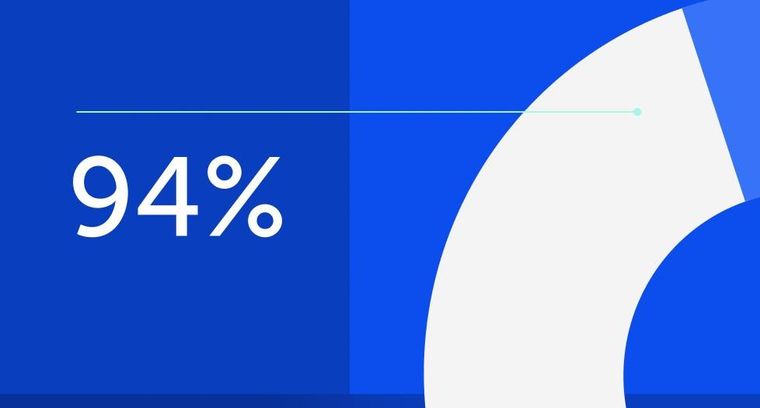
94% of researchers rate our articles as excellent or good
Learn more about the work of our research integrity team to safeguard the quality of each article we publish.
Find out more
ORIGINAL RESEARCH article
Front. Cell Dev. Biol., 29 April 2021
Sec. Cell Growth and Division
Volume 9 - 2021 | https://doi.org/10.3389/fcell.2021.645386
This article is part of the Research TopicCellular Mechanisms during Normal and Abnormal Craniofacial DevelopmentView all 22 articles
Epigenetic and chromatin regulation of craniofacial development remains poorly understood. Ankyrin Repeat Domain 11 (ANKRD11) is a chromatin regulator that has previously been shown to control neural stem cell fates via modulation of histone acetylation. ANKRD11 gene variants, or microdeletions of the 16q24.3 chromosomal region encompassing the ANKRD11 gene, cause KBG syndrome, a rare autosomal dominant congenital disorder with variable neurodevelopmental and craniofacial involvement. Craniofacial abnormalities include a distinct facial gestalt, delayed bone age, tooth abnormalities, delayed fontanelle closure, and frequently cleft or submucosal palate. Despite this, the dramatic phenotype and precise role of ANKRD11 in embryonic craniofacial development remain unexplored. Quantitative analysis of 3D images of KBG syndromic subjects shows an overall reduction in the size of the middle and lower face. Here, we report that mice with heterozygous deletion of Ankrd11 in neural crest cells (Ankrd11nchet) display a mild midfacial hypoplasia including reduced midfacial width and a persistent open fontanelle, both of which mirror KBG syndrome patient facial phenotypes. Mice with a homozygous Ankrd11 deletion in neural crest cells (Ankrd11ncko) die at birth. They show increased severity of several clinical manifestations described for KBG syndrome, such as cleft palate, retrognathia, midfacial hypoplasia, and reduced calvarial growth. At E14.5, Ankrd11 expression in the craniofacial complex is closely associated with developing bony structures, while expression at birth is markedly decreased. Conditional deletion of Ankrd11 leads to a reduction in ossification of midfacial bones, with several ossification centers failing to expand and/or fuse. Intramembranous bones show features of delayed maturation, with bone remodeling severely curtailed at birth. Palatal shelves remain hypoplastic at all developmental stages, with a local reduction in proliferation at E13.5. Our study identifies Ankrd11 as a critical regulator of intramembranous ossification and palate development and suggests that Ankrd11nchet and Ankrd11ncko mice may serve as pre-clinical models for KBG syndrome in humans.
Molecular studies in developmental biology have been instrumental in defining gene regulatory and signaling networks that control cell and tissue differentiation to a considerable extent. These studies have revealed that throughout tissue development, specific transcription factors or signaling molecules are often used in a reiterated fashion to elicit discrete, developmental stage-specific cellular responses. Epigenetic mechanisms, defined here as external modifications of chromatin, facilitate these stage-specific responses. Through control of global regulation of gene expression at the various developmental stages (Bannister and Kouzarides, 2011; Budhavarapu et al., 2013), they control cell identity and facilitate stage-specific molecular responses (Barrero et al., 2010; Mirabella et al., 2016). A fitting example for complex tissue development is craniofacial development, which involves the coordinated but often asynchronous development, growth, and maturation of initially independent bone and cartilage structures. Epigenetic control mechanisms help facilitate different bone-related processes: bone development, bone mass accumulation and growth, maintenance and remodeling, and bone loss at different stages (Strobl-Mazzulla et al., 2012; Hu et al., 2014; Marini et al., 2016). The complexity of chromatin regulation controlling DNA accessibility to transcriptional machinery during craniofacial morphogenesis is still poorly understood, although dysregulation of epigenetic mechanisms has been implicated in a number of musculoskeletal and rheumatic diseases (Jeffries and Sawalha, 2015).
The entry point for this study was the association of the chromatin regulator ANKRD11 (Ankyrin Repeat Domain 11; previously named ANCO-1) with KBG syndrome (OMIM #148050), a rare, autosomal dominant, congenital disorder characterized by a distinct craniofacial gestalt (Morel Swols and Tekin, 2018). ANKRD11 contains an Ankyrin domain consisting of five Ankyrin repeats, two repression domains, and one activation domain (Zhang et al., 2007), and regulates global gene expression (Gallagher et al., 2015) by interacting with a variety of histone acetylation modifying proteins, such as HDAC3 (histone deacetylase 3) (Zhang et al., 2004; Gallagher et al., 2015) and components of the P/CAF (p300/CBP-associated factor) acetyltransferase complex (Li et al., 2008). While the full-length ANKRD11 protein acts as a transcriptional repressor, the activation domain elicits transcriptional activation (Zhang et al., 2004, 2007; Li et al., 2008). Moreover, ANKRD11 interacts with and increases acetylation of p53, potentiating the ability of p53 to act as a transcription factor (Neilsen et al., 2008), which itself is involved in many aspects of craniofacial development (Bowen and Attardi, 2019).
KBG syndrome is associated with heterozygous mutations in ANKRD11 or micro-deletions of 16q24.3 encompassing ANKRD11. While patients with KBG syndrome display a range of phenotypes, a consistent feature is the distinct craniofacial gestalt (Morel Swols and Tekin, 2018). KBG syndrome diagnosis is typically suspected in an individual that displays macrodontia and/or characteristic facial appearance along with two additional criteria, such as palatal abnormalities, hearing loss, short stature, delayed bone age, scoliosis, learning difficulty, etc. (Morel Swols and Tekin, 2018). Delayed closure of a large anterior fontanelle is also frequently observed (Low et al., 2016).
The facial skeleton is mainly formed by neural crest cell-derived intramembranous bones, which develop from mesenchymal condensations at defined ossification centers (Berendsen and Olsen, 2015). During this process, osteochondroprogenitor cells sequentially differentiate into osteoblasts to form intramembranous bone. Osteoblasts deposit initially unmineralized osteoid that subsequently mineralizes (Berendsen and Olsen, 2015). As bone matures, osteoblasts become trapped within and terminally differentiate into osteocytes (St John et al., 2014). Maturation of osteocytes involves loss of organelles and changes in molecular properties (Irie et al., 2000). Newly formed bone matures through a process termed bone remodeling. This term describes the continuous process of bone resorption and bone formation that allows bone to attain its mature shape and optimal mechanical strength (Hadjidakis and Androulakis, 2006). These events require coordinated function of bone-resorbing osteoclasts, bone-depositing osteoblasts, and mature osteocytes. During this process, trabecular bone matures, and many osteocytes are resorbed.
Mineralization of the craniofacial complex begins in the mouse embryo around embryonic day 14 (Flaherty and Richtsmeier, 2018). While the involvement of epigenetics in the components of the ossification process is well documented (Schroeder et al., 2004; Li et al., 2009; Wein et al., 2015), there is little known about the overall contribution of chromatin regulators to craniofacial development.
Palate development describes the process by which the initially vertically growing maxillary appendages, termed palatal shelves, reorient to move above the tongue, grow horizontally and fuse to separate oral and nasal cavities (Kouskoura et al., 2011). The proliferation and differentiation of the palatal mesenchyme are regulated by intricate epithelial-mesenchymal interactions along the anterior-posterior axis. Palatal abnormalities, which occur when any of these required developmental events are disturbed, are relatively frequent and can present as complete, partial, or submucosal clefts. There is good evidence from the mouse for the involvement of epigenetic regulation during palate development (Kuriyama et al., 2008; Seelan et al., 2013; Juriloff et al., 2014) although direct evidence from humans is largely missing (Sharp et al., 2018).
Craniofacial and palate anomalies associated with KBG syndrome patients suggest a direct role for ANKRD11 during craniofacial development. A homozygous Ankrd11 missense mutation in mice is lethal in early embryonic stages (E9), preventing studies on craniofacial development with complete loss of Ankrd11 (Barbaric et al., 2008). As neural crest cells contribute significantly to development of the anterior craniofacial complex, we used a neural crest-specific Wnt1Cre2 Cre-lox mouse line (Lewis et al., 2013) to delete Ankrd11 in the developing neural crest.
We found that homozygous deletion of Ankrd11 in neural crest (conditional knockout; Ankrd11ncko) is perinatal lethal, while mice lacking one copy of Ankrd11 (conditional heterozygote; Ankrd11nchet) survive into adulthood. Adult heterozygous mice recapitulate some of the overall craniofacial phenotypes seen in KBG patients, whereas knockout embryos and pups display increased severity of numerous craniofacial anomalies commonly reported for patients with KBG syndrome (delayed ossification, cleft palate, midfacial hypoplasia, persistent anterior fontanelle, retrognathia). Our study identifies Ankrd11 as a critical regulator of intramembranous ossification and palate development and suggests that Ankrd11nchet and Ankrd11ncko mice may serve as novel pre-clinical models for KBG syndrome.
