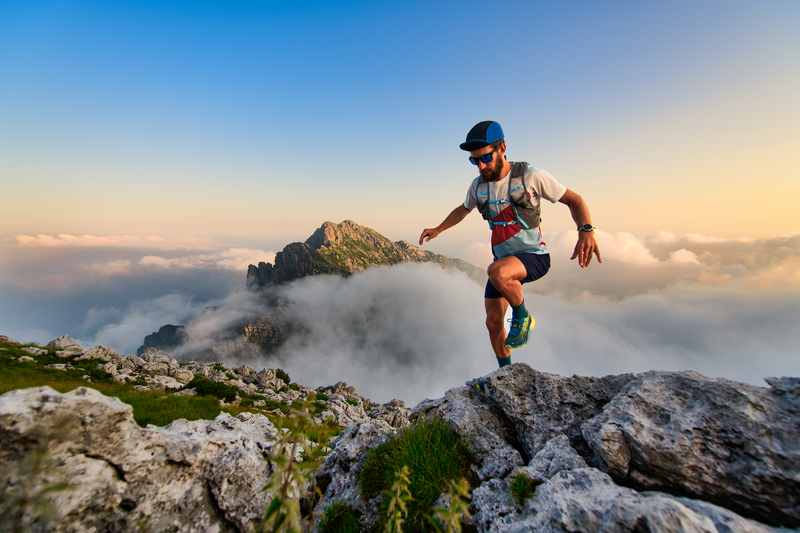
95% of researchers rate our articles as excellent or good
Learn more about the work of our research integrity team to safeguard the quality of each article we publish.
Find out more
ORIGINAL RESEARCH article
Front. Cell Dev. Biol. , 23 March 2021
Sec. Cellular Biochemistry
Volume 9 - 2021 | https://doi.org/10.3389/fcell.2021.644135
This article is part of the Research Topic Germ Cell Development and Reproductive Aging View all 21 articles
Tet enzymes participate in DNA demethylation and play critical roles in stem cell pluripotency and differentiation. DNA methylation alters with age. We find that Tet1 deficiency reduces fertility and leads to accelerated reproductive failure with age. Noticeably, Tet1-deficient mice at young age exhibit dramatically reduced follicle reserve and the follicle reserve further decreases with age, phenomenon consistent with premature ovarian failure (POF) syndrome. Consequently, Tet1-deficient mice become infertile by reproductive middle age, while age matched wild-type mice still robustly reproduce. Moreover, by single cell transcriptome analysis of oocytes, Tet1 deficiency elevates organelle fission, associated with defects in ubiquitination and declined autophagy, and also upregulates signaling pathways for Alzheimer’s diseases, but down-regulates X-chromosome linked genes, such as Fmr1, which is known to be implicated in POF. Additionally, Line1 is aberrantly upregulated and endogenous retroviruses also are altered in Tet1-deficient oocytes. These molecular changes are consistent with oocyte senescence and follicle atresia and depletion found in premature ovarian failure or insufficiency. Our data suggest that Tet1 enzyme plays roles in maintaining oocyte quality as well as oocyte number and follicle reserve and its deficiency can lead to POF.
Ten-eleven translocation (Tet) methylcytosine dioxygenases play a major role in shaping DNA methylation patterns through demethylation (Wu and Zhang, 2017). Tet enzymes have been demonstrated to play important roles in ESC pluripotency and differentiation (Ito et al., 2010; Koh et al., 2011). However, Tet1 or Tet2 -null mice are viable and overtly normal double Tet1/Tet2-deficient mice are also obtained (Dawlaty et al., 2011, 2013; Ko et al., 2011), but, some Tet1-deficient mice display a smaller body size at birth, which might reflect a developmental delay. Moreover, Tet1-mediated 5 hmC signals play important role in DNA demethylation during primordial germ cell (PGC) development and meiosis (Yamaguchi et al., 2012, 2013a; Hill et al., 2018). Tet1 deficiency results in meiotic defects of PGCs, including impaired homologous pairing and recombination in meiotic germ cells (meiocytes) during fetal development, probably due to insufficient demethylation and failed activation of meiotic genes (Yamaguchi et al., 2013a), such that Tet1-deficient mice at young age already exhibit reduced number of oocytes in the ovary and subfertility (Yamaguchi et al., 2012, 2013a). It also will be interesting to investigate whether Tet1 deficiency impacts reproductive aging of adult mice.
From the onset of reproductive maturity, organismal aging is characterized by declined fecundity, increased tissue dysfunction, and susceptibility to disease or mortality (Rando and Chang, 2012). The aging process is associated with altered epigenetic regulation, including DNA methylation, histone modification and chromatin remodeling (Zhang et al., 2020). DNA methylation involves in many aspects of cellular and molecular changes in aging (Lopez-Otin et al., 2013). Moreover, change to DNA methylation in aging has been used to predict the chronological age of human somatic tissues and individuals (Hannum et al., 2013; Horvath, 2013). Predictions have been made from heterogeneous samples such as lung, liver, and brain tissues as well as whole blood and peripheral blood mononuclear cells, and isolated CD4 + T cells, monocytes, and B cells (Horvath, 2013). Epigenetic clock sites have been defined also in multiple tissues in mouse (Stubbs et al., 2017) and age-related methylation changes between mouse and human were partially conserved (Maegawa et al., 2010). Deeper understanding of epigenetic alterations in aging and the molecular basis of the DNA methylation clock is of particular interest as epigenetic modifications can be reversed, allowing manipulation to potentially reverse aging and thus therapeutic potential (Rando and Chang, 2012; Cole et al., 2017; Chen and Kerr, 2019; Zhang et al., 2020). Nevertheless, it remains elusive whether Tet deficiency can impact aging in the adult.
Ovarian aging is mainly characterized by a sharp decrease in the number of oocyte and follicles and a decrease in oocyte quality (van Rooij et al., 2005; Broekmans et al., 2007, 2009; Tatone et al., 2008). The number of oocytes and follicles stored known as follicle reserve at birth is fixed, which cannot be replenished by germ cells in postnatal ovaries, but with age, periodic ovulation, follicle atresia and apoptosis are the main causes of a sharp decline in the number of follicles (Faddy, 2000; Bristol-Gould et al., 2006; Broekmans et al., 2007; Djahanbakhch et al., 2007; Notarianni, 2011; Zhang et al., 2012). Follicle depletion, in turn, causes lower levels of estrogen secretion in the body, eventually leading to menopause (Lawson et al., 2003).
Recently, we report that Tet2 deficiency accelerates reproductive aging in the adult female mice (Wang et al., 2020). Here we compared the fertility of mice deficient for Tet1 from young to reproductive old age. We report roles of Tet1 in maintaining oocyte quality in addition to oocyte number for preserving fertility with age.
Tet1 knockout (Tet1–/–) mice were generated from 129 × C57BL/6J mixed genetic background (Zhang et al., 2013). Most of wild-type (WT) and Tet1 knockout mice were generated from Tet1 heterozygotes mating. For fertility analysis, Tet1–/– females were crossed with WT male at young age and vaginal plugs were checked daily. All mice in the study were maintained in a mixed 129 × C57BL/6 background. All mouse experiments were carried out in accordance with the guidelines and regulations and approved by the Institutional Animal Care and Use Committee of Nankai University.
