelial cells in the pathogenesis of leprous neuropathy. Int. J. Lepros. Other Mycobact. Dis. 71, 328–340. doi: 10.1489/1544-581x2003071<0328:Usosca<2.0.Co;2
Lakatos, A., Franklin, R. J., and Barnett, S. C. (2000). Olfactory ensheathing cells and schwann cells differ in their in vitro interactions with astrocytes. Glia 32, 214–225.
Lankford, K. L., Sasaki, M., Radtke, C., and Kocsis, J. D. (2008). Olfactory ensheathing cells exhibit unique migratory, phagocytic, and myelinating properties in the x-irradiated spinal cord not shared by schwann cells. Glia 56, 1664–1678. doi: 10.1002/glia.20718
Le, N., Nagarajan, R., Wang, J. Y. T., Araki, T., Schmidt, R. E., and Milbrandt, J. (2005). Analysis of congenital hypomyelinating egr2(lo/lo) nerves identifies sox2 as an inhibitor of schwann cell differentiation and myelination. Proc. Natl. Acad. Sci. U.S.A. 102, 2596–2601. doi: 10.1073/pnas.0407836102
Ledouarin, N. M., and Smith, J. (1988). Development of the peripheral nervous-system from the neural crest. Annu. Rev. Cell Biol. 4, 375–404. doi: 10.1146/annurev.cellbio.4.1.375
Ledouarin, N., Dulac, C., Dupin, E., and Cameroncurry, P. (1991). Glial-cell lineages in the neural crest. Glia 4, 175–184.
Lee, C. Y. D., and Landreth, G. E. (2010). The role of microglia in amyloid clearance from the ad brain. J. Neural Trans. 117, 949–960. doi: 10.1007/s00702-010-0433-4
Lee, H., Park, C., Cho, I. H., Kim, H. Y., Jo, E. K., Lee, S., et al. (2007). Double-stranded rna induces inos gene expression in schwann cells, sensory neuronal death, and peripheral nerve demyelination. Glia 55, 712–722. doi: 10.1002/glia.20493
Lee, K. M., Chiu, K. B., Sansing, H. A., Didier, P. J., Ficht, T. A., Arenas-Gamboa, A. M., et al. (2013). Aerosol-induced brucellosis increases tlr-2 expression and increased complexity in the microanatomy of astroglia in rhesus macaques. Front. Cell. Infect. Microbiol. 3:86. doi: 10.3389/fcimb.2013.00086
Lee, S., Bazick, H., Chittoor-Vinod, V., Al Salihi, M. O., Xia, G. B., and Notterpek, L. (2018). Elevated peripheral myelin protein 22, reduced mitotic potential, and proteasome impairment in dermal fibroblasts from charcot-marie-tooth disease type 1a patients. Am. J. Pathol. 188, 728–738. doi: 10.1016/j.ajpath.2017.10.021
Leung, J. Y. K., Chapman, J. A., Harris, J. A., Hale, D., Chung, R. S., West, A. K., et al. (2008). Olfactory ensheathing cells are attracted to, and can endocytose, bacteria. Cell. Mol. Life Sci. 65, 2732–2739. doi: 10.1007/s00018-008-8184-1
Lewis, G. M., and Kucenas, S. (2014). Perineurial glia are essential for motor axon regrowth following nerve injury. J. Neurosci. 34, 12762–12777. doi: 10.1523/jneurosci.1906-14.2014
Li, R., Li, D. H., Wu, C. B., Ye, L. B., Wu, Y. Q., Yuan, Y., et al. (2020). Nerve growth factor activates autophagy in schwann cells to enhance myelin debris clearance and to expedite nerve regeneration. Theranostics 10, 1649–1677. doi: 10.7150/thno.40919
Li, Y. J., Zou, T., Xue, L. Y., Yin, Z. Q., Huo, S. J., and Xu, H. W. (2017). Tgf-beta 1 enhances phagocytic removal of neuron debris and neuronal survival by olfactory ensheathing cells via integrin/mfg-e8 signaling pathway. Mol. Cell. Neurosci. 85, 45–56. doi: 10.1016/j.mcn.2017.08.006
Li, Y., Li, D., and Raisman, G. (2005). Interaction of olfactory ensheathing cells with astrocytes may be the key to repair of tract injuries in the spinal cord: The ‘pathway hypothesis’. J. Neurocytol. 34, 343–351. doi: 10.1007/s11068-005-8361-1
Lindborg, J. A., Mack, M., and Zigmond, R. E. (2017). Neutrophils are critical for myelin removal in a peripheral nerve injury model of wallerian degeneration. J. Neurosci. 37, 10258–10277. doi: 10.1523/jneurosci.2085-17.2017
Lipson, A. C., Widenfalk, J., Lindqvist, E., Ebendal, T., and Olson, L. (2003). Neurotrophic properties of olfactory ensheathing glia. Exp. Neurol. 180, 167–171. doi: 10.1016/s0014-4886(02)00058-4
Little, C. S., Hammond, C. J., MacIntyre, A., Balin, B. J., and Appelt, D. M. (2004). Chlamydia pneumoniae induces alzheimer-like amyloid plaques in brains of balb/c mice. Neurobiol. Aging 25, 419–429. doi: 10.1016/s0197-4580(03)00127-1
Little, C. S., Joyce, T. A., Hammond, C. J., Matta, H., Cahn, D., Appelt, D. M., et al. (2014). Detection of bacterial antigens and alzheimer’s disease-like pathology in the central nervous system of balb/c mice following intranasal infection with a laboratory isolate of chlamydia pneumoniae. Front. Aging Neurosci. 6:304. doi: 10.3389/fnagi.2014.00304
Liu, C. C., Hu, J., Zhao, N., Wang, J., Wang, N., Cirrito, J. R., et al. (2017). Astrocytic lrp1 mediates brain a beta clearance and impacts amyloid deposition. J. Neurosci. 37, 4023–4031. doi: 10.1523/jneurosci.3442-16.2017
Liu, G. M., Xu, K., Li, J., and Luo, Y. G. (2016). Curcumin upregulates s100 expression and improves regeneration of the sciatic nerve following its complete amputation in mice. Neural Regenerat. Res. 11, 1304–1311. doi: 10.4103/1673-5374.189196
Liu, H. M. (1974). Schwann cell properties. 2. Identity of phagocytes in degenerating nerve. Am. J. Pathol. 75, 395–416.
Liu, H. M., Yang, L. H., and Yang, Y. J. (1995). Schwann cell properties: 3. C-fos expression, bfgf production, phagocytosis and proliferation during wallerian degeneration. J. Neuropathol. Exp. Neurol. 54, 487–496.
