- 1Cell Production and Tissue Engineering Unit, Virgen de las Nieves University Hospital, Granada, Spain
- 2Granada Biosanitary Research Institute (ibs. GRANADA), Granada, Spain
- 3Andalusian Network for the Design and Translation of Advanced Therapies, Seville, Spain
- 4Department of Dermatology, School of Medicine, University of Granada, Granada, Spain
- 5Department of Dermatology, Virgen de las Nieves University Hospital, Granada, Spain
The skin is the largest organ of the human body and its main functions include providing protection from external harmful agents, regulating body temperature, and homeostatic maintenance. Skin injuries can damage this important barrier and its functions so research focuses on approaches to accelerate wound healing and treat inflammatory skin diseases. Due to their regenerative and immunomodulatory properties, mesenchymal stromal cells (MSCs) have been reported to play a significant role in skin repair and regeneration. However, it seems that the secretome of these cells and exosomes in particular may be responsible for their functions in skin regeneration and the immunomodulation field. The present review aims to gather the available information about the role of MSC-derived exosomes for both in vitro and in vivo models of different skin conditions and to highlight the need for further research in order to overcome any limitations for clinical translation.
Introduction
The skin is the largest organ of the human body and constitutes a protective barrier that isolates us from harmful agents and injuries. Apart from its defensive function against physical, chemical, and biological agents, the skin also contributes to regulating the organism’s temperature, homeostatic maintenance, participation in the mechanisms of sensorial perception, as well as in regenerative processes (Oualla-Bachiri et al., 2020; Sanabria-de la Torre et al., 2020).
The skin is frequently damaged as a result of acute and chronic wounds such as extensive burns, trauma or diabetic ulcers and also because of other conditions like atopic dermatitis (AD), aging or oxidative stress (Cho et al., 2018; Lai et al., 2018; Roşca et al., 2018; Casado-Díaz et al., 2020; Zhang Y. et al., 2020). Patients with cutaneous wounds experience physical and mental health problems, and these wounds also have a huge socioeconomic burden (Wu et al., 2018). Recently, mesenchymal stromal cells (MSCs) have gained much attention in cutaneous repair and regeneration. Resident skin MSCs are actively involved in the wound-healing process, either by differentiating into fibroblasts, which are responsible for the matrix synthesis, or through the release of various molecules involved in tissue regeneration, such as anti-scarring, anti-apoptotic and pro-angiogenic factors. Thus, the paracrine activity of resident and recruited cells effects the suitability of the regenerative process (Ballini et al., 2018). In fact, several studies have applied exogenous MSCs to wounds in order to benefit from their regenerative properties, resulting in positive effects on both wound healing and scarring (Roşca et al., 2018). This therapeutic potential of MSCs is mainly due to their facility to be isolated and expanded in vitro and the possibility of being cryopreserved once isolated, without significant loss of their potential. Furthermore, MSCs are hypoimmunogenic since these cells express intermediate or low levels of the MHC class I and II molecules respectively (Casado-Díaz et al., 2020). MSCs can be isolated from different tissues, although the most widely used are bone marrow-derived MSCs (BM-MSCs), adipose tissue-derived MSCs (AT-MSCs), and umbilical cord-derived MSCs (UC-MSCs). Another promising source of MSCs is the oral cavity, including tissues such as dental pulp, human periapical inflamed cyst, dental pulp of human exfoliated deciduous teeth, periodontal ligament, dental follicle progenitors, root apical papilla of human teeth and gingiva (Spagnuolo et al., 2018). Moreover, MSCs have been isolated from other regions such as amniotic fluid, the periosteum and fetal tissues, all of which show phenotypic heterogeneity (Hoang et al., 2020). They can also be extracted from the blood, liver, spleen, and bone marrow of the human fetus in the first and second trimesters (Hoang et al., 2020).
However, despite the significant progress which has been made in the application of MSCs in wound repair and cutaneous regeneration, there are some limitations inherent to MSC cell therapy. For instance, there is considerable heterogeneity in the delivery protocols and MSC populations which makes it difficult to determine the impact of timing of delivery, number of cells produced and site of delivery on MSCs. In addition, there is no evidence that MSCs differentiate into phenotypes typical of resident cutaneous cells during skin wound healing (Hu et al., 2019). Lastly, current challenges for the use of MSCs concern the lack of universally accepted criteria for defining the MSC phenotype and their functional properties. Actually, MSCs mediate distinct immune modulating responses that are characterized by a pro-inflammatory MSC1 phenotype and an immunosuppressive MSC2 phenotype (Lai et al., 2018). Other limitations include their proliferation capacity, lifespan, potential contamination by handling and rejection (Ferreira and Gomes, 2019).
There is current evidence that MSCs achieve a therapeutic effect in vivo mainly through paracrine signaling (Wu et al., 2018; Hu et al., 2019; Casado-Díaz et al., 2020) and not only due to their capacity to proliferate and differentiate into the required cellular types in the damaged tissue but also because of their secretome (Ballini et al., 2017). The MSC– secretome has one free fraction, made of soluble factors and metabolites, and another encapsulated into extracellular vesicles (EVs).
Extracellular vesicles are typically classified into three subtypes according to size and biogenesis mechanisms: exosomes (50–100 nm), microvesicles (100–1000 nm), and apoptotic bodies (500–5000 nm) (Qiu et al., 2019). Exosomes are generated through endocytosis, from larger intracellular vesicles called multivesicular bodies (MVBs) through a sophisticated intracellular trafficking system (Aheget et al., 2020). MVBs are intraluminal vesicles, formed by internal budding of the endosomal membrane. The best-known mechanism of MVB and exosome generation is that carried out by the endosomal sorting complex required for transport (ESCRT), although other mechanisms such as hydrolysis of sphingomyelin into ceramides or proteins like tetraspanins have been reported (Andreu and Yáñez-Mó, 2014). MVBs migrate toward the edge of the cell where they fuse with the plasma membrane and exosomes are then released to the extracellular space via exocytosis (Qiu et al., 2019; Casado-Díaz et al., 2020). Thus, exosome biogenesis can be divided into three stages: the formation of endocytic vesicles, through the invagination of the plasma membrane; the formation of MVBs, by the inward budding of the endosomal membranes; and the fusion of MVBs with the plasma membrane and exosome release (Casado-Díaz et al., 2020). In addition to MSC surface antigens such as CD90, CD73, and CD105, exosomes have multiple specific marker proteins, including membrane transport and fusion proteins (GTPases, annexins, and flotillin), tetraspannins (CD9, CD63, and CD81), heat shock proteins (hsp60, hsp70, and hsp90), proteins involved in MVB biogenesis (Alix and tumor susceptibility gene 101 protein), as well as lipid-related proteins and phospholipases (Liu et al., 2018; Ferreira and Gomes, 2019; Casado-Díaz et al., 2020).
Exosomes are key bioactive vesicles responsible for the paracrine effects of MSCs, regulating many physiological and pathological processes by affecting the survival, proliferation, migration and gene expression of recipient cells and by reprogramming targeted cell behaviors (Wu et al., 2018). Several studies have indicated that some exosomes are involved in the skin’s physiological and pathological processes (Liu et al., 2018). Compared to MSC therapy, MSC exosomes have the following advantages: Firstly, MSC exosomes exert intense biological effects because of their direct fusion with target cells. Secondly, MSC exosomes can be stored and transported at −70°C for long periods of time since their effective components are protected by their membrane, which is not easily destroyed (Zhao et al., 2017). Thirdly, the concentration, dose, route and time of use are easy to control. Lastly, there is no risk of the immune rejection and tumorigenesis caused by cell transplantation therapy (Hu et al., 2019; An et al., 2020; Yu et al., 2020). In addition, exosomes can be sterilized by filtration during their preparation for clinical usage (Lai et al., 2018). Regarding exosome administration routes, the most common are intravenous and subcutaneous injections although they can also be administered through biocompatible scaffolds or hydrogels, which can serve as sustained release systems for these vesicles (Wang C. et al., 2019; Yang J. et al., 2020).
Due to the current interest in the potential therapeutic applications of MSC-derived exosomes, the main objective of the present review is to analyze their role in dermatology, focusing on wound healing and skin regeneration, GVHD, AD, psoriasis, photoaging, oxidative stress, and rejuvenation (Figure 1).
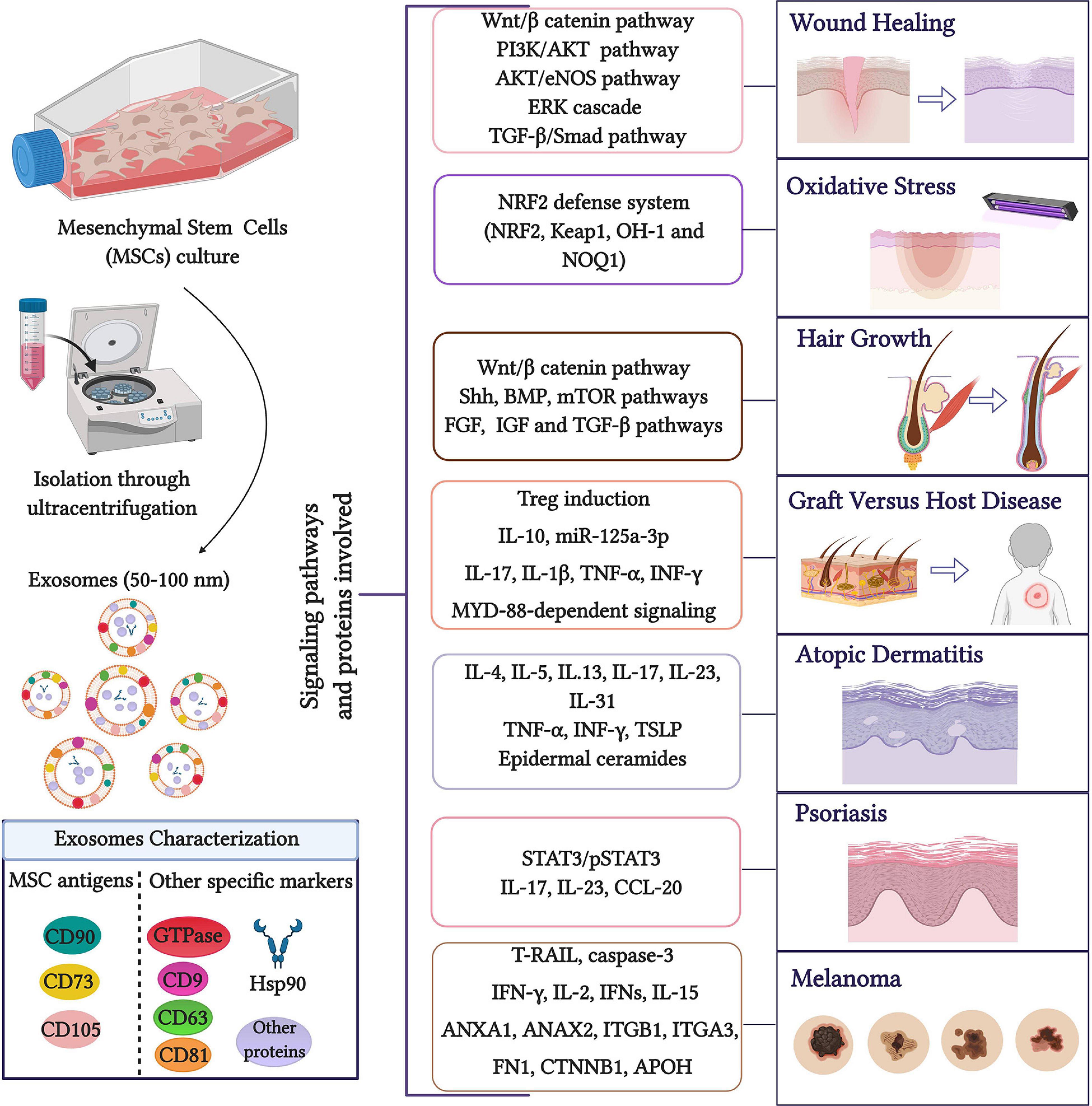
Figure 1. Role of MSC exosomes in dermatology. Exosomes are widely isolated through MSC culture media ultracentrifugation (Gardiner et al., 2016) although other isolation methods such as commercial kits, polyethylene glycol/polymer-based EV enrichment and size-based fractionation have been described (Witwer et al., 2019). The presence of MSC exosome markers can be analyzed by methods including Western blotting, enzyme-linked immunosorbent assays, classical flow cytometry of bead-captured EVs, or advanced flow cytometry at the single EV level. The main signaling pathways and proteins involved in the role of MSC exosomes are highlighted. Created with BioRender.com.
