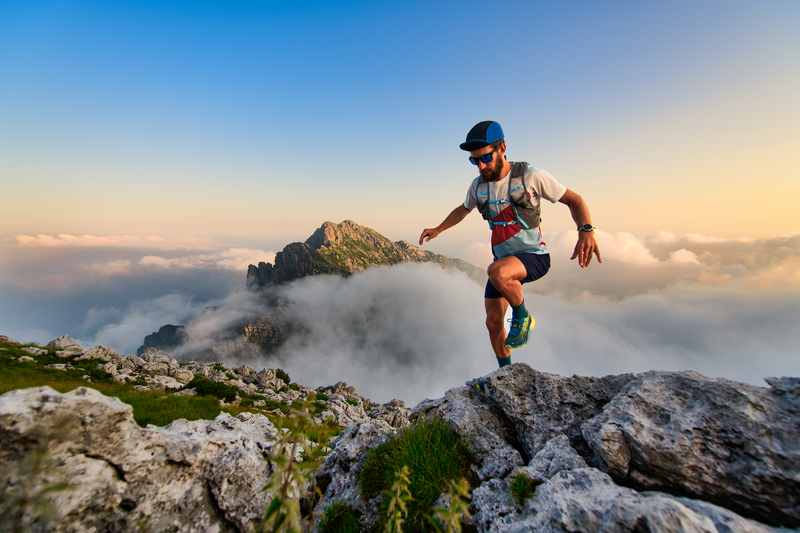
95% of researchers rate our articles as excellent or good
Learn more about the work of our research integrity team to safeguard the quality of each article we publish.
Find out more
REVIEW article
Front. Cell Dev. Biol. , 08 March 2021
Sec. Cell Growth and Division
Volume 9 - 2021 | https://doi.org/10.3389/fcell.2021.647149
This article is part of the Research Topic Biomimetic Materials for Tissue Regenerations View all 25 articles
For cosmetic and reconstructive purposes in the setting of small-volume adipose tissue damage due to aging, traumatic defects, oncological resections, and degenerative diseases, the current strategies for soft tissue replacement involve autologous fat grafts and tissue fillers with synthetic, bioactive, or tissue-engineered materials. However, they all have drawbacks such as volume shrinkage and foreign-body responses. Aiming to regenerate bioactive vascularized adipose tissue on biomaterial scaffolds, adipose tissue engineering (ATE) has emerged as a suitable substitute for soft tissue repair. The essential components of ATE include scaffolds as support, cells as raw materials for fat formation, and a tolerant local environment to allow regeneration to occur. The commonly loaded seeding cells are adipose-derived stem cells (ASCs), which are expected to induce stable and predictable adipose tissue formation. However, defects in stem cell enrichment, such as donor-site sacrifice, limit their wide application. As a promising alternative approach, cell-free bioactive scaffolds recruit endogenous cells for adipogenesis. In biomaterials without cell seeds, the key to sufficient adipogenesis relies on the recruitment of endogenous host cells and continuous induction of cell homing to scaffolds. Regeneration, rather than repair, is the fundamental dominance of an optimal mature product. To induce in situ adipogenesis, many researchers have focused on the mechanical and biochemical properties of scaffolds. In addition, efforts to regulate an angiogenic and adipogenic microenvironment in cell-free settings involve integrating growth factors or extracellular matrix (ECM) proteins onto bioactive scaffolds. Despite the theoretical feasibility and encouraging results in animal models, few of the reported cell-free biomaterials have been tested in humans, and failures of decellularized adipose tissues in adipogenesis have also been reported. In these cases, the most likely reason was the lack of supporting vasculature. This review summarizes the current status of biomaterials without cell seeds. Related mechanisms and influencing factors of in situ adipogenesis in cell-free biomaterials, dilemma in the development of biomaterials, and future perspectives are also addressed.
For cosmetic and reconstructive applications in the settings of adipose tissue damage due to aging and congenital or pathological conditions, such as traumatic defects, oncological resections, and degenerative diseases (Khan et al., 2012; Philips et al., 2014), the current strategies for delicate soft tissue replacement include autologous fat grafts, tissue fillers with synthetic materials, and adipose tissue engineering (ATE) (Gomillion and Burg, 2006; Choi et al., 2010). Autologous fat grafts have been acknowledged as comparatively ideal fillers because of their biocompatibility and lack of rejection immunoreaction. However, these drawbacks are also prominent. After autologous fat grafting, delayed revascularization, subsequent necrosis, and occupation of grafts by the host lead to oil cyst formation and progressive volume resorption up to 90% over time (Gomillion and Burg, 2006; Tanzi and Farè, 2009). Moreover, transplanted fat cells rarely proliferate. As a result, it is unreliable to obtain and maintain sufficient tissue augmentation only through free fat grafts. Tissue fillers remit the sacrifice of donor sites, but they often result in infection, allergic reactions, or foreign-body responses (Friedman, 1994; Gomillion and Burg, 2006; Sano et al., 2014). Similar to autologous fat grafts, they only temporarily augment volume without active self-regeneration of missing adipose tissue despite improvements in operative techniques and its popularity in the plastic market (Saylan, 2003; Itoi et al., 2010).
Aiming to regenerate bioactive vascularized adipose tissue on biomaterial scaffolds, ATE strategies have emerged as a suitable substitute for soft tissue repair (Tanzi and Farè, 2009). As outlined by Patrick (2000, 2001)) the three essential components of ATE include scaffolds as support, cells as raw materials for fat formation, and a tolerant local environment to allow these to happen. Adipogenesis can occur either in situ or de novo (Ahmed et al., 2008). In situ adipogenesis relies on pre-existing preadipocytes in the body, either from the surroundings or from other origins. The main techniques include fabricating and delivering biomaterials with or without bioactive factors and modifying a suitable microenvironment for the migration, proliferation, and differentiation of innate cells. This type of adipogenesis does not involve exogenous transplantation of cell seeds. De novo adipogenesis is based on encapsulating and transplanting cell seeds with the potential to proliferate and differentiate into adipose tissue and subsequent partial restoration of tissue functions at the expected body site (Hiraoka et al., 2006). Although preliminary research has revealed the clinical value of cell seeds, defects of stem cell enrichment, such as donor-site sacrifice, and most importantly, standardization, regulation, and concerns about biosafety, limit its wide application (Müller et al., 2020). As a promising alternative approach, cell-free bioactive scaffolds recruit endogenous cells for adipogenesis. This review summarizes the current status of biomaterials without cell seeds.
In clinical practice, soft tissue reconstruction can be used for cosmetic purposes and tissue defect repair. Common defect fillers include prostheses, autologous fillings, and bioactive materials. In addition, emerging tissue engineering techniques assist the above materials to improve the efficacy of reconstruction. The advantages and disadvantages of the major strategies used for the reconstruction of soft tissue defects are listed in Table 1.
Breast reconstruction is the most common strategy for soft tissue reconstruction for both esthetic and pathological repair purposes. Implants, including saline and silicone gel, have the advantages of low complication morbidity, mature technology, and the possibility of immediate reconstruction (Chang and Hammond, 2018). However, commercial implants may result in complications, such as infections (2.9%), capsular contracture (15–45%), and rupture (2–3.8%), and very low morbidity of implant-associated cancer, which could lead to implant loss (Prasad et al., 2019). This is due to the attachment and colonization of microorganisms and the formation of biofilms. However, the implants are also likely to suffer from migration and extrusion a long time after the procedure, which could cause patients to undergo a secondary surgery. More importantly, implants may only deal with soft tissue defects of certain shapes with available commercial products. The repair of irregular soft tissue defects or fine reconstruction of small-volume defects is less likely to be resolved by the prosthesis.
Autologous fillings are also widely used in soft tissue reconstruction, including autologous fat grafting and flaps. Autologous fat is considered an ideal filler because it is economical, accessible, and free of allergic reactions. It is used not only as a lipofiller but also for inducing adipose stem cells in tissue regenerative repair (Kling et al., 2013). As a result, it is widely used in facial, breast, and limb reconstruction (Simonacci et al., 2017). Moreover, the oncologic safety was also confirmed in a mouse residual breast cancer model (Silva et al., 2019). Nevertheless, the Achilles heel of fat grafting is necrosis, tissue resorption, and graft retention, with a high rate of weight and volume loss of less than 1 year after transplantation. Flap transplantation seems a better choice as it takes advantage of the vasculature of flaps and the recipient sites, which could guarantee long-term nutrition for the flaps (Abraham and Saint-Cyr, 2017). Despite the strengths of this technique, the decision regarding the size and location of the flap can only be roughly made, and the procedure always leaves obvious scars (Mohan et al., 2016; Abraham and Saint-Cyr, 2017). More importantly, the requirements of surgeons and the healthcare system limit its application (Alderman et al., 2011; Panchal and Matros, 2017).
Bioactive materials may also serve as soft tissue fillers, and some have already been approved by the Food and Drug Administration (FDA) and are commercially available. These materials, including hyaluronic acid, poly-L-lactic acid, calcium hydroxylapatite, and collagens, are absorbable and generally used in esthetic surgeries for small defects such as those of limbs and faces (Cohen et al., 2006; Ferneini and Ferneini, 2016). The surgical procedures using these soft tissue fillers are simple and rarely result in rejection reactions, although there are still risks of inflammation (Ferneini and Ferneini, 2016). However, these bioactive materials cannot be used to fill large volumes, such as the reconstruction of breasts (Lemperle et al., 2003). In addition, these fillers are gradually absorbed and cannot achieve long-term soft tissue reconstruction, which requires repeated injections (Ferneini and Ferneini, 2016).
Tissue engineering is an emerging technique for soft tissue reconstruction after the regeneration of adipose-derived stem cells (ASCs) in grafted fat. In current clinical applications, cell-assisted lipotransfer (CAL) and extracellular matrix (ECM)-based materials, based on tissue engineering techniques, can be used to compensate for the deficiencies of other materials.