Postnatal mice at 2 weeks old were genotyped using DNA extracted from their ears. PCR was carried out at 94°C for 2 min, followed by 35 cycles at 94°C for 30 s, 60°C for 30 s, and 72°C for 1 min. DNA fragments were visualized by agarose gel electrophoresis. The genotyping primers were listed as follows.
Ovaries were collected from different group mice (n > 10 mice for young and middle-age group and n = 7–8 mice for old group) and fixed by immersion in 4% paraformaldehyde (PFA) overnight at 4°C, and tissues were embedded with paraffin wax, based on previous methods (Liu et al., 2013). The follicles were categorized into primordial and primary, secondary and antral, accordingly (Myers et al., 2004). Follicles were classified as primordial and primary if they contained an oocyte surrounded by a single layer of squamous, or, cuboidal granulosa cells. Secondary follicles were identified as having more than one layer of granulosa cells with no visible antrum. Antral follicles possessed one or two small areas of follicular fluid (antrum) or a single large antral space.
For collection of oocytes for RNA-seq, female mice from the different groups were superovulated by intraperitoneal injection of 5 I U pregnant mare’s serum gonadotrophin (PMSG), followed 46-48 h later by 5 I U human chorionic gonadotrophin (hCG), to obtain MII oocytes. Granulosa cells attached to oocytes were removed in M2, or HKSOM supplemented with 0.03% hyaluronidase by gently pipetting.
RNA was isolated from ovaries using RNeasy mini kit (Qiagen), and subject to cDNA synthesis using Moloney Murine Leukemia Virus Reverse Transcriptase (Invitrogen). PCR reactions were set up in duplicates using the FastStart Universal SYBR Green Master (4913914001, Roche) and run on the Mastercycler® RealPlex2 real time PCR detection system (Eppendorf). The final PCR reaction volume in 20 μl contained 10 μl SYBR Green PCR Master Mix, 1 μl cDNA template, 2 μl primer mixture and 7 μl water. Thermal cycling was carried out with a 10 min denaturation step at 95°C, followed by two-step cycles, 15 s at 95°C and 1 min at 60°C. Each sample was analyzed using GAPDH as the internal control. Primers were designed as follows.
For paraffin sections, after deparaffinizing, rehydrating and washing in PBS (pH 7.2–7.4), the sections were subjected to high pressure antigen recovery sequentially in citrate buffer (pH 6.0) for 3 min, incubated with blocking solution (5% goat serum and 0.1% BSA in PBS) for 2 h at room temperature, and then incubated with the diluted primary antibodies [anti-5 hmC (39769, Active Motif), anti-Tet1 (MABE1144, Millipore), anti-Oct4 (SC-5279, Santa Cruz)] overnight at 4°C. After washing with PBS, sections were incubated with appropriate secondary antibodies (Alexa Fluor® FITC, 488, or 594). The sections were then stained with 1 μg/ml DAPI for 10 min to reveal nuclei, washed with PBS, and mounted in Vectashield (H-1000, Vector Laboratories, Burlingame, CA, United States). Relative integrated fluorescence intensity for 5 hmC was estimated by Image J software. The threshold was defined using non-specific background fluorescence.
MII oocytes were collected from young WT (n = 19), Tet1–/– (n = 15) or from old WT (n = 19), Tet1–/– (n = 14) mice in two biologically repeated experiments. Single MII oocyte was resuspended in PBS with 0.1% BSA (A3311-10g, Sigma), picked up in 1 μL 0.1% BSA using a micropipette with an epT.I.P.S. pipette tip (0030000838, Eppendorf) under a dissecting microscope, and transferred to the bottom of a 200-μL PCR tube (8-strip, nuclease-free, thin-walled PCR tubes with caps, PCR-0208-C, Axygen) containing oligo (dT) primer, Triton X-100, and Recombinant RNase Inhibitor (RRI, 2313A, Takara). Samples were frozen in liquid nitrogen and stored or used immediately.
Frozen or fresh samples were melted on ice and 1 μL deoxy-ribonucleoside triphosphate (R0191, Thermo Scientific) was added into the tubes, vortexed gently, and incubated at 37°C for 3 min. The cDNAs were then synthesized using SuperScriptTM II Reverse Transcriptase (18064071, Thermo Scientific) by slightly modified Smart-seq2 methods (Picelli et al., 2014), followed by 15 cycles of PCR using KAPA HotStart ReadyMix (KK2602, KAPA Biosystems) and then purified using Agencount AMPure XP beads (A63881, Beckman).
RNA-seq libraries were prepared using Smart-seq2 methods as previously described (Picelli et al., 2014). Briefly, the libraries were prepared by using TruePrep DNA Library Prep Kit V2 for Illumina® (TD503-02, Vazyme Biotech co.,ltd.) according to the manual instruction. Samples were barcoded during library preparation and multiplex sequenced, with a 150-bp pair-end sequencing strategy on a HiSeq 10x (Illumina).
Raw reads were processed using trim-galore and clean reads were mapped to mm 10 from UCSC genome1 by Hisat2 (v2.1.0) with default parameters (Kim et al., 2013, 2015). Uniquely mapped reads annotated in Gencode vM17 were calculated by Featurecounts (Liao et al., 2014), with default parameters (featureCounts −T 30 −O −Q 30 −p −a gencode.vM17.annotation.gtf.gz -o v17.txt ∗.bam). Sum factor normalization was applied with deconvolution of size factors within different batch samples using SCnorm (Bacher et al., 2017). Raw counts were normalized by library size via counts of exon model per million mapped reads (CPM). Gene expression was counted in individual oocyte when the transcript number was >1. Dynamic changes of differentially expressed genes (DEGs) between different groups were analyzed using Deseq2 (Love et al., 2014). DEGs were defined only if p value was <0.05, and a fold change was >1.5. Young Tet1–/– oocytes had 2,013 downregulated and 1,557 upregulated genes, compared with those of young WT oocytes. There were 1,448 downregulated and 1,696 upregulated genes in old Tet1–/– oocytes compared with those of old WT oocytes.
Gene ontology (GO) terms were collected from MGI (Bult et al., 2019) and kyoto encyclopedia of genes and genomes (KEGG) pathways from KEGG pathway database (Wixon and Kell, 2000). Enrichment results were obtained using clusterprofiler (Yu et al., 2012) and KOBAS (Xie et al., 2011).
Reads were mapped to mm 10 from UCSC genome see text footnote 1 by Hisat2 (v2.1.0) with default parameters as above. For estimation of retroelement expression, “repeatMasker” track, was downloaded from UCSC genome browser in GTF, based on the genomic coordination in GTF files. Annotation file of transposable elements was extracted and made as a SAF file, which is uploaded in https://github.com/LianaLiu/single. Reads were counted using featurecounts and only the unique mapped reads with completely overlapping retrotransposon coordinates were counted. Class and family of transposable elements are also provided in the supplementary datasheet Supplementary Table 1. Annotation of full-length retrotransposon elements was constructed based on methods described previously (Kaul et al., 2019).
