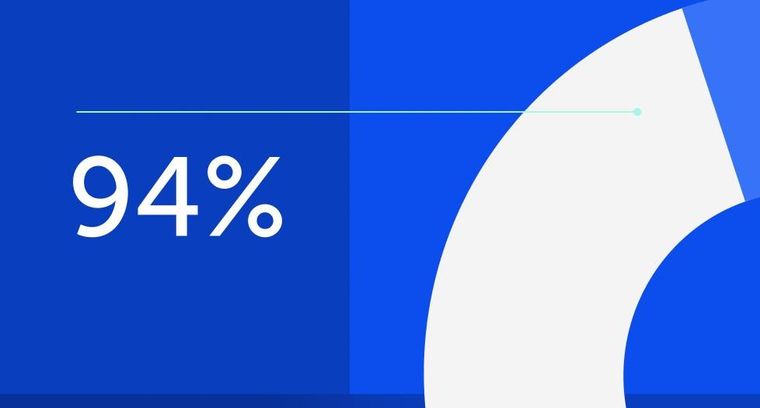
94% of researchers rate our articles as excellent or good
Learn more about the work of our research integrity team to safeguard the quality of each article we publish.
Find out more
REVIEW article
Front. Cell Dev. Biol., 21 January 2021
Sec. Signaling
Volume 9 - 2021 | https://doi.org/10.3389/fcell.2021.631623
This article is part of the Research TopicWnt Signaling at the Plasma Membrane: Activation, Regulation and Disease ConnectionView all 16 articles
Wnt signaling is one of the key signaling pathways that govern numerous physiological activities such as growth, differentiation and migration during development and homeostasis. As pathway misregulation has been extensively linked to pathological processes including malignant tumors, a thorough understanding of pathway regulation is essential for development of effective therapeutic approaches. A prominent feature of cancer cells is that they significantly differ from healthy cells with respect to their plasma membrane composition and lipid organization. Here, we review the key role of membrane composition and lipid order in activation of Wnt signaling pathway by tightly regulating formation and interactions of the Wnt-receptor complex. We also discuss in detail how plasma membrane components, in particular the ligands, (co)receptors and extracellular or membrane-bound modulators, of Wnt pathways are affected in lung, colorectal, liver and breast cancers that have been associated with abnormal activation of Wnt signaling. Wnt-receptor complex components and their modulators are frequently misexpressed in these cancers and this appears to correlate with metastasis and cancer progression. Thus, composition and organization of the plasma membrane can be exploited to develop new anticancer drugs that are targeted in a highly specific manner to the Wnt-receptor complex, rendering a more effective therapeutic outcome possible.
The Wnt signaling pathway is an evolutionarily conserved signal transduction cascade that controls a wide range of biological events from embryonic development to tissue regeneration (Nusse, 2005; Aman et al., 2018; Steinhart and Angers, 2018). The pathway is broadly divided into two branches as the canonical (β-catenin-dependent) Wnt signaling and the non-canonical (β-catenin-independent) Wnt signaling, which is further branched into the Wnt/planar cell polarity (PCP) and the Wnt/Ca2+ pathways. Wnt signaling pathways play a multitude of essential roles in cell fate determination, cell polarity, cell migration and patterning during embryonic development, adult tissue homeostasis and regeneration of various tissues and organs. Consequently, misregulation of Wnt signaling has been associated with a variety of human diseases including developmental defects, degenerative diseases, and many cancers. Although numerous components of the Wnt pathways have been characterized for their functional roles, many questions related to tight regulation and modifications of signaling remain unanswered. Besides, despite many efforts, so far no drugs have been approved to specifically target the pathway, leading to a gap in targeted therapy of Wnt-related diseases (Nusse and Clevers, 2017; Krishnamurthy and Kurzrock, 2018). A better understanding of the molecular mechanisms underlying Wnt-mediated cellular responses is especially critical for development of effective anticancer drugs that are expected to interfere with the highly activated Wnt signaling in many cancers (Jung and Park, 2020).
The plasma membrane plays a dual central role in regulation of cell signaling. On the one hand, it acts as a barrier and ensures spatial segregation between extracellular environment and the cytosolic compartment. At the same time, by harboring many cell surface receptors and regulators that are involved in cell signaling, it actively controls transmission of molecular signals from the exterior to the interior of a cell and precisely links them to downstream signaling events. The plasma membrane is likewise vital for initiation of Wnt signaling where Wnt ligands bind to their receptor complexes in specialized membrane domains that are considered as dynamic assemblies of various saturated lipids, sterols and lipid-anchored proteins (Sezgin et al., 2017a, b, 2015) (Figure 1). Owing to their central roles in initiation of signaling, Wnt pathway components acting at the plasma membrane have been frequently investigated as drug targets (Gurney et al., 2012; Krishnamurthy and Kurzrock, 2018; Zeng et al., 2018a). Since plasma membranes of healthy and diseased cells display major structural differences, the influence of membrane nanoenvironment on signaling function should be considered carefully when developing novel therapeutic approaches that target Wnt pathways at the membrane.
Figure 1. Structure of the plasma membrane. The plasma membrane consists of ordered and disordered domains. Basically, ordered domains are enriched in cholesterol, sphingolipids, glycerophosholipids, glycosylphosphatidylinositol (GPI)-anchored proteins, glycoproteins, dimeric proteins, and saturated lipids, whereas the disordered domains usually contain monomeric and transmembrane proteins and unsaturated lipids. Created with BioRender.com.
Here, we review the cellular mechanisms underlying regulation of Wnt signaling pathways at the plasma membrane domains with a specific focus on the role of lipid molecules. Next, we address how Wnt pathways are misregulated at the plasma membrane through their ligands, receptors and membrane associated pathway modulators in cancer. Since Wnt signaling has been associated with numerous cancers, here we will focus on four common cancers, i.e., lung, colorectal, liver, and breast cancers. We also discuss the therapeutic approaches that aim to inhibit aberrant signaling activity in cancer by targeting the Wnt-receptor complex at the plasma membrane.
Wnt signaling pathway regulates proliferation, survival, polarity, and migration, differentiation of cells as well as maintenance of stem cells of various lineages during embryonic development and tissue homeostasis (Clevers et al., 2014). Examples come from the stem cells that reside in different regions of the body including the digestive tract, hematopoietic system and the nervous system (Lee et al., 2000; Willert et al., 2003; Nusse et al., 2008). The Wnt target gene Lgr5 has been identified as a stem cell marker at the crypts of small intestine and colon (Barker et al., 2007). Further studies have revealed that Lgr5 and Axin2 are expressed in the stem cells of multiple organs such as the liver, mammary gland, stomach, brain, kidney, cochlea and ovary and associated with widespread self-renewal capacity (Barker et al., 2010, 2012; van Amerongen et al., 2012; Bowman et al., 2013; Flesken-Nikitin et al., 2013; Huch et al., 2013; Jan et al., 2013). Thus, by ensuring the formation of the stem cell pool and its continuity via asymmetric divisions, Wnt signals play critical roles in establishing niches for stem cells in various tissues and organs and determining molecular programs of tissue regeneration.
The Wnts consist of a large family of protein ligands that interact with a number of receptors and co-receptors at the plasma membrane. Based on 19 Wnt ligands and 10 Fz receptors in mammals, these interactions constitute one of the most complex relationships between extracellular ligands and cell surface receptors (Clevers and Nusse, 2012). While some Wnt ligands are associated with a particular Wnt signaling pathway, others that cannot easily be attributed to a single Wnt pathway are competent to initiate signaling in more than one branch.
Being the most studied Wnt pathway, canonical Wnt signaling operates through cytoplasmic accumulation of β-catenin protein and is essential for embryonic development, adult homeostasis and stem cell maintenance (Nusse and Clevers, 2017). In the absence of canonical Wnt ligand, β-catenin is phosphorylated by a multiprotein destruction complex that includes the serine-threonine kinases glycogen synthase kinase 3β (Gsk3β) and casein kinase 1α (Ck1α), the scaffold protein Axin and the cytoplasmic effector proteins dishevelled (Dvl) and Adenomatous Polyposis Coli (APC), and targeted for degradation by the ubiquitin-proteasome system (Kimelman and Xu, 2006; MacDonald et al., 2009). Active canonical Wnt ligand, however, binds to its cell surface receptor frizzled (Fz) and co-receptor low-density lipoprotein receptor-related protein 5/6 (Lrp5/6) and recruits Dvl and Axin to the plasma membrane, leading to disassembly of the destruction complex (Figure 2). β-catenin then accumulates in the cytoplasm, translocates into the nucleus and interacts with the T-cell factor/lymphoid enhancer factor (TCF/LEF) family of transcription factors to regulate the expression of target genes (Logan and Nusse, 2004; Taketo, 2004; Nusse, 2005; Angers and Moon, 2009; Clevers and Nusse, 2012).
Figure 2. The Wnt signaling pathways at the plasma membrane. Canonical Wnt signaling pathway: In the Wnt OFF state, defined by the absence of canonical Wnt ligand, β-catenin is phosphorylated by the destruction complex that is comprised of glycogen synthase kinase 3β (GSK3β) and casein kinase 1α (CK1α), Axin and dishevelled (DVL) and Adenomatous Polyposis Coli (APC). Ring finger protein 43 (RNF43) also inhibits the pathway by binding the Fz receptor via DVL. In the Wnt ON state, canonical Wnt ligand binds to the Frizzled (Fz) receptor and the low-density lipoprotein receptor-related protein 5/6 (LRP5/6) co-receptor. This interaction recruits DVL and AXIN to the Wnt-receptor complex and leads to disassembly of the destruction complex, causing stabilization of β-catenin in the cytosol. Binding of R-spondin to leucine-rich repeat-containing G-protein coupled receptor 5 (LGR5) enhances Wnt signaling activity by neutralizing RNF43. Non-canonical Wnt signaling pathways: In the PCP pathway, non-canonical Wnt ligands bind to the ROR/RYK-Fz receptor complex and recruit DVL. Next phosphorylation of VANGL activates the pathway. In calcium pathway, binding of non-canonical Wnt ligands to ROR/RYK-Fz recruits DVL and activated DVL binds to the small GTPase that further activates phospholipase C (PLC). Created with BioRender.com.
Acting independently of β-catenin, the non-canonical Wnt/PCP pathway coordinates cell movement and tissue polarity via small GTPase RhoA or c-Jun N-terminal kinase (JNK) (Katoh, 2005). The typical non-canonical ligands Wnt5a, Wnt5b, and Wnt11 initiate the PCP signaling via interacting with their receptors Fz3 or Fz6 and co-receptors Ror1, Ror2, or Ptk7 (Humphries and Mlodzik, 2018). The human planar cell polarity proteins Vangl1, Vangl2, Prickle1, and Prickle2, Cadherin EGF LAG seven-pass G-type receptors (Celsr1, Celsr2, Celsr3), Dvl1, Dvl2, Dvl3, and Ankyrin repeat domain 6 (Ankrd6) are involved in the signaling cascade (Figure 2). Dvl-dependent Wnt/PCP signals are transduced through the Dishevelled-associated activator of morphogenesis (Daam1 and Daam2) proteins or Mitogen activated protein (MAP) kinase kinase kinase (MAPKKK) and MAPKK4/7 and activate the Ras homolog family member A-Rho-associated protein kinase (RhoA-Rock) or Rac-JNK signaling cascades, respectively. On the other hand, the Dvl-independent G protein-dependent non-canonical Wnt pathway operates through G proteins, Receptor tyrosine kinases (RTKs) and Phospholipase C (PLC), leading to intracellular calcium release, activation of the serine/threonine protein phosphatase calcineurin and accumulation of Nuclear factor of activated T cells (NFAT) in the nucleus (Katoh, 2005; Kohn and Moon, 2005; Katoh and Katoh, 2017). Elevated cytoplasmic calcium can also activate Nemo-like kinase (NLK) signaling pathway through Calcium/calmodulin dependent kinase II (CaMKII).
Both canonical and non-canonical Wnt signaling pathways are tightly regulated by a number of modulators that are evolutionarily conserved and function either extracellularly to regulate ligand-receptor interactions or intracellularly to modify cytosolic or nuclear components of the pathway. Extracellular modulators can be either secreted, membrane-bound or transmembrane proteins and are broadly classified into two groups according to their functions: (1) The agonists that include Norrin, R-spondins (Rspos), GPI-anchored membrane proteins Ly6/Plaur domain-containing 6 (Lypd6) and Reck, G protein-coupled receptors Gpr124 and Gpr37 and (2) the antagonists that include secreted Dickkopf proteins (Dkks), Secreted frizzled-related proteins (Sfrps), secreted Wnt-inhibitory factor 1 (Wif-1), secreted Wise/Sost proteins, secreted protein Cerberus, secreted insulin-like growth-factor binding protein 4 (Igfbp-4), secreted palmitoleoyl-protein carboxylesterase Notum, single-transmembrane proteins Shisa, Wnt-activated inhibitory factor 1 (Waif1/5T4), adenomatosis polyposis coli down-regulated 1 (Apcdd1), membrane-bound metalloprotease Tiki1, transmembrane E3 ubiquitin ligase Zinc and ring finger 3 (Znrf3) and its functional homolog Ring finger protein 43 (Rnf43), (Cruciat and Niehrs, 2013; Jiang and Cong, 2016; Berger et al., 2017; Eubelen et al., 2018). Owing to their critical roles in ligand or receptor modification, ligand-receptor complex formation, regulation of signaling activity in membrane subdomains and receptor internalization, these secreted and membrane proteins are noteworthy candidates for therapeutic targeting of Wnt signaling pathways.
The plasma membrane has a complex heterogeneous and highly dynamic structure that is composed of lipids and proteins with varying features and compartmentalized into numerous smaller structures called micro- or nanodomains (Eggeling et al., 2009; Owen et al., 2012; Sarmento et al., 2020). This heterogeneity leads to formation of ordered membrane domains, also known as lipid rafts or lipid nanodomains, which are assembled from saturated lipids, sphingolipids, sterols, glycolipids, glycoproteins and certain lipid-anchored proteins such as glycosylphosphatidylinositol (GPI)-anchored proteins and fatty acylated proteins, leaving the relatively disordered domains occupied by unsaturated lipids and a large fraction of membrane proteins (Sezgin et al., 2015, 2017b; Fakhree et al., 2019; Kusumi et al., 2020; Figure 1). The ordered domains of the plasma membrane have been shown to be essential for cell signaling events by controlling membrane-cytoskeleton communication, ligand-receptor interaction and receptor clustering (Simons and Toomre, 2000; Thomas et al., 2004; Ozhan et al., 2013; Agarwal et al., 2018; Azbazdar et al., 2019). For example, activation of epidermal growth factor receptor (EGFR) signaling by the epidermal growth factor (EGF) has been proposed to induce coalescence of different lipid rafts and formation of signaling platforms (Hofman et al., 2009; Irwin et al., 2011). After exposure to the Gram-negative bacterial cell wall component lipopolysaccharides, Toll-like receptor 4 (TLR4) of the immune system cells likewise interacts with its sorting adaptor Toll/interleukin-1 receptor domain-containing adaptor protein (TIRAP) in the lipid rafts (Barnett and Kagan, 2020).
Interaction of the canonical Wnt ligand with its (co)receptors triggers Lrp6 phosphorylation and endocytosis of receptor complexes, both of which appear to occur preferentially in the ordered membrane domains (Yamamoto et al., 2008; Sakane et al., 2010). While appearing in a way unexpected due to the fact that majority of the receptor Fz and the co-receptor Lrp6 are preferentially localized in the disordered membrane domains, both processes are facilitated by the GPI-anchored Lypd6 protein that interacts with Lrp6, helps it to associate with the ordered membrane domains via its GPI anchor and enhances Wnt/β-catenin signaling by promoting Lrp6 phosphorylation in these domains (Ozhan et al., 2013). Lipid raft disruption via induction of cholesterol efflux from the membrane with methyl-β-cyclodextrin significantly reduces Lrp6 phosphorylation in neuroblastoma cells, indicating that membrane order and integrity is essential for Lrp6 activity (Riitano et al., 2020). Further work has revealed that the canonical Wnts bind to their receptor complexes selectively in the ordered membrane domains (Sezgin et al., 2017a). Disruption of the membrane order in vitro or in vivo using inhibitors that specifically target lipids associated with the ordered domains significantly reduces canonical Wnt signaling activity, underscoring the influence of the plasma membrane lipid content on early interaction of the canonical Wnt with its receptors and downstream signaling activity (Sezgin et al., 2017a). Presence of canonical Wnt ligand stimulates enrichment of cholesterol, an essential component of the ordered membrane domains, in the inner membrane leaflet and receptor clustering that is likely aided by other membrane components such as heparin sulfate and phosphatidylinositol-4,5-bisphosphate (PIP2) (Mii et al., 2017; Erazo-Oliveras et al., 2018). Defects in synthesis of cholesterol thus reduces Wnt signaling and results in abnormalities in craniofacial development and neural crest cell differentiation (Sezgin et al., 2017a; Castro et al., 2020). The glycosphingolipid mannosyl glucosylceramide, which assembles with sterols into ordered membrane domains, likewise enhances presynaptic Wnt1/Wingless (Wg) signaling in the lipid rafts and promotes synaptic bouton formation at the Drosophila neuromuscular junction (Huang et al., 2018). Wnt/β-catenin signaling can also act in combination with Reactive Oxygen Species (ROS) signaling to regulate nuclear β-catenin levels during neural differentiation in a lipid raft dependent manner (Haack et al., 2015).
