- 1Department of Cardiology, West China Hospital, Sichuan University, Chengdu, China
- 2Laboratory of Cardiovascular Diseases, Regenerative Medicine Research Center, West China Hospital, Sichuan University, Chengdu, China
Cell proliferation is an important cellular process for physiological tissue homeostasis and remodeling. The mechanisms of cell proliferation in response to pathological stresses are not fully understood. Mitochondria are highly dynamic organelles whose shape, number, and biological functions are modulated by mitochondrial dynamics, including fusion and fission. Mitofusin-2 (Mfn-2) is an essential GTPase-related mitochondrial dynamics protein for maintaining mitochondrial network and bioenergetics. A growing body of evidence indicates that Mfn-2 has a potential role in regulating cell proliferation in various cell types. Here we review these new functions of Mfn-2, highlighting its crucial role in several signaling pathways during the process of pathological cell proliferation. We conclude that Mfn-2 could be a new mediator of pathological cell proliferation and a potential therapeutic target.
Introduction
Cell proliferation is a precision–control process, which is essential for embryonic and postnatal development (Takeuchi and Nakamura, 2014). Under pathological conditions, abnormal cell proliferation is a central mechanism attributing to disease progressions. Abnormal cell proliferation includes both abnormal cell division and abnormal cell differentiation (Fajas, 2003). These processes are common in various diseases. For example, cardiac fibroblast proliferation and fibroblast-to-myofibroblast transition resulted in cardiac fibrosis (Nagpal et al., 2016), a pathological process characterized by the accumulation of extracellular matrix in the cardiac interstitium. Abnormal hepatic stellate cell (HSCs) trans-differentiation, activation, and proliferation of HSCs are the primary driving force to promote chronic cholestatic liver diseases and facilitate the progression of liver fibrosis (Liu et al., 2019). Proliferation is also a main characteristic of cancer cells and the base of metastasis (Carmeliet et al., 1998). Therefore, a thorough understanding of the underlying mechanisms regulating various pathological cell proliferations is the premise for developing new therapeutic strategies.
Previously, mitochondria have been regarded as static and isolated organelles. More recently, they are found to undergo constant changes in morphology, including fission, fusion, and network formation, and they can also relocate to different parts in cells (trafficking); all of these processes are termed mitochondrial dynamics. Mitochondrial dynamics is essential to maintain the normal function of cells such as energy metabolism, calcium homeostasis, and reactive oxygen species generation (Vafai and Mootha, 2012; Tsushima et al., 2018). Mitochondria dynamics (Figure 1) is closely related to cell proliferation. Previous evidence has set a link between mitochondria dynamics and cell proliferation; high levels of mitochondrial fission are associated with active proliferation (Chen and Chan, 2017). Mitra et al. (2009) reported that maintaining mitochondria in hyperfused morphology could regulate the cell transition from G1 to S phase. In addition, the number of mitochondria throughout the cell cycle is mostly constant, which is attributed to mitochondria dynamics to a great extent (Carlton et al., 2020). Oncocytes could alter mitochondria dynamics to support their proliferation property (Senft and Ronai, 2016).
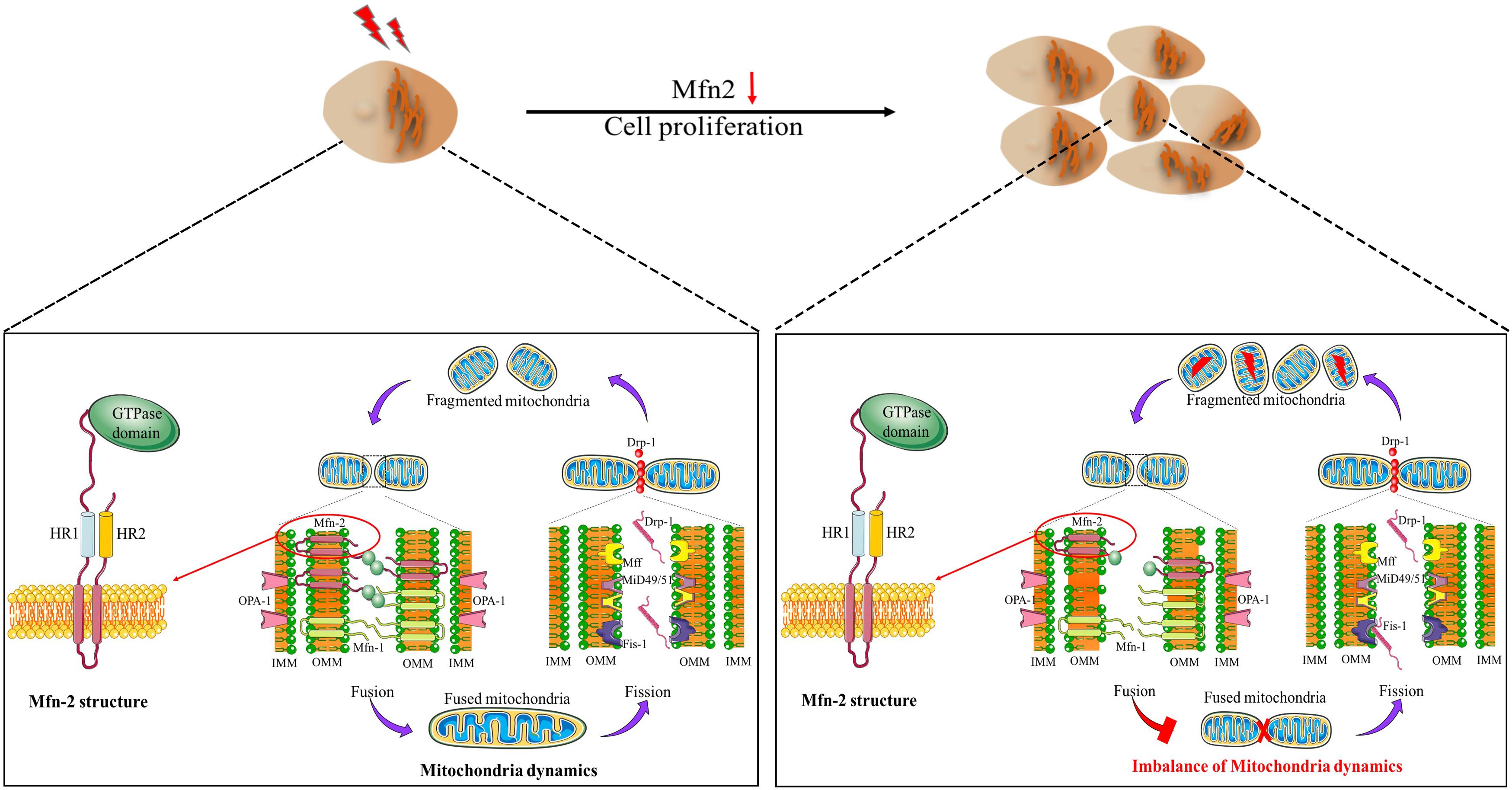
Figure 1. Mitochondria dynamics and Mfn-2 structure. Mfn-2 is one of the main factors regulating mitochondrial fusion. The N-terminal of Mfn-2 is the GTPase domain. There are two HR domains and two transmembrane domains.
