- 1Institute of Marine Research, Research Group Reproduction and Developmental Biology, Bergen, Norway
- 2Institute of Marine Research, Research Group Reproduction and Developmental Biology, Austevoll Research Station, Storebø, Norway
- 3Institute of Marine Research, Research Group Reproduction and Developmental Biology, Matre Research Station, Matredal, Norway
- 4Reproductive Biology Group, Division Developmental Biology, Department Biology, Science Faculty, Utrecht University, Utrecht, Netherlands
Entering meiosis strictly depends on stimulated by retinoic acid 8 (Stra8) gene function in mammals. This gene is missing in a number of fish species, including medaka and zebrafish, but is present in the majority of fishes, including Atlantic salmon. Here, we have examined the effects of removing stra8 on male fertility in Atlantic salmon. As in mammals, stra8 expression was restricted to germ cells in the testis, transcript levels increased during the start of puberty, and decreased when blocking the production of retinoic acid. We targeted the salmon stra8 gene with two gRNAs one of these were highly effective and produced numerous mutations in stra8, which led to a loss of wild-type (WT) stra8 expression in F0 salmon testis. In maturing stra8 crispants, the spermatogenetic tubuli were partially disorganized and displayed a sevenfold increase in germ cell apoptosis, in particular among type B spermatogonia and spermatocytes. The production of spermatogenic cysts, on the other hand, increased in maturing stra8 crispants. Gene expression analysis revealed unchanged (lin28a, ret) or reduced levels (egr1, dusp4) of transcripts associated with undifferentiated spermatogonia. Decreased expression was recorded for some genes expressed in differentiating spermatogonia including dmrt1 and ccnd2 or in spermatocytes, such as ccna1. Different from Stra8-deficient mammals, a large number of germ cells completed spermatogenesis, sperm was produced and fertilization rates were similar in WT and crispant males. While loss of stra8 increased germ cell apoptosis during salmon spermatogenesis, crispants compensated this cell loss by an elevated production of spermatogenic cysts, and were able to produce functional sperm. It appears that also in a fish species with a stra8 gene in the genome, the critical relevance this gene has attained for mammalian spermatogenesis is not yet given, although detrimental effects of the loss of stra8 were clearly visible during maturation.
Introduction
In mammals the start of meiosis strictly depends on Stra8 (Endo et al., 2015), and loss of this gene in mice leads to sterility in both males and females (Baltus et al., 2006; Anderson et al., 2008). STRA8 activates a broad transcriptional program in the mouse testis, that includes genes involved in the meiotic prophase, but also DNA replication and the G1-S cell cycle transition (Kojima et al., 2019). Considering that Stra8 transcripts were detectable already in undifferentiated spermatogonia in mice (Zhou et al., 2008), STRA8 may have functions, although perhaps not critical ones, also during the early mitotic phase of spermatogenesis. Retinoic acid (RA), a derivative of vitamin A, strongly up-regulates expression of Stra8 in mammalian germ cells which is critical for developmental transitions in spermatogenesis including entry into meiosis (Endo et al., 2015). Also in teleost fish which lack a stra8 gene (Pasquier et al., 2016), RA signaling promotes spermatogenesis such as the differentiation of spermatogonia in zebrafish (Crespo et al., 2019a), or the meiotic initiation in medaka (Adolfi et al., 2016) and tilapia (Feng et al., 2015). As these fish still are able to successfully complete spermatogenesis despite lacking a stra8 gene, the functional relevance for stra8 in fish needs further investigation. Zebrafish, medaka and tilapia belong to the two teleost fish taxa (Acanthomorpha and Cypriniformes) having lost the stra8 gene, while stra8 has been identified in at least fourteen other teleost fish including Southern catfish (Ictalurus punctatus) and three salmonids [Oncorhynchus mykiss, Salmo trutta and Salvelinus alpinus (Pasquier et al., 2016)], but there is little information on stra8 functions in fish. In catfish, stra8 is expressed in germ cells of both sexes, and functional experiments showed that RA, as in mammals, activated stra8 expression and meiotic initiation in the ovary (Dong et al., 2013; Li et al., 2016). However, direct evidence regarding stra8 functions in teleosts is currently missing. We therefore wanted to explore stra8 functions in Atlantic salmon, a fish species which has retained a copy of the stra8 gene. We here posed the following questions: is stra8 required for meiosis/fertility in Atlantic salmon males, as in mammals? If stra8 is not required, is this gene relevant at all in salmon spermatogenesis, and is a major RA function in mammals, the up-regulation of Stra8 gene expression, detectable in fish?
To address these questions we examined testis of salmon stra8 crispants histologically, quantified germ cell proliferation and apoptosis as well as analyzed a repertoire of genes involved in spermatogenesis. Testicular stra8 expression was nearly completely lost in stra8 crispants, correlating well with the high mutation rate found in F0 fish. Male crispants entered puberty but displayed disorganized spermatogenic tubules with an elevated incidence of apoptosis, in particular among type B spermatogonia and spermatocytes, during the rapid testis growth phase. This clear phenotype disappeared during the further progress through maturation. Potentially related to the increase in the production of spermatogenic cysts observed in crispants, we observed that in both, crispants and wild-type (WT) controls, testis weight did not differ, many germ cells completed spermatogenesis and sperm was able to fertilize eggs.
Materials and Methods
Guide RNA Design and Synthesis
High scoring guide RNA (gRNA) target sequences were predicted using CRISPRscan (Moreno-Mateos et al., 2015). Templates for producing gRNAs were then made according to protocols published by Gagnon et al. (2014) with minor modifications. Two partially complementary oligos, one forward gRNA oligo that encompasses the T7 polymerase II promoter, a 20 bp sequence that targets DNA and a 15 bp constant region that primes into a common reverse primer that contains the gRNA scaffold, were annealed and the protruding ends were filled in with a T4 polymerase to produce a full double stranded template. Templates were purified using the QIAGEN PCR purification kit and used as input for an in vitro transcription reaction using T7 HiScribe high yield kit (NEB). In vitro transcribed gRNAs were then treated with the TURBO DNA-free kit (Thermo Scientific) to remove template DNA, and purified using the QIAGEN RNeasy kit with a protocol modified for purification of small RNAs. Two gRNAs were prepared targeting the 5′-end and 3′-end of stra8, respectively (oligonucleotide sequences are shown in Figure 2A). Cas9 mRNA was prepared as previously described (Edvardsen et al., 2014).
Microinjection
Microinjection of fertilized and glutathione-softened salmon eggs was performed as previously described (Edvardsen et al., 2014). Two gRNAs targeting the 5′- and 3′-ends of stra8 were co-injected with a gRNA targeting slc45a2, aiding the selection of mutated individuals due to the loss of pigmentation (Wargelius et al., 2016). Sibling fish was kept as a control group.
Animal Housing and Tissue Sampling
The fish used in this study were reared and sampled at Matre Aquaculture Research Station (Matredal, Norway). The eggs used in the experiment were fertilized in November 2015, and full or partial albinos (slc45a2 knockouts) as well as sibling controls were started under standard conditions. In August 2016, a PIT-tag was implanted to allow individual identification of the fish, and a fin-clip was taken for genetic sex determination (Eisbrenner et al., 2014) and for analysis of mutation frequency with MiSeq. Equal numbers of sibling controls and stra8 crispants were then mixed into a common garden.
To initiate early male maturation in postsmolt males, fish were exposed to a 6-week stimulatory period from January-March 2017 according to established protocols (Fjelldal et al., 2011). Four (May 9th) and 8 months (September 26th) after starting the maturation regime, samplings took place from mutant and WT groups. Gonad and blood samples were collected, and gonado-somatic indices (GSI) calculated from stra8 crispants and WT fish. From the sampled fish, gonad tissue was collected in RNAlater (Thermo Fisher Scientific) for RNA extraction. In September 26th, three out of ten mature stra8 crispants exhibited gonads containing white-colored areas – as observed for all WT and most crispant males – but also gray areas, reminiscent of the coloration of maturing testis tissue. Samples for the analysis of gene expression were only taken from the white-colored areas in order to investigate gene expression changes in comparable stages of spermatogenesis. Gonad tissue pieces for subsequent histological analyses, however, were collected from white- and gray-colored areas (in case of the three mature crispants). Tissue samples were fixed in 4% paraformaldehyde or 4% glutaraldehyde and embedded in paraffin or plastic (Technovit 7100, Kulzer), respectively, as previously described (Wargelius et al., 2016; Kleppe et al., 2017).
In vivo testicular expression of genes involved in spermatogonial differentiation (sall4 and dusp4) and RA testicular function (stra8 and rec8) was investigated in salmon males before and after starting pubertal development. For that purpose, testicular tissue was collected from immature and maturing postsmolt males (13–15 months old) exposed to maturation-inducing conditions (Fjelldal et al., 2011) for 16 days. In response to this regime, part of the fish had started pubertal development, as indicated by GSI levels above 0.1% and the presence of type B spermatogonia, respectively. Testis tissue was fixed in 4% glutaraldehyde overnight at 4°C and embedded in Technovit 7100 for histological evaluation or collected in RNAlater for RNA extraction. Moreover, sall4, dusp4, stra8 and rec8 mRNA levels were analyzed in testicular fragments collected from immature postsmolts and cultured in basal and RA-free conditions (see Testis tissue culture section).
This experiment was approved by the Norwegian Animal Research Authority (NARA, permit number 5741) and the use of these experimental animals was in accordance with the Norwegian Animal Welfare Act.
MiSeq Analysis
Genomic DNA from fin clips were purified using the QIAGEN DNA extraction protocol. DNA was used in a two-step barcoding PCR targeting the two CRISPR locusts in stra8 as described by Gagnon et al. (2014) An amplicon for each of the targeted regions was prepared. For the exon 3 region a forward primer: 5′-TCT TTC CCT ACA CGA CGC TCT TCC GAT CTG GGG CAG CAT CAA TTA GCT T-3′ and reverse primer: 5′-TGG AGT TCA GAC GTG TGC TCT TCC GAT CTT GTC ATT CTG AGC ACC GTG G-3′ was used. For the exon 6 region a forward primer: 5′-TCT TTC CCT ACA CGA CGC TCT TCC GAT CTC ACC AGA TAA CAG GTT CTT CTC TCT-3′ and reverse primer: 5′-TGG AGT TCA GAC GTG TGC TCT TCC GAT CTG TGT CTT TCA TTT CAC CAG GAA CA-3′ was used. Each sample was then given a unique 6-mer barcode (TruSeq primers, Illumina) by a nested PCR with primers targeting 5′- overhangs of the first PCR products. The barcoded stra8 PCR products were mixed in equimolar ratios and the final denatured sequencing library was prepared at a concentration of 8 pM and spiked with 5% denatured phiX and sequenced on the MiSeq using MiSeq Reagent Kit v3 (600 cycle format). FastQ sequences were analyzed with CRISPResso2 (Clement et al., 2019) using the CRISPRessoPooled command in a customized Snakemake workflow (Köster and Rahmann, 2012). In brief, reads were filtered by retaining all reads with a minimum average Phred33 quality cutoff score of 20 and all bases with a minimum Phred33 score less than 20 were displayed as N. Trimming of Illumina adapters were enabled with the trimmomatic flag. In order to reduce sequencing errors occurring at the end of reads, paired-end reads were merged with FLASh and the preprocessed reads were then aligned by a global alignment algorithm aware of nuclease cut sites. An overview of the frequency and type of indels is shown in Supplementary Data 1.
Gamete Quality
On September 26th of 2017, mature WT and stra8 crispant males were stripped and the resulting sperm was then used for in vitro fertilization of WT eggs. Sperm samples from a total of five WT and six crispant males (of which three showed testes with gray areas) were used to individually fertilize ∼500–1000 eggs (average of ∼ 801 eggs per crossing for both genotypes) obtained from a single WT female. During the embryo incubation period, dead embryos were subsequently registered and removed daily. For all eleven different crosses, embryo survival was recorded post hatch on January 10th of 2018, as well as the number of embryos showing deformities.