Adipose-derived stem cells, along with autologous fat grafting, are the most used to improve the survival rate and reduce postoperative retention through cell enrichment. The efficiency was reported by a meta-analysis study in which 696 patients in 25 studies were reviewed and analyzed (Laloze et al., 2018). Furthermore, the advantage of CAL is only prominent in small-volume fat grafting. Kokai et al. (2019) applied an allograft adipose matrix (AAM) injection in the dorsal wrist of 15 patients, and all the patients displayed thickened wrist skin and diminished imprint of veins and tendons. However, wrist pain, injection site redness, and swelling were mostly reported in patients, and an average of 47% retention of the graft was observed.
In soft tissue regeneration, the clinical practice of the acellular dermal matrix (ADM) as a cell-free matrix dates back to as early as the 1990s. Similar to CAL, ADM is also applied with implants to overcome problems such as capsular contracture, seroma, and infection (Hunsicker et al., 2017). The matrix extract, as a soft tissue covering and prosthesis-based structural support, is sutured to the surface of the muscle, and then the prosthesis is fixed between the ADM and the muscle, which also provides an opportunity for immediate soft tissue reconstruction (Salzberg, 2012; Hadad et al., 2015). However, ADM is expensive, which limits its clinical application (Glasberg, 2017).
Adipose-derived stem cells are commonly loaded seeding cell populations in bioscaffolds (Bauer-Kreisel et al., 2010; Choi et al., 2010; Khan et al., 2012; Philips et al., 2014). Having been well described with the capacity to induce stable and predictable adipose tissue formation, ASCs within the stromal vascular fraction of adipose tissue have been suggested as a rational strategy to engineer mature adipose tissue (Zielins et al., 2016; Brett et al., 2017). In vitro studies have shown that human ASCs (hASCs) are capable of self-assembly in the presence of serum, ascorbic acid, and rosiglitazone and form dense adipocyte-containing cell sheets with an organized ECM (Vallee et al., 2009). In vivo experiments in animal models have comprehensively demonstrated the beneficial effects of ASC-loaded scaffolds on adipogenesis (Baglio et al., 2012). For example, when a scaffold composed of decellularized human adipose tissue and methacrylated glycol chitosan was seeded with 1 × 106 allogeneic rat ASCs isolated from the epididymal fat pad, the rate of scaffold degradation and inflammatory cell infiltration and angiogenesis in the scaffold regions were enhanced as compared with those for unseeded control scaffolds (Cheung et al., 2014). hASCs showed similar properties in that mature adipose tissue was formed after hASCs were subcutaneously implanted into nude mice (Tsuji et al., 2009). Another widely researched type of cell seed is stem cells derived from bone marrow (BMSCs) isolated from mature adult tissue (Pittenger et al., 1999; Brett et al., 2017). In in vitro studies, human BMSCs have been found to undergo adipogenic differentiation when cultured with basic fibroblast growth factor (bFGF), also known as fibroblast growth factor-2 (FGF-2) or fibroblast growth factor-beta (FGF-β), on gelatin scaffolds or polylactide-co-glycolide (Hong et al., 2005; Neubauer et al., 2005). In animal studies, rabbit BMSCs treated with adipogenic medium generated new adipose tissue after they were implanted in immunocompromised mice (Choi et al., 2005). Moreover, Alhadlaq et al. (2005) subcutaneously implanted poly(ethylene glycol) dacrylate-scaffold-encapsulated human BMSCs into SCID mice after in vitro adipogenic differentiation and observed de novo lipid-containing tissue. However, although mesenchymal stem cells (MSCs) have revealed potential in ATE in animal models, results from clinical studies have scarcely been reported. Apart from serving as a reservoir for the generation of new adipocytes, cell-rich fillers also reduce wound contraction and advance wound healing, in addition to promoting adipogenesis (Debels et al., 2017).
While the value of cell seeds in adipogenesis has been well described, the shortcomings of cells are intractable. First, since seeded cells are preferably homologous, donor-site injury is unavoidable. It could be troublesome for patients with low body weight where donor-site local liposuction is unwanted (Badylak, 2002; Gomillion and Burg, 2006). In this circumstance, multiple surgical procedures consume more time before the final cosmetic results are obtained, and patients need to bear an additional financial burden. Second, the isolation and culture techniques of ASCs have not been standardized, while ASCs are very sensitive to the manufacturing processes and might be profoundly influenced, thus wrecking the predictability of cell-seeded bioactive scaffolds (Bourin et al., 2013). Moreover, the high manufacturing cost of stem cell processes has limited its application in clinical use (Lee et al., 2014). Most crucially, difficulties in cell fate control are yet to be overcome in stem cell-assisted biomaterials (Baglio et al., 2012). Especially for patients with soft tissue defects resulting from malignant tumor resection, oncological safety could be the priority when considering accepting reconstruction. However, no study has achieved long-term observation of cell culture systems for a definite proof of safety or risks for in vivo applications to date (Lee et al., 2014).
Furthermore, the enhancement of adipogenesis through ASCs is believed to be contemporary. CAL, that is, ASC-enriched lipotransfer, has been promoted to address the variability in fat graft retention in autologous fat grafting (Kølle et al., 2013). In autologous fat grafting, adipocytes are predisposed to apoptosis and cell death due to ischemic conditions. However, with ASC assistance, a process of dynamic remodeling of adipose tissue after non-vascularized grafting was suggested (Eto et al., 2012). Fu et al. (2013) isolated green fluorescence protein (GFP)-positive ASCs from C57BL/6J-GFP mice, mixed them with minced inguinal adipose tissue harvested from wild-type C57BL/6J mice, and then co-implanted them into BALB/c nude mice. GFP-labeled adipocytes were observed 7 days after implantation. However, the fluorescence signal intensity fell drastically within the first 14 days and continued to drop thereafter (Fu et al., 2013), suggesting insufficient durability of these new adipocytes.
As a promising alternative approach, cell-free bioactive scaffolds overcome the limitations of stem cell seeding and recruit endogenous cells for adipogenesis (Hiraoka et al., 2006; Ju et al., 2014; Lih et al., 2016). The rationality and feasibility of in situ adipogenesis without cell seeds have been discussed (Lee et al., 2008).
Previous research has indicated that neovascularization is exclusively derived from endogenous cells through vascular remodeling, instead of exogenous cells in tissue-engineered vascular grafts (Marra et al., 2008). Rather than directly differentiating into blood vessels, exogenous cells of tissue-engineered grafts promoted the regeneration of endogenous cells (Hibino et al., 2011). Theoretically, soft tissue substitutions without seeding of exogenous cells could induce in situ adipogenesis and would be adequate for defect repair (Neels et al., 2004; Kelly et al., 2006; Marra et al., 2008). To induce in situ adipogenesis, many researchers have focused on the mechanical and biochemical properties of the adopted scaffolds. In addition, efforts have been made to regulate an angiogenic and adipogenic microenvironment in cell-free settings. The main strategies involved integrating growth factors or ECM proteins onto bioactive scaffolds (Yuksel et al., 2000a, b; Neels et al., 2004; Nillesen et al., 2007).
Extracellular matrices, a microenvironment with membrane proteins and growth factors, are closely associated with the induction of cell proliferation, migration, and differentiation. In natural processes, ECMs provide an environment that facilitates cell migration and nutrient diffusion. Based on the characteristics of ECMs, acellular adipose-derived matrix is the most common seed-free scaffold used in vivo, as it could provide a more biomimetic environment for adipogenesis and vascularization by promoting cell adhesion and proliferation, forming neo-formative adipose tissue similar to the natural morphology of adipocytes in native adipose tissue (Wu et al., 2012) and ensuring the long-term viability of adipose stem cells inside (Rossi et al., 2018).
Extracellular matrices are loose, porous structures composed of architectural and biochemical cues. The ECM derived from adipose tissue appears to be a white soft tissue and is always prepared with other compounds to form a hydrogel that can provide a stable cell niche and mechanical transduction pathway for adipose stem cell migration, proliferation, and differentiation (Kim et al., 2017). Flynn (2010) described the architecture of decellularized adipose tissue, which is composed of collagen fibers, decellularized vascular structures, and void spaces. The main components of the derived ECM structure include collagens, glycosaminoglycan elastin, and laminin. The structure determines the basic physical properties and basic functions of ECMs (Song et al., 2018; Thomas-Porch et al., 2018). As a physiological structure for cell activity, its porosity and extensive surface area play vital roles in cell penetration, adhesion, and proliferation (Song et al., 2018).
In addition, cell-free ECMs are highly biocompatible. According to Young et al. (2014), after the injection of decellularized adipose matrix hydrogels in mice, the mice showed high tolerance, presenting few adverse signs, and capsule formation was not observed.
The bioactive components in ECMs are essential for the initiation and progression of adipogenesis and regulate the surrounding cell activities. The biochemical cues contain a widely distributed basement membrane of ECMs, including laminin, elastin, fibronectin, and glycosaminoglycans (GAGs), which promote the structural adhesion and integrity of cells, as well as endogenous growth factors, including bFGF, vascular endothelial growth factor (VEGF), hepatocyte growth factor (HGF), platelet-derived growth factor (PDGF), insulin-like growth factor 1 (IGF-1), and transforming growth factor-beta 1(TGF-β1) (Flynn, 2010; Lu et al., 2014; Kim et al., 2015; Rossi et al., 2018).
