- 1Xiamen Cardiovascular Hospital, Xiamen University, Xiamen, China
- 2Center for Structural Biology, School of Life Sciences and School of Medicine, Tsinghua University, Beijing, China
The evolutionarily conserved NOTCH signaling displays pleotropic functions in almost every organ system with a simple signaling axis. Different from many other signaling pathways that can be amplified via kinase cascades, NOTCH signaling does not contain any intermediate to amplify signal. Thus, NOTCH signaling can be activated at distinct signaling strength levels, disruption of which leads to various developmental disorders. Here, we reviewed mechanisms establishing different NOTCH signaling strengths, developmental processes sensitive to NOTCH signaling strength perturbation, and transcriptional regulations influenced by NOTCH signaling strength changes. We hope this could add a new layer of diversity to explain the pleotropic functions of NOTCH signaling pathway.
Introduction
Since the observation of notched wing in Drosophila and subsequent discovery of notch gene one century ago (Morgan, 1917), NOTCH signaling has been extensively studied. On/off switch of NOTCH signaling is found to play fundamental roles in cell differentiation, proliferation, and apoptosis across all species (Artavanis-Tsakonas et al., 1999; Harper et al., 2003; Lai, 2004; Lasky and Wu, 2005; Bolós et al., 2007; Penton et al., 2012). Surprisingly, the signaling axis of NOTCH is relatively simple despite its pleiotropic functions. Canonically, cell membrane-tethered NOTCH ligand binds to NOTCH receptor on the neighboring cell, which induces enzymatic cleavages of NOTCH receptor. The released notch intracellular domain (NICD) subsequently migrates into cell nucleus, where it binds with transcriptional factor CSL (CBF-1/RBP-J in mammal, Su(H) in Drosophila, and Lag-1 in Caenorhabditis elegans) together with other transcription co-factors to activate gene transcription (Kopan and Ilagan, 2009). Different from many other signaling pathways that contain kinase cascade-mediated signaling amplification processes, NOTCH signaling does not contain signaling intermediate to amplify the signal. In addition, NICD-CSL binding also triggers NICD ubiquitination that leads to its subsequent degradation (Fryer et al., 2004). Therefore, the scale and duration of gene transcription is sensitive to the dosage of NICD presented in cell nucleus.
Previous studies reviewed that protein level reduction caused by heterozygous mutation of NOTCH signaling components can lead to multiple developmental defects (Eldadah et al., 2001; McCright et al., 2002; Saito et al., 2003; Gale et al., 2004; Hozumi et al., 2004; McDaniell et al., 2006; Warthen et al., 2006; McKellar et al., 2007; Wu et al., 2007; Rubio-Aliaga et al., 2009; Hassed et al., 2012; Sargin et al., 2013; Meester et al., 2015; Southgate et al., 2015; Fischer-Zirnsak et al., 2019; Blackwood et al., 2020), suggesting developmental processes are sensitive to NOTCH signaling dosage. In addition, certain binary cell fate specifications are dependent on high/low regulation of NOTCH signaling strength instead of on/off switch of NOTCH signaling (Van de Walle et al., 2009, 2013; Gama-Norton et al., 2015), further highlighting the importance of NOTCH signaling strength regulation during development. Here, we reviewed the mechanisms of NOTCH signaling strength regulation; NOTCH components exhibiting haploinsufficiency and cell differentiation processes rely on precise NOTCH signaling strength. We hope this can add an extra layer of diversity to NOTCH signaling that plays pleotropic functions in almost every organ system with a simple signaling axis.
Notch Signaling and Its Strength Regulation
NOTCH Signaling Can Be Activated at Different Strength Levels Resulting in Distinct Transcriptional Responses
The mechanism of NOTCH signaling activation is highly conserved during evolution except for slight difference in terms of the number of NOTCH ligands and receptors across different species. In mammals, there are five NOTCH ligands (Dll-1, Dll-4, Jag-1, and Jag-2 are activators, and Dll-3 is an inhibitor) and four NOTCH receptors (Notch-1, Notch-2, Notch-3, and Notch-4), all of which contain extracellular epidermal growth factor (EGF)-like domains executing ligand–receptor binding. Subsequently after binding, NOTCH receptor undergoes two successive enzymatic cleavages mediated by ADAM10 and γ-secretase, releasing the NICD into cell nucleus where it binds with NOTCH signaling transcription factor CSL together with other co-factors to activate gene transcription (Figure 1). The most conserved direct targets of NOTCH signaling are basic helix-loop-helix (bHLH) transcription factors of hairy/enhancer of split (Hes) family and hairy/enhancer of split related with YRPW motif (Hey) family (Iso et al., 2003; Borggrefe and Oswald, 2009).
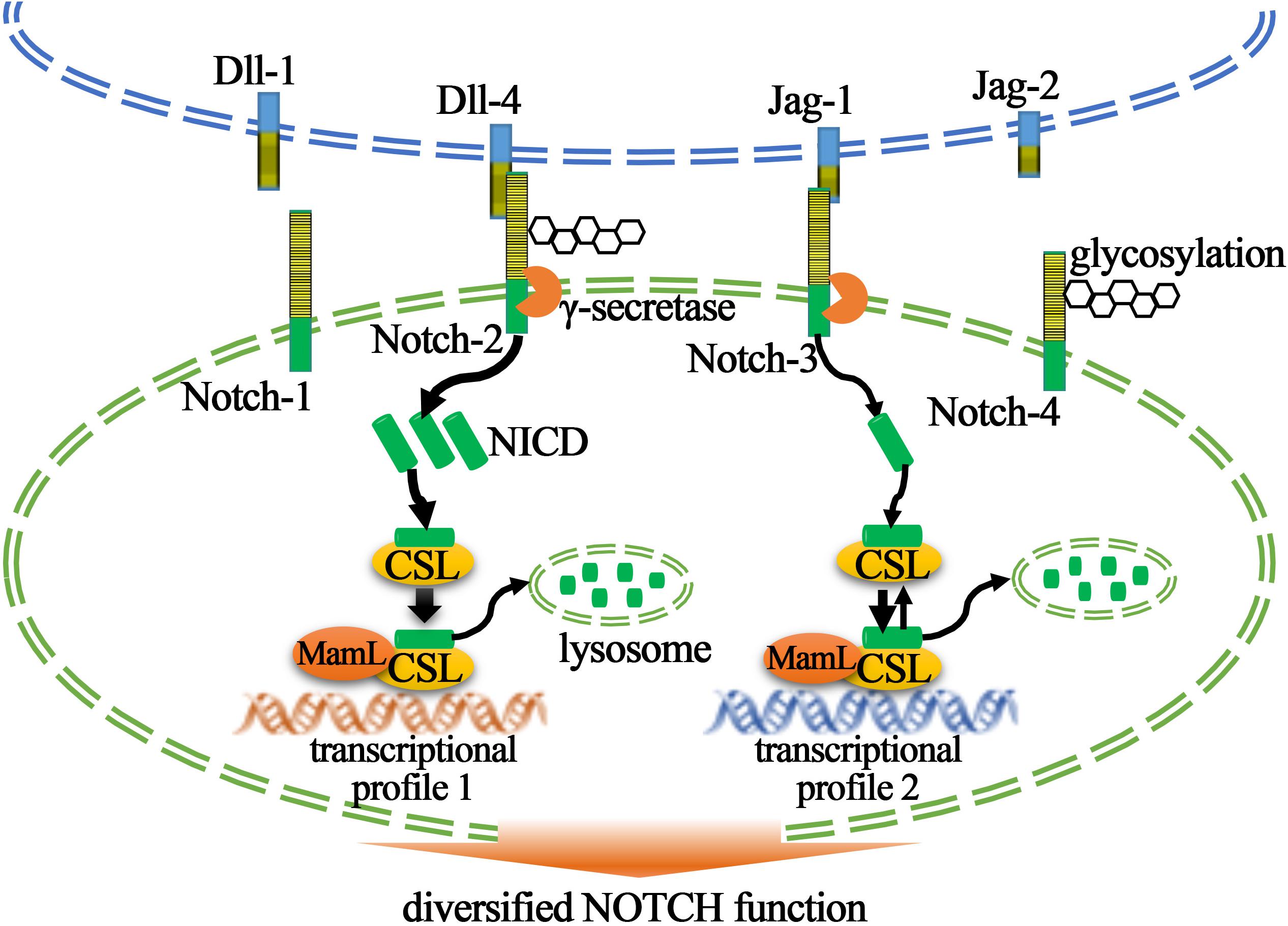
