- 1School of Life Sciences, Beijing Institute of Technology, Beijing, China
- 2School of Biological Sciences, Georgia Institute of Technology, Atlanta, GA, United States
Gain-of-function mutation of SHP2 is a central regulator in tumorigenesis and cancer progression through cell-autonomous mechanisms. Activating mutation of SHP2 in microenvironment was identified to promote cancerous transformation of hematopoietic stem cell in non-autonomous mechanisms. It is interesting to see whether therapies directed against SHP2 in tumor or microenvironmental cells augment antitumor efficacy. In this review, we summarized different types of gain-of-function SHP2 mutations from a human disease. In general, gain-of-function mutations destroy the auto-inhibition state from wild-type SHP2, leading to consistency activation of SHP2. We illustrated how somatic or germline mutation of SHP2 plays an oncogenic role in tumorigenesis, stemness maintenance, invasion, etc. Moreover, the small-molecule SHP2 inhibitors are considered as a potential strategy for enhancing the efficacy of antitumor immunotherapy and chemotherapy. We also discussed the interconnection between phase separation and activating mutation of SHP2 in drug resistance of antitumor therapy.
Introduction
Protein tyrosine phosphatases (PTPs) are widely expressed in most tissues. They play a regulatory role in various cell signaling events, such as mitogenic activation, metabolic control, transcription regulation, and cell migration. Src homology region 2 protein tyrosine phosphatase 2 (SHP2), encoded by PTPN11, is the first reported non-receptor protein oncogenic tyrosine phosphatase and required for the survival, proliferation, and differentiation of multiple cell types (Yang et al., 2013). Studies reported that germline mutations in the PTPN11 gene contribute to Noonan syndrome (NS) (Niemeyer, 2018; Pierpont and Digilio, 2018; Bellio et al., 2019), which is a multisystem developmental disorder disease characterized by short stature, chest deformity, webbed neck, bleeding diatheses, cardiac defects, and mental retardation (Grossmann et al., 2010; Roberts et al., 2013; Liu et al., 2020). Patients with NS tend to develop juvenile myelomonocytic leukemia (JMML)-like myeloproliferative neoplasm (MPN) (Strullu et al., 2014). Hyperactive Ras signaling is the main driving event caused by somatic mutations in KRAS, NRAS, or PTPN11 in about 50% of JMML patients (Tartaglia et al., 2004; Lipka et al., 2017). Mutations in NF1, NRAS, KRAS, CBL, and PTPN11 account for diagnosis in 85% of JMML patients (Stieglitz et al., 2015). Germline mutation of PTPN11 is found in 50% of the patients with NS (Dong et al., 2016). Somatic PTPN11 mutations are also associated with multiple types of human malignancies, such as leukemia and other solid tumors (Yang et al., 2013). According to previous reports, PTPN11 mutations affect disease progression by unblocking PTP activity and enhancement of the catalytic activity via disrupting the auto-inhibition status or regulating the substrate binding ability of the catalytic pocket (Guo et al., 2017). SHP2 is proved to promote tumor proliferation, invasion, metastasis, and chemotherapeutic resistance (Zhang et al., 2015).
Gain-of-function (GOF) mutation SHP2 promotes tumor progression in cell-autonomous and non-autonomous mechanisms. SHP2 plays a central and indispensable role in hematopoiesis and leukemogenesis via its complex involvement with cellular signaling pathways (Pandey et al., 2017). Furthermore, activating mutations SHP2 in the bone marrow microenvironment, but not in the tumor cells, also promote childhood MPN development and progression through detrimental effects on hematopoietic stem cells (HSCs) in non-autonomous mechanism (Dong et al., 2016). Thus, a comprehensive understanding of how SHP2 contributes to oncogenesis will provide novel insights into pathogenesis.
It was of great interest to discover small-molecule SHP2 inhibitors as a potential cancer therapeutic target in recent years. The study in SHP2 inhibition did not make a breakthrough until the discovery of inhibitors that occupied allosteric sites of SHP2 (Chen et al., 2016; Shen et al., 2020). This novel discovery shed light on efficient SHP2 inhibitors (Garcia Fortanet et al., 2016). Targeting these non-conserved allosteric sites tends to improve drug selectivity. Consequently, several other allosteric drugs were continuously discovered with higher expectation for cell permeability, oral availability, etc. (Chen et al., 2016; Shen et al., 2020). Currently, a few clinical trials of SHP2 allosteric inhibitors showed remarkable antitumor benefits (Liu et al., 2020).
In this review, we summarized the structural change and functional regulation of oncogenic SHP2 mutations. We discussed how SHP2 affects tumor progressions in cell-autonomous and non-autonomous mechanisms. Since SHP2 is considered as a novel antitumor target, we also summarized currently used SHP2 inhibitors as well as their potentials in the application of cancer treatment.
The Structural Conformation Changes and Functional Regulation of Oncogenic SHP2
SHP2 consists of one PTP catalytic domain that locates at the C-terminal region, two tandem C-SH2 and N-SH2 domains, and a C-terminal tail with tyrosyl phosphorylation sites (Feng et al., 1993). Human SHP2 encodes 593 amino acids, among which the N-SH2 domain locates at 3–104, C-SH2 domain locates at 112–216, the PTP domain locates at 221–524, and the C-terminal locates at 525–593. The N-SH2 domain has two non-overlapping ligand binding sites to regulate its de-phosphorylated activity. The C-SH2 domain provides binding energy and specificity (Zhang et al., 2015). The PTP domain contains the catalytic structures, such as the P ring (Yu et al., 2013), to de-phosphorylate substrates.
SHP2 activity is regulated by conformational switch that N-SH2 binds to PTP to block or binds to phosphorylated proteins to unblock its phosphatase activity (Zhang et al., 2015). SHP2 mainly exists in a closed self-inhibitory conformation (Zhang et al., 2020). In the inactive state, the D-E ring of the N-SH2 domain is inserted into the PTP domain to block the phosphatase activity site (Rehman et al., 2019). Studies reported that the stimulation of growth factor receptor [e.g., epidermal growth factor receptor (EGFR)] or the interaction between the N-SH2 domain with phosphorylated tyrosine residues of scaffold proteins led to the dissociation of N-SH2 with the PTP domain; thus, the active region of PTP will be exposed and SHP2 is activated (Liu et al., 2016). The structure and function regulation of SHP2 is shown in Figure 1. In addition, SHP2 is activated via the phosphorylation on two tyrosine residues (Y542 and Y580) within the C-terminal region (Voena et al., 2007).
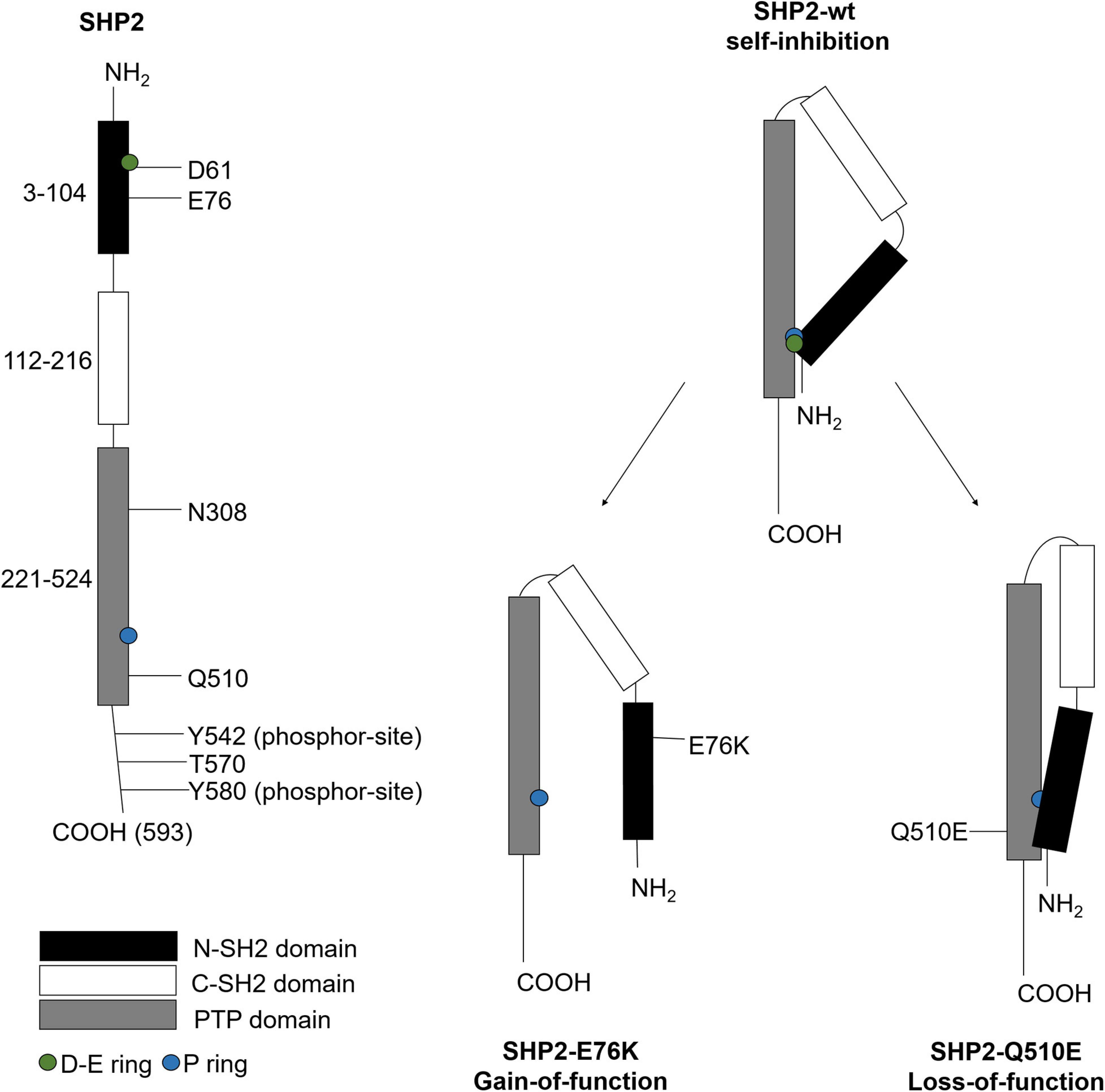
Figure 1. The structure and function regulation of SHP2. SHP2 contains 593 amino acids, including various important active regulatory sites. When these sites are mutated, SHP2 gains or loses function. The D-E ring and P ring on the three-dimensional structure of SHP2 play an important role in regulating catalytic activity. When SHP2 is in self-inhibition state, the P ring on the PTP domain will cover the D-E ring on the N-SH2 domain. SHP2 is activated by gain-of-function mutations, such as E76K, or inactivated by loss-of-function mutations, such as Q510E.
Gain-of-function mutations of SHP2 affect the interaction between N-SH2 and PTP (Tajan et al., 2015). GOF mutations mainly occur in the N-SH2 or PTP domains of cancer patients. These mutations lead to partial or complete dissociation of the binding domain of N-SH2 and PTP, and enhancement of phosphatase activity (Pannone et al., 2017). For example, the N-SH2 domain of PTPN11E76K/+ mutant leads to consistently expose the catalytic site of the PTP domain (LaRochelle et al., 2018).
