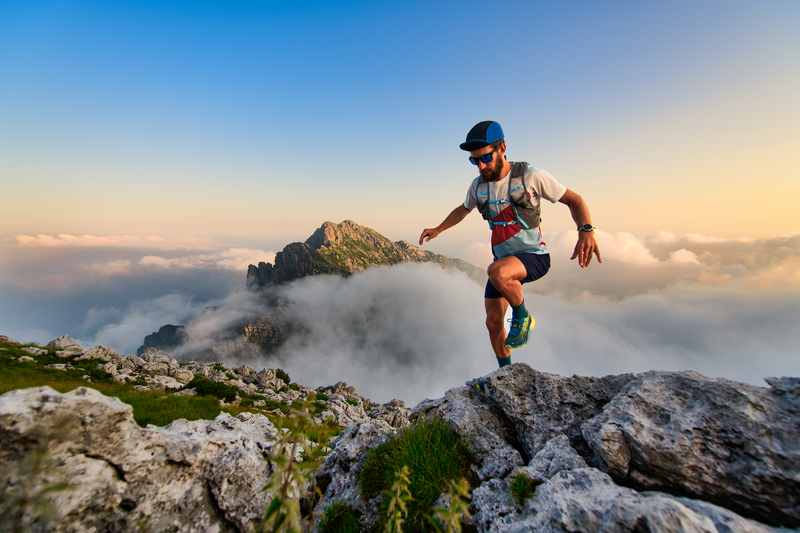
94% of researchers rate our articles as excellent or good
Learn more about the work of our research integrity team to safeguard the quality of each article we publish.
Find out more
ORIGINAL RESEARCH article
Front. Cell Dev. Biol. , 18 March 2021
Sec. Developmental Epigenetics
Volume 9 - 2021 | https://doi.org/10.3389/fcell.2021.621308
This article is part of the Research Topic Early Life Stress and Developmental Programming of Immune and Nervous System Responsivity View all 7 articles
Severe early life stress has long been associated with neuropsychological disorders in adulthood, including depression, schizophrenia, post-traumatic stress disorder, and memory dysfunction. To some extent, all of these conditions involve dysregulation of the hypothalamic-pituitary-adrenal (HPA) axis and reduced negative feedback inhibition of cortisol release in adulthood. However, the time course for mental health and hormonal outcomes across life stages and the attributes of early life stress that direct the behavioral and biological alterations is not fully understood. We designed our studies to compare outcomes of the two most common maternal deprivation schedules on cognitive ability prior to adulthood. We exposed rat pups to daily or randomly spaced maternal separation bouts within the first 3 weeks of life and examined cognitive performance, neurotrophic signaling, and stress and immune system markers during puberty. We found that the daily separation schedule impaired spatial learning while the randomly spaced schedule did not alter maze performance relative to normally reared control animals. Animals that underwent daily separation showed a tendency for reduced body weight compared to the randomly spaced condition, but there were no differences in adrenal weight. Thymus weight normalized by body weight was increased following daily separation compared to random separation and control conditions. Plasma corticosterone levels measured after behavior testing did not differ amongst experimental groups and there was no impact of TrKB receptor inhibition. Combined, the results show that different early life stress schedules produce different behavioral and biological outcomes when measured at puberty. Combined with prior findings from more mature animals, the results presented here suggest that daily neonatal stress produces varied alterations in spatial cognition at different life stages with a transient learning deficit at puberty preceding a more persistent and a progressive memory impairment through adulthood and into aging.
Survivors of early life abuse frequently suffer neuropsychological disorders in adulthood, including depression, schizophrenia, post-traumatic stress disorder, and memory dysfunction. All of these conditions are linked to dysregulation of the hormonal stress response, the hypothalamic-pituitary-adrenal (HPA) axis, with depression and memory loss more specifically associated with chronic increases in serum cortisol (corticosterone in rodents, abbreviated CORT). Maternal deprivation (MD) in animal models supports the notion that early life stress sets up a positive feedback loop whereby lasting elevations in CORT during development impair systems that regulate CORT release later in life (Stanton et al., 1988; Champagne, 2008). These cognitive and HPA impairments can result, in part, from atrophy and impaired function of the hippocampus (Huot et al., 2002; Li et al., 2010). Specifically, dendritic atrophy, disrupted synaptic plasticity, reduced neurogenesis (Karten et al., 2005), and progressive neuron loss (Brunson et al., 2005).
Rodent MD involves removing rat pups from the home cage for varying numbers of hours per day and varying numbers of days during the first three postnatal weeks, with outcomes measured in adulthood (Meaney et al., 1991; Plotsky and Meaney, 1993; van Oers, 1998; Aisa et al., 2007; Daskalakis et al., 2011; Suri et al., 2013). Many early life stress studies in rats can be more broadly classified as one of two MD paradigms, daily or intermittent/pseudo-random (Tractenberg et al., 2016). In the daily MD model, separation occurs every day, typically during the first postnatal week (Plotsky and Meaney, 1993; Kioukia-Fougia, 2002; Weaver et al., 2007). In this model, consistent findings include reductions in hippocampal expression levels for the glucocorticoid receptor (GR) (Ladd et al., 2004; Seo et al., 2020) and the neuroprotective factor, brain derived neurotrophic factor (BDNF) (Seo et al., 2016; Park et al., 2018), accompanied by hippocampal atrophy (Sapolsky, 1987), adrenal hyperplasia (Ulrich-Lai et al., 2006; Herman et al., 2016), excessive CORT release (Ulrich-Lai et al., 2006; Herman et al., 2016), thymus atrophy (Ulrich-Lai et al., 2006; Herman et al., 2016), and a long-term spatial memory deficit in adulthood (Brunson et al., 2005; Li et al., 2006; Herman et al., 2016). For the intermittent/pseudo-randomly spaced MD model, separation is not daily and does not occur at regular intervals (van Oers, 1998; Lehmann et al., 1999; Weaver et al., 2007). In this model, reductions in basal CORT (Kuhn and Schanberg, 1998; Daskalakis et al., 2011), increased sensitivity to glucocorticoid negative feedback (Kuhn and Schanberg, 1998; De Kloet et al., 2005; Daskalakis et al., 2011), increased adaptability to social settings (De Kloet et al., 2005; Oomen et al., 2010), and improved emotional resilience are evident (Price and Steckler, 2011; Schmidt et al., 2011). Because these different separation schedules have been employed independently, contrasting reports could result from any number of undocumented variables. Moreover, prior work has focused on outcome in middle aged and older rats, preventing a more complete understanding of the progressive alterations to immune, hormonal, and neural processes that lead to adult memory loss.
The reduced negative feedback of CORT release following daily MD stems from hypermethylation of the neuron-specific GR promoter, NR3C (Weaver et al., 2004), and a reduction in the hippocampal GRs (Anisman et al., 1998). A loss of hippocampal GRs and reduced negative feedback regulation of circulating CORT promotes synaptic depression, hippocampal atrophy, memory impairment, and psychological depression (McEwen et al., 1997; Kim and Diamond, 2002; Xiong and Krugers, 2015). Additionally, daily MD produces a reduction in the expression of BDNF in adulthood (Lippmann et al., 2007; Chourbaji et al., 2010; O’Sullivan et al., 2011; Lee et al., 2012). Impaired BDNF signaling results in a loss of a critical factor supporting late phase long-term potentiation of synaptic efficacy, dendritic growth, neurogenesis, and long-term memory (McEwen et al., 2015). Reductions in hippocampal GR and BDNF levels due to early life stress likely increase susceptibility to hippocampal related impairments later in life (Heim et al., 2004; Suri et al., 2013) and highly similar biological findings have emerged from human studies (Caffey and Silbey, 1960; Bonanno et al., 2002). A better understanding of alterations in CORT and BDNF signaling through adolescence might bring greater clarity to how the adult cognitive disability emerges and could reveal biological markers of impending memory failure.
