- 1Graduate School of Pharmaceutical Sciences, The University of Tokyo, Tokyo, Japan
- 2Department of Chemistry and Biotechnology, School of Engineering, The University of Tokyo, Tokyo, Japan
It has become apparent that our textbook illustration of singular isolated organelles is obsolete. In reality, organelles form complex cooperative networks involving various types of organelles. Light microscopic and ultrastructural studies have revealed that mitochondria–endoplasmic reticulum (ER) contact sites (MERCSs) are abundant in various tissues and cell types. Indeed, MERCSs have been proposed to play critical roles in various biochemical and signaling functions such as Ca2+ homeostasis, lipid transfer, and regulation of organelle dynamics. While numerous proteins involved in these MERCS-dependent functions have been reported, how they coordinate and cooperate with each other has not yet been elucidated. In this review, we summarize the functions of mammalian proteins that localize at MERCSs and regulate their formation. We also discuss potential roles of the MERCS proteins in regulating multiple organelle contacts.
Introduction
Electron microscopy (EM) studies have revealed that a significant portion of membranes from a variety of organelles are closely apposed but do not fuse. Among the membrane appositions within the range of 10–30 nm, membrane contact sites (MCSs) have been defined by the presence of proteins tethering two organelles (Scorrano et al., 2019). In recent years, it has become rapidly apparent that MCSs serve as unique intracellular platforms regulating a wide range of biochemical reactions. The mitochondria–endoplasmic reticulum (ER) contact sites (MERCSs) are the most frequently observed MCSs in many cell types, and extensive studies have revealed that MERCSs are hubs for the exchange of metabolites (Valm et al., 2017). For instance, the mitochondrial calcium uniporter (MCU) protein is activated only when the MCU complex is exposed to a high concentration of Ca2+ rarely reached in the cytoplasm of most cell types (1–5 μM depending on the components of the MCU complex) (Csordás et al., 2013; Patron et al., 2014; Petrungaro et al., 2015). These channel properties necessitate that the mitochondrial surface be very closely apposed to the ER surface, where Ca2+ is released from either inositol 1,4,5-trisphosphate (IP3) receptors (IP3Rs) and/or ryanodine receptors (RyRs) (Rizzuto et al., 1993; Cremer et al., 2020). Furthermore, lipid exchange between the ER and mitochondria is required for the coordinated synthesis of glycerophospholipids by lipid biosynthetic enzymes that localize at either the ER membrane (ERM) or the mitochondrial matrix. In the absence of vesicular transport between these organelles, transfer of intermediate lipid molecules relies on non-vesicular lipid transfer at contact sites (Vance, 1990; Petrungaro and Kornmann, 2019). In addition, MERCSs have been suggested to define sites of mitochondrial division and mitochondrial DNA (mtDNA) replication (Friedman et al., 2011; Murley et al., 2013; Lewis et al., 2016) as well as mitochondrial fusion (Guo et al., 2018; Abrisch et al., 2020). They also provide a platform for autophagosome biogenesis (Hailey et al., 2010; Hamasaki et al., 2013; Garofalo et al., 2016; Böckler and Westermann, 2014; Wu et al., 2016; Gomez-Suaga et al., 2017). In order to regulate this wide variety of functions at MERCSs, various protein complexes are specifically recruited and dynamically maintained at these unique contact sites.
In yeast, a protein complex called the ER–mitochondria encounter structure (ERMES) was identified as a molecular zipper bridging the ER and mitochondria (Kornmann et al., 2009). The ERMES complex consists of four core proteins: an ER-anchored maintenance of mitochondrial morphology 1 (Mmm1), mitochondrial distribution and morphology 10 (Mdm10) localized to the outer membrane of mitochondria (OMM), Mdm34 (Mmm2), and cytosolic Mdm12. The protein–protein interactions among these core components generate the tethering force between these two organelles. A few ERMES complex binding proteins such as a Ca2+-binding GTPase Gem1 (Kornmann et al., 2011; Stroud et al., 2011; Nguyen et al., 2012) and Mdm10 binding translocase of outer membrane 7 (Tom7) (Meisinger et al., 2006; Yamano et al., 2010; Becker et al., 2011; Ellenrieder et al., 2016) have been identified as the auxiliary subunits of ERMES complex. Gem1 is required for MERCS formation (Kornmann et al., 2011), whereas the function of Tom7 in regulating MERCSs is unclear.
Like Tom7, the sorting and assembly machinery (SAM) complex, which is responsible for the membrane insertion of mitochondrial outer membrane proteins, interacts with the β-barrel structure of Mdm10 at the opposite side of Mdm12 binding site (Ellenrieder et al., 2016). Given the competition between the ERMES and SAM complexes for Mdm10 binding, it is possible that MERCS formation and mitochondrial protein import are interrelated. Mmm1, Mdm12, and Mdm34 contain synaptotagmin-like mitochondrial lipid-binding protein (SMP) domains, which are homologous to the structurally well-characterized tubular lipid binding protein (TULIP) domain present in many lipid-binding proteins (I. Lee and Hong, 2006; Kopec et al., 2010). Structural analyses have shown that the SMP domain-containing proteins interact with a wide variety of glycerophospholipids (Schauder et al., 2014; AhYoung et al., 2015; Jeong et al., 2016, 2017). Thus, it has been hypothesized that ERMES might be a candidate in non-vesicular transfer of lipids between ER and mitochondria.
Likewise, characterizing the molecules responsible for MERCS formation in other eukaryotic cell types including multicellular organisms has been of great interest for a decade, which leads to the identification of proteins regulating this contact formation (Figure 1 and Table 1). The large variety of proteins identified indicates that the regulation of MERCSs in mammals is more complicated than in yeast. However, the coordination and dynamics of these protein complexes remain unclear and sometimes debated.