Protein tyrosine phosphatase is the catalytic domain that mediates a variety of cellular signaling processes including cell growth, differentiation, mitotic cycle, and oncogenic transformation. Several GOF mutations or loss-of-function (LOF) mutations of SHP2 are reported to be associated with cancer progression and other diseases (Table 1). Multiple researches reported that GOF mutations SHP2D61G and SHP2E76K are related to MPN (Xu et al., 2010), NS (De Rocca Serra-Nédélec et al., 2012), or JMML (Yang et al., 2008) through activation of Ras/Erk signaling in vivo and in vitro. SHP2E76K promotes tumorigenesis of colorectal cancer (CAC) and induces epithelial-to-mesenchymal transition (EMT) through the Wnt/β-catenin signaling pathway (Schneeberger et al., 2014). Studies reported that SHP2 is required for the growth of KRAS-mutant non-small-cell lung cancers (NSCLCs), and its inhibition leads to a potential antitumor therapy (Mainardi et al., 2018). More details are shown in Table 1.
A recent study reported that multivalent electrostatic interaction among the PTP domains leads to liquid–liquid phase separation (LLPS) of mutated SHP2. It recruits wild-type SHP2 and hyperactivates the phosphatase catalytic activity of SHP2, which further leads to hyperactivation of mitogen-activated protein kinase (MAPK) signaling pathway (Zhu et al., 2020). It also reported that SHP2 mutants in LEOPARD syndrome induce robust phase transition to liquid-like droplets in cells, which recruit and activate wild-type SHP2 to promote extracellular signal-regulated kinase1/2 (Erk1/2) activation (Zhu et al., 2020). It suggests that disease-associated SHP2 mutations promote GOF LLPS and consequently lead to overactivation of wild-type SHP2. The formation of LLPS is a mechanism of SHP2 activation and a potential contributor to developmental diseases and cancers.
Gain-of-Function SHP2 Promotes Tumor Progression in Cell-Autonomous or Non-Autonomous Mechanisms
The oncogenic SHP2 promotes cancer progression at a cellular level through two mechanisms. On the one hand, malignant proliferation results from tumor cell-autonomous oncogenic SHP2 mutations. Studies have reported that SHP2D61Y causes fatal myeloproliferative disorder via cell-autonomous effects on multiple stages of hematopoiesis (Chan et al., 2009). On the other hand, SHP2 in tumor microenvironment cells, such as mesenchymal stem cells (MSCs) and/or immunological cells, is responsible for tumor progression (Dong et al., 2016).
SHP2 Mutations Promote Tumor Progression in Cell-Autonomous Mechanism
It has been established that oncogenic alterations in the Ras/Raf/MEK/Erk pathway drive the neoplasia of multiple cancer types. SHP2 is expressed in multiple types of cells and regulates cell survival and proliferation through activation of the Ras/Erk signaling pathway (Chan et al., 2008; Bondeson, 2017). SHP2 negatively regulates the cytokine receptor-mediated JAK-STAT signaling pathway (Xu and Qu, 2008). Some studies also reported that SHP2E76K in the glioblastoma multiforme (GBM) cells promotes the malignant behavior of tumor cells through the Erk/cAMP responsive element binding protein (CREB) signaling pathway (Yang et al., 2019). These evidences indicated that activated SHP2 in tumor cells established oncogenic signaling pathways to promote tumor progression.
SHP2 established proliferative signaling pathways to promote tumorigenesis. Studies showed that SHP2E76K activates Erk and Src to promote the occurrence of lung tumors (Schneeberger et al., 2014). SHP2 dephosphorylates Ras to increase the association between Ras and Raf, thus activating the proliferation-promoting Ras/Erk/MAPK signaling pathway. Overexpression of SHP2 activates Erk/Akt signaling pathways and further leads to tumorigenesis of breast cancer (Hu et al., 2014). SHP2 activity is elevated by pathological analysis of astrocytes isolated from GBM. Patient-derived GBM specimens exhibit hyperactive Ras, while inhibition of SHP2 decelerates the progression of low-grade astrocytoma to GBM in a spontaneous transgenic glioma mouse model (Bunda et al., 2015). The observation that conditional knockout of SHP2 in the ErbB2 transgenic mice prevents tumorigenesis by blocking the expression of the ErbB2 indicates that SHP2 induces tumorigenesis through regulating the expression of oncogene (Zhao H. et al., 2019). Studies also revealed that SHP2 affects proliferation and tumorigenicity of glioblastoma stem cells (GSCs) through regulating the expression of transcription factor SOX2 (Roccograndi et al., 2017).
Oncogenic SHP2 promotes tumor progression. It has been demonstrated that targeting both Ras and its upstream or downstream proteins has no cancer-suppressing effect in Ras-mutant cancer (Bernards, 2012). However, studies revealed that SHP2 inhibition in KRAS-mutant NSCLCs in vivo under growth factor-limiting conditions triggers senescence response (Mainardi et al., 2018). Furthermore, genetic deletion of PTPN11 or inhibition of SHP2 in KRAS-mutant-driven tumors delays tumor progression (Ruess et al., 2018). Another study pointed out that SHP2 small molecular allosteric inhibitor RMC-4550 decreases oncogenic-related Ras/Raf/MEK/Erk signaling to impair the growth of cancer-bearing Ras-GTP-dependent oncogenic BRAF mutation, NF1 loss, or nucleotide-cycling oncogenic Ras (Nichols et al., 2018). Wang et al. showed that SHP2E76K and SHP2D61G induce cytokine allergy of hematopoietic cells by enhancing the production of reactive oxygen species (ROS). They interact with a new substrate in the mitochondria to increase the aerobic metabolism of the mitochondria and drive the development of myeloproliferative diseases and malignant leukemia (Xu et al., 2013).
Additionally, SHP2 promotes tumor metastasis. SHP2 decreases the phosphorylation of PAR3 (partitioning-defective 3) to impair the formation of polarity-regulating protein complex, resulting in a disrupted cell polarity, dysregulated cell–cell junctions, and increased EMT, which is one of the essential steps for prostate cancer metastasis (Zhang et al., 2016). Other studies demonstrated that SHP2 overexpression enhances ovarian tumor invasion by activating the PI3K/Akt axis (Hu et al., 2017). SHP2 knockdown inhibits cell migration in the HeLa and SiHa cervical cancer cell lines, while SHP2 overexpression has the opposite effects. This study further pointed out that the tumor-promoting effect of SHP2 is partially related to Akt signaling (Cao et al., 2019). Other studies reported that SHP2E76K promotes GBM tumor metastasis via the activation of Erk/CREB axis (Yang et al., 2019).
Gain-of-function mutations of SHP2 in cancer stem cells (CSCs) promote cell expansion, proliferation, and stemness maintenance and are responsible for drug resistance. Studies reported that activated SHP2 in CSCs promotes liver CSC expansion by activating β-catenin signaling (Xiang et al., 2017). Treatment of NSCLC by tyrosine kinase inhibitor (TKI) failed because SHP2 induces the stemness of KRAS-mutant NSCLCs. The inhibition of SHP2 attenuates the enhanced stemness (Jiang et al., 2019), suggesting the important role of tumor cell-autonomous SHP2 in stemness maintenance of CSCs. Other studies revealed that SHP2 catalytic activity is required for proliferation and tumorigenic transformation of GSCs (Roccograndi et al., 2017). A recent study revealed that PTPN11G226A mutation is essential in hematopoietic differentiation of JMML-derived induced pluripotent stem cells (iPSCs), suggesting the significant role of SHP2 in regulating stem cell bioactivity (Shigemura et al., 2019). The oncogenic function of tumor cell-autonomous SHP2 is shown in Figure 2.
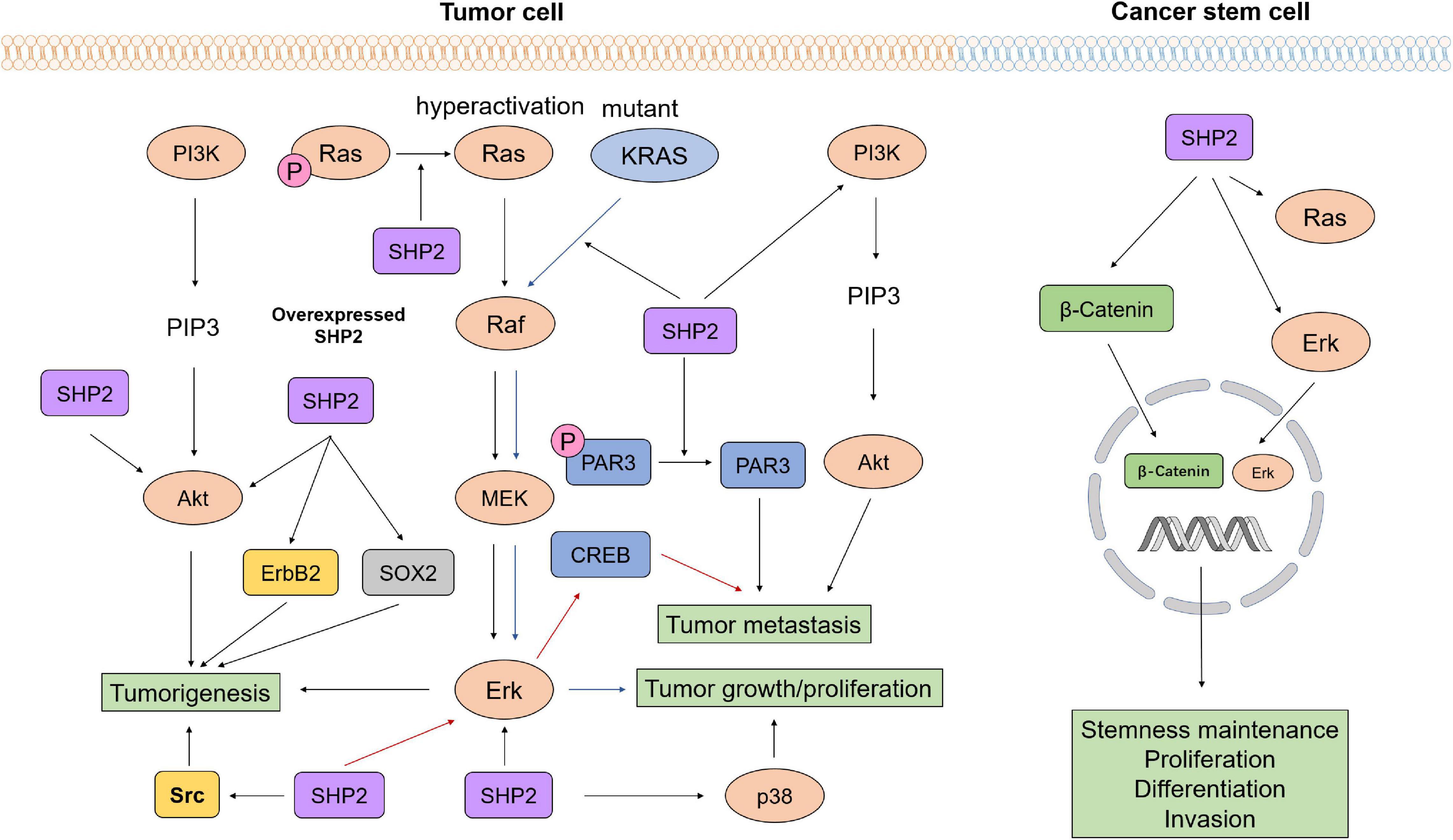
Figure 2. The oncogenic function of tumor cell-autonomous SHP2 gain-of-function mutations. As a signaling hub, SHP2 affects various tumor developmental stages by regulating multiple signaling pathways, such as PI3K/Akt, Ras/Raf/MEK/Erk signaling. SHP2 promotes proliferation, differentiation, and invasion and maintains stemness of cancer stem cells via activation of β-catenin and Ras/Erk signaling.