The few experiments that examined effects of MD at varying testing ages have shown that impacts on cognitive ability are not immediately observed (Workel et al., 2001; Pardon and Rattray, 2008; Nishi et al., 2013) and do not emerge until well into adulthood (Brunson et al., 2005; Zalosnik et al., 2014; Shu et al., 2015). The delayed onset of symptoms supports the notion that most, if not all of the health problems produced by early life stress may be substantially alleviated with targeted prophylactic therapy through identification of behavioral and biological markers in adolescents and young adults. In an attempt to discover early behavioral and biological markers for impending memory impairment and to better understand the impact of stress schedule, we applied daily or randomly spaced MD to neonatal rats and measured outcomes at puberty and compared behavioral, hormonal, immune, and health outcomes. We discovered that daily MD impaired spatial learning, while randomly spaced MD had no effect. Interestingly, while behavioral differences were observed, no alterations in plasma CORT levels were observed after behavior testing. Adrenal and thymus weights were unaltered, but body weight tended to be reduced following daily MD. These results further dissociate the daily and randomly spaced MD paradigms and reveal a potential behavioral marker in pubertal subjects for impending memory dysfunction in adulthood as a result of early life stress.
Long-Evans hooded rats bred in-house were for this study. Breeder males were obtained from Charles River and breeder females were bred in-house. Male offspring were used as test subjects because puberty related changes in the levels of reproductive hormones known to modulate hippocampal anatomy (Woolley and McEwen, 1993) and spatial cognition (Berry et al., 1997; Warren and Juraska, 1997; Korol et al., 2004) occur over a shorter timespan in female than in male rats (Ojeda and Skinner, 2006) and overlap to a greater extent with the experimental manipulations and test. Female offspring remained in the home cage with the dam at all times to buffer the dam from the stress of pup removal. Also, only the first litter from each dam was used for this study to control for effects of maternal history on dam-pup interactions. Day of birth was designated as postnatal day (P) 0. Litters were culled to 12 pups on P1, retaining even numbers of male and female rats when possible and litters of less than six pups were not used. Whole litters were randomly assigned to either MD condition or control on P1.
All animals were housed in individually ventilated laboratory rat cages (height: 20.3 cm; length: 30.5 cm; width: 35.5), under a 12 h light/dark cycle (lights on at 7:00 a.m.), and in a breeding colony with controlled temperature (23 ± 2°C) and humidity (30–70% relative humidity). Fresh food pellets (7012 Harlan Teklad LM-485, Fredrick, MD) and drinking water were available ad libitum. All experiments were conducted in accordance with the guidelines specified by the National Institutes of Health (NIH Guide for the Care and Use of Laboratory Animals; (NIH Publications No. 8023, revised 1978) and approved by the Institutional Animal Care and Use Committee of George Mason University.
Figure 1 summarizes the timeline for all experimental procedures. Following MD, male and female offspring were weaned at P22. From this point forward, females were group housed and males were individually housed. Test subjects were handled between MD and cannulation surgery at P30 followed by mock injections following cannulation surgery to desensitize the animals to the cannulation restraint prior to behavior testing. At P42, testing in the Morris water maze (MWM) ensued and drug (K252a, tyrosine receptor kinase B (TrKB) inhibitor) or vehicle was delivered directly to the hippocampus on the final day prior to the long-term memory test (the 24 h probe trial, 24-HP). Following behavior testing, subjects were euthanized and tissues were harvested. Each individual event is described below.
Figure 1. Event timeline. The onsets and durations for all experimental events are depicted. All animals were handled briefly on P23–28. Mock injections were performed on P34–39. Drug (K252a) or ACSF was infused 1 h prior to the 24-HP. Tissues were harvested after the 24-HP. IMM, Immediate probe trial performed on the same day as training.
Test subjects were separated from the dam according to two schedules. For daily MD (labeled P2–11), animals were separated from the dam once per day for ten consecutive days from P2 to P11. The random assignment group (labeled RAN) experienced the same number of separation days but spaced from P2 to P20. MD days for the RAN litters were determined by an on-line random number generator (random.org). Additional requirements for the randomization were that at least three of the separation days fall between P2 and P7 and that there were no more than three consecutive separation days anywhere on the schedule. The control litters (labeled CON) were left completely undisturbed until weaning except for a single cage change by the experimenter at ∼P12.
On the first day of MD, the dam from a single litter was removed to a separate transfer cage, male pups were removed to a different transfer cage, and female pups were removed to a new, clean temporary home cage before the dam was reunited with the female pups in the new cage. The dam and female pups remained in the housing room, while the male pups were brought to an adjacent room and individually placed in plexiglass isolation chambers without bedding (15 cm L × 15 cm W × 10 cm H) for 3 h per session. For pups in the RAN group, MD that occurred after P14 required the use of clear, plexiglass cage covers to prevent escape. Pups in all separation groups were warmed with an infrared heat lamp placed over the isolation chambers. The side of the chamber closest to the lamp was maintained at 32°C, the middle of the chamber at 30°C and the farthest point at 28°C. To mask vivarium noise and the sounds of the other pups, white noise was played through speakers at 75–80 dB as verified by an iPhone digital noise meter (Decibel Meter Pro). At the end of the 3 h period, male pups were put back into their transfer cage and returned to the housing room. The dam was moved to a temporary transfer cage while male pups were moved back to the permanent home cage at which time the dam was returned to the permanent home cage. After weaning on P22, male pups were singly housed and handled daily for 3 min per day on P23–28.
At P30, animals underwent stereotaxic surgery under Isoflurane anesthesia to implant bilateral indwelling guide cannulae. A mid-sagittal incision approximately 1.5 cm in length was made and the wound was held open with locking forceps. The fascia was scraped from the skull with the sharp edge of a pair of forceps and the skull was sterilized with hydrogen peroxide (3%) and ethyl alcohol (70%). Three small screws (MX-0080-02F-C, Small Parts, Amazon Inc.) were inserted into burr holes positioned around the intended cannula insertion zones to serve as anchors. Two burr holes were drilled at 3.6 mm posterior from bregma and ± 2.5 mm from the midline. One cannula (stainless steel hypodermic tubing, 26ga, Small Parts, Miramar, FL) was lowered into each hole simultaneously to 3.0 mm below dura so that the tips of both cannulae sat approximately 1 mm above the apex of the DG in both hippocampi (Dumas et al., 2010). Figure 2 shows a thin section from the brain of an animal with bilateral cannulae implants. Stylets extended approximately 0.5 mm beyond the tips of the cannulae and were left in place until mock injections were performed. Cannulae were cemented into place with dental cement (Grip Cement, Dentsply International Inc., Milford, DE). Once the dental cement had set, the scalp wound was clipped closed around the cement headcap and treated with topical antibiotic (bacitracin, Neosporin, Johnson & Johnson, New Brunswick, New Jersey). Rats were weighed prior to surgery and their tails were tattooed with a unique identifier during the surgery. Post-surgery, rats were given ketoprofen (5 mg/kg) subcutaneously two times a day for 3 days. Rats were allowed 10 days to recover from surgery prior to behavior testing. From P34 to P39, animals underwent brief head restraint and mock cannula insertions.
Figure 2. Image of a sagittal brain section showing cannula placement. Red arrows designate the most ventral extents of the cannulae tracts. The triangular notch in the right hemisphere designates brain orientation.
The selective tyrosine receptor kinase B (TrKB) inhibitor, K252a (Sigma Aldrich, St. Louis, MO) was diluted to 31 ng/μl in artificial cerebral spinal fluid (ACSF in mM: NaCl 124, KCl 2, MgSO4 2, CaCl2 2, KH2PO4 1.25, NaHCO3 26, and glucose 10, pH 7.4). This drug and dose were chosen to match other BDNF signaling and memory studies using rats of nearly the same age (Whitfield et al., 2011; Wang et al., 2012; Ju et al., 2015). One hour prior to the 24-HP probe (on P43), animals were infused with either K252a or artificial cerebrospinal fluid (ACSF) at a rate of 0.3 μL per minute for a total of 0.8 μL per hemisphere. Canulae remained in place for 2 min following infusion to optimize drug diffusion.
