- Institute of Bioorganic Chemistry, Polish Academy of Sciences, Poznań, Poland
Polyglutamine (PolyQ) diseases are neurodegenerative disorders caused by the CAG repeat expansion mutation in affected genes resulting in toxic proteins containing a long chain of glutamines. There are nine PolyQ diseases: Huntington’s disease (HD), spinocerebellar ataxias (types 1, 2, 3, 6, 7, and 17), dentatorubral-pallidoluysian atrophy (DRPLA), and spinal bulbar muscular atrophy (SBMA). In general, longer CAG expansions and longer glutamine tracts lead to earlier disease presentations in PolyQ patients. Rarely, cases of extremely long expansions are identified for PolyQ diseases, and they consistently lead to juvenile or sometimes very severe infantile-onset polyQ syndromes. In apparent contrast to the very long CAG tracts, shorter CAGs and PolyQs in proteins seems to be the evolutionary factor enhancing human cognition. Therefore, polyQ tracts in proteins can be modifiers of brain development and disease drivers, which contribute neurodevelopmental phenotypes in juvenile- and adult-onset PolyQ diseases. Therefore we performed a bioinformatics review of published RNAseq polyQ expression data resulting from the presence of polyQ genes in search of neurodevelopmental expression patterns and comparison between diseases. The expression data were collected from cell types reflecting stages of development such as iPSC, neuronal stem cell, neurons, but also the adult patients and models for PolyQ disease. In addition, we extended our bioinformatic transcriptomic analysis by proteomics data. We identified a group of 13 commonly downregulated genes and proteins in HD mouse models. Our comparative bioinformatic review highlighted several (neuro)developmental pathways and genes identified within PolyQ diseases and mouse models responsible for neural growth, synaptogenesis, and synaptic plasticity.
Early Brain Development in Health and PolyQ Disease
Normal brain development consists of cellular processes such as cell division, cell migration, cell differentiation, maturation, synaptogenesis, and apoptosis, which are precisely orchestrated by a molecular network of signaling pathways. Such orchestration is crucial for the correct generation of cellular layers, specialized neural regions, and the generation of complex neuronal wiring between brain structures. In brief, during the formation of the neural tube (neurulation) in the embryo, the neuroepithelial cells (NECs) perform symmetric cell divisions producing progenitors of different brain regions (Paridaen and Huttner, 2014). Pax6 and Emx2 signaling molecules expressed in opposing gradients from the anterior to posterior regions of the proliferative zone function as a primitive blueprint for the dividing NECs to give rise to the early structures of the forebrain, midbrain, and hindbrain (Stiles and Jernigan, 2010; Gibb and Kolb, 2018). Among others, neurulation gives rise to neural progenitors, neural crest, sensory placodes, and epidermis, all ectodermal derivatives (Haremaki et al., 2019). The appearance of these four lineages results from complex morphogenetic processes and several signaling activities, such as TGF-β inhibition and BMP4, Wnt, and FGF signaling pathways. The signaling molecules are represented already in non-linage committed iPSC from Huntington’s disease juvenile patients and mouse models, which show a range of molecular phenotypes such as MAPK, Wnt, and p53 pathways (Szlachcic et al., 2015, 2017).
Early human neurulation can be recapitulated in vitro by self-organizing neuruloids, containing cell populations present at the stage of neural tube closure in human development (days 21–25 post-fertilization; Haremaki et al., 2019). Interestingly such neuruloids generated from Huntington’s disease hESC demonstrated impaired neurogenesis resulting in aberrant rosette formation. In detail, HD 56Q neuruloids showed altered levels of Wnt/PCP pathway downregulation (for example, WNT5B, and RSPO3 specific in neuroepithelium) and RHOB and RAB5C in the neural crest. In addition, decreased expression of cytoskeleton-associated genes and actin-myosin contraction (EVL, MID1, RHOQ, and TMEM47) could be observed and hint toward an impairment in the actin-mediated tissue organization mechanism during neurulation (Haremaki et al., 2019). In another recent study, one-third of gene changes in RNA-seq analysis on HD patient-derived iPSCs were involved in pathways regulating neuronal development and maturation. When these dysregulated genes were mapped to stages of mouse striatal development, the profiles aligned mainly with earlier embryonic stages of neuronal differentiation (The HD iPSC Consortium, 2017). Moreover, sensory-motor network connectivity changes can be observed in the brains of HD patients, hinting at an effect of this PolyQ disease on brain connectivity (Pini et al., 2020).
During brain development, in a process called interkinetic nuclear migration coupled to the cell cycle, neural progenitors keep the balance between the cell renewal of progenitors and their differentiation. Their role is to control when and how many apical progenitor nuclei are exposed to proliferative versus neurogenic signals. Apical progenitors maintain their polarity through endocytosis and trafficking of glycans from the Golgi apparatus to the plasma membrane at the apical endfeet (Arai and Taverna, 2017). Interestingly, mislocalized expression of mHTT hinders both endosomal trafficking in apical progenitors, as well as the normal progression of cell cycle stages. Leading to a shift toward more neural differentiation and away from proliferation (Barnat et al., 2020). Afterward, neuroepithelial cells start expressing glial genes and thereby begin a differentiation process into radial glial cells (RGCs). At this stage, cell migration starts to play a decisive role. Neuronal cells originating from the ventricular and subventricular zones start migrating outward in a radial fashion, using the RGCs as guideposts. Some subsets of RDGs eventually differentiate into intermediate, immature, and finally into mature neurons or astrocytes (Franco and Müller, 2013; Gibb and Kolb, 2018). Other cell populations migrate to the cortex during later developmental stages and include the microglia, which mostly use vessels for guidance into the forebrain. Recent reports point toward glia, particularly microglia, as essential players for cortical morphogenesis via regulation of brain wiring and interneuronal migration in the cortical wall (Silva et al., 2019).
Over time, successive layers of the cortical mantle form, and the progenitor cells are becoming more restricted in the cell types that they can construct. Furthermore, in this cellular maturation process, neural cells start to extend dendrites and an axon to form connections with other cells and become an integral part of a communication network (Gibb and Kolb, 2018).
In the prenatal stage of life, the further development of the brain also starts to depend on degenerative processes such as programmed cell death or apoptosis. These processes are initiated to remove the brain cells which have failed to make connections or have underutilized connections (Chan et al., 2002). Also, the underused synapses are eliminated in a process called synaptic pruning. In these stages of brain development, a transcriptional repressor complex of Ataxin1 and Capicua (ATXN1-CIC) regulates cell lineage specification and is involved in the regulation of cell proliferation (Ahmad et al., 2019). Loss of the ATXN1-CIC complex may have severe neurodevelopmental consequences. Conditional knockout of either Atxn1-Atxn1l or Cic in mice leads to a decrease of cortical thickness, hyperactivity and memory deficits (Lu et al., 2017). Indeed, loss or reduction of functional ATXN1 has been observed in patients with autism spectrum disorder and attention-deficit/hyperactivity disorder (Celestino-Soper et al., 2012; Di Benedetto et al., 2013), suggesting that loss of ATXN1-CIC complexes causes a spectrum of neurobehavioral phenotypes (Lu et al., 2017). Expanded CAG tracts in ATXN1 have been shown to stimulate the proliferation of postnatal cerebellar stem cells in SCA1 mice, which tend to differentiate into GABAergic inhibitory interneurons rather than astrocytes (Edamakanti et al., 2018). These hyperproliferating cells lead to a significantly increased number of GABAergic inhibitory interneuron synaptic connections, which in turn disrupt the proper cerebellar Purkinje cell function (Edamakanti et al., 2018). On the other hand, SCA2 patient fibroblast cells exhibit higher levels of caspase-8- and caspase-9-mediated apoptotic activation than those of healthy controls, which contributes to the pathophysiology of SCA2 (Wardman et al., 2020). Also, the normal function of atrophin-1 and atrophin-2 proteins are related to the development and may be associated with regulation of cell polarity and transcriptional control of progenitors, which was reviewed previously (Shen and Peterson, 2009; Mannervik, 2014). Knockdown of Atn1 in neuronal progenitor cells (NPCs) in a rat led to severe aberrations in brain development. The study also highlighted ATN1 role as a direct target of the lysine-specific histone demethylase 1A (LSD1). LSD1 is known to have crucial developmental roles such as cortical neuronal migration or adult NPC proliferation (Zhang et al., 2014). Similarly, TATA Binding protein as part of the TFIID complexes may control promoter elements that can regulate developmental transcription (Ohler and Wassarman, 2010). As a general transcription factor, TBP is, directly and indirectly involved in numerous biological pathways. Studies confirmed many cellular processes impaired by mutant TBP via either gain of function or loss of function mechanisms, such as Notch signaling, TrkA signaling, Chaperone system, ER stress response, and muscle function (Yang et al., 2016).
Combined with the previously mentioned roles of HTT, ATXN1, ATXN2, ATXN3, ATN1, and TBP in transcription, translation, RNA metabolism, and ubiquitin-dependent protein quality control processes, a case can be made for the adverse effect of CAG tract extension on normal gene expression and protein regulation during neural development. Therefore, it can be proposed that other late-onset degenerative diseases may also be rooted in subtle developmental derailments. Deregulation of genes involved in cell migration, cell differentiation, maturation, synaptogenesis, and apoptosis can lead to severe neurodevelopmental disorders and may also contribute to the disease pathology of PolyQ diseases.
PolyQ Diseases and Juvenile Cases
Polyglutamine (PolyQ) diseases are neurodegenerative disorders caused by expansion mutations giving rise to abnormally long CAG tri-nucleotide repeat tracts in affected, otherwise unrelated genes. PolyQ disorders are dominantly inherited and autosomal, except for SBMA, which is X-linked. The expanded polyQ repeats disturb the function of the proteins encoded by the genes with CAG expansion, leading to loss or gain of function (Lim et al., 2008). To date, nine PolyQ diseases were identified; namely Huntington’s disease (HD), spinocerebellar ataxia (SCA) types 1, 2, 3, 6, 7, and 17, dentatorubral-pallidoluysian atrophy (DRPLA), and spinal bulbar muscular atrophy (SBMA) (Zoghbi and Orr, 2009).
Several PolyQ diseases may occur in younger patients, and in such cases, symptom presentation in juvenile disease usually differs from the adult form. Although the juvenile and infantile forms make up a minority of instances, the early onset and polyQ protein domains, usually much longer than in the adult forms, hint at the developmental nature of these cases. Since the etiology of the diseases is genetic and more defined, they may help to better understand the brain development in health and disease. The first aim of this work is to obtain a broader literature overview of the juvenile and infantile PolyQ disease cases, with very long CAG repeats, in the context of early brain development. Since brain development is primarily related to the forming of new cell populations, differentiation, and wiring of the brain, we also looked at what is known about these processes in the context of juvenile polyQ cases. In the second part of the work, we performed a bioinformatics analysis of RNAseq and proteomics data from polyQ patients and models in search of neurodevelopmental expression patterns and comparison between diseases. The expression data were collected from cell types reflecting stages of development such as iPSC, neuronal stem cell (NSCs), neural precursor cells (NPCs), and neurons, but also the adult patients and models for PolyQ disease. In addition, thanks to a broader selection of transcriptomic data in mice containing longer CAG tracts, we were able to compare gene expression profiles between different PolyQ diseases. Still, the bias toward HD in this work results from the available data sources. However, another aim of our work is the focus on juvenile cases of polyQ disorders other than HD, possible neurodevelopmental signs in the diseases, and what we could still learn from the juvenile forms about diseased brain development.
Juvenile and Infantile Huntington’s Disease
In HD, the CAG expansion mutation is located in the Huntingtin (HTT) gene (The Huntington’s Disease Collaborative Research Group, 1993), which is crucial for neural development [reviewed in Saudou and Humbert (2016) and Wiatr et al. (2018)]. The adult HD is a neurodegenerative choreic movement disorder characterized by motor disturbance, cognitive loss, and psychiatric manifestations that typically starts in the third to fifth decade of life and gradually worsens over the course of 10 to 20 years until death. Adult HD is characteristic for individuals who usually harbor over 39 trinucleotide repeats in HTT, but rarely more than 60 (Martin and Gusella, 1986; Latimer et al., 2017). The juvenile form of HD (Juvenile onset Huntington’s disease; JOHD) is defined as disease onset before the age of 20 with the number of CAG repeats between 60 (Quarrell et al., 2013) and 89 (Nance and Myers, 2001; Ribaï et al., 2007), and infantile HD with very rapid onset with number of CAG repeats above 90 and more (Fusilli et al., 2018; Stout, 2018). JOHD is also marked by a more rapid disease progression, leading to an earlier death (Fusilli et al., 2018). In JOHD, the symptoms are typically seizures, rigidity, and severe cognitive dysfunction (Nance and Myers, 2001; Vargas et al., 2003; Squitieri et al., 2006; Ribaï et al., 2007). In cases where the onset is very early (before 10 years of age, sometimes also referred to as “infantile-” or “ultra-juvenile HD”), epilepsy is also frequent (Barbeau, 1970). One of the youngest onset of JOHD and also one of the most severe presentations which have been described to date was a girl who had healthy development until 18 months of age and later at the age of 3,5 years, showed marked cerebellar atrophy. The patient was diagnosed to have 265 triplet repeats on the mutant HTT allele and 14 on the other (Milunsky et al., 2003). Other reports have described frequent speech difficulties as early symptoms before motor problems arise (Yoon et al., 2006; Sakazume et al., 2009). Behavioral problems, such as aggression, irritability, and hyperactivity, which are often reported signs of disturbed brain development, were also reported for juvenile HD (Yoon et al., 2006). In both juvenile- and adult-onset HD, the most affected cell types in the brain are striatal neurons (Tereshchenko et al., 2019). MRI data from JOHD cases show mostly cerebellar atrophy. The most substantial reduction in brain volume is observed in the caudate, putamen, as well as in globus pallidus and thalamus. Amygdala, hippocampus, and brainstem are slightly enlarged in HD patients (Hedjoudje et al., 2018). The significant difference between HD adults and children is seen in the cerebral cortex, which is mainly unaffected in children. Histopathological findings (Latimer et al., 2017) showed mild to moderate neuron loss in the brain tissue of adult-onset patients, while no significant loss of neocortical neurons was observed in JOHD. However, in JOHD patients, a significant neostriatal neuron loss and associated astrogliosis in the striatum were observed. In both disease onsets, HTT positive intranuclear and cytoplasmic neuronal inclusions can be found in the cerebral and striatum cortex.