Figure 1. Cartoon illustrating NOTCH signaling and its strength regulations. Binding of NOTCH ligand (Jag-1, Jag-2, Dll-1, and Dll-4) to NOTCH receptor (Notch1, Notch-2, Notch-3, and Notch-4), a process that can be influenced by glycosylation of epidermal growth factor (EGF) domain (yellow stripes), triggers γ-secretase-mediated NOTCH receptor cleavage. Notch intracellular domain (NICD) is then freed and migrates into cell nucleus where it binds with transcriptional factor CSL, increases CSL dwell time on DNA, and recruits co-factor MamL to initiate the gene transcription. The ultimate transcription profile is affected by the dosage of NICD, which is also regulated by lysosome-mediated NICD degradation.
Unlike many other signaling pathways, NOTCH signaling can be activated at distinct strength levels due to following three reasons: (1) one NOTCH receptor can only release one NICD after ligand–receptor binding, (2) no signal intermediate or kinase cascade is involved to amplify the initial signal, and (3) NICD is subjected to proteasome-mediated degradation after transcriptional activation (Fryer et al., 2004). Since CSL and other transcriptional co-factors are always readily present in cell nucleus, the level of NICD generated determines the strength and duration of NOTCH signaling. A recent study by Nandagopal et al. (2018) showed that ligand intracellular domain (ICD) determined distinct membrane distribution pattern of Dll-4 (which is dispersed over membrane) and Dll-1 (which is clustered in puncta), which triggered sustained and pulsatile release of NICD, respectively. Interestingly, the two patterns of NICD release resulted in distinct downstream gene expression and consequently a different cell fate choice during embryonic myogenesis (Nandagopal et al., 2018). This confirmed gene transcription and cell fate specification can be influenced by NOTCH signaling strength perturbations.
Mechanisms of NOTCH Signaling Strength Regulation
The frequency of NOTCH ligand and receptor binding determines the amount of NICD generated. In order to achieve a successful binding-induced NOTCH receptor cleavage, both ligand and receptor need to be presented on cell membrane in close proximity. Since all active NOTCH ligands and receptors are trans-membrane proteins that are subjected to consistent endocytosis, recycling, and degradation, the amount of NICD that could be potentially generated is affected by endocytic regulations of the ligands and receptors (Fortini and Bilder, 2009; Kandachar and Roegiers, 2012; Shen and Sun, 2020). Meanwhile, the four active NOTCH ligands (Jag-1, Jag-2, Dll-1, and Dll-4) in mammals exhibit different binding affinities (Benedito et al., 2009; Groot et al., 2014; Gama-Norton et al., 2015; Nandagopal et al., 2018), which further diversified the levels of NOTCH signaling strength in different cell contexts. The discovery of fringe glycosyltransferase also brought up the importance of EGF domain glycosylation, which can change ligand–receptor binding affinity and facilitate NOTCH receptor cleavage (Stanley and Okajima, 2010; Takeuchi and Haltiwanger, 2010; Kakuda and Haltiwanger, 2017). Thus, NOTCH signaling strength can be influenced by glycosylation of EGF domain in receptors. In addition to glycosylation, lipid composition of cell membrane can also influence NOTCH ligand–receptor binding via lipid–ligand interactions (Suckling et al., 2017). Underlying the binding affinity differences for different ligands, catch-bond that exhibited prolonged bond lifetimes upon tensile force application is shown to play important roles on modulating ligand–receptor binding (Luca et al., 2017). Collectively, the amount of ligand and receptor presented on cell membrane, the type of ligand binding to receptor, glycosylation of EGF domain, and lipid–ligand interaction all influence ligand–receptor binding and consequently the amount of NICD released.
The stability of NICD affects the duration of NOTCH signaling. NICD is generated following ligand–receptor binding and shuttles into cell nucleus, where it binds to transcription factor CSL together with co-factor of mastermind-like protein (MamL) and other chromatin modifiers to activate gene transcription. In addition to transcriptional regulations, CSL and MamL also recruit kinase CDK8 to phosphorylate NICD, which triggers protein ubiquitination on PEST (proline, glutamic acid, serine, and threonine-enriched) domain of NICD and proteasome-mediated NICD degradation (Fryer et al., 2004). Thus, NOTCH signaling is quickly turned down without re-supply of new NICD, which is a critical step to maintain proper levels of NOTCH signaling strength. Sustained NOTCH activation due to mutations in PEST domain can disrupt cell homeostasis and lead to various diseases, such as chronic lymphocytic leukemia (CCL; Ianni et al., 2009; Puente et al., 2011), marginal zone lymphoma (Trøen et al., 2008; Kiel et al., 2012), and increased proliferation of B-cell lymphoma cell (Zhang et al., 2014). Therefore, maintaining a proper level of NOTCH signaling strength is important.