Research in the past two decades or so have revealed that the mechanisms of mitochondrial dynamics are regulated by a group of highly conserved guanosine triphosphatases (GTPase) (Misaka et al., 2002; Rojo et al., 2002; Son et al., 2017). Mitofusin 1 (Mfn-1) and mitofusin 2 (Mfn-2) control the fusion of the outer mitochondrial membrane (OMM), while optic atrophy 1 (OPA1) regulates the fusion of the inner mitochondrial membrane. Mitochondrial fission is under-controlled by the dynamic-related protein 1 (Drp1), which interacts with several OMM proteins, such as Mid51 and Mid49. Mfns are outer mitochondria membrane proteins, with GTPase domain in the N-terminal, followed by a hydrophobic heptad repeat (HR1), the transmembrane anchor, and HR2. Mfns anchored to OMM by the C-terminal binding domain, and the N-terminal domain extruded to the cytoplasm (Fritz et al., 2001; Rojo et al., 2002; Figure 1). Mammalian Mfn-1 and Mfn-2 shared about 80% similarity, consisting of 737 and 757 amino acids, respectively. However, Mfn-1 exhibited higher GTPase activity than Mfn-2, while Mfn-2 exhibited higher GTP affinity. It is reported that Mfn-1 functions in mitochondria tethering, while Mfn-2 has other distinct functions in addition to the fusion reaction (Ishihara et al., 2004). For example, Mfn-2 has been reported to be involved in PINK/Parkin-mediated mitophagy process (Chen and Dorn, 2013). Mfn-2 is one of the first proteins identified to mediate the tethering of endoplasmic reticulum (ER) and mitochondria in mammals (Vance, 1990). This domain, named mitochondria-associated membranes (MAM), has been the new frontiers in bioenergetics and biophysical studies of intracellular organelles. ER lost its physiological morphology, and the ER–mitochondria interaction was disrupted after Mfn-2 ablation (de Brito and Scorrano, 2008). Increasing evidence has indicated that MAM is closely related to cell proliferation in various diseases (Wang et al., 2009; Danese et al., 2017; Yang et al., 2019). Comparing with Mfn-1, Mfn-2 may play a vital role in cell proliferation via various pathways.
In this review, we summarized recent advancements in the study of mitochondrial fusion protein Mfn-2. We focus on the link between altered Mfn-2 and pathological cell proliferation.
Overview of Mitofusin-2 and Cell Proliferation
Current evidence indicates that Mfn-2 plays various roles in many cellular processes, including cell proliferation and cell death (Chen et al., 2004; Wang et al., 2010; Zhao et al., 2012). Chen et al. (2004) found that Mfn-2 expression decreased in proliferative vascular smooth muscle cells (VSMCs), and overexpression of Mfn-2 could inhibit this proliferation process. Mfn-2 dysfunction has been associated with a variety of pathological conditions, including diabetes mellitus (Hernández-Alvarez et al., 2010), obesity (Bach et al., 2003), Charcot–Marie–Tooth disease (Züchner et al., 2004; Misko et al., 2012), atherosclerosis, hypertension (Chen et al., 2004), and cancer (Zhang et al., 2013; Xu et al., 2017). Collectively, the results of these studies have depicted Mfn-2 as a hyperplasia suppressor gene. In addition, energy is required in cell proliferation; in G1/S phase, mitochondrial elongation requires a higher amount of ATP to sustain cell duplication (Mitra et al., 2009). Mitochondria with intact structure are vital to supply enough ATP in this process, and Mfn-2 is necessary to maintain mitochondrial membrane potential, cellular oxygen consumption, etc. According to Sara’s research (Pich et al., 2005), the role of Mfn-2 in mitochondrial nutrient oxidation is a fusion-independent function. Mitochondria fusion is also necessary to maintain the stoichiometry of the protein components of mtDNA replisome. Mfn-2 mutation could cause diseases due to mtDNA depletion, disrupting mouse embryo fibroblast (MEF) proliferation and postnatal heart development (Silva Ramos et al., 2019). Here a more detailed discussion of how Mfn-2 regulates cell proliferation is provided (Figure 2).
Mitofusin-2 and Atherosclerosis
Atherosclerosis is the main cause of cardiovascular diseases. It has been clearly demonstrated that the development and progression of atherosclerosis involve inflammation, abnormal proliferation of VSMCs, remodeling of the vascular wall, and evolution of occlusive plaques.
It is reported that the antiproliferative effect of Mfn-2 in serum-evoked VSMC proliferation was mediated via the inhibition of Erk/mitogen-activated protein kinase (Erk/MAPKs) signaling followed by cell cycle arrest. Cell transition from G0/G1 phase to S phase is one of the important characteristics for proliferation. EdU assay indicated that downregulation of Mfn-2 resulted in a significant decrease of G0/G1 phase cells and a synchronous increase of the percentage of S-phase cells in homocysteine-induced VSMCs, and overexpression of Mfn-2 obtained the contrary results, suggesting that cell cycle arrest at G0/G1 phase is responsible for the anti-proliferation effects of Mfn-2 (Xu et al., 2019). Two related mechanisms involved in this process were reported: one is that Mfn-2 could bind and inhibit the proto-oncogene Ras directly via its p21 Ras-binding domain in the N-terminal to control cell proliferation (de Brito and Scorrano, 2009), while Mfn-2 mutant lacking amino acids 77–91 failed to pull down Ras, with no effect on its function in the regulation of mitochondria fusion (de Brito and Scorrano, 2009); the other is that Mfn-2 regulates NAD/NADH ratio to control the transition of cell phase (Li et al., 2015). Infection with Mfn-2 adenovirus could increase NADH level and reduce NAD level, blocking the cell cycle at G0/G1 phase.
The accumulation of cholesterol-containing low-density lipoproteins in the intima and endothelium promotes the recruitment of monocytes, which then differentiate into macrophages and initiate inflammatory activation. Inflammation cells release various cytokines and growth factors, the most powerful factors, such as platelet-derived growth factor (PDGF) and its receptors, inducing VSMCs to transform from quiescent state to secretion and proliferation state via many signaling pathways, such as MAPK, PI3K/Akt, etc. (Wang et al., 2018). One remarkable characteristic of this process is migration of VSMCs from the media to the intima, contributing to neo-intima formation. In addition, various inhibitors in the process of inflammation could inhibit VSMC proliferation (Uhrin et al., 2018). In Feng’s study, Mfn-2 expression was downregulated in proliferative VSMC primary cells treated with PDGF. Down-regulated Mfn-2 promotes cell proliferation and migration rate not only in PDGF-induced VSMCs but also in arterial smooth muscle cells (ASMCs) mediated by microRNA (miRNA) miR-31 (Huang et al., 2018), which targets the 3′UTR of Mfn-2 and downregulates its expression.