The regulation of cell recruitment, preadipocyte induction, and cell proliferation and differentiation depends on the bioactive cues inside the ECM. An effective ability to promote angiogenesis, adipo-induction, and lipid accumulation has been detected in decellularized ECM scaffolds (Jeon et al., 2020). Regardless of the addition of ASCs, the newly formed adipocytes were all host derived, suggesting that the ECM itself can recruit preadipocytes from hosts (Young et al., 2014). As observed by Kim et al. (2017), migration of host cells to the ECM scaffold was observed after angiogenesis, indicating the adipo-inducing function of vascularization factors. And this was confirmed by a study on DAT, which suggested that an enhanced concentration of bFGF could significantly improve adipogenesis (Lu et al., 2014). As one of the basic components of adipose-derived ECMs, bFGF not only promotes early angiogenesis by mobilizing endothelial cell infiltration for vascular network construction but also serves as an adipogenic-stimulating factor by inducing surrounding preadipocytes by enhancing the activity of factors that regulate both enhancer-binding protein-alpha (C/EBPα) and peroxisome proliferator-activated receptor-γ (PPAR-γ) expressions (Prusty et al., 2002; Marquez et al., 2017). Simultaneously, they compared the gene expression in endogenous adipose tissue with that in newly formed adipose tissue, and in addition to natural gene expression of PPAR-γ, adipocyte protein-2 (AP-2), pre-adipocyte factor-1 (Pref-1), and tumor necrosis factor α (TNF-α), the levels of C/EBP α, adiponectin, and glucose transporter-4 (Glut-4) were detected. ECMs can induce endogenous cell migration, proliferation, and differentiation by relying on a sequential multistep process that activates several transcription factors. In gene analysis, ECMs express major transcription factors in adipogenic differentiation including the expression of C/EBPα, which is able to induce adipogenesis in precursor cell lines that are susceptible to the expression of PPAR-γ, critical for adipogenesis promotion, as well as marker genes of the terminal process in adipogenic differentiation (Sarjeant and Stephens, 2012; Rossi et al., 2018; Hoefner et al., 2020; Figure 1). In addition, other adipogenic genes, such as aP2, adiponectin, leptin, and lipoprotein lipase, were observed in ECMs (Cheung et al., 2014; Jeon et al., 2020). These adipogenic genes may help promote a quicker induction of adipogenesis (Hoefner et al., 2020). Moreover, the increase in glycerol-3-phosphate dehydrogenase (GPDH) enzyme activity, representing the adipogenesis process, is closely associated with the adipogenic differentiation potential of ECMs without exogenous adipogenic factors (Cheung et al., 2014; Jeon et al., 2020).
Figure 1. Adipogenic flow in ECMs. ECMs express major transcription factors in the adipogenic differentiation including C/EBPα and PPAR-γ and induce endogenous cell migration, proliferation, and differentiation (ECM: extracellular matrix; PPARγ: peroxisome proliferator-activated receptor gamma; C/EBPα: CCAAT/enhancer-binding protein α).
With better biocompatibility and biodegradability compared with synthetic polymers, ECMs derived from living tissues provide a suitable microenvironment that interacts with cellular components and integrates with the surrounding tissues of recipient sites (Choi et al., 2014; Sano et al., 2014). As a natural bioscaffold, the microenvironment created by ECMs is tissue specific (Choi et al., 2012; Gattazzo et al., 2014). In particular, adipose-derived ECMs naturally consist of collagen, elastin, adhesion peptides, and sophisticated substances released by adipose tissue that facilitate cell recruitment, attachment, proliferation, and differentiation (Ramirez and Rifkin, 2003). Thus, both the adipo-inductive and adipo-conductive properties are preserved (Yu et al., 2013; Han et al., 2015). The current mainstream manufacturing technique for extracting ECMs from adipose tissues is decellularization (Tanzi and Farè, 2009; Choi et al., 2010). Ideally, decellularization eliminates cells and lipids in fat, while fully preserving the three-dimensional (3D) structural and adipose-like properties of ECMs (Tanzi and Farè, 2009). However, altered decellularization methods can result in varied structural and proteomic characteristics, and all current decellularization methods inevitably result in the disruption of the ECM structure and composition to some extent. Comparison among the three decellularization measures, including enzymatic-based, detergent-based, and solvent-based methods, showed varied and inadequate decellularization and breakage of tissue architecture in the solvent agent (Thomas-Porch et al., 2018), although the composition of the functional components was similar among the three different scaffolds. It should also be noted that none of the current decellularization strategy ensures the entire removal of cell and antigen components. After decellularization, the remaining adipose matrix has pore sizes ranging from 50 to 150 μm, which is optimum for cells to migrate and settle (Yannas et al., 1989; Roehm et al., 2016). Furthermore, ECMs can be made into injectable hydrogels to fill irregularly shaped defects (Young et al., 2011; Turner et al., 2012; Giatsidis et al., 2019). At present, both porcine and human adipose tissue-derived ECMs have been reported as cell-free scaffolds for adipogenesis.
Kim et al. (2015, 2017) manufactured a soluble ECM from human adipose tissue and prepared hydrogels by mixing it with a methylcellulose solution (Choi et al., 2011). After subcutaneous injection into the backs of nude mice, accumulated intracellular lipid droplets emerged, and their levels increased significantly from weeks 2 to 3, and well-organized lobule-like structures of adipose tissue and microvessel formation were observed at week 3 (Kim et al., 2017). As tested by RT-PCR, the expression of adipocyte protein 2 (aP2), the mouse gene for adipogenesis, as well as other adipogenic genes, PPARγ, adiponectin, and leptin were expressed. In addition, angiogenesis in such cell-free hydrogels can be strengthened by angiogenic factors, such as VEGF, by recruiting and activating endogenous endothelial cells (Kim et al., 2017). Another study implanted a human acellular adipose-derived matrix in rats. Adipogenesis was observed at the periphery of the implant, and satisfying fat integration was achieved starting at the edges of the scaffold (Wu et al., 2012). In an in vivo study, human decellularized adipose tissue was combined with methacrylated glycol chitosan or chondroitin sulfate forming hydrogels and injected subcutaneously into Wistar rats. By week 12, scanty adipocytes were still present in the unseeded arm (Cheung et al., 2014). According to Kokai et al. (2019), a human-derived, injectable allograft adipose matrix (AAM) extracted from cadaveric human adipose contains essential collagens and growth factors that play vital roles in adipogenesis, and the implantation of the matrix in mice could support adipose regeneration in situ. The AAM used in this experiment was obtained from the processed human adipose tissue of cadaveric donors, where cells were removed to minimize the immune reaction, which was also used by Giatsidis et al. (2019) to reconstruct soft tissue defects in mice. The advantages of injecting AAM to induce adipogenesis were reflected in the satisfactory quality of graft and neo-formative adipocytes, where the formation of cystic areas representing adipocyte necrosis was not observed, as well as a higher density of blood vessels. Researchers also introduced an external volume expansion (EVE) model, performed as skin preconditioning using a 1–3 cm silicone cup connected to a vacuum pump and applied to the dorsum of animals to suction at 25 mmHg six times per day. Compared to AAM alone, incorporation of both EVE and AAM resulted in better adipogenesis, presenting as a greater number of adipocytes and satisfactory angiogenesis. The application of the graft was also studied in humans, and satisfactorily enhanced skin and subcutaneous tissue was harvested with some minor observations, including wrist pain, redness, and swelling, as well as graft retention (Kokai et al., 2019). Easily available with no need for donor-site sacrifice, the acellular porcine adipose matrix has been thought to provide properties comparable to those of acellular human adipose matrices (Roehm et al., 2016). Tan et al. (2019) introduced a hydrogel from acellular porcine adipose tissue (HAPA) as a novel allogenic biomaterial that could induce adipocyte regeneration in wound tissue. Compared with those in the control group, better adipogenesis, angiogenesis, and wound healing were observed in mice injected with HAPA. Although neogenetic adipocytes and vessels in the ASC-seeded HAPA group were more satisfactory in this study than those for HAPA alone, the angiogenesis microenvironment that HAPA provided could trigger adipogenesis, probably by activating the associated transcription factors and inducing adipocyte regeneration. A novel adipogenic matrix, Adipogel, is derived from the ECMs of decellularized porcine adipose tissue and can also be manufactured from other species, including rats and humans (Debels et al., 2017). Subcutaneous thoracic implantation of Adipogel in rats depicts the clinical setting where soft tissue defects are adjacent to existing fat. Eight weeks after thoracic implantation, the majority of Adipogel implants demonstrated large clusters of adipocytes, although not as closely packed as in mature neighboring fat (Debels et al., 2017). After lumber implantations that mimicked defects where there was minimal adjacent fat, such as scarred tissue, implants were hardly found. However, adipocytes were observed wherever the implants were identified. At both implantation sites, differentiating preadipocytes were found among mature adipocytes, as depicted by perilipin staining. Thus, this cell-free biomaterial effectively maintained a space for tissue ingrowth and induced adipogenic differentiation from migrated endogenous cells (Debels et al., 2017).
Biosynthetic polymers have been widely investigated as scaffolds for ATE because their physical properties are modifiable and can be scalable. However, synthetic scaffolds can result in fibrous encapsulation or a severe inflammatory response and adverse events such as extrusion, cyst formation, and overlying skin ulceration. These inherent shortcomings have limited its development (O’Brien, 2011; Brett et al., 2017).
Chang et al. (2018) reported a synthetic cell-free Gelatin Cryogel (GC) scaffold coated with polydopamine (PDA) and immobilized platelets (Plts) that formed an injectable and safe chamber to regenerate large adipose tissues. This chamber sustained high levels of PDGF and VEGF released from Plts, which indicates more blood supplementation and less hypoxia. Moreover, GC is degradable, and the host cells can migrate into scaffolds after degradation and start a natural fiber network generation, which forms a natural ECM and induces less inflammatory reaction. These factors resulted in a large volume of adipose tissue.