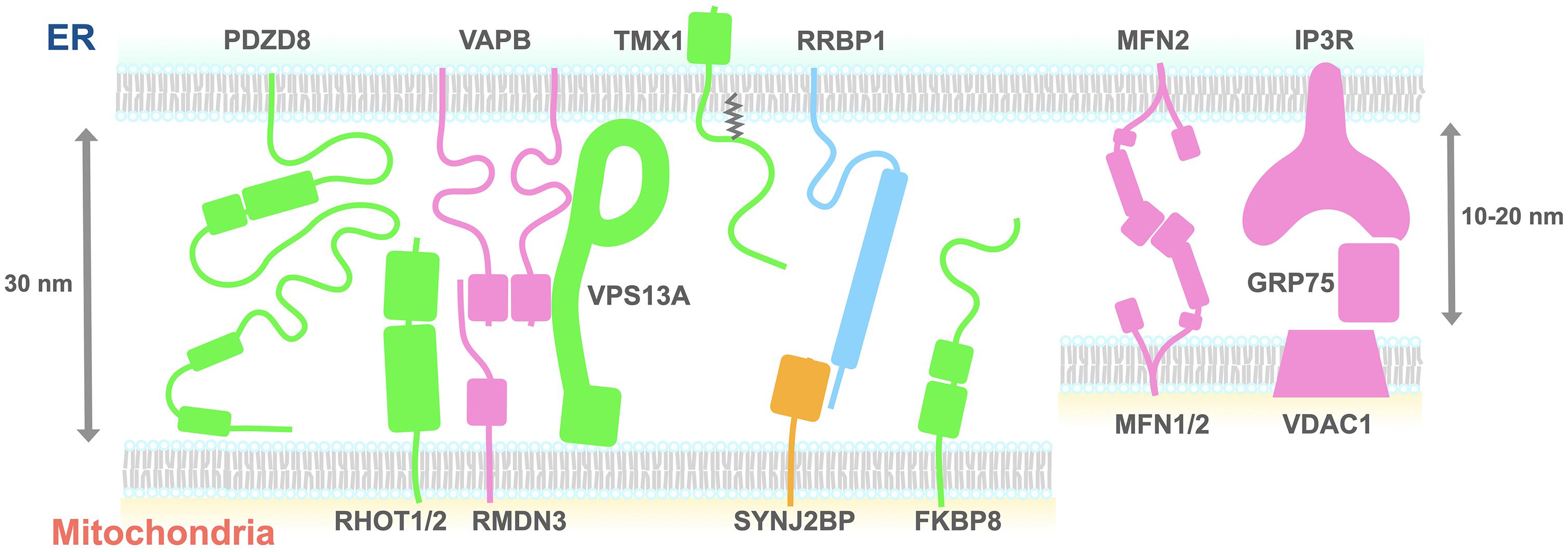
Figure 1. A schematic diagram of the proteins proposed to tether the ER and mitochondria. The size of each domain structure was either calculated from the structures deposited on the Protein Data Bank or estimated using the Phyre2 protein structure prediction tool. Proteins shown to be necessary (green, pink) or sufficient (yellow) for tethering the ER and mitochondria are depicted. Green indicates that tethering partners of the proteins remain to be determined. Light blue indicates that roles of the proteins need to be determined. ER, endoplasmic reticulum; FKBP8, FK506-binding protein 8; IP3R, inositol 1,4,5-trisphosphate receptor; Mfn2, mitofusin 2; PDZD8, PDZ domain-containing protein 8; RHOT1, Ras homolog family member T1; GRP75, glucose-regulated protein 75; RMDN3, regulator of microtubule dynamics protein 3; RRBP1, ribosome-binding protein 1; SYNJ2BP, synaptojanin-2-binding protein; TMX1, thioredoxin-related transmembrane protein 1; VAPB, VAMP-associated protein B; VPS13A, vacuolar protein sorting-associated protein 13 A; VDAC, voltage-dependent anion channel.
Mitochondria-Endoplasmic Reticulum Contact Site-Resident Proteins Involved in the Contact Formation
Yeast Endoplasmic Reticulum–Mitochondria Encounter Structure Complex and Functional Mammalian Homologs
Several orthologs of the ERMES complex proteins have been identified in metazoans, although until recently, only those of regulatory subunits of the complex were known. In mammals, mitochondria-localized GTPase family members Ras homolog family member T1 (RHOT1, also known as Miro1) and RHOT2 (Miro2) are identified as orthologs of Gem1. Confocal microscopy analysis in mouse embryonic fibroblasts (MEFs) showed that RHOT1 and RHOT2 double-knockout (DKO) resulted in the reduction of Mander’s coefficient between ER- and mitochondria-localized fluorescent proteins, as well as a slight decrease in the number of MERCSs identified by transmission EM (TEM) (Modi et al., 2019). Recruitment of RHOT1 to MERCS is promoted by its phosphorylation. Blocking the phosphorylation via a polo-like kinase 1 (PLK1) inhibitor reduced the interaction between voltage-dependent anion channel (VDAC) and IP3R, which tether the ER and mitochondria (see below) (S. Lee et al., 2016). It seems that the Tom7–SAM complex is conserved in mammals as a mitochondrial protein transport machinery; however, it remains unknown whether it is involved in MERCS formation.
Compared to the auxiliary subunits, orthologs of the core ERMES complex proteins had not been identified in metazoans because of low levels of conservation in the primary amino acid sequences. However, we recently identified the SMP domain-containing ER-resident protein called PDZ domain-containing protein 8 (PDZD8) as a functional homolog of yeast Mmm1 (Hirabayashi et al., 2017).
Mammalian Regulators of Mitochondria–Endoplasmic Reticulum Contact Sites
The recent development of the automatic Serial Scanning EM (SSEM) enabled us to visualize the three-dimensional (3D) structures of MERCSs. This technique unequivocally demonstrated that the aforementioned MMM1 homolog PDZD8 is required for MERCS formation in human HeLa cells without any effects on the 3D architecture of ER and mitochondria. Furthermore, dual-color Ca2+ imaging showed that ER–mitochondria tethering mediated by PDZD8 is essential for Ca2+ flux from the ER to the mitochondria in NIH3T3 cells and in mouse cortical pyramidal neurons (Hirabayashi et al., 2017). A recent in vitro assay suggested that SMP and PDZ domains of PDZD8 extract glycerophospholipids and ceramides, as well as cholesterol, albeit with low efficiency (Shirane et al., 2020). Thus, PDZD8 is potentially capable of transferring lipids in MCSs. Further studies are required to reveal the mitochondrial binding partner of PDZD8.
Mitochondria fusion protein mitofusin 2 (Mfn2) is the most intensively studied yet controversial ER–mitochondria tethering protein. It has been proposed that ER-localized Mfn2 engages in homotypic and heterotypic complexes with OMM localizing Mfn2 or Mnf1, respectively (De Brito and Scorrano, 2008). However, the role of Mfn2 complex in MERCS formation has been under intense debate (Filadi et al., 2018). Indeed, a recent study using split green fluorescent protein (GFP)-based contact site sensors (SPLICSs) showed that Mfn2 depletion increased and decreased the number of ER–mitochondria contact sites detected by SPLICS having spacers corresponding to short (8–10 nm) and long (40–50 nm) membrane distances, respectively (Cieri et al., 2018). Considering that the loss of Mfn1/2 complex increased the average distance between the two membranes at MERCSs only to 18 nm (De Brito and Scorrano, 2008), it is possible that the Mfn complex fine-tunes the membrane distance at MERCSs. It remains to be elucidated in what context and how Mfn2 controls MERCSs, as well as if it is related to its role in OMM fusion.
Consistent with the observation that mitochondrial fission occurs at MERCSs, the mitochondrial fission protein 1 (Fis1) is proposed to reside at MERCSs via the interaction with an ER-resident protein B-cell receptor-associated protein 31 (BAP31) (Iwasawa et al., 2011), although it remains uninvestigated whether the complex impacts MERCS formation. Also, the mitochondrial fission GTPase dynamin-related protein 1 (Drp1) has been shown to functionally stabilize MERCSs through mitochondrial E3 ubiquitin protein ligase 1 (Mul1, also known as MAPL)-dependent SUMOylation (Prudent et al., 2015).
The OMM-localized protein Spire1C, one of the splicing isoforms of Spire1, is yet another protein regulating mitochondrial fission at MERCSs. Spire1C interacts with the ER-localized isoform of Inverted formin 2 (INF2) via its kinase non-catalytic C-lobe domain (KIND) (Manor et al., 2015). The overexpression of KIND-deleted Spire1C decreased the overlap between the ER and mitochondria as identified by immunofluorescence imaging in light microscopy.
The yeast two-hybrid screening identified VDAC1–glucose-regulated protein (GRP)75–IP3R complex as a linker between the ER and mitochondria (Szabadkai et al., 2006). The OMM channel VDAC1 mediates Ca2+ channeling from microdomains with high Ca2+ concentration generated by the opening of the ER-resident Ca2+-release channel IP3Rs to the intermembrane space (Gincel et al., 2001; Rapizzi et al., 2002). IP3R2 is reported to interact not only with VDAC but also with the OMM-localized protein FUN14 domain-containing protein 1 (FUNDC1) at least in cardiac myocytes (S. Wu et al., 2017). Grp75 is a cytosolic regulator of the IP3R–VDAC complex that promotes the interaction between the channels to increase the efficiency of mitochondrial Ca2+ uptake (Szabadkai et al., 2006). The interaction between Grp75 and a MERCS-localized protein transglutaminase type 2 (TG2) has also been suggested to contribute to MERCS formation (D‘Eletto et al., 2018). Inferred from the 3D structure of each protein, the size of VDAC1–GRP75–IP3R complex is around 15 nm. Given that the triple-KO (TKO) of IP3R isoforms decreased more specifically the tight contact sites where the two membranes are less than 20 nm apart (Bartok et al., 2019), it is likely that this complex brings the ER and mitochondria in particularly close proximity. Tom70 was also reported to interact with IP3R3 to recruit it to the proximity of mitochondria, which results in promoting Ca2+ transfer from the ER to mitochondria (Filadi et al., 2018).