In patients diagnosed with type 2 diabetes, the incidence of atherosclerosis is high, and the expression of Mfn-2 in the muscle decreased; similar results are also observed in high-fat-diet mice. In addition, increasing the level of Mfn-2 could alleviate insulin resistance (Gan et al., 2013) through increasing the phosphorylation levels of PI3K-P85 and AKT2 insulin signaling pathways. Previous studies have identified that nuclear receptor superfamily member peroxisome proliferator-activated receptor-γ (PPARγ) is involved in atheromatous inflammation through the extracellular signal-regulated ERK/MAPKs and p38/MAPK pathways (Chen et al., 2004; Monsalve et al., 2013; Nagy et al., 2013; Yang et al., 2012). Liu et al. (2014) found that Mfn-2 inhibited atherosclerotic plaque formation by increasing PPARγ, and this process may be partially regulated by inactivation of the ERK1/2 and p38/MAPKs pathways. Xu et al. (2019) showed that, in homocysteine (Hcy)-induced atherosclerosis, c-Myc expression was increased, and it bound DNMT1 promoter to cause DNA hypermethylation of the Mfn-2 promoter, resulting in aberrant Mfn-2 transcription that led to VSMC proliferation.
As mentioned above, Mfn-2 is crucial in maintaining MAM structure. MAM is the central platform for the initiation of mitophagy and the formation of autophagosome (Hailey et al., 2010; Hamasaki et al., 2013). Chen and Dorn (2013) has linked Mfn-2 with PINK-Parkin-mediated senescent mitochondria elimination. In their model, PINK phosphorylates Mfn-2 to recruit Parkin to mitochondria, and Parkin, in turn, mediates Mfn-2 ubiquitination and initiates mitophagy. The effects of mitophagy on VSMC survival in response to stimuli in the pathogenesis of vascular disorders have been investigated in numerous studies (Culley and Chan, 2018; Marshall et al., 2018). He et al. (2019) reported that apelin-13 could induce VSMC proliferation and exacerbate atherosclerosis. PINK1/Parkin-mediated mitophagy could promote this process. In addition, a study on miR-145 found that the autophagy level was increased in the carotid intima hyperplasia in C57BL/6J mice (Wang et al., 2020), and activation of autophagy could stimulate aortic VSMC proliferation in metabolic hypertension rats (Wen et al., 2019). Although there is no direct evidence that Mfn-2 regulates cell proliferation via autophagy, considering the role of Mfn-2 in the maintenance of MAM, more studies are required to provide evidence linking Mfn-2-mediated autophagy with VSMC proliferation in the future.
MFN-2 and Pulmonary Hypertension
Pulmonary hypertension is another pathological condition attributed to VSMC proliferation. Pathophysiological changes involve all three layers of pulmonary arteries during vascular remodeling. For instance, endothelial angiogenesis, smooth muscle cell hyperplasia and hypertrophy, adventitial fibroblast proliferation, myofibroblast differentiation, and extracellular matrix deposition have all been reported (Howell et al., 2003; Wilkins et al., 2015). The excessive proliferation and impaired apoptosis of pulmonary artery smooth muscle cells (PASMCs) contribute to vascular obstruction in pulmonary hypertension patients.
The role of Mfn-2 in pulmonary hypertension is complicated. Zhang et al. (2012) reported that hypoxia induced Mfn-2 expression and knock-down Mfn-2 suppressed hypoxia-induced PASMCs proliferation through the PI3K/Akt signaling pathway. However, other studies indicated that the expression of Mfn-2 was decreased in pulmonary hypertension models, and increasing the Mfn-2 level may be a therapeutic strategy (Ryan et al., 2013, 2015; Fang et al., 2016; Lu et al., 2016). Deletion of thrombospondin motifs 8 (ADAMTS8), a secreted protein specifically expressed in the lung and the heart, reduced PASMC proliferation with Mfn-2 upregulation and mitochondrial function improvement (Omura et al., 2019). Downregulation of Mfn-2 may cause more cells to enter the S + G2/M phase of the cell cycle and inhibit the mitochondrial apoptosis pathway. These effects were reversed in PASMCs by Mfn-2 overexpression (Roy et al., 2009), indicating that Mfn-2 could be a therapeutic target in pulmonary hypertension (Ryan et al., 2013). While these discrepancies need to be resolved in future studies, it is clear that Mfn-2 is a critical regulator for pathological pulmonary hypertension.
Recent studies revealed that miRNAs play an important role in the pathogenesis of pulmonary hypertension by regulating ASMC proliferation (Thum et al., 2008; Roy et al., 2009; Bauersachs, 2010; Castoldi et al., 2012; Chen et al., 2014). Several miRNAs have been identified to participate in fibrosis and smooth muscle cell proliferation via targeting Mfn-2 directly. Huang et al. (2018) reported that the expression level of miR-31 was increased significantly in the arterial walls of patients with atherosclerosis obliterans. In addition, miR-31 promoted the proliferation and migration of human arterial smooth muscle cells, at least partially, through directly interacting with Mfn-2. In another study, Lu et al. (2016) revealed that miR-17 was upregulated in human PASMCs from patients with pulmonary artery hypertension, and the function of miR-17 in PASMC proliferation and apoptosis was partially mediated by downregulation of Mfn-2.
Mitofusin-2 and Fibroproliferative Diseases
Fibroblasts are a crucial component of connective tissues in various organs. Fibroblast activation and proliferation is characterized by an excessive accumulation of fibrous connective tissues in response to various stimuli (Kendall and Feghali-Bostwick, 2014). Fibrosis is a key pathological process in the development of various fibroproliferative diseases, such as cardiovascular fibrosis, pulmonary fibrosis, liver cirrhosis, systemic sclerosis, and kidney fibrosis. Fibroblasts produce the structural proteins of extracellular matrix (ECM), such as fibrous collagen (Kendall and Feghali-Bostwick, 2014). Fibroblast activation and proliferation may cause the accumulation of ECM’s components, ultimately leading to disruption of the architecture in various tissues and dysfunction of the organs, such as end-stage liver disease and kidney failure. Mfn-2 could inhibit non-alcoholic fatty liver by interacting with phosphatidylserine (PS) directly. Mfn-2 deficiency could reduce PS transfer from the ER to the mitochondria, inducing mitochondrial dysfunction (Hernandez-Alvarez et al., 2019). Knockdown of Mfn-2 could rescue mitochondria Ca2+ transfer and inhibit cell proliferation in kidney cysts (Kuo et al., 2019). In addition, overexpressed Mfn-2 could alleviate glomerular mesangial cell proliferation via the MAPK/ERK and PI3K/Akt pathways (Wan-Xin et al., 2012; Chen et al., 2019). Mfn-2 regulates mitochondria fusion and intracellular lipid metabolism, which are tightly linked with lung fibrosis (Chung et al., 2019). Prediabetes is a common pathological condition characterized by increased ventricular mass and wall thickness, in which fibroblast proliferation is one of the key factors. Although this is a complicated biological process, Mfn-2 is considered to play a vital role in this process (Koncsos et al., 2016).