Regulation of Wnt-receptor interaction in ordered domains through membrane proteins appears not to be restricted to canonical Wnt signaling. The heparan sulfate proteoglycan Glypican-4 (Gpc4) is one such bifunctional molecule that controls canonical and non-canonical Wnt pathways by binding to Wnt3a and Lrp6 in the ordered domains and to Wnt5a and Ror2 in the disordered domains, respectively (Sakane et al., 2012). Likewise, autocrine Wnt10b has been shown to be drafted to the ordered domains by the fibroblast-derived (FD) exosomes and activate mTOR signaling that in turn promotes axonal regeneration independently of β-catenin (Tassew et al., 2017). Conversely, inhibition of fatty acid synthase (FASN), the major source of long-chain fatty acids such as palmitate, interferes with lipid biosynthesis, disrupts ordered membrane architecture and inhibits Wnt/β-catenin signaling along with PI3K-AKT-mTOR pathways, most likely also inhibiting protein lipidation that is necessary for proper signal transduction (Ventura et al., 2015).
Apart from the components of the Wnt-receptor complex, N-terminally dephosphorylated (dephospho) β-catenin, the de novo synthesized form of β-catenin that correlates with Wnt signaling activity, has been reported to colocalize at the plasma membrane with Lrp6 and two members of the destruction complex, namely APC and Axin, shortly after Wnt stimulation and independently of E-cadherin (Hendriksen et al., 2008). Routing of dephospho-β-catenin to the membrane via the Wnt receptor complex appears to constitute a key step in regulation of its transcriptional activity and Wnt signaling. The activity of β-catenin as a membrane component may be controlled by other molecules under certain ambient conditions. For example, non-muscle myosin II induces accumulation of cortical F-actin and E-cadherin to the adherens junctions, resulting in corecruitment of β-catenin to the membrane to maintain cellular contraction and inhibition of Wnt signaling due to reduced levels of cytoplasmic β-catenin (Hall et al., 2019). Cytoskeletal networks have also been reported to regulate Wnt signaling activity through plasma membrane domains during differentiation of stem cells (von Erlach et al., 2018).
Interaction of Wnts ligands with their surface receptors within particular membrane domains activate an immediate biochemical response that triggers internalization of the Wnt signalosome, a dynamic signaling complex assembled by Dvl upon formation of the Wnt-receptor complex (Bienz, 2014; Gammons et al., 2016). Internalization of signalosome is essential for pathway activation that is balanced by degradation of excessive ligands and clearance of surface receptors, ultimately downregulating the signaling (Feng and Gao, 2015). At the same time, paradoxically, receptor-mediated endocytosis negatively regulates Wnt signaling through internalization and degradation of the receptor complex. The route of endocytosis is determined by multiple factors including the types of ligands and receptors, other molecules in the environment, feedback regulatory mechanisms and how the signal is terminated.
Canonical Wnt-mediated receptor complex is known to be endocytosed via the clathrin-independent route in the smooth invaginations of the plasma membrane, the so-called caveolae, which form as subset of the ordered membrane domains (Parton et al., 2006; Yamamoto et al., 2006; Bilic et al., 2007; Gong et al., 2008). Tyrosine-based motifs within the cytoplasmic tail of Lrp6 has been shown to be essential for determining its distribution within the membrane domains and its internalization routes, which is critical to keep signal activity under control (Liu et al., 2014). However, a large body of evidence supports that clathrin-dependent endocytosis of the Wnt-receptor complex can also enhance β-catenin-dependent signaling and that clathrin is a prerequisite for Wnt signalosome formation (Kim et al., 2013; Hagemann et al., 2014; Gammons et al., 2016; Brunt and Scholpp, 2018). Endocytosis has been proposed to regulate Wnt/β-Catenin signaling mainly via four alternative ways that include early endosomal acidification of the co-receptor Lrp6 for pathway activation, sequestration of GSK-3 to limit β-catenin proteolysis, clearance of ubiquitin ligases that target Wnt receptors for degradation and facilitation of signaling by stabilization of Dvl (Brunt and Scholpp, 2018).
β-catenin-independent Wnt pathway operates through clathrin-dependent endocytic route that mediates uptake of PCP components together with Syndecans, the transmembrane proteoglycans (Yamamoto et al., 2008; Ohkawara et al., 2011). Wnt/PCP signaling regulates cell adhesion and migration by regulating internalization of cadherins via protocadherins during vertebrate gastrulation (Brinkmann et al., 2016; Brunt and Scholpp, 2018). Recent data obtained from plasma membrane capacitance recordings have further supported that non-canonical Wnt5a is exclusively endocytosed via clathrin-coated vesicles (Bandmann et al., 2019).
Endocytosis of the Wnt-receptor complex can also be affected by different molecules and pathways. For example, in addition to regulating proteolytic degradation of β-catenin as part of the destruction complex, APC keeps Wnt receptor internalization and pathway activation under control by forming a complex with clathrin (Saito-Diaz et al., 2018). AP2-associated kinase 1 (Aak1), which is activated by Wnt/β-catenin signaling, likewise promotes clathrin-mediated endocytosis of Lrp6 and thus negatively regulates the pathway (Agajanian et al., 2019). The mammalian target of rapamycin complex 1 (mTORC1) signaling has also been shown to inhibit Wnt/β-catenin signaling by suppressing the expression of membrane Fz through enhancing its Dvl-dependent clathrin-mediated internalization (Zeng et al., 2018b). Further mechanistic studies on the interplay between Wnt signaling and other molecules will provide a deeper understanding of the tight control of pathway activity.
Wnts are 350–400 amino acid-long cysteine-rich secreted glycoproteins that are highly conserved in metazoans. Mammals have 19 Wnt ligands that act through the canonical or non-canonical Wnt pathway and thus contributes to the pathway specificity and complexity (Clevers and Nusse, 2012).
The high number of conserved cysteine residues that Wnt proteins harbor suggest that the intra- and inter- molecular disulfide bonds are important for the proper folding, multimerization and function of Wnt proteins (Tang et al., 2012). Wnts undergo two main types of posttranslational modifications, i.e., N-glycosylation and lipidation/acylation at the endoplasmic reticulum (ER) and are subsequently transported to the plasma membrane via the cargo protein Wntless (Wls)/Evi, which is essential for Wnt secretion (Glaeser et al., 2018; Gradilla et al., 2018). Various mutations introduced at N-linked glycosylation sites of different Wnt proteins have shown that glycosylation is essential for proper folding and secretion of Wnt proteins but dispensable for their signaling activity (Mason et al., 1992; Komekado et al., 2007; Kurayoshi et al., 2007; Tang et al., 2012).
Being a prominent mode of lipidation, acylation of Wnts takes place at conserved amino acid residues through palmitoylation and is mainly catalyzed by the protein Porcupine, a membrane-bound O-acyltransferase of the ER (Willert and Nusse, 2012; Gao and Hannoush, 2014; Nile and Hannoush, 2016). Initial mass spectrometry-based studies on mouse Wnt3a propounded two different sites for addition of palmitoyl moieties, which were a thioester-linked palmitic acid at a conserved cysteine and an oxyester-linked palmitoleic acid at a conserved serine (Willert et al., 2003; Takada et al., 2006). While the conserved serine has been confirmed as the consensus acylation site across all Wnts by high-resolution crystal structure analysis of Xenopus Wnt8, the conserved cysteine was found to be engaged in a disulfide bond, thus preventing it from serving as a lipidation site (Janda et al., 2012). Interestingly, canonical Wnts have been proposed to be acylated by palmitoleic acid, a monounsaturated fatty acid, which is assumed to exhibit a kinked conformation and thus fit into the cavity of the Porcupine (Takada et al., 2006; Nile et al., 2017; Lee et al., 2018, 2019). However, considering the fact that palmitoylation targets soluble proteins into ordered membrane domains, palmitoylation of Wnt by a monounsaturated fatty acid contradicts with its preferential binding in the ordered domains and ability to activate signaling therein (Zhai et al., 2004; Levental et al., 2010; Ozhan et al., 2013; Sezgin et al., 2017a). Computational structural analyses have indeed supported that canonical Wnt is likely modified by palmitic acid, a saturated fatty acid, by adopting a conformation compatible with the stereochemical features of Wnt modification (Azbazdar et al., 2019).
The role of lipidation in Wnt secretion and functionality has been investigated by mutagenesis of the conserved palmitoylation sites of various Wnt proteins (Kurayoshi et al., 2007; Franch-Marro et al., 2008; Tang et al., 2012; Luz et al., 2014; Hosseini et al., 2019; Speer et al., 2019). For example, palmitoylation mutants of mouse Wnt1 and Wnt3a were found to be secreted at varying levels while their signaling activities were significantly and consistently reduced (Takada et al., 2006; Doubravska et al., 2011; Galli and Burrus, 2011; Gao and Hannoush, 2014). Acylation mutant of Drosophila Wg was likewise found to be secreted normally but with markedly weaker signaling activity (Franch-Marro et al., 2008). Strikingly, several non-acylated mutants of Wnt1, Wnt3a, and Wnt8 from different species have been identified to vary dramatically in their rates of secretion, interactions with the receptor Fz and abilities to activate signaling in vitro or in vivo (Speer et al., 2019). Studies on the zebrafish canonical Wnt ligands have reported that Wnt palmitoylation is essential for activation of signaling but may be dispensable for secretion (Luz et al., 2014; Azbazdar et al., 2019). APT1-mediated depalmitoylation has been shown to be important for asymmetric localization of β-catenin and Wnt signaling activity during development (Stypulkowski et al., 2018). Interestingly, Drosophila WntD does not appear to undergo any glycolysis and acylation (Ching et al., 2008). Therefore, the impact of acylation on the secretion and function of different types of Wnts appears to vary significantly. We believe that individual and context-dependent characterization of Wnt ligands will provide critical insight into the how Wnt signaling activity is modified in different types of cancers and how therapeutic approaches could be specifically designed.
Wnt signaling pathways control a plethora of cellular responses involved in development, homeostasis and disease. Thus, the molecular interactions underlying initiation of Wnt pathways at the plasma membrane have the potential to serve as attractive drug targets, especially for cancer where Wnt signaling is broadly misregulated. The disclosure of the functional role of plasma membrane organization in Wnt/β-catenin signaling will shed light on how the membrane background can be exploited for therapeutic approaches:
(1) The drugs can be packed in specific lipid-based drug delivery systems to directly target them to the relatively ordered domains of the membrane where Wnt-receptor complex formation occurs.
(2) The peptide drugs can be modified by introducing particular lipid moieties that help them target Wnt-receptor complex more precisely. Posttranslational lipid modifications, in particular palmitoylation, might be a good candidate to enhance domain-specific receptor targeting.
(3) A very convenient strategy would be lipid fingerprinting of cancer cells to characterize membrane lipid profiles of individual cell types. This would enable selectively inhibition of aberrant activated Wnt signaling pathway by direct modification of membrane lipid composition.
Overall, we believe that unraveling plasma membrane organization with respect to (mis)regulation of Wnt signaling in health and disease will help not only develop new strategies on targeted anticancer therapies and but also increase target specificity of existing drugs that interfere with the ligand-receptor complex at the plasma membrane.
Dysregulation of plasma membrane domains with respect to its structural organization and dynamics, disruption of membrane protein and lipid homeostasis or mutations in genes encoding for membrane proteins can cause misregulation of cell signaling events and promote oncogenic signaling activities. For example, breast cancer cell lines with high levels of epidermal growth factor receptor (EGFR) have been found to be resistant for the tyrosine kinase inhibitors (TKIs) targeting EGFR because of EGFR accumulation in the lipid rafts at the membranes of these cells and pharmacological depletion of cholesterol from the rafts decreased this resistance (Irwin et al., 2011). The constitutively active mutant form of the non-receptor tyrosine kinase Src has likewise been reported to accumulate in the lipid rafts of small cell lung cancer (SCLC) cells and stimulate oncogenic phosphoinositide 3-kinase (PI3K) signaling by facilitating the interaction of particular PI3K isoforms with Src kinases (Arcaro et al., 2007). Epithelial-to-mesenchymal transition (EMT) has also been associated with modulation of lipid raft properties strongly suggesting that alterations of membrane biophysical phenotypes are required to maintain metastatic potential of cancer cells (Tisza et al., 2016). In this section we will review how misregulation of Wnt signaling mainly in four common cancers; i.e., lung, colorectal, liver, and breast, is linked to the defective signaling at the plasma membrane and discuss the potential therapeutic approaches based on targeting the Wnt pathway at the membrane in these cancers.
Being the most common cause of cancer-related death in the world, lung cancers are histologically classified as non-small-cell lung cancer (NSCLC), which comprises about 85% of lung cancers, and small-cell lung cancer (SCLC). The main subtypes of NSCLCs are adenocarcinoma (ADC), squamous cell carcinoma (SCC), and large cell carcinoma (LCC). Wnt signaling is frequently abnormally activated in lung cancers. Overexpression of Wnts1-3, Wnt5a, Wnt11, and Fz8 is common in NSCLC (Nakashima et al., 2012; Stewart, 2014; Huang et al., 2015; Rapp et al., 2016). Resected NSCLC samples with high levels of Wnt3 are characterized with a significantly higher Ki67 proliferation index and a significantly lower apoptotic index, resulting in a considerably lower survival rate in patients with high-Wnt tumors than in those with low-Wnt tumors (Nakashima et al., 2012). Wnt3a treatment has been found to decrease the expression of E-cadherin and increase that of N-cadherin and Vimentin, thereby promoting EMT and metastasis in NSCLC cells (Li et al., 2015). Intriguingly, overexpression of the cell surface heparan sulfate proteoglycan Glypican-5, which competitively binds to Wnt3a, inactivates Wnt/β-catenin pathway and consequently suppresses EMT and metastasis in lung ADC (Wang et al., 2016). Wnt7a and its receptor Fz9 are significantly downregulated in NSCLC compared to normal uninvolved lung tissue and, upon interaction; they likewise trigger a tumor suppressor pathway by inhibiting transformed cell growth and promoting epithelial differentiation through activation of JNK pathway but not the Wnt/ß-catenin pathway (Winn et al., 2005). On the other hand, upregulation of Ror 1, a member of the Ror family of RTKs, has been shown to promote lung carcinogenesis through activation of Wnt/PCP and Wnt/RTK signaling cascades (Katoh and Katoh, 2017).
In a recent analysis of correlation between expression of Wnt ligands and 23 immunosuppressive genes across all cancer types in the TCGA dataset, high levels of Wnt1 has been found to significantly and negatively correlate with CD8 + T cells, showing that it induces immune resistance in lung adenocarcinoma cells and thus immunologically cold tumors (Kerdidani et al., 2019). A meta-analysis based on the data from 1805 NSCLC patients has reported a similar overexpression of Wnt1 and Wnt5a with an inverse correlation to the overall survival of these patients (Jin et al., 2016) (Figure 3).
Figure 3. RNAseq gene expression levels of Wnt ligands in different cancers. (A) Lung cancer (B) Colon cancer (C) Liver cancer (D) Breast cancer. The data were downloaded from https://xenabrowser.net/on August 10, 2020. For each cancer type, data were filtered from the browser extension data set on the TCGA TARGET GTEx dataset, which includes approximately 13 thousand genes. Studies were obtained from GTEx project and TCGA. Gene expressions were analyzed using RSEM fpkm method. Significance was calculated using Welch’s t-test and graphs were generated using GraphPad Prism 7.
Another comparative study on NSCLC subtypes has reported that non-canonical Wnt5a was significantly upregulated in the SCC, while ADC was marked by a prominent expression of canonical Wnt7b (Vesel et al., 2017). In SCC, Wnt5a downregulates the ATP-binding cassette (ABC) transporter family members Abcb1 and Abcg2, which are involved in chemotherapy resistance and appear to be upregulated by canonical Wnt signaling when the cells are treated with the chemotherapeutic agent cisplatin (Vesel et al., 2017).
Aberrant pathway activation occurs as a result of mutations or polymorphisms in pathway genes, repression of pathway inhibitors or synergistic effect with other mutations such as Kras (Testa et al., 2018a). For example, Lrp6 rs10845498 polymorphism has been associated with a reduced risk of lung SCC while LRP6 rs6488507 polymorphism synergistically increased the risk of NSCLC in tobacco smokers (Deng et al., 2014). Loss of function mutations in the Wnt-feedback induced cell surface E3 ligases Rnf43 and Znrf3, which bind to Fz and target it for degradation and fusions of the Wnt agonists Rspo2 and Rspo3 have been reported in lung cancer (Katoh and Katoh, 2017). Tobacco smoking, as the main factor responsible for lung cancer, appears to activate Wnt signaling through polycomb-induced repression of the secreted Wnt antagonist Dkk1, resulting in a tumorigenic effect (Hussain et al., 2009). Moreover, concurrent activation of Wnt/β-catenin signaling and expression of the constitutively active Kras mutant KrasG12D in the bronchiolar epithelium of the adult mouse lung, significantly increased the tumor number and size (Pacheco-Pinedo et al., 2011).