Animal experiments were approved by the Research Ethics Office at the University of Alberta (Animal Care and Use Committee, AUP1149, AUP2527) in compliance with guidelines set by the Canadian Council of Animal Care. Mice on a C57Bl/6 background were housed in the animal facility at the University of Alberta. Ankrd11TM 1a(EUCOMM)Wtsi//IcsOrl mice, in which exon 7 was floxed, were rederived from sperm (EM:07651, the European Mouse Mutant Archive – Infrafrontier) and crossed with Flp recombinase expressing (Jackson Labs) mice to generate Ankrd11TM 1c conditional ready mice (Ankrd11fl/fl) as described (Skarnes et al., 2011). Exon 7 is located within the Ankyrin repeat domain. Ankrd11fl/fl mice were crossed with B6.Cg-E2f1Tg(Wnt1-cre)2Sor/J (Jackson Labs) (Lewis et al., 2013) mice for neural crest-specific deletion of Ankrd11 (Ankrd11ncko). Deletion of exon 7 results in out-of-frame splicing of exon 6 to exon 8, leading to a pre-mature stop and truncation of Ankrd11. Genotyping of mice was performed using Taq DNA Polymerase 2x Master Mix RED (Ampliqon, Denmark) from ear-notch or tissue biopsies. The following primers and PCR conditions were used: (1) for Ankrd11: forward, 5′-CTGTCTCAGAGAGGAGAGTGAGGAGGAC-3′; reverse, 5′-TACCTTACACCCTGAGACGGCGTC-3′; 34 cycles of: 94°C-30 s, 62°C-45s, 72°C-60s; (2) for the Cre transgene: forward, 5′-TTCCCGCAGAACCTGAAGATG-3′; reverse, 5′-CCCCAGAAATGCCAGATTACG-3′; Twsg1 internal control forward, 5′-AACAACAATGGCACAACCTAAT-3′, Twsg1 reverse, 5′-ACTTTCTCCCCACCCGTCTA-3′; 35 cycles of: 94°C-15s, 60°C-30s, 72°C-90s.
Mice were imaged using a MILabs μCT scanner at the School of Dentistry at the University of Alberta. Heads were fixed in 4% paraformaldehyde (PFA) for 24 h, then scanned at the following parameters: voltage = 50 kV, current = 0.24 mA, exposure time = 75 ms, 1600 exposures/full rotation. Scans were reconstructed at a voxel size of 25–35 μm, and the volumes were exported as NifTI-1 files. NifTI-1 files were directly analyzed using Amira software (version 2019.2, Life Technologies). NifTI-1 files were batch-converted to MINC-2 format using a custom made bash script and the MINC toolkit (Vincent et al., 2016).
We acquired four three-dimensional (3D) scans of confirmed KBG syndrome patients from FaceBase (Samuels et al., 2020). All human subjects provided informed consent for the use of facial image data as approved by Institutional Review Boards at the University of Calgary, University of Colorado, Denver and the University of California, San Francisco. Each scan was non-linearly registered to a dense atlas (27,000 vertices) using the non-rigid iterative closest point method described in Bannister et al. (2020). We used a linear model with the first 400 principal components of facial shape to adjust the dense registered meshes for the effects of age and sex as described in Hallgrímsson et al. (2020). We then compared the mean age and sex-adjusted KBG mesh to the mean non-syndromic mesh. We visualized the differences between means as a heatmap using the meshDist() function in the Morpho package for R (Schlager, 2017).
We used the MINC toolkit (Vincent et al., 2016) to gather 3D coordinates of 68 anatomical landmarks, from x-ray micro-CT volumes of the cranium of Ankrd11 and control mice (Supplementary Figure 1). We also placed landmarks on a mouse skull atlas (average) and extracted a surface mesh of its segmented cranium, using marching cubes in VTK (Schroeder et al., 1998) and the MINC toolkit (Vincent et al., 2016).
We imported the specimen landmark files and the atlas landmark data into R (R Core Team, 2020) using the functions tag2array() and tag2lm(), respectively (Vidal-García, 2021). We performed a Generalized Procrustes Analysis (GPA) using the function gpagen() in geomorph (Adams et al., 2020), in order to obtain shape coordinates and remove effects due to size, rotations, and translations in the 3D landmark data. We then calculated group means on the shape coordinates for Ankrd11 and control specimens, using the function mshape() in geomorph (Adams et al., 2020).
We mapped these 3D shape coordinate means to the atlas mesh and landmark coordinates using the function tps3d() in Morpho (Schlager, 2017). This function uses a thin plate spline method (Bookstein, 1989) to interpolate a target set of landmarks to the reference landmark set (atlas), which then warps the atlas mesh to fit the target landmark data. We visualized morphological differences between the Ankrd11 and control mean meshes with heatmaps, using the function meshDist() in Morpho (Schlager, 2017).
Three-dimensional segmentation was performed using 3D slicer software. Automated segmentation function was used utilizing island and scissor functions to isolate individual bones. Bone volume was determined using statistics functions.
Embryos were dissected from uterus at embryonic days (E) 13.5, 14.5, and 15.5 and collected at birth at postnatal day (P) 0. Embryos were decapitated and heads were fixed overnight at 4°C in 4% PFA. P0 heads were decalcified in 0.5M EDTA overnight before processing. Heads were processed and sectioned as previously described (Baddam et al., 2020). Before further analysis, slides were warmed in a 60°C oven, deparaffinized with xylene, and rehydrated through graded ethanol washes.
Rehydrated slides were stained with hematoxylin & eosin (H&E) (Harris modified hematoxylin Fisher SH30, eosin Sigma E4382), Alcian blue (1% Alcian blue solution, pH 1, 0.1N hydrochloric acid rinse, nuclear fast red counterstain), picrosirius red (Direct red 80 Fisher B21693, picric acid ACPchem P-2095), or Tartrate-Resistant Acid Phosphatase (TRAP). Briefly, TRAP staining was performed by incubating slides in pre-warmed enzymatic TRAP Staining Solution containing Fast Red Violet LB salt (Sigma F-331) at 37°C for 40 min, or until the control slide is slightly overstained. Slides were then counterstained with 0.02% Fast Green (Sigma F-7252). After staining, slides were dehydrated, cleared with xylene, and mounted with Permount (Fisher SP-15).
Immunofluorescent staining was performed as described previously (Malik et al., 2020). Details of primary and secondary antibodies and dilutions used are summarized in Supplementary Table 1. Images were either taken on an Olympus IX73 microscope using 10x, 20x, or 40x objectives to photograph in a single plane and images were captured using the included cellSens Dimension program. Analysis was performed on at least three independent biological replicates; a representative image is shown. Polarized light images were acquired using a polarizer and analyzer cube (Olympus).
Density of cells forming the palatal shelves was quantified at E13.5 using DAPI nuclear stain (not shown). Semi-automated cell counting was performed in ImageJ on spatially defined regions within the palatal shelves (oral or nasal domains).
The number of immunostained Ki67-positive cells were counted on a minimum of six separate images per age and genotype from a palatal shelf. Sections were from a minimum of five different embryos from at least three different litters. The region counted is indicated in Figure 5 with white dotted lines. Counting was automated in ImageJ using thresholding, watershed and particle analysis functions. Values presented in the graph are number of Ki67 positive cells divided over DAPI positive cells to normalize for hypoplasticity of shelves. Quantification of Runx2 and Sp7 domains at E12.5/13.5 was performed similarly, with values reported as percent of a defined field of view occupied by the stain and/or number of positive cells within the field of view. At least 3 (E14.5) or 6 (P0) sections were analyzed from 3 to 5 mice per genotype from at least three different litters. Statistical significance was determined via an unpaired two-tailed t-test.
Primary osteoblast cultures were established from calvaria for the gene expression time-course experiment. Calvaria were dissected and cut into small fragments, discarding cranial suture tissue. Calvarial bones were dissected into HBSS (Sigma H-9394) and used for osteoblast isolation using a modified protocol (Bakker and Klein-Nulend, 2012; Perpétuo et al., 2019). HBSS was replaced with 0.25% trypsin for 10-min digestion at 37°C, washed in αMEM (Gibco 12561-056), and digested twice with 0.2% Collagenase Type II solution (Worthington 4176) for 30 min each. Final digestion product was collected. Remaining bone pieces were washed with αMEM – solution was added to final digest and bone pieces were rinsed three times with cCM (complete culture medium: αMEM supplemented with Penicillin-Streptomycin [Gibco 15240-062], ascorbate [100 μg/mL, Sigma, A-8960], 10% Fetal Bovine Serum [Sigma F-1051]), then transferred to 75 cm2 flasks containing cCM. Final digest was centrifuged for 5 min at 1500 g and the pellet was resuspended in 1 mL αMEM per calvarium. Cell suspension was combined with bone pieces in flask and incubated at 37°C and 5% CO2 in air until confluent. cCM media changed every 3 days.
Once confluent, cells were trypsinized and seeded in a 12-well plate at 5–10 × 104 cells per well. Osteogenic medium (αMEM supplemented with ascorbate [50 μg/mL] and 2 mM β-glycerophosphate [Sigma G-5422]) was added when cells reached 80–90% confluency. Medium was changed every 3 days. Cells from three wells were harvested using Trizol (Invitrogen 15596026) at days 0, 3, 6, 9, 12, 15 for mRNA isolation and RT-qPCR quantification. Three independent experiments with two mice each were performed with three wells/time-point/experiment. For mRNA isolation, wells from within an experiment were combined. A representative result is shown.
RNA extraction, cDNA synthesis, and quantitative RT-qPCR were performed as previously described (Malik et al., 2020) using appropriate primer pairs normalized to 36B4 as a reference gene (see Supplementary Table 2). Fold change was calculated using 2–ΔΔ Ct method (Livak and Schmittgen, 2001). MIQE guidelines were followed (Bustin et al., 2009).
Three-dimensional facial images from four genetically confirmed KBG syndrome patients were combined and mapped against a reference face (Figure 1A) (Hallgrímsson et al., 2020). The resulting mesh heatmaps revealed a consensus hypoplastic mid-and lower faces, with an expansion of the upper third of the craniofacial complex. The Ankrd11 neural crest-specific haploinsufficient deletion was generated by crossing Ankrd11 floxed mice with Wnt1Cre2+ mice (Ankrd11nchet) (Figure 1B). Analysis of μCT scans from mice Ankrd11nchet mice revealed distinct changes to several craniofacial structures (Figure 1C): a persistent open fontanelle, a unique ossification defect in the posterior frontal suture, a slight change in pterygoid bone morphology evident from coronal ortho-slices anterior to the coronal suture (Figure 1C, lower panels). The angle of medial aspect of the pterygoid bone relative to ventrolateral was altered in Ankrd11nchet mice. Comparative mesh heatmaps of Ankrd11nchet and control scans revealed growth alterations in comparable regions as described for the human faces: reduced facial width, hypoplasia of midface, nasal region, and an expanded cranial vault (Figure 1D). Note: mandibles were omitted from analysis because of their variable position with respect to the skull.