Endoplasmic reticulum stress (ERS) is a cellular state in which the protein folding capacity of ER is overwhelmed due to increased protein load or disruption of the protein folding environment (Berridge, 2002). It has been reported that ERS is involved in fibroblast activation and proliferation in many organs such as the liver (Shin et al., 2013), heart (Spitler and Webb, 2014), kidney (Chiang et al., 2011), and lung (Baek et al., 2012). Previous reports also identified the relationship between Mfn-2 and ERS. For example, in cardiac fibrosis, we found that the expression of Mfn-2 was decreased, and upregulating Mfn-2 inhibited fibroblast proliferation via the p-PERK/ATF4 pathway but not the IREα/Xbp1s or c-ATF6 signaling pathway (Xin et al., 2019). Ngoh et al. (2012) and Muñoz et al. (2013) also reported that Mfn-2 was an ERS-inducible protein. Philippe and Gary wrote a review discussing the role of Mfn-2 in inflammation-induced ERS (Delmotte and Sieck, 2019). Downregulation of Mfn-2 aggravated ERS, attributing to the proliferation of airway smooth muscle.
A study from Sun et al. (2015) showed that miR-214 mediated ISO-induced proliferation and collagen synthesis in cardiac fibroblasts through directly targeting Mfn-2 and activating the downstream ERK1/2 MAPK signaling pathway.
Mitofusin-2 and Cancer
Abnormal cell proliferation could lead to the destabilization of chromosomal and genetic organization, resulting in the formation of neoplasm. This effect is regulated by a series of genes. Mutation of the genes will drive cells into tumor cells. The increase in glucose uptake and enhanced glycolytic rates indicate that metabolic alteration provides growth advantages for tumor cells (Lunt and Vander Heiden, 2011). In addition, tumor cells may exert different metabolic ways from normal cell proliferation. Warburg noticed that tumor cells exhibit a high rate of glycolysis even in the presence of oxygen (aerobic glycolysis) (Ortega et al., 2009). Some types of cancer, such as bladder cancer, cervical cancer, and breast cancer, are associated with altered mitochondrial morphology and metabolism (Filadi et al., 2018). The expression level of Mfn-2 is usually decreased in various types of cancer, and increasing Mfn-2 expression can suppress cell proliferation (Rehman et al., 2012), indicating that Mfn-2 is a tumor suppressor. One mechanism by which Mfn-2 suppresses cancer cell proliferation is inhibiting the metabolic flux to aerobic glycolysis by interreacting with pyruvate kinase 2 (PKM2) via the N-terminus (Li et al., 2009); phosphorylation of Mfn-2 enhances this interaction. Rictor is a subunit of mTORC1; Rictor deletion could block mitochondrial OXPHOS. Xu’s recent work showed that Mfn-2 plays a vital role in the inhibition of the mTORC2/Akt signaling pathway through the interaction with Rictor by binding its HR1 domain in breast cancer patients. Mfn-2 knockdown could enhance growth in breast cancer. All these evidence indicated that the mTORC/AKT pathway is closely linked with Mfn-2 in pathological cell proliferation.
Under physiological conditions, mitochondria take up calcium mainly from the ER. Calcium is released through inositol 1,4,5-trisphosphate (IP3) receptors (IP3R) and ryanodine receptors (RyRs) on the ER membrane (Marks, 1997) and enters mitochondria through voltage-dependent anion channels (VDACs). The relationship between calcium signaling pathway and cancer has been discussed in detail in previous reviews (Ivanova et al., 2017; Marchi et al., 2018). In this process, MAM play a vital role (Ivanova et al., 2017) because these harbor key calcium handling proteins such as IP3R, VDACs, and sigma-1 receptors (Hayashi and Su, 2007). In 2008, de Brito and Scorrano (2008) showed that Mfn-2 was required for mitochondrial calcium uptake, and Mfn-2 knockout MEFs exhibited a reduced calcium uptake rate. In addition, translation of the calcium signals is necessary for the regulation of cell death and survival. Lower ER calcium content could arouse the expression of anti-apoptotic protein expression, such as Bcl-2 (Pinton et al., 2001). Mfn-2 could restore calcium homeostasis and downregulate ER stress in mouse neuroblastoma N2a cells.
Besides the regulation of intracellular calcium transportation, Mfn-2 may also regulate other signaling pathways for cell proliferation. Transcription factor SP1 plays an essential role in the expression of PITPNM3 in cancer cells, and Mfn-2 was reported to have anti-tumor activity by interacting with transcription factor SP1 directly (Tang et al., 2020). Pang et al. (2019) found that Mfn-2 inhibited cell proliferation via the Wnt/β-catenin pathway in bladder cancer. Mfn-2 knockdown increased the translocation of β-catenin into the nucleus, resulting in larger tumor volumes and a higher proliferation index. Pharmacological inhibition of the Mfn-2/mTORC2/Akt pathway attenuated tumor growth (Xu et al., 2017). Several other studies also indicated that Mfn-2 interacted with the proapoptotic Bcl-2 family members Bax and Bak (Suen et al., 2008; Li et al., 2020).
Future Directions
Despite the significant advancements that deepened our understanding of the structure and functions of Mfn-2, several key questions remain to be elucidated:
(1) Although some evidence indicated that, in addition to fusion reaction, Mfn-2 has been reported to regulate cell proliferation via various signaling pathways, there are currently no studies that figured out whether these pathways are related to mitochondria fusion. More studies on this topic may be necessary in the future.
(2) Although there are many evidence about the role of Mfn-2 in neoplastic diseases, the microenvironment may change the impact of Mfn-2 in different types of cancers. In the future, a more detailed analysis of Mfn-2 may be necessary.
(3) Could altered Mfn-2 act as a potential biomarker in pathogenesis?
Conclusion
The machineries of mitochondrial fusion/fission and the significance of mitochondrial dynamics in regulating mitochondrial and cellular functions have been established. The mitochondrial fusion protein Mfn-2 regulates mitochondrial morphology, metabolism, calcium homeostasis, and mtDNA stability. Mfn-2 also plays a role via MAM formation and mitophagy to regulate cell proliferation and cell survival/death in different tissues. Many important questions await extensive investigation. In the future, studies on the role of Mfn-2 in cell proliferation may lead to the development of new strategies for treating various diseases, such as cancer and cardiovascular diseases.
Author Contributions
YX and JL draft the manuscript. WW revised and polished the manuscript. XL supervised the review and established the whole frame. All authors contributed to the article and approved the submitted version.
Funding
This study was supported by the National Natural Science Foundation of China (NSFC) projects: 12072215 and 11672197 and the Fundamental Research Funds for the Central Universities: 2020SCU12031.
Conflict of Interest
The authors declare that the research was conducted in the absence of any commercial or financial relationships that could be construed as a potential conflict of interest.