Misregulation of Wnt signaling in lung cancers have also been associated in many reports with abnormal expression of miRNAs that regulate the membrane components of Wnt pathways. For example, smoking-induced repression of miR-487b -a tumor suppressor miRNA that normally inhibits Wnt5a, Myc, and Kras and upregulates the Wnt antagonists Dkk1, Sfrp1, Sfrp4, and Wif1 to regulate lung stem cells- results in increased proliferation, invasion and metastatic potential of lung cancer cells (Xi et al., 2013). Expression of miR-148a is likewise significantly downregulated in primary cancer tissues of NSCLC patients compared to their adjacent normal lung tissues, and negatively correlates with the expression of Wnt1, a direct target of miRNA-148a (Chen et al., 2017). In contrast, elevated levels of miR-650 in NSCLC has been associated with promotion of cell proliferation and invasion through activation of Wnt1-mediated β-catenin signaling (Tang et al., 2019). Exosomal transfer of miR-1260b has been associated with increased tumor cell invasiveness in lung ADC proliferation through activation of Wnt/β-catenin signaling in neighboring cells (Xia et al., 2020). Interestingly, miR-1260b can induce biosynthesis of ceramides, depletion of which leads to accumulation of Wnt and inhibits Wnt signaling (Pepperl et al., 2013).
Several therapeutic approaches have been unraveled to suppress lung cancer progression via interfering with Wnt-receptor complex components at RNA or protein levels. For example, aspirin-induced miR-98 expression and long non-coding RNA (lncRNA) MIR503HG have been found to suppress proliferation of lung ADC and NSCLC cells via targeting Wnt1 and thus serve as tumor suppressors (Gan et al., 2019; Lin et al., 2019). Similarly, miR-5587-3p has been reported to suppress Wnt5b, a prognostic biomarker that is highly expressed in lung ADC and positively correlates with metastasis and cancer progression (Zhang et al., 2020). lncRNA AK126698 suppresses Wnt pathway by targeting Fz8 in NSCLC cells, and prevents their proliferation and migration (Fu et al., 2016). miR-135b has been identified to directly target Fz1 at its 3’UTR in NSCLC cells and enhance the chemosensitivity of cisplatin-resistant lung cancer cell lines (Su et al., 2016). On the other hand, antimalarial compounds artemisinin, dihydroartemisinin and artesunate can specifically suppress Wnt pathway by decreasing the protein level of Wnt5a/b (Tong et al., 2016). Qiyusanlong (QYSL) decoction, a formula composed of ten different traditional Chinese medicine, likewise reduces the protein levels of Wnt1, Wnt 2, and Wnt 5a, alone or with Cisplatin (Tong et al., 2018).
Colorectal cancer (CRC) ranks second among cancer-related deaths worldwide and majority of CRCs arise sporadically in patients with no family history of disease (Brenner et al., 2014). Hyperactivation of the Wnt pathway due to mutational inactivation of the APC tumor suppressor is thought to be the initiating event and key oncogenic driver in most sporadic and familial CRCs (Schatoff et al., 2017). Furthermore, mutations in both the components and the modulators of Wnt-receptor complex are frequently associated with CRC (Kuipers et al., 2015; Katoh and Katoh, 2017; Testa et al., 2018b). The Cancer Genome Atlas (TCGA) consortium report reveals that Wnt signaling is altered in up to 93% of all sporadic CRCs with at least one and up to sixteen alteration(s) in Wnt pathway components including APC, CTNNB1, TCF7L2, DKK family members, AXIN2 and the pathway negative regulator FAM123B/WTX (Cancer Genome Atlas Network, 2012).
Wnt2 and its receptor Fz7 have been found to be expressed at high levels in CRC as compared to normal colonic mucosa (Holcombe et al., 2002; Kalhor et al., 2018). Wnt3, Wnt6 and Wnt11 are likewise upregulated in CRC in correlation with poor survival rate, and when downregulated proliferation and migration are suppressed and apoptosis is induced (Zheng and Yu, 2018; Gorrono-Etxebarria et al., 2019; Nie et al., 2019; Peng et al., 2019) (Figure 3). Polymorphic adenoma-like protein 2, a zinc finger transcription factor, is also overexpressed in CRC and can promote Wnt6 expression by binding to its promoter region (Li et al., 2019b). The tubulin acetyltransferase αTAT1 promotes CRC progression via regulating subcellular localization of β-catenin and inducing expression of Wnt1 (Oh et al., 2017). Interestingly, Wnt1 is downregulated in response to Salmonella infection in CRC and this inhibits cancer cell invasion and migration (Wang et al., 2018). High levels of Wnt5a and its receptor Ror2 have been associated with drug resistance in CRC by concomitant induction of non-canonical Wnt signaling and suppression of canonical Wnt signaling (Bordonaro et al., 2011). Apart from these Wnt ligands, various Fz receptors including Fz4, Fz7, and Fz10, are also upregulated in CRC and their elevated levels have been associated with increased stemness, metastasis and recurrence (Cancer Genome Atlas Network, 2012; Ye et al., 2019; Chi et al., 2020). Wnt co-receptor Lrp6 is likewise significantly upregulated in many tumoral tissues of CRC in correlation with high malignancy and poor prognosis (Rismani et al., 2017). Various Lrp6 polymorphisms such as T867A, N789S, W239L have also been associated with susceptibility to early-onset CRC (de Voer et al., 2016).
Several membrane proteins have been reported to regulate Wnt signaling activity in CRC. For example the low-density lipoprotein receptor-related protein 1B (Lrp1b), which is downregulated in CRC, can suppress the growth and migration of cancer cells via inhibiting the interaction between Dvl2 and the Axin and hence the Wnt/β-catenin signaling (Wang et al., 2017). Expression of the cystic fibrosis transmembrane conductance regulator (Cftr) gene is likewise reduced in CRC, and this reduction enhances Wnt/β-catenin signaling via promoting interaction of Dvl-2 with the plasma membrane (Strubberg et al., 2018). On the contrary, the elevated expression of the type I transmembrane protein CUB-domain containing protein 1 (CDCP1) in CRC has been associated with high metastasis via promoting nuclear localization of β-catenin and Wnt signaling activity (He et al., 2020).
Wnt signaling is essential for normal intestinal function due to its roles in maintenance, proliferation and differentiation of intestinal stem cells. In particular, Lgr5 + intestinal stem cells exhibit high levels of canonical Wnt pathway activity reinforced by Rspo1-4 that drives a physical interaction between Lgr4/5 and Rnf43/Znrf3 (de Lau et al., 2014). Rspo fusions with protein tyrosine phosphatase receptor type K (PTPRK-RSPO3) and eukaryotic translation initiation factor subunit E (EIF3E-RSPO2) are frequently observed in colorectal traditional serrated adenomas and characterized by Rspo overexpression and activation of Wnt signaling (Sekine et al., 2017; Hashimoto et al., 2019). In contrast, Rspo2 appears to inhibit CRC metastasis by competing with the tumor-promoting Wnt5a for binding to Fz7 and thus antagonizing Wnt5a-driven non-canonical Wnt signaling (Dong et al., 2017). Although the majority of the studies conducted in primary clinical tissue have suggested a higher expression of the Rspo receptor Lgr5 in CRC cells relative to the adjacent normal tissue, a tumor-promoting role and enhanced chemoresistance, several studies have reported a potential tumor suppressive function of Lgr5 in CRC progression (Hsu et al., 2013; Morgan et al., 2018). N-terminal mutations of Rnf43, one of the most commonly mutated genes in CRC, have also been linked with enhanced Wnt/β-catenin signaling activity in colon cancer while C-terminal truncation mutants act similarly to the wild-type Rnf43 (Giannakis et al., 2014; Li et al., 2020).
Due to their potential function as tumor suppressors mentioned above, Lrp1b and Ctfr might offer promising strategies for the treatment of colon cancer. LiCl treatment also inhibits CRC cell proliferation via concomitant induction of the non-canonical ligand Wnt9 and suppression of β-catenin expression (Ali et al., 2016). Combination of inositol hexaphosphate and inositol can also reduce Wnt/β-catenin signaling via downregulating Wnt10b and β-catenin and suppress liver metastasis of CRC (Liu et al., 2020). Different microRNAs have been shown to affect CRC tumorigenesis as well. While miR-140-5p and miR-185 target Wnt1 and act as tumor suppressors, miR-410 targets Dkk1 and thus functions as an oncogene in CRC (Zhang et al., 2018; Wang et al., 2019b; Yeon et al., 2019). These findings reveal that miRNAs can be used as prognostic markers and to produce potential therapeutic agents for CRC patients.
Liver cancer is the fourth common cancer-related death in the world. Hepatocellular carcinoma (HCC), also referred to as hepatoma, is the most common type of liver cancer, constituting approximately 90% of all liver cancers. The molecular events that take place during multi-step initiation and progression of HCC are only partially understood. HCCs are broadly classified into the “proliferation class” and the “non-proliferation class” (Caruso et al., 2019). The proliferation class is further subdivided into the “Wnt-TGFβ subclass” with activated Wnt and TGFβ pathways and the “progenitor subclass” characterized by several features including overexpression of hepatic progenitor markers and mutations in AXIN1 (Rebouissou and Nault, 2020). On the other hand, the “non-proliferation class” of HCC includes tumors that are more heterogeneous, less aggressive, more differentiated with hepatocyte-like features, and contains at least two subclasses (Rebouissou and Nault, 2020). The most well described subclass is characterized by mutations in the β-catenin gene CTNNB1, leading to highly activated Wnt/β-catenin pathway, along with the TERT promoter and TP53 mutations (Yang et al., 2019a; Rebouissou and Nault, 2020). Thus, Wnt/β-catenin signaling is aberrantly activated in approximately 50% of HCC cases, in association with increased proliferation and inflammation, malignant tumor progression, poor prognosis, immune escape, and resistance to therapy (Yang et al., 2013; Khalaf et al., 2018; Jiang et al., 2019b; Ruiz de Galarreta et al., 2019).
Wnt-receptor complex components have been largely identified to take part in hepatocarcinogenesis. For example, several Fz receptors and the canonical Wnt ligands Wnt3 and, to a lesser extent, Wnt10b are strongly upregulated in a variety of HCC cells with different expression levels of hepatocyte lineage, epithelial and mesenchymal markers (Kim et al., 2008; Yuzugullu et al., 2009). Wnt2b, Wnt4, Wnt5a, Wnt5b, Wnt7b, Wnt8b, and Wnt9b are among the other ligands that have significantly increased expression in HCC cell lines (Yuzugullu et al., 2009) (Figure 3). Wnt1 is also highly expressed in HCC cell lines and has been associated with increased tumor recurrence after curative tumor resection in HBV- and HCV-related HCC patients (Lee et al., 2009; Wei et al., 2009). However, Wnt5a and Ror2 have been reported to be downregulated in HCC tissues with a poorer prognosis than HCC patients with elevated Wnt5a and Ror2 expression (Geng et al., 2012). In another study, Wnt5a overexpression was also found to decrease cell proliferation and tumor size in HCC, supporting that Wnt5a may serve as a tumor suppressor in HCC (Wang et al., 2019a). Another non-canonical ligand Wnt11a likewise decreases in HCC and its ectopic expression could suppress cell motility and migration via activation of RhoA/Rho kinase (Toyama et al., 2010). In addition to the Wnt ligands, high levels of Fz2, Fz7, Lrp5, and Lrp6 in HCC have also been found to be promote cell proliferation, migration, invasion, and EMT (Merle et al., 2004; Yuzugullu et al., 2009; Tung et al., 2012; Ou et al., 2019).
In search of novel potential therapeutic targets for HCC, the tumor-promoting function of Fz7 could be effectively reverted by small interfering RNAs that suppressed proliferation and metastasis of HCC cells and enhanced their apoptosis and sensitivity to chemotherapeutic drugs (Chen et al., 2016, 2018; Xue et al., 2018). Similarly, miR-542-3p, a common tumor-suppresser that is also downregulated in HCC tissues and cell lines, has been shown to inhibit HCC cell growth by targeting Fz7 and may thus represent a novel therapeutic target for HCC (Wu et al., 2017). An anti-Wnt1-antibody has been found to inhibit Wnt/β-catenin signaling and tumor growth in a xenograft mouse model (Wei et al., 2009). In contrast, anti-Wnt1 suppressed proliferation and apoptosis, but did not affect tumor size and growth in diethylnitrosamine-induced hepatocellular adenomas (Sklavos et al., 2018). Interestingly, garlic-derived compound S-allylmercaptocysteine reduced HCC tumorigenesis by directly targeting Lrp6 at the plasma membrane (Xiao et al., 2018).
Breast cancer is the leading cause of cancer death among females worldwide (Bray et al., 2018). Breast cancer is classified into three main subtypes based on the expression of estrogen receptor (ER) or progesterone receptor (PR) and amplification of the human epidermal growth factor 2 (ERBB2, commonly referred to as HER2): hormone receptor positive/ERBB2 negative (HR+/ERBB2-), ERBB2 positive (ERBB2+; HR+ or HR-) and triple-negative (lacking all three molecular markers) (Waks and Winer, 2019). Wnt signaling activity has been reported to increase in both cell lines and patient-derived metastatic cells of breast cancer and positively correlate with the ER expression (Lamb et al., 2013). Wnt/β-catenin pathway activation, characterized by reduction of membranous β-catenin with its concomitant nuclear accumulation, appears to correlate with poor clinical outcome in triple-negative breast cancer (TNBC) and basal-like breast cancer (BLBC), an aggressive subtype of breast cancer characterized by strong expression of basal markers such as cytokeratins (Khramtsov et al., 2010; Geyer et al., 2011; Xu et al., 2015).
The role of plasma membrane in breast cancer has been unraveled in a study that characterized the role of cell adhesion protein CD44, which appears to localize preferably to the lipid rafts due to palmitoylation. Raft affiliation of CD44 was observed to be higher in non-invasive breast cell lines, while decreasing in highly invasive cell lines or in case of mutagenesis of palmitoylation sites, suggesting that lipid raft association is a key regulatory mechanism in cancer cell migration (Babina et al., 2014). Interestingly γ-Tocotrienol, a natural isoform of vitamin E, appears to disrupt the lipid raft integrity, suppress Wnt/β-catenin signaling pathway and reduce cell motility in breast cancer (Ahmed et al., 2016). Thus, it will be very interesting to test how lipidation of Wnt and its receptors or raft association of Wnt-receptor complex influence migration and metastasis of these cells.
In a network correlation analysis of expression of >100 Wnt pathway components in healthy and cancerous breast tissues, the strong coherence in expression levels of the Wnt ligands and Fz receptors observed in the healthy breast tissue is dramatically lost in TNBC tissue and also varies widely in TNBC and non-TNBC (Koval and Katanaev, 2018) (Figure 3). Wnt4 and Wnt16 have been reported to be significantly upregulated in TNBC recurrence (Tsai et al., 2015). Wnt3a, 5a, 5b, 9a, and 11 are preferentially overexpressed due to gene amplifications in BLBC (Shi et al., 2014; Jiang et al., 2019a). Among them, Wnt5b has been identified as a key regulatory factor that governs the BLBC phenotype by activating both canonical and non-canonical Wnt signaling (Jiang et al., 2019a). Interestingly, Wnt5a expression was shown to decrease at both mRNA and protein levels in TNBC in association with poor prognosis and Wnt5a signaling was able to suppress tumor growth and metastasis (Borcherding et al., 2015; Zhong et al., 2016). Wnt3a and Wnt7a are likewise upregulated in metastatic breast cancer cell lines in association with poor prognosis and inhibition of Wnt/β-catenin signaling via Wnt1 knockdown could efficiently suppress cell proliferation and tumor growth (Jang et al., 2015; Avgustinova et al., 2016). The receptors Fz2, Fz3, Fz6, Fz7, and Fz10 as well as the co-receptor Lrp6 have also been reported to significantly increase in breast cancer and contribute to mesenchymal-like stemness, invasion, metastasis, and drug resistance (Liu et al., 2010; Yang et al., 2011; Gong et al., 2014; Simmons et al., 2014; Bell et al., 2017; Corda et al., 2017; Yin et al., 2020). Loss-of-function mutations of the tumor-suppressor-like molecules Rnf43 and Znrf3 and elevated expression levels of the Wnt agonists Rspo2 and Rspo4 can also be counted among the prognostic biomarkers of breast cancer (Ciriello et al., 2015; Coussy et al., 2017; Katoh and Katoh, 2017).