Figure 1. KBG syndrome patients and Ankrd11nchet mice share craniofacial similarities. (A) Mesh morphometric analysis of 4 KBG syndrome patient 3D images. Images shown are a mean representation of craniofacial changes on a reference face. Heat map representation in Procrustes distance (relative to superimposition). Red color indicates positive change in size relative to reference mesh and blue indicates negative size difference. White color indicates no change in highlighted region. (B) Brief summary of gene targeting and breeding strategy to produce Ankrd11nchet mice, shown in panels (C,D), and Ankrd11ncko mice. (C) Reconstructions of μCT scans of Ankrd11ctrl (Ankrd11wt/fl) and Ankrd11nchet mice. Top panel: lateral view of whole skull. Middle panel: Superior view of frontal bone; mandible and cranial base are clipped out of plane. Red dotted line indicates plane of sectioning for lower row of panels. Yellow dotted line highlights calvarial defect surrounding posterior frontal suture in Ankrd11nchet mice. Yellow arrows point to regions of hard tissue anomalies in Ankrd11nchet frontal bone. Lower panels: orthogonal slice through posterior frontal suture indicating pterygoid abnormalities (orange arrow) and open posterior frontal suture (yellow arrow). Inset in lower right corner of images is high contrast representation of cranial base structures affected in image. pb, parietal bone; fb, frontal bone; nb, nasal bone; post. fs, posterior frontal suture. (D) Mesh morphometric analysis of three Ankrd11nchet mouse skulls. Images shown are a mean mesh of three Ankrd11nchet skulls relative to a mean mesh of five Ankrd11ctrl skulls. Note: mandibles were excluded from analysis due to the variable position with respect to the skull. Colors depict Procrustes distances between Ankrd11ctrl and Ankrd11nchet mean meshes, with blue indicating negative values (Ankrd11nchet values fall within Ankrd11ctrl mesh) and red indicating positive values (Ankrd11nchet values fall outside of Ankrd11ctrl mesh). White values indicate no changes, meaning the vertices for these anatomical regions in both mice are very close.
While pups lacking both copies of Ankrd11 in the neural crest (Ankrd11ncko) died at birth, precluding our analysis at the postnatal and adult stage, visual inspection of the neonatal head at postnatal day 0 (P0) revealed partially open eyelids, variable midfacial hypoplasia, reduction of calvarial growth (evident as reduction in calvarial microvasculature), loss of pigment on the nose, fully penetrant cleft palate, and a smaller tongue relative to control mice (Supplementary Figure 2).
Skeletal preparations of P0 neonates demonstrated an underdeveloped midface and severe micrognathia in Ankrd11ncko mice when compared to littermate controls (Figure 2A). A cleft palate was evident, as was the reduced ossification of the palatine bones and the anterior cranial base (presphenoid and sphenoid) (Figure 2A). Micro-computed tomography (μCT) showed that all intramembranously formed orofacial bones were reduced in size, resulting in underdeveloped midface and mandible (Figure 2B). Ossification of the anterior cranial bones was similarly severely stunted, and bones failed to cover the majority of the cranium at birth when compared to control mice (Figure 2B). Ossification of the anterior cranial base (presphenoid and basisphenoid) was similarly reduced. Several of the primary ossification centers failed to expand and formation of the pterygoid wings was largely missing. In contrast, more posterior, mesoderm-derived components of the cranial base (basioccipital bone) appeared unaffected. Quantification of the volumes of isolated craniofacial bones confirmed 39% reduction of the mandible (p < 0.001), 83% reduction of the frontal bone (p < 0.001), and 76% reduction of parietal bone (p < 0.05), with interparietal, occipital and basioccipital bones being not significantly different (Figure 2C).
Figure 2. Ankrd11ncko mice have compromised ossification in craniofacial structures at birth. (A) Wholemount images of postnatal day 0 (P0) skull skeletal preparations, stained with Alizarin red and Alcian blue. Left panels: Orange dotted lines outline Ankrd11ctrl (Ankrd11fl/fl) mandible to indicate micrognathia in the mutant. Middle left panels: Red dotted lines outline Ankrd11ctrl (Ankrd11fl/fl) frontal bone to indicate its smaller size in the mutant. Middle right panels: Yellow arrows indicate extra space between presphenoid and palatine bones of the cranial base. Yellow asterisk to the right highlights clefting of hard palate in Ankrd11ncko mouse. Right panels: magnified view of palate. bs, basisphenoid bone; ps, presphenoid bone; pb, palatine bone. Scale bar represents 2 mm, third column represents 1 mm. (B) μCT scans of P0 Ankrd11ncko and Ankrd11ctrl skulls reconstructed in Amira. Blue dotted lines: Ankrd11ctrl skull outline. Green dotted lines: control frontal bone outline. Tan dotted fill in Ankrd11ncko: negative space between control mouse and mutant. Red dotted lines: control frontal bone outline. Yellow dotted lines: control basisphenoid and presphenoid hard tissue outline. White asterisk indicates reduction in palatine bone ossification in the mutant mouse. (C) representative segmentation of individual bones from μCT scans of P0 Ankrd11ncko and Ankrd11ctrl skulls. (D) Quantification of segmented bone volumes (mm3) from Ankrd11ncko and Ankrd11ctrl pups (independent two-sample t-test assuming unequal variances, **p < 0.001, *p < 0.05, n = 6). Segmentation and volume analysis performed using 3D Slicer.
Histological analysis confirmed the notable size difference of the head and the cleft palate phenotype (Figure 3A). All major structures were present and developed to a recognizable stage, with exception of the tongue and palatal shelves that remained hypoplastic (Figure 3A). Note that due to retrognathia, mandibular structures on the sections appear more anterior. The developing palatal shelves were hypoplastic and dysmorphic already at embryonic day 12.5 (E12.5) (Figure 3B). Hypoplasia of the tip of the palatal shelf remained evident at later developmental stages. Elevation of palatal shelves appeared normal, but hypoplastic shelves did not meet to fuse, resulting in failure to separate oral and nasal cavities (Figure 3B). Analysis of cell density in the oral and nasal domains of the palatal shelves at E13.5 revealed a 15% increase in cell density in the nasal domain only (p < 0.05), with no differences observed in the oral domain (Figure 3C).
Figure 3. Ankrd11ncko mice display craniofacial changes early in development. Hematoxylin and eosin (H&E) stain. (A) Representative images of P0 coronal sections of Ankrd11ctrl (top) and Ankrd11ncko (bottom) skulls. Note relative size difference between heads. Black asterisk indicates absence of complete palatine boundary separating oral and nasal cavities in Ankrd11ncko image. Second column images demonstrate overall differences in oral cavity, including smaller tongue and cleft palate. Differences in plane of section in mandible are due to retrognathia in Ankrd11ncko mice. Right panels focus on palatal shelves, with red arrow indicating unfused advancing medial edge of palatal shelf in mutant mouse. t, tongue; ps, palatal shelf; *cleft palate. Scale bar indicates: 1 mm (first column), 500 μm (second column), and 250 μm (third column). (B) Coronal sections of palatal shelves at E12.5, E13.5, and E14.5. Inset in lower right corner of each view is lower magnification image of same mouse, showing overall organization of oral cavity in each mouse. n = 3 from 3 litters. Scale bar represents 50 μm. (C) Analysis of cell density based on DAPI stain in E13.5 palatal shelves separated into oral and nasal domains. Left: representative H&E stain. Middle: ImageJ-generated density profile showing individual cells. Right: Statistical representation of analysis showing increased cell density in the nasal domain of Ankrd11ncko palatal shelves (independent two-sample T-test assuming unequal variances, *p < 0.05). n.d., nasal domain; o.d., oral domain.
To gauge when and how Ankrd11 could cause the above-described phenotypes, we performed expression analysis for Ankrd11 at E13.5, E14.5, and P0 (Figure 4A). At E13.5, Ankrd11 expression was discrete in orofacial regions with clear expression in a mesenchymal condensation in the developing maxillary region (sphenoid wing). Some expression was noted in the developing palatal shelves and lining oral epithelium. Note the distinct expression in the developing forebrain at this stage. At E14.5, Ankrd11 expression was overall stronger, more widely expressed, and could be observed in several developing mesenchymal structures. Distinct expression was observed in ossification centers and at sites of bone development in the mandible and maxilla, as well as in pre-odontoblasts in the developing molars. Ankrd11 was also present in some cartilaginous structures (nasal septum, developing turbinates), parts of the tongue, and the midline epithelial seam of the fusing palate. In the eye, expression was prominent in the developing lens as well as in the anterior segment (Figure 4A). Extent and intensity of Ankrd11 expression waned by P0, with the majority of Ankrd11 at this point restricted to the oral epithelium. Expression in the mandibular and maxillary bones was strongly reduced or absent, as was expression in teeth. In the eye, expression was now restricted to the posterior segment (Figure 4A). This indicates dynamic, but discrete, expression of Ankrd11 throughout development of craniofacial structures.
Figure 4. Ankrd11 is expressed in embryonic craniofacial tissues. Expression pattern of Ankrd11 on immunofluorescent staining of Ankrd11ctrl coronal paraffin sections. (A) Split view of coronal sections of C57/BL6 mice immunostained for Ankrd11 (left, green) or processed for H&E for morphological orientation (purple, right) at ages E13.5, E14.5 and P0. ns, nasal septum; mand, mandible; m, molar; mc, Meckel’s cartilage; olf bulb, olfactory bulb of brain; calv, calvaria. White dotted lines on P0 immunofluorescent panel outline bony structures of the craniofacial complex including calvaria, maxillary/palatine bones, and mandibular bone shown in (B). Scale bars represent 200 μm (left) and 500 μm (right). (B,C) High magnification representative images from coronal sections immunostained for Ankrd11 (red), with DAPI nuclear counterstain in blue from mandible and calvaria (B) as well as palatal shelves (C). White dotted lines in (B) (mandible panels) indicate boundaries of mandibular bone indicated in (A). Cranial/calvarial images are taken from comparable anatomic locations indicated in (A). Images in (B,C) are presented from E14.5 and P0. Images in (C) are presented from E12.5, E13.5, E14.5, and P0. Graphic representation of Ankrd11 expression at E12.5 and E14.5 in right panels created in Adobe Illustrator. Blue represents Ankrd11 expression, with larger dots indicating relatively brighter regions. n = 3 from 3 litters. Scale bars in (B) represent 50 μm, except for E14.5 calvaria (200 μm). Scale bars in (C) represent 100 μm. ps, palatal shelf; t, tongue; tb, tooth bud; nc, nasal cavity; oc, oral cavity; es, epithelial seam; max, developing maxillary bone; m(m), molar (mesenchymal portion); m (e), molar (epithelial portion).