SHP2 in Tumor Microenvironment Affects Tumor Progression via Non-autonomous Mechanism
Cre/LoxP system is applied to elucidate the specific role of non-autonomous SHP2 mutations in cancer. Some studies examined the detailed pathogenesis of metachondromatosis by deleting PTPN11 specifically in monocytes, macrophages, and osteoclasts (lysozyme M-Cre; LysM-Cre) or in cathepsin K (Ctsk)-expressing cells using PTPN11flox/flox and Cre recombinase transgenic mice (Yang et al., 2013). PTPN11 deletion in CD4+ cells driven by CD4 Cre recombinase demonstrated that although the ablation of SHP2 does not affect T cell development and functions, it causes cartilage tumors in a T cell-independent manner (Miah et al., 2017). More importantly, a previous study on the leukemogenic effect of SHP2 mutation in bone marrow microenvironment generated PTPN11E76K/+/Nestin-Cre+ transgenic mice with a neo cassette and a stop codon inserted ahead of PTPN11E76K, and thus, the SHP2E76K expressed with the deletion of neo cassette by Cre DNA recombinase (Dong et al., 2016). Ding et al. (2012) studied the cellular sources of Scf (stem cell factor) that affects HSC frequency and function by conditionally deleting Scf from hematopoietic cells, osteoblasts, nestin-cre- or nestin-creER-expressing cells, endothelial cells, or leptin receptor (Lepr)-expressing perivascular stromal cells. They found that HSCs were depleted when Scf was conditionally deleted in perivascular cells; thus, HSCs were proved to reside in a perivascular niche where they remained undifferentiated. Studying the oncogenic effects of SHP2 mutations in different cell populations in the tumor microenvironment could follow similar methods. In general, the use of transgenic mice combined with the Cre/LoxP system is a reliable approach for studying the role of SHP2 in diseases, especially in tumors.
SHP2 Mutations in Bone Marrow Microenvironment Promotes Leukemogenesis
SHP2 mutations promote the leukemogenesis of HSCs in non-cell-autonomous mechanisms. HSCs reside in distinct bone marrow niches defined by the surrounding stromal cells and the regulatory molecules they produce (Greenbaum et al., 2013), wherein the transduction signaling generated by surrounding microenvironment cells affects the self-renewal, proliferation of HSCs, and MPN formation. Studies have demonstrated that PTPN11 mutation in the bone marrow microenvironment, such as MSCs, promotes the development and progression of childhood MPN through the profound negative effects on HSCs (Dong et al., 2016), which demonstrated that not only tumor cell-autonomous SHP2 but also mutations in microenvironment cells leads to tumorigenesis. This study clarified the mechanism of leukemia recurrence. Using specifically expressed cre, such as Prx1-cre (Greenbaum et al., 2013), nestin-cre (Méndez-Ferrer et al., 2010), Lepr-cre (Ding et al., 2012), and Osterix-cre (Tang et al., 2016), to induce PTPN11 mutations in bone marrow mesenchymal cells leads to MPN, but SHP2 mutations in endothelial cells (VE-Cadherin-cre) and osteoblasts (Oc-cre) will not lead to MPN formation (Dong et al., 2016), which indicates that SHP2 mutations in the specific components of bone marrow microenvironment show leukemogenic effects (Figure 3).
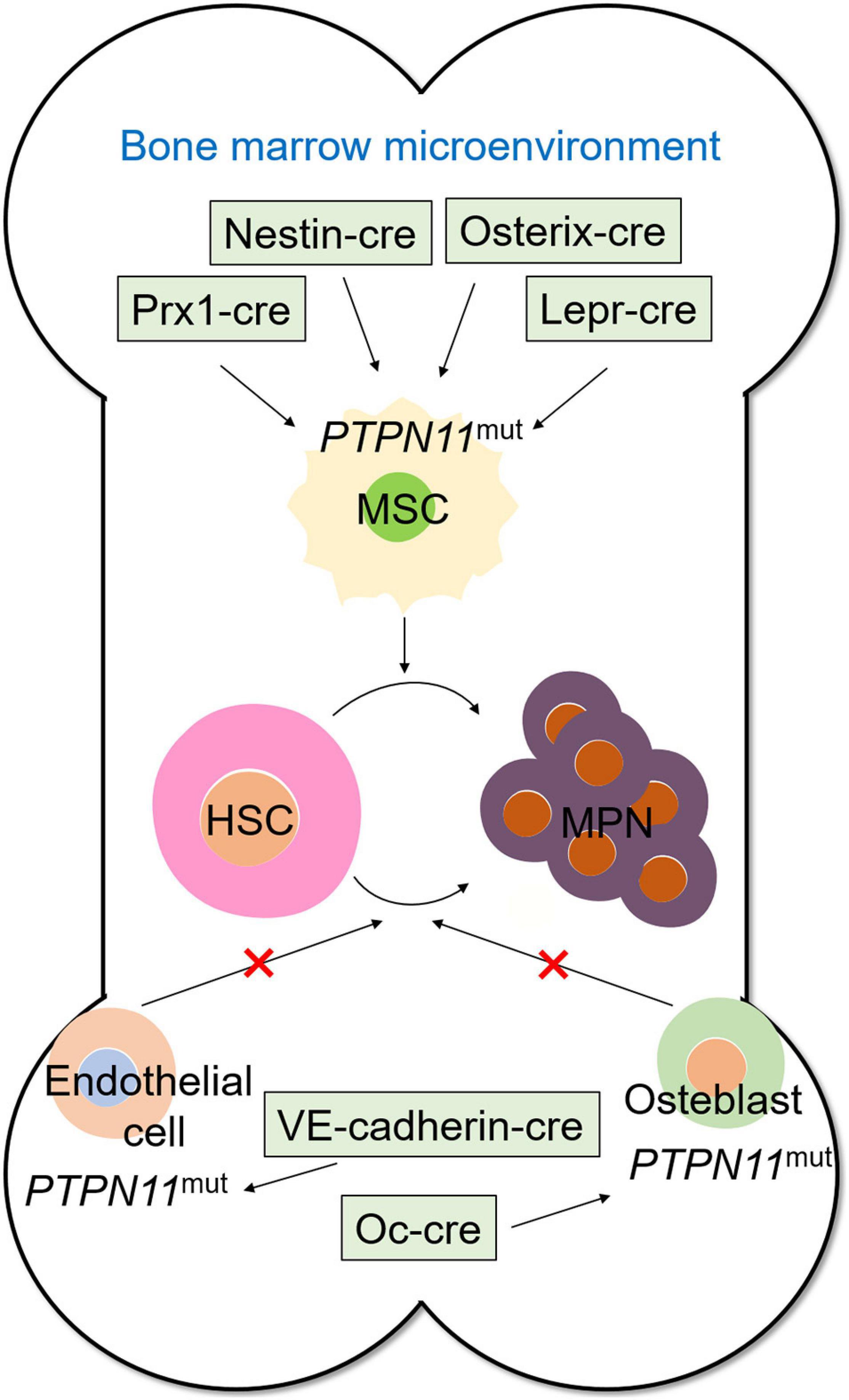
Figure 3. SHP2 mutation in bone marrow microenvironment promotes myeloproliferative neoplasm. PTPN11 mutation induced by Prx1-cre, Nestin-cre, Osterix-cre, and Lepr-cre in mesenchymal stem cells promotes tumorigenicity of hematopoietic stem cells, whereas PTPN11 mutation induced by VE-Cadherin-cre in endothelial cells and Oc-cre in osteoblasts shows no myeloproliferative neoplasm formation-promoting effect.
SHP2 in Immune Microenvironment Promotes Immune Escape of Tumor Cells
Gain-of-function mutations of SHP2 in tumor microenvironment cells affect tumor progression by non-autonomous mechanisms. SHP2 regulates immune cell functions in the tumor immune microenvironment to affect tumor progressions (Liu et al., 2020; Figure 4). For example, SHP2 regulates the function of T cells by binding to programmed cell death 1 (PD-1) (Liu et al., 2020). PD-1, a key immune checkpoint target for cancer immunotherapy and negative costimulatory receptor, is important to inhibit T cell activation. PD-1 binds to ligand PD-L1 and clusters with T cell receptor (TCR), which is temporarily related to phosphatase SHP2. These negative costimulatory clusters induce dephosphorylation of TCR signal molecules and inhibit the activation of T cells to block TCR induced stop signal (Yokosuka et al., 2012). Dimeric PD-1 activates SHP2-mediated immunosuppression by binding to SH2 domains of SHP2 (N-SH2 and C-SH2) via the C-terminal tyrosine-based switch motif (ITSM) of immune receptor (Okazaki et al., 2001; Sheppard et al., 2004; Yokosuka et al., 2012), thus promoting the immune escape of tumor cells. Other studies reported that cytotoxic T lymphocyte-associated antigen 4 (CTLA-4) is also an immune checkpoint and a negative regulator of T cell immune function (Buchbinder and Desai, 2016; Rowshanravan et al., 2018). Phosphorylation of YYKM motif in CTLA-4 cytoplasmic tail recruits SHP2 to dephosphorylate and inactivate CD28 (Salmond and Alexander, 2006; Lorenz, 2009; Rudd et al., 2009) and to promote the tumor cell survival. Furthermore, SHP2 regulates another signaling to impair the antitumor immunotherapy. SHP2 in the cytoplasm dephosphorylates STAT1, which ultimately inhibits the proliferation of T lymphocytes, leads to a decline in antitumor immunity, and promotes the development of cancer (Liu et al., 2020). Li et al. (2015) analyzed tumor-infiltrating and peripheral blood lymphocytes in head and neck squamous cell carcinoma patients and concluded on the inhibitory effect of SHP2-mediated PD-1 on tumor Th1 cell immunity and that the PD-1 or SHP2 blockade was sufficient to restore Th1 immune activity and to activate T cells, thus reversing immunosuppression in tumor microenvironment.