11-KT Quantification by ELISA
The levels of 11-ketotestosterone (11-KT) were analyzed by ELISA (Cuisset et al., 1994) on extracted plasma samples, as previously described (Andersson et al., 2013). Acetylcholine esterase-labeled tracers and microplates pre-coated with monoclonal mouse anti-rabbit IgG were supplied by Cayman Chemicals. Anti-11-KT was a kind gift from David E. Kime (Sheffield University, United Kingdom).
RNA Extraction and cDNA Preparation
A tissue piece of approximately 3 mm3 was homogenized in 400 μL of homogenization buffer and processed according to the Maxwell HT simplyRNA kit instructions (Promega) on a BioMek 4000 instrument (Beckton Dickinson). The quantity and purity of RNA samples were further assessed by spectrophotometry on a nanodrop ND-1000 instrument. cDNA was prepared by reverse transcription of 200 ng RNA using the SuperScript IV VILO Master Mix with ezDNase Enzyme according to the manufacturer’s recommendations (Thermo Fisher Scientific).
Quantitative PCR (qPCR)
The primers and conditions for running the vasa and ef1a qPCR assays have previously been described (Wargelius et al., 2016). Primers for the remaining targets were designed using NCBI primer blast1 and their respective sequences are listed fully in Supplementary Table 1. The forward primer for the stra8 gene was specifically designed to overlap the PAM site targeted by the exon-3 gRNA. A qPCR reaction was prepared according to the manufacturer descriptions to contain 800 nM of each forward and reverse primer in a 6 ul reaction containing a 1x concentration of the PowerUp SYBR Green master mix (Thermo Fisher Scientific). 2 μL of a 1/20 dilution of cDNA was added to the qPCR reaction and the reaction was subjected to thermocycling in a QuantStudio 5 Real-Time PCR system (Thermo Fisher Scientific) with an initial hold at 50°C for 2 min followed by an initial denaturation step at 95°C for 2 min. Thermocycling was conducted for 40 cycles using a denaturation step at 95°C for 1 s followed by a combined annealing and extension step at 60°C for 30 s. Data was processed at Thermo Fisher cloud using the relative quantification app.
Phylogenetics
Stra8 protein sequences for phylogenetic analysis were retrieved from UniProt2. Phylogenetic trees and multiple alignment of Stra8 from several species were produced using the ETE toolkit (Huerta-Cepas et al., 2016) with the default standard_fastree workflow utilizing ClustalOmega and FastTree. Blocks of local alignment are depicted as gray blocks in the multiple alignments. Support values (0–1) depicted in the proximity to branches are calculated by the standard_fastree workflow as the result of 1000 bootstrap resamples.
Apoptosis Detection by TUNEL
To determine the incidence of apoptosis, paraffin-embedded testis tissue was sectioned at 4 μm thickness and then subjected to deoxynucleotidyl transferase-mediated dUTP nick-end labeling (TUNEL). To this end, the sections were deparaffinized, rehydrated and then treated with permeabilization solution (20 μg/mL proteinase K in 10 mM Tris/HCl, pH 7.4) at 37°C for 30 min. Finally, slides were incubated with TUNEL reaction mixture (In Situ Cell Death Detection Kit, Fluorescein; Roche) in the dark at 37°C for 1 h. After washing twice in PBS, sections were counterstained with DAPI, mounted in Vectashield antifade mounting medium (Vector Laboratories) and imaged by confocal laser scanning microscopy (Zeiss LSM 700). Digital images were subjected to a quantification pipeline (see below). Negative and positive controls were included in each experimental set up (see Supplementary Figure 4B).
Proliferation Analysis by Phospho-Histone H3 (pH3) Immunostaining
In order to assess germ cell proliferation, paraffin-embedded testis tissue was sectioned at 4 μm thickness and then subjected to an immunocytochemical procedure to detect the endogenous proliferation marker phospho-histone H3 (pH3). Paraffin sections were deparaffinized and rehydrated according to standard protocols and subjected to pH3 immunohistochemistry using a polyclonal rabbit anti-human pH3 (Ser10) antibody (Upstate®; Sigma-Aldrich) as previously described (Almeida et al., 2008), except that the primary antibody was detected by undiluted HRP-conjugated goat anti-rabbit IgG (Brightvision Immunologic).
Image Quantification
Images from fluorescent stained sections (TUNEL) were quantified using a custom CellProfiler segmentation and quantification pipeline (Lamprecht et al., 2007). Images were first corrected for uneven illumination by the illumination correction function in CellProfiler. Thereafter a pixel classification was performed with Ilastik (Berg et al., 2019) to generate pixel probability maps for nuclei, background and TUNEL stained nuclei. The Watershed segmentation module in CellProfiler was then applied on the pixel probability maps. Objects identified by this module were then filtered by the filter and mask modules, before the final segmented, filtered and declumped objects were retained with the IdentifySecondaryObjects module. Five fields per individual and genotype were photographed at x20 magnification and the count of segmented nuclei and TUNEL labeled nuclei, as well as the percentage of TUNEL labeled nuclei, was then exported for analysis. Therefore, the total area investigated was the same for both WT and stra8 crispant groups.
A separate CellProfiler pipeline was constructed for the analysis of pH3 3,3′-diaminobenzidine (DAB) labeled images. The percentage of positive stained areas was calculated using the segmented positively stained areas obtained from the Otsu two class thresholding algorithm of the IdentifyPrimaryObjects module in CellProfiler. Five different fields of view were quantified for each section. To obtain the single cell proliferation data, five representative fields for each individual were photographed. The images were then analyzed and the total number of pH3 positive intratubular single cells (i.e., type A undifferentiated spermatogonia and Sertoli cells) quantified. The two cell types have characteristic size and shapes of their nucleus, that can be used to identify them (pH3 staining is restricted to the nucleus, so that the cells can be identified reliably). The nuclei of type A undifferentiated spermatogonia are round and show the largest diameter (∼12 μm) of all germ cell nuclei. Sertoli cell nuclei are triangular, kidney or banana shaped but not round and quite a bit smaller (∼2 μm for the short dimension). This approach has been used previously to quantify single cell proliferation, including studies in Atlantic salmon (Crespo et al., 2019b).
Testis Tissue Culture
A previously established primary testis tissue culture system developed for zebrafish (Leal et al., 2009a) was used, except that for salmon tissue incubations, the temperature was set to 14–15°C. Testis tissue was collected from immature males and incubated for 4 days in the absence or presence of 10 μM 4-diethylaminobenzaldehyde (DEAB, RA production inhibitor; Sigma-Aldrich) to investigate the effect of RA on gene expression in primary culture. At the end of the experiment, testis tissue was placed in RNAlater and stored at −80°C, until RNA extraction and qPCR analysis.
Statistical Analysis
GraphPad Prism 8 package (GraphPad Software, Inc.) was used for statistical analysis. Significant differences between groups were identified using Student’s t-test (paired or unpaired, as appropriate) (∗p < 0.05; ∗∗p < 0.01; ∗∗∗p < 0.001). Data are represented as mean ± SEM.
Results
Salmon stra8: Phylogenetic Analysis and Testicular Expression During the Onset of Puberty
In the current ICSASG_V2 salmon reference genome the stra8 gene is annotated as stimulated by retinoic acid gene 8 protein homolog. Within this reference there are four presumptive stra8 genes LOC106599152, LOC106598674, LOC106609832, and LOC106590959. LOC106599152, LOC106598674, and LOC106609832 encode partial transcripts where two of these (LOC106599152 and LOC106598674) are located on unplaced short contigs, whereas LOC106609832 is placed on chromosome 7. The fourth, LOC106590959, encodes a full length 1478 base pairs (bp) stra8 transcript, RefSeq accession number: XM_014182138.1. This transcript is located on an unplaced short contig and encodes a putative protein of 348 amino acids (XP_014037613.1), showing high sequence similarity to the stra8 found in rainbow trout (Oncorhynchus mykiss) and brown trout (Salmo trutta). Phylogenetic analysis of the salmon stra8 gene shows that it is clustered in the same branch as other salmonid species (Figure 1A).
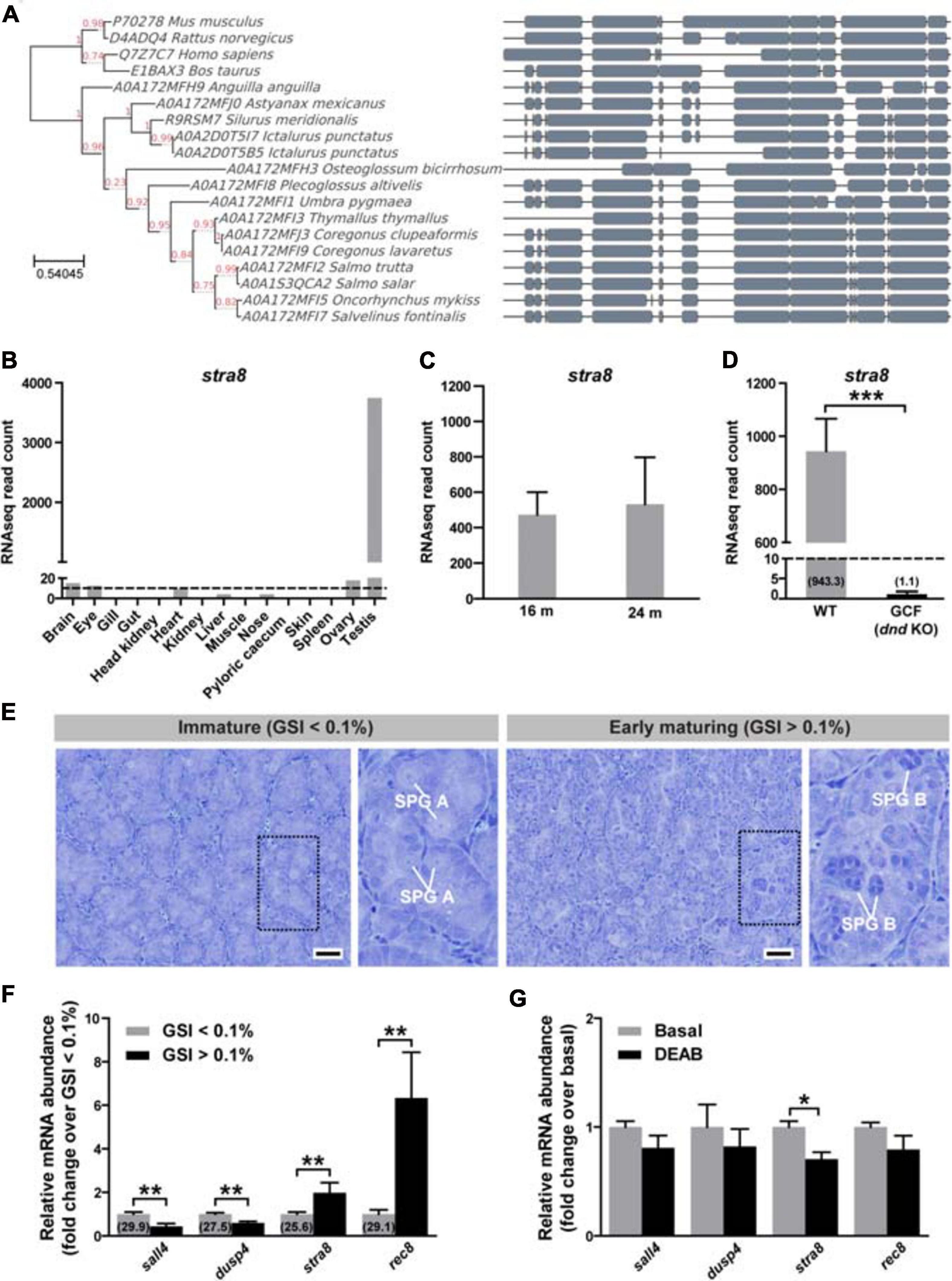
Figure 1. Phylogenetic tree of stra8 and potential involvement of retinoic acid (RA) in male Atlantic salmon at puberty. (A) Phylogenetic tree produced from a multiple alignment of STRA8. Blocks of local alignment are shown as gray boxes along the multiple alignment. Support values (0–1) generated from 1000 bootstrap resamples are shown next to the branches in red. UniProtKB accession numbers are displayed in the tree next to the species name. (B) stra8 expression levels in the different adult tissues used for making the reference annotation of the Atlantic salmon genome (GenBank GBRB00000000.1) (Lien et al., 2016). Results are expressed as RNAseq normalized read counts (N = 1). Dashed line indicates 10 RNAseq reads. (C) Testicular stra8 expression in immature salmon males before and after smoltification. Results are shown as mean RNAseq normalized read counts ± SEM (N = 3–8) retrieved from GenBank PRJNA380580 data set (Kjærner-Semb et al., 2018) (16 m males) and own unpublished data (24 m males). (D) Testicular stra8 expression in wild-type (WT) and germ cell-free (GCF) dead end (dnd) knockout salmon males, GenBank PRJNA550414 (Kleppe et al., 2020). Data are shown as mean RNAseq normalized read counts ± SEM (N = 3–4; ***p < 0.001). Numbers in brackets indicate average read counts for each group. Dashed line indicates 10 RNAseq reads. (E) Representative testis morphology of postsmolt males before (gonado-somatic index [GSI] < 0.1%) and after starting pubertal development (GSI > 0.1%). Insets show testis tissue magnified from the marked area (black dashed line), and representative type A (SPG A) and type B spermatogonia (SPG B) are labeled. Scale bar, 25 μm. (F) In vivo testicular expression of genes involved in spermatogonial differentiation and RA testicular function in postsmolt males before and after starting pubertal development. Numbers in brackets indicate Cq values obtained by qPCR analysis. Data are shown as mean fold change ± SEM (N = 8–9; **p < 0.01), and expressed relative to the control condition, which is set at 1. (G) Modulation of sall4, dusp4, stra8 and rec8 mRNA levels upon RA synthesis inhibition. Testicular fragments from immature males were cultured for 4 days at 14°C in the absence or presence of DEAB (10 μM). Data are shown as mean fold change ± SEM (N = 8–9; *p < 0.05), and expressed relative to the control condition, which is set at 1.