Exosomes and Wound Healing
Cutaneous wound healing is a complex, dynamic process in charge of restoring the structure and function of damaged tissues. It involves highly orchestrated multiple processes including hemostasis, inflammation, cell migration and proliferation, angiogenesis and extracellular matrix remodeling (Lai et al., 2018; Gao et al., 2020) which are strictly regulated by multiple diverse growth factors, cytokines, enzymes and structural matrix proteins generated by multiple cell types such as dermal fibroblasts, epidermal keratinocytes, and immune cells (Zhao et al., 2017; Hoang et al., 2020). Among the varied factors influencing wound repair, angiogenesis occupies a critical position, which results in the delivery of nutrients and oxygen to the wound sites, promoting fibroblast proliferation, collagen synthesis, and re-epithelialization (Liu et al., 2020; Yu et al., 2020). Under pathological conditions, the disruption and prolonging of the wound-healing process can lead to chronic, non-healing wounds such as diabetic wounds (Liu et al., 2020). The mechanisms underlying poor healing of diabetic wounds are still unclear although the main complications involve hypoxia, impaired angiogenesis, damage from reactive oxygen species (ROS) and neuropathy, leading to long-term medical burden and compromised quality of life in those patients (Wang C. et al., 2019; An et al., 2020).
Another problem associated with impaired wound healing is scarring. Cutaneous scar formation is a consequence of the wound-healing process and involves the coordination of a complex sequence of interactions between cells, ECM components and signaling molecules. Scar tissue is characterized by the exaggerated deposition of ECM components and a lack of skin appendages such as hair follicles and sweat glands. ECM remodeling, especially collagen synthesis and degradation, is the key cellular and molecular event contributing to scarring. Furthermore, the fibroblast-myofibroblast transition is critical in this process. In response to skin injury and wound damage, dermal fibroblasts undergo a phenotype transition into myofibroblasts, characterized by enhanced contractile ability and the expression of α-smooth muscle actin (α-SMA). Moreover, several studies have stated that the Transforming Growth Factor (TGF)-β1/Smad signaling pathway actively takes part in collagen formation and the fibroblast-myofibroblast transition (Wang et al., 2017). Interestingly, scarless healing occurs in the early or midgestation stages of embryonic development. Thus in fetal wound tissue, the ratio of collagen type III to type I is higher and there is also a higher ratio of TGF-β3 to TGF-β1 and of matrix metalloproteinases (MMPs) to tissue inhibitors of metalloproteinases (TIMPs).
Therapies based on MSCs showed great potential for wound healing due to their ability to recruit cells and release growth factors and proteins. In fact, MSCs have been tested as a promising cell-based therapy for diabetic wounds in vitro and in vivo because of their ability to accelerate wound closure with increased epithelialization, granulation tissue formation, and angiogenesis by differentiation into skin cells and paracrine pathways to repair injured cells. However, due to the disadvantages of MSCs, more efforts have been focused on the MSC secretome including cytokines, growth factors, chemokines and extracellular vesicles containing mRNA, proteins and microRNAs, as well as their role on the wound-healing process (An et al., 2020).
Mesenchymal stromal cell-derived exosomes can promote angiogenesis, cell migration, proliferation and the re-epithelialization process by activating a signaling route such as the Wnt/β-catenin, phosphatidylinositol 3-kinase/protein kinase B pathway (PI3K/AKT) or extracellular signal-regulated kinase (ERK) cascade, resulting in growth factors expression upregulation (Carrasco et al., 2019; Kucharzewski et al., 2019).
For instance, exosomes isolated from human AT-MSCs (hAT-MSCs) stimulate cell proliferation and migration and play an inhibitory role in the cell apoptosis of in vitro cultured human keratinocytes (HaCaTs) treated with hydrogen peroxide (H2O2) through Wnt/β-catenin signaling (Ma et al., 2019). Metastasis Associated Lung Adenocarcinoma Transcript 1 (MALAT1), a transcriptional regulator for numerous genes contained in these exosomes, can also mediate H2O2-induced wound healing by targeting miR-124 and activating this pathway (He et al., 2020). Zhang et al. showed that 14-3-3ζ protein in human UC-MSC (hUC-MSC) derived exosomes enhances the Hippo/Yes-associated protein (YAP) pathway, promoting self-regulation of Wnt/β-catenin signaling at the remodeling phase of cutaneous regeneration in vivo and restricting excessive skin cell expansion and collagen deposition 4 weeks after treatment in a rat deep second-degree burn model (Zhang et al., 2016). They found that exosomal 14-3-3ζ promoted the binding of p-LATS (large tumor suppressor) and YAP at high cell density, resulting in phosphorylation of YAP. Interestingly, miR-135a-mediated downregulation of large tumor suppressor 2 (LATS2) increased the migration of BJ cells in vitro and promoted wound healing in vivo (Gao et al., 2020). Regarding Wnt/β-catenin signaling, Wnt4 contained in hUC-MSC-derived exosomes promoted β-catenin nuclear translocation and activity to enhance proliferation and migration of HaCaTs in vitro and played a key role in wound re-epithelialization in a rat skin burn model in vivo (Zhang et al., 2015a). β-catenin nuclear translocation induced the increased expression of proliferating cell nuclear antigen (PCNA), cyclin D3, N-cadherin and β-catenin and the decreased expression of E-cadherin. Furthermore, Wnt4 induces β-catenin activation in endothelial cells and exerts proangiogenic effects in vitro, which could be an important mechanism for cutaneous wound healing (Zhang et al., 2015b). Other proliferative markers, growth factors and migration-related chemokines such as angiotensin-2 (Ang-2) (Liu et al., 2020), cyclin D1, cyclin A2 and C-X-C motif chemokine 12 (CXCL12) were significantly upregulated after hUC-MSCs-derived exosome treatments with human umbilical vein endothelial cells (HUVECs) in vitro, promoting proliferation, migration and angiogenesis (Li X. et al., 2020). In this study, in vivo administration of iron oxide nanoparticle-labeled exosomes significantly increased the number of exosomes accumulated at the injury site, enhancing endothelial cell proliferation, migration and angiogenic tubule formation as well as reducing scar formation due to the increased expression of CK19, PCNA, and collagen.
Another important signaling route in the wound-healing process, parallel to the Wnt/β-catenin pathway, is PI3K/AKT. Yang C. et al. (2020) reported that miR-21 packaged in hAT-MSC-derived exosomes enhances MMP-9 expression and decreases TIMP-1 through the PI3K/AKT pathway, promoting the proliferation of HaCaTs in vitro. Moreover, miR-126-mediated phosphatase and tensin homolog (PTEN) downregulation seems to stimulate angiogenesis in vitro through the PI3K/AKT pathway, contributing to the stimulation of wound healing and angiogenesis in diabetic rats in vivo (Ding et al., 2019). This route is also reported to be involved in promoting fibroblast proliferation and the optimization of collagen deposition in vitro after hAT-MSC-derived exosome treatment. In vivo, these exosomes significantly accelerated wound healing (Zhang W. et al., 2018). Regarding AKT, Yu et al. (2020) showed that exosomes from human BM-MSCs (hBM-MSCs) treated with atorvastatin (ATV) activated AKT/endothelial nitric oxide synthase (eNOS) signaling pathway to augment the angiogenesis of endothelial cells via upregulating miR-211-3p, resulting in accelerated wound regeneration in diabetic rats in vivo. The same effect has been reported after treatment with exosomes from the same origin pre-treated with serum of neonatal and adult mice (Qiu et al., 2020). In addition to AKT, hBM-MSC-derived exosomes are able to activate ERK1/2 and Signal transducer and activator of transcription 3 (STAT3), promoting the expression of trophic factors such as cyclin D2 in vitro. This stimulates fibroblast growth, migration and angiogenesis of endothelial cells in vitro (Shabbir et al., 2015). Induced mesenchymal stromal cell (iMSC) derived exosomes have also been proven to stimulate ERK1/2 thus promoting the proliferation of HaCaTs and human dermal fibroblasts (HDFs) in vivo (Kim et al., 2018). Moreover, exosomes obtained from hBM-MSCs, hAT-MSCs, and hUC-MSCs have been reported to be able to induce HaCaT and HDF proliferation and migration in vitro by inducing crucial wound healing-mediated growth factors (Choi et al., 2018) such as vascular endothelial growth factor A (VEGF-A), fibroblast growth factor 2 (FGF-2), hepatocyte growth factor (HGF) and platelet-derived growth factor BB (PDGF-BB), which can activate AKT, ERK, and STAT3 signaling (Hoang et al., 2020). Continuing with ERK proteins, Wang et al. (2017) reported that hAT-MSC-derived exosomes increased the MMP3 expression of skin dermal fibroblasts by activating the ERK/Mitogen-activated protein kinase (MAPK) pathway, leading to a high ratio of MMP3 to TIMP1, which is also beneficial for ECM remodeling. These exosomes also decreased the size of scars and increased the ratio of collagen III to collagen I in murine incisional wounds in vivo by preventing the differentiation of fibroblasts into myofibroblasts and increasing the ratio of TGF-β3 to TGF-β1 (Wang et al., 2017).
Regarding scarless wound healing, several studies have noted the role of the TGF-β/Smad signaling pathway. For instance, Jiang T. et al. (2020) showed that hBM-MSC-derived exosomes promoted HaCaT and HDF growth in vitro and accelerated scarless wound healing in vivo. They found significantly downregulated TGF-β1, Smad2, Smad3, and Smad4 expression, alongside upregulated TGF-β3 and Smad7 expression (Jiang T. et al., 2020). Furthermore, exosomes from hUC-MSCs reduced scar formation and myofibroblast accumulation in a skin defect mouse model in vivo. Specifically, Fang et al. (2016) reported that these exosomes contain specific microRNAs such as miR-21, miR-23a, miR-125b, and miR-145 which play key roles in suppressing myofibroblast formation by inhibiting the transforming TGF-β2/Smad2 pathway. In line with this, Jiang L. et al. (2020). demonstrated that tumor necrosis factor-inducible gene 6 (TSG-6) overexpressed hBM-MSC-derived exosomes effectively ameliorated scar pathological injury, decreased inflammatory molecular secretion and attenuated collagen deposition in a mouse skin wound model in vivo. The exosome treatment downregulated the expression of TGF-β1, p-Smad2 and p-Smad3 in the scar region (Jiang L. et al., 2020). Smad2/3 phosphorylation has also been found markedly decreased in fibroblasts treated with hUC-MSC-derived exosomes along with Collagen I and III and α-SMA (Hu et al., 2020). Considering the collagen I/III ratio, Dalirfardouei et al. (2019) reported that exosomes originating from human menstrual MSCs (hMen-MSCs) rapidly decreased this ratio leading to less scar formation in a diabetic mouse model and promoting re-epithelialization, wound closure and angiogenesis in vivo. Exosomes from hAT-MSCs exerted the same effects by increasing gene expression of N-cadherin, cyclin-1, PCNA and collagen I, III in vitro and collagen I and III production in the early stage of wound healing in vivo. Interestingly, in the late stage, exosomes inhibited collagen expression to reduce scar formation (Hu et al., 2016). In addition, human amniotic epithelial cell (hAEC) derived exosomes remarkably enhanced the proliferation and migration ability of fibroblasts in vitro and collagen I and collagen III were downregulated in these cells through stimulating the expression of MMP-1. In vivo wound assays also showed that exosome treatment facilitated the wound-healing process with well-arranged collagen fibers (Zhao et al., 2017).
Other signaling routes reported to be involved in the wound-healing process are the poly ADP ribose polymerase 1(PARP-1)/apoptosis-inducing factor (AIF) apoptosis pathway and Notch signaling pathway. The first seems to suppress HaCaT apoptosis induced with H2O2 by inhibiting nuclear translocation of AIF and upregulating PARP-1 after treatment with hUC-MSC-derived exosomes in vitro. In vivo experiments showed enhanced epidermal re-epithelialization and dermal angiogenesis (Zhao et al., 2020). Regarding the Notch signaling pathway, an in vivo study indicated that human fetal dermal mesenchymal stromal cell (hFD-MSC) derived exosomes could accelerate wound closure in a mouse full-thickness skin wound model by activating this route (Wang X. et al., 2019).
It should be noted that the administration route of exosomes in the in vivo studies described previously are mainly intravenous and subcutaneous injections of exosome solutions. However, some investigations reported hydrogel development as an efficient administration pathway. For example, a chitosan/silk hydrogel loaded with human gingival mesenchymal stromal cell (hG-MSC) derived exosomes accelerated skin defect healing in vivo (Shi et al., 2017) and a thermosensitive Pluronic F-127 (PF-127) hydrogel containing hUC-MSC-derived exosomes significantly accelerated wound closure rate in a diabetic animal model in vivo (Yang J. et al., 2020). Along with oxidative hyaluronic acid and poly-e-L-lysin (denoted as FHE hydrogel), PF-127 also significantly improved neovascularization and re-epithelialization in diabetic rats (Wang C. et al., 2019). Supplementary Tables 1, 2 summarize in vitro and in vivo studies respectively.