A number of studies have assessed the therapeutic potential of targeting Wnt signaling at the plasma membrane in TNBC. Being an important biomarker of TNBC, Fz7 is one of the well-investigated therapeutic options for breast cancer. A recombinant soluble peptide fragment (rhFzd7) has been shown to antagonize Fzd7 by competitively binding with Wnt3a and exhibit anti-tumor and anti-angiogenesis activities in TNBC (Xie et al., 2018). 2-cyano-3, 12-dioxooleana-1, 9 (11)-dien-28-oic acid-methyl ester (CDDO-Me) likewise targets Fz7 and Lrp6 and significantly inhibited tumor growth in breast cancer (Zhou et al., 2020). miR-142-3p, which is significantly down-regulated in breast cancer tissues, can also suppress Fz7 and thus serve as a tumor suppressor in breast cancer (Jia et al., 2018). A recent in silico study have identified several candidate molecules similar to palmitoleic acid that could potentially bind to the Fz7 transmembrane protein and inactivate the Wnt signaling pathway in TNBC cells (Alves Pinto and Freitas Da Silveira, 2020). In addition to Fz7, targeting Wnt ligands, Dkk1 or Lrp6 at the membrane may offer promising treatment options against breast cancer. For example, the antihelminthic niclosamide could sensitize TNBC cells to ionizing radiation (IR) by suppressing Wnt3a/β-catenin mediated radioresistance (Yin et al., 2016). A monoclonal anti-Wnt-1 antibody or Wnt-1 siRNA inhibit could induce apoptosis in a variety of human cancer cell lines including breast cancer (He et al., 2004). The polycomb protein chromobox homolog 7a (CBX7) likewise appears to inhibit breast tumorigenicity by enhancing the expression of the Wnt antagonist Dkk1 (Kim et al., 2015). The polyether ionophore antibiotic salinomycin, the milk thistle flavonolignan silibinin, the natural phenol echinacoside and the natural plant polyphenol rottlerin have all been shown to inhibit Wnt/β-catenin signaling by suppressing the Wnt co-receptor Lrp6 expression and exert anti-tumor effects in TNBC (Lu et al., 2012, 2014; Lu and Li, 2014; Tang et al., 2020).
It is noteworthy to mention that aberrant activation of Wnt signaling pathways is obviously not limited to the four cancer types mentioned below. Various Wnt ligands, the agonist Norrin, Fz receptors and the co-receptors Lrp6 and Ror1/2 have been reported to be abnormally expressed and associated with metastatic behavior, cancer progression and chemoresistance in ovarian cancer, glioblastoma multiforme, chronic lymphocytic leukemia, melanoma, multiple myeloma, post-transplant smooth muscle tumor, prostate cancer, pancreatic cancer gastric cancer, oral squamous cell carcinoma, Ewing sarcoma, osteosarcoma, and malignant peripheral nerve sheath tumors (Derksen et al., 2004; Larue and Delmas, 2006; Dissanayake et al., 2007; Yan et al., 2016; Yu et al., 2016; Li et al., 2017, 2019a; Liu et al., 2017; Pridgeon et al., 2017; Sandsmark et al., 2017; Jiang et al., 2018; Sinnberg et al., 2018; Teiken et al., 2018; Yang et al., 2019b; Chehover et al., 2020; El-Sehemy et al., 2020; Frenquelli et al., 2020; Kotrbova et al., 2020). Thus, a thorough understanding of misregulation of Wnt signaling pathways at the plasma membrane will pave the way for new therapeutic approaches for cancer.
Biological inhibitors and small molecules related with the Wnt pathways are widely exploited in therapeutic approaches to human diseases, including cancer, that have increased Wnt signaling activity. With its pivotal role in initiation and tight control of Wnt signaling activity, components and modulators of the Wnt-receptor complex constitute promising drug targets (Katoh and Katoh, 2017; Taciak et al., 2018; Goldsberry et al., 2019) (Figure 4). For example, Ipafricept (OMP-54F28), a recombinant fusion protein comprised of the Fz8 cysteine-rich domain and human IgG1 Fc fragment, acts as decoy receptor for Wnt ligands and exhibits antitumor activity (Le et al., 2015; Jimeno et al., 2017). OTSA 101-DTPA90Y, Vantictumab (OMP-18R5) and IgG-2919 are monoclonal antibodies targeting different Fz receptors and decrease tumor growth in different cancers (Gurney et al., 2012; Nielsen et al., 2015; Steinhart et al., 2017). The monoclonal antibodies DKN-01 and BHQ880 target DKK1 and likewise exert anti-tumorigenic activity in relapsed or refractory cancers including NSCLC, multiple myeloma and gastrointestinal cancers (Fulciniti et al., 2009; Edenfield et al., 2014; Iyer et al., 2014; Bendell et al., 2016). Rosmantuzumab (OMP-131R10) targets Rspo3 and evokes favorable responses against solid tumors and CRC (Diamond et al., 2016). The small molecule inhibitor KAN0439834, antibodies Cirmtuzumab (UC-961), ROR1-CD3-DART, APVO425, and ROR1R-CAR-T cells target Ror-1 with promising effects on different types of cancers (Berger et al., 2015; Yu et al., 2016; Katoh and Katoh, 2017). LGK974, ETC-159 (ETC1922159), RXC004, CGX1321, GNF-6231, XNM7201, IWP-2, WNT974, and WNT-C59 are all Porcupine inhibitors that are in the preclinical or phase I/II stage (Katoh and Katoh, 2017; Taciak et al., 2018; Goldsberry et al., 2019). When combined with pan-PI3K inhibitor GDC-0941, porcupine inhibitor ETC-159 has been shown to potently suppress in vivo tumor growth in pancreatic cancer (Zhong et al., 2019). Finally, Foxy-5, a small peptide that mimics Wnt5a, is considered to disrupt the migration and invasion of epithelial cancer cells and exhibit anti-metastatic impact in metastatic breast, colorectal and prostate cancers (Canesin et al., 2017).
Figure 4. Therapeutic molecules that target Wnt signaling pathway at the level of the plasma membrane. Different types of molecules are given with their target pathway component in boxes. Created with BioRender.com.
Clinical trials with several of these drugs have reported various adverse effects. For example, while being well tolerated by the patients with solid tumors, Ipafricept caused at least one of the treatment-emergent adverse events including dysgeusia, decreased appetite, fatigue, muscle spasms, and nausea, each of which were observed in at least 20% of patients (Jimeno et al., 2017). Different dose combinations of Vantictumab have been tested for 23 patients with advanced solid tumors, and likewise caused fatigue, nausea, vomiting, abdominal pain, constipation, and diarrhea as most common related adverse effects (Smith et al., 2013). First-in-human study of OTSA-101 on metastatic synovial sarcomna patients have reported lymphopenia, anemia, leucopenia, asthenia, hemoptysis, thrombocytopenia, neutropenia, and anorexia in some or all patients depending on the applied doses (Giraudet et al., 2018). The anti-DKK1 antibody DKN-01 also appears to cause adverse effects including cough, peripheral neuropathy, alopecia, leukopenia, neutropenia and fatigue in patients of refractory esophageal cancer or gastro-esophageal junction tumors (Bendell et al., 2016). Thus, further detailed investigations are absolutely necessary to assess the potential of Wnt inhibitors in therapeutic interventions.
The plasma membrane composition and organization play an important role in regulation of Wnt signaling by controlling ligand-receptor interaction and signal initiation. Since plasma membrane is highly dysregulated in cancer, it is essential to consider the unique organization of the Wnt-receptor complex for specific and effective targeting of the cancer cell. Understanding complex molecular interactions underlying Wnt-mediated cellular events at the plasma membrane has the potential to reveal attractive drug targets in cancers, and potentially other diseases, where Wnt signaling is extensively involved.
GO, YA, and MK wrote the manuscript and prepared the figures. EE contributed to the discussion. All authors contributed to the article and approved the submitted version.
The authors declare that the research was conducted in the absence of any commercial or financial relationships that could be construed as a potential conflict of interest.
Agajanian, M. J., Walker, M. P., Axtman, A. D., Ruela-de-Sousa, R. R., Serafin, D. S., Rabinowitz, A. D., et al. (2019). WNT Activates the AAK1 Kinase to Promote Clathrin-Mediated Endocytosis of LRP6 and Establish a Negative Feedback Loop. Cell Rep. 26, 79–93e78. doi: 10.1016/j.celrep.2018.12.023
Agarwal, S. R., Gratwohl, J., Cozad, M., Yang, P. C., Clancy, C. E., and Harvey, R. D. (2018). Compartmentalized cAMP Signaling Associated With Lipid Raft and Non-raft Membrane Domains in Adult Ventricular Myocytes. Front. Pharmacol. 9:332. doi: 10.3389/fphar.2018.00332
Ahmed, R. A., Alawin, O. A., and Sylvester, P. W. (2016). gamma-Tocotrienol reversal of epithelial-to-mesenchymal transition in human breast cancer cells is associated with inhibition of canonical Wnt signalling. Cell Prolif. 49, 460–470. doi: 10.1111/cpr.12270
Ali, I., Medegan, B., and Braun, D. P. (2016). Wnt9A Induction Linked to Suppression of Human Colorectal Cancer Cell Proliferation. Int. J. Mol. Sci. 17:495. doi: 10.3390/ijms17040495
Alves Pinto, I., and Freitas Da Silveira, N. J. (2020). In Silico Identification of Potential Inhibitors of the Wnt Signaling Pathway in Human Breast Cancer. J. Comput. Biol. 27, 999–1010. doi: 10.1089/cmb.2019.0311
Aman, A. J., Fulbright, A. N., and Parichy, D. M. (2018). Wnt/beta-catenin regulates an ancient signaling network during zebrafish scale development. Elife 7:37001. doi: 10.7554/eLife.37001
Angers, S., and Moon, R. T. (2009). Proximal events in Wnt signal transduction. Nat. Rev. Mol. Cell Biol. 10, 468–477.
Arcaro, A., Aubert, M., Espinosa, del Hierro, M. E., Khanzada, U. K., Angelidou, S., et al. (2007). Critical role for lipid raft-associated Src kinases in activation of PI3K-Akt signalling. Cell Signal 19, 1081–1092. doi: 10.1016/j.cellsig.2006.12.003
Avgustinova, A., Iravani, M., Robertson, D., Fearns, A., Gao, Q., Klingbeil, P., et al. (2016). Tumour cell-derived Wnt7a recruits and activates fibroblasts to promote tumour aggressiveness. Nat. Commun. 7:10305. doi: 10.1038/ncomms10305
Azbazdar, Y., Ozalp, O., Sezgin, E., Veerapathiran, S., Duncan, A. L., Sansom, M. S. P., et al. (2019). More Favorable Palmitic Acid Over Palmitoleic Acid Modification of Wnt3 Ensures Its Localization and Activity in Plasma Membrane Domains. Front. Cell Dev. Biol. 7:281. doi: 10.3389/fcell.2019.00281
Babina, I. S., McSherry, E. A., Donatello, S., Hill, A. D., and Hopkins, A. M. (2014). A novel mechanism of regulating breast cancer cell migration via palmitoylation-dependent alterations in the lipid raft affiliation of CD44. Breast Cancer Res. 16:R19. doi: 10.1186/bcr3614
Bandmann, V., Mirsanaye, A. S., Schafer, J., Thiel, G., Holstein, T., and Mikosch-Wersching, M. (2019). Membrane capacitance recordings resolve dynamics and complexity of receptor-mediated endocytosis in Wnt signalling. Sci. Rep. 9:12999. doi: 10.1038/s41598-019-49082-4
Barker, N., Huch, M., Kujala, P., van de Wetering, M., Snippert, H. J., van Es, J. H., et al. (2010). Lgr5+ve Stem Cells Drive Self-Renewal in the Stomach and Build Long-Lived Gastric Units In Vitro. Cell Stem Cell 6, 25–36. doi: 10.1016/j.stem.2009.11.013
Barker, N., Rookmaaker, Maarten, B., Kujala, P., Ng, A., Leushacke, M., et al. (2012). Lgr5+ve Stem/Progenitor Cells Contribute to Nephron Formation during Kidney Development. Cell Rep. 2, 540–552. doi: 10.1016/j.celrep.2012.08.018
Barker, N., van Es, J. H., Kuipers, J., Kujala, P., van den Born, M., Cozijnsen, M., et al. (2007). Identification of stem cells in small intestine and colon by marker gene Lgr5. Nature 449, 1003–1007. doi: 10.1038/nature06196
Barnett, K. C., and Kagan, J. C. (2020). Lipids that directly regulate innate immune signal transduction. Innate Immun. 26, 4–14. doi: 10.1177/1753425919852695
Bell, R., Barraclough, R., and Vasieva, O. (2017). Gene Expression Meta-Analysis of Potential Metastatic Breast Cancer Markers. Curr. Mol. Med. 17, 200–210. doi: 10.2174/1566524017666170807144946
Bendell, J. C., Murphy, J. E., Mahalingam, D., Halmos, B., Sirard, C. A., Landau, S. B., et al. (2016). Phase I study of DKN-01, an anti-DKK1 antibody, in combination with paclitaxel (pac) in patients (pts) with DKK1+ relapsed or refractory esophageal cancer (EC) or gastro-esophageal junction tumors (GEJ). J. Clin. Oncol. 34, 111–111. doi: 10.1200/jco.2016.34.4_suppl.111
Berger, B. S., Acebron, S. P., Herbst, J., Koch, S., and Niehrs, C. (2017). Parkinson’s disease-associated receptor GPR37 is an ER chaperone for LRP6. EMBO Rep. 18, 712–725. doi: 10.15252/embr.201643585
Berger, C., Sommermeyer, D., Hudecek, M., Berger, M., Balakrishnan, A., Paszkiewicz, P. J., et al. (2015). Safety of targeting ROR1 in primates with chimeric antigen receptor-modified T cells. Cancer Immunol. Res. 3, 206–216. doi: 10.1158/2326-6066.CIR-14-0163
Bienz, M. (2014). Signalosome assembly by domains undergoing dynamic head-to-tail polymerization. Trends Biochem. Sci. 39, 487–495. doi: 10.1016/j.tibs.2014.08.006
Bilic, J., Huang, Y. L., Davidson, G., Zimmermann, T., Cruciat, C. M., Bienz, M., et al. (2007). Wnt induces LRP6 signalosomes and promotes dishevelled-dependent LRP6 phosphorylation. Science 316, 1619–1622. doi: 10.1126/science.1137065
Borcherding, N., Kusner, D., Kolb, R., Xie, Q., Li, W., Yuan, F., et al. (2015). Paracrine WNT5A Signaling Inhibits Expansion of Tumor-Initiating Cells. Cancer Res. 75, 1972–1982. doi: 10.1158/0008-5472.CAN-14-2761
Bordonaro, M., Tewari, S., Cicco, C. E., Atamna, W., and Lazarova, D. L. (2011). A switch from canonical to noncanonical Wnt signaling mediates drug resistance in colon cancer cells. PLoS One 6:e27308. doi: 10.1371/journal.pone.0027308
Bowman, A. N., van Amerongen, R., Palmer, T. D., and Nusse, R. (2013). Lineage tracing with Axin2 reveals distinct developmental and adult populations of Wnt/β-catenin–responsive neural stem cells. Proc. Natl. Acad. Sci. 110, 7324–7329. doi: 10.1073/pnas.1305411110
Bray, F., Ferlay, J., Soerjomataram, I., Siegel, R. L., Torre, L. A., and Jemal, A. (2018). Global cancer statistics 2018: GLOBOCAN estimates of incidence and mortality worldwide for 36 cancers in 185 countries. CA Cancer J. Clinicians 68, 394–424. doi: 10.3322/caac.21492
Brenner, H., Kloor, M., and Pox, C. P. (2014). Colorectal cancer. Lancet 383, 1490–1502. doi: 10.1016/s0140-6736(13)61649-9
Brinkmann, E. M., Mattes, B., Kumar, R., Hagemann, A. I., Gradl, D., Scholpp, S., et al. (2016). Secreted Frizzled-related Protein 2 (sFRP2) Redirects Non-canonical Wnt Signaling from Fz7 to Ror2 during Vertebrate Gastrulation. J. Biol. Chem. 291, 13730–13742. doi: 10.1074/jbc.M116.733766
Brunt, L., and Scholpp, S. (2018). The function of endocytosis in Wnt signaling. Cell Mol. Life Sci. 75, 785–795. doi: 10.1007/s00018-017-2654-2
Cancer Genome Atlas Network (2012). Comprehensive molecular characterization of human colon and rectal cancer. Nature 487, 330–337. doi: 10.1038/nature11252
Canesin, G., Evans-Axelsson, S., Hellsten, R., Krzyzanowska, A., Prasad, C. P., Bjartell, A., et al. (2017). Treatment with the WNT5A-mimicking peptide Foxy-5 effectively reduces the metastatic spread of WNT5A-low prostate cancer cells in an orthotopic mouse model. PLoS One 12:e0184418. doi: 10.1371/journal.pone.0184418
Caruso, S., Calatayud, A. L., Pilet, J., La Bella, T., Rekik, S., Imbeaud, S., et al. (2019). Analysis of Liver Cancer Cell Lines Identifies Agents With Likely Efficacy Against Hepatocellular Carcinoma and Markers of Response. Gastroenterology 157, 760–776. doi: 10.1053/j.gastro.2019.05.001
Castro, V. L., Reyes-Nava, N. G., Sanchez, B. B., Gonzalez, C. G., and Quintana, A. M. (2020). Activation of WNT signaling restores the facial deficits in a zebrafish with defects in cholesterol metabolism. Biorxiv:2020.2002.2014.949958. Preprint. doi: 10.1101/2020.02.14.949958
Chehover, M., Reich, R., and Davidson, B. (2020). Expression of Wnt pathway molecules is associated with disease outcome in metastatic high-grade serous carcinoma. Virchows Arch. 477, 249–258. doi: 10.1007/s00428-019-02737-z
Chen, C., Xue, Y., Zhang, D., Xu, W., Xu, H., Yao, H., et al. (2016). Short hairpin RNA silencing of TGF-betaRII and FZD-7 synergistically suppresses proliferation and metastasis of hepatocellular carcinoma cells. Oncol. Lett. 11, 2039–2046. doi: 10.3892/ol.2016.4208
Chen, Y., Min, L., Ren, C., Xu, X., Yang, J., Sun, X., et al. (2017). miRNA-148a serves as a prognostic factor and suppresses migration and invasion through Wnt1 in non-small cell lung cancer. PLoS One 12:e0171751. doi: 10.1371/journal.pone.0171751
Chen, Z., Huang, C., Ma, T., Jiang, L., Tang, L., Shi, T., et al. (2018). Reversal effect of quercetin on multidrug resistance via FZD7/beta-catenin pathway in hepatocellular carcinoma cells. Phytomedicine 43, 37–45. doi: 10.1016/j.phymed.2018.03.040
Chi, J., Zhang, H., Hu, J., Song, Y., Li, J., Wang, L., et al. (2020). AGR3 promotes the stemness of colorectal cancer via modulating Wnt/beta-catenin signalling. Cell Signal 65:109419. doi: 10.1016/j.cellsig.2019.109419
Ching, W., Hang, H. C., and Nusse, R. (2008). Lipid-independent secretion of a Drosophila Wnt protein. J. Biol. Chem. 283, 17092–17098. doi: 10.1074/jbc.M802059200
Ciriello, G., Gatza, M. L., Beck, A. H., Wilkerson, M. D., Rhie, S. K., Pastore, A., et al. (2015). Comprehensive Molecular Portraits of Invasive Lobular Breast Cancer. Cell 163, 506–519. doi: 10.1016/j.cell.2015.09.033
Clevers, H., and Nusse, R. (2012). Wnt/beta-catenin signaling and disease. Cell 149, 1192–1205. doi: 10.1016/j.cell.2012.05.012
Clevers, H., Loh, K. M., and Nusse, R. (2014). An integral program for tissue renewal and regeneration: Wnt signaling and stem cell control. Science 346:1248012. doi: 10.1126/science.1248012
Corda, G., Sala, G., Lattanzio, R., Iezzi, M., Sallese, M., Fragassi, G., et al. (2017). Functional and prognostic significance of the genomic amplification of frizzled 6 (FZD6) in breast cancer. J. Pathol. 241, 350–361. doi: 10.1002/path.4841
Coussy, F., Lallemand, F., Vacher, S., Schnitzler, A., Chemlali, W., Caly, M., et al. (2017). Clinical value of R-spondins in triple-negative and metaplastic breast cancers. Br. J. Cancer 116, 1595–1603. doi: 10.1038/bjc.2017.131
Cruciat, C. M., and Niehrs, C. (2013). Secreted and transmembrane wnt inhibitors and activators. Cold Spring Harb. Perspect. Biol. 5:a015081. doi: 10.1101/cshperspect.a015081
de Lau, W., Peng, W. C., Gros, P., and Clevers, H. (2014). The R-spondin/Lgr5/Rnf43 module: regulator of Wnt signal strength. Genes Dev. 28, 305–316. doi: 10.1101/gad.235473.113
de Voer, R. M., Hahn, M. M., Weren, R. D., Mensenkamp, A. R., Gilissen, C., van Zelst-Stams, W. A., et al. (2016). Identification of Novel Candidate Genes for Early-Onset Colorectal Cancer Susceptibility. PLoS Genet. 12:e1005880. doi: 10.1371/journal.pgen.1005880
Deng, D., Zhang, Y., Bao, W., and Kong, X. (2014). Low-density lipoprotein receptor-related protein 6 (LRP6) rs10845498 polymorphism is associated with a decreased risk of non-small cell lung cancer. Int. J. Med. Sci. 11, 685–690. doi: 10.7150/ijms.8852
Derksen, P. W., Tjin, E., Meijer, H. P., Klok, M. D., MacGillavry, H. D., van Oers, M. H., et al. (2004). Illegitimate WNT signaling promotes proliferation of multiple myeloma cells. Proc. Natl. Acad. Sci. U S A. 101, 6122–6127. doi: 10.1073/pnas.0305855101
Diamond, J. R., Eckhardt, S. G., Bendell, J. C., Munster, P., Morris, V. K., Kopetz, S., et al. (2016). “Phase 1a/b study of OMP-131R10, an anti-RSPO3 antibody, in advanced solid tumors and previously treated metastatic colorectal cancer (CRC),” in Presented at: TAT 2016 Conference (Lausanne: Frontiers Media SA).
