- 1Department of Pharmacology and Toxicology, University of Louisville Health Science Center, Louisville, KY, United States
- 2University of Louisville Alcohol Research Center, Louisville, KY, United States
- 3Department of Medicine, University of Louisville, Louisville, KY, United States
- 4Department of Microbiology and Immunology, James Graham Brown Cancer Center, University of Louisville, Louisville, KY, United States
- 5Robley Rex Veterans Affairs Medical Center, Louisville, KY, United States
The neural crest cell (NCC) is a multipotent progenitor cell population that is sensitive to ethanol and is implicated in the Fetal Alcohol Spectrum Disorders (FASD). Studies have shown that sulforaphane (SFN) can prevent ethanol-induced apoptosis in NCCs. This study aims to investigate whether ethanol exposure can induce apoptosis in human NCCs (hNCCs) through epigenetically suppressing the expression of anti-apoptotic genes and whether SFN can restore the expression of anti-apoptotic genes and prevent apoptosis in ethanol-exposed hNCCs. We found that ethanol exposure resulted in a significant increase in the expression of DNMT3a and the activity of DNMTs. SFN treatment diminished the ethanol-induced upregulation of DNMT3a and dramatically reduced the activity of DNMTs in ethanol-exposed hNCCs. We also found that ethanol exposure induced hypermethylation at the promoter regions of two inhibitor of apoptosis proteins (IAP), NAIP and XIAP, in hNCCs, which were prevented by co-treatment with SFN. SFN treatment also significantly diminished ethanol-induced downregulation of NAIP and XIAP in hNCCs. The knockdown of DNMT3a significantly enhanced the effects of SFN on preventing the ethanol-induced repression of NAIP and XIAP and apoptosis in hNCCs. These results demonstrate that SFN can prevent ethanol-induced apoptosis in hNCCs by preventing ethanol-induced hypermethylation at the promoter regions of the genes encoding the IAP proteins and diminishing ethanol-induced repression of NAIP and XIAP through modulating DNMT3a expression and DNMT activity.
Introduction
Prenatal alcohol exposure can cause a spectrum of physical abnormalities and mental dysfunctions in children, which are defined as Fetal Alcohol Spectrum Disorders (FASD) (Koren et al., 2003; Sokol et al., 2003). Previous studies by Kotch and Sulik, as well as Dunty et al., have revealed that ethanol exposure during the early stages of development resulted in excessive cell death in neural crest cells (NCCs), which contributes heavily to ethanol-induced malformations (Kotch and Sulik, 1992; Dunty et al., 2001). Using a chick-specific antibody to NCCs, Cartwright and Smith confirmed that the prenatal ethanol exposure resulted in the loss of cranial NCCs in chicken embryos (Cartwright and Smith, 1995). Studies from our laboratory have also shown that ethanol exposure significantly increased apoptosis in NCCs in vitro (Chen et al., 2015; Li et al., 2019b).
Multiple signaling pathways have been shown to be involved in the ethanol-induced apoptosis in NCCs, including the Bcl2 family (Chen et al., 2015; Yuan et al., 2018), Seven in absentia homolog1 (Siah1) (Sun et al., 2014; Yuan et al., 2017), p53 and MAPK signaling (Yuan et al., 2017, 2020) and Nrf2 signaling (Chen et al., 2013). However, the potential role of the inhibitor of apoptosis proteins (IAP) in ethanol-induced apoptosis in NCCs remains to be defined. IAP family is composed of eight members presenting one to three BIR domains from the baculovirus (Birnbaum et al., 1994). They are frequently overexpressed in cancer cells that are resistant to apoptosis (Silke and Meier, 2013). DNA hypomethylation at the promoters of IAP genes and the overexpression of the IAPs have been found in different cancers, including ovarian, hepatocellular carcinoma (HCC), and glioma (Hervouet et al., 2013). Studies have also shown that the loss of methylation induced by DNA methyltransferase (DNMT) inhibitor at the promoters of the IAP genes is associated with apoptosis resistance (Hervouet et al., 2013).
It is well-known that DNA hypermethylation in the promoter regions of genes is associated with gene repression (Jones and Baylin, 2002) and plays an important role in the repression of many genes involved in various cellular functions, including DNA repair, cell adhesion, and apoptosis (Teodoridis et al., 2004; Gopisetty et al., 2006). DNA methylation has been shown to be associated with the repression of the apoptotic genes in cancer cells (Murphy et al., 2008; Malekzadeh et al., 2009; Hervouet et al., 2013). Hypermethylation at the Casp 8 promoter was correlated with a low Casp 8 expression in cancer cells and contributed to apoptosis resistance in different cancer cell lines, such as pediatric cancer (Harada et al., 2002), lung carcinomas (Shivapurkar et al., 2002), and breast cancer (Wu et al., 2010). Studies have shown that ethanol exposure can alter DNA methylation (Haycock and Ramsay, 2009; Ouko et al., 2009; Zhou et al., 2011) and histone modification (Zhong et al., 2010; Bekdash et al., 2013; Subbanna et al., 2013, 2014). An earlier study by Garro has also shown that fetal DNA was hypermethylated after ethanol exposure during embryonic development (Garro et al., 1991).
DNA methylation occurs on the cytosine residue of CpG dinucleotides through the transfer of 5-methylcytosine from the methyl donor S-adenosylmethionine (SAM) to the CpG. The reaction is catalyzed by a family of enzymes called DNA methyltransferases (DNMTs), including DNMT1, DNMT3a, and DNMT3b (Singal and Ginder, 1999). DNMT1 is responsible for the maintenance of established patterns of DNA methylation, while DNMT3a and DNMT3b mediate the establishment of new or de novo DNA methylation patterns (Chen et al., 2003; Jones and Liang, 2009b). Alteration of DNA methylation by DNMTs may trigger hypermethylation or hypomethylation of gene promoters and consequently result in activating or inhibiting gene expression (Hervouet et al., 2010, 2013).
Sulforaphane (SFN) is a vegetable-derived isothiocyanate that is abundant in cruciferous vegetables such as broccoli. Our previous studies have shown that SFN prevented ethanol-induced apoptosis through upregulating the antioxidant gene Nrf2 in NCCs (Chen et al., 2013). In addition to acting as an Nrf2 inducer, SFN has been found to regulate gene expression by inhibiting the activity of DNMTs (Meeran et al., 2012). It has also been reported that SFN can inhibit LPS-induced DNMT3a gene expression and confer resistance to LPS-induced apoptosis in porcine monocyte-derived dendritic cells (Qu et al., 2015).
In the present study, we determined whether SFN can prevent ethanol-induced apoptosis in hNCCs through epigenetic modulation of anti-apoptotic genes. We found that ethanol exposure resulted in a significant increase in the expression of DNMT3a and the activity of DNMTs. SFN treatment diminished the ethanol-induced upregulation of DNMT3a and dramatically reduced the activity of DNMTs in ethanol-exposed hNCCs. Ethanol exposure also induced hypermethylation at the promoter regions of two IAP proteins, NAIP and XIAP, in hNCCs, which were prevented by co-treatment with SFN. SFN treatment also significantly diminished ethanol-induced downregulation of NAIP and XIAP in hNCCs. The knockdown of DNMT3a significantly enhanced the effects of SFN on preventing the ethanol-induced repression of NAIP and XIAP and apoptosis in hNCCs. These results demonstrate that SFN can prevent ethanol-induced apoptosis in hNCCs by diminishing the ethanol-induced hypermethylation at the promoter regions of the genes encoding the IAP proteins.
Materials and Methods
Human Neural Crest Cell Differentiation, Culture and Treatment
hNCCs were differentiated from human embryonic stem cell (hESC) line H9 (WA09)), which was purchased from WiCell® (Madison, WI, United States). hESCs were maintained in mTeSRTM1 (StemCell Technologies, Inc., Vancouver, Canada) on hESCs-qualified MatrigelTM (BD Biosciences, San Jose, CA) coated plates, following the WiCell’s protocols. The differentiation of hNCCs from hESCs was performed as previously reported (Menendez et al., 2013; Avery and Dalton, 2016) with modification. Briefly, the hESCs® were first adapted to Accutase solution (Stem Cell Technologies, Vancouver, BC, Canada) and dissociated into single cells and cultured in the hESCs maintenance medium. When hESCs reached 75–85% confluence, the hESCs maintenance medium was removed, and the hESCs were detached by adding the Accutase® solution. The collected hESCs were resuspended and cultured in the hNCCs differentiation medium (DMEM/F-12 Medium, 14.3 M L-Glutamine + β-mercaptoethanol, MEM Non-Essential Amino Acid, 10 μg/mL Fgf2, 10 μg/mL Heregulin β-1, 200 μg/mL Long R3-IGF1, 10 mM CHIR 99021, 10 mM SB421542 and Penicillin and streptomycin). When cells reached 75–85% confluence, the differentiating cells were passed using Accutase® and then maintained in hNCCs differentiation medium. After culture in hNCCs-differentiation medium for around 10 days, hNCCs were collected, and NCC identity was analyzed by examining the NCC markers p75, HNK1, and AP2 using immunocytochemistry, flow cytometry and/or RT-PCR. For treatments, hNCCs were first pretreated with 1 μM SFN (LST Laboratories, St. Paul, MN) for 24 h, and then the cells were concurrently exposed to 1 μM SFN and 50 mM ethanol for an additional 24 h. To maintain the stable ethanol levels, the cell culture dishes were placed in a plastic desiccator containing ethanol in distilled water, as described previously (Yan et al., 2010).
