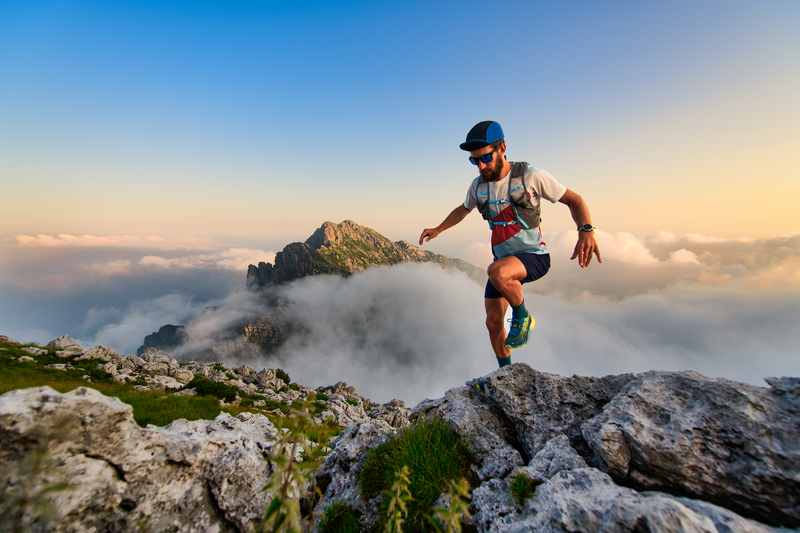
95% of researchers rate our articles as excellent or good
Learn more about the work of our research integrity team to safeguard the quality of each article we publish.
Find out more
REVIEW article
Front. Cell Dev. Biol. , 18 March 2021
Sec. Cellular Biochemistry
Volume 9 - 2021 | https://doi.org/10.3389/fcell.2021.658893
This article is part of the Research Topic Developmental Biology and Regulation of Osteoclasts View all 16 articles
Hypoxia-inducible factors (HIFs) have become key transcriptional regulators of metabolism, angiogenesis, erythropoiesis, proliferation, inflammation and metastases. HIFs are tightly regulated by the tissue microenvironment. Under the influence of the hypoxic milieu, HIF proteins allow the tissue to adapt its response. This is especially critical for bone, as it constitutes a highly hypoxic environment. As such, bone structure and turnover are strongly influenced by the modulation of oxygen availability and HIFs. Both, bone forming osteoblasts and bone resorbing osteoclasts are targeted by HIFs and modulators of oxygen tension. Experimental and clinical data have delineated the importance of HIF responses in different osteoclast-mediated pathologies. This review will focus on the influence of HIF expression on the regulation of osteoclasts in homeostasis as well as during inflammatory and malignant bone diseases.
Bone is a highly dynamic tissue that undergoes constant remodeling to adapt to changing functional and metabolic demands, but also to repair microdamages that naturally occur throughout life. For example, bone sensitively reacts to loading (e.g., weight-lifting activities) or unloading conditions (e.g., space flight) by increasing or decreasing bone mass, respectively. In addition, bone also adapts to meet changing metabolic demands (Zhang et al., 2015; Shahi et al., 2017; Loeffler et al., 2018), such as during lactation, when bone resorption increases to provide sufficient calcium for milk production (Kovacs, 2005). Thus, bone remodeling is a finely tuned and dynamic system that is required to maintain bone mass as well as mineral homeostasis during adulthood (Al-Bari and Al Mamun, 2020).
Bone remodeling is a temporally and spatially controlled process (Frost, 1963). In adults, about 10% of the bone surface is undergoing remodeling at a given time. The cells that contribute to bone remodeling are grouped into the basic multicellular unit (BMU) (Hauge et al., 2001; Andersen et al., 2009). Therein, osteoclasts, which are of hematopoietic origin, resorb bone (Teitelbaum, 2000). This is followed by a reversal phase, in which osteoclasts vacate the bone remodeling area and allow for osteoblasts, the bone-forming cells, to locate and refill the resorbed area with new bone matrix (Matsuo and Irie, 2008; Sims and Gooi, 2008). This process is estimated to take about 3 months in humans (Eriksen et al., 1984a,b). It is a coupled process, where osteoclasts regulate the differentiation and activity of osteoblasts and vice versa. Besides osteoclasts and osteoblasts, which are the two most important specialized cell types for bone remodeling, several other cell types have been shown to contribute to bone remodeling, such as the osteocytes, which appear to coordinate bone remodeling by sending signals to the osteoclasts and osteoblasts to regulate their activity (Sims and Martin, 2015).
In healthy adults, the amount of newly formed bone equals the amount of resorbed bone, thus, ensuring the maintenance of bone mass. However, several disease conditions including estrogen deficiency, chronic inflammation, and malignant disease lead to uncoupling of bone resorption and bone formation in which bone resorption exceeds bone formation, leading to bone loss and fragility (Roodman, 2004; Pacifici, 2008; Redlich and Smolen, 2012; Klein-Nulend et al., 2015). As such, osteoclasts play a prominent role in diseases characterized by bone loss and therefore are the main therapeutic target of anti-resorptive strategies to treat osteoporosis.
Importantly, both, inflammation and malignancy are characterized by hypoxia and also physiological bone remodeling is under the strict control of hypoxia-related signaling pathways. The latter may be explained by the rather hypoxic microenvironment of bone niche, with in vivo measurements in mice demonstrating local oxygen tension as low as 1.3 kPa (10 mmHg; tissues less than this are generally defined as hypoxic) (McNamee et al., 2013; Spencer et al., 2014).
Hypoxia-inducible factors (HIF) are heterodimeric transcription factors, consisting of an oxygen-labile alpha subunit (HIFα) and a constitutively-stable beta subunit (HIF1β), that exert pivotal roles in inducing cellular responses to hypoxia (Wang et al., 1995; Tian et al., 1997). HIF1α and HIF2α are structurally similar (Loboda et al., 2010). Their stability is post-transcriptionally regulated by oxygen availability through the iron-dependent enzymes prolylhydroxylases (PHDs) (Mole, 2010). In well-oxygenated environment, HIFα is subject to oxygen-dependent hydroxylation at proline residues 564 and/or 402 by PHDs, which leads to binding of the von Hippel Lindau protein (VHL) and an associated ubiquitin protein ligase complex. This leads to ubiquitination and proteasomal degradation of HIFα (Lee et al., 2004). Conversely, the hydroxylation reaction is inhibited under hypoxic condition, HIFα subunits are stabilized and translocate to the nucleus, where they heterodimerize with HIF1β and bind to HRE located within regulatory elements of HIF target genes (Dengler et al., 2014). These are involved in multiple processes such as angiogenesis (Vegf, Pdgf, and Fgf2), erythropoiesis (Epo, Tfr1, and Cp), metabolism (Glut1, Pdk1, Hk2, Ldha, and Mct4), proliferation (Tnfa, Ccnd1, and Igf2), inflammation (Il1b, Il6, and Il17) and metastasis (Met1, Lox1) (Flamme et al., 1997; Jaakkola et al., 2001; Mahon et al., 2001; Wenger et al., 2005; Semenza, 2014). Recent studies showed that many other proteins are involved in the regulation of basal HIF1α levels in an oxygen-independent manner. Luo et al. (2010) delineated that the heat shock protein 70 (HSP70) binds via its carboxy-terminus to HIF1α, leading to recruitment of HSP70-interaction protein (CHIP), a chaperone-dependent E3 ubiquitin ligase, which mediates HIF1α ubiquitination and proteasomal degradation. Additionally, it has been shown that PTEN-PI3K-AKT signaling axis controls E3 ubiquitin-protein ligase Murine double minute 2 (MDM2), which mediates HIF1α ubiquitination under hypoxic conditions in a proteasome-dependent manner (Joshi et al., 2014; Figure 1).
