- 1Institute of Vertebrate Biology, Czech Academy of Sciences, Brno, Czechia
- 2Department of Botany and Zoology, Faculty of Science, Masaryk University, Brno, Czechia
- 3Department of Zoology, Faculty of Science, Charles University, Prague, Czechia
- 4Department of Ecology, Faculty of Science, Charles University, Prague, Czechia
The fatty acid composition of biological membranes has been hypothesised to be a key molecular adaptation associated with the evolution of metabolic rates, ageing, and life span – the basis of the membrane pacemaker hypothesis (MPH). MPH proposes that highly unsaturated membranes enhance cellular metabolic processes while being more prone to oxidative damage, thereby increasing the rates of metabolism and ageing. MPH could, therefore, provide a mechanistic explanation for trade-offs between longevity, fecundity, and metabolic rates, predicting that short-lived species with fast metabolic rates and higher fecundity would have greater levels of membrane unsaturation. However, previous comparative studies testing MPH provide mixed evidence regarding the direction of covariation between fatty acid unsaturation and life span or metabolic rate. Moreover, some empirical studies suggest that an n-3/n-6 PUFA ratio or the fatty acid chain length, rather than the overall unsaturation, could be the key traits coevolving with life span. In this study, we tested the coevolution of liver fatty acid composition with maximum life span, annual fecundity, and basal metabolic rate (BMR), using a recently published data set comprising liver fatty acid composition of 106 avian species. While statistically controlling for the confounding effects of body mass and phylogeny, we found no support for long life span evolving with low fatty acid unsaturation and only very weak support for fatty acid unsaturation acting as a pacemaker of BMR. Moreover, our analysis provided no evidence for the previously reported links between life span and n-3 PUFA/total PUFA or MUFA proportion. Our results rather suggest that long life span evolves with long-chain fatty acids irrespective of their degree of unsaturation as life span was positively associated with at least one long-chain fatty acid of each type (i.e., SFA, MUFA, n-6 PUFA, and n-3 PUFA). Importantly, maximum life span, annual fecundity, and BMR were associated with different fatty acids or fatty acid indices, indicating that longevity, fecundity, and BMR coevolve with different aspects of fatty acid composition. Therefore, in addition to posing significant challenges to MPH, our results imply that fatty acid composition does not pose an evolutionary constraint underpinning life-history trade-offs at the molecular level.
Introduction
The fatty acid (FA) composition of biological membranes has a strong influence on many cellular processes (Grecco et al., 2011). This fact has sparked the development of hypotheses that propose that the membrane FA composition is a key molecular adaptation underpinning the evolution of metabolic rates, ageing, and life span (Hulbert and Else, 1999; Pamplona et al., 2002; Hulbert, 2010). The most prominent of these hypotheses is the membrane pacemaker hypothesis (MPH), which proposes that the level of membrane FA unsaturation could act as a pacemaker of metabolism and ageing, thereby determining species-specific life span (Hulbert and Else, 1999; Hulbert, 2008). More specifically, MPH proposes two interacting molecular mechanisms linking membrane FA composition to the rates of metabolism and ageing.
First, the incorporation of unsaturated FA (i.e., those containing at least one double bond) into biological membranes enhances membrane fluidity (Jaureguiberry et al., 2014) and the activity of membrane proteins, which may enable higher cellular and organismal metabolic rates (Hulbert, 2008). Mechanistically, the increase in fluidity with unsaturation is attributed to the presence of cis double bonds that bend the acyl chains of unsaturated FA, resulting in greater interchain distances and, consequently, in the decrease in van der Waals force of intermolecular attraction (Hulbert and Else, 1999). The addition of polyunsaturated FA (PUFA) also modifies the spatial distribution of lipid rafts (i.e., the relatively ordered and rigid membrane lipid regions), pushing them closer to each other and stabilising raft-associated proteins (Shaikh et al., 2015). Both membrane fluidity and the effect of PUFA on the spatial distribution of lipid rafts may enhance the activity of membrane proteins important for energetic metabolism, such as glucose transporter proteins (Weijers, 2016) and Na+/K+ ATPases (Lingwood et al., 2005; Welker et al., 2007), respectively. These effects at the molecular level may eventually lead to higher metabolic rates at the cellular and organismal level (Hulbert, 2008). Higher metabolic rates are often associated with increased generation of free radicals, which induce oxidative damage to vital cellular components (Perez-Campo et al., 1998; da Costa et al., 2016). Hence, in addition to enhanced metabolic rates, higher membrane unsaturation can increase the rate of senescence according to MPH because the accumulation of oxidative damage has been proposed as a major cause of senescence by the free-radical theory of ageing (Barja, 2013).
Second, owing to the presence of highly reactive hydrogen atoms in their double bonds, unsaturated FA are known to be more prone to oxidative damage by free radicals and other reactive oxygen species (ROS; Holman, 1954; Hulbert, 2005). Based on this knowledge, MPH suggests that the level of membrane unsaturation influences senescence rate not only through increased ROS generation accompanying fast metabolic rates but also through susceptibility of unsaturated membranes to oxidative damage (Pamplona et al., 2002; Pamplona and Barja, 2011).
The appeal of MPH lies in its potential to provide a simple mechanistic explanation of the central paradigm of the life-history theory, namely the physiological trade-off between metabolic rate and fecundity on one side and the rate of senescence and longevity on the other (Stearns, 1989, 1992; Ricklefs and Wikelski, 2002). In other words, MPH implies that membrane unsaturation may be a major molecular mechanism constraining the evolution of life histories (or pace of life) along the fast–slow continuum with highly fecund, short-lived species on one end and long-lived species with low fecundity on the opposite end (Williams et al., 2010; Jimenez et al., 2014; Gaillard et al., 2016).
However, the causal role of ROS in senescence has been questioned (Lapointe and Hekimi, 2010; Speakman and Selman, 2011), challenging both the free-radical theory of ageing and MPH although such challenges have been dismissed as misconceptions by defenders of those two hypotheses (Barja, 2013) or may have resulted from methodological pitfalls (Munro and Pamenter, 2019). Moreover, the empirical support for MPH has been mixed so far, showing negative, no, or positive correlation between membrane unsaturation and life span or metabolic rate although studies focused on life span provide relatively more support for MPH (reviewed in Calhoon et al., 2015; Jové et al., 2020). It is also worth noting that many early studies supporting MPH are based on limited sample sizes (often comparing one short-lived mammal with one longer-lived avian species) or do not control for the confounding effects of body mass and phylogenetic non-independence of different species (Naudí et al., 2013; Valencak and Azzu, 2014; Calhoon et al., 2015; Bozek et al., 2017; Jové et al., 2020). Statistical control for the confounding effect of body mass is particularly important as the observed relationships could potentially arise due to the correlation of membrane unsaturation with body mass while not being associated with longevity directly (Speakman, 2005b; Valencak and Azzu, 2014). When controlling for body mass and phylogenetic autocorrelation, comparative studies using sufficient numbers of mammalian or avian species found no (Valencak and Ruf, 2007) or even a positive (Galván et al., 2015) relationship between FA unsaturation and maximum life span.
Several lines of experimental evidence further suggest that the association between FA unsaturation and susceptibility to oxidative stress may not be as straightforward as commonly assumed. For example, the peroxidation rate of FA in an aqueous environment may not reflect their degree of unsaturation (Visioli et al., 1998). In cell cultures and plants, PUFA have even been reported to efficiently scavenge ROS and protect other biomolecules from oxidative damage (Richard et al., 2008; Mène-Saffrané et al., 2009). The ROS-scavenging effect was positively related to the degree of PUFA unsaturation with n-3 PUFA being the most effective ones. Such observations led to the hypothesis that membranes rich in PUFA may act as structural antioxidants (Richard et al., 2008; Mène-Saffrané et al., 2009; Farmer and Mueller, 2013; Schmid-Siegert et al., 2016). Such an antioxidant function of PUFA could provide a possible explanation for the positive relationship between maximum life span and the degree of liver FA unsaturation reported by a recent phylogenetic comparative study on 107 bird species (Galván et al., 2015). However, despite the mixed support for MPH and the emergence of alternative hypotheses about PUFA properties (Richard et al., 2008; Mène-Saffrané et al., 2009; Farmer and Mueller, 2013; Schmid-Siegert et al., 2016), it is still widely assumed that long-lived species evolve membranes with low FA unsaturation (Schroeder and Brunet, 2015; Johnson and Stolzing, 2019; Papsdorf and Brunet, 2019; Jové et al., 2020).