Analysis of stra8 transcript levels in deposited RNAseq data of the different adult tissues used in annotating the Atlantic salmon genome (GenBank GBRB00000000.1) (Lien et al., 2016) revealed high testicular expression (Figure 1B). Low read numbers were observed in the immature ovary, where most germ cells are primary previtellogenic oocytes arrested at the diplotene stage (data not shown). To obtain further information on stra8 transcript levels in testis tissue, we examined stra8 read numbers from three RNAseq data sets obtained from immature WT and germ cell-free mutant males (PRJNA380580 (Kjærner-Semb et al., 2018) and own unpublished data, 16 m and 24 m in Figure 1C, respectively; and PRJNA550414 (Kleppe et al., 2020), Figure 1D). Considerable expression was found in immature testis tissue from 16 or 24 months-old salmon (Figure 1C). No expression was found in testis tissue from germ cell-free dead end (dnd) knockout salmon confirming that the expression of stra8 is limited to germ cells (Figure 1D).
To investigate whether stra8 transcript levels change at the onset of pubertal development in male salmon, immature postsmolts (13–14 months old) were exposed to a maturation-inducing environmental regime (Fjelldal et al., 2011) for 16 days until the fish were sampled. In response to this regime, part of the fish had started pubertal development, as indicated by gonado-somatic index (GSI) levels above 0.1 and the presence of type B spermatogonia, respectively (Figure 1E). Elevated testicular transcript levels were recorded for stra8 and also for rec8, a gene important for meiotic recombination but not directly regulated by STRA8 in mammals (Kojima et al., 2019). A brief summary of stra8 and rec8 gene functions, and of the other genes mentioned below, is given in Table 1. Transcript levels of sall4 and dusp4, on the other hand, were halved in males, in which superallometric testis growth had started (GSI > 0.1%; Figure 1F). While stra8 levels had increased further in testis tissue that started to mature, it was remarkable that stra8 transcript levels were well above detection limit already in immature, prepubertal testis tissue, both before and after smoltification (16 m and 24 m, respectively; Figure 1C), that contained mainly type A undifferentiated spermatogonia (as shown in Figure 1E, left panel). Using immature testes in a primary tissue culture system, we found that adding the RA production inhibitor DEAB to the incubation medium down-regulated stra8 transcript levels (Figure 1G). indicating that stra8 expression also in salmon is stimulated by RA.
Genetic Ablation of stra8 in Salmon Transiently Disturbed Spermatogenesis
We generated stra8 crispants using CRISPR/Cas9. Guide RNAs (gRNAs) were designed to target exons 3 and 6 (Figure 2A). MiSeq analysis of fin clips of stra8 crispant fish, revealed that the gRNA targeting exon 3 induced mutations at the targeted locus at a high efficiency, whereas the second gRNA targeting exon 6 was non-functional. An overview of the frequency and type of indels can be found for each individual in Supplementary Data 1. qPCR of testis from the mutants showed a 4- or 12-log2 fold reduction of stra8 mRNA levels in the testis compared to WT siblings, in mature or maturing males during the rapid testicular growth phase (Figure 2B).
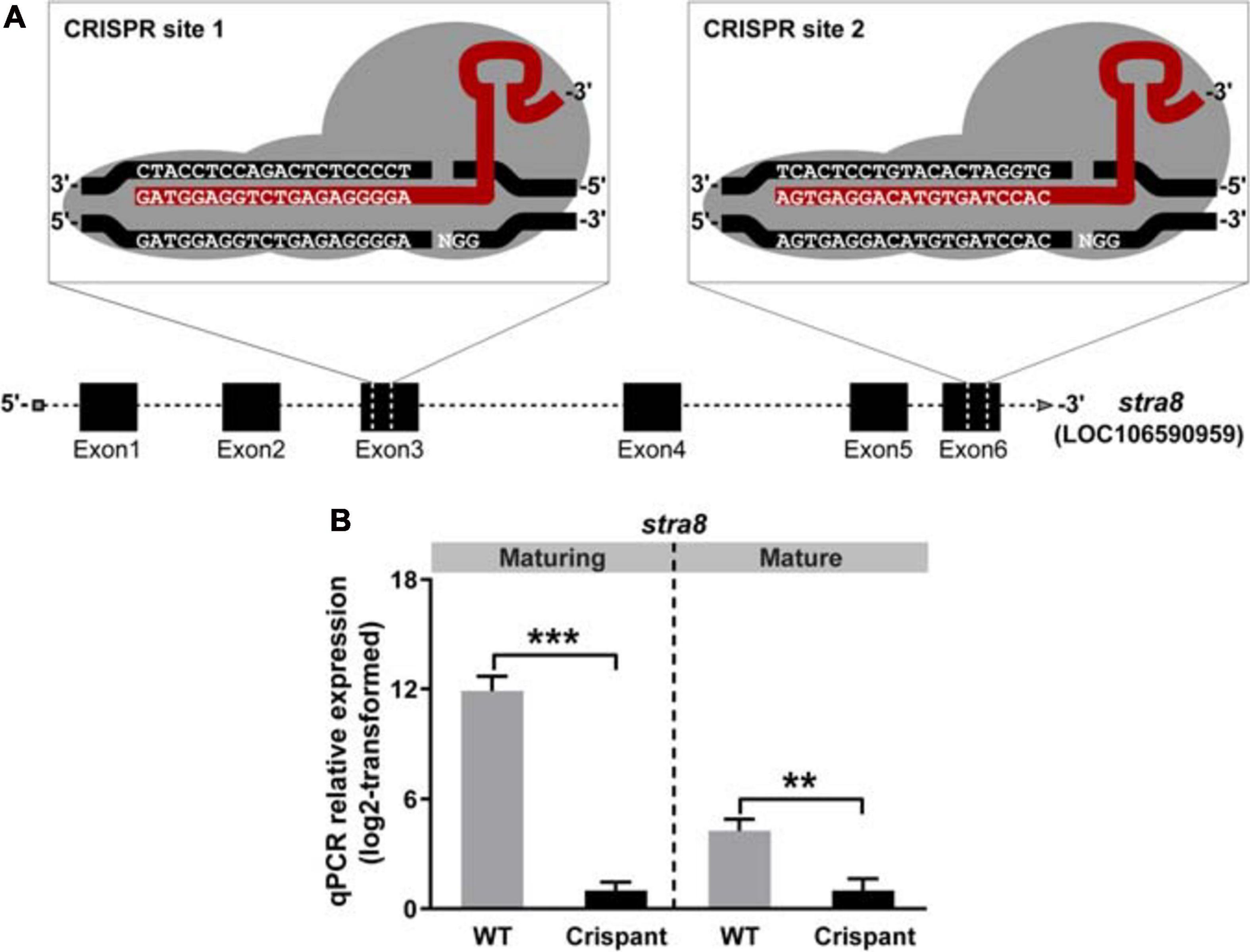
Figure 2. Efficient disruption of salmon stra8 by CRISPR/Cas9 and stra8 germ cell-specific expression. (A) Design of guide RNA target sites on exon 3 and exon 6 targeting the stra8 gene. (B) CRISPR/Cas9-mediated decrease of WT stra8 transcript levels in both maturing and mature Atlantic salmon crispant males. Data are shown as mean relative expression ± SEM (N = 7–10; **p < 0.01; ***p < 0.001). WT, wild-type.
The relative testis weight remained unaffected in maturing and mature stra8 crispants (GSI; Figure 3A). However, analyzing histological sections from maturing testes revealed clear effects on spermatogenesis in stra8 crispants, such as numerous apoptotic nuclei (white arrowheads in Figure 3B) and an irregular organization of spermatogenic cysts (Supplementary Figure 1A). Moreover, some stra8 crispant testes (e.g., #8 and #11; Supplementary Figure 1A) showed an accumulation of type B spermatogonia and few meiotic and postmeiotic cells, which differed from the appearance of testes sections from WT individuals. Neither plasma 11-KT (main androgen in fish) nor expression levels of steroidogenesis-related genes were affected in stra8 crispant males compared to WT siblings (Figure 3A and Supplementary Figure 2, respectively). Remarkably, 4 months later spermatogenesis had largely recovered in stra8 crispants. Many tubuli contained large numbers of spermatozoa. The spermatogenic cysts still present had progressed in development and mainly contained spermatocytes and spermatids, while the different generations of spermatogonia were still present but no longer in a prominent manner (Figure 3D). As observed in maturing males, GSI values and 11-KT plasma concentrations in mature stra8 crispants were similar to those in WT controls (Figure 3C). Despite the apparent spermatogenic recovery, some tubuli in mature stra8 crispant testes still contained several apoptotic cells (e.g., male #36 in Figure 3D), in particular in areas still comparatively rich in earlier stages of spermatogenesis with many type B spermatogonia and spermatocytes that macroscopically showed a gray coloration otherwise seen also in maturing testes (males #25, #30 and #32 in Supplementary Figures 3A,B), while the complete testis was white-colored and contained lumina with many spermatozoa in WT controls (Supplementary Figure 3B, male #29) and most – 7 out of 10 – stra8 crispants (Supplementary Figure 3B; e.g., male #28), but also in the white areas of the three stra8 crispant testes showing gray areas.