Another protein complex identified by the yeast two-hybrid screening is the VAMP-associated protein B (VAPB)–regulator of microtubule dynamics protein 3 (RMDN3, also called PTPIP51) complex (De vos et al., 2012). The quantification of the single-plane TEM images revealed that depletion of either VAPB or RMDN3 reduced MERCSs in human HEK293 cells (Stoica et al., 2014). The role of RMDN3 in MERCS formation was also shown in cerebellar Purkinje cells (Fecher et al., 2019). Knocking down either VAPB or RMDN3 caused a significant delay but only slight decrease in the mitochondrial Ca2+ uptake (De vos et al., 2012) and stimulated the induction of autophagy flux (Gomez-Suaga et al., 2017). Recently, the OMM protein mitoguardin 2 (Miga2) was also reported to interact with VAPA and VAPB (Freyre et al., 2019) and proposed to increase MERCS formation at least in flies (Xu et al., 2020). VAP-interacting protein vacuolar protein sorting-associated protein 13 A (VPS13A) has also been reported to localize to MERCSs and participate in their stabilization (Kumar et al., 2018; Muñoz-Braceras et al., 2019; Yeshaw et al., 2019). Interestingly, a recent study showed that RMDN3 recruits the oxysterol-binding protein (OSBP)-related proteins ORP5 and ORP8, which transfer phosphatidylinositol (PI) and phosphatidylserine (PS) at ER–plasma membrane (PM) contact sites (Chung et al., 2015; Moser von Filseck et al., 2015), to MERCSs (Galmes et al., 2016). Consistent with that, ORP5 and ORP8 are proposed to mediate PS transport, likely via the non-vesicular lipid transfer, at MERCSs (Rochin et al., 2019).
The OMM-localized fetal and adult testis-expressed transcript protein (FATE1) known as an antiapoptotic protein may also contribute to ER–mitochondria tethering. Overexpression of FATE1 partly decreased MERCSs as identified by TEM and confocal microscopy and also reduced Ca2+ uptake by mitochondria (Doghman-Bouguerra et al., 2016). EMD/Emerin is a potential interactor of FATE1 in the ER; however, the role of EMD in MERCS formation has not been investigated.
Posttranslational palmitoylation is found in several proteins localizing at MERCSs. The heterozygous KO of the redox-sensitive oxidoreductase thioredoxin-related transmembrane protein 1 (TMX1) decreased the average length of MERCSs as analyzed by TEM (Raturi et al., 2016). Importantly, the recruitment of TMX1 to MERCSs requires palmitoylation at the cytosolic domain (Roth et al., 2009; Lynes et al., 2012; Figure 1). Recruitment of the ER chaperone calnexin to MERCSs also requires palmitoylation (Lynes et al., 2012). While the palmitoylation of TMX1 is required for proper Ca2+ uptake from the ER to mitochondria, the ER-resident protein cytoskeleton-associated protein 4 (CKAP4) requires palmitoylation for sequestering VDAC2 from IP3R, which results in a decrease of MERCSs (Harada et al., 2020).
A proteomic analysis of the intersection between OMM and ERM-resident proteins obtained from ascorbate peroxidase (APEX)-mediated proximity biotinylation and subsequent mass spectrometry (MS) analysis identified the synaptojanin-2-binding protein (SYNJ2BP)–ribosome-binding protein 1 (RRBP1) complex as a potential tether that specifically regulates mitochondria–rough ER contact sites (Hung et al., 2017). TEM analysis showed that SYNJ2BP overexpression increased contacts between mitochondria and rough ER but not between mitochondria and smooth ER. In line with this, a recent report showed that RRBP1 is resident in mitochondria–rough ER contacts in mouse liver and works as a regulator of these contacts (Anastasia et al., 2021). The list of proteins identified in the intersection confirmed the localization of known MERCS proteins, even though many MERCS proteins, such as PDZD8, VAPs, and IP3Rs, listed in both ERM and OMM proteins are excluded from the intersection list because they also localized outside of MERCSs.
Recently, two split-pair proximity labeling enzymes, Contact-ID and Split-TurboID, were applied for the direct mapping of proteins localizing at MERCSs (Cho et al., 2020; Kwak et al., 2020). Although these methods are less sensitive than other methods, proteins identified with these methods are reliably localized to MERCS. Contact-ID identified FK506-binding protein 8 (FKBP8) as a novel MERCS-localizing protein. Indeed, knocking down FKBP8 reduces MERCSs in the TEM analysis and also diminishes mitochondrial Ca2+ uptake (Kwak et al., 2020).
Disease Association of Mitochondria–Endoplasmic Reticulum Contact Site Proteins
Genome-wide association studies have identified numerous gene mutations associated with neurological diseases. Among those, a significant number of mutations are found in genes related to MERCS formation, such as Mfn2, receptor expression-enhancing protein 1 (Reep1), solute carrier family 25 member 46 (SLC25A46), ATPase family AAA domain-containing protein 3A (ATAD3A), and CDGSH iron-sulfur domain-containing protein 2 (CISD2). Mutations in a genomic region coding an ER-resident protein Reep1 is associated with hereditary spastic paraplegias (HSPs) and distal hereditary motor neuropathy. A split-Renilla Luciferase 8 (RLuc8) reassembly assay suggested that Reep1 facilitates MERCS formation (Lim et al., 2015). The disease-associated Reep1 mutations impair REEP1’s ability to facilitate MERCSs, implying the relationship between HSP pathology and Reep1 function in MERCS formation (Lim et al., 2015).
A recent study using fibroblasts obtained from a patient suffering from Leigh syndrome identified a homozygous missense mutation in the genomic region coding for the mitochondrial protein SLC25A46. The study also proposed that SLC25A46 interacts with the conserved ER membrane protein complex (EMC) at MERCSs (Janer et al., 2016). Interestingly, EMC is suggested to be involved in MERCS formation in yeast (Lahiri et al., 2014). Moreover, it was shown that loss of SLC25A46 altered mitochondrial phospholipid composition, implying that SLC25A46 also plays a role in promoting lipid transfer at MERCSs (Janer et al., 2016). Further studies are still required to elucidate the function of SLC25A46 and EMC in MERCS formation in mammalian cells.
Another disease-associated gene, the inner mitochondrial membrane (IMM)-localized protein ATAD3A, has also been proposed to participate in MERCS formation (Issop et al., 2015; Baudier, 2018). Although the exact structure of ATAD3A remains unknown, a recent report proposed that the N-terminus of ATAD3A may insert into the OMM and associate with the ER (Issop et al., 2015). Thereby, ATAD3A may regulate the interactions among the IMM, OMM, and ERM.