To further complicate matters, a phylogenetic comparative study on 42 mammalian species found a negative relationship between maximum life span and n-3/n-6 PUFA ratio (Valencak and Ruf, 2007), which contrasts with the experimental evidence purporting antioxidant properties to n-3 PUFA. In addition, the length of the FA carbon chain could be a key trait coevolving with longevity as suggested by a recent comparative study on birds, which reported a positive relationship between life span and length of liver FA (Galván et al., 2015). However, such a positive relationship is in contrast with a mammalian comparative study, showing lower concentrations of long-chained FA in the blood plasma of long-lived species (Jové et al., 2013). It is unclear whether such contrasting results arise from physiological differences between taxa or because of different choices of tissues or statistical approaches among the studies. Consequently, the importance of membrane FA composition for life span or metabolic rate evolution remains an unresolved question (Calhoon et al., 2015).
Comparative studies may provide important insights into physiological and biochemical mechanisms modulating longevity and metabolic rates, considering the great variation in life-histories among various species even when body mass is accounted for Munro and Pamenter (2019). Here, we combine recently published data on liver FA of 106 European bird species (Galván et al., 2015) with published data on life-history traits (Fransson et al., 2017; Storchová and Hořák, 2018) and basal metabolic rate (BMR; McNab, 2009) to examine the links between FA composition and maximum life span, annual fecundity, or BMR. We include annual fecundity because reproduction is a highly energy-demanding process associated with elevated metabolic rates (Drent and Daan, 1980). To our knowledge, the coevolution of fecundity with FA composition has never been tested before even though it logically follows from the hypothesis that FA unsaturation should act as a pacemaker for metabolism and, hence, pace of life. The relationship between FA composition and maximum life span was analysed in the original study (Galván et al., 2015). The authors suggest that long life span evolves with higher proportions of long-chain and monounsaturated FA (MUFA), lower proportion of PUFA, and, surprisingly, higher overall level of unsaturation. They further observed that long life span was related to lower anti-inflammatory index, which is a ratio of anti-inflammatory FA to pre-inflammatory arachidonic acid. Nevertheless, Galván et al. (2015) relate life span to the first component of partial least squares regression, which, in addition to FA indexes, also include the effect of body mass and phylogeny. Hence, it is unclear to what extent the results of the original study may be affected by those confounding effects.
We employ Bayesian hierarchical (mixed effect) models, which allow us to perform beta regression within a phylogenetic context (Douma and Weedon, 2019) while controlling for the confounding effects of body mass and phylogeny. Beta regression is the preferred method to analyse proportional data, such as the proportions of FA because the commonly applied Gaussian regression of logit or square root–transformed data may yield biased estimates. The potential for such a bias is particularly high when the proportions are close to zero or one (Douma and Weedon, 2019) as is the case with many FA (Galván et al., 2015). Because differences in FA metabolism may evolve between sedentary and migratory species – either resulting from metabolic adaptations to long-distance flight (Jenni and Jenni-Eiermann, 1998; Guglielmo, 2010) or due to wintering in different environments (Calhoon et al., 2014; Jimenez et al., 2014) – we further include migratory behaviour as a covariate in the models. Additionally, sedentary and migratory species breeding in the temperate zone are also known to differ in their life-histories (Mönkkönen, 1992; Soriano-Redondo et al., 2020) and physiological components of pace of life (McNab, 2009; Gavrilov, 2014; Tomasek et al., 2019).
While statistically controlling for interspecific variation in body mass, migratory behaviour, and phylogeny, we aimed (i) to test whether maximum life span, annual fecundity, and BMR are associated with the aspects of FA composition proposed previously (i.e., FA unsaturation, n-3 PUFA/total PUFA proportion, average chain length, and anti-inflammatory index) and (ii) to carry out a detailed analysis exploring the associations between life-history traits and individual fatty acids.
Materials and Methods
FA Data
We obtained data on the total liver FA composition of 106 European bird species encompassing 15 taxonomic orders from Galván et al. (2015). The original data set consisted of 107 species, but we excluded common cuckoo (Cuculus canorus), which is an obligate brood parasite with indefinable clutch size and complete lack of parental care. The authors utilised liver tissues of free-living birds collected in Denmark to extract total lipids, followed by conversion of FA to FA methyl esters and quantification by gas chromatography. The molar proportions of individual FA were given as the mean values per species.
In addition to the proportions of individual FA, we further used the following set of FA indices available in the original data set: the proportions of saturated FA (SFA), MUFA, and PUFA; the proportion of n-3 PUFA to total PUFA (hereafter, n3 PUFA/total PUFA); average chain length (ACL); double bond index (DBI); peroxidizability index (PI); and anti-inflammatory index (AI). We calculated n-3 PUFA/total PUFA as an alternative to n-3/n6 PUFA ratio as analysing ratios in regression models is challenging (Sollberger and Ehlert, 2016). ACL indicates the average length of the FA carbon chain based on the proportional representation of individual FA. DBI gives the mean number of double bonds per 100 fatty acids. PI reflects the susceptibility of fatty acids to oxidative damage and is calculated as follows: PI = [(0.025 × Σmol% monoenoic) + (1 × Σmol% dienoic) + (2 × Σmol% trienoic) + (4 × Σmol% tetraenoic) + (6 × Σmol% pentaenoic) + (8 × Σmol% hexaenoic)] (Holman, 1954; Galván et al., 2015). AI is the percentage ratio of FA with anti-inflammatory properties (20:3n-6, 20:5n-3, 22:6n-3) to pre-inflammatory arachidonic acid (20:4n-6) (Galván et al., 2015).
Life-History and BMR Data
To control for the confounding effect of body mass (Speakman, 2005b), we used data on body mass from the original FA data set (Galván et al., 2015). We obtained maximum life span estimates predominantly from EURING (Fransson et al., 2017). Only for the water pipit (Anthus spinoletta), which had no maximum life span estimate in EURING, we used the corresponding record from AnAge longevity database (de Magalhães and Costa, 2009). We also extracted the number of recoveries of each species from EURING as capture effort is positively correlated with estimates of species maximum life span (Møller, 2008; Tomasek et al., 2019). To calculate annual fecundity (number of eggs laid per year), we utilised data on clutch size and number of clutches per year from Storchová and Hořák (2018).
Data on BMR came from the data set compiled by McNab (2009) except for the white-throated dipper (Cinclus cinclus) for which the value was obtained from a separate study (Bryant and Newton, 1994). BMR values were reported in kJ/h and adhered to the standard set of conditions necessary for metabolic rate measurements to be regarded as BMR (McKechnie and Wolf, 2004; McNab, 2009).
Migration Distance
Migration distance was calculated as a distance in thousands of kilometres between the centroid of the Jutland part of Denmark (original sampling location of data used in Galván et al., 2015) and the centroid of non-breeding distribution of each species using the haversine formula. Map data were obtained from BirdLife International and Handbook of the Birds of the World (2018). Centroids were calculated based on species distributions limited to Europe and Africa, using the ArcGIS 10.3 software (Esri, Redlands, CA, United States). The migration distance was set to zero in sedentary species.