Developmental Processes Sensitive to Notch Signaling Strength Perturbation
Haploinsufficiency of NOTCH Signaling Leads to Various Developmental Disorders and Diseases
Haploinsufficiency of NOTCH signaling components, a condition caused by heterozygous mutation producing only half amount of proteins, is related to the development of Adams–Oliver syndrome (AOS) characterized by congenital defects of limbs and scalp. Notch-1 haploinsufficiency has been discovered in AOS patients with variable levels of cardiovascular anomalies, such as ventricular septal defects, aortic stenosis, regurgitation, and coarctation (Southgate et al., 2015). In line with the Notch-1 haploinsufficiency discovered in AOS patients, another NOTCH signaling component RBP-J (coding the mammalian form of CSL) is also found to be haploinsufficient in AOS patients (Hassed et al., 2012), implying a causative role of reduced NOTCH signaling strength during the development of AOS. In addition, Dll-4 is likely the NOTCH ligand responsible for generating the proper level of NOTCH signaling in this case, as AOS patients are also reported to carry Dll-4 heterozygous mutation (Meester et al., 2015). Collectively, Dll-4, Notch-1, and RBP-J haploinsufficiencies are associated with AOS.
Haploinsufficiency of NOTCH signaling components also leads to the development of Alagille syndrome (AGS) that affects multiple organs including the liver, heart, eye, kidney, and bone. AGS patients are reported to carry heterozygous mutation of Jag-1 (Warthen et al., 2006) or Notch-2 (McDaniell et al., 2006) or both (Brennan and Kesavan, 2017). Mice models with heterozygous mutations of Jag-1 and Notch-2 also successfully recapitulated certain symptoms of AGS (McCright et al., 2002), suggesting that reduction of NOTCH signaling leads to the development of AGS. Interestingly, some AGS-related defects can also be associated with NOTCH signaling components’ haploinsufficiency independent of AGS. For example, tetralogy of Fallot, a congenital heart disease frequently observed in AGS patients, can be found patients carrying Jag-1 heterozygous mutation without diagnosis of AGS (Eldadah et al., 2001; Bauer et al., 2010). Another congenital heart disease named bicuspid aortic valve, a condition lacking one valve between the left ventricle and main artery, is associated with Notch-1 haploinsufficiency and also independent of AGS (McKellar et al., 2007). Therefore, NOTCH signaling haploinsufficiency can lead to the development of AGS and multiple cardiovascular defects.
Beyond above diseases, systematic examination of mice models also showed that NOTCH signaling haploinsufficiency can disrupt the development of many organs and tissues where NOTCH signaling plays essential functions. In addition to the reported AGS symptoms (Huppert, 2016), Jag-1± mice also showed degeneration of ganglionic eminence caused by suppressed neuronal progenitor cell proliferation (Blackwood et al., 2020) and spatial memory impairment (Sargin et al., 2013). Dll-4± mice displayed various artery defects including failed remodeling of yolk sac vasculature, artery regression, artery stenosis, atresia aorta, and defected arterial branching (Gale et al., 2004), consistent with the essential function of Dll-4 during artery development (Shutter et al., 2000; Cristofaro et al., 2013). Dll-1± mice on the other hand displayed smaller bodies, reduced cholesterol and triglyceride levels, suppressed immune system, bradycardia (Rubio-Aliaga et al., 2009), and brain disorders (Fischer-Zirnsak et al., 2019). Collectively, haploinsufficiency of NOTCH signaling components can lead to various developmental disorders.
NOTCH Signaling Strength in Arterial Endothelial Cells
The change of NOTCH signaling strength influences artery endothelial cell fate (vs. hematopoietic stem cell fate) specification and artery branching (vs. elongation). During early embryonic development, progenitor cells resident in aorta-gonad-mesonephros (AGM) region can give rise to either arterial endothelial cell or hematopoietic stem cell (HSC) depending on NOTCH signaling strength. In vivo study found that Dll-4 activated high NOTCH signaling while Jag-1 activated low NOTCH signaling in AGM region, where high NOTCH signaling specifies endothelial cell fates while low NOTCH signaling specifies HSC fates. Neutralization of Dll-4 via antibody blockage lowered NOTCH activity and forced AGM cells to differentiate into HSCs, suggesting a NOTCH-strength-dependent cell fate specification (Gama-Norton et al., 2015). In addition, NOTCH signaling strength also influences branching of the artery during the generation of vascular network. New tip cell specification, a requirement for artery branching, is inhibited by Dll-4-activated high NOTCH signaling while requires Jag-1-activated low NOTCH signaling. Jag-1 mutated mice, in which Dll-4 dominated in the artery and activated high NOTCH signaling, exhibited significantly reduced vascular branching. In contrast, overexpression of Jag-1 outcompeted Dll-4 and significantly promoted vascular branching. During the establishment of this signaling strength difference, fringe-mediated glycosylation of NOTCH played a critical role on potentiating Dll-4-activated high NOTCH signaling and suppressing Jag-1-mediated low NOTCH signaling. Removing fringe suppressed Dll-4-activated high NOTCH signaling and permitted Jag-1-activated low NOTCH signaling, resulting in enhanced vascular branching (Benedito et al., 2009).
Further study showed that the developmental difference resulting from Dll-4- and Jag-1-activated NOTCH signaling during vascular branching was purely due to the strength difference of NOTCH signaling. Administration of γ-secretase inhibitor (DAPT) into Jag-1-mutated mice lowered Dll-4-activated NOTCH signaling and rescued vascular branching defects (Benedito et al., 2009). Therefore, it is the strength of NOTCH signaling that determines the artery branching. Utilizing hybrid proteins created by swapping the ICD of Jag-1 and Dll-4 found that ICDs of NOTCH ligands determine the potential of NOTCH signaling strength that could be activated by Jag-1 or Dll-4. Cytoskeletal filament vimentin specifically binds to Jag-1 ICD and determines the pulling force critical for binding-induced NOTCH receptor cleavage. Hybrid protein fused by Jag1 ICD and Dll-4 extracellular domain (ECD) generated NOTCH signaling resembling ligand Jag-1 (Antfolk et al., 2017). Collectively, different NOTCH ligands hold distinct capacities to activate NOTCH signaling, and ligand ICDs play fundamental roles on influencing the activation potential.
NOTCH Signaling Strength in Vascular Smooth Muscle Cell
NOTCH signaling is required for vascular smooth muscle cell (VSMC) differentiation. VSMCs are recruited to vascular endothelial cells during vasculogenesis and play crucial roles on maintaining normal vascular tone in response to hemodynamic changes, especially in arteries where multiple layers of VSMCs are attached to endothelial cells (Zhuge et al., 2020). During vasculogenesis, VSMC progenitors are recruited to vascular bed where endothelial cell expresses Jag-1-activated NOTCH signaling in VSMC progenitor cells, resulting in expression of smooth cell markers of α-SMA and SM-22α and the final specification of VSMC fate (Noseda et al., 2006; High et al., 2008). Meanwhile, the activated NOTCH signaling in VSMCs also directly activated Jag-1 expression in the newly formed VSMCs, allowing further propagation of NOTCH signaling in the outer layers of VSMCs (Hoglund and Majesky, 2012; Manderfield et al., 2012; van Engeland et al., 2019). Therefore, Jag-1-activated NOTCH signaling is essential to maintain VSMC fate and form a multiple-layer structure of VSMCs in the artery.