Full length of L1 annotation file was also analyzed according to the previous method (Deininger et al., 2017; Kaul et al., 2019), with slight modifications, and 6,000 bp of L1 sequence were assumed to be the full-length elements. We utilized our paired-end RNA-seq reads aligned by Hisat2 and Samtools to create a sorted bam file and annotated based on our data.
Serum anti-Mullerian hormone (AMH) levels were assayed using ELISA kit (CK-E90200, Hangzhou EastBiopharm CO., LTD.). Quality control serum, sterilized distilled water, and five series diluted standard samples for a standard curve were tested for each serum sample. The intra- and inter-assay coefficients of variability for AMH were below 8 and 12%.
Data were analyzed by student’s t-test, χ2 test or fisher’s exact test for paired comparison, or by ANOVA and means compared by Fisher’s protected least-significant difference (PLSD) for multiple comparisons using the StatView from SAS Institute and the graphs made by GraphPad Prism 7 (GraphPad Software, San Diego, CA, United States). Kruskal-Wallis test was used to compare multiple groups using ggpubr R package. Linear regression analysis was done using GraphPad Prism 7. The data between groups were considered significant when P < 0.05 (∗), 0.01(∗∗) or 0.001(∗∗∗).
To study whether Tet1 regulates fertility with age, we employed Tet1 deficient mice generated using homologous recombination by deleting exons 11-13 (Zhang et al., 2013). Homozygous Tet1 knockout mice (Tet1–/–) were produced by crossing heterozygous mice (Supplementary Figure 1A). However, Tet1 deficiency resulted in death of a small proportion of embryos (Supplementary Figure 1B), consistent with previous study (Yamaguchi et al., 2012). The Tet1 knockout was evaluated based on genotyping, as well as the minimal mRNA expression level of Tet1 determined by qPCR (Supplementary Figure 1C). Also, Tet1 protein was absent in Tet1–/– blastocysts by immunofluorescence microscopy (Supplementary Figure 1D), further validating the successful knockout of the Tet1 gene. Representative primordial oocytes were shown by enclosed granulosa cells (Supplementary Figure 1E). The 5 hmC levels of primordial oocytes from Tet1–/– mice as shown by immunofluorescence were decreased compared to WT oocytes (Supplementary Figure 1F).
Wild-type (WT) and Tet1–/– female mice at the reproductive age of 2–4 months (young), 7–9 months (middle-age) and 10–12 months (old), were mated with young WT males, and the successfully mated females judged by the presence of mating plug next morning were allowed to deliver the pups to determine the fertility. WT mice exhibited reduced fertility with age as shown by smaller litter size, as expected for natural reproductive aging in females. Comparatively, Tet1–/– female mice manifested declined fertility and accelerated reproductive failure with age, as they produced smaller litter size than that of age-matched WT mice (Figure 1A). By linear regression analysis, WT and Tet1–/– mice with age displayed similar negative slope in the production of the offspring (Figure 1B). While WT females at middle-age produced an average of six pups, Tet1–/– deficient age-matched mice or at an older age failed to give birth to a pup (Figure 1A). Tet1–/– female mice were infertile at middle-age. This phenomenon is comparable to premature ovarian failure. Consistently, ovaries of Tet1–/– females at three age groups were lighter than those of age-matched WT females from young to old age (Figure 1C). By examining the ovarian serial sections by histology, the number of primordial and primary follicles as well as secondary and mature antral follicles was dramatically reduced in Tet1–/– females from the three age groups, compared to WT mice served as control (Figures 1D,E). Slope in the declined number of follicles in WT mice with age appeared to be much higher than did Tet1–/– mice, and the decrease of secondary and antral follicles in Tet1–/– mice with age actually was not significant (Figure 1F). This likely was because the follicle reserve was already dramatically lower in Tet1–/– than in WT mice at young age. Consistently, lower AMH levels indicative of ovarian reserve were found in serum of Tet1–/– than in WT mice at young age (Figure 1G). Therefore, Tet1 deficiency decreases fecundity particularly with age. The decreased fecundity is largely caused by prominent reduction of follicle reserve and development, and also probably by reduced oocyte quality.
Figure 1. Tet1 knockout causes subfertility and premature ovarian failure. (A) Average litter size of wild-type (WT) and Tet1 knockout (Tet1–/–) female mice at three different reproductive age (young, 2–4 months; middle-age, 7–9 months; old, 10–12 months). n > 10 mice for young and middle-age group and n = 7–8 mice for old group. (B) Linear regression analysis of the age effects on litter size between WT and Tet1–/– mice. n > 10 mice for young and middle-age group and n = 7–8 mice for old group. (C) Average weight per ovary from WT and Tet1–/– mice. n > 7. (D) Representative images of hematoxylin and eosin (H&E) staining of ovarian sections from young, middle-age and old groups. Scale bar, 500 μm. (E) Number of primordial and primary follicles, or secondary and antral follicles from WT and Tet1–/– mice. n = 4-6. (F) Linear regression analysis of the age effects on the number of follicles between WT and Tet1–/– mice. n = 3-4. ns, no significant difference. (G) AMH levels in serum of WT and Tet1–/– mice by ELISA assay (n = 4). The bars show mean ± SEM. *P < 0.05; **P < 0.01; ***P < 0.001. The P value is labeled if P > 0.05 (A,E).
To examine potential molecular changes in the oocytes, we performed single-cell RNA-seq detecting an average number of genes more than 15,000 (Supplementary Figure 2A) to analyze the oocytes of Tet1–/– (n = 15) and WT (n = 19) collected from young mice in two biological repeated experiments. Tet1–/– oocytes showed 2,013 downregulated (p value < 0.05, and a fold change <−1.5) and 1,557 upregulated genes (p value < 0.05, and a fold change >1.5), compared with WT oocytes (Figure 2A). Significantly downregulated genes in Tet1–/– oocytes included Fmr1 and Tet1, supporting an effective deletion of Tet1. Also, we analyzed differential gene expression among chromosomes (Supplementary Figure 2A). Notably, gene expression on chromosome X (Figure 2B) and in mitochondrial functions (Figure 2C) was significantly decreased in Tet1–/– oocytes compared with WT oocytes. About 39 genes located on X-chromosomes such as Nudf11, Tmem47, and Fmr1 were down-regulated in Tet1–/– oocytes. Due to the important roles of Fmr1, we used metascape to analyzed the potential protein-protein interaction enrichment analysis and found Fmr1 might interact with Hnrnpu and Pabpc1 (Supplementary Figure 2B). Moreover, the upregulated genes by Tet1 deficiency were enriched in homologous recombination, cell cycle, fanconi anemia pathway, Alzheimer’s disease, and metabolic pathways, while downregulated genes enriched in Ras signaling pathway, neurotrophin signaling pathway, which can cause premature ovarian failure (Dorfman et al., 2014), and notably autophagy and ubiquitin mediated proteolysis (Figures 2D–F). By GO enrichment analysis, downregulated genes after Tet1 deficiency were enriched in mRNA processing, regulation of mRNA stability, histone modification, negative regulation of apoptotic pathway such as Uri1, Rtkn2, Nr4a2, Bcl2l2, Akt1 and Psme3, and also protein polyubiquitination (Figures 2G,H). Interestingly, genes for DNA repair, nuclear division, chromosome segregation and organelle fission were upregulated in Tet1-deficient oocytes (Figures 2G,I). Increased apoptosis has been implicated in reduction of germ cell numbers (Yamaguchi et al., 2012). Together, these results suggest that elevated organelle fission and reduced protein polyubiquitination and autophagy might be implicated in reduced oocyte quality resulting from loss of Tet1.