Hyaluronan (HA) hydrogels have been widely used as defect fillers and have been used for tissue engineering in bones (Hulsart-Billström et al., 2018), cartilage (Toh et al., 2010), intervertebral disc (Calderon et al., 2010), and soft tissue (Sarkanen et al., 2012). Several injectable HA hydrogel materials have been approved by the FDA for clinical use, and HA hydrogel-related adverse events have been minimal (Rohrich et al., 2007). Sarkanen et al. (2011) incorporated adipose tissue extracts, a blend of a wide variety of components of mature adipose tissue, including VEGF, bFGF, adiponectin, angiogenin, and interleukin 6, into HA hydrogel to develop an acellular implant, namely, the ATE-HA implant. The implant gradually released bioactive factors ex vivo. In in vivo studies, the implants were placed under the dorsal subcutis of rodents, and triglyceride accumulation emerged as early as 1 week after implantation. Eventually, well-vascularized and nerved adipose tissues were observed at 40 weeks. The fat within the biomaterials was densely integrated and vessel accompanied, which was very similar to the native adipose tissue (Sarkanen et al., 2012). This acellular implant was considered to have performed well in terms of compatibility, bioactivity, and sustainability and has considerable potential for use in tissue engineering for sustained reconstruction of soft tissue defects.
In a study with 3D-printed biomaterials, polycaprolactone (PCL) particles were melted and 3D-printed as cylinders. Neutralized type I collagen solution was infused into the microchannels of the PCL scaffold, followed by gelation. PCL scaffolds were implanted in the inguinal fat pad in vivo in mice, and in situ adipogenesis was observed 4 weeks after implantation (Shah et al., 2017). However, such adipogenesis relied on the seeding of Pyrintegrin, an assisting substance that will be described in detail later in this review.
Despite the theoretical feasibility and encouraging results in animal models as well as in vitro experiments, failures of decellularized adipose tissues in adipogenesis have also been reported (Friedman, 1994; Yu et al., 2013). For example, an ECM product, Matrigel, was adipogenic only when it is in direct contact with adjacent fat in a mouse model (Kelly et al., 2006). The researchers inferred that the autograft could provide ECM components, paracrine factors, or chemokines that promote adipogenesis. For the chamber without cell seeding, the lack of a microenvironment for cell migration, adhesion, and proliferation was the probable reason for the failure. In an in vivo study, no vacuolated fat-like cells were found in bare bovine type I collagen 3 weeks after implantation in rat dorsal muscle if unseeded. Similarly, unsatisfactory adipogenic induction from direct implantation of decellularized adipose tissues alone was also reported (Zhang et al., 2016). In these cases, the most proposed reasons were a lack of supporting vasculature because of inadequate angiogenesis factors such as bFGF. A similar result was also observed for the decellularized ECM hydrogel that lacks bFGF, which could result in the disappearance of existing adipose tissue, indicating the vital role of bFGF during adipogenesis (Lu et al., 2014). In another study, hydrogel alone could induce little adipogenesis, while the addition of ASCs and/or TG could greatly improve adipogenesis by promoting vascularization, as stimulation of adipogenesis was observed following the formation of blood vessels. As a result, angiogenesis is closely associated with adipogenesis (Young et al., 2014). In summary, adipogenesis in unseeded materials should possess the ability to provide an appropriate environment and induce enough angiogenesis.
In biomaterials without cell seeds, the key to sufficient adipogenesis relies on the recruitment of endogenous host cells and continuous induction of cell homing to scaffolds because no exogenous mesenchymal cells could be utilized. Regeneration, rather than repair, is the fundamental dominance of an optimal mature product. In addition, it is expected to degrade smoothly and eventually be replaced by healthy functional host fat tissue. To achieve such properties, the adipogenic mechanisms in cell-free biomaterials should be explored. Influencing factors, including but not limited to recruiting potency, growth factor integration, biocompatibility, and mechanical characteristics, should be adequately considered and balanced.
In vivo studies have revealed better adipogenic efficacy of adipose-derived acellular matrix at fat-rich implantation sites than at fat-bare sites (Debels et al., 2017). For quite some time, it was widely believed that the cells migrating to cell-free scaffolds mainly come from the surrounding tissues. An up-to-date research suggested that hydrogels of decellularized porcine adipose tissue induce host-derived adipogenesis in nude mice. Unlike the ASC-seeded hydrogel, where islet-like adipocyte clusters seen with adipogenesis in the basal region deteriorated over time, adipocytes were scattered in the unseeded hydrogel with more adipogenesis observed on the basal side (Tan et al., 2017). As proposed by the authors, this disparity in the patterns of adipose tissue remodeling between seeded and unseeded matrices might be associated with differences in the cell origins of adipocytes. While little fat tissue existed subcutaneously in nude mice, adipocytes that emerged in the unseeded hydrogel were less likely to come from the surrounding fat.
Nearly any tissue in the body harbors some sort of progenitor cells, including, but not limited to, fat, muscle, dermis, brain, liver, heart, placenta, and circulating blood (Gage, 2000; Liu et al., 2009; Kalinina et al., 2011). The alternative origin of precursor cells could be bone marrow other than the direct addition of preadipocytes in an invasive way. The exact sources and migration routes of host cells remain unsolved. More specifically, are there any BMSCs homing to the cell-free scaffolds? If any, what percentage of cells was involved, and how did it occur? Answers to these questions are priceless for the development of bioactive fillers because bone marrow remains hemopoietic throughout the life span of a person and could be a natural inexhaustible cell reservoir for sustainable adipogenesis. Clinically, this might lead to a very stable long-term volume maintenance at the implant site.
The recruitment of BMSCs to regenerate the expected tissue is a sophisticated cascade of interactions. The remote signal is sensed before BMSCs are mobilized from niches in the bone marrow into the circulation. Then, BMSCs transit in the vascular system, adhere to the surface of endothelial cells across the vessels, and migrate toward the target areas. Subsequently, BMSCs proliferated and differentiated into mature adipocytes in situ (Liu et al., 2009; Figure 2).
Figure 2. Hypothetic sources of host cells in cell-free biomaterials. Around the biomaterials implanted in the soft tissue defects, ADSCs migrate from surrounding tissues toward the scaffolds and differentiate. BMSCs mobilize from niches in bone marrow into circulation, migrate to and colonize on the scaffolds, proliferation and differentiation into mature adipocytes. Other types of progenitor cells from other body parts may also serve as origins of host cells (ADSC: adipose-derived stem cell; BMSC: bone marrow-derived mesenchymal cell).
Cytokines and chemokines as chemoattractants largely influence the mobilization and homing of progenitor cells (Agrawal et al., 2011; Sicari et al., 2014). These chemoattractants can be released from the ECM scaffolds. Stem cell-activating molecules such as VEGF, HGF, IGF-1, and PDGF promote the recruitment of host ASCs from the nearby adipose tissue (Hu et al., 2015; Stuermer et al., 2015). While stromal cell-derived factor (SDF)-1a and its receptor, chemokine receptor type 4 (CXCR4), construct the most important functioning pathway in the homing of BMSCs (Liu et al., 2009), designing cell-free biomaterials that regulate the SDF-1-CXCR4 axis has not yet been attempted. Adipocytes are not the only type of cells in fat, and other types include inflammatory cells, pericytes, and fibroblasts (Eto et al., 2009; Yoshimura et al., 2011). Even though they constitute less than 10% of the fat volume summed up, they are indispensable in the whole picture of adipose tissue remodeling, and thus, they should not be neglected in the development of bioscaffolds.
In addition, implantation of unseeded materials induces the infiltration of immune cells such as neutrophils and macrophages from hosts. Adipose tissue macrophages, especially the M2 phenotype, are closely associated with working as major immune cell types in adipose tissues that are thought to play important roles in adipose tissue remodeling, which is an essential procedure during adipogenesis (Brown et al., 2012). Lilja et al. (2013) established a chamber to induce adipogenesis and found that the first host cells that migrated were macrophages and that adipose precursor cells accounted for only a minority of the migrated cells. Furthermore, the macrophages recruited from the host to ECM hydrogels induced adipose tissue remodeling and adipogenic activities (Kim et al., 2017).
Endogenous adipokines include bFGF, VEGF, TGF-β1, placental growth factor (PGF), IGF-1, HGF, and PDGF (Kim et al., 2017). One of the most widely adopted angiogenic factors to be enriched in biometric is bFGF (Lu et al., 2014). BFGF is naturally expressed in adipose tissue, and subcutaneous and omental adipose tissue mass can be regulated by bFGF, which is locally produced by adipocytes (Gabrielsson et al., 2002; Brett et al., 2017). Zhang et al. (2016) loaded allogeneic decellularized adipose tissue with bFGF and stem cells. Twelve weeks after injection in mice, only the bFGF-loaded implants resulted in significant in situ formation of adipose tissue that closely resembled the inherent fat in tissue architecture, whereas all the non-loaded implants degenerated (Zhang et al., 2016). bFGF was also incorporated onto minced collagen sponges and gelatin microspheres for release control. In the rabbit model, the histological area of in situ adipogenesis was enhanced over time by repeated administration (Lu et al., 2014). Furthermore, bFGF induced the formation of adipose tissue accompanied by microvasculature when subcutaneously injected into BALB/c mice with gelatin microspheres and Matrigel (Tabata et al., 2000).
Several other growth factors have been used in animal models. In a study by Yuksel et al., soluble IGF-1 was bound to poly(lactic-co-glycolic) acid (PLGA)/PEG microspheres and injected subcutaneously into the deep muscular fascia of the abdominal muscle wall in rats. Four weeks after injection, adipocyte formation was observed within the non-adipocyte cell depots (Yuksel et al., 2000c). Furthermore, the combination of growth factors (e.g., combination of bFGF, VEGF-A, and PDGF-BB) also significantly improved the formation of adipose tissue within subcutaneously implanted chambers in immunocompromised mice (Ting et al., 2014).
The effects of the incorporated growth factors on adipogenesis are dose sensitive. In an in vivo study on bFGF-loaded scaffolds, in situ adipogenesis accompanied with angiogenesis was observed in the implanted scaffold with 1.0 μg of bFGF per defect, and the extent was less at lower and higher bFGF doses. Presumably, an inadequate dose of bFGF was insufficient to exert its angiogenic or adipogenic effect, while bFGF overdose caused an inflammatory response at the implanted site and led to accelerated infiltration of fibrous tissues into the scaffold (Hiraoka et al., 2006). Therefore, finding an optimal concentration rather than simply adopting a maximal dose is pivotal in the development of biomaterials without cell seeds (Agrawal et al., 2011).