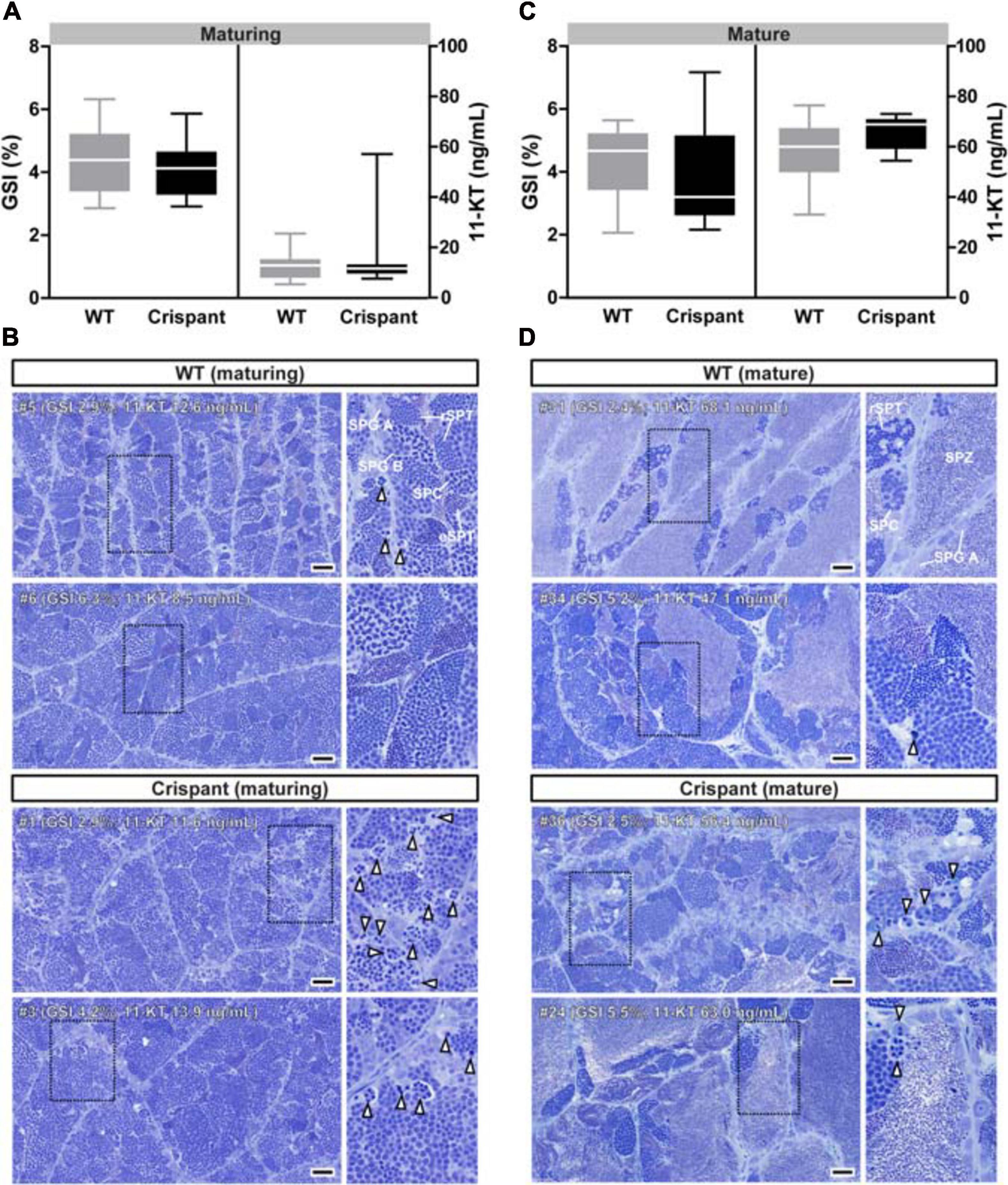
Figure 3. Evaluation of gonadal tissue and plasma androgen levels of WT and stra8 crispant salmon males. (A,C) Gonado-somatic indices (GSI) and plasma androgen (11-ketotestosterone; 11-KT) levels in WT and stra8 crispant males at samplings from May (maturing) and September 2017 (mature). (B,D) Histological images of maturing (B) and mature (D) Atlantic salmon testes obtained from WT and stra8 crispant fish. Insets show testis tissue magnified from the marked area (black dashed line). White arrowheads indicate apoptotic germ cells. Representative germ cell stages are shown in the upper right panels: type A spermatogonia (SPG A), type B spermatogonia (SPG B), spermatocytes (SPC), round spermatids (rSPT), elongated spermatids (eSPT) and spermatozoa (SPZ). WT, wild-type; Crispant, stra8 crispant. Scale bar, 50 μm.
Experiments on gamete quality (Supplementary Figure 3C) showed that sperm quality was indistinguishable for both genotypes, irrespectively of the gonad coloration of the crispants, considering that three of the six crispant sperm samples were collected from the males showing gray testis tissue parts. Fertilization capacity, embryo survival and percentage of embryos showing deformities, recorded at hatching stage, were all similar to the values observed in WT controls.
TUNEL analysis confirmed a sevenfold increased frequency of germ cell death by apoptosis in maturing, but not in mature, stra8 crispants (Figures 4A,B). In maturing mutants, DNA fragmentation was mainly observed in type B spermatogonia and spermatocytes, as identified by the shape and size of their DAPI-stained nuclei (Supplementary Figure 4A). Since GSI were not different despite elevated germ cell loss to apoptosis, we hypothesized that mutant testes may show a higher proliferation activity. While no differences were found in overall germ cell proliferation (Figures 4C,D left panel), single cell proliferation activity was significantly elevated in mutants (Figure 4C arrowheads and Figure 4D right panel). This increased activity involved single type Aund spermatogonia and associated Sertoli cells (white and black arrowheads, respectively, on Figure 4C and Supplementary Figure 4C).
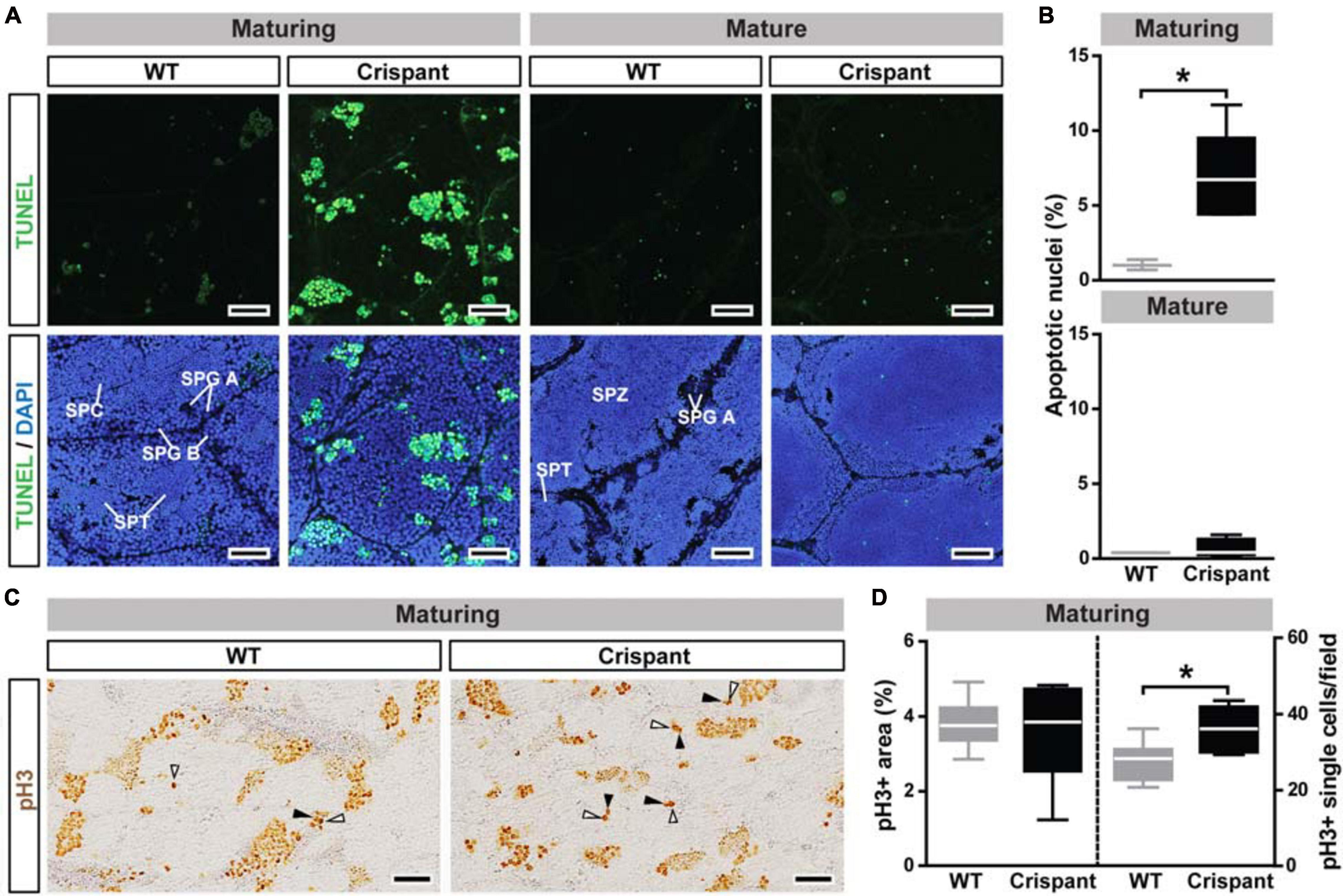
Figure 4. Germ cell apoptosis and proliferation in WT and stra8 crispant salmon testis tissue. (A,B) Detection (A) and quantification (B) of germ cell apoptosis/DNA damage by TUNEL analysis. TUNEL + cells/cysts are shown in green and DAPI counterstain in blue. Representative germ cell stages are labeled: type A spermatogonia (SPG A), type B spermatogonia (SPG B), spermatocytes (SPC), spermatids (SPT) and spermatozoa (SPZ). Scale bar, 30 μm. Quantification results are shown as mean ± SEM (N = 3–5; *p < 0.05). (C,D) Detection (C) and quantification (D) of cell proliferation by pH3 analysis. White and black arrowheads indicate pH3 + type A undifferentiated and Sertoli cells, respectively (examples of proliferating cells are shown at higher magnification in Supplementary Figure 4C). Scale bar, 50 μm. WT, wild-type; Crispant, stra8 crispant.
Analysis of Cell Stage Spermatogenic and Meiotic Markers in stra8 Crispants
To evaluate further potential effects in stra8 crispants on the three main phases of spermatogenesis (mitotic, meiotic, and spermiogenic phases), we quantified the expression levels of several genes expressed in germ cells, and of some genes expressed also or only in somatic cells (e.g., dmrt1 and egr1, respectively; see Table 1). Moreover, some of the genes analyzed (indicated by an asterisk in Table 1) are known direct downstream targets of STRA8 in mammals.
Considering the early generations of spermatogonia, several genes showed similar expression levels in maturing WT and stra8 crispants (e.g., lin28a, ret, nanos2, upp1, pou5f1; see Undifferentiated SPG in Figure 5 and Table 1), suggesting that the spermatogonial stem cells (SSCs) and progenitor generations among the type A spermatogonia were not drastically affected by the loss of stra8. This is in line with our morphological observations, since also in stra8 crispants the SSCs were able to self-renew and to produce differentiating spermatogonia, of which many completed the spermatogenic process. However, we did record significantly decreased transcript levels in maturing stra8 crispant testes of two genes (dusp4 and egr1; Figure 5), which encode proteins that in mammals are involved in the regulation of SSC proliferation and differentiation (Chan et al., 2017; Bhang et al., 2018). Considering transcripts expressed in differentiating spermatogonia, no significant differences were observed regarding dazl, piwil1 (Figure 5), or kit (Table 1), while three transcripts associated with differentiating spermatogonia and their mitotic expansion (ccnd2, dmrt1 and sall4) were significantly reduced in crispants (Figure 5). Also with respect to meiosis markers, we found genes that did (dmc1, prdm9, ccna1; Figure 5) or did not show (rec8, sycp3, spo11; Figure 5) significant expression changes in crispants. The only spermatid marker examined (sox30) showed reduced transcript levels in crispants, while no differences were found for the general germ cell marker vasa (Figure 5). The expression levels of growth factors known to regulate the differentiation and proliferation behavior of spermatogonia did not change in maturing stra8 crispants (Supplementary Figure 2). None of the genes investigated by qPCR in the present study showed significant changes in testis tissue of mature stra8 crispants (data not shown).