Loov, C., Hillered, L., Ebendal, T., and Erlandsson, A. (2012). Engulfing astrocytes protect neurons from contact-induced apoptosis following injury. PLoS One 7:e33090. doi: 10.1371/journal.pone.0033090
Lopez-Cebral, R., Silva-Correia, J., Reis, R. L., Silva, T. H., and Oliveira, J. M. (2017). Peripheral nerve injury: Current challenges, conventional treatment approaches, and new trends in biomaterials-based regenerative strategies. Acs Biomater. Sci. Eng. 3, 3098–3122. doi: 10.1021/acsbiomaterials.7b00655
Lopez-Vales, R., Garcia-Alias, G., Fores, J., Vela, J. M., Navarro, X., and Verdu, E. (2004). Transplanted olfactory ensheathing cells modulate the inflammatory response in the injured spinal cord. Neur. Glia Biol. 1, 201–209. doi: 10.1017/s1740925x05000037
Lorenzana, A. O., Lee, J. K., Mui, M., Chang, A., and Zheng, B. H. (2015). A surviving intact branch stabilizes remaining axon architecture after injury as revealed by in vivo imaging in the mouse spinal cord. Neuron 86, 947–954. doi: 10.1016/j.neuron.2015.03.061
Loring, J. F., and Erickson, C. A. (1987). Neural crest cell migratory pathways in the trunk of the chick-embryo. Dev. Biol. 121, 220–236. doi: 10.1016/0012-1606(87)90154-0
Louveau, A., Smirnov, I., Keyes, T. J., Eccles, J. D., Rouhani, S. J., Peske, J. D., et al. (2015). Structural and functional features of central nervous system lymphatic vessels. Nature 523:337. doi: 10.1038/nature14432
Lunnon, K., Teeling, J. L., Tutt, A. L., Cragg, M. S., Glennie, M. J., and Perry, V. H. (2011). Systemic inflammation modulates fc receptor expression on microglia during chronic neurodegeneration. J. Immunol. 186, 7215–7224. doi: 10.4049/jimmunol.0903833
Lutz, A. B., and Barres, B. A. (2014). Contrasting the glial response to axon injury in the central and peripheral nervous systems. Dev. Cell 28, 7–17. doi: 10.1016/j.devcel.2013.12.002
Lutz, A. B., Chung, W. S., Sloan, S. A., Carson, G. A., Zhou, L., Lovelett, E., et al. (2017). Schwann cells use tam receptor-mediated phagocytosis in addition to autophagy to clear myelin in a mouse model of nerve injury. Proc. Natl. Acad. Sci. U.S.A. 114, E8072–E8080. doi: 10.1073/pnas.1710566114
Macedo-Ramos, H., Batista, A. F., Carrier-Ruiz, A., Alves, L., Allodi, S., Ribeiro-Resende, V. T., et al. (2014). Evidence of involvement of the mannose receptor in the internalization of streptococcus pneumoniae by schwann cells. BMC Microbiol. 14:211. doi: 10.1186/s12866-014-0211-9
Macedo-Ramos, H., Campos, F. S. O., Carvalho, L. A., Ramos, I. B., Teixeira, L. M., De Souza, W., et al. (2011). Olfactory ensheathing cells as putative host cells for streptococcus pneumoniae: evidence of bacterial invasion via mannose receptor-mediated endocytosis. Neurosci. Res. 69, 308–313. doi: 10.1016/j.neures.2010.12.015
Macedo-Ramos, H., Ruiz-Mendoza, S., Mariante, R. M., Guimaraes, E. V., Quadros-De-Souza, L. C., Paiva, M. M., et al. (2016). Streptococcus pneumoniae resists intracellular killing by olfactory ensheathing cells but not by microglia. Sci. Rep. 6:36813. doi: 10.1038/srep36813
Mackaysim, A., and Kittel, P. (1991). Cell-dynamics in the adult-mouse olfactory epithelium – a quantitative autoradiographic study. J. Neurosci. 11, 979–984.
Man, L. L., Liu, F., Wang, Y. J., Song, H. H., Xu, H. B., Zhu, Z. W., et al. (2015). The hmgb1 signaling pathway activates the inflammatory response in schwann cells. Neural Regen. Res. 10, 1706–1712. doi: 10.4103/1673-5374.167773
Mariani, M. M., and Kielian, T. (2009). Microglia in infectious diseases of the central nervous system. J. Neuroimm. Pharmacol. 4, 448–461. doi: 10.1007/s11481-009-9170-6
Marinelli, S., Nazio, F., Tinari, A., Ciarlo, L., D’Amelio, M., Pieroni, L., et al. (2014). Schwann cell autophagy counteracts the onset and chronification of neuropathic pain. Pain 155, 93–107. doi: 10.1016/j.pain.2013.09.013
Martini, R., Klein, D., and Groh, J. (2013). Similarities between inherited demyelinating neuropathies and wallerian degeneration an old repair program may cause myelin and axon perturbation under non lesion conditions. Am. J. Pathol. 183, 655–660. doi: 10.1016/j.ajpath.2013.06.002
Marzolo, M. P., von Bernhardi, R., and Inestrosa, N. C. (1999). Mannose receptor is present in a functional state in rat microglial cells. J. Neurosci. Res. 58, 387–395. doi: 10.1002/(sici)1097-4547(19991101)58:3<387::Aid-jnr4<3.0.Co;2-l
Masaki, T., McGlinchey, A., Tomlinson, S. R., Qu, J., and Rambukkana, A. (2013a). Reprogramming diminishes retention of mycobacterium leprae in schwann cells and elevates bacterial transfer property to fibroblasts [version 3; referees: 3 approved]. F1000Research 2:198. doi: 10.12688/f1000research.2-198.v3
Masaki, T., McGlinchey, A., Cholewa-Waclaw, J., Qu, J. R., Tomlinson, S. R., and Rambukkana, A. (2014). Innate immune response precedes mycobacterium leprae-induced reprogramming of adult schwann cells. Cell. Reprogramm. 16, 9–17. doi: 10.1089/cell.2013.0064
Masaki, T., Qu, J. R., Cholewa-Waclaw, J., Burr, K., Raaum, R., and Rambukkana, A. (2013b). Reprogramming adult schwann cells to stem cell-like cells by leprosy bacilli promotes dissemination of infection. Cell 152, 51–67. doi: 10.1016/j.cell.2012.12.014
Mattos, K. A., Oliveira, V. G. C., D’Avila, H., Rodrigues, L. S., Pinheiro, R. O., Sarno, E. N., et al. (2011). Tlr6-driven lipid droplets in mycobacterium leprae-infected schwann cells: Immunoinflammatory platforms associated with bacterial persistence. J. Immunol. 187, 2548–2558. doi: 10.4049/jimmunol.1101344
Meinhardt, J., Radke, J., Dittmayer, C., Franz, J., Thomas, C., Mothes, R., et al. (2020). Olfactory transmucosal sars-cov-2 invasion as a port of central nervous system entry in individuals with covid-19. Nat. Neurosci. 24, 168–175. doi: 10.1038/s41593-020-00758-5
Mietto, B. S., Mostacada, K., and Martinez, A. M. B. (2015). Neurotrauma and inflammation: Cns and pns responses. Mediat. Inflamm. 2015:251204. doi: 10.1155/2015/251204
Miklossy, J., and Van der Loos, H. (1991). The long-distance effects of brain lesions: Visualization of myelinated pathways in the human brain using polarizing and fluorescence microscopy. J. Neuropathol. Exp. Neurol. 50, 1–15. doi: 10.1097/00005072-199101000-00001
Mills, E. A., Davis, C. H. O., Bushong, E. A., Boassa, D., Kim, K. Y., Ellisman, M. H., et al. (2015). Astrocytes phagocytose focal dystrophies from shortening myelin segments in the optic nerve of xenopus laevis at metamorphosis. Proc. Natl. Acad. Sci. U.S.A. 112, 10509–10514. doi: 10.1073/pnas.1506486112
Mirsky, R., Arthur-Farraj, P., Latouche, M., Wilton, D., Quintes, S., Chabrol, E., et al. (2013). Schwann cell c-jun is essential for successful peripheral nerve regeneration. Glia 61, S204–S205.