Figure 1. Regulation of HIFα stabilization in normoxia and hypoxia conditions as well in an oxygen independent pathway. In normoxia, HIFα is hydroxylated by prolyl hydroxylase domain (PHD) protein. The proline-hydroxylated HIFα is then recognized by von Hippel-Lindau E3 ubiquitin ligase (VHL) and subjected to be degraded via proteasome. In hypoxia, HIFα becomes stable and dimeries with HIF-1β. After translocation to the nucleus, the HIF heterodimer binds the hypoxia response element (HRE) of target genes to regulate transcription. Besides, NF-κb and STAT3 signaling activation induce Hifa mRNA transcription under normoxia or hypoxia.
Besides the post-transcriptional regulation of HIF1α protein stability, HIFα is also regulated at the transcriptional level. Increased transcription of Hif1a was found in cells after the stimulation of growth factors (FGF, EGF, and Heregulin), cytokines (TNF-α, IL-1, and IL-6) and pathogen associated molecular patterns (PAMP) (LPS and HBx) via the JAK/STAT and NF-κB signaling pathways (Frede et al., 2006; Figure 1). Recognition of pathogens by immune cells activates the mitogen activated protein kinase (MAPK) pathway via pattern recognition receptors signaling, such as toll like receptors (TLRs) (Frede et al., 2006), which leads to the induction of NF-κB and transactivation of Hif1a under normoxia (Rius et al., 2008). In addition, T cell receptor ligation induces substantial accumulation of HIF1α mRNA and protein, especially in the pro-inflammatory T helper 17 (Th17) cell lineage by a mechanism dependent of STAT3 signaling activation (Dang et al., 2011). Besides, MYD88-dependent NF-κB activity is crucial for LPS-induced HIF1α accumulation in dendritic cells (Jantsch et al., 2011). Taken together, growth factors, cytokines and factors stimulating PAMP are critical regulators of HIF1α or HIF2α level in normoxic and hypoxic conditions.
Given the pertinent role of osteoclasts in bone homeostasis and bone disease, and their regulation via hypoxia signaling, this review will summarize the current knowledge on the role of hypoxia signaling on osteoclasts and its potential as therapeutic target to inhibit osteoclast function in inflammatory and malignant bone diseases.
Osteoclasts originate from the erythromyeloid progenitors during embryogenesis and throughout life fuse with hematopoietic stem cells to produce long-lived, multinucleated cells that are capable to resorb bone (Jacome-Galarza et al., 2019). Mononuclear cells also appear to dissociate again from multinucleated osteoclasts, suggesting that osteoclasts are undergoing constant remodeling themselves. Receptor activator of NF-κB ligand (RANKL) is the key cytokine driving osteoclastogenesis. Upon binding to its receptor RANK, RANKL induces differentiation, fusion, and life span of osteoclasts via the activation of pathways downstream of TRAF6 including MAPK, NF-κB, and PI3K/Akt (Nakagawa et al., 1998; Kong et al., 1999; Yahara et al., 2020). These pathways culminate in the activation of NFATc1, AP-1, and NF-κB transcription factors, which induce the expression of typical osteoclastic genes such as cathepsin K or tartrate-resistant acid phosphatase (TRAP). RANKL is mainly produced by cells from the osteogenic lineage (i.e., osteoblasts and osteocytes) together with its natural antagonist osteoprotegerin (OPG). OPG is able to bind RANKL and prevent it from binding to RANK and thus initiating osteoclastogenesis. Therefore, the RANKL/OPG ratio is crucial for predicting the osteoclastic milieu of the environment. Once osteoclasts are formed they attach tightly to the bone matrix via integrins, most prominently αvβ3, and seal off the environment from the area that will be resorbed. Within this sealing zone, osteoclasts acidify the environment and secrete matrix-degrading enzymes such as cathepsin K into the resorption lacunae to resorb the mineralized and organic components of bone. Until recently, osteoclasts have been proposed to undergo apoptosis after bone resorption. However, newer concepts suggest that osteoclasts may recycle (parts) of themselves to fuse with new osteoclast syncytia and engage in new remodeling cycles (McDonald et al., 2019).
Hypoxia is a critical stimulator of osteoclastogenesis in mouse and human cell culture systems. Early studies have shown that low oxygen tension (2% O2) increases osteoclast differentiation and bone resorption in vitro (Arnett et al., 2003; Muzylak et al., 2006; Bozec et al., 2008), while hyperoxia suppresses osteoclastogenesis (Al Hadi et al., 2013; Yu et al., 2020). In an effort to analyze whether HIF1α was responsible for the pro-osteoclast effects, HIF1α protein was expressed in osteoclasts in vitro (Leger et al., 2010). However, in this study, osteoclast generation was inhibited by expression of a constitutively active form of HIF1α, suggesting that other hypoxia-responsive factors may contribute to osteoclastogenesis (Leger et al., 2010). Another study that investigated the potential of hypoxia mimetic PHD inhibitor dimethyloxallyl glycine (DMOG) to rescue ovariectomy-induced bone loss similarly found no effect of DMOG on osteoclast activity (Peng et al., 2014). Finally, Hulley et al. (2017) have shown that activation of HIF1α via deficiency of PHD2 does not affect osteoclast differentiation, but impairs bone resorption in vitro, suggesting that HIF1α may affect the bone resorbing activity of osteoclasts. However, it should be noted that in vivo, PHD2 +/− mice showed normal serum levels of CTX, a bone resorption marker, despite low bone mass (Rauner et al., 2016), suggesting that rather defective osteoblast function contributed to the low bone mass. HIFs are the canonical substrates for PHD-mediated protein hydroxylation. Increasing in vitro evidence indicates that PHD may also have alternative targets such as IKK-β, p105, p53, and FOXO3a (Cockman et al., 2019; Lee, 2019). However, the role of these non-HIF substrates in osteoclastogenesis under hypoxia is still elusive. Besides, neither osteoclast-specific knockout nor treatment with a HIF1α inhibitor altered bone mass or osteoclast numbers under physiological conditions, but in states of estrogen or testosterone deficiency, when osteoclasts are activated, HIF1α deficiency prevented bone loss by suppressing osteoclast activation (Miyauchi et al., 2013; Tando et al., 2016). Overall, direct effects of HIF1α on osteoclastogenesis during physiology appear negligible. However, metabolically, osteoclasts require oxidative phosphorylation during differentiation, while for bone resorption, osteoclasts rely on energy production via glycolysis (Czupalla et al., 2005; Jin et al., 2014; Lemma et al., 2016). As HIFs activate glycolysis, this may in part explain the stronger effect of HIF1α activation on osteoclastic bone resorption rather than differentiation. In fact, HIF1α has been identified as a critical metabolic switch to turn on anaerobic respiration to rapidly increase ATP production in osteoclasts (Morten et al., 2013).