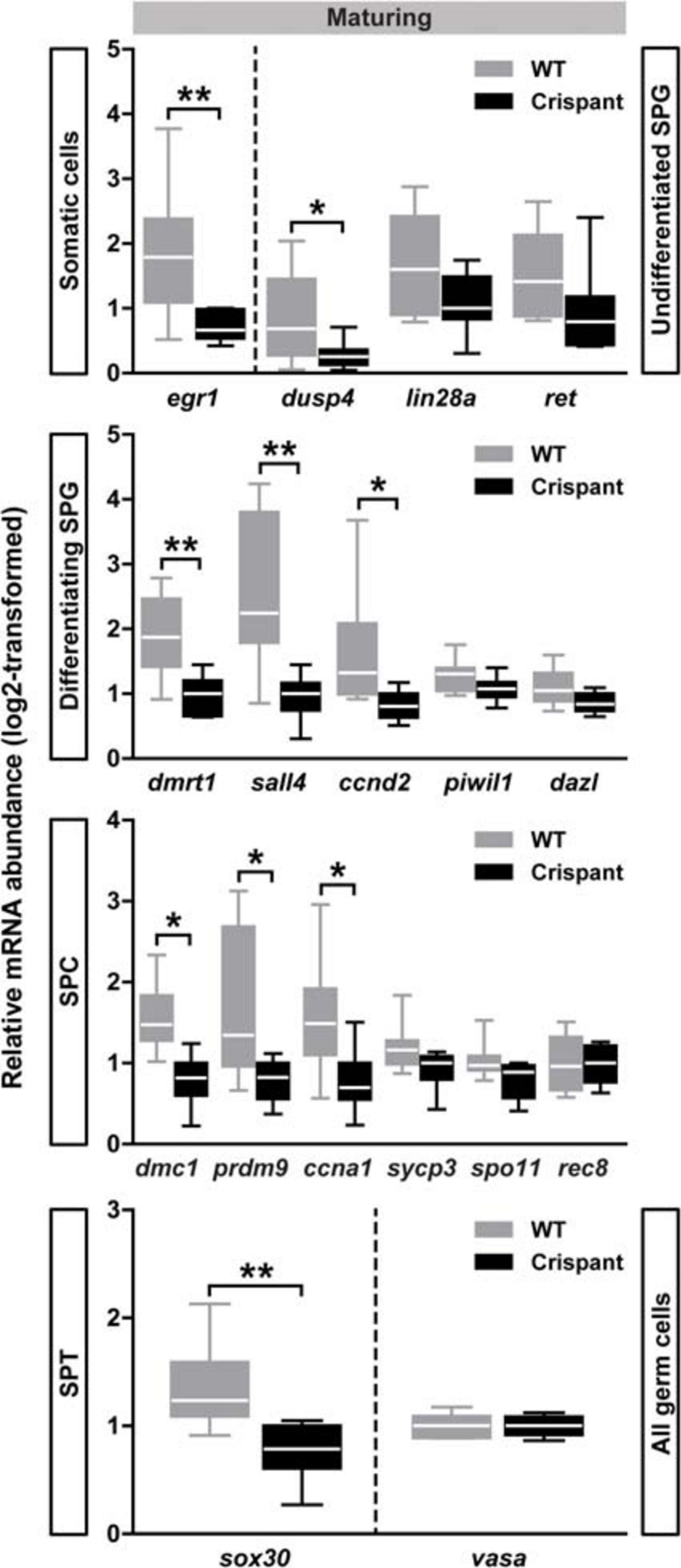
Figure 5. Testicular gene expression in maturing WT and stra8 crispant fish. Transcript levels of selected markers for germ cells at different stages of development are shown as mean ± SEM (N = 7–10; *p < 0.05, **p < 0.01), and expressed relative to ef1a expression. WT, wild-type; Crispant, stra8 crispant; SPG, spermatogonia; SPC, spermatocytes; SPT, spermatids.
Taken together, our observations show that the expression of stra8 was clearly detectable already in immature testes, confined to germ cells, up-regulated during the onset of puberty and decreased when blocking RA production. In maturing stra8 crispants, the expression of some genes important for the mitotic or meiotic phases of spermatogenesis was reduced, while several other transcripts remained unchanged. Reduced stra8 levels were associated with clear defects during spermatogenesis when the maturing salmon testis progressed through its rapid growth phase. However, these defects had been compensated when the males approached the fully mature stage of development.
Discussion
Phylogeny and Tissue Specificity of Salmon stra8
Our first aim was to identify stra8 in Atlantic salmon. While stra8 localized to a contig too short for synteny analysis, the phylogenetic analysis based on 14 fish species with an annotated stra8 gene (Figure 1A) indicated that the gene found in salmon was a stra8 ortholog. This annotation is supported by the germ cell-specific expression of this gene also in salmon (Kleppe et al., 2020) (Figure 1D), as previously reported in other fish species (Dong et al., 2013; Li et al., 2016; Pasquier et al., 2016). However, in contrast to the observation in Southern catfish (Dong et al., 2013), stra8 transcripts were barely detectable in immature salmon ovaries. It is possible that higher stra8 transcript levels can be recorded also in salmon when oogonia enter meiosis, but we did not collect samples covering the period that this (earlier) stage of female germ cell development is relatively prominent in the juvenile ovary. In any case, it seems that stra8 expression is not required to safeguard the existence of previtellogenic primary oocytes that have, as usual for oocytes until resumption of meiosis when approaching ovulation, arrested in diplonema.
stra8 in Salmon Testis
We found considerable amounts of stra8 transcripts in immature testes not containing differentiating spermatogonia or spermatocytes (Figures 1C,F,G). In contrast, Stra8 transcripts are not abundant in mice until they reach puberty, when RA strongly increased Stra8 levels in differentiating spermatogonia although low levels were already present in undifferentiated spermatogonia (Zhou et al., 2008). There is little information on the functional context of the expression of stra8 in immature salmon testis with spermatogenic tubules containing only undifferentiated spermatogonia and Sertoli cells. Early stra8 expression may prepare immature germ cells for an upcoming activation of spermatogonial differentiation/entry into meiosis, while the transcript may not be used yet, perhaps being idle while associated with RNA binding proteins. Unfortunately, we have no information on Stra8 protein levels in the testis of salmon or other fish species. Considering that stra8 transcript levels were reduced after exposing immature testis tissue to a compound preventing RA biosynthesis, or increased in testis entering puberty following photoperiod stimulation (Figures 1F,G), we assume that locally produced RA increased stra8 expression in the immature salmon testis to support the transition to differentiating spermatogonia (Zhou et al., 2008). Respective data is not available for testis tissue of other fish species. However, in Southern catfish ovaries, experimentally reduced or increased RA levels, also reduced or increased, respectively, the expression of stra8 (Li et al., 2016). These observations suggest that fish share RA-mediated regulation of stra8 gene expression with higher vertebrates. In fish lacking the stra8 gene, modulating gonadal RA levels still was relevant for spermatogenesis (Feng et al., 2015; Crespo et al., 2019a), demonstrating the activity of stra8-independent, RA-modulated pathways to promote the mitotic phase of spermatogenesis.
Maturing Testis of stra8 Crispants Are Characterized by Increased Apoptosis and Single Cell Proliferation
Loss of Stra8 in mice leads to sterility with an arrest of male germ cell differentiation at the spermatogonial stage and a hypoplastic testis (Anderson et al., 2008). In contrast to this finding in mice, testis weight or GSI did not differ in WT and stra8 crispant salmon, which also produced meiotic and postmeiotic germ cells. However, during the rapid growth phase of the maturing testis characterized by containing many type B spermatogonia, spermatocytes and spermatids, stra8 crispants displayed disorganized spermatogenic tubuli with a clearly higher incidence of germ cell apoptosis in comparison to WT controls, which mainly occurred in type B spermatogonia and spermatocytes. It is therefore conceivable that one of the functions of salmon Stra8 is to reduce germ cell apoptosis. Germ cell apoptosis is a common phenomenon in normal vertebrate spermatogenesis (Shaha et al., 2010). In zebrafish, for example, ∼40% of the germ cells are lost before they reach the stage of mature spermatozoa in WT males, and interestingly, the losses mainly occurred among type B spermatogonia and spermatocytes (Leal et al., 2009b). Also in Atlantic salmon, ongoing work in our group indicates that the main cell loss in WT testes occurs among type B spermatogonia and spermatocytes (unpublished results). While apoptotic germ cell loss is normal, the degree to which it occurred in maturing stra8 crispants was remarkably higher than in maturing WT controls. We have no information on the molecular mechanism(s) used by Stra8 to prevent germ cell apoptosis in salmon. However, recent work in mice showed that STRA8 inhibited germ cell caspases via the AKT pathway to exert an anti-apoptotic effect (Shen et al., 2018). In case in salmon a similar mechanism should be active, it may explain the higher apoptotic index observed in crispants, which mainly affected the germ cell stages also more susceptible to apoptosis in WT males.
Normal GSI values in maturing testes suggest that the apoptosis-related loss of germ cells somehow was compensated, for example by changes in proliferation activity. While we did not find differences in the overall testicular proliferation activity, we detected an increase in the single cell proliferation activity in stra8 crispants, involving type Aund spermatogonia and associated Sertoli cells. In the cystic type of spermatogenesis typically seen in fish, Sertoli cells envelope and accompany a single germ cell clone during spermatogenesis (Schulz et al., 2010), thus forming the functional unit of fish spermatogenesis, known as spermatogenic cyst. Since new cysts are formed when a type Aund spermatogonium derived from a self-renewal division associated with a Sertoli cell, we assume that the elevated level of single cell proliferation of specifically these two cell types increased the production of spermatogenic cysts in maturing stra8 crispants. Producing more cysts may allow the crispants to reach normal GSI despite losing many more germ cells to apoptosis than WT controls. In salmon, there is no information on possible drivers of Sertoli cell and type Aund proliferation. However, in both zebrafish (Nóbrega et al., 2015; Safian et al., 2018) and mice (reviewed by Oduwole et al., 2018), Fsh-mediated effects are very important for the proliferation of these cells. It will be interesting to investigate in future studies if, for example, stra8-deficient testis responds differently to Fsh than WT testis. It is not clear how the increased apoptotic loss of germ cells is perceived and then communicated to the (as yet also unknown) regulatory system controlling cyst production in stra8 crispants. However, once the signaling in the newly formed cyst changes to induce differentiation, resulting in a series of mitotic divisions that increases germ cell number geometrically, elevated cyst production seems a feasible route to compensate the apoptotic germ cell loss.
Expressional Changes Associated With the Mitotic Phase
As discussed above, the response of the immature testis to the loss of stra8 may be linked to an expansion of the stem cell reservoir, eventually resulting in additional spermatogenic cysts. Proteins encoded by gsdf, igf3, insl3 or amh stimulate or inhibit, respectively, spermatogonial proliferation (Sawatari et al., 2007; Assis et al., 2016; Morais et al., 2017). However, none of them changed (Supplementary Figure 2). Considering that three of these growth factors respond to endocrine regulation by Fsh and/or androgens (Sambroni et al., 2013; Melo et al., 2015; Nóbrega et al., 2015; Crespo et al., 2016), and that circulating androgen levels in stra8 crispants were similar to those in WT controls (Figures 3A,C), it seems unlikely that mutant males differed largely from controls regarding the gonadotropin and/or androgen mediated regulation of spermatogenesis. However, local testicular signaling systems may well have responded to the loss of stra8. In this regard, the decreased dusp4 transcript levels may be relevant, considering that in mice, the protein phosphatase DUSP4 inhibits JNK-mediated stimulation of SSC self-renewal (Chan et al., 2017). Assuming that a similar mechanism is active in the salmon testis, decreased dusp4 transcript levels may contribute to the observed increase in proliferation of single type Aund spermatogonia that feeds into the production of additional spermatogenic cysts. However, other transcripts associated with SSC or progenitor spermatogonia remained unaffected in stra8 crispants (e.g., ret, lin28a, pou5f and upp1). Taken together, there is little molecular and no morphological evidence for the notion that SSCs or progenitor spermatogonia functions are compromised in stra8 crispants. In contrast, our observations rather indicate an elevated activity, leading to an increased production of spermatogenic cysts.