Early Onset in PolyQ Spinocerebellar Ataxias
Most severe cases of juvenile or infantile-onset were reported for SCA2 (ATXN2 gene; ataxin-2 protein), SCA7 (ATXN7; Ataxin-7 protein), SCA17 (TBP gene; TATA Binding protein), and DRPLA (ATN1 gene; atrophin-1 protein). Juvenile-onsets were also reported for SCA3/MJD with more severe presentation compared to adult forms. One of the reasons for the occurrence of very severe developmental signs may be the function of ATXN2, ATXN7, TBP, and ATN1, which can be summarized as a very pleiotropic and broad influence on transcriptional regulation. The function of the genes, including their impact on transcription, has been well-reviewed previously (Shen and Peterson, 2009; Yang et al., 2016; Lee J. et al., 2018; Niewiadomska-Cimicka and Trottier, 2019). In SCA1 (ATXN1 gene; Ataxin-1 protein), SCA3/MJD (ATXN3 gene; ataxin-3), and SCA6 (CACNA1A gene; α1A subunit of the voltage-gated P/Q type channel), the cases with the earliest reported onset were mostly showing signs shortly before adolescence.
In SCA2, the CAG repeat in affected adults varies in length from 34 to 59 CAG repeats. The expansion mutation in ATXN2 in infantile cases can be very severe, reaching the range of 124 and 750 CAGs, and the range between 62 and 92 defines onset in early childhood. Typically, SCA2 presents with progressive involuntary movements of the limbs, sensorimotor neuropathy, and slowed eye movements. The abnormal eye movements and myoclonic jerks are generally the first symptoms seen in infantile and early childhood cases, with the onset of disease as early as 2 months of age (Moretti et al., 2004; Vinther-Jensen et al., 2013; Singh et al., 2014; Sánchez-Corona et al., 2020). Besides these, pigmentary retinopathy, seizures, dysphagia, and early death are unfortunately also standard features of juvenile SCA2 (Babovic-Vuksanovic et al., 1998; Mao et al., 2002). The MRI of children with very early-onset SCA2 (age from 7 to 17 months) revealed enlarged lateral ventricles, markedly small cerebellum and vermis, and associated atrophy involving the brainstem and both cerebral hemispheres. Moreover, increasing cerebral white matter loss, dysmyelination, pontocerebellar atrophy, and thinning of the corpus callosum was observed during SCA2 disease progression (Moretti et al., 2004; Ramocki et al., 2008; Paciorkowski et al., 2011; Vinther-Jensen et al., 2013; Singh et al., 2014). Histopathology findings in the cerebellar cortex showed a profound loss of Purkinje and granular neurons with severe attenuation of the molecular layer (Paciorkowski et al., 2011).
Abnormally long polyQ tract in the ataxin 7 (ATXN7) gene primarily manifests as cerebellar ataxia in SCA7. Healthy alleles of this gene contain up to 35 CAG repeats, whereas SCA7 affected individuals have more than 39 repeats (David et al., 1997; Stevanin et al., 1998). The childhood-onset of SCA7 is the consequence of more than 100 CAG repeats in the ATXN7 gene (La Spada, 2020). It is a severe developmental syndrome with patient death reported as early as 6 weeks of age (Neetens et al., 1990). The unique symptom is retinal degeneration, which often is the first presenting symptom (Niewiadomska-Cimicka and Trottier, 2019). Besides the classic symptoms of progressive cerebellar ataxia and retinal degeneration, the juvenile cases of SCA7 presented with absent or depressed deep tendon reflexes, which is not the case in the adult-onset type of the disease (Enevoldson et al., 1994). Other studies reported symptoms such as seizures, dysphagia, myoclonus, head lag, the absence of cough reflex, and severe hypotonia, but also symptoms more uncommon for PolyQ diseases such as cardiac involvement, hepatomegaly, multiple hemangiomas, atrial septum defect, patent ductus arteriosus, and congestive heart failure accompany ataxia (Benton et al., 1998; Johansson et al., 1998; van de Warrenburg et al., 2001; Ansorge et al., 2004). Concerning histopathology, adult SCA7 is characterized by neural loss, mainly in the cerebellum and regions of the brainstem, particularly the inferior olivary complex (Holmberg, 1998). Juvenile cases present marked atrophy of both the cerebrum and cerebellum, ventricular dilation, as well as delayed myelination (Benton et al., 1998). Other reports show diffuse brain volume reduction and increased atrophy of the brainstem and cerebellum during SCA7 disease progression (Donis et al., 2015). The most affected cell types in SCA7 are retinal, cerebellar, and medullar neurons (Naphade et al., 2019).
In DRPLA, the affected gene is ATN1 (Koide et al., 1994), a transcriptional regulator involved in the brain and other organ development (Palmer et al., 2019). In the case of the ATN1 gene, CAG repeat sizes can vary between 6 and 35 in healthy individuals, while the expansion of more than 48 repeats results in full penetrance and gives rise to the disease (Nagafuchi et al., 1994). Patients with juvenile-onset DRPLA often have progressive myoclonic epilepsy as one of the first symptoms (Tomoda et al., 1991), and the onset in the first years of life with CAG repeats between 70 and 80 (Veneziano and Frontali, 1993; Hasegawa et al., 2010). Disease onset could occur as early as 6 months of age (with an extreme number of CAG repeats of 90 and 93), when hyperkinetic and involuntary movements, the difficulty of controlling head movements, and seizures developed (Shimojo et al., 2001). In general, DRPLA is characterized by severe neuronal loss in the dentatorubral and pallidal-subthalamic nucleus (corpus Luysii). Juvenile-onset can be characterized by more marked pallidoluysian degeneration than dentatorubral degeneration, which is opposite to late-adult onset degeneration pattern (Yamada, 2010). MRI data of children with DRPLA showed severe atrophy of the cerebrum and cerebellum, delayed myelination, and thin corpus callosum (Shimojo et al., 2001). However, atrophy of the brainstem and spinal cord was noticed as mild (Takeda and Takahashi, 1996). Histochemistry revealed mild neuronal loss with gliosis in the cerebral cortex (Hayashi et al., 1998; Tsuchiya et al., 1998). The most affected cell types in DRPLA are striatal medium spiny neurons and pallidal neurons (Naphade et al., 2019).
SCA17 is caused by an abnormal number (more than 45-47) of CAG or CAA repeats in the TATA box-binding protein (TBP) (Gao et al., 2008; Toyoshima and Takahashi, 2018). In SCA17, a small gain in CAG number in the TBP gene results in a very severe level of genetic anticipation (Maltecca et al., 2003; Rasmussen et al., 2007). For instance, CAG repeats in the range of 55–58 may cause the disease onset at age 20, 61 CAG was associated with onset at age 11, while 66 CAGs resulted in onset at the age of 3 years (Koide et al., 1999; Maltecca et al., 2003; Rasmussen et al., 2007). Common features of the disease are ataxic gait, dysarthria, loss of muscle control, seizures, spasticity, tremor, and intellectual disability. Given the strong anticipation resulting from only low intergenerational expansion, SCA17 and TBP may strongly influence the brain development and transcriptional control of developmental genes. MRI data of 14 years old female with SCA17 showed prominent cerebellar atrophy accompanied by a dilatation of the fourth ventricle and mild cerebral atrophy as well as dilatation of the lateral ventricles (Koide et al., 1999). It is familiar with neuroimaging studies of a family with age at onset range from very early to adult-onset that showed cerebral and cerebellar atrophy in all patients (Maltecca et al., 2003). The most affected cell types in SCA17 are Purkinje, medium spiny cortical, and dopaminergic neurons (Naphade et al., 2019).
In SCA3, disease occurs above 51 or more CAG repeats in ATXN3, while healthy individuals have 12–43 repeats. SCA3 early childhood-onset, described in 2016, involved the range of CAG repeat between 80 and 91 (Donis et al., 2016). The progression of the disease was faster compared to adolescent cases and the signs observed were ataxia, pyramidal findings, and dystonia. In previous SCA3/MJD cohorts, the maximal number of CAGs was 86 (Todd and Paulson, 2010; Tezenas du Montcel et al., 2014). Pathological examination of juvenile SCA3 patients has shown degeneration and mild gliosis of the substantia nigra, dentate, pontine and cranial nerve nuclei, anterior horns, and Clarke’s columns, with the consequent loss of fibers of the superior and middle cerebellar peduncles and spinocerebellar tracts (Coutinho et al., 1982). The most affected cells in adult SCA3 are motor neurons (Naphade et al., 2019). However, in juvenile cases of SCA3 severe nerve cell loss was observed in the dorsal root and trigeminal ganglia (Coutinho et al., 1982).
SCA6 is caused by a polyQ mutation in the calcium channel gene CACNA1A (Zhuchenko et al., 1997). SCA6 develops due to a relatively low number of CAG repeats, with 5 to 20 repeats being considered healthy and 21 repeats and above giving rise to the disease (Ishikawa et al., 1997). A study by Wang et al. (2010) showed that neurodegeneration in SCA6 also occurs in the spinal cord. Results of an autopsy of siblings with early-onset SCA6 revealed severe neurodegeneration in the cerebellum, dentate nucleus, and olivary nuclei (Wang et al., 2010). The most affected cell type in both adult and juvenile SCA6 are Purkinje cells (Wang et al., 2010; Naphade et al., 2019).
The length of CAG repeats in infantile or childhood PolyQ diseases highly influences the onset and severity of the disease. Moreover, genetic anticipation, earlier (and more severe) disease onset in successive generations, is playing a crucial role in the majority of these disorders (Jones et al., 2017).
Spinal bulbar muscular atrophy, also referred to as Kennedy disease, is a form of spinal muscular atrophy that is recessive and X-linked, and therefore only occurs in males. The cause of SBMA is a CAG repeat expansion in exon one of the androgen receptor gene, and the CAG triplet number is mostly in the range of 38 to 62. Unlike in other PolyQ diseases discussed here, the number of CAG repeats only poorly predicts the age of onset (muscle weakness) (Sperfeld et al., 2002; Echaniz-Laguna et al., 2005). Grunseich (Grunseich et al., 2014) presents juvenile onset with 68 CAG repeats. Juvenile onset commonly manifests itself with limb atrophy and gynecomastia between 8 to 15 years of age (Echaniz-Laguna et al., 2005). Neurodegeneration in adult SBMA is mainly characterized by loss of motor neurons in the spinal cord and brainstem, white matter atrophy, and partial androgen insensitivity (Arnold and Merry, 2019). Quantitative brain imaging studies of SBMA patients demonstrated white matter alterations in the corticospinal tracts (CST), limbic system (Kassubek et al., 2007; Unrath et al., 2010), and cerebellum (Pieper et al., 2013). Likewise degeneration of the dorsal root ganglia, loss of lower motor neurons in the anterior horn of the spinal cord as well as in the brainstem motor nuclei except for the third, fourth and sixth cranial nerves. On a cellular level, there is a presence of nuclear inclusions, especially in residual motor neurons in the brainstem, spinal cord, and non-neuronal tissues such as the prostate, testes, and skin (Naphade et al., 2019).
Review of Juvenile- and Adult-Onset HD and Other PolyQ Diseases Transcriptomic Data: Dysregulated Genes Overlap and Go Terms Over-Representation Analysis
To obtain a broader view of the role of the very long CAG repeats and very long polyQ tracts in proteins in early brain development, we collected published transcriptomic data from human juvenile- and adult-onset HD (An et al., 2012; Feyeux et al., 2012; HD iPSC Consortium, 2012; Chiu et al., 2015; Ring et al., 2015; Nekrasov et al., 2016; The HD iPSC Consortium, 2017; Mehta et al., 2018; Świtońska et al., 2019; Al-Dalahmah et al., 2020; Smith-Geater et al., 2020) and also published RNAseq or microarray data from different PolyQ mouse models (Suzuki et al., 2012; Aikawa et al., 2015; Agostoni et al., 2016; Pflieger et al., 2017; Driessen et al., 2018; Hervás-Corpión et al., 2018; Malik et al., 2019; Liu et al., 2020; Stoyas et al., 2020). The published mouse data from SCA1, SCA2, SCA6, SCA7, SCA17, and DRPLA were originally collected from different brain regions, however, data from SBMA mice were collected from primary motor neurons in the spinal cord. An overview of data from all papers included in the analysis can be found in Supplementary Table 1. Transcriptomic data were retrieved from the Gene Expression Omnibus (GEO) repository, if possible, or from the Supplementary Material provided with the original publication. A cut-off of p-value < 0.05 was considered as significant. In papers with much higher number of identified genes, we set a cut-off of p-value < 0.001 (HD iPSC Consortium, 2012; The HD iPSC Consortium, 2017). The analysis of overlapping dysregulated genes (DEGs) between diseases was created and visualized with R software 3.6.3 (R Core Team, 2018) and its three packages: UpSetR (Conway et al., 2017), ComplexHeatmap (Gu et al., 2016), and VennDiagram (Chen and Boutros, 2011). GO terms over-representation analysis was conducted in Cytoscape (Shannon et al., 2003) and its ClueGO app (Bindea et al., 2009, 2013). In order to conduct a thorough comparative study, and look separately into up- and downregulation in various cell populations, we had to combine datas identified by several different methods, such as RNAseq and microarrays. In the summary table, we added a short note about the type of method used in each publication for obtaining the transcriptomic data (Supplementary Table 1).