Figure 2. Differential gene expression of Tet1–/– and WT oocytes. (A) Scatter-plots showing gene expression profile between young Tet1–/– (n = 15) and WT (n = 19) oocytes from two biological repeats. (B) Boxplot showing gene expression on chromosome X and downregulated in young Tet1–/– oocytes compared with young WT oocytes. ****P < 0.0001. (C) Boxplot showing expression of genes on mitochondrial function and Downregulated in young Tet1–/– oocytes. (D) KEGG enrichment results showing genes downregulated or upregulated after Tet1 deficiency. X-axis represents the corrected p value (FDR) using Benjamini and Hochberg. (E) Pheatmap showing genes enriched in autophagy signaling pathway down regulated in Tet1 deficient oocytes. (F) Pheatmap showing genes enriched in Alzheimer’s disease upregulated in Tet1 deficient oocytes. (G) GO enrichment results showing genes downregulated or upregulated after Tet1 deficiency. X-axis represents the corrected p value (FDR) using Benjamini and Hochberg. (H) Pheatmap showing genes enriched in protein polyubiquitination in Tet1 deficient oocytes. (I) Pheatmap showing genes enriched in organelle fission upregulated in Tet1 deficient oocytes.
Further, we compared our single cell RNA-seq data on oocytes with published data on PGCs (Yamaguchi et al., 2012) and found that 63 genes including Rnf8, Tet3, Fmr1nb, and Xlr5a/b/c were commonly downregulated in both Tet1-deficient oocytes and PGCs (Supplementary Figures 3A,B). We also compared our data using oocytes with the differentially expressed genes (DEG) associated with differential methylated regions (DMR) from published data using PGCs (Yamaguchi et al., 2012). About 20 genes, including Tet1, Sycp3, Dazl, and Sirt6, exhibited reduced expression in association with increased methylation after Tet1 deficiency (Supplementary Figure 3C). The analysis shows that Tet1 also plays a role in maturing oocytes in addition to its function in PGCs and meiosis.
We further analyzed differential gene expression between old WT (n = 19) and Tet1–/– oocytes (n = 14) in two biological repeats. Tet1, as expected, and Dazl were downregulated in old Tet1–/– oocytes compared with old WT oocytes, while Dnmt3a was highly expressed in old Tet1–/– oocytes (Figure 3A). Downregulated genes in old Tet1–/– oocytes were enriched in insulin signaling pathway, Parkinson’s disease, pathways in cancer and sphingolipid signaling pathway, and genes upregulated in old Tet1–/– oocytes enriched in spliceosome, choline metabolism in cancer, and phospholipase D signaling pathway (Figure 3B). The expression of genes enriched in insulin signaling pathway (Figure 3C), were decreased and genes enriched in spliceosome (Figure 3D) were increased in Tet1–/– oocytes. By GO enrichment analysis, genes upregulated in old Tet1–/– oocytes were enriched in cellular component disassembly, negative regulation of organelle organization, small GTPase mediated signal transduction, DNA repair, regulation of DNA metabolic process and protein localization to Golgi apparatus, whereas genes downregulated enriched in organelle fission, protein dephosphorylation, proteasomal protein catabolic process and negative regulation of apoptotic pathway including genes including Akt1, Bcl2, Nr4a2, etc (Figure 3E). Expression of genes enriched in proteasomal protein catabolic process were decreased (Figure 3F), and those in DNA repair were increased (Figure 3G). Implication of increased DNA repair is not known, but possibly suggests increased DNA damage stress due to loss of Tet1.
Figure 3. Differential gene expression of old Tet1–/– and WT oocytes. (A) Scatter-plots showing gene expression profile between old Tet1–/– (n = 14) and WT (n = 19) oocytes from two biological repeats. (B) KEGG enrichment results showing genes downregulated or upregulated after Tet1 deficiency. X-axis represents the corrected p value (FDR) using Benjamini and Hochberg. (C) Pheatmap showing genes enriched in insulin signaling pathway in Tet1 deficient oocytes. (D) Pheatmap showing genes enriched in spliceosome in Tet1 deficient oocytes. (E) GO enrichment results showing genes downregulated or upregulated after Tet1 deficiency. X-axis represents the corrected p value (FDR) using Benjamini and Hochberg. (F) Pheatmap showing genes enriched in proteasomal protein catabolic process in Tet1 deficient oocytes. (G) Pheatmap showing genes enriched in DNA repair in Tet1 deficient oocytes.
Additionally, we analyzed the differential gene expression between young and old Tet1–/– oocytes to explore the aging effect on Tet1-deficiency. There were 1,117 upregulated and 1,216 downregulated genes in old Tet1–/– mouse oocytes compared with young Tet1–/– oocytes (Figure 4A). Comparatively, more genes were upregulated and downregulated in old WT oocytes (Figure 4B). By comparing the differential gene number between WT and Tet1–/– oocytes with mouse age, interestingly, aging affects gene expression more in WT than in Tet1–/– oocytes (Figure 4C). However, the mismatch repair was increased in old Tet1–/– oocytes compared with young Tet1–/– oocytes by KEGG enrichment analysis (Figure 4D), while apoptotic process was enriched in young Tet1–/– oocytes (Figure 4E). These results show that Tet1 deficiency may overlap some of the aging effect on gene expression. Alternatively, based on complex analysis above, Tet1 deficiency induces partial aging effects on oocyte function.
Figure 4. Differential gene expression between young and old Tet1–/– oocytes. (A) Scatter plot displaying differential gene expression between young and old Tet1–/– oocytes. (B) Venn plot showing the upregulated and downregulated genes between young and old WT oocytes, and young and old Tet1–/– oocytes. (C) Slope plot showing the change between WT and Tet1–/– oocytes. (D) KEGG enrichment results of differential genes between young and old Tet1–/– oocytes. X-axis represents the p-value. (E) GO enrichment results of differential genes between young and old Tet1–/– oocytes. X-axis represents the p-value.