Phylogenetic Data
Species phylogenetic non-independence is a form of autocorrelation due to shared ancestry (some species are more closely related to each other than to other species) and needs to be controlled for statistically to avoid biased parameter estimates (Speakman, 2005b). To control for the effect of phylogenetic non-independence, we used the most complete molecular phylogeny of extant bird species to date1 based on the phylogenetic hypotheses of Hackett et al. (2008) and Jetz et al. (2012). For the 106 species included in our study, we downloaded 10,000 phylogenetic trees and generated a consensus tree using the TreeAnnotator tool implemented in the BEAST 2.6.3 software (Bouckaert et al., 2019).
Statistical Analysis
We analysed the data using Bayesian hierarchical models implemented in the brms package (Bürkner, 2018) in R 4.0.3 software (R Core Team, 2020). We used this approach rather than the phylogenetic least squares because it allowed us to control for species-level phylogenetic non-independence while applying beta regression, which is the preferred method for continuous proportional data (Douma and Weedon, 2019). In addition, Bayesian inference does not need corrections for multiple testing because it estimates the probability of an effect of interest given the prior belief and the observed data and, unlike the frequentist framework, does not make assumptions about the distribution of p values in theoretical repetitions of an experiment (Berry and Hochberg, 1999; Gelman et al., 2012; Sjölander and Vansteelandt, 2019). Nevertheless, we follow the suggestion of Sjölander and Vansteelandt (2019) and adjust for multiple testing informally by discussing the effects while considering their dependence (Figure 2) and relative support (Figure 3).
In the beta regression models, we fitted proportions of the individual FA, SFA, MUFA, PUFA, or n-3 PUFA/total PUFA as response variables. In addition, we used Gaussian regression to model non-proportional response variables, namely ACL, DBI, PI, and log-transformed AI.
We fitted four types of regression models differing in the fixed-effect structure. First, to estimate the effect of body mass, we fitted simple phylogenetic regression models with body mass as the sole predictor (Supplementary Table 1). Second, to analyse the association of FA composition with life span, the models contained maximum life span as the focal predictor (Supplementary Table 2). These models further included body mass, number of recoveries, and migration distance among the predictors to control for their effects. Third, to analyse the association of FA composition with fecundity, we fitted models with annual fecundity, body mass, and migration distance as predictors (Supplementary Table 3). The fourth set of models included total BMR along with body mass as the predictors (Supplementary Table 4). We log-transformed body mass, annual fecundity, BMR, and the number of recoveries before fitting them in the models. We also standardised (z-transformed) each predictor and non-proportional response variable (ACL, DBI, PI, log-transformed AI) by subtracting its mean from the raw values and dividing them by standard deviation of the variable. Phylogeny was included in all the models as a random effect (Garamszegi, 2014). We used default priors calculated by the brms R package and ran the models in 25 chains, each with 6,000 iterations, 2,000 burn-in, and thinning set to 10. Potential scale reduction factor was <1.01 in all cases, indicating good model convergence (Gelman and Rubin, 1992).
We present resulting estimates as posterior means together with their equal-tailed 95% credible intervals based on quantiles (Bürkner, 2017). In the Bayesian framework, the 95% credible interval represents a range of values that, given the observed data, contain the true effect value with 95% probability. We consider the support for an effect to be significant if 95% credible intervals do not contain zero (Hespanhol et al., 2019).
Results
Interspecific Variation in Liver FA Composition
The abundance and interspecific variation in liver FA is visualised in Figure 1. The most abundant liver FA according to their median values were palmitic acid (C16:0; 23.3%), stearic acid (C18:0; 22.2%), oleic acid (C18:1n9; 21.1%), linoleic acid (C18:2n6; 9.3%), arachidonic acid (C20:4n6; 8.5%), docosahexaenoic acid (C22:6n3; 3.5%), and palmitoleic acid (C16:1n7; 1.3%). Medians of all the other FA were lower than 1%.
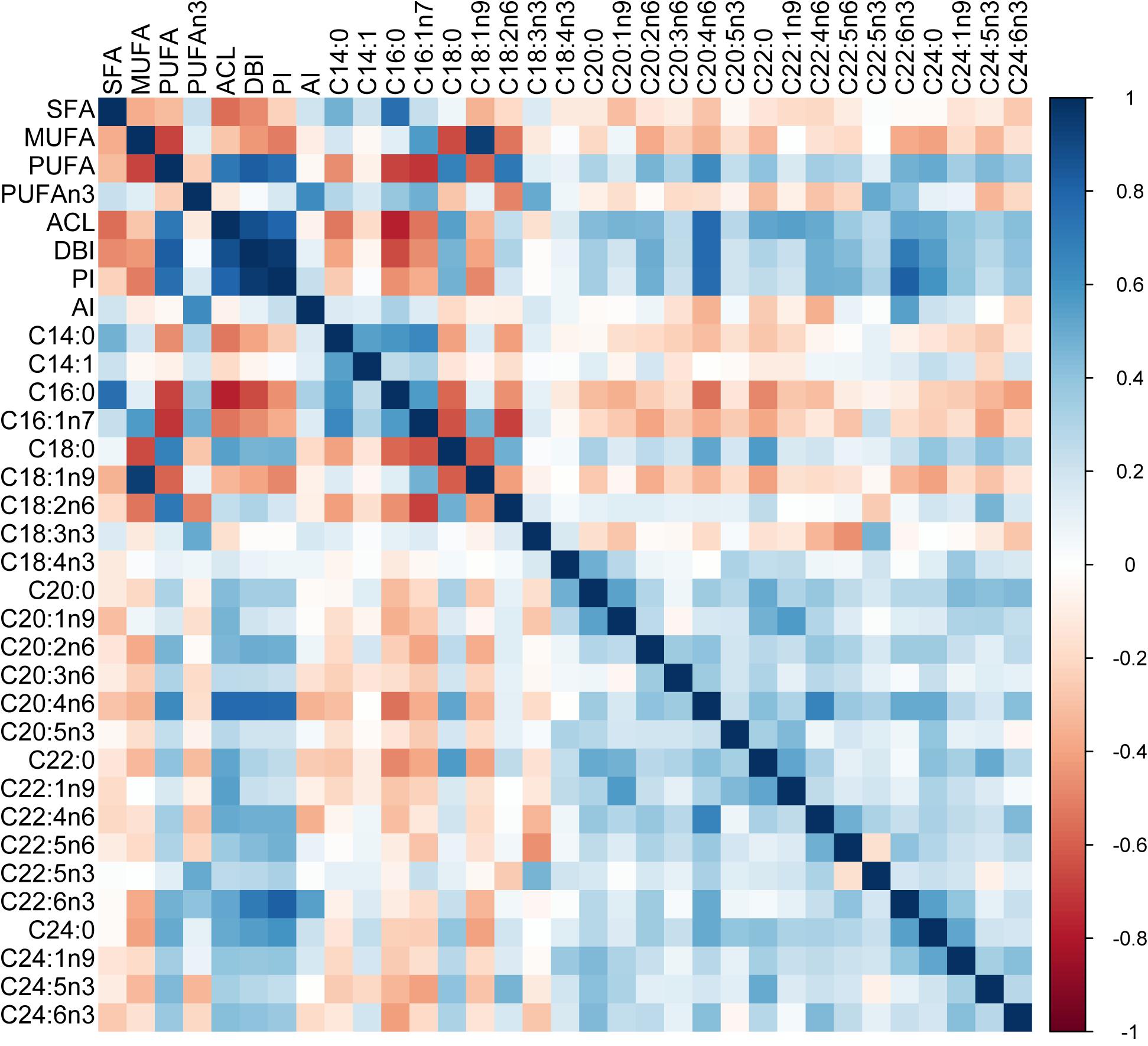
Figure 2. Pairwise correlations between residuals of FA indices and individual FA. Correlations were calculated using residuals from phylogenetic generalised least squares regression on body mass. The proportional variables were logit-transformed, and AI was log-transformed before the analysis. SFA – saturated fatty acids, MUFA – monounsaturated fatty acids, PUFA – polyunsaturated fatty acids, DBI – double bond index, PI – peroxidizability index, ACL – average chain length, AI – anti-inflammatory index.