Exosomes and Oxidative Stress, Photoaging and Rejuvenation
Some studies have analyzed whether MSC-derived exosomes can enhance skin rejuvenation by preventing oxidative stress and photoaging effects. Oxidative stress is a major cause of skin injury induced by ultraviolet (UV) irradiation and other stimuli which can damage the cellular lipids, proteins and DNA of skin cells by promoting the production of ROS and decreasing antioxidant enzyme activity. This results in sunburn, premature aging, and carcinogenesis. Keratinocytes constitute a barrier against environmental damage by modulating oxidative stress, glucose metabolism and inflammatory mediators through the nuclear factor E2-related factor 2 (NRF2) signaling pathway, which is a central player in regulating the expression of antioxidant enzymes following skin injury or inflammation (Schäfer et al., 2012; Wang et al., 2020). Wang et al. (2020) investigated the effects of hUC-MSC-derived exosomes on oxidative injury in H2O2-stimulated epidermal keratinocytes in vitro and a UV-irradiated mouse model in vivo. They found reduced ROS generation, DNA damage, aberrant calcium signaling and mitochondrial changes in addition to alleviated cellular and histological responses to inflammation and oxidation (Table 1). Furthermore, the NRF2 signaling pathway was involved in this antioxidation activity since its knockdown attenuated the antioxidant capacities of exosomes both in vitro and in vivo (Wang et al., 2020) (Table 1 and Supplementary Table 3).
In terms of skin rejuvenation, the ECM and especially collagen and elastin play a crucial role in skin growth and elasticity. Collagen is responsible for the mechanical protection of the body, prevention of skin dehydration, elasticity maintenance, tissue firmness and skin wrinkle minimization. Elastin is a major structural protein of body tissue and a fibrous protein which provides strength and natural elasticity (Kim et al., 2017). Kim and colleagues studied the capacity of hUC-MSC-derived exosomes to rejuvenate skin by modulating collagen production and permeation (Kim et al., 2017; Ferreira and Gomes, 2019). They reported stimulation of HDF migration and collagen synthesis in vitro and promotion of collagen I and elastin production in ex vivo human skin (Kim et al., 2017) (Table 1 and Supplementary Table 3). Interestingly, they also found decreased MMP-1 production, which works as a collagenase and encodes a secreted enzyme which breaks down the types I, II, and III interstitial collagens (Tallant et al., 2010; Kim et al., 2017). Lastly, Zhang K. et al. (2020) studied whether the marine sponge Haliclona sp. spicules (SHSs) could effectively enhance the skin delivery of exosomes from hUC-MSCs by evaluating its topical application in rejuvenating photoaged mouse skin. It was reported that the combination of exosomes and SHSs showed significant anti-photoaging effects in mice, including reducing microwrinkles, alleviating histopathological changes and promoting the expression of extracellular matrix constituents. Moreover, skin irritation tests on a guinea pig animal model showed slight irritation (Zhang K. et al., 2020) (Table 1 and Supplementary Table 3).
Taken together, these investigations prove the significant role of exosomes, mainly derived from hUC-MSCs, in preventing oxidative skin damage and promoting rejuvenation. This could lead to the potential use of MSC-derived exosomes in the development and application of cosmetics, although further studies about their effects on in vivo human skin are required.
Exosomes and Hair Growth
Alopecia is a common medical problem which has serious negative impacts on affected individuals. It is related to various factors including nutritional deficiencies, hormonal changes and drug treatment (Rajendran et al., 2017). Alopecia areata and androgenic alopecia are challenging conditions for dermatologists nowadays, with a lack of effective treatments. Advanced therapies are a promising therapeutic option and have shown good results (Martinez-Lopez et al., 2020).
Various dermatological compounds have been developed to combat alopecia. Finasteride and minoxidil are the mainstay treatments for alopecia. However, they only have a short-term effect and discontinuation of treatment leads to rapid hair loss and has known side effects (Mysore, 2012; Suchonwanit et al., 2019; Ha et al., 2020). Autologous follicle transplantation has also been tried, but the number of donor follicles is limited, it is an invasive procedure and the graft survival rate largely depends on the surgeon. Therefore, alopecia is an unsolved problem requiring effective long-term strategies (Rajendran et al., 2017).
The hair follicle cycle is a complex process involving alternating phases of rapid growth (anagen), regression (catagen), and quiescence (telogen) (Alonso and Fuchs, 2006). Hair follicles are epidermal appendages that contain both epithelial and mesenchymal compartments. The dermal papilla (DP) is located at the base of the hair follicle and plays a fundamental role in the hair follicle cycle (Kishimoto et al., 2000; Rajendran et al., 2017). AT-MSCs are able to promote the proliferation of DP cells in vitro and promote hair growth in mice and humans (Fukuoka et al., 2017; Ha et al., 2020).
The Wnt/β-catenin signaling and Shh signaling pathways, in addition to the secretion of growth factors such as FGF-5 or insulin-like growth factor-1 (IGF-1), are crucial for hair follicle development and hair growth (Ha et al., 2020). Other routes involved in the telogen to anagen transition include the estrogen receptor pathway, Bone Morphogenetic Protein (BMP) signaling, mammalian Target of Rapamycin (mTOR) signaling, FGF and TGF-β signaling pathways (Rajendran et al., 2017).
Direct transplantation of DP cells has been tested and their ability to induce follicles has been demonstrated (Weinberg et al., 1993). However, cell transplantation therapy is generally associated with the risk of tumor formation, graft rejection and ethical concerns. DP-MSC-derived exosomes have been reported to induce the passage from telogen to anagen, as well as delayed transition from anagen to catagen in vivo. Furthermore, these exosomes stimulated the expression of β-catenin and Shh, regulators of the hair follicle cycle and the proliferation and migration of outer root sheath keratinocytes, increasing their entry into S and S/G1 phase. The result was the formation of hair shafts of greater length and diameter (Zhou et al., 2018). Moreover, Kwack et al. (2019) stated that DP-exosomes were able to induce the development of in vitro cultured human hair follicles and also hair growth in vivo mice models. Interestingly, Yan et al. (2019) found 111 miRNAs that are differentially expressed between DP cells and DP exosomes. There were 34 major miRNAs that pointed to exosomes as regulators of hair follicle stem cell proliferation and differentiation. Specifically, miR22-5p inhibits hair follicle stem cell proliferation by targeting the Lymphoid enhancer-binding factor 1 (LEF1) gene, an important regulator of the β-catenin signaling pathway (Yan et al., 2019).
Rajendran et al. (2017) have produced the only publication to date on MSC exosomes, where the effect of BM-MSC-derived exosomes on hair growth was studied (Rajendran et al., 2017) (Table 1 and Supplementary Table 3). They found that in vitro treatment of DP cells with these exosomes caused activation by phosphorylation of AKT, as well as of the antiapoptotic protein Bcl-2, thus increasing their survival and migration. On the other hand, the expression and release of VEGF and IGF-1 genes were significantly increased in a dose-dependent manner by treatment with MSC-EV. These factors promote hair growth, an increase in follicle size and hair thickness. In vivo effects of these exosomes led to an increase in Wnt3a and Wnt5a signaling, so the treatment could be useful for activating human hair follicle stem cells, resulting in anagen initiation through Wnt/β-catenin activation (Rajendran et al., 2017). BM-MSC exosomes significantly promoted the conversion of telogen to anagen and increased the thickness of the dermis, which also indirectly reflects the improvement of hair growth. Additionally, no major organ damage was observed, indicating that BM-MSC exosomes could be a non-toxic treatment option (Rajendran et al., 2017).
Alopecia areata and androgenic alopecia are challenging conditions for dermatologists nowadays, with a lack of effective treatments. Advanced therapies are a promising therapeutic option that have shown good results (Martinez-Lopez et al., 2020). Although few studies have focused on the use of exosomes to stimulate hair growth, the findings so far are promising. In fact, a patented study points to MSC exosomes as the central component of a pharmaceutical composition aimed at promoting hair growth (Lim et al., 2015). It is very likely that the role of MSC exosomes in hair follicle dynamics will become a high-impact tool in skin regenerating cosmetics and biomedicine (Carrasco et al., 2019).
Exosomes and Graft Versus Host Disease
Graft versus host disease (GVHD) occurs when donor cells attack host cells. There are two ways of developing GVHD: acute GVHD (aGVHD) which appears earlier and usually remains as a skin rash, and chronic GVHD (cGVHD) which appears later and may affect more tissues and organs. cGVHD is the primary cause of long-term morbidity and mortality after allogeneic hematopoietic stem cell transplantation (Lai et al., 2018). The epithelial target tissues affected by classic cGVHD are the gastrointestinal tract, liver, skin, and lungs. In fact, pulmonary complications are currently considered diagnostic evidence of cGVHD and are characterized by a frequent lack of response to treatment and irreversibility. However, other systems such as the oral, esophageal, musculoskeletal, joint, fascial, ocular and lymphohematopoietic can also be damaged. A proposed conceptual model divides the pathophysiology of cGVHD into three stages: early inflammation (phase 1), later chronic inflammation, thymic injury and dysregulated B cell and T cell immunity (phase 2), and finally tissue repair with fibrosis (phase 3) (Zeiser and Blazar, 2017).
Firstly, the translocation of bacteria and fungi to the tissue creates damage that causes the release of pathogen-associated molecular patterns (PAMPs), as well as damage-associated molecular patterns (DAMPs), leading to a cascade of activation of toll-like receptors (TLRs), nucleotide-binding oligomerization domain-like receptors (NOD-R) and the NOD-like receptor protein 3 inflammasome (NLRP3) (Zeiser and Blazar, 2017). As the intima of the vessels is damaged by inflammation, endothelial cells are lost and the microvasculature is disorganized. In addition, T cells are activated during the initiation phase of chronic GVHD.
In the second stage, T cells are polarized toward type 1, type 2, and type 17 helper T (Th1, Th2, and Th17) cells. In addition, thymic epithelial cells are lost, which are necessary for the generation of regulatory T (Treg) cells and for positive selection of T lymphocytes (Zeiser and Blazar, 2017). The Th17/Treg ratio has been considered a specific marker of cGVHD progression (Lai et al., 2018).
Lastly, platelet-derived growth factor α (PDGF-α) and TGF-β activate fibroblasts, causing extracellular matrix production and ultimately the sclerotic phenotype. In addition, the production of isotype-switched immunoglobulin by differentiated B cells results in pathogenic immunoglobulin deposition in various organs, which contributes to organ damage and fibrosis (Zeiser and Blazar, 2017). The chronic inflammatory state is maintained by the Th17 cells that escaped immune regulation in the second stage (MacDonald et al., 2017).
Due to their immunomodulatory properties, MSCs are a promising therapy for preventing cGVHD. MSCs regulate the Th17/Treg balance and promote transportation tolerance (Weng et al., 2012). MSC exosomes mediate the paracrine effects of MSCs and promote tissue repair and homeostasis recovery, making them potential candidates for cell-free therapies (Lai et al., 2018). MSC exosomes are able to inhibit inflammation by suppressing the activation and migration of autoreactive T cells. Exosomes secreted by immune and non-immune cells can stimulate and inhibit the immune system. The effects of exosomes on immunity include the activation of T cells, polarization of T cells into Treg cells, immunosuppression, anti-inflammatory and others (Wang W. M. et al., 2019).
In vivo and in vitro experiments have demonstrated the ability of MSC exosomes to inhibit cGVHD by promoting the expansion of Treg cells whilst inhibiting pro-inflammatory Th17 cells (Table 1 and Supplementary Table 3). In fact, hBM-MSCs-derived exosomes exert marked immunosuppressive effects on cytokine production (Lai et al., 2018). Specifically, these exosomes inhibit the expression of pro-inflammatory cytokines such as IL-17A, a characteristic cytokine of Th17 cells. Furthermore, they are capable of inducing the production of IL-10, a characteristic cytokine of Treg. In this way, hBM-MSCs-derived exosomes carry out regulatory immune responses capable of attenuating cGVHD. Also, they are a promising therapeutic tool for treating pulmonary complications in cGVHD (Lai et al., 2018).
Several studies reveal the effective immunomodulatory potential of MSC exosomes for the treatment of cGVHD by regulating Treg through different pathways, such as MYD88-dependent signaling (Zhang et al., 2014) (Supplementary Table 3) or by pathways dependent on antigen-presenting cells. Furthermore, it appears that induction of Tregs mediated by human embryonic stem cell–derived MSCs (hESC-MSCs)-derived-exosomes requires previous T cell activation (Zhang B. et al., 2018) (Table 1 and Supplementary Table 3). The immunosuppressive effect of MSC exosomes has been evaluated in a mouse model of myocardial ischemia/reperfusion injury, renal fibrosis, liver injury, etc. (Lai et al., 2010; Lee et al., 2012; Li et al., 2013). Furthermore, hBM-MSC-derived exosomes had immunoregulatory effects on peripheral blood mononuclear cells in vitro and could prolong the survival of mice with aGVHD and improve aGvHD damage in vivo (Fujii et al., 2018) (Table 1 and Supplementary Table 3).