Importantly, there is strong evidence that HIF1α controls osteoclastogenesis via the regulation of the RANKL/OPG ratio and also IL-33 levels in osteoblasts (Wu et al., 2015; Kang et al., 2017; Zhu et al., 2019) and osteocytes even under physiological conditions (Stegen et al., 2018).
In contrast to HIF1α, HIF2α appears to have direct effects on osteoclasts. Overexpression of HIF2α in progenitors increased osteoclast numbers and marker gene expression in vitro (Lee et al., 2019) by upregulating TRAF6 expression. Moreover, osteoclast-specific knockout of HIF2α increased bone mass by decreasing osteoclast numbers (Lee et al., 2019). However, also in case of HIF2α, osteoblast-mediated regulation of osteoclastogenesis via RANKL/OPG seems to play an important role as osteoblast-specific knockout of HIF2α also decreased osteoclast numbers in vivo.
Taken together, HIFs appear to play a more prominent role in osteoblast-to-osteoclast communication rather than directly affecting osteoclastogenesis. Moreover, activation of hypoxia signaling pathways may be more relevant in disease states than during physiological bone remodeling. In the following sections, we will discuss the role of hypoxia-related proteins in inflammatory and malignant diseases.
Rheumatoid arthritis (RA) is a systemic autoimmune disorder that manifests as chronic inflammation and joint tissue destruction (Komatsu and Takayanagi, 2012). Macrophages, T lymphocytes and B lymphocytes are crucial cells in the development and progression of RA (Ma and Pope, 2005; Cope et al., 2007; Marston et al., 2010). Oxygen tension in the synovial fluid of RA patients (range from 18 to 33 mmHg, equivalent to 2 to 4%) was found lower than in healthy controls (range from 69 to 89 mmHg, equivalent to 9 to 12%) (Giatromanolaki et al., 2003; Muz et al., 2009). In addition, tissue oximeters were used to confirm that hypoxia is a feature of RA synovial tissue and correlates with the intensity of the inflammatory process during RA development (Quinonez-Flores et al., 2016). HIFs (HIF1α and HIF2α) could therefore interfere with joint inflammation, angiogenesis and cartilage destruction in RA (Westra et al., 2010; Quinonez-Flores et al., 2016). Different aspects of RA are influenced by the expression of HIFs in stromal cells and immune cells. Hypoxia induces vascular cell adhesion molecule-1 (VCAM1) and stromal cell-derived factor-1 (SDF-1) expression in synovial fibroblasts and promotes lymphocyte homing to joints of RA patients (Hitchon et al., 2002; Hu et al., 2016). Studies have also shown that NF-κB-HIF1α pathway activation drives the migration and invasion of synovial fibroblasts by increasing the expression of MMP2 and MMP9 (Lee et al., 2012). Interestingly, HIF2α was expressed mainly in fibroblast-like synoviocytes (FLS) of RA synovium and regulated the production of RANKL and several catabolic factors such as matrix-degrading enzymes (MMP3, MMP9, MMP12, MMP13, and ADAMTS4), chemokines (CCL2, CCL5, CCL7, CXCL1, CXCL2, CXCL4, CXCL5, and CXCL10) and inflammatory mediators (COX2 and iNOS) (Huh et al., 2015). Moreover, HIF2α expression in FLS controls IL-6 induction and enhances Th17 cell differentiation during RA pathogenesis (Ryu et al., 2014). Also the induction of IL-1, TNF-α, and IL-33 was reported to be increased in FLS via HIFs, which subsequently was reflected by T cell functions with expansion of Th1 and Th17 cells (Sarkar et al., 2010; Samarpita et al., 2018), but also B cell autoantibody production. By a feedback loop by TNF-α, IL-33 manages the control of HIF. Besides HIF2α, also HIF1α increases IL-6 production in RA. Further evidence shows that HIF1α is highly expressed in Th17 cells and that loss of HIF1α in Th17 cells impairs their differentiation and IL-17 production, suggesting that HIF1α expression in Th17 cells might control synovial inflammation in arthritis (Dang et al., 2011). Finally, HIF1α participation in collagen-induced arthritis has been demonstrated by studying conditional HIF1α deletion in B cells, which results in less IL-10-producing B cells and exacerbated Th17 cells mediated inflammation (Meng et al., 2018; Figure 2).
Figure 2. HIFs involvement in the regulation of osteoclastogenesis in arthritic joint. HIFs expression induce the secretion of a number of cytokines from synovial fibroblast and macrophages that enhance osteoclastogenesis. In addition, hypoxia stimulates IL-6 production by synovial fibroblast and macrophages, thereby increasing Th17 differentiation from naïve CD4 T cells. However, HIFs activation in Breg cells inhibit Th17 differentiation via suppressive factor IL-10.
Altogether, HIF1α and HIF2α indirectly regulate osteoclast-induced bone erosion through the control of the pro-inflammatory milieu. However, it remains unclear whether there is a direct involvement of these factors in osteoclasts under inflammatory conditions. Several therapeutic agents use the hypoxic milieu to get activated and to deliver the therapeutic agents to hypoxic cells on a site specific. However, the off-target effects might be an important challenge since hypoxic conditions also appear physiologically (Phillips, 2016). Several agents targeting the HIF pathway, such as specific HIF inhibitors, showed promising results in cancers or in hypoxia-related diseases (Fallah and Rini, 2019). Regarding RA, it has been suggested that local administration of these compounds could avoid their early systemic degradation. Another promising route may be achieved via delivery carriers for example via the delivery of gene therapy targeting HIFs. However, before these therapies approach clinics, several challenges still need to be addressed. In RA, downstream targets of HIFs have been therapeutically targeted, such as antibodies against VEGF, or small molecules against its receptor. In preclinical studies, these approaches showed a significant reduction of inflammation, particularly in the early phase of inflammatory RA development (Lu et al., 2000; De Bandt et al., 2003; Maruotti et al., 2014). Based on the above-mentioned studies, it is evident that HIFs are promising targets for RA.