Mombaerts, P., Wang, F., Dulac, C., Chao, S. K., Nemes, A., Mendelsohn, M., et al. (1996). Visualizing an olfactory sensory map. Cell 87, 675–686. doi: 10.1016/s0092-8674(00)81387-2
Monk, K. R., Feltri, M. L., and Taveggia, C. (2015). New insights on schwann cell development. Glia 63, 1376–1393. doi: 10.1002/glia.22852
Morizawa, Y. M., Hirayama, Y., Ohno, N., Shibata, S., Shigetomi, E., Sui, Y., et al. (2017). Reactive astrocytes function as phagocytes after brain ischemia via abca1-mediated pathway. Nat. Commun. 8:37. doi: 10.1038/s41467-017-00037-1
Moseman, E. A. (2020). Battling brain-eating amoeba: Enigmas surrounding immunity to naegleria fowleri. PLoS Pathog. 16:e1008406. doi: 10.1371/journal.ppat.1008406
Mosley, K., and Cuzner, M. L. (1996). Receptor-mediated phagocytosis of myelin by macrophages and microglia: Effect of opsonization and receptor blocking agents. Neurochem. Res. 21, 481–487. doi: 10.1007/bf02527713
Mosser, C. A., Baptista, S., Arnoux, I., and Audinat, E. (2017). Microglia in cns development: shaping the brain for the future. Progr. Neurobiol. 149-150, 1–20. doi: 10.1016/j.pneurobio.2017.01.002
Mucignat-Caretta, C., Redaelli, M., and Caretta, A. (2012). One nose, one brain: Contribution of the main and accessory olfactory system to chemosensation. Front. Neuroanat. 6:46. doi: 10.3389/fnana.2012.00046
Mueller, M., Leonhard, C., Wacker, K., Ringelstein, E. B., Okabe, M., Hickey, W. F., et al. (2003). Macrophage response to peripheral nerve injury: the quantitative contribution of resident and hematogenous macrophages. Laborat. Investigat. 83, 175–185. doi: 10.1097/01.Lab.0000056993.28149.Bf
Murtaza, M., Chacko, A., Delbaz, A., Reshamwala, R., Rayfield, A., McMonagle, B., et al. (2019). Why are olfactory ensheathing cell tumors so rare? Cancer Cell Int. 19:260. doi: 10.1186/s12935-019-0989-5
Mutso, M., St John, J. A., Ling, Z. L., Burt, F. J., Poo, Y. S., Liu, X., et al. (2020). Basic insights into zika virus infection of neuroglial and brain endothelial cells. J. Gen. Virol. 101, 622–634. doi: 10.1099/jgv.0.001416
Nagarajan, R., Le, N., Mahoney, H., Araki, T., and Milbrandt, J. (2002). Deciphering peripheral nerve myelination by using schwann cell expression profiling. Proc. Natl. Acad. Sci. U.S.A. 99, 8998–9003. doi: 10.1073/pnas.132080999
Napoli, I., Noon, L. A., Ribeiro, S., Kerai, A. P., Parrinello, S., Rosenberg, L. H., et al. (2012). A central role for the erk-signaling pathway in controlling schwann cell plasticity and peripheral nerve regeneration in vivo. Neuron 73, 729–742. doi: 10.1016/j.neuron.2011.11.031
Nathaniel, E. J. H., and Pease, D. C. (1963). Degenerative changes in rat dorsal roots during wallerian degeneration. J. Ultrastruct. Res. 9:511. doi: 10.1016/s0022-5320(63)80082-9
Nau, R., Ribes, S., Djukic, M., and Eiffert, H. (2014). Strategies to increase the activity of microglia as efficient protectors of the brain against infections. Front. Cell. Neurosci. 8:138. doi: 10.3389/fncel.2014.00138
Nazareth, L., Chen, M., Shelper, T., Shah, M., Velasquez, J. T., Walkden, H., et al. (2019). Novel insights into the glia limitans of the olfactory nervous system. J. Comparat. Neurol. 527, 1228–1244. doi: 10.1002/cne.24618
Nazareth, L., Lineburg, K. E., Chuah, M. I., Velasquez, J. T., Chehrehasa, F., St John, J. A., et al. (2015a). Olfactory ensheathing cells are the main phagocytic cells that remove axon debris during early development of the olfactory system. J. Comparat. Neurol. 523, 479–494. doi: 10.1002/cne.23694
Nazareth, L., Shelper, T. B., Chacko, A., Basu, S., Delbaz, A., Lee, J. Y. P., et al. (2020). Key differences between olfactory ensheathing cells and schwann cells regarding phagocytosis of necrotic cells: Implications for transplantation therapies. Sci. Rep. 10:18936. doi: 10.1038/s41598-020-75850-8
Nazareth, L., Velasquez, J. T., Lineburg, K. E., Chehrehasa, F., St John, J. A., and Ekberg, J. A. K. (2015b). Differing phagocytic capacities of accessory and main olfactory ensheathing cells and the implication for olfactory glia transplantation therapies. Mol. Cell. Neurosci. 65, 92–101. doi: 10.1016/j.mcn.2015.03.005
Nazareth, L., Walkden, H., Chacko, A., Delbaz, A., Shelper, T. B., Armitage, C. W., et al. (2021). Chlamydia muridarum can invade the central nervous system via the olfactory and trigeminal nerves and infect peripheral nerve glial cells. Front. Cell. Infect. Microbiol. 10:e607779. doi: 10.3389/fcimb.2020.607779
Neal, J. W., and Gasque, P. (2016). The role of primary infection of schwann cells in the aetiology of infective inflammatory neuropathies. J. Infect. 73, 402–418. doi: 10.1016/j.jinf.2016.08.006
Neher, J. J., Emmrich, J. V., Fricker, M., Mander, P. K., Thery, C., and Brown, G. C. (2013). Phagocytosis executes delayed neuronal death after focal brain ischemia. Proc. Natl. Acad. Sci. U.S.A. 110, E4098–E4107. doi: 10.1073/pnas.1308679110
Nicks, J., Lee, S., Harris, A., Falk, D. J., Todd, A. G., Arredondo, K., et al. (2014). Rapamycin improves peripheral nerve myelination while it fails to benefit neuromuscular performance in neuropathic mice. Neurobiol. Dis. 70, 224–236. doi: 10.1016/j.nbd.2014.06.023
Niemi, J. P., DeFrancesco-Lisowitz, A., Roldan-Hernandez, L., Lindborg, J. A., Mandell, D., and Zigmond, R. E. (2013). A critical role for macrophages near axotomized neuronal cell bodies in stimulating nerve regeneration. J. Neurosci. 33, 16236–16248. doi: 10.1523/jneurosci.3319-12.2013
Nugent, S. G., Osullivan, V. R., Fraher, J. P., and Rea, B. B. (1991). Central peripheral transitional zone of the spinal accessory nerve in the rat. J. Anat. 175, 19–25.
Odaly, J. A., and Imaeda, T. (1967). Electron microscopic study of wallerian degeneration in cutaneous nerves caused by mechanical injury. Laborat. Investigat. 17:744.
Oliveira, R. B., Ochoa, M. T., Sieling, P. A., Rea, T. H., Rambukkana, A., Sarno, E. N., et al. (2003). Expression of toll-like receptor 2 on human schwann cells: a mechanism of nerve damage in leprosy. Infect. Immun. 71, 1427–1433. doi: 10.1128/iai.71.3.1427-1433.2003
Olson, J. K., and Miller, S. D. (2004). Microglia initiate central nervous system innate and adaptive immune responses through multiple tlrs. J. Immunol. 173, 3916–3924. doi: 10.4049/jimmunol.173.6.3916
Oulton, M. R., and Mezei, C. (1976). Characterization of myelin of chick sciatic nerve. J. Lipid Res. 17, 167–175.