In transcripts encoding proteins exerting functions in either differentiating spermatogonia (ccnd2, sall4, dmrt1, piwil1 and dazl) or in Sertoli cells (dmrt1 and egr1), decreased transcript levels were observed for ccnd2, sall4, dmrt1 and egr1, while piwil1 and dazl remained unchanged in maturing stra8 crispants. These observations suggest that the transition from undifferentiated to differentiating spermatogonia was partially compromised in stra8 crispants. Studies in mice linked CCND2 to spermatogonial differentiation (Beumer et al., 2000). Also in mice, Sall4 gene expression is, as Stra8, directly induced by RA, upon which SALL4 sequesters the transcriptional inhibitor ZBTB16 (a.k.a. PLZF), eventually promoting spermatogonial differentiation (Gely-Pernot et al., 2015). Lower levels of the two germ cell-specific transcripts sall4 and ccnd2 in stra8 crispants therefore suggest that the transition from undifferentiated to differentiating spermatogonia was partially compromised. The transcription factor dmrt1 is expressed in both Sertoli cells and spermatogonia in mice and zebrafish and germ cell-specific deletion of Dmrt1 in mice caused spermatogonia to precociously exit mitotic cell cycling and enter meiosis (Matson et al., 2010). In zebrafish, the generalized loss of dmrt1 also resulted in a hypoplastic testis, but in contrast to mice, the small number of spermatogonia are also unable to enter meiosis and eventually, testis tissue becomes devoid of germ cells (Webster et al., 2017), suggesting that spermatogonial stem cell maintenance was dysregulated as well. The more severe phenotype in zebrafish may reflect the loss of dmrt1 function also from Sertoli cells, since the combination of inability to enter meiosis and loss of stem cells was also described in mice with a generalized loss of Dmrt1 (Raymond et al., 2000). Interestingly the phenotype also included overproliferation of Sertoli cells, reminiscent of what we see in stra8 crispants. Possibly, Stra8 can stimulate Sertoli cell proliferation and cyst formation through a yet unknown pathway that may involve germ-to-Sertoli cell signaling, since stra8 crispants also showed reduced levels of egr1, encoding a transcription factor that stimulates Dmrt1 expression in murine Sertoli cells (Lei and Heckert, 2002). There is no experimental evidence for germ-to-soma signaling in the salmon testis so far, but in mice germ cells use Notch ligands to stimulate Sertoli cell Notch receptors (Garcia et al., 2017). Overall, more work is required in salmon to clarify the mechanisms connecting stra8 to sall4, dmrt1 and egr1, considering that in mice, SALL4 functions in parallel to and independent of STRA8 while DMRT1 is up-stream of STRA8 in germ cells (Matson et al., 2010; Gely-Pernot et al., 2015; Zhang and Zarkower, 2017). The fact that piwil1 was not modulated in crispants may reflect the constitutive functions of Piwi proteins for germ cell genome stability (Thomson and Lin, 2009). Also dazl transcript levels were stable. This gene encodes an RNA binding protein that promotes the more robust translation of a large fraction (∼30%) of transcripts in spermatogonia, thereby facilitating the differentiating proliferation of progenitor spermatogonia in mice (Zagore et al., 2018; Mikedis et al., 2020). Our observations on piwil1 and dazl thus are in line with the notion that their stable expression contributes to the requirements for germ cell survival and their developmental transition into differentiating spermatogonia that is still taking place in stra8 crispants, although partially compromised.
Expressional Changes Associated With Meiosis and Spermiogenesis
In mice, STRA8-deficient spermatocytes initiate, but fail to complete meiosis, so that mutant germ cells still express genes needed for the initiation of meiosis, such as Sycp3, Spo11 and Rec8 (Mark et al., 2008). Likewise, we observed no differences in the expression of these genes between control males and stra8 crispants during the rapid growth phase of the maturing testis (Figure 5), while other meiosis-associated factors including ccna1, prdm9 and dmc1 were reduced in mutants. In mice, loss of Ccna1 disrupts spermatogenesis by arresting the first meiotic cell cycle in the late diplotene stage, resulting in the loss of these spermatocytes to apoptosis (Wolgemuth, 2011). It is unknown if stra8 controls ccna1 expression in salmon, but the increased apoptosis among spermatocytes in stra8 crispants may indicate that reduced ccna1 and elevated apoptosis are linked in the salmon testis as well. PRDM9 is a zinc finger containing histone H3K4 trimethylase that is expressed in early meiosis and Prdm9-deficient mice are sterile (Parvanov et al., 2010). STRA8 directly modulated Prdm9 gene expression in mice (Ma et al., 2018; Kojima et al., 2019). PRDM9 interacts with SPO11, initiating DNA double-strand breaks (DSBs) that are repaired by the recombinases DMC1 and RAD51 in the meiosis crossover process (Parvanov et al., 2010; Grey et al., 2018; Paiano et al., 2020). A shortage of either Prdm9 or Dmc1 protein in salmon stra8 crispants may have resulted in DSB repair problems and hence elevated apoptosis among spermatocytes. Based on a stra8 mouse mutant data (Koubova et al., 2014; Kojima et al., 2019), finding reduced dmc1 transcript levels in stra8 crispant salmon was not surprising. Finally, Sox30 in mice is expressed at the end of meiosis and during spermiogenesis (Bai et al., 2018). The reduced sox30 transcript levels in stra8 crispants may reflect a smaller number of spermatids resulting in a cumulative manner from the several effects discussed above during the mitotic and meiotic phases. Overall, our data suggests that also in the Atlantic salmon, stra8 supported the completion of meiosis, but different from mammals, is not required for meiosis.
Phenotype and Compensation in the Mature stra8 Crispant Testis
Eventually, stra8 crispant testes reached the mature stage and produced functional sperm (Figure 3D and Supplementary Figure 3), which could fertilize eggs at a similar rate as control sperm (Supplementary Figure 3C). These results further indicate that alternative pathways to complete meiosis exist also in fish species that do have a stra8 gene, pathways possibly similar to those used in, for example, zebrafish, medaka and tilapia (Feng et al., 2015; Adolfi et al., 2016; Crespo et al., 2019a), which miss a stra8 gene in their genome. Nevertheless, the removal of stra8 did have noticeable consequences, such as increased apoptotic loss of germ cells and increased single germ and Sertoli cell proliferation. The latter probably reflects the production of additional spermatogenic cysts, which can be understood as compensatory response to level out the increased loss of germ cells to apoptosis. This compensation took place during the growth phase of the maturing testis. When GSI values reach their maximum during the annual reproductive cycle of Atlantic salmon, this indicates that the production of new spermatogenic cysts stops while existing cysts continue to differentiate so that the testis becomes filled with mature sperm; single undifferentiated spermatogonia are the only other germ cell type present and remain quiescent until they resume activity at the beginning of the next annual cycle (Schulz et al., 2010). The mature status of both WT controls and stra8 crispants is indicated by the large number of mature sperm and androgen plasma levels of 60–80 ng/mL (Figure 3). Therefore, it was not surprising that neither cyst production nor apoptosis were different when comparing the two mature groups. After all, the processes were largely completed and the cell types involved had reached postmeiotic stages of development, or were lost to apoptosis, and hence no longer present in the mature testis. Also, while RA deficiency completely blocks spermatogonial differentiation in mammals, blocking RA production reduced, but did not block, sperm production/fertility in zebrafish (Pradhan and Olsson, 2015). These observations suggest that also stra8-independent but RA-regulated processes are not strictly required for spermatogenesis in fish. It appears that in teleost fish, signaling pathways regulating spermatogenesis operate in parallel, such that failure of a single pathway can be compensated at least in part in many cases. In mammals, on the other hand, some of these pathways apparently are organized in a sequential manner, such that when one of the elements operating in sequence fails, the spermatogenic process is blocked at this then crucial bottleneck with no option for alternative routes. In this context it is relevant to consider that (in context with the long generation time in salmon) we carried out our work with F0 crispants. The mosaicism of the F0 crispants and hence the presence of low amounts of WT in frame mutations may have provided, if any, only minor rescue effects in the crispants, considering the less than 1% WT sequence found in any of the crispants (see Supplementary Data 1).
Data Availability Statement
The datasets presented in this study can be found in online repositories. The names of the repository/repositories and accession number(s) can be found below: https://www.ncbi.nlm.nih.gov/, PRJNA380580; https://www.ncbi.nlm.nih.gov/, PRJNA550414; and https://www.ncbi.nlm.nih.gov/genbank/, GBRB00000000.1.
Ethics Statement
The animal study was reviewed and approved by the Norwegian Animal Research Authority (NARA, permit number 5741) and the use of these experimental animals was in accordance with the Norwegian Animal Welfare Act.
Author Contributions
KOS, DC, LK, EA, RBE, BN, and AW performed the experiments. KOS, DC, AW, and RWS analyzed and contributed to the interpretation of the results. AW, RWS, TJH, PGF, and RBE conceived the project, secured funding, and provided the supervision. KOS, DC, RWS, and AW wrote the manuscript. All authors contributed to the article and approved the submitted version.
Funding
This study was funded by the Research Council of Norway projects SALMOSTERILE (221648) and STERWELL (267610).
Conflict of Interest
The authors declare that the research was conducted in the absence of any commercial or financial relationships that could be construed as a potential conflict of interest.
Acknowledgments
We would like to thank Lise Dyrhovden and Ivar Helge Matre for rearing and handling all fish, and Anne Torsvik for expert technical assistance.