The Morris water maze (MWM) was used to test spatial learning and memory. A black circular pool (1.7 m in diameter) was filled with tap water (23–24°C) to 10 cm below the rim of the pool, a level that covered a stationary escape platform (17.5 cm diameter) by 1 cm. White curtains displaying black spatial cues surrounded the pool (Figure 3). MWM procedures were followed as described previously (Dumas et al., 2010) except that the water was made opaque with non-toxic white paint and the escape platform was covered with white, non-slip, rubber shelf-liner. A video camera (Model WV-CP280-N, 1/3″ CCD, Panasonic) was positioned approximately four feet above the center of the pool and video recording equipment (a PC with ULead Video Studio, Corel) was located 1.5–2.5 m from the pool behind the curtains surrounding the pool. Transfer cages were kept in a biocontainment hood on an opposite wall ∼ 4.5 m from the pool.
Figure 3. MWM and depiction of spatial analyses. The escape platform is just barely visible in the Goal quadrant (with dotted circle). Spatial cues can be seen attached to curtains that surround the pool. Boxed letters indicate the pseudo-randomized starting locations used during training and probe trials. Bold boxes indicate potential start locations for probe trials for the platform location shown in the figure. Dashed gray lines indicate the boundaries of the four quadrants (Goal, two Adjacent, and Opposite). Distance to platform (DtP) analysis is conducted by noting the linear distance between the center of the rat and the platform center (D) in video snapshots separated by 1 s (tx).
Escape training was performed in six blocks of three trials on a single training day (massed training), with a 15–30 min inter-block interval. The goal quadrant remained stationary throughout training but was varied across testing cohorts. Four starting locations, offset from the platform locations by 45°, were equally spaced around the pool (Figure 3). The starting location for each trial was chosen pseudo-randomly maintaining roughly equal representation of each starting location. Just prior to training, each rat was positioned to climb onto the escape platform three times from different directions. The first block of training trials immediately followed climbing practice. For each training trial, the animal was allowed a maximum of 1 min to find the platform. At the end of each trial, a fifteen second latency was imposed for the rat to spend on the platform. If the rat was unable to find the platform after 1 min, it was gently guided to the platform where it would remain for 15 s. After the sixth training block, an immediate probe trial (IMM) was performed in which the platform was removed, and the rat was allowed to explore the maze for 1 min. The platform was then replaced, and three more refresher trials were performed (not included in data analyses). Twenty-four hours after the IMM probe, the rat was placed in the MWM for a second, 1-min probe trial (24-HR probe).
Following anesthesia (Isoflurane, >5% vapor) and before perfusion, adrenal and thymus glands were removed, placed on filter papers, and individually weighed. The animal was then perfused with 4% paraformaldehyde, the headcap was gently removed, and the brain was extracted.
Blood was collected 30 min after completion of the 24-HR probe either from decapitation during fresh tissue collection or by nicking the renal artery prior to perfusion. This time point was chosen because prior studies have shown that, while the exact time for CORT levels to peak following an acute stressor may vary somewhat, CORT remains significantly elevated at 30 min (Kim et al., 1998; Zimomra et al., 2011; Gil-Lozano et al., 2013). One half of each litter had blood collected from the renal artery prior to perfusion, and the other half from the trunk post decapitation. Blood was immediately centrifuged at 10,000 rpm at 4°C for 5 min. Plasma was then poured off into fresh Eppendorf tubes and stored at −20°C until assayed with a corticosterone ELISA kit (Enzo life sciences ADI-900-097, Farmingdale, NY).
For each training trial in the water maze, latency to escape and path length were measured. For each probe trial, the amount of time spent in each quadrant and distance to platform center (DtP, linear distance between the nose of the animal and the platform center sampled at 1 Hz) were analyzed (Figure 3). Proximity analyses like DtP have been shown to better separate learning impaired from normal subjects (Maei et al., 2009; Pereira and Burwell, 2015; Kapadia et al., 2016).
Repeated measures ANOVA were applied to the escape learning curves (repeating across trial blocks) and DtP measurement during the probe trials (repeating across seconds). None of the data passed Mauchly’s sphericity test, so the Greenhouse-Geisser correction was applied. Chi-squared tests were used to identify likelihood trends for categorical data, as determined by quadrant preference for both the IMM and 24-HR probes. ANOVAs were used to identify stress schedule effects on plasma CORT levels and tissue weights followed by Tukey post hoc tests. Data were averaged within litter prior to analysis or data were averaged within animal and litter number was added as a random factor, where appropriate.
A total of 21 litters were used for this study (P2–11: 4; RAN: 6; CON: 11). Figure 4A shows mean escape latency for each MD condition at each block of training. Litter mean escape latency decreased significantly across training blocks [F(2.606, 46.902) = 79.634, p < 0.001; Greenhouse-Geisser correction for non-sphericity], and there was no significant main effect of MD condition [F(2) = 2.789, p = 0.088]. There was no difference in escape learning between groups, suggesting no impairment in escape learning or performance selective to condition.
Figure 4. Summary of MD effects on escape learning in the MWM. (A) Litter mean escape latency in seconds for each training block across MD conditions. Sample sizes: CON = 11; P2–11 = 4; RAN = 6. (B) Bar graphs showing litter mean quadrant dwell time for each MD condition during the IMM probe trial. Asterisks denote significant Chi Square tests for quadrant (∗∗∗p < 0.001, ∗∗p < 0.01). (C) Each point marks the litter’s average distance between the animal and the platform location (DtP) across the first fifteen seconds of the IMM probe for each MD condition. The asterisk denotes significant RMANOVA main effect of MD condition (p < 0.05). The pound symbol (#) denotes significant Tukey effect of MD condition (p < 0.05). Sample sizes: CON = 11; P2–11 = 4; RAN = 6. The open circle on the Y-axis marks the starting location distance for all animals (120 cm).
For the IMM probe trial performed on the same day as the training trials, mean dwell time per quadrant was calculated for each litter’s MD group (Figure 4B). Chi Square tests and distribution shape indicated a goal quadrant bias for CON and P2–11 groups; CON [χ2(3, 11) = 33.000, p < 0.0001], RAN [χ2(3, 6) = 7.333, p = 0.0620], P2–11 [χ2(3, 4) = 12.000, p < 0.01]. We also analyzed the litter’s distance to the platform location (DtP) at each second for the first 15 s of the IMM probe trial to examine platform approach behavior. This measure has been compared to other measures of spatial accuracy and shown to be a more powerful tool in separating groups with varying spatial abilities (Maei et al., 2009; Pereira and Burwell, 2015; Kapadia et al., 2016). A two-way repeated measures ANOVA of litter means revealed main effects of sample time [F(2.653, 47.749) = 31.326, p < 0.001; Greenhouse-Geisser correction] and MD condition [F(2, 18) = 4.009, p < 0.05] (Figure 4C). Only the litters in the P2–11 group were consistently farther from the platform location than the CON group [Tukey: CON and P2–11: p < 0.05; p < 0.05 for second 7, p < 0.06 for seconds 6, 11; RAN and CON: p = 0.303; RAN and P2: p = 0.920]. These DtP results are not a function of group differences in swim speeds [cm/s across first 15 s ANOVA with litter as a random factor: CON, 13.17 ± 0.52; P2–11, 14.84 ± 0.70; RAN, 12.43 ± 0.89; F(2) = 2.261, p = 0.109]. Overall, these findings indicate that although P2–11 animals spent a similar amount of time in the goal quadrant as the CON and RAN animals, the P2–11 group was less direct in its initial approach to the platform location.
Spatial memory was assessed in the 24-HR probe trial. Typical of massed training protocols (Commins et al., 2003), some forgetting occurred in all groups and there was no clear goal quadrant bias (no significant Chi Square results) for any MD group following delivery of ACSF (Figure 5A, left side) or K252A (Figure 5A, right side). Two-way repeated measures ANOVAs on DtP of litter means indicated no main effect of sample time [F(5.086, 43.229) = 0.423 p = 0.866], or of MD condition [F(2) = 1.162, p = 0.336] following ACSF delivery (Figure 5B, left side) or K252a [time—F(5.926, 44.448) = 0.826, p < 0.555; MD condition—F(2) = 0.045, p = 0.956] (Figure 5B, right side).