Painter, M. W., Lutz, A. B., Cheng, Y. C., Latremoliere, A., Duong, K., Miller, C. M., et al. (2014). Diminished schwann cell repair responses underlie age-associated impaired axonal regeneration. Neuron 83, 331–343. doi: 10.1016/j.neuron.2014.06.016
Panni, P., Ferguson, I. A., Beacham, I., Mackay-Sim, A., Ekberg, J. A. K., and St John, J. A. (2013). Phagocytosis of bacteria by olfactory ensheathing cells and schwann cells. Neurosci. Lett. 539, 65–70. doi: 10.1016/j.neulet.2013.01.052
Papadopoulos, Z., Herz, J., and Kipnis, J. (2020). Meningeal lymphatics: from anatomy to central nervous system immune surveillance. J. Immunol. 204, 286–293. doi: 10.4049/jimmunol.1900838
Parkinson, D. B., Bhaskaran, A., Droggiti, A., Dickinson, S., D’Antonio, M., Mirsky, R., et al. (2004). Krox-20 inhibits jun-nh2-terminal kinase/c-jun to control schwann cell proliferation and death. J. Cell Biol. 164, 385–394. doi: 10.1083/jcb.200307132
Parrinello, S., Napoli, I., Ribeiro, S., Digby, P. W., Fedorova, M., Parkinson, D. B., et al. (2010). Ephb signaling directs peripheral nerve regeneration through sox2-dependent schwann cell sorting. Cell 143, 145–155. doi: 10.1016/j.cell.2010.08.039
Pastrana, E., Moreno-Flores, M. T., Gurzov, E. N., Avila, J., Wandosell, F., and Diaz-Nido, J. (2006). Genes associated with adult axon regeneration promoted by olfactory ensheathing cells: A new role for matrix metalloproteinase 2. J. Neurosci. 26, 5347–5359. doi: 10.1523/jneurosci.1111-06.2006
Pearse, D. D., Bastidas, J., Izabel, S. S., and Ghosh, M. (2018). Schwann cell transplantation subdues the pro-inflammatory innate immune cell response after spinal cord injury. Int. J. Mol. Sci. 19:2550. doi: 10.3390/ijms19092550
Pearse, D. D., Marcillo, A. E., Oudega, M., Lynch, M. P., Wood, P. M., and Bunge, M. B. (2004). Transplantation of schwann cells and olfactory ensheathing glia after spinal cord injury: does pretreatment with methylprednisolone and interleukin-10 enhance recovery? J. Neurotraum. 21, 1223–1239. doi: 10.1089/neu.2004.21.1223
Peluffo, H., Solari-Saquieres, P., Negro-Demontel, M. L., Francos-Quijorna, I., Navarro, X., Lopez-Vales, R., et al. (2015). Cd300f immunoreceptor contributes to peripheral nerve regeneration by the modulation of macrophage inflammatory phenotype. J. Neuroinflamm. 12:364. doi: 10.1186/s12974-015-0364-y
Pereira, R. A., Tscharke, D. C., and Simmons, A. (1994). Up-regulation of class-i major histocompatibility complex gene-expression in primary sensory neurons, satellite cells, and schwann-cells of mice in response to acute but not latent herpes-simplex virus-infection in-vivo. J. Exp. Med. 180, 841–850. doi: 10.1084/jem.180.3.841
Perera, S. N., Williams, R. M., Lyne, R., Stubbs, O., Buehler, D. P., Sauka-Spengler, T., et al. (2020). Insights into olfactory ensheathing cell development from a laser-microdissection and transcriptome-profiling approach. Glia 68, 2550–2584. doi: 10.1002/glia.23870
Perry, V. H., Brown, M. C., and Gordon, S. (1987). The macrophage response to central and peripheral nerve injury- a posible role for macrophages in regeneration. J. Exp. Med. 165, 1218–1223. doi: 10.1084/jem.165.4.1218
Perry, V. H., Tsao, J. W., Fearn, S., and Brown, M. C. (1995). Radiation-induced reductions in macrophage recruitment have only slight effects on myelin degeneration in sectioned peripheral-nerves of mice. Eur. J. Neurosci. 7, 271–280. doi: 10.1111/j.1460-9568.1995.tb01063.x
Pinard, A., Levesque, S., Vallee, J., and Robitaille, R. (2003). Glutamatergic modulation of synaptic plasticity at a pns vertebrate cholinergic synapse. Eur. J. Neurosci. 18, 3241–3250. doi: 10.1111/j.1460-9568.2003.03028.x
Ponath, G., Ramanan, S., Mubarak, M., Housley, W., Lee, S., Sahinkaya, F. R., et al. (2017). Myelin phagocytosis by astrocytes after myelin damage promotes lesion pathology. Brain 140, 399–413. doi: 10.1093/brain/aww298
Prinz, M., and Priller, J. (2017). The role of peripheral immune cells in the cns in steady state and disease. Nat. Neurosci. 20, 136–144. doi: 10.1038/nn.4475
Quintes, S., and Brinkmann, B. G. (2017). Transcriptional inhibition in schwann cell development and nerve regeneration. Neural Regenerat. Res. 12:1241. doi: 10.4103/1673-5374.213537
Radtke, C., Wewetzer, K., Reimers, K., and Vogt, P. M. (2011). Transplantation of olfactory ensheathing cells as adjunct cell therapy for peripheral nerve injury. Cell Transplant. 20, 145–152. doi: 10.3727/096368910x522081
Rajbhandari, L., Tegenge, M. A., Shrestha, S., Kumar, N., Malik, A., Mithal, A., et al. (2014). Toll-like receptor 4 deficiency impairs microglial phagocytosis of degenerating axons. Glia 62, 1982–1991. doi: 10.1002/glia.22719
Ramaglia, V., King, R. H. M., Nourallah, M., Wolterman, R., de Jonge, R., Ramkema, M., et al. (2007). The membrane attack complex of the complement system is essential for rapid wallerian degeneration. J. Neurosci. 27, 7663–7672. doi: 10.1523/jneurosci.5623-06.2007
Ramesh, G., Santana-Gould, L., Inglis, F. M., England, J. D., and Philipp, M. T. (2013). The lyme disease spirochete borrelia burgdorferi induces inflammation and apoptosis in cells from dorsal root ganglia. J. Neuroinflamm. 10:88. doi: 10.1186/1742-2094-10-88
Ramón-Cueto, A., Plant, G. W., Avila, J., and Bunge, M. B. (1998). Long-distance axonal regeneration in the transected adult rat spinal cord is promoted by olfactory ensheathing glia transplants. J. Neurosci. 18, 3803–3815. doi: 10.1523/jneurosci.18-10-03803.1998
Rangaraju, S., and Notterpek, L. (2011). Autophagy aids membrane expansion by neuropathic schwann cells. Autophagy 7, 238–239. doi: 10.4161/auto.7.2.14278
Rasmussen, J. P., Sack, G. S., Martin, S. M., and Sagasti, A. (2015). Vertebrate epidermal cells are broad-specificity phagocytes that clear sensory axon debris. J. Neurosci. 35, 559–570. doi: 10.1523/jneurosci.3613-14.2015
Régnier-Vigouroux, A. (2003). The mannose receptor in the brain. Int. Rev. Cytol. 226, 321–342. doi: 10.1016/s0074-7696(03)01006-4
Reichert, F., and Rotshenker, S. (2003). Complement-receptor-3 and scavenger-receptor-ai/ii mediated myelin phagocytosis in microglia and macrophages. Neurobiol. Dis. 12, 65–72. doi: 10.1016/s0969-9961(02)00008-6
Reina, M. A., Sala-Blanch, X., Arriazu, R., and Machés, F. (2015). “Microscopic morphology and ultrastructure of human peripheral nerves,” in Nerves and Nerve Injuries, eds R. S. Tubbs, E. Rizk, M. M. Shoja, M. Loukas, N. Barbaro, and R. J. Spinner (San Diego, CA: Academic Press), 91–106.
Reshamwala, R., Shah, M., St John, J., and Ekberg, J. (2019). Survival and integration of transplanted olfactory ensheathing cells are crucial for spinal cord injury repair: insights from the last 10 years of animal model studies. Cell Transplant. 28(Suppl. 1), 132S–159S. doi: 10.1177/0963689719883823
Rickmann, M., Fawcett, J. W., and Keynes, R. J. (1985). The migration of neural crest cells and the hrowth of motor axons through the rostral half of the chick somite. J. Embryol. Exp. Morphol. 90, 437–455.