Previously Published Transcriptomic Data Show Molecular Downregulation in Juvenile-Onset Human HD and Highlights Organism Morphogenesis, Neurodevelopment and Synaptic Transmission
We first focused on the publications with human data where the main aim was to compare genes dysregulated in two types of HD onset in a more detailed way. We assessed the overlap of DEGs between different cell types and between different types of HD disease onset (Figures 1, 2). We focused on data from embryonic stem cells (ESC), induced pluripotent stem cells (iPSC), neural stem cells (NSC), and neurons. With such a collection, we were able to check whether there are genes downregulated in HD from the very beginning and at the same time through the whole “neurodifferentiation axis”. The analyses revealed two genes shared between iPSC, NSC, and neurons in data from JOHD, TBX15, and HOXB6 (Figures 1A,B and Supplementary Table 1). These two genes encode transcription factors that regulate a variety of developmental processes. We identified 12 and 22 genes shared between iPSC and NSC with neurons, respectively, in JOHD (Figures 1A,B and Supplementary Table 1). The firstly mentioned 12 genes are again connected in the majority with the regulation of transcription. The NSC/neurons shared genes are involved in developmental biology and particularly on embryonic skeletal system morphogenesis. Regarding adult-onset HD, we did not identify genes commonly downregulated in every cell type and only identified a group of 11 genes shared between ESC and NSC (Figure 1C and Supplementary Table 1). Altogether, the created Venn diagrams highlight the fact that in JOHD, molecular processes and genes downregulated on very early stages of organism development may have a direct impact on the later brain and neuronal formation, hence resulting in a much earlier disease onset. The UpSetR diagram did not show much of an overlap of downregulated genes between juvenile and adult HD (Figure 1A). Nonetheless, 27 significantly dysregulated genes were identified in neurons obtained from both the adult and juvenile disease types (Figures 1D–E). Those are involved, among others, in the cerebral cortex GABAergic interneuron differentiation, which aberration leads to an imbalance between excitatory and inhibitory signaling, affecting motor and cognitive processes during HD pathogenesis (Hsu et al., 2018). We also analyzed which biological processes include genes downregulated only in juvenile or only in adult HD. This resulted in a big cluster of various early neurodevelopmental processes, organism morphogenesis, and signal transduction for JOHD (Figure 3), which was not the case for adult HD. Besides some neuronal GO terms connected with genes downregulated in adult HD, no obvious cluster of connected processes was identified. Particularly interesting were the four papers with transcriptomic data on human juvenile-onset HD neurons and four articles concerning human adult-onset HD neurons, which we compared (HD iPSC Consortium, 2012; Chiu et al., 2015; Nekrasov et al., 2016; Mehta et al., 2018; Świtońska et al., 2019; Al-Dalahmah et al., 2020; Smith-Geater et al., 2020). A total of 27 downregulated and 48 upregulated genes in neurons were found to be shared between juvenile-onset and adult-onset HD (Figures 1D, 2D). A total of 758 downregulated and 632 upregulated genes in neurons were found to be unique for juvenile-onset HD, and an additional 108 downregulated, and 451 upregulated genes in neurons were unique to adult-onset HD (Figures 1D, 2D). A complete list of common and uniquely dysregulated genes can be found in the Supplementary Data of this work (Supplementary Table 1).
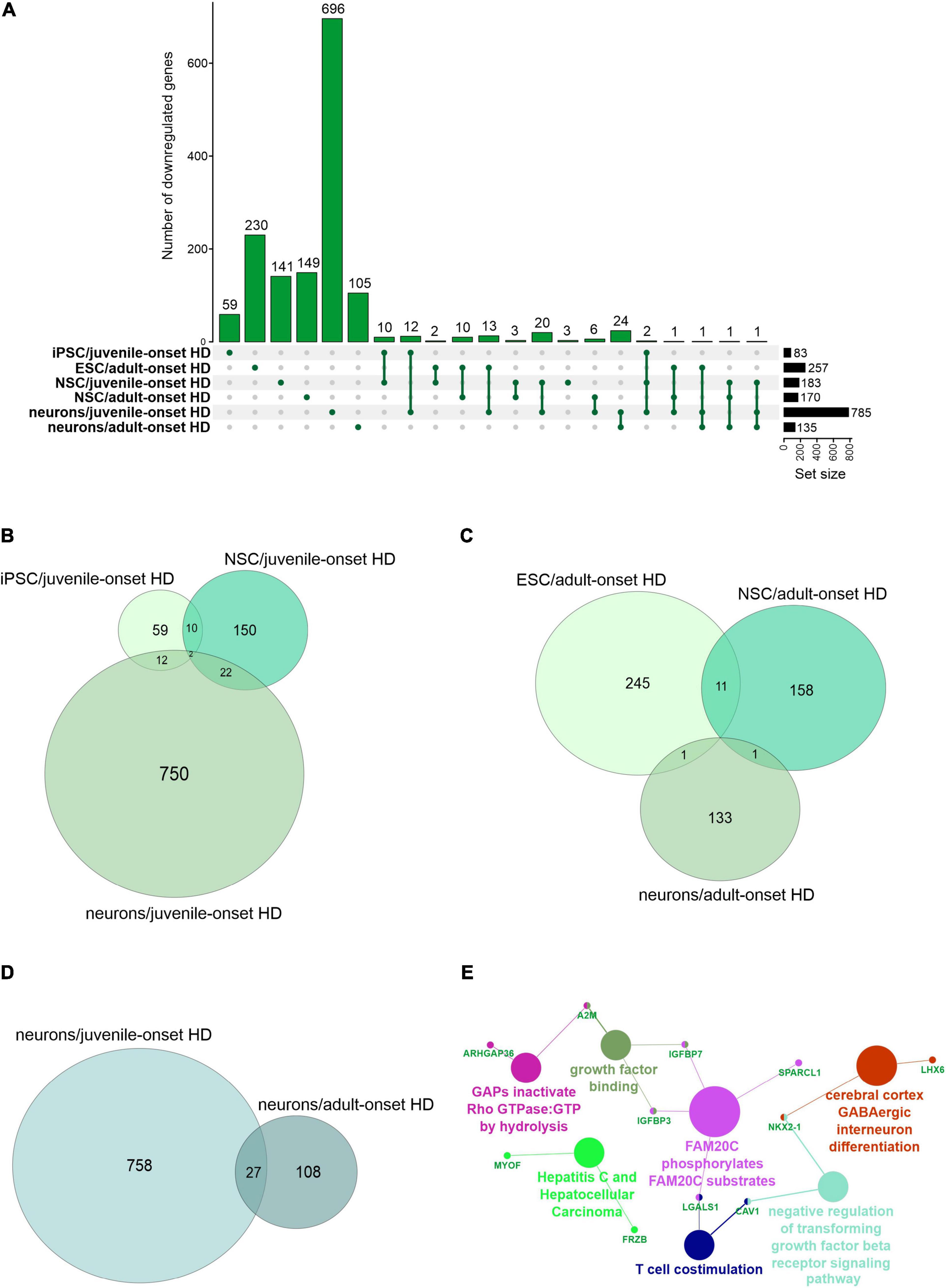
Figure 1. Analysis of Juvenile- and adult Huntington disease transcriptomic data demonstrates mostly specific sets of downregulated genes for each type of onset. (A) UpsetR graph showing the intersection between genes identified in the different HD cell types. Venn diagrams were used to visualize the overlap between genes from juvenile HD iPSC, NSC, and neurons (B), for genes from adult HD iPSC, NSC, and neurons (C), and for genes from juvenile and adult neurons (D). Interestingly, although both the juvenile and adult neurons contain a mutation in HTT, their transcriptomic dysregulated genes vastly differ, showing just 27 genes in common. These commonly downregulated genes are visualized with a CluGO plot (E).
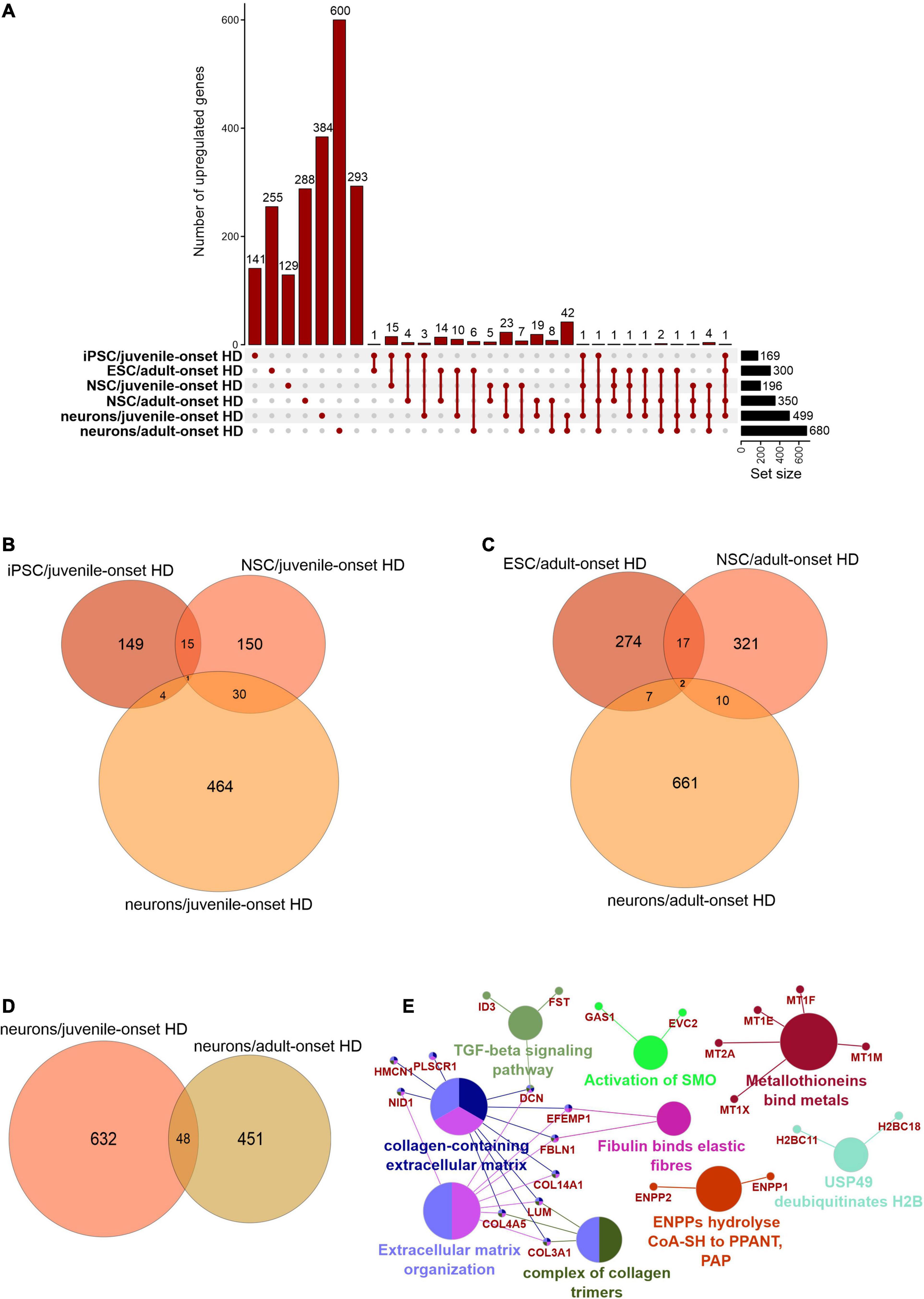
Figure 2. Analysis of Juvenile- and adult Huntington disease transcriptomic data demonstrates mostly specific sets of upregulated genes for each type of onset. (A) UpsetR graph showing the overlap between genes identified in the different HD cell types. Venn diagrams were used to visualize the overlap between genes from juvenile HD iPSC, NSC, and neurons (B), for genes from adult HD iPSC, NSC, and neurons (C), and for genes from juvenile and adult neurons (D). Similar to Supplementary Figure 1D, the juvenile HD and adult HD neurons vastly differ in dysregulated genes, showing only 48 genes in common. These commonly downregulated genes are visualized with a CluGO plot (E).
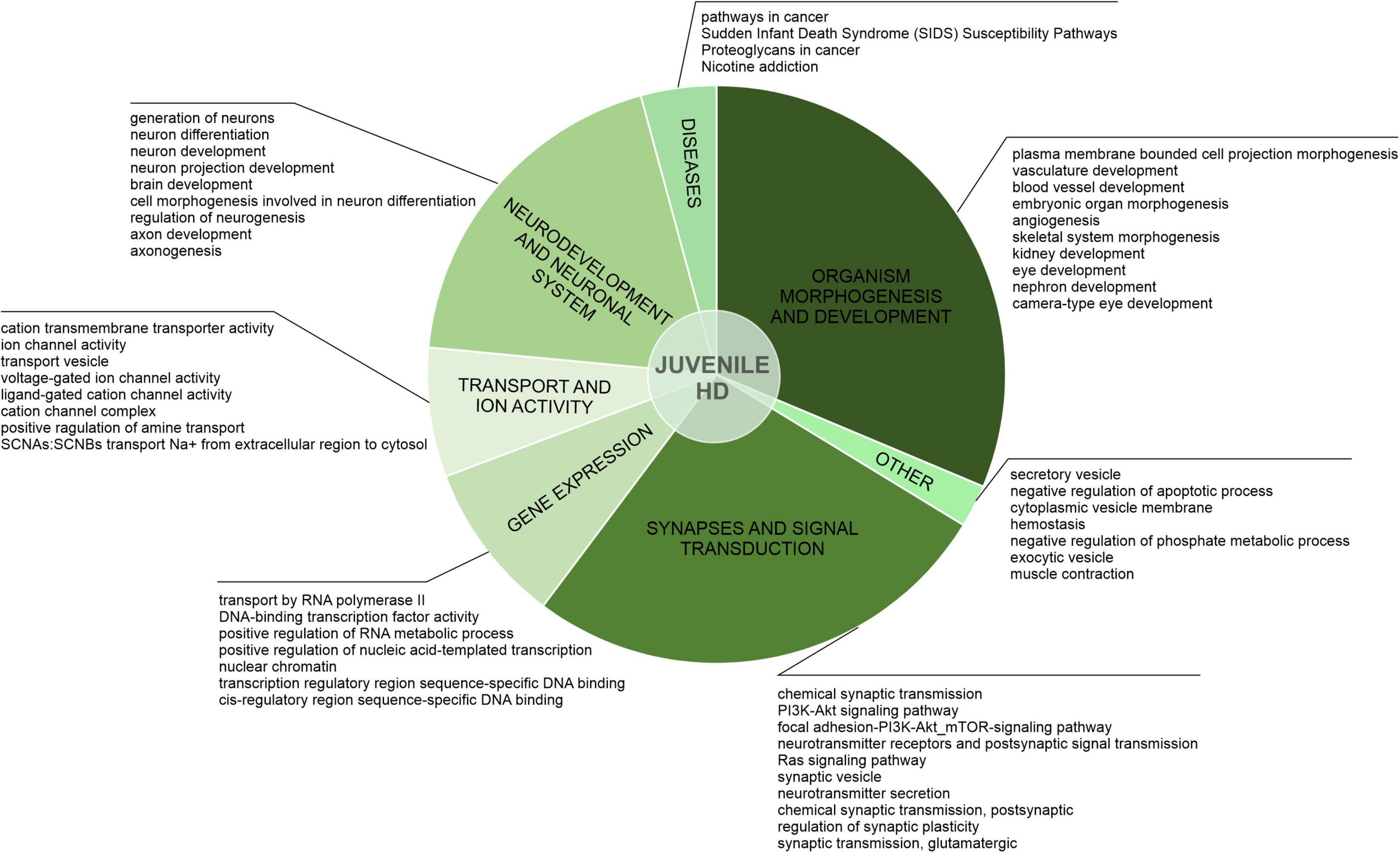
Figure 3. Uniquely downregulated DEGs in JOHD are involved in developmental processes, organism morphogenesis, and signal transduction. A pie-chart with a ClueGO analysis of genes downregulated only in neurons from juvenile-onset HD patients was used to visualize the biological processes they are involved in. Top dysregulated processes for each bigger cluster were listed.