Transposable elements (TEs) constitute approximately 40% of the mouse genome (Bourc’his and Bestor, 2004) and DNA methylation plays roles in repressing TEs (Wu and Zhang, 2010; Cardelli, 2018). Previously, loss of Tet1 was associated with increased methylation and reduced transcriptional activation of TEs in PGCs (Hill et al., 2018). We analyzed transcripts of TEs in oocytes of young and old mice based on our single cell RNA-seq data. Proportion of LINE and SINE was slightly higher in Tet1–/– oocytes compared with young WT oocytes (Figure 5A). Further analysis of different ERVs revealed that the proportion of ERVL-MaLR was higher in Tet1–/– than that in WT oocytes (Figure 5B). Moreover, we used z-score to compare the expression of ERVL-MaLR belonging to ERVL-Ma, and showed that it was increased in Tet1–/– oocytes (Figure 5C). To further analyze the difference between young Tet1–/– and WT oocytes, we performed differential analysis using Deseq2 and found that L1-mus1 and L1-mus3 transcripts were increased in young Tet1–/– oocytes compared with age-matched WT oocytes (Figure 5D). Based on previous study, only 1/10 L1 elements were full-length with lower coding for retrotranspositionally competent L1 elements (Deininger et al., 2017). We further analyzed the full-length L1 family and found that L1Md_F3 was notably highly expressed in Tet1–/– deficient oocytes, in contrast to WT oocytes (Figure 5E). These results together suggest that Tet1 deficiency also may alter transcription of TEs in oocytes.
Figure 5. Expression of transposon elements (TEs) of Tet1–/– and WT oocytes. (A) Barplot showing the proportion of TEs in young Tet1–/–, WT, old Tet1–/–, and WT oocytes. (B) Barplot displaying the proportion of ERVs in young Tet1–/–, WT, old Tet1–/–, and WT oocytes. (C) Z-score showing expression of ERVL-MaLR between young Tet1–/– and WT oocytes. Data are shown as mean ± SEM. **P < 0.01. (D) Volcano showing the differential TEs between young Tet1–/– and WT oocytes. Orange represented upregulated TEs compared with young WT oocytes. (E) Expression of Full-length L1 family in young Tet1–/– and WT oocytes, and old Tet1–/– and WT oocytes.
Here, we show that Tet1 deficiency leads to POF by influencing the quality of oocytes, including aberrant X-chromosome inactivation, and increased expression of L1-mus, as well as the oocyte number and follicle reserve. Previous elegant study demonstrated critical role of Tet1 in meiosis/PGCs during fetal development (Yamaguchi et al., 2012). Tet1 deficiency leads to aberrant meiosis, increased methylation on meiosis genes and subfertility in young mice (Yamaguchi et al., 2012). We extended the study by evaluating age effects on the fertility and oocytes in adult mice deficient in Tet1. We find that Tet1 deficient mice at reproductive middle-age already are completely infertile, consistent with POF or primary ovarian insufficiency (POI), which is characterized by the premature depletion of ovarian follicles and infertility at mid-reproductive age (Shah and Nagarajan, 2014; Delcour et al., 2019).
We show that Tet1 deficiency upregulates genes for Alzheimer’s diseases, but reduces expression of X-chromosome-linked genes, such as Fmr1. These defects might be linked in POF. Women with primary ovarian insufficiency (POI) or POF are at increased risk for non-communicable diseases such as, Alzheimer’s disease, cardiovascular disease, and osteoporosis (Harlow and Signorello, 2000; Shah and Nagarajan, 2014). POF has repeatedly been associated to X-chromosome deletions and haploinsufficiency of X-linked genes can be on the basis of POF (Ferreira et al., 2010). X chromosome inactivation could underlie POF linked to cognitive impairment in Alzheimer’s disease (Al-Hinti et al., 2007; Bretherick et al., 2007; Davey, 2013), regardless existing discrepancy on X chromosome in POF. Moreover, fragile X mental retardation type 1 (FMR1) gene premutation on the X chromosome have been frequently found in POF or POI (Jin et al., 2012; Shamilova et al., 2013; Bouali et al., 2015; Qin et al., 2015; Mila et al., 2018). FMR1 gene premutation allele’s carrier women have an increased risk for, or, susceptibility to POF (Ferreira et al., 2010; Pu et al., 2014). FMR1 gene premutation is the first single-gene cause of primary ovarian failure (Fragile X-associated primary ovarian insufficiency) and one of the most common causes of ataxia (fragile X-associated tremor/ataxia syndrome), and multiple additional phenotypes including neuropathy and neuropsychiatric alterations (Mila et al., 2018).
Moreover, loss of Tet1 increases DNA methylation as shown by increased expression of Dnmt3a and declined 5hmC levels in oocytes. Tet enzyme can regulate DNA demethylation as well as transcription (Wu and Zhang, 2017; Greenberg and Bourc’his, 2019). DNA methylation is one of the best-characterized epigenetic modifications and has been implicated in numerous biological processes, including transposable element silencing, genomic imprinting and X chromosome inactivation (Wu and Zhang, 2010; Dai et al., 2016). Oxidation of 5-methylcytosine by TET dioxygenases can lead to global demethylation (Dai et al., 2016; Wu and Zhang, 2017). About 60–80% of the CpG sites in the mammalian genome are modified by 5 mC (Smith and Meissner, 2013). TET-mediated oxidation has a locus-specific effect (Yamaguchi et al., 2012, 2013b; Dawlaty et al., 2013; Wu and Zhang, 2017). For instance, integrative Genomics Viewer reveals that the binding of Tet1 and 5 hmC levels on Fmr1 locus in WT is decreased in Tet1-knockdown ESCs by analysis of published data GEO datasets (GSE24841) (Williams et al., 2011), likely reducing expression of Fmr1. Indeed, blocking DNA methylation by 5-azacytidine (5-azaC or 5-azadC) has achieved a significant reactivation of FMR1 gene expression in fragile X syndrome cellular models (Bar-Nur et al., 2012). Also, L1 and ERVL-MaLR are aberrantly expressed in Tet1-deficient oocytes. L1 is highly expressed during mouse oocyte development and embryo cleavage stage (Jachowicz et al., 2017). But, elevated L1 expression correlates with fetal oocyte attrition, oocyte aneuploidy and embryonic lethality (Malki et al., 2014).
Additionally, Tet1 deficiency results in declined ubiquitination and autophagy but increased organelle fission in oocytes, and these defects presumably can be detrimental to clearance of damaged, or, senescent organelles in the cells. Impaired autophagy can lead to POF (Gawriluk et al., 2014; Delcour et al., 2019). Tet1 deficiency also decreases Ras signaling pathways. Appropriate activation of RAS signaling is crucial for directing normal follicle development (Fan et al., 2008).
Further experiments are required to investigate the underlying molecular mechanisms of how Tet1 regulates these signaling pathways associated with POF or POI. Also, the question remains as to whether Tet1 has similar function in human fertility and in POF patients. Nevertheless, our findings that Tet1 may involve in autophagy, X-chromosome activation and Alzheimer’s disease provide additional insights into molecular basis of POF.
RNA-seq data have been deposited in the GEO database under the accession number GSE142163.
The animal study was reviewed and approved by the Institutional Animal Care and Use Committee, Nankai University. Written informed consent was obtained from the owners for the participation of their animals in this study.
HW performed the experiments on fertility and histology, and prepared the manuscript. LinlLiu analyzed the RNA-seq data and prepared the manuscript. G_LX provided the materials, advised the project, and revised the manuscript. LinLiu conceived the study, designed experiments, and wrote the manuscript. All authors contributed to the article and approved the submitted version.
This work was supported by the National Natural Science Foundation of China (31571546 and 91749129).
The authors declare that the research was conducted in the absence of any commercial or financial relationships that could be construed as a potential conflict of interest.