Figure 5. Summary of MD effects on spatial memory in the 24 h probe trial. (A) Quadrant dwell time distributions of litter means across MD and drug conditions. (B) Litter distance to platform location across MD and drug conditions. Sample sizes: ACSF (CON = 10; P2–11 = 4; RAN = 6); K252a (CON = 10; P2–11 = 3; RAN = 5). The open circle on the Y-axis marks the starting location distance for all animals (120 cm).
We first compared CORT levels between collection procedures (trunk blood following decapitation or by nicking the renal artery). There were no differences in mean CORT level between different blood collection methods [F(1, 49) = 0.146, p = 0.704]. Therefore, values from each collection method were combined and averaged within experimental group. A two-way ANOVA of litter means revealed no main effect of MD condition [F(2) = 0.082, p = 0.921] or drug treatment [F(1) = 0.233, p = 0.635] on CORT level [F(2) = 0.254, p = 0.778; Figures 6A,B].
Figure 6. Mean plasma CORT levels collected after the 24-HR probe trial across MD and drug conditions. Dots represent individual litter means. (A) CORT levels for animals treated with ACSF before the 24-HR probe trial. (B) CORT levels for animals treated with K252a before the 24-HR probe trial. Sample sizes: ACSF: CON = 4, P2–11 = 4, RAN = 5; K252a: CON = 6, P2–11 = 2, RAN = 4.
Body weight at time of surgery was different between MD conditions when litter was treated as a random factor [F(2, 95) = 21.920, p < 0.001] CON (M = 140.60 g) and RAN (M = 142.23 g) animals weighed more than P2–11 (M = 114.99 g) animals (Tukey; p < 0.001), suggesting less robust physical development after P2–11 MD and more robust development after RAN MD (Figure 7A). There was no effect of MD condition on normalized adrenal gland when litter was treated as a random factor [F(2, 95) = 0.424, p = 0.656)] (Figure 7B) and there was an effect of MD on normalized thymus gland weight when litter was treated as a random factor [F(2, 95) = 4.660, p = 0.012) (Figure 7C). P2–11 thymus was heavier than CON and RAN (Tukey; P2–11 to CON, p < 0.05; P2–11 to RAN, p < 0.01).
Figure 7. Body and tissue weights across MD groups. Dots represent individual litter means. (A) Body weights at time of surgery (P30) compared across MD condition with litter as a random factor. Body weight sample sizes: CON = 58; P2–11 = 24; RAN = 33. (B) Adrenal weight normalized by body weight across MD condition. Adrenal weight sample sizes: CON = 58; P2–11 = 24; RAN = 33. (C) Thymus weight normalized by body weight across MD condition. Thymus weight sample sizes CON = 58; P2–11 = 24; RAN = 33; RAN = 10. Asterisks denote significance (∗∗∗p < 0.001, ∗∗p < 0.01, ∗p < 0.05).
Prior rodent studies of early life stress incorporated MD schedules of varying types and assays performed in midlife (Plotsky and Meaney, 1993; van Oers, 1998; Weaver et al., 2007; Daskalakis et al., 2011; Suri et al., 2013). This study aimed to differentiate the effects of two schedules of MD, daily (P2–11) and random randomly spaced sessions (RAN), on spatial learning and memory ability in pubertal rats. Based on quadrant dwell time for the entire probe trial, RAN animals appear to be less persistent in their goal location search compared to CON and P2–11 subjects. However, this traditional measure is subject to off-target searching later in the probe trial. Compared to CON and RAN animals, the P2–11 schedule produced an impairment in the initial trajectory and proximity to the goal location. These measures have been shown to be better separators of spatial learning (IMM probe) and memory (24-HR probe) abilities across testing groups in MWM probe trials (Maei et al., 2009; Pereira and Burwell, 2015; Kapadia et al., 2016). There was a trend for an effect in the RAN group (p = 0.06), but the initial trajectory for this group aligns almost exactly with the CON group. Thus, the platform approach deficit observed when MD occurs from P2 to P11 is due to more frequent MD episodes during the first two postnatal weeks or the daily nature of the MD. Conversely, the lack of an approach deficit in the RAN group may have resulted from less intense MD during the first two postnatal weeks or the random spacing of the MD. There was no effect of MD schedule or K252A on 24-HR probe performance suggesting no impact neonatal stress on spatial memory or BDNF/TrKB signaling at this life stage. Future experiments will need to address the remaining outstanding variable of total age range of MD bouts (10 versus 20 days) and employ additional cognitive measures to better define the degree (spaced training in the MWM) and specificity (non-spatial learning and memory tasks) of the spatial learning impairment following daily MD. This study suffered somewhat from a low N that was mitigated in part by the reduced variability gained by averaging pup data within a litter. Larger litter numbers per group would add more statistical power and enable more concrete conclusions.
P2–11 animals weighed significantly less than RAN, but not CON subjects. These results suggest that growth reduction due to daily neonatal stress may begin at or near puberty. When normalized by body weight, the wet weights of the adrenal and thymus glands were unaltered, supporting the idea that the adrenal hyperplasia and thymus atrophy observed in adulthood emerge after puberty (Margarinos and McEwen, 1995; Ulrich-Lai et al., 2006).
The effects of early life stress on spatial learning are schedule-dependent and may differ when viewed at puberty (this study) versus adulthood (prior studies) (Figure 8). While caution should be taken when comparing across life stages (because age of testing is not the only difference between studies), the current findings support the need for studies directly comparing across various life stages. Only after the P2–11 separation schedule was spatial learning impaired. Due to the massed training protocol, all groups showed forgetting. A lack of performance differences across conditions suggests that neither of the MD conditions was able to improve long-term memory following massed training. While not performed within the same study, this is in sharp contrast to effects observed in adulthood (Brunson, 2005; Bolton et al., 2017; Chaby et al., 2017). Long-term memory may be more completely understood after a spaced training paradigm that enhances long-term memories. These findings show that the effects of different MD schedules can be differentiated at puberty and support the notion that more consistent early life stress is required to produce cognitive dysfunction at life stages earlier than adulthood. However, it is not clear if the behavioral outcome after MD from P2 to P11 is due to the full 10 consecutive days of MD or some critical number of separation bouts during the first postnatal week (Lehmann and Feldon, 2000; Litvin et al., 2010; Tractenberg et al., 2016; Borges-Aguiar et al., 2018; Orso et al., 2019).
Figure 8. Table listing results from prior studies of MD with daily separation side-by-side with the current study. The Prior Reports column shows the consensus of research studies utilizing a daily (Daily) separation schedule with assays performed in adulthood (Anisman et al., 1998; Huot et al., 2002; Heim et al., 2004; Bartolomucci et al., 2005; Brunson et al., 2005; Greisen et al., 2005; Ladd et al., 2005; Reber et al., 2007; Chourbaji et al., 2010; O’Sullivan et al., 2011; Lee et al., 2012; McEwen et al., 2015; Holubová et al., 2016; Bolton et al., 2017; Chaby et al., 2017; Récamier-Carballo et al., 2017). The Current Study columns summarize the results from the P2–11 and RAN separation schedules. Arrows indicate direction of change in each parameter relative to non-stressed controls. No Change indicates that there is no difference between stressed and non-stressed controls. No Consensus indicates that there is no clear agreement in the literature. TBD indicates “to be determined.”
Inclusion of only male subjects polarizes the CORT data as females show a differential CORT response to early life stress at puberty (Romeo et al., 2004; Hodes and Epperson, 2019). Specifically, intact females exhibit an extended CORT response whereas males more rapidly return to baseline after an acute stressor. This manifests in the males showing greater resilience to acute stress during adolescence and adulthood while females exhibiting more persistent, negative behavioral effects (Bourke and Neigh, 2011). Moreover, gender differences in MD responsiveness (Weaver et al., 2007; Oreland et al., 2009) warrant replication of this study on female rats.