Quantitative Real-Time PCR
Quantitative real-time PCR was performed as previously described (Fan et al., 2019). Briefly, total RNA was isolated from control and treated hNCCs using a QIAGEN RNeasy mini kit (QIAGEN, Valencia, CA) according to the manufacturer’s instruction. The cDNAs were synthesized using QuantiTect Reverse Transcription Kit (QIAGEN, Valencia, CA) following the manufacturer’s protocol. Quantitative RT-PCR was performed using FastStart SYBR Green Master (Roche Applied, Indianapolis, IN, United States) on a Rotor-Gene 600 Real-time PCR system (Corbett LifeScience, Mannheim, Germany). The following primer pairs were used for this analysis: NAIP (Homo sapiens) forward: 5′-GCCTAGATGCAGTTCAGTTGG-3′, reverse: 3′-GGCACCAAAGAGGATTAGGCT-3′; XIAP (Homo sapiens) forward: 5′-GGTGAAGGAGATACCGTGCG-3′, reverse: 3′-GCATGTGTCTCAGATGGCCT-5′; β-Actin (Homo sapiens) forward: 5′-AGAAGGATTCCTATGTGGGCG-3′, reverse: 3′-GGATAGCACAGCCTGGATAGCA-5′. The primers were synthesized by Integrated DNA Technologies, Inc. (IDT, Coralville, IA, United States). All assays were carried out in triplicate. Relative quantitative analysis was performed by comparing the threshold cycle number for target genes and a reference β-Actin mRNA.
Western Blotting
Western blotting was performed by the standard protocol as previously described (Yuan et al., 2017). Briefly, hNCCs from control and experimental groups were washed with PBS and then lysed in cold RIPA lysis buffer with 1 mM PMSF and protease cocktail inhibitors. Whole-cell lysis was centrifuged at 12,000 × g for 10 min at 4°C, and the supernatants were used for Western blot. Protein concentrations were measured by using BSA Protein Assay Reagent Kit (Pierce, Thermo Scientific, Waltham, MA). Proteins were probed with the following antibodies: anti-cleaved caspase-3 rabbit mAb (Cell Signaling, Beverly, MA, United States), anti-DNMT3a rabbit mAb (Cell Signaling, Beverly, MA, United States), anti-NAIP sheep pAb (R&D system, Minneapolis, MN, United States), anti-XIAP rabbit mAb (Cell Signaling, Beverly, MA, United States), and anti-β–Actin mouse mAb (Santa Cruz, Santa Cruz, CA). The densitometry of the blots was analyzed using ImageJ software (National Institute of Health, United States). All Western blot analyses were performed in triplicate.
Analysis of DNMT Activity
hNCCs from the control and treated groups were harvested, and nuclear extracts were isolated by using the EpiQuik Nuclear Extraction Kit (Epigentek, Farmingdale, NY) according to the manufacturer’s protocol. The protein concentrations of nuclear extraction were measured using the BCA protein assay kit (Thermo Scientific, Irvine, CA). DNMT activity was determined with an EpiQuik DNA Methyltransferase Activity Assay kit (Epigentek, Farmingdale, NY), following the manufacturer’s instruction.
Methylated Specific PCR (MSP)
The methylation status of CpG island on the promoters of NAIP and XAIP was determined by MSP. Genomic DNA was extracted from control and treated hNCCs using DNeasy Blood & Tissue Kit (QIAGEN, Valencia, CA) according to the manufacturer’s instruction. Bisulfite conversion of the genomic DNA was performed using the EpiTect Bisulfite Kit (QIAGEN, Valencia, CA). Bisulfite-modified DNA was amplified using two primer sets specific for the detection of the methylated (M) and unmethylated (U) sequences. The following primer pairs were used for MSP analysis. NAIP (M) forward: 5′-AGGTTGGAGTATCGTGGC-3′, reverse: 3′-GAACGTAATAACGAACGCCT-5′, NAIP (U) forward: 5′-TTTAGGTTGGAGTATTGTGGT-3′, reverse: 3′-ACCAAACATAATAACAAACACCT-5′; XIAP (M) forward: 5′-GGCGGAGGTTGTAGTGAGTC-3′, reverse: 3′-ATAAACATAAACCACCGCGC-5′, XIAP (U) forward: 5′-GGAGGTGGAGGTTGTAGTGAGTT-3′, reverse: 3′-TATAAACATAAACCACCACACCC-5′. Real-time qMSP assays were performed on a Rotor-Gene 600 Real-time PCR system (Corbett LifeScience, Mannheim, Germany), and the PCR products were separated on 2% agarose gel. The intensity of DNA bands was measured by using NIH ImageJ software (National Institute of Health, United States). Methylation levels were calculated as the intensity of the methylated DNA band relative to the combined intensity of the methylated and unmethylated DNA bands.
siRNA Transfection
hNCCs were transfected with DNMT3a siRNA (SMART pool: On-TARGET plus human DNMT3a) (GE Healthcare Dharmacon, Lafayette, CO) or scramble control siRNA (IDT, Coralville, IA) in a final concentration of 25 nM by using LipofectamineTM2000 (Thermo Fisher, Waltham, MA), according to the manufacturer’s instruction. The cells were harvested 24 h after transfection for experiments.
Analysis of Cell Viability and Apoptosis
Cell viability was measured using an MTS (3-(4,5-dimethylthiazol-2-yl)-5-(3- arboxymethoxyphenyl)-2-(4- sulfophenyl)-2H-tetrazolium salt) assay kit (Promega, Madison, WI), as described previously (Chen et al., 2013). Apoptosis was determined by the analysis of caspase-3 cleavage by Western blot and by flow cytometry using a FITC Annexin V apoptosis detection kit (BD Bioscience, Franklin Lakes, NJ, United States) as described previously (Li et al., 2019b).
Statistical Analysis
Statistical analyses were performed using GraphPad Prism software (GraphPad Software, San Diego, CA, United States). All data were expressed as mean ± SEM of at least three independent experiments. Comparisons between groups were analyzed by one-way ANOVA. Multiple comparison post-tests were conducted using Bonferroni’s test. Differences between groups were considered significant at p < 0.05.
Results
SFN Treatment Significantly Diminished Ethanol-Induced Apoptosis in hNCCs
For this study, hNCCs were differentiated from human embryonic stem cells and validated by examining the expression of NCC marker HNK1 using immunocytochemistry (Figure 1A). To determine whether SFN treatment can diminish the ethanol-induced reduction in cell viability in hNCCs, the cells were treated with ethanol alone or pretreated with SFN at different concentrations for 24 h, followed by concurrent exposure to SFN and ethanol for an additional 24 h. As shown in Figure 1B, SFN at 1 μM significantly diminished the reduction of cell viability in hNCCs exposed to 50 mM ethanol. Treatment with SFN also significantly reduced caspase-3 activation in hNCCs exposed to 50 mM ethanol for 24 h (Figure 1C).
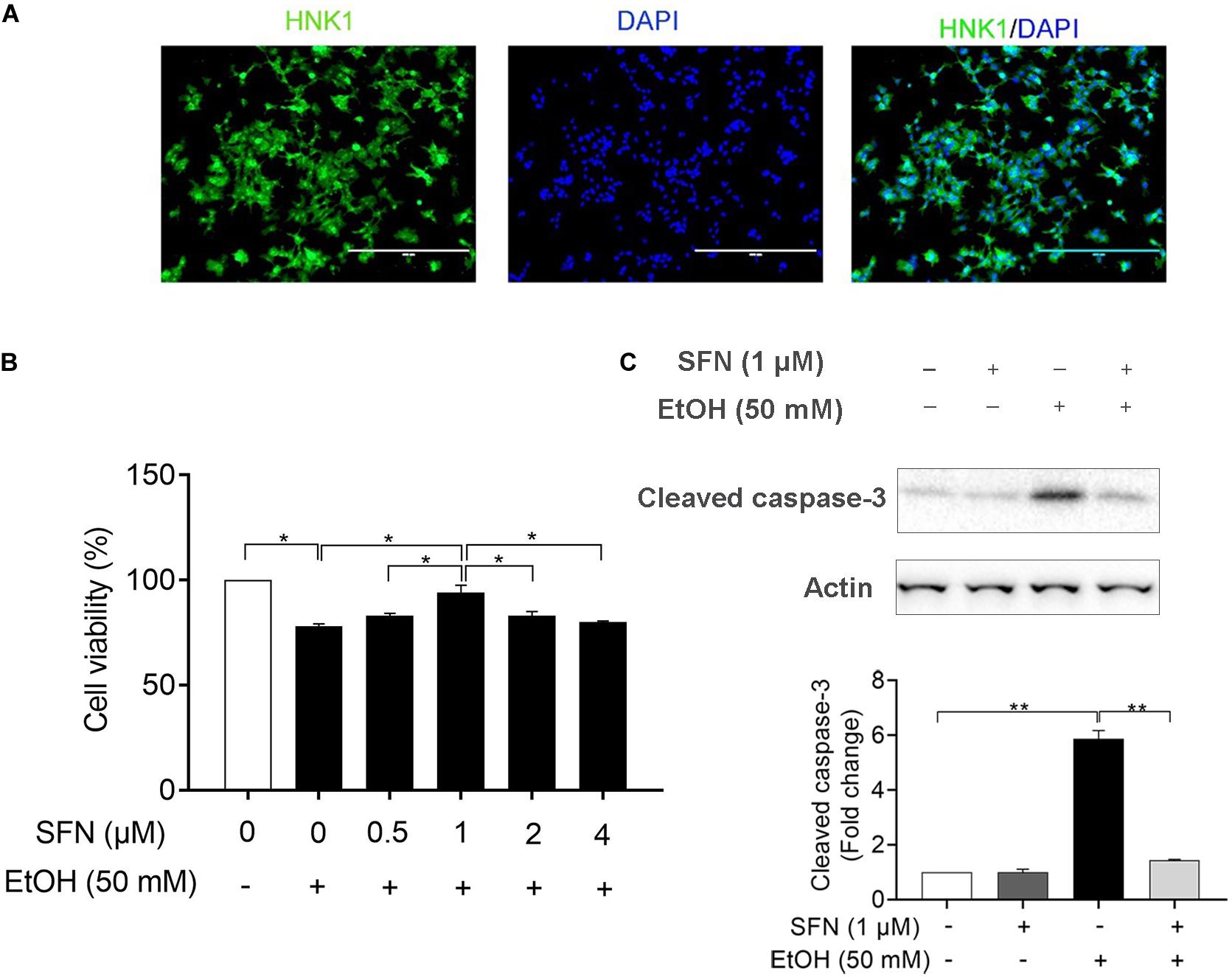
Figure 1. SFN significantly diminished the ethanol-induced apoptosis in hNCCs. (A) Immunocytochemistry of NCC marker, HNK1, in hNCCs differentiated from hESCs. (B) hNCCs were treated with 50 mM ethanol alone or pretreated with SFN at different concentrations for 24 h, followed by concurrent exposure to SFN and ethanol for an additional 24 h. Cell viability was determined by MTS assay. (C) hNCCs were treated with 1 μM SFN for 24 h, followed by concurrent exposure to 1 μM SFN and 50 mM ethanol for an additional 24 h. Apoptosis was determined by the analysis of caspase-3 cleavage using Western blotting. Data are expressed as a percentage of control (B) or fold change over control (C) and represent the mean ± SEM of three separated experiments. *p < 0.05, **p < 0.01.