After the assessment of gene overlaps, we performed pathway analysis with ClueGO app (Cytoscape). We found that the DEGs uniquely downregulated in juvenile-onset HD neurons are significantly involved in developmental processes, such as Dopaminergic Neurogenesis (PW:0000394), Differentiation Pathway (WP2848), spinal cord development (GO:0021510), Neuronal System (R-HSA-112316.7), Neural Crest Differentiation (WP2064), presynaptic active zone assembly (GO:1904071), anterior/posterior axon guidance (GO:0033564, metencephalon development (GO:0022037), Potassium Channels (WP2669), and DNA-binding transcription activator activity, RNA polymerase II-specific (GO:0001228) Besides developmental processes, a substantial subset of the uniquely downregulated genes in JOHD-derived neurons is involved in synaptic processes, regulation of synaptic transmission, glutamatergic (GO:0051967 and GO:0051968), Cholinergic synapse (GO:0098981), neurotransmitter secretion (GO:0007269), axon terminus (GO:0043679), positive regulation of dopamine secretion (GO:0033603), regulation of neuronal synaptic plasticity (GO:0048168), and regulation of dendrite morphogenesis (GO:0048814). In Supplementary Table 2, we present a list of the most significantly involved pathways in uniquely downregulated DEGs in JOHD or adult-onset HD, grouped by biological processes, and highlight the input genes found in those pathways. The GO terms unique to neurons of adult-onset HD patients suggest a more developed, more mature cellular expression pattern compared to the juvenile-onset HD.
Inspired by transcriptomic data generated by Haremaki and colleagues (Haremaki et al., 2019) we decided to extend our bioinformatic study with one additional comparative analysis. As previously mentioned, Haremaki and colleagues succeeded in recapitulating human neurulation by generating neuruloids harboring neural progenitors, neural crest, sensory placode, and epidermis. These self-organizing structures provide a great opportunity to study the developmental aspects of many human diseases, especially HD. Having the insight into single-cell transcriptomics from healthy and HD neuruloids, we decided to compare these data with the ones collected for our comparative study. We compared down- and upregulated genes from our cohort to each group of markers specific to a particular cell population identified in scRNA-seq of healthy neuruloids, neuroepithelial identity NE1 and NE2, neurons, skin, neural crest (NC), placode and U1 neurons, and also to a list of differentially expressed genes in NE and NC populations in HD neuruloids (Supplementary Table 4). We identified a significant number of genes shared between markers for neuruloid neurons population and downregulated genes in stem cell-derived neurons in juvenile-onset HD (Supplementary Table 4). This is coherent with GO term over-representation analysis and again highlights the significant downregulation of crucial genes and thus many biological processes during the very early neurogenesis.
HD and SCA1 Seems to Have More Common Transcriptionally Dysregulated Genes Than Other PolyQ Diseases in Mice
In rare PolyQ diseases availability of patient samples and RNAseq data is limited. Therefore, more data can be acquired from mouse models of PolyQ diseases. An extensive review of polyQ mouse models can be found in the works of Figiel et al. (2012) and Switonski et al. (2012). The high CAG repeat numbers are needed in polyQ mouse models to express a disease phenotype; therefore, they may be considered as polyQ models of juvenile-onset type. Therefore, the second data collection for this bioinformatic review was from nine publications concerning mouse brain transcriptomics in several PolyQ diseases, such as HD, SCA1, SCA2, SCA6, SCA7, SCA17, DRPLA, and SBMA (Suzuki et al., 2012; Aikawa et al., 2015; Agostoni et al., 2016; Pflieger et al., 2017; Driessen et al., 2018; Hervás-Corpión et al., 2018; Malik et al., 2019; Liu et al., 2020; Stoyas et al., 2020) (Supplementary Table 1). After adjusting p-value cut-off, the following number of genes was collected: 697 downregulated and 167 upregulated DEGs in HD and, respectively, 643 and 144 in SCA1, 134 and 80 in SCA2, 493 and 349 in SCA6, 64 and 27 in SCA7, 246 and 187 in SCA17, 250 and 162 in SBMA, 225 and 318 in DRPLA (Figures 4, 5 and Supplementary Table 1). The most significant subset of commonly shared DEGs were 87 downregulated genes common between HD and SCA1 (Figure 4B and Supplementary Table 3). ClueGo analysis revealed the involvement of DEGs in Amphetamine addiction (KEGG hsa05031), Opioid signaling (WP1978), neuronal cell body membrane (GO:0032809), and integrin cell surface markers (WP1833) (Figure 4C and Supplementary Table 5). SBMA stood out as the least common of the PolyQ diseases, with 235 out of 250 downregulated and 152 out of 162 upregulated genes being uniquely expressed in SBMA only (Figures 4A, 5A).
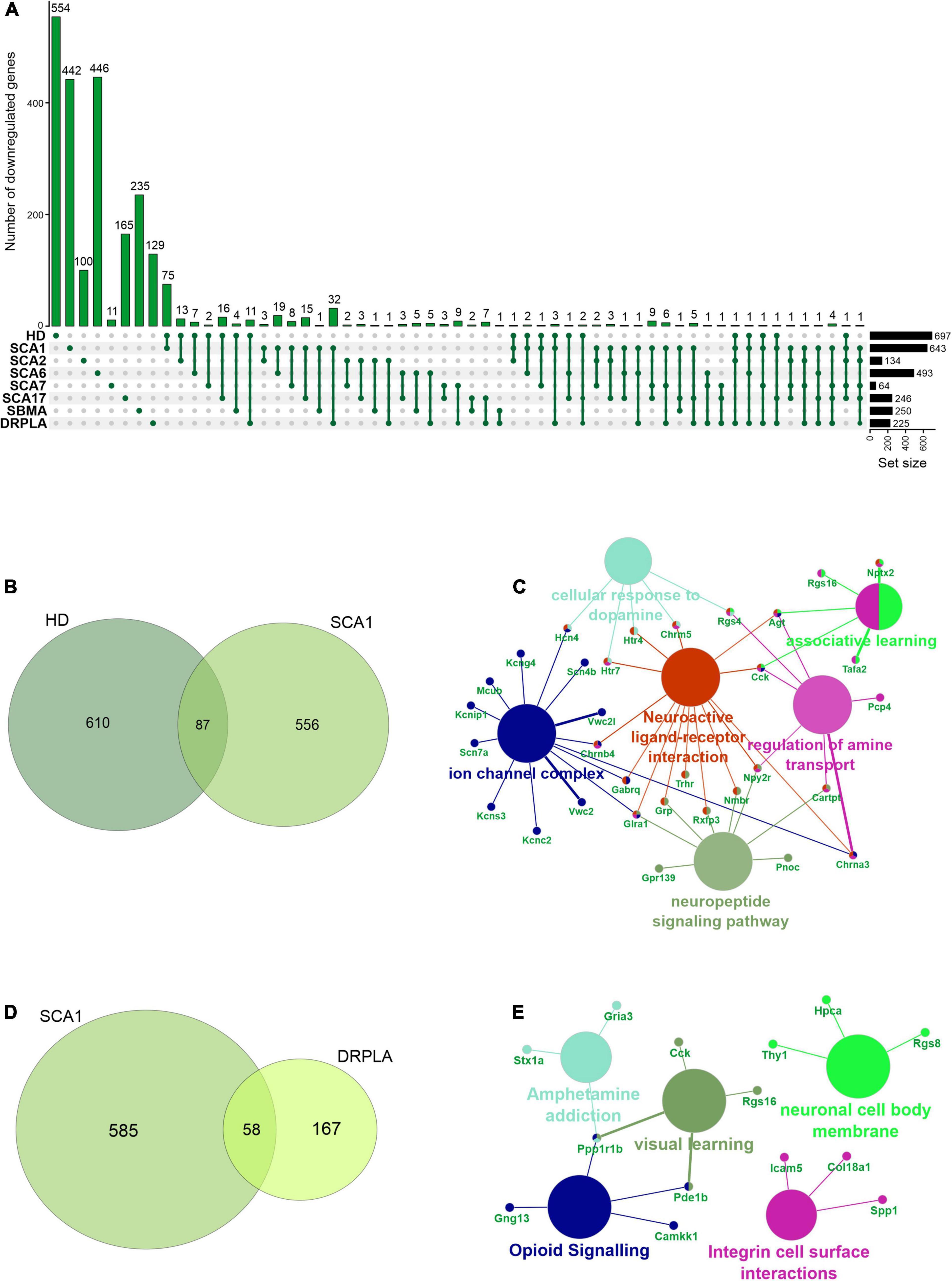
Figure 4. Overlap of significantly downregulated genes from mice transcriptomic data from different PolyQ diseases. (A) UpsetR analysis was used to see the overlap between downregulated genes identified in different PolyQ diseases. Venn diagrams visualizing the overlap between downregulated genes in HD and SCA1; (B) and for overlapping genes downregulated in SCA1 and DRPLA (D). ClueGO analysis was used to visualize the biological processes in which the commonly downregulated genes between HD and SCA1 (C) and between SCA1 and DRPLA (E) are involved.
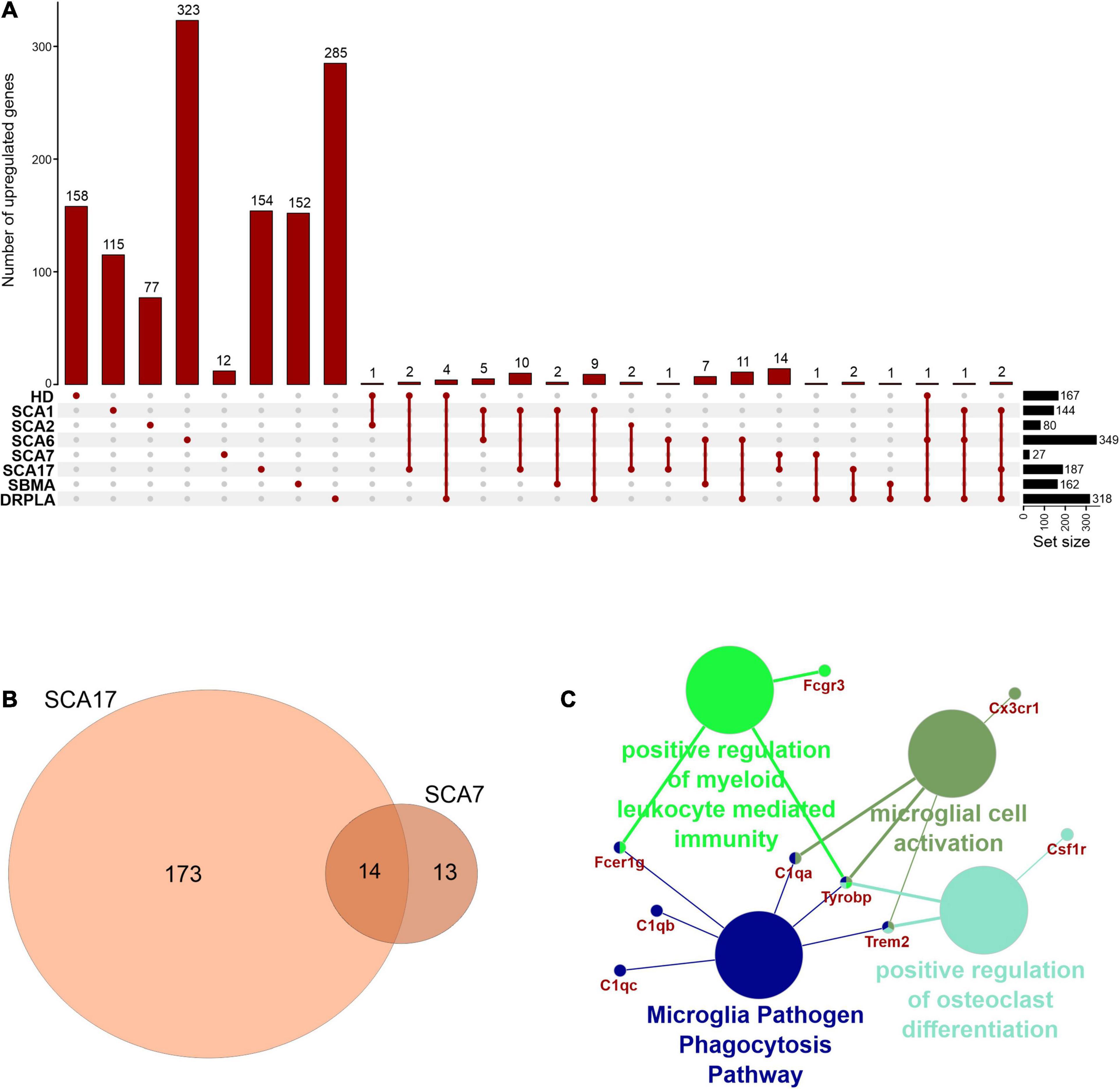
Figure 5. Overlap of significantly upregulated genes from mice transcriptomic data from different PolyQ diseases. (A) UpsetR analysis was used to see the overlap between upregulated genes identified in different PolyQ diseases. (B) Venn diagrams visualizing the overlap between genes upregulated in SCA7 and SCA17. (C) ClueGO analysis of genes commonly upregulated in SCA7 and SCA17.
Two genes were shared between five of the PolyQ diseases (G Protein Subunit Gamma 13 (Gng13) in SCA1, 2, 7, 17, and DRPLA, and Glutamate receptor delta two interacting protein (Grid2ip) in HD, Sca1, 2, 7, and 17). Gng13 encodes the gamma subunit of heterotrimeric G proteins, which are signal transducers for the seven-transmembrane-helix G protein-coupled receptors (Li et al., 2006). Grid2ip is a Purkinje cell-specific postsynaptic protein, where it may serve to link Glutamate receptor delta 2 (GRID2) with the actin cytoskeleton and various signaling molecules. GRID2 has been reported to play crucial roles in synaptogenesis and synaptic plasticity and may control GRID2 signaling in Purkinje cells (Matsuda et al., 2006). Other notable DEGs are Regulator Of G Protein Signaling 8 (Rgs8), Regulator Of G Protein Signaling 16 (Rgs16), and Purkinje Cell Protein 4 (Pcp4), commonly dysregulated in HD, SCA1, DRPLA, and either SCA6 (Rgs16), or SCA7 (Rgs8 and Pcp4). These DEGs are all involved in calmodulin-binding, which acts as part of a calcium signal transduction pathway and has roles in cellular mechanisms, including metabolism, synaptic plasticity, nerve growth, smooth muscle contraction (Hyman and Pfenninger, 1985; Xia and Storm, 2005; Kleerekoper and Putkey, 2009; Mouton-Liger et al., 2011; Wang and Putkey, 2016).