Dissanayake, S. K., Wade, M., Johnson, C. E., O’Connell, M. P., Leotlela, P. D., French, A. D., et al. (2007). The Wnt5A/protein kinase C pathway mediates motility in melanoma cells via the inhibition of metastasis suppressors and initiation of an epithelial to mesenchymal transition. J. Biol. Chem. 282, 17259–17271. doi: 10.1074/jbc.M700075200
Dong, X., Liao, W., Zhang, L., Tu, X., Hu, J., Chen, T., et al. (2017). RSPO2 suppresses colorectal cancer metastasis by counteracting the Wnt5a/Fzd7-driven noncanonical Wnt pathway. Cancer Lett. 402, 153–165. doi: 10.1016/j.canlet.2017.05.024
Doubravska, L., Krausova, M., Gradl, D., Vojtechova, M., Tumova, L., Lukas, J., et al. (2011). Fatty acid modification of Wnt1 and Wnt3a at serine is prerequisite for lipidation at cysteine and is essential for Wnt signalling. Cell Signal 23, 837–848. doi: 10.1016/j.cellsig.2011.01.007
Edenfield, W. J., Richards, D. A., Vukelja, S. J., Weiss, G. J., Sirard, C. A., Landau, S. B., et al. (2014). A phase 1 study evaluating the safety and efficacy of DKN-01, an investigational monoclonal antibody (Mab) in patients (pts) with advanced non-small cell lung cancer. J. Clin. Oncol. 32, 8068–8068. doi: 10.1200/jco.2014.32.15_suppl.8068
Eggeling, C., Ringemann, C., Medda, R., Schwarzmann, G., Sandhoff, K., Polyakova, S., et al. (2009). Direct observation of the nanoscale dynamics of membrane lipids in a living cell. Nature 457, 1159–1162. doi: 10.1038/nature07596
El-Sehemy, A., Selvadurai, H., Ortin-Martinez, A., Pokrajac, N., Mamatjan, Y., Tachibana, N., et al. (2020). Norrin mediates tumor-promoting and -suppressive effects in glioblastoma via Notch and Wnt. J. Clin. Invest. 130, 3069–3086. doi: 10.1172/JCI128994
Erazo-Oliveras, A., Fuentes, N. R., Wright, R. C., and Chapkin, R. S. (2018). Functional link between plasma membrane spatiotemporal dynamics, cancer biology, and dietary membrane-altering agents. Cancer Metastasis Rev. 37, 519–544. doi: 10.1007/s10555-018-9733-1
Eubelen, M., Bostaille, N., Cabochette, P., Gauquier, A., Tebabi, P., Dumitru, A. C., et al. (2018). A molecular mechanism for Wnt ligand-specific signaling. Science 361:aat1178. doi: 10.1126/science.aat1178
Fakhree, M. A. A., Blum, C., and Claessens, M. (2019). Shaping membranes with disordered proteins. Arch. Biochem. Biophys. 677:108163. doi: 10.1016/j.abb.2019.108163
Feng, Q., and Gao, N. (2015). Keeping Wnt signalosome in check by vesicular traffic. J. Cell Physiol. 230, 1170–1180. doi: 10.1002/jcp.24853
Flesken-Nikitin, A., Hwang, C.-I., Cheng, C.-Y., Michurina, T. V., Enikolopov, G., and Nikitin, A. Y. (2013). Ovarian surface epithelium at the junction area contains a cancer-prone stem cell niche. Nature 495, 241–245. doi: 10.1038/nature11979
Franch-Marro, X., Wendler, F., Griffith, J., Maurice, M. M., and Vincent, J. P. (2008). In vivo role of lipid adducts on Wingless. J. Cell Sci. 121(Pt 10), 1587–1592. doi: 10.1242/jcs.015958
Frenquelli, M., Caridi, N., Antonini, E., Storti, F., Vigano, V., Gaviraghi, M., et al. (2020). The WNT receptor ROR2 drives the interaction of multiple myeloma cells with the microenvironment through AKT activation. Leukemia 34, 257–270. doi: 10.1038/s41375-019-0486-9
Fu, X., Li, H., Liu, C., Hu, B., Li, T., and Wang, Y. (2016). Long noncoding RNA AK126698 inhibits proliferation and migration of non-small cell lung cancer cells by targeting Frizzled-8 and suppressing Wnt/beta-catenin signaling pathway. Onco Targets Ther. 9, 3815–3827. doi: 10.2147/OTT.S100633
Fulciniti, M., Tassone, P., Hideshima, T., Vallet, S., Nanjappa, P., Ettenberg, S. A., et al. (2009). Anti-DKK1 mAb (BHQ880) as a potential therapeutic agent for multiple myeloma. Blood 114, 371–379. doi: 10.1182/blood-2008-11-191577
Galli, L. M., and Burrus, L. W. (2011). Differential palmit(e)oylation of Wnt1 on C93 and S224 residues has overlapping and distinct consequences. PLoS One 6:e26636. doi: 10.1371/journal.pone.0026636
Gammons, M. V., Renko, M., Johnson, C. M., Rutherford, T. J., and Bienz, M. (2016). Wnt Signalosome Assembly by DEP Domain Swapping of Dishevelled. Mol. Cell 64, 92–104. doi: 10.1016/j.molcel.2016.08.026
Gan, H., Lin, L., Hu, N., Yang, Y., Gao, Y., Pei, Y., et al. (2019). Aspirin ameliorates lung cancer by targeting the miR-98/WNT1 axis. Thorac. Cancer 10, 744–750. doi: 10.1111/1759-7714.12992
Gao, X., and Hannoush, R. N. (2014). Single-cell imaging of Wnt palmitoylation by the acyltransferase porcupine. Nat. Chem. Biol. 10, 61–68. doi: 10.1038/nchembio.1392
Geng, M., Cao, Y. C., Chen, Y. J., Jiang, H., Bi, L. Q., and Liu, X. H. (2012). Loss of Wnt5a and Ror2 protein in hepatocellular carcinoma associated with poor prognosis. World J. Gastroenterol. 18, 1328–1338. doi: 10.3748/wjg.v18.i12.1328
Geyer, F. C., Lacroix-Triki, M., Savage, K., Arnedos, M., Lambros, M. B., MacKay, A., et al. (2011). beta-Catenin pathway activation in breast cancer is associated with triple-negative phenotype but not with CTNNB1 mutation. Mod. Pathol. 24, 209–231. doi: 10.1038/modpathol.2010.205
Giannakis, M., Hodis, E., Jasmine, Mu, X., Yamauchi, M., Rosenbluh, J., et al. (2014). RNF43 is frequently mutated in colorectal and endometrial cancers. Nat. Genet. 46, 1264–1266. doi: 10.1038/ng.3127
Giraudet, A. L., Cassier, P. A., Iwao-Fukukawa, C., Garin, G., Badel, J. N., Kryza, D., et al. (2018). A first-in-human study investigating biodistribution, safety and recommended dose of a new radiolabeled MAb targeting FZD10 in metastatic synovial sarcoma patients. BMC Cancer 18:646. doi: 10.1186/s12885-018-4544-x
Glaeser, K., Urban, M., Fenech, E., Voloshanenko, O., Kranz, D., Lari, F., et al. (2018). ERAD-dependent control of the Wnt secretory factor Evi. EMBO J. 37:201797311. doi: 10.15252/embj.201797311
Goldsberry, W. N., Londoño, A., Randall, T. D., Norian, L. A., and Arend, R. C. (2019). A Review of the Role of Wnt in Cancer Immunomodulation. Cancers 11:cancers11060771. doi: 10.3390/cancers11060771
Gong, C., Qu, S., Lv, X.-B., Liu, B., Tan, W., Nie, Y., et al. (2014). BRMS1L suppresses breast cancer metastasis by inducing epigenetic silence of FZD10. Nat. Commun. 5:5406. doi: 10.1038/ncomms6406
Gong, Q., Huntsman, C., and Ma, D. (2008). Clathrin-independent internalization and recycling. J. Cell Mol. Med. 12, 126–144. doi: 10.1111/j.1582-4934.2007.00148.x
Gorrono-Etxebarria, I., Aguirre, U., Sanchez, S., Gonzalez, N., Escobar, A., Zabalza, I., et al. (2019). Wnt-11 as a Potential Prognostic Biomarker and Therapeutic Target in Colorectal Cancer. Cancers 11:11070908. doi: 10.3390/cancers11070908
Gradilla, A. C., Sanchez-Hernandez, D., Brunt, L., and Scholpp, S. (2018). From top to bottom: Cell polarity in Hedgehog and Wnt trafficking. BMC Biol. 16:37. doi: 10.1186/s12915-018-0511-x
Gurney, A., Axelrod, F., Bond, C. J., Cain, J., Chartier, C., Donigan, L., et al. (2012). Wnt pathway inhibition via the targeting of Frizzled receptors results in decreased growth and tumorigenicity of human tumors. Proc. Natl. Acad. Sci. U S A. 109, 11717–11722. doi: 10.1073/pnas.1120068109
Haack, F., Lemcke, H., Ewald, R., Rharass, T., and Uhrmacher, A. M. (2015). Spatio-temporal model of endogenous ROS and raft-dependent WNT/beta-catenin signaling driving cell fate commitment in human neural progenitor cells. PLoS Comput. Biol. 11:e1004106. doi: 10.1371/journal.pcbi.1004106
Hagemann, A. I., Kurz, J., Kauffeld, S., Chen, Q., Reeves, P. M., Weber, S., et al. (2014). In vivo analysis of formation and endocytosis of the Wnt/beta-catenin signaling complex in zebrafish embryos. J. Cell Sci. 127(Pt 18), 3970–3982. doi: 10.1242/jcs.148767
Hall, E. T., Hoesing, E., Sinkovics, E., and Verheyen, E. M. (2019). Actomyosin contractility modulates Wnt signaling through adherens junction stability. Mol. Biol. Cell 30, 411–426. doi: 10.1091/mbc.E18-06-0345
Hashimoto, T., Ogawa, R., Yoshida, H., Taniguchi, H., Kojima, M., Saito, Y., et al. (2019). EIF3E-RSPO2 and PIEZO1-RSPO2 fusions in colorectal traditional serrated adenoma. Histopathology 75, 266–273. doi: 10.1111/his.13867
He, B., You, L., Uematsu, K., Xu, Z., Lee, A. Y., Matsangou, M., et al. (2004). A monoclonal antibody against Wnt-1 induces apoptosis in human cancer cells. Neoplasia 6, 7–14. doi: 10.1016/s1476-5586(04)80048-4
He, Y., Davies, C. M., Harrington, B. S., Hellmers, L., Sheng, Y., Broomfield, A., et al. (2020). CDCP1 enhances Wnt signaling in colorectal cancer promoting nuclear localization of beta-catenin and E-cadherin. Oncogene 39, 219–233. doi: 10.1038/s41388-019-0983-3
Hendriksen, J., Jansen, M., Brown, C. M., van der Velde, H., van Ham, M., Galjart, N., et al. (2008). Plasma membrane recruitment of dephosphorylated beta-catenin upon activation of the Wnt pathway. J. Cell Sci. 121, 1793–1802. doi: 10.1242/jcs.025536
Hofman, E. G., Bader, A. N., Gerritsen, H. C., van Bergen, and En Henegouwen, P. M. (2009). EGF induces rapid reorganization of plasma membrane microdomains. Commun. Integr. Biol. 2, 213–214. doi: 10.4161/cib.2.3.7877
Holcombe, R. F., Marsh, J. L., Waterman, M. L., Lin, F., Milovanovic, T., and Truong, T. (2002). Expression of Wnt ligands and Frizzled receptors in colonic mucosa and in colon carcinoma. Mol. Pathol. 55, 220–226. doi: 10.1136/mp.55.4.220
Hosseini, V., Dani, C., Geranmayeh, M. H., Mohammadzadeh, F., Nazari Soltan, Ahmad, S., et al. (2019). Wnt lipidation: Roles in trafficking, modulation, and function. J. Cell Physiol. 234, 8040–8054. doi: 10.1002/jcp.27570
Hsu, H. C., Liu, Y. S., Tseng, K. C., Hsu, C. L., Liang, Y., Yang, T. S., et al. (2013). Overexpression of Lgr5 correlates with resistance to 5-FU-based chemotherapy in colorectal cancer. Int. J. Colorectal Dis. 28, 1535–1546. doi: 10.1007/s00384-013-1721-x
Huang, C., Ma, R., Xu, Y., Li, N., Li, Z., Yue, J., et al. (2015). Wnt2 promotes non-small cell lung cancer progression by activating WNT/beta-catenin pathway. Am. J. Cancer Res. 5, 1032–1046.