A considerable portion of efforts in the development of biomaterials has been devoted to enriching biomaterials with structural ECM components (Badylak, 2002). In ECM biomaterials, structural proteins offer architectural support to cells. Collagen types I–VI are dominant in structural proteins of adipose-derived ECM (Divoux and Clement, 2011). Interacting with other molecules, collagen VI regulates the extension of fat and insulin sensitivity (Mariman and Wang, 2010). Other structural proteins, including elastin, fibronectin, and glycosaminoglycans, may profoundly regulate cellular behaviors during adipogenesis (Nakajima et al., 2002). When structural proteins are applied, it should be noted that the effects of incorporated structural proteins in adipogenesis are dose sensitive. Specifically, while maintaining structural integrity and facilitating in vivo adipogenesis, excessive deposition of collagen is associated with pathological fibrosis during fat remodeling (Chun, 2012).
Other substances or particles have been suggested to assist adipogenesis in cell-free biomaterials. Pyrintegrin is a 2,4-disubstituted pyrimidine that induces the activation of β1 integrin and multiple growth factor receptors, including FGF receptor 1 (FGFR1), IGF-1 receptor (IGFR1), epidermal growth factor receptor 1 (EGFR1), and human epidermal growth factor receptor 2 (HER2) (Xu et al., 2010). It is capable of improving the survival of human embryonic stem cells (Xu et al., 2010), which upregulates PPARγ and C/EBPα, a pair of molecules that promote adipose cell differentiation toward fibroblasts or myoblasts (Kawai et al., 1985). Shah et al. (2017) implanted Pyrintegrin-adsorbed 3D-bioprinted PCL scaffolds in the inguinal fat pad in vivo in C57BL/6 mice and observed the formation of adipose tissue that was positive for Oil Red O. Moreover, mouse PPARγ was significantly overexpressed in the harvested tissue within the PCL collagen gel scaffolds (Shah et al., 2017). Given that no cell seeds were transplanted, the new adipose tissue formed by host cells in the native environment demonstrated in situ adipogenesis with no need for stem cell assistance.
On the one hand, cell-seeded materials remain the mainstream with great potential in the field of fine soft tissue reconstruction with biomaterials. On the other hand, the inherent shortcomings of stem cells make cell-free biomaterials an important alternative option. Adipogenic biomaterials without cell seeds overcome the limitations of stem cell enrichment, including donor-site sacrifice, potential oncological concerns, troublesome manufacturing processes, and extra cost.
While bearing notable advantages, the most significant restriction of cell-free bioscaffolds lies in the recruitment of endogenous host cells. Owing to the difficulties in cell labeling, the origins and mobilizing routes of host cells in in situ adipogenesis remain ambiguous. Once the potential pathways of BMSC homing are sufficiently explored and steadily manipulated in implanted biomaterials, long-term clinical volume maintenance could be realistic. In the research of cell-free biomaterials, apart from the extensively attempted importation of structural proteins and growth factors, some other influencing factors have been mentioned but have yet to be thoroughly investigated, including stiffness and pore diameters of scaffold and genetic homology of ECMs.
Despite the encouraging results of in vivo studies, only a few of the reported cell-free biomaterials have been tested in humans. Therefore, more efforts are needed to create practical and affordable biomaterials with optimal long-term cosmetic outcomes.
All authors made substantial contributions to the conception of this review and the critical appraisal of the literature summarized herein, wrote the manuscript, and approved the final version of this article before submission.
This work was supported by the China Postdoctoral Science Foundation Funded Project (2019M663511) and the Science and Technology Bureau of Sichuan (2020YJ0293).
The authors declare that the research was conducted in the absence of any commercial or financial relationships that could be construed as a potential conflict of interest.
Abraham, J. T., and Saint-Cyr, M. (2017). Keystone and pedicle perforator flaps in reconstructive surgery: new modifications and applications. Clin. Plast. Surg. 44, 385–402. doi: 10.1016/j.cps.2016.12.005
Agrawal, V., Tottey, S., Johnson, S. A., Freund, J. M., Siu, B. F., and Badylak, S. F. (2011). Recruitment of progenitor cells by an extracellular matrix cryptic peptide in a mouse model of digit amputation. Tissue Eng. Part A 17, 2435–2443. doi: 10.1089/ten.tea.2011.0036
Ahmed, T. A., Dare, E. V., and Hincke, M. (2008). Fibrin: a versatile scaffold for tissue engineering applications. Tissue Eng. Part B Rev. 14, 199–215. doi: 10.1089/ten.teb.2007.0435
Alderman, A. K., Atisha, D., Streu, R., Salem, B., Gay, A., Abrahamse, P., et al. (2011). Patterns and correlates of postmastectomy breast reconstruction by U.S. Plastic surgeons: results from a national survey. Plast. Reconstr. Surg. 127, 1796–1803. doi: 10.1097/PRS.0b013e31820cf183
Alhadlaq, A., Tang, M., and Mao, J. J. (2005). Engineered adipose tissue from human mesenchymal stem cells maintains predefined shape and dimension: implications in soft tissue augmentation and reconstruction. Tissue Eng. 11, 556–566. doi: 10.1089/ten.2005.11.556
Badylak, S. F. (2002). The extracellular matrix as a scaffold for tissue reconstruction. Semin. Cell Dev. Biol. 13, 377–383. doi: 10.1016/S1084952102000940
Baglio, S. R., Pegtel, D. M., and Baldini, N. (2012). Mesenchymal stem cell secreted vesicles provide novel opportunities in (stem) cell-free therapy. Front. Physiol. 3:359. doi: 10.3389/fphys.2012.00359
Bauer-Kreisel, P., Goepferich, A., and Blunk, T. (2010). Cell-delivery therapeutics for adipose tissue regeneration. Adv. Drug Deliv. Rev. 62, 798–813. doi: 10.1016/j.addr.2010.04.003
Bourin, P., Bunnell, B. A., Casteilla, L., Dominici, M., Katz, A. J., March, K. L., et al. (2013). Stromal cells from the adipose tissue-derived stromal vascular fraction and culture expanded adipose tissue-derived stromal/stem cells: a joint statement of the International Federation for Adipose Therapeutics and Science (IFATS) and the International Society for Cellular Therapy (ISCT). Cytotherapy 15, 641–648. doi: 10.1016/j.jcyt.2013.02.006
Brett, E., Chung, N., Leavitt, W. T., Momeni, A., Longaker, M. T., and Wan, D. C. (2017). A review of cell-based strategies for soft tissue reconstruction. Tissue Eng. Part B Rev. 23, 336–346. doi: 10.1089/ten.teb.2016.0455
Brown, B. N., Londono, R., Tottey, S., Zhang, L., Kukla, K. A., Wolf, M. T., et al. (2012). Macrophage phenotype as a predictor of constructive remodeling following the implantation of biologically derived surgical mesh materials. Acta Biomater. 8, 978–987. doi: 10.1016/j.actbio.2011.11.031
Calderon, L., Collin, E., Murphy, M., O’Halloran, D., and Pandit, A. (2010). Type II collagen-hyaluronan hydrogel-a step towards a scaffold for intervertebral disc tissue engineering. Eur. Cell Mater. 20, 134–148. doi: 10.22203/eCM.v020a12
Chang, E. I., and Hammond, D. C. (2018). Clinical results on innovation in breast implant design. Plast. Reconstr. Surg. 142(4S The Science of Breast Implants), 31s–38s. doi: 10.1097/prs.0000000000005000
Chang, Q., Cai, J., Wang, Y., Yang, R., Xing, M., and Lu, F. (2018). Large adipose tissue generation in a mussel-inspired bioreactor of elastic-mimetic cryogel and platelets. J. Tissue Eng. 9:2041731418808633. doi: 10.1177/2041731418808633
Cheung, H. K., Han, T. T., Marecak, D. M., Watkins, J. F., Amsden, B. G., and Flynn, L. E. (2014). Composite hydrogel scaffolds incorporating decellularized adipose tissue for soft tissue engineering with adipose-derived stem cells. Biomaterials 35, 1914–1923. doi: 10.1016/j.biomaterials.2013.11.067
Choi, B., Kim, S., Lin, B., Wu, B. M., and Lee, M. (2014). Cartilaginous extracellular matrix-modified chitosan hydrogels for cartilage tissue engineering. ACS Appl. Mater. Interfaces 6, 20110–20121. doi: 10.1021/am505723k
Choi, J. H., Gimble, J. M., Lee, K., Marra, K. G., Rubin, J. P., Yoo, J. J., et al. (2010). Adipose tissue engineering for soft tissue regeneration. Tissue Eng. Part B Rev. 16, 413–426. doi: 10.1089/ten.teb.2009.0544