Finally, several Cerebellin (Cbln1, 2, 3, and 4), Matrix Metalloproteinases (Mmp8, 9, 16, 17, and 20), and Collagen (Col5a1, Col6a4, Col11a1, Col18a1, Col20a1, and Col25a1) isoforms are downregulated in compared PolyQ diseases. While no commonly dysregulated isoform was found, the downregulation of these proteins is important for synaptic activity and the modulation of the extracellular matrix, further hinting to an important role of WM alterations in PolyQ diseases.
Commonly Downregulated Genes and Proteins in HD Mouse Brain Are Connected to Neuron Development, Synapses and Signal Transduction, and Cellular Transport
Additionally, we collected published proteomic data from human juvenile- and adult-onset HD (Chen et al., 2012; Schönberger et al., 2013; McQuade et al., 2014; Ratovitski et al., 2016; Ramdzan et al., 2017; Ooi et al., 2019; Świtońska et al., 2019), mice HD models (Deschepper et al., 2012; Skotte et al., 2018; Sap et al., 2019; Sapp et al., 2020) and SCA1 mouse model (Stucki et al., 2016). No data from SCA2, SCA6, SCA7, SCA17, DRPLA, and SBMA were found. Since the availability of published proteomic data is very limited, our analysis of this data is also limited compared to the transcriptomic part. Therefore, we mainly focused on GO term analysis and searching for similarities between dysregulated genes and proteins in human and mouse HD. GO terms over-representation analysis was conducted in ConsensusPathDB (CPDB) (Herwig et al., 2016). An overview of collected data and all of the GO terms analyses for particular sets of proteins can be found in Supplementary Table 5.
Firstly, we focused on comparing proteins and genes dysregulated in the corresponding cell populations in human HD (Supplementary Table 5). The comparison didn’t result in any bigger common group, but nonetheless we were able to identify three shared downregulated genes/proteins: TP53, ELAVL2, COL1A2, together connected with mRNA and protease binding, and four commonly upregulated genes/proteins: LAMB1, CD44, SPP1 and HIST1H1B, which are connected with cell morphogenesis and neuron development. Cell-specific CPDB GO term analysis of human HD proteomic data highlighted mainly neurodevelopment and organism morphogenesis, various metabolic processes, cellular transport, gene expression and DNA damage and apoptosis. Secondly, the comparative analysis of mouse data resulted in identifying an overlap between 13 genes and proteins significantly downregulated in HD mouse brain (Figure 6 and Supplementary Table 5). GO term analysis highlighted four main clusters of biological processes to which the identified genes are connected, such as transport regulation, synapses and signaling, neuron development and metabolism.
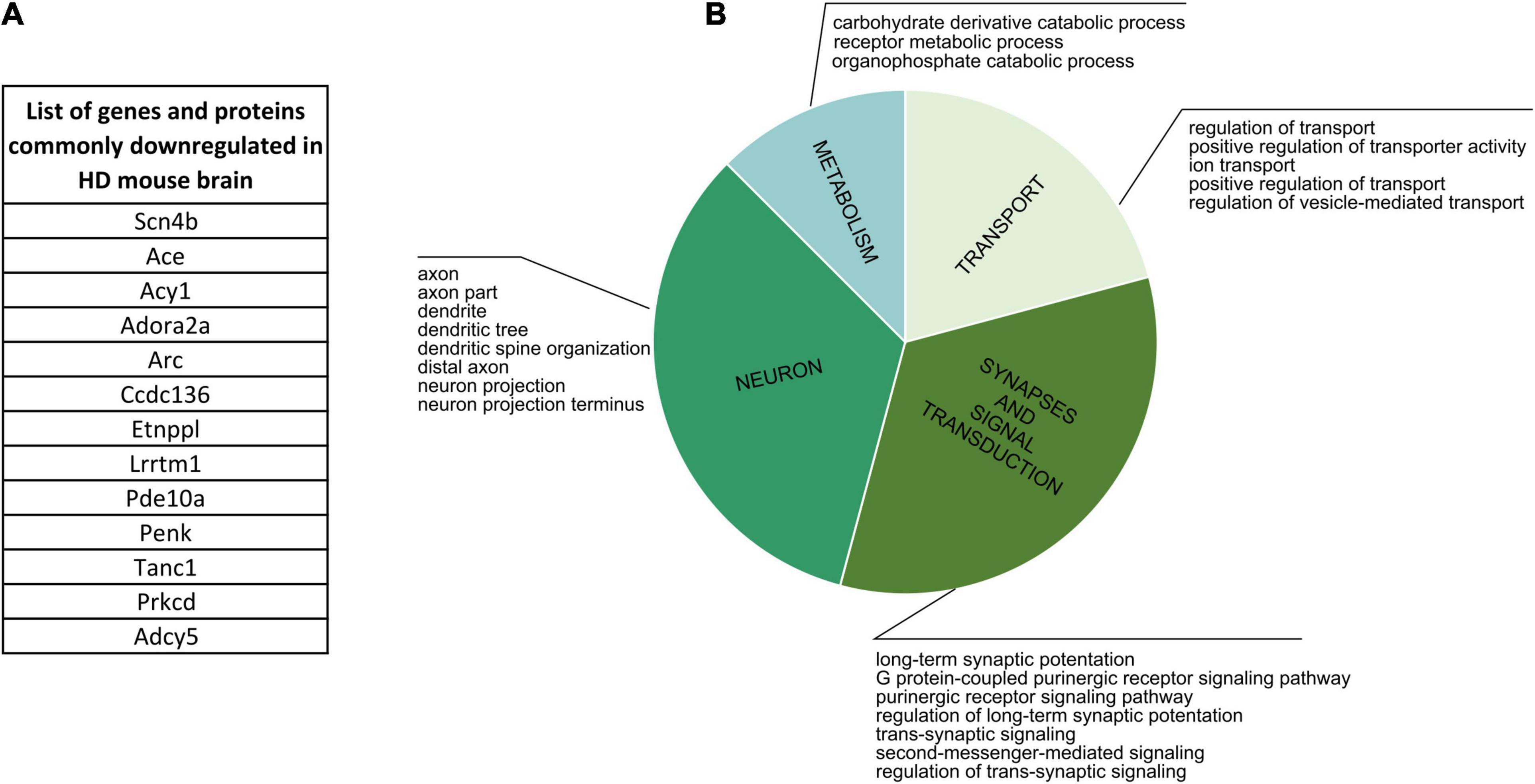
Figure 6. Commonly Downregulated Genes and Proteins in HD Mouse Brain. (A) A list of 13 genes and proteins which were identified as dowregulated in HD mouse brain in several different publications. (B) CPDB GO term over-representation analysis for the identified group of shared genes and proteins.
Finally, we analyzed lists of dysregulated proteins from mouse HD brain to further characterize if there are specific clusters of biological processes connected uniquely with up- or downregulation of certain proteins. We identified two main groups of GO terms for downregulated proteins: transport and ion activity and synapses and signal transduction, and two other main groups for upregulated proteins: DNA and nucleus, and neurodevelopment and organism morphogenesis.
CPDB over-representation analysis of proteins downregulated in SCA1 mouse brain also highlighted synapses and signal transduction and transport-related processes, while upregulation was more connected to metabolism and developmental aspects.
Discussion and Concluding Remarks
Although the juvenile and infantile forms make up a minority of PolyQ disease cases, the early onset makes these diseases an example of neurodevelopmental disorders. Indeed, the results of our bioinformatic study of the available transcriptomic data reveal that uniquely dysregulated genes in juvenile-onset HD neurons are involved in several (neuro)developmental pathways leading to early symptoms in patients. Our group and others have previously demonstrated a neurodevelopmental component in HD pathogenesis, and further exciting evidence was delivered only very recently (Kubera et al., 2019; Barnat et al., 2020). Moreover, HTT has an impact on the cortical volume and brain connections, leading to beter general intelligence score (IQ) in people with larger (sub-disease) PolyQ repeats (Lee et al., 2017; Lee J. K. et al., 2018). An increasing number of studies created a body of evidence for transcriptional modulators of PolyQ tracts not only in HD but also in other PolyQ diseases, like SCAs, mentioned in this manuscript (Paulson et al., 2017; Buijsen et al., 2019).
Our analysis combines numerous data sets on polyQ transcriptomics into one collection and demonstrates several neurodevelopmental transcriptomic commonalities to the diseases. There are genes unique in JOHD neurons and individual genes that are downregulated in four or more of the independent PolyQ diseases mouse models. The genes were involved in neural growth, synaptogenesis, and synaptic plasticity, and extracellular matrix remodeling, suggesting a critical role of brain connections and WM changes roles in PolyQ disease pathology. HTT, ATN1, TBP, and Ataxins have previously been identified as transcriptional regulators (Benn et al., 2008; Kumar et al., 2014; Gao et al., 2019) therefore, our results are in agreement with the previously formulated hypothesis that transcriptional dysregulation is a solid feature of several PolyQ diseases (Helmlinger et al., 2006). The addition of proteomic dataset further highlights neural growth and synaptogenesis and signal transduction as main biological processes in which dysregulated proteins are involved.
Polyglutamine diseases are relatively rare, thus, only a limited number of publications with transcriptomic data were available for our bioinformatic analysis. More research into PolyQ diseases is needed to understand better the mechanistic aspects of the disease pathology. Moreover, studies that will focus on the unique differences between juvenile- and adult-onset would be of interest, as the longer CAG repeat mutations augment the transcriptional potential of the affected protein, which may leading to compromised of neurodevelopment.
Author Contributions
KŚ-K, BK, JD, and MF wrote the manuscript. KŚ-K performed all bioinformatics associated with R software, ClueGO and CPDB analyzes of data. All authors read and approved the final manuscript. MF was responsible for the concept of this review and for obtaining funding.
Funding
This work was supported by the grant from the National Science Centre (grant number 2018/31/B/NZ3/03621).
Conflict of Interest
The authors declare that the research was conducted in the absence of any commercial or financial relationships that could be construed as a potential conflict of interest.
Supplementary Material
The Supplementary Material for this article can be found online at: https://www.frontiersin.org/articles/10.3389/fcell.2021.642773/full#supplementary-material
Supplementary Table 1 | Transcriptomic data included in the comparative bioinformatic study. Data were retrieved from the Gene Expression Omnibs (GEO) repository, if possible, or from the Supplementary Material provided with the publication. A cut-off of p-value < 0.05 was considered as significant. In two publications, with a greater number of identified genes, we set a cut-off of p-value < 0.001.
Supplementary Table 2 | Biological processes, molecular function, and cellular components ClueGO analysis for genes downregulated in neurons from juvenile-onset HD patients (stem cell-derived or collected post-mortem). Top downregulated processes were visualized in Figure 3.
Supplementary Table 3 | Biological processes, molecular function, and cellular components ClueGO analysis for common transcriptionally downregulated genes in HD and SCA1 mice.
Supplementary Table 4 | Comparative analysis between scRNA-seq data from neuruloid paper (Haremaki et al., 2019) and human data collected for our comparative study. We compared down- and upregulated genes from our cohort to each group of markers specific to a particular cell population identified in scRNA-seq of healthy neuruloids, neuroepithelial identity NE1 and NE2, neurons, skin, neural crest (NC), placode and U1 neurons, and also to a list of differentially expressed genes in NE and NC populations in HD neuruloids.
Supplementary Table 5 | Proteomic data included in the bioinformatic comparative study and CPDB GO term over-representation analysis of collected data. Up- and downregulated proteins were retrieved from the main figures or tables or from Supplementary Material provided with the publications. A cut-off of p-value < 0.05 was generally considered as significant.
References
Agostoni, E., Michelazzi, S., Maurutto, M., Carnemolla, A., Ciani, Y., Vatta, P., et al. (2016). Effects of Pin1 loss in Hdh(Q111) knock-in mice. Front. Cell. Neurosci. 10:110. doi: 10.3389/fncel.2016.00110
Ahmad, S. T., Rogers, A. D., Chen, M. J., Dixit, R., Adnani, L., Frankiw, L. S., et al. (2019). Capicua regulates neural stem cell proliferation and lineage specification through control of Ets factors. Nat. Commun. 10:2000. doi: 10.1038/s41467-019-09949-6
Aikawa, T., Mogushi, K., Iijima-Tsutsui, K., Ishikawa, K., Sakurai, M., Tanaka, H., et al. (2015). Loss of MyD88 alters neuroinflammatory response and attenuates early Purkinje cell loss in a spinocerebellar ataxia type 6 mouse model. Hum. Mol. Genet. 24, 4780–4791. doi: 10.1093/hmg/ddv202
Al-Dalahmah, O., Sosunov, A. A., Shaik, A., Ofori, K., Liu, Y., Vonsattel, J. P., et al. (2020). Single-nucleus RNA-seq identifies Huntington disease astrocyte states. Acta Neuropathol. Commun. 8:19. doi: 10.1186/s40478-020-0880-6
An, M. C., Zhang, N., Scott, G., Montoro, D., Wittkop, T., Mooney, S., et al. (2012). Genetic correction of Huntington’s disease phenotypes in induced pluripotent stem cells. Cell Stem Cell 11, 253–263. doi: 10.1016/j.stem.2012.04.026
Ansorge, O., Giunti, P., Michalik, A., Van Broeckhoven, C., Harding, B., Wood, N., et al. (2004). Ataxin-7 aggregation and ubiquitination in infantile SCA7 with 180 CAG repeats. Ann. Neurol. 56, 448–452. doi: 10.1002/ana.20230
Arai, Y., and Taverna, E. (2017). Neural progenitor cell polarity and cortical development. Front. Cell. Neurosci. 11:384. doi: 10.3389/fncel.2017.00384
Arnold, F. J., and Merry, D. E. (2019). Molecular mechanisms and therapeutics for SBMA/Kennedy’s disease. Neurotherapeutics 16, 928–947. doi: 10.1007/s13311-019-00790-9
Babovic-Vuksanovic, D., Snow, K., Patterson, M. C., and Michels, V. V. (1998). Spinocerebellar ataxia type 2 (SCA 2) in an infant with extreme CAG repeat expansion. Am. J. Med. Genet. 79, 383–387.