SFN Diminished Ethanol-Induced Increase in DNMT Activity and Up-Regulation of DNMT3a in hNCCs
To determine the effects of ethanol and SFN on the activity and expression of DNMT, hNCCs were pretreated with 1 μM SFN for 24 h, followed by 24 h of concurrent exposure to 1 μM SFN and 50 mM ethanol. As shown in Figure 2A, ethanol exposure significantly increased the DNMT activity in hNCCs. Treatment with SFN dramatically reduced the ethanol-induced increase in the activity of DNMT in hNCCs. To determine whether ethanol and SFN also affect the expression of DNMT, the protein expression of DNMT3a, one of the three DNMTs, which is involved in DNA methylation in eukaryotic cells (Leonhardt and Bestor, 1993; Jones and Liang, 2009a), was analyzed by Western blotting. We found that exposure to 50 mM ethanol resulted in a significant increase in the protein expression of DNMT3a in hNCCs. Co-treatment with SFN and ethanol significantly reduced ethanol-induced increase in DNMT3a protein expression (Figure 2B).
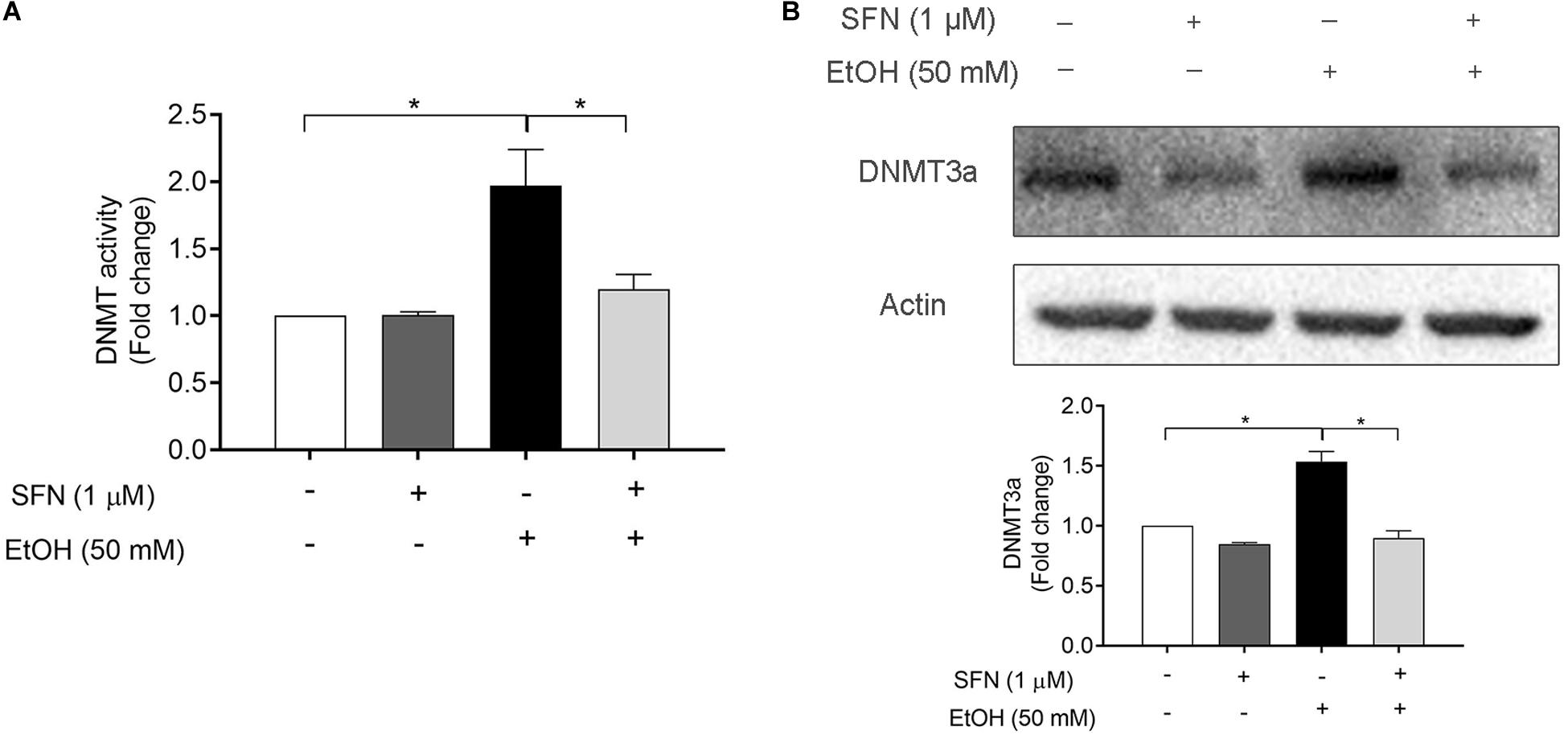
Figure 2. SFN diminished ethanol-induced increase in DNMT activity and the up-regulation of DNMT3a in hNCCs. hNCCs were treated with 1 μM SFN for 24 h, followed by concurrent exposure to 1 μM SFN and 50 mM ethanol for an additional 24 h. (A) The DNMT activity was determined by using an EpiQuik DNA Methyltransferase Activity Assay kit. (B) The protein expression of DNMT3a was determined by Western blotting. Data are expressed as fold change over control and represent the mean ± SEM of three separated experiments. *p < 0.05.
SFN Diminished the Ethanol-Induced Hypermethylation at the Promoters of NAIP and XIAP in hNCCs
To determine whether ethanol-induced up-regulation of DNMT3a and increase in DNMT activity can result in hypermethylation at the promoters of the IAP genes and whether SFN can diminish ethanol-induced hypermethylation at the promoters of these genes, the methylation status of the CpG islands at the promoters of XIAP and NIAP in hNCCs was analyzed by using methylation-specific PCR (MSP). As shown in Figure 3, exposure of hNCCs to ethanol for 24 h resulted in a significant increase in the methylation levels at the promoters of XIAP and NIAP. Co-treatment with SFN significantly diminished ethanol-induced hypermethylation at the promoters of these anti-apoptotic genes in hNCCs. These results demonstrate that ethanol exposure can induce hypermethylation at the promoters of NAIP and XIAP in hNCCs, which can be prevented by co-treatment with SFN.