Finally, another disease-related gene coding for a MERCS-regulating protein is CISD2, which is a causative gene associated with Wolfram syndrome. A recent study showed that MERCS formation and Ca2+ uptake in mitochondria are upregulated in patient-derived fibroblasts (Rouzier et al., 2017). In mouse white adipose tissues and fibroblasts, CISD2 is proposed to interact with GTPase immunity-associated protein (IMAP) family member 5 (GIMAP5) at MERCSs and modulates mitochondrial Ca2+ uptake (C. H. Wang et al., 2014).
Mitochondria–Endoplasmic Reticulum Contact Site Proteins at Other Organelle Contact Sites
The recent development of high-speed super-resolution microscopy revealed that the ER contacts various organelles (Valm et al., 2017). Interestingly, some of the MERCSs regulating ER-resident proteins are also found at other organelle contact sites, which implies that those proteins mediate crosstalk among multiple types of organelle contacts.
A major common function of the membrane contact sites is non-vesicular lipid transfer. In this regard, VAPA and VAPB play essential roles in diverse contact sites by transferring phospholipids and ceramides. VAP proteins interact with FFAT motifs of protein partners located on the opposing membrane or the ERM (H. Wu et al., 2018). Besides RMDN3 (PTPIP51) at MERCSs, VAP proteins form complexes with proteins such as Nir2, ceramide transferase 1 (CERT), and OSBP at the ER–Golgi contacts, and OSBP, StAR-related lipid transfer protein 3 (STARD3), Protrudin, and ORP1L at the ER–endolysosome contacts.
As mentioned above, ORP5 and ORP8, which promote the exchange of PI and PS at EM–PM contacts, also localize to MERCSs (Galmes et al., 2016). Further ORP5 is suggested to localize to ER–lipid droplet contacts and regulates the exchange of phosphatidylinositol-4-phosphate (PI4P) and PS at these contacts (Du et al., 2020).
Another example of a lipid-binding MERCS protein found in other organelle contact sites is PDZD8. Although it is still controversial if PDZD8 localizes to lysosomal-associated membrane protein 1 (LAMP1)-positive lysosomes, overexpressed PDZD8 directly interacts with Protrudin and GTP-bound Rab7, both of which localize to late endosomes (Guillén-Samander et al., 2019; Elbaz-Alon et al., 2020; Shirane et al., 2020). Interestingly, overexpressed PDZD8 and Rab7 colocalize at the three-way junction of ER, endosomes, and mitochondria, thereby inducing the association of the endosome and mitochondria. Furthermore, a recent study indicates that PDZD8 participates in the VAP complex (Cabukusta et al., 2020), which implies PDZD8’s roles in multiple different organelle contact sites. Since overexpression of MERCS proteins can disrupt their localization and therefore their functions, future studies will need to elucidate the location of endogenous PDZD8 among those contact sites. This statement is true for most proteins studied at MERCSs. The rapid development of clustered regularly interspaced short palindromic repeats (CRISPR)-Cas9-mediated knockin strategies (as used for PDZD8 in Hirabayashi et al., 2017) in various cell types will be a key step in cell biology of MERCS protein complexes.
VPS13A is yet another lipid-binding MERCS protein that localizes to additional MCSs. Yeast Vps13 resides at mitochondria–vacuole contacts (vacuole and mitochondria patches; v-CLAMPs) and ER–vacuole contacts (nuclear–vacuole junction; NVJ) and has a redundant role with the ERMES complex. It has been hypothesized that Vps13 creates an alternative lipid transport route between the ER and mitochondria through other organelles (Lang et al., 2015; Petrungaro and Kornmann, 2019). Consistent with this idea, the mammalian orthologs of Vps13, VPS13A, and VPS13C, have been reported to possess the ability to transfer glycerophospholipids between membranes in vitro (Kumar et al., 2018). Furthermore, VPS13A has been reported to localize at mitochondria–endosome/lysosome contacts and ER–lipid droplet contacts as well as MERCSs, whereas VPS13C is distributed to ER–endosome contacts and ER–lipid droplet contacts, loss of which causes mitochondrial dysfunction (Lesage et al., 2016; Kumar et al., 2018). These reports imply the function of VPS13 family proteins in lipid transport at multiple MCSs, although they also play distinct roles at each contact site, such as MERCS formation, autophagy induction, and regulation of lipid droplet motility (Muñoz-Braceras et al., 2015, 2019; Kumar et al., 2018; Yeshaw et al., 2019). Since both VPS13A and VPS13C are recruited to the ER via the FFAT motif (Kumar et al., 2018; Yeshaw et al., 2019), it is plausible that their localization at ER–other organelle contacts might be regulated through the interaction with VAPs. It also has been shown that VPS13A interacts with Rab7 (Muñoz-Braceras et al., 2019), which may result in VPS13A’s recruitment to mitochondria–endosome contacts.
Mitochondria also form contact sites with organelles other than the ER. Several MERCS-localized mitochondrial proteins have also been reported to reside at other organelle contact sites. Mfn2 localizes at the contact sites between mitochondria and the lysosome-related organelle of pigment cells melanosome (Daniele et al., 2014). Considering that Fzo1, a yeast homolog of Mfn, is suggested to reside at mitochondria–peroxisome contacts (Shai et al., 2018), Mfns might participate in the contact formation between mitochondria and other various organelles. The mitochondria–lysosome contacts mark at the site of mitochondrial fission. At this fission site, Fis1 recruits the Rab7 GTPase-activating protein TBC1 domain family member 15 (TBC1D15), which results in untethering of the contacts (Wong et al., 2018). This suggests that Fis1 localizes at the mitochondria–lysosome contact sites, as well as at MERCSs.
Conclusion
In recent years, owing to advances in microscopy and the development of new biochemical tools, the list of proteins involved in the regulation of MERCSs has been dramatically expanded. Given that PDZD8 remains the only identified mammalian ortholog of the ERMES core subunits (Mmm1), it is conceivable that the mammalian ER and mitochondria tethering protein complexes have not directly evolved from the yeast ERMES complex. Therefore, MERCSs might have evolved various cell type-specific roles in mammals, which are just beginning to be explored. Provided that the properties of MERCS proteins, such as domain structure, size, and localization, are quite diverse, it is plausible to assume that they work at different subdomains of MERCSs, different steps of MERCS formation, or in different cell types. This complex regulation of MERCSs might be required for the precise control of biochemical reactions in response to the various cellular demands unique to each cell type. Since many MERCS proteins also reside at other organelle contact sites, investigation of the dynamic localization of endogenous, as opposed to overexpressed, proteins in a variety of cellular contexts will improve our understanding of the complex spatiotemporal regulation of MERCSs and pave the way to reveal the physiological roles of these contact sites.
Author Contributions
Both authors wrote the manuscript. Both authors contributed to the article and approved the submitted version.
Conflict of Interest
The authors declare that the research was conducted in the absence of any commercial or financial relationships that could be construed as a potential conflict of interest.
Acknowledgments
We apologize to the many scientists whose work we were not able to credit owing to space restrictions. We thank Drs. Franck Polleux and Heike Blockus for comments on the manuscript.