Supplementary Material
The Supplementary Material for this article can be found online at: https://www.frontiersin.org/articles/10.3389/fcell.2021.657192/full#supplementary-material
Footnotes
References
Adolfi, M. C., Herpin, A., Regensburger, M., Sacquegno, J., Waxman, J. S., and Schartl, M. (2016). Retinoic acid and meiosis induction in adult versus embryonic gonads of medaka. Sci. Rep. 6:34281. doi: 10.1038/srep34281
Almeida, F. F. L., Kristoffersen, C., Taranger, G. L., and Schulz, R. W. (2008). Spermatogenesis in Atlantic Cod (Gadus morhua): a novel model of cystic germ cell development. Biol. Reprod. 78, 27–34. doi: 10.1095/biolreprod.107.063669
Anderson, E. L., Baltus, A. E., Roepers-Gajadien, H. L., Hassold, T. J., de Rooij, D. G., van Pelt, A. M. M., et al. (2008). Stra8 and its inducer, retinoic acid, regulate meiotic initiation in both spermatogenesis and oogenesis in mice. Proc. Natl. Acad. Sci. U.S.A. 105, 14976–14980. doi: 10.1073/pnas.0807297105
Andersson, E., Schulz, R. W., Male, R., Bogerd, J., Patiña, D., Benedet, S., et al. (2013). Pituitary gonadotropin and ovarian gonadotropin receptor transcript levels: seasonal and photoperiod-induced changes in the reproductive physiology of female Atlantic salmon (Salmo salar). Gen. Comp. Endocrinol. 191, 247–258. doi: 10.1016/j.ygcen.2013.07.001
Assis, L. H. C., Crespo, D., Morais, R. D. V. S., França, L. R., Bogerd, J., and Schulz, R. W. (2016). INSL3 stimulates spermatogonial differentiation in testis of adult zebrafish (Danio rerio). Cell Tissue Res. 363, 579–588. doi: 10.1007/s00441-015-2213-9
Bai, S., Fu, K., Yin, H., Cui, Y., Yue, Q., Li, W., et al. (2018). Sox30 initiates transcription of haploid genes during late meiosis and spermiogenesis in mouse testes. Dev. Camb. Engl. 145:dev164855. doi: 10.1242/dev.164855
Baltus, A. E., Menke, D. B., Hu, Y.-C., Goodheart, M. L., Carpenter, A. E., de Rooij, D. G., et al. (2006). In germ cells of mouse embryonic ovaries, the decision to enter meiosis precedes premeiotic DNA replication. Nat. Genet. 38, 1430–1434. doi: 10.1038/ng1919
Beer, R. L., and Draper, B. W. (2013). nanos3 maintains germline stem cells and expression of the conserved germline stem cell gene nanos2 in the zebrafish ovary. Dev. Biol. 374, 308–318. doi: 10.1016/j.ydbio.2012.12.003
Bellaiche, J., Lareyre, J.-J., Cauty, C., Yano, A., Allemand, I., and Gac, F. L. (2014). Spermatogonial stem cell quest: nanos2, marker of a subpopulation of undifferentiated a spermatogonia in trout testis1. Biol. Reprod. 90:79. doi: 10.1095/biolreprod.113.116392
Berg, S., Kutra, D., Kroeger, T., Straehle, C. N., Kausler, B. X., Haubold, C., et al. (2019). ilastik: interactive machine learning for (bio)image analysis. Nat. Methods 16, 1226–1232. doi: 10.1038/s41592-019-0582-9
Beumer, T. L., Roepers-Gajadien, H. L., Gademan, I. S., Kal, H. B., and de Rooij, D. G. (2000). Involvement of the D-type cyclins in germ cell proliferation and differentiation in the mouse. Biol. Reprod. 63, 1893–1898. doi: 10.1095/biolreprod63.6.1893
Bhang, D. H., Kim, B.-J., Kim, B. G., Schadler, K., Baek, K.-H., Kim, Y. H., et al. (2018). Testicular endothelial cells are a critical population in the germline stem cell niche. Nat. Commun. 9:4379. doi: 10.1038/s41467-018-06881-z
Chakraborty, P., Buaas, F. W., Sharma, M., Snyder, E., de Rooij, D. G., and Braun, R. E. (2014). LIN28A marks the spermatogonial progenitor population and regulates its cyclic expansion. Stem Cells Dayt. Ohio 32, 860–873. doi: 10.1002/stem.1584
Chan, A.-L., La, H. M., Legrand, J. M. D., Mäkelä, J.-A., Eichenlaub, M., De Seram, M., et al. (2017). Germline stem cell activity is sustained by SALL4-dependent silencing of distinct tumor suppressor genes. Stem Cell Rep. 9, 956–971. doi: 10.1016/j.stemcr.2017.08.001
Clement, K., Rees, H., Canver, M. C., Gehrke, J. M., Farouni, R., Hsu, J. Y., et al. (2019). CRISPResso2 provides accurate and rapid genome editing sequence analysis. Nat. Biotechnol. 37, 224–226. doi: 10.1038/s41587-019-0032-3
Crespo, D., Assis, L. H. C., Furmanek, T., Bogerd, J., and Schulz, R. W. (2016). Expression profiling identifies Sertoli and Leydig cell genes as Fsh targets in adult zebrafish testis. Mol. Cell. Endocrinol. 437, 237–251. doi: 10.1016/j.mce.2016.08.033
Crespo, D., Assis, L. H. C., Kant, H. J. G., van de Waard, S., de Safian, D., Lemos, M. S., et al. (2019a). Endocrine and local signaling interact to regulate spermatogenesis in zebrafish: follicle-stimulating hormone, retinoic acid and androgens. Development 146:dev178665. doi: 10.1242/dev.178665
Crespo, D., Bogerd, J., Sambroni, E., LeGac, F., Andersson, E., Edvardsen, R. B., et al. (2019b). The initiation of puberty in Atlantic salmon brings about large changes in testicular gene expression that are modulated by the energy status. BMC Genomics 20:475. doi: 10.1186/s12864-019-5869-9
Cuisset, B., Pradelles, P., Kime, D. E., Kühn, E. R., Babin, P., Davail, S., et al. (1994). Enzyme immunoassay for 11-ketotestosterone using acetylcholinesterase as laberl: application to the measurement of 11-ketotestosterone in plasma of Siberian sturgeon. Comp. Biochem. Physiol. C Pharmacol. Toxicol. Endocrinol. 108, 229–241. doi: 10.1016/1367-8280(94)90035-3
Dong, R., Yang, S., Jiao, J., Wang, T., Shi, H., Zhou, L., et al. (2013). Characterization of Stra8 in Southern catfish (Silurus meridionalis): evidence for its role in meiotic initiation. BMC Mol. Biol. 14:11. doi: 10.1186/1471-2199-14-11
Edvardsen, R. B., Leininger, S., Kleppe, L., Skaftnesmo, K. O., and Wargelius, A. (2014). Targeted mutagenesis in Atlantic salmon (Salmo salar L.) using the CRISPR/Cas9 system induces complete knockout individuals in the F0 generation. PLoS One 9:e108622. doi: 10.1371/journal.pone.0108622
Eisbrenner, W. D., Botwright, N., Cook, M., Davidson, E. A., Dominik, S., Elliott, N. G., et al. (2014). Evidence for multiple sex-determining loci in tasmanian atlantic salmon (Salmo salar). Heredity 113, 86–92. doi: 10.1038/hdy.2013.55
Endo, T., Romer, K. A., Anderson, E. L., Baltus, A. E., Rooij, D. G., and de, et al. (2015). Periodic retinoic acid–STRA8 signaling intersects with periodic germ-cell competencies to regulate spermatogenesis. Proc. Natl. Acad. Sci. U.S.A. 112, E2347–E2356. doi: 10.1073/pnas.1505683112
Feng, C.-W. A., Spiller, C., Merriner, D. J., O’Bryan, M. K., Bowles, J., and Koopman, P. (2017). SOX30 is required for male fertility in mice. Sci. Rep. 7:17619. doi: 10.1038/s41598-017-17854-5
Feng, R., Fang, L., Cheng, Y., He, X., Jiang, W., Dong, R., et al. (2015). Retinoic acid homeostasis through aldh1a2 and cyp26a1 mediates meiotic entry in Nile tilapia (Oreochromis niloticus). Sci. Rep. 5:10131. doi: 10.1038/srep10131
Fjelldal, P. G., Hansen, T., and Huang, T. (2011). Continuous light and elevated temperature can trigger maturation both during and immediately after smoltification in male Atlantic salmon (Salmo salar). Aquaculture 321, 93–100. doi: 10.1016/j.aquaculture.2011.08.017
Gagnon, J. A., Valen, E., Thyme, S. B., Huang, P., Akhmetova, L., Ahkmetova, L., et al. (2014). Efficient mutagenesis by Cas9 protein-mediated oligonucleotide insertion and large-scale assessment of single-guide RNAs. PLoS One 9:e98186. doi: 10.1371/journal.pone.0098186
Garcia, T. X., Parekh, P., Gandhi, P., Sinha, K., and Hofmann, M. C. (2017). The NOTCH ligand JAG1 regulates GDNF expression in sertoli cells. Stem Cells Dev. 26, 585–598. doi: 10.1089/scd.2016.0318
Gely-Pernot, A., Raverdeau, M., Teletin, M., Vernet, N., Féret, B., Klopfenstein, M., et al. (2015). Retinoic acid receptors control spermatogonia cell-fate and induce expression of the SALL4A transcription factor. PLoS Genet. 11:e1005501. doi: 10.1371/journal.pgen.1005501
Green, C. D., Ma, Q., Manske, G. L., Shami, A. N., Zheng, X., Marini, S., et al. (2018). A comprehensive roadmap of murine spermatogenesis defined by single-cell RNA-Seq. Dev. Cell 46, 651–667.e10. doi: 10.1016/j.devcel.2018.07.025
Grey, C., Baudat, F., and de Massy, B. (2018). PRDM9, a driver of the genetic map. PLoS Genet. 14:e1007479. doi: 10.1371/journal.pgen.1007479
Grive, K. J., Hu, Y., Shu, E., Grimson, A., Elemento, O., Grenier, J. K., et al. (2019). Dynamic transcriptome profiles within spermatogonial and spermatocyte populations during postnatal testis maturation revealed by single-cell sequencing. PLoS Genet. 15:e1007810. doi: 10.1371/journal.pgen.1007810
Houwing, S., Kamminga, L. M., Berezikov, E., Cronembold, D., Girard, A., van den Elst, H., et al. (2007). A role for Piwi and piRNAs in germ cell maintenance and transposon silencing in Zebrafish. Cell 129, 69–82. doi: 10.1016/j.cell.2007.03.026
Huerta-Cepas, J., Serra, F., and Bork, P. (2016). ETE 3: reconstruction, analysis, and visualization of phylogenomic data. Mol. Biol. Evol. 33, 1635–1638. doi: 10.1093/molbev/msw046
Keeney, S. (2008). Spo11 and the formation of DNA double-strand breaks in meiosis. Genome Dyn. Stab. 2, 81–123. doi: 10.1007/7050_2007_026
Kjærner-Semb, E., Ayllon, F., Kleppe, L., Sørhus, E., Skaftnesmo, K., Furmanek, T., et al. (2018). Vgll3 and the Hippo pathway are regulated in Sertoli cells upon entry and during puberty in Atlantic salmon testis. Sci. Rep. 8:1912. doi: 10.1038/s41598-018-20308-1
Kleppe, L., Andersson, E., Skaftnesmo, K. O., Edvardsen, R. B., Fjelldal, P. G., Norberg, B., et al. (2017). Sex steroid production associated with puberty is absent in germ cell-free salmon. Sci. Rep. 7:12584. doi: 10.1038/s41598-017-12936-w
Kleppe, L., Edvardsen, R. B., Furmanek, T., Andersson, E., Skaftnesmo, K. O., Thyri Segafredo, F., et al. (2020). Transcriptomic analysis of dead end knockout testis reveals germ cell and gonadal somatic factors in Atlantic salmon. BMC Genomics 21:99. doi: 10.1186/s12864-020-6513-4
Kojima, M. L., de Rooij, D. G., and Page, D. C. (2019). Amplification of a broad transcriptional program by a common factor triggers the meiotic cell cycle in mice. ELife 8:e43738. doi: 10.7554/eLife.43738
Köster, J., and Rahmann, S. (2012). Snakemake—a scalable bioinformatics workflow engine. Bioinformatics 28, 2520–2522. doi: 10.1093/bioinformatics/bts480
Koubova, J., Hu, Y.-C., Bhattacharyya, T., Soh, Y. Q. S., Gill, M. E., Goodheart, M. L., et al. (2014). Retinoic acid activates two pathways required for meiosis in mice. PLoS Genet. 10:e1004541. doi: 10.1371/journal.pgen.1004541
La, H. M., Mäkelä, J.-A., Chan, A.-L., Rossello, F. J., Nefzger, C. M., Legrand, J. M. D., et al. (2018). Identification of dynamic undifferentiated cell states within the male germline. Nat. Commun. 9:2819. doi: 10.1038/s41467-018-04827-z
Lamprecht, M. R., Sabatini, D. M., and Carpenter, A. E. (2007). CellProfiler: free, versatile software for automated biological image analysis. BioTechniques 42, 71–75. doi: 10.2144/000112257