Bone metastases are incurable, cause pathological fractures, hypercalcemia and reduce the quality of life (Macedo et al., 2017). Initially, the hypoxic bone microenvironment provides an excellent soil for tumor cells to thrive. Once homed, these cells start producing a variety of cytokines and growth factors that activate cells, including osteoclasts (Maurizi and Rucci, 2018). In turn, this will lead to bone absorption and destruction of the microenvironment, which eventually stimulates the proliferation of tumor cells. As such a vicious cycle between tumor cells and osteoclasts is established (Huang et al., 2020). Although osteolytic lesions have been observed in several cancer types, it has been especially detected in breast cancers; a tumor with great avidity for bone metastasizing in up to 80% of stage IV breast cancer patients (Brook et al., 2018). These tumor cells typically produce parathyroid hormone related protein (PTHrP), which stimulates calcium release from bone (Poole and Reeve, 2005), just like PTH. Interestingly, the expression of PTHrP is driven by HIF2α, but not HIF1α, and is not only confined to metastatic tumor cells, but has also been detected in chondrocytes where it is also induced by HIF1α (Browe et al., 2019). PTHrP stimulates osteoblasts to generate RANKL, simultaneously preventing OPG production (Huang et al., 2004). RANKL and RANK have also been shown to be produced by tumor cells in a HIF1α-dependent manner (Tang et al., 2011), suggesting that osteolysis is potentially also feasible through an interaction between the tumor cells and osteoclasts. As mentioned before, in this setting, osteoclastogenesis is driven forward due to an enhanced RANKL/OPG ratio. The consequence is enhanced bone resorption and release of other growth factors including TGFα and PDGF, both stimulating tumor growth and eventually also osteolysis (Janssens et al., 2005). Nevertheless, there are also other reports suggesting RANKL is not HIF-dependent. In that respect, deletion of PHD2 and PHD3 in osteoblast (progenitors) (Osx:cre line) resulted in increased bone volume as a consequence of OPG induction, whereas RANKL levels were not changed (Wu et al., 2015). This finding was also confirmed using a VHL knock-out strategy in primary osteoblasts (Shao et al., 2015) and by us, showing that PHD2 deletion in osteoblasts (Osx:cre line) causes high bone density (Rauner et al., 2016; Stegen et al., 2016). Although more research will be necessary to unravel the background of these opposing results, hypoxia and hypoxia pathway proteins have an impact on stromal cells of the bone/bone marrow environment that directly regulate bone homeostasis and therefore probably also osteolytic lesions.
Interestingly, the impact of hypoxia signaling in bone can also influence the growth and dissemination of external tumors finally ending up in the bone. Devignes and colleagues elegantly showed that HIF-induced CXCL12 production in osteoblast progenitors directly promotes systemic tumor growth and dissemination. In fact, mice conditionally deficient for HIF1α in osteoprogenitors displayed reduced CXCL12+ cells whereas VHL deficiency resulted in the opposite outcome (Devignes et al., 2018). The chemokine CXCL12/stromal cell-derived factor 1 alpha (SDF1) has not only been shown in a variety of different tumor types (Shi et al., 2020), but also plays a central role in the bone marrow niche where it controls hematopoietic stem cell quiescence in conjunction with its receptor CXCR4. In the context of breast cancer cell dissemination, this signaling appears to work via CXCR4 on the tumor cells, underscoring local hypoxic signaling in the BM niche exerting control on distant tumors, impacting growth and metastasis (Xu et al., 2015). This suggests that targeting CXCL12/CXCR4 would be beneficial, but different experimental approaches reveal case-by-case differences (Zielinska and Katanaev, 2020). Indeed, although systemic CXCR4 inhibition might be beneficial in breast cancer growth, deficiency of CXCR4 in osteoclasts was shown to enhance osteoclastogenesis, which in turn may again promote bone metastasis and stimulate the vicious cycle (Zielinska and Katanaev, 2020).
Conversely, hypoxia in a variety of different tumors outside the bone/bone marrow area can also affect the bone and its environment by enhancing future colonization of tumor cells and even promoting pre-osteolysis. First indications for this paradigm were reported almost two decades ago, as researchers found a clear correlation between HIF1α expression in primary breast cancers and the presence of (micro)metastasis in the bone marrow of these patients (Woelfle et al., 2003). HIF1α-induced lysyl oxidase (LOX), a copper-dependent amine oxidases, is such a molecule that can cause tumor cell dissemination and tumor driven osteolytic lesions (Bondareva et al., 2009). At the same time, it promotes RANKL-dependent differentiation of osteoclasts, while inhibiting osteoblast differentiation (Reynaud et al., 2017). This suggests that LOX secreted by tumor cells induces osteoclastogenesis thereby creating a pre-metastatic niche that would favor tumor homing and growth. Interestingly, LOX induction itself also enhances HIF1α expression, underscoring the synergism between LOX/HIF in regulating the adaptation of tumor cells to hypoxia (Pez et al., 2011) and beyond.
The role of hypoxia and HIFs is evident in bone physiology and in numerous pathophysiological diseases where osteoclasts are activated and induce bone loss. However, the exact role of HIF1α or HIF2α in osteoclast remains quite vague and largely appear to be mediated indirectly via other cells like stromal cells. However, they should be taken into consideration when thinking of the indirect pathway of osteoclast activation, notably by their function in the immune cells, in particular in Th17/Treg cells or in macrophages. Therefore, HIF inhibitors would likely target osteoclast activation and secondary bone loss in numerous diseases. As example, a recent study discovered an increased bone mass in mice treated with HIF1α inhibitor 2ME2 (Miyauchi et al., 2013). However, it still remains inconclusive whether HIF inhibitors would act the same way in human bone diseases as in murine models. Future studies on HIF signaling and its clinical relevance may improve our understanding of the role of HIF in osteoclastogenesis and eventually lead to effective treatments for human diseases involving bone homeostasis.
MR and AB contributed equally to the work. XM, BW, MR, and AB conceived and wrote the review. All authors contributed to the article and approved the submitted version.
The authors declare that the research was conducted in the absence of any commercial or financial relationships that could be construed as a potential conflict of interest.
This work was supported by grants from the German Research Foundation (μBone SPP 2084) to BW, MR, and AB as well as a grant from the German-Israeli-Foundation (No. I-1433-203.13_2017) to BW and MR.
Al Hadi, H., Smerdon, G. R., and Fox, S. W. (2013). Hyperbaric oxygen therapy suppresses osteoclast formation and bone resorption. J. Orthop. Res. 31, 1839–1844. doi: 10.1002/jor.22443
Al-Bari, A. A., and Al Mamun, A. (2020). Current advances in regulation of bone homeostasis. FASEB Bioadv. 2, 668–679. doi: 10.1096/fba.2020-00058
Andersen, T. L., Sondergaard, T. E., Skorzynska, K. E., Dagnaes-Hansen, F., Plesner, T. L., Hauge, E. M., et al. (2009). A physical mechanism for coupling bone resorption and formation in adult human bone. Am. J. Pathol. 174, 239–247. doi: 10.2353/ajpath.2009.080627
Arnett, T. R., Gibbons, D. C., Utting, J. C., Orriss, I. R., Hoebertz, A., Rosendaal, M., et al. (2003). Hypoxia is a major stimulator of osteoclast formation and bone resorption. J. Cell Physiol. 196, 2–8. doi: 10.1002/jcp.10321
Bondareva, A., Downey, C. M., Ayres, F., Liu, W., Boyd, S. K., Hallgrimsson, B., et al. (2009). The lysyl oxidase inhibitor, beta-aminopropionitrile, diminishes the metastatic colonization potential of circulating breast cancer cells. PLoS One 4:e5620. doi: 10.1371/journal.pone.0005620
Bozec, A., Bakiri, L., Hoebertz, A., Eferl, R., Schilling, A. F., Komnenovic, V., et al. (2008). Osteoclast size is controlled by Fra-2 through LIF/LIF-receptor signalling and hypoxia. Nature 454, 221–225. doi: 10.1038/nature07019
Brook, N., Brook, E., Dharmarajan, A., Dass, C. R., and Chan, A. (2018). Breast cancer bone metastases: pathogenesis and therapeutic targets. Int. J. Biochem. Cell Biol. 96, 63–78. doi: 10.1016/j.biocel.2018.01.003
Browe, D. C., Coleman, C. M., Barry, F. P., and Elliman, S. J. (2019). Hypoxia activates the PTHrP -MEF2C pathway to attenuate hypertrophy in mesenchymal stem cell derived cartilage. Sci. Rep. 9:13274. doi: 10.1038/s41598-019-49499-x
Cockman, M. E., Lippl, K., Tian, Y. M., Pegg, H. B., Figg, W. D. J., Abboud, M. I., et al. (2019). Lack of activity of recombinant HIF prolyl hydroxylases (PHDs) on reported non-HIF substrates. Elife 8:e46490. doi: 10.7554/eLife.46490
Cope, A. P., Schulze-Koops, H., and Aringer, M. (2007). The central role of T cells in rheumatoid arthritis. Clin. Exp. Rheumatol. 25(5 Suppl. 46), S4–S11.