Acknowledgments
We thank Wang and Pei Wang for the critical comments.
References
Bach, D., Pich, S., Soriano, F. X., Vega, N., Baumgartner, B., and Oriola, J. (2003). Mitofusin-2 determines mitochondrial network architecture and mitochondrial metabolism. a novel regulatory mechanism altered in obesity. J. Biol. Chem. 278, 17190–17197. doi: 10.1074/jbc.m212754200
Baek, H. A., Kim, D. S., Park, H. S., Jang, K. Y., Kang, M. J., and Lee, D. G. (2012). Involvement of endoplasmic reticulum stress in myofibroblastic differentiation of lung fibroblasts. Am. J. Respir. Cell Mol. Biol. 46, 731–739. doi: 10.1165/rcmb.2011-0121oc
Bauersachs, J. (2010). Regulation of myocardial fibrosis by MicroRNAs. J. Cardiovasc. Pharmacol. 56, 454–459. doi: 10.1097/fjc.0b013e3181ee81df
Berridge, M. J. (2002). The endoplasmic reticulum: a multifunctional signaling organelle. Cell Calcium 32, 235–249. doi: 10.1016/s0143416002001823
Carlton, J. G., Jones, H., and Eggert, U. S. (2020). Membrane and organelle dynamics during cell division. Nat. Rev. Mol. Cell Biol. 21, 151–166. doi: 10.1038/s41580-019-0208-1
Carmeliet, P., Dor, Y., Herbert, J. M., Fukumura, D., Brusselmans, K., and Dewerchin, M. (1998). Role of HIF-1alpha in hypoxia-mediated apoptosis, cell proliferation and tumour angiogenesis. Nature 394, 485–490. doi: 10.1038/28867
Castoldi, G., Di Gioia, C. R. T., Bombardi, C., Catalucci, D., Corradi, B., and Gualazzi, M. G. (2012). MiR-133a regulates collagen 1A1: potential role of miR-133a in myocardial fibrosis in angiotensin II-dependent hypertension. J. Cell. Physiol. 227, 850–856. doi: 10.1002/jcp.22939
Chen, H., and Chan, D. C. (2017). Mitochondrial dynamics in regulating the unique phenotypes of cancer and stem cells. Cell Metab. 26, 39–48. doi: 10.1016/j.cmet.2017.05.016
Chen, H., Du, Y., Li, Y., Zeng, J., Miao, J., and Jiang, X. (2019). Jixuecao (Herba Centellae Asiaticae) alleviates mesangial cell proliferation in IgA nephropathy by inducing mitofusin 2 expression. J. Tradit. Chin. Med. 39, 346–355.
Chen, K.-H., Guo, X., Ma, D., Guo, Y., Li, Q., Yang, D., et al. (2004). Dysregulation of HSG triggers vascular proliferative disorders. Nat. Cell Biol. 6, 872–883. doi: 10.1038/ncb1161
Chen, S., Puthanveetil, P., Feng, B., Matkovich, S. J., Dorn, G. W., and Chakrabarti, S. (2014). Cardiac miR-133a overexpression prevents early cardiac fibrosis in diabetes. J. Cell Mol. Med. 18, 415–421. doi: 10.1111/jcmm.12218
Chen, Y., and Dorn, G. W. (2013). PINK1-phosphorylated mitofusin 2 is a Parkin receptor for culling damaged mitochondria. Science 340, 471–475. doi: 10.1126/science.1231031
Chiang, C.-K., Hsu, S.-P., Wu, C.-T., Huang, J.-W., Cheng, H.-T., and Chang, Y.-W. (2011). Endoplasmic reticulum stress implicated in the development of renal fibrosis. Mol. Med. 17, 1295–1305. doi: 10.2119/molmed.2011.00131
Chung, K. P., Hsu, C. L., Fan, L. C., Huang, Z., Bhatia, D., and Chen, Y.-J. (2019). Mitofusins regulate lipid metabolism to mediate the development of lung fibrosis. Nat. Commun. 10:3390.
Culley, M. K., and Chan, S. Y. (2018). Mitochondrial metabolism in pulmonary hypertension: beyond mountains there are mountains. J. Clin. Invest. 128, 3704–3715. doi: 10.1172/jci120847
Danese, A., Patergnani, S., Bonora, M., Wieckowski, M. R., Previati, M., Giorgi, C., et al. (2017). Calcium regulates cell death in cancer: roles of the mitochondria and mitochondria-associated membranes (MAMs). Biochimica et Biophysica Acta Bioenergetics 1858, 615–627. doi: 10.1016/j.bbabio.2017.01.003
de Brito, O. M., and Scorrano, L. (2008). Mitofusin 2 tethers endoplasmic reticulum to mitochondria. Nature 456, 605–610. doi: 10.1038/nature07534
de Brito, O. M., and Scorrano, L. (2009). Mitofusin-2 regulates mitochondrial and endoplasmic reticulum morphology and tethering: the role of Ras. Mitochondrion 3, 222–226. doi: 10.1016/j.mito.2009.02.005
Delmotte, P., and Sieck, G. C. (2019). Endoplasmic reticulum stress and mitochondrial function in airway smooth muscle. Front. Cell Dev. Biol. 7:374.
Fajas, L. (2003). Adipogenesis: a cross-talk between cell proliferation and cell differentiation. Ann. Med. 35, 79–85. doi: 10.1080/07853890310009999
Fang, X., Chen, X., Zhong, G., Chen, Q., and Hu, C. (2016). Mitofusin 2 downregulation triggers pulmonary artery smooth muscle cell proliferation and apoptosis imbalance in rats with hypoxic pulmonary hypertension Via the PI3K/Akt and mitochondrial apoptosis pathways. J. Cardiovasc. Pharmacol. 67, 164–174. doi: 10.1097/fjc.0000000000000333
Filadi, R., Pendin, D., and Pizzo, P. (2018). Mitofusin 2: from functions to disease. Cell Death Dis. 9:330.
Fritz, S., Rapaport, D., Klanner, E., Neupert, W., and Westermann, B. (2001). Connection of the mitochondrial outer and inner membranes by Fzo1 is critical for organellar fusion. J. Cell Biol. 152, 683–692. doi: 10.1083/jcb.152.4.683
Gan, K.-X., Wang, C., Chen, J.-H., Zhu, C.-J., and Song, G.-Y. (2013). Mitofusin-2 ameliorates high-fat diet-induced insulin resistance in liver of rats. World Gastroenterol. 19, 1572–1581. doi: 10.3748/wjg.v19.i10.1572
Hailey, D. W., Rambold, A. S., Satpute-Krishnan, P., Mitra, K., Sougrat, R., Kim, P. K., et al. (2010). Mitochondria supply membranes for autophagosome biogenesis during starvation. Cell 141, 656–667. doi: 10.1016/j.cell.2010.04.009
Hamasaki, M., Furuta, N., Matsuda, A., Nezu, A., Yamamoto, A., and Fujita, N. (2013). Autophagosomes form at ER-mitochondria contact sites. Nature 495, 389–393. doi: 10.1038/nature11910
Hayashi, T., and Su, T.-P. (2007). Sigma-1 receptor chaperones at the ER-mitochondrion interface regulate Ca(2+) signaling and cell survival. Cell 131, 596–610. doi: 10.1016/j.cell.2007.08.036
He, L., Zhou, Q., Huang, Z., Xu, J., Zhou, H., and Lv, D. (2019). PINK1/Parkin-mediated mitophagy promotes apelin-13-induced vascular smooth muscle cell proliferation by AMPKα and exacerbates atherosclerotic lesions. J. Cell Physiol. 234, 8668–8682. doi: 10.1002/jcp.27527
Hernandez-Alvarez, M. I., Sebastian, D., Vives, S., Ivanova, S., Bartoccioni, P., and Kakimoto, P. (2019). Deficient endoplasmic reticulum-mitochondrial phosphatidylserine transfer causes liver disease. Cell 177, 881–895.e17.