We thank Wei Deng, Qin Xu, Guian Huang, Jiao Yang, and Haiying Wang for help in the experiments.
The Supplementary Material for this article can be found online at: https://www.frontiersin.org/articles/10.3389/fcell.2021.644135/full#supplementary-material
Supplementary Figure 1 | Loss of Tet1 in female mice reduces 5 hmC levels in oocytes. (A) PCR genotyping of Tet1 mutant mice. Primer sequences are listed in Supplementary Table 2. (B) Table summarizing the litter size and Mendelian ratio of Tet1–/– mice. (C) Tet1 mRNA expression level in ovaries of young mice by qPCR analysis. (D) Tet1 protein expression level in E3.5 blastocysts by immunofluorescence. Scale bar, 10 μm. (E) Representative images of primordial follicles from young WT mouse ovary. (F) Representative images of primordial follicles stained with 5 hmC antibody. Dashed line indicates the primordial oocyte. Scale bar, 10 μm. Bottom panel, Relative 5 hmC level in primordial oocytes from young mice. n = 21 oocytes counted for each group. Data represents mean ± SEM. ***P < 0.001.
Supplementary Figure 2 | Distribution of differentially expressed genes across chromosomes. (A) Scatter plot illustrating the chromosomal distribution of differential genes Between young WT and Tet1–/– oocytes. (B) Protein-protein interactome network analysis using Metascape (http://metascape.org/gp/index.html#) showing the potential interaction of Fmr1, Hnrnpu and Pabpc1 and with other proteins.
Supplementary Figure 3 | Coverage of Smart-seq2 of oocytes and comparison with transcriptome data on PGCs from Yamaguchi et al., 2012. (A) Boxplot displaying number of genes sequenced in oocytes. (B) Venn plot showing the overlapped, downregulated genes between oocytes based on our data and PGCs from Yamaguchi et al., 2012. (C) Venn plot showing the overlapped, downregulated genes between oocytes and PGCss associated with DMRs, based on Yamaguchi et al., 2012.
Al-Hinti, J. T., Nagan, N., and Harik, S. I. (2007). Fragile X premutation in a woman with cognitive impairment, tremor, and history of premature ovarian failure. Alzheimer Dis. Assoc. Disord. 21, 262–264. doi: 10.1097/wad.0b013e31811ec130
Bacher, R., Chu, L. F., Leng, N., Gasch, A. P., Thomson, J. A., Stewart, R. M., et al. (2017). SCnorm: robust normalization of single-cell RNA-seq data. Nat. Methods 14, 584–586. doi: 10.1038/nmeth.4263
Bar-Nur, O., Caspi, I., and Benvenisty, N. (2012). Molecular analysis of FMR1 reactivation in fragile-X induced pluripotent stem cells and their neuronal derivatives. J. Mol. Cell. Biol. 4, 180–183. doi: 10.1093/jmcb/mjs007
Bouali, N., Hmida, D., Mougou, S., Bouligand, J., Lakhal, B., Dimessi, S., et al. (2015). Analysis of FMR1 gene premutation and X chromosome cytogenetic abnormalities in 100 Tunisian patients presenting premature ovarian failure. Ann. Endocrinol. 76, 671–678. doi: 10.1016/j.ando.2015.10.001
Bourc’his, D., and Bestor, T. H. (2004). Meiotic catastrophe and retrotransposon reactivation in male germ cells lacking Dnmt3L. Nature 431, 96–99. doi: 10.1038/nature02886
Bretherick, K. L., Metzger, D. L., Chanoine, J. P., Panagiotopoulos, C., Watson, S. K., Lam, W. L., et al. (2007). Skewed X-chromosome inactivation is associated with primary but not secondary ovarian failure. Am. J. Med. Genet. A 143A, 945–951. doi: 10.1002/ajmg.a.31679
Bristol-Gould, S. K., Kreeger, P. K., Selkirk, C. G., Kilen, S. M., Mayo, K. E., Shea, L. D., et al. (2006). Fate of the initial follicle pool: empirical and mathematical evidence supporting its sufficiency for adult fertility. Dev. Biol. 298, 149–154. doi: 10.1016/j.ydbio.2006.06.023
Broekmans, F. J., Knauff, E. A., te Velde, E. R., Macklon, N. S., and Fauser, B. C. (2007). Female reproductive ageing: current knowledge and future trends. Trends Endocrinol. Metab. 18, 58–65. doi: 10.1016/j.tem.2007.01.004
Broekmans, F. J., Soules, M. R., and Fauser, B. C. (2009). Ovarian aging: mechanisms and clinical consequences. Endocr. Rev. 30, 465–493. doi: 10.1210/er.2009-0006
Bult, C. J., Blake, J. A., Smith, C. L., Kadin, J. A., Richardson, J. E., and Mouse Genome Database Group (2019). Mouse Genome Database (MGD) 2019. Nucleic Acids Res. 47, D801–D806.
Cardelli, M. (2018). The epigenetic alterations of endogenous retroelements in aging. Mech. Ageing Dev. 174, 30–46. doi: 10.1016/j.mad.2018.02.002
Chen, D., and Kerr, C. (2019). The epigenetics of stem cell aging comes of age. Trends Cell Biol. 29, 563–568. doi: 10.1016/j.tcb.2019.03.006
Cole, J. J., Robertson, N. A., Rather, M. I., Thomson, J. P., McBryan, T., Sproul, D., et al. (2017). Diverse interventions that extend mouse lifespan suppress shared age-associated epigenetic changes at critical gene regulatory regions. Genome Biol. 18:58.