Choi, J. S., Kim, B. S., Kim, J. Y., Kim, J. D., Choi, Y. C., Yang, H. J., et al. (2011). Decellularized extracellular matrix derived from human adipose tissue as a potential scaffold for allograft tissue engineering. J. Biomed. Mater. Res. A 97, 292–299. doi: 10.1002/jbm.a.33056
Choi, J. S., Kim, J. D., Yoon, H. S., and Cho, Y. W. (2012). Full-thickness skin wound healing using human placenta-derived extracellular matrix containing bioactive molecules. Tissue Eng. Part A 19, 329–339. doi: 10.1089/ten.tea.2011.0738
Choi, Y. S., Park, S.-N., and Suh, H. (2005). Adipose tissue engineering using mesenchymal stem cells attached to injectable PLGA spheres. Biomaterials 26, 5855–5863. doi: 10.1016/j.biomaterials.2005.02.022
Chun, T. H. (2012). Peri-adipocyte ECM remodeling in obesity and adipose tissue fibrosis. Adipocyte 1, 89–95. doi: 10.4161/adip.19752
Cohen, S. R., Berner, C. F., Busso, M., Gleason, M. C., Hamilton, D., Holmes, R. E., et al. (2006). ArteFill: a long-lasting injectable wrinkle filler material–summary of the U.S. Food and Drug Administration trials and a progress report on 4- to 5-year outcomes. Plast. Reconstr. Surg. 118(Suppl. 3), 64s–76s. doi: 10.1097/01.prs.0000234873.00905.a4
Coroneos, C. J., Selber, J. C., Offodile, A. C. II, Butler, C. E., and Clemens, M. W. (2019). US FDA breast implant postapproval studies: long-term outcomes in 99,993 patients. Ann. Surg. 269, 30–36. doi: 10.1097/sla.0000000000002990
Debels, H., Gerrand, Y. W., Poon, C. J., Abberton, K. M., Morrison, W. A., and Mitchell, G. M. (2017). An adipogenic gel for surgical reconstruction of the subcutaneous fat layer in a rat model. J. Tissue Eng. Regen. Med. 11, 1230–1241. doi: 10.1002/term.2025
Divoux, A., and Clement, K. (2011). Architecture and the extracellular matrix: the still unappreciated components of the adipose tissue. Obes. Rev. 12, e494–e503. doi: 10.1111/j.1467-789X.2010.00811.x
Duteille, F., Perrot, P., Bacheley, M. H., and Stewart, S. (2018). Eight-year safety data for round and anatomical silicone gel breast implants. Aesthet. Surg. J. 38, 151–161. doi: 10.1093/asj/sjx117
Eto, H., Kato, H., Suga, H., Aoi, N., Doi, K., Kuno, S., et al. (2012). The fate of adipocytes after nonvascularized fat grafting: evidence of early death and replacement of adipocytes. Plast. Reconstr. Surg. 129, 1081–1092. doi: 10.1097/PRS.0b013e31824a2b19
Eto, H., Suga, H., Matsumoto, D., Inoue, K., Aoi, N., Kato, H., et al. (2009). Characterization of structure and cellular components of aspirated and excised adipose tissue. Plast. Reconstr. Surg. 124, 1087–1097. doi: 10.1097/PRS.0b013e3181b5a3f1
Ferneini, E. M., and Ferneini, A. M. (2016). An overview of vascular adverse events associated with facial soft tissue fillers: recognition, prevention, and treatment. J. Oral Maxillofac. Surg. 74, 1630–1636. doi: 10.1016/j.joms.2016.03.009
Flynn, L. E. (2010). The use of decellularized adipose tissue to provide an inductive microenvironment for the adipogenic differentiation of human adipose-derived stem cells. Biomaterials 31, 4715–4724. doi: 10.1016/j.biomaterials.2010.02.046
Friedman, R. J. (1994). Silicone breast prostheses implantation and explantation. Semin. Arthritis Rheum. 24, 8–10. doi: 10.1016/0049-0172(94)90103-1
Fu, S., Luan, J., Xin, M., Wang, Q., Xiao, R., and Gao, Y. (2013). Fate of adipose-derived stromal vascular fraction cells after co-implantation with fat grafts: evidence of cell survival and differentiation in ischemic adipose tissue. Plast. Reconstr. Surg. 132, 363–373. doi: 10.1097/PRS.0b013e31829588b3
Gabrielsson, B. G., Johansson, J. M., Jennische, E., Jernås, M., Itoh, Y., Peltonen, M., et al. (2002). Depot-specific expression of fibroblast growth factors in human adipose tissue. Obes. Res. 10, 608–616. doi: 10.1038/oby.2002.83
Gage, F. H. (2000). Mammalian neural stem cells. Science 287, 1433–1438. doi: 10.1126/science.287.5457.1433
Gattazzo, F., Urciuolo, A., and Bonaldo, P. (2014). Extracellular matrix: a dynamic microenvironment for stem cell niche. Biochim. Biophys. 1840, 2506–2519. doi: 10.1016/j.bbagen.2014.01.010
Giatsidis, G., Succar, J., Waters, T. D., Liu, W., Rhodius, P., Wang, C., et al. (2019). Tissue-engineered soft-tissue reconstruction using noninvasive mechanical preconditioning and a shelf-ready allograft adipose matrix. Plast. Reconstr. Surg. 144, 884–895. doi: 10.1097/prs.0000000000006085
Glasberg, S. B. (2017). The economics of prepectoral breast reconstruction. Plast. Reconstr. Surg. 140(6S Prepectoral Breast Reconstruction), 49s–52s. doi: 10.1097/prs.0000000000004051
Gomillion, C. T., and Burg, K. J. (2006). Stem cells and adipose tissue engineering. Biomaterials 27, 6052–6063. doi: 10.1016/j.biomaterials.2006.07.033
Hadad, I., Liu, A. S., and Guo, L. (2015). A new approach to minimize acellular dermal matrix use in prosthesis-based breast reconstruction. Plast. Reconstr. Surg. Glob. Open 3:e472. doi: 10.1097/gox.0000000000000433
Han, T. T., Toutounji, S., Amsden, B. G., and Flynn, L. E. (2015). Adipose-derived stromal cells mediate in vivo adipogenesis, angiogenesis and inflammation in decellularized adipose tissue bioscaffolds. Biomaterials 72, 125–137. doi: 10.1016/j.biomaterials.2015.08.053
Hibino, N., Villalona, G., Pietris, N., Duncan, D. R., Schoffner, A., Roh, J. D., et al. (2011). Tissue-engineered vascular grafts form neovessels that arise from regeneration of the adjacent blood vessel. FASEB J. 25, 2731–2739. doi: 10.1096/fj.11-182246
Hiraoka, Y., Yamashiro, H., Yasuda, K., Kimura, Y., Inamoto, T., and Tabata, Y. (2006). In situ regeneration of adipose tissue in rat fat pad by combining a collagen scaffold with gelatin microspheres containing basic fibroblast growth factor. Tissue Eng. 12, 1475–1487. doi: 10.1089/ten.2006.12.1475
Hoefner, C., Muhr, C., Horder, H., Wiesner, M., Wittmann, K., Lukaszyk, D., et al. (2020). Human adipose-derived mesenchymal stromal/stem cell spheroids possess high adipogenic capacity and acquire an adipose tissue-like extracellular matrix pattern. Tissue Eng. Part A 26, 915–926. doi: 10.1089/ten.TEA.2019.0206
Hong, L., Peptan, I., Clark, P., and Mao, J. J. (2005). Ex vivo adipose tissue engineering by human marrow stromal cell seeded gelatin sponge. Ann. Biomed. Eng. 33, 511–517. doi: 10.1007/s10439-005-2510-7
Hu, L., Yang, G., Hägg, D., Sun, G., Ahn, J. M., Jiang, N., et al. (2015). IGF1 promotes adipogenesis by a lineage bias of endogenous adipose stem/progenitor cells. Stem Cells 33, 2483–2495. doi: 10.1002/stem.2052
Hulsart-Billström, G., Selvaraju, R. K., Estrada, S., Lubberink, M., Asplund, V., Bergman, K., et al. (2018). Non-invasive tri-modal visualisation via PET/SPECT/μCT of recombinant human bone morphogenetic protein-2 retention and associated bone regeneration: a proof of concept. J. Control. Release 285, 178–186. doi: 10.1016/j.jconrel.2018.07.012
Hunsicker, L. M., Ashikari, A. Y., Berry, C., Koch, R. M., and Salzberg, C. A. (2017). Short-term complications associated with acellular dermal matrix-assisted direct-to-implant breast reconstruction. Ann. Plast. Surg. 78, 35–40. doi: 10.1097/sap.0000000000000742
Itoi, Y., Takatori, M., Hyakusoku, H., and Mizuno, H. (2010). Comparison of readily available scaffolds for adipose tissue engineering using adipose-derived stem cells. J. Plast. Reconstr. Aesthet. Surg. 63, 858–864. doi: 10.1016/j.bjps.2009.01.069
Jeon, E. Y., Joo, K. I., and Cha, H. J. (2020). Body temperature-activated protein-based injectable adhesive hydrogel incorporated with decellularized adipose extracellular matrix for tissue-specific regenerative stem cell therapy. Acta Biomater. 114, 244–255. doi: 10.1016/j.actbio.2020.07.033
Ju, Y. M., Atala, A., Yoo, J. J., and Lee, S. J. (2014). In situ regeneration of skeletal muscle tissue through host cell recruitment. Acta Biomater. 10, 4332–4339. doi: 10.1016/j.actbio.2014.06.022
Kalinina, N., Sysoeva, V. Y., Rubina, K. A., Parfenova, Y. V., and Tkachuk, V. A. (2011). Mesenchymal stem cells in tissue growth and repair. Acta Nat. 3, 30–37. doi: 10.32607/20758251-2011-3-4-30-37
Kawai, K., Tamaki, A., and Hirohata, K. (1985). Steroid-induced accumulation of lipid in the osteocytes of the rabbit femoral head. A histochemical and electron microscopic study. J. Bone Joint Surg. Am. 67, 755–763. doi: 10.2106/00004623-198567050-00010
Kelly, J. L., Findlay, M. W., Knight, K. R., Penington, A., Thompson, E. W., Messina, A., et al. (2006). Contact with existing adipose tissue is inductive for adipogenesis in matrigel. Tissue Eng. 12, 2041–2047. doi: 10.1089/ten.2006.12.2041