Hernández-Alvarez, M. I., Thabit, H., Burns, N., Shah, S., Brema, I., and Hatunic, M. (2010). Subjects with early-onset type 2 diabetes show defective activation of the skeletal muscle PGC-1{alpha}/Mitofusin-2 regulatory pathway in response to physical activity. Diabetes Care 33, 645–651. doi: 10.2337/dc09-1305
Howell, K., Preston, R. J., and McLoughlin, P. (2003). Chronic hypoxia causes angiogenesis in addition to remodelling in the adult rat pulmonary circulation. J. Physiol. 547(Pt 1), 133–145. doi: 10.1113/jphysiol.2002.030676
Huang, S., Chen, Z., Wu, W., Wang, M., Wang, R., and Cui, J. (2018). MicroRNA-31 promotes arterial smooth muscle cell proliferation and migration by targeting mitofusin-2 in arteriosclerosis obliterans of the lower extremitie. Exp. Ther. Med. 15, 633–640.
Ishihara, N., Eura, Y., and Mihara, K. (2004). Mitofusin 1 and 2 play distinct roles in mitochondrial fusion reactions via GTPase activity. J. Cell Sci. 117(Pt 26), 6535–6546. doi: 10.1242/jcs.01565
Ivanova, H., Kerkhofs, M., La Rovere, R. M., and Bultynck, G. (2017). Endoplasmic reticulum-mitochondrial ca fluxes underlying cancer cell survival. Front. Oncol. 7:70.
Kendall, R. T., and Feghali-Bostwick, C. A. (2014). Fibroblasts in fibrosis: novel roles and mediators. Front. Pharmacol. 5:123.
Koncsos, G., Varga, Z. V., Baranyai, T., Boengler, K., Rohrbach, S., and Li, L. (2016). Diastolic dysfunction in prediabetic male rats: role of mitochondrial oxidative stress. Am. J. Physiol. Heart Circ. Physiol. 311, H927–H943.
Kuo, I. Y., Brill, A. L., Lemos, F. O., Jiang, J. Y., Falcone, J. L., and Kimmerling, E. P. (2019). Polycystin 2 regulates mitochondrial Ca 2+ signaling, bioenergetics, and dynamics through mitofusin 2. Sci. Signal. 12:eaat7397. doi: 10.1126/scisignal.aat7397
Li, D., Li, X., Guan, Y., and Guo, X. (2015). Mitofusin-2-mediated tethering of mitochondria and endoplasmic eticulum promotes cell cycle arrest of vascular smooth muscle cells in G0/G1 phase. Acta Biochim. Biophys. Sin. 47, 441–450. doi: 10.1093/abbs/gmv035
Li, H.-B., Zhang, X.-Z., Sun, Y., Zhou, Q., Song, J.-N., and Hu, Z.-F. (2020). HO-1/PINK1 regulated mitochondrial fusion/fission to inhibit pyroptosis and attenuate septic acute kidney injury. BioMed. Res. Int. 2020:2148706.
Li, T., Han, J., Jia, L., Hu, X., Chen, L., and Wang, Y. (2009). PKM2 coordinates glycolysis with mitochondrial fusion and oxidative phosphorylation. Protein Cell 10, 583–594. doi: 10.1007/s13238-019-0618-z
Liu, C., Ge, B., He, C., Zhang, Y., Liu, X., Liu, K., et al. (2014). Mitofusin 2 decreases intracellular lipids in macrophages by regulating peroxisome proliferator-activated receptor-γ. Biochem. Biophys. Res. Commun. 450, 500–506. doi: 10.1016/j.bbrc.2014.06.005
Liu, R., Li, X., Zhu, W., Wang, Y., Zhao, D., and Wang, X. (2019). Cholangiocyte-derived exosomal long noncoding RNA H19 promotes hepatic stellate cell activation and cholestatic liver fibrosis. Hepatology 70, 1317–1335. doi: 10.1002/hep.30662
Lu, Z., Li, S., Zhao, S., and Fa, X. (2016). Upregulated miR-17 regulates hypoxia-mediated human pulmonary artery smooth muscle cell proliferation and apoptosis by targeting Mitofusin 2. Med. Sci. Monit. 22, 3301–3308. doi: 10.12659/msm.900487
Lunt, S. Y., and Vander Heiden, M. G. (2011). Aerobic glycolysis: meeting the metabolic requirements of cell proliferation. Annu. Rev. Cell Dev. Biol. 27, 441–464. doi: 10.1146/annurev-cellbio-092910-154237
Marchi, S., Patergnani, S., Missiroli, S., Morciano, G., Rimessi, A., Wieckowski, M. R., et al. (2018). Mitochondrial and endoplasmic reticulum calcium homeostasis and cell death. Cell Calcium 69, 62–72. doi: 10.1016/j.ceca.2017.05.003
Marks, A. R. (1997). Intracellular calcium-release channels: regulators of cell life and death. Am. J. Physiol. 272(2 Pt 2), H597–H605.
Marshall, J. D., Bazan, I., Zhang, Y., Fares, W. H., and Lee, P. J. (2018). Mitochondrial dysfunction and pulmonary hypertension: cause, effect, or both. Am. J. Physiol. Lung. Cell Mol. Physiol. 314, L782–L796.
Misaka, T., Miyashita, T., and Kubo, Y. (2002). Primary structure of a dynamin-related mouse mitochondrial GTPase and its distribution in brain, subcellular localization, and effect on mitochondrial morphology. J. Biol. Chem. 277, 15834–15842. doi: 10.1074/jbc.m109260200
Misko, A. L., Sasaki, Y., Tuck, E., Milbrandt, J., and Baloh, R. H. (2012). Mitofusin2 mutations disrupt axonal mitochondrial positioning and promote axon degeneration. J. Neurosci. 32, 4145–4155. doi: 10.1523/jneurosci.6338-11.2012
Mitra, K., Wunder, C., Roysam, B., Lin, G., and Lippincott-Schwartz, J. (2009). A hyperfused mitochondrial state achieved at G1-S regulates cyclin E buildup and entry into S phase. Proc. Natl. Acad. Sci. USA 106, 11960–11965. doi: 10.1073/pnas.0904875106
Monsalve, F. A., Pyarasani, R. D., Delgado-Lopez, F., and Moore-Carrasco, R. (2013). Peroxisome proliferator-activated receptor targets for the treatment of metabolic diseases. Mediators Inflamm. 2013:549627.