Baseline CORT is highly variable amongst laboratory rats housed under the same conditions (García and Armario, 2001). By clamping this natural variability, via adrenalectomy with CORT replacement, or by employing within-animal CORT comparisons before and after MWM testing, effects on plasma CORT might have been revealed. As well, collecting blood at multiple time points following maze exposure to analyze CORT might have revealed more information about the changes in CORT over time and the reason for low CORT levels in this particular study. In addition, many studies have shown that handling of pups during their juvenile period, which we did, may result in decreased anxiety and a more positive reaction to later stressors (Chapillon et al., 2002), including altering of the corticosterone response (Levine et al., 1967; Meaney et al., 1985; Levine, 2000). These factors may have reduced the overall impact of MD in our study.
Finally, maternal effects due to the use of first litters instead of those from seasoned dams may have also influenced the outcome (Pawluski et al., 2009). Significant increases in CORT are found from P1 in primi- versus multiparous females (Grajeda and Pérez-Escamilla, 2002; Pawluski et al., 2009) and is correlated with increased licking and grooming in rats (Pawluski et al., 2009), though the differences in CORT and maternal care between primi- and multiparous females disappears by P14. It may be informative to further investigate connections between BDNF and CORT in regulating the progression of cognitive alterations following early life stress by measuring BDNF and CORT levels at numerous timepoints leading up to puberty.
One of the important aspects of this study is the identification of a uniquely timed stressor and its effects on the adult stress response. Most studies that look at the effects of MD focus on the first two postnatal weeks rather than the entire juvenile period (Wang et al., 2020). Some studies examine manipulations occurring daily for the first three postnatal weeks (Shimada et al., 1990; Meerlo et al., 1999; Pryce et al., 2001, 2003; Kosten et al., 2006). One study of maternal deprivation through P21 reported enhanced spatial learning in the MWM when deprived subjects were tested at 3–6 months of age (Pryce et al., 2003). Given variations in adult behavior based on frequency of MD bouts, total age span of MD bouts, and age of testing, larger studies directly comparing across these variables are needed to gain a more complete perspective on the lifespan impacts of MD. Moreover, while the relevance of animal studies to human MD equivalents is clear (Bolton et al., 2017), this field would benefit from early life stress models that more closely approximate human development. Randomized MD may provide greater insight into the lasting effects of more persistent early life stress occurring in an unpredictable manner, which may be more akin to human early life stressors.
This study has illuminated effects of daily MD on stress biology and cognitive ability at a younger age than typically studied. Additional experiments at varying developmental stages are necessary to fully understand the trajectory of the cognitive and health impacts of early life stress. Currently, the spatial learning deficit at puberty may serve as a behavioral marker of future memory failure that can be assessed non-invasively and inexpensively at the human level. Testing of other types of learning and memory might reveal additional markers for impending memory loss and possibly provide a novel platform for prophylaxis aimed at averting not only cognitive dysfunction later in life but also the overwhelming cost of lifetime treatment for childhood abuse. For all individuals identified in a given year, the total cost to society is estimated to run upward of $100 billion dollars per year (Fang et al., 2012). Thus, for both quality of life and economic reasons, it is imperative that we further identify the underlying mechanisms of early life stress that leads to adult disease.
The raw data supporting the conclusions of this article will be made available by the authors without undue reservation.
The animal study was reviewed and approved by the Institutional Animal Care and Use Committee, George Mason University.
ES and TD designed the study. ES, DM, SS-B, NL, CL, JE, and KB collected the data. ES analyzed the data. ES and TD co-wrote the manuscript. ES, DM, SS-B, NL, CL, JE, KB, and TD revised the manuscript. All authors contributed to the article and approved the submitted version.
This work was supported in part by the Thomas and Kate Miller Jeffress Memorial Trust Fund, the Department of Defense (ONR#N00014-10-1-0198), and the National Institutes of Health (1R15AG045820-01A1 and 1R15AG060461-01).
The authors declare that the research was conducted in the absence of any commercial or financial relationships that could be construed as a potential conflict of interest.
We would like to extend sincere appreciation to Drs. Alex Carlisle, Carolina Salvador-Morales, and Nadine Kabbani for sharing equipment and Dr. Daniela Kaufer for critical scientific advising.
Aisa, B., Tordera, R., Lasheras, B., Del Rio, J., and Ramirez, M. J. (2007). Cognitive impairment associated to HPA axis hyperactivity after maternal separation in rats. Psychoneuroendocrinology 32, 256–266.
Anisman, H., Zaharia, M. D., Meaney, M. J., and Merali, Z. (1998). Do early-life events permanently alter behavioral and hormonal responses to stressors? Int. J. Dev. Neurosci. 16, 149–164.
Bartolomucci, A., Palanza, P., Sacerdote, P., Panerai, A. E., Sgoifo, A., Dantzer, R., et al. (2005). Social factors and individual vulnerability to chronic stress exposure. Neurosci. Biobehav. Rev. 29, 67–81. doi: 10.1016/j.neubiorev.2004.06.009
Berry, B., McMahan, R., and Gallagher, M. (1997). Spatial learning and memory at defined points of the estrous cycle: effects on performance of a hippocampal-dependent task. Behav. Neurosci. 111, 267–274.
Bolton, J. L., Molet, J., Ivy, A., and Baram, T. Z. (2017). New insights into early-life stress and behavioral outcomes. Curr. Opin. Behav. Sci. 14, 133–139. doi: 10.1016/j.cobeha.2016.12.012
Bonanno, G. A., Papa, A., and O’Neill, K. (2002). Loss and human resilience. Appl. Preven. Psychol. 10, 193–206.
Borges-Aguiar, A. C., Schauffer, L. Z., de Kloet, E. R., and Schenberg, L. C. (2018). Daily maternal separations during stress hyporesponsive period decrease the thresholds of panic-like behaviors to electrical stimulation of the dorsal periaqueductal gray of the adult rat. Behav. Brain Res. 344, 132–144. doi: 10.1016/j.bbr.2018.02.020
Bourke, C. H., and Neigh, G. N. (2011). Behavioral effects of chronic adolescent stress are sustained and sexually dimorphic. Horm. Behav. 60, 112–120. doi: 10.1016/j.yhbeh.2011.03.011
Brunson, K. L. (2005). Mechanisms of late-onset cognitive decline after early-life stress. J. Neurosci. 25, 9328–9338. doi: 10.1523/JNEUROSCI.2281-05.2005
Brunson, K. L., Kramár, E., Lin, B., Chen, Y., Colgin, L. L., Yanagihara, T. K., et al. (2005). Mechanisms of late-onset cognitive decline after early-life stress. J. Neurosci. 25, 9328–9338. doi: 10.1523/JNEUROSCI.2281-05.2005
Caffey, J., and Silbey, R. (1960). Regrowth and overgrowth of the thymus after atrophy induced by the oral administration of adrenocorticosteroids to human infants. Pediatrics 26, 762–770.