Czupalla, C., Mansukoski, H., Pursche, T., Krause, E., and Hoflack, B. (2005). Comparative study of protein and mRNA expression during osteoclastogenesis. Proteomics 5, 3868–3875. doi: 10.1002/pmic.200402059
Dang, E. V., Barbi, J., Yang, H. Y., Jinasena, D., Yu, H., Zheng, Y., et al. (2011). Control of T(H)17/T(reg) balance by hypoxia-inducible factor 1. Cell 146, 772–784. doi: 10.1016/j.cell.2011.07.033
De Bandt, M., Ben Mahdi, M. H., Ollivier, V., Grossin, M., Dupuis, M., Gaudry, M., et al. (2003). Blockade of vascular endothelial growth factor receptor I (VEGF-RI), but not VEGF-RII, suppresses joint destruction in the K/BxN model of rheumatoid arthritis. J. Immunol. 171, 4853–4859. doi: 10.4049/jimmunol.171.9.4853
Dengler, V. L., Galbraith, M., and Espinosa, J. M. (2014). Transcriptional regulation by hypoxia inducible factors. Crit. Rev. Biochem. Mol. Biol. 49, 1–15. doi: 10.3109/10409238.2013.838205
Devignes, C. S., Aslan, Y., Brenot, A., Devillers, A., Schepers, K., Fabre, S., et al. (2018). HIF signaling in osteoblast-lineage cells promotes systemic breast cancer growth and metastasis in mice. Proc. Natl. Acad. Sci. U.S.A. 115, E992–E1001. doi: 10.1073/pnas.1718009115
Eriksen, E. F., Gundersen, H. J., Melsen, F., and Mosekilde, L. (1984a). Reconstruction of the formative site in iliac trabecular bone in 20 normal individuals employing a kinetic model for matrix and mineral apposition. Metab. Bone Dis. Relat. Res. 5, 243–252. doi: 10.1016/0221-8747(84)90066-3
Eriksen, E. F., Melsen, F., and Mosekilde, L. (1984b). Reconstruction of the resorptive site in iliac trabecular bone: a kinetic model for bone resorption in 20 normal individuals. Metab. Bone Dis. Relat. Res. 5, 235–242. doi: 10.1016/0221-8747(84)90065-1
Fallah, J., and Rini, B. I. (2019). HIF inhibitors: status of current clinical development. Curr. Oncol. Rep. 21:6. doi: 10.1007/s11912-019-0752-z
Flamme, I., Frohlich, T., von Reutern, M., Kappel, A., Damert, A., and Risau, W. (1997). HRF, a putative basic helix-loop-helix-PAS-domain transcription factor is closely related to hypoxia-inducible factor-1 alpha and developmentally expressed in blood vessels. Mech. Dev. 63, 51–60. doi: 10.1016/s0925-4773(97)00674-6
Frede, S., Stockmann, C., Freitag, P., and Fandrey, J. (2006). Bacterial lipopolysaccharide induces HIF-1 activation in human monocytes via p44/42 MAPK and NF-kappaB. Biochem. J. 396, 517–527. doi: 10.1042/BJ20051839
Giatromanolaki, A., Sivridis, E., Maltezos, E., Athanassou, N., Papazoglou, D., Gatter, K. C., et al. (2003). Upregulated hypoxia inducible factor-1alpha and -2alpha pathway in rheumatoid arthritis and osteoarthritis. Arthritis Res. Ther. 5, R193–R201. doi: 10.1186/ar756
Hauge, E. M., Qvesel, D., Eriksen, E. F., Mosekilde, L., and Melsen, F. (2001). Cancellous bone remodeling occurs in specialized compartments lined by cells expressing osteoblastic markers. J. Bone Miner. Res. 16, 1575–1582. doi: 10.1359/jbmr.2001.16.9.1575
Hitchon, C., Wong, K., Ma, G., Reed, J., Lyttle, D., and El-Gabalawy, H. (2002). Hypoxia-induced production of stromal cell-derived factor 1 (CXCL12) and vascular endothelial growth factor by synovial fibroblasts. Arthritis Rheum. 46, 2587–2597. doi: 10.1002/art.10520
Hu, F., Liu, H., Xu, L., Li, Y., Liu, X., Shi, L., et al. (2016). Hypoxia-inducible factor-1alpha perpetuates synovial fibroblast interactions with T cells and B cells in rheumatoid arthritis. Eur. J. Immunol. 46, 742–751. doi: 10.1002/eji.201545784
Huang, J. C., Sakata, T., Pfleger, L. L., Bencsik, M., Halloran, B. P., Bikle, D. D., et al. (2004). PTH differentially regulates expression of RANKL and OPG. J. Bone Miner. Res. 19, 235–244. doi: 10.1359/JBMR.0301226
Huang, Y., Xiao, Z., Guan, Z., Zeng, Z., Shen, Y., Xu, X., et al. (2020). Bone-seeking nanoplatform co-delivering cisplatin and zoledronate for synergistic therapy of breast cancer bone metastasis and bone resorption. Acta Pharm. Sin. B 10, 2384–2403. doi: 10.1016/j.apsb.2020.06.006
Huh, Y. H., Lee, G., Lee, K. B., Koh, J. T., Chun, J. S., and Ryu, J. H. (2015). HIF-2alpha-induced chemokines stimulate motility of fibroblast-like synoviocytes and chondrocytes into the cartilage-pannus interface in experimental rheumatoid arthritis mouse models. Arthritis Res. Ther. 17:302. doi: 10.1186/s13075-015-0816-x
Hulley, P. A., Bishop, T., Vernet, A., Schneider, J. E., Edwards, J. R., Athanasou, N. A., et al. (2017). Hypoxia-inducible factor 1-alpha does not regulate osteoclastogenesis but enhances bone resorption activity via prolyl-4-hydroxylase 2. J. Pathol. 242, 322–333. doi: 10.1002/path.4906
Jaakkola, P., Mole, D. R., Tian, Y. M., Wilson, M. I., Gielbert, J., Gaskell, S. J., et al. (2001). Targeting of HIF-alpha to the von Hippel-Lindau ubiquitylation complex by O2-regulated prolyl hydroxylation. Science 292, 468–472. doi: 10.1126/science.1059796
Jacome-Galarza, C. E., Percin, G. I., Muller, J. T., Mass, E., Lazarov, T., Eitler, J., et al. (2019). Developmental origin, functional maintenance and genetic rescue of osteoclasts. Nature 568, 541–545. doi: 10.1038/s41586-019-1105-7
Janssens, K., ten Dijke, P., Janssens, S., and Van Hul, W. (2005). Transforming growth factor-beta1 to the bone. Endocr. Rev. 26, 743–774. doi: 10.1210/er.2004-0001