Robitaille, R. (1998). Modulation of synaptic efficacy and synaptic depression by glial cells at the frog neuromuscular junction. Neuron 21, 847–855. doi: 10.1016/s0896-6273(00)80600-5
Rodrigues, M. C. O., Rodrigues, A. A., Glover, L. E., Voltarelli, J., and Borlongan, C. V. (2012). Peripheral nerve repair with cultured schwann cells: Getting closer to the clinics. Sci. World J. 2012, 1–10. doi: 10.1100/2012/413091
Roet, K. C. D., and Verhaagen, J. (2014). Understanding the neural repair-promoting properties of olfactory ensheathing cells. Exp. Neurol. 261, 594–609. doi: 10.1016/j.expneurol.2014.05.007
Roet, K. C. D., Bossers, K., Franssen, E. H. P., Ruitenberg, M. J., and Verhaagen, J. (2011). A meta-analysis of microarray-based gene expression studies of olfactory bulb-derived olfactory ensheathing cells. Exp. Neurol. 229, 10–45. doi: 10.1016/j.expneurol.2011.03.001
Rosenberg, A. F., Wolman, M. A., Franzini-Armstrong, C., and Granato, M. (2012). In vivo nerve-macrophage interactions following peripheral nerve injury. J. Neurosci. 32, 3898–3909. doi: 10.1523/jneurosci.5225-11.2012
Rotshenker, S., Reichert, F., Gitik, M., Haklai, R., Elad-Sfadia, G., and Kloog, Y. (2008). Galectin-3/mac-2, ras and pi3k activate complement receptor-3 and scavenger receptor-ai/ii mediated myelin phagocytosis in microglia. Glia 56, 1607–1613. doi: 10.1002/glia.20713
Ruiz-Mendoza, S., Macedo-Ramos, H., Santos, F. A., Quadros-De-Souza, L. C., Paiva, M. M., Pinto, T. C. A., et al. (2016). Streptococcus pneumoniae infection regulates expression of neurotrophic factors in the olfactory bulb and cultured olfactory ensheathing cells. Neuroscience 317, 149–161. doi: 10.1016/j.neuroscience.2016.01.016
Sakita, M., Murakami, S., and Fujino, H. (2016). Age-related morphological regression of myelinated fibers and capillary architecture of distal peripheral nerves in rats. BMC Neurosci. 17:4. doi: 10.1186/s12868-016-0277-4
Samuel, N. M., Mirsky, R., Grange, J. M., and Jessen, K. R. (1987). Expression of major histocompatibility complex class-i and class-ii antigens in human schwann-cell cultures and effects of infection with mycobacterium-leprae. Clin. Exp. Immunol. 68, 500–509.
Satinsky, D., Liu, C. N., and Pepe, F. A. (1964). Neurilemma cell in peripheral nerve degeneration + regeneration. Exp. Neurol. 9:441. doi: 10.1016/0014-4886(64)90052-4
Schafer, D. P., Lehrman, E. K., Kautzman, A. G., Koyama, R., Mardinly, A. R., Yamasaki, R., et al. (2012). Microglia sculpt postnatal neural circuits in an activity and complement-dependent manner. Neuron 74, 691–705. doi: 10.1016/j.neuron.2012.03.026
Scheib, J. L., and Hoke, A. (2016). An attenuated immune response by schwann cells and macrophages inhibits nerve regeneration in aged rats. Neurobiol. Aging 45, 1–9. doi: 10.1016/j.neurobiolaging.2016.05.004
Schröder, J. M., May, R., and Weis, J. (1993). Perineurial cells are the first to traverse gaps of peripheral nerves in silicone tubes. Clin. Neurol. Neurosurg. 95(Suppl.), S78–S83. doi: 10.1016/0303-8467(93)90040-n
Schulz, N. T., Paulhiac, C. I., Lee, L., and Zhou, R. P. (1995). Isolation and expression analysis of tyro3, a murine growth-factor receptor tyrosine kinase preferentially expressed in adult brain. Mol. Brain Res. 28, 273–280. doi: 10.1016/0169-328x(94)00216-2
Schuster, C. M., Davis, G. W., Fetter, R. D., and Goodman, C. S. (1996). Genetic dissection of structural and functional components of synaptic plasticity. 2. Fasciclin ii controls presynaptic structural plasticity. Neuron 17, 655–667. doi: 10.1016/s0896-6273(00)80198-1
Shacham-Silverberg, V., Shalom, H. S., Goldner, R., Golan-Vaishenker, Y., Gurwicz, N., Gokhman, I., et al. (2018). Phosphatidylserine is a marker for axonal debris engulfment but its exposure can be decoupled from degeneration. Cell Death Dis. 9:1155. doi: 10.1038/s41419-018-1155-z
Shanthaveerappa, T. R., and Bourne, G. H. (1963). The perinural epithelium: nature and significance. Nature 199, 577–579. doi: 10.1038/199577a0
Sharghi-Namini, S., Turmaine, M., Meier, C., Sahni, V., Umehara, F., Jessen, K. R., et al. (2006). The structural and functional integrity of peripheral nerves depends on the glial-derived signal desert hedgehog. J. Neurosci. 26, 6364–6376. doi: 10.1523/jneurosci.0157-06.2006
Shimeld, C., Efstathiou, S., and Hill, T. (2001). Tracking the spread of a lacz-tagged herpes simplex virus type 1 between the eye and the nervous system of the mouse: Comparison of primary and recurrent infection. J. Virol. 75, 5252–5262. doi: 10.1128/jvi.75.11.5252-5262.2001
Shimoji, Y., Ng, V., Matsumura, K., Fischetti, V. A., and Rambukkana, A. (1999). A 21-kda surface protein of mycobacterium leprae binds peripheral nerve laminin-2 and mediates schwann cell invasion. Proc. Natl. Acad. Sci. U.S.A. 96, 9857–9862. doi: 10.1073/pnas.96.17.9857
Shivkumar, M., Milho, R., May, J. S., Nicoll, M. P., Efstathiou, S., and Stevenson, P. G. (2013). Herpes simplex virus 1 targets the murine olfactory neuroepithelium for host entry. J. Virol. 87, 10477–10488. doi: 10.1128/jvi.01748-13
Sierra, A., Encinas, J. M., Deudero, J. J. P., Chancey, J. H., Enikolopov, G., Overstreet-Wadiche, L. S., et al. (2010). Microglia shape adult hippocampal neurogenesis through apoptosis-coupled phagocytosis. Cell Stem Cell 7, 483–495. doi: 10.1016/j.stem.2010.08.014
Sjolinder, H., and Jonsson, A. B. (2010). Olfactory nerve-a novel invasion route of neisseria meningitidis to reach the meninges. PLoS One 5:e0014034. doi: 10.1371/journal.pone.0014034
Soares, L., Parisi, M., and Bonini, N. M. (2014). Axon injury and regeneration in the adult drosophila. Sci. Rep. 4:6199. doi: 10.1038/srep06199
Song, J. W., Misgeld, T., Kang, H., Knecht, S., Lu, J., Cao, Y., et al. (2008). Lysosomal activity associated with developmental axon pruning. J. Neurosci. 28, 8993–9001. doi: 10.1523/jneurosci.0720-08.2008
Squarzoni, P., Oller, G., Hoeffel, G., Pont-Lezica, L., Rostaing, P., Low, D., et al. (2014). Microglia modulate wiring of the embryonic forebrain. Cell Rep. 8, 1271–1279. doi: 10.1016/j.celrep.2014.07.042
St John, J. A., Ekberg, J. A. K., Dando, S. J., Meedeniya, A. C. B., Horton, R. E., Batzloff, M., et al. (2014). Burkholderia pseudomallei penetrates the brain via destruction of the olfactory and trigeminal nerves: Implications for the pathogenesis of neurological melioidosis. Mbio 5:14. doi: 10.1128/mBio.00025-14
St John, J. A., Walkden, H., Nazareth, L., Beagley, K. W., Ulett, G. C., Batzloff, M. R., et al. (2016). Burkholderia pseudomallei rapidly infects the brain stem and spinal cord via the trigeminal nerve after intranasal inoculation. Infect. Immun. 84, 2681–2688. doi: 10.1128/iai.00361-16
Stamou, M., Grodzki, A. C., van Oostrum, M., Wollscheid, B., and Lein, P. J. (2018). Fc gamma receptors are expressed in the developing rat brain and activate downstream signaling molecules upon cross-linking with immune complex. J. Neuroinflamm. 15:1050. doi: 10.1186/s12974-017-1050-z
Stephan, A. H., Barres, B. A., and Stevens, B. (2012). The complement system: an unexpected role in synaptic pruning during development and disease. Annu. Rev. Neurosci. 35, 369–389. doi: 10.1146/annurev-neuro-061010-113810
Stevens, B., Allen, N. J., Vazquez, L. E., Howell, G. R., Christopherson, K. S., Nouri, N., et al. (2007). The classical complement cascade mediates cns synapse elimination. Cell 131, 1164–1178. doi: 10.1016/j.cell.2007.10.036
Stolinski, C. (1995). Structure and composition of the outer connective tissue sheaths of peripheral nerve. J. Anat. 186(Pt 1), 123–130.