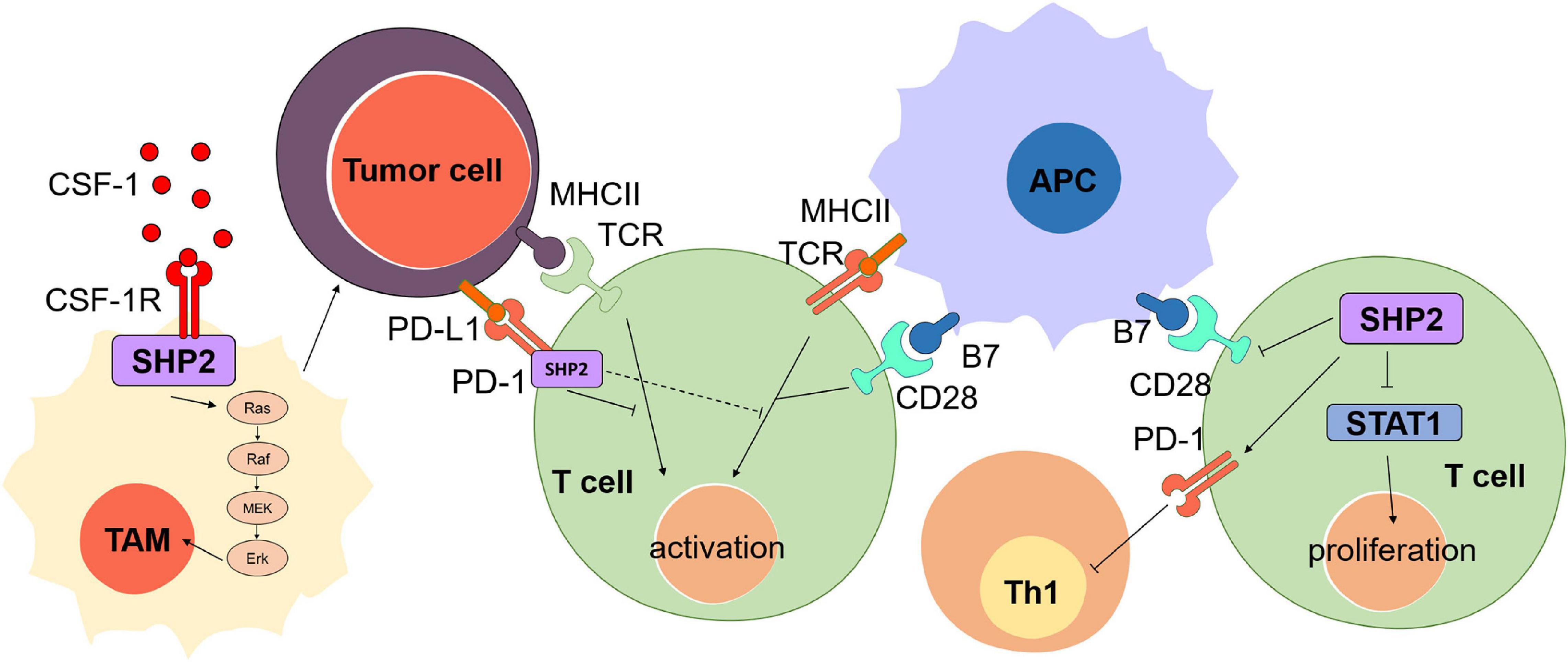
Figure 4. The oncogenic role of SHP2 in tumor immune microenvironment. SHP2 binds to the T cell receptor (TCR) and prevents the activation of T cell induced by the signaling transduction among tumor cells, antigen-presenting cells (APCs), and T cells. SHP2 binds to the colony-stimulating factor-1 receptor (CSF-1R) under the stimulation of CSF-1 and then consequently activates Ras/Raf/MEK/Erk signaling and promotes the proliferation of tumor-associated macrophage (TAM), thus boosting the cancer cell survival. SHP2 in T cells inhibits the activation of T cell via blockage of the function of CD28 and STAT1.
SHP2 is involved in multiple signaling pathways in tumor-associated macrophages. Stimulated by the colony-stimulating factor-1 (CSF-1), SHP2 binds to the CSF receptor (CSF-1R) complex on the inner membrane of tumor-associated macrophage (TAM), leading to the activation of the Ras/Erk signaling pathway in TAM and supporting the survival, proliferation, and migration of tumor cells (Achkova and Maher, 2016). Furthermore, SHP2 in the macrophages is associated with chronic inflammation-related cancers. Recently, Barkal et al. (2019) have shown that tumor-expressed CD24 binds macrophage sialic-acid-binding Ig-like lectin 10 (Siglec-10) to promote tumor avoidance in the tumor microenvironment by recruiting SHP2 to the cytoplasmic tail ITIM motif of Siglec-10. Besides, Xiao et al. (2019) found that SHP2 deficiency in macrophages disrupts the IL-10/STAT3 signaling pathway, worsening the colons of mice.
Gain-of-Function SHP2 Promotes Chemoresistance in Cell-Autonomous and/or Non-autonomous Mechanisms
SHP2 promotes chemoresistance in cell-autonomous and/or non-autonomous mechanisms. A previous study observed high expression of SHP2 in both chemoresistant hepatocellular carcinomas (HCCs) and recurrent HCCs derived from patients (Xiang et al., 2017), suggesting a relationship between aberrant SHP2 and chemoresistance. In fact, numerous studies reported that tumor cell-autonomous SHP2 participates in multiple signaling that promotes chemoresistance. For example, SHP2 mutations in tumor cells induce Erk inhibitor resistance through feedback activation of receptor tyrosine kinase (RTK) signaling and rebounding of Erk activity in Erk-driven tumors (Ahmed et al., 2019). SHP2 activates several different tyrosine kinases to drive anaplastic lymphoma kinase (ALK) inhibitor resistance during chemotherapy of ALK-rearranged NSCLCs (Dardaei et al., 2018). SHP2 mediates cisplatin resistance by inhibiting apoptosis and activating the Ras/PI3K/Akt/survivin pathway in lung cancer cells (Tang et al., 2018). Other studies demonstrated that SHP2 activation mutation confers resistance to imatinib in drug-tolerant chronic myeloid leukemia cells. The blockage of Raf/MEK/Erk and PI3K/Akt/mTOR pathways via SHP2 inhibition leads to apoptosis of drug-resistant cells (Li et al., 2018). In PTEN-null senescent tumors, there is a downregulation of SHP2 and activation of JAK/STAT3 pathway, which contributes to the establishment of an immunosuppressive tumor microenvironment that promotes chemoresistance (Toso et al., 2014). SHP2 also influences cancer resistance through other mechanisms. SHP2E76K activation mutation in bone marrow mesenchymal stromal cells (BMSCs) upregulates vascular cell adhesion molecule 1 (VCAM-1) expression by increasing the PI3K/Akt phosphorylation level and further induces BMSC-mediated chemoresistance in B-cell acute lymphoblastic leukemia (B-ALL) (Yu et al., 2020). This is a typical instance of SHP2 promoting drug resistance in non-autonomous mechanism. In general, these findings illuminate a pivotal oncogenic function of SHP2 in cancers; thus, pharmacological inhibition of SHP2 is a valid therapeutic approach for the treatment of cancers.
SHP2 Inhibition Is a Promising Antitumor Strategy
Small-Molecule Inhibitors of SHP2
SHP2 is a potential target for cancer therapy. At present, several SHP2 small-molecule inhibitors are available (Table 2). One study reported an allosteric small-molecule SHP2 inhibitor SHP099, which binds to a tunnel-like pocket formed by the confluence of three domains of SHP2 to stabilize its self-inhibiting conformation, while it has no significant activity against other PTP families (including SHP1) and kinases (Fodor et al., 2018). In addition, SHP099 inhibits the proliferation of RTK-driven human cancer cells by inhibiting Ras/Erk signaling, so drug inhibition of SHP2 is one of the effective strategies for cancer treatment (Chen et al., 2016). Meanwhile, Chen et al. identified a weak SHP2 inhibitor SHP244. X-ray crystallography shows that SHP244 binds to SHP2 and stabilizes the inactive closed conformation of SHP2 by forming cracks at the N-terminal interface between SH2 and PTP (Fodor et al., 2018). In addition, it is possible that the allosteric sites are occupied by SHP099 and SHP244 at the same time, and the combination of SHP099 and SHP244 enhances the pharmacological inhibition of cells (Fodor et al., 2018). A recent study demonstrated that mutated SHP2-mediated LLPS formation is inhibited by SHP2 allosteric inhibitors, which prevent SHP2 from releasing the self-inhibition status. Therefore, the application of SHP2 inhibitors is a promising therapeutic strategy to treat SHP2-involved developmental disorders and tumors (Zhu et al., 2020).
Recently, Bagdanoff et al. (2019) identified SHP2 inhibitor SHP389, which regulates MAPK signal in vivo. Another study improved the basis of the allosteric inhibitors described previously and identified a new effective oral SHP2 inhibitor, SHP394, which shows high lipid efficiency, improved efficacy, and enhanced pharmacokinetics (Sarver et al., 2019). Nichols et al. (2018) showed that MRC-4550 affects human tumor models. MRC-4550 treatment reduces Ras/Raf/MEK/Erk signal transduction and cancer growth. RMC-4630 is an oral and effective allotropic selective inhibitor of SHP2 in the Ras signaling pathway. This inhibitor is currently in clinical trials and is being evaluated in a multi-cohort phase 1/2 clinical program (Moore et al., 2020). An effective SHP2 variable structure allosteric inhibitor PCC0208023 was synthesized recently. It non-competitively inhibits the activity of SHP2. In addition, PCC0208023 inhibits the proliferation of human CAC cells driven by KRAS mutation by inhibiting Ras/MAPK signaling pathway in vitro. It also shows an antitumor effect on KRAS-driven xenograft model (Chen et al., 2020). Other studies have identified NSC-87877, which binds to the catalytic cracking of SHP2 PTP (Chen et al., 2006). Additionally, PHPS1 is an effective cell permeation inhibitor, which inhibits SHP2-dependent cell processes, such as hepatocyte growth factor/dispersant factor (HGF/SF)-induced epithelial cell scattering and branching. PHPS1 also blocks the SHP2-associated downstream signaling pathway, such that it inhibits the SHP2E76K-mediated activation of Erk1/2 to prevent the growth of a variety of human tumor cell lines (Hellmuth et al., 2008). Wang et al. found that cefsulodin blocks SHP2-mediated signaling transduction and proliferation of several cancer cell lines (He et al., 2015).
Small-Molecule Inhibition of Cell-Autonomous SHP2 to Prevent Chemoresistance
SHP2 is a potential therapeutic target for cancer treatment, as it plays a significant role in promoting chemoresistance. Chemotherapy is one of the most commonly used methods in the clinical diagnosis and treatment of malignant tumors. It is an effective means of systemic treatment for not only the tumors at the treatment site but also the clinical metastasis tumors at the potential lesions (Arbour and Riely, 2019). However, the resistance of tumor cells to chemotherapy drugs often impairs its efficacy and finally leads to failure, which has become a huge challenge for cancer treatment (Vasan et al., 2019). Increasing evidences have shown that mutated SHP2 plays an important role in chemoresistance (Ruess et al., 2018). At present, multiple allosteric inhibitors of SHP2 are discovered (Chen et al., 2016), and traditional chemotherapeutic drugs combined with SHP2 inhibition have become a potential approach to enhance efficacy of chemotherapy and immunotherapy.
Some studies reported that the combination of SHP2 inhibitors with other drugs shows promising application prospects (Figure 5). SHP099 exhibited antitumor activity either as a single agent or in combination with temozolomide (TMZ) and provided significant survival benefits for GBM tumor xenograft-bearing animals (Sang et al., 2019). MEK inhibitors show limited efficacy, because of the rapid development of adaptive resistance, whereas SHP2 inhibitor SHP099 combined with MEK inhibition prevents adaptive resistance in multiple KRAS-driven malignancies (Fedele et al., 2018). Pharmacologically targeting Erk signaling in Erk-dependent tumors is also limited by adaptive resistance, due to the feedback activation of RTK signaling, which is mediated by SHP2. Thus, targeting Erk signaling and SHP2 prevents such resistance in Erk-dependent tumors (Ahmed et al., 2019). Most ALK-rearranged NSCLCs initially respond to small-molecule ALK inhibitors, but drug resistance often develops. Researchers identified SHP2 as a common targetable resistance node in multiple ALK inhibitor-resistance patient-derived cells (PDCs), and treatment with SHP099 in combination with the ALK TKI ceritinib blocked the growth of resistant PDCs by preventing compensatory Ras and Erk reactivation (Dardaei et al., 2018). The survival benefit of sorafenib for patients with HCC is unsatisfactory due to the development of adaptive resistance, and SHP2 was observably upregulated in sorafenib-resistant HCC cell lines as well as patient-derived xenografts (Leung et al., 2019). SHP2 inhibition by SHP099 in combination with sorafenib attenuated the adaptive resistance to sorafenib by impeding RTK-induced reactivation of the MEK/Erk and Akt signaling pathways. Dioscin inhibits MEK/Erk and PI3K/Akt signaling pathways to abrogate TKI resistance through dysregulation of SHP2 expression in lung adenocarcinoma (Wang Y.C. et al., 2018). Thus, the combination of dioscin and TKI is potentially therapeutic for chemoresistant tumor treatment.