Chaby, L. E., Zhang, L., and Liberzon, I. (2017). The effects of stress in early life and adolescence on posttraumatic stress disorder, depression, and anxiety symptomatology in adulthood. Curr. Opin. Behav. Sci. 14, 86–93. doi: 10.1016/j.cobeha.2017.01.001
Champagne, F. A. (2008). Epigenetic mechanisms and the transgenerational effects of maternal care. Front. Neuroendocr. 29, 386–397. doi: 10.1016/j.yfrne.2008.03.003.Epigenetic
Chapillon, P., Patin, V., Roy, V., Vincent, A., and Caston, J. (2002). Effects of pre- and postnatal stimulation on developmental, emotional, and cognitive aspects in rodents: a review. Dev. Psychobiol. 41, 373–387. doi: 10.1002/dev.10066
Chourbaji, S., Brandwein, C., and Gass, P. (2010). Altering BDNF expression by genetics and/or environment: Impact for emotional and depression-like behaviour in laboratory mice. Neurosci. Biobehav. Rev. 35, 599–611. doi: 10.1016/j.neubiorev.2010.07.003
Commins, S., Cunningham, L., Harvey, D., and Walsh, D. (2003). Massed but not spaced training impairs spatial memory. Behav. Brain Res. 139, 215–223. doi: 10.1016/S0166-4328(02)00270-X
Daskalakis, N. P., Claessens, S. E. F., Laboyrie, J. J. L., Enthoven, L., Oitzl, M. S., Champagne, D. L., et al. (2011). The newborn rat’s stress system readily habituates to repeated and prolonged maternal separation, while continuing to respond to stressors in context dependent fashion. Horm. Behav. 60, 165–176. doi: 10.1016/j.yhbeh.2011.04.003
De Kloet, E. R., Sibug, R. M., Helmerhorst, F. M., and Schmidt, M. (2005). Stress, genes and the mechanism of programming the brain for later life. Neurosci. Biobehav. Rev. 29, 271–281. doi: 10.1016/j.neubiorev.2004.10.008
Dumas, T. C., Gillette, T., Ferguson, D., Hamilton, K., and Sapolsky, R. M. (2010). Anti-glucocorticoid gene therapy reverses the impairing effects of elevated corticosterone on spatial memory, hippocampal neuronal excitability, and synaptic plasticity. J. Neurosci. 30, 1712–1720. doi: 10.1523/JNEUROSCI.4402-09.2010
Fang, X., Brown, D. S., Florence, C., and Mercy, J. A. (2012). The economic burden of child maltreatment in the united states and implications for prevention. Child Abus. Negl. 36, 156–165. doi: 10.1016/j.chiabu.2011.10.006
García, A., and Armario, A. (2001). Individual differences in the recovery of the hypothalamic-pituitary-adrenal axis after termination of exposure to a severe stressor in outbred male sprague-dawley rats. Psychoneuroendocrinology 26, 363–374.
Gil-Lozano, M., Romani-Perez, M., Outeirino-Iglesias, V., Vigo, E., Brubaker, P. L., Gonzalez-Matias, L. C., et al. (2013). Effects of prolonged exendin-4 administration on hypothalamic-pituitary-adrenal axis activity and water balance. AJP Endocrinol. Metab. 304, E1105–E1117. doi: 10.1152/ajpendo.00529.2012
Grajeda, R., and Pérez-Escamilla, R. (2002). Stress during labor and delivery is associated with delayed onset of lactation among urban guatemalan women. J. Nutr. 132, 3055–3060. doi: 10.1093/jn/131.10.3055
Greisen, M. H., Altar, C. A., Bolwig, T. G., Whitehead, R., and Wörtwein, G. (2005). Increased adult hippocampal brain-derived neurotrophic factor and normal levels of neurogenesis in maternal separation rats. J. Neurosci. Res. 79, 772–778. doi: 10.1002/jnr.20418
Heim, C., Plotsky, P. M., and Nemeroff, C. B. (2004). Importance of studying the contributions of early adverse experience to neurobiological findings in depression. Neuropsychopharmacology 29, 641–648. doi: 10.1038/sj.npp.1300397
Herman, J. P., Mcklveen, J. M., Ghosal, S., Kopp, B., Wulsin, A., Makinson, R., et al. (2016). Regulation of the hypothalamic-pituitary-adrenocortical stress response. Compr. Physiol. 6, 603–621. doi: 10.1002/cphy.c150015.Regulation
Hodes, G. E., and Epperson, C. N. (2019). Sex Differences in vulnerability and resilience to stress across the life span. Biol. Psychiatry 86, 421–432. doi: 10.1016/j.biopsych.2019.04.028
Holubová, A., Štofková, A., Jurčovičová, J., and Šlamberová, R. (2016). The effect of neonatal maternal stress on plasma levels of adrenocorticotropic hormone, corticosterone, leptin, and ghrelin in adult male rats exposed to acute heterotypic stressor. Physiol. Res. 65, 557–566.
Huot, R. L., Plotsky, P. M., Lenox, R. H., and McNamara, R. K. (2002). Neonatal maternal separation reduces hippocampal mossy fiber density in adult long evans rats. Brain Res. 950, 52–63. doi: 10.1016/S0006-8993(02)02985-2
Ju, Y. Y., Long, J. D., Liu, Y., and Liu, J. G. (2015). Formation of aversive memories associated with conditioned drug withdrawal requires BDNF expression in the amygdala in acute morphine-dependent rats. Acta Pharmacol. Sin. 36, 1437–1443. doi: 10.1038/aps.2015.94
Kapadia, M., Xu, J., and Sakic, B. (2016). The water maze paradigm in experimental studies of chronic cognitive disorders: theory, protocols, analysis, and inference. Neurosci. Biobehav. Rev. 68, 195–217. doi: 10.1016/j.neubiorev.2016.05.016
Karten, Y. J. G., Olariu, A., and Cameron, H. A. (2005). Stress in early life inhibits neurogenesis in adulthood. Trends Neurosci. 28, 171–172. doi: 10.1016/j.tins.2005.01.009
Kim, D. H., Jung, J. S., Song, D. K., Suh, H. W., Huh, S. O., and Kim, Y. H. (1998). Intracerebroventricular injection-induced increase in plasma corticosterone levels in the mouse: a stress model. J. Pharmacol. Toxicol. Methods 39, 71–73.
Kim, J. J., and Diamond, D. M. (2002). The stressed hippocampus, synaptic plasticity and lost memories. Nat. Rev. Neurosci. 3, 453–462. doi: 10.1038/nrn849
Kioukia-Fougia, N. (2002). The effects of stress exposure on the hypothalamic–pituitary–adrenal axis, thymus, thyroid hormones and glucose levels. Prog. Neuro Psychopharmacol. Biol. Psychiatry 26, 823–830. doi: 10.1016/S0278-5846(01)00297-4
Korol, D. L., Malin, E. L., Borden, K. A., Busby, R. A., and Couper-Leo, J. (2004). Shifts in preferred learning strategy across the estrous cycle in female rats. Horm. Behav. 45, 330–338. doi: 10.1016/j.yhbeh.2004.01.005
Kosten, T. A., Lee, H. J., and Kim, J. J. (2006). Early life stress impairs fear conditioning in adult male and female rats. Brain Res. 1087, 142–150. doi: 10.1016/j.brainres.2006.03.009
Kuhn, C. M., and Schanberg, S. M. (1998). Responses to maternal separation: mechanisms and mediators. Int. J. Dev. Neurosci. 16, 261–270. doi: 10.1016/S0736-5748(98)00034-3
Ladd, C. O., Huot, R. L., Thrivikraman, K. V., Nemeroff, C. B., and Plotsky, P. M. (2004). Long-term adaptations in glucocorticoid receptor and mineralocorticoid receptor mRNA and negative feedback on the hypothalamo-pituitary-adrenal axis following neonatal maternal separation. Biol. Psychiatry 55, 367–375. doi: 10.1016/j.biopsych.2003.10.007
Ladd, C. O., Thrivikraman, K. V., Huot, R. L., and Plotsky, P. M. (2005). Differential neuroendocrine responses to chronic variable stress in adult long evans rats exposed to handling-maternal separation as neonates. Psychoneuroendocrinology 30, 520–533. doi: 10.1016/j.psyneuen.2004.12.004
Lee, K.-Y., Miki, T., Yokoyama, T., Ueki, M., Warita, K., Suzuki, S., et al. (2012). Neonatal repetitive maternal separation causes long-lasting alterations in various neurotrophic factor expression in the cerebral cortex of rats. Life Sci. 90, 578–584. doi: 10.1016/j.lfs.2012.01.021
Lehmann, J., and Feldon, J. (2000). Long-term biobehavioral effects of maternal separation in the rat: consistent or confusing? Rev. Neurosci. 11, 383–408. doi: 10.1515/REVNEURO.2000.11.4.383
Lehmann, J., Pryce, C. R., Bettschen, D., and Feldon, J. (1999). The maternal separation paradigm and adult emotionality and cognition in male and female wistar rats. Pharmacol. Biochem. Behav. 64, 705–715.