Barbeau, A. (1970). Parental ascent in the juvenile form of Huntington’s chorea. Lancet 2:937. doi: 10.1016/s0140-6736(70)92119-7
Barnat, M., Capizzi, M., Aparicio, E., Boluda, S., Wennagel, D., Kacher, R., et al. (2020). Huntington’s disease alters human neurodevelopment. Science 369, 787–793. doi: 10.1126/science.aax3338
Benn, C. L., Sun, T., Sadri-Vakili, G., McFarland, K. N., DiRocco, D. P., Yohrling, G. J., et al. (2008). Huntingtin modulates transcription, occupies gene promoters in vivo, and binds directly to DNA in a polyglutamine-dependent manner. J. Neurosci. 28, 10720–10733. doi: 10.1523/JNEUROSCI.2126-08.2008
Benton, C. S., de Silva, R., Rutledge, S. L., Bohlega, S., Ashizawa, T., and Zoghbi, H. Y. (1998). Molecular and clinical studies in SCA-7 define a broad clinical spectrum and the infantile phenotype. Neurology 51, 1081–1086. doi: 10.1212/wnl.51.4.1081
Bindea, G., Galon, J., and Mlecnik, B. (2013). CluePedia Cytoscape plugin: pathway insights using integrated experimental and in silico data. Bioinformatics 29, 661–663. doi: 10.1093/bioinformatics/btt019
Bindea, G., Mlecnik, B., Hackl, H., Charoentong, P., Tosolini, M., Kirilovsky, A., et al. (2009). ClueGO: a Cytoscape plug-in to decipher functionally grouped gene ontology and pathway annotation networks. Bioinformatics 25, 1091–1093. doi: 10.1093/bioinformatics/btp101
Buijsen, R. A. M., Toonen, L. J. A., Gardiner, S. L., and van Roon-Mom, W. M. C. (2019). Genetics, mechanisms, and therapeutic progress in polyglutamine spinocerebellar Ataxias. Neurotherapeutics 16, 263–286. doi: 10.1007/s13311-018-00696-y
Celestino-Soper, P. B., Skinner, C., Schroer, R., Eng, P., Shenai, J., Nowaczyk, M. M., et al. (2012). Deletions in chromosome 6p22.3-p24.3, including ATXN1, are associated with developmental delay and autism spectrum disorders. Mol. Cytogenet. 5:17. doi: 10.1186/1755-8166-5-17
Chan, W. Y., Lorke, D. E., Tiu, S. C., and Yew, D. T. (2002). Proliferation and apoptosis in the developing human neocortex. Anat. Rec. 267, 261–276. doi: 10.1002/ar.10100
Chen, H., and Boutros, P. C. (2011). VennDiagram: a package for the generation of highly-customizable Venn and Euler diagrams in R. BMC Bioinformatics 12:35. doi: 10.1186/1471-2105-12-35
Chen, S., Lu, F. F., Seeman, P., and Liu, F. (2012). Quantitative proteomic analysis of human substantia nigra in Alzheimer’s Disease, Huntington’s disease and multiple sclerosis. Neurochem. Res. 37, 2805–2813. doi: 10.1007/s11064-012-0874-2
Chiu, F.-L., Lin, J.-T., Chuang, C.-Y., Chien, T., Chen, C.-M., Chen, K.-H., et al. (2015). Elucidating the role of the A2A adenosine receptor in neurodegeneration using neurons derived from Huntington’s disease iPSCs. Hum. Mol. Genet. 24, 6066–6079. doi: 10.1093/hmg/ddv318
Conway, J. R., Lex, A., and Gehlenborg, N. (2017). UpSetR: an R package for the visualization of intersecting sets and their properties. Bioinformatics 33, 2938–2940. doi: 10.1093/bioinformatics/btx364
Coutinho, P., Guimarães, A., and Scaravilli, F. (1982). The pathology of Machado-Joseph disease. Report of a possible homozygous case. Acta Neuropathol. 58, 48–54. doi: 10.1007/BF00692697
David, G., Abbas, N., Stevanin, G., Dürr, A., Yvert, G., Cancel, G., et al. (1997). Cloning of the SCA7 gene reveals a highly unstable CAG repeat expansion. Nat. Genet. 17, 65–70. doi: 10.1038/ng0997-65
Deschepper, M., Hoogendoorn, B., Brooks, S., Dunnett, S. B., and Jones, L. (2012). Proteomic changes in the brains of Huntington’s disease mouse models reflect pathology and implicate mitochondrial changes. Brain Res. Bull. 88, 210–222. doi: 10.1016/j.brainresbull.2011.01.012
Di Benedetto, D., Di Vita, G., Romano, C., Giudice, M. L., Vitello, G. A., Zingale, M., et al. (2013). 6p22.3 deletion: report of a patient with autism, severe intellectual disability and electroencephalographic anomalies. Mol. Cytogenet. 6:4. doi: 10.1186/1755-8166-6-4
Donis, K. C., Mattos, E. P., Silva, A. A., Furtado, G. V., Saraiva-Pereira, M. L., Jardim, L. B., et al. (2015). Infantile spinocerebellar ataxia type 7: case report and a review of the literature. J. Neurol. Sci. 354, 118–121. doi: 10.1016/j.jns.2015.04.040
Donis, K. C., Saute, J. A. M., Krum-Santos, A. C., Furtado, G. V., Mattos, E. P., Saraiva-Pereira, M. L., et al. (2016). Spinocerebellar ataxia type 3/Machado-Joseph disease starting before adolescence. Neurogenetics 17, 107–113. doi: 10.1007/s10048-016-0473-5
Driessen, T. M., Lee, P. J., and Lim, J. (2018). Molecular pathway analysis towards understanding tissue vulnerability in spinocerebellar ataxia type 1. ELife 7:e39981. doi: 10.7554/eLife.39981
Echaniz-Laguna, A., Rousso, E., Anheim, M., Cossée, M., and Tranchant, C. (2005). A family with early-onset and rapidly progressive X-linked spinal and bulbar muscular atrophy. Neurology 64, 1458–1460. doi: 10.1212/01.WNL.0000158617.41819.F3
Edamakanti, C. R., Do, J., Didonna, A., Martina, M., and Opal, P. (2018). Mutant ataxin1 disrupts cerebellar development in spinocerebellar ataxia type 1. J. Clin. Invest. 128, 2252–2265. doi: 10.1172/JCI96765
Enevoldson, T. P., Sanders, M. D., and Harding, A. E. (1994). Autosomal dominant cerebellar ataxia with pigmentary macular dystrophy. A Clinical and genetic study of eight families. Brain 117(Pt 3), 445–460. doi: 10.1093/brain/117.3.445
Feyeux, M., Bourgois-Rocha, F., Redfern, A., Giles, P., Lefort, N., Aubert, S., et al. (2012). Early transcriptional changes linked to naturally occurring Huntington’s disease mutations in neural derivatives of human embryonic stem cells. Hum. Mol. Genet. 21, 3883–3895. doi: 10.1093/hmg/dds216
Figiel, M., Szlachcic, W. J., Switonski, P. M., Gabka, A., and Krzyzosiak, W. J. (2012). Mouse models of polyglutamine diseases: review and data table. Part I. Mol. Neurobiol. 46, 393–429. doi: 10.1007/s12035-012-8315-4
Franco, S. J., and Müller, U. (2013). Shaping our minds: stem and progenitor cell diversity in the mammalian neocortex. Neuron 77, 19–34. doi: 10.1016/j.neuron.2012.12.022
Fusilli, C., Migliore, S., Mazza, T., Consoli, F., De Luca, A., Barbagallo, G., et al. (2018). Biological and clinical manifestations of juvenile Huntington’s disease: a retrospective analysis. Lancet Neurol. 17, 986–993. doi: 10.1016/S1474-4422(18)30294-1
Gao, R., Chakraborty, A., Geater, C., Pradhan, S., Gordon, K. L., Snowden, J., et al. (2019). Mutant huntingtin impairs PNKP and ATXN3, disrupting DNA repair and transcription. Elife 8:e42988. doi: 10.7554/eLife.42988
Gao, R., Matsuura, T., Coolbaugh, M., Zühlke, C., Nakamura, K., Rasmussen, A., et al. (2008). Instability of expanded CAG/CAA repeats in spinocerebellar ataxia type 17. Eur. J. Hum. Genet. 16, 215–222. doi: 10.1038/sj.ejhg.5201954
Gibb, R., and Kolb, B. (2018). The Neurobiology of Brain and Behavioral Development. London: Academic Press. doi: 10.1016/C2015-0-00695-5
Grunseich, C., Kats, I. R., Bott, L. C., Rinaldi, C., Kokkinis, A., Fox, D., et al. (2014). Early onset and novel features in a spinal and bulbar muscular atrophy patient with a 68 CAG repeat. Neuromuscul. Disord. 24, 978–981. doi: 10.1016/j.nmd.2014.06.441
Gu, Z., Eils, R., and Schlesner, M. (2016). Complex heatmaps reveal patterns and correlations in multidimensional genomic data. Bioinformatics 32, 2847–2849. doi: 10.1093/bioinformatics/btw313
Haremaki, T., Metzger, J. J., Rito, T., Ozair, M. Z., Etoc, F., and Brivanlou, A. H. (2019). Self-organizing neuruloids model developmental aspects of Huntington’s disease in the ectodermal compartment. Nat. Biotechnol. 37, 1198–1208. doi: 10.1038/s41587-019-0237-5
Hasegawa, A., Ikeuchi, T., Koike, R., Matsubara, N., Tsuchiya, M., Nozaki, H., et al. (2010). Long-term disability and prognosis in dentatorubral-pallidoluysian atrophy: a correlation with CAG repeat length. Mov. Disord. 25, 1694–1700. doi: 10.1002/mds.23167
Hayashi, Y., Kakita, A., Yamada, M., Egawa, S., Oyanagi, S., Naito, H., et al. (1998). “Hereditary dentatorubral-pallidoluysian atrophy: ubiquitinated filamentous inclusions in the cerebellar dentate nucleus neurons. Acta Neuropathologica 95, 479–482. doi: 10.1007/s004010050828
HD iPSC Consortium. (2012). Induced pluripotent stem cells from patients with Huntington’s disease show CAG-repeat-expansion-associated phenotypes. Cell Stem Cell 11, 264–278. doi: 10.1016/j.stem.2012.04.027
Hedjoudje, A., Nicolas, G., Goldenberg, A., Vanhulle, C., Dumant-Forrest, C., Deverrière, G., et al. (2018). Morphological features in juvenile Huntington disease associated with cerebellar atrophy — magnetic resonance imaging morphometric analysis. Pediatr. Radiol. 48, 1463–1471. doi: 10.1007/s00247-018-4167-z
Helmlinger, D., Tora, L., and Devys, D. (2006). Transcriptional alterations and chromatin remodeling in polyglutamine diseases. Trends Genet. 22, 562–570. doi: 10.1016/j.tig.2006.07.010
Hervás-Corpión, I., Guiretti, D., Alcaraz-Iborra, M., Olivares, R., Campos-Caro, A., and Barco, Á, et al. (2018). Early alteration of epigenetic-related transcription in Huntington’s disease mouse models. Sci. Rep. 8:9925. doi: 10.1038/s41598-018-28185-4
Herwig, R., Hardt, C., Lienhard, M., and Kamburov, A. (2016). Analyzing and interpreting genome data at the network level with ConsensusPathDB. Nat. Protoc. 11, 1889–1907. doi: 10.1038/nprot.2016.117
Holmberg, M. (1998). Spinocerebellar ataxia type 7 (SCA7): a neurodegenerative disorder with neuronal intranuclear inclusions. Hum. Mol. Genet. 7, 913–918. doi: 10.1093/hmg/7.5.913
Hsu, Y.-T., Chang, Y.-G., and Chern, Y. (2018). Insights into GABAAergic system alteration in Huntington’s disease. Open Biol. 8:180165. doi: 10.1098/rsob.180165
Hyman, C., and Pfenninger, K. H. (1985). Intracellular regulators of neuronal sprouting: calmodulin-binding proteins of nerve growth cones. J. Cell Biol. 101, 1153–1160. doi: 10.1083/jcb.101.3.1153
Ishikawa, K., Tanaka, H., Saito, M., Ohkoshi, N., Fujita, T., Yoshizawa, K., et al. (1997). Japanese families with autosomal dominant pure cerebellar ataxia map to chromosome 19p13.1-p13.2 and are strongly associated with mild CAG expansions in the spinocerebellar ataxia type 6 gene in chromosome 19p13.1. Am. J. Hum. Genet. 61, 336–346. doi: 10.1086/514867
Johansson, J., Forsgren, L., Sandgren, O., Brice, A., Holmgren, G., and Holmberg, M. (1998). Expanded CAG repeats in Swedish spinocerebellar ataxia type 7 (SCA7) patients: effect of CAG repeat length on the clinical manifestation. Hum. Mol. Genet. 7, 171–176. doi: 10.1093/hmg/7.2.171
Jones, L., Houlden, H., and Tabrizi, S. J. (2017). DNA repair in the trinucleotide repeat disorders. Lancet Neurol. 16, 88–96. doi: 10.1016/S1474-4422(16)30350-7
Kassubek, J., Juengling, F. D., and Sperfeld, A.-D. (2007). Widespread white matter changes in Kennedy disease: a voxel based morphometry study. J. Neurol. Neurosurg. Psychiatry 78, 1209–1212. doi: 10.1136/jnnp.2006.112532
Kleerekoper, Q. K., and Putkey, J. A. (2009). PEP-19, an intrinsically disordered regulator of calmodulin signaling. J. Biol. Chem. 284, 7455–7464. doi: 10.1074/jbc.M808067200
Koide, R., Ikeuchi, T., Onodera, O., Tanaka, H., Igarashi, S., Endo, K., et al. (1994). Unstable expansion of CAG repeat in hereditary dentatorubral-pallidoluysian atrophy (DRPLA). Nat. Genet. 6, 9–13. doi: 10.1038/ng0194-9
Koide, R., Kobayashi, S., Shimohata, T., Ikeuchi, T., Maruyama, M., Saito, M., et al. (1999). A neurological disease caused by an expanded CAG trinucleotide repeat in the TATA-binding protein gene: a new polyglutamine disease? Hum. Mol. Genet. 8, 2047–2053. doi: 10.1093/hmg/8.11.2047
Kubera, K. M., Schmitgen, M. M., Hirjak, D., Wolf, R. C., and Orth, M. (2019). Cortical neurodevelopment in pre-manifest Huntington’s disease. Neuroimage Clin. 23:101913. doi: 10.1016/j.nicl.2019.101913
Kumar, A., Vaish, M., and Ratan, R. R. (2014). Transcriptional dysregulation in Huntington’s disease: a failure of adaptive transcriptional homeostasis. Drug Discov. Today 19, 956–962. doi: 10.1016/j.drudis.2014.03.016
La Spada, A. R. (2020). “Spinocerebellar Ataxia Type 7,” in GeneReviews®, eds M. P. Adam, H. H. Ardinger, R. A. Pagon, S. E. Wallace, L. J. Bean, K. Stephens, et al. Seattle, WA: University of Washington, Seattle). Available online at: http://www.ncbi.nlm.nih.gov/books/NBK1256/ (accessed November 23, 2020).