References
Abrisch, R. G., Gumbin, S. C., Wisniewski, B. T., Lackner, L. L., and Voeltz, G. K. (2020). Fission and fusion machineries converge at ER contact sites to regulate mitochondrial morphology. J. Cell Biol. 219:e201911122. doi: 10.1083/jcb.201911122
AhYoung, A. P., Jiang, J., Zhang, J., Dang, X. K., Loo, J. A., Zhou, Z. H., et al. (2015). Conserved SMP domains of the ERMES complex bind phospholipids and mediate tether assembly. Proc. Natl. Acad. Sci. U S A. 112, E3179–E3188. doi: 10.1073/pnas.1422363112
Anastasia, I., Ilacqua, N., Raimondi, A., Lemieux, P., Ghandehari-Alavijeh, R., Faure, G., et al. (2021). Mitochondria-rough-er contacts in the liver regulate systemic lipid homeostasis. Cell Rep. 34:108873. doi: 10.1016/j.celrep.2021.108873
Bartok, A., Weaver, D., Golenár, T., Nichtova, Z., Katona, M., Bánsághi, S., et al. (2019). IP3 receptor isoforms differently regulate ER-mitochondrial contacts and local calcium transfer. Nat. Commun. 10:3726. doi: 10.1038/s41467-019-11646-3
Baudier, J. (2018). ATAD3 proteins: brokers of a mitochondria-endoplasmic reticulum connection in mammalian cells. Biol. Rev. 93, 827–844. doi: 10.1111/brv.12373
Becker, T., Wenz, L. S., Thornton, N., Stroud, D., Meisinger, C., Wiedemann, N., et al. (2011). Biogenesis of mitochondria: dual role of Tom7 in modulating assembly of the preprotein translocase of the outer membrane. J. Mol. Biol. 405, 113–124. doi: 10.1016/j.jmb.2010.11.002
Böckler, S., and Westermann, B. (2014). Mitochondrial ER contacts are crucial for mitophagy in yeast. Dev. Cell. 28, 450–458. doi: 10.1016/j.devcel.2014.01.012
Bosc, C., Broin, N., Fanjul, M., Saland, E., Farge, T., Courdy, C., et al. (2020). Autophagy regulates fatty acid availability for oxidative phosphorylation through mitochondria-endoplasmic reticulum contact sites. Nat. Commun. 11:4056. doi: 10.1038/s41467-020-17882-2
Cabukusta, B., Berlin, I., van Elsland, D. M., Forkink, I., Spits, M., de Jong, A. W. M., et al. (2020). Human VAPome analysis reveals MOSPD1 and MOSPD3 as membrane contact site proteins interacting with FFAT-Related FFNT motifs. Cell Rep. 33:108475. doi: 10.1016/j.celrep.2020.108475
Cho, K. F., Branon, T. C., Rajeev, S., Svinkina, T., Udeshi, N. D., Thoudam, T., et al. (2020). Split-TurboID enables contact-dependent proximity labeling in cells. Proc. Natl. Acad. Sci. U S A. 117, 12143–12154. doi: 10.1073/pnas.1919528117
Chung, J., Torta, F., Masai, K., Lucast, L., Czapla, H., Tanner, L. B., et al. (2015). PI4P/phosphatidylserine countertransport at ORP5- and ORP8-mediated ER - plasma membrane contacts. Science 349, 428–432. doi: 10.1126/science.aab1370
Cieri, D., Vicario, M., Giacomello, M., Vallese, F., Filadi, R., Wagner, T., et al. (2018). SPLICS: a split green fluorescent protein-based contact site sensor for narrow and wide heterotypic organelle juxtaposition. Cell Death Differ. 25, 1131–1145. doi: 10.1038/s41418-017-0033-z
Cosson, P., Marchetti, A., Ravazzola, M., and Orci, L. (2012). Mitofusin-2 independent juxtaposition of endoplasmic reticulum and mitochondria: an ultrastructural study. PLoS One. 7:e46293. doi: 10.1371/journal.pone.0046293
Cremer, T., Neefjes, J., and Berlin, I. (2020). The journey of Ca2+ through the cell – pulsing through the network of ER membrane contact sites. J. Cell Sci. 133:jcs249136. doi: 10.1242/jcs.249136
Csordás, G., Golenár, T., Seifert, E. L., Kamer, K. J., Sancak, Y., Perocchi, F., et al. (2013). MICU1 controls both the threshold and cooperative activation of the mitochondrial Ca2+ uniporter. Cell. Metabol. 17, 976–987. doi: 10.1016/j.cmet.2013.04.020
Daniele, T., Hurbain, I., Vago, R., Casari, G., Raposo, G., Tacchetti, C., et al. (2014). Mitochondria and melanosomes establish physical contacts modulated by Mfn2 and involved in organelle biogenesis. Curr. Biol. 24, 393–403. doi: 10.1016/j.cub.2014.01.007
De Brito, O. M., and Scorrano, L. (2008). Mitofusin 2 tethers endoplasmic reticulum to mitochondria. Nature 456, 605–610. doi: 10.1038/nature07534
De vos, K. J., Mórotz, G. M., Stoica, R., Tudor, E. L., Lau, K. F., Ackerley, S., et al. (2012). VAPB interacts with the mitochondrial protein PTPIP51 to regulate calcium homeostasis. Hum. Mol. Genet. 21, 1299–1311. doi: 10.1093/hmg/ddr559
Doghman-Bouguerra, M., Granatiero, V., Sbiera, S., Sbiera, I., Lacas-Gervais, S., Brau, F., et al. (2016). FATE 1 antagonizes calcium- and drug-induced apoptosis by uncoupling ER and mitochondria. EMBO Rep. 17, 1264–1280. doi: 10.15252/embr.201541504
Du, X., Zhou, L., Aw, Y. C., Mak, H. Y., Xu, Y., Rae, J., et al. (2020). ORP5 localizes to ER-lipid droplet contacts and regulates the level of PI(4)P on lipid droplets. J. Cell Biol. 219:e201905162. doi: 10.1083/jcb.201905162
D‘Eletto, M., Rossin, F., Occhigrossi, L., Farrace, M. G., Faccenda, D., Desai, R., et al. (2018). Transglutaminase type 2 regulates ER-mitochondria contact sites by interacting with GRP75. Cell. Rep. 25, 3573–3581. doi: 10.1016/j.celrep.2018.11.094
Elbaz-Alon, Y., Guo, Y., Segev, N., Harel, M., Quinnell, D. E., Geiger, T., et al. (2020). PDZD8 interacts with protrudin and Rab7 at ER-late endosome membrane contact sites associated with mitochondria. Nat. Commun. 11:3645. doi: 10.1038/s41467-020-17451-7
Ellenrieder, L., Opaliłski, Ł, Becker, L., Krüger, V., Mirus, O., Straub, S. P., et al. (2016). Separating mitochondrial protein assembly and endoplasmic reticulum tethering by selective coupling of Mdm10. Nat. Commun. 7:13021. doi: 10.1038/ncomms13021
Fecher, C., Trovò, L., Müller, S. A., Snaidero, N., Wettmarshausen, J., Heink, S., et al. (2019). Cell-type-specific profiling of brain mitochondria reveals functional and molecular diversity. Nat. Neurosci. 22, 1731–1742. doi: 10.1038/s41593-019-0479-z
Filadi, R., Greotti, E., and Pizzo, P. (2018). Highlighting the endoplasmic reticulum-mitochondria connection: focus on mitofusin 2. Pharmacol. Res. 128, 42–51. doi: 10.1016/j.phrs.2018.01.003
Filadi, R., Greotti, E., Turacchio, G., Luini, A., Pozzan, T., and Pizzo, P. (2015). Mitofusin 2 ablation increases endoplasmic reticulum-mitochondria coupling. Proc. Natl. Acad. Sci. U S A. 112, E2174–E2181. doi: 10.1073/pnas.1504880112
Filadi, R., Greotti, E., Turacchio, G., Luini, A., Pozzan, T., and Pizzo, P. (2016). Presenilin 2 modulates endoplasmic reticulum-mitochondria coupling by tuning the antagonistic effect of mitofusin 2. Cell Rep. 15, 2226–2238. doi: 10.1016/j.celrep.2016.05.013
Filadi, R., Leal, N. S., Schreiner, B., Rossi, A., Dentoni, G., Pinho, C. M., et al. (2018). TOM70 sustains cell bioenergetics by promoting IP3R3-mediated ER to mitochondria Ca2+ transfer. Curr. Biol. 28, 369–382. doi: 10.1016/j.cub.2017.12.047
Freyre, C. A. C., Rauher, P. C., Ejsing, C. S., and Klemm, R. W. (2019). MIGA2 links mitochondria, the ER, and lipid droplets and promotes De novo lipogenesis in adipocytes. Mol. Cell. 76, 811–825. doi: 10.1016/j.molcel.2019.09.011
Friedman, J. R., Lackner, L. L., West, M., DiBenedetto, J. R., Nunnari, J., and Voeltz, G. K. (2011). ER tubules mark sites of mitochondrial division. Science 334, 358–362. doi: 10.1126/science.1207385
Galmes, R., Houcine, A., Vliet, A. R., Agostinis, P., Jackson, C. L., and Giordano, F. (2016). ORP5/ORP8 localize to endoplasmic reticulum–mitochondria contacts and are involved in mitochondrial function. EMBO Rep. 17, 800–810. doi: 10.15252/embr.201541108
Garofalo, T., Matarrese, P., Manganelli, V., Marconi, M., Tinari, A., Gambardella, L., et al. (2016). Evidence for the involvement of lipid rafts localized at the ER-mitochondria associated membranes in autophagosome formation. Autophagy 12, 917–935. doi: 10.1080/15548627.2016.1160971
Gincel, D., Zaid, H., and Shoshan-Barmatz, V. (2001). Calcium binding and translocation by the voltage-dependent anion channel: a possible regulatory mechanism in mitochondrial function. Biochem. J. 358, 147–155.