Muñoz, J. P., Ivanova, S., Sánchez-Wandelmer, J., Martínez-Cristóbal, P., Noguera, E., and Sancho, A. (2013). Mfn2 modulates the UPR and mitochondrial function via repression of PERK. EMBO J. 32, 2348–2361. doi: 10.1038/emboj.2013.168
Nagpal, V., Rai, R., Place, A. T., Murphy, S. B., Verma, S. K., and Ghosh, A. K. (2016). MiR-125b is critical for fibroblast-to-myofibroblast transition and cardiac fibrosis. Circulation 133, 291–301. doi: 10.1161/circulationaha.115.018174
Nagy, Z. S., Czimmerer, Z., and Nagy, L. (2013). Nuclear receptor mediated mechanisms of macrophage cholesterol metabolism. Mol. Cell. Endocrinol. 368, 85–98. doi: 10.1016/j.mce.2012.04.003
Ngoh, G. A., Papanicolaou, K. N., and Walsh, K. (2012). Loss of mitofusin 2 promotes endoplasmic reticulum stress. J. Biol. Chem. 287, 20321–20332. doi: 10.1074/jbc.m112.359174
Omura, J., Saton, K., Kikuchi, N., Satoh, T., Kurosawa, R., and Nogi, M. (2019). ADAMTS8 promotes the development of pulmonary arterial hypertension and right ventricular failure. Circ. Res. 125, 884–906.
Ortega, A. D., Sánchez-Aragó, M., Giner-Sánchez, D., Sánchez-Cenizo, L., Willers, I., and Cuezva, J. M. (2009). Glucose avidity of carcinomas. Cancer Lett. 276, 125–135. doi: 10.1016/j.canlet.2008.08.007
Pang, G., Xie, Q., and Yao, J. (2019). Mitofusin 2 inhibits bladder cancer cell proliferation and invasion via the Wnt/β-catenin pathway. Oncol. Lett. 18, 2434–2442.
Pich, S., Bach, D., Briones, P., Liesa, M., Camps, M., and Testar, X. (2005). The Charcot-Marie-Tooth type 2A gene product, Mfn2, up-regulates fuel oxidation through expression of OXPHOS system. Hum. Mol. Genet. 14, 1405–1415. doi: 10.1093/hmg/ddi149
Pinton, P., Ferrari, D., Rapizzi, E., Di Virgilio, F., Pozzan, T., and Rizzuto, R. (2001). The Ca2+ concentration of the endoplasmic reticulum is a key determinant of ceramide-induced apoptosis: significance for the molecular mechanism of Bcl-2 action. EMBO J. 20, 2690–2701. doi: 10.1093/emboj/20.11.2690
Rehman, J., Zhang, H. J., Toth, P. T., Zhang, Y., Marsboom, G., Hong, Z., et al. (2012). Inhibition of mitochondrial fission prevents cell cycle progression in lung cancer. FASEB J. 26, 2175–2186. doi: 10.1096/fj.11-196543
Rojo, M., Legros, F., Chateau, D., and Lombès, A. (2002). Membrane topology and mitochondrial targeting of mitofusins, ubiquitous mammalian homologs of the transmembrane GTPase Fzo. J. Cell Sci. 115(Pt 8), 1663–1674.
Roy, S., Khanna, S., Hussain, S.-R. A., Biswas, S., Azad, A., and Rink, C. (2009). MicroRNA expression in response to murine myocardial infarction: miR-21 regulates fibroblast metalloprotease-2 via phosphatase and tensin homologue. Cardiovasc. Res. 82, 21–29. doi: 10.1093/cvr/cvp015
Ryan, J. J., Marsboom, G., Fang, Y.-H., Toth, P. T., Morrow, E., and Luo, N. (2013). PGC1α-mediated mitofusin-2 deficiency in female rats and humans with pulmonary arterial hypertension. Am. J. Respir. Crit. Care Med. 187, 865–878. doi: 10.1164/rccm.201209-1687oc
Ryan, J., Dasgupta, A., Huston, J., Chen, K.-H., and Archer, S. L. (2015). Mitochondrial dynamics in pulmonary arterial hypertension. J. Mol. Med. 93, 229–242.
Senft, D., and Ronai, Z. A. (2016). Regulators of mitochondrial dynamics in cancer. Curr. Opin. Cell Biol. 39, 43–52. doi: 10.1016/j.ceb.2016.02.001
Shin, J., He, M., Liu, Y., Paredes, S., Villanova, L., and Brown, K. (2013). SIRT7 represses Myc activity to suppress ER stress and prevent fatty liver disease. Cell Rep. 5, 654–665. doi: 10.1016/j.celrep.2013.10.007
Silva Ramos, E., Motori, E., Brüser, C., Kühl, I., Yeroslaviz, A., and Ruzzenente, B. (2019). Mitochondrial fusion is required for regulation of mitochondrial DNA replication. PLoS Genet. 15:e1008085. doi: 10.1371/journal.pgen.1008085
Son, J. M., Sarsour, E. H., Kakkerla, Balaraju, A., Fussell, J., and Kalen, A. L. (2017). Mitofusin 1 and optic atrophy 1 shift metabolism to mitochondrial respiration during aging. Aging Cell 16, 1136–1145. doi: 10.1111/acel.12649
Spitler, K. M., and Webb, R. C. (2014). Endoplasmic reticulum stress contributes to aortic stiffening via proapoptotic and fibrotic signaling mechanisms. Hypertension 63, e40–e45.
Suen, D.-F., Norris, K. L., and Youle, R. J. (2008). Mitochondrial dynamics and apoptosis. Genes Dev. 22, 1577–1590. doi: 10.1101/gad.1658508
Sun, M., Yu, H., Zhang, Y., Li, Z., and Gao, W. (2015). MicroRNA-214 mediates isoproterenol-induced proliferation and collagen synthesis in cardiac fibroblasts. Sci. Rep. 5:18351.
Takeuchi, T., and Nakamura, H. (2014). Cell proliferation and development. Preface. Dev. Growth Differ. 56:323.
Tang, T., Tao, X., Bao, X., Chen, J., Dai, J., Ye, J., et al. (2020). Mitofusin-2 (Mfn-2) might have anti-cancer effect through interaction with transcriptional factor SP1 and consequent regulation on phosphatidylinositol transfer protein 3 (PITPNM3) expression. Med. Sci. Monit. 26:e918599.