Elevated NOTCH signaling strength triggered the proliferation of VSMCs. Unlike skeletal muscle cells or cardiomyocytes, both of which are terminally differentiated and quiescent, VSMCs holds the ability to proliferate, dedifferentiate, and even transdifferentiate into macrophage-like cells in response to vascular injury or environmental stimulus (Bennett et al., 2016; Basatemur et al., 2019; Zhuge et al., 2020). The elevated NOTCH signaling has been observed in arterial injury (Wang et al., 2002) and atherosclerotic lesions (Davis-Knowlton et al., 2019), both of which involve VSMC proliferation. An in vitro study also showed that over-expression of NICD in VSMCs can increase cell proliferation (Sweeney et al., 2004), which seems contradictory to the fact that NOTCH signaling promotes the quiescent status of VSMCs characterized by the expression of α-SMA. However, NICD over-expression generally resulted in sustained high NOTCH signaling, and detailed in vitro studies clarified that NOTCH downstream targets of Hey are promoted under this condition and are responsible for increased VSMC proliferation and suppressed α-SMA expression via negative feedback loops. Specifically, the increased Hey, a transcriptional repressor, can in term prevent the transcription of α-SMA through directly binding to α-SMA promoter (Tang et al., 2008). Meanwhile, Hey also inhibits the expression of cyclin-dependent kinase inhibitor P27kip1, allowing re-entering into the cell cycle (Havrda et al., 2006). Conclusively, NOTCH signaling is required for expression of VSMC markers to maintain VSMC fate, while elevated NOTCH signaling can suppress VSMC marker expression and promote VSMC proliferation.
NOTCH Signaling Strength in T-Cell Lineage
NOTCH signaling strength determines αβ T-cell (vs. γδ T-cell) specification in T-cell lineage. Postnatal development of T immune cell in the thymus requires activation of NOTCH signaling in the hematopoietic progenitor cells (HPCs) that migrated from the bone marrow. NOTCH signaling activation inhibits non-T-cell cells including myeloid lineage during early stages and B-cell during late stages (Wilson et al., 2001). However, after T-cell fate is committed, the strength of NOTCH in T-cell lineage determines T-cell sub-lineage specifications between αβ T-cell and γδ T-cell. An in vitro study showed that human OP9-Dll1/4 that served as NOTCH signal-sending cell can stimulate the differentiation of human HPCs into T-cells populated with both αβ T-cell and γδ T-cell. Interestingly, lowering NOTCH signaling strength via adding a series of γ-secretase inhibitor (DAPT) with increasing concentrations gradually switched γδ T-cell into αβ T-cell (Van de Walle et al., 2009), documenting NOTCH-strength-dependent cell fate determination between the two T-cell subtypes.
The strength changes of NOTCH signaling in T-cell lineage is caused by binding with different NOTCH ligands that hold distinct receptor binding affinities. Still in human HPCs, Jag-2 exhibited strong NOTCH activation potential and directed HPCs predominantly into γδ T-cell (Van de Walle et al., 2011, 2013); Dll-4 instead induced a relatively weak NOTCH signaling and generated both γδ T-cell and αβ T-cell, while Jag-1 induced the weakest NOTCH signaling and generated mainly αβ T-cell (Van de Walle et al., 2013). Collectively, the diverted expression of NOTCH ligands maintained a diverse range of NOTCH signaling strength, which balanced the population of αβ T-cell and γδ T-cell. Surprisingly, mice HPCs also utilize the strength difference of NOTCH signaling to determine the αβ T-cell fate and γδ T-cell fate but in an opposite way that low NOTCH signaling favors γδ T-cell (Washburn et al., 1997). It is intriguing how this difference between human and mouse is generated, while the NOTCH-strength-dependent cell fate determination is indeed conserved during evolution.
NOTCH Signaling Strength in Marginal Zone B Cell
Differentiation of marginal zone B (MZB) cell, an immune cell developed in marginal zone of the spleen, relies on NOTCH signaling dosage. Notch-2 is preferentially expressed in B-cell and is prominent in splenic marginal zone, suggesting its potential function on MZB cell differentiation. Homozygous mutation of Notch-2 completely eliminated MZB cells. Interestingly, heterozygous mutation of Notch-2, in which 50% of Notch-2 mRNA still expressed, resulted in partial reduction of MZB cells (Saito et al., 2003). Consistent with Notch-2 mutant, mutational study of MamL-1 (transcriptional co-factor of CSL) showed a similar dose-dependent regulation of MZB cell differentiation. Wild-type, heterozygous mutation of MamL-1 and homozygous mutation of MamL-1 showed sequential reduction of MZB cell number (Wu et al., 2007), suggesting all these defects are due to reduction of NOTCH signaling strength.
Other studies on NOTCH ligands found that it is the ligand of Dll-1 that activates Notch-2 for MZB cell differentiation in the spleen. Mutational study of Dll-1 similarly showed a sequential reduction of MZB cell among wild-type, heterozygous mutation of Dll-1 and homozygous mutation of Dll-1 (Hozumi et al., 2004). A later study in splenic stromal cells further confirmed that Dll-1 expressed by these cells are responsible for activating NOTCH signaling required for MZB cell differentiation (Fasnacht et al., 2014). Collectively, these comparison studies on heterozygous mutation of Notch-2, MamL-1, and Dll-1 suggest that MZB cell differentiation is dependent on NOTCH dosage. MZB cell differentiation likely requires NOTCH signaling to be above certain threshold. Haploinsufficiency of these NOTCH signaling components made many cells fail to reach this threshold and resulted in big reduction of mature MZB cells.
NOTCH Signaling Strength in Pancreatic Progenitor Cells
Stepwise downregulation of NOTCH signaling strength in pancreatic endocrine progenitor cells drives the transition from quiescence to proliferation and from proliferation to differentiation. NOTCH signaling is well-known for its function on maintaining pancreatic progenitor cells, and suppressing NOTCH signaling triggered the progenitor cells to differentiate into pancreatic secreting cells (Apelqvist et al., 1999). Interestingly, lineage tracing observation of pancreas development in zebrafish discovered a stepwise downregulation of NOTCH signaling strength in quiescent endocrine progenitor cells, proliferating endocrine progenitor cells and differentiated mature endocrine cells. Lowering NOTCH signaling strength via applying low concentration of γ-secretase inhibitor (DAPT) to the developing pancreas promoted progenitor cell proliferation and consequently expanded pancreatic endocrine progenitor pool. However, saturated DAPT led to differentiation of pancreatic secreting cells accompanied by drastically reduced progenitor pool (Ninov et al., 2012), confirming moderate NOTCH signaling strength is required for the proliferation of pancreatic endocrine progenitor cells.