In a preliminary clinical study, a patient with resistant grade IV aGVHD was treated with hBM-MSC-derived exosomes (Kordelas et al., 2014) (Supplementary Table 3). The levels of IL-1β, TNF-α, and IFN-γ produced by peripheral blood mononucleated cells (PBMC) were reduced by more than 50% after the last application. In this context, the clinical outcome of aGVHD improved significantly shortly after the start of MSC exosome therapy (Kordelas et al., 2014). Therefore, MSC-derived exosomes may potentially provide a new and safe tool for treating therapy-refractory GVHD and other diseases potentially associated with inflammation (Leung, 2013).
In addition to GVHD, allograft rejection is the main reason for the failure of organ transplantation. A novel study analyzed the role of exosomes as RNA transport vehicles to induce immune tolerance in patients with skin grafts. The RNA delivery system of targeted dendritic cells (DC exosomes) was constructed based on MSC exosomes. DC exosomes were able to induce immune tolerance 3, 7, and 14 days after skin transplantation. Furthermore, the long-term immune tolerance of the graft was maintained in the murine model (Li C. et al., 2020).
Exosomes and Atopic Dermatitis
Chronic uncontrolled inflammatory responses are associated with various inflammatory diseases, including allergic skin diseases such as AD (Shin et al., 2020). Due to their intrinsic immunosuppressive properties, MSCs are a key element in the regulation of inflammation and therefore in the treatment of these allergic diseases. Furthermore, the clinical value of MSCs in AD has been confirmed in clinical trials (phase I/IIa) (Carrasco et al., 2019). Specifically, MSC exosomes represent an excellent alternative to MSC cell therapy since MSC exosomes have biological functions similar to those of parent cells, while they are more stable and have lower immunogenicity. The anti-inflammatory and immunomodulatory functions of MSC exosomes have been widely described (Ha et al., 2020).
Specifically, AD is a chronic skin disease with serious erythematous lesions and severe systemic inflammation. AD is related to genetic, immunological and environmental factors and its prevalence is higher in developed countries, particularly in recent years (Sanabria-de la Torre et al., 2020). Different factors must be taken into account in order to develop an effective treatment against AD. On one hand, elevated levels of Th2 cytokines are associated with abnormal immune responses that increase susceptibility to AD (Leung, 2000). On the other hand, the abnormal expression of genes responsible for epidermal barrier function is a crucial factor in the development of AD (Silverberg and Silverberg, 2015). The pathogenesis of AD includes changes in the skin barrier, abnormal immune signaling and defective terminal differentiation of keratinocytes, leading to decreased levels of ceramides, filaggrin and antimicrobial peptides (Leung, 2013). Alteration of the skin barrier leads to severe skin inflammation, allowing the entry of pathogens, allergens and toxic environmental pollutants. Ceramides contribute to keratinocyte differentiation and thus epidermal barrier function (Mizutani et al., 2009). In fact, AD patients have reduced ceramide levels (Imokawa et al., 1990).
Regarding the epidermal barrier in AD, Shin et al. (2020) demonstrate that hAT-MSC-derived exosomes are capable of improving the barrier functions of epidermal permeability, which coincides with a significant increase in ceramides and a reduction in immune responses during AD progression (Supplementary Table 3). In addition, MSC exosomes stimulated the production of epidermal ceramides and the formation of laminar bilayers at the stratum granulosa-stratum corneum interface, which contributes to the differentiation of keratinocytes and helps form an adequate epidermal permeability barrier. Finally, MSC exosomes were also found to activate genes associated with keratinocyte differentiation (Shin et al., 2020). Therefore, MSC exosomes offer a promising cell-free therapeutic option for the treatment of AD, thanks to their role in the de novo synthesis of ceramides, in the activation of genes involved in keratinocyte differentiation, lipid metabolism, cell cycle as well as their intervention in the regulation of the immune response.
Regarding their role as mediators of inflammation, hAT-MSC-derived exosomes significantly decreased the production of pro-inflammatory cytokines [Interleukin (IL) −4, 5, 13, 17], tumor necrosis factor α (TNF-α), interferon gamma (IFN-γ) and thymic stromal lymphopoeitin (TSLP) in the murine model of AD in a dose-dependent manner. Since TSLP is also known to be an important itch inducer, this result implied that AT-MSC exosomes helped reduce itching (Shin et al., 2020) (Supplementary Table 3). Moreover, MSC exosomes promote anti-inflammatory polarization of M2 macrophages and reduce pro-inflammatory polarization of M1 macrophages. In this way, MSC exosomes reduce the expression of Th2 cytokines, including IL-4, IL-13, IL-23, and IL-31, therapeutic targets for AD (Cho et al., 2018; Ha et al., 2020; Shin et al., 2020). In fact, Cho et al. (2018) studied the intravenous (IV) and subcutaneous (SC) administration of hAT-MSC-derived exosomes finding AD symptoms significantly decreased in a dose-dependent manner in both murine models (Supplementary Table 3). These exosomes were able to reduce the clinical score, the numbers of MCs, CD86+ cells and CD206+ cells in the dermal tissue, as well as the mRNA levels of associated inflammatory factors (Cho et al., 2018).
Exosomes and Psoriasis
Psoriasis is one of the most common skin diseases, affecting over 125 million people worldwide. It is a chronic skin disease represented by red squamous plaques that usually appear on the elbows, knees, sacroiliac region, nails, and scalp. Its histological features are characterized by epidermal hyperplasia, increased angiogenesis, and immune cell infiltration (Zhang Y. et al., 2020). There is also an extensive list of comorbidities associated with this disease (including Crohn’s disease, psoriatic arthritis, atherogenic dyslipidemia, hypertension, diabetes, as well as increased carotid intima-media thickness) (Martinez-Lopez et al., 2018; Sanabria-de la Torre et al., 2020). It is fundamentally associated with immunological and genetic factors. Treatments are varied and tend to be aimed at reducing skin lesions; this is a current area of research. The therapeutic effects of MSCs on psoriasis have been reported in experimental studies and clinical cases (Chen et al., 2016; De Jesus et al., 2016; Sah et al., 2016). In two clinical cases of psoriasis vulgaris treated with MSCs, both of them remained relapse-free for 4 or 5 years.
As mentioned above, MSC exosomes have therapeutic effects on various relapsing inflammatory disorders such as AD and cGVHD. Accumulative evidence has indicated that MSC exosomes exhibit potent immunomodulatory effects by regulating the activation of immune cells and inhibiting the expression of various inflammatory cytokines (Kordelas et al., 2014; Cho et al., 2018; Lai et al., 2018).
In fact, Zhang Y. et al. (2020) demonstrated that hUC-MSC-derived exosomes prevented the progression and reduced the severity of psoriasis by regulating immune cells, through inhibiting in vitro the maturation and activation of DCs and Th17 cells, along with HaCaTs. In addition, treatment with these exosomes effectively blocked the induction of inflammatory cytokines and reduced both histopathological symptoms and immune responses in a mouse model in vivo (Table 1 and Supplementary Table 3).
Exosomes and Melanoma
The tumor necrosis factor-related apoptosis-inducing ligand (TRAIL) is a member of the TNF family with a selective effect on cancer cells. As a promising agent for cancer therapy, TRAIL can induce cell apoptosis by interaction with its receptors, death receptor 4 and 5 (DR4 and DR5) on tumor cells. Human melanoma cells express TRAIL DR5 (Kurbanov et al., 2005). Murine melanoma cells (B16) express a moderate level of TRAIL-R on their cell surface (Dufour et al., 2017). Mueller et al. demonstrated that TRAIL-modified MSCs (MSC-TRAIL) could effectively induce apoptosis in sensitive advanced colorectal carcinoma cells in vitro and also inhibit tumor growth in animal models (Mueller et al., 2011). Yuan et al. (2017) showed that exosomes derived from MSC-TRAIL (TRAIL exosomes) were capable of inducing effective apoptosis in a wide range of cancer cell lines including lung adenocarcinoma, primary normal human bronchial epithelial cells, renal cancer cells, breast adenocarcinoma and neuroblastoma cell lines in vitro. Compared to recombinant soluble TRAIL, TRAIL exosomes exert a greater killing efficiency of cancer cells. Due to the fluidic nature of the bilayer membrane, the lipid membrane of TRAIL exosomes allows higher TRAIL oligomerization and subsequently higher clustering of its receptors (Yuan et al., 2017). Also, the low pH condition of tumor microenvironment potentially influenced exosome trafficking and uptake by cancer cells (Parolini et al., 2009).
In a recent study, Shamili et al. (2018) investigated the anti-tumor effect of TRAIL exosomes, drawing on the combined use of tumor-specific cytotoxicity of TRAIL and intrinsic properties of BM-MSC-derived exosomes on tumor cells. Specifically, the anti-tumor activity of MSC exosomes and TRAIL exosomes was analyzed in vitro and in three in vivo models (Table 1 and Supplementary Table 3).
In vitro analysis (Table 1) consisted of a co-culture of the exosomes with murine melanoma cells (B16F0 cells). There was a significant difference in cell viability between cells treated with TRAIL exosomes and those treated with MSC exosomes. Specifically, TRAIL exosomes and MSC exosomes induced 11.93 and 4.69% apoptosis in B16F0 cells, respectively (Shamili et al., 2018). To evaluate the in vivo anti-tumor activity of these exosomes (Supplementary Table 3), three models of melanoma tumor-bearing mice were developed. In the co-delivery model, tumor cells were co-injected with exosomes and in the non-co-delivery model exosomes were injected after tumor appearance in a single dose or in multiple doses. The co-delivery model resulted in a delayed appearance of the tumor for 6 days and a reduction in size. The non-co-delivery administration with a single dose showed anti-tumor activity in early days, delayed tumor growth and an increased life expectancy of 4 days. Finally, non-co-delivery administration with multiples doses also resulted in a significant reduction of tumor size and an increased life expectancy of 8 days. Delayed tumor growth was higher compared to the single doses. In summary, it was demonstrated that MSC exosomes and TRAIL exosomes have potential capacity as targeted cancer treatment, with the best option being TRAIL exosomes. Specifically, the best administration option was delivery after tumor appearance of TRAIL exosomes in multidose. These exosomes could be exploited for drug delivery purposes to deliver therapeutic agents. In addition, this study highlights the possibility of combining TRAIL exosomes and chemotherapeutics as a promising anti-tumor strategy (Shamili et al., 2018).
de Araujo Farias et al. (2018) investigated the role of exosomes derived from irradiated MSCs (irMSC exosomes) A375 melanoma cell line in vitro and in tumor growth and metastasis retardation after treatment with MSC + radiotherapy in vivo (Table 1 and Supplementary Table 3). In this study, the authors report the possible implication of exosomes cargo, specifically annexins (ANXA1 and ANAX2), integrins (ITGB1 and ITGA3), fibronectin 1 (FN1), catenin β1 (CTNNB1) and apolipoprotein H (APOH), in their suppressive action on A375 cells survival. Regarding in vivo outcomes, the ability of MSCs to accumulate at tumor sites makes them extremely attractive for targeted cancer therapy; in addition, the tumor tropism of MSCs has been reported to increase with radiation therapy (Kim et al., 2010). MSCs combined with radiation therapy enhances the effects of radiation on the metastatic spread of melanoma cells and exosome-derived factors could be involved in these effects (de Araujo Farias et al., 2018). MSC exosomes appear to bind to specific membrane microdomains in tumor cells, amplifying the action of radiation therapy, stimulating tumor cell death, thus increasing the sensitivity of cells to radiation and promoting systemic effects (Kim et al., 2010). Radiation therapy itself may not be systemic, although it could contribute to a systemic effect when used in combination with MSCs due to the ability of irMSC exosomes to increase the control of tumor growth and metastasis (de Araujo Farias et al., 2018).
Discussion
Exosomes derived from MSCs are characterized by having immunomodulatory and regenerative properties and because of this they have gained much attention as a new potential cell-free approach in this field by overcoming the inherent limitations of the use of MSCs in the treatment of inflammatory skin diseases. Although the role of MSC exosomes in wound healing has been discussed (Roşca et al., 2018; Hu et al., 2019; Casado-Díaz et al., 2020), exosomes have been reported to play crucial roles in other skin conditions. In the present review, the function of MSC exosomes not only in wound healing and skin regeneration but also in oxidative stress and rejuvenation, hair growth, melanoma and autoimmune disorders such as GVHD, psoriasis and AD has been analyzed.