Jantsch, J., Wiese, M., Schodel, J., Castiglione, K., Glasner, J., Kolbe, S., et al. (2011). Toll-like receptor activation and hypoxia use distinct signaling pathways to stabilize hypoxia-inducible factor 1alpha (HIF1A) and result in differential HIF1A-dependent gene expression. J. Leukoc. Biol. 90, 551–562. doi: 10.1189/jlb.1210683
Jin, Z., Wei, W., Yang, M., Du, Y., and Wan, Y. (2014). Mitochondrial complex I activity suppresses inflammation and enhances bone resorption by shifting macrophage-osteoclast polarization. Cell Metab. 20, 483–498. doi: 10.1016/j.cmet.2014.07.011
Joshi, S., Singh, A. R., and Durden, D. L. (2014). MDM2 regulates hypoxic hypoxia-inducible factor 1alpha stability in an E3 ligase, proteasome, and PTEN-phosphatidylinositol 3-kinase-AKT-dependent manner. J. Biol. Chem. 289, 22785–22797. doi: 10.1074/jbc.M114.587493
Kang, H., Yang, K., Xiao, L., Guo, L., Guo, C., Yan, Y., et al. (2017). Osteoblast hypoxia-inducible factor-1alpha pathway activation restrains osteoclastogenesis via the interleukin-33-MicroRNA-34a-Notch1 pathway. Front. Immunol. 8:1312. doi: 10.3389/fimmu.2017.01312
Klein-Nulend, J., van Oers, R. F., Bakker, A. D., and Bacabac, R. G. (2015). Bone cell mechanosensitivity, estrogen deficiency, and osteoporosis. J. Biomech. 48, 855–865. doi: 10.1016/j.jbiomech.2014.12.007
Komatsu, N., and Takayanagi, H. (2012). Inflammation and bone destruction in arthritis: synergistic activity of immune and mesenchymal cells in joints. Front. Immunol. 3:77. doi: 10.3389/fimmu.2012.00077
Kong, Y. Y., Yoshida, H., Sarosi, I., Tan, H. L., Timms, E., Capparelli, C., et al. (1999). OPGL is a key regulator of osteoclastogenesis, lymphocyte development and lymph-node organogenesis. Nature 397, 315–323. doi: 10.1038/16852
Kovacs, C. S. (2005). Calcium and bone metabolism during pregnancy and lactation. J. Mammary Gland Biol. Neoplasia 10, 105–118. doi: 10.1007/s10911-005-5394-0
Lee, J. W., Bae, S. H., Jeong, J. W., Kim, S. H., and Kim, K. W. (2004). Hypoxia-inducible factor (HIF-1)alpha: its protein stability and biological functions. Exp. Mol. Med. 36, 1–12. doi: 10.1038/emm.2004.1
Lee, S. Y., Park, K. H., Yu, H. G., Kook, E., Song, W. H., Lee, G., et al. (2019). Controlling hypoxia-inducible factor-2alpha is critical for maintaining bone homeostasis in mice. Bone Res. 7:14. doi: 10.1038/s41413-019-0054-y
Lee, Y. A., Choi, H. M., Lee, S. H., Hong, S. J., Yang, H. I., Yoo, M. C., et al. (2012). Hypoxia differentially affects IL-1beta-stimulated MMP-1 and MMP-13 expression of fibroblast-like synoviocytes in an HIF-1alpha-dependent manner. Rheumatology (Oxford) 51, 443–450. doi: 10.1093/rheumatology/ker327
Leger, A. J., Altobelli, A., Mosquea, L. M., Belanger, A. J., Song, A., Cheng, S. H., et al. (2010). Inhibition of osteoclastogenesis by prolyl hydroxylase inhibitor dimethyloxallyl glycine. J. Bone Miner. Metab. 28, 510–519. doi: 10.1007/s00774-010-0171-6
Lemma, S., Sboarina, M., Porporato, P. E., Zini, N., Sonveaux, P., Di Pompo, G., et al. (2016). Energy metabolism in osteoclast formation and activity. Int. J. Biochem. Cell Biol. 79, 168–180. doi: 10.1016/j.biocel.2016.08.034
Loboda, A., Jozkowicz, A., and Dulak, J. (2010). HIF-1 and HIF-2 transcription factors–similar but not identical. Mol. Cells 29, 435–442. doi: 10.1007/s10059-010-0067-2
Loeffler, J., Duda, G. N., Sass, F. A., and Dienelt, A. (2018). The metabolic microenvironment steers bone tissue regeneration. Trends Endocrinol. Metab. 29, 99–110. doi: 10.1016/j.tem.2017.11.008
Lu, J., Kasama, T., Kobayashi, K., Yoda, Y., Shiozawa, F., Hanyuda, M., et al. (2000). Vascular endothelial growth factor expression and regulation of murine collagen-induced arthritis. J. Immunol. 164, 5922–5927. doi: 10.4049/jimmunol.164.11.5922
Luo, W., Zhong, J., Chang, R., Hu, H., Pandey, A., and Semenza, G. L. (2010). Hsp70 and CHIP selectively mediate ubiquitination and degradation of hypoxia-inducible factor (HIF)-1alpha but not HIF-2alpha. J. Biol. Chem. 285, 3651–3663. doi: 10.1074/jbc.M109.068577
Ma, Y., and Pope, R. M. (2005). The role of macrophages in rheumatoid arthritis. Curr. Pharm. Des. 11, 569–580. doi: 10.2174/1381612053381927
Macedo, F., Ladeira, K., Pinho, F., Saraiva, N., Bonito, N., Pinto, L., et al. (2017). Bone metastases: an overview. Oncol. Rev. 11:321. doi: 10.4081/oncol.2017.321
Mahon, P. C., Hirota, K., and Semenza, G. L. (2001). FIH-1: a novel protein that interacts with HIF-1alpha and VHL to mediate repression of HIF-1 transcriptional activity. Genes Dev. 15, 2675–2686. doi: 10.1101/gad.924501
Marston, B., Palanichamy, A., and Anolik, J. H. (2010). B cells in the pathogenesis and treatment of rheumatoid arthritis. Curr. Opin. Rheumatol. 22, 307–315. doi: 10.1097/BOR.0b013e3283369cb8
Maruotti, N., Cantatore, F. P., and Ribatti, D. (2014). Putative effects of potentially anti-angiogenic drugs in rheumatic diseases. Eur. J. Clin. Pharmacol. 70, 135–140. doi: 10.1007/s00228-013-1605-6
Matsuo, K., and Irie, N. (2008). Osteoclast-osteoblast communication. Arch. Biochem. Biophys. 473, 201–209. doi: 10.1016/j.abb.2008.03.027
Maurizi, A., and Rucci, N. (2018). The osteoclast in bone metastasis: player and target. Cancers (Basel) 10:218. doi: 10.3390/cancers10070218
McDonald, M., Khoo, W. H., Mohanty, S., Terry, R., Chai, R., Quinn, J., et al. (2019). Intravital imaging of osteoclasts in vivo reveals novel osteoclast fate which may underlie the therapeutic response to Denosumab withdrawal. J. Bone Miner. Res. 34, 44–45.