Huang, Y., Huang, S., Di Scala, C., Wang, Q., Wandall, H. H., Fantini, J., et al. (2018). The glycosphingolipid MacCer promotes synaptic bouton formation in Drosophila by interacting with Wnt. Elife 7:38183. doi: 10.7554/eLife.38183
Huch, M., Dorrell, C., Boj, S. F., van Es, J. H., Li, V. S. W., van de Wetering, M., et al. (2013). In vitro expansion of single Lgr5+ liver stem cells induced by Wnt-driven regeneration. Nature 494, 247–250. doi: 10.1038/nature11826
Humphries, A. C., and Mlodzik, M. (2018). From instruction to output: Wnt/PCP signaling in development and cancer. Curr. Opin. Cell Biol. 51, 110–116. doi: 10.1016/j.ceb.2017.12.005
Hussain, M., Rao, M., Humphries, A. E., Hong, J. A., Liu, F., Yang, M., et al. (2009). Tobacco smoke induces polycomb-mediated repression of Dickkopf-1 in lung cancer cells. Cancer Res. 69, 3570–3578. doi: 10.1158/0008-5472.CAN-08-2807
Irwin, M. E., Mueller, K. L., Bohin, N., Ge, Y., and Boerner, J. L. (2011). Lipid raft localization of EGFR alters the response of cancer cells to the EGFR tyrosine kinase inhibitor gefitinib. J. Cell Physiol. 226, 2316–2328. doi: 10.1002/jcp.22570
Iyer, S. P., Beck, J. T., Stewart, A. K., Shah, J., Kelly, K. R., Isaacs, R., et al. (2014). A Phase IB multicentre dose-determination study of BHQ880 in combination with anti-myeloma therapy and zoledronic acid in patients with relapsed or refractory multiple myeloma and prior skeletal-related events. Br. J. Haematol. 167, 366–375. doi: 10.1111/bjh.13056
Jan, T. A., Chai, R., Sayyid, Z. N., van Amerongen, R., Xia, A., Wang, T., et al. (2013). Tympanic border cells are Wnt-responsive and can act as progenitors for postnatal mouse cochlear cells. Development 140, 1196–1206. doi: 10.1242/dev.087528
Janda, C. Y., Waghray, D., Levin, A. M., Thomas, C., and Garcia, K. C. (2012). Structural basis of Wnt recognition by Frizzled. Science 337, 59–64. doi: 10.1126/science.1222879
Jang, G. B., Kim, J. Y., Cho, S. D., Park, K. S., Jung, J. Y., Lee, H. Y., et al. (2015). Blockade of Wnt/beta-catenin signaling suppresses breast cancer metastasis by inhibiting CSC-like phenotype. Sci. Rep. 5:12465. doi: 10.1038/srep12465
Jia, X. P., Meng, L. L., Fang, J. C., Wang, H. W., Chen, J., Zhou, J., et al. (2018). Aberrant Expression of miR-142-3p and its Target Gene HMGA1 and FZD7 in Breast Cancer and its Clinical Significance. Clin. Lab. 64, 915–921. doi: 10.7754/Clin.Lab.2017.171114
Jiang, K., Li, S., Li, L., Wang, X., Gu, Y., and Jin, Z. (2018). WNT6 is an effective marker for osteosarcoma diagnosis and prognosis. Medicine 97:e13011. doi: 10.1097/MD.0000000000013011
Jiang, S., Zhang, M., Zhang, Y., Zhou, W., Zhu, T., Ruan, Q., et al. (2019a). WNT5B governs the phenotype of basal-like breast cancer by activating WNT signaling. Cell Commun. Signal 17:109. doi: 10.1186/s12964-019-0419-2
Jiang, X., and Cong, F. (2016). Novel Regulation of Wnt Signaling at the Proximal Membrane Level. Trends Biochem. Sci. 41, 773–783. doi: 10.1016/j.tibs.2016.06.003
Jiang, Y., Han, Q. J., and Zhang, J. (2019b). Hepatocellular carcinoma: Mechanisms of progression and immunotherapy. World J. Gastroenterol. 25, 3151–3167. doi: 10.3748/wjg.v25.i25.3151
Jimeno, A., Gordon, M., Chugh, R., Messersmith, W., Mendelson, D., Dupont, J., et al. (2017). A First-in-Human Phase I Study of the Anticancer Stem Cell Agent Ipafricept (OMP-54F28), a Decoy Receptor for Wnt Ligands, in Patients with Advanced Solid Tumors. Clin. Cancer Res. 23, 7490–7497. doi: 10.1158/1078-0432.ccr-17-2157
Jin, J., Zhan, P., Qian, H., Wang, X., Katoh, M., Phan, K., et al. (2016). Prognostic value of wingless-type proteins in non-small cell lung cancer patients: a meta-analysis. Transl. Lung Cancer Res. 5, 436–442. doi: 10.21037/tlcr.2016.08.08
Jung, Y.-S., and Park, J.-I. (2020). Wnt signaling in cancer: therapeutic targeting of Wnt signaling beyond β-catenin and the destruction complex. Exp. Mol. Med. 52, 183–191. doi: 10.1038/s12276-020-0380-6
Kalhor, H., Poorebrahim, M., Rahimi, H., Shabani, A. A., Karimipoor, M., Akbari Eidgahi, M. R., et al. (2018). Structural and dynamic characterization of human Wnt2-Fzd7 complex using computational approaches. J. Mol. Model 24:274. doi: 10.1007/s00894-018-3788-3
Katoh, M., and Katoh, M. (2017). Molecular genetics and targeted therapy of WNT-related human diseases (Review). Int. J. Mol. Med. 40, 587–606. doi: 10.3892/ijmm.2017.3071
Kerdidani, D., Chouvardas, P., Arjo, A. R., Giopanou, I., Ntaliarda, G., Guo, Y. A., et al. (2019). Wnt1 silences chemokine genes in dendritic cells and induces adaptive immune resistance in lung adenocarcinoma. Nat. Commun. 10:1405. doi: 10.1038/s41467-019-09370-z
Khalaf, A. M., Fuentes, D., Morshid, A. I., Burke, M. R., Kaseb, A. O., Hassan, M., et al. (2018). Role of Wnt/β-catenin signaling in hepatocellular carcinoma, pathogenesis, and clinical significance. J. Hepatocell. Carcinoma 5, 61–73. doi: 10.2147/JHC.S156701
Khramtsov, A. I., Khramtsova, G. F., Tretiakova, M., Huo, D., Olopade, O. I., and Goss, K. H. (2010). Wnt/beta-catenin pathway activation is enriched in basal-like breast cancers and predicts poor outcome. Am. J. Pathol. 176, 2911–2920. doi: 10.2353/ajpath.2010.091125
Kim, H. Y., Park, J. H., Won, H. Y., Lee, J. Y., and Kong, G. (2015). CBX7 inhibits breast tumorigenicity through DKK-1-mediated suppression of the Wnt/β-catenin pathway. FASEB J. 29, 300–313. doi: 10.1096/fj.14-253997
Kim, I., Pan, W., Jones, S. A., Zhang, Y., Zhuang, X., and Wu, D. (2013). Clathrin and AP2 are required for PtdIns(4,5)P2-mediated formation of LRP6 signalosomes. J. Cell Biol. 200, 419–428. doi: 10.1083/jcb.201206096
Kim, M., Lee, H. C., Tsedensodnom, O., Hartley, R., Lim, Y. S., Yu, E., et al. (2008). Functional interaction between Wnt3 and Frizzled-7 leads to activation of the Wnt/beta-catenin signaling pathway in hepatocellular carcinoma cells. J. Hepatol. 48, 780–791. doi: 10.1016/j.jhep.2007.12.020
Kimelman, D., and Xu, W. (2006). beta-catenin destruction complex: insights and questions from a structural perspective. Oncogene 25, 7482–7491. doi: 10.1038/sj.onc.1210055
Kohn, A. D., and Moon, R. T. (2005). Wnt and calcium signaling: beta-catenin-independent pathways. Cell Calcium 38, 439–446. doi: 10.1016/j.ceca.2005.06.022
Komekado, H., Yamamoto, H., Chiba, T., and Kikuchi, A. (2007). Glycosylation and palmitoylation of Wnt-3a are coupled to produce an active form of Wnt-3a. Genes Cells 12, 521–534. doi: 10.1111/j.1365-2443.2007.01068.x
Kotrbova, A., Ovesna, P., Gybel, T., Radaszkiewicz, T., Bednarikova, M., Hausnerova, J., et al. (2020). WNT signaling inducing activity in ascites predicts poor outcome in ovarian cancer. Theranostics 10, 537–552. doi: 10.7150/thno.37423
Koval, A., and Katanaev, V. L. (2018). Dramatic dysbalancing of the Wnt pathway in breast cancers. Sci. Rep. 8:7329. doi: 10.1038/s41598-018-25672-6
Krishnamurthy, N., and Kurzrock, R. (2018). Targeting the Wnt/beta-catenin pathway in cancer: Update on effectors and inhibitors. Cancer Treat. Rev. 62, 50–60. doi: 10.1016/j.ctrv.2017.11.002
Kuipers, E. J., Grady, W. M., Lieberman, D., Seufferlein, T., Sung, J. J., Boelens, P. G., et al. (2015). Colorectal cancer. Nat. Rev. Dis. Primers 1:15065. doi: 10.1038/nrdp.2015.65
Kurayoshi, M., Yamamoto, H., Izumi, S., and Kikuchi, A. (2007). Post-translational palmitoylation and glycosylation of Wnt-5a are necessary for its signalling. Biochem. J. 402, 515–523. doi: 10.1042/BJ20061476
Kusumi, A., Fujiwara, T. K., Tsunoyama, T. A., Kasai, R. S., Liu, A. A., Hirosawa, K. M., et al. (2020). Defining raft domains in the plasma membrane. Traffic 21, 106–137. doi: 10.1111/tra.12718
Lamb, R., Ablett, M. P., Spence, K., Landberg, G., Sims, A. H., and Clarke, R. B. (2013). Wnt pathway activity in breast cancer sub-types and stem-like cells. PLoS One 8:e67811. doi: 10.1371/journal.pone.0067811
Larue, L., and Delmas, V. (2006). The WNT/Beta-catenin pathway in melanoma. Front. Biosci. 11:733–742. doi: 10.2741/1831
Le, P. N., McDermott, J. D., and Jimeno, A. (2015). Targeting the Wnt pathway in human cancers: therapeutic targeting with a focus on OMP-54F28. Pharmacol. Therapeut. 146, 1–11. doi: 10.1016/j.pharmthera.2014.08.005
Lee, C. J., Rana, M. S., Bae, C., Li, Y., and Banerjee, A. (2019). In vitro reconstitution of Wnt acylation reveals structural determinants of substrate recognition by the acyltransferase human Porcupine. J. Biol. Chem. 294, 231–245. doi: 10.1074/jbc.RA118.005746
Lee, H. H., Uen, Y. H., Tian, Y. F., Sun, C. S., Sheu, M. J., Kuo, H. T., et al. (2009). Wnt-1 protein as a prognostic biomarker for hepatitis B-related and hepatitis C-related hepatocellular carcinoma after surgery. Cancer Epidemiol. Biomarkers Prev. 18, 1562–1569. doi: 10.1158/1055-9965.EPI-09-0039
Lee, H. J., Li, J., Vickman, R. E., Li, J., Liu, R., Durkes, A. C., et al. (2018). Cholesterol Esterification Inhibition Suppresses Prostate Cancer Metastasis by Impairing the Wnt/beta-catenin Pathway. Mol. Cancer Res. 16, 974–985. doi: 10.1158/1541-7786.MCR-17-0665
Lee, S. M., Tole, S., Grove, E., and McMahon, A. P. (2000). A local Wnt-3a signal is required for development of the mammalian hippocampus. Development 127, 457–467.