Conclusively, high NOTCH signaling strength maintains quiescent state of pancreatic endocrine progenitor cell, moderate NOTCH signaling strength triggers its proliferation, and low NOTCH signaling strength leads to its final differentiation. Combining NOTCH haploinsufficiency-related disorders, precise strength requirements in artery endothelial cell, VSMC, T-cell, and MZB cell, developmental processes can be sensitive to NOTCH signaling strength perturbations (summarized in Table 1). Therefore, re-examining NOTCH-regulated processes with consideration of signaling strength would likely offer new insights to explain the pleotropic functions of NOTCH.
Mechanisms Underlying Distinct Transcriptional Activation by Different Strengths of Notch Signaling
Different duration and dynamics of NOTCH signaling strengths yield distinct transcriptional responses, which resulted in distinct developmental consequences. A new study reported by Nandagopal et al. (2018) showed that the conserved NOTCH direct target genes of Hes1 and Hey1/L responded differently to the level changes of NICD, the active component of NOTCH signaling. Interestingly, Hes1 and Hey1/L did not simply adjust their expression levels in proportion to the amount of NICD generated. High and sustained NOTCH activation induced by Dll-4 initiated Hey1/L transcription compared to pulsatile NOTCH activation induced by Dll-1, instead of proportionally increasing Hes1 that was already transcribed under pulsatile NOTCH activation (Nandagopal et al., 2018). Considering NICD pattern change alone can initiate new gene expression, and it is likely that level changes of NICD would mediate chromatin modifications for gene transcription.
Dosage change of NICD can influence CSL-DNA binding kinetics, NICD dimerization, and chromatin opening. A recent study using live cell imaging changed the classical model that CSL stays on chromatin to repress gene transcription until the arrival of NICD that switches CSL into a transcriptional activator (Morel et al., 2001; Borggrefe and Oswald, 2009). Instead, CSL consistently binds to and detaches from DNA, and NICD along or together with co-factor of MamL can change the binding dynamics of CSL onto DNA by increasing its chromatin dwell time and potentially the binding loci (Gomez-Lamarca et al., 2018). NICD dimerization, utilizing two NICDs for gene transcription, is essential for certain NOTCH downstream gene expression. Suppressing of NICD dimerization leads to various developmental disorders including heart anomalies and defective MZB cell differentiation; therefore, shifted balance between dimerized NICD and monomer NICD that can be influenced by NICD dosage is going to affect transcriptional profile as well (Hass et al., 2015; Kobia et al., 2020). The changing of gene transcription under different NICD dosages can be further proven by the observation that NICD can change chromatin structure (Gomez-Lamarca et al., 2018), opening of which permits more gene transcription. Collectively, NICD not only switches CSL into transcription activator but also modulates the dynamics of CSL-DNA binding, NICD dimerization, and chromatin structure, thus affecting gene transcription both qualitatively and quantitatively.
Conclusion
The on/off switch of NOTCH signaling is well-recognized for its function on regulating cell differentiation, proliferation, and apoptosis. The pleotropic function of NOTCH signaling seems contradictory to the simple setting of NOTCH signaling axis. Genetic studies discovered heterozygous mutations of NOTCH signaling components in various developmental disorders and diseases, suggesting that organ development is also sensitive to NOTCH signaling dosage. More importantly, simple changes of NOTCH signaling strength influences the binary cell fate determinations and cell proliferation and differentiation during artery, postnatal T-cell, MZB cell, and pancreas development, suggesting that NOTCH signaling strength changes can be as important as on/off switch of the signaling. Underlying NOTCH signaling strength changes and NICD–CSL complex-mediated gene transcriptions are changed both quantitatively and qualitatively to direct distinct cellular responses. Therefore, NOTCH signaling strength can add a new layer of diversity to explain the pleotropic functions of NOTCH.
Author Contributions
WS and YW conceived the study. All authors contributed their expertise in NOTCH signaling, epigenetic regulation, and cardiovascular development, respectively and contributed to the finalization of this manuscript significantly.
Funding
This work was supported by Xiamen Cardiovascular Hospital.
Conflict of Interest
The authors declare that the research was conducted in the absence of any commercial or financial relationships that could be construed as a potential conflict of interest.
Acknowledgments
We thank Dr. Jun Li from Shanghai Jiao Tong University for his discussions on this topic.
References
Antfolk, D., Sjöqvist, M., Cheng, F., Isoniemi, K., Duran, C. L., Rivero-Muller, A., et al. (2017). Selective regulation of Notch ligands during angiogenesis is mediated by vimentin. PNAS 114, E4574–E4581. doi: 10.1073/pnas.1703057114
Apelqvist, A., Li, H., Sommer, L., Beatus, P., Anderson, D. J., Honjo, T., et al. (1999). Notch signalling controls pancreatic cell differentiation. Nature 400, 877–881. doi: 10.1038/23716
Artavanis-Tsakonas, S., Rand, M. D., and Lake, R. J. (1999). Notch signaling: cell fate control and signal integration in development. Science 284, 770–776.