McNamee, E. N., Korns Johnson, D., Homann, D., and Clambey, E. T. (2013). Hypoxia and hypoxia-inducible factors as regulators of T cell development, differentiation, and function. Immunol. Res. 55, 58–70. doi: 10.1007/s12026-012-8349-8
Meng, X., Grotsch, B., Luo, Y., Knaup, K. X., Wiesener, M. S., Chen, X. X., et al. (2018). Hypoxia-inducible factor-1alpha is a critical transcription factor for IL-10-producing B cells in autoimmune disease. Nat. Commun. 9:251. doi: 10.1038/s41467-017-02683-x
Miyauchi, Y., Sato, Y., Kobayashi, T., Yoshida, S., Mori, T., Kanagawa, H., et al. (2013). HIF1alpha is required for osteoclast activation by estrogen deficiency in postmenopausal osteoporosis. Proc. Natl. Acad. Sci. U.S.A. 110, 16568–16573. doi: 10.1073/pnas.1308755110
Mole, D. R. (2010). Iron homeostasis and its interaction with prolyl hydroxylases. Antioxid. Redox Signal. 12, 445–458. doi: 10.1089/ars.2009.2790
Morten, K. J., Badder, L., and Knowles, H. J. (2013). Differential regulation of HIF-mediated pathways increases mitochondrial metabolism and ATP production in hypoxic osteoclasts. J. Pathol. 229, 755–764. doi: 10.1002/path.4159
Muz, B., Khan, M. N., Kiriakidis, S., and Paleolog, E. M. (2009). Hypoxia. The role of hypoxia and HIF-dependent signalling events in rheumatoid arthritis. Arthritis Res. Ther. 11:201. doi: 10.1186/ar2568
Muzylak, M., Price, J. S., and Horton, M. A. (2006). Hypoxia induces giant osteoclast formation and extensive bone resorption in the cat. Calcif. Tissue Int. 79, 301–309. doi: 10.1007/s00223-006-0082-7
Nakagawa, N., Kinosaki, M., Yamaguchi, K., Shima, N., Yasuda, H., Yano, K., et al. (1998). RANK is the essential signaling receptor for osteoclast differentiation factor in osteoclastogenesis. Biochem. Biophys. Res. Commun. 253, 395–400. doi: 10.1006/bbrc.1998.9788
Pacifici, R. (2008). Estrogen deficiency, T cells and bone loss. Cell Immunol. 252, 68–80. doi: 10.1016/j.cellimm.2007.06.008
Peng, J., Lai, Z. G., Fang, Z. L., Xing, S., Hui, K., Hao, C., et al. (2014). Dimethyloxalylglycine prevents bone loss in ovariectomized C57BL/6J mice through enhanced angiogenesis and osteogenesis. PLoS One 9:e112744. doi: 10.1371/journal.pone.0112744
Pez, F., Dayan, F., Durivault, J., Kaniewski, B., Aimond, G., Le Provost, G. S., et al. (2011). The HIF-1-inducible lysyl oxidase activates HIF-1 via the Akt pathway in a positive regulation loop and synergizes with HIF-1 in promoting tumor cell growth. Cancer Res. 71, 1647–1657. doi: 10.1158/0008-5472.CAN-10-1516
Phillips, R. M. (2016). Targeting the hypoxic fraction of tumours using hypoxia-activated prodrugs. Cancer Chemother. Pharmacol. 77, 441–457. doi: 10.1007/s00280-015-2920-7
Poole, K. E., and Reeve, J. (2005). Parathyroid hormone – a bone anabolic and catabolic agent. Curr. Opin. Pharmacol. 5, 612–617. doi: 10.1016/j.coph.2005.07.004
Quinonez-Flores, C. M., Gonzalez-Chavez, S. A., and Pacheco-Tena, C. (2016). Hypoxia and its implications in rheumatoid arthritis. J. Biomed. Sci. 23:62. doi: 10.1186/s12929-016-0281-0
Rauner, M., Franke, K., Murray, M., Singh, R. P., Hiram-Bab, S., Platzbecker, U., et al. (2016). Increased EPO levels are associated with bone loss in mice lacking PHD2 in EPO-producing cells. J. Bone Miner. Res. 31, 1877–1887. doi: 10.1002/jbmr.2857
Redlich, K., and Smolen, J. S. (2012). Inflammatory bone loss: pathogenesis and therapeutic intervention. Nat. Rev. Drug Discov. 11, 234–250. doi: 10.1038/nrd3669
Reynaud, C., Ferreras, L., Di Mauro, P., Kan, C., Croset, M., Bonnelye, E., et al. (2017). Lysyl oxidase is a strong determinant of tumor cell colonization in bone. Cancer Res. 77, 268–278. doi: 10.1158/0008-5472.CAN-15-2621
Rius, J., Guma, M., Schachtrup, C., Akassoglou, K., Zinkernagel, A. S., Nizet, V., et al. (2008). NF-kappaB links innate immunity to the hypoxic response through transcriptional regulation of HIF-1alpha. Nature 453, 807–811. doi: 10.1038/nature06905
Roodman, G. D. (2004). Pathogenesis of myeloma bone disease. Blood Cells Mol. Dis. 32, 290–292. doi: 10.1016/j.bcmd.2004.01.001
Ryu, J. H., Chae, C. S., Kwak, J. S., Oh, H., Shin, Y., Huh, Y. H., et al. (2014). Hypoxia-inducible factor-2alpha is an essential catabolic regulator of inflammatory rheumatoid arthritis. PLoS Biol. 12:e1001881. doi: 10.1371/journal.pbio.1001881
Samarpita, S., Doss, H. M., Ganesan, R., and Rasool, M. (2018). Interleukin 17 under hypoxia mimetic condition augments osteoclast mediated bone erosion and expression of HIF-1alpha and MMP-9. Cell Immunol. 332, 39–50. doi: 10.1016/j.cellimm.2018.07.005
Sarkar, S., Cooney, L. A., and Fox, D. A. (2010). The role of T helper type 17 cells in inflammatory arthritis. Clin. Exp. Immunol. 159, 225–237. doi: 10.1111/j.1365-2249.2009.04016.x
Semenza, G. L. (2014). Oxygen sensing, hypoxia-inducible factors, and disease pathophysiology. Annu. Rev. Pathol. 9, 47–71. doi: 10.1146/annurev-pathol-012513-104720
Shahi, M., Peymani, A., and Sahmani, M. (2017). Regulation of bone metabolism. Rep. Biochem. Mol. Biol. 5, 73–82.