Levental, I., Lingwood, D., Grzybek, M., Coskun, U., and Simons, K. (2010). Palmitoylation regulates raft affinity for the majority of integral raft proteins. Proc. Natl. Acad. Sci. U S A. 107, 22050–22054. doi: 10.1073/pnas.1016184107
Li, C., Nguyen, V., Clark, K. N., Zahed, T., Sharkas, S., Filipp, F. V., et al. (2019a). Down-regulation of FZD3 receptor suppresses growth and metastasis of human melanoma independently of canonical WNT signaling. Proc. Natl. Acad. Sci. U S A. 116, 4548–4557. doi: 10.1073/pnas.1813802116
Li, C., Song, G., Zhang, S., Wang, E., and Cui, Z. (2015). Wnt3a increases the metastatic potential of non-small cell lung cancer cells in vitro in part via its upregulation of Notch3. Oncol. Rep. 33, 1207–1214. doi: 10.3892/or.2014.3700
Li, L., Xu, C., Liu, P., and Huang, J. (2017). Correlation study of DNA methylation of WNT6 gene with osteosarcoma in children. Oncol. Lett. 14, 271–275. doi: 10.3892/ol.2017.6135
Li, N., Li, D., Du, Y., Su, C., Yang, C., Lin, C., et al. (2019b). Overexpressed PLAGL2 transcriptionally activates Wnt6 and promotes cancer development in colorectal cancer. Oncol. Rep. 41, 875–884. doi: 10.3892/or.2018.6914
Li, S., Lavrijsen, M., Bakker, A., Magierowski, M., Magierowska, K., Liu, P., et al. (2020). Commonly observed RNF43 mutations retain functionality in attenuating Wnt/beta-catenin signaling and unlikely confer Wnt-dependency onto colorectal cancers. Oncogene 39, 3458–3472. doi: 10.1038/s41388-020-1232-5
Lin, H., Li, P., Zhang, N., Cao, L., Gao, Y. F., and Ping, F. (2019). Long non-coding RNA MIR503HG serves as a tumor suppressor in non-small cell lung cancer mediated by wnt1. Eur. Rev. Med. Pharmacol. Sci. 23, 10818–10826. doi: 10.26355/eurrev_201912_19785
Liu, B., Chen, W., Cao, G., Dong, Z., Xu, J., Luo, T., et al. (2017). MicroRNA-27b inhibits cell proliferation in oral squamous cell carcinoma by targeting FZD7 and Wnt signaling pathway. Arch. Oral. Biol. 83, 92–96. doi: 10.1016/j.archoralbio.2017.07.009
Liu, C. C., Kanekiyo, T., Roth, B., and Bu, G. (2014). Tyrosine-based signal mediates LRP6 receptor endocytosis and desensitization of Wnt/beta-catenin pathway signaling. J. Biol. Chem. 289, 27562–27570. doi: 10.1074/jbc.M113.533927
Liu, C. C., Prior, J., Piwnica-Worms, D., and Bu, G. (2010). LRP6 overexpression defines a class of breast cancer subtype and is a target for therapy. Proc. Natl. Acad. Sci. U S A. 107, 5136–5141. doi: 10.1073/pnas.0911220107
Liu, X., Liu, C., Chen, C., Sun, W., Ci, Y., Li, Q., et al. (2020). Combination of Inositol Hexaphosphate and Inositol Inhibits Liver Metastasis of Colorectal Cancer in Mice Through the Wnt/beta-Catenin Pathway. Onco Targets Ther. 13, 3223–3235. doi: 10.2147/OTT.S247646
Logan, C. Y., and Nusse, R. (2004). The Wnt signaling pathway in development and disease. Annu. Rev. Cell Dev. Biol. 20, 781–810. doi: 10.1146/annurev.cellbio.20.010403.113126
Lu, W., and Li, Y. (2014). Salinomycin suppresses LRP6 expression and inhibits both Wnt/beta-catenin and mTORC1 signaling in breast and prostate cancer cells. J. Cell Biochem. 115, 1799–1807. doi: 10.1002/jcb.24850
Lu, W., Lin, C., and Li, Y. (2014). Rottlerin induces Wnt co-receptor LRP6 degradation and suppresses both Wnt/β-catenin and mTORC1 signaling in prostate and breast cancer cells. Cell. Signal. 26, 1303–1309. doi: 10.1016/j.cellsig.2014.02.018
Lu, W., Lin, C., King, T. D., Chen, H., Reynolds, R. C., and Li, Y. (2012). Silibinin inhibits Wnt/β-catenin signaling by suppressing Wnt co-receptor LRP6 expression in human prostate and breast cancer cells. Cell. Signal. 24, 2291–2296. doi: 10.1016/j.cellsig.2012.07.009
Luz, M., Spannl-Muller, S., Ozhan, G., Kagermeier-Schenk, B., Rhinn, M., Weidinger, G., et al. (2014). Dynamic association with donor cell filopodia and lipid-modification are essential features of Wnt8a during patterning of the zebrafish neuroectoderm. PLoS One 9:e84922. doi: 10.1371/journal.pone.0084922
MacDonald, B. T., Tamai, K., and He, X. (2009). Wnt/beta-catenin signaling: components, mechanisms, and diseases. Dev. Cell 17, 9–26. doi: 10.1016/j.devcel.2009.06.016
Mason, J. O., Kitajewski, J., and Varmus, H. E. (1992). Mutational analysis of mouse Wnt-1 identifies two temperature-sensitive alleles and attributes of Wnt-1 protein essential for transformation of a mammary cell line. Mol. Biol. Cell 3, 521–533. doi: 10.1091/mbc.3.5.521
Merle, P., de la Monte, S., Kim, M., Herrmann, M., Tanaka, S., Von Dem Bussche, A., et al. (2004). Functional consequences of frizzled-7 receptor overexpression in human hepatocellular carcinoma. Gastroenterology 127, 1110–1122. doi: 10.1053/j.gastro.2004.07.009
Mii, Y., Yamamoto, T., Takada, R., Mizumoto, S., Matsuyama, M., Yamada, S., et al. (2017). Roles of two types of heparan sulfate clusters in Wnt distribution and signaling in Xenopus. Nat. Commun. 8:1973. doi: 10.1038/s41467-017-02076-0
Morgan, R. G., Mortensson, E., and Williams, A. C. (2018). Targeting LGR5 in Colorectal Cancer: therapeutic gold or too plastic? Br. J. Cancer 118, 1410–1418. doi: 10.1038/s41416-018-0118-6
Nakashima, N., Liu, D., Huang, C. L., Ueno, M., Zhang, X., and Yokomise, H. (2012). Wnt3 gene expression promotes tumor progression in non-small cell lung cancer. Lung Cancer 76, 228–234. doi: 10.1016/j.lungcan.2011.10.007
Nie, X., Xia, F., Liu, Y., Zhou, Y., Ye, W., Hean, P., et al. (2019). Downregulation of Wnt3 Suppresses Colorectal Cancer Development Through Inhibiting Cell Proliferation and Migration. Front. Pharmacol. 10:1110. doi: 10.3389/fphar.2019.01110
Nielsen, T. O., Poulin, N. M., and Ladanyi, M. (2015). Synovial sarcoma: recent discoveries as a roadmap to new avenues for therapy. Cancer Discov. 5, 124–134. doi: 10.1158/2159-8290.CD-14-1246
Nile, A. H., and Hannoush, R. N. (2016). Fatty acylation of Wnt proteins. Nat. Chem. Biol. 12, 60–69. doi: 10.1038/nchembio.2005
Nile, A. H., Mukund, S., Stanger, K., Wang, W., and Hannoush, R. N. (2017). Unsaturated fatty acyl recognition by Frizzled receptors mediates dimerization upon Wnt ligand binding. Proc. Natl. Acad. Sci. U S A. 114, 4147–4152. doi: 10.1073/pnas.1618293114
Nusse, R. (2005). Wnt signaling in disease and in development. Cell Res. 15, 28–32. doi: 10.1038/sj.cr.7290260
Nusse, R., and Clevers, H. (2017). Wnt/beta-Catenin Signaling, Disease, and Emerging Therapeutic Modalities. Cell 169, 985–999. doi: 10.1016/j.cell.2017.05.016
Nusse, R., Fuerer, C., Ching, W., Harnish, K., Logan, C., Zeng, A., et al. (2008). Wnt signaling and stem cell control. Cold Spring Harb. Symposia Quantitat. Biol. 73, 59–66. doi: 10.1101/sqb.2008.73.035
Oh, S., You, E., Ko, P., Jeong, J., Keum, S., and Rhee, S. (2017). Genetic disruption of tubulin acetyltransferase, alphaTAT1, inhibits proliferation and invasion of colon cancer cells through decreases in Wnt1/beta-catenin signaling. Biochem. Biophys. Res. Commun. 482, 8–14. doi: 10.1016/j.bbrc.2016.11.039
Ohkawara, B., Glinka, A., and Niehrs, C. (2011). Rspo3 binds syndecan 4 and induces Wnt/PCP signaling via clathrin-mediated endocytosis to promote morphogenesis. Dev. Cell 20, 303–314. doi: 10.1016/j.devcel.2011.01.006
Ou, H., Chen, Z., Xiang, L., Fang, Y., Xu, Y., Liu, Q., et al. (2019). Frizzled 2-induced epithelial-mesenchymal transition correlates with vasculogenic mimicry, stemness, and Hippo signaling in hepatocellular carcinoma. Cancer Sci. 110, 1169–1182. doi: 10.1111/cas.13949
Owen, D. M., Williamson, D. J., Magenau, A., and Gaus, K. (2012). Sub-resolution lipid domains exist in the plasma membrane and regulate protein diffusion and distribution. Nat. Commun. 3:1256. doi: 10.1038/ncomms2273
Ozhan, G., Sezgin, E., Wehner, D., Pfister, A. S., Kuhl, S. J., Kagermeier-Schenk, B., et al. (2013). Lypd6 enhances Wnt/beta-catenin signaling by promoting Lrp6 phosphorylation in raft plasma membrane domains. Dev. Cell 26, 331–345. doi: 10.1016/j.devcel.2013.07.020
Pacheco-Pinedo, E. C., Durham, A. C., Stewart, K. M., Goss, A. M., Lu, M. M., Demayo, F. J., et al. (2011). Wnt/beta-catenin signaling accelerates mouse lung tumorigenesis by imposing an embryonic distal progenitor phenotype on lung epithelium. J. Clin. Invest. 121, 1935–1945. doi: 10.1172/JCI44871
Parton, R. G., Hanzal-Bayer, M., and Hancock, J. F. (2006). Biogenesis of caveolae: a structural model for caveolin-induced domain formation. J. Cell Sci. 119(Pt 5), 787–796. doi: 10.1242/jcs.02853
Peng, J., Zhao, Y., Luo, Q., Chen, H., Fan, W., Pan, Z., et al. (2019). High WNT6 expression indicates unfavorable survival outcome for patients with colorectal liver metastasis after liver resection. J. Cancer 10, 2619–2627. doi: 10.7150/jca.32817
Pepperl, J., Reim, G., Luthi, U., Kaech, A., Hausmann, G., and Basler, K. (2013). Sphingolipid depletion impairs endocytic traffic and inhibits Wingless signaling. Mech. Dev. 130, 493–505. doi: 10.1016/j.mod.2013.04.001
Pridgeon, M. G., Grohar, P. J., Steensma, M. R., and Williams, B. O. (2017). Wnt Signaling in Ewing Sarcoma, Osteosarcoma, and Malignant Peripheral Nerve Sheath Tumors. Curr. Osteoporos Rep. 15, 239–246. doi: 10.1007/s11914-017-0377-9
Rapp, J., Kiss, E., Meggyes, M., Szabo-Meleg, E., Feller, D., Smuk, G., et al. (2016). Increased Wnt5a in squamous cell lung carcinoma inhibits endothelial cell motility. BMC Cancer 16:915. doi: 10.1186/s12885-016-2943-4
Rebouissou, S., and Nault, J. C. (2020). Advances in molecular classification and precision oncology in hepatocellular carcinoma. J. Hepatol. 72, 215–229. doi: 10.1016/j.jhep.2019.08.017
Riitano, G., Manganelli, V., Capozzi, A., Mattei, V., Recalchi, S., Martellucci, S., et al. (2020). LRP6 mediated signal transduction pathway triggered by tissue plasminogen activator acts through lipid rafts in neuroblastoma cells. J. Cell Communicat. Signal. 14, 315–323. doi: 10.1007/s12079-020-00551-w
Rismani, E., Fazeli, M. S., Mahmoodzadeh, H., Movassagh, A., Azami, S., Karimipoor, M., et al. (2017). Pattern of LRP6 gene expression in tumoral tissues of colorectal cancer. Cancer Biomark 19, 151–159. doi: 10.3233/CBM-160175
Ruiz de Galarreta, M., Bresnahan, E., Molina-Sanchez, P., Lindblad, K. E., Maier, B., et al. (2019). beta-Catenin Activation Promotes Immune Escape and Resistance to Anti-PD-1 Therapy in Hepatocellular Carcinoma. Cancer Discov. 9, 1124–1141. doi: 10.1158/2159-8290.CD-19-0074
Saito-Diaz, K., Benchabane, H., Tiwari, A., Tian, A., Li, B., Thompson, J. J., et al. (2018). APC Inhibits Ligand-Independent Wnt Signaling by the Clathrin Endocytic Pathway. Dev. Cell 44, 566–581e568. doi: 10.1016/j.devcel.2018.02.013
Sakane, H., Yamamoto, H., and Kikuchi, A. (2010). LRP6 is internalized by Dkk1 to suppress its phosphorylation in the lipid raft and is recycled for reuse. J. Cell Sci. 123, 360–368. doi: 10.1242/jcs.058008
Sakane, H., Yamamoto, H., Matsumoto, S., Sato, A., and Kikuchi, A. (2012). Localization of glypican-4 in different membrane microdomains is involved in the regulation of Wnt signaling. J. Cell Sci. 125(Pt 2), 449–460. doi: 10.1242/jcs.091876
Sandsmark, E., Hansen, A. F., Selnaes, K. M., Bertilsson, H., Bofin, A. M., Wright, A. J., et al. (2017). A novel non-canonical Wnt signature for prostate cancer aggressiveness. Oncotarget 8, 9572–9586. doi: 10.18632/oncotarget.14161
Sarmento, M. J., Hof, M., and Šachl, R. (2020). Interleaflet Coupling of Lipid Nanodomains – Insights From in vitro Systems. Front. Cell Dev. Biol. 8:284. doi: 10.3389/fcell.2020.00284
Schatoff, E. M., Leach, B. I., and Dow, L. E. (2017). Wnt Signaling and Colorectal Cancer. Curr. Colorectal Cancer Rep. 13, 101–110. doi: 10.1007/s11888-017-0354-9
Sekine, S., Ogawa, R., Hashimoto, T., Motohiro, K., Yoshida, H., Taniguchi, H., et al. (2017). Comprehensive characterization of RSPO fusions in colorectal traditional serrated adenomas. Histopathology 71, 601–609. doi: 10.1111/his.13265
Sezgin, E., Azbazdar, Y., Ng, X. W., Teh, C., Simons, K., Weidinger, G., et al. (2017a). Binding of canonical Wnt ligands to their receptor complexes occurs in ordered plasma membrane environments. FEBS J. 284, 2513–2526. doi: 10.1111/febs.14139
Sezgin, E., Gutmann, T., Buhl, T., Dirkx, R., Grzybek, M., Coskun, U., et al. (2015). Adaptive lipid packing and bioactivity in membrane domains. PLoS One 10:e0123930. doi: 10.1371/journal.pone.0123930
Sezgin, E., Levental, I., Mayor, S., and Eggeling, C. (2017b). The mystery of membrane organization: composition, regulation and roles of lipid rafts. Nat. Rev. Mol. Cell Biol. 18, 361–374. doi: 10.1038/nrm.2017.16
Shi, J., Wang, Y., Zeng, L., Wu, Y., Deng, J., Zhang, Q., et al. (2014). Disrupting the interaction of BRD4 with diacetylated Twist suppresses tumorigenesis in basal-like breast cancer. Cancer Cell 25, 210–225. doi: 10.1016/j.ccr.2014.01.028
Simmons, G. E. Jr., Pandey, S., Nedeljkovic-Kurepa, A., Saxena, M., Wang, A., and Pruitt, K. (2014). Frizzled 7 Expression Is Positively Regulated by SIRT1 and β-Catenin in Breast Cancer Cells. PLoS One 9:e98861. doi: 10.1371/journal.pone.0098861
Simons, K., and Toomre, D. (2000). Lipid rafts and signal transduction. Nat. Rev. Mol. Cell Biol. 1, 31–39. doi: 10.1038/35036052
Sinnberg, T., Levesque, M. P., Krochmann, J., Cheng, P. F., Ikenberg, K., Meraz-Torres, F., et al. (2018). Wnt-signaling enhances neural crest migration of melanoma cells and induces an invasive phenotype. Mol. Cancer 17:59. doi: 10.1186/s12943-018-0773-5
Sklavos, A., Poutahidis, T., Giakoustidis, A., Makedou, K., Angelopoulou, K., Hardas, A., et al. (2018). Effects of Wnt-1 blockade in DEN-induced hepatocellular adenomas of mice. Oncol. Lett. 15, 1211–1219. doi: 10.3892/ol.2017.7427
Smith, D. C., Rosen, L. S., Chugh, R., Goldman, J. W., Xu, L., Kapoun, A., et al. (2013). First-in-human evaluation of the human monoclonal antibody vantictumab (OMP-18R5; anti-Frizzled) targeting the WNT pathway in a phase I study for patients with advanced solid tumors. J. Clin. Oncol. 31, 2540–2540. doi: 10.1200/jco.2013.31.15_suppl.2540
Speer, K. F., Sommer, A., Tajer, B., Mullins, M. C., Klein, P. S., and Lemmon, M. A. (2019). Non-acylated Wnts Can Promote Signaling. Cell Rep. 26, 875–883e875. doi: 10.1016/j.celrep.2018.12.104
Steinhart, Z., and Angers, S. (2018). Wnt signaling in development and tissue homeostasis. Development 145:146589. doi: 10.1242/dev.146589
Steinhart, Z., Pavlovic, Z., Chandrashekhar, M., Hart, T., Wang, X., Zhang, X., et al. (2017). Genome-wide CRISPR screens reveal a Wnt-FZD5 signaling circuit as a druggable vulnerability of RNF43-mutant pancreatic tumors. Nat. Med. 23, 60–68. doi: 10.1038/nm.4219
Stewart, D. J. (2014). Wnt signaling pathway in non-small cell lung cancer. J. Natl. Cancer Inst. 106:djt356. doi: 10.1093/jnci/djt356
Strubberg, A. M., Liu, J., Walker, N. M., Stefanski, C. D., MacLeod, R. J., Magness, S. T., et al. (2018). Cftr Modulates Wnt/beta-Catenin Signaling and Stem Cell Proliferation in Murine Intestine. Cell Mol. Gastroenterol. Hepatol. 5, 253–271. doi: 10.1016/j.jcmgh.2017.11.013
Stypulkowski, E., Asangani, I. A., and Witze, E. S. (2018). The depalmitoylase APT1 directs the asymmetric partitioning of Notch and Wnt signaling during cell division. Sci. Signal 11:aam8705. doi: 10.1126/scisignal.aam8705
Su, W., Mo, Y., Wu, F., Guo, K., Li, J., Luo, Y., et al. (2016). miR-135b reverses chemoresistance of non-small cell lung cancer cells by downregulation of FZD1. Biomed. Pharmacother. 84, 123–129. doi: 10.1016/j.biopha.2016.09.027