In this context, different signaling routes and molecules where exosomes are implicated have been proposed in the available bibliography, depending on the specific study. Regarding wound healing and skin regeneration, the most-reported signaling pathways include Wnt/β-catenin, PI3K/AKT, ERK, and TGF-β/Smad. Wnt/β-catenin and AKT cascades seem to participate in hair growth as well. Different miRNA and other molecules may also be involved. The NRF2 defense system is related to the oxidative stress process. With respect to autoimmune disorders, MSC exosomes seem to reduce pro-inflammatory cytokines and to promote induction of regulatory T cells. Finally, in melanomas, the administration of exosomes is capable of reducing tumor growth and size and promoting life span of in vivo models.
In addition to the mechanism of action, the origin of the exosomes can also affect their role. It is important to analyze the differences between fetal and adult MSC-derived exosomes. Firstly, regarding the source of MSCs, several aspects have to been taken into account. Commonly used adults MSCs as source of exosomes include AT-MSCs and BM-MSCs. AT-MSCs are usually isolated from left over biological material generated during liposuction, lipoplasty, or lipectomy procedures while BM-MSCs are obtained from bone marrow aspirate, an invasive and painful procedure (Hass et al., 2011). The most used fetal MSC source of exosomes is UC-MSCs. Other fetal MSCs include ESC-MSCs and FD-MSCs. Advantages derived from the use of these MSCs over adult MSCs comprise their ready availability and their obtaining from no invasive procedures. Furthermore, fetal MSCs have shown improved proliferative capacity, life span and differentiation potential (Hass et al., 2011). Considering the role of exosomes derived from both fetal and adult MSCs, several points need to be highlighted. Regarding fetal MSC-derived exosomes, UC-MSC-derived exosomes are implicated in wound healing, oxidative stress defense process, rejuvenation and psoriasis. No other source, fetal or adult, of exosomes has been used for testing its role in the oxidative stress defense process, rejuvenation and psoriasis. Regarding wound healing, FD-MSCs and iMSC (from Wharton’s Jelly derived IPSCs) have also been used. ESC-MSC-derived exosomes have showed beneficial effects in GVHD. Concerning adult MSC, AT-MSC-derived exosomes are implicated in wound healing and AD. No other source of MSC has been used for testing exosome’s role in AD. Among adult MSCs, just BM-MSC-derived exosomes have been shown improvements on acute, chronic and refractory GVHD, melanoma and hair growth. Men-MSC- and G-MSC-derived exosomes have improved wound healing outcomes. Comparative studies are lacking about specific differences between exosomes from fetal MSC and from adult MSC.
Regarding the possible clinical translation of exosomes, it is essential to determine the optimal source of MSCs. Cell senescence due to culture passages and time can alter the exosome cargos and therefore their properties. In this sense, iMSC is a strategy for exosome production with several advantages. Thus, established iPSCs can expand indefinitely and easily become iMSCs within 20 days. Moreover, the in vitro growth and differentiation potential of MSCs is affected by the culture period, the donor’s age and the donor’s health status, factors that are also resolved with the use of iMSC. Furthermore, iMSCs avoid ethical problems and immune rejection (Kim et al., 2018). In this sense, MSCs from oral cavities are more easily harvestable than other anatomic sites and have shown a great plasticity toward the main lineages, specifically toward bone tissues. Human periapical inflamed cyst in particular represents a promising source of MSCs because of the reduced ethical and biological issues as it is usually considered biological waste (Spagnuolo et al., 2018; Tatullo et al., 2019). Moreover, it is important to determine the optimal culture conditions. As mentioned throughout this review, it is feasible to condition MSC media in order to enhance determined exosome features depending on the study. In addition, the methodology for isolating and purifying exosomes must be highly controlled and optimized.
However, due to the lack of clinical trials, the heterogeneity of MSC products prepared by different laboratories, diverse strategies of MSC isolation and absence of standardization across groups, it is difficult to achieve the MSC exosome bench to bedside translation as any new therapeutic platform requires the establishment of GMP conditions and standards for production. In case of exosomes, there are some limitations to overcome. These limitations include large-scale production, downstream purification methods, and quality control systems (Jafari et al., 2020). Regarding limitations on exosome’s production, studies about scaling up cell culture have focused on technologies to maximize surface area (Colao et al., 2018). Hollow-fiber bioreactors, which are high-density systems for culturing cells, allow continuously production of exosomes for more than 10 weeks, without splitting or subculturing the cells, increasing the exosome production from 10 to 100 fold (Whitford et al., 2015; Colao et al., 2018; Jafari et al., 2020). Other strategies include genetic manipulation of MSCs for producing an immortalized cell line, which continuously produced exosomes or the design of PEGylated wells for the aggregation of cells and the formation of MSC spheroids to enhance cell-to-cell communication (Jafari et al., 2020). Once scaling up cell culture of MSC for large-scale production of exosomes is achieve, it is necessary to define them physically, biochemically and functionally by quantifiable features and using reproducible and standardized assays. Members of the Society for Clinical Research and Translation of Extracellular Vesicles Singapore (SOCRATES), the International Society for Extracellular Vesicles (ISEV), the International Society for Cell and Gene Therapy (ISCT) and the International Society of Blood Transfusion (ISBT) have proposed specific harmonization criteria for MSC-small EVs (sEVs) to facilitate data sharing and comparison (Witwer et al., 2019). Precisely, MSC-sEVs should be defined by quantifiable metrics to identify the cellular origin of the sEVs in a preparation, presence of lipid-membrane vesicles and the degree of physical and biochemical integrity of the vesicles. Therefore, specifications related to purity, identity, quantity, potency, and sterility need to be defined in accordance with the regulations for pharmaceutical manufacturing (Gimona et al., 2017). Quality can be assessed by producing them through validated procedures. Sterility testing is achieved by stablished protocols for detection of microbiological and endotoxin contaminations. Potency issues relies on more molecular biology and physiology information. In fact, qualified potency assays in disease-relevant in vitro and in vivo models are necessary to elucidate their mechanism of action (Gimona et al., 2017; Jafari et al., 2020). Limitations on downstream purification and characterization methods make it difficult to determine purity and identity of exosomes. In these aspects, heterogeneity is a critical issue. Research efforts are focus on strategies to improve the isolation of exosomes, although there is no consensus on the most suitable method for exosomes enrichment and purification (Gimona et al., 2017). Some recent technologies include serial filtration, tangential flow filtration (TFF) or polymer precipitation (Gimona et al., 2017; Colao et al., 2018; Jafari et al., 2020). Standardized GMP-compliant TFF systems are available on the market, offering the possibility of validated process control. Another promising strategy comprises sequential filtration followed by affinity-based chromatography that specific surface proteins (Jafari et al., 2020). Considering identity of exosomes, the ISEV has established a set of criteria for their proteomic identification with a minimal list of requirements: exosomes should (1) have transmembrane proteins to provide evidence of a membrane; (2) have cytosolic proteins to provide evidence of membrane- or receptor-binding; (3) be free of protein impurities from intracellular compartments not associated with the plasma membranes or endosomes; and (4) be free of co-isolating extracellular proteins (Colao et al., 2018). Characterization approaches of exosomes include nanoparticle tracking analysis (NTA) and dynamic light scattering (DLS) for size distribution and zeta potential determination, ELISA assay, Western blotting, and flow cytometry for exosome protein analysis and quantitative reverse transcription-PCR (qRT-PCR), mass spectrometry, and miRNA array for exosome content determination (Jafari et al., 2020). However, since exosomes are still difficult to purify, minimal acceptance and release criteria include these statements: (1) MSCs display an ISCT-compliant surface marker profile at the time of secretome harvest; (2) EVs within the secretome fraction must comply with the minimal criteria of ISEV, at least for a number of membrane markers; (3) The size range should be in the range of exosomes (50–150 nm) and (4) Sterility and endotoxin levels must comply with regulatory requirements (Gimona et al., 2017).
Another factor to consider is the in vivo administration of exosomes. As has been shown, subcutaneous and intravenous are the most frequently used administrations although it is possible to deliver them through different kinds of hydrogels. In this context, scaffolds have recently gained much attention. In fact, the geometrical and mechanical properties of scaffolds are able to influence the secretome, interactome and cell behavior (Ballini et al., 2017). Computer-aided design (CAD) has emerged as a promising strategy for biomimetic scaffold manufacturing as it allows the development of standardized design to test different types of scaffolds by reducing bias during measurements (Marrelli et al., 2016; Ballini et al., 2017). Thus, it is important to optimize the application of EVs for skin wound healing. Therefore, it would be convenient to optimize and analyze the advantages and disadvantages of the different application methodologies for each specific clinical objective.
In conclusion, the currently available data in this field shows how MSC exosomes can improve critical parameters of preclinical and clinical models of several skin conditions, including wound healing, oxidative stress, photoaging and rejuvenation, AD, psoriasis, GVHD and melanoma. Exosomes stimulate the migration and proliferation of fibroblast and keratinocytes, promote angiogenesis, collagen synthesis, re-epithelization and wound closure in wound healing, stimulate hair growth and have immunoregulatory effects on inflammatory skin diseases. Regarding oxidative stress, they are able to decrease ROS generation and DNA damage. Lastly, in melanoma, exosomes reduce tumor growth and promote life span. However, very few studies have reported their role on human skin disease models. Kwon et al. (2020) conducted a 12-week prospective, double-blind, randomized, split-face, comparative study to evaluate the clinical efficacy of application of hAT-MSC exosomes after fractional CO2 laser in the treatment of facial acne scars. The application of exosomes treatment yielded more favorable responses, a shorter recovery time, and fewer side-effects (Kwon et al., 2020). Regarding clinical trials, to date there are only two about MSC exosome treatment on humans (NCT04173650 and NCT04213248). The first trial will analyze the impact of MSC exosomes on dystrophic epidermolysis bullosa human wounds and the second their effect on dry eye symptoms in patients with cGVHD. However, both are still in the recruitment phase.
Therefore, further research is required for a better understanding of the molecular mechanisms underlying the action and therapeutic potency of MSC exosomes in the clinical context. In order to assess their clinical translation, methods for large-scale production should be optimized and standardized, including the source of MSCs, their isolation and culture conditions as well as the administration protocols for exosomes.
Author Contributions
MQ-V had the conception, revised the bibliography, and wrote the manuscript. RS-DT and AF-G revised the bibliography and the different versions of the manuscript. MS-D, ÁS-S, and TM-V revised the different versions of the manuscript. SA-S had the conception, revised bibliography and the different versions of the manuscript. All the authors contributed to the article and approved the submitted version.
Funding
This study has been funded by the Carlos III Health Institute of Spain through the PI13/02576 and PI17/02083 projects [co-funded by European Regional Development Fund “A way to make Europe” and Andalusian Regional Government Finance (SAS PI-0458-2016)]. The work of MQ-V was supported by a predoctoral fellowship (BOE 22/10/2019) from the Spanish Ministry of Science, Innovation and Universities. This study is part of her doctoral research in the Biomedicine program at the University of Granada.
Conflict of Interest
The authors declare that the research was conducted in the absence of any commercial or financial relationships that could be construed as a potential conflict of interest.
Acknowledgments
We gratefully acknowledge financial support from the Carlos III Health Institute of Spain (PI13/02576 and PI17/02083) and Andalusian Regional Government Finance (SAS PI-0458-2016). The work of MQ-V was supported by a predoctoral fellowship (BOE 22/10/2019) from the Spanish Ministry of Science, Innovation and Universities. This study is part of her doctoral research in the Biomedicine program at the University of Granada.
Supplementary Material
The Supplementary Material for this article can be found online at: https://www.frontiersin.org/articles/10.3389/fcell.2021.647012/full#supplementary-material
Supplementary Table 1 | In vitro studies of MSC exosomes in wound healing.
Supplementary Table 2 | In vivo studies of MSC exosomes in wound healing.
Supplementary Table 3 | In vivo studies of MSC exosomes in other skin conditions.