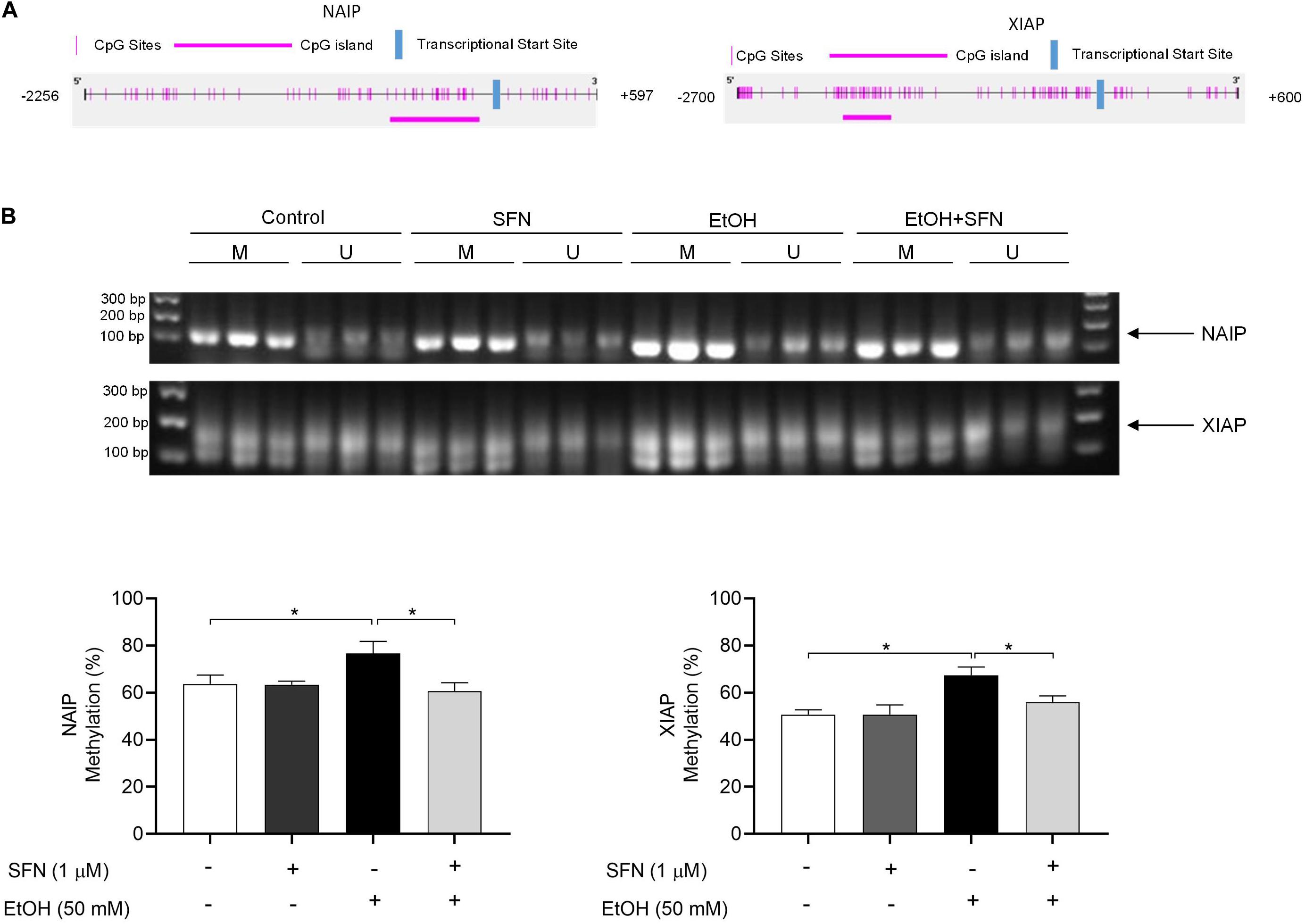
Figure 3. SFN significantly diminished the ethanol-induced hypermethylation at the promoters of XIAP and NAIP in hNCCs. hNCCs were treated with 1 μM SFN for 24 h, followed by concurrent exposure to 1 μM SFN and 50 mM ethanol for an additional 24 h. (A) Schematic illustration of the CpG sites and CpG island in the promoters of NAIP and XIAP. The selected CpG island in the NAIP gene promoter is in the region from –634 to –64 bp in the promoter of NAIP. The selected CpG island in the XIAP gene promoter is in the region from –2156 to –1734 bp in the promoter of XIAP. (B) DNA methylation status at the CpG island of the promoters of NAIP and XIAP was determined by using MSP. Data are expressed as the percentage of methylation and represent the mean ± SEM of three separated experiments. *p < 0.05. M, methylated DNA, U, unmethylated DNA.
SFN Diminished Ethanol-Induced Down-Regulation of IAP Genes in hNCCs
To determine whether ethanol-induced hypermethylation at the promoters of NAIP and XIAP can repress the expression of NAIP and XIAP and whether SFN can prevent ethanol-induced down-regulation of NAIP and XIAP by diminishing ethanol-induced hypermethylation at the promoters of these genes in hNCCs, the mRNA expression of NAIP and XIAP was analyzed in hNCCs. As shown in Figure 4, exposure to 50 mM ethanol for 24 h resulted in a dramatic reduction in mRNA expression of NAIP and XIAP in hNCCs. Treatment with SFN significantly diminished ethanol-induced down-regulation of NAIP and XIAP in hNCCs, indicating that SFN can diminish ethanol-induced repression of NAIP and XIAP in hNCCs by preventing ethanol-induced hypermethylation at the promoters of NAIP and XIAP.
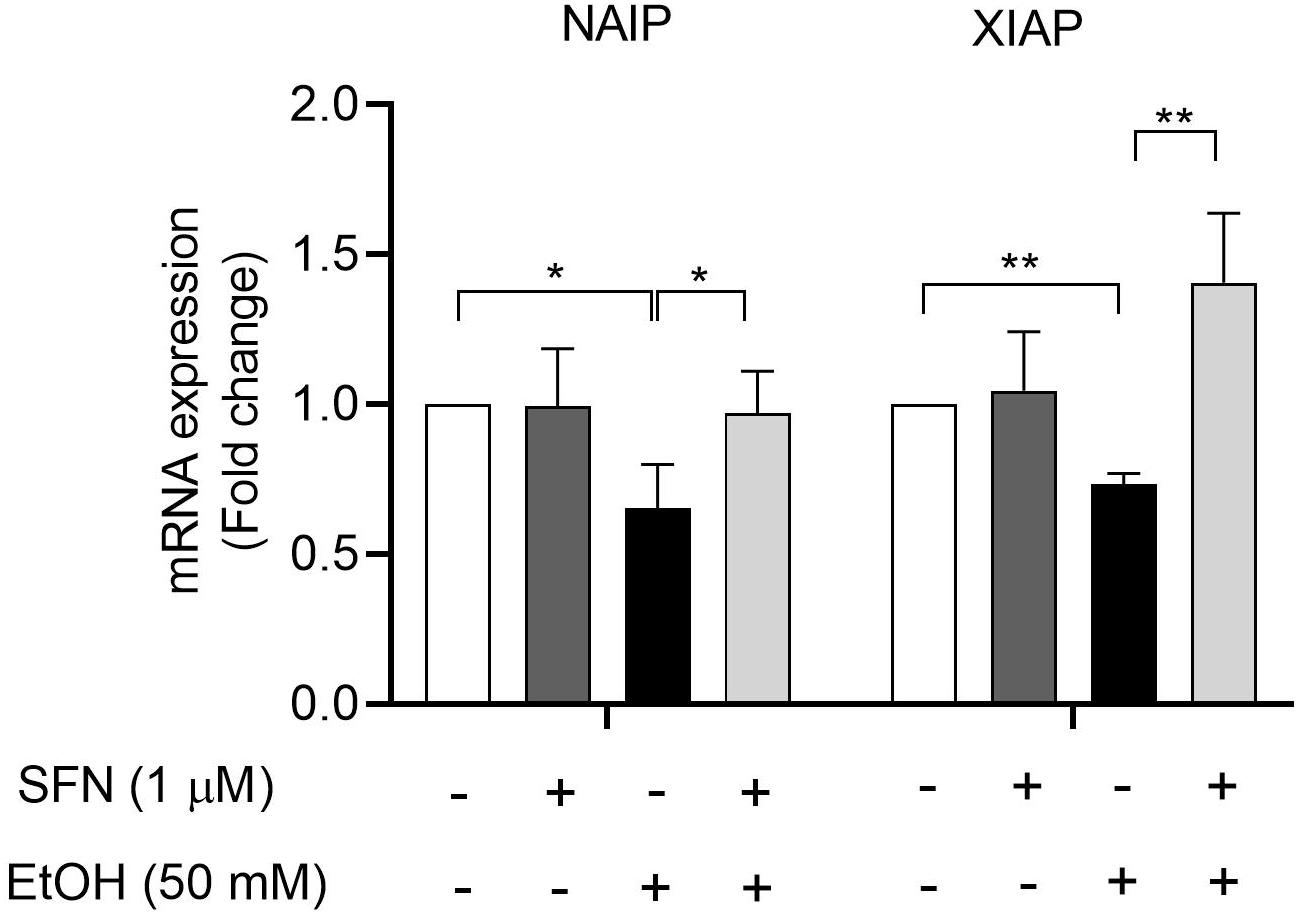
Figure 4. SFN diminished ethanol-induced down-regulation of IAP genes in hNCCs. hNCCs were treated with 1 μM SFN for 24 h, followed by concurrent exposure to 1 μM SFN and 50 mM ethanol for an additional 24 h. The mRNA expression of the NAIP and XIAP was determined by using qRT-PCR. The data were expressed as fold change over control and represent the mean ± SEM of three separated experiments. *p < 0.05, **P < 0.01.
Knockdown of DNMT3a Significantly Diminished Ethanol-Induced Down-Regulation of NAIP and XIAP and Enhanced the Effects of SFN Against Ethanol-Induced Down-Regulation of NIAP and XIAP in hNCCs
To determine whether the upregulation of DNMT3a contributes to the ethanol-induced down-regulation of NAIP and XIAP, DNMT3a was knocked down by siRNA in hNCCs before treatment with ethanol or/and SFN. We found that the knockdown of DNMT3a significantly diminished ethanol-induced decreases in the mRNA expression of NAIP and XIAP. Down-regulation of DNMT3a by siRNA also enhanced the effects of SFN against ethanol-induced down-regulation of NIAP and XIAP in hNCCs. In addition, knockdown of DNMT3a significantly diminished ethanol-induced decreases in the protein expression of NAIP and XIAPI and significantly enhanced the effects of SFN against ethanol-induced reductions in the protein expression of NAIP and XIAP (Figure 5). These results indicate that the down-regulation of DNMT3a and the inhibition of DNMT activity by SFN can diminish ethanol-induced repression of anti-apoptotic genes in hNCCs.