Basatemur, G. L., Jørgensen, H. F., Clarke, M. C. H., Bennett, M. R., and Mallat, Z. (2019). Vascular smooth muscle cells in atherosclerosis. Nat. Rev. Cardiol. 16, 727–744. doi: 10.1038/s41569-019-0227-9
Bauer, R. C., Laney, A. O., Smith, R., Gerfen, J., Morrissette, J. J. D., Woyciechowski, S., et al. (2010). Jagged1 Mutations in Patients with Tetralogy of Fallot or Pulmonic Stenosis. Hum. Mutat. 31, 594–601. doi: 10.1002/humu.21231
Benedito, R., Roca, C., Sörensen, I., Adams, S., Gossler, A., Fruttiger, M., et al. (2009). The notch ligands Dll4 and Jagged1 have opposing effects on angiogenesis. Cell 137, 1124–1135. doi: 10.1016/j.cell.2009.03.025
Bennett, M. R., Sinha, S., and Owens, G. K. (2016). Vascular smooth muscle cells in atherosclerosis. Circ. Res. 118, 692–702. doi: 10.1161/CIRCRESAHA.115.306361
Blackwood, C. A., Bailetti, A., Nandi, S., Gridley, T., and Hébert, J. M. (2020). Notch Dosage: Jagged1 Haploinsufficiency Is Associated With Reduced Neuronal Division and Disruption of Periglomerular Interneurons in Mice. Front. Cell Dev. Biol. 8:00113. doi: 10.3389/fcell.2020.00113
Bolós, V., Grego-Bessa, J., and de la Pompa, J. L. (2007). Notch Signaling in Development and Cancer. Endocr. Rev. 28, 339–363. doi: 10.1210/er.2006-0046
Borggrefe, T., and Oswald, F. (2009). The Notch signaling pathway: transcriptional regulation at Notch target genes. Cell Mol. Life Sci. 66, 1631–1646. doi: 10.1007/s00018-009-8668-7
Brennan, A., and Kesavan, A. (2017). Novel Heterozygous Mutations in JAG1 and NOTCH2 Genes in a Neonatal Patient with Alagille Syndrome. Case Rep. Pediatr. 2017:1368189. doi: 10.1155/2017/1368189
Cristofaro, B., Shi, Y., Faria, M., Suchting, S., Leroyer, A. S., Trindade, A., et al. (2013). Dll4-Notch signaling determines the formation of native arterial collateral networks and arterial function in mouse ischemia models. Development 140, 1720–1729. doi: 10.1242/dev.092304
Davis-Knowlton, J., Turner, J. E., Turner, A., Damian-Loring, S., Hagler, N., Henderson, T., et al. (2019). Characterization of smooth muscle cells from human atherosclerotic lesions and their responses to Notch signaling. Lab. Investig. 99, 290–304. doi: 10.1038/s41374-018-0072-1
Eldadah, Z. A., Hamosh, A., Biery, N. J., Montgomery, R. A., Duke, M., Elkins, R., et al. (2001). Familial Tetralogy of Fallot caused by mutation in the jagged1 gene. Hum. Mol. Genet. 10, 163–169. doi: 10.1093/hmg/10.2.163
Fasnacht, N., Huang, H.-Y., Koch, U., Favre, S., Auderset, F., Chai, Q., et al. (2014). Specific fibroblastic niches in secondary lymphoid organs orchestrate distinct Notch-regulated immune responses. J. Exp. Med. 211, 2265–2279. doi: 10.1084/jem.20132528
Fischer-Zirnsak, B., Segebrecht, L., Schubach, M., Charles, P., Alderman, E., Brown, K., et al. (2019). Haploinsufficiency of the Notch Ligand DLL1 Causes Variable Neurodevelopmental Disorders. Am. J. Hum. Genet. 105, 631–639. doi: 10.1016/j.ajhg.2019.07.002
Fortini, M. E., and Bilder, D. (2009). Endocytic regulation of Notch signaling. Curr. Opin. Genet. Dev. 19, 323–328. doi: 10.1016/j.gde.2009.04.005
Fryer, C. J., White, J. B., and Jones, K. A. (2004). Mastermind recruits CycC:CDK8 to phosphorylate the Notch ICD and coordinate activation with turnover. Mol. Cell 16, 509–520. doi: 10.1016/j.molcel.2004.10.014
Gale, N. W., Dominguez, M. G., Noguera, I., Pan, L., Hughes, V., Valenzuela, D. M., et al. (2004). Haploinsufficiency of delta-like 4 ligand results in embryonic lethality due to major defects in arterial and vascular development. PNAS 101, 15949–15954. doi: 10.1073/pnas.0407290101
Gama-Norton, L., Ferrando, E., Ruiz-Herguido, C., Liu, Z., Liu, Z., Guiu, J., et al. (2015). Notch signal strength controls cell fate in the haemogenic endothelium. Nat. Commun. 6:8510. doi: 10.1038/ncomms9510
Gomez-Lamarca, M. J., Falo-Sanjuan, J., Stojnic, R., Abdul Rehman, S., Muresan, L., Jones, M. L., et al. (2018). Activation of the Notch Signaling Pathway In Vivo Elicits Changes in CSL Nuclear Dynamics. Dev. Cell 44, 611.e–623.e. doi: 10.1016/j.devcel.2018.01.020
Groot, A. J., Habets, R., Yahyanejad, S., Hodin, C. M., Reiss, K., Saftig, P., et al. (2014). Regulated Proteolysis of NOTCH2 and NOTCH3 Receptors by ADAM10 and Presenilins. Mole. Cell. Biol. 34, 2822–2832. doi: 10.1128/MCB.00206-14
Harper, J. A., Yuan, J. S., Tan, J. B., Visan, I., and Guidos, C. J. (2003). Notch signaling in development and disease. Clin. Genet. 64, 461–472.
Hass, M. R., Liow, H., Chen, X., Sharma, A., Inoue, Y. U., Inoue, T., et al. (2015). SpDamID: Marking DNA Bound by Protein Complexes Identifies Notch-Dimer Responsive Enhancers. Mol. Cell 59, 685–697. doi: 10.1016/j.molcel.2015.07.008
Hassed, S. J., Wiley, G. B., Wang, S., Lee, J.-Y., Li, S., Xu, W., et al. (2012). RBPJ Mutations Identified in Two Families Affected by Adams-Oliver Syndrome. Am. J. Hum. Genet. 91, 391–395. doi: 10.1016/j.ajhg.2012.07.005
Havrda, M. C., Johnson, M. J., O’Neill, C. F., and Liaw, L. (2006). A novel mechanism of transcriptional repression of p27kip1 through Notch/HRT2 signaling in vascular smooth muscle cells. Thromb. Haemost. 96, 361–370. doi: 10.1160/TH06-04-0224
High, F. A., Lu, M. M., Pear, W. S., Loomes, K. M., Kaestner, K. H., and Epstein, J. A. (2008). Endothelial expression of the Notch ligand Jagged1 is required for vascular smooth muscle development. Proc. Natl. Acad. Sci. U S A 105, 1955–1959. doi: 10.1073/pnas.0709663105
Hoglund, V. J., and Majesky, M. W. (2012). Patterning the artery wall by lateral induction of Notch signaling. Circulation 125, 212–215. doi: 10.1161/CIRCULATIONAHA.111.075937
Hozumi, K., Negishi, N., Suzuki, D., Abe, N., Sotomaru, Y., Tamaoki, N., et al. (2004). Delta-like 1 is necessary for the generation of marginal zone B cells but not T cells in vivo. Nat. Immunol. 5, 638–644. doi: 10.1038/ni1075