Thum, T., Gross, C., Fiedler, J., Fischer, T., Kissler, S., and Bussen, M. (2008). MicroRNA-21 contributes to myocardial disease by stimulating MAP kinase signalling in fibroblasts. Nature 456, 980–984. doi: 10.1038/nature07511
Tsushima, K., Bugger, H., Wende, A. R., Soto, J., Jenson, G. A., and Tor, A. R. (2018). Mitochondrial reactive oxygen species in lipotoxic hearts induce post-translational modifications of AKAP121, DRP1, and OPA1 that promote mitochondrial fission. Circ. Res. 122, 58–73. doi: 10.1161/circresaha.117.311307
Uhrin, P., Wang, D., Mocan, A., Waltenberger, B., Breuss, J. M., and Tewari, D. (2018). Vascular smooth muscle cell proliferation as a therapeutic target. Part 2: natural products inhibiting proliferation. Biotechnol. Adv. 36, 1608–1621. doi: 10.1016/j.biotechadv.2018.04.002
Vafai, S. B., and Mootha, V. K. (2012). Mitochondrial disorders as windows into an ancient organelle. Nature 491, 374–383. doi: 10.1038/nature11707
Vance, J. E. (1990). Phospholipid synthesis in a membrane fraction associated with mitochondria. J. Biol. Chem. 265, 7248–7256. doi: 10.1016/s0021-9258(19)39106-9
Wang, D., Uhrin, P., Mocan, A., Waltenberger, B., Breuss, J. M., and Tewari, D. (2018). Vascular smooth muscle cell proliferation as a therapeutic target. Part 1: molecular targets and pathways. Biotechnol. Adv. 36, 1586–1607. doi: 10.1016/j.biotechadv.2018.04.006
Wang, W., Chen, L., Shang, C., Jin, Z., Yao, F., Bai, L., et al. (2020). miR-145 inhibits the proliferation and migration of vascular smooth muscle cells by regulating autophagy. J. Cell Mol. Med. 24, 6658–6669. doi: 10.1111/jcmm.15316
Wang, W., Cheng, X., Lu, J., Wei, J., Fu, G., and Zhu, F. (2010). Mitofusin-2 is a novel direct target of p53. Biochem. Biophys. Res. Commun. 400, 587–592. doi: 10.1016/j.bbrc.2010.08.108
Wang, Y., Cao, W., Yu, Z., and Liu, Z. (2009). Downregulation of a mitochondria associated protein SLP-2 inhibits tumor cell motility, proliferation and enhances cell sensitivity to chemotherapeutic reagents. Cancer Biol. Ther. 8, 1651–1658. doi: 10.4161/cbt.8.17.9283
Wan-Xin, T., Tian-Lei, C., Ben, W., Wei-Hua, W., and Ping, F. (2012). Effect of mitofusin 2 overexpression on the proliferation and apoptosis of high-glucose-induced rat glomerular mesangial cells. J. Nephrol. 25, 1023–1030. doi: 10.5301/jn.5000089
Wen, J., Wang, J., Guo, L., Cai, W., Wu, Y., Chen, W., et al. (2019). Chemerin stimulates aortic smooth muscle cell proliferation and migration via activation of autophagy in VSMCs of metabolic hypertension rats. Am. J. Transl. Res. 11, 1327–1342.
Wilkins, M. R., Ghofrani, H.-A., Weissmann, N., Aldashev, A., and Zhao, L. (2015). Pathophysiology and treatment of high-altitude pulmonary vascular disease. Circulation 131, 582–590. doi: 10.1161/circulationaha.114.006977
Xin, Y., Wu, W., Qu, J., Wang, X., Lei, S., Yuan, L., et al. (2019). Inhibition of Mitofusin-2 promotes cardiac fibroblast activation via the PERK/ATF4 pathway and reactive oxygen species. Oxid. Med. Cell. Longev. 2019:3649808.
Xu, K., Chen, G., Li, X., Wu, X., Chang, Z., and Xu, J. (2017). MFN2 suppresses cancer progression through inhibition of mTORC2/Akt signaling. Sci. Rep. 7:41718.
Xu, L., Hao, H., Hao, Y., Wei, G., Li, G., and Ma, P. (2019). Aberrant MFN2 transcription facilitates homocysteine-induced VSMCs proliferation via the increased binding of c-Myc to DNMT1 in atherosclerosis. J. Cell Mol. Med. 23, 4611–4626. doi: 10.1111/jcmm.14341
Yang, C.-M., Lu, Y.-L., Chen, H.-Y., and Hu, M.-L. (2012). Lycopene and the LXRα agonist T0901317 synergistically inhibit the proliferation of androgen-independent prostate cancer cells via the PPARγ-LXRα-ABCA1 pathway. J. Nutr. Biochem. 23, 1155–1162. doi: 10.1016/j.jnutbio.2011.06.009
Yang, Y.-D., Li, M.-M., Xu, G., Feng, L., Zhang, E.-L., and Chen, J. (2019). Nogo-B receptor directs mitochondria-associated membranes to regulate vascular smooth muscle cell proliferation. Int. J. Mol. Sci. 20:2319. doi: 10.3390/ijms20092319
Zhang, D., Ma, C., Li, S., Ran, Y., Chen, J., Lu, P., et al. (2012). Effect of Mitofusin 2 on smooth muscle cells proliferation in hypoxic pulmonary hypertension. Microvasc. Res. 84, 286–296. doi: 10.1016/j.mvr.2012.06.010
Zhang, G.-E., Jin, H.-L., Lin, X.-K., Chen, C., Liu, X.-S., Zhang, Q., et al. (2013). Anti-tumor effects of Mfn2 in gastric cancer. Int. J. Mol. Sci. 14, 13005–13021. doi: 10.3390/ijms140713005
Zhao, T., Huang, X., Han, L., Wang, X., Cheng, H., and Zhao, Y. (2012). Central role of mitofusin 2 in autophagosome-lysosome fusion in cardiomyocytes. J. Biol. Chem. 287, 23615–23625. doi: 10.1074/jbc.m112.379164
Keywords: mitochondria, Mfn-2, cell proliferation, target, apoptosis
Citation: Xin Y, Li J, Wu W and Liu X (2021) Mitofusin-2: A New Mediator of Pathological Cell Proliferation. Front. Cell Dev. Biol. 9:647631. doi: 10.3389/fcell.2021.647631
Received: 30 December 2020; Accepted: 02 March 2021;
Published: 01 April 2021.
Edited by:
Xiaoqiang Tang, Sichuan University, ChinaReviewed by:
Xiaoqiu Tan, Southwest Medical University, ChinaSichong Ren, Chengdu Medical College, China
Copyright © 2021 Xin, Li, Wu and Liu. This is an open-access article distributed under the terms of the Creative Commons Attribution License (CC BY). The use, distribution or reproduction in other forums is permitted, provided the original author(s) and the copyright owner(s) are credited and that the original publication in this journal is cited, in accordance with accepted academic practice. No use, distribution or reproduction is permitted which does not comply with these terms.
*Correspondence: Xiaojing Liu, bGl1eHFAc2N1LmVkdS5jbg==
†These authors have contributed equally to this work