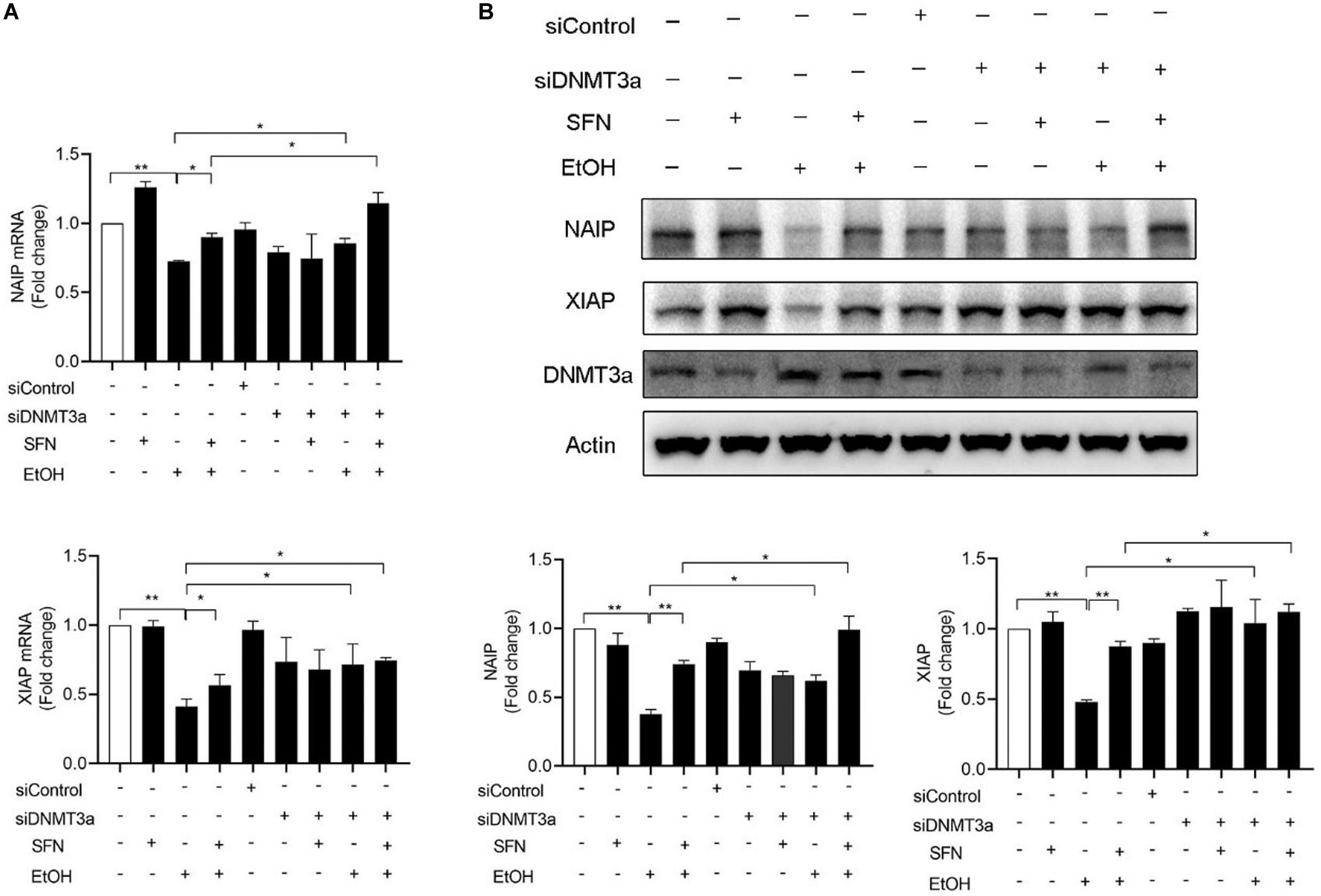
Figure 5. The knockdown of DNMT3a diminished ethanol-induced down-regulation of NAIP and XIAP and enhanced the effects of SFN against ethanol-induced down-regulation of NAIP and XIAP in hNCCs. hNCCs transfected with control-siRNA or DNMT3a-siRNA were treated with 1 μM SFN for 24 h, followed by concurrent exposure to 1 μM SFN and 50 mM ethanol for an additional 24 h. (A) The mRNA expression of NAIP and XIAP was determined by qRT-PCR. (B) The protein expression of NAIP and XIAP was determined by Western blotting. Data are expressed as fold change over control and represent the mean ± SEM of three separated experiments. *p < 0.05, **p < 0.01.
Knockdown of DNMT3a Diminished Ethanol-Induced Apoptosis and Enhanced the Protective Effects of SFN Against Ethanol-Induced Apoptosis in hNCCs
To determine whether ethanol-induced upregulation of DNMT3a and subsequent repression of anti-apoptotic genes, NAIP and XIAP, contributes to ethanol-induced apoptosis and whether SFN can prevent ethanol-induced apoptosis by diminishing ethanol-induced upregulation of DNMT3a, DNMT3a was knocked down by siRNA in hNCCs. Apoptosis was determined by the analysis of caspase-3 activation using Western blot. As shown in Figure 6A, the knockdown of DNMT3a by siRNA significantly reduced ethanol-induced caspase-3 activation, indicating that the knockdown of DNMT3a can diminish ethanol-induced apoptosis in hNCCs. These results were further confirmed by the results from the flow cytometric analysis of Annexin V staining, which have clearly shown that knockdown of DNMT3a can significantly reduce the number of early apoptotic cells in ethanol-exposed hNCCs (Figure 6B). The knockdown of DNMT3a also significantly enhanced the protective effects of SFN against ethanol-induced apoptosis. These results demonstrate that ethanol-induced upregulation of DNMT3a contributes to ethanol-induced apoptosis and that SFN can prevent ethanol-induced apoptosis in hNCCs by diminishing ethanol-induced upregulation of DNMT3a and reducing the activity of DNMTs.
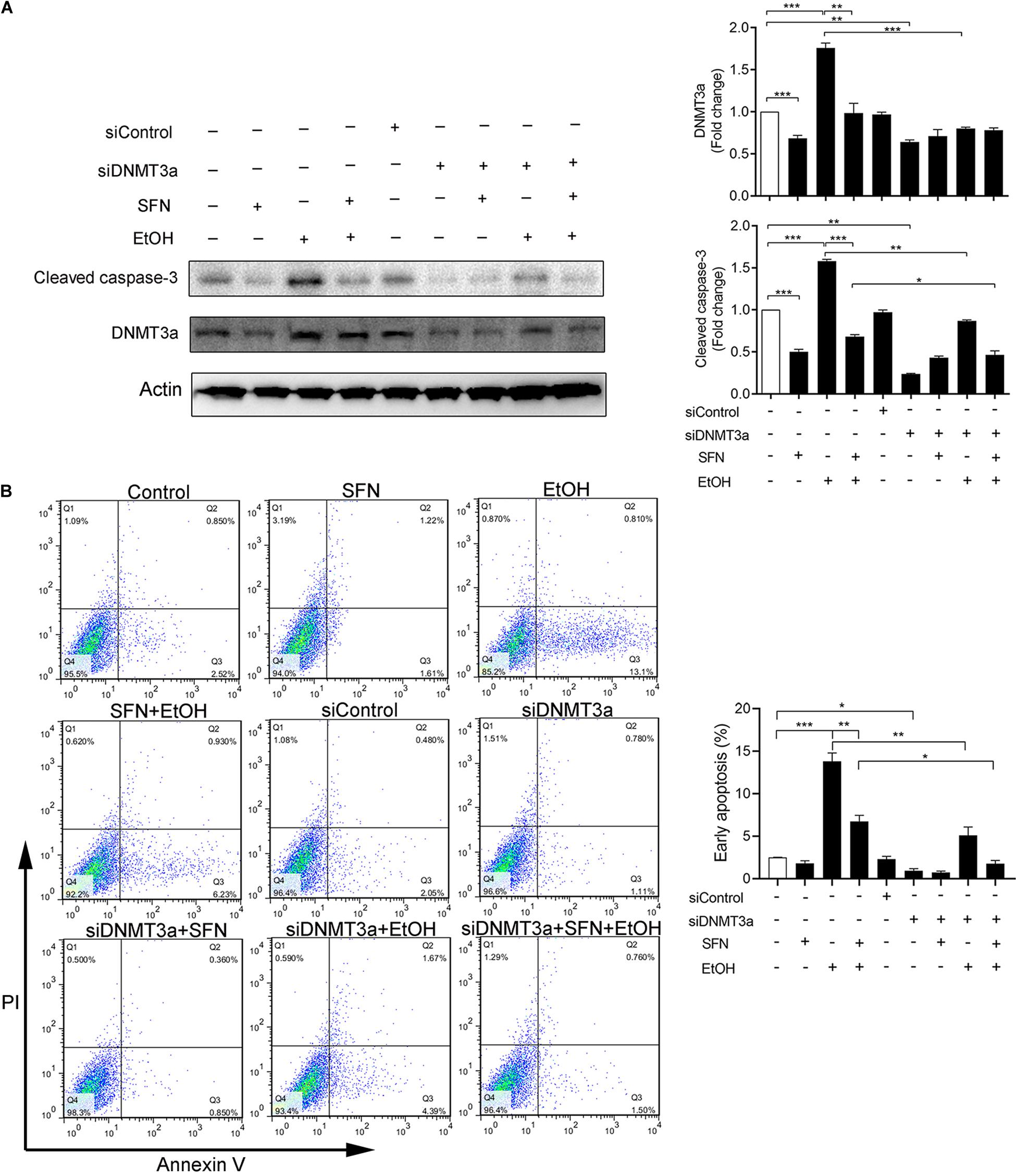
Figure 6. The knockdown of DNMT3a diminished ethanol-induced apoptosis and enhanced the protective effects of SFN in hNCCs. hNCCs transfected with control-siRNA or DNMT3a-siRNA were treated with 1 μM SFN for 24 h, followed by concurrent exposure to 1 μM SFN and 50 mM ethanol for an additional 24 h. The protein expression of DNMT3a was determined by Western blotting (A). Apoptosis was determined by the analysis of caspase-3 cleavage by Western blotting (A) or flow cytometry with Annexin V-FITC apoptosis detection kit (B). Data are expressed as fold change over control (A) or percentage (B) and represent the mean ± SEM of three separated experiments. *p < 0.05, **p < 0.01, ***p < 0.001.