Levine, S. (2000). Influence of psychological variables on the activity of the hypothalamic-pituitary-adrenal axis. Eur. J. Pharmacol. 405, 149–160. doi: 10.1016/S0014-2999(00)00548-3
Levine, S., Haltmeyer, G. C., Karas, G. G., and Denenberg, V. H. (1967). Physiological and behavioral effects of infantile stimulation. Physiol. Behav. 2, 55–59. doi: 10.1016/0031-9384(67)90011-X
Li, G., Cherrier, M. M., Tsuang, D. W., Petrie, E. C., Colasurdo, E. A., Craft, S., et al. (2006). Salivary cortisol and memory function in human aging. Neurobiol. Aging 27, 1705–1714. doi: 10.1016/j.neurobiolaging.2005.09.031
Li, X. M., Han, F., Liu, D. J., and Shi, Y. X. (2010). Single-prolonged stress induced mitochondrial-dependent apoptosis in hippocampus in the rat model of post-traumatic stress disorder. J. Chem. Neuroanat. 40, 248–255. doi: 10.1016/j.jchemneu.2010.07.001
Lippmann, M., Bress, A., Nemeroff, C. B., Plotsky, P. M., and Monteggia, L. M. (2007). Long-term behavioural and molecular alterations associated with maternal separation in rats. Eur. J. Neurosci. 25, 3091–3098. doi: 10.1111/j.1460-9568.2007.05522.x
Litvin, Y., Tovote, P., Pentkowski, N. S., Zeyda, T., King, L. B., Vasconcellos, A. J., et al. (2010). Maternal separation modulates short-term behavioral and physiological indices of the stress response. Horm. Behav. 58, 241–249. doi: 10.1016/j.yhbeh.2010.03.010
Maei, H. R., Zaslavsky, K., Teixeira, C. M., and Frankland, P. W. (2009). What is the most sensitive measure of water maze probe test performance? Front. Integr. Neurosci. 3:4. doi: 10.3389/neuro.07.004.2009
Margarinos, A., and McEwen, B. S. (1995). Stress-induced atrophy of apical dendrites of hippocampal CA3c neurons: comparison of stressors. Neuroscience 69, 83–88.
McEwen, B. S., Bowles, N. P., Gray, J. D., Hill, M. N., Hunter, R. G., Karatsoreos, I. N., et al. (2015). Mechanisms of stress in the brain. Nat. Neurosci. 18, 1353–1363. doi: 10.1038/nn.4086
McEwen, B. S., Conrad, C. D., Kuroda, Y., Frankfurt, M., Magarinos, A. M., and McKittrick, C. (1997). Prevention of stress-induced morphological and cognitive consequences. Eur. Neuropsychopharmacol. 7, 323–328.
Meaney, M. J., Aitken, D. H., Bhatnagar, S., and Sapolsky, R. M. (1991). Postnatal handling attenuates certain neuroendocrine, anatomical, and cognitive dysfunctions associated with aging in female rats. Neurobiol. Aging 12, 31–38. doi: 10.1016/0197-4580(91)90036-J
Meaney, M. J., Aitken, D. H., Bodnoff, S. R., Iny, L. J., Tatarewicz, J. E., and Sapolsky, R. M. (1985). Early postnatal handling alters glucocorticoid receptor concentrations in selected brain regions. Behav. Neurosci. 99, 765–770. doi: 10.1037/a0034187
Meerlo, P., Horvath, K. M., Nagy, G. M., Bohus, B., and Koolhaas, J. M. (1999). The influence of postnatal handling on adult neuroendocrine and behavioural stress reactivity. J. Neuroendocrinol. 11, 925–933. doi: 10.1046/j.1365-2826.1999.00409.x
Nishi, M., Horii-Hayashi, N., Sasagawa, T., and Matsunaga, W. (2013). Effects of early life stress on brain activity: implications from maternal separation model in rodents. Gen. Comp. Endocrinol. 181, 306–309. doi: 10.1016/j.ygcen.2012.09.024
Ojeda, S., and Skinner, M. (2006). “Puberty in the Rat,” in Physiology of Reproduction, eds E. Knobil and J. Neill (Amsterdam, NL: Elsevier Inc), 2061–2126.
Oomen, C. A., Soeters, H., Audureau, N., Vermunt, L., Van Hasselt, F. N., Manders, E. M. M., et al. (2010). Severe early life stress hampers spatial learning and neurogenesis, but improves hippocampal synaptic plasticity and emotional learning under high-stress conditions in adulthood. J. Neurosci. 30, 6635–6645. doi: 10.1523/JNEUROSCI.0247-10.2010
Oreland, S., Pickering, C., Gökturk, C., Oreland, L., Arborelius, L., and Nylander, I. (2009). Two repeated maternal separation procedures differentially affect brain 5-hydroxytryptamine transporter and receptors in young and adult male and female rats. Brain Res. 1305, S37–S49. doi: 10.1016/j.brainres.2009.08.069
Orso, R., Creutzberg, K. C., Wearick-Silva, L. E., Wendt Viola, T., Tractenberg, S. G., Benetti, F., et al. (2019). How early life stress impact maternal care: a systematic review of rodent studies. Front. Behav. Neurosci. 13:197. doi: 10.3389/fnbeh.2019.00197
O’Sullivan, E., Barrett, E., Grenham, S., Fitzgerald, P., Stanton, C., Ross, R. P., et al. (2011). BDNF expression in the hippocampus of maternally separated rats: does Bifidobacterium breve 6330 alter BDNF levels? Benef. Microbes 2, 199–207. doi: 10.3920/BM2011.0015
Pardon, M. C., and Rattray, I. (2008). What do we know about the long-term consequences of stress on ageing and the progression of age-related neurodegenerative disorders? Neurosci. Biobehav. Rev. 32, 1103–1120. doi: 10.1016/j.neubiorev.2008.03.005
Park, S. W., Seo, M. K., Lee, J. G., Hien, L. T., and Kim, Y. H. (2018). Effects of maternal separation and antidepressant drug on epigenetic regulation of the brain-derived neurotrophic factor exon I promoter in the adult rat hippocampus. Psychiatry Clin. Neurosci. 72, 255–265. doi: 10.1111/pcn.12609
Pawluski, J. L., Charlier, T. D., Lieblich, S. E., Hammond, G. L., and Galea, L. A. M. (2009). Reproductive experience alters corticosterone and CBG levels in the rat dam. Physiol. Behav. 96, 108–114. doi: 10.1016/j.physbeh.2008.09.004
Pereira, T., and Burwell, R. (2015). Using the spatial learning index to evaluate performance on the water maze. Behav. Neurosci. 129, 533–539. doi: 10.1037/bne0000078.Using
Plotsky, P. M., and Meaney, M. J. (1993). Early, postnatal experience alters hypothalamic corticotropin-releasing factor (CRF) mRNA, median eminence CRF content and stress-induced release in adult rats. Brain Res. Mol. Brain Res. 18, 195–200.