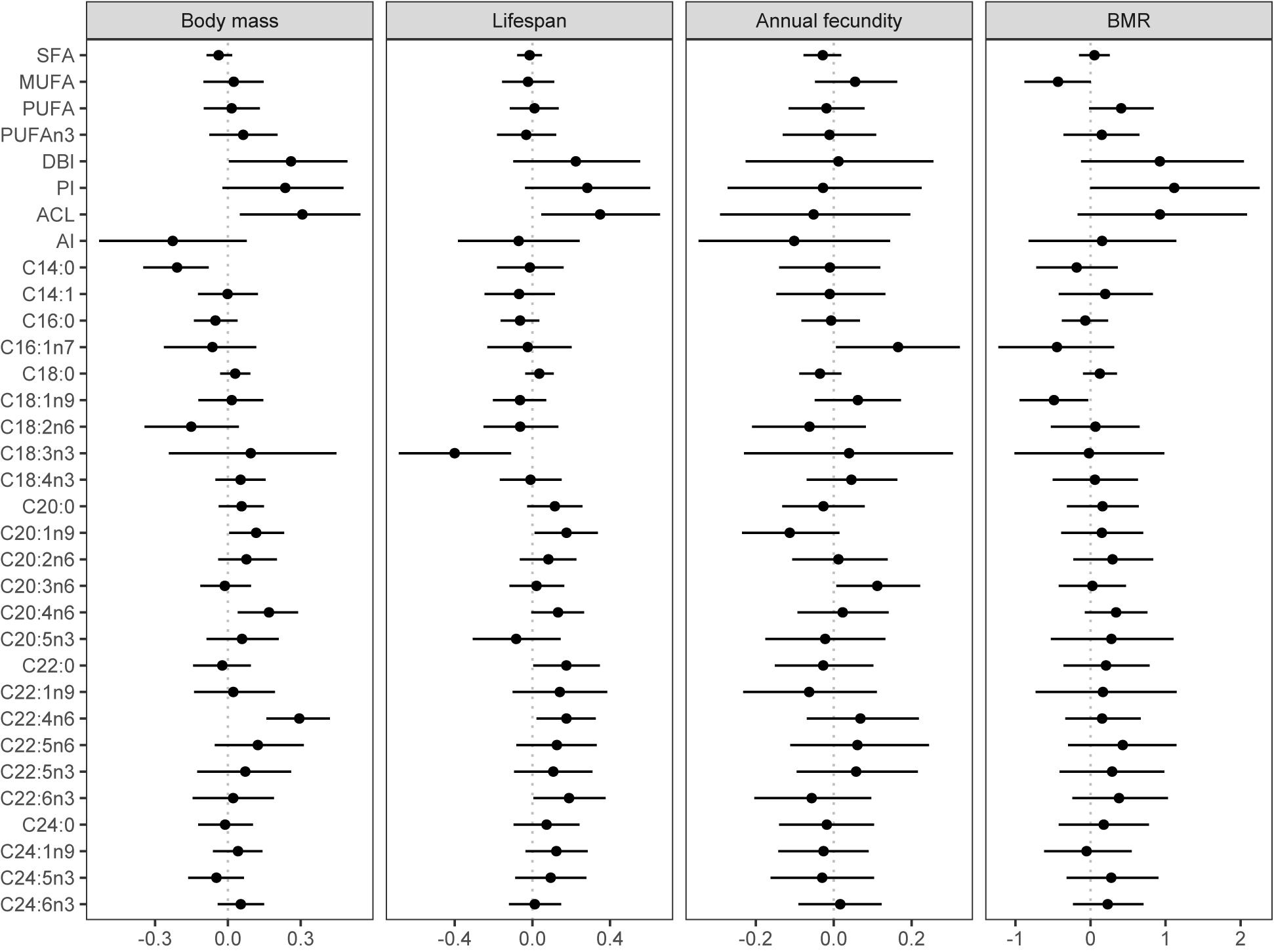
Figure 3. Effects of body mass, life span, annual fecundity, and BMR on liver FA composition. Shown are average marginal effects from Bayesian phylogenetic models and their 95% credible intervals. The 95% credible interval represents a range of values that, given the observed data, contain the true effect value with 95% probability. We consider the effect to be significantly supported if the 95% credible interval do not contain zero. Effect of body mass is from a model with body mass as a sole fixed-effect predictor. The effect of life span was controlled for body mass, number of recoveries, and migration distance. The effect of annual fecundity was controlled for body mass and migration distance. The effect of BMR was controlled for body mass. Body mass, life span, number of recoveries, annual fecundity, BMR, and anti-inflammatory index were log-transformed. All the non-proportional response and predictor variables were standardised by z-transformation. Beta regression and Gaussian regression were used for proportional and non-proportional data, respectively. SFA – saturated fatty acids, MUFA – monounsaturated fatty acids, PUFA – polyunsaturated fatty acids, DBI – double bond index, PI – peroxidizability index, ACL – average chain length, AI – anti-inflammatory index.
Index of overall FA unsaturation (DBI) and PI were strongly positively correlated to ACL and PUFA (Figure 2). At the level of individual FA, DBI, and PI showed the strongest positive correlations with C20:4n6 and C22:6n3 and the strongest negative correlation with C16:0. ACL was most strongly associated with C20:4n6. Besides this, C18:0 and all the FA with 20 or more carbons in their chains contributed to ACL as all of them showed positive correlations with ACL. In contrast, ACL was negatively correlated with C14:0, C16:0, C16:1n7, and C18:1n9. Moreover, the pattern of pairwise correlations between individual FA suggests that ACL is the primary axis of variation. We only show correlations of residuals from a phylogenetic generalised least squares regression on body mass in Figure 2 because there was no difference between correlations based on raw values. This indicates that the correlation structure of liver FA is independent of body mass and phylogeny.
Scaling of Liver FA Composition With Body Mass
With regards to the FA indices, only DBI and ACL exhibited a positive covariation with body mass (Figure 3; Supplementary Table 1). Body mass was not significantly associated with most individual FA. Only C14:0 showed a negative association with body mass, whereas C20:1n9, C20:4n6, and C22:4n6 showed a negative association.
Liver FA Composition and Life Span
In contradiction to MPH, our analysis did not reveal a significant relationship between SFA, MUFA, PUFA, DBI, or PI and maximum life span while controlling for confounding effects of body mass, migratory behaviour, and phylogeny (Supplementary Table 2; Figure 3). Such a result indicates that species life span is not determined by FA unsaturation. There was also no association of life span with n-3 PUFA/total PUFA or anti-inflammatory index. The only integrative component of FA composition that did correlate in a significant manner with maximum life span was ACL (Figure 3).
At the level of individual FA, long life span was associated with low levels of C18:3n3 and high levels of C20:1n9, C22:0, C22:4n6, and C22:6n3. There was also weak support for a positive covariation of maximum life span with 20:4n6, but the 95% credible intervals of the estimate marginally contained zero (Figure 3).
Liver FA Composition and Annual Fecundity
Our analysis did not reveal a significant correlation between any of the FA composition indices and annual fecundity. The only individual FA that were associated with annual fecundity were C16:1n7 and C20:3n6, both exhibiting positive association (Figure 3; Supplementary Table 3).
Liver FA Composition and BMR
Basal metabolic rate was not significantly associated with any of the indices of the liver FA composition although there were marginally non-significant trends toward a positive covariation with PUFA, DBI, and PI and a negative covariation with MUFA. Among the individual FA, only C18:1n9 showed a significant association in the negative direction (Figure 3; Supplementary Table 4).