Huppert, S. S. (2016). A faithful JAGGED1 haploinsufficiency mouse model of arteriohepatic dysplasia (Alagille syndrome) after all. Hepatology 63, 365–367. doi: 10.1002/hep.28338
Ianni, M. D., Baldoni, S., Rosati, E., Ciurnelli, R., Cavalli, L., Martelli, M. F., et al. (2009). A new genetic lesion in B-CLL: a NOTCH1 PEST domain mutation. Br. J. Haematol. 146, 689–691. doi: 10.1111/j.1365-2141.2009.07816.x
Iso, T., Kedes, L., and Hamamori, Y. (2003). HES and HERP families: Multiple effectors of the notch signaling pathway. J. Cell. Physiol. 194, 237–255. doi: 10.1002/jcp.10208
Kakuda, S., and Haltiwanger, R. S. (2017). Deciphering the Fringe-Mediated Notch Code: Identification of Activating and Inhibiting Sites Allowing Discrimination between Ligands. Dev. Cell 40, 193–201. doi: 10.1016/j.devcel.2016.12.013
Kandachar, V., and Roegiers, F. (2012). Endocytosis and control of Notch signaling. Curr. Opin. Cell Biol. 24, 534–540. doi: 10.1016/j.ceb.2012.06.006
Kiel, M. J., Velusamy, T., Betz, B. L., Zhao, L., Weigelin, H. G., Chiang, M. Y., et al. (2012). Whole-genome sequencing identifies recurrent somatic NOTCH2 mutations in splenic marginal zone lymphoma. J. Exp. Med. 209, 1553–1565. doi: 10.1084/jem.20120910
Kobia, F. M., Preusse, K., Dai, Q., Weaver, N., Hass, M. R., Chaturvedi, P., et al. (2020). Notch dimerization and gene dosage are important for normal heart development, intestinal stem cell maintenance, and splenic marginal zone B-cell homeostasis during mite infestation. PLoS Biol. 18:e3000850. doi: 10.1371/journal.pbio.3000850
Kopan, R., and Ilagan, M. A. (2009). The Canonical Notch Signaling Pathway: Unfolding the Activation Mechanism. Cell 137, 216–233. doi: 10.1016/j.cell.2009.03.045
Lai, E. C. (2004). Notch signaling: control of cell communication and cell fate. Development 131, 965–973. doi: 10.1242/dev.01074
Lasky, J. L., and Wu, H. (2005). Notch Signaling, Brain Development, and Human Disease. Pediatr. Res. 57, 104R–109R. doi: 10.1203/01.PDR.0000159632.70510.3D
Luca, V. C., Kim, B. C., Ge, C., Kakuda, S., Wu, D., Roein-Peikar, M., et al. (2017). Notch-Jagged complex structure implicates a catch bond in tuning ligand sensitivity. Science 355, 1320–1324. doi: 10.1126/science.aaf9739
Manderfield, L. J., High, F. A., Engleka, K. A., Liu, F., Li, L., Rentschler, S., et al. (2012). Notch activation of Jagged1 contributes to the assembly of the arterial wall. Circulation 125, 314–323. doi: 10.1161/CIRCULATIONAHA.111.047159
McCright, B., Lozier, J., and Gridley, T. (2002). A mouse model of Alagille syndrome: Notch2 as a genetic modifier of Jag1 haploinsufficiency. Dev. 129, 1075–1082.
McDaniell, R., Warthen, D. M., Sanchez-Lara, P. A., Pai, A., Krantz, I. D., Piccoli, D. A., et al. (2006). NOTCH2 mutations cause Alagille syndrome, a heterogeneous disorder of the notch signaling pathway. Am. J. Hum. Genet. 79, 169–173. doi: 10.1086/505332
McKellar, S. H., Tester, D. J., Yagubyan, M., Majumdar, R., Ackerman, M. J., and Sundt, T. M. (2007). Novel NOTCH1 mutations in patients with bicuspid aortic valve disease and thoracic aortic aneurysms. J. Thorac. Cardiovasc. Surg. 134, 290–296. doi: 10.1016/j.jtcvs.2007.02.041
Meester, J. A. N., Southgate, L., Stittrich, A.-B., Venselaar, H., Beekmans, S. J. A., den Hollander, N., et al. (2015). Heterozygous Loss-of-Function Mutations in DLL4 Cause Adams-Oliver Syndrome. Am. J. Hum. Genet. 97, 475–482. doi: 10.1016/j.ajhg.2015.07.015
Morel, V., Lecourtois, M., Massiani, O., Maier, D., Preiss, A., and Schweisguth, F. (2001). Transcriptional repression by suppressor of hairless involves the binding of a hairless-dCtBP complex in Drosophila. Curr. Biol. 11, 789–792. doi: 10.1016/s0960-9822(01)00224-x
Nandagopal, N., Santat, L. A., LeBon, L., Sprinzak, D., Bronner, M. E., and Elowitz, M. B. (2018). Dynamic Ligand Discrimination in the Notch Signaling Pathway. Cell 172, 869.e–880.e. doi: 10.1016/j.cell.2018.01.002
Ninov, N., Borius, M., and Stainier, D. Y. R. (2012). Different levels of Notch signaling regulate quiescence, renewal and differentiation in pancreatic endocrine progenitors. Development 139, 1557–1567. doi: 10.1242/dev.076000
Noseda, M., Fu, Y., Niessen, K., Wong, F., Chang, L., McLean, G., et al. (2006). Smooth Muscle alpha-actin is a direct target of Notch/CSL. Circ. Res. 98, 1468–1470. doi: 10.1161/01.RES.0000229683.81357.26
Penton, A. L., Leonard, L. D., and Spinner, N. B. (2012). Notch signaling in human development and disease. Semin. Cell Dev. Biol. 23, 450–457. doi: 10.1016/j.semcdb.2012.01.010
Puente, X. S., Pinyol, M., Quesada, V., Conde, L., Ordóñez, G. R., Villamor, N., et al. (2011). Whole-genome sequencing identifies recurrent mutations in chronic lymphocytic leukaemia. Nature 475, 101–105. doi: 10.1038/nature10113
Rubio-Aliaga, I., Przemeck, G. K. H., Fuchs, H., Gailus-Durner, V., Adler, T., Hans, W., et al. (2009). Dll1 Haploinsufficiency in Adult Mice Leads to a Complex Phenotype Affecting Metabolic and Immunological Processes. PLoS One 4:e6054. doi: 10.1371/journal.pone.0006054
Saito, T., Chiba, S., Ichikawa, M., Kunisato, A., Asai, T., Shimizu, K., et al. (2003). Notch2 Is Preferentially Expressed in Mature B Cells and Indispensable for Marginal Zone B Lineage Development. Immunity 18, 675–685. doi: 10.1016/S1074-7613(03)00111-0
Sargin, D., Botly, L. C. P., Higgs, G., Marsolais, A., Frankland, P. W., Egan, S. E., et al. (2013). Disrupting Jagged1-Notch signaling impairs spatial memory formation in adult mice. Neurobiol. Learn. Mem. 103, 39–49. doi: 10.1016/j.nlm.2013.03.001