This dynamic expression is nicely illustrated in the developing mandibular bone (Figure 4B), one of the earliest bones to mature in the craniofacial complex (Flaherty and Richtsmeier, 2018). While at E14.5, at the onset of ossification, Ankrd11 signal lined the forming mandibular bone, expression was negligible in the comparable region at P0 (Figure 4B). Similarly, in the cranial vault, Ankrd11 was localized to the forming ossification centers at E14.5, whereas at P0 weak expression was observed in cells lining the calvarial bone, but not in osteocytes within the mature bone (Figure 4B).
In comparison, Ankrd11 expression in the developing palatal shelves and the developing maxilla was more discrete. At E12.5, a mostly epithelial expression pattern was observed in palatal shelves (Figure 4C). A few Ankrd11-positive mesenchymal cells were evident in the buccal aspect toward its tip, as well as in its upper, dorsal aspects (Figure 4C). At E13.5 and E14.5, the epithelial and mesenchymal expression largely remained comparable to E12.5. Overall, at E14.5, Ankrd11 expression was strongest in epithelium of the newly forming nasal cavity, the oral epithelium, the developing maxillary bones, as well as in the fusing palatal shelf, restricted to the midline epithelial seam. With the exception of some epithelial structures, Ankrd11 expression at P0 was reduced in all of these places. Residual weak expression in presumptive bone-lining cells was noted (Figure 4C).
To assess the cause for the hypoplastic palatal shelves in Ankrd11ncko embryos, we assessed apoptosis and proliferation. No significant apoptosis was observed at any of the time points between E12.5–E13.5 (Supplementary Figure 3). The overall ratio of Ki67-positive cells to DAPI-positive nuclei was comparable at E12.5 and E13.5 in Ankrd11ncko palatal shelves when compared to Ankrd11ctrl (Figures 5A,B). When shelves were separated into oral and nasal domains and a spatially restricted quantitative analysis was performed (Figure 5A, right panels), a 40% decrease in mesenchymal proliferation became obvious in the E13.5 oral domain (n ≥ 5 each, p < 0.05) (Figure 5B). To test for changes to cellular and extracellular organization, E13.5 palatal sections were stained with Alcian blue to identify distribution of glycosaminoglycans (GAGs). The overall organization and direction of cell orientation differs between Ankrd11ncko and control shelves (Figure 5C). In addition, a disorganization of the mesenchymal cells lining the shelf epithelium was noticed (Figure 5C). These findings indicate that loss of Ankrd11 has multiple subtle effects on palatal shelf organization and maturation, which likely underlie the cleft palate phenotype.
Figure 5. Palatal shelf proliferation is locally affected in Ankrd11ncko embryos. (A) Representative images of palatal shelves at each age and timepoint immunostained for Ki67 (red). DAPI-labeled nuclei are in blue. White dotted line delineates region counted for quantification shown in (B). White scale bars represent 50 μm. Right panels: Magnification of E13.5 palatal shelf showing heatmap of Ki67 expression using ImageJ. White dotted line separates the nasal and oral domains of the palatal shelf. Scale bars represent 50 μm. (B) Left panel: Quantification of Ki67-positive cells relative to DAPI-positive nuclei plotted for each genotype (Ankrd11ctrl depicted in black and Ankrd11ncko depicted in red at E12.5 and E13.5). Middle and right panel: Cell proliferation in nasal and oral domains respectively (independent two-sample T-test assuming unequal variances, minimum of six sections/genotype from minimum of five embryos per genotype, *p < 0.05, n = 6). (C) Alcian blue/nuclear fast red staining of E13.5 palatal shelves. Red arrow indicates mesenchymal cells lining epithelium on buccal side of shelf. n = 3/genotype, representative images shown. Scale bars represent 50 μm.
To better understand the reduction in intramembranous ossification observed in Figure 2, protein expression of two master regulators of ossification, Runx2 and Sp7 (Hojo et al., 2016; Komori, 2017) was assessed within the field of view indicated in Figure 6A. At E14.5, expression of Runx2 and Sp7 quite homogenously outlined the growing maxillary bones in control embryos (Figure 6A, top row). In contrast, in Ankrd11ncko mutant embryos, Runx2 and Sp7 expression was only strong in the center, becoming more diffuse toward the periphery. Sp7 expression was more contained in comparison to Runx2 (Figure 6A). Quantification of the respective expression domains indicated that expression of both Runx2 and Sp7 was more contained, however the differences in the area occupied did not reach statistical significance (Figure 6B).
Figure 6. Bone formation in Ankrd11ncko mice is dysregulated in late embryonic development. (A) Palatal region in E14.5 Ankrd11ctrl (upper row) and Ankrd11ncko (lower row) embryos H&E stained for orientation (left column) and immunostained for runt-related transcription factor 2 (Runx2) and Osterix (Sp7) (middle and right columns). Scale bars indicate 100 μm. (B) Quantification of % occupancy of field of view stained for Runx2 and Sp7 (independent two-sample T-test assuming unequal variances). (C) Low and high magnification images of palatal bone in P0 Ankrd11ctrl (upper row) and Ankrd11ncko (lower row) pups immunostained for Runx2 (left two columns) and Sp7 (right two columns). DAPI (blue) was used to counterstain nuclei. (D) Representation of individual cells expressing Runx2 and Sp7 generated using ImageJ showing altered cell distribution in the mutant. Red dotted line and arrowhead indicates extended trabeculae in control, whereas red circles and yellow arrowheads indicate more contained cellular clusters in the mutant. Scale bars in first and third columns represent 100 μm, and second and fourth represent 50 μm. (E) Quantification of Runx2 and Sp7 expression at P0 (left: number of positive cells; right: percent area per field of view) showing significant reduction of the area of Runx2 expression, whereas Sp7 expression domain was more contained but not statistically different at P0 (n = minimum of six sections form 3–5 pups/genotype, independent two-sample T-test assuming unequal variances, **p < 0.001).
Differences in expression became even more striking at P0, a time-point of significant maxillary bone growth and remodeling. Expression of Runx2 and Sp7 could be observed in osteoblasts lining the newly formed trabeculae in control neonates (Figure 6C, top row). In Ankrd11ncko, this trabecular pattern appeared disturbed. Expression of both Runx2 and Sp7 appeared patchy and locally more restricted to the small, sparse trabeculae in maxillary bone (Figure 6C, bottom row). Tracing the outlines of cells expressing Runx2 and Sp7 revealed the presence of extended, Runx2-positive cellular assemblies reminiscent of developing trabecula (Figure 6D, red dotted line), while the cellular structures in the mutant were more self-contained (Figure 6D, yellow arrowheads). Quantification indicated an 85% reduction of the Runx2-expression domain (p < 0.001) as well as 76% reduction in the number of Runx2-positive cells (p < 0.001). In contrast, despite a trend toward lower values, neither the number nor area of Sp7-positive cells were significantly different (Figure 6E). To test whether Ankrd11 is indeed expressed and changes during osteoblast differentiation, we performed an in vitro time course of calvarial osteoblast differentiation. RT-qPCR analysis revealed that expression of Ankrd11 changes dynamically over the course of osteogenic differentiation (Figure 7A). For reference, expression of several markers of osteoblast differentiation is shown: osteocalcin (Ocn), integrin binding sialoprotein (Ibsp), Osteopontin (Opn), alkaline phosphatase 1 (Alp1). This co-expression was mirrored in vivo, where Ankrd11, albeit more discrete, largely aligned with expression of Sp7 at E14.5, but not at P0 (Figure 7B).
Figure 7. Ankrd11 is upregulated with bone induction. (A) RT-qPCR analysis of Ankrd11, Ocn, Ibsp, Opn, and Alp1 mRNA expression during in vitro osteogenic differentiation of P6 calvarial osteoblast precursor cells from wild-type mice. Data was normalized to 36B4 housekeeping gene. Results are relative to d15 expression from four independent biological replicates with d15 values equated to 100%. (B) Low magnification of approximate location (blue box, H&E stain) of panels showing immunostaining for Osterix (Sp7, red, top row) and Ankrd11 (red, bottom row) in E14.5 and P0 Ankrd11ctrl mice. Images indicate similar expression domains of Sp7 and Ankrd11 at embryonic stages with expression pattern varying at birth. DAPI (blue) was used to counterstain nuclei. n = 3. D, days; E, embryonic; P, postnatal.
The altered appearance of maxillary bones in Figure 7 prompted us to analyze maxilla, mandible, and calvaria trabeculae in more detail. In control mice, discrete trabeculae with widely interspaced osteocytes of mostly elongated appearance were readily identified (Figure 8A, top row). In contrast, trabeculae appeared less refined with a higher number of poorly aligned osteocytes evident in all bones from Ankrd11ncko mice (Figure 8A, bottom row). Staining for the Wnt antagonist Sclerostin (Sost), a marker for mature osteocytes and an important regulator of bone remodeling (Winkler et al., 2003; Husain and Jeffries, 2017), revealed a strong reduction in Ankrd11ncko mice (Figure 8A, right column), suggesting that osteocyte maturation is delayed and bone remodeling might be compromised. To better illustrate this, we stained coronal sections of the craniofacial complex with Picrosirius red to reveal collagen fiber networks and osteoclast-specific tartrate resistant alkaline phosphatase (TRAP). An overall reduction in trabeculation was seen in the maxilla, calvaria (Figure 8B), and mandible (Figure 8C). This was mirrored by a 99% reduction in TRAP-positive regions in the maxilla, 85% reduction in the mandible, and 99.5% reduction in the calvaria (Figure 8D bottom graph; p < 0.05) despite presence of comparable amounts of bone within the region of interest (Figure 8D top graph; n.s.). In the calvaria (Figure 8B, bottom panels), a single-layered bone was observed in Ankrd11ncko mice indicative of reduced or defective bone remodeling. TRAP staining revealed an almost complete lack of bone resorption and, by extension, remodeling. Birefringent imaging under polarized light of picrosirius red-stained sections confirmed changes in trabeculation, interconnectivity, and crosslinking of collagen fibrils (Figure 8E). Thus, Ankrd11 appears to be comparatively more important in bone growth and remodeling than the initial induction of intramembranous bone, despite its prominent expression in ossification centers.