Stoll, G., Griffin, J. W., Li, C. Y., and Trapp, B. D. (1989). Wallerian degeneration in the peripheral nervous system: Participation of both schwann cells and macrophages in myelin degradation. J. Neurocytol. 18, 671–683. doi: 10.1007/bf01187086
Stratton, J. A., and Shah, P. T. (2016). Macrophage polarization in nerve injury: Do schwann cells play a role? Neural Regenerat. Res. 11, 53–57. doi: 10.4103/1673-5374.175042
Stuve, O., and Zettl, U. (2014). Neuroinflammation of the central and peripheral nervous system: an update. Clin. Exp. Immunol. 175, 333–335. doi: 10.1111/cei.12260
Su, Z. D., Chen, J. J., Qiu, Y., Yuan, Y. M., Zhu, F., Zhu, Y. L., et al. (2013). Olfactory ensheathing cells: the primary innate immunocytes in the olfactory pathway to engulf apoptotic olfactory nerve debris. Glia 61, 490–503. doi: 10.1002/glia.22450
Sulaiman, O. A. R., and Gordon, T. (2000). Effects of short- and long-term schwann cell denervation on peripheral nerve regeneration, myelination, and size. Glia 32, 234–246. doi: 10.1002/1098-1136(200012)32:3<234::Aid-glia40<3.0.Co;2-3
Sun, T., Ye, C., Zhang, Z., Wu, J., and Huang, H. (2013). Cotransplantation of olfactory ensheathing cells and schwann cells combined with treadmill training promotes functional recovery in rats with contused spinal cords. Cell Trans. 22(Suppl. 1), S27–S38. doi: 10.3727/096368913x672118
Suzuki, J., and Osumi, N. (2015). Neural crest and placode contributions to olfactory development. Curr. Top. Dev. Biol. 111, 351–374. doi: 10.1016/bs.ctdb.2014.11.010
Suzuki, K., Lovera, M., Schmachtenberg, O., and Couve, E. (2015). Axonal degeneration in dental pulp precedes human primary teeth exfoliation. J. Dent. Res. 94, 1446–1453. doi: 10.1177/0022034515593055
Takami, T., Oudega, M., Bates, M. L., Wood, P. M., Kleitman, N., and Bunge, M. B. (2002). Schwann cell but not olfactory ensheathing glia transplants improve hindlimb locomotor performance in the moderately contused adult rat thoracic spinal cord. J. Neurosci. 22, 6670–6681. doi: 10.1523/jneurosci.22-15-06670.2002
Taskinen, H. S., and Roytta, M. (1997). The dynamics of macrophage recruitment after nerve transection. Acta Neuropathol. 93, 252–259. doi: 10.1007/s004010050611
Thomas, P. K. (1963). The connective tissue of peripheral nerve: an electron microscope study. J. Anat. 97(Pt 1), 35–44.
Thumm, M., and Simons, M. (2015). Myelinophagy: schwann cells dine in. J. Cell Biol. 210, 9–10. doi: 10.1083/jcb.201506039
Tofaris, G. K., Patterson, P. H., Jessen, K. R., and Mirsky, R. (2002). Denervated schwann cells attract macrophages by secretion of leukemia inhibitory factor (lif) and monocyte chemoattractant protein-1 in a process regulated by interleukin-6 and lif. J. Neurosci. 22, 6696–6703. doi: 10.1523/jneurosci.22-15-06696.2002
Touma, E., Kato, S., Fukui, K., and Koike, T. (2007). Calpain-mediated cleavage of collapsin response mediator protein(crmp)-2 during neurite degeneration in mice. Eur. J. Neurosci. 26, 3368–3381. doi: 10.1111/j.1460-9568.2007.05943.x
Treloar, H. B., Feinstein, P., Mombaerts, P., and Greer, C. A. (2002). Specificity of glomerular targeting by olfactory sensory axons. J. Neurosci. 22, 2469–2477. doi: 10.1523/jneurosci.22-07-02469.2002
Tremblay, M. E., Cookson, M. R., and Civiero, L. (2019). Glial phagocytic clearance in parkinson’s disease. Mol. Neurodegener. 14:314. doi: 10.1186/s13024-019-0314-8
Tufail, Y., Cook, D., Fourgeaud, L., Powers, C. J., Merten, K., Clark, C. L., et al. (2017). Phosphatidylserine exposure controls viral innate immune responses by microglia. Neuron 93:574. doi: 10.1016/j.neuron.2016.12.021
Ulrich, R., Imbschweiler, I., Kalkuhl, A., Lehmbecker, A., Ziege, S., Kegler, K., et al. (2014). Transcriptional profiling predicts overwhelming homology of schwann cells, olfactory ensheathing cells, and schwann cell-like glia. Glia 62, 1559–1581. doi: 10.1002/glia.22700
Ushiki, T., and Ide, C. (1990). Three-dimensional organization of the collagen fibrils in the rat sciatic nerve as revealed by transmission- and scanning electron microscopy. Cell Tissue Res. 260, 175–184. doi: 10.1007/bf00297503
Valles, S. L., Iradi, A., Aldasoro, M., Vila, J. M., Aldasoro, C., de la Torre, J., et al. (2019). Function of glia in aging and the brain diseases. Int. J. Med. Sci. 16, 1473–1479. doi: 10.7150/ijms.37769
Valverde, F., Santacana, M., and Heredia, M. (1992). Formation of an olfactory glomerulus - morphological aspects of development and organization. Neuroscience 49, 255–275. doi: 10.1016/0306-4522(92)90094-i
van Ginkel, F. W., McGhee, J. R., Watt, J. M., Campos-Torres, A., Parish, L. A., and Briles, D. E. (2003). Pneumococcal carriage results in ganglioside-mediated olfactory tissue infection. Proc. Natl. Acad. Sci. U.S.A. 100, 14363–14367. doi: 10.1073/pnas.2235844100
Van Hove, H., Martens, L., Scheyltjens, I., De Vlaminck, K., Antunes, A. R. P., De Prijck, S., et al. (2019). A single-cell atlas of mouse brain macrophages reveals unique transcriptional identities shaped by ontogeny and tissue environment. Nat. Neurosci. 22, 1021–1035. doi: 10.1038/s41593-019-0393-4
van Paassen, B. W., van Ruissen, F., van der Kooi, A. J., de Visser, M., Verhamme, C., and Baas, F. (2019). Neuropathy related genes as possible modifiers of pmp22 related neuropathies. Eur. J. Hum. Genet. 27, 1474–1474.