Khan, W. S., Adesida, A. B., Tew, S. R., Longo, U. G., and Hardingham, T. E. (2012). Fat pad derived mesenchymal stem cells as a potential source for cell-based adipose tissue repair strategies. Cell Prolif. 45, 111–120. doi: 10.1111/j.1365-2184.2011.00804.x
Kim, E. J., Choi, J. S., Kim, J. S., Choi, Y. C., and Cho, Y. W. (2015). Injectable and thermosensitive soluble extracellular matrix and methylcellulose hydrogels for stem cell delivery in skin wounds. Biomacromolecules 17, 4–11. doi: 10.1021/acs.biomac.5b01566
Kim, J. S., Choi, J. S., and Cho, Y. W. (2017). Cell-free hydrogel system based on a tissue-specific extracellular matrix for in situ adipose tissue regeneration. ACS Appl. Mater. Interfaces 9, 8581–8588. doi: 10.1021/acsami.6b16783
Kling, R. E., Mehrara, B. J., Pusic, A. L., Young, V. L., Hume, K. M., Crotty, C. A., et al. (2013). Trends in autologous fat grafting to the breast: a national survey of the American society of plastic surgeons. Plast. Reconstr. Surg. 132, 35–46. doi: 10.1097/PRS.0b013e318290fad1
Kokai, L. E., Schilling, B. K., Chnari, E., Huang, Y. C., Imming, E. A., Karunamurthy, A., et al. (2019). Injectable allograft adipose matrix supports adipogenic tissue remodeling in the nude mouse and human. Plast. Reconstr. Surg. 143, 299e–309e. doi: 10.1097/PRS.0000000000005269
Kølle, S.-F. T., Fischer-Nielsen, A., Mathiasen, A. B., Elberg, J. J., Oliveri, R. S., Glovinski, P. V., et al. (2013). Enrichment of autologous fat grafts with ex-vivo expanded adipose tissue-derived stem cells for graft survival: a randomised placebo-controlled trial. Lancet 382, 1113–1120. doi: 10.1016/S0140-6736(13)61410-5
Laloze, J., Varin, A., Gilhodes, J., Bertheuil, N., Grolleau, J. L., Brie, J., et al. (2018). Cell-assisted lipotransfer: friend or foe in fat grafting? Systematic review and meta-analysis. J. Tissue Eng. Regen. Med. 12, e1237–e1250. doi: 10.1002/term.2524
Lee, H. Y., Yang, H. J., Rhie, J. W., and Han, K. T. (2014). Adipose tissue regeneration in vivo using micronized acellular allogenic dermis as an injectable scaffold. Aesth. Plast. Surg. 38, 1001–1010. doi: 10.1007/s00266-014-0379-2
Lee, S. J., Van Dyke, M., Atala, A., and Yoo, J. J. (2008). Host cell mobilization for in situ tissue regeneration. Rejuvenation Res. 11, 747–756. doi: 10.1089/rej.2008.0691
Lemperle, G., Morhenn, V., and Charrier, U. (2003). Human histology and persistence of various injectable filler substances for soft tissue augmentation. Aesth. Plast. Surg. 27, 354–366; discussion367. doi: 10.1007/s00266-003-3022-1
Lih, E., Park, K. W., Chun, S. Y., Kim, H., Kwon, T. G., Joung, Y. K., et al. (2016). Biomimetic porous PLGA scaffolds incorporating decellularized extracellular matrix for kidney tissue regeneration. ACS Appl. Mater. Interfaces 8, 21145–21154. doi: 10.1021/acsami.6b03771
Lilja, H. E., Morrison, W. A., Han, X.-L., Palmer, J., Taylor, C., Tee, R., et al. (2013). An adipoinductive role of inflammation in adipose tissue engineering: key factors in the early development of engineered soft tissues. Stem Cells Dev. 22, 1602–1613. doi: 10.1089/scd.2012.0451
Liu, Z. J., Zhuge, Y., and Velazquez, O. C. (2009). Trafficking and differentiation of mesenchymal stem cells. J. Cell. Biochem. 106, 984–991. doi: 10.1002/jcb.22091
Lu, Q., Li, M., Zou, Y., and Cao, T. (2014). Delivery of basic fibroblast growth factors from heparinized decellularized adipose tissue stimulates potent de novo adipogenesis. J. Control. Release 174, 43–50. doi: 10.1016/j.jconrel.2013.11.007
Mariman, E. C., and Wang, P. (2010). Adipocyte extracellular matrix composition, dynamics and role in obesity. Cell. Mol. Life Sci. 67, 1277–1292. doi: 10.1007/s00018-010-0263-4
Marquez, M. P., Alencastro, F., Madrigal, A., Jimenez, J. L., Blanco, G., Gureghian, A., et al. (2017). The role of cellular proliferation in adipogenic differentiation of human adipose tissue-derived mesenchymal stem cells. Stem Cells Dev. 26, 1578–1595. doi: 10.1089/scd.2017.0071
Marra, K. G., DeFail, A. J., Clavijo-Alvarez, J. A., Badylak, S. F., Taieb, A., Schipper, B. M., et al. (2008). FGF-2 enhances vascularization for adipose tissue engineering. Plast. Reconstr. Surg. 121, 1153–1164. doi: 10.1097/01.prs.0000305517.93747.72
Mohan, A. T., Sur, Y. J., Zhu, L., Morsy, M., Wu, P. S., Moran, S. L., et al. (2016). The concepts of propeller, perforator, keystone, and other local flaps and their role in the evolution of reconstruction. Plast. Reconstr. Surg. 138, 710e–729e. doi: 10.1097/prs.0000000000002610
Müller, H., Dagher, G., Loibner, M., Stumptner, C., Kungl, P., and Zatloukal, K. (2020). Biobanks for life sciences and personalized medicine: importance of standardization, biosafety, biosecurity, and data management. Curr. Opin. Biotechnol. 65, 45–51. doi: 10.1016/j.copbio.2019.12.004
Nakajima, I., Muroya, S., Tanabe, R., and Chikuni, K. (2002). Positive effect of collagen V and VI on triglyceride accumulation during differentiation in cultures of bovine intramuscular adipocytes. Differentiation 70, 84–91. doi: 10.1046/j.1432-0436.2002.700203.x
Neels, J. G., Thinnes, T., and Loskutoff, D. J. (2004). Angiogenesis in an in vivo model of adipose tissue development. FASEB J. 18, 983–985. doi: 10.1096/fj.03-1101fje
Neubauer, M., Hacker, M., Bauer-Kreisel, P., Weiser, B., Fischbach, C., Schulz, M. B., et al. (2005). Adipose tissue engineering based on mesenchymal stem cells and basic fibroblast growth factor in vitro. Tissue Eng. 11, 1840–1851. doi: 10.1089/ten.2005.11.1840
Nillesen, S. T., Geutjes, P. J., Wismans, R., Schalkwijk, J., Daamen, W. F., and van Kuppevelt, T. H. (2007). Increased angiogenesis and blood vessel maturation in acellular collagen–heparin scaffolds containing both FGF2 and VEGF. Biomaterials 28, 1123–1131. doi: 10.1016/j.biomaterials.2006.10.029
O’Brien, F. J. (2011). Biomaterials & scaffolds for tissue engineering. Mater. Today 14, 88–95. doi: 10.1016/S1369-7021(11)70058-X
Panchal, H., and Matros, E. (2017). Current trends in postmastectomy breast reconstruction. Plast. Reconstr. Surg. 140(5S Advances in Breast Reconstruction), 7s–13s. doi: 10.1097/prs.0000000000003941
Patrick, C. W. (2000). Adipose tissue engineering: the future of breast and soft tissue reconstruction following tumor resection. Semin. Surg. Oncol. 19, 302–311. doi: 10.1002/1098-2388(200010/11)19:3<302::AID-SSU12>3.0.CO;2-S
Patrick, C. W. (2001). Tissue engineering strategies for adipose tissue repair. Anat. Rec. 263, 361–366. doi: 10.1002/ar.1113
Philips, B. J., Marra, K. G., and Rubin, J. P. (2014). Healing of grafted adipose tissue: current clinical applications of adipose-derived stem cells for breast and face reconstruction. Wound Repair Regen. 22, 11–13.
Pittenger, M. F., Mackay, A. M., Beck, S. C., Jaiswal, R. K., Douglas, R., Mosca, J. D., et al. (1999). Multilineage potential of adult human mesenchymal stem cells. Science 284, 143–147.
Prasad, K., Zhou, R., Zhou, R., Schuessler, D., Ostrikov, K. K., and Bazaka, K. (2019). Cosmetic reconstruction in breast cancer patients: opportunities for nanocomposite materials. Acta Biomater. 86, 41–65. doi: 10.1016/j.actbio.2018.12.024
Prusty, D., Park, B.-H., Davis, K. E., and Farmer, S. R. (2002). Activation of MEK/ERK signaling promotes adipogenesis by enhancing peroxisome proliferator-activated receptor γ (PPARγ) and C/EBPα gene expression during the differentiation of 3T3-L1 preadipocytes. J. Biol. Chem. 277, 46226–46232.
Ramirez, F., and Rifkin, D. B. (2003). Cell signaling events: a view from the matrix. Matrix Biol. 22, 101–107.
Roehm, K. D., Hornberger, J., and Madihally, S. V. (2016). In vitro characterization of acelluar porcine adipose tissue matrix for use as a tissue regenerative scaffold. J. Biomed. Mater. Res. A 104, 3127–3136.
Rohrich, R. J., Ghavami, A., and Crosby, M. A. (2007). The role of hyaluronic acid fillers (Restylane) in facial cosmetic surgery: review and technical considerations. Plast. Reconstr. Surg. 120, 41S–54S.
Rossi, E., Guerrero, J., Aprile, P., Tocchio, A., Kappos, E. A., Gerges, I., et al. (2018). Decoration of RGD-mimetic porous scaffolds with engineered and devitalized extracellular matrix for adipose tissue regeneration. Acta Biomater. 73, 154–166. doi: 10.1016/j.actbio.2018.04.039
Salzberg, C. A. (2012). Focus on technique: one-stage implant-based breast reconstruction. Plast. Reconstr. Surg. 130(5 Suppl. 2), 95s–103s. doi: 10.1097/PRS.0b013e318262e1a1
Sano, H., Orbay, H., Terashi, H., Hyakusoku, H., and Ogawa, R. (2014). Acellular adipose matrix as a natural scaffold for tissue engineering. J. Plast. Reconstr. Aesthet. Surg. 67, 99–106.