Discussion
Ethanol-induced apoptosis in NCCs is one of the major mechanisms underlying the pathogenesis of FASD. Previous studies have demonstrated that ethanol exposure during the early stages of development resulted in excessive cell death in NCCs in mouse embryos (Kotch and Sulik, 1992; Dunty et al., 2001). Using an in vitro model of mouse NCCs, JoMa1.3, our laboratory has also shown that ethanol exposure significantly increased apoptosis in NCCs (Chen et al., 2015; Li et al., 2019b). In this study, we have shown that exposure to ethanol resulted in a significant increase in apoptosis in hNCCs. This is the first study that demonstrates that ethanol can induce apoptosis in human NCCs.
Apoptosis can be induced and regulated by multiple signaling pathways, including the inhibitor of apoptosis proteins (IAP), a group of negative regulators of apoptosis. IAPs contribute to cell survival by controlling the formation of cell-death-activating platforms, including the apoptosome in Drosophila and the Ripoptosome in mammals, and mediating activation of NF-kB and subsequent induction of pro-survival gene transcription through their ability to functions as E3 ligases (Silke and Meier, 2013). IAPs have been implicated in the pathology of human cancers by inhibiting apoptosis, and the overexpression of IAPs has been found in various cancers (Wright and Duckett, 2005). Studies have also revealed the importance of IAPs in the adaptive response to cell damages (Marivin et al., 2012). It was reported that XIAP and NIAP are involved in the adaptive response of neuronal cells to hypoxic-ischemia-induced injury (Holcik et al., 2000; Russell et al., 2008; West et al., 2009). The down-regulation of XIAP has also been linked to neurodegenerative disorders, including Wilson’s and Huntington’s diseases (Goffredo et al., 2005; Weiss et al., 2010). In addition, IAPs were found to be critical for embryonic development. The knockdown of birc5a resulted in multiple defects in the nervous system, the cardiovascular system, and the hematopoietic system (Delvaeye et al., 2009). In this study, we have shown that ethanol exposure can significantly decrease the expression of NAIP and XIAP and induce apoptosis in hNCCs and that SFN treatment that can diminish ethanol-induced down-regulation of NAIP and XIAP can also decrease the ethanol-induced apoptosis in hNCCs, demonstrating that down-regulation of NAIP and XIAP contributes to ethanol-induced apoptosis in hNCCs.
Epigenetic modifications, including DNA methylation and histone modifications, are well-known mechanisms for the regulation of gene expression and are involved in the regulation of many genes associated with various cellular functions, including apoptosis (Boyanapalli and Kong, 2015; Sui et al., 2015; Kang et al., 2019). Studies have shown that epigenetic modifications contribute to the detrimental consequences of alcohol abuse during pregnancy in the developing fetus (Basavarajappa and Subbanna, 2016). Recent studies in our laboratory have demonstrated that ethanol exposure significantly increased apoptosis through decreasing histone acetylation at the promoters of the anti-apoptotic gene, Bcl2 (Yuan et al., 2018). We have also shown that ethanol-induced reduction in the enrichment of trimethylation of histone H3 Lysine 4 (H3K4me3) at the promoters of Snail1 contributes to ethanol-induced apoptosis in NCCs (Li et al., 2019b). In this study, we have shown that ethanol exposure resulted in a significant increase in the methylation levels at the promoters of XIAP and NIAP and decreased the expression of these anti-apoptotic genes in hNCCs. These results indicate that, in addition to histone modification, DNA methylation also contributes to the regulation of the anti-apoptotic genes in ethanol-exposed NCCs.
DNMTs are key enzymes that catalyze the DNA methylation process by transferring a methyl group to DNA (Robertson et al., 1999; Uysal et al., 2015). DNMT3a is the main enzyme involved in DNA methylation in mammals (Singal and Ginder, 1999). It is well-known that DNMT3a plays a critical role in the establishment of methylation patterns during development (Zahnow et al., 2016). In addition, the defect of DNMT3a was reported to result in non-progressive neurodevelopmental conditions (Weissman et al., 2014). Environmental stimuli in pregnant rat have been found to increase DNMT3a expression and alter DNA methylation levels in their offspring (Jensen Pena et al., 2012). A study has also demonstrated that ethanol exposure increased the expression of DNMT3a in rats (Sakharkar et al., 2014). In addition, it has been reported that DNMT3a can regulate the expression of apoptosis-related genes in cancer cells through changing the methylation levels at the promoter regions of the apoptosis-related genes, such as Bad, Bax and Bcl2 (Jing et al., 2019; Li et al., 2019a; Wei et al., 2019). Consistent with these findings, we found that ethanol exposure significantly increased the expression of DNMT3a in hNCCs. Ethanol exposure also resulted in a significant increase in the activity of DNMT. In addition, knockdown of DNMT3a dramatically diminished ethanol-induced down-regulation of NAIP and XIAP, demonstrating that the ethanol-induced upregulation of DNMT3a contributes to the hypermethylation at the promoters of NAIP and XIAP and the down-regulation of these anti-apoptotic genes in hNCCs exposed to ethanol.
It has been reported that SFN can act as an inhibitor of histone deacetylase (HDAC) and DNMTs to modulate epigenetic modification of genes in varied types of cells (Ali Khan et al., 2015; Su et al., 2018; Yuan et al., 2018). Studies have demonstrated that SFN can suppress the LPS-induced apoptosis in monocyte-derived dendritic cells through down-regulating DNMT3a and the suppression of TGF-β signaling (Qu et al., 2015). Recent studies from our laboratory have also demonstrated that SFN can reduce ethanol-induced apoptosis in NCCs by diminishing ethanol-induced reduction of histone acetylation at the promoter of Bcl2 and preventing the down-regulation of Bcl2 in ethanol-exposed NCCs (Yuan et al., 2018). In the present study, we found that SFN treatment diminished ethanol-induced upregulation of DNMT3a and dramatically reduce the activity of DNMTs in ethanol-exposed hNCCs. Treatment with SFN also diminished the ethanol-induced hypermethylation at the promoters of NAIP and XIAP and the down-regulation of NAIP and XIAP and subsequently reduced ethanol-induced apoptosis in hNCCs. These results demonstrate that SFN can prevent ethanol-induced apoptosis in hNCCs by epigenetically modulating the DNA methylation at the promoters of anti-apoptotic genes.
In conclusion, the present study has demonstrated that SFN treatment diminished the ethanol-induced upregulation of DNMT3a and dramatically reduced the activity of DNMTs in ethanol-exposed hNCCs. We also found that ethanol exposure induced hypermethylation at the promoter regions of NAIP and XIAP, which were prevented by co-treatment with SFN. SFN also diminished ethanol-induced down-regulation of NAIP and XIAP and apoptosis in hNCCs. The knockdown of DNMT3a significantly enhanced the effects of SFN on preventing the ethanol induced repression of NAIP and XIAP and apoptosis in hNCCs. These results demonstrate that ethanol-induced upregulation of DNMT3a and the hypermethylation at the promoters of NAIP and XIAP and subsequent down-regulation of NAIP and XIAP contribute to ethanol-induced apoptosis in hNCCs and that SFN can prevent ethanol-induced apoptosis in hNCCs by preventing ethanol-induced hypermethylation at the promoters of the genes encoding the IAP proteins. These findings suggest that SFN may represent a promising and effective agent for the prevention of ethanol-induced apoptosis and FASD.
Data Availability Statement
All datasets generated in this study are included in the article.
Author Contributions
YL and S-YC conceptualized and designed the experiments and participated in data interpretation and manuscript preparation. YL, HF, and JL performed the experiments and participated in data analysis. FY, LL, WF, and H-GZ participated in data interpretation and discussion. All authors reviewed the manuscript.
Funding
This work was supported by the National Institutes of Health Grants AA028435, AA021434, AA024337 (S-YC), and AA023190 (WF) from the National Institute on Alcohol Abuse and Alcoholism. H-GZ was supported by a Research Career Scientist (RCS) award.
Conflict of Interest
The authors declare that the research was conducted in the absence of any commercial or financial relationships that could be construed as a potential conflict of interest.
Abbreviations
DNMT, DNA methyltransferase; FASD, Fetal Alcohol Spectrum Disorders; hNCCs, Human neural crest cells; IAP, Inhibitor of apoptosis protein; MSP, Methylation-specific PCR; SFN, Sulforaphane.