References
Aheget, H., Tristán-Manzano, M., Mazini, L., Cortijo-Gutierrez, M., Galindo-Moreno, P., Herrera, C., et al. (2020). Exosome: a new player in translational nanomedicine. J. Clin. Med. 9:2380. doi: 10.3390/jcm9082380
An, T., Chen, Y., Tu, Y., and Lin, P. (2020). Mesenchymal stromal cell-derived extracellular vesicles in the treatment of diabetic foot ulcers: application and challenges. Stem Cell Rev. Rep. 1–10. doi: 10.1007/s12015-020-10014-9
Andreu, Z., and Yáñez-Mó, M. (2014). Tetraspanins in extracellular vesicle formation and function. Front. Immunol. 5:442. doi: 10.3389/fimmu.2014.00442
Bai, Y., Han, Y. D., Yan, X. L., Ren, J., Zeng, Q., Li, X. D., et al. (2018). Adipose mesenchymal stem cell-derived exosomes stimulated by hydrogen peroxide enhanced skin flap recovery in ischemia-reperfusion injury. Biochem. Biophys. Res. Commun. 500, 310–317. doi: 10.1016/j.bbrc.2018.04.065
Ballini, A., Boccaccio, A., Saini, R., Van Pham, P., and Tatullo, M. (2017). Dental-derived stem cells and their secretome and interactions with Bioscaffolds/Biomaterials in regenerative medicine: from the in vitro research to translational applications. Stem Cells Int. 2017, 15–17. doi: 10.1155/2017/6975251
Ballini, A., Cantore, S., Scacco, S., Coletti, D., and Tatullo, M. (2018). Mesenchymal stem cells as promoters, enhancers, and playmakers of the translational regenerative medicine 2018. Stem Cells Int. 2018:6927401. doi: 10.1155/2018/6927401
Carrasco, E., Soto-Heredero, G., and Mittelbrunn, M. (2019). The role of extracellular vesicles in cutaneous remodeling and hair follicle dynamics. Int. J. Mol. Sci. 20:2758. doi: 10.3390/ijms20112758
Casado-Díaz, A., Quesada-Gómez, J. M., and Dorado, G. (2020). Extracellular vesicles derived from mesenchymal stem cells (MSC) in regenerative medicine: applications in skin wound healing. Front. Bioeng. Biotechnol. 8:146. doi: 10.3389/fbioe.2020.00146
Chen, H., Niu, J. W., Ning, H. M., Pan, X., Li, X. B., Li, Y., et al. (2016). Treatment of psoriasis with mesenchymal stem cells. Am. J. Med. 129, e13–e14. doi: 10.1016/j.amjmed.2015.11.001
Cho, B. S., Kim, J. O., Ha, D. H., and Yi, Y. W. (2018). Exosomes derived from human adipose tissue-derived mesenchymal stem cells alleviate atopic dermatitis. Stem Cell Res. Ther. 9, 1–5. doi: 10.1186/s13287-018-0939-5
Choi, E. W., Seo, M. K., Woo, E. Y., Kim, S. H., Park, E. J., and Kim, S. (2018). Exosomes from human adipose-derived stem cells promote proliferation and migration of skin fibroblasts. Exp. Dermatol. 27, 1170–1172. doi: 10.1111/exd.13451
Colao, I. L., Corteling, R., Bracewell, D., and Wall, I. (2018). Manufacturing exosomes: a promising therapeutic platform. Trends Mol. Med. 24, 242–256. doi: 10.1016/j.molmed.2018.01.006
Dalirfardouei, R., Jamialahmadi, K., Jafarian, A. H., and Mahdipour, E. (2019). Promising effects of exosomes isolated from menstrual blood-derived mesenchymal stem cell on wound-healing process in diabetic mouse model. J. Tissue Eng. Regen. Med. 13, 555–568. doi: 10.1002/term.2799
de Araujo Farias, V., O’Valle, F., Serrano-Saenz, S., Anderson, P., Andrés, E., López-Peñalver, J., et al. (2018). Exosomes derived from mesenchymal stem cells enhance radiotherapy-induced cell death in tumor and metastatic tumor foci. Mol. Cancer 17, 1–12. doi: 10.1186/s12943-018-0867-0
De Jesus, M. M., Santiago, J. S., Trinidad, C. V., See, M. E., Semon, K. R., Fernandez, M. O., et al. (2016). Autologous adipose-derived mesenchymal stromal cells for the treatment of psoriasis vulgaris and psoriatic arthritis: a case report. Cell Transplant. 25, 2063–2069. doi: 10.3727/096368916X691998
Ding, J., Wang, X., Chen, B., Zhang, J., and Xu, J. (2019). Exosomes derived from human bone marrow mesenchymal stem cells stimulated by deferoxamine accelerate cutaneous wound healing by promoting angiogenesis. Biomed. Res. Int. 2019:9742765. doi: 10.1155/2019/9742765
Dufour, F., Rattier, T., Shirley, S., Picarda, G., Constantinescu, A. A., Morlé, A., et al. (2017). N-glycosylation of mouse TRAIL-R and human TRAIL-R1 enhances TRAIL-induced death. Cell Death Differ. 24, 500–l17. N-glycosylation of mouse TRAIL-R and human TRAIL-R1 enhances TRAIL-induced death. Cell Death Differ. 24, 500–510. doi: 10.1038/cdd.2016.150
El-Tookhy, O. S., Shamaa, A. A., Shehab, G. G., Abdallah, A. N., and Azzam, O. M. (2017). Histological evaluation of experimentally induced critical size defect skin wounds using exosomal solution of mesenchymal stem cells derived microvesicles. Int. J. Stem Cells 10, 144–153. doi: 10.15283/ijsc17043
Fang, S., Xu, C., Zhang, Y., Xue, C., Yang, C., Bi, H., et al. (2016). Umbilical cord-derived mesenchymal stem cell-derived exosomal MicroRNAs suppress myofibroblast differentiation by inhibiting the transforming growth factor-b/SMAD2 pathway during wound healing. Stem Cells Transl. Med. 5, 1425–1439.
Ferreira, A. D. F., and Gomes, D. A. (2019). Stem cell extracellular vesicles in skin repair. Bioengineering 6, 1–18. doi: 10.3390/bioengineering6010004
Fujii, S., Miura, Y., Fujishiro, A., Shindo, T., Shimazu, Y., Hirai, H., et al. (2018). Graft-versus-host disease amelioration by human bone marrow mesenchymal stromal/stem cell-derived extracellular vesicles is associated with peripheral preservation of naive T Cell populations. Stem Cells 36, 434–445. doi: 10.1002/stem.2759
Fukuoka, H., Narita, K., and Suga, H. (2017). Hair regeneration therapy: application of adipose-derived stem cells. Curr. Stem Cell Res. Ther. 12, 531–534. doi: 10.2174/1574888x12666170522114307
Gao, S., Chen, T., Hao, Y., Zhang, F., Tang, X., Wang, D., et al. (2020). Exosomal miR-135a derived from human amnion mesenchymal stem cells promotes cutaneous wound healing in rats and fibroblast migration by directly inhibiting LATS2 expression. Stem Cell Res. Ther. 11, 1–11. doi: 10.1186/s13287-020-1570-9
Gardiner, C., Di Vizio, D., Sahoo, S., Théry, C., Witwer, K. W., Wauben, M., et al. (2016). Techniques used for the isolation and characterization of extracellular vesicles: results of a worldwide survey. J. Extracell. Vesicles 5, 1–6. doi: 10.3402/jev.v5.32945
Gimona, M., Pachler, K., Laner-Plamberger, S., Schallmoser, K., and Rohde, E. (2017). Manufacturing of human extracellular vesicle-based therapeutics for clinical use. Int. J. Mol. Sci. 18:1190. doi: 10.3390/ijms18061190
Ha, D. H., Kim, H., Lee, J., Kwon, H. H., Park, G., Yang, S. H., et al. (2020). Mesenchymal Stem/Stromal cell-derived exosomes for immunomodulatory therapeutics and skin regeneration. Cells 9:1157.
Hass, R., Kasper, C., Böhm, S., and Jacobs, R. (2011). Different populations and sources of human mesenchymal stem cells (MSC): a comparison of adult and neonatal tissue-derived MSC. Cell Commun. Signal. 9, 1–14. doi: 10.1186/1478-811X-9-12
He, L., Zhu, C., Jia, J., Hao, X. Y., Yu, X. Y., Liu, X. Y., et al. (2020). ADSC-Exos containing MALAT1 promotes wound healing by targeting miR-124 through activating Wnt/β-catenin pathway. Biosci. Rep. 40, 1–13. doi: 10.1042/BSR20192549
Hoang, D. H., Nguyen, T. D., Nguyen, H. P., Nguyen, X. H., Do, P. T. X., Dang, V. D., et al. (2020). Differential wound healing capacity of mesenchymal stem cell-derived exosomes originated from bone marrow, adipose tissue and umbilical cord under serum- and xeno-free condition. Front. Mol. Biosci. 7:119. doi: 10.3389/fmolb.2020.00119
Hu, J., Chen, Y., Huang, Y., and Su, Y. (2020). Human umbilical cord mesenchymal stem cell-derived exosomes suppress dermal fibroblasts-myofibroblats transition via inhibiting the TGF-β1/Smad 2/3 signaling pathway. Exp. Mol. Pathol. 115:104468. doi: 10.1016/j.yexmp.2020.104468
Hu, L., Wang, J., Zhou, X., Xiong, Z., Zhao, J., Yu, R., et al. (2016). Exosomes derived from human adipose mensenchymal stem cells accelerates cutaneous wound healing via optimizing the characteristics of fibroblasts. Sci. Rep. 6, 1–11. doi: 10.1038/srep32993
Hu, P., Yang, Q., Wang, Q., Shi, C., Wang, D., Armato, U., et al. (2019). Mesenchymal stromal cells-exosomes: a promising cell-free therapeutic tool for wound healing and cutaneous regeneration. Burn. Trauma 7, 1–10. doi: 10.1186/s41038-019-0178-8
Imokawa, G., Abe, A., Jin, K., Higaki, Y., Kawashima, M., and Hidano, A. (1990). Decreased level of ceramides in stratum corneum of atopic dermatitis: an etiologic factor in atopic dry skin? J. Invest. Dermatol. 96, 523–526.
Jafari, D., Malih, S., Eini, M., Jafari, R., Gholipourmalekabadi, M., Sadeghizadeh, M., et al. (2020). Improvement, scaling-up, and downstream analysis of exosome production. Crit. Rev. Biotechnol. 40, 1098–1112. doi: 10.1080/07388551.2020.1805406
Jiang, L., Zhang, Y., Liu, T., Wang, X., Wang, H., Song, H., et al. (2020). Exosomes derived from TSG-6 modified mesenchymal stromal cells attenuate scar formation during wound healing. Biochimie 177, 40–49. doi: 10.1016/j.biochi.2020.08.003
Jiang, T., Wang, Z., and Sun, J. (2020). Human bone marrow mesenchymal stem cell-derived exosomes stimulate cutaneous wound healing mediates through TGF-β/Smad signaling pathway. Stem Cell Res. Ther. 11, 1–10. doi: 10.1186/s13287-020-01723-6
Kim, S., Lee, S. K., Kim, H., and Kim, T. M. (2018). Exosomes secreted from induced pluripotent stem cell-derived mesenchymal stem cells accelerate skin cell proliferation. Int. J. Mol. Sci. 19:3119. doi: 10.3390/ijms19103119
Kim, S. M., Oh, J. H., Park, S. A., Rhu, C. H., Lim, J. Y., Kim, D. S., et al. (2010). Irradiation enhances the tumor tropism and therapeutic potential of tumor necrosis factor-related apoptosis-inducing ligand-secreting human umbilical cord blood-derived mesenchymal stem cells in glioma therapy. Stem Cells 28, 2217–2228. doi: 10.1002/stem.543
Kim, Y. J., Yoo, S. M., Park, H. H., Lim, H. J., Kim, Y. L., Lee, S., et al. (2017). Exosomes derived from human umbilical cord blood mesenchymal stem cells stimulates rejuvenation of human skin. Biochem. Biophys. Res. Commun. 493, 1102–1108. doi: 10.1016/j.bbrc.2017.09.056
Kishimoto, J., Burgeson, R. E., and Morgan, B. A. (2000). Wnt signaling maintains the hair-inducing activity of the dermal papilla. Genes Dev. 14, 1181–1185. doi: 10.1101/gad.14.10.1181
Kordelas, L., Rebmann, V., Ludwig, A. K., Radtke, S., Ruesing, J., Doeppner, T. R., et al. (2014). MSC-derived exosomes: a novel tool to treat therapy-refractory graft-versus-host disease. Leukemia 28, 970–973. doi: 10.1038/leu.2014.41
Kucharzewski, M., Rojczyk, E., Wilemska-Kucharzewska, K., Wilk, R., Hudecki, J., and Los, M. J. (2019). Novel trends in application of stem cells in skin wound healing. Eur. J. Pharmacol. 843, 307–315. doi: 10.1016/j.ejphar.2018.12.012
Kurbanov, B. M., Geilen, C. C., Fecker, L. F., Orfanos, C. E., and Eberle, J. (2005). Efficient TRAIL-R1/DR4-mediated apoptosis in melanoma cells by tumor necrosis factor-related apoptosis-inducing ligand (TRAIL). J. Invest. Dermatol. 125, 1010–1019. doi: 10.1111/j.0022-202X.2005.23900.x
Kwack, M. H., Seo, C. H., Gangadaran, P., Ahn, B. C., Kim, M. K., Kim, J. C., et al. (2019). Exosomes derived from human dermal papilla cells promote hair growth in cultured human hair follicles and augment the hair-inductive capacity of cultured dermal papilla spheres. Exp. Dermatol. 28, 854–857. doi: 10.1111/exd.13927
Kwon, H. H., Yang, S. H., Lee, J., Park, B. C., Park, K. Y., Jung, J. Y., et al. (2020). Combination treatment with human adipose tissue stem cellderived exosomes and fractional co2 laser for acne scars: a 12-week prospective, double-blind, randomized, split-face study. Acta Derm. Venereol. 100, 1–8. doi: 10.2340/00015555-3666
Lai, P., Chen, X., Guo, L., Wang, Y., Liu, X., Liu, Y., et al. (2018). A potent immunomodulatory role of exosomes derived from mesenchymal stromal cells in preventing cGVHD. J. Hematol. Oncol. 11, 1–15. doi: 10.1186/s13045-018-0680-7
Lai, R. C., Arslan, F., Lee, M. M., Sze, N. S. K., Choo, A., Chen, T. S., et al. (2010). Exosome secreted by MSC reduces myocardial ischemia/reperfusion injury. Stem Cell Res. 4, 214–222. doi: 10.1016/j.scr.2009.12.003
Lee, C., Mitsialis, S. A., Aslam, M., Vitali, S. H., Vergadi, E., Konstantinou, G., et al. (2012). Exosomes mediate the cytoprotective action of mesenchymal stromal cells on hypoxia-induced pulmonary hypertension. Circulation 126, 2601–2611. doi: 10.1161/CIRCULATIONAHA.112.114173
Leung, D. Y. M. (2000). Atopic dermatitis: new insights and opportunities for therapeutic intervention. J. Allergy Clin. Immunol. 105, 860–876. doi: 10.1067/mai.2000.106484
Leung, D. Y. M. (2013). New insights into atopic dermatitis: role of skin barrier and immune dysregulation. Allergol. Int. 62, 151–161. doi: 10.2332/allergolint.13-RAI-0564
Li, C., Guo, F., Wang, X., Liu, D., Wu, B., Wang, F., et al. (2020). Exosome-based targeted RNA delivery for immune tolerance induction in skin transplantation. J. Biomed. Mater. Res. Part A 108, 1493–1500. doi: 10.1002/jbm.a.36919
Li, X., Wang, Y., Shi, L., Li, B., Li, J., Wei, Z., et al. (2020). Magnetic targeting enhances the cutaneous wound healing effects of human mesenchymal stem cell-derived iron oxide exosomes. J. Nanobiotechnol. 18, 1–14. doi: 10.1186/s12951-020-00670-x
Li, T., Yan, Y., Wang, B., Qian, H., Zhang, X., Shen, L., et al. (2013). Exosomes derived from human umbilical cord mesenchymal stem cells alleviate liver fibrosis. Stem Cells Dev. 22, 845–854. doi: 10.1089/scd.2012.0395
Lim, S. K., Yeo, M. S. W., Chen, T. S., and Lai, R. C. (2015). Use of Exosomes to Promote or Enhance Hair Growth. U.S. Patent Application No 14/444,113, 22 Ene. 2015. Munich: European Patent Office.