Discussion
Our comparative analysis does not support the MPH as a whole because none of the key indices of FA unsaturation (DBI, PI, SFA, MUFA, and PUFA) covaried with maximum life span, BMR, or annual fecundity when controlled for the confounding effects of body mass, migration behaviour and phylogeny. Contrary to the previous analysis of the same data set (Galván et al., 2015), our results do not support the conclusion that long life span evolves with high MUFA and low PUFA content, which is commonly considered to be one of the mechanisms underpinning negative covariation between unsaturation and longevity (Schroeder and Brunet, 2015; Johnson and Stolzing, 2019; Papsdorf and Brunet, 2019; Jové et al., 2020). Moreover, maximum life span tended to positively covary, although non-significantly, with DBI and PI in our study, providing strong evidence against the prediction that long-lived species evolve membranes with low FA unsaturation.
Although the early comparative studies support a negative relationship between FA unsaturation and longevity, those studies are mostly based on only a low number of species, sometimes comparing one short-lived mammal species with one longer-lived bird species, and do not control for the confounding effects of body mass and phylogenetic non-independence (Naudí et al., 2013; Valencak and Azzu, 2014; Calhoon et al., 2015; Bozek et al., 2017; Jové et al., 2020). Modern phylogenetic comparative studies utilising larger sample sizes and controlling for body mass and phylogeny found no (Valencak and Ruf, 2007) or even positive (Galván et al., 2015) association between FA unsaturation and maximum life span. Similarly, the positive associations between FA unsaturation and BMR or calcium ATPase in muscles have not been supported in phylogenetic comparative studies on mammals and fish, respectively (Valencak and Ruf, 2007; Gonzalez et al., 2015). Moreover, one of the fundamental assumptions of MPH coming from the original rate-of-living theory (Pearl, 1928) – i.e., the negative correlation between metabolic rates and life span – has been refuted, at least at the interspecific level, by large comparative studies controlling for body mass and phylogeny (Speakman, 2005a; de Magalhães et al., 2007; Furness and Speakman, 2008; Møller, 2008). Our results fit into such emerging comparative evidence against MPH, specifically against the prediction that evolution of long life span is underpinned by low FA unsaturation. Nevertheless, marginally non-significant positive association between PI and BMR may provide some, although very weak, support for the second prediction of MPH, namely that higher FA unsaturation enhances metabolic rates (Hulbert and Else, 1999).
It is important to note, however, that the total liver lipids analysed in our study and that by Galván et al. (2015) also comprise lipids other than membrane phospholipids, such as triglycerides derived from the diet. Hence, caution should be exercised when interpreting the observed patterns as reflecting variation in membrane FA composition. However, it seems reasonable to assume that phospholipid FA contribute considerably to the patterns because phospholipids represent the majority of lipids in the avian liver (Grande and Prigge, 1970; Galván et al., 2015). Moreover, a phylogenetic analysis comparing the composition of muscle phospholipids across mammals similarly reported a lack of an association between life span and FA unsaturation or the contents of SFA, MUFA, and PUFA (Valencak and Ruf, 2007), suggesting that the absence of such relationships in our study is probably not due to a confounding effect of dietary triglycerides.
Our analysis does not support several conclusions of the original study analysing the same data set (Galván et al., 2015), namely associations of maximum life span with proportions of MUFA and PUFA, anti-inflammatory index, or several individual FA, including C18:1n9, C18:2n6, C20:3n6, C22:1n9, and C22:5n6. Instead, we identified some other FA to be associated with maximum life span (C18:3n3, C20:4n6, C22:0, and C22:6n3). Such a discrepancy is most probably down to the different statistical approaches employed. The conclusion of the original study was based on the significant contributions of those indices and FA to the life span–predicting component of partial least square regression; however, that component integrated variation shared by all the FA indices, body mass, and phylogeny. It is, therefore, unclear as to what extent MUFA covaried with maximum life span directly. Because the conventional phylogenetic regression model used in our study is more straightforward to interpret, the discrepancies between the studies suggest potential pitfalls of the interpretation of individual predictors in partial least squares regression. Another factor potentially contributing to such discrepant conclusions could be that the original study used square-root transformation of proportional data, which may lead to biased estimates (Douma and Weedon, 2019). In contrast, we used beta regression in our study, which is the preferred statistical method for proportional data (Douma and Weedon, 2019).
In accordance with the conclusions of the original study (Galván et al., 2015), our results show a positive covariation between maximum life span and ACL, supporting the role of FA chain length in life span evolution. Considering the differences in statistical approaches explained above, our analysis provides an improved interpretation of the positive longevity–ACL relationship as being independent of the confounding effects of body mass and phylogeny. Our analysis of individual FA further indicates that this covariation was mainly driven by long life span being associated with low levels of α-linolenic acid (C18:3n3) and high levels of gondoic acid (C20:1n9), behenic acid (C22:0), adrenic acid (C22:4n6), and docosahexaenoic acid (DHA; C22:6n3). Nevertheless, except for the eicosapentaenoic acid (C20:5n3), all the other FA with 20 or more carbons in their chains probably contributed to the longevity–ACL relationship because their models show positive regression coefficients of life span although with 95% credible intervals containing zero. Taken together, these results suggest that longevity does not evolve with any particular type of FA as life span was positively associated with at least one long-chain FA of each type (i.e., SFA, MUFA, n-6 PUFA, and n-3 PUFA).
Fatty acid unsaturation was positively associated with maximum life span in the original study (Galván et al., 2015). Here, we observed a similar, although non-significant, trend. On one hand, such a positive covariation between life span and unsaturation is consistent with the hypothesis that membranes rich in long-chain PUFA function as structural antioxidants (Richard et al., 2008; Mène-Saffrané et al., 2009; Farmer and Mueller, 2013; Schmid-Siegert et al., 2016). On the other hand, the fact that all the types of long-chain FA, irrespective of their unsaturation, covaried positively with life span in our analysis indicates that unsaturation is not the key trait in life span evolution. Moreover, ACL showed a much stronger association with life span than DBI or PI in our study, suggesting that the positive covariation of unsaturation indices with life span more likely reflects a tight relationship between fatty acid unsaturation and chain length (Figure 2).
Another feature of FA composition proposed to be linked to life span evolution is the n-3/n-6 PUFA ratio (Valencak and Ruf, 2007). Specifically, long life span is reported to evolve with relatively smaller proportions of n-3 PUFA in muscle phospholipids across mammals (Valencak and Ruf, 2007). It is further suggested that a low level of DHA, a highly unsaturated n-3 PUFA, is particularly important for the evolution of long life span due to its high susceptibility to peroxidation (Pamplona et al., 1998; Hulbert, 2003). Our results do not support the importance of n-3/n-6 PUFA ratio, however, as the n-3 PUFA/total PUFA did not show any association with maximum life span. Moreover, DHA was significantly positively related to maximum life span in our analysis, which is contrary to the proposed negative correlation (Pamplona et al., 1998; Hulbert, 2003). The positive association between DHA and life span is intriguing and has not been observed in previous comparative studies, including the original study analysing the same data set using partial least squares analysis (Valencak and Ruf, 2007; Galván et al., 2015). Such a pattern provides other evidence against MPH. It is rather consistent with the hypothesis that membranes with long-chain PUFA act as structural antioxidants (Richard et al., 2008; Mène-Saffrané et al., 2009; Farmer and Mueller, 2013; Schmid-Siegert et al., 2016). As discussed, however, the positive association of DHA with life span may merely reflect the overall importance of long-chain FA irrespective of their unsaturation.
Docosahexaenoic acid was further proposed as an important pacemaker of metabolic rate (Hulbert and Else, 1999; Hulbert, 2003) based on its observed enhancing effect on the molecular activity of Na+/K+-ATPase (Turner et al., 2003). Our results do not support such a role of DHA at the interspecific level in birds as DHA was not significantly associated with BMR. This is in accordance with the findings of a previous comparative study in mammals, which also reported no relation between DHA and BMR (Valencak and Ruf, 2007). Hence, DHA is probably not an important molecular adaptation underpinning metabolic rate evolution.