References
Ali Khan, M., Kedhari Sundaram, M., Hamza, A., Quraishi, U., Gunasekera, D., Ramesh, L., et al. (2015). Sulforaphane reverses the expression of various tumor suppressor genes by targeting DNMT3B and HDAC1 in human cervical cancer cells. Evid. Based Complement. Alternat. Med. 2015:412149. doi: 10.1155/2015/412149
Avery, J., and Dalton, S. (2016). Methods for derivation of multipotent neural crest cells derived from human pluripotent stem cells. Methods Mol. Biol. 1341, 197–208. doi: 10.1007/7651_2015_234
Basavarajappa, B. S., and Subbanna, S. (2016). Epigenetic mechanisms in developmental alcohol-induced neurobehavioral deficits. Brain Sci. 6:12. doi: 10.3390/brainsci6020012
Bekdash, R. A., Zhang, C., and Sarkar, D. K. (2013). Gestational choline supplementation normalized fetal alcohol-induced alterations in histone modifications, DNA methylation, and proopiomelanocortin (POMC) gene expression in beta-endorphin-producing POMC neurons of the hypothalamus. Alcohol Clin. Exp. Res. 37, 1133–1142. doi: 10.1111/acer.12082
Birnbaum, M. J., Clem, R. J., and Miller, L. K. (1994). An apoptosis-inhibiting gene from a nuclear polyhedrosis virus encoding a polypeptide with Cys/His sequence motifs. J. Virol. 68, 2521–2528. doi: 10.1128/jvi.68.4.2521-2528.1994
Boyanapalli, S. S., and Kong, A. T. (2015). “Curcumin, the King of Spices”: epigenetic regulatory mechanisms in the prevention of cancer, neurological, and inflammatory diseases. Curr. Pharmacol. Rep. 1, 129–139. doi: 10.1007/s40495-015-0018-x
Cartwright, M. M., and Smith, S. M. (1995). Increased cell death and reduced neural crest cell numbers in ethanol-exposed embryos: partial basis for the fetal alcohol syndrome phenotype. Alcohol Clin. Exp. Res. 19, 378–386. doi: 10.1111/j.1530-0277.1995.tb01519.x
Chen, T., Ueda, Y., Dodge, J. E., Wang, Z., and Li, E. (2003). Establishment and maintenance of genomic methylation patterns in mouse embryonic stem cells by Dnmt3a and Dnmt3b. Mol. Cell. Biol. 23, 5594–5605. doi: 10.1128/mcb.23.16.5594-5605.2003
Chen, X., Liu, J., and Chen, S. Y. (2013). Sulforaphane protects against ethanol-induced oxidative stress and apoptosis in neural crest cells by the induction of Nrf2-mediated antioxidant response. Br. J. Pharmacol. 169, 437–448. doi: 10.1111/bph.12133
Chen, X., Liu, J., Feng, W. K., Wu, X., and Chen, S. Y. (2015). MiR-125b protects against ethanol-induced apoptosis in neural crest cells and mouse embryos by targeting Bak 1 and PUMA. Exp. Neurol. 271, 104–111. doi: 10.1016/j.expneurol.2015.04.026
Delvaeye, M., De Vriese, A., Zwerts, F., Betz, I., Moons, M., Autiero, M., et al. (2009). Role of the 2 zebrafish survivin genes in vasculo-angiogenesis, neurogenesis, cardiogenesis and hematopoiesis. BMC Dev. Biol. 9:25. doi: 10.1186/1471-213X-9-25
Dunty, W. C. Jr., Chen, S. Y., Zucker, R. M., Dehart, D. B., and Sulik, K. K. (2001). Selective vulnerability of embryonic cell populations to ethanol-induced apoptosis: implications for alcohol-related birth defects and neurodevelopmental disorder. Alcohol Clin. Exp. Res. 25, 1523–1535. doi: 10.1111/j.1530-0277.2001.tb02156.x
Fan, H., Yuan, F., Yun, Y., Wu, T., Lu, L., Liu, J., et al. (2019). MicroRNA-34a mediates ethanol-induced impairment of neural differentiation of neural crest cells by targeting autophagy-related gene 9a. Exp. Neurol. 320:112981. doi: 10.1016/j.expneurol.2019.112981
Garro, A. J., McBeth, D. L., Lima, V., and Lieber, C. S. (1991). Ethanol consumption inhibits fetal DNA methylation in mice: implications for the fetal alcohol syndrome. Alcohol Clin. Exp. Res. 15, 395–398. doi: 10.1111/j.1530-0277.1991.tb00536.x
Goffredo, D., Rigamonti, D., Zuccato, C., Tartari, M., Valenza, M., and Cattaneo, E. (2005). Prevention of cytosolic IAPs degradation: a potential pharmacological target in Huntington’s Disease. Pharmacol. Res. 52, 140–150. doi: 10.1016/j.phrs.2005.01.006
Gopisetty, G., Ramachandran, K., and Singal, R. (2006). DNA methylation and apoptosis. Mol. Immunol. 43, 1729–1740. doi: 10.1016/j.molimm.2005.11.010
Harada, K., Toyooka, S., Shivapurkar, N., Maitra, A., Reddy, J. L., Matta, H., et al. (2002). Deregulation of caspase 8 and 10 expression in pediatric tumors and cell lines. Cancer Res. 62, 5897–5901.
Haycock, P. C., and Ramsay, M. (2009). Exposure of mouse embryos to ethanol during preimplantation development: effect on DNA methylation in the h19 imprinting control region. Biol. Reprod. 81, 618–627. doi: 10.1095/biolreprod.108.074682
Hervouet, E., Cheray, M., Vallette, F. M., and Cartron, P. F. (2013). DNA methylation and apoptosis resistance in cancer cells. Cells 2, 545–573. doi: 10.3390/cells2030545
Hervouet, E., Vallette, F. M., and Cartron, P. F. (2010). Impact of the DNA methyltransferases expression on the methylation status of apoptosis-associated genes in glioblastoma multiforme. Cell Death Dis. 1:e8. doi: 10.1038/cddis.2009.7
Holcik, M., Thompson, C. S., Yaraghi, Z., Lefebvre, C. A., MacKenzie, A. E., and Korneluk, R. G. (2000). The hippocampal neurons of neuronal apoptosis inhibitory protein 1 (NAIP1)-deleted mice display increased vulnerability to kainic acid-induced injury. Proc. Natl. Acad. Sci. U.S.A. 97, 2286–2290. doi: 10.1073/pnas.040469797
Jensen Pena, C., Monk, C., and Champagne, F. A. (2012). Epigenetic effects of prenatal stress on 11beta-hydroxysteroid dehydrogenase-2 in the placenta and fetal brain. PLoS One 7:e39791. doi: 10.1371/journal.pone.0039791
Jing, W., Song, N., Liu, Y. P., Qu, X. J., Qi, Y. F., Li, C., et al. (2019). DNMT3a promotes proliferation by activating the STAT3 signaling pathway and depressing apoptosis in pancreatic cancer. Cancer Manag. Res. 11, 6379–6396. doi: 10.2147/CMAR.S201610
Jones, P. A., and Baylin, S. B. (2002). The fundamental role of epigenetic events in cancer. Nat. Rev. Genet. 3, 415–428. doi: 10.1038/nrg816
Jones, P. A., and Liang, G. (2009a). Rethinking how DNA methylation patterns are maintained. Nat. Rev. Genet. 10, 805–811.
Jones, P. A., and Liang, G. N. (2009b). OPINION Rethinking how DNA methylation patterns are maintained. Nat. Rev. Genet. 10, 805–811. doi: 10.1038/nrg2651
Kang, K. A., Piao, M. J., Hyun, Y. J., Zhen, A. X., Cho, S. J., Ahn, M. J., et al. (2019). Luteolin promotes apoptotic cell death via upregulation of Nrf2 expression by DNA demethylase and the interaction of Nrf2 with p53 in human colon cancer cells. Exp. Mol. Med. 51, 1–14. doi: 10.1038/s12276-019-0238-y
Koren, G., Nulman, I., Chudley, A. E., and Loocke, C. (2003). Fetal alcohol spectrum disorder. CMAJ 169, 1181–1185.