Gomez-Suaga, P., Paillusson, S., Stoica, R., Noble, W., Hanger, D. P., and Miller, C. C. J. (2017). The ER-mitochondria tethering complex VAPB-PTPIP51 regulates autophagy. Curr. Biol. 27, 371–385. doi: 10.1016/j.cub.2016.12.038
Guillén-Samander, A., Bian, X., and de Camilli, P. (2019). PDZD8 mediates a Rab7-dependent interaction of the ER with late endosomes and lysosomes. Proc. Natl. Acad. Sci. U S A. 116, 22619–22623. doi: 10.1073/pnas.1913509116
Guo, Y., Li, D., Zhang, S., Yang, Y., Liu, J. J., Wang, X., et al. (2018). Visualizing intracellular organelle and cytoskeletal interactions at nanoscale resolution on millisecond timescales. Cell 175, 1430–1442. doi: 10.1016/j.cell.2018.09.057
Hailey, D. W., Rambold, A. S., Satpute-Krishnan, P., Mitra, K., Sougrat, R., Kim, P. K., et al. (2010). Mitochondria supply membranes for autophagosome biogenesis during starvation. Cell 141, 656–667. doi: 10.1016/j.cell.2010.04.009
Hamasaki, M., Furuta, N., Matsuda, A., Nezu, A., Yamamoto, A., Fujita, N., et al. (2013). Autophagosomes form at ER-mitochondria contact sites. Nature 495, 389–393. doi: 10.1038/nature11910
Harada, T., Sada, R., Osugi, Y., Matsumoto, S., Matsuda, T., Hayashi-Nishino, M., et al. (2020). Palmitoylated CKAP4 regulates mitochondrial functions through an interaction with VDAC2 at ER-mitochondria contact sites. J. Cell. Sci. 133:jcs249045. doi: 10.1242/jcs.249045
Hirabayashi, Y., Kwon, S. K., Paek, H., Pernice, W. M., Paul, M. A., Lee, J., et al. (2017). ER-mitochondria tethering by PDZD8 regulates Ca2+ dynamics in mammalian neurons. Science 358, 623–630. doi: 10.1126/science.aan6009
Hung, V., Lam, S. S., Udeshi, N. D., Svinkina, T., Guzman, G., Mootha, V. K., et al. (2017). Proteomic mapping of cytosol-facing outer mitochondrial and ER membranes in living human cells by proximity biotinylation. Elife 6:e24463. doi: 10.7554/eLife.24463
Issop, L., Fan, J., Lee, S., Rone, M. B., Basu, K., Mui, J., et al. (2015). Mitochondria-associated membrane formation in hormone-stimulated leydig cell steroidogenesis: role of ATAD3. Endocrinology 156, 334–345. doi: 10.1210/en.2014-1503
Iwasawa, R., Mahul-Mellier, A. L., Datler, C., Pazarentzos, E., and Grimm, S. (2011). Fis1 and Bap31 bridge the mitochondria-ER interface to establish a platform for apoptosis induction. EMBO J. 30, 556–568. doi: 10.1038/emboj.2010.346
Janer, A., Prudent, J., Paupe, V., Fahiminiya, S., Majewski, J., Sgarioto, N., et al. (2016). SLC25A46 is required for mitochondrial lipid homeostasis and cristae maintenance and is responsible for Leigh syndrome. EMBO Mol. Med. 8, 1019–1038. doi: 10.15252/emmm.201506159
Jeong, H., Park, J., Jun, Y., and Lee, C. (2017). Crystal structures of Mmm1 and Mdm12–Mmm1 reveal mechanistic insight into phospholipid trafficking at ER-mitochondria contact sites. Proc. Natl. Acad. Sci. U S A. 114, E9502–E9511. doi: 10.1073/pnas.1715592114
Jeong, H., Park, J., and Lee, C. (2016). Crystal structure of Mdm12 reveals the architecture and dynamic organization of the ERMES complex. EMBO Rep. 17, 1857–1871. doi: 10.15252/embr.201642706
Kopec, K. O., Alva, V., and Lupas, A. N. (2010). Homology of SMP domains to the TULIP superfamily of lipid-binding proteins provides a structural basis for lipid exchange between ER and mitochondria. Bioinformatics 26, 1927–1931. doi: 10.1093/bioinformatics/btq326
Kornmann, B., Currie, E., Collins, S. R., Schuldiner, M., Nunnari, J., Weissman, J. S., et al. (2009). An ER-mitochondria tethering complex revealed by a synthetic biology screen. Science 325, 477–481. doi: 10.1126/science.1175088
Kornmann, B., Osman, C., and Walter, P. (2011). The conserved GTPase Gem1 regulates endoplasmic reticulum-mitochondria connections. Proc. Natl. Acad. Sci. U S A. 108, 14151–14156. doi: 10.1073/pnas.1111314108
Kumar, N., Leonzino, M., Hancock-Cerutti, W., Horenkamp, F. A., Li, P. Q., Lees, J. A., et al. (2018). VPS13A and VPS13C are lipid transport proteins differentially localized at ER contact sites. J. Cell. Biol. 217, 3625–3639. doi: 10.1083/JCB.201807019
Kwak, C., Shin, S., Park, J. S., Jung, M., My Nhung, T. T., Kang, M. G., et al. (2020). Contact-ID, a tool for profiling organelle contact sites, reveals regulatory proteins of mitochondrial-associated membrane formation. Proc. Natl. Acad. Sci. U S A. 117, 12109–12120. doi: 10.1073/pnas.1916584117
Lahiri, S., Chao, J. T., Tavassoli, S., Wong, A. K. O., Choudhary, V., Young, B. P., et al. (2014). A conserved endoplasmic reticulum membrane protein complex (EMC) facilitates phospholipid transfer from the ER to mitochondria. PLoS Biol. 12:1001969. doi: 10.1371/journal.pbio.1001969
Lang, A. B., John Peter, A. T. A. T., Walter, P., and Kornmann, B. (2015). ER-mitochondrial junctions can be bypassed by dominant mutations in the endosomal protein Vps13. J. Cell. Biol. 210, 883–890. doi: 10.1083/jcb.201502105
Lee, I., and Hong, W. (2006). Diverse membrane-associated proteins contain a novel SMP domain. FASEB J. 20, 202–206. doi: 10.1096/fj.05-4581hyp
Lee, S., Lee, K. S., Huh, S., Liu, S., Lee, D. Y., Hong, S. H., et al. (2016). Polo kinase phosphorylates miro to control ER-mitochondria contact sites and mitochondrial Ca2+ homeostasis in neural stem cell development. Dev. Cell. 37, 174–189. doi: 10.1016/j.devcel.2016.03.023
Lesage, S., Drouet, V., Majounie, E., Deramecourt, V., Jacoupy, M., Nicolas, A., et al. (2016). Loss of VPS13C function in autosomal-recessive parkinsonism causes mitochondrial dysfunction and increases PINK1/Parkin-dependent mitophagy. Am. J. Hum. Genet. 98, 500–513. doi: 10.1016/j.ajhg.2016.01.014