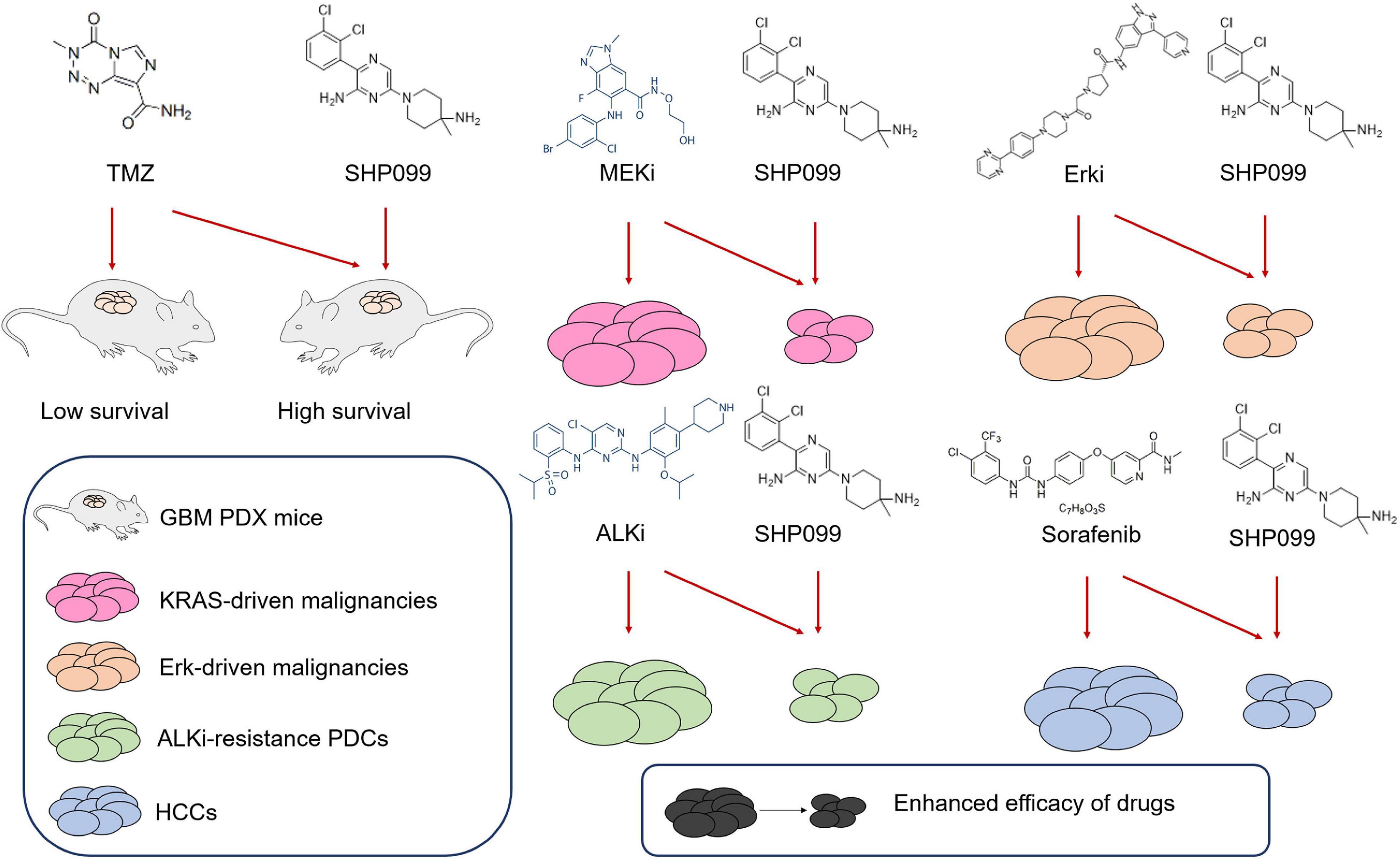
Figure 5. Combination of SHP099 with other drugs enhances antitumor efficacy. In patient-derived xenograft (PDX) mouse model, temozolomide (TMZ) combines with SHP099 to increase the survival rate of tumor-carrying mice. The combination of SHP099 with MEK inhibitor, Erk inhibitor, ALK inhibitor, or sorafenib impairs the survival of malignancies, which indicates that the drug toxicity is enhanced through the drug combination.
SHP2 Inhibition Is a Potential Strategy for Immunotherapy
SHP2 inhibition is a potential strategy for enhancing the efficacy of antitumor immunotherapy. Pharmacological inhibition of SHP2 through SHP099 combined with PD-1 antibody is a valid therapeutic approach for the treatment of cancers through enhancing the efficacy of antitumor immunity (Zhao M. et al., 2019). Some preclinical findings revealed that SHP2 promotes immune suppression in the tumor microenvironment; thus, the allosteric inhibition of SHP2 by RMC-4550 could induce antitumor immunity (Quintana et al., 2020). Other studies demonstrated that the co-inhibition of CSF1-R and SHP2 using nanoparticles loaded with inhibitors for tumor TAM activation and enhancement of phagocytosis is an effective strategy for macrophage-based antitumor immunotherapy (Ramesh et al., 2019). A recent study reported that allosteric inhibition of SHP2 leads to direct and selective depletion of pro-tumorigenic M2 macrophages and promotes antitumor immunity, suggesting a therapeutic approach for Ras-driven cancers (Quintana et al., 2020).
Conclusion and Discussion
SHP2 serves as a pivotal hub to connect multiple oncogenic signaling pathways, such as PI3K/Akt, Ras/Raf/MAPK, and PD-1/PD-L1 pathways. It promotes tumor progression via cell-autonomous and non-cell-autonomous mechanisms. That is, on the one hand, activation mutations of SHP2 in specific cells directly establish tumorigenic phenotype to promote the tumor progressions; on the other hand, SHP2 mutations in the tumor microenvironment promote tumor development. Oncogenic SHP2 is regarded as a potential cancer treatment target. Recently, multiple types of SHP2 inhibitors have been discovered to enhance cancer treatments.
At present, there are several techniques to study gene functions and explore new antitumor targets. Except for the Cre/LoxP system, clustered regularly interspaced short palindromic repeats (CRISPR)/Cas9-based genome-wide screening is widely applied to study the gene functions and to discover novel targets for treatment. CRISPR/Cas9 is a gene-editing tool for operating specific genes in the genome, which was first found as part of the adaptive immune system in bacteria. In recent years, CRISPR/Cas9 has been widely applied in altering genomes to activate or to repress the expression of genes; thus, its application accelerates the study of the mechanism of tumorigenesis and the development of cancer therapy (Lee et al., 2019). A recent study demonstrated that genome-scale CRISPR/Cas9 gene-knockout screening is applied in discovering potential therapeutic antitumor targets in the cancer cells’ genomes and identified the important role of PTPN11 in pediatric rhabdoid tumors (Oberlick et al., 2019). Genome-scale CRISPR/Cas9 gene-knockout screening can also be applied to study protein function and to explore new therapeutic targets. Recent studies applied the CRISPR/Cas9 system to gene therapy. AAV-CRISPR/Cas9-mediated gene editing corrects Ldlr mutation in vivo and effectively ameliorates atherosclerosis phenotypes, which is a potential therapeutic approach for patients with familial hypercholesterolemia (Zhao et al., 2020). Since transfusing the PD-1 knockout T-cells to patients with solid tumor induces immunological responses against tumor cells, the CRISPR/Cas9 system is regarded as therapeutic tool (Zhan et al., 2019). With the development of drug delivery systems (Chen et al., 2017; Wang P. et al., 2018), whether CRISPR/Cas9 could be applied to edit SHP2 mutation for enhancement of cancer therapy is worth exploring.
Other targeting SHP2 degradation techniques are also potential adjuvant approaches for enhancement of cancer therapeutic efficacy, including proteolysis-targeting chimera (PROTAC). PROTAC is designed to allosterically target specific proteins and recruit the E3 ligase Von Hippel-Lindau (VHL), resulting in ubiquitination and subsequent degradation of the target protein (Burslem et al., 2019). This technique is widely applied to drug development and research on mechanisms of chemoresistance. To date, about 50 proteins, including clinically validated drug targets, are targeted by PROTAC for degradation, and these PROTACs have been successfully developed in clinical trials for cancer therapy (Li and Song, 2020). For example, PROTAC-induced bromodomain and extra-terminal (BET) protein degradation showed anti-prostate cancer efficacy (Raina et al., 2016). A recent study demonstrated that induced SHP2 degradation through PROTAC is an effective approach to inhibit the function of SHP2, and it further pointed out that optimization of these SHP2 degraders may lead to the development of a new class of therapies for cancers and other human diseases (Wang et al., 2020).
Recently, hyperactivation of SHP2 through the formation of LLPS has been elucidated (Zhu et al., 2020). Both GOF and LOF disease-associated SHP2 variants promote LLPS to increase the catalytic activity of mutant and wild-type SHP2, leading to MAPK hyperactivation. SHP2 is an important signal hub in normal conditions, and its hyperactivation will undoubtedly lead to the breakdown of cell signal balance. Therefore, in developmental diseases, especially tumors, LLPS possibly acts as an important driver for disease occurrence and chemotherapeutic resistance. As it has been found that allosteric inhibitors of SHP2 have an inhibitory effect on the formation of LLPS, it is very promising to develop new therapies based on SHP2 inhibition and LLPS blockage.
Author Contributions
LD and DH drafted the manuscript and designed the structure. QX, MX, and XM proposed useful comments, suggestions, and revised the manuscript. CZ revised the language of the manuscript. All authors contributed to the article and approved the submitted version.
Funding
This work was supported by grants from the National Natural Science Foundation of China (81870123), China Postdoctoral Science Foundation Grant (2018M641206), and the National Science Foundation for Young Scientists of China (81902545).
Conflict of Interest
The authors declare that the research was conducted in the absence of any commercial or financial relationships that could be construed as a potential conflict of interest.
Acknowledgments
We thank the Biological and Medical Engineering Core Facilities, School of Life Sciences, Beijing Institute of Technology, for providing experimental study assistance.