Nevertheless, the main factor hypothesised to underpin a variation in metabolic rates is membrane unsaturation (Hulbert and Else, 1999). Our findings provide weak support for this hypothesis, revealing marginally non-significant positive covariation of BMR with DBI, PI, and PUFA and negative covariation with MUFA. These patterns contrast with the results reported by the Valencak and Ruf (2007) comparative study on mammals, which found no link between BMR and DBI, PUFA, or MUFA and even a negative association of BMR with PI. However, the high level of MUFA in species with low BMR observed in our analysis is in accordance with the recent study in dogs, demonstrating a decrease in cellular metabolic rate following an enrichment of fibroblasts with MUFA (Jimenez et al., 2020). It is important to note, however, that 95% credible intervals of our estimates marginally contained zero, and we performed a high number of comparisons in our analysis. Clearly, more phylogenetic comparative studies focused on FA composition of phospholipids are needed to validate the hypothesised macroevolutionary link between membrane unsaturation and metabolic rates.
In conclusion, our analysis presents significant challenges to the current hypotheses about the role of FA composition in life span and life-history evolution. We found no support for membrane unsaturation, MUFA/PUFA ratio, or n-3/n-6 PUFA ratio playing an important role in life span evolution as commonly assumed based on MPH (Hulbert, 2003; Schroeder and Brunet, 2015; Johnson and Stolzing, 2019; Papsdorf and Brunet, 2019; Jové et al., 2020). Our results rather support the importance of FA chain length for life span evolution as proposed by the previous study (Galván et al., 2015). Our contribution to such a conclusion lies in a clear demonstration that the positive longevity–ACL relationship is independent of body mass and phylogeny. We only found weak support for the second MPH prediction, namely that high membrane unsaturation promotes high metabolic rates, thereby functioning as a pacemaker of metabolic rates; however, the weakness of the effect prevents any firm conclusion in this regard. We also identified specific types of FA associated with maximum life span, annual fecundity, and BMR evolution that may represent promising avenues for future research. A striking finding of our study is that life span, fecundity, and BMR are each associated with different FA or FA indices. Not even a single FA showed significant association with more than one life-history trait or BMR. Such a pattern indicates that maximum life span, annual fecundity, and BMR coevolve with different aspects of liver FA composition. Such differences imply that liver FA composition does not pose molecular constraints underpinning life-history trade-offs.
Data Availability Statement
The original contributions presented in the study are included in the article/Supplementary Material, further inquiries can be directed to the corresponding author.
Ethics Statement
Ethical review and approval was not required for the animal study because publicly available data were only used in this study.
Author Contributions
OT conceived and designed the study with input from TA. SK, OK, and OT collected and processed the data. SK and OT analysed data with input from TA. SK and OT wrote the manuscript with input from TA and OK. All authors approved the submitted version.
Funding
This study was funded through the Czech Science Foundation (GA17-24782S to TA and GA21-22160S to TA and OT).
Conflict of Interest
The authors declare that the research was conducted in the absence of any commercial or financial relationships that could be construed as a potential conflict of interest.
Acknowledgments
Maps of species distribution were kindly provided by BirdLife International and Handbook of the Birds of the World. Computational resources were supplied by the project “e-Infrastruktura CZ” (e-INFRA LM2018140) provided within the program Projects of Large Research, Development and Innovations Infrastructures.
Supplementary Material
The Supplementary Material for this article can be found online at: https://www.frontiersin.org/articles/10.3389/fcell.2021.638501/full#supplementary-material
Abbreviations
ACL, average chain length; AI, anti-inflammatory index; DBI, double bond index; DHA, docosahexaenoic acid; MPH, membrane pacemaker hypothesis; PI, peroxidizability index.
Footnotes
References
Barja, G. (2013). Updating the mitochondrial free radical theory of aging: an integrated view, key aspects, and confounding concepts. Antioxid. Redox Signal. 19, 1420–1445. doi: 10.1089/ars.2012.5148
Berry, D. A., and Hochberg, Y. (1999). Bayesian perspectives on multiple comparisons. J. Statist. Plan. Infer. 82, 215–227. doi: 10.1016/S0378-3758(99)00044-0
BirdLife International and Handbook of the Birds of the World (2018). Bird Species Distribution Maps of the World. Version 2018.1. Available online at: http://datazone.birdlife.org/species/requestdis (accessed November 29, 2019).
Bouckaert, R., Vaughan, T. G., Barido-Sottani, J., Duchêne, S., Fourment, M., Gavryushkina, A., et al. (2019). BEAST 2.5: an advanced software platform for Bayesian evolutionary analysis. PLoS Comput. Biol. 15:e1006650. doi: 10.1371/journal.pcbi.1006650
Bozek, K., Khrameeva, E. E., Reznick, J., Omerbašić, D., Bennett, N. C., Lewin, G. R., et al. (2017). Lipidome determinants of maximal lifespan in mammals. Sci. Rep. 7:5. doi: 10.1038/s41598-017-00037-7
Bryant, D. M., and Newton, A. V. (1994). Metabolic costs of dominance in dippers, Cinclus cinclus. Anim. Behav. 48, 447–455. doi: 10.1006/anbe.1994.1258
Bürkner, P.-C. (2017). brms: an R package for Bayesian multilevel models using Stan. J. Stat. Soft. 80, 1–28. doi: 10.18637/jss.v080.i01
Bürkner, P.-C. (2018). Advanced Bayesian multilevel modeling with the R package brms. R. J. 10:395. doi: 10.32614/RJ-2018-017
Calhoon, E. A., Jimenez, A. G., Harper, J. M., Jurkowitz, M. S., and Williams, J. B. (2014). Linkages between mitochondrial lipids and life history in temperate and tropical birds. Physiol. Biochem. Zool. 87, 265–275. doi: 10.1086/674696
Calhoon, E. A., Ro, J., and Williams, J. B. (2015). Perspectives on the membrane fatty acid unsaturation/pacemaker hypotheses of metabolism and aging. Chem. Phys. Lipids 191, 48–60. doi: 10.1016/j.chemphyslip.2015.08.008
da Costa, J. P., Vitorino, R., Silva, G. M., Vogel, C., Duarte, A. C., and Rocha-Santos, T. (2016). A synopsis on aging—Theories, mechanisms and future prospects. Age. Res. Rev. 29, 90–112. doi: 10.1016/j.arr.2016.06.005
de Magalhães, J. P., and Costa, J. (2009). A database of vertebrate longevity records and their relation to other life-history traits. J. Evol. Biol. 22, 1770–1774. doi: 10.1111/j.1420-9101.2009.01783.x
de Magalhães, J. P., Costa, J., and Church, G. M. (2007). An analysis of the relationship between metabolism, developmental schedules, and longevity using phylogenetic independent contrasts. J. Gerontol. A Biol. Sci. Med. 62, 149–160. doi: 10.1093/gerona/62.2.149
Douma, J. C., and Weedon, J. T. (2019). Analysing continuous proportions in ecology and evolution: a practical introduction to beta and Dirichlet regression. Methods Ecol. Evol. 10, 1412–1430. doi: 10.1111/2041-210X.13234
Drent, R. H., and Daan, S. (1980). The prudent parent: energetic adjustments in avian breeding. Ardea 38–90, 225–252. doi: 10.5253/arde.v68.p225
Farmer, E. E., and Mueller, M. J. (2013). ROS-mediated lipid peroxidation and RES-activated signaling. Annu. Rev. Plant Physiol. 64, 429–450. doi: 10.1146/annurev-arplant-050312-120132
Fransson, T., Jansson, L., Kolehmainen, T., Kroon, C., and Wenninger, T. (2017). EURING List of Longevity Records for European Birds. Available online at: https://euring.org/data-and-codes/longevity-list (accessed November 24, 2018).