Lewis, S. C., Uchiyama, L. F., and Nunnari, J. (2016). ER-mitochondria contacts couple mtDNA synthesis with mitochondrial division in human cells. Science 353:aaf5549. doi: 10.1126/science.aaf5549
Lim, Y., Cho, I. T., Schoel, L. J., Cho, G., and Golden, J. A. (2015). Hereditary spastic paraplegia-linked REEP1 modulates endoplasmic reticulum/mitochondria contacts. Annal. Neurol. 78, 679–696. doi: 10.1002/ana.24488
Lynes, E. M., Bui, M., Yap, M. C., Benson, M. D., Schneider, B., Ellgaard, L., et al. (2012). Palmitoylated TMX and calnexin target to the mitochondria-associated membrane. EMBO J. 31, 457–470. doi: 10.1038/emboj.2011.384
Manor, U., Bartholomew, S., Golani, G., Christenson, E., Kozlov, M., Higgs, H., et al. (2015). A mitochondria-anchored isoform of the actin-nucleating spire protein regulates mitochondrial division. ELife 4:e08828. doi: 10.7554/eLife.08828
Meisinger, C., Wiedemann, N., Rissler, M., Strub, A., Milenkovic, D., Schönfisch, B., et al. (2006). Mitochondrial protein sorting: differentiation of β-barrel assembly by tom7-mediated segregation of Mdm10. J. Biol. Chem. 281, 22819–22826. doi: 10.1074/jbc.M602679200
Modi, S., López-Doménech, G., Halff, E. F., Covill-Cooke, C., Ivankovic, D., Melandri, D., et al. (2019). Miro clusters regulate ER-mitochondria contact sites and link cristae organization to the mitochondrial transport machinery. Nat. Commun. 10:4399. doi: 10.1038/s41467-019-12382-4
Moser von Filseck, J., Čopič, A., Delfosse, V., Vanni, S., Jackson, C. L., Bourguet, W., et al. (2015). Phosphatidylserine transport by ORP/Osh proteins is driven by phosphatidylinositol 4-phosphate. Science 349, 432–436. doi: 10.1126/science.aab1346
Muñoz-Braceras, S., Calvo, R., and Escalante, R. (2015). TipC and the chorea-acanthocytosis protein VPS13A regulate autophagy in Dictyostelium and human HeLa cells. Autophagy 11, 918–927. doi: 10.1080/15548627.2015.1034413
Muñoz-Braceras, S., Tornero-Écija, A. R., Vincent, O., and Escalante, R. (2019). VPS13A is closely associated with mitochondria and is required for efficient lysosomal degradation. Dis. Model. Mech. 12:dmm036681. doi: 10.1242/DMM.036681
Murley, A., Lackner, L. L., Osman, C., West, M., Voeltz, G. K., Walter, P., et al. (2013). ER-associated mitochondrial division links the distribution of mitochondria and mitochondrial DNA in yeast. ELife 2:e00422. doi: 10.7554/eLife.00422
Nagashima, S., Takeda, K., Ohno, N., Ishido, S., Aoki, M., Saitoh, Y., et al. (2019). MITOL deletion in the brain impairs mitochondrial structure and ER tethering leading to oxidative stress. Life Sci. Alliance 2:e201900308. doi: 10.26508/lsa.201900308
Naon, D., Zaninello, M., Giacomello, M., Varanita, T., Grespi, F., Lakshminaranayan, S., et al. (2016). Critical reappraisal confirms that Mitofusin 2 is an endoplasmic reticulum-mitochondria tether. Proc. Natl. Acad. Sci. U S A. 113, 11249–11254. doi: 10.1073/pnas.1606786113
Nguyen, T. T., Lewandowska, A., Choi, J. Y., Markgraf, D. F., Junker, M., Bilgin, M., et al. (2012). Gem1 and ERMES do not directly affect phosphatidylserine transport from ER to mitochondria or mitochondrial inheritance. Traffic 13, 880–890. doi: 10.1111/j.1600-0854.2012.01352.x
Patron, M., Checchetto, V., Raffaello, A., Teardo, E., VecellioReane, D., Mantoan, M., et al. (2014). MICU1 and MICU2 finely tune the mitochondrial Ca2+ uniporter by exerting opposite effects on MCU activity. Mol. Cell. 53, 726–737. doi: 10.1016/j.molcel.2014.01.013
Petrungaro, C., and Kornmann, B. (2019). Lipid exchange at ER-mitochondria contact sites: a puzzle falling into place with quite a few pieces missing. Curr. Opin. Cell. Biol. 57, 71–76. doi: 10.1016/j.ceb.2018.11.005
Petrungaro, C., Zimmermann, K. M., Küttner, V., Fischer, M., Dengjel, J., Bogeski, I., et al. (2015). The Ca2+-dependent release of the Mia40-induced MICU1-MICU2 dimer from MCU regulates mitochondrial Ca2+ uptake. Cell Metabol. 22, 721–733. doi: 10.1016/j.cmet.2015.08.019
Prudent, J., Zunino, R., Sugiura, A., Mattie, S., Shore, G. C., and McBride, H. M. (2015). MAPL SUMOylation of Drp1 stabilizes an ER/mitochondrial platform required for cell death. Mol. Cell. 59, 941–955. doi: 10.1016/j.molcel.2015.08.001
Puri, R., Cheng, X. T., Lin, M. Y., Huang, N., and Sheng, Z. H. (2019). Mul1 restrains Parkin-mediated mitophagy in mature neurons by maintaining ER-mitochondrial contacts. Nat. Comm. 10. doi: 10.1038/s41467-019-11636-5
Rapizzi, E., Pinton, P., Szabadkai, G., Wieckowski, M. R., Vandecasteele, G., Baird, G., et al. (2002). Recombinant expression of the voltage-dependent anion channel enhances the transfer of Ca2+ microdomains to mitochondria. J. Cell Biol. 159, 613–624. doi: 10.1083/jcb.200205091
Raturi, A., Gutiérrez, T., Ortiz-Sandoval, C., Ruangkittisakul, A., Herrera-Cruz, M. S., Rockley, J. P., et al. (2016). TMX1 determines cancer cell metabolism as a thiolbased modulator of ER-mitochondria Ca2+ flux. J. Cell Biol. 214, 433–444. doi: 10.1083/jcb.201512077
Rizzuto, R., Brini, M., Murgia, M., and Pozzan, T. (1993). Microdomains with high Ca2+ close to IP3-sensitive channels that are sensed by neighboring mitochondria. Science 262, 744–747. doi: 10.1126/science.8235595
Rochin, L., Sauvanet, C., Jääskeläinen, E., Houcine, A., Kivelä, A., Xingjie, M. A., et al. (2019). Orp5 transfers phosphatidylserine to mitochondria and regulates mitochondrial calcium uptake at endoplasmic reticulum - mitochondria contact sites. bioRxiv 2019:695577. doi: 10.1101/695577
Roth, D., Lynes, E., Riemer, J., Hansen, H. G., Althaus, N., Simmen, T., et al. (2009). A di-arginine motif contributes to the ER localization of the type I transmembrane ER oxidoreductase TMX4. Biochem. J. 425, 195–208. doi: 10.1042/BJ20091064