Dai, H. Q., Wang, B. A., Yang, L., Chen, J. J., Zhu, G. C., Sun, M. L., et al. (2016). TET-mediated DNA demethylation controls gastrulation by regulating Lefty-Nodal signalling. Nature 538, 528–532. doi: 10.1038/nature20095
Davey, D. A. (2013). Alzheimer’s disease, dementia, mild cognitive impairment and the menopause: a ‘window of opportunity’? Womens Health 9, 279–290. doi: 10.2217/whe.13.22
Dawlaty, M. M., Breiling, A., Le, T., Raddatz, G., Barrasa, M. I., Cheng, A. W., et al. (2013). Combined deficiency of Tet1 and Tet2 causes epigenetic abnormalities but is compatible with postnatal development. Dev. Cell 24, 310–323. doi: 10.1016/j.devcel.2012.12.015
Dawlaty, M. M., Ganz, K., Powell, B. E., Hu, Y. C., Markoulaki, S., Cheng, A. W., et al. (2011). Tet1 is dispensable for maintaining pluripotency and its loss is compatible with embryonic and postnatal development. Cell Stem Cell 9, 166–175. doi: 10.1016/j.stem.2011.07.010
Deininger, P., Morales, M. E., White, T. B., Baddoo, M., Hedges, D. J., Servant, G., et al. (2017). A comprehensive approach to expression of L1 loci. Nucleic Acids Res. 45:e31. doi: 10.1093/nar/gkw1067
Delcour, C., Amazit, L., Patino, L. C., Magnin, F., Fagart, J., Delemer, B., et al. (2019). ATG7 and ATG9A loss-of-function variants trigger autophagy impairment and ovarian failure. Genet. Med. 21, 930–938. doi: 10.1038/s41436-018-0287-y
Djahanbakhch, O., Ezzati, M., and Zosmer, A. (2007). Reproductive ageing in women. J. Pathol. 211, 219–231. doi: 10.1002/path.2108
Dorfman, M. D., Garcia-Rudaz, C., Alderman, Z., Kerr, B., Lomniczi, A., Dissen, G. A., et al. (2014). Loss of Ntrk2/Kiss1r signaling in oocytes causes premature ovarian failure. Endocrinology 155, 3098–3111. doi: 10.1210/en.2014-1111
Faddy, M. J. (2000). Follicle dynamics during ovarian ageing. Mol. Cell. Endocrinol. 163, 43–48. doi: 10.1016/s0303-7207(99)00238-5
Fan, H. Y., Shimada, M., Liu, Z., Cahill, N., Noma, N., Wu, Y., et al. (2008). Selective expression of KrasG12D in granulosa cells of the mouse ovary causes defects in follicle development and ovulation. Development 135, 2127–2137. doi: 10.1242/dev.020560
Ferreira, S. I., Matoso, E., Pinto, M., Almeida, J., Liehr, T., Melo, J. B., et al. (2010). X-chromosome terminal deletion in a female with premature ovarian failure: haploinsufficiency of X-linked genes as a possible explanation. Mol. Cytogenet. 3:14. doi: 10.1186/1755-8166-3-14
Gawriluk, T. R., Ko, C., Hong, X., and Christenson, L. K. (2014). Beclin-1 deficiency in the murine ovary results in the reduction of progesterone production to promote preterm labor. Proc. Natl. Acad. Sci. U.S.A. 111, E4194–E4203.
Greenberg, M. V. C., and Bourc’his, D. (2019). The diverse roles of DNA methylation in mammalian development and disease. Nat. Rev. Mol. Cell Biol. 20, 590–607. doi: 10.1038/s41580-019-0159-6
Hannum, G., Guinney, J., Zhao, L., Zhang, L., Hughes, G., Sadda, S., et al. (2013). Genome-wide methylation profiles reveal quantitative views of human aging rates. Mol. Cell. 49, 359–367. doi: 10.1016/j.molcel.2012.10.016
Harlow, B. L., and Signorello, L. B. (2000). Factors associated with early menopause. Maturitas 35, 3–9. doi: 10.1016/s0378-5122(00)00092-x
Hill, P. W. S., Leitch, H. G., Requena, C. E., Sun, Z., Amouroux, R., Roman-Trufero, M., et al. (2018). Epigenetic reprogramming enables the transition from primordial germ cell to gonocyte. Nature 555, 392–396. doi: 10.1038/nature25964
Ito, S., D’Alessio, A. C., Taranova, O. V., Hong, K., Sowers, L. C., and Zhang, Y. (2010). Role of Tet proteins in 5mC to 5hmC conversion, ES-cell self-renewal and inner cell mass specification. Nature 466, 1129–1133. doi: 10.1038/nature09303
Jachowicz, J. W., Bing, X., Pontabry, J., Boskovic, A., Rando, O. J., and Torres-Padilla, M. E. (2017). LINE-1 activation after fertilization regulates global chromatin accessibility in the early mouse embryo. Nat. Genet. 49, 1502–1510. doi: 10.1038/ng.3945
Jin, M., Yu, Y., and Huang, H. (2012). An update on primary ovarian insufficiency. Sci. China Life Sci. 55, 677–686.
Kaul, T., Morales, M. E., Smither, E., Baddoo, M., Belancio, V. P., and Deininger, P. (2019). RNA next-generation sequencing and a bioinformatics pipeline to identify expressed LINE-1s at the locus-specific level. J. Vis. Exp. 147: 59771.
Kim, D., Langmead, B., and Salzberg, S. L. (2015). HISAT: a fast spliced aligner with low memory requirements. Nat. Methods 12, 357–360. doi: 10.1038/nmeth.3317
Kim, D., Pertea, G., Trapnell, C., Pimentel, H., Kelley, R., and Salzberg, S. L. (2013). TopHat2: accurate alignment of transcriptomes in the presence of insertions, deletions and gene fusions. Genome Biol. 14:R36.
Ko, M., Bandukwala, H. S., An, J., Lamperti, E. D., Thompson, E. C., Hastie, R., et al. (2011). Ten-Eleven-Translocation 2 (TET2) negatively regulates homeostasis and differentiation of hematopoietic stem cells in mice. Proc. Natl. Acad. Sci. U.S.A. 108, 14566–14571. doi: 10.1073/pnas.1112317108
Koh, K. P., Yabuuchi, A., Rao, S., Huang, Y., Cunniff, K., Nardone, J., et al. (2011). Tet1 and Tet2 regulate 5-hydroxymethylcytosine production and cell lineage specification in mouse embryonic stem cells. Cell Stem Cell 8, 200–213. doi: 10.1016/j.stem.2011.01.008
Lawson, R., El-Toukhy, T., Kassab, A., Taylor, A., Braude, P., Parsons, J., et al. (2003). Poor response to ovulation induction is a stronger predictor of early menopause than elevated basal FSH: a life table analysis. Hum. Reprod. 18, 527–533. doi: 10.1093/humrep/deg101
Liao, Y., Smyth, G. K., and Shi, W. (2014). featureCounts: an efficient general purpose program for assigning sequence reads to genomic features. Bioinformatics 30, 923–930. doi: 10.1093/bioinformatics/btt656
Liu, M., Yin, Y., Ye, X., Zeng, M., Zhao, Q., Keefe, D. L., et al. (2013). Resveratrol protects against age-associated infertility in mice. Hum. Reprod. 28, 707–717.
Lopez-Otin, C., Blasco, M. A., Partridge, L., Serrano, M., and Kroemer, G. (2013). The hallmarks of aging. Cell 153, 1194–1217.
Love, M. I., Huber, W., and Anders, S. (2014). Moderated estimation of fold change and dispersion for RNA-seq data with DESeq2. Genome Biol. 15:550.