Furness, L. J., and Speakman, J. R. (2008). Energetics and longevity in birds. Age 30, 75–87. doi: 10.1007/s11357-008-9054-3
Gaillard, J.-M., Lemaître, J.-F., Berger, V., Bonenfant, C., Devillard, S., Douhard, M., et al. (2016). “Life histories, axes of variation in,” in Encyclopedia of Evolutionary Biology, Vol. 2, ed. R. M. Kliman (Amsterdam: Elsevier), 312–323. doi: 10.1016/B978-0-12-800049-6.00085-8
Galván, I., Naudí, A., Erritzře, J., Møller, A. P., Barja, G., and Pamplona, R. (2015). Long lifespans have evolved with long and monounsaturated fatty acids in birds. Evolution 69, 2776–2784. doi: 10.1111/evo.12754
Garamszegi, L. Z. (2014). “Uncertainties due to within-species variation in comparative studies: measurement errors and statistical weights,” in Modern Phylogenetic Comparative Methods and Their Application in Evolutionary Biology, ed. L. Z. Garamszegi (Heidelberg: Springer), 157–199.
Gavrilov, V. M. (2014). Ecological and scaling analysis of the energy expenditure of rest, activity, flight, and evaporative water loss in passeriformes and non-passeriformes in relation to seasonal migrations and to the occupation of boreal stations in high and moderate latitudes. Q. Rev. Biol. 89, 107–150. doi: 10.1086/676046
Gelman, A., Hill, J., and Yajima, M. (2012). Why we (usually) don’t have to worry about multiple comparisons. J. Res. Educ. Effect. 5, 189–211. doi: 10.1080/19345747.2011.618213
Gelman, A., and Rubin, D. B. (1992). Inference from iterative simulation using multiple sequences. Statist. Sci. 7, 457–472. doi: 10.1214/ss/1177011136
Gonzalez, A., Pagé, B., and Weber, J.-M. (2015). Membranes as a possible pacemaker of metabolism in cypriniform fish: does phylogeny matter? J. Exp. Biol. 218, 2563–2572. doi: 10.1242/jeb.117630
Grande, F., and Prigge, W. F. (1970). Glucagon infusion, plasma FFA and triglycerides, blood sugar, and liver lipids in birds. Am. J. Physiol. 218, 1406–1411. doi: 10.1152/ajplegacy.1970.218.5.1406
Grecco, H. E., Schmick, M., and Bastiaens, P. I. H. (2011). Signaling from the living plasma membrane. Cell 144, 897–909. doi: 10.1016/j.cell.2011.01.029
Guglielmo, C. G. (2010). Move that fatty acid: fuel selection and transport in migratory birds and bats. Integr. Comp. Biol. 50, 336–345. doi: 10.1093/icb/icq097
Hackett, S. J., Kimball, R. T., Reddy, S., Bowie, R. C. K., Braun, E. L., Braun, M. J., et al. (2008). A phylogenomic study of birds reveals their evolutionary history. Science 320, 1763–1768. doi: 10.1126/science.1157704
Hespanhol, L., Vallio, C. S., Costa, L. M., and Saragiotto, B. T. (2019). Understanding and interpreting confidence and credible intervals around effect estimates. Braz. J. Phys. Ther. 23, 290–301. doi: 10.1016/j.bjpt.2018.12.006
Holman, R. T. (1954). Autoxidation of fats and related substances. Prog. Chem. Fats Other Lipids 2, 51–98. doi: 10.1016/0079-6832(54)90004-X
Hulbert, A. (2003). Life, death and membrane bilayers. J. Exp. Biol. 206, 2303–2311. doi: 10.1242/jeb.00399
Hulbert, A. J. (2005). On the importance of fatty acid composition of membranes for aging. J. Theor. Biol. 234, 277–288. doi: 10.1016/j.jtbi.2004.11.024
Hulbert, A. J. (2008). The links between membrane composition, metabolic rate and lifespan. Comp. Biochem. Physiol. A Mol. Integr. Physiol. 150, 196–203. doi: 10.1016/j.cbpa.2006.05.014
Hulbert, A. J. (2010). Metabolism and longevity: is there a role for membrane fatty acids? Integr. Comp. Biol. 50, 808–817. doi: 10.1093/icb/icq007
Hulbert, A. J., and Else, P. L. (1999). Membranes as possible pacemakers of metabolism. J. Theor. Biol. 199, 257–274. doi: 10.1006/jtbi.1999.0955
Jaureguiberry, M. S., Tricerri, M. A., Sanchez, S. A., Finarelli, G. S., Montanaro, M. A., Prieto, E. D., et al. (2014). Role of plasma membrane lipid composition on cellular homeostasis: learning from cell line models expressing fatty acid desaturases. Acta Biochim. Biophys. Sin. 46, 273–282. doi: 10.1093/abbs/gmt155
Jenni, L., and Jenni-Eiermann, S. (1998). Fuel supply and metabolic constraints in migrating birds. J. Avian Biol. 29, 521–528. doi: 10.2307/3677171
Jetz, W., Thomas, G. H., Joy, J. B., Hartmann, K., and Mooers, A. O. (2012). The global diversity of birds in space and time. Nature 491, 444–448. doi: 10.1038/nature11631
Jimenez, A. G., Cooper-Mullin, C., Calhoon, E. A., and Williams, J. B. (2014). Physiological underpinnings associated with differences in pace of life and metabolic rate in north temperate and neotropical birds. J. Comp. Physiol. B 184, 545–561. doi: 10.1007/s00360-014-0825-0
Jimenez, A. G., Winward, J. D., Walsh, K. E., and Champagne, A. M. (2020). Effects of membrane fatty acid composition on cellular metabolism and oxidative stress in dermal fibroblasts from small and large breed dogs. J. Exp. Biol. 223:jeb221804. doi: 10.1242/jeb.221804
Johnson, A. A., and Stolzing, A. (2019). The role of lipid metabolism in aging, lifespan regulation, and age-related disease. Aging Cell 18:e13048. doi: 10.1111/acel.13048
Jové, M., Mota-Martorell, N., Pradas, I., Galo-Licona, J. D., Martín-Gari, M., and Obis, È (2020). The lipidome fingerprint of longevity. Molecules 25:4343. doi: 10.3390/molecules25184343
Jové, M., Naudí, A., Aledo, J. C., Cabré, R., Ayala, V., Portero-Otin, M., et al. (2013). Plasma long-chain free fatty acids predict mammalian longevity. Sci. Rep. 3:sre03346. doi: 10.1038/srep03346
Lapointe, J., and Hekimi, S. (2010). When a theory of aging ages badly. Cell. Mol. Life Sci. 67, 1–8. doi: 10.1007/s00018-009-0138-8
Lingwood, D., Harauz, G., and Ballantyne, J. S. (2005). Regulation of fish gill Na(+)-K(+)-ATPase by selective sulfatide-enriched raft partitioning during seawater adaptation. J. Biol. Chem. 280, 36545–36550. doi: 10.1074/jbc.M506670200
McKechnie, A. E., and Wolf, B. O. (2004). The allometry of avian basal metabolic rate: good predictions need good data. Physiol. Biochem. Zool. 77, 502–521. doi: 10.1086/383511
McNab, B. K. (2009). Ecological factors affect the level and scaling of avian BMR. Comp. Biochem. Physiol. A 152, 22–45. doi: 10.1016/j.cbpa.2008.08.021
Mène-Saffrané, L., Dubugnon, L., Chételat, A., Stolz, S., Gouhier-Darimont, C., and Farmer, E. E. (2009). Nonenzymatic oxidation of trienoic fatty acids contributes to reactive oxygen species management in Arabidopsis. J. Biol. Chem. 284, 1702–1708. doi: 10.1074/jbc.M807114200
Møller, A. P. (2008). Relative longevity and field metabolic rate in birds. J. Evol. Biol. 21, 1379–1386. doi: 10.1111/j.1420-9101.2008.01556.x
Mönkkönen, M. (1992). Life history traits of Palaearctic and Nearctic migrant passerines. Ornis Fennica 69, 161–172.