Latimer, C. S., Flanagan, M. E., Cimino, P. J., Jayadev, S., Davis, M., Hoffer, Z. S., et al. (2017). Neuropathological comparison of adult onset and juvenile Huntington’s disease with cerebellar atrophy: a report of a father and son. J. Huntingtons Dis. 6, 337–348. doi: 10.3233/JHD-170261
Lee, J. K., Conrad, A., Epping, E., Mathews, K., Magnotta, V., Dawson, J. D., et al. (2018). Effect of trinucleotide repeats in the Huntington’s gene on intelligence. EBioMedicine 31, 47–53. doi: 10.1016/j.ebiom.2018.03.031
Lee, J. K., Ding, Y., Conrad, A. L., Cattaneo, E., Epping, E., Mathews, K., et al. (2017). Sex-specific effects of the Huntington gene on normal neurodevelopment. J. Neurosci. Res. 95, 398–408. doi: 10.1002/jnr.23980
Lee, J., Kim, M., Itoh, T. Q., and Lim, C. (2018). Ataxin-2: a versatile posttranscriptional regulator and its implication in neural function. Wiley Interdiscip. Rev. RNA 9:e1488. doi: 10.1002/wrna.1488
Li, Z., Benard, O., and Margolskee, R. F. (2006). Ggamma13 interacts with PDZ domain-containing proteins. J. Biol. Chem. 281, 11066–11073. doi: 10.1074/jbc.M600113200
Lim, J., Crespo-Barreto, J., Jafar-Nejad, P., Bowman, A. B., Richman, R., Hill, D. E., et al. (2008). Opposing effects of polyglutamine expansion on native protein complexes contribute to SCA1. Nature 452, 713–718. doi: 10.1038/nature06731
Liu, Q., Huang, S., Yin, P., Yang, S., Zhang, J., Jing, L., et al. (2020). Cerebellum-enriched protein INPP5A contributes to selective neuropathology in mouse model of spinocerebellar ataxias type 17. Nat. Commun. 11:1101. doi: 10.1038/s41467-020-14931-8
Lu, H.-C., Tan, Q., Rousseaux, M. W. C., Wang, W., Kim, J.-Y., Richman, R., et al. (2017). Disruption of the ATXN1-CIC complex causes a spectrum of neurobehavioral phenotypes in mice and humans. Nat. Genet. 49, 527–536. doi: 10.1038/ng.3808
Malik, B., Devine, H., Patani, R., La Spada, A. R., Hanna, M. G., and Greensmith, L. (2019). Gene expression analysis reveals early dysregulation of disease pathways and links Chmp7 to pathogenesis of spinal and bulbar muscular atrophy. Sci. Rep. 9:3539. doi: 10.1038/s41598-019-40118-3
Maltecca, F., Filla, A., Castaldo, I., Coppola, G., Fragassi, N. A., Carella, M., et al. (2003). Intergenerational instability and marked anticipation in SCA-17. Neurology 61, 1441–1443. doi: 10.1212/01.wnl.0000094123.09098.a0
Mannervik, M. (2014). Control of Drosophila embryo patterning by transcriptional co-regulators. Exp. Cell Res. 321, 47–57. doi: 10.1016/j.yexcr.2013.10.010
Mao, R., Aylsworth, A. S., Potter, N., Wilson, W. G., Breningstall, G., Wick, M. J., et al. (2002). Childhood-onset ataxia: testing for large CAG-repeats in SCA2 and SCA7. Am. J. Med. Genet. 110, 338–345. doi: 10.1002/ajmg.10467
Martin, J. B., and Gusella, J. F. (1986). Huntingtons disease. N. Engl. J. Med. 315, 1267–1276. doi: 10.1056/NEJM198611133152006
Matsuda, K., Matsuda, S., Gladding, C. M., and Yuzaki, M. (2006). Characterization of the delta2 glutamate receptor-binding protein delphilin: splicing variants with differential palmitoylation and an additional PDZ domain. J. Biol. Chem. 281, 25577–25587. doi: 10.1074/jbc.M602044200
McQuade, L. R., Balachandran, A., Scott, H. A., Khaira, S., Baker, M. S., and Schmidt, U. (2014). Proteomics of Huntington’s disease-affected human embryonic stem cells reveals an evolving pathology involving mitochondrial dysfunction and metabolic disturbances. J. Proteome Res. 13, 5648–5659. doi: 10.1021/pr500649m
Mehta, S. R., Tom, C. M., Wang, Y., Bresee, C., Rushton, D., Mathkar, P. P., et al. (2018). Human Huntington’s Disease iPSC-derived cortical neurons display altered transcriptomics, morphology, and maturation. Cell Rep. 25, 1081–1096.e6. doi: 10.1016/j.celrep.2018.09.076
Milunsky, J. M., Maher, T. A., Loose, B. A., Darras, B. T., and Ito, M. (2003). XL PCR for the detection of large trinucleotide expansions in juvenile Huntington’s disease. Clin. Genet. 64, 70–73. doi: 10.1034/j.1399-0004.2003.00108.x
Moretti, P., Blazo, M., Garcia, L., Armstrong, D., Lewis, R. A., Roa, B., et al. (2004). Spinocerebellar ataxia type 2 (SCA2) presenting with ophthalmoplegia and developmental delay in infancy. Am. J. Med. Genet. A 124A, 392–396. doi: 10.1002/ajmg.a.20428
Mouton-Liger, F., Thomas, S., Rattenbach, R., Magnol, L., Larigaldie, V., Ledru, A., et al. (2011). PCP4 (PEP19) overexpression induces premature neuronal differentiation associated with Ca(2+) /calmodulin-dependent kinase II-δ activation in mouse models of Down syndrome. J. Comp. Neurol. 519, 2779–2802. doi: 10.1002/cne.22651
Nagafuchi, S., Yanagisawa, H., Sato, K., Shirayama, T., Ohsaki, E., Bundo, M., et al. (1994). Dentatorubral and pallidoluysian atrophy expansion of an unstable CAG trinucleotide on chromosome 12p. Nat. Genet. 6, 14–18. doi: 10.1038/ng0194-14
Nance, M. A., and Myers, R. H. (2001). Juvenile onset Huntington’s disease–clinical and research perspectives. Ment. Retard. Dev. Disabil. Res. Rev. 7, 153–157. doi: 10.1002/mrdd.1022
Naphade, S., Tshilenge, K.-T., and Ellerby, L. M. (2019). Modeling polyglutamine expansion diseases with induced pluripotent stem cells. Neurotherapeutics 16, 979–998. doi: 10.1007/s13311-019-00810-8
Neetens, A., Martin, J. J., Libert, J., and Den Ende, P. V. (1990). Autosomal dominant cone dystrophy-cerebellar atrophy (ADCoCA) (modified ADCA Harding II). Neuro Ophthalmol. 10, 261–275. doi: 10.3109/01658109008997294
Nekrasov, E. D., Vigont, V. A., Klyushnikov, S. A., Lebedeva, O. S., Vassina, E. M., Bogomazova, A. N., et al. (2016). Manifestation of Huntington’s disease pathology in human induced pluripotent stem cell-derived neurons. Mol. Neurodegener. 11:27. doi: 10.1186/s13024-016-0092-5
Niewiadomska-Cimicka, A., and Trottier, Y. (2019). Molecular targets and therapeutic strategies in spinocerebellar ataxia Type 7. Neurotherapeutics 16, 1074–1096. doi: 10.1007/s13311-019-00778-5
Ohler, U., and Wassarman, D. A. (2010). Promoting developmental transcription. Development 137, 15–26. doi: 10.1242/dev.035493
Ooi, J., Langley, S. R., Xu, X., Utami, K. H., Sim, B., Huang, Y., et al. (2019). Unbiased profiling of isogenic huntington disease hPSC-Derived CNS and peripheral cells reveals strong cell-type specificity of CAG length effects. Cell Rep. 26, 2494–2508.e7.
Paciorkowski, A. R., Shafrir, Y., Hrivnak, J., Patterson, M. C., Tennison, M. B., Clark, H. B., et al. (2011). Massive expansion of SCA2 with autonomic dysfunction, retinitis pigmentosa, and infantile spasms. Neurology 77, 1055–1060. doi: 10.1212/WNL.0b013e31822e5627
Palmer, E. E., Hong, S., Al Zahrani, F., Hashem, M. O., Aleisa, F. A., Ahmed, H. M. J., et al. (2019). De novo variants disrupting the HX repeat motif of ATN1 cause a recognizable non-progressive neurocognitive syndrome. Am. J. Hum. Genet. 104, 542–552. doi: 10.1016/j.ajhg.2019.01.013
Paridaen, J. T., and Huttner, W. B. (2014). Neurogenesis during development of the vertebrate central nervous system. EMBO Rep. 15, 351–364. doi: 10.1002/embr.201438447
Paulson, H. L., Shakkottai, V. G., Clark, H. B., and Orr, H. T. (2017). Polyglutamine spinocerebellar ataxias — from genes to potential treatments. Nat. Rev. Neurosci. 18, 613–626. doi: 10.1038/nrn.2017.92
Pflieger, L. T., Dansithong, W., Paul, S., Scoles, D. R., Figueroa, K. P., Meera, P., et al. (2017). Gene co-expression network analysis for identifying modules and functionally enriched pathways in SCA2. Hum. Mol. Genet. 26, 3069–3080. doi: 10.1093/hmg/ddx191
Pieper, C. C., Konrad, C., Sommer, J., Teismann, I., and Schiffbauer, H. (2013). Structural changes of central white matter tracts in Kennedy’s disease - a diffusion tensor imaging and voxel-based morphometry study. Acta Neurol. Scand. 127, 323–328. doi: 10.1111/ane.12018
Pini, L., Jacquemot, C., Cagnin, A., Meneghello, F., Semenza, C., Mantini, D., et al. (2020). Aberrant brain network connectivity in presymptomatic and manifest Huntington’s disease: a systematic review. Hum. Brain Mapp. 41, 256–269. doi: 10.1002/hbm.24790
Quarrell, O. W. J., Nance, M. A., Nopoulos, P., Paulsen, J. S., Smith, J. A., and Squitieri, F. (2013). Managing juvenile Huntington’s disease. Neurodegener. Dis. Manag. 3, 267–276. doi: 10.2217/nmt.13.18
R Core Team (2018). R: A Language and Environment for Statistical Computing. Vienna: R Foundation for Statistical Computing. Available online at: https://www.R-project.org
Ramdzan, Y. M., Trubetskov, M. M., Ormsby, A. R., Newcombe, E. A., Sui, X., Tobin, M. J., et al. (2017). Huntingtin inclusions trigger cellular quiescence, deactivate apoptosis, and lead to delayed necrosis. Cell Rep. 19, 919–927. doi: 10.1016/j.celrep.2017.04.029
Ramocki, M. B., Chapieski, L., McDonald, R. O., Fernandez, F., and Malphrus, A. D. (2008). Spinocerebellar Ataxia Type 2 presenting with cognitive regression in childhood. J. Child Neurol. 23, 999–1001. doi: 10.1177/0883073808315622
Rasmussen, A., De Biase, I., Fragoso-Benítez, M., Macías-Flores, M. A., Yescas, P., Ochoa, A., et al. (2007). Anticipation and intergenerational repeat instability in spinocerebellar ataxia type 17: anticipation in SCA17. Ann. Neurol. 61, 607–610. doi: 10.1002/ana.21139
Ratovitski, T., Chaerkady, R., Kammers, K., Stewart, J. C., Zavala, A., Pletnikova, O., et al. (2016). Quantitative proteomic analysis reveals similarities between Huntington’s disease (HD) and Huntington’s disease-like 2 (HDL2) human brains. J. Proteome Res. 15, 3266–3283. doi: 10.1021/acs.jproteome.6b00448
Ribaï, P., Nguyen, K., Hahn-Barma, V., Gourfinkel-An, I., Vidailhet, M., Legout, A., et al. (2007). Psychiatric and cognitive difficulties as indicators of juvenile huntington disease onset in 29 patients. Arch. Neurol. 64, 813–819. doi: 10.1001/archneur.64.6.813
Ring, K. L., An, M. C., Zhang, N., O’Brien, R. N., Ramos, E. M., Gao, F., et al. (2015). Genomic analysis reveals disruption of striatal neuronal development and therapeutic targets in human Huntington’s disease neural stem cells. Stem Cell Rep. 5, 1023–1038. doi: 10.1016/j.stemcr.2015.11.005
Sakazume, S., Yoshinari, S., Oguma, E., Utsuno, E., Ishii, T., Narumi, Y., et al. (2009). A patient with early onset Huntington disease and severe cerebellar atrophy. Am. J. Med. Genet. A 149A, 598–601. doi: 10.1002/ajmg.a.32707
Sánchez-Corona, J., Ramirez-Garcia, S. A., Castañeda-Cisneros, G., Gutiérrez-Rubio, S. A., Volpini, V., Sánchez-Garcia, D. M., et al. (2020). A clinical report of the massive CAG repeat expansion in spinocerebellar ataxia type 2: severe onset in a Mexican child and review previous cases. Genet. Mol. Biol. 43:e20190325. doi: 10.1590/1678-4685-GMB-2019-0325
Sap, K. A., Guler, A. T., Bezstarosti, K., Bury, A. E., Juenemann, K., Demmers, J. A. A., et al. (2019). Global proteome and ubiquitinome changes in the soluble and insoluble fractions of Q175 Huntington mice brains. Mol. Cell. Proteomics 18, 1705–1720. doi: 10.1074/mcp.RA119.001486
Sapp, E., Seeley, C., Iuliano, M., Weisman, E., Vodicka, P., DiFiglia, M., et al. (2020). Protein changes in synaptosomes of Huntington’s disease knock-in mice are dependent on age and brain region. Neurobiol. Dis. 141:104950. doi: 10.1016/j.nbd.2020.104950
Saudou, F., and Humbert, S. (2016). The biology of huntingtin. Neuron 89, 910–926. doi: 10.1016/j.neuron.2016.02.003
Schönberger, S. J., Jezdic, D., Faull, R. L. M., and Cooper, G. J. S. (2013). Proteomic analysis of the human brain in Huntington’s disease indicates pathogenesis by molecular processes linked to other neurodegenerative diseases and to Type-2 diabetes. J. Huntingtons Dis. 2, 89–99. doi: 10.3233/JHD-120044