Maegawa, S., Hinkal, G., Kim, H. S., Shen, L., Zhang, L., Zhang, J., et al. (2010). Widespread and tissue specific age-related DNA methylation changes in mice. Genome Res. 20, 332–340. doi: 10.1101/gr.096826.109
Malki, S., van der Heijden, G. W., O’Donnell, K. A., Martin, S. L., and Bortvin, A. (2014). A role for retrotransposon LINE-1 in fetal oocyte attrition in mice. Dev. Cell 29, 521–533. doi: 10.1016/j.devcel.2014.04.027
Mila, M., Alvarez-Mora, M. I., Madrigal, I., and Rodriguez-Revenga, L. (2018). Fragile X syndrome: an overview and update of the FMR1 gene. Clin. Genet. 93, 197–205. doi: 10.1111/cge.13075
Myers, M., Britt, K. L., Wreford, N. G., Ebling, F. J., and Kerr, J. B. (2004). Methods for quantifying follicular numbers within the mouse ovary. Reproduction 127, 569–580. doi: 10.1530/rep.1.00095
Notarianni, E. (2011). Reinterpretation of evidence advanced for neo-oogenesis in mammals, in terms of a finite oocyte reserve. J. Ovarian Res. 4:1. doi: 10.1186/1757-2215-4-1
Picelli, S., Faridani, O. R., Bjorklund, A. K., Winberg, G., Sagasser, S., and Sandberg, R. (2014). Full-length RNA-seq from single cells using Smart-seq2. Nat. Protoc. 9, 171–181. doi: 10.1038/nprot.2014.006
Pu, D., Xing, Y., Gao, Y., Gu, L., and Wu, J. (2014). Gene variation and premature ovarian failure: a meta-analysis. Eur. J. Obstet. Gynecol. Reprod. Biol. 182, 226–237. doi: 10.1016/j.ejogrb.2014.09.036
Qin, Y., Jiao, X., Simpson, J. L., and Chen, Z. J. (2015). Genetics of primary ovarian insufficiency: new developments and opportunities. Hum. Reprod. Update 21, 787–808. doi: 10.1093/humupd/dmv036
Rando, T. A., and Chang, H. Y. (2012). Aging, rejuvenation, and epigenetic reprogramming: resetting the aging clock. Cell 148, 46–57. doi: 10.1016/j.cell.2012.01.003
Shah, D., and Nagarajan, N. (2014). Premature menopause - meeting the needs. Post Reprod. Health 20, 62–68. doi: 10.1177/2053369114531909
Shamilova, N. N., Marchenko, L. A., Dolgushina, N. V., Zaletaev, D. V., and Sukhikh, G. T. (2013). The role of genetic and autoimmune factors in premature ovarian failure. J. Assist. Reprod. Genet. 30, 617–622. doi: 10.1007/s10815-013-9974-4
Smith, Z. D., and Meissner, A. (2013). DNA methylation: roles in mammalian development. Nat. Rev. Genet. 14, 204–220. doi: 10.1038/nrg3354
Stubbs, T. M., Bonder, M. J., Stark, A. K., Krueger, F., Team, B. I. A. C., von Meyenn, F., et al. (2017). Multi-tissue DNA methylation age predictor in mouse. Genome Biol. 18:68.
Tatone, C., Amicarelli, F., Carbone, M. C., Monteleone, P., Caserta, D., Marci, R., et al. (2008). Cellular and molecular aspects of ovarian follicle ageing. Hum. Reprod. Update 14, 131–142. doi: 10.1093/humupd/dmm048
van Rooij, I. A., Broekmans, F. J., Scheffer, G. J., Looman, C. W., Habbema, J. D., de Jong, F. H., et al. (2005). Serum antimullerian hormone levels best reflect the reproductive decline with age in normal women with proven fertility: a longitudinal study. Fertil. Steril. 83, 979–987. doi: 10.1016/j.fertnstert.2004.11.029
Wang, H., Liu, L., Gou, M., Huang, G., Tian, C., Yang, J., et al. (2020). Roles of Tet2 in meiosis, fertility and reproductive aging. Protein Cell [Epub ahead of print].
Williams, K., Christensen, J., Pedersen, M. T., Johansen, J. V., Cloos, P. A., Rappsilber, J., et al. (2011). TET1 and hydroxymethylcytosine in transcription and DNA methylation fidelity. Nature 473, 343–348. doi: 10.1038/nature10066
Wu, S. C., and Zhang, Y. (2010). Active DNA demethylation: many roads lead to Rome. Nat. Rev. Mol. Cell Biol. 11, 607–620. doi: 10.1038/nrm2950
Wu, X., and Zhang, Y. (2017). TET-mediated active DNA demethylation: mechanism, function and beyond. Nat. Rev. Genet. 18, 517–534. doi: 10.1038/nrg.2017.33
Xie, C., Mao, X., Huang, J., Ding, Y., Wu, J., Dong, S., et al. (2011). KOBAS 2.0: a web server for annotation and identification of enriched pathways and diseases. Nucleic Acids Res. 39, W316–W322.
Yamaguchi, S., Hong, K., Liu, R., Inoue, A., Shen, L., Zhang, K., et al. (2013a). Dynamics of 5-methylcytosine and 5-hydroxymethylcytosine during germ cell reprogramming. Cell Res. 23, 329–339. doi: 10.1038/cr.2013.22
Yamaguchi, S., Shen, L., Liu, Y., Sendler, D., and Zhang, Y. (2013b). Role of Tet1 in erasure of genomic imprinting. Nature 504, 460–464. doi: 10.1038/nature12805
Yamaguchi, S., Hong, K., Liu, R., Shen, L., Inoue, A., Diep, D., et al. (2012). Tet1 controls meiosis by regulating meiotic gene expression. Nature 492, 443–447. doi: 10.1038/nature11709
Yu, G., Wang, L. G., Han, Y., and He, Q. Y. (2012). clusterProfiler: an R package for comparing biological themes among gene clusters. OMICS 16, 284–287. doi: 10.1089/omi.2011.0118
Zhang, H., Zheng, W., Shen, Y., Adhikari, D., Ueno, H., and Liu, K. (2012). Experimental evidence showing that no mitotically active female germline progenitors exist in postnatal mouse ovaries. Proc. Natl. Acad. Sci. U.S.A. 109, 12580–12585. doi: 10.1073/pnas.1206600109
Zhang, R. R., Cui, Q. Y., Murai, K., Lim, Y. C., Smith, Z. D., and Jin, S. (2013). Tet1 regulates adult hippocampal neurogenesis and cognition. Cell Stem Cell 13, 237–245. doi: 10.1016/j.stem.2013.05.006
Keywords: Tet1, aging, epigenetics, oocyte, premature ovarian failure
Citation: Liu L, Wang H, Xu G_L and Liu L (2021) Tet1 Deficiency Leads to Premature Ovarian Failure. Front. Cell Dev. Biol. 9:644135. doi: 10.3389/fcell.2021.644135
Received: 20 December 2020; Accepted: 26 February 2021;
Published: 23 March 2021.
Edited by:
Francesca Elizabeth Duncan, Northwestern University, United StatesReviewed by:
Michael Klutstein, Hebrew University of Jerusalem, IsraelCopyright © 2021 Liu, Wang, Xu and Liu. This is an open-access article distributed under the terms of the Creative Commons Attribution License (CC BY). The use, distribution or reproduction in other forums is permitted, provided the original author(s) and the copyright owner(s) are credited and that the original publication in this journal is cited, in accordance with accepted academic practice. No use, distribution or reproduction is permitted which does not comply with these terms.
*Correspondence: Lin Liu, bGl1bGluQG5hbmthaS5lZHUuY24=
†These authors have contributed equally to this work
Disclaimer: All claims expressed in this article are solely those of the authors and do not necessarily represent those of their affiliated organizations, or those of the publisher, the editors and the reviewers. Any product that may be evaluated in this article or claim that may be made by its manufacturer is not guaranteed or endorsed by the publisher.
Research integrity at Frontiers
Learn more about the work of our research integrity team to safeguard the quality of each article we publish.