Munro, D., and Pamenter, M. E. (2019). Comparative studies of mitochondrial reactive oxygen species in animal longevity: technical pitfalls and possibilities. Aging Cell 18:e13009. doi: 10.1111/acel.13009
Naudí, A., Jové, M., Ayala, V., Portero-Otín, M., Barja, G., and Pamplona, R. (2013). Membrane lipid unsaturation as physiological adaptation to animal longevity. Front. Physiol. 4:372. doi: 10.3389/fphys.2013.00372
Pamplona, R., and Barja, G. (2011). An evolutionary comparative scan for longevity-related oxidative stress resistance mechanisms in homeotherms. Biogerontology 12:409. doi: 10.1007/s10522-011-9348-1
Pamplona, R., Barja, G., and Portero-Otín, M. (2002). Membrane fatty acid unsaturation, protection against oxidative stress, and maximum life span: a homeoviscous-longevity adaptation? Ann. N. Y. Acad. Sci. 959, 475–490. doi: 10.1111/j.1749-6632.2002.tb02118.x
Pamplona, R., Portero-Otín, M., Riba, D., Ruiz, C., Prat, J., Bellmunt, M. J., et al. (1998). Mitochondrial membrane peroxidizability index is inversely related to maximum life span in mammals. J. Lipid Res. 39, 1989– 1994.
Papsdorf, K., and Brunet, A. (2019). Linking lipid metabolism to chromatin regulation in aging. Trends Cell Biol. 29, 97–116. doi: 10.1016/j.tcb.2018.09.004
Perez-Campo, R., Lopez-Torres, M., Cadenas, S., Rojas, C., and Barja, G. (1998). The rate of free radical production as a determinant of the rate of aging: evidence from the comparative approach. J. Comp. Physiol. B Biochem. Syst. Environ. Physiol. 168, 149–158.
R Core Team (2020). R: A Language and Environment for Statistical Computing. Vienna: R Foundation for Statistical Computing.
Richard, D., Kefi, K., Barbe, U., Bausero, P., and Visioli, F. (2008). Polyunsaturated fatty acids as antioxidants. Pharmacol. Res. 57, 451–455. doi: 10.1016/j.phrs.2008.05.002
Ricklefs, R. E., and Wikelski, M. (2002). The physiology/life-history nexus. Trends. Ecol. Evol. 17, 462–468. doi: 10.1016/S0169-5347(02)02578-8
Schmid-Siegert, E., Stepushenko, O., Glauser, G., and Farmer, E. E. (2016). Membranes as structural antioxidants. Recycling of malondialdehyde to its source in oxidation-sensitive chloroplast fatty acids. J. Biol. Chem. 291, 13005–13013. doi: 10.1074/jbc.M116.729921
Schroeder, E. A., and Brunet, A. (2015). Lipid profiles and signals for long life. Trends Endocrinol. Metab. 26, 589–592. doi: 10.1016/j.tem.2015.08.007
Shaikh, S. R., Kinnun, J. J., Leng, X., Williams, J. A., and Wassall, S. R. (2015). How polyunsaturated fatty acids modify molecular organization in membranes: insight from NMR studies of model systems. Biochim. Biophys. Acta Biomembr. 1848, 211–219. doi: 10.1016/j.bbamem.2014.04.020
Sjölander, A., and Vansteelandt, S. (2019). Frequentist versus Bayesian approaches to multiple testing. Eur. J. Epidemiol. 34, 809–821. doi: 10.1007/s10654-019-00517-2
Sollberger, S., and Ehlert, U. (2016). How to use and interpret hormone ratios. Psychoneuroendocrinology 63, 385–397. doi: 10.1016/j.psyneuen.2015.09.031
Soriano-Redondo, A., Gutiérrez, J. S., Hodgson, D., and Bearhop, S. (2020). Migrant birds and mammals live faster than residents. Nat. Commun. 11:5719. doi: 10.1038/s41467-020-19256-0
Speakman, J. R. (2005a). Body size, energy metabolism and lifespan. J. Exp. Biol. 208, 1717–1730. doi: 10.1242/jeb.01556
Speakman, J. R. (2005b). Correlations between physiology and lifespan - two widely ignored problems with comparative studies. Aging Cell 4, 167–175. doi: 10.1111/j.1474-9726.2005.00162.x
Speakman, J. R., and Selman, C. (2011). The free-radical damage theory: accumulating evidence against a simple link of oxidative stress to ageing and lifespan. Bioessays 33, 255–259. doi: 10.1002/bies.201000132
Storchová, L., and Hořák, D. (2018). Life-history characteristics of European birds. Glob. Ecol. Biogeogr. 27, 400–406. doi: 10.1111/geb.12709
Tomasek, O., Bobek, L., Kralova, T., Adamkova, M., and Albrecht, T. (2019). Fuel for the pace of life: baseline blood glucose concentration co-evolves with life-history traits in songbirds. Funct. Ecol. 33, 239–249. doi: 10.1111/1365-2435.13238
Turner, N., Else, P. L., and Hulbert, A. J. (2003). Docosahexaenoic acid (DHA) content of membranes determines molecular activity of the sodium pump: implications for disease states and metabolism. Naturwissenschaften 90, 521–523. doi: 10.1007/s00114-003-0470-z
Valencak, T. G., and Azzu, V. (2014). Making heads or tails of mitochondrial membranes in longevity and aging: a role for comparative studies. Longev. Healthspan 3:3. doi: 10.1186/2046-2395-3-3
Valencak, T. G., and Ruf, T. (2007). N-3 polyunsaturated fatty acids impair lifespan but have no role for metabolism. Aging Cell 6, 15–25. doi: 10.1111/j.1474-9726.2006.00257.x
Visioli, F., Colombo, C., and Galli, C. (1998). Oxidation of individual fatty acids yields different profiles of oxidation markers. Biochem. Biophys. Res. Commun. 245, 487–489. doi: 10.1006/bbrc.1998.8463
Weijers, R. N. M. (2016). Membrane flexibility, free fatty acids, and the onset of vascular and neurological lesions in type 2 diabetes. J. Diabetes Metab. Disord. 15:13. doi: 10.1186/s40200-016-0235-9
Welker, P., Geist, B., Frühauf, J.-H., Salanova, M., Groneberg, D. A., Krause, E., et al. (2007). Role of lipid rafts in membrane delivery of renal epithelial Na+-K+-ATPase, thick ascending limb. Am. J. Physiol. Regul. Integr. Comp. Physiol. 292, R1328–R1337. doi: 10.1152/ajpregu.00166.2006
Keywords: membrane pacemaker hypothesis, life-history trade-offs, pace-of-life syndromes, membrane unsaturation, evolution of longevity, ageing, aging, senescence
Citation: Kumar SA, Albrecht T, Kauzál O and Tomášek O (2021) No Evidence for Trade-Offs Between Lifespan, Fecundity, and Basal Metabolic Rate Mediated by Liver Fatty Acid Composition in Birds. Front. Cell Dev. Biol. 9:638501. doi: 10.3389/fcell.2021.638501
Received: 06 December 2020; Accepted: 23 February 2021;
Published: 29 March 2021.
Edited by:
Owen Jones, University of Southern Denmark, DenmarkReviewed by:
Arne Sahm, Leibniz Institute on Aging, Fritz Lipmann Institute (FLI), GermanyHerbert Fuhrmann, Leipzig University, Germany
Copyright © 2021 Kumar, Albrecht, Kauzál and Tomášek. This is an open-access article distributed under the terms of the Creative Commons Attribution License (CC BY). The use, distribution or reproduction in other forums is permitted, provided the original author(s) and the copyright owner(s) are credited and that the original publication in this journal is cited, in accordance with accepted academic practice. No use, distribution or reproduction is permitted which does not comply with these terms.
*Correspondence: Oldřich Tomášek, dG9tYXNla0BpdmIuY3o=