Taciak, B., Pruszynska, I., Kiraga, L., Bialasek, M., and Krol, M. (2018). Wnt signaling pathway in development and cancer. J. Physiol. Pharmacol. 69:7. doi: 10.26402/jpp.2018.2.07
Takada, R., Satomi, Y., Kurata, T., Ueno, N., Norioka, S., Kondoh, H., et al. (2006). Monounsaturated fatty acid modification of Wnt protein: its role in Wnt secretion. Dev. Cell 11, 791–801. doi: 10.1016/j.devcel.2006.10.003
Taketo, M. M. (2004). Shutting down Wnt signal-activated cancer. Nat. Genet. 36, 320–322. doi: 10.1038/ng0404-320
Tang, C., Gong, L., Lvzi, X., Qiu, K., Zhang, Z., and Wan, L. (2020). Echinacoside inhibits breast cancer cells by suppressing the Wnt/beta-catenin signaling pathway. Biochem. Biophys. Res. Commun. 526, 170–175. doi: 10.1016/j.bbrc.2020.03.050
Tang, X., Ding, Y., Wang, X., Wang, X., Zhao, L., and Bi, H. (2019). miR-650 promotes non-small cell lung cancer cell proliferation and invasion by targeting ING4 through Wnt-1/beta-catenin pathway. Oncol. Lett. 18, 4621–4628. doi: 10.3892/ol.2019.10805
Tang, X., Wu, Y., Belenkaya, T. Y., Huang, Q., Ray, L., Qu, J., et al. (2012). Roles of N-glycosylation and lipidation in Wg secretion and signaling. Dev. Biol. 364, 32–41. doi: 10.1016/j.ydbio.2012.01.009
Tassew, N. G., Charish, J., Shabanzadeh, A. P., Luga, V., Harada, H., Farhani, N., et al. (2017). Exosomes Mediate Mobilization of Autocrine Wnt10b to Promote Axonal Regeneration in the Injured CNS. Cell Rep. 20, 99–111. doi: 10.1016/j.celrep.2017.06.009
Teiken, K., Kuehnel, M., Rehkaemper, J., Kreipe, H., Laenger, F., Hussein, K., et al. (2018). Non-canonical WNT6/WNT10A signal factor expression in EBV+ post-transplant smooth muscle tumors. Clin. Sarcoma Res. 8:10. doi: 10.1186/s13569-018-0096-8
Testa, U., Castelli, G., and Pelosi, E. (2018a). Lung Cancers: Molecular Characterization, Clonal Heterogeneity and Evolution, and Cancer Stem Cells. Cancers 10:10080248. doi: 10.3390/cancers10080248
Testa, U., Pelosi, E., and Castelli, G. (2018b). Colorectal cancer: genetic abnormalities, tumor progression, tumor heterogeneity, clonal evolution and tumor-initiating cells. Med. Sci. 6:6020031. doi: 10.3390/medsci6020031
Thomas, S., Preda-Pais, A., Casares, S., and Brumeanu, T. D. (2004). Analysis of lipid rafts in T cells. Mol. Immunol. 41, 399–409. doi: 10.1016/j.molimm.2004.03.022
Tisza, M. J., Zhao, W., Fuentes, J. S., Prijic, S., Chen, X., Levental, I., et al. (2016). Motility and stem cell properties induced by the epithelial-mesenchymal transition require destabilization of lipid rafts. Oncotarget 7, 51553–51568. doi: 10.18632/oncotarget.9928
Tong, J. B., Zhang, X. X., Wang, X. H., Zeng, S. J., Wang, D. Y., Zhang, Z. Q., et al. (2018). Qiyusanlong decoction suppresses lung cancer in mice via Wnt/beta-catenin pathway. Mol. Med. Rep. 17, 5320–5327. doi: 10.3892/mmr.2018.8478
Tong, Y., Liu, Y., Zheng, H., Zheng, L., Liu, W., Wu, J., et al. (2016). Artemisinin and its derivatives can significantly inhibit lung tumorigenesis and tumor metastasis through Wnt/beta-catenin signaling. Oncotarget 7, 31413–31428. doi: 10.18632/oncotarget.8920
Toyama, T., Lee, H. C., Koga, H., Wands, J. R., and Kim, M. (2010). Noncanonical Wnt11 inhibits hepatocellular carcinoma cell proliferation and migration. Mol. Cancer Res. 8, 254–265. doi: 10.1158/1541-7786.MCR-09-0238
Tsai, C. H., Chiu, J. H., Yang, C. W., Wang, J. Y., Tsai, Y. F., Tseng, L. M., et al. (2015). Molecular characteristics of recurrent triple-negative breast cancer. Mol. Med. Rep. 12, 7326–7334. doi: 10.3892/mmr.2015.4360
Tung, E. K., Wong, B. Y., Yau, T. O., and Ng, I. O. (2012). Upregulation of the Wnt co-receptor LRP6 promotes hepatocarcinogenesis and enhances cell invasion. PLoS One 7:e36565. doi: 10.1371/journal.pone.0036565
van Amerongen, R., Bowman, Angela, N., and Nusse, R. (2012). Developmental Stage and Time Dictate the Fate of Wnt/β-Catenin-Responsive Stem Cells in the Mammary Gland. Cell Stem Cell 11, 387–400. doi: 10.1016/j.stem.2012.05.023
Ventura, R., Mordec, K., Waszczuk, J., Wang, Z., Lai, J., Fridlib, M., et al. (2015). Inhibition of de novo Palmitate Synthesis by Fatty Acid Synthase Induces Apoptosis in Tumor Cells by Remodeling Cell Membranes, Inhibiting Signaling Pathways, and Reprogramming Gene Expression. EBioMed. 2, 808–824. doi: 10.1016/j.ebiom.2015.06.020
Vesel, M., Rapp, J., Feller, D., Kiss, E., Jaromi, L., Meggyes, M., et al. (2017). ABCB1 and ABCG2 drug transporters are differentially expressed in non-small cell lung cancers (NSCLC) and expression is modified by cisplatin treatment via altered Wnt signaling. Respir. Res. 18:52. doi: 10.1186/s12931-017-0537-6
von Erlach, T. C., Bertazzo, S., Wozniak, M. A., Horejs, C.-M., Maynard, S. A., Attwood, S., et al. (2018). Cell-geometry-dependent changes in plasma membrane order direct stem cell signalling and fate. Nat. Mater. 17, 237–242. doi: 10.1038/s41563-017-0014-0
Waks, A. G., and Winer, E. P. (2019). Breast Cancer Treatment: A Review. JAMA 321, 288–300. doi: 10.1001/jama.2018.19323
Wang, J., Lu, R., Fu, X., Dan, Z., Zhang, Y. G., Chang, X., et al. (2018). Novel Regulatory Roles of Wnt1 in Infection-Associated Colorectal Cancer. Neoplasia 20, 499–509. doi: 10.1016/j.neo.2018.03.001
Wang, S., Qiu, M., Xia, W., Xu, Y., Mao, Q., Wang, J., et al. (2016). Glypican-5 suppresses Epithelial-Mesenchymal Transition of the lung adenocarcinoma by competitively binding to Wnt3a. Oncotarget 7, 79736–79746. doi: 10.18632/oncotarget.12945
Wang, T., Liu, X., and Wang, J. (2019a). Up-regulation of Wnt5a inhibits proliferation and migration of hepatocellular carcinoma cells. J. Cancer Res. Ther. 15, 904–908. doi: 10.4103/jcrt.JCRT_886_18
Wang, W., He, Y., Rui, J., and Xu, M. Q. (2019b). miR-410 acts as an oncogene in colorectal cancer cells by targeting dickkopf-related protein 1 via the Wnt/beta-catenin signaling pathway. Oncol. Lett. 17, 807–814. doi: 10.3892/ol.2018.9710
Wang, Z., Sun, P., Gao, C., Chen, J., Li, J., Chen, Z., et al. (2017). Down-regulation of LRP1B in colon cancer promoted the growth and migration of cancer cells. Exp. Cell Res. 357, 1–8. doi: 10.1016/j.yexcr.2017.04.010
Wei, W., Chua, M. S., Grepper, S., and So, S. K. (2009). Blockade of Wnt-1 signaling leads to anti-tumor effects in hepatocellular carcinoma cells. Mol. Cancer 8:76. doi: 10.1186/1476-4598-8-76
Willert, K., and Nusse, R. (2012). Wnt proteins. Cold Spring Harb. Perspect. Biol. 4:a007864. doi: 10.1101/cshperspect.a007864
Willert, K., Brown, J. D., Danenberg, E., Duncan, A. W., Weissman, I. L., Reya, T., et al. (2003). Wnt proteins are lipid-modified and can act as stem cell growth factors. Nature 423, 448–452.
Winn, R. A., Marek, L., Han, S. Y., Rodriguez, K., Rodriguez, N., Hammond, M., et al. (2005). Restoration of Wnt-7a expression reverses non-small cell lung cancer cellular transformation through frizzled-9-mediated growth inhibition and promotion of cell differentiation. J. Biol. Chem. 280, 19625–19634. doi: 10.1074/jbc.M409392200
Wu, W., Dang, S., Feng, Q., Liang, J., Wang, Y., and Fan, N. (2017). MicroRNA-542-3p inhibits the growth of hepatocellular carcinoma cells by targeting FZD7/Wnt signaling pathway. Biochem. Biophys. Res. Commun. 482, 100–105. doi: 10.1016/j.bbrc.2016.10.136
Xi, S., Xu, H., Shan, J., Tao, Y., Hong, J. A., Inchauste, S., et al. (2013). Cigarette smoke mediates epigenetic repression of miR-487b during pulmonary carcinogenesis. J. Clin. Invest. 123, 1241–1261. doi: 10.1172/JCI61271
Xia, Y., Wei, K., Hu, L. Q., Zhou, C. R., Lu, Z. B., Zhan, G. S., et al. (2020). Exosome-mediated transfer of miR-1260b promotes cell invasion through Wnt/beta-catenin signaling pathway in lung adenocarcinoma. J. Cell Physiol. 235, 6843–6853. doi: 10.1002/jcp.29578
Xiao, J., Xing, F., Liu, Y., Lv, Y., Wang, X., Ling, M. T., et al. (2018). Garlic-derived compound S-allylmercaptocysteine inhibits hepatocarcinogenesis through targeting LRP6/Wnt pathway. Acta Pharm. Sin B 8, 575–586. doi: 10.1016/j.apsb.2017.10.003
Xie, W., Zhang, Y., He, Y., Zhang, K., Wan, G., Huang, Y., et al. (2018). A novel recombinant human Frizzled-7 protein exhibits anti-tumor activity against triple negative breast cancer via abating Wnt/beta-catenin pathway. Int. J. Biochem. Cell Biol. 103, 45–55. doi: 10.1016/j.biocel.2018.08.004
Xu, J., Prosperi, J. R., Choudhury, N., Olopade, O. I., and Goss, K. H. (2015). beta-Catenin is required for the tumorigenic behavior of triple-negative breast cancer cells. PLoS One 10:e0117097. doi: 10.1371/journal.pone.0117097
Xue, Y., Chen, C., Xu, W., Xu, H., Zheng, J., and Gu, Y. (2018). Downregulation of Frizzled-7 induces the apoptosis of hepatocellular carcinoma cells through inhibition of NF-kappaB. Oncol. Lett. 15, 7693–7701. doi: 10.3892/ol.2018.8292
Yamamoto, H., Komekado, H., and Kikuchi, A. (2006). Caveolin is necessary for Wnt-3a-dependent internalization of LRP6 and accumulation of beta-catenin. Dev. Cell 11, 213–223. doi: 10.1016/j.devcel.2006.07.003
Yamamoto, S., Nishimura, O., Misaki, K., Nishita, M., Minami, Y., Yonemura, S., et al. (2008). Cthrc1 selectively activates the planar cell polarity pathway of Wnt signaling by stabilizing the Wnt-receptor complex. Dev. Cell 15, 23–36. doi: 10.1016/j.devcel.2008.05.007
Yan, J., Liu, T., Zhou, X., Dang, Y., Yin, C., and Zhang, G. (2016). FZD6, targeted by miR-21, represses gastric cancer cell proliferation and migration via activating non-canonical wnt pathway. Am. J. Transl. Res. 8, 2354–2364.
Yang, J. D., Hainaut, P., Gores, G. J., Amadou, A., Plymoth, A., and Roberts, L. R. (2019a). A global view of hepatocellular carcinoma: trends, risk, prevention and management. Nat. Rev. Gastroenterol. Hepatol. 16, 589–604. doi: 10.1038/s41575-019-0186-y
Yang, J., Ye, Z., Mei, D., Gu, H., and Zhang, J. (2019b). Long noncoding RNA DLX6-AS1 promotes tumorigenesis by modulating miR-497-5p/FZD4/FZD6/Wnt/beta-catenin pathway in pancreatic cancer. Cancer Manag. Res. 11, 4209–4221. doi: 10.2147/CMAR.S194453
Yang, L., Wu, X., Wang, Y., Zhang, K., Wu, J., Yuan, Y. C., et al. (2011). FZD7 has a critical role in cell proliferation in triple negative breast cancer. Oncogene 30, 4437–4446. doi: 10.1038/onc.2011.145
Yang, M., Li, S. N., Anjum, K. M., Gui, L. X., Zhu, S. S., Liu, J., et al. (2013). A double-negative feedback loop between Wnt-beta-catenin signaling and HNF4alpha regulates epithelial-mesenchymal transition in hepatocellular carcinoma. J. Cell Sci. 126(Pt 24), 5692–5703. doi: 10.1242/jcs.135053
Ye, C., Xu, M., Lin, M., Zhang, Y., Zheng, X., Sun, Y., et al. (2019). Overexpression of FZD7 is associated with poor survival in patients with colon cancer. Pathol. Res. Pract. 215:152478. doi: 10.1016/j.prp.2019.152478
Yeon, M., Lee, S., Lee, J. E., Jung, H. S., Kim, Y., and Jeoung, D. (2019). CAGE-miR-140-5p-Wnt1 Axis Regulates Autophagic Flux, Tumorigenic Potential of Mouse Colon Cancer Cells and Cellular Interactions Mediated by Exosomes. Front. Oncol. 9:1240. doi: 10.3389/fonc.2019.01240
Yin, L., Gao, Y., Zhang, X., Wang, J., Ding, D., Zhang, Y., et al. (2016). Niclosamide sensitizes triple-negative breast cancer cells to ionizing radiation in association with the inhibition of Wnt/beta-catenin signaling. Oncotarget 7, 42126–42138. doi: 10.18632/oncotarget.9704
Yin, P., Wang, W., Gao, J., Bai, Y., Wang, Z., Na, L., et al. (2020). Fzd2 Contributes to Breast Cancer Cell Mesenchymal-Like Stemness and Drug Resistance. Oncol. Res. 28, 273–284. doi: 10.3727/096504020X15783052025051
Yu, J., Chen, L., Cui, B., Widhopf, G. F. II, Shen, Z., Wu, R., et al. (2016). Wnt5a induces ROR1/ROR2 heterooligomerization to enhance leukemia chemotaxis and proliferation. J. Clin. Invest. 126, 585–598. doi: 10.1172/JCI83535
Yuzugullu, H., Benhaj, K., Ozturk, N., Senturk, S., Celik, E., Toylu, A., et al. (2009). Canonical Wnt signaling is antagonized by noncanonical Wnt5a in hepatocellular carcinoma cells. Mol. Cancer 8:90. doi: 10.1186/1476-4598-8-90
Zeng, C. M., Chen, Z., and Fu, L. (2018a). Frizzled Receptors as Potential Therapeutic Targets in Human Cancers. Int. J. Mol. Sci. 19:19051543. doi: 10.3390/ijms19051543
Zeng, H., Lu, B., Zamponi, R., Yang, Z., Wetzel, K., Loureiro, J., et al. (2018b). mTORC1 signaling suppresses Wnt/beta-catenin signaling through DVL-dependent regulation of Wnt receptor FZD level. Proc. Natl. Acad. Sci. U S A. 115, E10362–E10369. doi: 10.1073/pnas.1808575115
Zhai, L., Chaturvedi, D., and Cumberledge, S. (2004). Drosophila wnt-1 undergoes a hydrophobic modification and is targeted to lipid rafts, a process that requires porcupine. J. Biol. Chem. 279, 33220–33227. doi: 10.1074/jbc.M403407200
Zhang, Q., Fan, H., Liu, H., Jin, J., Zhu, S., Zhou, L., et al. (2020). WNT5B exerts oncogenic effects and is negatively regulated by miR-5587-3p in lung adenocarcinoma progression. Oncogene 39, 1484–1497. doi: 10.1038/s41388-019-1071-4
Zhang, W., Sun, Z., Su, L., Wang, F., Jiang, Y., Yu, D., et al. (2018). miRNA-185 serves as a prognostic factor and suppresses migration and invasion through Wnt1 in colon cancer. Eur. J. Pharmacol. 825, 75–84. doi: 10.1016/j.ejphar.2018.02.019
Zheng, X. L., and Yu, H. G. (2018). Wnt6 contributes tumorigenesis and development of colon cancer via its effects on cell proliferation, apoptosis, cell-cycle and migration. Oncol. Lett. 16, 1163–1172. doi: 10.3892/ol.2018.8729
Zhong, Z., Sepramaniam, S., Chew, X. H., Wood, K., Lee, M. A., Madan, B., et al. (2019). PORCN inhibition synergizes with PI3K/mTOR inhibition in Wnt-addicted cancers. Oncogene 38, 6662–6677. doi: 10.1038/s41388-019-0908-1
Zhong, Z., Shan, M., Wang, J., Liu, T., Shi, Q., and Pang, D. (2016). Decreased Wnt5a Expression is a Poor Prognostic Factor in Triple-Negative Breast Cancer. Med. Sci. Monit. 22, 1–7. doi: 10.12659/msm.894821
Keywords: Wnt, frizzled, plasma membrane, cancer, lipid raft
Citation: Azbazdar Y, Karabicici M, Erdal E and Ozhan G (2021) Regulation of Wnt Signaling Pathways at the Plasma Membrane and Their Misregulation in Cancer. Front. Cell Dev. Biol. 9:631623. doi: 10.3389/fcell.2021.631623
Received: 20 November 2020; Accepted: 04 January 2021;
Published: 21 January 2021.
Edited by:
Serge Roche, Institut National de la Santé et de la Recherche Médicale (INSERM), FranceReviewed by:
Julie Pannequin, Centre National de la Recherche Scientifique (CNRS), FranceCopyright © 2021 Azbazdar, Karabicici, Erdal and Ozhan. This is an open-access article distributed under the terms of the Creative Commons Attribution License (CC BY). The use, distribution or reproduction in other forums is permitted, provided the original author(s) and the copyright owner(s) are credited and that the original publication in this journal is cited, in accordance with accepted academic practice. No use, distribution or reproduction is permitted which does not comply with these terms.
*Correspondence: Gunes Ozhan, Z3VuZXMub3poYW5AaWJnLmVkdS50cg==
†These authors have contributed equally to this work
Disclaimer: All claims expressed in this article are solely those of the authors and do not necessarily represent those of their affiliated organizations, or those of the publisher, the editors and the reviewers. Any product that may be evaluated in this article or claim that may be made by its manufacturer is not guaranteed or endorsed by the publisher.
Research integrity at Frontiers
Learn more about the work of our research integrity team to safeguard the quality of each article we publish.