Shen, W., and Sun, J. (2020). Different modes of Notch activation and strength regulation in the spermathecal secretory lineage. Development 2020:184390. doi: 10.1242/dev.184390
Shutter, J. R., Scully, S., Fan, W., Richards, W. G., Kitajewski, J., Deblandre, G. A., et al. (2000). Dll4, a novel Notch ligand expressed in arterial endothelium. Genes Dev. 14, 1313–1318. doi: 10.1101/gad.14.11.1313
Southgate, L., Sukalo, M., Karountzos, A. S. V., Taylor, E. J., Collinson, C. S., Ruddy, D., et al. (2015). Haploinsufficiency of the NOTCH1 Receptor as a Cause of Adams–Oliver Syndrome With Variable Cardiac Anomalies. Circulation 8, 572–581. doi: 10.1161/CIRCGENETICS.115.001086
Stanley, P., and Okajima, T. (2010). Roles of glycosylation in Notch signaling. Curr. Top Dev. Biol. 92, 131–164. doi: 10.1016/S0070-2153(10)92004-8
Suckling, R. J., Korona, B., Whiteman, P., Chillakuri, C., Holt, L., Handford, P. A., et al. (2017). Structural and functional dissection of the interplay between lipid and Notch binding by human Notch ligands. EMBO J. 36, 2204–2215. doi: 10.15252/embj.201796632
Sweeney, C., Morrow, D., Birney, Y. A., Coyle, S., Hennessy, C., Scheller, A., et al. (2004). Notch 1 and 3 receptors modulate vascular smooth muscle cell growth, apoptosis and migration via a CBF-1/RBP-Jk dependent pathway. FASEB J. 18, 1421–1423. doi: 10.1096/fj.04-1700fje
Takeuchi, H., and Haltiwanger, R. S. (2010). Role of Glycosylation of Notch in Development. Semin. Cell Dev. Biol. 21:638. doi: 10.1016/j.semcdb.2010.03.003
Tang, Y., Urs, S., and Liaw, L. (2008). Hairy-Related Transcription Factors Inhibit Notch-Induced Smooth Muscle α-Actin Expression by Interfering With Notch Intracellular Domain/CBF-1 Complex Interaction With the CBF-1–Binding Site. Circulat. Res. 102, 661–668. doi: 10.1161/CIRCRESAHA.107.165134
Trøen, G., Wlodarska, I., Warsame, A., Llodrà, S. H., Wolf-Peeters, C. D., and Delabie, J. (2008). NOTCH2 mutations in marginal zone lymphoma. Haematologica 93, 1107–1109. doi: 10.3324/haematol.11635
Van de Walle, I., De Smet, G., De Smedt, M., Vandekerckhove, B., Leclercq, G., Plum, J., et al. (2009). An early decrease in Notch activation is required for human TCR-αβ lineage differentiation at the expense of TCR-γδ T cells. Blood 113, 2988–2998. doi: 10.1182/blood-2008-06-164871
Van de Walle, I., De Smet, G., Gärtner, M., De Smedt, M., Waegemans, E., Vandekerckhove, B., et al. (2011). Jagged2 acts as a Delta-like Notch ligand during early hematopoietic cell fate decisions. Blood 117, 4449–4459. doi: 10.1182/blood-2010-06-290049
Van de Walle, I., Waegemans, E., De Medts, J., De Smet, G., De Smedt, M., Snauwaert, S., et al. (2013). Specific Notch receptor-ligand interactions control human TCR-αβ/γδ development by inducing differential Notch signal strength. J. Exp. Med. 210, 683–697. doi: 10.1084/jem.20121798
van Engeland, N. C. A., Suarez Rodriguez, F., Rivero-Müller, A., Ristori, T., Duran, C. L., Stassen, O. M. J. A., et al. (2019). Vimentin regulates Notch signaling strength and arterial remodeling in response to hemodynamic stress. Sci. Rep. 9:12415. doi: 10.1038/s41598-019-48218-w
Wang, W., Campos, A. H., Prince, C. Z., Mou, Y., and Pollman, M. J. (2002). Coordinate Notch3-hairy-related transcription factor pathway regulation in response to arterial injury. Mediator role of platelet-derived growth factor and ERK. J. Biol. Chem. 277, 23165–23171. doi: 10.1074/jbc.M201409200
Warthen, D. M., Moore, E. C., Kamath, B. M., Morrissette, J. J. D., Sanchez-Lara, P. A., Sanchez, P., et al. (2006). Jagged1 (JAG1) mutations in Alagille syndrome: increasing the mutation detection rate. Hum. Mutat. 27, 436–443. doi: 10.1002/humu.20310
Washburn, T., Schweighoffer, E., Gridley, T., Chang, D., Fowlkes, B. J., Cado, D., et al. (1997). Notch activity influences the alphabeta versus gammadelta T cell lineage decision. Cell 88, 833–843. doi: 10.1016/s0092-8674(00)81929-7
Wilson, A., MacDonald, H. R., and Radtke, F. (2001). Notch 1-deficient common lymphoid precursors adopt a B cell fate in the thymus. J. Exp. Med. 194, 1003–1012. doi: 10.1084/jem.194.7.1003
Wu, L., Maillard, I., Nakamura, M., Pear, W. S., and Griffin, J. D. (2007). The transcriptional coactivator Maml1 is required for Notch2-mediated marginal zone B-cell development. Blood 110, 3618–3623. doi: 10.1182/blood-2007-06-097030
Zhang, X., Shi, Y., Weng, Y., Lai, Q., Luo, T., Zhao, J., et al. (2014). The Truncate Mutation of Notch2 Enhances Cell Proliferation through Activating the NF-κB Signal Pathway in the Diffuse Large B-Cell Lymphomas. PLoS One 9:e108747. doi: 10.1371/journal.pone.0108747
Keywords: Notch signaling, NICD, signaling strength, cell fate specification, development
Citation: Shen W, Huang J and Wang Y (2021) Biological Significance of NOTCH Signaling Strength. Front. Cell Dev. Biol. 9:652273. doi: 10.3389/fcell.2021.652273
Received: 12 January 2021; Accepted: 23 February 2021;
Published: 26 March 2021.
Edited by:
Juan Jose Sanz-Ezquerro, Centro Nacional de Biotecnología, Consejo Superior de Investigaciones Científicas (CSIC), SpainReviewed by:
Ivan Maillard, University of Pennsylvania, United StatesLuis Luna-Zurita, Spanish National Centre for Cardiovascular Research, Spain
Copyright © 2021 Shen, Huang and Wang. This is an open-access article distributed under the terms of the Creative Commons Attribution License (CC BY). The use, distribution or reproduction in other forums is permitted, provided the original author(s) and the copyright owner(s) are credited and that the original publication in this journal is cited, in accordance with accepted academic practice. No use, distribution or reproduction is permitted which does not comply with these terms.
*Correspondence: Wei Shen, c2hlbndlaUB4bWhlYXJ0LmNvbQ==; Yan Wang, d3lAbWVkbWFpbC5jb20uY24=