Price, L. H., and Steckler, T. (2011). Editorial: early life stress and psychopharmacology. Psychopharmacology (Berl). 214, 1–3. doi: 10.1007/s00213-011-2217-4
Pryce, C. R., Bettschen, D., and Feldon, J. (2001). Comparison of the effects of early handling and early deprivation on maternal care in the rat. Dev. Psychobiol. 38, 239–251. doi: 10.1002/dev.1018
Pryce, C. R., Bettschen, D., Nanz-Bahr, N. I., and Feldon, J. (2003). Comparison of the effects of early handling and early deprivation on conditioned stimulus, context, and spatial learning and memory in adult rats. Behav. Neurosci. 117, 883–893. doi: 10.1037/0735-7044.117.5.883
Reber, S. O., Birkeneder, L., Veenema, A. H., Obermeier, F., Falk, W., Straub, R. H., et al. (2007). Adrenal insufficiency and colonic inflammation after a novel chronic psycho-social stress paradigm in mice: Implications and mechanisms. Endocrinology 148, 670–682. doi: 10.1210/en.2006-0983
Récamier-Carballo, S., Estrada-Camarena, E., and López-Rubalcava, C. (2017). Maternal separation induces long-term effects on monoamines and brain-derived neurotrophic factor levels on the frontal cortex, amygdala, and hippocampus: differential effects after a stress challenge. Behav. Pharmacol. 28, 545–557. doi: 10.1097/FBP.0000000000000324
Romeo, R. D., Lee, S. J., and McEwen, B. S. (2004). Differential stress reactivity in intact and ovariectomized prepubertal and adult female rats. Neuroendocrinology 80, 387–393. doi: 10.1159/000084203
Sapolsky, R. M. (1987). Glucocorticoids and hippocampal damage. Trends Neurosci. 10, 346–349. doi: 10.1016/S0079-6123(08)63163-5
Schmidt, M. V., Wang, X. D., and Meijer, O. C. (2011). Early life stress paradigms in rodents: potential animal models of depression? Psychopharmacology (Berl). 214, 131–140. doi: 10.1007/s00213-010-2096-0
Seo, M. K., Kim, S. G., Seog, D. H., Bahk, W. M., Kim, S. H., Park, S. W., et al. (2020). Effects of early life stress on epigenetic changes of the glucocorticoid receptor 17 promoter during adulthood. Int. J. Mol. Sci. 21, 1–12. doi: 10.3390/ijms21176331
Seo, M. K., Ly, N. N., Lee, C. H., Cho, H. Y., Choi, C. M., Nhu, L. H., et al. (2016). Early life stress increases stress vulnerability through BDNF gene epigenetic changes in the rat hippocampus. Neuropharmacology 105, 388–397. doi: 10.1016/j.neuropharm.2016.02.009
Shimada, C., Kurumiya, S., Noguchi, Y., and Umemoto, M. (1990). The effect of neonatal exposure to chronic footshock on pain-responsiveness and sensitivity to morphine after maturation in the rat. Behav. Brain Res. 36, 105–111. doi: 10.1016/0166-4328(90)90165-B
Shu, C., Xiao, L., Tang, J., Wang, G., Zhang, X., and Wang, X. (2015). Blunted behavioral and molecular responses to chronic mild stress in adult rats with experience of infancy maternal separation. Tohoku J. Exp. Med. 235, 81–87. doi: 10.1620/tjem.235.81.Correspondence
Stanton, M. E., Gutierrez, Y. R., and Levine, S. (1988). Maternal deprivation potentiates pituitary-adrenal stress responses in infant rats. Behav. Neurosci. 102, 692–700.
Suri, D., Veenit, V., Sarkar, A., Thiagarajan, D., Kumar, A., Nestler, E. J., et al. (2013). Early stress evokes age-dependent biphasic changes in hippocampal neurogenesis, BDNF expression, and cognition. Biol. Psychiatry 73, 658–666. doi: 10.1016/j.biopsych.2012.10.023
Tractenberg, S. G., Levandowski, M. L., de Azeredo, L. A., Orso, R., Roithmann, L. G., Hoffmann, E. S., et al. (2016). An overview of maternal separation effects on behavioural outcomes in mice: evidence from a four-stage methodological systematic review. Neurosci. Biobehav. Rev. 68, 489–503. doi: 10.1016/j.neubiorev.2016.06.021
Ulrich-Lai, Y. M., Figueiredo, H. F., Ostrander, M. M., Choi, D. C., Engeland, W. C., and Herman, J. P. (2006). Chronic stress induces adrenal hyperplasia and hypertrophy in a subregion-specific manner. Am. J. Physiol. Metab. 291, E965–E973. doi: 10.1152/ajpendo.00070.2006
van Oers, H. J. J. (1998). The ontogeny of glucocorticoid negative feedback: influence of maternal deprivation. Endocrinology 139, 2838–2846. doi: 10.1210/en.139.6.2838
Wang, D., Levine, J. L. S., Avila-Quintero, V., Bloch, M., and Kaffman, A. (2020). Systematic review and meta-analysis: effects of maternal separation on anxiety-like behavior in rodents. Transl. Psychiatry 10:174. doi: 10.1038/s41398-020-0856-0
Wang, W.-S., Kang, S., Liu, W.-T., Li, M., Liu, Y., Yu, C., et al. (2012). Extinction of aversive memories associated with morphine withdrawal requires ERK-mediated epigenetic regulation of brain-derived neurotrophic factor transcription in the rat ventromedial prefrontal cortex. J. Neurosci. 32, 13763–13775. doi: 10.1523/JNEUROSCI.1991-12.2012
Warren, S. G., and Juraska, J. M. (1997). Spatial and nonspatial learning across the rat estrous cycle. Behav. Neurosci. 111, 259–266. doi: 10.1037//0735-7044.111.2.259
Weaver, I. C., Cervoni, N., Champagne, F. A., D’Alessio, A. C., Sharma, S., Seckl, J. R., et al. (2004). Epigenetic programming by maternal behavior. Nat. Neurosci. 7, 847–854.
Weaver, S. A., Diorio, J., and Meaney, M. J. (2007). Maternal separation leads to persistent reductions in pain sensitivity in female rats. J. Pain 8, 962–969. doi: 10.1016/j.jpain.2007.07.001
Whitfield, T. W., Shi, X., Sun, W. L., and McGinty, J. F. (2011). The suppressive effect of an intra-prefrontal cortical infusion of BDNF on cocaine-seeking is TRK receptor and extracellular signal-regulated protein kinase mitogen-activated protein kinase dependent. J. Neurosci. 31, 834–842. doi: 10.1523/JNEUROSCI.4986-10.2011
Woolley, C. S., and McEwen, B. S. (1993). Roles of estradiol and progesterone in regulation of hippocampal dendritic spine density during the estrous cycle in the rat. J. Comp. Neurol. 336, 293–306. doi: 10.1002/cne.903360210
Workel, J. O., Oitzl, M. S., Fluttert, M., Lesscher, H., Karssen, A., and De Kloet, E. R. (2001). Differential and age-dependent effects of maternal deprivation on the hypothalamic-pituitary-adrenal axis of brown norway rats from youth to senescence. J. Neuroendocrinol. 13, 569–580. doi: 10.1046/j.1365-2826.2001.00668.x
Xiong, H., and Krugers, H. J. (2015). Tuning hippocampal synapses by stress-hormones: relevance for emotional memory formation. Brain Res. 1621, 114–120. doi: 10.1016/j.brainres.2015.04.010
Zalosnik, M. I., Pollano, A., Trujillo, V., Suárez, M. M., and Durando, P. E. (2014). Effect of maternal separation and chronic stress on hippocampal-dependent memory in young adult rats: evidence for the match-mismatch hypothesis. Stress 3890, 1–19. doi: 10.3109/10253890.2014.936005
Keywords: brain derived neuronal factor, corticosterone, hippocampus, glucocorticoid receptor, maternal deprivation, tyrosine receptor kinase B, spatial learning
Citation: Stoneham ET, McHail DG, Samipour-Biel S, Liehr N, Lee CM, Evans JC, Boggs K and Dumas TC (2021) Spatial Learning Is Impaired in Male Pubertal Rats Following Neonatal Daily but Not Randomly Spaced Maternal Deprivation. Front. Cell Dev. Biol. 9:621308. doi: 10.3389/fcell.2021.621308
Received: 26 October 2020; Accepted: 26 February 2021;
Published: 18 March 2021.
Edited by:
Erik R. Duboué, Florida Atlantic University, United StatesReviewed by:
Michael A. Burman, University of New England, United StatesCopyright © 2021 Stoneham, McHail, Samipour-Biel, Liehr, Lee, Evans, Boggs and Dumas. This is an open-access article distributed under the terms of the Creative Commons Attribution License (CC BY). The use, distribution or reproduction in other forums is permitted, provided the original author(s) and the copyright owner(s) are credited and that the original publication in this journal is cited, in accordance with accepted academic practice. No use, distribution or reproduction is permitted which does not comply with these terms.
*Correspondence: Theodore C. Dumas, dGR1bWFzQGdtdS5lZHU=
Disclaimer: All claims expressed in this article are solely those of the authors and do not necessarily represent those of their affiliated organizations, or those of the publisher, the editors and the reviewers. Any product that may be evaluated in this article or claim that may be made by its manufacturer is not guaranteed or endorsed by the publisher.
Research integrity at Frontiers
Learn more about the work of our research integrity team to safeguard the quality of each article we publish.