Rouzier, C., Moore, D., Delorme, C., Lacas-Gervais, S., Ait-El-Mkadem, S., Fragaki, K., et al. (2017). A novel CISD2 mutation associated with a classical wolfram syndrome phenotype alters Ca2+ homeostasis and ER-mitochondria interactions. Hum. Mol. Genet. 26, 1599–1611. doi: 10.1093/hmg/ddx060
Schauder, C. M., Wu, X., Saheki, Y., Narayanaswamy, P., Torta, F., Wenk, M. R., et al. (2014). Structure of a lipid-bound extended synaptotagmin indicates a role in lipid transfer. Nature 510, 552–555. doi: 10.1038/nature13269
Scorrano, L., De Matteis, M. A., Emr, S., Giordano, F., Hajnóczky, G., Kornmann, B., et al. (2019). Coming together to define membrane contact sites. Nat. Commun. 10:1287. doi: 10.1038/s41467-019-09253-3
Shai, N., Yifrach, E., Van Roermund, C. W. T., Cohen, N., Bibi, C., Ijlst, L., et al. (2018). Systematic mapping of contact sites reveals tethers and a function for the peroxisome-mitochondria contact. Nat. Commun. 9:1761. doi: 10.1038/s41467-018-03957-8
Shirane, M., Wada, M., Morita, K., Hayashi, N., Kunimatsu, R., Matsumoto, Y., et al. (2020). Protrudin and PDZD8 contribute to neuronal integrity by promoting lipid extraction required for endosome maturation. Nat. Commun. 11:4576. doi: 10.1038/s41467-020-18413-9
Stoica, R., De Vos, K. J., Paillusson, S., Mueller, S., Sancho, R. M., Lau, K. F., et al. (2014). ER-mitochondria associations are regulated by the VAPB-PTPIP51 interaction and are disrupted by ALS/FTD-associated TDP-43. Nat. Commun. 5:3996. doi: 10.1038/ncomms4996
Stroud, D. A., Oeljeklaus, S., Wiese, S., Bohnert, M., Lewandrowski, U., Sickmann, A., et al. (2011). Composition and topology of the endoplasmic reticulum-mitochondria encounter structure. J. Mol. Biol. 413, 743–750. doi: 10.1016/j.jmb.2011.09.012
Sugiura, A., Nagashima, S., Tokuyama, T., Amo, T., Matsuki, Y., Ishido, S., et al. (2013). MITOL regulates endoplasmic reticulum-mitochondria contacts via Mitofusin2. Mol. Cell 51, 20–34. doi: 10.1016/j.molcel.2013.04.023
Szabadkai, G., Bianchi, K., Várnai, P., De Stefani, D., Wieckowski, M. R., Cavagna, D., et al. (2006). Chaperone-mediated coupling of endoplasmic reticulum and mitochondrial Ca2+ channels. J. Cell Biol. 175, 901–911. doi: 10.1083/jcb.200608073
Valm, A. M., Cohen, S., Legant, W. R., Melunis, J., Hershberg, U., Wait, E., et al. (2017). Applying systems-level spectral imaging and analysis to reveal the organelle interactome. Nature 546, 162–167. doi: 10.1038/nature22369
Vance, J. E. (1990). Phospholipid synthesis in a membrane fraction associated with mitochondria. J. Biol. Chem. 265, 7248–7256. doi: 10.1016/S0021-9258(19)39106-9
Wang, C. H., Chen, Y. F., Wu, C. Y., Wu, P. C., Huang, Y. L., Kao, C. H., et al. (2014). Cisd2 modulates the differentiation and functioning of adipocytes by regulating intracellular Ca2+ homeostasis. Hum. Mol. Genet. 23, 4770–4785. doi: 10.1093/hmg/ddu193
Wang, P. T. C., Garcin, P. O., Fu, M., Masoudi, M., St-Pierre, P., Panté, N., et al. (2015). Distinct mechanisms controlling rough and smooth endoplasmic reticulum contacts with mitochondria. J. Cell Sci. 128, 2759–2765. doi: 10.1242/jcs.171132
Wong, Y. C., Ysselstein, D., and Krainc, D. (2018). Mitochondria-lysosome contacts regulate mitochondrial fission via RAB7 GTP hydrolysis. Nature 554, 382–386. doi: 10.1038/nature25486
Wu, H., Carvalho, P., and Voeltz, G. K. (2018). Here, there, and everywhere: the importance of ER membrane contact sites. Science 361, eaan5835. doi: 10.1126/science.aan5835
Wu, S., Lu, Q., Wang, Q., Ding, Y., Ma, Z., Mao, X., et al. (2017). Binding of FUNDC1 with inositol 1,4,5-trisphosphate receptor in mitochondria-associated endoplasmic reticulum (ER) membranes maintains mitochondrial dynamics and function in hearts in vivo. Circulation 136, 2248–2266. doi: 10.1161/CIRCULATIONAHA.117.030235
Wu, W., Lin, C., Wu, K., Jiang, L., Wang, X., Li, W., et al. (2016). FUNDC 1 regulates mitochondrial dynamics at the ER –mitochondrial contact site under hypoxic conditions. EMBO J. 35, 1368–1384. doi: 10.15252/embj.201593102
Xu, L., Wang, X., Zhou, J., Qiu, Y., Shang, W., Liu, J. P., et al. (2020). Miga-mediated endoplasmic reticulum– mitochondria contact sites regulate neuronal homeostasis. ELife 9, 1–26. doi: 10.7554/eLife.56584
Yamano, K., Tanaka-Yamano, S., and Endo, T. (2010). Tom7 regulates Mdm10-mediated assembly of the mitochondrial import channel protein TOM40. J. Biol. Chem. 285, 41222–41231. doi: 10.1074/jbc.M110.163238
Keywords: mitochondria, ER, organelle contact sites, mammalian protein, tether
Citation: Aoyama-Ishiwatari S and Hirabayashi Y (2021) Endoplasmic Reticulum–Mitochondria Contact Sites—Emerging Intracellular Signaling Hubs. Front. Cell Dev. Biol. 9:653828. doi: 10.3389/fcell.2021.653828
Received: 15 January 2021; Accepted: 06 April 2021;
Published: 20 May 2021.
Edited by:
Yasunori Saheki, Nanyang Technological University, SingaporeReviewed by:
Julien Prudent, University of Cambridge, United KingdomJaakko Saraste, University of Bergen, Norway
Copyright © 2021 Aoyama-Ishiwatari and Hirabayashi. This is an open-access article distributed under the terms of the Creative Commons Attribution License (CC BY). The use, distribution or reproduction in other forums is permitted, provided the original author(s) and the copyright owner(s) are credited and that the original publication in this journal is cited, in accordance with accepted academic practice. No use, distribution or reproduction is permitted which does not comply with these terms.
*Correspondence: Yusuke Hirabayashi, aGlyYWJheWFzaGlAY2hlbWJpby50LnUtdG9reW8uYWMuanA=