Vargas, M. E., and Barres, B. A. (2007). Why is wallerian degeneration in the cns so slow? Annu. Rev. Neurosci. 30, 153–179. doi: 10.1146/annurev.neuro.30.051606.094354
Vedeler, C. A., Nilsen, R., and Matre, R. (1989). Localization of fc-gamma-receptors and complement receptors-cr1 on human peripheral-nerve fibers by immunoelectron microscopy. J. Neuroimmunol. 23, 29–33. doi: 10.1016/0165-5728(89)90069-6
Vedeler, C. A., Scarpini, E., Beretta, S., Doronzo, R., and Matre, R. (1990). The ontogenesis of fcγ receptors and complement receptors cr1 in human peripheral nerve. Acta Neuropathol. 80, 35–40. doi: 10.1007/BF00294219
Velasquez, J. T., St John, J. A., Nazareth, L., and Ekberg, J. A. K. (2018). Schwann cell lamellipodia regulate cell-cell interactions and phagocytosis. Mol. Cell Neurosci. 88, 189–200. doi: 10.1016/j.mcn.2018.01.001
Velasquez, J. T., Watts, M. E., Todorovic, M., Nazareth, L., Pastrana, E., Diaz-Nido, J., et al. (2014). Low-dose curcumin stimulates proliferation, migration and phagocytic activity of olfactory ensheathing cells. PLoS One 9:e0111787. doi: 10.1371/journal.pone.0111787
Verdu, E., Ceballos, D., Vilches, J. J., and Navarro, X. (2000). Influence of aging on peripheral nerve function and regeneration. J. Peripher. Nerv. Syst. 5, 191–208. doi: 10.1046/j.1529-8027.2000.00026.x
Viader, A., Golden, J. P., Baloh, R. H., Schmidt, R. E., Hunter, D. A., and Milbrandt, J. (2011). Schwann cell mitochondrial metabolism supports long-term axonal survival and peripheral nerve function. J. Neurosci. 31, 10128–10140. doi: 10.1523/jneurosci.0884-11.2011
Vincent, A. J., Choi-Lundberg, D. L., Harris, J. A., West, A. K., and Chuah, M. I. (2007). Bacteria and pamps activate nuclear factor kb and gro production in a subset of olfactory ensheathing cells and astrocytes but not in schwann cells. Glia 55, 905–916. doi: 10.1002/glia.20512
Vincent, A. J., Taylor, J. M., Choi-Lundberg, D. L., West, A. K., and Chuah, M. I. (2005). Genetic expression profile of olfactory ensheathing cells is distinct from that of schwann cells and astrocytes. Glia 51, 132–147. doi: 10.1002/glia.20195
Voas, M. G., Glenn, T. D., Raphael, A. R., and Talbot, W. S. (2009). Schwann cells inhibit ectopic clustering of axonal sodium channels. J. Neurosci. 29, 14408–14414. doi: 10.1523/jneurosci.0841-09.2009
Volney, W. A. (1851). Experiments on the section of the glossopharyngeal and hypoglossal nerves of the frog, and observations of the alterations produced thereby in the structure of their primitive fibres. Proc. R. Soc. Lond. 5, 924–925. doi: 10.1098/rspl.1843.0224
Wagstaff, L. J., Gomez-Sanchez, J. A., Mirsky, R., and Jessen, K. R. (2017). The relationship between schwann cell c-jun and regeneration failures due to ageing and long-term injury. Glia 65, E532–E532.
Walkden, H., Delbaz, A., Nazareth, L., Batzloff, M., Shelper, T., Beacham, I. R., et al. (2020). Burkholderia pseudomallei invades the olfactory nerve and bulb after epithelial injury in mice and causes the formation of multinucleated giant glial cells in vitro. PLoS Neglect. Trop. Dis. 14:e0008017. doi: 10.1371/journal.pntd.0008017
Wang, P. L., Yim, A. K. Y., Kim, K. W., Avey, D., Czepielewski, R. S., Colonna, M., et al. (2020). Peripheral nerve resident macrophages share tissue-specific programming and features of activated microglia. Nat. Commun. 11:2552. doi: 10.1038/s41467-020-16355-w
Watanabe, T., Nagase, K., Chosa, M., and Tobinai, K. (2010). Schwann cell autophagy induced by saha, 17-aag, or clonazepam can reduce bortezomib-induced peripheral neuropathy. Br. J. Cancer 103, 1580–1587. doi: 10.1038/sj.bjc.6605954
Watts, R. J., Schuldiner, O., Perrino, J., Larsen, C., and Luo, L. Q. (2004). Glia engulf degenerating axons during developmental axon pruning. Curr. Biol. 14, 678–684. doi: 10.1016/j.cub.2004.03.035
Webster, H. D. (1971). The geometry of peripheral myelin sheaths during their formation and growth in rat sciatic nerves. J. Cell Biol. 48, 348–367. doi: 10.1083/jcb.48.2.348
Webster, H. D., Martin, R., and O’Connell, M. F. (1973). The relationships between interphase schwann cells and axons before myelination: a quantitative electron microscopic study. Dev. Biol. 32, 401–416. doi: 10.1016/0012-1606(73)90250-9
Wei, P. X., Bao, R. X., and Fan, Y. B. (2020). Brainstem encephalitis caused by listeria monocytogenes. Pathogens 9:e9090715. doi: 10.3390/pathogens9090715
Weinger, J. G., Brosnan, C. F., Loudig, O., Goldberg, M. F., Macian, F., Arnett, H. A., et al. (2011). Loss of the receptor tyrosine kinase axl leads to enhanced inflammation in the cns and delayed removal of myelin debris during experimental autoimmune encephalomyelitis. J. Neuroinflamm. 8:49. doi: 10.1186/1742-2094-8-49
Weinkauf, C., and PereiraPerrin, M. (2009). Trypanosoma cruzi promotes neuronal and glial cell survival through the neurotrophic receptor trkc. Infect. Immun. 77, 1368–1375. doi: 10.1128/iai.01450-08
Weinstein, J. R., Quan, Y., Hanson, J. F., Colonna, L., Iorga, M., Honda, S., et al. (2015). Igm-dependent phagocytosis in microglia is mediated by complement receptor 3, not fc alpha/mu receptor. J. Immunol. 195, 5309–5317. doi: 10.4049/jimmunol.1401195
Weiss, P., and Wang, H. (1945). Transformation of adult schwann cells in macrophages. Proc. Soc. Exp. Biol. Med. 58, 273–275.
Weiss, T., Taschner-Mandl, S., Bileck, A., Slany, A., Kromp, F., Rifatbegovic, F., et al. (2016). Proteomics and transcriptomics of peripheral nerve tissue and cells unravel new aspects of the human schwann cell repair phenotype. Glia 64, 2133–2153. doi: 10.1002/glia.23045
Welleford, A. S., Quintero, J. E., El Seblani, N., Blalock, E., Gunewardena, S., Shapiro, S. M., et al. (2020). Rna sequencing of human peripheral nerve in response to injury: distinctive analysis of the nerve repair pathways. Cell Transplant. 29:157. doi: 10.1177/0963689720926157
Wewetzer, K., Verdu, E., Angelov, D. N., and Navarro, X. (2002). Olfactory ensheathing glia and schwann cells: two of a kind? Cell Tissue Res. 309, 337–345. doi: 10.1007/s00441-002-0607-y
Windus, L. C. E., Lineburg, K. E., Scott, S. E., Claxton, C., Mackay-Sim, A., Key, B., et al. (2010). Lamellipodia mediate the heterogeneity of central olfactory ensheathing cell interactions. Cell. Mol. Life Sci. 67, 1735–1750. doi: 10.1007/s00018-010-0280-3
Wolbert, J., Li, X., Heming, M., Mausberg, A. K., Akkermann, D., Frydrychowicz, C., et al. (2020). Redefining the heterogeneity of peripheral nerve cells in health and autoimmunity. Proc. Natl. Acad. Sci. U.S.A. 117, 9466–9476. doi: 10.1073/pnas.1912139117
Wong, W. F., Chambers, J. P., Gupta, R., and Arulanandam, B. P. (2019). Chlamydia and its many ways of escaping the host immune system. J. Pathog. 2019:8604958. doi: 10.1155/2019/8604958
Woodhall, E., West, A. K., and Chuah, M. I. (2001). Cultured olfactory ensheathing cells express nerve growth factor, brain-derived neurotrophic factor, glia cell line-derived neurotrophic factor and their receptors. Mol. Brain Res. 88, 203–213. doi: 10.1016/s0169-328x(01)00044-4