Figure 8. Bone maturation is delayed in Ankrd11ncko mice. (A) Representative sections of maxillary, mandibular, and calvarial bone from Ankrd11ctrl (top row) and Ankrd11ncko (bottom row) mice at P0. Sections were stained simultaneously for H&E to reveal cell morphology and bone organization. Images were taken at anatomically matching locations. Images in right column depict sections immunostained for sclerostin (Sost, red) in the P0 maxillary bone, demonstrating a reduction in Sost+ cells in Ankrd11ncko maxillary bone. DAPI (blue) was used to counterstain nuclei. Scale bars in first two columns represent 25 μm, and last two represent 50 μm. (B) Sirius red and tartrate-resistant acid phosphatase (TRAP) staining of maxilla and calvaria of Ankrd11ctrl and Ankrd11ncko mice at P0 on neighboring sections. Red dotted line outlines region of bone in Ankrd11ncko maxilla with no TRAP-positive cells, indicating a reduction in remodeling. Calvarial images are taken at the same point midway between the eye and fontanelle. Scale bars represent 100 μm. (C) Sirius red and TRAP staining of the mandible in Ankrd11ctrl and Ankrd11ncko mice at P0 showing a decrease in TRAP-positive cells in the mandibular bone. Scale bars represent 100 μm. (D) Quantification of images shown in (B,C) (independent two-sample T-test assuming unequal variances, *p < 0.05, n = 5). (E) Polarized light images of sirius red stained maxilla, calvaria, and mandible in control and Ankrd11ncko mice at P0 showing differences in extent of collagen fibril interconnection and orientation. Scale bars represent 50 μm.
Neural crest-specific deletion of Ankrd11 reveals direct roles for Ankrd11 in various aspects of craniofacial development. Our study focused on intramembranous bone formation of facial bones and the skull, as well as palate formation, as these structures are commonly affected in patients with KBG syndrome. Similarly to KBG syndrome, adult Ankrd11nchet mice present with discrete phenotypic changes that match the descriptions of ‘triangular’ face, persistent anterior fontanelle, and palatal abnormalities in humans. These observations complement the phenotypic description of heterozygous “Yoda” mice, in which an ENU-induced point-mutation in the C-terminus of Ankrd11 leads to a shorter and wider face, decreased bone mineral density, incomplete closure of the interfrontal suture, and persistent opening of the anterior fontanelle (Barbaric et al., 2008). While both heterozygous Yoda and Ankrd11nchet mutants are valuable to relate effects of Ankrd11 mutations on development, they do not allow for attribution of systemic consequences and overall developmental delay to discrete roles of Ankrd11. In contrast, relating phenotypic consequences of homozygous, cell-specific deletion of Ankrd11 to cellular expression of Ankrd11 elucidates the involvement of Ankrd11 in a precise and applicable manner. In this study, we focused on neural crest and craniofacial development. Ankrd11ncko neonates exhibit a triangular shape face, cleft palate, midfacial hypoplasia, retrognathia, bone defects including an expanded anterior fontanelle, hypoplastic frontal bones, and a delay in bone maturation. Ankrd11ncko die at birth, which underscores the overall importance of Ankrd11 in neural crest-derived structures. Our findings on intramembranous ossification and palate development indicate that Ankrd11 controls neural crest-derived cell progenitor proliferation or differentiation in a spatio-temporal manner, without affecting apoptosis. These data support and extend previous reports, where knockdown of Ankrd11 in neural precursors decreases their proliferation and resulting number of differentiated neurons during murine cortical development (Gallagher et al., 2015). Our findings that Ankrd11 impinges on intramembranous bone formation and maturation allow comparison to 3D morphometric analysis in both mice and humans to make precise conclusions on functional impact. Thus, the homozygous deletion of Ankrd11 in a tissue-specific manner provides a powerful approach to model the KBG syndrome phenotypes in mice. Furthermore, this approach highlights how discrete perturbations in chromatin and epigenetic control can compromise tissue development and function long-term.
Neural crest-specific deletion reveals the broad involvement of Ankrd11 during craniofacial development. In line with this, Ankrd11 shows restricted and dynamic expression in many craniofacial tissues. At E13.5 and E14.5, Ankrd11 is localized to discrete parts of the eye, bones, teeth, cartilage, and several muscular components. At a later developmental stage, expression in these regions is comparatively low and is primarily restricted to oral epithelium. Some expression in muscular compartments and olfactory bulb was noted in line with Gallagher et al. (2015). Relating expression to phenotypic manifestations allows strong predictions on the involvement of Ankrd11 in the development of these different structures. For instance, Ankrd11 expression is noted from E13.5 onward in mesenchymal condensations and at E14.5 in ossification centers. This implies that the bony defects observed in Ankrd11ncko mice might precipitate from differences during bone induction, although some of the defects such as osteocyte maturation and remodeling manifest only at much later stages. A similar pattern is seen in the maxillary and calvarial bones, where Ankrd11 is strongly expressed early in development, yet the impact on bone maturation and remodeling is noted only in much later stages when Ankrd11 expression is markedly reduced. It was previously suggested that development of bone involves epigenetic mechanisms, which might in part be controlled by lineage-specific transcription factors such as Sp7/Osterix (Park-Min, 2017). Whereas induction of ossification centers appears not to be compromised, significant differences are observed with respect to growth and maturation of these ossification centers. Expression domains of Runx2 and Sp7 are differentially affected. Thus, our study illustrates how expression of these two master genes are variably affected by loss of Ankrd11. At present, it is unclear if the weak expression of Ankrd11 seen in more mature bone indicates a continuing requirement for Ankrd11 to maintain established epigenetic signatures, or whether it indicates ongoing, not-yet-understood de novo genomic fine-tuning.
Both 3D morphological as well as phenotypic analysis indicate that Ankrd11 is particularly important for intramembranous ossification. Establishment of ossification centers appears not to be compromised in the Ankrd11ncko embryos; however, they universally fail to fully expand. This was not due to changes in proliferation or apoptosis (Supplementary Figure 3) but rather appeared to be the consequence of other, not fully understood mechanisms affecting specification, maturation, cell orientation, or migration of the induced bone-forming cells. In this light, Yoda mice or mice with Ankrd11 knockdown in cortical progenitors display aberrant positioning of neurons in the developing cortex linked to dysregulated epigenetic mechanisms (Gallagher et al., 2015). Together with our craniofacial data, this could underscore that control of cell migration may be a common mechanism.
Intramembranous calvarial bone development generally occurs in two stages: (1) development of bone primordia from mesenchymal condensations, e.g. anterior-superior of eye for the frontal bone (beginning at E12.5), and (2) expansion, e.g. of the frontal bone front to cover the top of the skull (Yoshida et al., 2008). Defects in either or both of these stages result in insufficient bone formation. Our results suggest that loss of Ankrd11 predominantly affects extension of the bone via growth at the osteogenic front and bone remodeling. The frontal bone insufficiency seen in Ankrd11ncko mice mirrors the phenotype observed in the Beetlejuice mouse, which carries a mutation in Prickle1 (Prickle1bj/bj) (Wan et al., 2018). Prickle1bj/bj mice also have hypoplastic frontal bones, an expanded anterior fontanelle, and frequently cleft palate. The Prickle1 mutation is thought to affect a Wnt/Planar cell polarity (Wnt/PCP) signaling pathway. Apoptosis and proliferation are not affected, but unlike the Ankrd11 mutant, a notable decrease in Sp7 alongside minimal changes to Runx2 expression is observed (Wan et al., 2018). Mutations in core components of the Wnt/PCP pathway cause Robinow syndrome which, like KBG syndrome, results in a wider midface and shorter stature. Notwithstanding these phenotypic similarities, changes in Ankrd11ncko mice are likely caused by inappropriate epigenetic regulation in differentiating osteoblasts. Histones associated with several gene loci for key osteogenic factors including Runx2, Sp7, and Alp undergo dynamic acetylation changes throughout the ossification process (Zhang et al., 2015). Of interest, Runx2 interacts directly with HDAC3, a histone deacetylase previously shown to directly interact with Ankrd11 (Schroeder et al., 2004; Zhang et al., 2004; Gallagher et al., 2015). It is thus possible that Ankrd11 and Runx2 participate in common gene regulatory networks to set up osteoblast differentiation during craniofacial bone development. Potential changes in epigenetic signatures were not investigated as part of this study, because craniofacial structures are too heterogeneous following different developmental kinetics, posing significant challenges for the analysis of subtle epigenetic changes.
Bone remodeling begins soon after bone formation. In Ankrd11ncko mice, bone remodeling was severely compromised. The only notable remodeling occurred in the mandible, but even there it was dramatically reduced. Osteocytes appeared to retain an immature phenotype, evidenced by their increased numbers, plump morphology, apparent failure to align along stress/force lines, and lack of Sclerostin (Sost) expression, a characteristic of immature osteocytes. This could be the consequence of a delay in bone formation or an intrinsic defect in osteocyte differentiation. As Ankrd11 is not expressed in osteocytes (Figure 4B), this phenotype is likely caused by abnormal osteoblast differentiation, possibly involving inappropriate epigenetic osteoblast programming or specification as discussed earlier.
Immature osteocytes lack the exquisite mechanosensory properties of mature osteocytes. The primary cilium has been suggested to play an important role in bone mechanotransduction (Temiyasathit and Jacobs, 2010). Inability to sense or respond to mechanical cues would manifest as failure to align along stress lines, increased cell number, and failure to induce bone remodeling (exemplified by loss of TRAP staining). On the other hand, osteocyte maturation is dependent on mineralization, as the transition to mature osteocytes is triggered by differences in mechanosensing of mineralized and unmineralized matrix (Irie et al., 2008). Changes to ordered mineralization in Ankrd11ncko bones could equally explain the failure in osteocyte maturation and associated bone remodeling. Osteoclasts are related to monocytes and are of hematopoietic origin. Neural crest-specific Ankrd11 deletion would not directly affect these cells. The defect in remodeling is therefore most likely due to defective mechanosensing or defective communication with osteoclasts, underlining interconnection of these systems.