References
Achkova, D., and Maher, J. (2016). Role of the colony-stimulating factor (CSF)/CSF-1 receptor axis in cancer. Biochem. Soc. Trans. 44, 333–341. doi: 10.1042/bst20150245
Ahmed, T. A., Adamopoulos, C., Karoulia, Z., Wu, X., Sachidanandam, R., Aaronson, S. A., et al. (2019). SHP2 drives adaptive resistance to ERK signaling inhibition in molecularly defined subsets of ERK-dependent tumors. Cell Rep. 26, 65–78.e65. doi: 10.1016/j.celrep.2018.12.013
Arbour, K. C., and Riely, G. J. (2019). Systemic therapy for locally advanced and metastatic non-small cell lung cancer: a review. JAMA 322, 764–774. doi: 10.1001/jama.2019.11058
Bagdanoff, J. T., Chen, Z., Acker, M., Chen, Y. N., Chan, H., Dore, M., et al. (2019). Optimization of fused bicyclic allosteric SHP2 inhibitors. J. Med. Chem. 62, 1781–1792. doi: 10.1021/acs.jmedchem.8b01725
Barkal, A. A., Brewer, R. E., Markovic, M., Kowarsky, M., Barkal, S. A., Zaro, B. W., et al. (2019). CD24 signalling through macrophage Siglec-10 is a target for cancer immunotherapy. Nature 572, 392–396. doi: 10.1038/s41586-019-1456-0
Bellio, M., Garcia, C., Edouard, T., Voisin, S., Neel, B. G., Cabou, C., et al. (2019). Catalytic dysregulation of SHP2 leading to Noonan syndromes affects platelet signaling and functions. Blood 134, 2304–2317. doi: 10.1182/blood.2019001543
Bernards, R. (2012). A missing link in genotype-directed cancer therapy. Cell 151, 465–468. doi: 10.1016/j.cell.2012.10.014
Bondeson, M. L. (2017). Key insights into the protein tyrosine phosphatase PTPN11/SHP2 associated with noonan syndrome and cancer. Hum. Mutat. 38:337. doi: 10.1002/humu.23084
Buchbinder, E. I., and Desai, A. (2016). CTLA-4 and PD-1 pathways: similarities, differences, and implications of their inhibition. Am. J. Clin. Oncol. 39, 98–106. doi: 10.1097/coc.0000000000000239
Bunda, S., Burrell, K., Heir, P., Zeng, L., Alamsahebpour, A., Kano, Y., et al. (2015). Inhibition of SHP2-mediated dephosphorylation of Ras suppresses oncogenesis. Nat. Commun. 6:8859. doi: 10.1038/ncomms9859
Burslem, G. M., Schultz, A. R., Bondeson, D. P., Eide, C. A., Savage Stevens, S. L., Druker, B. J., et al. (2019). Targeting BCR-ABL1 in chronic myeloid leukemia by PROTAC-mediated targeted protein degradation. Cancer Res. 79, 4744–4753. doi: 10.1158/0008-5472.Can-19-1236
Cao, M., Gao, D., Zhang, N., Duan, Y., Wang, Y., Mujtaba, H., et al. (2019). Shp2 expression is upregulated in cervical cancer, and Shp2 contributes to cell growth and migration and reduces sensitivity to cisplatin in cervical cancer cells. Pathol. Res. Pract. 215:152621. doi: 10.1016/j.prp.2019.152621
Chan, G., Kalaitzidis, D., and Neel, B. G. (2008). The tyrosine phosphatase Shp2 (PTPN11) in cancer. Cancer Metastasis Rev. 27, 179–192. doi: 10.1007/s10555-008-9126-y
Chan, G., Kalaitzidis, D., Usenko, T., Kutok, J. L., Yang, W., Mohi, M. G., et al. (2009). Leukemogenic Ptpn11 causes fatal myeloproliferative disorder via cell-autonomous effects on multiple stages of hematopoiesis. Blood 113, 4414–4424. doi: 10.1182/blood-2008-10-182626
Chen, J., Cao, Z., and Guan, J. (2018). SHP2 inhibitor PHPS1 protects against atherosclerosis by inhibiting smooth muscle cell proliferation. BMC Cardiovasc. Disord. 18:72. doi: 10.1186/s12872-018-0816-2
Chen, L., Sung, S. S., Yip, M. L., Lawrence, H. R., Ren, Y., Guida, W. C., et al. (2006). Discovery of a novel shp2 protein tyrosine phosphatase inhibitor. Mol. Pharmacol. 70, 562–570. doi: 10.1124/mol.106.025536
Chen, X., Zou, F., Hu, Z., Du, G., Yu, P., Wang, W., et al. (2020). PCC0208023, a potent SHP2 allosteric inhibitor, imparts an antitumor effect against KRAS mutant colorectal cancer. Toxicol. Appl. Pharmacol. 398:115019. doi: 10.1016/j.taap.2020.115019
Chen, Y. N., LaMarche, M. J., Chan, H. M., Fekkes, P., Garcia-Fortanet, J., Acker, M. G., et al. (2016). Allosteric inhibition of SHP2 phosphatase inhibits cancers driven by receptor tyrosine kinases. Nature 535, 148–152. doi: 10.1038/nature18621
Chen, Z., Liu, F., Chen, Y., Liu, J., Wang, X., Chen, A. T., et al. (2017). Targeted delivery of CRISPR/Cas9-mediated cancer gene therapy via liposome-templated hydrogel nanoparticles. Adv. Funct. Mater. 27:1703036. doi: 10.1002/adfm.201703036
Dardaei, L., Wang, H. Q., Singh, M., Fordjour, P., Shaw, K. X., Yoda, S., et al. (2018). SHP2 inhibition restores sensitivity in ALK-rearranged non-small-cell lung cancer resistant to ALK inhibitors. Nat. Med. 24, 512–517. doi: 10.1038/nm.4497
De Rocca Serra-Nédélec, A., Edouard, T., Tréguer, K., Tajan, M., Araki, T., Dance, M., et al. (2012). Noonan syndrome-causing SHP2 mutants inhibit insulin-like growth factor 1 release via growth hormone-induced ERK hyperactivation, which contributes to short stature. Proc. Natl. Acad. Sci. U.S.A. 109, 4257–4262. doi: 10.1073/pnas.1119803109
Ding, L., Saunders, T. L., Enikolopov, G., and Morrison, S. J. (2012). Endothelial and perivascular cells maintain haematopoietic stem cells. Nature 481, 457–462. doi: 10.1038/nature10783
Dong, L., Yu, W. M., Zheng, H., Loh, M. L., Bunting, S. T., Pauly, M., et al. (2016). Leukaemogenic effects of Ptpn11 activating mutations in the stem cell microenvironment. Nature 539, 304–308. doi: 10.1038/nature20131
Fedele, C., Ran, H., Diskin, B., Wei, W., Jen, J., Geer, M. J., et al. (2018). SHP2 inhibition prevents adaptive resistance to MEK inhibitors in multiple cancer models. Cancer Discov. 8, 1237–1249. doi: 10.1158/2159-8290.Cd-18-0444
Feng, G. S., Hui, C. C., and Pawson, T. (1993). SH2-containing phosphotyrosine phosphatase as a target of protein-tyrosine kinases. Science 259, 1607–1611. doi: 10.1126/science.8096088
Fodor, M., Price, E., Wang, P., Lu, H., Argintaru, A., Chen, Z., et al. (2018). Dual Allosteric Inhibition of SHP2 Phosphatase. ACS Chem. Biol. 13, 647–656. doi: 10.1021/acschembio.7b00980
Garcia Fortanet, J., Chen, C. H., Chen, Y. N., Chen, Z., Deng, Z., Firestone, B., et al. (2016). Allosteric inhibition of SHP2: identification of a potent, selective, and orally efficacious phosphatase inhibitor. J. Med. Chem. 59, 7773–7782. doi: 10.1021/acs.jmedchem.6b00680
Greenbaum, A., Hsu, Y. M., Day, R. B., Schuettpelz, L. G., Christopher, M. J., Borgerding, J. N., et al. (2013). CXCL12 in early mesenchymal progenitors is required for haematopoietic stem-cell maintenance. Nature 495, 227–230. doi: 10.1038/nature11926
Grossmann, K. S., Rosário, M., Birchmeier, C., and Birchmeier, W. (2010). The tyrosine phosphatase Shp2 in development and cancer. Adv. Cancer Res. 106, 53–89. doi: 10.1016/s0065-230x(10)06002-1
Guo, W., Liu, W., Chen, Z., Gu, Y., Peng, S., Shen, L., et al. (2017). Tyrosine phosphatase SHP2 negatively regulates NLRP3 inflammasome activation via ANT1-dependent mitochondrial homeostasis. Nat. Commun. 8:2168. doi: 10.1038/s41467-017-02351-0
He, R., Yu, Z. H., Zhang, R. Y., Wu, L., Gunawan, A. M., Lane, B. S., et al. (2015). Exploring the existing drug space for Novel pTyr mimetic and SHP2 inhibitors. ACS Med. Chem. Lett. 6, 782–786. doi: 10.1021/acsmedchemlett.5b00118
Hellmuth, K., Grosskopf, S., Lum, C. T., Würtele, M., Röder, N., von Kries, J. P., et al. (2008). Specific inhibitors of the protein tyrosine phosphatase Shp2 identified by high-throughput docking. Proc. Natl. Acad. Sci. U.S.A. 105, 7275–7280. doi: 10.1073/pnas.0710468105
Hu, Z., Fang, H., Wang, X., Chen, D., Chen, Z., and Wang, S. (2014). Overexpression of SHP2 tyrosine phosphatase promotes the tumorigenesis of breast carcinoma. Oncol. Rep. 32, 205–212. doi: 10.3892/or.2014.3201
Hu, Z., Li, J., Gao, Q., Wei, S., and Yang, B. (2017). SHP2 overexpression enhances the invasion and metastasis of ovarian cancer in vitro and in vivo. Onco Targets Ther. 10, 3881–3891. doi: 10.2147/ott.S138833
Hu, Z., Wang, X., Fang, H., Liu, Y., Chen, D., Zhang, Q., et al. (2016). A tyrosine phosphatase SHP2 gain-of-function mutation enhances malignancy of breast carcinoma. Oncotarget 7, 5664–5676. doi: 10.18632/oncotarget.6561
Ishida, H., Kogaki, S., Narita, J., Ichimori, H., Nawa, N., Okada, Y., et al. (2011). LEOPARD-type SHP2 mutant Gln510Glu attenuates cardiomyocyte differentiation and promotes cardiac hypertrophy via dysregulation of Akt/GSK-3β/β-catenin signaling. Am. J. Physiol. Heart Circ. Physiol. 301, H1531–H1539. doi: 10.1152/ajpheart.00216.2011
Jiang, L., Xu, W., Chen, Y., and Zhang, Y. (2019). SHP2 inhibitor specifically suppresses the stemness of KRAS-mutant non-small cell lung cancer cells. Artif. Cells Nanomed. Biotechnol. 47, 3231–3238. doi: 10.1080/21691401.2019.1646748
LaRochelle, J. R., Fodor, M., Vemulapalli, V., Mohseni, M., Wang, P., Stams, T., et al. (2018). Structural reorganization of SHP2 by oncogenic mutations and implications for oncoprotein resistance to allosteric inhibition. Nat. Commun. 9:4508. doi: 10.1038/s41467-018-06823-9
Lee, Y. R., Chen, M., Lee, J. D., Zhang, J., Lin, S. Y., Fu, T. M., et al. (2019). Reactivation of PTEN tumor suppressor for cancer treatment through inhibition of a MYC-WWP1 inhibitory pathway. Science 364:eaau0159. doi: 10.1126/science.aau0159
Leung, C. O. N., Tong, M., Chung, K. P. S., Zhou, L., Che, N., Tang, K. H., et al. (2019). Overriding adaptive resistance to sorafenib through combination therapy with Src homology 2 domain-containing phosphatase 2 blockade in hepatocellular carcinoma. Hepatology 72, 155–168. doi: 10.1002/hep.30989