Woodhoo, A., and Sommer, L. (2008). Development of the schwann cell lineage: from the neural crest to the myelinated nerve. Glia 56, 1481–1490. doi: 10.1002/glia.20723
Woodhoo, A., Dean, C. H., Droggiti, A., Mirsky, R., and Jessen, K. R. (2004). The trunk neural crest and its early glial derivatives: a study of survival responses, developmental schedules and autocrine mechanisms. Mol. Cell. Neurosci. 25, 30–41. doi: 10.1016/j.mcn.2003.09.006
Woods, J. J., Skelding, K. A., Martin, K. L., Aryal, R., Sontag, E., Johnstone, D. M., et al. (2020). Assessment of evidence for or against contributions of chlamydia pneumoniae infections to alzheimer’s disease etiology. Brain Behav. Immun. 83, 22–32. doi: 10.1016/j.bbi.2019.10.014
Wright, A. A., Todorovic, M., Murtaza, M., St John, J. A., and Ekberg, J. A. (2020). Macrophage migration inhibitory factor and its binding partner htra1 are expressed by olfactory ensheathing cells. Mol. Cell. Neurosci. 102:103450. doi: 10.1016/j.mcn.2019.103450
Wright, A. A., Todorovic, M., Tello-Velasquez, J., Rayfield, A. J., St John, J. A., and Ekberg, J. A. (2018). Enhancing the therapeutic potential of olfactory ensheathing cells in spinal cord repair using neurotrophins. Cell Trans. 27, 867–878. doi: 10.1177/0963689718759472
Wu, H. H., Bellmunt, E., Scheib, J. L., Venegas, V., Burkert, C., Reichardt, L. F., et al. (2009). Glial precursors clear sensory neuron corpses during development via jedi-1, an engulfment receptor. Nat. Neurosci. 12, 1534–U1579. doi: 10.1038/nn.2446
Wyatt, R. M., and Balice-Gordon, R. J. (2003). Activity-dependent elimination of neuromuscular synapses. J. Neurocytol. 32, 777–794. doi: 10.1023/b:Neur.0000020623.62043.33
Xia, B., Gao, J. B., Li, S. Y., Huang, L. L., Ma, T., Zhao, L. H., et al. (2019). Extracellular vesicles derived from olfactory ensheathing cells promote peripheral nerve regeneration in rats. Front. Cell. Neurosci. 13:548. doi: 10.3389/fncel.2019.00548
Xiao, Y., Faucherre, A., Pola-Morell, L., Heddleston, J. M., Liu, T.-L., Chew, T.-L., et al. (2015). High-resolution live imaging reveals axon-glia interactions during peripheral nerve injury and repair in zebrafish. Dis. Models Mech. 8, 553–564. doi: 10.1242/dmm.018184
Xie, J., Li, Y. J., Dai, J. M., He, Y., Sun, D. Y., Dai, C., et al. (2019). Olfactory ensheathing cells grafted into the retina of rcs rats suppress inflammation by down-regulating the jak/stat pathway. Front. Cell. Neurosci. 13:341. doi: 10.3389/fncel.2019.00341
Yan, S. D., Chen, X., Fu, J., Chen, M., Zhu, H. J., Roher, A., et al. (1996). Rage and amyloid-beta peptide neurotoxicity in alzheimer’s disease. Nature 382, 685–691. doi: 10.1038/382685a0
Yao, R., Murtaza, M., Velasquez, J. T., Todorovic, M., Rayfield, A., Ekberg, J., et al. (2018). Olfactory ensheathing cells for spinal cord injury: sniffing out the issues. Cell Transplant. 27, 879–889. doi: 10.1177/0963689718779353
Ydens, E., Demon, D., Lornet, G., De Winter, V., Timmerman, V., Lamkanfi, M., et al. (2015). Nlrp6 promotes recovery after peripheral nerve injury independently of inflammasomes. J. Neuroinflamm. 12:143. doi: 10.1186/s12974-015-0367-8
Ydens, E., Lornet, G., Smits, V., Goethals, S., Timmerman, V., and Janssens, S. (2013). The neuroinflammatory role of schwann cells in disease. Neurobiol. Dis. 55, 95–103. doi: 10.1016/j.nbd.2013.03.005
Yi, S., Liu, Q. Y., Wang, X. H., Qian, T. M., Wang, H. K., Zha, G. B., et al. (2019). Tau modulates schwann cell proliferation, migration and differentiation following peripheral nerve injury. J. Cell Sci. 132:222059. doi: 10.1242/jcs.222059
You, H., Wei, L., Liu, Y., Oudega, M., Jiao, S. S., Feng, S. N., et al. (2011). Olfactory ensheathing cells enhance schwann cell-mediated anatomical and functional repair after sciatic nerve injury in adult rats. Exp. Neurol. 229, 158–167. doi: 10.1016/j.expneurol.2010.08.034
Yuan, X. D., Klein, D., Kerscher, S., West, B. L., Weis, J., Katona, I., et al. (2018). Macrophage depletion ameliorates peripheral neuropathy in aging mice. J. Neurosci. 38, 4610–4620. doi: 10.1523/jneurosci.3030-17.2018
Zhang, G., Bogdanova, N., Gao, T., Song, J. J., Cragg, M. S., Glennie, M. J., et al. (2014). Fc gamma receptor-mediated inflammation inhibits axon regeneration. PLoS One 9:e0088703. doi: 10.1371/journal.pone.0088703
Zhang, J. Y., Chen, H. J., Duan, Z. X., Chen, K. J., Liu, Z., Zhang, L., et al. (2017). The effects of co-transplantation of olfactory ensheathing cells and schwann cells on local inflammation environment in the contused spinal cord of rats. Mol. Neurobiol. 54, 943–953. doi: 10.1007/s12035-016-9709-5
Zhang, J. Y., Ren, J. Y., Liu, Y., Huang, D. X., and Lu, L. J. (2020). Resveratrol regulates the recovery of rat sciatic nerve crush injury by promoting the autophagy of schwann cells. Life Sci. 256:117959. doi: 10.1016/j.lfs.2020.117959
Zhang, L. J., Zhuang, X. Q., Kotitalo, P., Keller, T., Krzyczmonik, A., Haaparanta-Solin, M., et al. (2021). Intravenous transplantation of olfactory ensheathing cells reduces neuroinflammation after spinal cord injury via interleukin-1 receptor antagonist. Theranostics 11, 1147–1161. doi: 10.7150/thno.52197
Zhang, S. T., Hu, L., Jiang, J. L., Li, H. J., Wu, Q., Ooi, K., et al. (2020). Hmgb1/rage axis mediates stress-induced rvlm neuroinflammation in mice via impairing mitophagy flux in microglia. J. Neuroinflamm. 17:1673. doi: 10.1186/s12974-019-1673-3
Zhao, Z. W., Li, X. L., and Li, Q. (2017). Curcumin accelerates the repair of sciatic nerve injury in rats through reducing schwann cells apoptosis and promoting myelinization. Biomed. Pharmacother. 92, 1103–1110. doi: 10.1016/j.biopha.2017.05.099
Zhou, J. H., Li, S. Y., Gao, J. B., Hu, Y. W., Chen, S. H., Luo, X. L., et al. (2020). Epothilone b facilitates peripheral nerve regeneration by promoting autophagy and migration in schwann cells. Front. Cell. Neurosci. 14:143. doi: 10.3389/fncel.2020.00143
Keywords: olfactory ensheathing cell, Schwann cell, cell debris, bacteria, macrophage, neuropathy
Citation: Nazareth L, St John J, Murtaza M and Ekberg J (2021) Phagocytosis by Peripheral Glia: Importance for Nervous System Functions and Implications in Injury and Disease. Front. Cell Dev. Biol. 9:660259. doi: 10.3389/fcell.2021.660259
Received: 29 January 2021; Accepted: 17 March 2021;
Published: 08 April 2021.
Edited by:
Nathan Anthony Smith, Children’s National Hospital, United StatesReviewed by:
Cody J. Smith, University of Notre Dame, United StatesYehong Du, Chongqing Medical University, China
Copyright © 2021 Nazareth, St John, Murtaza and Ekberg. This is an open-access article distributed under the terms of the Creative Commons Attribution License (CC BY). The use, distribution or reproduction in other forums is permitted, provided the original author(s) and the copyright owner(s) are credited and that the original publication in this journal is cited, in accordance with accepted academic practice. No use, distribution or reproduction is permitted which does not comply with these terms.
*Correspondence: Jenny Ekberg, ai5la2JlcmdAZ3JpZmZpdGguZWR1LmF1