Shannon, P., Markiel, A., Ozier, O., Baliga, N. S., Wang, J. T., Ramage, D., et al. (2003). Cytoscape: a software environment for integrated models of biomolecular interaction networks. Genome Res. 13, 2498–2504. doi: 10.1101/gr.1239303
Shen, Y., and Peterson, A. S. (2009). Atrophins’ emerging roles in development and neurodegenerative disease. Cell. Mol. Life Sci. 66, 437–446. doi: 10.1007/s00018-008-8403-9
Shimojo, Y., Osawa, Y., Fukumizu, M., Hanaoka, S., Tanaka, H., Ogata, F., et al. (2001). Severe infantile dentatorubral pallidoluysian atrophy with extreme expansion of CAG repeats. Neurology 56, 277–278. doi: 10.1212/wnl.56.2.277
Silva, C. G., Peyre, E., and Nguyen, L. (2019). Cell migration promotes dynamic cellular interactions to control cerebral cortex morphogenesis. Nat. Rev. Neurosci. 20, 318–329. doi: 10.1038/s41583-019-0148-y
Singh, A., Faruq, M., Mukerji, M., Dwivedi, M. K., Pruthi, S., and Kapoor, S. (2014). Infantile onset spinocerebellar Ataxia 2 (SCA2): a clinical report with review of previous cases. J. Child Neurol. 29, 139–144. doi: 10.1177/0883073813509015
Skotte, N. H., Andersen, J. V., Santos, A., Aldana, B. I., Willert, C. W., Nørremølle, A., et al. (2018). Integrative characterization of the R6/2 mouse model of Huntington’s disease reveals dysfunctional astrocyte metabolism. Cell Rep. 23, 2211–2224. doi: 10.1016/j.celrep.2018.04.052
Smith-Geater, C., Hernandez, S. J., Lim, R. G., Adam, M., Wu, J., Stocksdale, J. T., et al. (2020). Aberrant development corrected in adult-onset Huntington’s disease iPSC-derived neuronal cultures via WNT signaling modulation. Stem Cell Rep. 14, 406–419. doi: 10.1016/j.stemcr.2020.01.015
Sperfeld, A. D., Karitzky, J., Brummer, D., Schreiber, H., Häussler, J., Ludolph, A. C., et al. (2002). X-linked bulbospinal neuronopathy: kennedy disease. Arch. Neurol. 59:1921. doi: 10.1001/archneur.59.12.1921
Squitieri, F., Frati, L., Ciarmiello, A., Lastoria, S., and Quarrell, O. (2006). Juvenile Huntington’s disease: does a dosage-effect pathogenic mechanism differ from the classical adult disease? Mech. Ageing Dev. 127, 208–212. doi: 10.1016/j.mad.2005.09.012
Stevanin, G., Giunti, P., Belal, G. D., Dürr, A., Ruberg, M., Wood, N., et al. (1998). De novo expansion of intermediate alleles in spinocerebellar ataxia 7. Hum. Mol. Genet. 7, 1809–1813. doi: 10.1093/hmg/7.11.1809
Stiles, J., and Jernigan, T. L. (2010). The basics of brain development. Neuropsychol. Rev. 20, 327–348. doi: 10.1007/s11065-010-9148-4
Stout, J. C. (2018). Juvenile Huntington’s disease: left behind? Lancet Neurol. 17, 932–933. doi: 10.1016/S1474-4422(18)30334-X
Stoyas, C. A., Bushart, D. D., Switonski, P. M., Ward, J. M., Alaghatta, A., Tang, M., et al. (2020). Nicotinamide pathway-dependent sirt1 activation restores calcium homeostasis to achieve neuroprotection in spinocerebellar ataxia type 7. Neuron 105, 630–644.e9. doi: 10.1016/j.neuron.2019.11.019
Stucki, D. M., Ruegsegger, C., Steiner, S., Radecke, J., Murphy, M. P., Zuber, B., et al. (2016). Mitochondrial impairments contribute to Spinocerebellar ataxia type 1 progression and can be ameliorated by the mitochondria-targeted antioxidant MitoQ. Free Radic. Biol. Med. 97, 427–440. doi: 10.1016/j.freeradbiomed.2016.07.005
Suzuki, K., Zhou, J., Sato, T., Takao, K., Miyagawa, T., Oyake, M., et al. (2012). DRPLA transgenic mouse substrains carrying single copy of full-length mutant human DRPLA gene with variable sizes of expanded CAG repeats exhibit CAG repeat length- and age-dependent changes in behavioral abnormalities and gene expression profiles. Neurobiol. Dis. 46, 336–350. doi: 10.1016/j.nbd.2012.01.014
Świtońska, K., Szlachcic, W. J., Handschuh, L., Wojciechowski, P., Marczak, Ł, Stelmaszczuk, M., et al. (2019). Identification of altered developmental pathways in human juvenile HD iPSC With 71Q and 109Q using transcriptome profiling. Front. Cell. Neurosci. 12:528. doi: 10.3389/fncel.2018.00528
Switonski, P. M., Szlachcic, W. J., Gabka, A., Krzyzosiak, W. J., and Figiel, M. (2012). Mouse models of polyglutamine diseases in therapeutic approaches: review and data table. Part II. Mol. Neurobiol. 46, 430–466. doi: 10.1007/s12035-012-8316-3
Szlachcic, W. J., Switonski, P. M., Krzyzosiak, W. J., Figlerowicz, M., and Figiel, M. (2015). Huntington disease iPSCs show early molecular changes in intracellular signaling, the expression of oxidative stress proteins and the p53 pathway. Dis. Model. Mech. 8, 1047–1057. doi: 10.1242/dmm.019406
Szlachcic, W. J., Wiatr, K., Trzeciak, M., Figlerowicz, M., and Figiel, M. (2017). The generation of mouse and human huntington disease iPS cells suitable for in vitro studies on huntingtin function. Front. Mol. Neurosci. 10:253. doi: 10.3389/fnmol.2017.00253
Takeda, S., and Takahashi, H. (1996). Neuropathology of dentatorubropallidoluysian atrophy. Neuropathology 16, 48–55. doi: 10.1111/j.1440-1789.1996.tb00155.x
Tereshchenko, A., Magnotta, V., Epping, E., Mathews, K., Espe-Pfeifer, P., Martin, E., et al. (2019). Brain structure in juvenile-onset Huntington disease. Neurology 92, e1939–e1947. doi: 10.1212/WNL.0000000000007355
Tezenas du Montcel, S., Durr, A., Bauer, P., Figueroa, K. P., Ichikawa, Y., Brussino, A., et al. (2014). Modulation of the age at onset in spinocerebellar ataxia by CAG tracts in various genes. Brain 137, 2444–2455. doi: 10.1093/brain/awu174
The HD iPSC Consortium (2017). Developmental alterations in Huntington’s disease neural cells and pharmacological rescue in cells and mice. Nat. Neurosci. 20, 648–660. doi: 10.1038/nn.4532
The Huntington’s Disease Collaborative Research Group (1993). A novel gene containing a trinucleotide repeat that is expanded and unstable on Huntington’s disease chromosomes. Cell 72, 971–983.
Todd, P. K., and Paulson, H. L. (2010). RNA-mediated neurodegeneration in repeat expansion disorders. Ann. Neurol. 67, 291–300. doi: 10.1002/ana.21948
Tomoda, A., Ikezawa, M., Ohtani, Y., Miike, T., and Kumamoto, T. (1991). Progressive myoclonus epilepsy: dentato-rubro-pallido-luysian atrophy (DRPLA) in childhood. Brain Dev. 13, 266–269. doi: 10.1016/s0387-7604(12)80061-1
Toyoshima, Y., and Takahashi, H. (2018). “Spinocerebellar Ataxia Type 17 (SCA17),” in Polyglutamine Disorders Advances in Experimental Medicine and Biology, eds C. Nóbrega and L. Pereira de Almeida (Cham: Springer International Publishing), 219–231. doi: 10.1007/978-3-319-71779-1_10
Tsuchiya, K., Oyanagi, S., Arima, K., Ikeda, K., Akashi, T., Ando, S., et al. (1998). Dentatorubropallidoluysian atrophy: clinicopathological study of dementia and involvement of the nucleus basalis of meynert in seven autopsy cases. Acta Neuropathol. 96, 502–508. doi: 10.1007/s004010050925
Unrath, A., Müller, H.-P., Riecker, A., Ludolph, A. C., Sperfeld, A.-D., and Kassubek, J. (2010). Whole brain-based analysis of regional white matter tract alterations in rare motor neuron diseases by diffusion tensor imaging. Hum. Brain Mapp. 31, 1727–1740. doi: 10.1002/hbm.20971
van de Warrenburg, B. P., Frenken, C. W., Ausems, M. G., Kleefstra, T., Sinke, R. J., Knoers, N. V., et al. (2001). Striking anticipation in spinocerebellar ataxia type 7: the infantile phenotype. J. Neurol. 248, 911–914. doi: 10.1007/s004150170082
Vargas, A. P., Carod-Artal, F. J., Bomfim, D., Vázquez-Cabrera, C., and Dantas-Barbosa, C. (2003). Unusual early-onset Huntington’s disease. J. Child Neurol. 18, 429–432. doi: 10.1177/08830738030180061301
Veneziano, L., and Frontali, M. (1993). “DRPLA,” in GeneReviews®, eds M. P. Adam, H. H. Ardinger, R. A. Pagon, S. E. Wallace, L. J. Bean, K. Stephens, et al. (Seattle, WA: University of Washington, Seattle). Available online at: http://www.ncbi.nlm.nih.gov/books/NBK1491/ (accessed November 24, 2020).
Vinther-Jensen, T., Ek, J., Duno, M., Skovby, F., Hjermind, L. E., Nielsen, J. E., et al. (2013). Germ-line CAG repeat instability causes extreme CAG repeat expansion with infantile-onset spinocerebellar ataxia type 2. Eur. J. Hum. Genet. 21, 626–629. doi: 10.1038/ejhg.2012.231
Wang, X., and Putkey, J. A. (2016). PEP-19 modulates calcium binding to calmodulin by electrostatic steering. Nat. Commun. 7:13583. doi: 10.1038/ncomms13583
Wang, X., Wang, H., Xia, Y., Jiang, H., Shen, L., Wang, S., et al. (2010). A neuropathological study at autopsy of early onset spinocerebellar ataxia 6. J. Clin. Neurosci. 17, 751–755. doi: 10.1016/j.jocn.2009.10.007
Wardman, J. H., Henriksen, E. E., Marthaler, A. G., Nielsen, J. E., and Nielsen, T. T. (2020). Enhancement of autophagy and solubilization of ataxin-2 alleviate apoptosis in spinocerebellar ataxia type 2 patient cells. The Cerebellum 19, 165–181. doi: 10.1007/s12311-019-01092-8
Wiatr, K., Szlachcic, W. J., Trzeciak, M., Figlerowicz, M., and Figiel, M. (2018). Huntington disease as a neurodevelopmental disorder and early signs of the disease in stem cells. Mol. Neurobiol. 55, 3351–3371. doi: 10.1007/s12035-017-0477-7
Xia, Z., and Storm, D. R. (2005). The role of calmodulin as a signal integrator for synaptic plasticity. Nat. Rev. Neurosci. 6, 267–276. doi: 10.1038/nrn1647
Yamada, M. (2010). Dentatorubral-pallidoluysian atrophy (DRPLA): the 50th Anniversary of Japanese Society of Neuropathology. Neuropathology 30, 453–457. doi: 10.1111/j.1440-1789.2010.01120.x
Yang, S., Li, X.-J., and Li, S. (2016). Molecular mechanisms underlying Spinocerebellar Ataxia 17 (SCA17) pathogenesis. Rare Dis. 4:e1223580. doi: 10.1080/21675511.2016.1223580
Yoon, G., Kramer, J., Zanko, A., Guzijan, M., Lin, S., Foster-Barber, A., et al. (2006). Speech and language delay are early manifestations of juvenile-onset Huntington disease. Neurology 67, 1265–1267. doi: 10.1212/01.wnl.0000238390.86304.4e
Zhang, F., Xu, D., Yuan, L., Sun, Y., and Xu, Z. (2014). Epigenetic regulation of Atrophin1 by lysine-specific demethylase 1 is required for cortical progenitor maintenance. Nat. Commun. 5:5815. doi: 10.1038/ncomms6815
Zhuchenko, O., Bailey, J., Bonnen, P., Ashizawa, T., Stockton, D. W., Amos, C., et al. (1997). Autosomal dominant cerebellar ataxia (SCA6) associated with small polyglutamine expansions in the alpha 1A-voltage-dependent calcium channel. Nat. Genet. 15, 62–69. doi: 10.1038/ng0197-62
Keywords: Huntington’s disease, juvenile, spinocerebellar ataxia, DRPLA, transcriptomics, neurodevelopment, bioinformatic review, proteomics
Citation: Świtońska-Kurkowska K, Krist B, Delimata J and Figiel M (2021) Juvenile Huntington’s Disease and Other PolyQ Diseases, Update on Neurodevelopmental Character and Comparative Bioinformatic Review of Transcriptomic and Proteomic Data. Front. Cell Dev. Biol. 9:642773. doi: 10.3389/fcell.2021.642773
Received: 16 December 2020; Accepted: 10 June 2021;
Published: 01 July 2021.
Edited by:
Andreas Hermann, University Hospital Rostock, GermanyReviewed by:
Dan Lindholm, University of Helsinki, FinlandChandrasekar Raman, Joslin Diabetes Center, Harvard Medical School, United States
Copyright © 2021 Świtońska-Kurkowska, Krist, Delimata and Figiel. This is an open-access article distributed under the terms of the Creative Commons Attribution License (CC BY). The use, distribution or reproduction in other forums is permitted, provided the original author(s) and the copyright owner(s) are credited and that the original publication in this journal is cited, in accordance with accepted academic practice. No use, distribution or reproduction is permitted which does not comply with these terms.
*Correspondence: Maciej Figiel, bWZpZ2llbEBpYmNoLnBvem5hbi5wbA==