Kotch, L. E., and Sulik, K. K. (1992). Patterns of ethanol-induced cell death in the developing nervous system of mice; neural fold states through the time of anterior neural tube closure. Int. J. Dev. Neurosci. 10, 273–279. doi: 10.1016/0736-5748(92)90016-s
Leonhardt, H., and Bestor, T. H. (1993). Structure, function and regulation of mammalian DNA methyltransferase. EXS 64, 109–119. doi: 10.1007/978-3-0348-9118-9_5
Li, J., Su, X., Dai, L., Chen, N., Fang, C., Dong, Z., et al. (2019a). Temporal DNA methylation pattern and targeted therapy in colitis-associated cancer. Carcinogenesis 41, 235–244. doi: 10.1093/carcin/bgz199
Li, Y., Yuan, F., Wu, T., Lu, L., Liu, J., Feng, W., et al. (2019b). Sulforaphane protects against ethanol-induced apoptosis in neural crest cells through restoring epithelial-mesenchymal transition by epigenetically modulating the expression of Snail1. Biochim. Biophys. Acta Mol. Basis Dis. 1865, 2586–2594. doi: 10.1016/j.bbadis.2019.07.002
Malekzadeh, K., Sobti, R. C., Nikbakht, M., Shekari, M., Hosseini, S. A., Tamandani, D. K., et al. (2009). Methylation patterns of Rb1 and Casp-8 promoters and their impact on their expression in bladder cancer. Cancer Invest. 27, 70–80. doi: 10.1080/07357900802172085
Marivin, A., Berthelet, J., Plenchette, S., and Dubrez, L. (2012). The inhibitor of apoptosis (IAPs) in adaptive response to cellular stress. Cells 1, 711–737. doi: 10.3390/cells1040711
Meeran, S. M., Patel, S. N., Li, Y., Shukla, S., and Tollefsbol, T. O. (2012). Bioactive dietary supplements reactivate ER expression in ER-negative breast cancer cells by active chromatin modifications. PLoS One 7:e37748. doi: 10.1371/journal.pone.0037748
Menendez, L., Kulik, M. J., Page, A. T., Park, S. S., Lauderdale, J. D., Cunningham, M. L., et al. (2013). Directed differentiation of human pluripotent cells to neural crest stem cells. Nat. Protoc. 8, 203–212. doi: 10.1038/nprot.2012.156
Murphy, T. M., Perry, A. S., and Lawler, M. (2008). The emergence of DNA methylation as a key modulator of aberrant cell death in prostate cancer. Endocr. Relat. Cancer 15, 11–25. doi: 10.1677/Erc-07-0208
Ouko, L. A., Shantikumar, K., Knezovich, J., Haycock, P., Schnugh, D. J., and Ramsay, M. (2009). Effect of alcohol consumption on CpG methylation in the differentially methylated regions of H19 and IG-DMR in male gametes: implications for fetal alcohol spectrum disorders. Alcohol Clin. Exp. Res. 33, 1615–1627. doi: 10.1111/j.1530-0277.2009.00993.x
Qu, X. Q., Proll, M., Neuhoff, C., Zhang, R., Cinar, M. U., Hossain, M. M., et al. (2015). Sulforaphane epigenetically regulates innate immune responses of porcine monocyte-derived dendritic cells induced with lipopolysaccharide. PLoS One 10:e0121574. doi: 10.1371/journal.pone.0121574
Robertson, K. D., Uzvolgyi, E., Liang, G., Talmadge, C., Sumegi, J., Gonzales, F. A., et al. (1999). The human DNA methyltransferases (DNMTs) 1, 3a and 3b: coordinate mRNA expression in normal tissues and overexpression in tumors. Nucleic Acids Res. 27, 2291–2298. doi: 10.1093/nar/27.11.2291
Russell, J. C., Whiting, H., Szuflita, N., and Hossain, M. A. (2008). Nuclear translocation of X-linked inhibitor of apoptosis (XIAP) determines cell fate after hypoxia ischemia in neonatal brain. J. Neurochem. 106, 1357–1370. doi: 10.1111/j.1471-4159.2008.05482.x
Sakharkar, A. J., Tang, L., Zhang, H., Chen, Y., Grayson, D. R., and Pandey, S. C. (2014). Effects of acute ethanol exposure on anxiety measures and epigenetic modifiers in the extended amygdala of adolescent rats. Int. J. Neuropsychopharmacol. 17, 2057–2067. doi: 10.1017/S1461145714001047
Shivapurkar, N., Toyooka, S., Eby, M. T., Huang, C. X., Sathyanarayana, U. G., Cunningham, H. T., et al. (2002). Differential inactivation of caspase-8 in lung cancers. Cancer Biol. Ther. 1, 65–69. doi: 10.4161/cbt.1.1.45
Silke, J., and Meier, P. (2013). Inhibitor of apoptosis (IAP) proteins-modulators of cell death and inflammation. Cold Spring Harb. Perspect. Biol. 5:a008730. doi: 10.1101/cshperspect.a008730
Sokol, R. J., Delaney-Black, V., and Nordstrom, B. (2003). Fetal alcohol spectrum disorder. JAMA 290, 2996–2999. doi: 10.1001/jama.290.22.2996
Su, X., Jiang, X., Meng, L., Dong, X., Shen, Y., and Xin, Y. (2018). Anticancer activity of sulforaphane: the epigenetic mechanisms and the Nrf2 signaling pathway. Oxid. Med. Cell. Longev. 2018:5438179. doi: 10.1155/2018/5438179
Subbanna, S., Nagre, N. N., Umapathy, N. S., Pace, B. S., and Basavarajappa, B. S. (2014). Ethanol exposure induces neonatal neurodegeneration by enhancing CB1R Exon1 histone H4K8 acetylation and up-regulating CB1R function causing neurobehavioral abnormalities in adult mice. Int. J. Neuropsychopharmacol. 18:yu028. doi: 10.1093/ijnp/pyu028
Subbanna, S., Shivakumar, M., Umapathy, N. S., Saito, M., Mohan, P. S., Kumar, A., et al. (2013). G9a-mediated histone methylation regulates ethanol-induced neurodegeneration in the neonatal mouse brain. Neurobiol. Dis. 54, 475–485. doi: 10.1016/j.nbd.2013.01.022
Sui, X., Zhu, J., Zhou, J., Wang, X., Li, D., Han, W., et al. (2015). Epigenetic modifications as regulatory elements of autophagy in cancer. Cancer Lett. 360, 106–113. doi: 10.1016/j.canlet.2015.02.009
Sun, H., Chen, X., Yuan, F., Liu, J., Zhao, Y., and Chen, S. Y. (2014). Involvement of seven in absentia homolog-1 in ethanol-induced apoptosis in neural crest cells. Neurotoxicol. Teratol. 46, 26–31. doi: 10.1016/j.ntt.2014.08.006
Teodoridis, J. M., Strathdee, G., and Brown, R. (2004). Epigenetic silencing mediated by CpG island methylation: potential as a therapeutic target and as a biomarker. Drug Resist. Updat. 7, 267–278. doi: 10.1016/j.drup.2004.06.005
Uysal, F., Akkoyunlu, G., and Ozturk, S. (2015). Dynamic expression of DNA methyltransferases (DNMTs) in oocytes and early embryos. Biochimie 116, 103–113. doi: 10.1016/j.biochi.2015.06.019
Wei, D., Yu, G., and Zhao, Y. (2019). MicroRNA-30a-3p inhibits the progression of lung cancer via the PI3K/AKT by targeting DNA methyltransferase 3a. Onco. Targets Ther. 12, 7015–7024. doi: 10.2147/OTT.S213583
Weiss, K. H., Runz, H., Noe, B., Gotthardt, D. N., Merle, U., Ferenci, P., et al. (2010). Genetic analysis of BIRC4/XIAP as a putative modifier gene of Wilson disease. J. Inherit. Metab. Dis. 33(Suppl. 3), S233–S240. doi: 10.1007/s10545-010-9123-5
Weissman, J., Naidu, S., and Bjornsson, H. T. (2014). Abnormalities of the DNA methylation mark and its machinery: an emerging cause of neurologic dysfunction. Semin. Neurol. 34, 249–257. doi: 10.1055/s-0034-1386763
West, T., Stump, M., Lodygensky, G., Neil, J. J., Deshmukh, M., and Holtzman, D. M. (2009). Lack of X-linked inhibitor of apoptosis protein leads to increased apoptosis and tissue loss following neonatal brain injury. ASN Neuro. 1:e00004. doi: 10.1042/AN20090005
Wright, C. W., and Duckett, C. S. (2005). Reawakening the cellular death program in neoplasia through the therapeutic blockade of IAP function. J. Clin. Invest. 115, 2673–2678. doi: 10.1172/JCI26251
Wu, Y., Alvarez, M., Slamon, D. J., Koeffler, P., and Vadgama, J. V. (2010). Caspase 8 and maspin are downregulated in breast cancer cells due to CpG site promoter methylation. BMC Cancer 10:32. doi: 10.1186/1471-2407-10-32
Yan, D., Dong, J., Sulik, K. K., and Chen, S. Y. (2010). Induction of the Nrf2-driven antioxidant response by tert-butylhydroquinone prevents ethanol-induced apoptosis in cranial neural crest cells. Biochem. Pharmacol. 80, 144–149. doi: 10.1016/j.bcp.2010.03.004
Yuan, F., Chen, X., Liu, J., Feng, W., Cai, L., Wu, X., et al. (2018). Sulforaphane restores acetyl-histone H3 binding to Bcl-2 promoter and prevents apoptosis in ethanol-exposed neural crest cells and mouse embryos. Exp. Neurol. 300, 60–66. doi: 10.1016/j.expneurol.2017.10.020
Yuan, F., Chen, X., Liu, J., Feng, W., Wu, X., and Chen, S. Y. (2017). Up-regulation of Siah1 by ethanol triggers apoptosis in neural crest cells through p38 MAPK-mediated activation of p53 signaling pathway. Arch. Toxicol. 91, 775–784. doi: 10.1007/s00204-016-1746-3
Yuan, F., Yun, Y., Fan, H., Li, Y., Lu, L., Liu, J., et al. (2020). MicroRNA-135a protects against ethanol-induced apoptosis in neural crest cells and craniofacial defects in zebrafish by modulating the siah1/p38/p53 pathway. Front. Cell Dev. Biol. 8:1058.
Zahnow, C. A., Topper, M., Stone, M., Murray-Stewart, T., Li, H., Baylin, S. B., et al. (2016). Inhibitors of DNA methylation, histone deacetylation, and histone demethylation: a perfect combination for cancer therapy. Adv. Cancer Res. 130, 55–111. doi: 10.1016/bs.acr.2016.01.007
Zhong, L., Zhu, J., Lv, T., Chen, G., Sun, H., Yang, X., et al. (2010). Ethanol and its metabolites induce histone lysine 9 acetylation and an alteration of the expression of heart development-related genes in cardiac progenitor cells. Cardiovasc. Toxicol. 10, 268–274. doi: 10.1007/s12012-010-9081-z
Keywords: sulforaphane, ethanol, apoptosis, DNA methylation, IAP proteins, neural crest cells
Citation: Li Y, Fan H, Yuan F, Lu L, Liu J, Feng W, Zhang H-G and Chen S-Y (2021) Sulforaphane Protects Against Ethanol-Induced Apoptosis in Human Neural Crest Cells Through Diminishing Ethanol-Induced Hypermethylation at the Promoters of the Genes Encoding the Inhibitor of Apoptosis Proteins. Front. Cell Dev. Biol. 9:622152. doi: 10.3389/fcell.2021.622152
Received: 27 October 2020; Accepted: 20 January 2021;
Published: 09 February 2021.
Edited by:
Marta Magarinos, Autonomous University of Madrid, SpainReviewed by:
Laura Maria Frago, Autonomous University of Madrid, SpainJuan Antonio Montero, University of Cantabria, Spain
Copyright © 2021 Li, Fan, Yuan, Lu, Liu, Feng, Zhang and Chen. This is an open-access article distributed under the terms of the Creative Commons Attribution License (CC BY). The use, distribution or reproduction in other forums is permitted, provided the original author(s) and the copyright owner(s) are credited and that the original publication in this journal is cited, in accordance with accepted academic practice. No use, distribution or reproduction is permitted which does not comply with these terms.
*Correspondence: Shao-Yu Chen, c2hhb3l1LmNoZW5AbG91aXN2aWxsZS5lZHU=
†These authors have contributed equally to this work