While lack of Sost expression could reflect a failure of osteocyte maturation, it must be noted that Sost itself is under epigenetic control. HDAC5 deficiency results in increased Sost expression, impaired osteogenesis, and low bone density (Wein et al., 2015). Sost activity-blocking antibodies are an approved treatment modality for osteoporosis aiming to reduce bone resorption. A more detailed analysis will be required to carefully dissect the cause and consequence of these bone-related phenotypes.
In contrast to bone primordia, the pattern of Ankrd11 expression in the palate is relatively discrete. It also differs from the often broad expression domains described for many transcription factors and signaling molecules in the developing palatal shelves (He et al., 2011; Danescu et al., 2015). This restricted Ankrd11 expression was seen at all stages from E12.5-E14.5 and could be attributed to discrete cells organizing palate development, or a tight window of expression governing reprogramming. Thus, Ankrd11 might be involved in the spatial organization of the palatal shelf, possibly regulating the expression of a growth factor or its receptors, facilitating growth of the palatal shelf secondarily. Indeed, changes in cellular distribution were observed.
Furthermore, an asymmetric pattern of proliferation was observed at E13.5, with a decrease in the buccal half of the shelf. The affected region roughly corresponds to the gene expression domain of the Hedgehog signaling receptor Patched (Ptch) (Xiong et al., 2009), raising the possibility that Ankrd11 somehow intersects with Hh signaling and cilia. Hedgehog and Wnt signaling are indeed both critical for cilia maturation, and functional cilia are required for normal palatal development (Brugmann et al., 2010; Nakaniwa et al., 2019). Recently, Ankrd11 was directly associated with ciliopathies (Breslow et al., 2018). The interplay between Hedgehog and Wnt signaling and chromatin remodeling by Ankrd11 may provide important clues for understanding both palate and bone phenotypes and will be investigated in future studies.
Mesh analysis of four patients with KBG syndrome revealed hypoplastic mid- and lower face and enlarged upper face. This is largely mimicked in Ankrd11nchet and Ankrd11ncko mice (Figures 1, 2). Given the stunted intramembranous ossification in the Ankrd11ncko mouse, we propose that the expansion of the upper 1/3 of the face is a consequence of underdeveloped midfacial bones as well as intramembranously formed extensions of the cranial base – the pterygoid wings. The resultant narrower cranial base and midface would necessitate increased cranial bone growth to accommodate the expanding brain, leading to a triangular face. The calvaria would not be able to meet the increased demand in bone growth (as they are compromised in bone formation themselves) resulting in a persistent anterior fontanelle.
Patients with KBG syndrome show various accompanying craniofacial anomalies. Macrodontia, dental crowding, and hypo/oligodontia are often observed, necessitating invasive jaw surgery (Ockeloen et al., 2015; Low et al., 2016; Morel Swols and Tekin, 2018). Our results and expression analysis suggest that these malformations may be due to abnormal tooth and jaw development. Moreover, speech and feeding difficulties are frequently observed in KBG syndrome patients (Ockeloen et al., 2015; Low et al., 2016; Morel Swols and Tekin, 2018). The anomalies in palate development, more severe in Ankrd11ncko mice but evident also in Ankrd11nchet mice, can provide a structural explanation for these observations. High-arched palate or submucosal cleft, considered milder variants of defective palate development, lead to similar problems. Thus, our results will precipitate further investigations into the underpinnings of the various phenotypic craniofacial anomalies associated with ANKRD11.
ANKRD11 is one of the most disrupted genes in monogenic neurodevelopmental disorders with de novo mutations (Wilfert et al., 2017; Satterstrom et al., 2020). Yet, most patients with KBG syndrome are not diagnosed until 20–30 years of age, if at all (personal communication with Drs. Charlotte Ockeloen, Tjitske Kleefstra, and Peter Kannu). The gold standard for diagnosis of KBG syndrome is genetic testing to detect ANKRD11 variants or 16q24.3 deletion involving the gene. Specific craniofacial features such as persistent anterior fontanelle, submucosal or high-arched palate, macrodontia, and reduced bone mineral density are currently only suggestive of KBG syndrome and clinical phenotypic criteria for proper diagnosis remain poorly defined (Morel Swols and Tekin, 2018). This study confirms the power of 3D morphometric analysis to assist with syndrome diagnosis (Hallgrímsson et al., 2020) and illustrates the power of disease modeling in the mouse to unearth the underlying cellular basis for the malformation. Indeed, many craniofacial features observed in KBG syndrome (Ockeloen et al., 2015; Low et al., 2016; Morel Swols and Tekin, 2018) are recapitulated in the Ankrd11ncko mouse. This study has begun to unravel some of the unknowns of Ankrd11-mediated regulation of craniofacial development and has allowed assessment of craniofacial malformations as a result of Ankrd11 deletion or loss-of-function. Systematic assessment of cataloged craniofacial features in patients with KBG syndrome can potentially drive further understanding of clinical penetrance and future establishment of definitive phenotypic diagnostic criteria to achieve earlier differential diagnosis, as well as clarify the impact of ANKRD11 variants of unknown significance (VUS). Similarly, studies in other organ systems often involved in KBG syndrome will further clarify the role of Ankrd11 in epigenetic and genomic control of tissue and organ development.
Our phenotypic characterization of conditional ablation of Ankrd11 in the murine neural crest revealed novel roles of Ankrd11 in craniofacial development, specifically in intramembranous bone formation and palate development. Our results will help improve clinical assessment of patients with KBG syndrome based on craniofacial phenotypic diagnosis, particularly early in life. Ultimately, early diagnosis and better understanding of ANKRD11 function will assist with genetic counseling for patients with KBG syndrome and their affected families.
The raw data supporting the conclusions of this article will be made available by the authors, without undue reservation.
The studies involving human participants were reviewed and approved by Institutional Review Boards at the University of Calgary, University of Colorado, Denver, and the University of California, San Francisco. Written informed consent to participate in this study was provided by the participants’ legal guardian/next of kin. The animal study was reviewed and approved by Research Ethics Office at the University of Alberta (Animal Care and Use Committee) under AUP1149 and AUP2527.
DR performed the experiments, analyzed the results, and wrote the manuscript. PB performed the experiments, analyzed the results, and edited the manuscript. HL and MV-G performed the experiments and analyzed the results. S-TD and DGo performed the preliminary experiments. AW and TF performed the supporting experiments and mouse line management. NS and SE generated the Ankrd11fl/fl mice. MV-G, JA, and BH performed the morphometric analyses of human subjects and mice and edited the manuscript. DGr and AV conceived the study, analyzed the results, wrote and edited the manuscript, and provided funding. All the authors contributed to the article and approved the submitted version.
This work was supported by the Gilbert K. Winter Fund from the University of Alberta Hospital Foundation (AV and DGr), the School of Dentistry (DGr), the Alberta Dental Association & College Chair for Oral Health Research (DGr), the Canada Research Chair Tier II in Neural Stem Cell Biology (AV), CIHR (SE), and NSERC 238992-17, CIHR Foundation grant (BH).
The authors declare that the research was conducted in the absence of any commercial or financial relationships that could be construed as a potential conflict of interest.
We acknowledge the microCT facility of the School of Dentistry, University of Alberta and the Centre for Prions and Protein Folding Diseases for the use of their NanoZoomer equipment. We thank Dr. David Callen for his generous gift of the α-Ankrd11 polyclonal antibody. We would also like to acknowledge the generous support of trainees through the following scholarships: NSERC Postgraduate (Doctoral) Scholarship (PB), Alberta Graduate Excellence Scholarship (PB and AW), LiKaShing Foundation (HL), Medical Sciences Graduate Program (DR, PB, and AW), 75th Anniversary Award of the Faculty of Medicine (PB and AW), Violett Kilburn Scholarship (PB), School of Dentistry (DR), Alberta Children’s Hospital Research Institute (ACHRI) PDF (MV-G) and Alberta Innovates PDF in Health Innovation (MV-G), Women and Children Research Institute (WCHRI) Graduate Studentship Award (AW), and the NSERC Undergraduate Student Research Award (S-TD).
The Supplementary Material for this article can be found online at: https://www.frontiersin.org/articles/10.3389/fcell.2021.645386/full#supplementary-material
Supplementary Figure 1 | Landmarks used for mesh morphometric analysis. Sixty-eight anatomical landmarks placed on micro-CT volumes of the cranium using the MINC toolkit.
Supplementary Figure 2 | Ankrd11ncko mice are born with a macroscopically recognizable craniofacial phenotype. Left column: Ankrd11ctrl P0 mice. Right column: Ankrd11ncko P0 mice. (A,B) Lateral view of head, with a white arrow indicating layer of skin covering eye, incomplete in Ankrd11ncko mice. Ankrd11ncko mice also have a growth deficiency in the midface and retrognathia. (C,D) Superior view of head. Ankrd11ncko appears paler, with a more domed head shape. (E,F) View of the snout from the front. White arrow indicates black pigment that is absent in Ankrd11ncko mice. (G,H) View of dissected palate, viewed from below. Left side is anterior. Asterisk highlights cleft palate in Ankrd11ncko mouse. (I,J) View of dissected mandible and tongue, viewed from above. Left side is anterior. Note tongue (central, spanning left to right in image) is thinner and shorter in Ankrd11ncko mouse. Consistent amongst three mice from different litters.
Supplementary Figure 3 | Cleaved Caspase 3 (CC3) dependent apoptosis does not differ between Ankrd11ctrl and Ankrd11ncko palatal shelves. Representative immunofluorescent staining for cleaved caspase 3 (CC3) on coronal paraffin sections (green). Nuclei are counterstained with DAPI (red). n = 3 from 3 l. Scale bar represents 50 μm on all images.
Supplementary Table 1 | Antibodies used for immunofluorescent staining.
Supplementary Table 2 | Oligonucleotide primer pairs used for quantitative RT-PCR.