Liu, Y., Wang, H., and Wang, J. (2018). Exosomes as a novel pathway for regulating development and diseases of the skin. Biomed. Rep. 8, 207–214. doi: 10.3892/br.2018.1054
Liu, J., Yan, Z., Yang, F., Huang, Y., Yu, Y., Zhou, L., et al. (2020). Exosomes derived from human umbilical cord mesenchymal stem cells accelerate cutaneous wound healing by enhancing angiogenesis through delivering angiopoietin-2. Stem Cell Rev. Rep. 1–13. doi: 10.1007/s12015-020-09992-7
Ma, T., Fu, B., Yang, X., Xiao, Y., and Pan, M. (2019). Adipose mesenchymal stem cell-derived exosomes promote cell proliferation, migration, and inhibit cell apoptosis via Wnt/β-catenin signaling in cutaneous wound healing. J. Cell. Biochem. 120, 10847–10854. doi: 10.1002/jcb.28376
MacDonald, K. P. A., Hill, G. R., and Blazar, B. R. (2017). Chronic graft-versus-host disease: biological insights from preclinical and clinical studies. Blood 129, 13–21. doi: 10.1182/blood-2016-06-686618
Marrelli, M., Pujia, A., Palmieri, F., Gatto, R., Falisi, G., Gargari, M., et al. (2016). Innovative approach for the in vitro research on biomedical scaffolds designed and customized with CAD-CAM technology. Int. J. Immunopathol. Pharmacol. 29, 778–783. doi: 10.1177/0394632016646121
Martinez-Lopez, A., Blasco-Morente, G., Perez-Lopez, I., Tercedor-Sanchez, J., and Arias-Santiago, S. (2018). Studying the effect of systemic and biological drugs on intima-media thickness in patients suffering from moderate and severe psoriasis. J. Eur. Acad. Dermatol. Venereol. 32, 1492–1498. doi: 10.1111/jdv.14841
Martinez-Lopez, A., Montero-Vilchez, T., Sierra-Sánchez, Á, Molina-Leyva, A., and Arias-Santiago, S. (2020). Advanced medical therapies in the management of non-scarring alopecia: areata and androgenic alopecia. Int. J. Mol. Sci. 21, 1–28. doi: 10.3390/ijms21218390
Mizutani, Y., Mitsutake, S., Tsuji, K., Kihara, A., and Igarashi, Y. (2009). Ceramide biosynthesis in keratinocyte and its role in skin function. Biochimie 91, 784–790. doi: 10.1016/j.biochi.2009.04.001
Mueller, L. P., Luetzkendorf, J., Widder, M., Nerger, K., Caysa, H., and Mueller, T. (2011). TRAIL-transduced multipotent mesenchymal stromal cells (TRAIL-MSC) overcome TRAIL resistance in selected CRC cell lines in vitro and in vivo. Cancer Gene Ther. 18, 229–239. doi: 10.1038/cgt.2010.68
Mysore, V. (2012). Finasteride and sexual side effects. Indian Dermatol. Online J. 3, 62–65. doi: 10.4103/2229-5178.93496
Oualla-Bachiri, W., Fernández-gonzález, A., Quiñones-vico, M. I., and Arias-santiago, S. (2020). From grafts to human bioengineered vascularized skin substitutes. Int. J. Mol. Sci. 21, 1–27. doi: 10.3390/ijms21218197
Parolini, I., Federici, C., Raggi, C., Lugini, L., Palleschi, S., De Milito, A., et al. (2009). Microenvironmental pH is a key factor for exosome traffic in tumor cells. J. Biol. Chem. 284, 34211–34222. doi: 10.1074/jbc.M109.041152
Qiu, G., Zheng, G., Ge, M., Wang, J., Huang, R., Shu, Q., et al. (2019). Functional proteins of mesenchymal stem cell-derived extracellular vesicles. Stem Cell Res. Ther. 10, 1–11. doi: 10.1186/s13287-019-1484-6
Qiu, X., Liu, J., Zheng, C., Su, Y., Bao, L., Zhu, B., et al. (2020). Exosomes released from educated mesenchymal stem cells accelerate cutaneous wound healing via promoting angiogenesis. Cell Prolif. 53, 1–17. doi: 10.1111/cpr.12830
Rajendran, R. L., Gangadaran, P., Bak, S. S., Oh, J. M., Kalimuthu, S., Lee, H. W., et al. (2017). Extracellular vesicles derived from MSCs activates dermal papilla cell in vitro and promotes hair follicle conversion from telogen to anagen in mice. Sci. Rep. 7, 1–12. doi: 10.1038/s41598-017-15505-3
Roşca, A. M., Ţuţuianu, R., and Titorencu, I. D. (2018). Mesenchymal stromal cells derived exosomes as tools for chronic wound healing therapy. Rom. J. Morphol. Embryol. 59, 655–662.
Sah, S. K., Park, K. H., Yun, C. O., Kang, K. S., and Kim, T. Y. (2016). Effects of human mesenchymal stem cells transduced with superoxide dismutase on imiquimod-induced psoriasis-like skin inflammation in mice. Antioxidants Redox Signal. 24, 233–248. doi: 10.1089/ars.2015.6368
Sanabria-de la Torre, R., Fernández-gonzález, A., and Quiñones-vico, M. I. (2020). Bioengineered skin intended as in vitro model for pharmacosmetics, skin disease study and environmental skin impact analysis. Biomedicines 8:464. doi: 10.3390/biomedicines8110464
Schäfer, M., Farwanah, H., Willrodt, A. H., Huebner, A. J., Sandhoff, K., Roop, D., et al. (2012). Nrf2 links epidermal barrier function with antioxidant defense. EMBO Mol. Med. 4, 364–379. doi: 10.1002/emmm.201200219
Shabbir, A., Cox, A., Rodriguez-Menocal, L., Salgado, M., and Van Badiavas, E. (2015). Mesenchymal stem cell exosomes induce proliferation and migration of normal and chronic wound fibroblasts, and enhance angiogenesis in vitro. Stem Cells Dev. 24, 1635–1647. doi: 10.1089/scd.2014.0316
Shamili, F. H., Bayegi, H. R., Salmasi, Z., Sadri, K., Mahmoudi, M., Kalantari, M., et al. (2018). Exosomes derived from TRAIL-engineered mesenchymal stem cells with effective anti-tumor activity in a mouse melanoma model. Int. J. Pharm. 549, 218–229. doi: 10.1016/j.ijpharm.2018.07.067
Shi, Q., Qian, Z., Liu, D., Sun, J., Wang, X., Liu, H., et al. (2017). GMSC-derived exosomes combined with a chitosan/silk hydrogel sponge accelerates wound healing in a diabetic rat skin defect model. Front. Physiol. 8:904. doi: 10.3389/fphys.2017.00904
Shi, R., Jin, Y., Hu, W., Lian, W., Cao, C., Han, S., et al. (2020). Exosomes derived from mmu_circ_0000250-modified adipose-derived mesenchymal stem cells promote wound healing in diabetic mice by inducing miR-128-3p/SIRT1-mediated autophagy. Am. J. Physiol. Cell Physiol. 318, C848–C856. doi: 10.1152/ajpcell.00041.2020
Shin, K.-O., Ha, D. H., Kim, J. O., Crumrine, D. A., Meyer, J. M., Wakefield, J. S., et al. (2020). Exosomes from human adipose tissue-derived mesenchymal stem cells promote epidermal barrier repair by inducing de novo synthesis of ceramides in atopic dermatitis. Cells 9:680. doi: 10.3390/cells9030680
Silverberg, N. B., and Silverberg, J. I. (2015). Inside out or outside in: does atopic dermatitis disrupt barrier function or does disruption of barrier function trigger atopic dermatitis? Cutis 96, 359–361.
Spagnuolo, G., Codispoti, B., Marrelli, M., Rengo, C., Rengo, S., and Tatullo, M. (2018). Commitment of oral-derived stem cells in dental and maxillofacial applications. Dent. J. 6, 1–8. doi: 10.3390/dj6040072
Suchonwanit, P., Thammarucha, S., and Leerunyakul, K. (2019). Minoxidil and its use in hair disorders: a review. Drug Des. Devel. Ther. 13, 2777–2786. doi: 10.2147/DDDT.S214907
Tallant, C., Marrero, A., and Gomis-Rüth, F. X. (2010). Matrix metalloproteinases: fold and function of their catalytic domains. Biochim. Biophys. Acta Mol. Cell Res. 1803, 20–28. doi: 10.1016/j.bbamcr.2009.04.003
Tatullo, M., Spagnuolo, G., Codispoti, B., Zamparini, F., Zhang, A., Esposti, M. D., et al. (2019). PLA-based mineral-doped scaffolds seeded with human periapical cyst-derived MSCs: a promising tool for regenerative healing in dentistry. Materials 12, 1–17. doi: 10.3390/ma12040597
Wang, C., Wang, M., Xu, T., Zhang, X., Lin, C., Gao, W., et al. (2019). Engineering bioactive self-healing antibacterial exosomes hydrogel for promoting chronic diabetic wound healing and complete skin regeneration. Theranostics 9, 65–76. doi: 10.7150/thno.29766
Wang, W. M., Wu, C., and Jin, H. Z. (2019). Exosomes in chronic inflammatory skin diseases and skin tumors. Exp. Dermatol. 28, 213–218. doi: 10.1111/exd.13857
Wang, X., Jiao, Y., Pan, Y., Zhang, L., Gong, H., Qi, Y., et al. (2019). Fetal dermal mesenchymal stem cell-derived exosomes accelerate cutaneous wound healing by activating Notch signaling. Stem Cells Int. 2019:2402916. doi: 10.1155/2019/2402916
Wang, L., Hu, L., Zhou, X., Xiong, Z., Zhang, C., Shehada, H. M. A., et al. (2017). Exosomes secreted by human adipose mesenchymal stem cells promote scarless cutaneous repair by regulating extracellular matrix remodelling. Sci. Rep. 7, 1–12. doi: 10.1038/s41598-017-12919-x
Wang, T., Jian, Z., Baskys, A., Yang, J., Li, J., Guo, H., et al. (2020). MSC-derived exosomes protect against oxidative stress-induced skin injury via adaptive regulation of the NRF2 defense system. Biomaterials 257:120264. doi: 10.1016/j.biomaterials.2020.120264
Weinberg, W. C., Goodman, L. V., George, C., Morgan, D. L., Ledbetter, S., Yuspa, S. H., et al. (1993). Reconstitution of hair follicle development In vivo: determination of follicle formation, hair growth, and hair quality by dermal cells. J. Invest. Dermatol. 100, 229–236. doi: 10.1111/1523-1747.ep12468971
Weng, J., He, C., Lai, P., Luo, C., Guo, R., Wu, S., et al. (2012). Mesenchymal stromal cells treatment attenuates dry eye in patients with chronic graft-versus-host disease. Mol. Ther. 20, 2347–2354. doi: 10.1038/mt.2012.208
Whitford, W., Ludlow, J. W., and Cadwell, J. J. S. (2015). Continuous production of exosomes: utilizing the technical advantages of hollow-fiber bioreactor technology. Genet. Eng. & Biotechnol. News 35, 34–34.