Leal, M. C., Cardoso, E. R., Nóbrega, R. H., Batlouni, S. R., Bogerd, J., França, L. R., et al. (2009b). Histological and stereological evaluation of zebrafish (Danio rerio) spermatogenesis with an emphasis on spermatogonial generations. Biol. Reprod. 81, 177–187. doi: 10.1095/biolreprod.109.076299
Leal, M. C., de Waal, P. P., García-López, A., Chen, S. X., Bogerd, J., and Schulz, R. W. (2009a). Zebrafish primary testis tissue culture: an approach to study testis function ex vivo. Gen. Comp. Endocrinol. 162, 134–138. doi: 10.1016/j.ygcen.2009.03.003
Lei, N., and Heckert, L. L. (2002). Sp1 and Egr1 regulate transcription of the Dmrt1 gene in sertoli cells. Biol. Reprod. 66, 675–684. doi: 10.1095/biolreprod66.3.675
Li, M., Feng, R., Ma, H., Dong, R., Liu, Z., Jiang, W., et al. (2016). Retinoic acid triggers meiosis initiation via stra8-dependent pathway in Southern catfish, Silurus meridionalis. Gen. Comp. Endocrinol. 232, 191–198. doi: 10.1016/j.ygcen.2016.01.003
Lien, S., Koop, B. F., Sandve, S. R., Miller, J. R., Kent, M. P., Nome, T., et al. (2016). The Atlantic salmon genome provides insights into rediploidization. Nature 533, 200–205. doi: 10.1038/nature17164
Ma, H.-T., Niu, C.-M., Xia, J., Shen, X.-Y., Xia, M.-M., Hu, Y.-Q., et al. (2018). Stimulated by retinoic acid gene 8 (Stra8) plays important roles in many stages of spermatogenesis. Asian J. Androl. 20, 479–487. doi: 10.4103/aja.aja_26_18
Mark, M., Jacobs, H., Oulad-Abdelghani, M., Dennefeld, C., Féret, B., Vernet, N., et al. (2008). STRA8-deficient spermatocytes initiate, but fail to complete, meiosis and undergo premature chromosome condensation. J. Cell Sci. 121, 3233–3242. doi: 10.1242/jcs.035071
Matson, C. K., Murphy, M. W., Griswold, M. D., Yoshida, S., Bardwell, V. J., and Zarkower, D. (2010). The mammalian doublesex homolog DMRT1 is a transcriptional gatekeeper that controls the mitosis versus meiosis decision in male germ cells. Dev. Cell 19, 612–624. doi: 10.1016/j.devcel.2010.09.010
Melo, M. C., van Dijk, P., Andersson, E., Nilsen, T. O., Fjelldal, P. G., Male, R., et al. (2015). Androgens directly stimulate spermatogonial differentiation in juvenile Atlantic salmon (Salmo salar). Gen. Comp. Endocrinol. 211, 52–61. doi: 10.1016/j.ygcen.2014.11.015
Mikedis, M. M., Fan, Y., Nicholls, P. K., Endo, T., Jackson, E. K., Cobb, S. A., et al. (2020). DAZL mediates a broad translational program regulating expansion and differentiation of spermatogonial progenitors. ELife 9:e56523. doi: 10.7554/eLife.56523
Morais, R. D. V. S., Crespo, D., Nóbrega, R. H., Lemos, M. S., van de Kant, H. J. G., de França, L. R., et al. (2017). Antagonistic regulation of spermatogonial differentiation in zebrafish (Danio rerio) by Igf3 and Amh. Mol. Cell. Endocrinol. 454, 112–124. doi: 10.1016/j.mce.2017.06.017
Moreno-Mateos, M. A., Vejnar, C. E., Beaudoin, J.-D., Fernandez, J. P., Mis, E. K., Khokha, M. K., et al. (2015). CRISPRscan: designing highly efficient sgRNAs for CRISPR-Cas9 targeting in vivo. Nat. Methods 12, 982–988. doi: 10.1038/nmeth.3543
Naughton, C. K., Jain, S., Strickland, A. M., Gupta, A., and Milbrandt, J. (2006). Glial cell-line derived neurotrophic factor-mediated RET signaling regulates spermatogonial stem cell fate. Biol. Reprod. 74, 314–321. doi: 10.1095/biolreprod.105.047365
Nóbrega, R. H., Morais, R. D. V., de, S., Crespo, D., de Waal, P. P., de França, L. R., et al. (2015). Fsh stimulates spermatogonial proliferation and differentiation in zebrafish via Igf3. Endocrinology 156, 3804–3817. doi: 10.1210/en.2015-1157
Oduwole, O. O., Peltoketo, H., and Huhtaniemi, I. T. (2018). Role of follicle-stimulating hormone in spermatogenesis. Front. Endocrinol. 9:763. doi: 10.3389/fendo.2018.00763
Paiano, J., Wu, W., Yamada, S., Sciascia, N., Callen, E., Paola Cotrim, A., et al. (2020). ATM and PRDM9 regulate SPO11-bound recombination intermediates during meiosis. Nat. Commun. 11:857. doi: 10.1038/s41467-020-14654-w
Parisi, S., McKay, M. J., Molnar, M., Thompson, M. A., van der Spek, P. J., van Drunen-Schoenmaker, E., et al. (1999). Rec8p, a meiotic recombination and sister chromatid cohesion phosphoprotein of the Rad21p family conserved from fission yeast to humans. Mol. Cell. Biol. 19, 3515–3528. doi: 10.1128/mcb.19.5.3515
Parvanov, E. D., Petkov, P. M., and Paigen, K. (2010). Prdm9 controls activation of mammalian recombination hotspots. Science 327:835. doi: 10.1126/science.1181495
Pasquier, J., Cabau, C., Nguyen, T., Jouanno, E., Severac, D., Braasch, I., et al. (2016). Gene evolution and gene expression after whole genome duplication in fish: the PhyloFish database. BMC Genomics 17:368. doi: 10.1186/s12864-016-2709-z
Pradhan, A., and Olsson, P.-E. (2015). Inhibition of retinoic acid synthesis disrupts spermatogenesis and fecundity in zebrafish. Gen. Comp. Endocrinol. 217–218, 81–91. doi: 10.1016/j.ygcen.2015.02.002
Raymond, C. S., Murphy, M. W., O’Sullivan, M. G., Bardwell, V. J., and Zarkower, D. (2000). Dmrt1, a gene related to worm and fly sexual regulators, is required for mammalian testis differentiation. Genes Dev. 14, 2587–2595. doi: 10.1101/gad.834100
Rossi, P., Sette, C., Dolci, S., and Geremia, R. (2000). Role of c-kit in mammalian spermatogenesis. J. Endocrinol. Invest. 23, 609–615. doi: 10.1007/BF03343784
Safian, D., Ryane, N., Bogerd, J., and Schulz, R. W. (2018). Fsh stimulates Leydig cell Wnt5a production, enriching zebrafish type A spermatogonia. J. Endocrinol. 239, 351–363. doi: 10.1530/JOE-18-0447
Sambroni, E., Lareyre, J.-J., and Le Gac, F. (2013). Fsh controls gene expression in fish both independently of and through steroid mediation. PLoS One 8:e76684. doi: 10.1371/journal.pone.0076684
Sawatari, E., Shikina, S., Takeuchi, T., and Yoshizaki, G. (2007). A novel transforming growth factor-β superfamily member expressed in gonadal somatic cells enhances primordial germ cell and spermatogonial proliferation in rainbow trout (Oncorhynchus mykiss). Dev. Biol. 301, 266–275. doi: 10.1016/j.ydbio.2006.10.001
Schulz, R. W., de França, L. R., Lareyre, J.-J., LeGac, F., Chiarini-Garcia, H., Nobrega, R. H., et al. (2010). Spermatogenesis in fish. Gen. Comp. Endocrinol. 165, 390–411. doi: 10.1016/j.ygcen.2009.02.013
Shaha, C., Tripathi, R., and Mishra, D. P. (2010). Male germ cell apoptosis: regulation and biology. Philos. Trans. R. Soc. Lond. B Biol. Sci. 365, 1501–1515. doi: 10.1098/rstb.2009.0124
Shami, A. N., Zheng, X., Munyoki, S. K., Ma, Q., Manske, G. L., Green, C. D., et al. (2020). Single-Cell RNA sequencing of human, macaque, and mouse testes uncovers conserved and divergent features of mammalian spermatogenesis. Dev. Cell 54, 529–547.e12. doi: 10.1016/j.devcel.2020.05.010
Shen, X., Niu, C., Guo, J., Xia, M., Xia, J., Hu, Y., et al. (2018). Stra8 may inhibit apoptosis during mouse spermatogenesis via the AKT signaling pathway. Int. J. Mol. Med. 42, 2819–2830. doi: 10.3892/ijmm.2018.3825
Thomson, T., and Lin, H. (2009). The biogenesis and function of PIWI proteins and piRNAs: progress and prospect. Annu. Rev. Cell Dev. Biol. 25, 355–376. doi: 10.1146/annurev.cellbio.24.110707.175327
Wargelius, A., Leininger, S., Skaftnesmo, K. O., Kleppe, L., Andersson, E., Taranger, G. L., et al. (2016). Dnd knockout ablates germ cells and demonstrates germ cell independent sex differentiation in Atlantic salmon. Sci. Rep. 6:21284. doi: 10.1038/srep21284
Webster, K. A., Schach, U., Ordaz, A., Steinfeld, J. S., Draper, B. W., and Siegfried, K. R. (2017). Dmrt1 is necessary for male sexual development in zebrafish. Dev. Biol. 422, 33–46. doi: 10.1016/j.ydbio.2016.12.008
Wolgemuth, D. J. (2011). Function of the A-type cyclins during gametogenesis and early embryogenesis. Results Probl. Cell Differ. 53, 391–413. doi: 10.1007/978-3-642-19065-0_17
Yoshida, K., Kondoh, G., Matsuda, Y., Habu, T., Nishimune, Y., and Morita, T. (1998). The mouse RecA-like gene Dmc1 is required for homologous chromosome synapsis during meiosis. Mol. Cell 1, 707–718. doi: 10.1016/S1097-2765(00)80070-2
Yuan, L., Liu, J.-G., Zhao, J., Brundell, E., Daneholt, B., and Höög, C. (2000). The murine SCP3 gene is required for synaptonemal complex assembly, chromosome synapsis, and male fertility. Mol. Cell 5, 73–83. doi: 10.1016/S1097-2765(00)80404-9
Zagore, L. L., Sweet, T. J., Hannigan, M. M., Weyn-Vanhentenryck, S. M., Jobava, R., Hatzoglou, M., et al. (2018). DAZL regulates germ cell survival through a network of PolyA-Proximal mRNA interactions. Cell Rep. 25, 1225–1240.e6. doi: 10.1016/j.celrep.2018.10.012
Zhang, T., and Zarkower, D. (2017). DMRT proteins and coordination of mammalian spermatogenesis. Stem Cell Res. 24, 195–202. doi: 10.1016/j.scr.2017.07.026
Zheng, Y., Phillips, L. J., Hartman, R., An, J., and Dann, C. T. (2016). Ectopic POU5F1 in the male germ lineage disrupts differentiation and spermatogenesis in mice. Reprod. Camb. Engl. 152, 363–377. doi: 10.1530/REP-16-0140
Zhou, Q., Nie, R., Li, Y., Friel, P., Mitchell, D., Hess, R. A., et al. (2008). Expression of stimulated by retinoic acid gene 8 (Stra8) in spermatogenic cells induced by retinoic acid: an in vivo study in vitamin A-sufficient postnatal murine testes. Biol. Reprod. 79, 35–42. doi: 10.1095/biolreprod.107.066795
Keywords: stimulated by retinoic acid 8, gene editing, spermatogenesis, apoptosis, single cell proliferation
Citation: Skaftnesmo KO, Crespo D, Kleppe L, Andersson E, Edvardsen RB, Norberg B, Fjelldal PG, Hansen TJ, Schulz RW and Wargelius A (2021) Loss of stra8 Increases Germ Cell Apoptosis but Is Still Compatible With Sperm Production in Atlantic Salmon (Salmo salar). Front. Cell Dev. Biol. 9:657192. doi: 10.3389/fcell.2021.657192
Received: 22 January 2021; Accepted: 29 March 2021;
Published: 16 April 2021.
Edited by:
Fei Gao, Institute of Zoology, Chinese Academy of Sciences (CAS), ChinaReviewed by:
Qi-En Yang, Northwest Institute of Plateau Biology (CAS), ChinaMaria Eugenia Teves, Virginia Commonwealth University, United States
Copyright © 2021 Skaftnesmo, Crespo, Kleppe, Andersson, Edvardsen, Norberg, Fjelldal, Hansen, Schulz and Wargelius. This is an open-access article distributed under the terms of the Creative Commons Attribution License (CC BY). The use, distribution or reproduction in other forums is permitted, provided the original author(s) and the copyright owner(s) are credited and that the original publication in this journal is cited, in accordance with accepted academic practice. No use, distribution or reproduction is permitted which does not comply with these terms.
*Correspondence: Anna Wargelius, YW5uYS53YXJnZWxpdXNAaGkubm8=
†These authors have contributed equally to this work