Sarjeant, K., and Stephens, J. M. (2012). Adipogenesis. Cold Spring Harb. Perspect. Biol. 4:a008417. doi: 10.1101/cshperspect.a008417
Sarkanen, J. R., Kaila, V., Mannerström, B., Räty, S., Kuokkanen, H., Miettinen, S., et al. (2011). Human adipose tissue extract induces angiogenesis and adipogenesis in vitro. Tissue Eng. Part A 18, 17–25.
Sarkanen, J. R., Ruusuvuori, P., Kuokkanen, H., Paavonen, T., and Ylikomi, T. (2012). Bioactive acellular implant induces angiogenesis and adipogenesis and sustained soft tissue restoration in vivo. Tissue Eng. Part A 18, 2568–2580.
Shah, B. S., Chen, M., Suzuki, T., Embree, M., Kong, K., Lee, C. H., et al. (2017). Pyrintegrin induces soft tissue formation by transplanted or endogenous cells. Sci. Rep. 7:36402.
Sicari, B. M., Dziki, J. L., Siu, B. F., Medberry, C. J., Dearth, C. L., and Badylak, S. F. (2014). The promotion of a constructive macrophage phenotype by solubilized extracellular matrix. Biomaterials 35, 8605–8612.
Silva, M. M., Kokai, L. E., Donnenberg, V. S., Fine, J. L., Marra, K. G., Donnenberg, A. D., et al. (2019). Oncologic safety of fat grafting for autologous breast reconstruction in an animal model of residual breast cancer. Plast. Reconstr. Surg. 143, 103–112.
Simonacci, F., Bertozzi, N., Grieco, M. P., Grignaffini, E., and Raposio, E. (2017). Procedure, applications, and outcomes of autologous fat grafting. Ann. Med. Surg. 20, 49–60. doi: 10.1016/j.amsu.2017.06.059
Song, M., Liu, Y., and Hui, L. (2018). Preparation and characterization of acellular adipose tissue matrix using a combination of physical and chemical treatments. Mol. Med. Rep. 17, 138–146.
Strong, A. L., Cederna, P. S., Rubin, J. P., Coleman, S. R., and Levi, B. (2015). The current state of fat grafting: a review of harvesting, processing, and injection techniques. Plast. Reconstr. Surg. 136, 897–912. doi: 10.1097/prs.0000000000001590
Stuermer, E. K., Lipenksy, A., Thamm, O., Neugebauer, E., Schaefer, N., Fuchs, P., et al. (2015). The role of SDF-1 in homing of human adipose-derived stem cells. Wound Repair Regen. 23, 82–89.
Tabata, Y., Miyao, M., Inamoto, T., Ishii, T., Hirano, Y., Yamaoki, Y., et al. (2000). De novo formation of adipose tissue by controlled release of basic fibroblast growth factor. Tissue Eng. 6, 279–289.
Tan, Q. W., Tang, S. L., Zhang, Y., Yang, J. Q., Wang, Z. L., Xie, H. Q., et al. (2019). Hydrogel from acellular porcine adipose tissue accelerates wound healing by inducing intradermal adipocyte regeneration. J. Invest. Dermatol. 139, 455–463. doi: 10.1016/j.jid.2018.08.013
Tan, Q. W., Zhang, Y., Luo, J. C., Zhang, D., Xiong, B. J., Yang, J. Q., et al. (2017). Hydrogel derived from decellularized porcine adipose tissue as a promising biomaterial for soft tissue augmentation. J. Biomed. Mater. Res. A 105, 1756–1764.
Tanzi, M. C., and Farè, S. (2009). Adipose tissue engineering: state of the art, recent advances and innovative approaches. Expert Rev. Med. Devices 6, 533–551.
Thomas-Porch, C., Li, J., Zanata, F., Martin, E. C., Pashos, N., Genemaras, K., et al. (2018). Comparative proteomic analyses of human adipose extracellular matrices decellularized using alternative procedures. J. Biomed. Mater. Res. A 106, 2481–2493.
Ting, A. C., Craft, R. O., Palmer, J. A., Gerrand, Y.-W., Penington, A. J., Morrison, W. A., et al. (2014). The adipogenic potential of various extracellular matrices under the influence of an angiogenic growth factor combination in a mouse tissue engineering chamber. Acta Biomater. 10, 1907–1918.
Toh, W. S., Lee, E. H., Guo, X.-M., Chan, J. K., Yeow, C. H., Choo, A. B., et al. (2010). Cartilage repair using hyaluronan hydrogel-encapsulated human embryonic stem cell-derived chondrogenic cells. Biomaterials 31, 6968–6980.
Tsuji, W., Inamoto, T., Yamashiro, H., Ueno, T., Kato, H., Kimura, Y., et al. (2009). Adipogenesis induced by human adipose tissue–derived stem cells. Tissue Eng. Part A 15, 83–93.
Turner, A. E., Yu, C., Bianco, J., Watkins, J. F., and Flynn, L. E. (2012). The performance of decellularized adipose tissue microcarriers as an inductive substrate for human adipose-derived stem cells. Biomaterials 33, 4490– 4499.
Vallee, M., Côté, J.-F., and Fradette, J. (2009). Adipose-tissue engineering: taking advantage of the properties of human adipose-derived stem/stromal cells. Pathol. Biol. 57, 309–317.
Wu, I., Nahas, Z., Kimmerling, K. A., Rosson, G. D., and Elisseeff, J. H. (2012). An injectable adipose matrix for soft-tissue reconstruction. Plast. Reconstr. Surg. 129, 1247–1257. doi: 10.1097/PRS.0b013e31824ec3dc
Xu, Y., Zhu, X., Hahm, H. S., Wei, W., Hao, E., Hayek, A., et al. (2010). Revealing a core signaling regulatory mechanism for pluripotent stem cell survival and self-renewal by small molecules. Proc. Natl. Acad. Sci. U.S.A. 107, 8129– 8134.
Yannas, I. V., Lee, E., Orgill, D. P., Skrabut, E. M., and Murphy, G. F. (1989). Synthesis and characterization of a model extracellular matrix that induces partial regeneration of adult mammalian skin. Proc. Natl. Acad. Sci. U.S.A. 86, 933–937.
Yoshimura, K., Eto, H., Kato, H., Doi, K., and Aoi, N. (2011). In vivo manipulation of stem cells for adipose tissue repair/reconstruction. Regen. Med. 6, 33–41.
Young, D. A., Bajaj, V., and Christman, K. (2014). Decellularized adipose matrix hydrogels stimulate in vivo neovascularization and adipose formation. J. Biomed. Mater. Res. A 102, 1641–1651.
Young, D. A., Ibrahim, D. O., Hu, D., and Christman, K. L. (2011). Injectable hydrogel scaffold from decellularized human lipoaspirate. Acta Biomater. 7, 1040–1049.
Yu, C., Bianco, J., Brown, C., Fuetterer, L., Watkins, J. F., Samani, A., et al. (2013). Porous decellularized adipose tissue foams for soft tissue regeneration. Biomaterials 34, 3290–3302.
Yuksel, E., Weinfeld, A. B., Cleek, R., Jensen, J., Wamsley, S., Waugh, J. M., et al. (2000a). Augmentation of adipofascial flaps using the long-term local delivery of insulin and insulin-like growth factor-1. Plast. Reconstr. Surg. 106, 373–382.
Yuksel, E., Weinfeld, A. B., Cleek, R., Wamsley, S., Jensen, J., Boutros, S., et al. (2000b). Increased free fat-graft survival with the long-term, local delivery of insulin, insulin-like growth factor-I, and basic fibroblast growth factor by PLGA/PEG microspheres. Plast. Reconstr. Surg. 105, 1712–1720.
Yuksel, E., Weinfeld, A. B., Cleek, R., Waugh, J. M., Jensen, J., Boutros, S., et al. (2000c). De novo adipose tissue generation through long-term, local delivery of insulin and insulin-like growth factor-1 by PLGA/PEG microspheres in an in vivo rat model: a novel concept and capability. Plast. Reconstr. Surg. 105, 1721–1729.
Zhang, S., Lu, Q., Cao, T., and Toh, W. S. (2016). Adipose tissue and extracellular matrix development by injectable decellularized adipose matrix loaded with basic fibroblast growth factor. Plast. Reconstr. Surg. 137, 1171–1180.
Keywords: adipose tissue engineering, biomaterials, extracellular matrix, scaffold, cell-free
Citation: Yang J, Zhou C, Fu J, Yang Q, He T, Tan Q and Lv Q (2021) In situ Adipogenesis in Biomaterials Without Cell Seeds: Current Status and Perspectives. Front. Cell Dev. Biol. 9:647149. doi: 10.3389/fcell.2021.647149
Received: 29 December 2020; Accepted: 08 February 2021;
Published: 08 March 2021.
Edited by:
Naresh Kumar Rajendran, University of Rochester, United StatesCopyright © 2021 Yang, Zhou, Fu, Yang, He, Tan and Lv. This is an open-access article distributed under the terms of the Creative Commons Attribution License (CC BY). The use, distribution or reproduction in other forums is permitted, provided the original author(s) and the copyright owner(s) are credited and that the original publication in this journal is cited, in accordance with accepted academic practice. No use, distribution or reproduction is permitted which does not comply with these terms.
*Correspondence: Qing Lv, bHZxaW5nd2VzdGNoaW5hQDE2My5jb20=
†These authors have contributed equally to this work and share first authorship
Disclaimer: All claims expressed in this article are solely those of the authors and do not necessarily represent those of their affiliated organizations, or those of the publisher, the editors and the reviewers. Any product that may be evaluated in this article or claim that may be made by its manufacturer is not guaranteed or endorsed by the publisher.
Research integrity at Frontiers
Learn more about the work of our research integrity team to safeguard the quality of each article we publish.