Li, J., Jie, H. B., Lei, Y., Gildener-Leapman, N., Trivedi, S., Green, T., et al. (2015). PD-1/SHP-2 inhibits Tc1/Th1 phenotypic responses and the activation of T cells in the tumor microenvironment. Cancer Res. 75, 508–518. doi: 10.1158/0008-5472.Can-14-1215
Li, X., Pang, J., Xue, W., Wang, Y., Tian, T., Elgehama, A., et al. (2018). Inducible SHP-2 activation confers resistance to imatinib in drug-tolerant chronic myeloid leukemia cells. Toxicol. Appl. Pharmacol. 360, 249–256. doi: 10.1016/j.taap.2018.09.044
Li, X., and Song, Y. (2020). Proteolysis-targeting chimera (PROTAC) for targeted protein degradation and cancer therapy. J. Hematol. Oncol. 13:50. doi: 10.1186/s13045-020-00885-3
Lipka, D. B., Witte, T., Toth, R., Yang, J., Wiesenfarth, M., Nöllke, P., et al. (2017). RAS-pathway mutation patterns define epigenetic subclasses in juvenile myelomonocytic leukemia. Nat. Commun. 8:2126. doi: 10.1038/s41467-017-02177-w
Liu, Q., Qu, J., Zhao, M., Xu, Q., and Sun, Y. (2020). Targeting SHP2 as a promising strategy for cancer immunotherapy. Pharmacol. Res. 152:104595. doi: 10.1016/j.phrs.2019.104595
Liu, X., Zheng, H., Li, X., Wang, S., Meyerson, H. J., Yang, W., et al. (2016). Gain-of-function mutations of Ptpn11 (Shp2) cause aberrant mitosis and increase susceptibility to DNA damage-induced malignancies. Proc. Natl. Acad. Sci. U.S.A. 113, 984–989. doi: 10.1073/pnas.1508535113
Lorenz, U. (2009). SHP-1 and SHP-2 in T cells: two phosphatases functioning at many levels. Immunol. Rev. 228, 342–359. doi: 10.1111/j.1600-065X.2008.00760.x
Mainardi, S., Mulero-Sánchez, A., Prahallad, A., Germano, G., Bosma, A., Krimpenfort, P., et al. (2018). SHP2 is required for growth of KRAS-mutant non-small-cell lung cancer in vivo. Nat. Med. 24, 961–967. doi: 10.1038/s41591-018-0023-9
Méndez-Ferrer, S., Michurina, T. V., Ferraro, F., Mazloom, A. R., Macarthur, B. D., Lira, S. A., et al. (2010). Mesenchymal and haematopoietic stem cells form a unique bone marrow niche. Nature 466, 829–834. doi: 10.1038/nature09262
Miah, S. M. S., Jayasuriya, C. T., Salter, A. I., Reilly, E. C., Fugere, C., Yang, W., et al. (2017). Ptpn11 deletion in CD4(+) cells does not affect T cell development and functions but causes cartilage tumors in a T cell-independent manner. Front. Immunol. 8:1326. doi: 10.3389/fimmu.2017.01326
Moore, A. R., Rosenberg, S. C., McCormick, F., and Malek, S. (2020). RAS-targeted therapies: is the undruggable drugged? Nat. Rev. Drug Discov. 19, 533–552. doi: 10.1038/s41573-020-0068-6
Nichols, R. J., Haderk, F., Stahlhut, C., Schulze, C. J., Hemmati, G., Wildes, D., et al. (2018). RAS nucleotide cycling underlies the SHP2 phosphatase dependence of mutant BRAF-, NF1- and RAS-driven cancers. Nat. Cell Biol. 20, 1064–1073. doi: 10.1038/s41556-018-0169-1
Niemeyer, C. M. (2018). JMML genomics and decisions. Hematol. Am. Soc. Hematol. Educ. Program 2018, 307–312. doi: 10.1182/asheducation-2018.1.307
Noda, S., Takahashi, A., Hayashi, T., Tanuma, S., and Hatakeyama, M. (2016). Determination of the catalytic activity of LEOPARD syndrome-associated SHP2 mutants toward parafibromin, a bona fide SHP2 substrate involved in Wnt signaling. Biochem. Biophys. Res. Commun. 469, 1133–1139. doi: 10.1016/j.bbrc.2015.12.117
Oberlick, E. M., Rees, M. G., Seashore-Ludlow, B., Vazquez, F., Nelson, G. M., Dharia, N. V., et al. (2019). Small-molecule and CRISPR screening converge to reveal receptor tyrosine kinase dependencies in pediatric rhabdoid tumors. Cell Rep 28, 2331–2344.e2338. doi: 10.1016/j.celrep.2019.07.021
Okazaki, T., Maeda, A., Nishimura, H., Kurosaki, T., and Honjo, T. (2001). PD-1 immunoreceptor inhibits B cell receptor-mediated signaling by recruiting src homology 2-domain-containing tyrosine phosphatase 2 to phosphotyrosine. Proc. Natl. Acad. Sci. U.S.A. 98, 13866–13871. doi: 10.1073/pnas.231486598
Pandey, R., Saxena, M., and Kapur, R. (2017). Role of SHP2 in hematopoiesis and leukemogenesis. Curr. Opin. Hematol. 24, 307–313. doi: 10.1097/moh.0000000000000345
Pannone, L., Bocchinfuso, G., Flex, E., Rossi, C., Baldassarre, G., Lissewski, C., et al. (2017). Structural, functional, and clinical characterization of a novel PTPN11 mutation cluster underlying noonan syndrome. Hum. Mutat. 38, 451–459. doi: 10.1002/humu.23175
Pierpont, M. E., and Digilio, M. C. (2018). Cardiovascular disease in Noonan syndrome. Curr. Opin. Pediatr. 30, 601–608. doi: 10.1097/mop.0000000000000669
Quintana, E., Schulze, C. J., Myers, D. R., Choy, T. J., Mordec, K., Wildes, D., et al. (2020). Allosteric inhibition of SHP2 stimulates anti-tumor immunity by transforming the immunosuppressive environment. Cancer Res. 80, 2889–2902. doi: 10.1158/0008-5472.Can-19-3038
Raina, K., Lu, J., Qian, Y., Altieri, M., Gordon, D., Rossi, A. M., et al. (2016). PROTAC-induced BET protein degradation as a therapy for castration-resistant prostate cancer. Proc. Natl. Acad. Sci. U.S.A. 113, 7124–7129. doi: 10.1073/pnas.1521738113
Ramesh, A., Kumar, S., Nandi, D., and Kulkarni, A. (2019). CSF1R- and SHP2-inhibitor-loaded nanoparticles enhance cytotoxic activity and phagocytosis in tumor-associated macrophages. Adv. Mater. 31:e1904364. doi: 10.1002/adma.201904364
Rehman, A. U., Rafiq, H., Rahman, M. U., Li, J., Liu, H., Luo, S., et al. (2019). Gain-of-function SHP2 E76Q mutant rescuing autoinhibition mechanism associated with juvenile myelomonocytic leukemia. J. Chem. Inf. Model. 59, 3229–3239. doi: 10.1021/acs.jcim.9b00353
Roberts, A. E., Allanson, J. E., Tartaglia, M., and Gelb, B. D. (2013). Noonan syndrome. Lancet 381, 333–342. doi: 10.1016/s0140-6736(12)61023-x
Roccograndi, L., Binder, Z. A., Zhang, L., Aceto, N., Zhang, Z., Bentires-Alj, M., et al. (2017). SHP2 regulates proliferation and tumorigenicity of glioma stem cells. J. Neurooncol. 135, 487–496. doi: 10.1007/s11060-017-2610-x
Rowshanravan, B., Halliday, N., and Sansom, D. M. (2018). CTLA-4: a moving target in immunotherapy. Blood 131, 58–67. doi: 10.1182/blood-2017-06-741033
Rudd, C. E., Taylor, A., and Schneider, H. (2009). CD28 and CTLA-4 coreceptor expression and signal transduction. Immunol. Rev. 229, 12–26. doi: 10.1111/j.1600-065X.2009.00770.x
Ruess, D. A., Heynen, G. J., Ciecielski, K. J., Ai, J., Berninger, A., Kabacaoglu, D., et al. (2018). Mutant KRAS-driven cancers depend on PTPN11/SHP2 phosphatase. Nat. Med. 24, 954–960. doi: 10.1038/s41591-018-0024-8
Salem, I. H., Plante, S., Gounni, A. S., Rouabhia, M., and Chakir, J. (2018). A shift in the IL-6/STAT3 signalling pathway imbalance towards the SHP2 pathway in severe asthma results in reduced proliferation process. Cell. Signal. 43, 47–54. doi: 10.1016/j.cellsig.2017.12.001
Salmond, R. J., and Alexander, D. R. (2006). SHP2 forecast for the immune system: fog gradually clearing. Trends Immunol. 27, 154–160. doi: 10.1016/j.it.2006.01.007
Sang, Y., Hou, Y., Cheng, R., Zheng, L., Alvarez, A. A., Hu, B., et al. (2019). Targeting PDGFRα-activated glioblastoma through specific inhibition of SHP-2-mediated signaling. Neuro Oncol. 21, 1423–1435. doi: 10.1093/neuonc/noz107
Sarver, P., Acker, M., Bagdanoff, J. T., Chen, Z., Chen, Y. N., Chan, H., et al. (2019). 6-Amino-3-methylpyrimidinones as potent, selective, and orally efficacious SHP2 inhibitors. J. Med. Chem. 62, 1793–1802. doi: 10.1021/acs.jmedchem.8b01726
Schneeberger, V. E., Luetteke, N., Ren, Y., Berns, H., Chen, L., Foroutan, P., et al. (2014). SHP2E76K mutant promotes lung tumorigenesis in transgenic mice. Carcinogenesis 35, 1717–1725. doi: 10.1093/carcin/bgu025
Schramm, C., Fine, D. M., Edwards, M. A., Reeb, A. N., and Krenz, M. (2012). The PTPN11 loss-of-function mutation Q510E-Shp2 causes hypertrophic cardiomyopathy by dysregulating mTOR signaling. Am. J. Physiol. Heart Circ. Physiol. 302, H231–H243. doi: 10.1152/ajpheart.00665.2011
Shen, D., Chen, W., Zhu, J., Wu, G., Shen, R., Xi, M., et al. (2020). Therapeutic potential of targeting SHP2 in human developmental disorders and cancers. Eur. J. Med. Chem. 190:112117. doi: 10.1016/j.ejmech.2020.112117
Sheppard, K. A., Fitz, L. J., Lee, J. M., Benander, C., George, J. A., Wooters, J., et al. (2004). PD-1 inhibits T-cell receptor induced phosphorylation of the ZAP70/CD3zeta signalosome and downstream signaling to PKCtheta. FEBS Lett. 574, 37–41. doi: 10.1016/j.febslet.2004.07.083
Shi, Y., Ma, I. T., Patel, R. H., Shang, X., Chen, Z., Zhao, Y., et al. (2015). NSC-87877 inhibits DUSP26 function in neuroblastoma resulting in p53-mediated apoptosis. Cell Death Dis. 6:e1841. doi: 10.1038/cddis.2015.207
Shigemura, T., Matsuda, K., Kurata, T., Sakashita, K., Okuno, Y., Muramatsu, H., et al. (2019). Essential role of PTPN11 mutation in enhanced haematopoietic differentiation potential of induced pluripotent stem cells of juvenile myelomonocytic leukaemia. Br. J. Haematol. 187, 163–173. doi: 10.1111/bjh.16060
Song, M., Park, J. E., Park, S. G., Lee, D. H., Choi, H. K., Park, B. C., et al. (2009). NSC-87877, inhibitor of SHP-1/2 PTPs, inhibits dual-specificity phosphatase 26 (DUSP26). Biochem. Biophys. Res. Commun. 381, 491–495. doi: 10.1016/j.bbrc.2009.02.069
Stieglitz, E., Taylor-Weiner, A. N., Chang, T. Y., Gelston, L. C., Wang, Y. D., Mazor, T., et al. (2015). The genomic landscape of juvenile myelomonocytic leukemia. Nat. Genet. 47, 1326–1333. doi: 10.1038/ng.3400