Witwer, K. W., Van Balkom, B. W. M., Bruno, S., Choo, A., Dominici, M., Gimona, M., et al. (2019). Defining mesenchymal stromal cell (MSC) -derived small extracellular vesicles for therapeutic applications. J. Extracell. Vesicles 8:1609206. doi: 10.1080/20013078.2019.1609206
Wu, P., Zhang, B., Shi, H., Qian, H., and Xu, W. (2018). MSC-exosome: a novel cell-free therapy for cutaneous regeneration. Cytotherapy 20, 291–301. doi: 10.1016/j.jcyt.2017.11.002
Yan, H., Gao, Y., Ding, Q., Liu, J., Li, Y., Jin, M., et al. (2019). Exosomal micro RNAs derived from dermal papilla cells mediate hair follicle stem cell proliferation and differentiation. Int. J. Biol. Sci. 15, 1368–1382. doi: 10.7150/ijbs.33233
Yang, C., Luo, L., Bai, X., Shen, K., Liu, K., Wang, J., et al. (2020). Highly-expressed micoRNA-21 in adipose derived stem cell exosomes can enhance the migration and proliferation of the HaCaT cells by increasing the MMP-9 expression through the PI3K/AKT pathway. Arch. Biochem. Biophys. 681:108259. doi: 10.1016/j.abb.2020.108259
Yang, J., Chen, Z., Pan, D., Li, H., and Shen, J. (2020). Umbilical cord-derived mesenchymal stem cell-derived exosomes combined pluronic F127 hydrogel promote chronic diabetic wound healing and complete skin regeneration. Int. J. Nanomed. 15, 5911–5926. doi: 10.2147/IJN.S249129
Yu, M., Liu, W., Li, J., Lu, J., Lu, H., Jia, W., et al. (2020). Exosomes derived from atorvastatin-pretreated MSC accelerate diabetic wound repair by enhancing angiogenesis via AKT/eNOS pathway. Stem Cell Res. Ther. 11, 1–17. doi: 10.1186/s13287-020-01824-2
Yuan, Z. Q., Kolluri, K. K., Gowers, K. H. C., and Janes, S. M. (2017). TRAIL delivery by MSC-derived extracellular vesicles is an effective anticancer therapy. J. Extracell. Vesicles 6:1265291. doi: 10.1080/20013078.2017.1265291
Zeiser, R., and Blazar, B. R. (2017). Pathophysiology of chronic graft-versus-host disease and therapeutic targets. N. Engl. J. Med. 377, 2565–2579. doi: 10.1056/nejmra1703472
Zhang, B., Shi, Y., Gong, A., Pan, Z., Shi, H., Yang, H., et al. (2016). HucMSC Exosome-delivered 14-3-3ζ orchestrates self-control of the wnt response via modulation of YAP during cutaneous regeneration. Stem Cells 34, 2485–2500. doi: 10.1002/stem.2432
Zhang, B., Wang, M., Gong, A., Zhang, X., Wu, X., Zhu, Y., et al. (2015a). HucMSc-exosome mediated-Wnt4 signaling is required for cutaneous wound healing. Stem Cells 33, 2158–2168. doi: 10.1002/stem.1771
Zhang, B., Wu, X., Zhang, X., Sun, Y., Yan, Y., Shi, H., et al. (2015b). Human umbilical cord mesenchymal stem cell exosomes enhance angiogenesis through the Wnt4/β-Catenin pathway. Stem Cells Transl. Med. 4, 513–522. doi: 10.5966/sctm.2014-0267
Zhang, B., Yeo, R. W. Y., Lai, R. C., Sim, E. W. K., Chin, K. C., and Lim, S. K. (2018). Mesenchymal stromal cell exosome–enhanced regulatory T-cell production through an antigen-presenting cell–mediated pathway. Cytotherapy 20, 687–696. doi: 10.1016/j.jcyt.2018.02.372
Zhang, W., Bai, X., Zhao, B., Li, Y., Zhang, Y., Li, Z., et al. (2018). Cell-free therapy based on adipose tissue stem cell-derived exosomes promotes wound healing via the PI3K/Akt signaling pathway. Exp. Cell Res. 370, 333–342. doi: 10.1016/j.yexcr.2018.06.035
Zhang, B., Yin, Y., Lai, R. C., Tan, S. S., Choo, A. B. H., and Lim, S. K. (2014). Mesenchymal stem cells secrete immunologically active exosomes. Stem Cells Dev. 23, 1233–1244. doi: 10.1089/scd.2013.0479
Zhang, K., Yu, L., Li, F. R., Li, X., Wang, Z., Zou, X., et al. (2020). Topical application of exosomes derived from human umbilical cord mesenchymal stem cells in combination with sponge spicules for treatment of photoaging. Int. J. Nanomed. 15, 2859–2872. doi: 10.2147/IJN.S249751
Zhang, Y., Yan, J., Li, Z., and Sun, Q. (2020). Exosomes derived from human umbilical cord mesenchymal stem cells alleviate psoriasis-like skin inflammation. J. Invest. Dermatol. 1–21. doi: 10.21203/rs.3.rs-23914/v1
Zhao, B., Zhang, Y., Han, S., Zhang, W., Zhou, Q., Guan, H., et al. (2017). Exosomes derived from human amniotic epithelial cells accelerate wound healing and inhibit scar formation. J. Mol. Histol. 48, 121–132. doi: 10.1007/s10735-017-9711-x
Zhao, G., Liu, F., Liu, Z., Zuo, K., Wang, B., Zhang, Y., et al. (2020). MSC-derived exosomes attenuate cell death through suppressing AIF nucleus translocation and enhance cutaneous wound healing. Stem Cell Res. Ther. 11, 1–18. doi: 10.1186/s13287-020-01616-8
Glossary
Glossary: AD, atopic dermatitis; AT-MSCs, adipose tissue mesenchymal stromal cells; aGVHD, acute graft versus host disease; AKT, protein kinase B; AIF, apoptosis-inducing factor; Ang-2, angiotensin-2; ANX, annexin; APOH, apolipoprotein H; ARG, arginine; ATV, atorvastatin; BM-MSCs, bone marrow mesenchymal stromal cells; BMP, bone morphogenetic protein signaling; CAD, computer-aided design; CCL20, C-C motif chemokine ligand 20; CTNNB1, catenin β1; cGVHD, chronic graft versus host disease; CXCL12, C-X-C motif chemokine 12; DAMPs, damage-associated molecular patterns; DCs, dendritic cells; DP, dermal papilla; DP-MSCs, dermal papilla mesenchymal stromal cells; DR, death receptor; ECM, extracellular matrix; ERK, extracellular signal-regulated kinase; EVs, extracellular vesicles; eNOS, endothelial nitric oxide synthase; ESCRT, endosomal sorting complex required for transport; Exos, exosomes; FD-MSCs, fetal dermal mesenchymal stromal cells; FGF, fibroblast growth factor; FN1, fibronectin 1; GMP, good manufacturing practice; G-MSCs, gingival mesenchymal stromal cells; GVHD, graft versus host disease; HaCaTs, human cultured keratinocytes; hAD-MSCs, human adipose tissue mesenchymal stromal cells; hAECs, human amniotic epithelial cells; hBM-MSCs, human bone marrow mesenchymal stromal cells; HDFs, human dermal fibroblasts; hESC-MSCs, human embryonic stem cell–derived MSCs; HGF, hepatocyte growth factor; HO-1, heme oxygenase 1; hsp, head shock protein; hUC-MSCs, human umbilical cord mesenchymal stromal cells; HUVECs, human umbilical vein endothelial cells; IFN-γ, interferon gamma; IGF, insulin-like growth factor; IL, interleukin; iMSCs, induced mesenchymal stromal cells; iNOS, inducible nitric oxide synthase; irMSCs, irradiated mesenchymal stromal cells; ISBT, International Society of Blood Transfusion; ISCT, International Society for Cell and Gene Therapy; ISEV, International Society of Extracellular Vesicles; ITG, integrin; Keap1, Kelch-like ECH-associated protein 1; KGF, keratinocyte growth factor; LATS, large tumor suppressor; LEF1, lymphoid enhancer-binding factor 1; MALAT1, metastasis associated lung adenocarcinoma transcript 1; MAPK, mitogen-activated protein kinase; Men-MSCs, menstrual blood mesenchymal stromal cells; MMPs, matrix metalloproteinases; MSCs, mesenchymal stromal cells; MSC-TRAIL, tumor necrosis factor-related apoptosis-inducing ligand modified mesenchymal stromal cells; mTOR, mammalian target of rapamycin; MVBs, multivesicular bodies; MWCO, molecular weight cutoff; NLRP3, NOD-like receptor protein 3 inflammasome; NOD-R, nucleotide-binding oligomerization domain-like receptors; NQO1, NAD(P)H quinone dehydrogenase 1; NRF2, nuclear factor E2-related factor 2; PAMPs, pathogen-associated molecular patterns; PBS, phosphate-buffered saline; PARP-1, poly (ADP ribose) polymerase 1; PCNA, proliferating cell nuclear antigen; PDGF-BB, platelet-Derived Growth Factor BB; PDGF-α, platelet-derived growth factor α; PF-127, pluronic F-127; PI3K/AKT, phosphatidylinositol 3-kinase/protein kinase B pathway; PTEN, phosphatase and tensin homolog; ROS, reactive oxygen species; α-SMA, α-smooth muscle actin; SHSs, Haliclona sp. spicules; STAT3, signal transducer and activator of transcription 3; SOCRATES, Society for Clinical Research and Translation of Extracellular Vesicles Singapore; Th, helper T cells; TGF-β1, transforming growth factor β1; TGF-β3, transforming growth factor β3; TIMPs, tissue inhibitor of matrix metalloproteinases; TLRs, toll-like receptors; TNF-α, tumor necrosis factor α; TRAIL, tumor necrosis factor-related apoptosis-inducing ligand; Tregs, regulatory T cells; TSG-6, tumor necrosis factor-inducible gene 6 protein; TSLP, thymic stromal lymphopoeitin; UC-MSCs, umbilical cord mesenchymal stromal cells; UV, ultraviolet; VEGF-A, vascular endothelial growth factor A; YAP, yes-associated protein.
Keywords: exosomes-based therapy, immunomodulation, mesenchymal stem cell-derived exosomes, regenerative medicine, skin autoimmune diseases, skin wound healing
Citation: Quiñones-Vico MI, Sanabria-de la Torre R, Sánchez-Díaz M, Sierra-Sánchez Á, Montero-Vílchez T, Fernández-González A and Arias-Santiago S (2021) The Role of Exosomes Derived From Mesenchymal Stromal Cells in Dermatology. Front. Cell Dev. Biol. 9:647012. doi: 10.3389/fcell.2021.647012
Received: 28 December 2020; Accepted: 01 March 2021;
Published: 07 April 2021.
Edited by:
Vicente Herranz-Pérez, University of Valencia, SpainReviewed by:
Marco Tatullo, University of Bari Medical School, ItalyMelania Lo Iacono, Azienda Ospedaliera Universitaria Policlinico Paolo Giaccone, Italy
Copyright © 2021 Quiñones-Vico, Sanabria-de la Torre, Sánchez-Díaz, Sierra-Sánchez, Montero-Vílchez, Fernández-González and Arias-Santiago. This is an open-access article distributed under the terms of the Creative Commons Attribution License (CC BY). The use, distribution or reproduction in other forums is permitted, provided the original author(s) and the copyright owner(s) are credited and that the original publication in this journal is cited, in accordance with accepted academic practice. No use, distribution or reproduction is permitted which does not comply with these terms.
*Correspondence: Manuel Sánchez-Díaz, bWFub2xvLjk0LnNhbmNoZXpAZ21haWwuY29t