Ankrd11, Ankyrin Repeat Domain 11; ncko, neural crest knockout; nchet, neural crest heterozygous deletion; E__, embryonic day __; 36B4, acidic ribosomal phosphoprotein P0; Alpl, alkaline phosphatase; CBP, CREB-associated factor; CC3, cleaved caspase 3; CREB, cAMP-response element-binding protein; EDTA, ethylenediaminetetraacetic acid; ENU, N-ethyl -N-nitrosourea mutagenesis; Flp, flippase; HBSS, Hanks’ Balanced Salt Solution; HDAC3/5, histone deacetylase 3/5; Hh, Hedgehog; Ibsp, integrin-binding sialoprotein; Ki67, Marker of Proliferation Ki-67; Ocn, osteocalcin; Opn, osteopontin; P/CAF, p300/CBP-associated acetyltransferase complex; P0, postnatal day 0; PFA, paraformaldehyde; Prickle1, Prickle Planar Cell Polarity Protein 1; RT-qPCR, reverse transcriptase quantitative polymerase chain reaction; Runx2, Runt-related transcription factor 2; Sost, sclerostin; Sp7, osterix; TRAP, tartrate-resistant acid phosphatase; Wnt1, Wnt family member 1; Wnt/PCP, Wnt planar cell polarity; αMEM, minimum essential medium α; μCT, micro-computed tomography.
Adams, D., Collyer, M., and Kaliontzopoulou, A. (2020). geomorph: Geometric Morphometric Analyses of 2D/3D Landmark Data. Available online at: https://CRAN.R-project.org/package=geomorph (accessed Decembaer 20, 2020).
Baddam, P., Kung, T., Adesida, A. B., and Graf, D. (2020). Histological and molecular characterization of the growing nasal septum in mice. J. Anat. 238, 751–764. doi: 10.1111/joa.13332
Bakker, A. D., and Klein-Nulend, J. (2012). “osteoblast isolation from murine calvaria and long bones,” in Bone Research Protocols Methods in Molecular Biology, eds M. H. Helfrich and S. H. Ralston (Totowa, NJ: Humana Press), 19–29. doi: 10.1007/978-1-61779-415-5_2
Bannister, A. J., and Kouzarides, T. (2011). Regulation of chromatin by histone modifications. Cell Res. 21, 381–395. doi: 10.1038/cr.2011.22
Bannister, J. J., Crites, S. R., Aponte, J. D., Katz, D. C., Wilms, M., Klein, O. D., et al. (2020). Fully automatic landmarking of syndromic 3D facial surface scans using 2D Images. Sensors 20:3171. doi: 10.3390/s20113171
Barbaric, I., Perry, M. J., Dear, T. N., Rodrigues Da Costa, A., Salopek, D., Marusic, A., et al. (2008). An ENU-induced mutation in the Ankrd11 gene results in an osteopenia-like phenotype in the mouse mutant Yoda. Physiol. Genomics 32, 311–321. doi: 10.1152/physiolgenomics.00116.2007
Barrero, M. J., Boué, S., and Izpisúa Belmonte, J. C. (2010). Epigenetic mechanisms that regulate cell identity. Cell Stem Cell 7, 565–570. doi: 10.1016/j.stem.2010.10.009
Berendsen, A. D., and Olsen, B. R. (2015). Bone development. Bone 80, 14–18. doi: 10.1016/j.bone.2015.04.035
Bookstein, F. L. (1989). Principal warps: thin-plate splines and the decomposition of deformations. IEEE Trans. Pattern Anal. Mach. Intell. 11, 567–585. doi: 10.1109/34.24792
Bowen, M. E., and Attardi, L. D. (2019). The role of p53 in developmental syndromes. J. Mol. Cell Biol. 11, 200–211. doi: 10.1093/jmcb/mjy087
Breslow, D. K., Hoogendoorn, S., Kopp, A. R., Morgens, D. W., Vu, B. K., Kennedy, M. C., et al. (2018). A CRISPR-based screen for Hedgehog signaling provides insights into ciliary function and ciliopathies. Nat. Genet. 50, 460–471. doi: 10.1038/s41588-018-0054-7
Brugmann, S. A., Cordero, D. R., and Helms, J. A. (2010). Craniofacial ciliopathies: a new classification for craniofacial disorders. Am. J. Med. Genet. A 152A, 2995–3006. doi: 10.1002/ajmg.a.33727
Budhavarapu, V. N., Chavez, M., and Tyler, J. K. (2013). How is epigenetic information maintained through DNA replication? Epigenet. Chromatin 6:32. doi: 10.1186/1756-8935-6-32
Bustin, S. A., Benes, V., Garson, J. A., Hellemans, J., Huggett, J., Kubista, M., et al. (2009). The MIQE guidelines: minimum information for publication of quantitative real-time PCR experiments. Clin. Chem. 55, 611–622. doi: 10.1373/clinchem.2008.112797
Danescu, A., Mattson, M., Dool, C., Diewert, V. M., and Richman, J. M. (2015). Analysis of human soft palate morphogenesis supports regional regulation of palatal fusion. J. Anat. 227, 474–486. doi: 10.1111/joa.12365
Flaherty, K., and Richtsmeier, J. T. (2018). It’s about time: ossification center formation in C57BL/6 mice from E12–E16. J. Dev. Biol. 6:31. doi: 10.3390/jdb6040031
Gallagher, D., Voronova, A., Zander, M. A., Cancino, G. I., Bramall, A., Krause, M. P., et al. (2015). Ankrd11 is a chromatin regulator involved in autism that is essential for neural development. Dev. Cell 32, 31–42. doi: 10.1016/j.devcel.2014.11.031
Hadjidakis, D. J., and Androulakis, I. I. (2006). Bone remodeling. Ann. N. Y. Acad. Sci. 1092, 385–396. doi: 10.1196/annals.1365.035
Hallgrímsson, B., Aponte, J. D., Katz, D. C., Bannister, J. J., Riccardi, S. L., Mahasuwan, N., et al. (2020). Automated syndrome diagnosis by three-dimensional facial imaging. Genet. Med. 22, 1682–1693. doi: 10.1038/s41436-020-0845-y
He, F., Xiong, W., Wang, Y., Li, L., Liu, C., Yamagami, T., et al. (2011). Epithelial Wnt/β-catenin signaling regulates palatal shelf fusion through regulation of Tgfβ3 expression. Dev. Biol. 350, 511–519. doi: 10.1016/j.ydbio.2010.12.021
Hojo, H., Ohba, S., He, X., Lai, L. P., and McMahon, A. P. (2016). Sp7/osterix is restricted to bone-forming vertebrates where it acts as a Dlx Co-factor in osteoblast specification. Dev. Cell 37, 238–253. doi: 10.1016/j.devcel.2016.04.002
Hu, N., Strobl-Mazzulla, P. H., and Bronner, M. E. (2014). Epigenetic regulation in neural crest development. Dev. Biol. 396, 159–168. doi: 10.1016/j.ydbio.2014.09.034
Husain, A., and Jeffries, M. A. (2017). Epigenetics and bone remodeling. Curr. Osteoporos. Rep. 15, 450–458. doi: 10.1007/s11914-017-0391-y
Irie, K., Ejiri, S., Sakakura, Y., Shibui, T., and Yajima, T. (2008). Matrix mineralization as a trigger for osteocyte maturation. J. Histochem. Cytochem. 56, 561–567. doi: 10.1369/jhc.2008.950527
Irie, K., Ozawa, H., and Yajima, T. (2000). The histochemical and cytochemical changes from formative to resorptive osteocytes. Acta Histochem. Cytochem. 33, 385–391. doi: 10.1267/ahc.33.385
Jeffries, M. A., and Sawalha, A. H. (2015). Autoimmune disease in the epigenetic era: how has epigenetics changed our understanding of disease and how can we expect the field to evolve? Expert Rev. Clin. Immunol. 11, 45–58. doi: 10.1586/1744666X.2015.994507
Juriloff, D. M., Harris, M. J., Mager, D. L., and Gagnier, L. (2014). Epigenetic mechanism causes Wnt9b deficiency and nonsyndromic cleft lip and palate in the A/WySn mouse strain. Birth Defects Res. Part A Clin. Mol. Teratol. 100, 772–788. doi: 10.1002/bdra.23320
Komori, T. (2017). “Roles of Runx2 in skeletal development,” in RUNX Proteins in Development and Cancer Advances in Experimental Medicine and Biology, eds Y. Groner, Y. Ito, P. Liu, J. C. Neil, N. A. Speck, and A. van Wijnen (Singapore: Springer), 83–93. doi: 10.1007/978-981-10-3233-2_6
Kouskoura, T., Fragou, N., Alexiou, M., John, N., Sommer, L., Graf, D., et al. (2011). The genetic basis of craniofacial and dental abnormalities. Schweiz Monatsschr Zahnmed 121, 636–646.
Kuriyama, M., Udagawa, A., Yoshimoto, S., Ichinose, M., Sato, K., Yamazaki, K., et al. (2008). DNA methylation changes during cleft palate formation induced by retinoic acid in mice. Cleft Palate Craniofac. J. 45, 545–551. doi: 10.1597/07-134.1
Lewis, A. E., Vasudevan, H. N., O’Neill, A. K., Soriano, P., and Bush, J. O. (2013). The widely used Wnt1-Cre transgene causes developmental phenotypes by ectopic activation of Wnt signaling. Dev. Biol. 379, 229–234. doi: 10.1016/j.ydbio.2013.04.026
Li, C.-W., Dinh, G. K., Zhang, A., and Chen, J. D. (2008). Ankyrin repeats-containing cofactors interact with ADA3 and modulate its co-activator function. Biochem. J. 413, 349–357. doi: 10.1042/BJ20071484
Li, H., Xie, H., Liu, W., Hu, R., Huang, B., Tan, Y.-F., et al. (2009). A novel microRNA targeting HDAC5 regulates osteoblast differentiation in mice and contributes to primary osteoporosis in humans. J. Clin. Invest. 119, 3666–3677. doi: 10.1172/JCI39832
Livak, K. J., and Schmittgen, T. D. (2001). Analysis of relative gene expression data using real-time quantitative PCR and the 2(-Delta Delta C(T)) Method. Methods 25, 402–408. doi: 10.1006/meth.2001.1262
Low, K., Ashraf, T., Canham, N., Clayton-Smith, J., Deshpande, C., Donaldson, A., et al. (2016). Clinical and genetic aspects of KBG syndrome. Am. J. Med. Genet. A 170, 2835–2846. doi: 10.1002/ajmg.a.37842
Malik, Z., Roth, D. M., Eaton, F., Theodor, J. M., and Graf, D. (2020). Mesenchymal Bmp7 controls onset of tooth mineralization: a novel way to regulate molar cusp shape. Front. Physiol. 11:698. doi: 10.3389/fphys.2020.00698
Marini, F., Cianferotti, L., and Brandi, M. L. (2016). Epigenetic mechanisms in bone biology and osteoporosis: can they drive therapeutic choices? Int. J. Mol. Sci. 17:1329. doi: 10.3390/ijms17081329
Mirabella, A. C., Foster, B. M., and Bartke, T. (2016). Chromatin deregulation in disease. Chromosoma 125, 75–93. doi: 10.1007/s00412-015-0530-0
Morel Swols, D., and Tekin, M. (2018). “KBG Syndrome,” in Gene Reviews, eds M. P. Adam, H. H. Ardinger, R. A. Pagon, S. E. Wallace, L. J. Bean, K. Stephens, et al. (Seattle, WA: University of Washington, Seattle).