Strullu, M., Caye, A., Lachenaud, J., Cassinat, B., Gazal, S., Fenneteau, O., et al. (2014). Juvenile myelomonocytic leukaemia and Noonan syndrome. J. Med. Genet. 51, 689–697. doi: 10.1136/jmedgenet-2014-102611
Tajan, M., de Rocca Serra, A., Valet, P., Edouard, T., and Yart, A. (2015). SHP2 sails from physiology to pathology. Eur. J. Med. Genet. 58, 509–525. doi: 10.1016/j.ejmg.2015.08.005
Tang, C., Luo, H., Luo, D., Yang, H., and Zhou, X. (2018). Src homology phosphotyrosyl phosphatase 2 mediates cisplatin-related drug resistance by inhibiting apoptosis and activating the Ras/PI3K/Akt1/survivin pathway in lung cancer cells. Oncol. Rep. 39, 611–618. doi: 10.3892/or.2017.6109
Tang, Y., Feinberg, T., Keller, E. T., Li, X. Y., and Weiss, S. J. (2016). Snail/Slug binding interactions with YAP/TAZ control skeletal stem cell self-renewal and differentiation. Nat. Cell Biol. 18, 917–929. doi: 10.1038/ncb3394
Tartaglia, M., Martinelli, S., Cazzaniga, G., Cordeddu, V., Iavarone, I., Spinelli, M., et al. (2004). Genetic evidence for lineage-related and differentiation stage-related contribution of somatic PTPN11 mutations to leukemogenesis in childhood acute leukemia. Blood 104, 307–313. doi: 10.1182/blood-2003-11-3876
Toso, A., Revandkar, A., Di Mitri, D., Guccini, I., Proietti, M., Sarti, M., et al. (2014). Enhancing chemotherapy efficacy in Pten-deficient prostate tumors by activating the senescence-associated antitumor immunity. Cell Rep. 9, 75–89. doi: 10.1016/j.celrep.2014.08.044
Vasan, N., Baselga, J., and Hyman, D. M. (2019). A view on drug resistance in cancer. Nature 575, 299–309. doi: 10.1038/s41586-019-1730-1
Voena, C., Conte, C., Ambrogio, C., Boeri Erba, E., Boccalatte, F., Mohammed, S., et al. (2007). The tyrosine phosphatase Shp2 interacts with NPM-ALK and regulates anaplastic lymphoma cell growth and migration. Cancer Res. 67, 4278–4286. doi: 10.1158/0008-5472.Can-06-4350
Wang, M., Lu, J., Wang, M., Yang, C. Y., and Wang, S. (2020). Discovery of SHP2-D26 as a first, potent, and effective PROTAC degrader of SHP2 protein. J. Med. Chem 64, 906–908. doi: 10.1021/acs.jmedchem.0c00471
Wang, P., Zhang, L., Zheng, W., Cong, L., Guo, Z., Xie, Y., et al. (2018). Thermo-triggered release of CRISPR-Cas9 system by lipid-encapsulated gold nanoparticles for tumor therapy. Angew. Chem. Int. Ed. Engl. 57, 1491–1496. doi: 10.1002/anie.201708689
Wang, Y. C., Wu, D. W., Wu, T. C., Wang, L., Chen, C. Y., and Lee, H. (2018). Dioscin overcome TKI resistance in EGFR-mutated lung adenocarcinoma cells via down-regulation of tyrosine phosphatase SHP2 expression. Int. J. Biol. Sci. 14, 47–56. doi: 10.7150/ijbs.22209
Xiang, D., Cheng, Z., Liu, H., Wang, X., Han, T., Sun, W., et al. (2017). Shp2 promotes liver cancer stem cell expansion by augmenting β-catenin signaling and predicts chemotherapeutic response of patients. Hepatology 65, 1566–1580. doi: 10.1002/hep.28919
Xiao, P., Zhang, H., Zhang, Y., Zheng, M., Liu, R., Zhao, Y., et al. (2019). Phosphatase Shp2 exacerbates intestinal inflammation by disrupting macrophage responsiveness to interleukin-10. J. Exp. Med. 216, 337–349. doi: 10.1084/jem.20181198
Xu, D., Liu, X., Yu, W. M., Meyerson, H. J., Guo, C., Gerson, S. L., et al. (2011). Non-lineage/stage-restricted effects of a gain-of-function mutation in tyrosine phosphatase Ptpn11 (Shp2) on malignant transformation of hematopoietic cells. J. Exp. Med. 208, 1977–1988. doi: 10.1084/jem.20110450
Xu, D., and Qu, C. K. (2008). Protein tyrosine phosphatases in the JAK/STAT pathway. Front. Biosci. 13:4925–4932. doi: 10.2741/3051
Xu, D., Wang, S., Yu, W. M., Chan, G., Araki, T., Bunting, K. D., et al. (2010). A germline gain-of-function mutation in Ptpn11 (Shp-2) phosphatase induces myeloproliferative disease by aberrant activation of hematopoietic stem cells. Blood 116, 3611–3621. doi: 10.1182/blood-2010-01-265652
Xu, D., Zheng, H., Yu, W. M., and Qu, C. K. (2013). Activating mutations in protein tyrosine phosphatase Ptpn11 (Shp2) enhance reactive oxygen species production that contributes to myeloproliferative disorder. PLoS One 8:e63152. doi: 10.1371/journal.pone.0063152
Yang, F., Xu, M., Wang, S., Song, L., Yu, D., Li, Y., et al. (2019). Gain-of-function E76K-mutant SHP2 promotes cell proliferation, metastasis, and tumor growth in glioblastoma through Activation Of the ERK/CREB pathway. Onco Targets Ther. 12, 9435–9447. doi: 10.2147/OTT.S222881
Yang, W., Wang, J., Moore, D. C., Liang, H., Dooner, M., Wu, Q., et al. (2013). Ptpn11 deletion in a novel progenitor causes metachondromatosis by inducing hedgehog signalling. Nature 499, 491–495. doi: 10.1038/nature12396
Yang, Z., Li, Y., Yin, F., and Chan, R. J. (2008). Activating PTPN11 mutants promote hematopoietic progenitor cell-cycle progression and survival. Exp. Hematol. 36, 1285–1296. doi: 10.1016/j.exphem.2008.04.016
Yokosuka, T., Takamatsu, M., Kobayashi-Imanishi, W., Hashimoto-Tane, A., Azuma, M., and Saito, T. (2012). Programmed cell death 1 forms negative costimulatory microclusters that directly inhibit T cell receptor signaling by recruiting phosphatase SHP2. J. Exp. Med. 209, 1201–1217. doi: 10.1084/jem.20112741
Yu, K., Yin, Y., Ma, D., Lu, T., Wei, D., Xiong, J., et al. (2020). Shp2 activation in bone marrow microenvironment mediates the drug resistance of B-cell acute lymphoblastic leukemia through enhancing the role of VCAM-1/VLA-4. Int. Immunopharmacol. 80:106008. doi: 10.1016/j.intimp.2019.106008
Yu, Z. H., Xu, J., Walls, C. D., Chen, L., Zhang, S., Zhang, R., et al. (2013). Structural and mechanistic insights into LEOPARD syndrome-associated SHP2 mutations. J. Biol. Chem. 288, 10472–10482. doi: 10.1074/jbc.M113.450023
Zhan, T., Rindtorff, N., Betge, J., Ebert, M. P., and Boutros, M. (2019). CRISPR/Cas9 for cancer research and therapy. Semin. Cancer Biol. 55, 106–119. doi: 10.1016/j.semcancer.2018.04.001
Zhang, J., Zhang, F., and Niu, R. (2015). Functions of Shp2 in cancer. J. Cell. Mol. Med. 19, 2075–2083. doi: 10.1111/jcmm.12618
Zhang, K., Zhao, H., Ji, Z., Zhang, C., Zhou, P., Wang, L., et al. (2016). Shp2 promotes metastasis of prostate cancer by attenuating the PAR3/PAR6/aPKC polarity protein complex and enhancing epithelial-to-mesenchymal transition. Oncogene 35, 1271–1282. doi: 10.1038/onc.2015.184
Zhang, Q., Li, Y., Zhao, R., Wang, X., Fan, C., Xu, Y., et al. (2018). The gain-of-function mutation E76K in SHP2 promotes CAC tumorigenesis and induces EMT via the Wnt/β-catenin signaling pathway. Mol. Carcinog. 57, 619–628. doi: 10.1002/mc.22785
Zhang, R. Y., Yu, Z. H., Chen, L., Walls, C. D., Zhang, S., Wu, L., et al. (2020). Mechanistic insights explain the transforming potential of the T507K substitution in the protein-tyrosine phosphatase SHP2. J. Biol. Chem. 295, 6187–6201. doi: 10.1074/jbc.RA119.010274
Zhao, H., Li, Y., He, L., Pu, W., Yu, W., Li, Y., et al. (2020). In vivo AAV-CRISPR/Cas9-mediated gene editing ameliorates atherosclerosis in familial hypercholesterolemia. Circulation 141, 67–79. doi: 10.1161/circulationaha.119.042476
Zhao, H., Martin, E., Matalkah, F., Shah, N., Ivanov, A., Ruppert, J. M., et al. (2019). Conditional knockout of SHP2 in ErbB2 transgenic mice or inhibition in HER2-amplified breast cancer cell lines blocks oncogene expression and tumorigenesis. Oncogene 38, 2275–2290. doi: 10.1038/s41388-018-0574-8
Zhao, M., Guo, W., Wu, Y., Yang, C., Zhong, L., Deng, G., et al. (2019). SHP2 inhibition triggers anti-tumor immunity and synergizes with PD-1 blockade. Acta pharmaceutica Sin. B 9, 304–315. doi: 10.1016/j.apsb.2018.08.009
Zhao, Y., Lin, L., Zhang, Y., and Geng, D. (2017). SHP-2 activating mutation promotes malignant biological behaviors of glioma cells. Med. Sci. Monit. 23, 2931–2938. doi: 10.12659/msm.904381
Zheng, H., Yu, W. M., Waclaw, R. R., Kontaridis, M. I., Neel, B. G., and Qu, C. K. (2018). Gain-of-function mutations in the gene encoding the tyrosine phosphatase SHP2 induce hydrocephalus in a catalytically dependent manner. Sci. Signal. 11:eaao1591. doi: 10.1126/scisignal.aao1591
Keywords: SHP2 mutation, tumor, cell-autonomous/non-cell autonomous mechanisms, SHP2 inhibition, tumor microenvironment
Citation: Dong L, Han D, Meng X, Xu M, Zheng C and Xia Q (2021) Activating Mutation of SHP2 Establishes a Tumorigenic Phonotype Through Cell-Autonomous and Non-Cell-Autonomous Mechanisms. Front. Cell Dev. Biol. 9:630712. doi: 10.3389/fcell.2021.630712
Received: 18 November 2020; Accepted: 04 January 2021;
Published: 11 March 2021.
Edited by:
Serge Roche, Institut National de la Santé et de la Recherche Médicale (INSERM), FranceReviewed by:
Jean-max Pasquet, Institut National de la Santé et de la Recherche Médicale (INSERM), FranceErnesto Diaz-Flores, University of California, San Francisco, United States
Copyright © 2021 Dong, Han, Meng, Xu, Zheng and Xia. This is an open-access article distributed under the terms of the Creative Commons Attribution License (CC BY). The use, distribution or reproduction in other forums is permitted, provided the original author(s) and the copyright owner(s) are credited and that the original publication in this journal is cited, in accordance with accepted academic practice. No use, distribution or reproduction is permitted which does not comply with these terms.
*Correspondence: Qin Xia, cXhpYUBiaXQuZWR1LmNu
†These authors share first authorship