Shao, J., Zhang, Y., Yang, T., Qi, J., Zhang, L., and Deng, L. (2015). HIF-1alpha disturbs osteoblasts and osteoclasts coupling in bone remodeling by up-regulating OPG expression. In Vitro Cell. Dev. Biol. Anim. 51, 808–814. doi: 10.1007/s11626-015-9895-x
Shi, Y., Riese, D. J. II, and Shen, J. (2020). The role of the CXCL12/CXCR4/CXCR7 chemokine axis in cancer. Front. Pharmacol. 11:574667. doi: 10.3389/fphar.2020.574667
Sims, N. A., and Gooi, J. H. (2008). Bone remodeling: multiple cellular interactions required for coupling of bone formation and resorption. Semin. Cell Dev. Biol. 19, 444–451. doi: 10.1016/j.semcdb.2008.07.016
Sims, N. A., and Martin, T. J. (2015). Coupling signals between the osteoclast and osteoblast: how are messages transmitted between these temporary visitors to the bone surface? Front. Endocrinol. (Lausanne) 6:41. doi: 10.3389/fendo.2015.00041
Spencer, J. A., Ferraro, F., Roussakis, E., Klein, A., Wu, J., Runnels, J. M., et al. (2014). Direct measurement of local oxygen concentration in the bone marrow of live animals. Nature 508, 269–273. doi: 10.1038/nature13034
Stegen, S., Stockmans, I., Moermans, K., Thienpont, B., Maxwell, P. H., Carmeliet, P., et al. (2018). Osteocytic oxygen sensing controls bone mass through epigenetic regulation of sclerostin. Nat Commun. 9:2557. doi: 10.1038/s41467-018-04679-7
Stegen, S., van Gastel, N., Eelen, G., Ghesquiere, B., D’Anna, F., Thienpont, B., et al. (2016). HIF-1alpha promotes glutamine-mediated redox homeostasis and glycogen-dependent bioenergetics to support postimplantation bone cell survival. Cell Metab. 23, 265–279. doi: 10.1016/j.cmet.2016.01.002
Tando, T., Sato, Y., Miyamoto, K., Morita, M., Kobayashi, T., Funayama, A., et al. (2016). Hif1alpha is required for osteoclast activation and bone loss in male osteoporosis. Biochem. Biophys. Res. Commun. 470, 391–396. doi: 10.1016/j.bbrc.2016.01.033
Tang, Z. N., Zhang, F., Tang, P., Qi, X. W., and Jiang, J. (2011). Hypoxia induces RANK and RANKL expression by activating HIF-1alpha in breast cancer cells. Biochem. Biophys. Res. Commun. 408, 411–416. doi: 10.1016/j.bbrc.2011.04.035
Teitelbaum, S. L. (2000). Bone resorption by osteoclasts. Science 289, 1504–1508. doi: 10.1126/science.289.5484.1504
Tian, H., McKnight, S. L., and Russell, D. W. (1997). Endothelial PAS domain protein 1 (EPAS1), a transcription factor selectively expressed in endothelial cells. Genes Dev. 11, 72–82. doi: 10.1101/gad.11.1.72
Wang, G. L., Jiang, B. H., Rue, E. A., and Semenza, G. L. (1995). Hypoxia-inducible factor 1 is a basic-helix-loop-helix-PAS heterodimer regulated by cellular O2 tension. Proc. Natl. Acad. Sci. U.S.A. 92, 5510–5514. doi: 10.1073/pnas.92.12.5510
Wenger, R. H., Stiehl, D. P., and Camenisch, G. (2005). Integration of oxygen signaling at the consensus HRE. Sci. STKE 2005:re12. doi: 10.1126/stke.3062005re12
Westra, J., Molema, G., and Kallenberg, C. G. (2010). Hypoxia-inducible factor-1 as regulator of angiogenesis in rheumatoid arthritis – therapeutic implications. Curr. Med. Chem. 17, 254–263. doi: 10.2174/092986710790149783
Woelfle, U., Cloos, J., Sauter, G., Riethdorf, L., Janicke, F., van Diest, P., et al. (2003). Molecular signature associated with bone marrow micrometastasis in human breast cancer. Cancer Res. 63, 5679–5684.
Wu, C., Rankin, E. B., Castellini, L., Alcudia, J. F., LaGory, E. L., Andersen, R., et al. (2015). Oxygen-sensing PHDs regulate bone homeostasis through the modulation of osteoprotegerin. Genes Dev. 29, 817–831. doi: 10.1101/gad.255000.114
Xu, C., Zhao, H., Chen, H., and Yao, Q. (2015). CXCR4 in breast cancer: oncogenic role and therapeutic targeting. Drug Des. Devel. Ther. 9, 4953–4964. doi: 10.2147/DDDT.S84932
Yahara, Y., Barrientos, T., Tang, Y. J., Puviindran, V., Nadesan, P., Zhang, H., et al. (2020). Erythromyeloid progenitors give rise to a population of osteoclasts that contribute to bone homeostasis and repair. Nat. Cell Biol. 22, 49–59. doi: 10.1038/s41556-019-0437-8
Yu, X., Jiang, H., Cheng, G., Shang, W., and Zhang, S. (2020). High levels of HIF-1a in hypoxic dental pulps associated with teeth with severe periodontitis. J. Mol. Histol. 51, 265–275. doi: 10.1007/s10735-020-09878-5
Zhang, Q., Riddle, R. C., and Clemens, T. L. (2015). Bone and the regulation of global energy balance. J. Intern. Med. 277, 681–689. doi: 10.1111/joim.12348
Zhu, J., Tang, Y., Wu, Q., Ji, Y. C., Feng, Z. F., and Kang, F. W. (2019). HIF-1alpha facilitates osteocyte-mediated osteoclastogenesis by activating JAK2/STAT3 pathway in vitro. J. Cell. Physiol. 234, 21182–21192. doi: 10.1002/jcp.28721
Keywords: hypoxia, HIF, osteoclast, osteoblast, bone homeostasis, inflammation, malignant bone disease
Citation: Meng X, Wielockx B, Rauner M and Bozec A (2021) Hypoxia-Inducible Factors Regulate Osteoclasts in Health and Disease. Front. Cell Dev. Biol. 9:658893. doi: 10.3389/fcell.2021.658893
Received: 26 January 2021; Accepted: 18 February 2021;
Published: 18 March 2021.
Edited by:
Ari Elson, Weizmann Institute of Science, IsraelReviewed by:
Cormac Taylor, University College Dublin, IrelandCopyright © 2021 Meng, Wielockx, Rauner and Bozec. This is an open-access article distributed under the terms of the Creative Commons Attribution License (CC BY). The use, distribution or reproduction in other forums is permitted, provided the original author(s) and the copyright owner(s) are credited and that the original publication in this journal is cited, in accordance with accepted academic practice. No use, distribution or reproduction is permitted which does not comply with these terms.
*Correspondence: Aline Bozec, YWxpbmUuYm96ZWNAdWstZXJsYW5nZW4uZGU=
†These authors have contributed equally to this work
Disclaimer: All claims expressed in this article are solely those of the authors and do not necessarily represent those of their affiliated organizations, or those of the publisher, the editors and the reviewers. Any product that may be evaluated in this article or claim that may be made by its manufacturer is not guaranteed or endorsed by the publisher.
Research integrity at Frontiers
Learn more about the work of our research integrity team to safeguard the quality of each article we publish.