Nakaniwa, M., Kawasaki, M., Kawasaki, K., Yamada, A., Meguro, F., Takeyasu, M., et al. (2019). Primary cilia in murine palatal rugae development. Gene Expression Patterns 34:119062. doi: 10.1016/j.gep.2019.119062
Neilsen, P., Cheney, K. M., Li, C.-W., Chen, J., Cawrse, J. E., Schulz, R. B., et al. (2008). Identification of ANKRD11 as a p53 coactivator. J. Cell Sci. 121(Pt 21), 3541–3552. doi: 10.1242/jcs.026351
Ockeloen, C. W., Willemsen, M. H., de Munnik, S., van Bon, B. W. M., de Leeuw, N., Verrips, A., et al. (2015). Further delineation of the KBG syndrome phenotype caused by ANKRD11 aberrations. Eur. J. Hum. Genet. 23, 1176–1185. doi: 10.1038/ejhg.2014.253
Park-Min, K. H. (2017). Epigenetic regulation of bone cells. Connect Tissue Res. 58, 76–89. doi: 10.1080/03008207.2016.1177037
Perpétuo, I. P., Bourne, L. E., and Orriss, I. R. (2019). Isolation and generation of osteoblasts. Methods Mol Biol 1914, 21–38. doi: 10.1007/978-1-4939-8997-3_2
R Core Team (2020). R: A Language and Environment for Statistical Computing. Available online at: https://www.R-project.org/ (accessed December 20, 2020).
Samuels, B. D., Aho, R., Brinkley, J. F., Bugacov, A., Feingold, E., Fisher, S., et al. (2020). FaceBase 3: analytical tools and FAIR resources for craniofacial and dental research. Development 147:dev191213. doi: 10.1242/dev.191213
Satterstrom, F. K., Kosmicki, J. A., Wang, J., Breen, M. S., De Rubeis, S., An, J.-Y., et al. (2020). Large-scale exome sequencing study implicates both developmental and functional changes in the neurobiology of autism. Cell 180, 568.e23–584.e23. doi: 10.1016/j.cell.2019.12.036
Schlager, S. (2017). “Chapter 9 - morpho and rvcg – shape analysis in R: R-Packages for geometric morphometrics, shape analysis and surface manipulations,” in Statistical Shape and Deformation Analysis, eds G. Zheng, S. Li, and G. Székely (Cambridge, MA: Academic Press), 217–256. doi: 10.1016/B978-0-12-810493-4.00011-0
Schroeder, T. M., Kahler, R. A., Li, X., and Westendorf, J. J. (2004). Histone deacetylase 3 Interacts with Runx2 to repress the osteocalcin promoter and regulate osteoblast differentiation. J. Biol. Chem. 279, 41998–42007. doi: 10.1074/jbc.M403702200
Schroeder, W., Martin, K., and Lorensen, W. (1998). The Visualization Toolkit : An Object-Oriented Approach to 3D Graphics, 2nd Edn. Hoboken, NJ: Prentice Hall.
Seelan, R. S., Mukhopadhyay, P., Warner, D. R., Webb, C. L., Pisano, M., and Greene, R. M. (2013). Epigenetic regulation of Sox4 during palate development. Epigenomics 5, 131–146. doi: 10.2217/epi.13.1
Sharp, G., Stergiakouli, E., Sandy, J., and Relton, C. (2018). Epigenetics and orofacial clefts: a brief introduction. Cleft Palate Craniofac. J. 55, 795–797. doi: 10.1597/16-124
Skarnes, W. C., Rosen, B., West, A. P., Koutsourakis, M., Bushell, W., Iyer, V., et al. (2011). A conditional knockout resource for the genome-wide study of mouse gene function. Nature 474, 337–342. doi: 10.1038/nature10163
St John, H. C., Bishop, K. A., Meyer, M. B., Benkusky, N. A., Leng, N., Kendziorski, C., et al. (2014). The osteoblast to osteocyte transition: epigenetic changes and response to the vitamin D3 hormone. Mol. Endocrinol. 28, 1150–1165. doi: 10.1210/me.2014-1091
Strobl-Mazzulla, P. H., Marini, M., and Buzzi, A. (2012). Epigenetic landscape and miRNA involvement during neural crest development. Dev. Dyn. 241, 1849–1856. doi: 10.1002/dvdy.23868
Temiyasathit, S., and Jacobs, C. R. (2010). Osteocyte primary cilium and its role in bone mechanotransduction. Ann. N. Y. Acad. Sci. 1192, 422–428. doi: 10.1111/j.1749-6632.2009.05243.x
Vidal-García, M. (2021). morpho. Tools. GM v1.0.0: A Set of R Tools to Help With Geometric Morphometric Analyses of 3D Data. doi: 10.5281/zenodo.4673771
Vincent, R. D., Neelin, P., Khalili-Mahani, N., Janke, A. L., Fonov, V. S., Robbins, S. M., et al. (2016). MINC 2.0: a flexible format for multi-modal images. Front. Neuroinform. 10:35. doi: 10.3389/fninf.2016.00035
Wan, Y., Lantz, B., Cusack, B. J., and Szabo-Rogers, H. L. (2018). Prickle1 regulates differentiation of frontal bone osteoblasts. Sci. Rep. 8:18021. doi: 10.1038/s41598-018-36742-0
Wein, M. N., Spatz, J., Nishimori, S., Doench, J., Root, D., Babij, P., et al. (2015). HDAC5 controls MEF2C-driven sclerostin expression in osteocytes. J. Bone Miner Res. 30, 400–411. doi: 10.1002/jbmr.2381
Wilfert, A. B., Sulovari, A., Turner, T. N., Coe, B. P., and Eichler, E. E. (2017). Recurrent de novo mutations in neurodevelopmental disorders: properties and clinical implications. Genome Med. 9:101. doi: 10.1186/s13073-017-0498-x
Winkler, D. G., Sutherland, M. K., Geoghegan, J. C., Yu, C., Hayes, T., Skonier, J. E., et al. (2003). Osteocyte control of bone formation via sclerostin, a novel BMP antagonist. EMBO J. 22, 6267–6276. doi: 10.1093/emboj/cdg599
Xiong, W., He, F., Morikawa, Y., Yu, X., Zhang, Z., Lan, Y., et al. (2009). Hand2 is required in the epithelium for palatogenesis in mice. Dev. Biol. 330, 131–141. doi: 10.1016/j.ydbio.2009.03.021
Yoshida, T., Vivatbutsiri, P., Morriss-Kay, G., Saga, Y., and Iseki, S. (2008). Cell lineage in mammalian craniofacial mesenchyme. Mech. Dev. 125, 797–808. doi: 10.1016/j.mod.2008.06.007
Zhang, A., Li, C.-W., and Chen, J. D. (2007). Characterization of transcriptional regulatory domains of ankyrin repeat cofactor-1. Biochem. Biophys. Res. Commun. 358, 1034–1040. doi: 10.1016/j.bbrc.2007.05.017
Zhang, A., Yeung, P. L., Li, C.-W., Tsai, S.-C., Dinh, G. K., Wu, X., et al. (2004). Identification of a novel family of ankyrin repeats containing cofactors for p160 nuclear receptor coactivators. J. Biol. Chem. 279, 33799–33805. doi: 10.1074/jbc.M403997200
Keywords: KBG syndrome, epigenetic regulation, craniofacial development and malformations, intramembranous ossification, bone remodeling, cleft palate, neurodevelopmental disorders, chromatin regulation
Citation: Roth DM, Baddam P, Lin H, Vidal-García M, Aponte JD, De Souza S-T, Godziuk D, Watson AES, Footz T, Schachter NF, Egan SE, Hallgrímsson B, Graf D and Voronova A (2021) The Chromatin Regulator Ankrd11 Controls Palate and Cranial Bone Development. Front. Cell Dev. Biol. 9:645386. doi: 10.3389/fcell.2021.645386
Received: 23 December 2020; Accepted: 31 March 2021;
Published: 29 April 2021.
Edited by:
Poongodi Geetha-Loganathan, SUNY Oswego, United StatesReviewed by:
Steven Goudy, Emory University, United StatesCopyright © 2021 Roth, Baddam, Lin, Vidal-García, Aponte, De Souza, Godziuk, Watson, Footz, Schachter, Egan, Hallgrímsson, Graf and Voronova. This is an open-access article distributed under the terms of the Creative Commons Attribution License (CC BY). The use, distribution or reproduction in other forums is permitted, provided the original author(s) and the copyright owner(s) are credited and that the original publication in this journal is cited, in accordance with accepted academic practice. No use, distribution or reproduction is permitted which does not comply with these terms.
*Correspondence: Daniel Graf, ZGdyYWZAdWFsYmVydGEuY2E=; Anastassia Voronova, dm9yb25vdmFAdWFsYmVydGEuY2E=
†ORCID: Daniela Marta Roth, orcid.org/0000-0001-8156-4681; Pranidhi Baddam, orcid.org/0000-0003-0232-2022; Haiming Lin, orcid.org/0000-0002-9925-8188; Marta Vidal-García, orcid.org/0000-0001-7617-7329; Jose David Aponte, orcid.org/0000-0002-1608-8612; Sarah-Thea De Souza, orcid.org/0000-0003-3081-2766; Sean E. Egan, orcid.org/0000-0003-0189-3202; Benedikt Hallgrímsson, orcid.org/0000-0002-7192-9103; Daniel Graf, orcid.org/0000-0003-1163-8117; Anastassia Voronova, orcid.org/0000-0001-7504-1905
Disclaimer: All claims expressed in this article are solely those of the authors and do not necessarily represent those of their affiliated organizations, or those of the publisher, the editors and the reviewers. Any product that may be evaluated in this article or claim that may be made by its manufacturer is not guaranteed or endorsed by the publisher.
Research integrity at Frontiers
Learn more about the work of our research integrity team to safeguard the quality of each article we publish.